- 1 Division of Oral Biology and Medicine, School of Dentistry, University of California, Los Angeles, CA, USA
- 2 Department of Molecular and Medical Pharmacology, David Geffen School of Medicine, University of California, Los Angeles, CA, USA
- 3 Department of Anesthesiology, University of Pittsburgh, Pittsburgh, PA, USA
- 4 Department of Pharmacology and Chemical Biology, University of Pittsburgh, Pittsburgh, PA, USA
- 5 Division of Biochemistry and Molecular Biology, Center for Brain Research, Medical University Vienna, Vienna, Austria
There is considerable evidence that ethanol (EtOH) potentiates γ-aminobutyric acid type A receptor (GABAAR) action, but only GABAARs containing δ subunits appear sensitive to low millimolar EtOH. The α4 and δ subunits co-assemble into GABAARs which are relatively highly expressed at extrasynaptic locations in the dentate gyrus where they mediate tonic inhibition. We previously demonstrated reversible- and time-dependent changes in GABAAR function and subunit composition in rats after single-dose EtOH intoxication. We concluded that early tolerance to EtOH occurs by over-activation and subsequent internalization of EtOH-sensitive extrasynaptic α4βδ-GABAARs. Based on this hypothesis, any highly EtOH-sensitive GABAARs should be subject to internalization following exposure to suitably high EtOH doses. To test this, we studied the GABAARs in mice with a global deletion of the α4 subunit (KO). The dentate granule cells of these mice exhibited greatly reduced tonic currents and greatly reduced potentiation by acutely applied EtOH, whereas synaptic currents showed heightened sensitivity to low EtOH concentrations. The hippocampus of naive KO mice showed reduced δ subunit protein levels, but increased α2, and γ2 levels compared to wild-type (WT) controls, suggesting at least partial compensation by these subunits in synaptic, highly EtOH-sensitive GABAARs of KO mice. In WT mice, cross-linking and Western blot analysis at 1 h after an EtOH challenge (3.5 g/kg, i.p.) revealed increased intracellular fraction of the α1, α4, and δ, but not α2, α5, or γ2 subunits. By contrast, we observed significant internalization of α1, α2, δ, and γ2 subunits after a similar EtOH challenge in KO mice. Synaptic currents from naïve KO mice were more sensitive to potentiation by zolpidem (0.3 μM, requiring α1/α2, inactive at α4/5 GABAARs) than those from naïve WT mice. At 1 h after EtOH, synaptic currents of WT mice were unchanged, whereas those of KO mice were significantly less sensitive to zolpidem, suggesting decreases in functional α1/2βγ GABAARs. These data further support our hypothesis that EtOH intoxication induces GABAAR plasticity via internalization of highly EtOH-sensitive GABAARs.
Introduction
The mammalian γ-aminobutyric acid type A receptors (GABAARs) are heteropentameric chloride channel proteins formed from a family of 19 related subunits, named α1–6, β1–3, γ1–3, δ, ε, θ, π, and ρ1–3 (Olsen and Sieghart, 2008). Synaptic GABAARs mediate the bulk of fast (phasic) inhibition, while extrasynaptic GABAARs provide a sustained (tonic) inhibitory influence on brain neurotransmission. GABAARs are important targets for drugs such as benzodiazepines (BZs), barbiturates, neurosteroids, ethanol (EtOH), other general anesthetics, and a number of picrotoxin-like convulsants. Differences in GABAAR subunit composition are major determinants of channel kinetics, sensitivity to GABA, and cellular localization to synaptic and extrasynaptic membranes (Olsen and Sieghart, 2009). For example, the presence of α4 and δ subunits in GABAARs results in their extrasynaptic localization (Nusser et al., 1998; Wei et al., 2003; Liang et al., 2006), sensitivity to low concentrations of ambient GABA (Wei et al., 2003; Chandra et al., 2006), insensitivity to the classical BZs such as diazepam, and responsiveness to low millimolar concentrations of EtOH (Sundstrom-Poromaa et al., 2002; Wallner et al., 2003; Wei et al., 2004; Liang et al., 2008). EtOH potentiation of extrasynaptic GABAARs is not universally accepted, several labs have reported a lack of EtOH potentiation of recombinant δ subunit-containing GABAARs (Borghese and Harris, 2007; Korpi et al., 2007). Also, at least some of EtOH’s acute effects on GABAergic transmission are mediated by enhancement of presynaptic GABA release (Roberto et al., 2006).
It is widely accepted that an important aspect of EtOH’s acute action on the brain is enhancement of inhibition mediated by GABAARs, while withdrawal involves a reduction in GABAAR-mediated inhibition (Kumar et al., 2009). A well-substantiated theory of how repeated use of EtOH leads to dependence is that the chronic repetition of the mini-withdrawals leads to a persistent state of withdrawal (alcohol withdrawal syndrome, AWS) in which the withdrawals are more severe and long-lasting, eventually permanent (Becker, 2008). Many of the behavioral and pharmacological changes associated with AWS and dependence may be explained by alterations in the function of GABAARs (Kumar et al., 2009). Chronic EtOH, particularly chronic intermittent EtOH (CIE) administration in rodents produces long-lasting alterations in GABAAR subunit composition, localization, and function (Mhatre and Ticku, 1992; Devaud et al., 1997; Cagetti et al., 2003; Liang et al., 2006). Even a single intoxicating EtOH dose is capable of inducing such alterations, albeit fully reversible (Liang et al., 2007). However, the cellular and molecular mechanisms behind EtOH-induced GABAAR plasticity are not well understood.
Here we studied the early events in the reversible GABAAR plasticity produced by EtOH intoxication. We hypothesized that early tolerance to EtOH occurs by over-activation and subsequent internalization of EtOH-sensitive extrasynaptic α4βδ-GABAARs (Liang et al., 2007; Shen et al., 2011). Based on this hypothesis, any highly EtOH-sensitive GABAARs should be subject to internalization following exposure to moderate-high EtOH doses. To test this, we examined the biochemistry and pharmacology of GABAARs in mice with a global deletion of the α4 subunit (KO). We previously demonstrated that dentate granule cells (DGCs) of these mice exhibit greatly reduced tonic currents (Chandra et al., 2006; Liang et al., 2008) and greatly reduced potentiation by acutely applied EtOH (Liang et al., 2008). However, behavioral responses of KO mice to acute EtOH are almost unchanged (Chandra et al., 2008); this may be attributed to the heightened sensitivity of synaptic currents to low EtOH concentrations (Liang et al., 2008). Therefore, we examined the function, pharmacology and subunit composition of synaptic GABAARs before and after EtOH intoxication in KO mice and their wild-type (WT) counterparts. Our data suggest that genetic deletion of α4 subunits results in compensatory alterations in synaptic GABAAR subunits which undergo rapid internalization following EtOH intoxication.
Materials and Methods
Mice
The α4 KO and WT littermate control mice were produced from heterozygous breeding pairs on a C57BL/6J N7 genetic background. Details of mouse production and genotyping have been previously reported (Chandra et al., 2006). The Institutional Animal Care and Use Committee approved all animal experiments. Only adult male (3–9 months old) mice were used in the experiments described here. Animals of different age were distributed randomly across the different experiments and both biochemical and electrophysiological experiments were often conducted on different hippocampal slices from the same animal. In some experiments, mice received an injection of pyrazole (68 mg/kg, i.p., Sigma, St Louis, MO, USA) 30 min prior to injection of saline or EtOH (3.5 g/kg, i.p.).
Brain Slice Preparation and Western Blotting
Coronal slices (400 μm thick) of mouse dorsal hippocampus were obtained using standard techniques (Spigelman et al., 2003). Briefly, mice were anesthetized with isoflurane, decapitated, and sections obtained using a vibrating blade microtome (VT1200S, Leica Microsystems, Bannockburn, IL, USA) while immersed in cold (0–4°C) artificial cerebrospinal fluid (ACSF) composed of (in mM): NaCl, 125; KCl, 2.5; CaCl2, 2; MgCl2, 2; NaHCO3, 26 and D-glucose, 10. The ACSF was continuously bubbled with a 95/5% mixture of O2/CO2 to ensure adequate oxygenation of slices and a pH of 7.4. The dentate gyrus and CA1 regions were microdissected from individual sections, and incubated in a small volume chamber with or without the protein cross-linking reagent, bis(sulfosuccinimidyl)suberate (BS3) in ACSF at 4°C according to (Grosshans et al., 2002). BS3 is bifunctional and cross-links all proteins exposed to the medium, i.e., cell-surface proteins. These large complexes do not enter the gel and are retained at the top; thus the band of protein at the identified Mr corresponds to that fraction that is intracellular only. The difference between that value and the amount from an equivalent adjacent slice, untreated, and thus total, represents the surface pool. After incubation of brain sections in the absence (total) or presence (intracellular) of BS3, sections were washed three-times with Tris wash buffer (pH 7.6) and homogenized in a buffer composed of 1% SDS, 1 mM EDTA, and 10 mM Tris, pH 8.0. Protein aliquots (40 μg) from samples were separated on 10% SDS-polyacrylamide gel electrophoresis under reducing conditions using the BioRad Mini-Protean 3 Cell system. Proteins were transferred to PVDF membranes (Immun-Blot PVDF membrane, 0.2 mm, BioRad) by wet transfer (BioRad, Hercules, CA, USA). Blots were probed with anti-peptide α1 (proprietary N-terminus sequence), α2 (322–357), α4 (379–421), α5 (337–388), γ1 (1–39), γ2 (319–366), or δ (1–44) antibodies (all at 1 mg/ml), followed by HRP-conjugated anti-rabbit secondary antibody (1:5000 dilution) and bands detected by ECL detection kit (Amersham Pharmacia UK), apposed to X-ray film under non-saturating conditions. The α1 GABAAR antibody was obtained from Novus Biologicals (Littleton, CO, USA). β-actin (Sigma, St. Louis, MO, USA) or GAPDH (Santa Cruz Biotech, Santa Cruz, CA, USA) antibodies were used as a loading controls. Bands from different samples corresponding to the appropriate subunit were analyzed and absorbance values compared by densitometry using ImageJ (NIH, Bethesda, MD, USA). Group differences were evaluated by unpaired t-test. p < 0.05 was considered statistically significant.
Electrophysiological Recordings
Whole-cell patch clamp recordings were obtained from cells located in the DGC layers at 34 ± 0.5°C during perfusion with ACSF using patch pipettes containing (in mM): cesium gluconate, 135; MgCl2, 2; CaCl2, 1; ethylene glycol-bis(β-aminoethyl ether)-N,N,N′,N′-tetraacetic acid, 11; N-2-hydroxyethylpiperazine-N′-2-ethanesulfonic acid, 10; K2ATP, 2; Na2GTP, 0.2; pH adjusted to 7.25 with CsOH. GABAAR-mediated mIPSCs were pharmacologically isolated by adding tetrodotoxin (TTX, 0.5 μM), D(-)-2-amino-5-phosphonopentanoate (APV, 40 μM), 6-cyano-7-nitroquinoxaline-2,3-dione (CNQX, 10 μM), and CGP 54626 (1 μM) to the ACSF from stock solutions. Stock solutions of CNQX were made with pure dimethyl sulfoxide (DMSO). Final concentration of DMSO did not exceed 42 μM in the recording chamber. Signals were recorded in voltage-clamp mode with an amplifier (Axoclamp-2B, Molecular Devices, Sunnyvale, CA, USA). Whole-cell access resistances were in the range of 8–20 MΩ before electrical compensation by ~70%. During voltage-clamp recordings, access resistance was monitored by measuring the size of the capacitative transient in response to a 5-mV step command, and experiments were abandoned if changes >20% were encountered. At least 10 min was allowed for equilibration of the pipette solution with the intracellular milieu before commencing recordings. Data were acquired with pClamp 9 software (Molecular Devices), digitized at 20 kHz (Digidata 1200B, Molecular Devices) and analyzed using the Clampfit software (Molecular Devices) and the Mini Analysis Program (versions 5.2.2 and 5.4.8, Synaptosoft).
Detection and Analysis of mIPSCs
The recordings were low-pass filtered off-line (Clampfit software) at 2 kHz. The mIPSCs were detected with threshold criteria of: 5 pA, amplitude and 20 fC, charge transfer. Frequency of mIPSCs was determined from all automatically detected events in a given 100 s recording period. For kinetic analysis, only single event mIPSCs with a stable baseline, sharp rising phase, and exponential decay were chosen during visual inspection of the recording trace. Double and multiple peak mIPSCs were excluded. The mIPSC kinetics were obtained from analysis of the averaged chosen single events (>120 events/100 s recording period) aligned with half rise time in each cell. Decay time constants were obtained by fitting a double exponential to the falling phase of the averaged mIPSCs. The investigator performing the recordings and mIPSC analysis was blind to the mouse genotypes.
Results
Compensatory Changes in GABAAR Subunit Levels in KO Mice
We first studied compensatory changes in GABAAR subunit levels in the microdissected DG/CA1 areas of hippocampal slices from untreated α4 KO mice compared to their WT counterparts. The DG/CA1 area was kept intact to maximize tissue yield for the protein assays and because previous studies demonstrated similar EtOH-induced alterations in the two hippocampal regions (Liang et al., 2007). As illustrated in Figure 1, KO mice showed significant decreases in both α4 and δ subunit levels (p < 0.05). By contrast, significant increases were observed in the α2 (p = 0.032) and γ2 (p = 0.007) subunit levels. Although increases in γ2 subunits confirmed previous results (Liang et al., 2008), the compensatory increases in α2 subunits were surprising given the normally limited abundance of the predominantly extrasynaptic α4 and δ subunits in WT mice. There was considerable variability in the γ1 subunit expression and the apparent increases did not reach statistical significance (p = 0.28). Although compensatory increases in α5 subunit were expected given its relatively high abundance in the extrasynaptic GABAARs of the CA1 region (Caraiscos et al., 2004), these were not observed, nor was there any significant change in α1 subunit levels (p = 0.25).
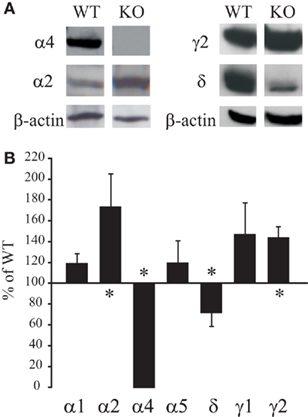
Figure 1. Altered hippocampal GABAAR subunit levels in untreated a4 KO. The hippocampal CA1/dentate gyrus (DG) regions were microdissected from untreated WT and KO mice and prepared for Western blot detection using appropriate antibodies. (A) Example blots of differences in total protein levels of select GABAAR subunits between WT and KO mice. Absorbance values were first normalized to the loading control (β-actin or GAPDH) signal and then expressed as % of total WT signal from the same gel. (B) Summary graph of differences in GABAAR subunit levels between WT and KO mice. Data are mean ± SEM from (n = 4–12 mice/group). †p < 0.05 (unpaired t-test) compared to WT mice.
Altered Identity of GABAAR Subunits Internalized by EtOH Treatment in α4 KO Mice
We next compared the effects of in vivo EtOH exposure (3.5 g/kg, i.p.) on the intracellular levels of GABAAR subunits between WT and KO mice. Since mice rapidly metabolize EtOH, all mice were pretreated with the alcohol dehydrogenase inhibitor, pyrazole (68 mg/kg, i.p.) 30 min prior to saline or EtOH treatment. Mice in both EtOH-treated groups maintained loss of righting reflex (LORR) by the time isoflurane anesthesia was administered (50–59 min post-EtOH). By comparing blots of microdissected slices incubated with or without the membrane-impermeable cross-linking reagent BS3, we were able to identify the intracellular pools of GABAAR subunits (Grosshans et al., 2002). Cell-surface proteins form high molecular weight aggregates with BS3, such that they remain at the top of the gel. By contrast, intracellular proteins are not accessed by the membrane-impermeant reagent and thus can be quantified through Western blot analysis.
As illustrated in Figure 2, analyses of the intracellular levels of WT EtOH-treated, compared to saline-treated mice, revealed a significant increase in the internal levels of α1 (p = 0.02), α4 (p < 0.01), and δ (p = 0.03) subunits, whereas α2, α5, and γ2 subunits remained unchanged (p > 0.05). These data are consistent with the rapid internalization of α4/δ subunit-containing GABAARs previously observed after EtOH intoxication in the rat (Liang et al., 2007). The EtOH-induced increases in internal α1 subunit levels are consistent with the presence of EtOH-sensitive α1βδ-GABAARs localized to hippocampal interneuron subpopulations in WT and α4KO mice (Glykys et al., 2007). In EtOH-treated compared to saline-treated KO mice there was a significant increase in α1 (p = 0.01), α2 (p = 0.01), δ (p = 0.02), and γ2 (p = 0.046) subunits, while α5 (p > 0.05) was unchanged and α4 subunit was not detected. The intracellular pool of α2 was significantly increased in KO EtOH-treated mice compared to WT EtOH-treated mice (p = 0.02). Consistent with our previous findings in rats (Liang et al., 2007), we also demonstrated a lack of EtOH-induced α5 subunit internalization in WT and KO mice (Figure 2C). These data suggested that in the absence of the highly EtOH-sensitive α4/δ subunit-containing GABAARs, EtOH intoxication results in the internalization of α1, α2, δ and γ2 subunit-containing GABAARs in KO mice.
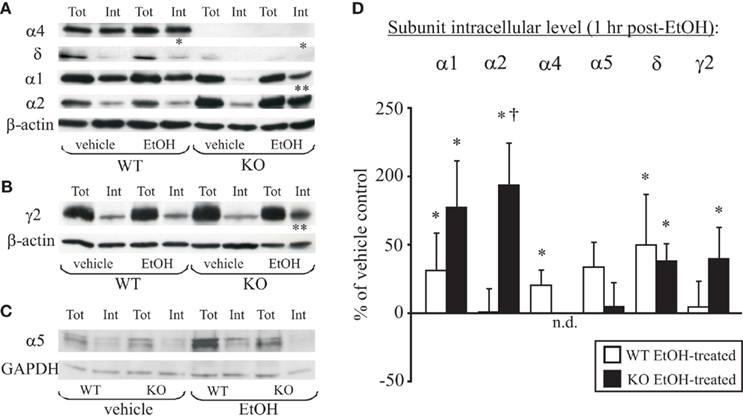
Figure 2. EtOH-induced internalization of α1, α2, and δ subunits in the hippocampus of α4 KO mice. (A) Representative gels from the hippocampal CA1 and dentate gyrus (DG) regions microdissected from WT and KO mice at 1 h after vehicle (saline) or EtOH (3.5 g/kg, i.p.) injection. Note the increases in the intracellular fraction of α1, α4, and δ subunits (*) after EtOH treatment in WT mice. The α4 signal is absent while α5 and δ signal reduced in KO mice. In comparison to vehicle treatment, EtOH treatment induces internalization of α1, α2, δ and γ2 subunits (*) in tissue collected from KO mice at 1 h after treatment. EtOH treatment also caused a significant increase in internalization of α2 subunits in KO mice (†) as compared to EtOH-treated WT mice. (B) Similar experiments in a different gel illustrate internalization of the γ2 subunit (*) at 1 h after EtOH treatment in KO but not WT mice. (C) Example gel illustrating the lack of α5 subunit internalization after EtOH treatment of WT or KO mice. (D) Summary graph of changes in internal subunit levels of WT (open bars) and KO (closed bars) mice 1 h after EtOH intoxication relative to vehicle-treated mice (horizontal 100% line). Data are mean ± SEM (n = 4–12 mice/group). The α4 signal was not detected (0%) in the α4 KO samples. *p < 0.05 (unpaired t-test) compares EtOH-treated with vehicle-treated mice; †p < 0.05 (unpaired t-test) compares WT EtOH-treated to α4KO EtOH-treated groups.
Altered Zolpidem Sensitivity of Synaptic GABAARs in α4 KO Mice
Based on the compensatory increases in α2 and γ2 subunits in α4 KO mice (Figure 1), we hypothesized that synaptic GABAARs containing these subunits should also exhibit increased sensitivity to potentiation by benzodiazepine site ligands. To test this, we compared the responsiveness of mIPSCs in WT and KO mice to zolpidem (0.3 μM). Zolpidem exhibits highest affinity for α1βγ2 GABAARs; its affinity for α2/3βγ2 GABAARs is 2–10-fold lower; its affinity for α4/5-containing GABAARs is >1000-fold lower (Wafford et al., 1993; Möhler et al., 2000). In hippocampal neurons of α1 KO mice, 0.3 μM zolpidem still potentiates mIPSCs by prolonging their decay time (Goldstein et al., 2002). Thus, zolpidem at 0.3 μM is expected to potentiate both α1βγ2 and α2βγ2 populations of GABAARs. We further hypothesized, based on the observed EtOH-induced internalization of α1, α2, and γ2 subunit-containing GABAARs in α4 KO mice, that zolpidem potentiation of synaptic GABAARs should be selectively reduced by EtOH treatment in KO mice.
Analysis of mIPSC kinetics revealed differences between saline-treated WT and KO mice analogous to those reported in detail previously (Chandra et al., 2006; Liang et al., 2008). The main differences were decreased amplitude and frequency of mIPSCs in KO mice (Table 1). For both phenotypes, the mIPSC kinetics did not significantly differ between saline- and EtOH-treated mice, except for a post-EtOH increase in mIPSC decay τ2 of WT mice.
In our recording conditions of 34.5°C the main effect of zolpidem was an increase in mIPSC decay time for both WT and KO DG cells (Table 2). As hypothesized, we observed significantly greater potentiation of mIPSCs by zolpidem in recordings from saline-treated KO mice compared to saline-treated WT mice (Table 2 and Figures 3A–C). Further analysis revealed that zolpidem potentiation of mIPSCs was unchanged at 1 h post-EtOH treatment in WT mice, but was significantly reduced at 1 h post-EtOH treatment in KO mice (Table 2 and Figures 3A–C). These data provided pharmacological evidence that the synaptic GABAARs in DGCs of α4 KO mice exhibit increased benzodiazepine sensitivity and are rapidly internalized after exposure to high EtOH concentrations.
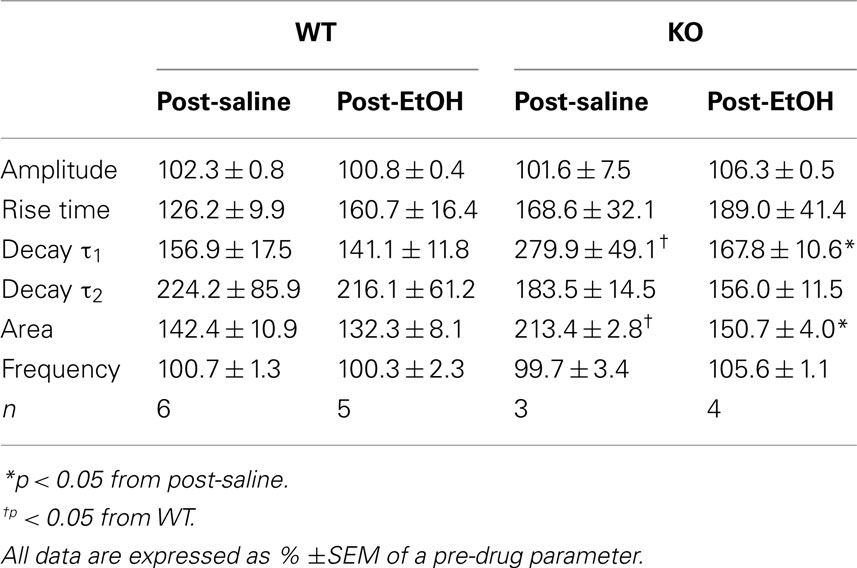
Table 2. Zolpidem (0.3 μM) effects on mIPSC kinetics in DG cells from saline- and EtOH-exposed WT and KO mice.
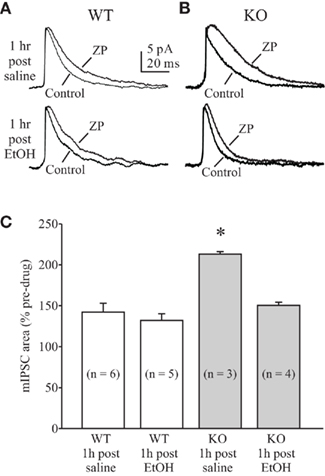
Figure 3. Altered enhancement of mIPSCs by zolpidem in DG cells from α4 KO mice. Examples of averaged mIPSCs before and after zolpidem (ZP, 0.3 μM) application in DG cells from saline- (upper traces) and EtOH-treated (lower traces) WT (A) and α4 KO (B) mice. Slices were prepared at 50–60 min after EtOH administration. Cells were voltage-clamped at 0 mV and averaged mIPSCs were aligned at baseline. (C): summary graph of mIPSC potentiation by zolpidem in WT and KO mice. Note the unchanged mIPSC potentiation by zolpidem at 1 h after EtOH administration in WT mice. Also note the increased mIPSC potentiation in saline-treated KO mice and decreased mIPSC potentiation in EtOH-treated KO mice. *p < 0.05 from other groups (one-way RM ANOVA).
Discussion
Here we demonstrate that global deletion of the α4 subunit produces compensatory alterations in the subunit composition and function of synaptic GABAARs. Specifically, loss of the α4 subunit leads to a reduction in δ subunit levels. Normally these subunits co-assemble into peri- and extra-synaptically located α4βδ-GABAARs (Nusser et al., 1998; Wei et al., 2003; Liang et al., 2006) which exhibit low conductance, high affinity for GABA (Wei et al., 2003; Chandra et al., 2006), insensitivity to BZs, and sensitivity to low millimolar concentrations of EtOH (Sundstrom-Poromaa et al., 2002; Wallner et al., 2003; Wei et al., 2004; Liang et al., 2008). Consistent with the extrasynaptic localization and functional properties of α4βδ-GABAARs, previous studies demonstrated that DGCs of mice with global deletions of either δ or α4 subunits exhibit greatly reduced tonic inhibitory currents (Chandra et al., 2006; Liang et al., 2008) and reduced sensitivity to potentiation by acute EtOH (Glykys et al., 2007; Liang et al., 2008). Surprisingly, the synaptic currents in DGCs of δ KO (Liang et al., 2006), and particularly α4 KO (Liang et al., 2008) mice exhibit enhanced sensitivity to low concentrations of EtOH. This enhanced synaptic responsiveness provides a plausible physiological explanation for the apparently normal behavioral responses to acute EtOH of these KO mice, despite clear reductions in EtOH-enhanced tonic inhibitory currents in hippocampal neurons (Mihalek et al., 2001; Chandra et al., 2008). It must be noted that the normal responses to acute EtOH do not extend to chronic EtOH consumption or the more long-term effects of EtOH intoxication and subsequent withdrawal symptoms which are attenuated in δ KO mice.
Global loss of the δ subunit leads to compensatory decreases in α4 and increases in γ2 subunit protein levels (Tretter et al., 2001; Peng et al., 2002). Increased co-immunoprecipitation of these remaining two subunits in δ KO mice (Korpi et al., 2002) suggests formation of functional synaptic GABAARs, which might be the cause of an enhanced responsiveness to low concentrations of EtOH (Liang et al., 2006). This conclusion is supported by the finding that rats withdrawn from acute or chronic EtOH administration exhibit marked increases in α4 and γ2 subunit protein levels, and increased sensitivity of synaptic GABAARs to low concentrations of acute EtOH (Liang et al., 2007). Chronic EtOH administration also results in large increases in α4 subunit incorporation within GABAergic synapses of DGCs (Liang et al., 2006). Here we demonstrate that global deletion of the α4 subunit leads to compensatory decreases in δ and increases in α2 and γ2 subunit protein levels (Figure 1). These data confirm our previous findings of selective increases in γ2 subunits in hippocampus and thalamus, but not cortex of α4 KO mice (Liang et al., 2008). Interestingly, we did not observe compensatory increases in the α5 subunit levels in the hippocampus. This subunit is normally expressed at relatively high levels in the extrasynaptic GABAARs of the CA1 region, which contribute to tonic inhibition (Caraiscos et al., 2004). Notably, α5 subunit-containing GABAARs also contribute to tonic inhibition in DG neurons because the reduced tonic currents in δ subunit KO are completely eliminated in the δ/α5 double knockout mice (Glykys et al., 2008). The previously demonstrated large decreases in tonic currents of DG neurons from α4 KO mice (Liang et al., 2008) are consistent with the lack of compensatory increases in α5 subunit levels observed here. It would be interesting to determine if the magnitude of tonic currents in the CA1 region are affected by the loss of the α4 subunit.
An obvious question is whether the compensatory alterations in subunit composition are directly responsible for the altered function and pharmacological properties of synaptic GABAARs in α4 KO mice, particularly with respect to enhanced EtOH sensitivity (Liang et al., 2008). Previously, we localized a relatively small (~25%) but significant proportion of α4 subunits to the central portion of GABAergic synapses in DGCs of rats (Liang et al., 2006). Loss of such synaptic α4 subunits is consistent with the altered mIPSC kinetics of α4 KO mice (Chandra et al., 2006; Liang et al., 2008). Here we hypothesized that in the absence of α4 subunits, synaptic GABAARs with increases in relative proportions of α2/γ subunits should also exhibit greater sensitivity to BZs. To test this, we compared synaptic responsiveness of WT and KO DGCs to zolpidem, a BZ site ligand which is inactive at α4/5-containing GABAARs and preferentially potentiates α1–3/γ2-containing GABAARs (Wafford et al., 1993; Möhler et al., 2000). Our data revealed significantly greater zolpidem potentiation of mIPSCs from KO mice compared to WT mice (Figure 3), supporting our hypothesis. However, the increased presence of α2 and γ2 subunits at synapses does not explain the high EtOH sensitivity of these compensatory synaptic GABAARs. In addition to the multitude of novel subunit combinations, one cannot help suggest that some unknown factor(s) might also affect synaptic EtOH sensitivity. Perhaps this is related to GABAAR-associated proteins and/or some protein phosphorylation event(s).
The presence of highly EtOH-sensitive synaptic receptors in tissues which virtually lack extrasynaptic tonic currents and EtOH responsiveness gives us the opportunity to explore several aspects of the EtOH-induced GABAAR plasticity hypothesis (Liang et al., 2007). Here, we focused on the early events in EtOH intoxication, hypothesized to involve EtOH-induced overstimulation of EtOH-sensitive GABAARs resulting in their rapid internalization. Thus, we reasoned that if the EtOH-sensitive synaptic GABAARs of α4 KO mice were exposed to high doses of EtOH, we should be able to detect their internalization and its functional consequences with biochemical and electrophysiological techniques. Indeed, cross-linking and Western blot analysis of hippocampal tissue collected from KO mice within 1 h of EtOH exposure showed internalization of α1, α2, δ, and γ2 subunits (Figure 2). By contrast, only α1, α4, and δ subunits showed EtOH-induced internalization in WT mice. Moreover, the zolpidem sensitivity of synaptic GABAARs of KO mice was significantly reduced at 1 h after EtOH treatment, whereas zolpidem sensitivity of WT mice was unchanged by EtOH treatment, suggesting functional decreases in α1–3/γ-containing synaptic GABAARs only in EtOH-treated KO mice.
Although δ subunit levels were significantly reduced in the α4 KO hippocampus, we could still detect EtOH-induced internalization of δ subunits in KO mice. Previous studies have demonstrated that unlike DGCs, certain hippocampal GABAergic interneurons express α1/δ-containing GABAARs which are responsive to low concentrations of EtOH (Glykys et al., 2007). We suspect that it is these remaining EtOH-sensitive δ-containing GABAARs that account for the EtOH-induced δ-subunit internalization signal in KO mice.
Use-dependent decrease of receptor function is a common mechanism of drug tolerance development. This process is a complex series of events which may include receptor desensitization, uncoupling between agonist binding and channel activation, receptor internalization, and transcriptional down-regulation. These separable mechanisms of drug tolerance development are specific to the class of receptors under study. For example, the development of acute tolerance to opioid analgesics was shown to involve receptor desensitization via phosphorylation and functional uncoupling of receptors from G proteins, while receptor internalization was shown to counteract desensitization through rapid recycling of opioid receptors back to the surface in a reactivated state (Koch and Hollt, 2008). Pathology-induced chronic opioid tolerance appears to involve transcriptional decreases in receptor expression (Abdulla and Smith, 1998). Acute tolerance to BZs is also thought to involve uncoupling between BZ and GABA binding sites of GABAARs (Hu and Ticku, 1994; Primus et al., 1996; Ali and Olsen, 2001). By contrast, development of acute tolerance to EtOH has been related to early internalization of extrasynaptic GABAARs (Liang et al., 2007), whereas protracted tolerance following chronic EtOH administration appears to involve transcriptional alterations in GABAAR subunit composition and function (Matthews et al., 1998; Cagetti et al., 2003; Liang et al., 2006), reviewed in (Kumar et al., 2009). Fairly rapid transcriptionally mediated increases in α4 subunit levels have also been demonstrated following EtOH exposure (Pignataro et al., 2007). Such increases in α4 subunit levels were recently determined to require selective activation of the protein kinase γ isozyme (Werner et al., 2010). Rapid decreases in inhibition and BZ tolerance during status epilepticus have also been demonstrated to involve internalization of GABAARs (Goodkin et al., 2005, 2008; Naylor et al., 2005) and subsequent long-term alterations in GABAAR subunit composition were demonstrated to involve transcriptional events (Brooks-Kayal et al., 1998; Roberts et al., 2005).
In summary, our studies showed that global genetic deletion of the α4 subunit leads to compensatory changes in synaptic GABAAR subunit composition and function. These functional changes include increased zolpidem sensitivity. We also demonstrate that EtOH intoxication in the KO mice leads to rapid internalization of these zolpidem-sensitive synaptic receptors. The α4 KO mice should prove useful in examining the long-term consequences of EtOH withdrawal and dependence.
Conflict of Interest Statement
The authors declare that the research was conducted in the absence of any commercial or financial relationships that could be construed as a potential conflict of interest.
Acknowledgments
Supported by NIH grants AA07680, AA13004, and AA16100.
References
Abdulla, F. A., and Smith, P. A. (1998). Axotomy reduces the effect of analgesic opioids yet increases the effect of nociceptin on dorsal root ganglion neurons. J. Neurosci. 18, 9685–9694.
Ali, N. J., and Olsen, R. W. (2001). Chronic benzodiazepine treatment of cells expressing recombinant GABAA receptors uncouples allosteric binding: studies on possible mechanisms. J. Neurochem. 79, 1100–1108.
Becker, H. C. (2008). Alcohol dependence, withdrawal, and relapse. Alcohol Res. Health 31. Available at: http://pubs.niaaa.nih.gov/publicatio ns/arh314/348-361.htm
Borghese, C. M., and Harris, R. A. (2007). Studies of ethanol actions on recombinant δ-containing γ-aminobutyric acid type A receptors yield contradictory results. Alcohol 41, 155–162.
Brooks-Kayal, A. R., Shumate, M. D., Jin, H., Rikhter, T. Y., and Coulter, D. A. (1998). Selective changes in single cell GABA(A) receptor subunit expression and function in temporal lobe epilepsy. Nat. Med. 4, 1166–1172.
Cagetti, E., Liang, J., Spigelman, I., and Olsen, R. W. (2003). Withdrawal from chronic intermittent ethanol treatment changes subunit composition, reduces synaptic function, and decreases behavioral responses to positive allosteric modulators of GABAA receptors. Mol. Pharmacol. 63, 53–64.
Caraiscos, V. B., Elliott, E. M., You, T., Cheng, V. Y., Belelli, D., Newell, J. G., Jackson, M. F., Lambert, J. J., Rosahl, T. W., Wafford, K. A., MacDonald, J. F., and Orser, B. A. (2004). Tonic inhibition in mouse hippocampal CA1 pyramidal neurons is mediated by α5 subunit-containing γ-aminobutyric acid type A receptors. Proc. Natl. Acad. Sci. U.S.A. 101, 3662–3667.
Chandra, D., Jia, F., Liang, J., Peng, Z., Suryanarayanan, A., Werner, D. F., Spigelman, I., Houser, C. R., Olsen, R. W., Harrison, N. L., and Homanics, G. E. (2006). GABAA receptor α4 subunits mediate extrasynaptic inhibition in thalamus and dentate gyrus and the action of gaboxadol. Proc. Natl. Acad. Sci. U.S.A. 103, 15230–15235.
Chandra, D., Werner, D. F., Liang, J., Suryanarayanan, A., Harrison, N. L., Spigelman, I., Olsen, R. W., and Homanics, G. E. (2008). Normal acute behavioral responses to moderate/high dose ethanol in GABAA receptor α4 subunit knockout mice. Alcohol. Clin. Exp. Res. 32, 10–18.
Devaud, L. L., Fritschy, J. M., Sieghart, W., and Morrow, A. L. (1997). Bidirectional alterations of GABAA receptor subunit peptide levels in rat cortex during chronic ethanol consumption and withdrawal. J. Neurochem. 69, 126–130.
Glykys, J., Mann, E. O., and Mody, I. (2008). Which GABAA receptor subunits are necessary for tonic inhibition in the hippocampus? J. Neurosci. 28, 1421–1426.
Glykys, J., Peng, Z., Chandra, D., Homanics, G. E., Houser, C. R., and Mody, I. (2007). A new naturally occurring GABAA receptor subunit partnership with high sensitivity to ethanol. Nat. Neurosci. 10, 40–48.
Goldstein, P. A., Elsen, F. P., Ying, S. W., Ferguson, C., Homanics, G. E., and Harrison, N. L. (2002). Prolongation of hippocampal miniature inhibitory postsynaptic currents in mice lacking the GABAA receptor α1 subunit. J. Neurophysiol. 88, 3208–3217.
Goodkin, H. P., Joshi, S., Mtchedlishvili, Z., Brar, J., and Kapur, J. (2008). Subunit-specific trafficking of GABAA receptors during status epilepticus. J. Neurosci. 28, 2527–2538.
Goodkin, H. P., Yeh, J. L., and Kapur, J. (2005). Status epilepticus increases the intracellular accumulation of GABAA receptors. J. Neurosci. 25, 5511–5520.
Grosshans, D. R., Clayton, D. A., Coultrap, S. J., and Browning, M. D. (2002). Analysis of glutamate receptor surface expression in acute hippocampal slices. Sci. STKE 2002, L8.
Hu, X. J., and Ticku, M. K. (1994). Chronic benzodiazepine agonist treatment produces functional uncoupling of the gamma-aminobutyric acid-benzodiazepine receptor ionophore complex in cortical neurons. Mol. Pharmacol. 45, 618–625.
Koch, T., and Hollt, V. (2008). Role of receptor internalization in opioid tolerance and dependence. Pharmacol. Ther. 117, 199–206.
Korpi, E. R., Debus, F., Linden, A. M., Malecot, C., Leppa, E., Vekovischeva, O., Rabe, H., Bohme, I., Aller, M. I., Wisden, W., and Luddens, H. (2007). Does ethanol act preferentially via selected brain GABAA receptor subtypes? The current evidence is ambiguous. Alcohol 41, 163–176.
Korpi, E. R., Mihalek, R. M., Sinkkonen, S. T., Hauer, B., Hevers, W., Homanics, G. E., Sieghart, W., and Luddens, H. (2002). Altered receptor subtypes in the forebrain of GABAA receptor δ subunit-deficient mice: recruitment of γ2 subunits. Neuroscience 109, 733–743.
Kumar, S., Porcu, P., Werner, D. F., Matthews, D. B., az-Granados, J. L., Helfand, R. S., and Morrow, A. L. (2009). The role of GABAA receptors in the acute and chronic effects of ethanol: a decade of progress. Psychopharmacology (Berl.) 205, 529–564.
Liang, J., Suryanarayanan, A., Abriam, A., Snyder, B., Olsen, R. W., and Spigelman, I. (2007). Mechanisms of reversible GABAA receptor plasticity after ethanol intoxication. J. Neurosci. 27, 12367–12377.
Liang, J., Suryanarayanan, A., Chandra, D., Homanics, G. E., Olsen, R. W., and Spigelman, I. (2008). Functional consequences of GABAA receptor α4 subunit deletion on synaptic and extrasynaptic currents in mouse dentate granule cells. Alcohol. Clin. Exp. Res. 32, 19–26.
Liang, J., Zhang, N., Cagetti, E., Houser, C. R., Olsen, R. W., and Spigelman, I. (2006). Chronic intermittent ethanol-induced switch of ethanol actions from extrasynaptic to synaptic hippocampal GABAA receptors. J. Neurosci. 26, 1749–1758.
Matthews, D. B., Devaud, L. L., Fritschy, J. M., Sieghart, W., and Morrow, A. L. (1998). Differential regulation of GABAA receptor gene expression by ethanol in the rat hippocampus versus cerebral cortex. J. Neurochem. 70, 1160–1166.
Mhatre, M. C., and Ticku, M. K. (1992). Chronic ethanol administration alters gamma-aminobutyric acid A receptor gene expression. Mol. Pharmacol. 42, 415–422.
Mihalek, R. M., Bowers, B. J., Wehner, J. M., Kralic, J. E., VanDoren, M. J., Morrow, A. L., and Homanics, G. E. (2001). GABAA-receptor δ subunit knockout mice have multiple defects in behavioral responses to ethanol. Alcohol. Clin. Exp. Res. 25, 1708–1718.
Möhler, H., Benke, D., Fritschy, J. M., and Benson, J. (2000). “The benzodiazepine site of GABAA receptors,” in GABA in the Nervous System: The View at Fifty Years, eds D. L. Martin and R. W. Olsen (Philadelphia: Lippincott Williams & Wilkins), 97–112.
Naylor, D. E., Liu, H., and Wasterlain, C. G. (2005). Trafficking of GABAA receptors, loss of inhibition, and a mechanism for pharmacoresistance in status epilepticus. J. Neurosci. 25, 7724–7733.
Nusser, Z., Sieghart, W., and Somogyi, P. (1998). Segregation of different GABAA receptors to synaptic and extrasynaptic membranes of cerebellar granule cells. J. Neurosci. 18, 1693–1703.
Olsen, R. W., and Sieghart, W. (2008). International Union of Pharmacology. LXX. Subtypes of gamma-aminobutyric acid(A) receptors: classification on the basis of subunit composition, pharmacology, and function. Update. Pharmacol. Rev. 60, 243–260.
Olsen, R. W., and Sieghart, W. (2009). GABA A receptors: subtypes provide diversity of function and pharmacology. Neuropharmacology 56, 141–148.
Peng, Z., Hauer, B., Mihalek, R. M., Homanics, G. E., Sieghart, W., Olsen, R. W., and Houser, C. R. (2002). GABAA receptor subunit changes in δ subunit-deficient mice: altered expression of α4 and γ2 subunits in the forebrain. J. Comp. Neurol. 446, 179–197.
Pignataro, L., Miller, A. N., Ma, L., Midha, S., Protiva, P., Herrera, D. G., and Harrison, N. L. (2007). Alcohol regulates gene expression in neurons via activation of heat shock factor 1. J. Neurosci. 27, 12957–12966.
Primus, R. J., Yu, J., Xu, J., Hartnett, C., Meyyappan, M., Kostas, C., Ramabhadran, T. V., and Gallager, D. W. (1996). Allosteric uncoupling after chronic benzodiazepine exposure of recombinant gamma-aminobutyric acid(A) receptors expressed in Sf9 cells: ligand efficacy and subtype selectivity. J. Pharmacol. Exp. Ther. 276, 882–890.
Roberto, M., Treistman, S. N., Pietrzykowski, A. Z., Weiner, J., Galindo, R., Mameli, M., Valenzuela, F., Zhu, P. J., Lovinger, D., Zhang, T. A., Hendricson, A. H., Morrisett, R., and Siggins, G. R. (2006). Actions of acute and chronic ethanol on presynaptic terminals. Alcohol. Clin. Exp. Res. 30, 222–232.
Roberts, D. S., Raol, Y. H., Bandyopadhyay, S., Lund, I. V., Budreck, E. C., Passini, M. A., Wolfe, J. H., Brooks-Kayal, A. R., and Russek, S. J. (2005). Egr3 stimulation of GABRA4 promoter activity as a mechanism for seizure-induced up-regulation of GABAA receptor α4 subunit expression. Proc. Natl. Acad. Sci. U.S.A. 102, 11894–11899.
Shen, Y., Lindemeyer, A. K., Spigelman, I., Sieghart, W., Olsen, R. W., and Liang, J. (2011). Plasticity of GABAA receptors following ethanol pre-exposure in cultured hippocampal neurons. Mol. Pharmacol. 79, 432–442.
Spigelman, I., Li, Z., Liang, J., Cagetti, E., Samzadeh, S., Mihalek, R. M., Homanics, G. E., and Olsen, R. W. (2003). Reduced inhibition and sensitivity to neurosteroids in hippocampus of mice lacking the GABAA receptor δ subunit. J. Neurophysiol. 90, 903–910.
Sundstrom-Poromaa, I., Smith, D. H., Gong, Q. H., Sabado, T. N., Li, X., Light, A., Wiedmann, M., Williams, K., and Smith, S. S. (2002). Hormonally regulated α4β2δ GABAA receptors are a target for alcohol. Nat. Neurosci. 5, 721–722.
Tretter, V., Hauer, B., Nusser, Z., Mihalek, R. M., Hoger, H., Homanics, G. E., Somogyi, P., and Sieghart, W. (2001). Targeted disruption of the GABAA receptor δ subunit gene leads to an upregulation of γ2 subunit-containing receptors in cerebellar granule cells. J. Biol. Chem. 276, 10532–10538.
Wafford, K. A., Whiting, P. J., and Kemp, J. A. (1993). Differences in affinity and efficacy of benzodiazepine receptor ligands at recombinant γ-aminobutyric acidA receptor subtypes. Mol. Pharmacol. 43, 240–244.
Wallner, M., Hanchar, H. J., and Olsen, R. W. (2003). Ethanol enhances α4β3δ and α6β3δ γ-aminobutyric acid type A receptors at low concentrations known to affect humans. Proc. Natl. Acad. Sci. U.S.A. 100, 15218–15223.
Wei, W., Faria, L. C., and Mody, I. (2004). Low ethanol concentrations selectively augment the tonic inhibition mediated by δ subunit-containing GABAA receptors in hippocampal neurons. J. Neurosci. 24, 8379–8382.
Wei, W., Zhang, N., Peng, Z., Houser, C. R., and Mody, I. (2003). Perisynaptic localization of δ subunit-containing GABAA receptors and their activation by GABA spillover in the mouse dentate gyrus. J. Neurosci. 23, 10650–10661.
Keywords: tolerance, dependence, withdrawal, internalization, dentate gyrus, synaptic transmission, alcohol, receptor trafficking
Citation: Suryanarayanan A, Liang J, Meyer EM, Lindemeyer AK, Chandra D, Homanics GE, Sieghart W, Olsen RW and Spigelman I (2011) Subunit compensation and plasticity of synaptic GABAA receptors induced by ethanol in α4 subunit knockout mice. Front. Neurosci. 5:110. doi: 10.3389/fnins.2011.00110
Received: 11 January 2011;
Accepted: 29 August 2011;
Published online: 23 September 2011.
Edited by:
A. Leslie Morrow, University of North Carolina School of Medicine, USAReviewed by:
Enrico Sanna, University of Cagliari, ItalyBrian McCool, Wake Forest School of Medicine, USA
Copyright: © 2011 Suryanarayanan, Liang, Meyer, Lindemeyer, Chandra, Homanics, Sieghart, Olsen and Spigelman. This is an open-access article subject to a non-exclusive license between the authors and Frontiers Media SA, which permits use, distribution and reproduction in other forums, provided the original authors and source are credited and other Frontiers conditions are complied with.
*Correspondence: Igor Spigelman, University of California, Los Angeles, 63-078 CHS, 10833 Le Conte Avenue, Los Angeles, CA 90095-1668, USA. e-mail:aWdvckB1Y2xhLmVkdQ==