Introduction
Glaucoma is a multifactorial neurodegenerative disease affecting 64.3 million people worldwide (Tham et al., 2014). Despite vigorous research on new treatments, those that reduce intraocular pressure (IOP) remain the gold standard. However, their effectiveness has been questioned as they only slow down degeneration without significantly reversing or stopping the disease (Osborne et al., 2016b). Recent studies have, therefore, investigated the causative roles of other processes, including glutamate toxicity, glial overactivation, etc., (Mann et al., 2005; Chong and Martin, 2015; Lopez Sanchez et al., 2016; Vecino et al., 2016).
Mitochondrial dysfunction is another widely studied causal process in the development of glaucoma and has also been investigated as a potential drug target. For example, red light therapy, manipulation of the mammalian target of rapamycin (mTOR) pathway, and nicotinamide treatment are three recently investigated clinical therapies for glaucoma-related mitochondrial dysfunction (Osborne et al., 2016a,b; Williams et al., 2017). Mitochondrial activity is intimately linked to oxidative metabolism and reactive oxygen species (ROS) formation (Schieke et al., 2006). ROS production is known to cause retinal ganglion cell (RGC) apoptosis and subsequent vision loss. Furthermore, while mitochondrial function is regulated by multiple pathways, calcium signaling likely plays a key role (Vosler et al., 2008; Hurst et al., 2017). In fact, plasma membrane calcium channel inhibitors were recently found to arrest acute axonal degeneration and improve regeneration after optic nerve crush (Ribas et al., 2017). A different combination of calcium permeability inhibitors also preserved optokinetic reflex following partial optic nerve transection (Savigni et al., 2013). While the inhibitors utilized in these studies targeted calcium channels in the plasma membrane, their effects indicate that ROS generation and calcium signaling, which are significantly regulated by the mitochondria, are critical during glaucoma pathogenesis.
Recently, a mitochondrial-specific drug delivery system was shown to be effective in increasing drug concentration in mitochondria in hepatic injuries and drug-resistant cancer cells (Yamada and Harashima, 2017; Yamada et al., 2017). However, the full potential of this system (and other similar systems) has not been fully evaluated with regards to calcium regulation in the diseased retina. In this opinion article, we provide a brief discussion concerning the role of mitochondrial calcium regulation during glaucoma pathogenesis as well as insight concerning the potential use of mitochondrial-specific drug delivery during disease treatment. We believe that the extensive research and overlap in the fields of glaucoma and mitochondrial disease/aging (including calcium signaling dysfunction) ultimately lead to the therapeutic utilization of mitochondrial-specific delivery of calcium channel regulators during glaucoma and other retinal/neurodegenerative diseases (Figure 1).
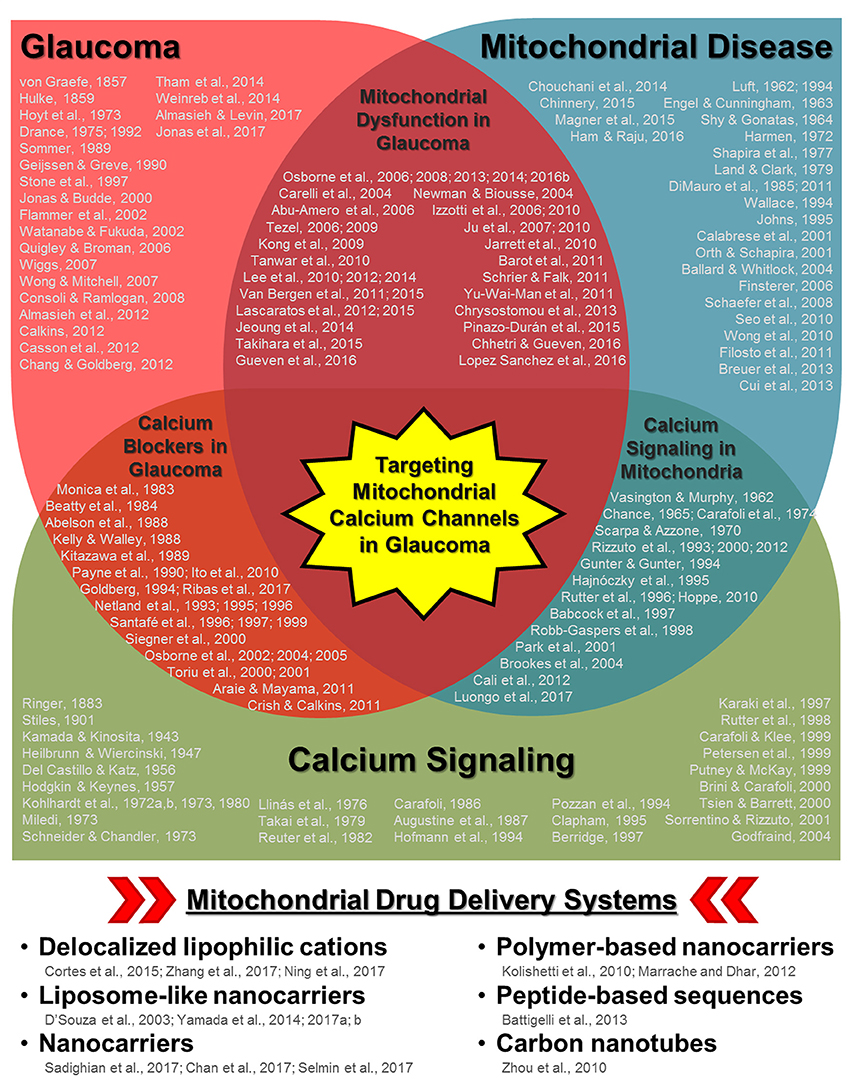
Figure 1. Schematic diagram highlighting the relationships between glaucoma, mitochondrial disease/aging, and calcium signaling along with multiple keystone studies and reviews from prominent research groups. Some of the earliest published research concerning disease pathology and mechanisms are listed for each respective field as well as in areas of overlap (e.g., mitochondrial dysfunction in glaucoma, calcium channel treatment in glaucoma, and calcium signaling in mitochondria). While it is not possible to list all of the influential research published in each field, those listed include some of the key historical publications, with particular emphasis on relationships with the ocular environment or neurodegeneration when applicable. The cumulative research reported in these publications (and those cited within) in each respective field as well as other disease contexts has led to the development of multiple mitochondria-specific drug delivery systems, listed in the bottom panel. Their validation in parallel with the continued investigation of mitochondrial calcium signaling during disease pathogenesis indicate that targeting mitochondrial calcium channels during glaucoma could be a powerful therapeutic tool.
Glaucoma Pathophysiology
Glaucoma is a two phase degenerative disease. The first phase involves a primary insult to the RGCs (Levkovitch-Verbin et al., 2003). Confirmed risk factors/insults for glaucoma include high IOP, ischemia, and aging. While these direct insults have classically been investigated as the cause of glaucoma-related vision loss, recent evidence indicates that damage to the visual cortex and/or optic nerve (i.e., distal axonopathy), which is then propagated to the retina following stress on axonal transport systems, may play a significant role in the initiation of the disease (Calkins and Horner, 2012; Crish and Calkins, 2015). Ultimately, all of these insults disrupt oxygen supply and alter retinal function. Furthermore, mitochondrial oxidative phosphorylation is significantly less effective in the affected RGCs, and energy production depends more on glycolysis and the tricarboxylic acid cycle. This change in energy supply causes oxidative stress and reduced ROS consumption, leading to mitochondrial damage and further ROS accumulation (Nguyen et al., 2011). While it has been hypothesized that RGCs can still function normally in this reduced energy state (Osborne et al., 2016b), they are more susceptible to secondary insults.
Secondary affronts to the RGCs can come in various forms. For example, primary insult-induced activation of retinal microglia and astrocytes as well as altered Müller cell function have detrimental secondary consequences related to the release of pro-inflammatory markers as well as other cytotoxic substances, including glutamate, nitrogen oxide, etc., in the extracellular space surrounding the RGCs. Furthermore, aged/dysfunctional mitochondria within the RGCs can also act as secondary stressors. In aged mitochondria, the initial increase in oxidative stress and reduced ROS consumption is amplified, resulting in a vicious positive feedback loop involving ROS along with damage to mitochondrial and nuclear DNA (Nguyen et al., 2011). This damage is largely irreversible as the repair mechanisms are often impaired in aged mitochondria. ATP production in cells with damaged mitochondria also becomes increasingly more difficult, ultimately leading to calcium dysregulation. As calcium is a known trigger for glutamate release (Neher and Sakaba, 2008), disrupted calcium signaling in aged mitochondria can further exacerbate primary insult-induced glutamate toxicity. Interestingly, increased glutamate concentration also mediates calcium influx (Wojda et al., 2008), indicating multiple points of crosstalk between mitochondrial calcium signaling and neuronal function.
Mitochondrial Calcium as a Key Player in Glaucoma
Mitochondria have two membrane layers. At the outer membrane, calcium influx is largely mediated through voltage-dependent anion channels (VDACs) (Cali et al., 2012; Rizzuto et al., 2012). Some reports suggest that open-state VDACs facilitate metabolite flow and prevent cytochrome C release, while closed-state VDACs mediate the opposite (Tan and Colombini, 2007; Hoppe, 2010; Williams et al., 2013). Further, elevated intracellular calcium concentrations appear to increase VDAC1 oligomerization and downstream apoptosis (Keinan et al., 2013). This increase in oligomerization has been demonstrated to be mediated specifically by mitochondrial, rather than cytosolic, calcium, providing a direct link between mitochondrial calcium and apoptosis.
At the inner membrane, calcium influx from the intermembrane space into the matrix is largely regulated by calcium-activated mitochondrial calcium uniporters (MCUs) (Cali et al., 2012). Although MCU calcium affinity is low, their effect on calcium concentration is significant as they mediate calcium inflow in response to the negative membrane potential/calcium gradient created by pumping protons across the membrane during oxidative phosphorylation. Thus, MCUs are functionally dependent on both intracellular calcium concentration and energy demand (Tsai et al., 2017). The links between calcium and energy are further strengthened by the calcium-dependent activation of three metabolic enzymes, pyruvate, α-ketoglutarate, and isocitrate dehydrogenases, all of which function in the tricarboxylic acid cycle (Cali et al., 2012). MCU function also depends on the proximity of the mitochondria to other calcium regulating organelles, including the endoplasmic reticulum, sarcoplasmic reticulum, and plasma membrane, which can alter the local calcium concentration (Kirichok et al., 2004; Rizzuto et al., 2012).
Cellular calcium homeostasis, whereby nanomolar levels of free calcium are found in the cytosol, is maintained, at least in part, via effective buffering mechanisms, including pH and phosphate/adenosine availability. Additional intermitochondrial buffering mechanisms involve calcium efflux via electrogenic Ca2+/3Na+ exchangers (mNCXs) and/or electroneutral Ca2+/2H+ exchangers (mHCXs) located on the inner mitochondrial membrane. mNCXs are the main efflux channels in excitable tissues, including RGCs, while mHCXs are found mainly in non-excitable tissues (Hoppe, 2010). Interestingly, these efflux systems appear to change into influx pathways during glaucoma (Wojda et al., 2008). In aged neurons, the expression of calcium buffering proteins, including calbindin-D28k, calretinin, and parvalbumin, is also reduced (Bu et al., 2003). Together, these changes in efflux levels and calcium buffering protein expression significantly alter calcium gradients, cytosolic calcium levels (Williams et al., 2013), and mitochondrial membrane polarization (Wojda et al., 2008), resulting in altered/inefficient oxidative phosphorylation, ROS accumulation, downstream changes in mitochondrial function, and neuronal cell survival.
In retinal neurons, intracellular free calcium overload triggers calpain activation which can subsequently initiate apoptotic cascades (Sharma and Rohrer, 2004; Huang et al., 2010; Kar et al., 2010). Calpain is a calcium-dependent cysteine protease that, once activated, cleaves pro-apoptotic B-cell lymphoma (Bcl)-2 family members as well as apoptosis-inducing factor (AIF) and inner membrane mNCXs (Vosler et al., 2008). Calpain activation is also related to the formation and opening of mitochondrial permeability transition pores (mPTPs) (Cali et al., 2012; Bernardi and Di Lisa, 2015). mPTPs are multi-protein complexes that facilitate calcium efflux. However, unlike mNCXs, once the mPTPs are opened, the inner membrane is irreversibly permeabilized, resulting in uncontrolled dissipation of the electrochemical gradient, ATP depletion, ROS production, cytochrome C efflux, and mitochondrial swelling (Rasheed et al., 2017). Notably, increased cytoplasmic cytochrome C levels not only facilitate additional calpain activation, but the coupling of cytochrome C with apoptosis protease-activating factor (APAF)-1 results in apoptosome formation. Apoptosomes recruit and activate caspase-9 and downstream caspase-mediated apoptosis. Interestingly, activation of various caspases also feeds back into the process to further activate pro-apoptotic Bcl-2 proteins family members and increase mitochondrial permeability.
Calcium-Related Drug Treatments in the Ocular Environment
Various calcium regulating therapies have been investigated for their use in treating visual neurodegeneration (Kamel et al., 2017). Indeed, in the dorsal lateral geniculate nucleus and superior colliculus as well as RGCs, lomerizine, a well-known plasma membrane calcium channel blocker, has been used to manage neuronal degeneration (Ito et al., 2010; Selt et al., 2010). Various combinations of calcium channel inhibitors, including the L-/N-type channel blocker amlodipine, T-type channel blocker amiloride, α-amino-3-hydroxy-5-methyl-4-isoxazolepropionic acid (AMPA) receptor blockers, and purinergic receptor blockers, also increase RGC survival, reduce axonal degeneration, and increase axonal regeneration in both partial optic nerve transection (Savigni et al., 2013) and crush models (Ribas et al., 2017). However, the effects on visual function preservation were not significant.
In glaucoma and retinitis pigmentosa models, inhibition of calpain signaling has also been demonstrated to be beneficial for RGC and photoreceptor survival, respectively. This is not surprising as the detrimental effects of calcium overload are mediated largely through calpain activation. Latanoprost, an ocular anti-hypertension drug, for example, modulates its neuroprotective effects in this calpain-mediated manner (Yamamoto et al., 2017).
Unfortunately, beyond these studies using agents against calcium signaling in the plasma membrane, other mitochondria-specific calcium channel regulators to block detrimental calcium changes have not been intensively studied and none have been investigated as a glaucoma treatment option. Notably, calcium can be transported across the outer and inner mitochondrial membranes via VDACs and MCUs, respectively, as well as during unregulated diffusion through mPTPs, making each of these protein complexes a potential target for calcium regulation during disease. While a number of agents have been used to block mPTPs (Kajitani et al., 2007; Halestrap and Richardson, 2015), treatment with these agents leaves the causative upstream changes in calcium concentration and channel function largely unchecked. Thus, targeting VDACs and/or MCUs and avoiding mPTP formation altogether would potentially be more advantageous. For example, an anti-VDAC antibody has been shown to reduce cytochrome C release from mitochondria (Madesh and Hajnóczky, 2001). Unfortunately, directly altering VDAC function in this manner can also manipulate the transport of other essential metabolites (Camara et al., 2017). In cancer, blocking VDACs results in apoptosis (Shoshan-Barmatz et al., 2017), which is counterproductive to the cellular rescue required during glaucoma treatment. Alternatively, an MCU blocker, Ru360, was demonstrated to alter ion transport through these channels as well as block iron overload and has the advantage of having minimal effects on other cellular functions (Sripetchwandee et al., 2013). This same blocker was also previously shown to prevent the accumulation of mitochondrial free calcium despite high cytosolic free calcium concentrations in post-ischaemic rat heart cells (de Jesús García-Rivas et al., 2005). Lastly, this drug also maintains normal oxidative phosphorylation levels and prevents mPTP opening, while other organelles and cellular processes are unaffected, making it a drug of interest for glaucoma therapy.
Mitochondrial-Specific Drug Delivery as a Means to Treat Glaucoma
Organelle-specific drug targeting itself is not novel, being reviewed in multiple excellent publications (Sakhrani and Padh, 2013; Zhang and Zhang, 2016). While mitochondrial-specific drug targeting has not been applied to glaucoma, researchers have been actively proposing new mitochondrial delivery/transporter systems to target this organelle in other diseases. For example, a liposomal-based carrier was recently described that uses octaarginine modification, electrostatic attraction, and membrane fusion to promote mitochondrial uptake (Yamada and Harashima, 2017). This style of “MITO-porter” was then used to deliver coenzyme Q10 in mice with hepatic ischemic/reperfusion injuries and mediated a significant decrease in serum alanine aminotransferase (ALT) (Yamada et al., 2015). Another study utilized a MITO-porter system to target doxorubicin to the mitochondria of drug-resistant cancer cells, successfully destroying these cells (Yamada et al., 2017). Other nanotechnology techniques have also been employed, including a recent hybrid of polylactide-co-glycolide nanoparticles and mitochondria-penetrating particles (Selmin et al., 2017; Figure 1, bottom panel). Taken together, the evidence emerging from these investigations provides a solid foundation for the continued study of these delivery systems in other cellular contexts.
Delivery of agents used to modulate channel function along with the expression of other essential compounds (e.g., cytochrome C, ATP, etc.,) in concentrated amounts directly to the mitochondria during glaucoma using these systems would allow some of the downstream detrimental changes to be managed before vision loss. While some current (e.g., Ru360) and future drugs targeting mitochondrial calcium channels already innately target the mitochondria, the use of MITO-porters and similar delivery systems would not only allow higher concentrations to be delivered, but would also avoid any unknown effects on other organelles. Furthermore, delivery systems could also be used to package multiple drugs/compounds together in order to have the greatest therapeutic effect. Ultimately, these drugs would collectively reduce calcium efflux and restore calcium homeostasis as well as prevent mPTP formation, ATP depletion, ROS production, and cytochrome C dissipation. Doing so would prevent the second wave of apoptosis, allowing the cells to function normally even after the initial insult. While these drug delivery systems are not currently used as an ocular disease treatment, their potential to transport drugs to the retina and/or optic nerve/visual cortex that will subsequently manipulate mitochondrial function is a promising research avenue for novel treatment development for glaucoma as well as other retinal pathologies.
Conclusions
Mitochondrial dysfunction and the associated changes in calcium homeostasis, ROS production, and energy supply are intimately related to RGC death/dysfunction during glaucoma, making it an attractive treatment target. Mitochondrial-targeting drug delivery systems, which have been developed and validated in other cellular environments, could potentially avoid these issues by packaging multiple drugs and delivering them at high concentrations directly to the mitochondria. In discussing the recent advances in these techniques within the context of mitochondrial calcium regulation during glaucoma for the first time, we pose the question: Is this the future of glaucoma treatment? The relationships highlighted in multiple keystone studies investigating glaucoma and mitochondrial disease/aging in addition to the essential role of calcium signaling in these processes indicate an affirmative answer. Thus, while the full potential of these systems has yet to be fully established, we believe that mitochondrial-specific delivery of calcium channel regulators could effectively change how glaucoma and other neurodegenerative diseases affecting the retina are treated.
Author Contributions
All authors listed have made a substantial, direct and intellectual contribution to the work, and approved it for publication.
Funding
This work was supported by a grant from Seed Funding for Basic Science Research at the University of Hong Kong.
Conflict of Interest Statement
The authors declare that the research was conducted in the absence of any commercial or financial relationships that could be construed as a potential conflict of interest.
References
Abelson, M. B., Gilbert, C. M., and Smith, L. M. (1988). Sustained reduction of intraocular pressure in humans with the calcium channel blocker verapamil. Am. J. Ophthalmol. 105, 155–159. doi: 10.1016/0002-9394(88)90179-1
Abu-Amero, K. K., Morales, J., and Bosley, T. M. (2006). Mitochondrial abnormalities in patients with primary open-angle glaucoma. Invest. Ophthalmol. Vis. Sci. 47, 2533–2541. doi: 10.1167/iovs.05-1639
Almasieh, M., and Levin, L. A. (2017). Neuroprotection in glaucoma: animal models and clinical trials. Annu. Rev. Vis. Sci. 3, 91–120. doi: 10.1146/annurev-vision-102016-061422
Almasieh, M., Wilson, A. M., Morquette, B., Cueva Vargas, J. L., and Di Polo, A. (2012). The molecular basis of retinal ganglion cell death in glaucoma. Prog. Retin. Eye Res. 31, 152–181. doi: 10.1016/j.preteyeres.2011.11.002
Araie, M., and Mayama, C. (2011). Use of calcium channel blockers for glaucoma. Prog. Retin. Eye Res. 30, 54–71. doi: 10.1016/j.preteyeres.2010.09.002
Augustine, G. J., Charlton, M. P., and Smith, S. J. (1987). Calcium action in synaptic transmitter release. Annu. Rev. Neurosci. 10, 633–693. doi: 10.1146/annurev.ne.10.030187.003221
Babcock, D. F., Herrington, J., Goodwin, P. C., Park, Y. B., and Hille, B. (1997). Mitochondrial participation in the intracellular Ca2+ network. J. Cell Biol. 136, 833–844. doi: 10.1083/jcb.136.4.833
Ballard, J. W., and Whitlock, M. C. (2004). The incomplete natural history of mitochondria. Mol. Ecol. 13, 729–744. doi: 10.1046/j.1365-294X.2003.02063.x
Barot, M., Gokulgandhi, M. R., and Mitra, A. K. (2011). Mitochondrial dysfunction in retinal diseases. Curr. Eye Res. 36, 1069–1077. doi: 10.3109/02713683.2011.607536
Battigelli, A., Russier, J., Venturelli, E., Fabbro, C., Petronilli, V., Bernardi, P., et al. (2013). Peptide-based carbon nanotubes for mitochondrial targeting. Nanoscale 5, 9110–9117. doi: 10.1039/c3nr02694a
Beatty, J. F., Krupin, T., Nichols, P. F., and Becker, B. (1984). Elevation of intraocular pressure by calcium channel blockers. Arch. Ophthalmol. 102, 1072–1076. doi: 10.1001/archopht.1984.01040030866035
Bernardi, P., and Di Lisa, F. (2015). The mitochondrial permeability transition pore: molecular nature and role as a target in cardioprotection. J. Mol. Cell. Cardiol. 78, 100–106. doi: 10.1016/j.yjmcc.2014.09.023
Berridge, M. J. (1997). Elementary and global aspects of calcium signalling. J. Physiol. 499(Pt 2), 291–306. doi: 10.1113/jphysiol.1997.sp021927
Breuer, M. E., Koopman, W. J., Koene, S., Nooteboom, M., Rodenburg, R. J., Willems, P. H., et al. (2013). The role of mitochondrial OXPHOS dysfunction in the development of neurologic diseases. Neurobiol. Dis. 51, 27–34. doi: 10.1016/j.nbd.2012.03.007
Brini, M., and Carafoli, E. (2000). Calcium signalling: a historical account, recent developments and future perspectives. Cell. Mol. Life Sci. 57, 354–370. doi: 10.1007/PL00000698
Brookes, P. S., Yoon, Y., Robotham, J. L., Anders, M. W., and Sheu, S. S. (2004). Calcium, ATP, and ROS: a mitochondrial love-hate triangle. Am. J. Physiol. Cell Physiol. 287, C817–C833. doi: 10.1152/ajpcell.00139.2004
Bu, J., Sathyendra, V., Nagykery, N., and Geula, C. (2003). Age-related changes in calbindin-D28k, calretinin, and parvalbumin-immunoreactive neurons in the human cerebral cortex. Exp. Neurol. 182, 220–231. doi: 10.1016/S0014-4886(03)00094-3
Calabrese, V., Scapagnini, G., Giuffrida Stella, A. M., Bates, T. E., and Clark, J. B. (2001). Mitochondrial involvement in brain function and dysfunction: relevance to aging, neurodegenerative disorders and longevity. Neurochem. Res. 26, 739–764. doi: 10.1023/A:1010955807739
Cali, T., Ottolini, D., and Brini, M. (2012). Mitochondrial Ca2+ as a key regulator of mitochondrial activities. Adv. Exp. Med. Biol. 942, 53–73. doi: 10.1007/978-94-007-2869-1_3
Calkins, D. J. (2012). Critical pathogenic events underlying progression of neurodegeneration in glaucoma. Prog. Retin. Eye Res. 31, 702–719. doi: 10.1016/j.preteyeres.2012.07.001
Calkins, D. J., and Horner, P. J. (2012). The cell and molecular biology of glaucoma: axonopathy and the brain. Invest. Ophthalmol. Vis. Sci. 53, 2482–2484. doi: 10.1167/iovs.12-9483i
Camara, A. K. S., Zhou, Y., Wen, P. C., Tajkhorshid, E., and Kwok, W. M. (2017). Mitochondrial VDAC1: a key gatekeeper as potential therapeutic target. Front. Physiol. 8:460. doi: 10.3389/fphys.2017.00460
Carafoli, E. (1986). Membrane transport in the cellular homeostasis of calcium. J. Cardiovasc. Pharmacol. 8 (Suppl. 8), S3–S6. doi: 10.1097/00005344-198600088-00002
Carafoli, E., and Klee, C. (1999). Calcium as a Cellular Regulator. New York, NY: Oxford University Press.
Carafoli, E., Tiozzo, R., Lugli, G., Crovetti, F., and Kratzing, C. (1974). The release of calcium from heart mitochondria by sodium. J. Mol. Cell. Cardiol. 6, 361–371. doi: 10.1016/0022-2828(74)90077-7
Carelli, V., Ross-Cisneros, F. N., and Sadun, A. A. (2004). Mitochondrial dysfunction as a cause of optic neuropathies. Prog. Retin. Eye Res. 23, 53–89. doi: 10.1016/j.preteyeres.2003.10.003
Casson, R. J., Chidlow, G., Wood, J. P., Crowston, J. G., and Goldberg, I. (2012). Definition of glaucoma: clinical and experimental concepts. Clin. Exp. Ophthalmol. 40, 341–349. doi: 10.1111/j.1442-9071.2012.02773.x
Chan, M. S., Liu, L. S., Leung, H. M., and Lo, P. K. (2017). Cancer-cell-specific mitochondria-targeted drug delivery by dual-ligand-functionalized nanodiamonds circumvent drug resistance. ACS Appl. Mater. Interfaces 9, 11780–11789. doi: 10.1021/acsami.6b15954
Chance, B. (1965). The energy-linked reaction of calcium with mitochondria. J. Biol. Chem. 240, 2729–2748.
Chang, E. E., and Goldberg, J. L. (2012). Glaucoma 2.0: neuroprotection, neuroregeneration, neuroenhancement. Ophthalmology 119, 979–986. doi: 10.1016/j.ophtha.2011.11.003
Chhetri, J., and Gueven, N. (2016). Targeting mitochondrial function to protect against vision loss. Expert Opin. Ther. Targets 20, 721–736. doi: 10.1517/14728222.2015.1134489
Chinnery, P. F. (2015). Mitochondrial disease in adults: what's old and what's new? EMBO Mol. Med. 7, 1503–1512. doi: 10.15252/emmm.201505079
Chong, R. S., and Martin, K. R. (2015). Glial cell interactions and glaucoma. Curr. Opin. Ophthalmol. 26, 73–77. doi: 10.1097/ICU.0000000000000125
Chouchani, E. T., Pell, V. R., Gaude, E., Aksentijević, D., Sundier, S. Y., Robb, E. L., et al. (2014). Ischaemic accumulation of succinate controls reperfusion injury through mitochondrial ROS. Nature 515, 431–435. doi: 10.1038/nature13909
Chrysostomou, V., Rezania, F., Trounce, I. A., and Crowston, J. G. (2013). Oxidative stress and mitochondrial dysfunction in glaucoma. Curr. Opin. Pharmacol. 13, 12–15. doi: 10.1016/j.coph.2012.09.008
Consoli, D., and Ramlogan, R. (2008). Out of sight: problem sequences and epistemic boundaries of medical know-how on glaucoma. J. Evol. Econ. 18, 31–56. doi: 10.1007/s00191-007-0074-4
Cortes, L. A., Castro, L., Pesce, B., Maya, J. D., Ferreira, J., Castro-Castillo, V., et al. (2015). Novel gallate triphenylphosphonium derivatives with potent antichagasic activity. PLoS ONE 10:e0136852. doi: 10.1371/journal.pone.0136852
Crish, S. D., and Calkins, D. J. (2011). Neurodegeneration in glaucoma: progression and calcium-dependent intracellular mechanisms. Neuroscience 176, 1–11. doi: 10.1016/j.neuroscience.2010.12.036
Crish, S. D., and Calkins, D. J. (2015). Central visual pathways in glaucoma: evidence for distal mechanisms of neuronal self-repair. J. Neuroophthalmol. 35(Suppl. 1), S29–S37. doi: 10.1097/WNO.0000000000000291
Cui, H., Li, F., Chen, D., Wang, G., Truong, C. K., Enns, G. M., et al. (2013). Comprehensive next-generation sequence analyses of the entire mitochondrial genome reveal new insights into the molecular diagnosis of mitochondrial DNA disorders. Genet. Med. 15, 388–394. doi: 10.1038/gim.2012.144
de Jesús García-Rivas, G., Guerrero-Hernández, A., Guerrero-Serna, G., Rodríguez-Zavala, J. S., and Zazueta, C. (2005). Inhibition of the mitochondrial calcium uniporter by the oxo-bridged dinuclear ruthenium amine complex (Ru360) prevents from irreversible injury in post-ischemic rat heart. FEBS J. 272, 3477–3488. doi: 10.1111/j.1742-4658.2005.04771.x
Del Castillo, J., and Katz, B. (1956). Biophysical aspects of neuro-muscular transmission. Prog. Biophys. Biophys. Chem. 6, 121–170.
DiMauro, S. (2011). A history of mitochondrial diseases. J. Inherit. Metab. Dis. 34, 261–276. doi: 10.1007/s10545-010-9082-x
DiMauro, S., Bonilla, E., Zeviani, M., Nakagawa, M., and DeVivo, D. C. (1985). Mitochondrial myopathies. Ann. Neurol. 17, 521–538. doi: 10.1002/ana.410170602
Drance, S. M. (1975). Doyne Memorial Lecture, 1975. Correlation of optic nerve and visual field defects in simple glaucoma. Trans. Ophthalmol. Soc. U. K. 95, 288–296.
Drance, S. M. (1992). Bowman Lecture. Glaucoma–changing concepts. Eye (Lond) 6(Pt 4), 337–345. doi: 10.1038/eye.1992.69
D'Souza, G. G., Rammohan, R., Cheng, S. M., Torchilin, V. P., and Weissig, V. (2003). DQAsome-mediated delivery of plasmid DNA toward mitochondria in living cells. J. Control. Release 92, 189–197. doi: 10.1016/S0168-3659(03)00297-9
Engel, W. K., and Cunningham, G. G. (1963). Rapid examination of muscle tissue. An improved trichrome method for fresh-frozen biopsy sections. Neurology 13, 919–923. doi: 10.1212/WNL.13.11.919
Filosto, M., Scarpelli, M., Cotelli, M. S., Vielmi, V., Todeschini, A., Gregorelli, V., et al. (2011). The role of mitochondria in neurodegenerative diseases. J. Neurol. 258, 1763–1774. doi: 10.1007/s00415-011-6104-z
Finsterer, J. (2006). Central nervous system manifestations of mitochondrial disorders. Acta Neurol. Scand. 114, 217–238. doi: 10.1111/j.1600-0404.2006.00671.x
Flammer, J., Orgül, S., Costa, V. P., Orzalesi, N., Krieglstein, G. K., Serra, L. M., et al. (2002). The impact of ocular blood flow in glaucoma. Prog. Retin. Eye Res. 21, 359–393. doi: 10.1016/S1350-9462(02)00008-3
Geijssen, H. C., and Greve, E. L. (1990). Focal ischaemic normal pressure glaucoma versus high pressure glaucoma. Doc. Ophthalmol. 75, 291–301. doi: 10.1007/BF00164843
Godfraind, T. (2004). “Historical perspective: from early steps on calcium research to the identification of calcium antagonist prototypes and of the signaling functions of Ca2+,” in Calcium Channel Blockers. Milestones in Drug Therapy, eds M. J. Parnham and J. Bruinvels (Basel: Birkhäuser), 1–9.
Goldberg, I. (1994). Therapeutic possibilities for calcium channel blockers in glaucoma. Prog. Retin. Eye Res. 13, 593–604. doi: 10.1016/1350-9462(94)90023-X
Gueven, N., Nadikudi, M., Daniel, A., and Chhetri, J. (2016). Targeting mitochondrial function to treat optic neuropathy. Mitochondrion 36, 7–14. doi: 10.1016/j.mito.2016.07.013
Gunter, K. K., and Gunter, T. E. (1994). Transport of calcium by mitochondria. J. Bioenerg. Biomembr. 26, 471–485. doi: 10.1007/BF00762732
Hajnóczky, G., Robb-Gaspers, L. D., Seitz, M. B., and Thomas, A. P. (1995). Decoding of cytosolic calcium oscillations in the mitochondria. Cell 82, 415–424. doi: 10.1016/0092-8674(95)90430-1
Halestrap, A. P., and Richardson, A. P. (2015). The mitochondrial permeability transition: a current perspective on its identity and role in ischaemia/reperfusion injury. J. Mol. Cell. Cardiol. 78, 129–141. doi: 10.1016/j.yjmcc.2014.08.018
Ham, P. B. III., and Raju, R. (2016). Mitochondrial function in hypoxic ischemic injury and influence of aging. Prog Neurobiol. 157, 92–116. doi: 10.1016/j.pneurobio.2016.06.006
Harman, D. (1972). The biologic clock: the mitochondria? J. Am. Geriatr. Soc. 20, 145–147. doi: 10.1111/j.1532-5415.1972.tb00787.x
Heilbrunn, L. V., and Wiercinski, F. J. (1947). The action of various cations on muscle protoplasm. J. Cell. Comp. Physiol. 29, 15–32. doi: 10.1002/jcp.1030290103
Hodgkin, A. L., and Keynes, R. D. (1957). Movements of labelled calcium in squid giant axons. J. Physiol. 138, 253–281. doi: 10.1113/jphysiol.1957.sp005850
Hofmann, F., Biel, M., and Flockerzi, V. (1994). Molecular basis for Ca2+ channel diversity. Annu. Rev. Neurosci. 17, 399–418. doi: 10.1146/annurev.ne.17.030194.002151
Hoppe, U. C. (2010). Mitochondrial calcium channels. FEBS Lett. 584, 1975–1981. doi: 10.1016/j.febslet.2010.04.017
Hoyt, W. F., Frisén, L., and Newman, N. M. (1973). Fundoscopy of nerve fiber layer defects in glaucoma. Invest. Ophthalmol. 12, 814–829.
Huang, W., Fileta, J., Rawe, I., Qu, J., and Grosskreutz, C. L. (2010). Calpain activation in experimental glaucoma. Invest. Ophthalmol. Vis. Sci. 51, 3049–3054. doi: 10.1167/iovs.09-4364
Hurst, S., Hoek, J., and Sheu, S. S. (2017). Mitochondrial Ca2+ and regulation of the permeability transition pore. J. Bioenerg. Biomembr. 49, 27–47. doi: 10.1007/s10863-016-9672-x
Ito, Y., Nakamura, S., Tanaka, H., Tsuruma, K., Shimazawa, M., Araie, M., et al. (2010). Lomerizine, a Ca2+ channel blocker, protects against neuronal degeneration within the visual center of the brain after retinal damage in mice. CNS Neurosci. Ther. 16, 103–114. doi: 10.1111/j.1755-5949.2009.00081.x
Izzotti, A., Bagnis, A., and Saccà, S. C. (2006). The role of oxidative stress in glaucoma. Mutat. Res. 612, 105–114. doi: 10.1016/j.mrrev.2005.11.001
Izzotti, A., Saccà, S. C., Longobardi, M., and Cartiglia, C. (2010). Mitochondrial damage in the trabecular meshwork of patients with glaucoma. Arch. Ophthalmol. 128, 724–730. doi: 10.1001/archophthalmol.2010.87
Jarrett, S. G., Lewin, A. S., and Boulton, M. E. (2010). The importance of mitochondria in age-related and inherited eye disorders. Ophthalmic Res. 44, 179–190. doi: 10.1159/000316480
Jeoung, J. W., Seong, M. W., Park, S. S., Kim, D. M., Kim, S. H., and Park, K. H. (2014). Mitochondrial DNA variant discovery in normal-tension glaucoma patients by next-generation sequencing. Invest. Ophthalmol. Vis. Sci. 55, 986–992. doi: 10.1167/iovs.13-12968
Johns, D. R. (1995). The ophthalmologic manifestations of mitochondrial disease. Semin. Ophthalmol. 10, 295–302. doi: 10.3109/08820539509063800
Jonas, J. B., Aung, T., Bourne, R. R., Bron, A. M., Ritch, R., and Panda-Jonas, S. (2017). Glaucoma. Lancet 390, 2183–2193. doi: 10.1016/S0140-6736(17)31469-1
Jonas, J. B., and Budde, W. M. (2000). Diagnosis and pathogenesis of glaucomatous optic neuropathy: morphological aspects. Prog. Retin. Eye Res. 19, 1–40. doi: 10.1016/S1350-9462(99)00002-6
Ju, W. K., Kim, K. Y., Duong-Polk, K. X., Lindsey, J. D., Ellisman, M. H., and Weinreb, R. N. (2010). Increased optic atrophy type 1 expression protects retinal ganglion cells in a mouse model of glaucoma. Mol. Vis. 16, 1331–1342.
Ju, W. K., Liu, Q., Kim, K. Y., Crowston, J. G., Lindsey, J. D., Agarwal, N., et al. (2007). Elevated hydrostatic pressure triggers mitochondrial fission and decreases cellular ATP in differentiated RGC-5 cells. Invest. Ophthalmol. Vis. Sci. 48, 2145–2151. doi: 10.1167/iovs.06-0573
Kajitani, N., Kobuchi, H., Fujita, H., Yano, H., Fujiwara, T., Yasuda, T., et al. (2007). Mechanism of A23187-induced apoptosis in HL-60 cells: dependency on mitochondrial permeability transition but not on NADPH oxidase. Biosci. Biotechnol. Biochem. 71, 2701–2711. doi: 10.1271/bbb.70304
Kamada, T., and Kinosita, H. (1943). Disturbances initiated from naked surface of muscle protoplasm. Jpn. J. Zool. 10, 469–493.
Kamel, K., Farrell, M., and O'Brien, C. (2017). Mitochondrial dysfunction in ocular disease: focus on glaucoma. Mitochondrion 35, 44–53. doi: 10.1016/j.mito.2017.05.004
Kar, P., Samanta, K., Shaikh, S., Chowdhury, A., Chakraborti, T., and Chakraborti, S. (2010). Mitochondrial calpain system: an overview. Arch. Biochem. Biophys. 495, 1–7. doi: 10.1016/j.abb.2009.12.020
Karaki, H., Ozaki, H., Hori, M., Mitsui-Saito, M., Amano, K., Harada, K., et al. (1997). Calcium movements, distribution, and functions in smooth muscle. Pharmacol. Rev. 49, 157–230.
Keinan, N., Pahima, H., Ben-Hail, D., and Shoshan-Barmatz, V. (2013). The role of calcium in VDAC1 oligomerization and mitochondria-mediated apoptosis. Biochim. Biophys. Acta 1833, 1745–1754. doi: 10.1016/j.bbamcr.2013.03.017
Kelly, S. P., and Walley, T. J. (1988). Effect of the calcium antagonist nifedipine on intraocular pressure in normal subjects. Br. J. Ophthalmol. 72, 216–218. doi: 10.1136/bjo.72.3.216
Kirichok, Y., Krapivinsky, G., and Clapham, D. E. (2004). The mitochondrial calcium uniporter is a highly selective ion channel. Nature 427, 360–364. doi: 10.1038/nature02246
Kitazawa, Y., Shirai, H., and Go, F. J. (1989). The effect of Ca2+ -antagonist on visual field in low-tension glaucoma. Graefes Arch. Clin. Exp. Ophthalmol. 227, 408–412. doi: 10.1007/BF02172889
Kohlhardt, M., Bauer, B., Krause, H., and Fleckenstein, A. (1972a). Differentiation of the transmembrane Na and Ca channels in mammalian cardiac fibres by the use of specific inhibitors. Pflugers Arch. 335, 309–322. doi: 10.1007/BF00586221
Kohlhardt, M., Bauer, B., Krause, H., and Fleckenstein, A. (1972b). New selective inhibitors of the transmembrane Ca conductivity in mammalian myocardial fibres. Studies with the voltage clamp technique. Experientia 28, 288–289. doi: 10.1007/BF01928693
Kohlhardt, M., Bauer, B., Krause, H., and Fleckenstein, A. (1973). Selective inhibition of the transmembrane Ca conductivity of mammalian myocardial fibres by Ni, Co and Mn ions. Pflugers Arch. 338, 115–123. doi: 10.1007/BF00592747
Kohlhardt, M., and Fleckenstein, A. (1980). Inhibition of the slow inward current by nifedipine in mammalian ventricular myocardium. Br. J. Clin. Pract. 8(Suppl. 1), 3–8.
Kolishetti, N., Dhar, S., Valencia, P. M., Lin, L. Q., Karnik, R., Lippard, S. J., et al. (2010). Engineering of self-assembled nanoparticle platform for precisely controlled combination drug therapy. Proc. Natl. Acad. Sci. U.S.A. 107, 17939–17944. doi: 10.1073/pnas.1011368107
Kong, G. Y., Van Bergen, N. J., Trounce, I. A., and Crowston, J. G. (2009). Mitochondrial dysfunction and glaucoma. J. Glaucoma 18, 93–100. doi: 10.1097/IJG.0b013e318181284f
Land, J. M., and Clark, J. B. (1979). Mitochondrial myopathies. Biochem. Soc. Trans. 7, 231–245. doi: 10.1042/bst0070231
Lascaratos, G., Chau, K. Y., Zhu, H., Gkotsi, D., King, R., Gout, I., et al. (2015). Resistance to the most common optic neuropathy is associated with systemic mitochondrial efficiency. Neurobiol. Dis. 82, 78–85. doi: 10.1016/j.nbd.2015.05.012
Lascaratos, G., Garway-Heath, D. F., Willoughby, C. E., Chau, K. Y., and Schapira, A. H. (2012). Mitochondrial dysfunction in glaucoma: understanding genetic influences. Mitochondrion 12, 202–212. doi: 10.1016/j.mito.2011.11.004
Lee, D., Shim, M. S., Kim, K. Y., Noh, Y. H., Kim, H., Kim, S. Y., et al. (2014). Coenzyme Q10 inhibits glutamate excitotoxicity and oxidative stress-mediated mitochondrial alteration in a mouse model of glaucoma. Invest. Ophthalmol. Vis. Sci. 55, 993–1005. doi: 10.1167/iovs.13-12564
Lee, S., Sheck, L., Crowston, J. G., Van Bergen, N. J., O'Neill, E. C., O'Hare, F., et al. (2012). Impaired complex-I-linked respiration and ATP synthesis in primary open-angle glaucoma patient lymphoblasts. Invest. Ophthalmol. Vis. Sci. 53, 2431–2437. doi: 10.1167/iovs.12-9596
Lee, S., Van Bergen, N. J., Kong, G. Y., Chrysostomou, V., Waugh, H. S., O'Neill, E. C., et al. (2011). Mitochondrial dysfunction in glaucoma and emerging bioenergetic therapies. Exp. Eye Res. 93, 204–212. doi: 10.1016/j.exer.2010.07.015
Levkovitch-Verbin, H., Quigley, H. A., Martin, K. R., Zack, D. J., Pease, M. E., and Valenta, D. F. (2003). A model to study differences between primary and secondary degeneration of retinal ganglion cells in rats by partial optic nerve transection. Invest. Ophthalmol. Vis. Sci. 44, 3388–3393. doi: 10.1167/iovs.02-0646
Llinás, R., Steinberg, I. Z., and Walton, K. (1976). Presynaptic calcium currents and their relation to synaptic transmission: voltage clamp study in squid giant synapse and theoretical model for the calcium gate. Proc. Natl. Acad. Sci. U.S.A. 73, 2918–2922. doi: 10.1073/pnas.73.8.2918
Lopez Sanchez, M. I., Crowston, J. G., Mackey, D. A., and Trounce, I. A. (2016). Emerging mitochondrial therapeutic targets in optic neuropathies. Pharmacol. Ther. 165, 132–152. doi: 10.1016/j.pharmthera.2016.06.004
Luft, R. (1994). The development of mitochondrial medicine. Proc. Natl. Acad. Sci. U.S.A. 91, 8731–8738. doi: 10.1073/pnas.91.19.8731
Luft, R., Ikkos, D., Palmieri, G., Ernster, L., and Afzelius, B. (1962). A case of severe hypermetabolism of nonthyroid origin with a defect in the maintenance of mitochondrial respiratory control: a correlated clinical, biochemical, and morphological study. J. Clin. Invest. 41, 1776–1804. doi: 10.1172/JCI104637
Luongo, T. S., Lambert, J. P., Gross, P., Nwokedi, M., Lombardi, A. A., Shanmughapriya, S., et al. (2017). The mitochondrial Na+/Ca2+ exchanger is essential for Ca2+ homeostasis and viability. Nature 545, 93–97. doi: 10.1038/nature22082
Madesh, M., and Hajnóczky, G. (2001). VDAC-dependent permeabilization of the outer mitochondrial membrane by superoxide induces rapid and massive cytochrome c release. J. Cell. Bio. 155, 1003–1016. doi: 10.1083/jcb.200105057
Magner, M., Kolárová, H., Honzik, T., Švandová, I., and Zeman, J. (2015). Clinical manifestation of mitochondrial diseases. Dev. Period Med. 19, 441–449.
Mann, M., Haq, W., Zabel, T., Guenther, E., Zrenner, E., and Ladewig, T. (2005). Age-dependent changes in the regulation mechanisms for intracellular calcium ions in ganglion cells of the mouse retina. Eur. J. Neurosci. 22, 2735–2743. doi: 10.1111/j.1460-9568.2005.04475.x
Marrache, S., and Dhar, S. (2012). Engineering of blended nanoparticle platform for delivery of mitochondria-acting therapeutics. Proc. Natl. Acad. Sci. U.S.A. 109, 16288–16293. doi: 10.1073/pnas.1210096109
Miledi, R. (1973). Transmitter release induced by injection of calcium ions into nerve terminals. Proc. R. Soc. Lond. B. Biol. Sci. 183, 421–425. doi: 10.1098/rspb.1973.0026
Monica, M. L., Hesse, R. J., and Messerli, F. H. (1983). The effect of a calcium-channel blocking agent on intraocular pressure. Am. J. Ophthalmol. 96:814. doi: 10.1016/S0002-9394(14)71934-8
Neher, E., and Sakaba, T. (2008). Multiple roles of calcium ions in the regulation of neurotransmitter release. Neuron 59, 861–872. doi: 10.1016/j.neuron.2008.08.019
Netland, P. A., Chaturvedi, N., and Dreyer, E. B. (1993). Calcium channel blockers in the management of low-tension and open-angle glaucoma. Am. J. Ophthalmol. 115, 608–613. doi: 10.1016/S0002-9394(14)71458-8
Netland, P. A., Feke, G. T., Konno, S., Goger, D. G., and Fujio, N. (1996). Optic nerve head circulation after topical calcium channel blocker. J. Glaucoma 5, 200–206. doi: 10.1097/00061198-199606000-00010
Netland, P. A., Grosskreutz, C. L., Feke, G. T., and Hart, L. J. (1995). Color Doppler ultrasound analysis of ocular circulation after topical calcium channel blocker. Am. J. Ophthalmol. 119, 694–700. doi: 10.1016/S0002-9394(14)72772-2
Newman, N. J., and Biousse, V. (2004). Hereditary optic neuropathies. Eye 18, 1144–1160. doi: 10.1038/sj.eye.6701591
Nguyen, D., Alavi, M. V., Kim, K. Y., Kang, T., Scott, R. T., Noh, Y. H., et al. (2011). A new vicious cycle involving glutamate excitotoxicity, oxidative stress and mitochondrial dynamics. Cell Death Dis. 2:e240. doi: 10.1038/cddis.2011.117
Ning, J., Huang, B., Wei, Z., Li, W., Zheng, H., Ma, L., et al. (2017). Mitochondria targeting and near-infrared fluorescence imaging of a novel heptamethine cyanine anticancer agent. Mol. Med. Rep. 15, 3761–3766. doi: 10.3892/mmr.2017.6451
Orth, M., and Schapira, A. H. (2001). Mitochondria and degenerative disorders. Am. J. Med. Genet. 106, 27–36. doi: 10.1002/ajmg.1425
Osborne, N. N., Álvarez, C. N., and del Olmo Aguado, S. (2014). Targeting mitochondrial dysfunction as in aging and glaucoma. Drug Discov. Today 19, 1613–1622. doi: 10.1016/j.drudis.2014.05.010
Osborne, N. N., and del Olmo-Aguado, S. (2013). Maintenance of retinal ganglion cell mitochondrial functions as a neuroprotective strategy in glaucoma. Curr. Opin. Pharmacol. 13, 16–22. doi: 10.1016/j.coph.2012.09.002
Osborne, N. N., Lascaratos, G., Bron, A. J., Chidlow, G., and Wood, J. P. (2006). A hypothesis to suggest that light is a risk factor in glaucoma and the mitochondrial optic neuropathies. Br. J. Ophthalmol. 90, 237–241. doi: 10.1136/bjo.2005.082230
Osborne, N. N., Li, G. Y., Ji, D., Mortiboys, H. J., and Jackson, S. (2008). Light affects mitochondria to cause apoptosis to cultured cells: possible relevance to ganglion cell death in certain optic neuropathies. J. Neurochem. 105, 2013–2028. doi: 10.1111/j.1471-4159.2008.05320.x
Osborne, N. N., Núñez-Álvarez, C., Del Olmo-Aguado, S., and Merrayo-Lloves, J. (2016a). Visual light effects on mitochondria: the potential implications in relation to glaucoma. Mitochondrion 36, 29–35. doi: 10.1016/j.mito.2016.11.009
Osborne, N. N., Núñez-Álvarez, C., Joglar, B., and Del Olmo-Aguado, S. (2016b). Glaucoma: focus on mitochondria in relation to pathogenesis and neuroprotection. Eur. J. Pharmacol. 787, 127–133. doi: 10.1016/j.ejphar.2016.04.032
Osborne, N. N., Wood, J. P., and Chidlow, G. (2005). Invited review: neuroprotective properties of certain beta-adrenoceptor antagonists used for the treatment of glaucoma. J. Ocul. Pharmacol. Ther. 21, 175–181. doi: 10.1089/jop.2005.21.175
Osborne, N. N., Wood, J. P., Chidlow, G., Casson, R., DeSantis, L., and Schmidt, K. G. (2004). Effectiveness of levobetaxolol and timolol at blunting retinal ischaemia is related to their calcium and sodium blocking activities: relevance to glaucoma. Brain Res. Bull. 62, 525–528. doi: 10.1016/S0361-9230(03)00070-4
Osborne, N. N., Wood, J. P., Cupido, A., Melena, J., and Chidlow, G. (2002). Topical flunarizine reduces IOP and protects the retina against ischemia-excitotoxicity. Invest. Ophthalmol. Vis. Sci. 43, 1456–1464.
Park, M. K., Ashby, M. C., Erdemli, G., Petersen, O. H., and Tepikin, A. V. (2001). Perinuclear, perigranular and sub-plasmalemmal mitochondria have distinct functions in the regulation of cellular calcium transport. EMBO J. 20, 1863–1874. doi: 10.1093/emboj/20.8.1863
Payne, L. J., Slagle, T. M., Cheeks, L. T., and Green, K. (1990). Effect of calcium channel blockers on intraocular pressure. Ophthalmic Res. 22, 337–341. doi: 10.1159/000267044
Petersen, O. H., Burdakov, D., and Tepikin, A. V. (1999). Polarity in intracellular calcium signaling. Bioessays 21, 851–860. doi: 10.1002/(SICI)1521-1878(199910)21:10<851::AID-BIES7>3.0.CO;2-F
Pinazo-Durán, M. D., Zanón-Moreno, V., Gallego-Pinazo, R., and García-Medina, J. J. (2015). Oxidative stress and mitochondrial failure in the pathogenesis of glaucoma neurodegeneration. Prog. Brain Res. 220, 127–153. doi: 10.1016/bs.pbr.2015.06.001
Pozzan, T., Rizzuto, R., Volpe, P., and Meldolesi, J. (1994). Molecular and cellular physiology of intracellular calcium stores. Physiol. Rev. 74, 595–636.
Putney, J. W. Jr., and McKay, R. R. (1999). Capacitative calcium entry channels. Bioessays 21, 38–46. doi: 10.1002/(SICI)1521-1878(199901)21:1<38::AID-BIES5>3.0.CO;2-S
Quigley, H. A., and Broman, A. T. (2006). The number of people with glaucoma worldwide in 2010 and 2020. Br. J. Ophthalmol. 90, 262–267. doi: 10.1136/bjo.2005.081224
Rasheed, M. Z., Tabassum, H., and Parvez, S. (2017). Mitochondrial permeability transition pore: a promising target for the treatment of Parkinson's disease. Protoplasma 254, 33–42. doi: 10.1007/s00709-015-0930-2
Reuter, H., Stevens, C. F., Tsien, R. W., and Yellen, G. (1982). Properties of single calcium channels in cardiac cell culture. Nature 297, 501–504. doi: 10.1038/297501a0
Ribas, V. T., Koch, J. C., Michel, U., Bähr, M., and Lingor, P. (2017). Attenuation of axonal degeneration by calcium channel inhibitors improves retinal ganglion cell survival and regeneration after optic nerve crush. Mol. Neurobiol. 54, 72–86. doi: 10.1007/s12035-015-9676-2
Ringer, S. (1883). A further contribution regarding the influence of the different constituents of the blood on the contraction of the heart. J. Physiol. 4, 29–42. doi: 10.1113/jphysiol.1883.sp000120
Rizzuto, R., Bernardi, P., and Pozzan, T. (2000). Mitochondria as all-round players of the calcium game. J. Physiol. 529 (Pt 1), 37–47. doi: 10.1111/j.1469-7793.2000.00037.x
Rizzuto, R., Brini, M., Murgia, M., and Pozzan, T. (1993). Microdomains with high Ca2+ close to IP3-sensitive channels that are sensed by neighboring mitochondria. Science 262, 744–747. doi: 10.1126/science.8235595
Rizzuto, R., De Stefani, D., Raffaello, A., and Mammucari, C. (2012). Mitochondria as sensors and regulators of calcium signalling. Nat. Rev. Mol. Cell Biol. 13, 566–578. doi: 10.1038/nrm3412
Robb-Gaspers, L. D., Burnett, P., Rutter, G. A., Denton, R. M., Rizzuto, R., and Thomas, A. P. (1998). Integrating cytosolic calcium signals into mitochondrial metabolic responses. EMBO J. 17, 4987–5000. doi: 10.1093/emboj/17.17.4987
Rutter, G. A., Burnett, P., Rizzuto, R., Brini, M., Murgia, M., Pozzan, T., et al. (1996). Subcellular imaging of intramitochondrial Ca2+ with recombinant targeted aequorin: significance for the regulation of pyruvate dehydrogenase activity. Proc. Natl. Acad. Sci. U.S.A. 93, 5489–5494. doi: 10.1073/pnas.93.11.5489
Rutter, G. A., Fasolato, C., and Rizzuto, R. (1998). Calcium and organelles: a two-sided story. Biochem. Biophys. Res. Commun. 253, 549–557. doi: 10.1006/bbrc.1998.9727
Sadighian, S., Rostamizadeh, K., Hosseini, M. J., Hamidi, M., and Hosseini-Monfared, H. (2017). Magnetic nanogels as dual triggered anticancer drug delivery: toxicity evaluation on isolated rat liver mitochondria. Toxicol. Lett. 278, 18–29. doi: 10.1016/j.toxlet.2017.06.004
Sakhrani, N. M., and Padh, H. (2013). Organelle targeting: third level of drug targeting. Drug Des. Devel. Ther. 7, 585–599. doi: 10.2147/DDDT.S45614
Santafé, J., Martínez de Ibarreta, M. J., Segarra, J., and Melena, J. (1997). A long-lasting hypotensive effect of topical diltiazem on the intraocular pressure in conscious rabbits. Naunyn Schmiedebergs. Arch. Pharmacol. 355, 645–650. doi: 10.1007/PL00004996
Santafé, J., Martínez de Ibarreta, M. J., Segarra, J., and Melena, J. (1999). The effect of topical diltiazem on ocular hypertension induced by water loading in rabbits. Gen. Pharmacol. 32, 201–205. doi: 10.1016/S0306-3623(98)00196-7
Santafé, J., Martínez de Ibarreta, M. J., Segarra, J., Melena, J., and Garrido, M. (1996). A complex interaction between topical verapamil and timolol on intraocular pressure in conscious rabbits. Naunyn Schmiedebergs. Arch. Pharmacol. 354, 198–204. doi: 10.1007/BF00178721
Savigni, D. L., O'Hare Doig, R. L., Szymanski, C. R., Bartlett, C. A., Lozić, I., Smith, N. M., et al. (2013). Three Ca2+ channel inhibitors in combination limit chronic secondary degeneration following neurotrauma. Neuropharmacology 75, 380–390. doi: 10.1016/j.neuropharm.2013.07.034
Scarpa, A., and Azzone, G. F. (1970). The mechanism of ion translocation in mitochondria. 4. Coupling of K+ efflux with Ca2+ uptake. Eur. J. Biochem. 12, 328–335. doi: 10.1111/j.1432-1033.1970.tb00854.x
Schaefer, A. M., McFarland, R., Blakely, E. L., He, L., Whittaker, R. G., Taylor, R. W., et al. (2008). Prevalence of mitochondrial DNA disease in adults. Ann. Neurol. 63, 35–39. doi: 10.1002/ana.21217
Schieke, S. M., Phillips, D., McCoy, J. P. Jr., Aponte, A. M., Shen, R. F., Balaban, R. S., et al. (2006). The mammalian target of rapamycin (mTOR) pathway regulates mitochondrial oxygen consumption and oxidative capacity. J. Biol. Chem. 281, 27643–27652. doi: 10.1074/jbc.M603536200
Schneider, M. F., and Chandler, W. K. (1973). Voltage dependent charge movement of skeletal muscle: a possible step in excitation-contraction coupling. Nature 242, 244–246. doi: 10.1038/242244a0
Schrier, S. A., and Falk, M. J. (2011). Mitochondrial disorders and the eye. Curr. Opin. Ophthalmol. 22, 325–331. doi: 10.1097/ICU.0b013e328349419d
Selmin, F., Magri, G., Gennari, C. G., Marchianò, S., Ferri, N., and Pellegrino, S. (2017). Development of poly(lactide-co-glycolide) nanoparticles functionalized with a mitochondria penetrating peptide. J. Pept. Sci. 23, 182–188. doi: 10.1002/psc.2952
Selt, M., Bartlett, C. A., Harvey, A. R., Dunlop, S. A., and Fitzgerald, M. (2010). Limited restoration of visual function after partial optic nerve injury; a time course study using the calcium channel blocker lomerizine. Brain Res. Bull. 81, 467–471. doi: 10.1016/j.brainresbull.2009.11.004
Seo, A. Y., Joseph, A. M., Dutta, D., Hwang, J. C., Aris, J. P., and Leeuwenburgh, C. (2010). New insights into the role of mitochondria in aging: mitochondrial dynamics and more. J. Cell Sci. 123(Pt 15), 2533–2542. doi: 10.1242/jcs.070490
Shapira, Y., Harel, S., and Russell, A. (1977). Mitochondrial encephalomyopathies: a group of neuromuscular disorders with defects in oxidative metabolism. Isr. J. Med. Sci. 13, 161–164. doi: 10.1159/000400702
Sharma, A. K., and Rohrer, B. (2004). Calcium-induced calpain mediates apoptosis via caspase-3 in a mouse photoreceptor cell line. J. Biol. Chem. 279, 35564–35572. doi: 10.1074/jbc.M401037200
Shoshan-Barmatz, V., Krelin, Y., Shteinfer-Kuzmine, A., and Arif, T. (2017). Voltage-dependent anion channel 1 as an emerging drug target for novel anti-cancer therapeutics. Front. Oncol. 7:154. doi: 10.3389/fonc.2017.00154
Shy, G. M., and Gonatas, N. K. (1964). Human myopathy with giant abnormal mitochondria. Science 145, 493–496. doi: 10.1126/science.145.3631.493
Siegner, S. W., Netland, P. A., Schroeder, A., and Erickson, K. A. (2000). Effect of calcium channel blockers alone and in combination with antiglaucoma medications on intraocular pressure in the primate eye. J. Glaucoma 9, 334–339. doi: 10.1097/00061198-200008000-00009
Sommer, A. (1989). Intraocular pressure and glaucoma. Am. J. Ophthalmol. 107, 186–188. doi: 10.1016/0002-9394(89)90221-3
Sorrentino, V., and Rizzuto, R. (2001). Molecular genetics of Ca2+ stores and intracellular Ca2+ signalling. Trends Pharmacol. Sci. 22, 459–464. doi: 10.1016/S0165-6147(00)01760-0
Sripetchwandee, J., Sanit, J., Chattipakorn, N., and Chattipakorn, S. C. (2013). Mitochondrial calcium uniporter blocker effectively prevents brain mitochondrial dysfunction caused by iron overload. Life Sci. 92, 298–304. doi: 10.1016/j.lfs.2013.01.004
Stiles, P. G. (1901). On the rhythmic activity of the oesophagus and the influence upon it of various media. Am. J. Physiol. 5, 338–357.
Stone, E. M., Fingert, J. H., Alward, W. L., Nguyen, T. D., Polansky, J. R., Sunden, S. L., et al. (1997). Identification of a gene that causes primary open angle glaucoma. Science 275, 668–670. doi: 10.1126/science.275.5300.668
Takai, Y., Kishimoto, A., Iwasa, Y., Kawahara, Y., Mori, T., and Nishizuka, Y. (1979). Calcium-dependent activation of a multifunctional protein kinase by membrane phospholipids. J. Biol. Chem. 254, 3692–3695.
Takihara, Y., Inatani, M., Eto, K., Inoue, T., Kreymerman, A., Miyake, S., et al. (2015). In vivo imaging of axonal transport of mitochondria in the diseased and aged mammalian CNS. Proc. Natl. Acad. Sci. U.S.A. 112, 10515–10520. doi: 10.1073/pnas.1509879112
Tan, W., and Colombini, M. (2007). VDAC closure increases calcium ion flux. Biochim. Biophys. Acta 1768, 2510–2515. doi: 10.1016/j.bbamem.2007.06.002
Tanwar, M., Dada, T., Sihota, R., and Dada, R. (2010). Mitochondrial DNA analysis in primary congenital glaucoma. Mol. Vis. 16, 518–533.
Tezel, G. (2006). Oxidative stress in glaucomatous neurodegeneration: mechanisms and consequences. Prog. Retin. Eye Res. 25, 490–513. doi: 10.1016/j.preteyeres.2006.07.003
Tezel, G. (2009). The role of glia, mitochondria, and the immune system in glaucoma. Invest. Ophthalmol. Vis. Sci. 50, 1001–1012. doi: 10.1167/iovs.08-2717
Tham, Y. C., Li, X., Wong, T. Y., Quigley, H. A., Aung, T., and Cheng, C. Y. (2014). Global prevalence of glaucoma and projections of glaucoma burden through 2040: a systematic review and meta-analysis. Ophthalmology 121, 2081–2090. doi: 10.1016/j.ophtha.2014.05.013
Toriu, N., Akaike, A., Yasuyoshi, H., Zhang, S., Kashii, S., Honda, Y., et al. (2000). Lomerizine, a Ca2+ channel blocker, reduces glutamate-induced neurotoxicity and ischemia/reperfusion damage in rat retina. Exp. Eye Res. 70, 475–484. doi: 10.1006/exer.1999.0809
Toriu, N., Sasaoka, M., Shimazawa, M., Sugiyama, T., and Hara, H. (2001). Effects of lomerizine, a novel Ca2+ channel blocker, on the normal and endothelin-1-disturbed circulation in the optic nerve head of rabbits. J. Ocul. Pharmacol. Ther. 17, 131–149. doi: 10.1089/10807680151125456
Tsai, C. W., Wu, Y., Pao, P. C., Phillips, C. B., Williams, C., Miller, C., et al. (2017). Proteolytic control of the mitochondrial calcium uniporter complex. Proc. Natl. Acad. Sci. U.S.A. 114, 4388–4393. doi: 10.1073/pnas.1702938114
Tsien, R. W., and Barrett, C. F. (2000). “A brief history of calcium channel discovery,” in Madame Curie Bioscience Database [Internet]. (Austin, TX: Landes Bioscience).
Van Bergen, N. J., Chakrabarti, R., O'Neill, E. C., Crowston, J. G., and Trounce, I. A. (2011). Mitochondrial disorders and the eye. Eye Brain 3, 29–47. doi: 10.1097/ICU.0b013e328349419d
Van Bergen, N. J., Crowston, J. G., Craig, J. E., Burdon, K. P., Kearns, L. S., Sharma, S., et al. (2015). Measurement of systemic mitochondrial function in advanced primary open-angle glaucoma and Leber hereditary optic neuropathy. PLoS ONE 10:e0140919. doi: 10.1371/journal.pone.0140919
Vasington, F. D., and Murphy, J. V. (1962). Ca ion uptake by rat kidney mitochondria and its dependence on respiration and phosphorylation. J. Biol. Chem. 237, 2670–2677.
Vecino, E., Rodriguez, F. D., Ruzafa, N., Pereiro, X., and Sharma, S. C. (2016). Glia-neuron interactions in the mammalian retina. Prog. Retin. Eye Res. 51, 1–40. doi: 10.1016/j.preteyeres.2015.06.003
von Graefe, A. (1857). Ueber kie Wirkung der Iridectomie bei Glaukom. Graefes Arch. Ophthalmol. 3:456.
Vosler, P. S., Brennan, C. S., and Chen, J. (2008). Calpain-mediated signaling mechanisms in neuronal injury and neurodegeneration. Mol. Neurobiol. 38, 78–100. doi: 10.1007/s12035-008-8036-x
Wallace, D. C. (1994). Mitochondrial DNA sequence variation in human evolution and disease. Proc. Natl. Acad. Sci. U.S.A. 91, 8739–8746. doi: 10.1073/pnas.91.19.8739
Watanabe, M., and Fukuda, Y. (2002). Survival and axonal regeneration of retinal ganglion cells in adult cats. Prog. Retin. Eye Res. 21, 529–553. doi: 10.1016/S1350-9462(02)00037-X
Weinreb, R. N., Aung, T., and Medeiros, F. A. (2014). The pathophysiology and treatment of glaucoma: a review. JAMA 311, 1901–1911. doi: 10.1001/jama.2014.3192
Wiggs, J. L. (2007). Genetic etiologies of glaucoma. Arch. Ophthalmol. 125, 30–37. doi: 10.1001/archopht.125.1.30
Williams, G. S., Boyman, L., Chikando, A. C., Khairallah, R. J., and Lederer, W. J. (2013). Mitochondrial calcium uptake. Proc. Natl. Acad. Sci. U.S.A. 110, 10479–10486. doi: 10.1073/pnas.1300410110
Williams, P. A., Harder, J. M., Foxworth, N. E., Cochran, K. E., Philip, V. M., Porciatti, V., et al. (2017). Vitamin B3 modulates mitochondrial vulnerability and prevents glaucoma in aged mice. Science 355, 756–760. doi: 10.1126/science.aal0092
Wojda, U., Salinska, E., and Kuznicki, J. (2008). Calcium ions in neuronal degeneration. IUBMB Life 60, 575–590. doi: 10.1002/iub.91
Wong, L. J., Scaglia, F., Graham, B. H., and Craigen, W. J. (2010). Current molecular diagnostic algorithm for mitochondrial disorders. Mol. Genet. Metab. 100, 111–117. doi: 10.1016/j.ymgme.2010.02.024
Wong, T. Y., and Mitchell, P. (2007). The eye in hypertension. Lancet 369, 425–435. doi: 10.1016/S0140-6736(07)60198-6
Yamada, Y. (2014). Development of the MITO-porter, a nano device for mitochondrial drug delivery via membrane fusion. Yakugaku Zasshi 134, 1143–1155. doi: 10.1248/yakushi.14-00191
Yamada, Y., and Harashima, H. (2017). MITO-Porter for mitochondrial delivery and mitochondrial functional analysis. Handb. Exp. Pharmacol. 240, 475–472. doi: 10.1007/164_2016_4
Yamada, Y., Munechika, R., Kawamura, E., Sakurai, Y., Sato, Y., and Harashima, H. (2017). Mitochondrial delivery of doxorubicin using MITO-Porter kills drug-resistant renal cancer cells via mitochondrial toxicity. J. Pharm. Sci. 106, 2428–2437. doi: 10.1016/j.xphs.2017.04.058
Yamada, Y., Nakamura, K., Abe, J., Hyodo, M., Haga, S., Ozaki, M., et al. (2015). Mitochondrial delivery of Coenzyme Q10 via systemic administration using a MITO-Porter prevents ischemia/reperfusion injury in the mouse liver. J. Control. Release 213, 86–95. doi: 10.1016/j.jconrel.2015.06.037
Yamamoto, K., Sato, K., Yukita, M., Yasuda, M., Omodaka, K., Ryu, M., et al. (2017). The neuroprotective effect of latanoprost acts via klotho-mediated suppression of calpain activation after optic nerve transection. J. Neurochem. 140, 495–508. doi: 10.1111/jnc.13902
Yu-Wai-Man, P., Griffiths, P. G., and Chinnery, P. F. (2011). Mitochondrial optic neuropathies - disease mechanisms and therapeutic strategies. Prog. Retin. Eye Res. 30, 81–114. doi: 10.1016/j.preteyeres.2010.11.002
Zhang, X. Y., and Zhang, P. Y. (2016). Mitochondria targeting nano agents in cancer therapeutics. Oncol. Lett. 12, 4887–4890. doi: 10.3892/ol.2016.5302
Zhang, Y., Zhang, C., Chen, J., Liu, L., Hu, M., Li, J., et al. (2017). Trackable mitochondria-targeting nanomicellar loaded with doxorubicin for overcoming drug resistance. ACS Appl. Mater. Interfaces 9, 25152–25163. doi: 10.1021/acsami.7b07219
Keywords: calcium, mitochondria, glaucoma, mitochondrial drug delivery system, calcium channel blockers
Citation: Cheung LTY, Manthey AL, Lai JSM and Chiu K (2017) Targeted Delivery of Mitochondrial Calcium Channel Regulators: The Future of Glaucoma Treatment? Front. Neurosci. 11:648. doi: 10.3389/fnins.2017.00648
Received: 05 September 2017; Accepted: 07 November 2017;
Published: 22 November 2017.
Edited by:
Victor Tapias, Weill Cornell Medical College, United StatesReviewed by:
Rolf Sprengel, Max Planck Institute for Medical Research (MPG), GermanySelva Baltan, Cleveland Clinic Lerner College of Medicine, United States
Copyright © 2017 Cheung, Manthey, Lai and Chiu. This is an open-access article distributed under the terms of the Creative Commons Attribution License (CC BY). The use, distribution or reproduction in other forums is permitted, provided the original author(s) or licensor are credited and that the original publication in this journal is cited, in accordance with accepted academic practice. No use, distribution or reproduction is permitted which does not comply with these terms.
*Correspondence: Kin Chiu, ZGF0d2FpQGhrdS5oaw==