- 1Laboratory of Neuropharmacology and Preclinical Toxicology, Institute of Basic Health Sciences, Universidade Federal do Rio Grande do Sul, Porto Alegre, Brazil
- 2Laboratory of Pain Pharmacology and Neuromodulation, Preclinical Studies - Pharmacology Department, Institute of Basic Health Sciences, Universidade Federal do Rio Grande do Sul, Porto Alegre, Brazil
- 3Postgraduate Program in Biological Sciences, Pharmacology and Therapeutics, Institute of Basic Health Sciences, Universidade Federal Rio Grande do Sul, Porto Alegre, Brazil
- 4Postgraduate Program in Medical Sciences, School of Medicine Universidade Federal Rio Grande do Sul, Porto Alegre, Brazil
- 5Centro Universitário FADERGS, Health and Wellness School Laureate International Universities, Porto Alegre, Brazil
- 6Laboratory of Neuromodulation, Department of Physical Medicine & Rehabilitation, Spaulding Rehabilitation Hospital and Massachusetts General Hospital, Harvard University, Boston, MA, United States
Epilepsy is a chronic brain syndrome characterized by recurrent seizures resulting from excessive neuronal discharges. Despite the development of various new antiepileptic drugs, many patients are refractory to treatment and report side effects. Non-invasive methods of brain stimulation, such as transcranial direct current stimulation (tDCS), have been tested as alternative approaches to directly modulate the excitability of epileptogenic neural circuits. Although some pilot and initial clinical studies have shown positive results, there is still uncertainty regarding the next steps of investigation in this field. Therefore, we reviewed preclinical and clinical studies using the following framework: (1) preclinical studies that have been successfully translated to clinical studies, (2) preclinical studies that have failed to be translated to clinical studies, and (3) clinical findings that were not previously tested in preclinical studies. We searched PubMed, Web of Science, Embase, and SciELO (2002–2017) using the keywords “tDCS,” “epilepsy,” “clinical trials,” and “animal models.” Our initial search resulted in 64 articles. After applying inclusion and exclusion criteria, we screened 17 full-text articles to extract findings about the efficacy of tDCS, with respect to the therapeutic framework used and the resulting reduction in seizures and epileptiform patterns. We found that few preclinical findings have been translated into clinical research (number of sessions and effects on seizure frequency) and that most findings have not been tested clinically (effects of tDCS on status epilepticus and absence epilepsy, neuroprotective effects in the hippocampus, and combined use with specific medications). Finally, considering that clinical studies on tDCS have been conducted for several epileptic syndromes, most were not previously tested in preclinical studies (Rasmussen's encephalitis, drug resistant epilepsy, and hippocampal sclerosis-induced epilepsy). Overall, most studies report positive findings. However, it is important to underscore that a successful preclinical study may not indicate success in a clinical study, considering the differences highlighted herein. Although most studies report significant findings, there are still important insights from preclinical work that must be tested clinically. Understanding these factors may improve the evidence for the potential use of this technique as a clinical tool in the treatment of epilepsy.
Introduction
Techniques involving stimulation of the central nervous system have been extensively studied in recent years. These techniques have been shown to improve symptoms in a range of neurological disorders. Both invasive and non-invasive brain stimulation techniques have been described. Non-invasive techniques can be divided into transcranial magnetic stimulation (TMS), transcranial alternating current stimulation, and transcranial direct current stimulation (tDCS) (Woods et al., 2016).
tDCS relies on the modification of the neuronal resting membrane potential to induce changes in cortical excitability. This technique consists on applying a weak, direct, constant, and low intensity electric current over the scalp using two electrodes: an anode and a cathode (Gomez Palacio Schjetnan et al., 2013). The electrodes are arranged in different assemblies, creating a flow of low-level continuous electrical current targeting a specific region of the cerebral cortex. Anodal stimulation induces depolarization of the neuronal membrane, and therefore facilitates neuronal firing. In contrast, cathodal stimulation has the opposite effect, hyperpolarizing the neuronal membrane (Figure 1; Jackson et al., 2016). tDCS is applied at intensities ranging from 0.5–2 mA across saline-soaked electrodes placed on the human or animal scalp (Figure 2) [from author].
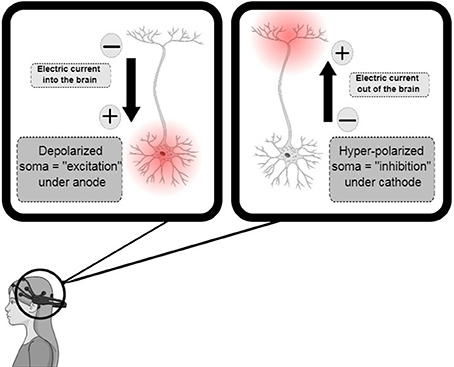
Figure 1. Transcranial direct-current stimulation design, demonstrating anodal, and cathodal stimuli with consequent depolarization (increase in excitability) and hyperpolarization (decrease in excitability), respectively. Adapted from Jackson et al. (2016).
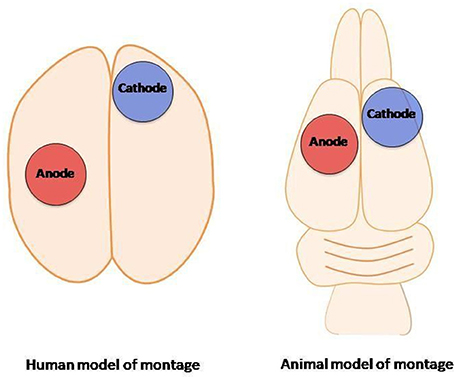
Figure 2. Transcranial direct-current stimulation: human montage vs. transcranial direct-current stimulation animal model (from author).
tDCS has been shown to improve symptoms in patients with depression, stroke, focal dystonia, migraine, chronic pain, and epilepsy (Liebetanz et al., 2006a). Epilepsy represents a chronic brain syndrome of diverse etiology, characterized by recurrent seizures resulting from excessive neuronal discharge (Chindo et al., 2014). This syndrome affects ~0.5–1% of the world's population (Dhir et al., 2005), and is associated with a variety of clinical symptoms such as impaired consciousness, movement and sensation (Chindo et al., 2014). Epileptical discharges are generated in response to a loss of balance between excitatory and inhibitory connections, resulting in tonic depolarization of brain circuits (McCormick and Contreras, 2001). The pathophysiology of epilepsy includes hyperactivity of excitatory glutamatergic transmission, and a deficit of inhibitory signaling, mainly resulting from insufficient neurotransmission mediated by γ-aminobutyric acid A receptors (GABAAR) (Lason et al., 2013).
Despite the diversity of new antiepileptic drugs, a large proportion of individuals suffering from epilepsy is refractory to pharmacology treatment (Loscher, 2002), and/or reports side effects which hinder the use of drugs. Epidemiological data indicate that 20–40% of patients with newly diagnosed epilepsy become refractory to treatment (Loscher and Schmidt, 2004; French, 2007). For this reason, there is a continuous search for new therapeutic strategies, from which tDCS has emerged as a possible alternative. In this study, we aimed to summarize the evidence concerning the effects of tDCS in epilepsy in both clinical and preclinical studies. We used a framework to provide insight into the translation rate of preclinical into clinical studies. In addition, we attempted to determine important areas for clinical testing, to verify if these results are complementary, and to identify possible limitations of these studies.
Methods
Search Strategy
This systematic review was based on a literature search using PubMed, Web of Science, Embase and SciELO. The keyword “tDCS” was used in combination with other keywords such as “epilepsy,” “clinical trials,” and “animal models.” The term “AND” was used in each combination (Figure 3). In addition, the reference sections of the studies that met our inclusion criteria were manually screened for relevant publications.
Inclusion and Exclusion Criteria
Studies had to meet the following criteria: (1) publication in English between 2002 and 2017, (2) report original research, and (3) report case reports. Exclusion criterion were: (1) lack of original data (e.g. review articles, editorial material, articles reporting duplicate data); (2) articles addressing only effects of other brain stimulation techniques, such as alternating electrical current stimulation, or TMS.
Data Extraction and Outcomes
Two investigators (GGR and CO) extracted data from the full articles independently. Any disagreements were resolved by a third investigator (PP). However, we summarized the results in a narrative format. The primary outcome of our study was seizure suppression (SS). Relevant articles reporting the outcome of interest were identified, and standardized tables were utilized to extract the following variables: experiment, total sessions, interval between sessions, number of individuals (N), montage, contact area of electrodes, sham group and results/insights. The primary author collected the name of the authors, titles and study design. When further information about the study was needed, the authors were contacted by email.
Risk of Bias Assessment
Risk of bias was assessed by two reviewers (GGR and CO), for each included preclinical study, using SYRCLE's Risk of Bias tool for animal studies (Hooijmans et al., 2014). We extracted study characteristics related to construct and external validity (Henderson et al., 2013). For construct validity, we included sex, species and strain, type of epilepsy model, total treatment sessions and the interval between them, and the use of any other intervention. For clinical studies, we ranked each of the following as having a high, low, or unclear risk of bias when included allocation concealment, blinding of participants, personnel and outcome assessors, incomplete outcome data, and selective outcome reporting (Higgins and Green, 2011). Since the primary outcome included only the tDCS effectiveness, not harmful effects, results may be biased.
Framework of Translation From Preclinical Studies to Clinical Studies
In order to analyze the results for this review, we used a framework to understand whether preclinical findings were translated into clinical studies, and whether or not this translational research confirmed preclinical findings. Therefore, we created three main categories: (1) preclinical studies that have been successfully translated in clinical studies, (2) preclinical studies that have failed to translate in clinical studies, (3) clinical findings that were not tested previously in preclinical studies.
Results
The final search identified 64 studies. After applying the inclusion and exclusion criteria, we included 17 articles with different types of designs (5 preclinical/12 clinical) for full-text analysis. We screened the articles according to the main outcome, the suppression of seizures, and summarized the results separately for basic (Table 1) and clinical (Table 2) research. Interestingly, the results obtained from the search in PubMed and Web of Science were the same. We summarized our findings using the framework discussed above.
From Tables 1,2, we observed that most clinical studies showed significant results with respect to epileptiform activity, though only 11 studies (91.67%) had positive results in clinical outcomes such as seizure frequency reduction. For safety, none of them showed an increase in seizure frequency or moderate to severe adverse effects. On the other hand, two preclinical studies confirmed the initial hypothesis that cathodal-tDCS induces anticonvulsant effect.
Preclinical Studies That Have Been Successfully Translated to Clinical Studies
Reduction in Seizure Frequency by Cathodal tDCS
The first study analyzed results of tDCS in rats subjected to a cortical ramp model of focal epilepsy. In this model, the anticonvulsive effect induced by cathodal-tDCS (c-tDCS) varied according to the duration of stimulation and strength of the current. In addition, the effect may be associated to modulation of cortical excitability that outlasts the actual stimulation (Liebetanz et al., 2006b). Kamida et al. (2013) reported similar results using amygdala-kindled rats; c-tDCS treatment significantly improved the seizure stage, decreased after-discharge duration, and elevated after-discharge threshold 1 day after the last tDCS session. Similar findings have also been reported in clinical studies by Fregni et al. (2006), Yook et al. (2011), San-Juan et al. (2011), Auvichayapat et al. (2013), Assenza et al. (2014), Tekturk et al. (2016a,b), Auvichayapat et al. (2016), Zoghi et al. (2016), Assenza et al. (2017), and San-Juan et al. (2017), conducted in a total of 138 patients.
Preclinical Studies That Have Failed to Be Translated to Clinical Studies
Status Epilepticus
In 2011, Kamida et al. (2011) used a model of pilocarpine-induced status epilepticus (SE) in immature rats, and demonstrated a 21% reduction in convulsions on postnatal day 55. There are no studies evaluating the effects of tDCS in patients experiencing SE. However, there is a case report (that does not meet the inclusion criteria for this review) that has tested this approach (Grippe et al., 2015). Therefore, this design might be a good opportunity for a future trial.
Neuroprotective Effects in the Hippocampus
c-tDCS has been reported to exert neuroprotective effects in the immature rat hippocampus, reducing SE-induced hippocampal cell loss, as well as supragranular and CA3 sprouting (Kamida et al., 2011). This hippocampal impairment is caused by seizure-induced neuronal damage and synaptic reorganization, which starts soon after SE (Covolan and Mello, 2000). This type of a study is difficult to perform in a clinical setting; however, it would clearly be worthwhile to test, for example after brain injury that may lead to further impairment in cortical areas and result in epileptogenic foci (D'Ambrosio and Perucca, 2004).
Effects in Absence Epilepsy
Zobeiri and van Luijtelaar (2013) using a genetic absence model of epilepsy, showed that c-tDCS reduces slow-wave discharges (SWDs) in rats during stimulation and affects the mean duration of SWDs after stimulation, both in an intensity-dependent manner. Behavioral changes were also observed in response to the highest stimulation intensity. Spectral analysis of EEG during stimulation revealed an increase in sub-delta and delta frequency ranges, suggesting that cortical cells were hyperpolarized. These preclinical findings have not been replicated clinically, as no clinical study has evaluated tDCS in patients with absence epilepsy. This constitutes another opportunity for future studies.
Combination of tDCS With Specific Drugs to Test Synergistic Effects
In an acute seizure induced by pentylenetetrazole, c-tDCS reduced EEG spike bursts, and suppressed clinical seizures in rats. c-tDCS, in combination with lorazepam, was more effective in SS compared with either tDCS or lorazepam alone, and prevented loss of motor cortex inhibition during paired-pulse transcranial magnetic stimulation (ppTMS) accompanied by pentylenetetrazole injection. This study provides evidence of the neural substrate of the antiepileptic effects of tDCS through ppTMS measures and demonstrates that c-tDCS enhances GABAergic intracortical inhibition mediated by GABAA signaling. Further, c-tDCS prevented the loss of GABAergic ppTMS inhibition that is expected with PTZ-mediated GABAA antagonism. This corroborates the hypothesis that tDCS may influence neurotransmitter levels and receptor function in humans (Medeiros et al., 2012). Thus, a combination of c-tDCS and GABAergic pharmacotherapy could be proposed, for example with benzodiazepine treatment (Dhamne et al., 2015).
Clinical Findings Not Replicated in Preclinical Studies
Epilepsy is characterized by multiple heterogeneous syndromes with various etiologies and symptoms, insufficiently addressed in current animal models despite the number of experimental options (Kandratavicius et al., 2014; Depaulis and Hamelin, 2015). The choice of appropriate protocol remains a challenge; most animal models used in epilepsy research are models of epileptic seizures rather than epilepsy per se, making differentiation subjective (Löscher, 2011). Thus, there are still limitations and shortcomings regarding models of refractory epilepsy and epilepsy because of hippocampal sclerosis, which often compromise the translational application of preclinical findings. Nonetheless, such clinical findings represent an opportunity to perform preclinical studies in an attempt to establish reproducible animal models and clarify the mechanisms involved in both pathology and treatment with tDCS.
Effects in Rasmussen's Encephalitis
Rasmussen's encephalitis is a rare and progressive inflammatory disease that reaches one cerebral hemisphere, and leads to intractable partial-onset seizures. Currently, the only effective treatment is hemispherectomy, but this procedure may cause irreversible neurological deficits. In a case report, San-Juan et al. (2011) reported that one patient with Rasmussen's encephalitis was seizure free and another showed a 50% reduction in seizure frequency within 6 months of follow-up after tDCS treatment. In a recent study, two patients with Rasmussen's encephalitis received modulated c-tDCS (2 mA for 30 min on 3 consecutive days) and demonstrated reduced seizure frequency following stimulation. One patient showed more than 50% reduction in seizure frequency, and the longest positive effect lasted for 1 month (Tekturk et al., 2016a). Thus, tDCS may be used to treat this pathology in order to avoid or delay surgical intervention (San-Juan et al., 2011).
In another study, patients with Lennox-Gastaut syndrome received pharmacological treatment for 5 consecutive days and 2 mA c-tDCS over the primary motor cortex (M1) for 20 min. This combination was more effective in reducing seizure frequency and epileptic discharges than pharmacological treatment alone. This reduction was sustained for 3 weeks after treatment (Auvichayapat et al., 2016).
Effects in Drug Resistant Epilepsy
Approximately one-third of epilepsy patients develop drug resistance, and only 50% can take benefit from the surgical removal of an epileptic focus (Assenza et al., 2017). Although surgery is an option in cases of drug resistant epilepsy, many patients have no access to medical centers that perform respective epilepsy surgery. Furthermore, some patients may have a seizure focus located in eloquent cortex where resection is likely to cause deficit (Auvichayapat et al., 2013).
Many models of refractory epilepsy have been developed over the past 20 years, which use two approaches: (1) seizures or epilepsy models resistant to antiepileptic drugs (for example, 6-Hz psychomotor seizure model in mice) and (2) chronic epilepsy models, such as kindling. Kindling involves the application of repeated excitatory stimuli to induce partial seizures, followed by subsequent generalized seizures. This leads to increased seizure length and severity with continuous stimulation (Löscher, 2011). It is important highlight that we did not find preclinical studies relating the use of tDCS in refractory epilepsy, resulting in a lack of mechanistic and neurochemical clarifications. An alternative treatment is neuromodulation, which represents an attempt to improve the quality of life of patients with refractory epilepsy.
In 2006, Fregni et al. (2006) conducted a controlled study applying c-tDCS in 19 patients with refractory epilepsy; this unprecedented study investigated the electrographic and clinical response to c-tDCS in this epileptic condition. Patients underwent one session of c-tDCS (20 min, 1 mA) targeting the epileptogenic focus. The active stimulation did not induce seizures and was well-tolerated, and the treatment promoted a large reduction in number of epileptiform discharges (EDs) in the EEG and in the frequency of seizures. These parameters were measured and compared before (baseline), immediately after, and 15 and 30 days after either sham or active stimulation.
Yook et al. (2011) demonstrated in a case report of bilateral perisylvian syndrome that tDCS, when applied over the midpoint between P4 and T4, had a lasting effect over a 2-month period following treatment termination, decreasing the duration of each seizure episode. For 2 months after the second treatment session, only one seizure attack occurred, a considerable improvement over the eight seizure attacks per month prior to tDCS, when the patient was treated only with antiepileptic drugs.
The large reduction in interictal epileptiform EEG discharges in two subjects with drug-refractory continuous spike-wave discharges during slow sleep suggests that the simultaneous application of tDCS treatment and EEG recording allows the assessment of safety parameters during treatment. This methodology ensures that the stimulation is sufficiently focal and provides a detailed evaluation of epileptic activity changes induced by tDCS, representing an attractive outlook for epilepsy treatment (Faria et al., 2012).
Auvichayapat et al. (2013) showed that a single session of active tDCS treatment was associated with significant reductions in epileptic discharge frequency in children with refractory epilepsy immediately, 24, and 48 h after tDCS treatment. In addition, 4 weeks after treatment, a small decrease in seizure frequency was detected.
In focal resistant epilepsy, two patients received c-tDCS (constant current of 1 mA) during a real session in a single-blind, sham-controlled study, followed by 1 month of observation. During this period, the patients or caregivers provided a detailed seizure calendar (frequency per week at basal, post-sham and post-tDCS time points). These patients experienced reduction in seizure frequencies of ~70 and 50% (Assenza et al., 2014).
Effects in Epilepsy Due to Hippocampal Sclerosis
Animals subjected to kainate or pilocarpine-induced SE develop spontaneous seizures after a pre-epileptic period or seizure free, this can be due hippocampal injury, resulting in an animal model of mesial temporal lobe epilepsy (MTLE) with hippocampal sclerosis (Sloviter, 2008). Although MTLE is well-described in in clinical studies, with respect to electrophysiological and histological parameters, it remains partially reproduced in most rodent models (Depaulis and Hamelin, 2015). Even if there animal models described, remains some doubt about their reliability and extrapolation of their findings to the clinical setting, which represents a limitation in the conduction of preclinical studies using tDCS in models of MTLE due to hippocampal sclerosis.
In a randomized, placebo-controlled, double-blinded clinical trial with 3 sessions, 5 sessions and placebo stimulation, 3 and 5 sessions of c-tDCS stimulation decreased the frequency of seizures and interictal epileptiform discharge (immediately post-tDCS vs. baseline) in adults with MTLE and hippocampal sclerosis, compared to sham tDCS (San-Juan et al., 2017). It is known that MTLE with hippocampal sclerosis is a drug-resistant focal epilepsy syndrome. Another study showed that 83.33% of MTLE patients who received modulated c-tDCS (2 mA for 30 min on 3 consecutive days) showed more than 50% reduction in seizure frequency during a 1-month follow-up. Moreover, 50% these patients were seizure-free in the 1-month period post-tDCS (Tekturk et al., 2016b).
Recently, the case of a patient with drug-resistant temporal lobe epilepsy was reported. tDCS reduced seizure frequency from 6–10 per day to 0–3 per day. Seizure diaries revealed that seizure rates remained low (from 0 to 3 per day) for 4 months, and then began to increase (Zoghi et al., 2016). A recent database of published tDCS clinical trials, authored by Lefaucheur, presents a detailed list of studies assessing the clinical effect of tDCS, including in epileptic patients (Lefaucheur, 2016).
Discussion
In this study, we performed a systematic review of clinical and preclinical studies using tDCS as a therapeutic approach in the treatment of epilepsy. Most studies presented here involved the use of c-tDCS, and several have investigated the effects of c-tDCS on spontaneous neural activity and evoked motor responses of the central and peripheral nervous system. These studies provide evidence that the effects of tDCS involve a non-synaptic mechanism of action, based on changes in neural membrane function (Ardolino et al., 2005).
tDCS has been applied in the treatment of epilepsy, spasticity, movement disorders, peripheral vascular disease, and certain psychiatric disorders (Raghavan et al., 2008). The acute effects of weak tDCS on ongoing epileptiform activity are well-established in animal models. However, the underlying mechanism by which prolonged tDCS modulates seizure initiation propensity and epileptogenesis remains unknown (Jackson et al., 2016). In addition, animal studies suggest that prolonged cathodal tDCS (c-tDCS) has anticonvulsant effects. On the other hand, anodal tDCS (a-tDCS) has contrary effects, decreasing the threshold for producing the seizure activity (evident in EEG), while behavioral changes are not observed (Hayashi et al., 1988; Liebetanz et al., 2006b). Nonetheless, Tekturk et al. attempted to prevent the generation and propagation of seizures by applying a-tDCS. This attempt was based on the hypothesis that even if c-tDCS decreases cortical excitability, a-tDCS increases the effects of inhibitory connections (Tekturk et al., 2016a). Therefore, they used c-tDCS targeting the epileptic foci and a-tDCS targeting the surrounding normal cortical tissue, an approach that was not effective in reducing the frequency of seizures.
Epilepsy is a pathology with the intrinsic characteristic of hypersynchronous brain activity. Therefore, epilepsy represents a model for abnormal hyperexcitatory plastic changes within cortical circuitry (San-Juan et al., 2017). The heterogeneity reported when using tDCS to treat refractory epilepsy may partly be attributed to the different etiology of that pathology. Accordingly, different approaches may be assessed in distinct types of epilepsy, due to the paucity of studies available. For example, in drug-resistant post-traumatic epilepsy patients, only one double-blinded randomized control trial has been published (Fregni et al., 2006). The causes of refractoriness of epilepsy to drugs and surgical treatment remain unknown. However, one possible explanation is the presence of neuronal damage affecting other brain areas besides the hippocampus (Petrovski et al., 2010; Zhang et al., 2017). A morphometric study using magnetic resonance imaging showed that neuronal damage in patients with temporal lobe epilepsy extends beyond the hippocampus, and affects regions that connect to the hippocampus functionally and anatomically (van Elst et al., 2000). This finding suggests the presence of a neural network injury which underlies the clinical manifestations in these patients (Andrade-Valença et al., 2008). More specifically, MTLE is commonly associated with hippocampal sclerosis (Andrade-Valença et al., 2008).
A previous review by our research group (Medeiros et al., 2012) discusses the presumed mechanisms of action of tDCS, attempting to elucidate the underlying neurobiology and cell-signaling pathways involved. There, we suggest that tDCS induces plasticity, improves neuronal viability and morphology, modulates synaptic transmission, and biosynthesis of molecules.
tDCS has consistently been reported to be safe, and a recent review confirmed the absence of evidence for serious adverse effects (Bikson et al., 2016). tDCS is a technique that can be applied with low risk and little discomfort, and when used in repeated sessions, can have long-lasting effects (Nitsche et al., 2008). The effects of tDCS in the short term occur due to a decrease (anodal) or increase (cathodal) in neuronal firing threshold (Ruscheweyh et al., 2011). However, long-term effects involve the participation of brain-derived neuronal factor (BDNF) and glutamatergic N-methyl-d-aspartate (NMDA) receptors in synaptic plasticity mechanisms (Fertonani et al., 2010). Brain damage induced by the formation of toxic products does not occur using this technique, because there is no direct contact of electrodes with the cerebral cortex (Nitsche et al., 2003). Magnetic resonance imaging before and after 30 and 60 min of stimulation applied to the prefrontal and motor cortex did not exhibit pathological signal alterations. As such, it was concluded that tDCS does not induce cerebral edema, or render abnormal the blood brain barrier or brain tissue (Rosen et al., 2009). Finally, Accornero et al. (2007) showed no abnormal variations in heart rate, blood pressure, or temperature during and 20 min after the end of the stimulation. Therefore, tDCS is a safe method for use in humans, and has the advantage of being easily combined with other interventions, as pharmacological treatment.
San-Juan et al. (2015) reviewed the efficacy and safety of tDCS in epilepsy. The authors analyzed 9 articles using different methodologies (3 pre-clinical/6 clinical). Moreover, in vivo and in vitro animal studies demonstrated that direct current stimulation could induce suppression of epileptiform activity without neurological injury. Four out of six (67%) clinical studies revealed an effective decrease in epileptic seizures, and five out of six (83%) showed a reduction of interictal epileptiform activity (San-Juan et al., 2015; Scorza and Brunoni, 2015). In fact, in this review we did not find evidence that tDCS in epilepsy may lead to an increase in seizures or any other significant adverse effects (Pereira et al., 2016). Additional studies involving a large cohort of patients are required to investigate the effects of tDCS in drug-resistant epilepsy.
In order to develop optimal stimulation protocols and long-term follow-up, animal studies and larger prospective clinical trials with homogenous epileptic conditions are needed. Every study uses different patient categories, stimulation protocols, electrode sizes, stimulation sites, and stimulation current strength. Therefore, conclusions drawn from the comparison of these studies should be used to provide standardized measures, in order to improve reproducibility of outcomes (Gschwind and van Mierlo, 2016). Epileptogenesis involves an increase in excitatory synaptic strength, and seizure foci are characterized by a pathological reduction of inhibitory (GABA-releasing) terminals and an increase in excitatory (glutamatergic) terminals (Figure 4; Fregni and Pascual-Leone, 2007). Hence, the principle mechanism of action of tDCS might be the induction of long-term-depression-like (LTD) effects, i.e., reducing cortical excitability and the probability of paroxysmal activity in epileptogenic cortical regions (Nitsche and Paulus, 2009). While the immediate anticonvulsant effects of c-tDCS involve the hyperpolarization of neuronal soma and desynchronization of neuronal activity, its long-term effects seem to occur through the modulation of synaptic transmission, causing LTD in the thalamus-cingulate pathway. This process appears to be N-methyl-D aspartate (NMDA) receptor- and duration-dependent (Chang et al., 2015), thus c-tDCS seems to promote intracortical inhibition. On the other hand, a-tDCS facilitates synaptic plasticity mediated by a long-term potentiation (LTP)-like mechanism, as well as previous studies presented that brief seizures could induce LTP and mossy fiber sprouting in the hippocampus; therefore, the mechanism of LTP formation might be similar to the mechanism of epileptogenesis (Chang et al., 2015; Rroji et al., 2015). Finally, the mechanisms underlying the effects of tDCS seem to be involved not only in local polarity-related modifications of cortical excitability, but also in more complex interhemispheric connections (Tatti et al., 2016).
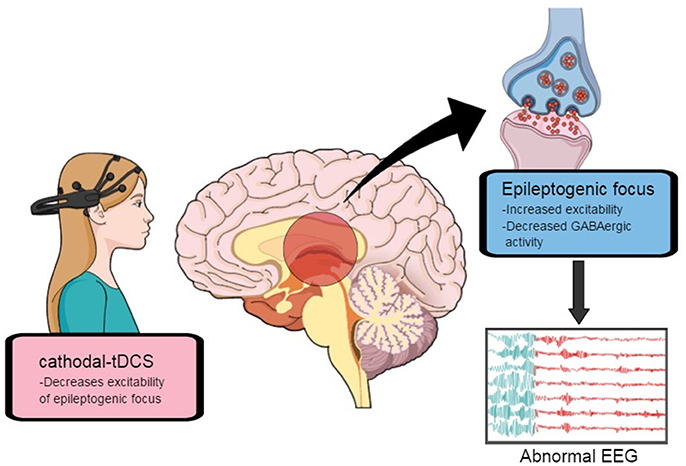
Figure 4. Excitability in epileptogenic focus might be decreased by cathodal transcranial direct-current stimulation. Adapted from Fregni and Pascual-Leone (2007).
Conclusions
Considering the data obtained in this review, we conclude that tDCS should be considered a viable therapeutic option in refractory epilepsy, particularly in patients who are unable to undergo surgery. In general, animal studies used c-tDCS with currents ranging from 100 to 200 μA; even with defined montages, the stimulation appears to be bicephalic, due to the animal's skull size. At present, clinical studies involving c-tDCS use ranging from 1 to 2 mA, and the cathode is placed over the epileptic foci in majority. Cathodal tDCS appears to decrease excitability through hyperpolarization associated to LTD-like mechanisms. Moreover, because tDCS is simple to use, low cost, and easily accessible, it is a good option in countries with limited resources (Scorza and Brunoni, 2015; Zoghi et al., 2016). Therefore, despite the intriguing possibility of modulating neural networks, tDCS still requires a more in-depth analysis of the most beneficial protocols and elucidation of the underlying mechanism of action. Thus, this non-invasive technique still requires further sham-controlled, double-blind larger multi-center studies, which may be justified for cost-effectiveness and surgery complication avoidance. Novel methods of real-time assessment with EEG, and use of other neural markers, may also help with understanding the clinical effects of tDCS in epilepsy (Faria et al., 2012; Leite et al., 2017). Although there are several trials published in this field, further evidence is needed to understand the potential role of using tDCS to treat epilepsy.
Author Contributions
All authors participated in the design of the study and drafted the manuscript. IT and PP participated in study coordination and helped draft the manuscript. FF and DL helped finalize the manuscript. GR, CdO, and RV have designed and prepared the manuscript figures. All authors read and approved the final manuscript.
Conflict of Interest Statement
The authors declare that the research was conducted in the absence of any commercial or financial relationships that could be construed as a potential conflict of interest.
Acknowledgments
The authors were supported by the Brazilian's agencies: Conselho Nacional de Desenvolvimento Científico e Tecnológico (IT; PP), Federal University of Rio Grande do Sul and Brazilian Federal Agency for Support and Evaluation of Graduate Education—CAPES/PNPD (CdO); CAPES Edital MD-PhD/2015 (DL). We also want to thank the Engineering Division from the HCPA for having developed the tDCS stimulator, MCT/FINEP—COENG/2013.
References
Accornero, N., Li Voti, P., La Riccia, M., and Gregori, B. (2007). Visual evoked potentials modulation during direct current cortical polarization. Exp. Brain Res. 178, 261–266. doi: 10.1007/s00221-006-0733-y
Andrade-Valença, L. P., Valença, M. M., Velasco, T. R., Carlotti, C. G., Assirati, J. A., Galvis-Alonso, O. Y., et al. (2008). Mesial temporal lobe epilepsy: clinical and neuropathologic findings of familial and sporadic forms. Epilepsia 49, 1046–1054. doi: 10.1111/j.1528-1167.2008.01551.x
Ardolino, G., Bossi, B., Barbieri, S., and Priori, A. (2005). Non-synaptic mechanisms underlie the after-effects of cathodal transcutaneous direct current stimulation of the human brain. J. Physiol. 568, 653–663. doi: 10.1113/jphysiol.2005.088310
Assenza, G., Campana, C., Assenza, F., Pellegrino, G., Di Pino, G., Fabrizio, E., et al. (2017). Cathodal transcranial direct current stimulation reduces seizure frequency in adults with drug-resistant temporal lobe epilepsy: a sham controlled study. Brain Stimul. 10, 333–335. doi: 10.1016/j.brs.2016.12.005
Assenza, G., Campana, C., Formica, D., Schena, E., Taffoni, F., Di Pino, G., et al. (2014). Efficacy of cathodal transcranial direct current stimulation in drug-resistant epilepsy: a proof of principle. Conf. Proc. IEEE Eng. Med. Biol. Soc. 2014, 530–533. doi: 10.1109/EMBC.2014.6943645
Auvichayapat, N., Rotenberg, A., Gersner, R., Ngodklang, S., Tiamkao, S., Tassaneeyakul, W., et al. (2013). Transcranial direct current stimulation for treatment of refractory childhood focal epilepsy. Brain Stimul. 6, 696–700. doi: 10.1016/j.brs.2013.01.009
Auvichayapat, N., Sinsupan, K., Tunkamnerdthai, O., and Auvichayapat, P. (2016). Transcranial direct current stimulation for treatment of childhood pharmacoresistant lennox-gastaut syndrome: a pilot study. Front. Neurol. 7:66. doi: 10.3389/fneur.2016.00066
Bikson, M., Grossman, P., Thomas, C., Zannou, A. L., Jiang, J., Adnan, T., et al. (2016). Safety of transcranial direct current stimulation: evidence based update 2016. Brain Stimul. 9, 641–661. doi: 10.1016/j.brs.2016.06.004
Chang, W. P., Lu, H. C., and Shyu, B. C. (2015). Treatment with direct-current stimulation against cingulate seizure-like activity induced by 4-aminopyridine and bicuculline in an in vitro mouse model. Exp. Neurol. 265, 180–192. doi: 10.1016/j.expneurol.2015.02.002
Chindo, B. A., Ya, 'U. J., Danjuma, N. M., Okhale, S. E., Gamaniel, K. S., and Becker, A. (2014). Behavioral and anticonvulsant effects of the standardized extract of Ficus platyphylla stem bark. J. Ethnopharmacol. 154, 351–360. doi: 10.1016/j.jep.2014.03.061
Covolan, L., and Mello, L. E. (2000). Temporal profile of neuronal injury following pilocarpine or kainic acid-induced status epilepticus. Epilepsy Res. 39, 133–152. doi: 10.1016/S0920-1211(99)00119-9
D'Ambrosio, R., and Perucca, E. (2004). Epilepsy after head injury. Curr. Opin. Neurol. 17, 731–735. doi: 10.1097/00019052-200412000-00014
Depaulis, A., and Hamelin, S. (2015). Animal models for mesiotemporal lobe epilepsy: the end of a misunderstanding? Rev. Neurol. 171, 217–226. doi: 10.1016/j.neurol.2015.01.558
Dhamne, S. C., Ekstein, D., Zhuo, Z., Gersner, R., Zurakowski, D., Loddenkemper, T., et al. (2015). Acute seizure suppression by transcranial direct current stimulation in rats. Ann. Clin. Trans. Neurol. 2, 843–856. doi: 10.1002/acn3.226
Dhir, A., Naidu, O. S., and Kulkarni, S. K. (2005). Effect of naproxen, a non-selective cyclo-oxygenase inhibitor, on pentylenetetrazol-induced kindling in mice. Clin. Exp. Pharmacol. Physiol. 32, 579–584. doi: 10.1111/j.1440-1681.2005.04233.x
Faria, P., Fregni, F., Sebastião, F., Dias, A. I., and Leal, A. (2012). Feasibility of focal transcranial DC polarization with simultaneous EEG recording: preliminary assessment in healthy subjects and human epilepsy. Epilepsy Behav. 25, 417–425. doi: 10.1016/j.yebeh.2012.06.027
Fertonani, A., Rosini, S., Cotelli, M., Rossini, P. M., and Miniussi, C. (2010). Naming facilitation induced by transcranial direct current stimulation. Behav. Brain Res. 208, 311–318. doi: 10.1016/j.bbr.2009.10.030
Fregni, F., and Pascual-Leone, A. (2007). Technology insight: noninvasive brain stimulation in neurology-perspectives on the therapeutic potential of rTMS and tDCS. Nat. Clin. Pract. Neurol. 3, 383–393. doi: 10.1038/ncpneuro0530
Fregni, F., Thome-Souza, S., Nitsche, M. A., Freedman, S. D., Valente, K. D., and Pascual-Leone, A. (2006). A controlled clinical trial of cathodal DC polarization in patients with refractory epilepsy. Epilepsia 47, 335–342. doi: 10.1111/j.1528-1167.2006.00426.x
French, J. A. (2007). Refractory epilepsy: clinical overview. Epilepsia 48, 3–7. doi: 10.1111/j.1528-1167.2007.00992.x
Gomez Palacio Schjetnan, A., Faraji, J., Metz, G. A., Tatsuno, M., and Luczak, A. (2013). Transcranial direct current stimulation in stroke rehabilitation: a review of recent advancements. Stroke Res. Treat. 2013:170256. doi: 10.1155/2013/170256
Grippe, T. C., Brasil-Neto, J. P., Boechat-Barros, R., Cunha, N. S., and Oliveira, P. L. (2015). Interruption of Epilepsia Partialis Continua by Transcranial Direct Current Stimulation. Brain Stimul. 8, 1227–1228. doi: 10.1016/j.brs.2015.08.004
Gschwind, M., and van Mierlo, P. (2016). Difficulty of comparing the multiple heterogeneous approaches: comment to transcranial direct current stimulation in epilepsy. Brain Stimul. 9, 459–461. doi: 10.1016/j.brs.2016.03.003
Hayashi, Y., Hattori, Y., Asaki, H., Moriwaki, A., and Hori, Y. (1988). Effects of prolonged weak anodal direct current on electrocorticogram in awake rabbit. Acta Med. Okayama 42, 293–296.
Henderson, V. C., Kimmelman, J., Fergusson, D., Grimshaw, J. M., and Hackam, D. G. (2013). Threats to validity in the design and conduct of preclinical efficacy studies: a systematic review of guidelines for in vivo animal experiments. PLoS Med. 10:e1001489. doi: 10.1371/journal.pmed.1001489
Higgins, J., and Green, S. (eds.). (2011). Cochrane Handbook for Systematic Reviews of Interventions. Version 5.1.0. The Cochrane Collaboration.
Hooijmans, C. R., Rovers, M. M., de Vries, R. B., Leenaars, M., Ritskes-Hoitinga, M., and Langendam, M. W. (2014). SYRCLE's risk of bias tool for animal studies. BMC Med. Res. Methodol. 14:43. doi: 10.1186/1471-2288-14-43
Jackson, M. P., Rahman, A., Lafon, B., Kronberg, G., Ling, D., Parra, L. C., et al. (2016). Animal models of transcranial direct current stimulation: methods and mechanisms. Clin. Neurophysiol. 127, 3425–3454. doi: 10.1016/j.clinph.2016.08.016
Kamida, T., Kong, S., Eshima, N., Abe, T., Fujiki, M., and Kobayashi, H. (2011). Transcranial direct current stimulation decreases convulsions and spatial memory deficits following pilocarpine-induced status epilepticus in immature rats. Behav. Brain Res. 217, 99–103. doi: 10.1016/j.bbr.2010.08.050
Kamida, T., Kong, S., Eshima, N., and Fujiki, M. (2013). Cathodal transcranial direct current stimulation affects seizures and cognition in fully amygdala-kindled rats. Neurol. Res. 35, 602–607. doi: 10.1179/1743132813Y.0000000170
Kandratavicius, L., Balista, P. A., Lopes-Aguiar, C., Ruggiero, R. N., Umeoka, E. H., Garcia-Cairasco, N., et al. (2014). Animal models of epilepsy: use and limitations. Neuropsychiatr. Dis. Treat. 10, 1693–1705. doi: 10.2147/NDT.S50371
Lason, W., Chlebicka, M., and Rejdak, K. (2013). Research advances in basic mechanisms of seizures and antiepileptic drug action. Pharmacol. Rep. 65, 787–801. doi: 10.1016/S1734-1140(13)71060-0
Lefaucheur, J. P. (2016). A comprehensive database of published tDCS clinical trials (2005-2016). Neurophysiol. Clin. 46, 319–398. doi: 10.1016/j.neucli.2016.10.002
Leite, J., Morales-Quezada, L., Carvalho, S., Thibaut, A., Doruk, D., Chen, C. F., et al. (2017). Surface EEG-transcranial direct current stimulation (tDCS) closed-loop system. Int. J. Neural Syst. 27:1750026. doi: 10.1142/S0129065717500265
Liebetanz, D., Fregni, F., Monte-Silva, K. K., Oliveira, M. B., Amâncio-dos-Santos, A., Nitsche, M. A., et al. (2006a). After-effects of transcranial direct current stimulation (tDCS) on cortical spreading depression. Neurosci. Lett. 398, 85–90. doi: 10.1016/j.neulet.2005.12.058
Liebetanz, D., Klinkerm, F., Hering, D., Koch, R., Nitsche, M. A., Potschka, H., et al. (2006b). Anticonvulsant effects of transcranial direct-current stimulation (tDCS) in the rat cortical ramp model of focal epilepsy. Epilepsia 47, 1216–1224. doi: 10.1111/j.1528-1167.2006.00539.x
Loscher, W. (2002). Current status and future directions in the pharmacotherapy of epilepsy. Trends Pharmacol. Sci. 23, 113–118. doi: 10.1016/S0165-6147(00)01974-X
Löscher, W. (2011). Critical review of current animal models of seizures and epilepsy used in the discovery and development of new antiepileptic drugs. Seizure 20, 359–368. doi: 10.1016/j.seizure.2011.01.003
Loscher, W., and Schmidt, D. (2004). New horizons in the development of antiepileptic drugs: the search for new targets. Epilepsy Res. 60, 77–159. doi: 10.1016/j.eplepsyres.2004.06.004
McCormick, D. A., and Contreras, D. (2001). On the cellular and network bases of epileptic seizures. Annu. Rev. Physiol. 63, 815–846. doi: 10.1146/annurev.physiol.63.1.815
Medeiros, L. F., de Souza, I. C., Vidor, L. P., de Souza, A., Deitos, A., Volz, M. S., et al. (2012). Neurobiological effects of transcranial direct current stimulation: a review. Front. Psychiatr. 3:110. doi: 10.3389/fpsyt.2012.00110
Nitsche, M. A., Cohen, L. G., Wassermann, E. M., Priori, A., Lang, N., Antal, A., et al. (2008). Transcranial direct current stimulation: state of the art. Brain Stimul. 1, 206–223. doi: 10.1016/j.brs.2008.06.004
Nitsche, M. A., Liebetanz, D., Lang, N., Antal, A., Tergau, F., and Paulus, W. (2003). Safety criteria for transcranial direct current stimulation (tDCS) in humans. Clin. Neurophysiol. 114, 2220–2222. doi: 10.1016/S1388-2457(03)00235-9
Nitsche, M. A., and Paulus, W. (2009). Noninvasive brain stimulation protocols in the treatment of epilepsy: current state and perspectives. Neurotherapeutics 6, 244–250. doi: 10.1016/j.nurt.2009.01.003
Pereira, L. S., Müller, V. T., da Mota Gomes, M., Rotenberg, A., and Fregni, F. (2016). Safety of repetitive transcranial magnetic stimulation in patients with epilepsy: a systematic review. Epilepsy Behav. 57, 167–176. doi: 10.1016/j.yebeh.2016.01.015
Petrovski, S., Szoeke, C. E. I., Jones, N. C., Salzberg, M. R., Sheffield, L. J., Huggins, R. M., et al. (2010). Neuropsychiatric symptomatology predicts seizure recurrence in newly treated patients. Neurology 75, 1015–1021. doi: 10.1212/WNL.0b013e3181f25b16
Raghavan, S., Eldabe, S., and Strachan, R. (2008). Novel trends in pain management – Neuromodulation. Curr. Anaesth. Crit. Care 19, 344–348. doi: 10.1016/j.cacc.2008.07.005
Rosen, A. C., Ramkumar, M., Nguyen, T., and Hoeft, F. (2009). Noninvasive transcranial brain stimulation and pain. Curr. Pain Headache Rep. 13, 12–17. doi: 10.1007/s11916-009-0004-2
Rroji, O., van Kuyck, K., Nuttin, B., and Wenderoth, N. (2015). Anodal tDCS over the primary motor cortex facilitates long-term memory formation reflecting use-dependent plasticity. PLoS ONE 10:e0127270. doi: 10.1371/journal.pone.0127270
Ruscheweyh, R., Wilder-Smith, O., Drdla, R., Liu, X. G., and Sandkühler, J. (2011). Long-term potentiation in spinal nociceptive pathways as a novel target for pain therapy. Mol. Pain 7:20. doi: 10.1186/1744-8069-7-20
San-Juan, D., Calcáneo, Jde, D., González-Aragón, M. F., Maldonado, L. B., Avellán, A. M., Argumosa, E. V., et al. (2011). Transcranial direct current stimulation in adolescent and adult Rasmussen's encephalitis. Epilepsy Behav. 20, 126–131. doi: 10.1016/j.yebeh.2010.10.031
San-Juan, D., Espinoza López, D. A., Vázquez Gregorio, R., Trenado, C., Fernández-González Aragón, M., Morales-Quezada, L., et al. (2017). Transcranial direct current stimulation in mesial temporal lobe epilepsy and Hippocampal Sclerosis. Brain Stimul. 10, 28–35. doi: 10.1016/j.brs.2016.08.013
San-Juan, D., Morales-Quezada, L., Orozco Garduño, A. J., Alonso-Vanegas, M., González-Aragón, M. F., Espinoza López, D. A., et al. (2015). Transcranial direct current stimulation in epilepsy. Brain Stimul. 8, 455–464. doi: 10.1016/j.brs.2015.01.001
Scorza, F. A., and Brunoni, A. R. (2015). Transcranial direct current stimulation against sudden unexpected death in epilepsy: press that button again, please. Brain Stimul. 8, 839–840. doi: 10.1016/j.brs.2015.04.006
Sloviter, R. S. (2008). Hippocampal epileptogenesis in animal models of mesial temporal lobe epilepsy with hippocampal sclerosis: the importance of the “latent period” and other concepts. Epilepsia 49, 85–92. doi: 10.1111/j.1528-1167.2008.01931.x
Tatti, E., Rossi, S., Innocenti, I., Rossi, A., and Santarnecchi, E. (2016). Non-invasive brain stimulation of the aging brain: state of the art and future perspectives. Ageing Res. Rev. 29, 66–89. doi: 10.1016/j.arr.2016.05.006
Tekturk, P., Erdogan, E. T., Kurt, A., Kocagoncu, E., Kucuk, Z., Kinay, D., et al. (2016a). Transcranial direct current stimulation improves seizure control in patients with Rasmussen encephalitis. Epileptic Disord. 18, 58–66. doi: 10.1684/epd.2016.0796
Tekturk, P., Erdogan, E. T., Kurt, A., Vanli-Yavuz, E. N., Ekizoglu, E., Kocagoncu, E., et al. (2016b). The effect of transcranial direct current stimulation on seizure frequency of patients with mesial temporal lobe epilepsy with hippocampal sclerosis. Clin. Neurol. Neurosurg. 149, 27–32. doi: 10.1016/j.clineuro.2016.07.014
van Elst, L. T., Woermann, F. G., Lemieux, L., Thompson, P. J., and Trimble, M. R. (2000). Affective aggression in patients with temporal lobe epilepsy: a quantitative MRI study of the amygdale. Brain 123, 234–243. doi: 10.1093/brain/123.2.234
Woods, A. J., Antal, A., Bikson, M., Boggio, P. S., Brunoni, A. R., Celnik, P., et al. (2016). A technical guide to tDCS, and related non-invasive brain stimulation tools. Clin. Neurophysiol. 127, 1031–1048. doi: 10.1016/j.clinph.2015.11.012
Yook, S. W., Park, S. H., Seo, J. H., Kim, S. J., and Ko, M. H. (2011). Suppression of seizure by cathodal transcranial direct current stimulation in an epileptic patient - a case report. Ann. Rehabil. Med. 35, 579–582. doi: 10.5535/arm.2011.35.4.579
Zhang, Z., Liao, W., Xu, Q., Wei, W., Zhou, H. J., Sun, K., et al. (2017). Hippocampus-associated causal network of structural covariance measuring structural damage progression in temporal lobe epilepsy. Hum. Brain Mapp. 38, 753–766. doi: 10.1002/hbm.23415
Zobeiri, M., and van Luijtelaar, G. (2013). Noninvasive transcranial direct current stimulation in a genetic absence model. Epilepsy Behav. 26, 42–50. doi: 10.1016/j.yebeh.2012.10.018
Keywords: animal models, clinical trials, epilepsy, non-invasive brain stimulation, transcranial direct current stimulation
Citation: Regner GG, Pereira P, Leffa DT, de Oliveira C, Vercelino R, Fregni F and Torres ILS (2018) Preclinical to Clinical Translation of Studies of Transcranial Direct-Current Stimulation in the Treatment of Epilepsy: A Systematic Review. Front. Neurosci. 12:189. doi: 10.3389/fnins.2018.00189
Received: 14 December 2017; Accepted: 08 March 2018;
Published: 22 March 2018.
Edited by:
Francisco Lopez-Munoz, Universidad Camilo José Cela, SpainReviewed by:
Vikas Mishra, Babasaheb Bhimrao Ambedkar University, IndiaVincenzo Marra, University of Leicester, United Kingdom
Copyright © 2018 Regner, Pereira, Leffa, de Oliveira, Vercelino, Fregni and Torres. This is an open-access article distributed under the terms of the Creative Commons Attribution License (CC BY). The use, distribution or reproduction in other forums is permitted, provided the original author(s) and the copyright owner are credited and that the original publication in this journal is cited, in accordance with accepted academic practice. No use, distribution or reproduction is permitted which does not comply with these terms.
*Correspondence: Patrícia Pereira, cGF0cmljaWFwZXJlaXJhQHVmcmdzLmJy