- 1Department of Biochemistry and Biophysics, Universidade Federal da Bahia, Salvador, Brazil
- 2Department of Nutrition, Universidade Federal de Pernambuco, Recife, Brazil
Astrocytes can protect neurons against oxidative stress and excitability-dependent disorders, such as epilepsy. Valeriana officinalis has been used as anticonvulsant and can exert an antioxidant effect, which may underlie its opposing action against the toxic effects of the pesticide rotenone. We investigated the V. officinalis/rotenone interaction in the cortical spreading depression (CSD), a phenomenon that depends upon brain excitability (in vivo model). In addition, we analyzed the protective action of V. officinalis against the cytotoxic effects of rotenone in cultures of rat C6 glioma cells (in vitro model). For the CSD study, Wistar rats received either V. officinalis (250 mg/kg/day via gavage for 15 days; n = 8) or 10 mg/kg/day rotenone via subcutaneous injections for 7 days (n = 7), or they received both substances (n = 5). Two control groups received either saline (vehicle for V. officinalis; n = 8) or 1% Tween-80 aqueous solution (vehicle for rotenone; n = 9). After treatment, CSD was recorded for 4 h. The rotenone- and V. officinalis-treated groups presented, respectively, with lower (2.96 ± 0.14 mm/min), and higher CSD propagation velocity (3.81 ± 0.10 mm/min) when compared with the controls (Tween-80, 3.37 ± 0.06 mm/min and saline, 3.35 ± 0.08 mm/min; p < 0.05). The rotenone plus V. officinalis-treated group displayed a CSD velocity (3.38 ± 0.07 mm/min) that was similar to controls. In line with these results, in vitro experiments on rat glioma C6 cells revealed a protective effect (MTT assay) of V. officinalis against rotenone-induced cytotoxicity. These results suggest the therapeutic potential of V. officinalis for treating neurological diseases involving redox imbalance and astrocyte dysfunction.
Introduction
Astrocytes are intimately involved in diverse neuronal functions, such as modulating synaptic activity and plasticity; regulating the extracellular microenvironment by buffering neurotransmitter, ion, and water content, and regulating local blood flow and the delivery of energy substrates (Devinsky et al., 2012). Dysregulation of astrocyte function may cause seizures or promote epileptogenesis (Wetherington et al., 2008; Chan et al., 2019).
The brain is very sensitive to oxidative stress because of its high rate of O2 and energy consumption, relatively low levels of antioxidants, and large amount of oxidizable unsaturated fatty acids (Coimbra-Costa et al., 2017). The combined occurrence of high utilization of energy and impairment of oxidative phosphorylation jeopardizes the cells’ capacity to regulate their energy levels. This can lead to excessive production of reactive oxygen species (ROS), causing neuronal injury. Impaired energy metabolism may play a critical role in the neuronal injury caused by oxidant substance-induced epilepsy (Gupta et al., 2000; Bolson and Steinhäuser, 2018).
The plant known as Valeriana officinalis L., Valerianaceae has long been used for the treatment of insomnia, and it is recognized as an effective herbal sedative worldwide. In some countries, V. officinalis was additionally a reasonably popular anticonvulsant remedy in the past (Gorji and Khaleghi Ghadiri, 2001; Eadie, 2004). The aqueous extract of V. officinalis exerts effective antiepileptic action in the model of temporal lobe epilepsy (Rezvani et al., 2010; Wu et al., 2017). It is believed that the pharmacological activity of V. officinalis depends on its antioxidant action, and data in the literature have pointed to a protective effect of V. officinalis in rotenone-induced neural toxicity (Oliveira et al., 2009; Sudati et al., 2013). Despite the fact that in those studies V. officinalis protected neurons against rotenone-induced cytotoxicity, the protective effect was not tested in cells of glial origin. This is important because astrocytes supposedly play a role in the loss of dopaminergic neurons in Parkinson’s disease (Nagatsu and Sawada, 2005). Our work advances the knowledge in the field by studying (to the best of our knowledge for the first time) the V. officinalis effects on cells of glial origin. Furthermore, we demonstrated a novel action of V. officinalis extract on the cortical spreading depression (CSD) phenomenon in vivo (see below), which also represents an advancement of the knowledge in this field. Supported by these results, the present study investigated the hypothesis that V. officinalis methanol extract protects cells of glial origin against rotenone-induced cytotoxicity in vitro.
Rotenone is an aromatic compound that is largely employed as pesticide (Jenner, 2001). Rotenone passes across cell membranes without difficulty, as it is a very lipophilic compound and does not depend upon membrane transporters (Greenamyre et al., 2001). Rotenone inhibits the mitochondrial enzyme NADH dehydrogenase, acting within complex I of the respiratory chain (Talpade et al., 2000; Thiffault et al., 2000; Pamies et al., 2018) producing free-radical-dependent oxidative injury (Chen et al., 2008). Therefore, exposing rats to rotenone constitutes an interesting and useful model for studying brain disorders that are related to redox imbalance. Indeed, studies have stressed the importance of astrocytes in the protection of neurons from rotenone (Rathinam et al., 2012; Goswami et al., 2015). Furthermore, therapeutic efforts aimed at the removal of free radicals or prevention of their formation in the brain may be beneficial in epilepsy. Natural products represent an important source of chemical compounds, as they often have potent biological activities with promising pharmacological profiles.
The in vivo experiments in this study investigated the electrophysiological effects of rotenone administration and the counteracting effect of treatment with V. officinalis on the velocity of propagation of CSD in the rat brain. CSD is a reversible response of the brain tissue to electrical, mechanical, or chemical stimuli. The response consists of cell depolarization with subsequent electrical depression, which propagates slowly over the tissue (Leão, 1944, 1947; Guedes and Abadie-Guedes, 2019). CSD has been further electrophysiologically described in many animal species (Gorji, 2001) and in humans (Dreier et al., 2019). Modifications in brain excitability (Koroleva and Bures, 1980) and in the redox homeostasis of the brain (El-Bachá et al., 1998; Mendes-da-Silva et al., 2014) are factors that modulate CSD. Analyzing some CSD features (e.g., velocity of propagation) constitutes a very useful way of evaluating brain sensitivity to CSD under conditions that can modify brain excitability (Amaral et al., 2009; Guedes and Abadie-Guedes, 2019) and therefore are clinically relevant. Certain experimental treatments can facilitate CSD propagation, whereas other treatments can antagonize CSD (Guedes and Abadie-Guedes, 2019). These treatments can help in the comprehension of the CSD mechanisms and perhaps shed light on the mechanisms of CSD-related diseases, such as epilepsy (Leão, 1944, 1972; Guedes and Cavalheiro, 1997; Guedes and Abadie-Guedes, 2019).
Extracts of medicinal plants have beneficial effects in experimental models both in vivo (Rezvani et al., 2010 for V. officinalis, and Ilesanmi et al., 2019 for Antiaris africana) and in vitro (Areiza-Mazo et al., 2018 for Physalis peruviana). Therefore, in addition to the CSD model, we analyzed the V. officinalis/rotenone interaction in a set of in vitro experiments in the rat C6 glioma cell culture model (Grobben et al., 2002; Krylova et al., 2019). Rat C6 glioma cells have been used to study the effects of valproic acid as an inhibitor of histone deacetylase (Ximenes et al., 2012). This short-chain fatty acid is an analog of valeric acid, which is present in V. officinalis. Since these cells preserve several characteristics of astrocytes and are easy to cultivate, they were used in this work to investigate the protective effect of the methanolic extract of V. officinalis root powder against rotenone-induced cytotoxicity.
Using the CSD and the C6 glioma cell culture models, we addressed the following two questions: first, how does the administration of rotenone or V. officinalis affect CSD propagation and glial cell viability in culture, and second, does V. officinalis protect the rat brain against rotenone-induced toxicity? Here, we provide evidence that V. officinalis counteracts rotenone effects on CSD in the rat brain in vivo and protects against rotenone cytotoxicity on the viability of rat glioma C6 cells in vitro.
Materials and Methods
In vivo Experiments
Male Wistar rats (2 months old; 240–270 g body weight; n = 37) were housed in polypropylene cages (51 × 35.5 × 18.5 cm) under controlled temperature (23 ± 1°C), and a 12:12 h light:dark cycle (lights on at 7:00 a.m.); animals were fed a lab chow diet containing 23% protein. All experiments were performed between 13:00 and 18:00 h. The animals were handled in accordance with the norms of the Ethics Committee for Animal Research of our University. These norms are coherent with the “Principles of Laboratory Animal Care” (NIH; Bethesda, United States). All efforts were made to minimize animal suffering and to reduce the number of animals used.
Valeriana officinalis root powder was obtained from “Farmácia Homeopática Única,” a local manipulation pharmacy. The powder from the pulverized rhizome of the plant was diluted in water (125 mg/ml), heated to 100°C for 10 min, and then centrifuged in the water bath at 672 g for 10 min. Then, the aqueous extract was filtered and stored at 4°C. The extract and saline (vehicle) solutions were administered orally by gavage for 15 days (postnatal days 60–74). Each animal (n = 8 vehicle-treated and 8 valerian-treated rats) received either a single daily gavage of 2 ml/kg vehicle or V. officinalis extract at a dose of 250 mg/kg/d in a volume of 2 ml/kg. The doses of V. officinalis were based on the daily recommended dose for an adult human, which is 3,060 mg (as suggested on the bottle of a commercially available product).
Rotenone (obtained commercially from Sigma, St. Louis, MO, United States) was suspended in 1% Tween-80 solution in water and dispersed by sonication. Administration of rotenone consisted of daily subcutaneous injections at a dose of 10 mg/kg/d for 7 days (postnatal days 68–74) in a volume of 1 ml/kg (n = 7 rats). This dose was chosen based on previous studies in rodents (Dodiya et al., 2018; Perez-Pardo et al., 2018). The solution was freshly prepared every day. The control group (n = 9) was treated with the vehicle (1% Tween-80 solution) in a similar volume and time schedule. In the group that received V. officinalis and rotenone treatment simultaneously (n = 5), the subcutaneous rotenone injection occurred over the last 7 days of the 15-day period of V. officinalis administration.
The electrophysiological recording of CSD occurred on postnatal day 75–80. The animals were anesthetized with a mixture of 1 g/kg urethane plus 40 mg/kg chloralose via intraperitoneal injection. Three trephine holes (2–3 mm in diameter, aligned in the frontal-to-occipital direction, and parallel to the midline) were drilled into the right side of the skull. The CSD-eliciting stimulus (a 1–2 mm diameter cotton ball saturated with a 2% KCl solution) was applied for 1 min to the frontal hole. This stimulus, which elicited a single CSD episode, was repeated at 20-min intervals over the 4 h of the recording session. Two recording electrodes (Ag-AgCl type) were placed on the two parietal holes. These electrodes were glued one-to-the-other with cyanoacrylate glue, and presented a fixed interelectrode distance. A third Ag-AgCl electrode was positioned on the nasal bones and was used as common reference electrode (Lima et al., 2014). We calculated the CSD velocity of propagation dividing the interelectrode distance by the time required for a CSD wave to pass that distance. Rectal temperature was maintained at 37 ± 1°C with the help of a heating blanket. At the end of the recording session, the animals were euthanized with an overdose of anesthetic.
In vitro Experiments
The C6 rat glioma cell culture was prepared following previously described procedures (Goswami et al., 2015). Briefly, cells were placed on plastic plates (10 cm diameter) with Dulbecco’s modified Eagle’s medium (DMEM) supplemented with 1 mM pyruvic acid, 2 mM L-(+)-glutamine, 44 mM NaHCO3, 10% fetal bovine serum, 100 IU/ml penicillin, and 100 μg/ml streptomycin. The culture medium was renewed every other day. Cultures were maintained at 37°C until complete confluence (approximately 2–4 days), then they were trypsinized and placed on 96-well plates (3.1 × 104 cells/cm2). In a triplicate experiment, cells were treated with rotenone concentrations ranging from 1 to 200 nM or with 0.5% DMSO (vehicle) for 48 h to determine the cytotoxic concentration of rotenone that killed 50% of cells (EC50). This concentration was used to investigate the protective effect of V. officinalis. The root powder of V. officinalis was successively extracted three times for 3 days in petroleum ether, dichloromethane, and methanol. The methanol extract was used in this work containing between 0.2 μg/ml and 153 μg/ml of the V. officinalis powder in a duplicate experiment. We evaluated cell viability by using the dye 3-(4,5-dimethylthiazol-2-yl)-2,5-diphenyltetrazolium bromide (MTT assay; Hansen et al., 1989). Briefly, 1 mg/ml MTT was added to each well, and after a 2 h-incubation at 37°C, 100 μl of lysis buffer [20% (w/v) sodium dodecyl sulfate (SDS) in 50% (v/v) N,N-dimethylformamide, pH 4.7] was added to each well. The optical density, which is proportional to the number of viable cells, was evaluated with a microplate reader at 595 nm. The cell morphology was examined by phase-contrast microscopy.
Statistical Analysis
For the in vivo experiments, statistical differences were analyzed using Sigmastat® statistical software (version 3.5, San Jose, CA, United States). Intergroup differences were compared using ANOVA. When indicated, ANOVA was followed by a post hoc (Holm-Sidak) test. Differences were considered significant when p < 0.05.
For the in vitro experiments, we used GraphPad Prism version 5.00 for Windows (GraphPad Software, San Diego, CA, United States). The D’Agostino and Person omnibus normality test was used to identify whether data presented normal distribution. Data were expressed as the mean ± SEM or median and ranges according to the distribution. Additionally, according to the distribution, parametric or nonparametric statistic tests were chosen, as indicated in the figure legends. Probability values of p < 0.05 were accepted as indications of statistically significant differences. Nonlinear regressions were carried out to fit cell viability data in order to determine the rotenone concentration that killed 50% of cells (EC50).
Results
In vivo Experiments
The body weights of the rats in the five groups are presented in Figure 1. At the beginning of the treatment (postnatal day 60), body weights were comparable in the five groups (mean weights ranging from 257.6 ± 14.1 g to 260.0 ± 13.5 g). At the end of the treatment period (postnatal day 75), the rotenone-treated group had a lower mean body weight (267.9 ± 19.7 g) when compared with the Tween-80 group (299.2 ± 28.3 g), the saline group (293.5 ± 21.5 g), and the V. officinalis group (289.9 ± 24.6 g; p < 0.05).
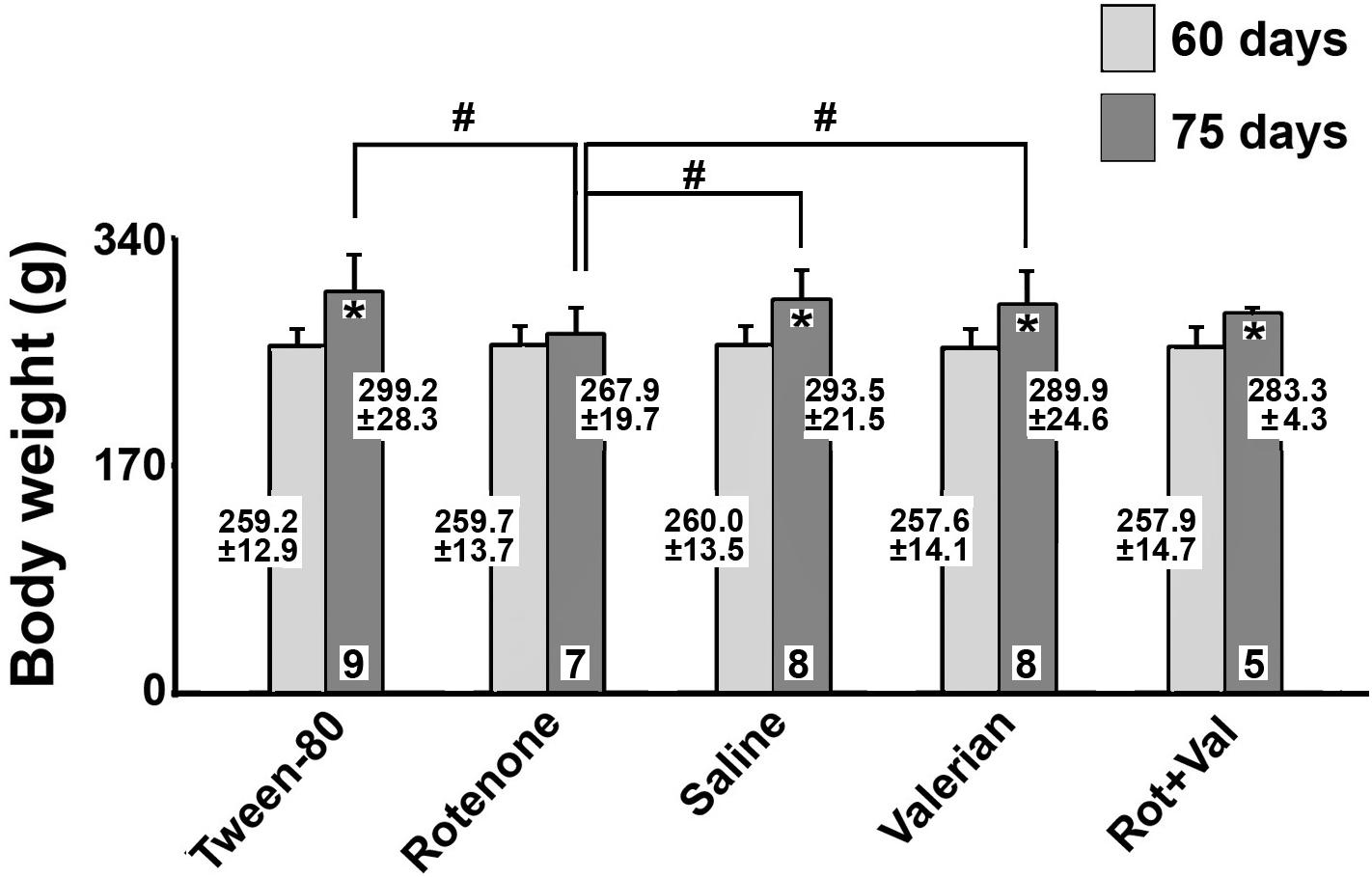
Figure 1. Body weights (mean ± standard deviation; values are given at the bars) of Wistar rats treated via subcutaneous injection of Tween-80 or rotenone or via gavage with saline solution, valerian, or with rotenone + valerian (Rot+Val). Body weights were measured on postnatal day 60 (beginning of experiment), and postnatal day 75 (end of experiment). *p < 0.05 compared with the corresponding 60 day-value. #p < 0.05 as indicated by the lines (one-way ANOVA followed by the Holm-Sidak test).
CSD Propagation Velocity
Topical application of 2% KCl for 1 min on the frontal cortex initiated CSD that was recorded at two points on the parietal surface. In parts A and B of Figure 2 we show examples of CSD recordings in five rats that were treated with saline, valerian, Tween-80%, rotenone, or valerian plus rotenone. After a KCl-elicited CSD initiates, the cerebral cortex activity recovers within a few minutes (usually less than 10 min; Leão, 1944). Considering this recovery time, we established 20 min as time-interval to repeat the KCl stimulation over the 4 h of the recording session.
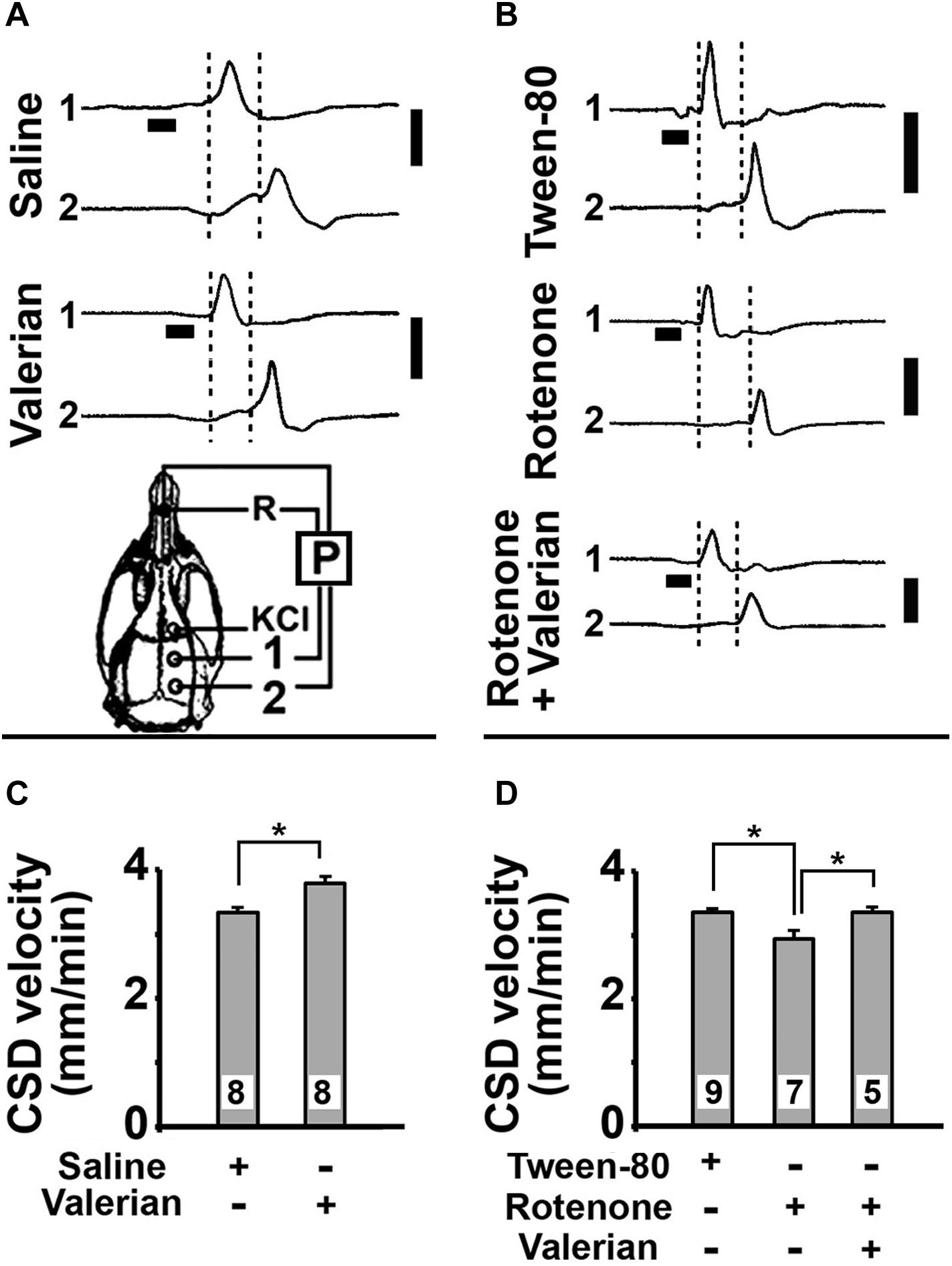
Figure 2. (A,B) Electrophysiological recordings (slow potential changes, P) of CSD in five rats that were treated per gavage with V. officinalis (250 mg/kg/d) or saline solution for 15 days, or with s.c. injections of Tween-80 (control group) or rotenone (10 mg/kg/d) for 7 days, or treated with s.c. injections of rotenone plus gavage with V. officinalis. The vertical solid bars at the right of the traces indicate 10 mV (negative upwards). The horizontal bars under the traces from the recording point 1 indicate the time (1 min) of application of the CSD-eliciting stimulus (a 2 mm diameter cotton ball soaked with 2% KCl solution) on the intact dura mater. Once elicited in the frontal cortex, CSD propagated and was recorded by the two cortical electrodes located at the parietal cortex (skull diagram, points 1 and 2). A third electrode of the same type was placed on the nasal bones and served as a common reference (R) for the recording electrodes. The vertical dashed lines delimited the latency for the CSD wave to cross the interelectrode distance. When compared with the corresponding controls, the latencies in the V. officinalis group and in the rotenone group were, respectively, shorter and longer. (C,D) CSD velocity of propagation in the 75–80-day-old rats of the five groups described in (A,B). Values are presented as the mean ± standard deviation of the 12 CSD measurements per rat, along the 4 h recording period, with the number of animals given at the bottom part of the bars. Asterisks indicate intergroup differences (p < 0.05; ANOVA plus the Holm-Sidak test).
Figures 2C,D presents the mean CSD propagation velocities in the five groups of rats that were studied in vivo. ANOVA revealed a significant difference between the treatment groups [F(4,32) = 75.98; p < 0.001]. The post hoc (Holm-Sidak) test revealed that CSD velocity was significantly higher in the valerian-treated group (3.81 ± 0.10 mm/min) compared to the saline-treated group (3.35 ± 0.08 mm/min; p < 0.005). The rotenone-treated animals displayed lower CSD velocities (2.96 ± 0.14 mm/min) in comparison to the Tween-80-treated controls (3.37 ± 0.06 mm/min; p < 0.007). The group treated with rotenone plus valerian displayed a mean CSD velocity (3.38 ± 0.07 mm/min) that was similar to the controls (saline: 3.35 ± 0.08 mm/min; Tween-80: 3.37 ± 0.06 mm/min).
In vitro Experiments
The Extract of V. officinalis Protected Cell Cultures Against Rotenone-Induced Cytotoxicity
Rotenone treatment induced a cytotoxic effect to C6 cells after 48 h in a concentration-dependent manner. The median EC50 for rotenone was 3.5 nM (Range: 0.4–4.1 nM; n = 3). Data from the experiment corresponding to the median value are shown in Figure 3. Data fitted to Equation (below) using nonlinear regression:
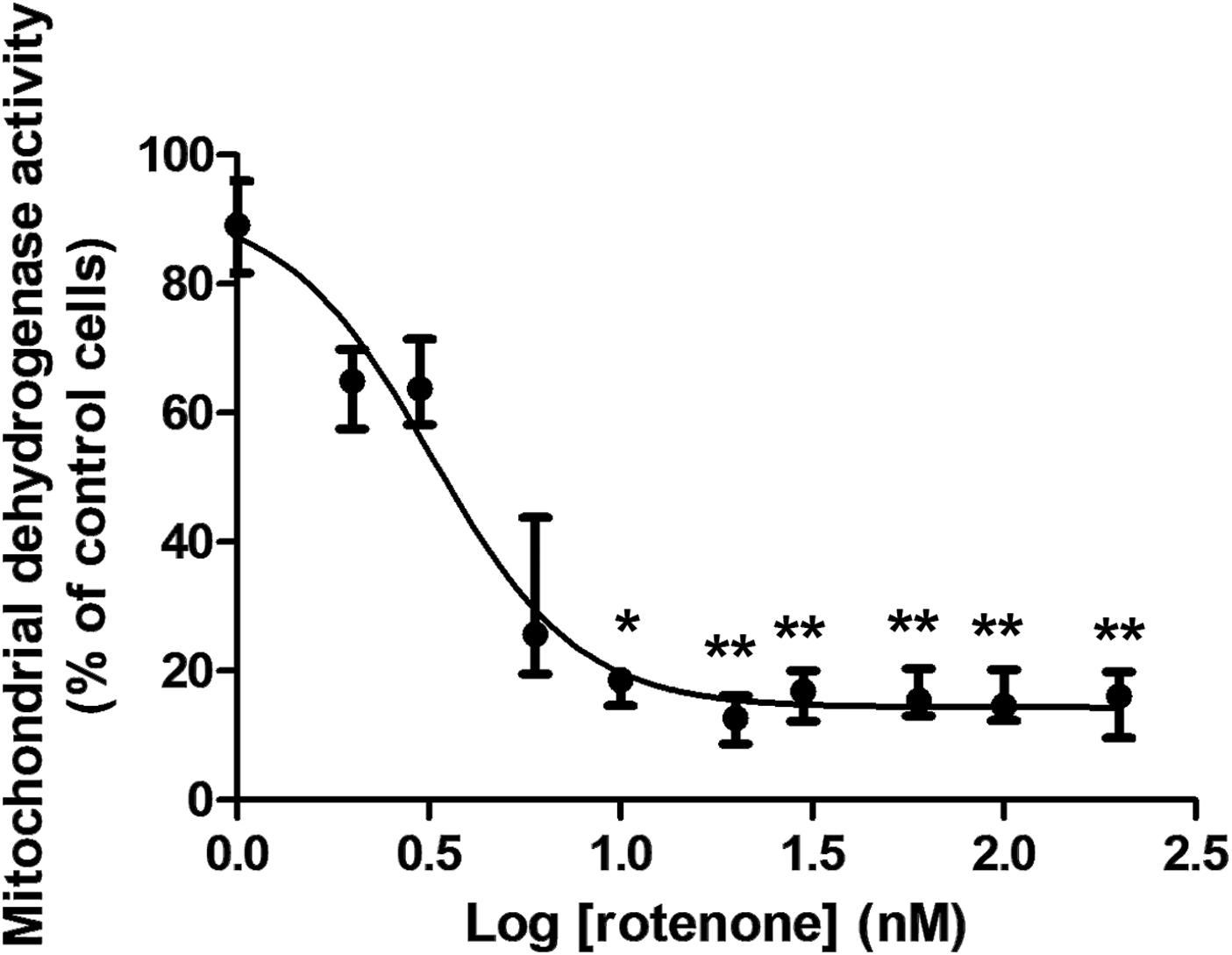
Figure 3. Cytotoxic effect of rotenone to rat glioma C6 cells after 48 h measured by the MTT assay. The graph represents the experiment corresponding to the median EC50, which was 3.5 nM. Since the data did not follow a normal distribution, they were represented by medians and ranges. Cell viability corresponds to the mitochondrial dehydrogenase activity measured at 595 nm. Data were normalized to the median absorbance of the control cells treated only with 0.5% DMSO (Median: 1.041; Range: 1.026–1.237; n = 8), which was considered a 100% cell viability. Data analyzed by the Kruskal–Wallis test showed a significant effect for rotenone concentration (p < 0.0001). Groups were compared by Dunn’s multiple comparison test (n = 8 for every concentration). Statistical significance: *p < 0.05; **p < 0.0001 compared to control cells.
in which V is the percentage of cell viability and C is the rotenone concentration.
In comparison to the vehicle-treated cultures (treated with 0.5% DMSO; Figure 4A), rotenone treatment induced a concentration-dependent cytotoxic effect on C6 cells after 48 h, as evaluated by decreased cell viability (Figure 4B). Treatment with V. officinalis did protect the cells against the toxic action of rotenone (Figure 4C).
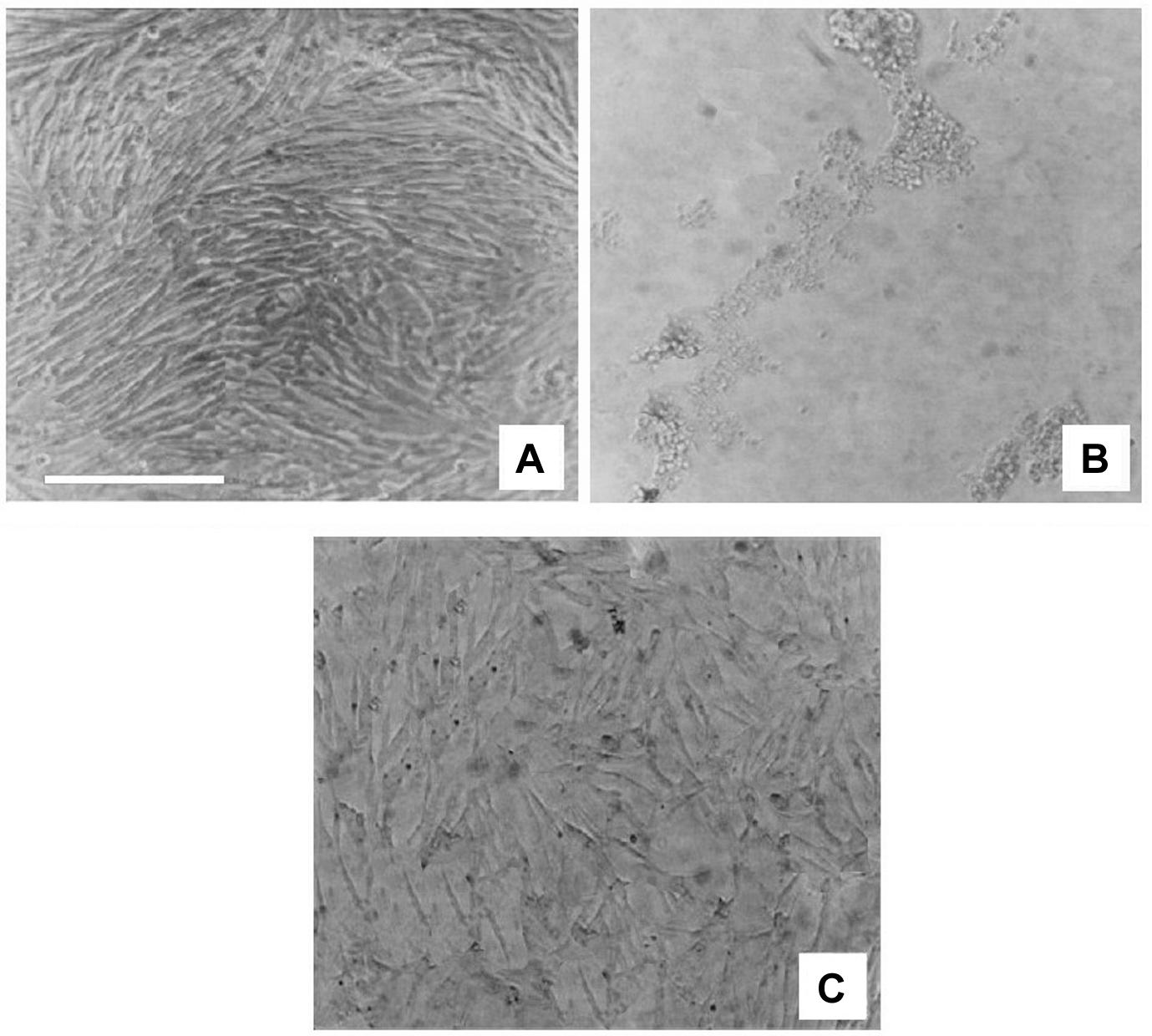
Figure 4. Photomicrographs (phase-contrast microscopy) of C6 rat glioma cell cultures. In (A), we see a culture that received vehicle treatment (DMSO 0.5%). After rotenone application, cell viability decreased significantly (B). The application of 132.1 μg/ml V. officinalis to a rotenone-treated culture (C) protected the culture against the rotenone toxic effect. Scale bar = 100 μm.
Therefore, the rotenone concentration of 3.5 nM was used for further experiments in order to study the protective effects of the methanolic extract of V. officinalis root powder (Figure 5). A significant protective effect of V. officinalis against rotenone-induced cytotoxicity was observed. The median of the minimal cytoprotective concentration of V. officinalis was 74.8 μg/ml (Range: 40.0–105.6 μg/mL; n = 2).
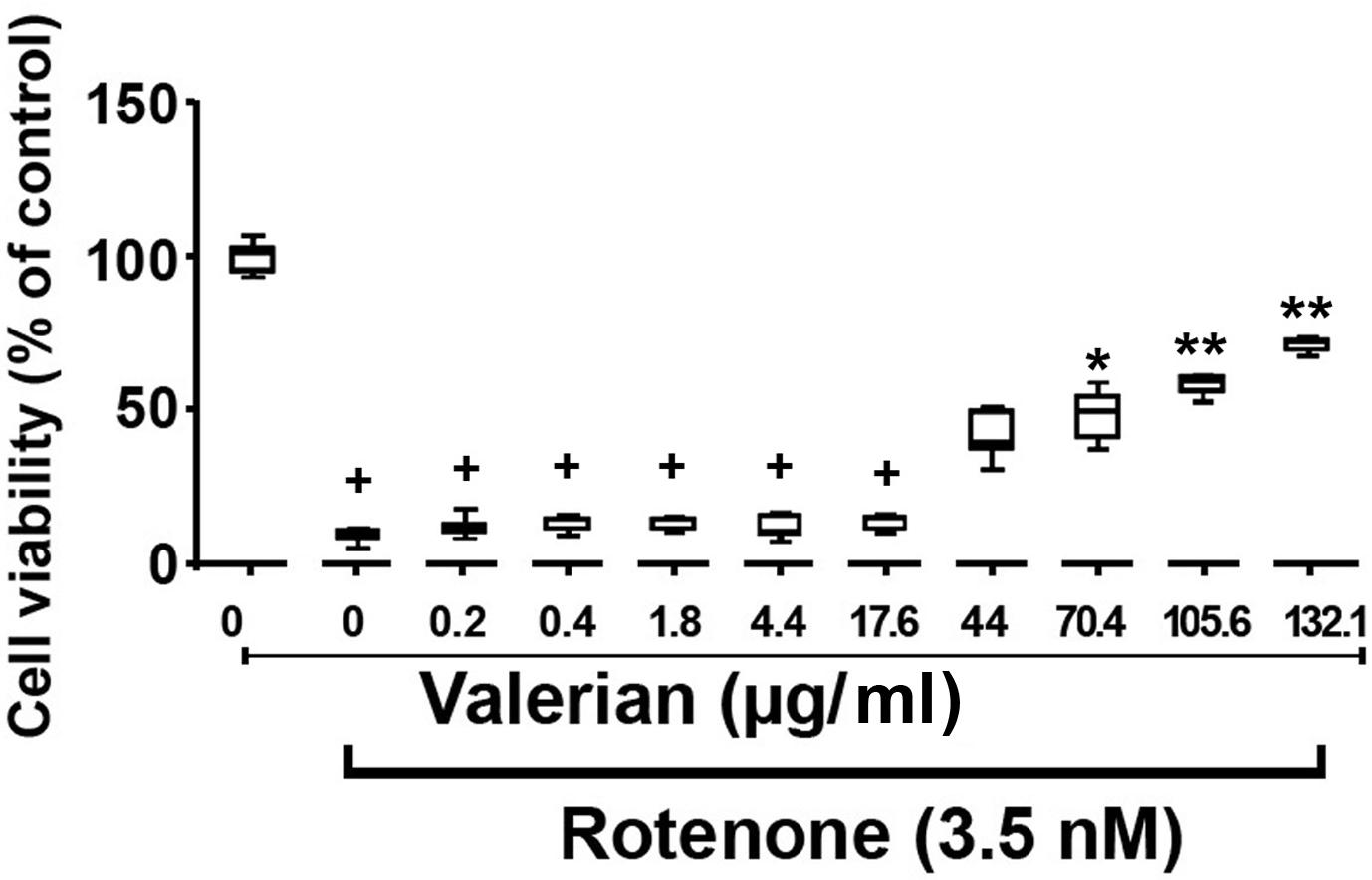
Figure 5. Cytoprotective effect of the methanolic extract of V. officinalis root powder against rotenone-induced cytotoxicity in rat glioma C6 cells after 48 h. Cell viability corresponds to the mitochondrial dehydrogenase activity measured by the MTT assay. Data were normalized to the control group, in which cells were treated only with the solvent (0.5% DMSO), which was considered to have 100% cell viability. Since data did not follow a normal distribution, they were represented by their medians and ranges. The group treated with 3.5 nM rotenone was compared with the group treated only with DMSO by the Mann-Whitney test. All groups treated with 3.5 nM rotenone and the methanolic extract of V. officinalis root powder between 0.0–132.1 μg/ml were analyzed by the Kruskal–Wallis nonparametric test followed by Dunn’s multiple comparisons test. Statistical significance: +p < 0.0005 compared to cells treated only with DMSO; *p < 0.05; **p < 0.0005 compared to cells treated only with 3.5 nM rotenone.
Discussion
The present results constitute the first evidence that systemic administration of V. officinalis and rotenone exert opposite effects on CSD propagation in the rat cortex. Data on this novel electrophysiological action of V. officinalis indicate that the treatment with this substance accelerated CSD propagation, whereas rotenone treatment decelerated CSD. Moreover, the combined administration of valerian plus rotenone in the same animal showed that valerian has the ability to protect the brain against the CSD velocity alterations induced by rotenone. Because ovarian hormones seem to influence CSD (Accioly et al., 2012; Accioly and Guedes, 2017), we decided to use only male rats.
The observation that epileptiform EEG waves appeared during CSD (Leão, 1944) originated the speculation that the mechanisms of CSD and epilepsy would be related (Leão, 1944, 1972). During epileptic seizures, redox imbalance occurs, with free radical production and lipid peroxidation; interestingly, all of these processes can cause tissue damage (Tuszkiewicz-Misztal et al., 2000; Ilhan et al., 2006) and can be counteracted by antioxidant compounds that may be present in V. officinalis (Rezvani et al., 2010). However, since in the present study redox balance was not investigated, we cannot discuss this potential mechanism as being involved in the plant extract action.
There are some experimental and clinical pieces of evidence suggesting a positive effect of V. officinalis against convulsive disorders. In folkloric medicine, V. officinalis has been claimed as having an effective anticonvulsant action (Gorji and Khaleghi Ghadiri, 2001; Eadie, 2004). The anticonvulsant action of the aqueous extract of V. officinalis was shown by Rezvani et al. (2010) in amygdala-kindled rats using the ethanolic extract of V. officinalis, a similar effect reported by Hiller and Zetler (1996), and Petkov and Manolov (1975), respectively, in picrotoxin-induced, and pentylenetetrazole- and strychnine-induced convulsions in mice.
In the in vivo part of this study, the effect of an aqueous extract of valerian on CSD velocity was evaluated. Our data suggest that administration of V. officinalis was associated with brain changes that resulted in acceleration of CSD propagation. Because no CSD alteration was found in gavage-treated rats (as controls), we can discard the possibility that the CSD effect in the V. officinalis group would be produced by the stress of the gavage process, as recently postulated for other compound (Gondim-Silva et al., 2019). This finding confirms previous data indicating that substances with anticonvulsant activity (e.g., dipyrone; Doretto et al., 1998) enhance the CSD velocity (Amaral et al., 2009). Moreover, these data are similar to previous findings showing that low and high doses of the antioxidant vitamin ascorbic acid decelerated and accelerated CSD, respectively, in rats (Mendes-da-Silva et al., 2014, 2018). Taken together, these findings are in accordance with the idea that the CSD, as an interesting phenomenon, can help in extending our knowledge about the involvement of redox imbalance into the pathogenesis of neurological diseases (Guedes et al., 2012) with the possible involvement of astrocytes (Torrente et al., 2014). This possibility shall be investigated in the near future.
Another important finding of the present study was the protective effect of V. officinalis on CSD-velocity changes induced by rotenone. Two interpretations can derive from the group treated simultaneously with V. officinalis+rotenone (Figures 2B–D): (a) the plant extract protects against the rotenone effects on CSD propagation, or (b) rotenone inhibits the increment of CSD velocity induced by V. officinalis extracts. The first interpretation (a) is supported by our in vitro outcome (Figures 3–5), showing a protecting action of V. officinalis against the rotenone effects on rat C6 glioma cells. Our data on body weight effects (Figure 1) also favor this first interpretation, which is, in addition, coherent with data from others showing several kinds of neural protection that is exerted by V. officinalis (Sudati et al., 2013; Becker et al., 2014; Torres-Hernández et al., 2015; Mineo et al., 2017). Further specific investigations are required to test the hypotheses that have been raised to explain the mechanisms, through which V. officinalis protects the brain against convulsions. At this stage, we cannot disregard the hypothesis that several mechanisms may operate in producing seizures, depending on the distinct brain regions that are involved (Guedes et al., 1992; Amâncio-dos-Santos et al., 2006).
The underlying mechanisms of the beneficial CNS effects of V. officinalis is still a matter of debate. The involvement of the GABAergic system has been postulated based on in vitro studies (Mennini et al., 1993). However, mechanisms other than the GABAergic have to be considered. For example, the effects of some components of V. officinalis seem to be associated with their antioxidant activities. Indeed, Sudati et al. (2009) showed that V. officinalis prevents prooxidant-induced brain oxidative damage. Redox imbalance is associated with cell death that occurs in convulsive states and brain ischemia; interestingly, the treatment with antioxidant agents diminishes this effect (Pestana et al., 2010; Betti et al., 2011).
Since CSD’s are linked to a huge sustained depolarization of the glial membrane potential (Sugaya et al., 1975) the rotenone cytotoxicity to a cell of glial origin was investigated. Rotenone was cytotoxic to rat glioma C6 cells in the present work with an EC50 of 3.5 nM after 48 h. This compound acts as an inhibitor of mitochondrial complex I (Nicholls and Budd, 2000). Furthermore, 500 nM rotenone caused apoptosis after 24 h in exposed human dopaminergic SH-SY5Y neuroblastoma cells (Wang et al., 2002). In the cortex exposed to the combination of CSD and the injection of fluorocitrate, which is taken up selectively by astrocytes inhibiting the enzyme aconitase, potentially injured cells expressing the 72-kDa heat shock protein have been observed (Lian and Stringer, 2004). Rotenone is a complex aromatic compound that presents a modified catechol group in its molecular structure. Aromatic compounds such as catechol may be toxic to cells from the central nervous system (Lima et al., 2008). 1,3-Dinitrobenzene, which also induces a metabolic impairment, killed 50% of C6 cells after 36 h at the concentration of 630 μM (Miller et al., 2011). Therefore, rotenone was more cytotoxic than this compound. Rotenone at the concentration of 10 μM killed 50% of the non-dopaminergic neuronal Neuro-2a cells after 4 h (Swarnkar et al., 2012a). Rotenone at 1 μM killed approximately 50% of C6 cells after 24 h (Swarnkar et al., 2012b). Therefore, it seems that rotenone cytotoxicity to C6 cells is concentration- and time-dependant. Rotenone at 10 μM was more cytotoxic to differentiated neuroblastoma SH-SY5Y cells induced by retinoic acid than to undifferentiated ones after 24 h treatment (Jantas et al., 2013). This suggests that dopaminergic neuronal cells are more sensitive to rotenone than non-dopaminergic ones. Furthermore, the infusion of rotenone into the right substantia nigra in rats lowered the expression of tyrosine hydroxylase in mid brain after 7 days in a dose-dependent manner, which suggests the damage of dopaminergic neurons (Swarnkar et al., 2013). The compound 1-Methyl-4-phenyl-pyridine (MPP+) is another inhibitor of mitochondrial complex I that killed 50% of wild-type mouse astrocytes in cultures at the approximate concentration of 14 μM after 48 h (Khan et al., 2014). Therefore, rotenone was more cytotoxic to C6 cells than MPP+ was to mouse astrocytes. It seems that rotenone was also more cytotoxic to C6 rat glioma cells than to T98G human glioblastoma cells, since the EC50 was 50 μM after 24 h (Cabezas et al., 2015). Glial maturation factor, which is a highly conserved neuroinflammatory acidic protein that is released by damaged cells, decreases the viability of N27 rat dopaminergic neuronal cells in a concentration-dependent manner (Selvakumar et al., 2018). This means that this protein released by rotenone-damaged glial cells may mediate dopaminergic neuronal cell death. The use of rotenone in vitro and in vivo is a successful neurodegenerative model of Parkinson’s disease due to similarities between the neural rotenone effects and the symptoms of that neurological disorder (Cabezas et al., 2019). Pretreatment with medicinal plants such as Pistacia atlantica have demonstrated to be neuroprotective against Parkinson’s disease-inducing neurotoxins, like 6-hydroxydopamine and rotenone (Moeini et al., 2019). Therefore, the present study investigated the neuroprotective potential of V. officinalis.
The aqueous extract of V. officinalis was not cytotoxic up to 391 μg/ml but it presented an EC50 of 2.8 mg/ml to human neuroblastoma SH-SY5Y (Oliveira et al., 2009). Valproic acid, a compound derived from valeric acid, which is produced by V. officinalis, can counteract by long-term administration selective alterations of α-synuclein caused by rotenone in rodents (Chateauvieux et al., 2010). Valproate also mitigated the oxidative stress produced by FeCl3 in primary cultured rat cerebrocortical cells (Ximenes et al., 2012). Another molecule present in V. officinalis is 2S-hesperidin, a flavonoid with sedative effects in the central nervous system (Roohbakhsh et al., 2014).
In the present work, the median of the minimal cytoprotective concentration of the methanolic extract of V. officinalis against rotenone-induced cytotoxicity to C6 cells (Figure 5) was 74.8 μg/ml. V. officinalis was considered as a potential alternative phytodrug against neurodegenerative diseases (Perez-Hernandez et al., 2016). It also presented protective effects in Drosophila melanogaster subjects that had been challenged with rotenone (Sudati et al., 2013; Ríos et al., 2016). Another valerian species, V. amurensis P. Smirn. ex Kom, increased the cerebral cholinergic function, and decreased apoptosis induced by the amyloid-β peptide (1–40) in mice (Dey et al., 2017). People from the Pikuni-Blackfoot tribe use a mix of V. officinalis with Hypericum perforatum L. to make a tea with relaxant effects to treat the symptoms of Parkinson’s disease (de Rus Jacquet et al., 2017). The ethnopharmacological use of V. officinalis to treat dementia has also been considered (Tewari et al., 2018). V. officinalis is among the 12 herbal preparations officially listed by the European Medicines Agency for use as a medicinal product (Forni et al., 2019). Overall, these pieces of evidence allow us to postulate the methanolic extract of V. officinalis root powder as a promising product that deserves further fractionation to search compounds that exert medicinal effects. The fact that the composition of the valerian extract remains to be determined does not invalidate the publication of the present data that clearly show the extract’s protective effects. We hope that these data spark the interest of research agencies to fund new projects aiming to isolate and characterize these compounds. Despite the fact that there are currently no effective therapies for Parkinson’s disease and no available treatments to slow down its progression, new drugs (either synthesized or from natural products) as well as non-drug therapeutic options continue to be searched, such as cell transplantation and gene therapies (Jamebozorgi et al., 2019). In both cases (drug and no-drug therapy), pre-clinical in vitro and in vivo studies, specially using rodent models, are ethically necessary before these strategies can move toward clinical trials and applications, in order to solve some biosecurity aspects. The extrapolation of experimental findings from animals to humans is a complex and challenging process, which requires cautiousness. Despite this, our data globally permit the conclusion that investigation of the brain effects of natural products can benefit from experimental models, such as the two models here employed.
We conclude that the present results suggest the beneficial effect of V. officinalis in counteracting the alterations of CSD propagation velocity as well as of C6 rat glioma cytotoxicity induced by rotenone. This beneficial action of V. officinalis, which has been documented both in vivo and in vitro, deserves further pharmacological and molecular exploration aimed at its translational application.
Data Availability Statement
The raw data supporting the conclusions of this article will be made available by the authors, without undue reservation.
Ethics Statement
The animal study was reviewed and approved by Ethics Committee for Animal Research of the Federal University of Bahia, Brazil.
Author Contributions
AA and RG conceived and performed the in vivo experiments. AA and RE-B conceived and performed the in vitro experiments. IG participated substantially in the conduction of the in vivo experiments. AA, RE-B, and RG conducted the statistical analysis and prepared the manuscript. All authors contributed to the article and approved the submitted version.
Funding
Funding for this project was provided in part by the BNB/FUNDECI 2012.0004, Conselho Nacional de Desenvolvimento Científico e Tecnológico (CNPq no. 305998/2018-8), MCT/FINEP/CT-INFRA – PROINFRA – 01/2008, Instituto Nacional de Ciência e Tecnologia em Excitotoxicidade e Neuroproteção’ – Edital INCT/MCT/CNPq, and Capes (Finance Code 001; Edital 043/2013 Ciências Do Mar II and BEX 2036/15-0).
Conflict of Interest
The authors declare that the research was conducted in the absence of any commercial or financial relationships that could be construed as a potential conflict of interest.
References
Accioly, N. E., Benevides, R. D. L., Costa, B. L. S. A., and Guedes, R. C. A. (2012). Ovariectomy in the developing rat decelerates cortical spreading depression in adult brain. Int. J. Dev. Neuroci. 30, 405–410. doi: 10.1016/j.ijdevneu.2012.01.012
Accioly, N. E., and Guedes, R. C. A. (2017). Neonatal treatment with ovarian hormones and suckling among distinct litter sizes: differential effects on recognition memory and spreading depression at adulthood. Nutr. Neurosci. 22, 174–184. doi: 10.1080/1028415X.2017.1358472
Amâncio-dos-Santos, A., Pinheiro, P. C. F., Lima, D. C. F., Ozias, M. G., Oliveira, M. B., Guimarães, N. X., et al. (2006). Fluoxetine inhibits cortical spreading depression in weaned and adult rats suckled under favorable and unfavorable lactation conditions. Exp. Neurol. 200, 275–282. doi: 10.1016/j.expneurol.2006.02.014
Amaral, A. P., Barbosa, M. S., Souza, V. C., Ramos, I. L., and Guedes, R. C. A. (2009). Drug/nutrition interaction in the developing brain: dipyrone enhances spreading depression in rats. Exp. Neurol. 219, 492–498. doi: 10.1016/j.expneurol.2009.06.017
Areiza-Mazo, N., Robles, J., Zamudio-Rodriguez, J. A., Giraldez, L., Echeverria, V., Barrera-Bailon, B., et al. (2018). Extracts of Physalis peruviana protect astrocytic cells under oxidative stress with rotenone. Front. Chem. 6:276. doi: 10.3389/fchem.2018.00276
Becker, A., Felgentreff, F., Schröder, H., Meier, B., and Brattström, A. (2014). The anxiolytic effects of a Valerian extract is based on Valerenic acid. Complement. Alternat. Medicine 2014:267. doi: 10.1186/1472-6882-14-267
Betti, M., Minelli, A., Ambrogini, P., Ciuffoli, S., Viola, V., Galli, F., et al. (2011). Dietary supplementation with α-tocopherol reduces neuroinflammation and neuronal degeneration in the rat brain after kainic acid-induced status epilepticus. Free Radic. Res. 45, 1136–1142. doi: 10.3109/10715762.2011.597750
Bolson, D., and Steinhäuser, C. (2018). Epilepsy and astrocyte energy metabolism. Glia. 66, 1235–1243. doi: 10.1002/glia.23247
Cabezas, R., Baez-Jurado, E., Hidalgo-Lanussa, O., Echeverria, V., Ashrad, G. M., Sahebkar, A., et al. (2019). Growth factors and neuroglobin in astrocyte protection against neurodegeneration and oxidative stress. Molec. Neurobiol. 56, 2339–2351. doi: 10.1007/s12035-018-1203-9
Cabezas, R., Fidel Avila, Gonzalez, J., El-Bacha, R. S., and Barreto, G. E. (2015). PDGF-BB protects mitochondria from rotenone in T98G cells. Neurotox. Res. 27, 355–367. doi: 10.1007/s12640-014-9509-5
Chan, F., Lax, N. Z., Voss, C. M., Aldana, B. I., Whyte, S., Jenkins, A., et al. (2019). The role of astrocytes in seizure generation: insights from a novel in vitro seizure model based on mitochondrial dysfunction. Brain 142, 391–411. doi: 10.1093/brain/awy320
Chateauvieux, S., Morceau, F., Dicato, M., and Diederich, M. (2010). Molecular and therapeutic potential and toxicity of valproic acid. J. Biomed. Biotechnol. 2010:479364. doi: 10.1155/2010/479364
Chen, L., Ding, Y., Cagniard, B., Van Laar, A. D., Mortimer, A., Chi, W., et al. (2008). Unregulated cytosolic dopamine causes neurodegeneration associated with oxidative stress in mice. J. Neurosci. 28, 425–433. doi: 10.1523/jneurosci.3602-07.2008
Coimbra-Costa, D., Alva, N., Duran, M., Carbonell, T., and Rama, R. (2017). Oxidative stress and apoptosis after acute respiratory hypoxia and reoxygenation in rat brain. Redox Biol. 12, 216–225. doi: 10.1016/j.redox.2017.02.014
de Rus Jacquet, A., Tambe, M. A., Ma, S. Y., McCabe, G. P., Vest, J. H. C., and Rochet, J.-C. (2017). Pikuni-Blackfeet traditional medicine: neuroprotective activities of medicinal plants used to treat Parkinson’s disease-related symptoms. J. Ethnopharmacol. 206, 393–407. doi: 10.1016/j.jep.2017.01.001
Devinsky, O., Vezzani, A., Najjar, S., Lanerolle, N. C., and Rogawski, M. A. (2012). Glia and epilepsy: excitability and inflammation. Trends Neurosci. 36, 174–184. doi: 10.1016/j.tins.2012.11.008
Dey, A., Bhattacharya, R., Mukherjee, A., and Pandey, D. K. (2017). Natural products against Alzheimer’s disease: pharmaco-therapeutics and biotechnological interventions. Biotechnol. Adv. 35, 178–216. doi: 10.1016/j.biotechadv.2016.12.005
Dodiya, H. B., Forsyth, C. B., Voigt, R. M., Engen, P. A., Patel, J., Shaikh, M., et al. (2018). Chronic stress-induced gut dysfunction exacerbates Parkinson’s disease phenotype and pathology in a rotenone-induced mouse model of Parkinson’s disease. Neurobiol. Dis. 135:104352. doi: 10.1016/j.nbd.2018.12.012
Doretto, M. C., Garcia-Cairasco, N., Pimenta, N. J., Souza, D. A., and Tatsuo, M. A. (1998). Dipyrone, a novel anticonvulsant agent? Insights from three experimental epilepsy models. Neuroreport 9, 2415–2421. doi: 10.1097/00001756-199807130-00048
Dreier, J. P., Major, S., Lemale, C. L., Kola, V., Reiffurth, C., Schoknecht, K., et al. (2019). Correlates of spreading depolarization, spreading depression, and negative ultraslow potential in epidural versus subdural electrocorticography. Front. Neurosci. 13:373. doi: 10.3389/fnins.2019.00373
Eadie, M. J. (2004). Could valerian have been the first anticonvulsant? Epilepsia 45, 1338–1343. doi: 10.1111/j.0013-9580.2004.27904.x
El-Bachá, R. S., Lima-Filho, J. L., and Guedes, R. C. A. (1998). Dietary antioxidant deficiency facilitates cortical spreading depression induced by photo-activated riboflavin. Nutr. Neurosci. 1, 205–212. doi: 10.1080/1028415x.1998.11747230
Forni, C., Facchiano, F., Bartoli, M., Pieretti, S., Facchiano, A., D’Arcangelo, D., et al. (2019). Beneficial role of phytochemicals on oxidative stress and age-related diseases. BioMed Res. Int. 2019, 1–16. doi: 10.1155/2019/8748253
Gondim-Silva, K. R., da-Silva, J. M., de-Souza, L. A. V., and Guedes, R. C. A. (2019). Neonatal pyridoxine administration long lastingly accelerates cortical spreading depression in male rats, without affecting anxiety-like behavior. Nutr. Neurosci. doi: 10.1080/1028415X.2019.1632570 [Epub ahead of print].
Gorji, A. (2001). Spreading depression: a review of the clinical relevance. Brain Res. Rev. 38, 33–60. doi: 10.1016/s0165-0173(01)00081-9
Gorji, A., and Khaleghi Ghadiri, M. (2001). History of epilepsy in medieval iranian medicine. Neurosci. Biobehav. Rev. 25, 455–461. doi: 10.1016/s0149-7634(01)00025-2
Goswami, P., Gupta, S., Joshi, N., Sharma, S., and Singh, S. (2015). Astrocyte activation and neurotoxicity: a study in different rat brain regions and in rat C6 astroglial cells. Environ. Toxicol. Pharmacol. 40, 122–139. doi: 10.1016/j.etap.2015.06.001
Greenamyre, J. T., Betarbet, R., Sherer, T., and Panov, A. (2001). Response: Parkinson’s disease, pesticides and mitochondrial dysfunction. Trends Neurosci. 24:247. doi: 10.1016/s0166-2236(00)01788-4
Grobben, B., De Deyn, P. P., and Slegers, H. (2002). Rat C6 glioma as experimental model system for the study of glioblastoma growth and invasion. Cell Tissue Res. 310, 257–270. doi: 10.1007/s00441-002-0651-7
Guedes, R. C. A., and Abadie-Guedes, R. (2019). Brain aging and electrophysiological signaling: revisiting the spreading depression model. Front. Aging Neurosci. 11:136. doi: 10.3389/fnagi.2019.00136
Guedes, R. C. A., Abadie-Guedes, R., and Bezerra, R. S. (2012). The use of cortical spreading depression for studying the brain actions of antioxidants. Nutr. Neurosci. 15, 111–119. doi: 10.1179/1476830511y.0000000024
Guedes, R. C. A., Cabral-Filho, J. E., and Teodósio, N. R. (1992). “GABAergic mechanisms involved in cortical spreading depression in normal and malnourished rats,” in Spreading Depression: Experimental Brain Research Series, Vol. 23, ed. R. J. Do Carmo (Berlin: Springer), 17–26. doi: 10.1007/978-3-642-77551-2_3
Guedes, R. C. A., and Cavalheiro, E. A. (1997). Blockade of spreading depression in chronic epileptic rats: reversion by diazepam. Epilepsy Res. 27, 33–40. doi: 10.1016/s0920-1211(96)01017-0
Gupta, R., Milatovic, D., Zivin, M., and Dettbarn, W. D. (2000). Seizure-induced changes in energy metabolites and effects of N-tert-butyl-α-phenylnitrone (PNB) and vitamin E in rats. Pflugers. Arch. 440, R160–R162.
Hansen, M. B., Nielsen, S. E., and Berg, K. (1989). Re-examination and further development of a precise and rapid dye method for measuring cell growth/cell kill. J. Immunol. Methods 119, 203–210. doi: 10.1016/0022-1759(89)90397-9
Hiller, K. O., and Zetler, G. (1996). Neuropharmacological studies on ethanol extracts of Valeriana officinalis L.: behavioural and anticonvulsant properties. Phytother. Res. 51, 505–512.
Ilesanmi, O. B., Akinmoladun, A. C., Josiah, S. S., Olaleye, M. T., and Akindahunsi, A. A. (2019). Modulation of key enzymes linked to Parkinsonism and neurologic disorders by Antiaris africana in rotenone-toxified rats. J. Basic Clin. Physiol. Pharmacol. 31:20190014. doi: 10.1515/jbcpp-2019-0014
Ilhan, A., Iraz, M., Kamisli, S., and Yigitoglu, R. (2006). Pentyleneterazol-induced kindling seizure attenuated by Ginko biloba extract (Egb 761) in mice. Prog. NeuroPsychopharmacol. Biol. Psychiatry 30, 1504–1510. doi: 10.1016/j.pnpbp.2006.05.013
Jamebozorgi, K., Taghizadeh, E., Rostami, D., Pormasoumi, H., Barreto, G. E., Hayat, S. M. G., et al. (2019). Cellular and molecular aspects of parkinson treatment: future therapeutic perspectives. Mol. Neurobiol. 56, 4799–4811. doi: 10.1007/s12035-018-1419-8
Jantas, D., Roman, A., Kuśmierczyk, J., Lorenc-Koci, E., Konieczny, J., Lenda, T., et al. (2013). The extent of neurodegeneration and neuroprotection in two chemical in vitro models related to Parkinson’s disease is critically dependent on cell culture conditions. Neurotox. Res. 24, 41–54. doi: 10.1007/s12640-012-9374-z
Jenner, P. (2001). Parkinson’s disease, pesticides and mitochondrial dysfunction. Trends Neurosci. 24, 245–247.
Khan, M. M., Kempuraj, D., Zaheer, S., and Zaheer, A. (2014). Glia maturation factor deficiency suppresses 1-methyl-4-phenylpyridinium-induced oxidative stress in astrocytes. J. Molec. Neurosci. 53, 590–599. doi: 10.1007/s12031-013-0225-z
Koroleva, V. I., and Bures, J. (1980). Blockade of cortical spreading depression in electrically and chemically stimulated areas of cerebral cortex in rats. Electroencephalogr. Clin. Neurophysiol. 48, 1–15. doi: 10.1016/0013-4694(80)90038-3
Krylova, N. G., Drobysh, M. S., Semenkova, G. N., Kulahava, T. A., Pinchuk, S. V., and Shadyro, O. I. (2019). Cytotoxic and antiproliferative efects of thymoquinone on rat C6 glioma cells depend on oxidative stress. Molec. Cell. Biochem. 462, 195–206. doi: 10.1007/s11010-019-03622-8
Leão, A. A. P. (1944). Spreading depression of activity in the cerebral cortex. J. Neurophysiol. 7, 359–390. doi: 10.1152/jn.1944.7.6.359
Leão, A. A. P. (1947). Further observations on the spreading depression of activity in the cerebral cortex. J. Neurophysiol. 10, 409–414. doi: 10.1152/jn.1947.10.6.409
Leão, A. A. P. (1972). “Spreading depression,” in Experimental Models of Epilepsy, eds D. P. Purpura, K. Penry, D. B. Tower, D. M. Woodbury, and R. D. Walter (New York, NY: Raven Press), 173–195.
Lian, X. Y., and Stringer, J. L. (2004). Energy failure in astrocytes increases the vulnerability of neurons to spreading depression. Eur. J. Neurosci. 19, 2446–2454. doi: 10.1111/j.0953-816x.2004.03289.x
Lima, C. B., Soares, G. S. F., Vitor, S. M., Andrade-da-Costa, B. L. S., Castellano, B., and Guedes, R. C. A. (2014). Spreading depression features and Iba1 immunoreactivity in the cerebral cortex of developing rats submitted to treadmill exercise after treatment with monosodium glutamate. Int. J. Devel. Neurosci. 33, 98–105. doi: 10.1016/j.ijdevneu.2013.12.008
Lima, R. M. F., Alvarez, L. D. G., Costa, M. F. D., Costa, S. L., Clarencio, J., and El-Bacha, R. S. (2008). Cytotoxic effects of catechol to neuroblastoma N2a cells. Gen. Physiol. Biophys. 27, 306–314.
Mendes-da-Silva, R. F., Cunha-Lopes, A. A., Bandim-da-Silva, M. E., Cavalcanti, G. A., Rodrigues, A. R. O., Andrade-da-Costa, B. L. S., et al. (2014). Prooxidant versus antioxidant brain action of ascorbic acid in well-nourished and malnourished rats as a function of dose: a cortical spreading depression and malondialdehyde analysis. Neuropharmacology 86, 155–160. doi: 10.1016/j.neuropharm.2014.06.027
Mendes-da-Silva, R. F., Francisco, E. S., and Guedes, R. C. A. (2018). Pilocarpine/ascorbic acid interaction in the immature brain: electrophysiological and oxidative effects in well-nourished and malnourished rats. Brain Res. Bull. 142, 414–421. doi: 10.1016/j.brainresbull.2018.09.008
Mennini, T., Bernasconi, T., and Morazzoni, P. (1993). In vitro study on the interaction of extracts and pure compounds from Valeriana officinalis roots with GABA, benzodiazepine and barbiturate receptors in rat brain. Fitoterapia 64, 291–300.
Miller, J. A., Runkle, S. A., Tjalkens, R. B., and Philbert, M. A. (2011). 1,3-Dinitrobenzene-induced metabolic impairment through selective inactivation of the pyruvate dehydrogenase complex. Toxicol. Sci. 122, 502–511. doi: 10.1093/toxsci/kfr102
Mineo, L., Concerto, C., Patel, D., Mayorga, T., Paula, M., Chusid, E., et al. (2017). Valeriana officinalis root extract modulates cortical excitatory circuits in humans. Neuropsychobiology 75, 46–51. doi: 10.1159/000480053
Moeini, R., Memariani, Z., Asadi, F., Bozorgi, M., and Gorji, N. (2019). Pistacia genus as a potential source of neuroprotective natural products. Planta Med. 85, 1326–1350. doi: 10.1055/a-1014-1075
Nagatsu, T., and Sawada, M. (2005). Inflammatory process in parkinsons disease: role for cytokines. Curr. Pharmaceut. Design 11, 999–1016. doi: 10.2174/1381612053381620
Nicholls, D. G., and Budd, S. L. (2000). Mitochondria and neuronal survival. Physiol. Rev. 80, 315–360. doi: 10.1152/physrev.2000.80.1.315
Oliveira, D. M., Barreto, G., Andrade, D. V. G., Saraceno, E., Aon-Bertolino, L., Capani, F., et al. (2009). Cytoprotective effect of Valeriana officinalis extract on an in vitro experimental model of parkinson disease. Neurochem. Res. 34, 215–220. doi: 10.1007/s11064-008-9749-y
Pamies, D., Block, K., Lau, P., Gribaldo, L., Pardo, C. A., Barreras, P., et al. (2018). Rotenone exerts developmental neurotoxicity in a human brain spheroid model. Toxicol. Appl. Pharmacol. 354, 101–114. doi: 10.1016/j.taap.2018.02.003
Perez-Hernandez, J., Zaldivar-Machorro, V. J., Villanueva-Porras, D., Vega-Avila, E., and Chavarria, A. (2016). A potential alternative against neurodegenerative diseases: phytodrugs. Oxidat. Med. Cell. Longevity 2016, 19. doi: 10.1155/2016/8378613
Perez-Pardo, P., Dodiya, H. B., Broersen, L. M., Douna, H., van Wijk, N., Lopes da Silva, S., et al. (2018). Gut–brain and brain–gut axis in Parkinson’s disease models: effects of a uridine and fish oil diet. Nutr. Neurosci. 21, 391–402. doi: 10.1080/1028415X.2017.1294555
Pestana, R. R. F., Kinjo, E. R., Hernandes, M. S., and Britto, L. R. G. (2010). Reactive oxygen species generated by NADPH oxidase are involved in neurodegeneration in the pilocarpine model of temporal lobe epilepsy. Neurosci. Lett. 484, 187–191. doi: 10.1016/j.neulet.2010.08.049
Rathinam, M. L., Watts, L. T., Narasimhan, M., Riar, A. K., Mahimainathan, L., and Henderson, G. I. (2012). Astrocyte mediated protection of fetal cerebral cortical neurons from rotenone and paraquat. Environ. Toxicol. Pharmacol. 33, 353–360. doi: 10.1016/j.etap.2011.12.027
Rezvani, M. E., Roohbakhsh, A., Allahtavakoli, M., and Shamsizadeh, A. (2010). Anticonvulsant effect of aqueous extract of Valeriana officinalis in amygdale-kindled rats: possible involvement of adenosine. J. Ethnopharmacol. 127, 313–318. doi: 10.1016/j.jep.2009.11.002
Ríos, J.-L., Onteniente, M., Picazo, D., and Montesinos, M.-C. (2016). Medicinal plants and natural products as potential sources for antiparkinson drugs. Planta Med. 82, 942–951. doi: 10.1055/s-0042-107081
Roohbakhsh, A., Parhiz, H., Soltani, F., Rezaee, R., and Iranshahi, M. (2014). Neuropharmacological properties and pharmacokinetics of the citrus flavonoids hesperidin and hesperetin — A mini-review. Life Sci. 113, 1–6. doi: 10.1016/j.lfs.2014.07.029
Selvakumar, G. P., Iyer, S. S., Kempuraj, D., Raju, M., Thangavel, R., Saeed, D., et al. (2018). Glia maturation factor dependent inhibition of mitochondrial PGC-1α triggers oxidative stress-mediated apoptosis in N27 rat dopaminergic neuronal cells. Mol. Neurobiol. 55, 7132–7152. doi: 10.1007/s12035-018-0882-6
Sudati, J. H., Fachinetto, R., Pereira, R. P., Boligon, A. A., Athayde, M. L., Soares, F. A., et al. (2009). In vitro antioxidant activity of Valeriana officinalis against different neurotoxic agents. Neurochem. Res. 34, 1372–1379. doi: 10.1007/s11064-009-9917-8
Sudati, J. H., Vieira, F. A., Pavin, S. S., Dias, G. R. M., Seeger, R. L., Golombieski, R., et al. (2013). Valeriana officinalis attenuates the rotenone-induced toxicity in Drosophila melanogaster. NeuroToxicology 37, 118–126. doi: 10.1016/j.neuro.2013.04.006
Sugaya, E., Takato, M., and Noda, Y. (1975). Neuronal and glial activity during spreading depression in cerebral cortex of cat. J. Neurophysiol. 38, 822–841. doi: 10.1152/jn.1975.38.4.822
Swarnkar, S., Goswami, P., Kamat, P. K., Patro, I. K., Singh, S., and Nath, C. (2013). Rotenone-induced neurotoxicity in rat brain areas: a study on neuronal and neuronal supportive cells. Neuroscience 230, 172–183. doi: 10.1016/j.neuroscience.2012.10.034
Swarnkar, S., Goswami, P., Kamat, P. K., Gupta, S., Patro, I. K., Singh, S., et al. (2012a). Rotenone-induced apoptosis and role of calcium: a study on Neuro-2a cells. Arch. Toxicol. 86, 1387–1397. doi: 10.1007/s00204-012-0853-z
Swarnkar, S., Singh, S., Goswami, P., Mathur, R., Patro, I. K., and Nath, C. (2012b). Astrocyte activation: a key step in rotenone induced cytotoxicity and DNA damage. Neurochem. Res. 37, 2178–2189. doi: 10.1007/s11064-012-0841-y
Talpade, D. J., Greene, J. G., Higgins, D. S. Jr., and Greenamyre, J. T. (2000). In vivo labeling of mitochondrial complex I (NADH:ubiquinone oxidoreductase) in rat brain using [(3)H] dihydrorotenone. J. Neurochem. 75, 2611–2621. doi: 10.1046/j.1471-4159.2000.0752611.x
Tewari, D., Stankiewicz, A. M., Mocan, A., Sah, A. N., Tzvetkov, N. T., Huminiecki, L., et al. (2018). Ethnopharmacological approaches for dementia therapy and significance of natural products and herbal drugs. Front. Aging Neurosci. 10:3. doi: 10.3389/fnagi.2018.00003
Thiffault, C., Langston, J. W., and Di Monte, D. A. (2000). Increased striatal dopamine turnover following acute administration of rotenone to mice. Brain Res. 885, 283–288. doi: 10.1016/s0006-8993(00)02960-7
Torrente, D., Mendes-da-Silva, R. F., Lopes, A. A. C., González, J., Barreto, G., and Guedes, R. C. A. (2014). Increased calcium influx triggers and accelerates cortical spreading depression in vivo in male adult rats. Neurosci. Lett. 558, 87–90. doi: 10.1016/j.neulet.2013.11.004
Torres-Hernández, B. A., Del Valle-Mojica, L. M., and Ortíz, J. G. (2015). Valerenic acid and Valeriana officinalis extracts delay onset of Pentylenetetrazole (PTZ)-Induced seizures in adult Danio rerio (Zebrafish). Complement. Alternat. Med. 15:228.
Tuszkiewicz-Misztal, E., Opoka-Winiarska, V., and Postêpski, J. (2000). Significance of dietary antioxidants for child proper development and health. Pediatr. Pol. 75, 359–366.
Wang, X., Qin, Z. H., Leng, Y., Wang, Y., Jin, X., Chase, T. N., et al. (2002). Prostaglandin A1 inhibits rotenone-induced apoptosis in SH-SY5Y cells. J. Neurochem. 83, 1094–1102. doi: 10.1046/j.1471-4159.2002.01224.x
Wetherington, J., Serrano, G., and Dingledine, R. (2008). Astrocytes in the epileptic brain. Neuron 58, 168–178. doi: 10.1016/j.neuron.2008.04.002
Wu, A., Ye, X., Huang, Q., Dai, W. M., and Zhang, J. M. (2017). Anti-epileptic effects of valepotriate isolated from valeriana jatamansi jones and its possible mechanisms. Pharmacogn. Mag. 13, 512–516. doi: 10.4103/0973-1296.211027
Keywords: rat, Valeriana officinalis, cortical spreading depression, rotenone, brain excitability, toxic effects, astrocyte
Citation: Amaral de Brito AP, Galvão de Melo IMS, El-Bachá RS and Guedes RCA (2020) Valeriana officinalis Counteracts Rotenone Effects on Spreading Depression in the Rat Brain in vivo and Protects Against Rotenone Cytotoxicity Toward Rat Glioma C6 Cells in vitro. Front. Neurosci. 14:759. doi: 10.3389/fnins.2020.00759
Received: 26 March 2020; Accepted: 29 June 2020;
Published: 23 July 2020.
Edited by:
Jacob Raber, Oregon Health & Science University, United StatesReviewed by:
Charles K. Meshul, Oregon Health & Science University, United StatesSantiago J. Ballaz, Yachay Tech University, Ecuador
Copyright © 2020 Amaral de Brito, Galvão de Melo, El-Bachá and Guedes. This is an open-access article distributed under the terms of the Creative Commons Attribution License (CC BY). The use, distribution or reproduction in other forums is permitted, provided the original author(s) and the copyright owner(s) are credited and that the original publication in this journal is cited, in accordance with accepted academic practice. No use, distribution or reproduction is permitted which does not comply with these terms.
*Correspondence: Rubem Carlos Araújo Guedes, guedes.rca@gmail.com; rguedes@ufpe.br
†Present address: Ana Paula Amaral de Brito, Universidade do Estado da Bahia – UNEB, Salvador, Brazil