- 1Department of Neuroscience, Psychology, Drug Research and Child Health (NEUROFARBA), Section of Pharmacology and Toxicology, University of Florence, Florence, Italy
- 2Department of Experimental and Clinical Biomedical Sciences, University of Florence, Florence, Italy
Oligodendrocyte-formed myelin sheaths allow fast synaptic transmission in the brain. Impairments in the process of myelination, or demyelinating insults, might cause chronic diseases such as multiple sclerosis (MS). Under physiological conditions, remyelination is an ongoing process throughout adult life consisting in the differentiation of oligodendrocyte progenitor cells (OPCs) into mature oligodendrocytes (OLs). During pathological events, this process fails due to unfavorable environment. Adenosine and sphingosine kinase/sphingosine 1-phosphate signaling axes (SphK/S1P) play important roles in remyelination processes. Remarkably, fingolimod (FTY720), a sphingosine analog recently approved for MS treatment, plays important roles in OPC maturation. We recently demonstrated that the selective stimulation of A2B adenosine receptors (A2BRs) inhibit OPC differentiation in vitro and reduce voltage-dependent outward K+ currents (IK) necessary to OPC maturation, whereas specific SphK1 or SphK2 inhibition exerts the opposite effect. During OPC differentiation A2BR expression increases, this effect being prevented by SphK1/2 blockade. Furthermore, selective silencing of A2BR in OPC cultures prompts maturation and, intriguingly, enhances the expression of S1P lyase, the enzyme responsible for irreversible S1P catabolism. Finally, the existence of an interplay between SphK1/S1P pathway and A2BRs in OPCs was confirmed since acute stimulation of A2BRs activates SphK1 by increasing its phosphorylation. Here the role of A2BR and SphK/S1P signaling during oligodendrogenesis is reviewed in detail, with the purpose to shed new light on the interaction between A2BRs and S1P signaling, as eventual innovative targets for the treatment of demyelinating disorders.
Introduction
In the central nervous system (CNS), oligodendrocytes (OLs) are responsible for myelin production, which allows fast signal transmission and provides metabolic support to axons (Nave, 2010; Saab et al., 2016). During development, OLs are generated in the germinal zones of the brain, i.e., the subventricular zone (SVZ) (Yu et al., 1994), from migratory bipolar oligodendrocyte precursor cells (OPCs), which are renowned for the expression of the proteoglycan nerve-glial antigen 2 (NG2) (Grinspan, 2002; Brazel et al., 2004). Thanks to their migratory ability, OPCs spread and populate the embryonic brain and spinal cord (Emery, 2010) to differentiate into myelinating OLs. However, a pool of immature OPCs, comprising the 5-8% of total glial cells (Levine et al., 2001), persists within the adult CNS where they represent the major population of cycling cells (Dawson et al., 2003). This process guarantees, under physiological conditions, myelin turnover and remodeling in response to life experience (Malerba et al., 2015; Bergles and Richardson, 2016) and, in conditions of tissue damage, the generation of new OLs able to remyelinate the brain after a lesion or injury, an ability that might be lost through normal aging or chronic diseases. However, under pathological conditions such those characterized by chronic neuroinflammation and neurodegeneration, this process fails, leading to improper nerve conduction due to discontinuities in the myelin sheet (Nave, 2010; Franklin and Ffrench-Constant, 2017). For this reason, it is of critical relevance to identify innovative targets able to encourage OPC differentiation toward the mature, myelin-producing OL phenotype.
Among the factors influencing oligodendrogliogenesis, interest has been focused in the last years on the neuromodulator adenosine (Coppi et al., 2021), on one hand, and on the pleiotropic signaling molecule sphingosine 1-phosphate (S1P).
This review will focus on S1P and adenosine receptor-mediated effects in oligodendrocyte progenitors relevant for oligodendrogenesis and their possible functional interplay with the purpose to deepen the knowledge of molecular mechanisms involved in A2B receptor- (A2BR-) or S1P-mediated effects and their cross-talk in oligodendrocyte biology.
Oligodendrocyte Differentiation
Before being able to produce myelin, oligodendroglial cells progress through a series of highly regulated steps of differentiation from OPCs to mature OLs (de Castro and Bribian, 2005; Barateiro and Fernandes, 2014). This process is characterized by the loss of proliferative and migratory activity and the acquisition of an elaborate and highly ramified morphology (de Castro and Bribian, 2005). Oligodendrogliogenesis implicates a sequence of distinct maturational stages each of them identified by distinct morphological changes and by the expression of specific antigens (Levi et al., 1986; Gard and Pfeiffer, 1990; Warrington et al., 1992; Jung et al., 1996). On these bases, three major stages of differentiation have been proposed: a proliferating, bipolar OPC phase, characterized by the expression of platelet-derived growth factor (PDGF) receptor alpha (PDGFα), NG2 and the transcription factor Olig2 (Pringle et al., 1992; Ligon et al., 2006), a post-mitotic, moderately ramified pre-oligodendrocyte phase (pre-OL), positive for the markers O4 (Szuchet et al., 2011) and the recently deorphanized (Ciana et al., 2006) P2Y-like GPR17 receptor (Lecca et al., 2008; Emery, 2010; Fumagalli et al., 2011; Coppi et al., 2013b), and a mature myelinating OL phase, characterized by a highly ramified morphology and by the expression of galactocerebroside (GC), a specific marker for the oligodendrocyte membrane, myelin specific structural proteins such as 2’,3’-Cyclic-nucleotide-3’-phosphodiesterase (CNPase), myelin associated glycoprotein (MAG) and myelin basic protein (MBP) (Scolding et al., 1989; Zhang, 2001; Szuchet et al., 2011; Coppi et al., 2013a, 2015). Mature OLs synthesize large amounts of myelin, giving rise to multilamellar myelin sheaths that wrap and insulate neuronal axons which allow electrical isolation and saltatory conduction of electric impulses.
It is known that, during their maturation, oligodendroglial cells display functional voltage-gated ion channels whose expression changes during differentiation (Sontheimer et al., 1989; Barres et al., 1990; Williamson et al., 1997; Spitzer et al., 2019) including either inward or outward rectifying K+ channels (Kir and Kv, respectively), Na+ channels (Nav) and different subtypes of Ca2+ channels (Cav) (Verkhratsky et al., 1990).
During mouse brain development, the first ion channel subtype detected in OPCs is the Kv voltage-dependent, outwardly rectifying K+ conductance, at embryonic day 18 (E18) (Spitzer et al., 2019). However, a fraction of OPCs are described to express also NaV currents that sharply increase around birth. This OPC population, with high NaV and KV densities, reflect a proliferative and migratory state during myelin generation (Gautier et al., 2015; Spitzer et al., 2019). Intriguingly, not all OPCs express NaV, but only a fraction, described to be around 60% (Kettenmann et al., 1991). Of note, a subpopulation of electrically excitable, spiking, NG2+ OPCs, able to generate full action potentials when stimulated by depolarizing current injection, have been described in brain slices (Karadottir et al., 2008), but the functional role of this “electrically excitable” OPC subpopulation is still unknown. Of note, single action potentials have also been detected in a minority of cultured OPCs (Barres et al., 1990).
The functional role of action potentials (APs) in OPC is still a matter of debate since, up to now, no functional differences have been detected between firing and not-firing OPCs (Bonetto et al., 2020). As long as OPCs undergo functional maturation, the density of Nav currents decreases as well as the expression of outward Kv conductances. In this phase, OPC differentiation potential declines and thus it can be considered a “quiescent” OPC state.
Among Kv currents, OPCs show outward conductances mainly composed by tetraetylammonium (TEA)-sensitive, delayed rectifying K+ currents (IK) (Sontheimer and Kettenmann, 1988) characterized by scarce time- and voltage-dependent inactivation and by a threshold for activation around -40 mV (Gutman et al., 2005). They also express a transient outward K+ current, or IA (Gallo et al., 1996; Coppi et al., 2013b; Coppi et al., 2015), characterized by a rapid time-dependent inactivation (approximately 50 ms) and a voltage-dependent inactivation at potentials above -80 mV.
During maturation, outward K+ conductances (both IK and IA), as well as INa, undergo a strong downregulation up to almost completely disappearance in mature OLs (Sontheimer and Kettenmann, 1988; Sontheimer et al., 1989; Barres et al., 1990; Coppi et al., 2013a). Concomitantly, a gradual increase in the expression of inwardly rectifying K+ currents (Kir), activated at potentials lower than −100 mV, appears. Indeed, Kir currents are the main conductance observed in mature OLs (Knutson et al., 1997). Among the mentioned currents, TEA-sensitive IK are crucially linked to cell cycle regulation and hence to myelin formation (Chittajallu et al., 2005), as demonstrated by the fact that when OPC cultures are grown in the presence of TEA, a significant inhibition of their proliferation and differentiation is observed (Gallo et al., 1996; Knutson et al., 1997; Chittajallu et al., 2005; Coppi et al., 2013b). Hence, compounds that modulate these currents may affect oligodendrocyte proliferation and myelination, as well as neurotransmitters, cytokines and growth factors acting on specific metabotropic receptors described to modify K+ current expression in OPCs (Stellwagen and Malenka, 2006; Zonouzi et al., 2011; Lundgaard et al., 2013; Malerba et al., 2015; Spitzer et al., 2019).
Adenosine as a Neuromodulator
Adenosine is an intermediary metabolite acting as a building molecule for nucleic acids and a component of the biological energy currency ATP. In addition, adenosine is one of the most evolutionarily ancient signaling molecules (Verkhratsky and Burnstock, 2014), acting through the stimulation of four distinct adenosine-sensitive metabotropic P1 receptors denoted as A1, A2A, A2B and A3 adenosine receptors (A1Rs, A2ARs, A2BRs and A3Rs) (Fredholm et al., 2011). These receptors are widely expressed both in the periphery or in the central nervous system (CNS) and have been implicated in a myriad of biological functions (Pedata et al., 2007; Coppi et al., 2012).
High extracellular concentrations of the nucleoside adenosine are found under conditions of tissue damage or when an imbalance in oxygen supply occurs (Latini et al., 1999; Pedata et al., 2014). Adenosine is short-lived in the extracellular space due to enzymatic degradation by adenosine deaminase (ADA) or adenosine kinase (AK) (Chen et al., 2013) or re-uptake operated by the equilibrative nucleoside transporters (ENT) isoforms ENT1 and ENT2 (Inoue, 2017), so its effects in the CNS are mainly described as autocrine and/or paracrine.
Among the four different P1 receptor subtypes, different affinities have been shown for the endogenous ligand. The activation of A1Rs is achieved as long as extracellular adenosine falls in the low nanomolar range (1-10 nM), a concentration generally present in most tissues and organs throughout the body (Latini and Pedata, 2001). A1R-mediated signal activates Gi/o proteins leading to the inhibition of adenylyl cyclase (AC) and to a decrease in intracellular cAMP levels (Antonioli et al., 2019). A1Rs are the predominant P1 receptor subtype in the CNS, with high levels of expression reported in the cerebral cortex, hippocampus, cerebellum, thalamus, brainstem and spinal cord. It is well known that A1R activation inhibits synaptic transmission (Corradetti et al., 1984; Dunwiddie, 1984) leading to sedative, anticonvulsant (Muzzi et al., 2013) and anxiolytic (Vincenzi et al., 2016) effects in the CNS whereas, at cardiovascular levels, they are potent bradycardic agents (Jacobson and Gao, 2006).
The A2AR subtype is known to stimulate AC by Gs protein activation, leading to the production of cAMP which acts as a second messenger by activating protein kinase A (PKA) (Antonioli et al., 2019). Within the brain, this receptor subtype is widely expressed with particularly high levels found in the striatum/caudate-putamen nuclei (Peterfreund et al., 1996). In the periphery, its expression is abundant on blood vessels and inflammatory/immune cells (Yu L. Q. et al., 2004). The functional effect of A2ARs in the brain is at variance from A1R subtype, as they enhance glutamate release and promote cell excitability (Goncalves and Ribeiro, 1996; Lopes et al., 2002). In accordance, A2AR activation participates to excitotoxic damage due to extracellular glutamate overload during an ischemic-like insult obtained in vitro by oxygen and glucose deprivation (OGD) (Colotta et al., 2012; Maraula et al., 2013; Maraula et al., 2014). Concerning peripheral functions of A2ARs, it is worth to note that adenosine, thanks to its actions on this receptor subtype, is one of the most powerful endogenous anti-inflammatory agents (Antonioli et al., 2019). Indeed, A2ARs are highly expressed in inflammatory cells including lymphocytes, granulocytes and monocytes/macrophages, where their activation reduces pro-inflammatory cytokines, i.e., tumor necrosis factor-alpha (TNFα), interleukin-1β (IL-1 β) and interleukin-6 (IL-6) (Varani et al., 2011) and enhances the release of anti-inflammatory mediators, such as interleukin-10 (IL-10) (Bortoluzzi et al., 2016).
The A2BR subtype is somewhat the most enigmatic and less studied among the four P1 receptors as its pharmacological and physiological characterization has long been precluded by the lack of suitable ligands able to discriminate among the other adenosine receptor subtypes (Popoli and Pepponi, 2012; Chandrasekaran et al., 2019). The central distribution of A2BRs on neurons and glia is scarce but widespread, whereas in the periphery abundant levels of A2BRs are observed in bronchial epithelia, smooth muscles, inflammatory cells such as mast, neutrophils and monocytes, vasculature and digestive tracts like ileum and colon (Feoktistov et al., 1998; Chandrasekaran et al., 2019). It is reported that A2BR activation stimulates Gs and, in some cases, also Gq/11 proteins, thus activating either or both cAMP-pathway and IP3- related pathways. In this second case, the activation of phospholipase C (PLC) leads to the production of inositol-(1,4,5)-trisphosphate (IP3) and diacylglycerol (DAG), that increases the intracellular levels of Ca2+ and activates protein kinase C (PKC), respectively (Antonioli et al., 2019).
Differently from high affinity A1Rs and A2ARs, activated by physiological levels of extracellular adenosine (low nM and high nM, respectively (Coppi et al., 2020b), the A2BR needs much higher adenosine concentrations (in the μM range) reached only in conditions of tissue damage or injury (Chandrasekaran et al., 2019). Such a low affinity of A2BRs for the endogenous agonist implies that they represent a good therapeutic target, since they are activated only by high adenosine efflux reached under pathological conditions or injury, when a massive release of adenosine occurs (Popoli and Pepponi, 2012) or that they can be driven to function by selective agonists (Coppi et al., 2020b). All the adenosine receptors mentioned above are also associated with mitogen-activated protein kinase (MAPK) pathways, which involves the activation of extracellular signal-regulated kinase 1 and 2 (ERK1 and ERK2), whose action can mediate numerous cellular responses, and of JUN N-terminal kinase (JNK) and p38 MAPK (Melani et al., 2009). In particular, A1Rs and A2BRs promote the activation of ERK1, ERK2, p38 MPAK and JNK, instead A2ARs and A3Rs are involved only in the stimulation of ERK1 and ERK2 signaling (Antonioli et al., 2013).
As mentioned above for the cognate A2AR subtype, A2BR activation within the CNS is reported to increase glutamate release (Goncalves et al., 2015; Fusco et al., 2019) but the mechanism is at variance from the former. In fact, Cunha and co-workers demonstrated that the A2BR selective agonist BAY60-6583 attenuates the predominant A1R-mediated inhibitory control of synaptic transmission in the CA1 hippocampus (Goncalves et al., 2015). These data are consistent with the relatively abundant expression of A2BRs in hippocampal presynaptic sites, demonstrated by means of synaptosome preparation, reported by the same authors (Goncalves et al., 2015). The facilitatory effect of A2BRs on glutamatergic neurotransmission was confirmed by us in acute hippocampal slices by using the electrophysiological protocol of paired pulse facilitation (PPF) (Fusco et al., 2019). We reported that A2BR activation decreases PPF in the CA1 hippocampus, an effect known to be ascribed to enhanced glutamate release (Zucker and Regehr, 2002). Furthermore, the effect of BAY60-6583 was prevented not only by the A2BR antagonists MRS1754 and PSB-603, but also by the A1R blocker DPCPX (Fusco et al., 2019), confirming the fact that A1R activation in necessary for the enhancing effect of A2BRs on glutamate release. Furthermore, we extended results to a newly synthetized A2BR-selective agonist, the recently described BAY60-6583-analog P453 (Betti et al., 2018) which proved higher affinity for the A2BR (50 nM vs. 200 nM, respectively) than the commercially available BAY 60-6583. In addition, as reported for A2ARs, also A2BR activation participates to OGD-induced synaptic failure in the hippocampus (Fusco et al., 2018).
In the periphery, A2BRs are present on hematic cells, such as lymphocytes and neutrophils, with the highest expression levels found on macrophages (Gessi et al., 2005; Yang et al., 2006). Here, A2BRs in most cases are co-expressed with A2ARs and their activation exert anti-inflammatory effects by inhibiting migration and vascular adhesion (Yang et al., 2006) of inflammatory cells (Wakai et al., 2001; Eckle et al., 2008).
The A3R subtype is known to couple to Gi/o proteins and to inhibit AC but, under particular conditions or in different cell types, activation of Gq/11 by A3R agonists has also been reported (Antonioli et al., 2019). This receptor subtype presents large interspecies differences, with only 74% sequence homology between rat and human (Koscso et al., 2011). Its expression is not uniform throughout the body: low levels are found in the brain and spinal cord whereas a predominance of this receptor subtype is described in peculiar regions at the periphery, i.e., in the testis, lung, kidneys, placenta, heart, brain, spleen and liver (Gessiaa et al., 2008). Interestingly, most of the cell types of the immune system express functional A3Rs on their surface (Hasko et al., 2008) and its activation is one of the most powerful stimuli for mast cells degranulation (Ramkumar et al., 1993).
Adenosine in Oligodendrogliogenesis
Oligodendrocyte turnover is rather slow under physiological conditions and guarantees myelin turnover and remodeling in response to life experience (Bergles and Richardson, 2016). However, a disruption in this process, for example in case of a maturation block, could have devastating consequences during aging and in pathological conditions, such as multiple sclerosis (MS).
Recruitment of OPCs to injured areas is in fact one of the most important events to promote remyelination after CNS injury (Simon et al., 2011; Neumann et al., 2019). Unfortunately, it is still not clear why this process fails, or is insufficient to provide myelin repair, during chronic demyelinating diseases (Romanelli et al., 2016). In fact, most OPCs fail to mature into myelin-producing OLs in MS, indicating that remyelination by adult OPCs is hindered principally due to a failure of OPC differentiation into mature OLs rather than a failure of repopulation or migration of OPCs (Chang et al., 2002; Patel and Klein, 2011).
A deep knowledge of the intricate processes regulating OPC maturation to OLs is mandatory to investigate new therapeutic targets aimed at counteracting demyelinating diseases and repair myelin damage in the long run.
Among the neuromodulators contributing to the balance between proliferating, immature OPCs and myelinating, mature OLs there are purines (Stevens et al., 2002; Fields, 2004; Fields and Burnstock, 2006) and, in particular, adenosine (Coppi et al., 2020b).
All P1 receptors are expressed by maturating oligodendroglial progenitors as well as by mature OLs (Stevens et al., 2002; Coppi et al., 2020a) and exert a key role in cell development (Coppi et al., 2015, 2020b). Furthermore, the expression by oligodendrocytes of the nucleoside transporters ENT1 and ENT2, as well as adenosine degrading enzymes ADA and AK, suggests that these cells are able to sense and finely tune extracellular adenosine levels (Gonzalez-Fernandez et al., 2014), thus supporting the notion that purinergic signaling exerts a prominent role in these cells (Burnstock et al., 2011).
Indeed, our research group contributed to demonstrate that adenosine can affect numerous OPC functions such as migration, proliferation and maturation (Fields and Stevens-Graham, 2002; Stevens et al., 2002; Coppi et al., 2013a,b, 2015; Cherchi et al., 2021), with distinct effects mediated by different receptor subtypes, as summarized in Figure 1.
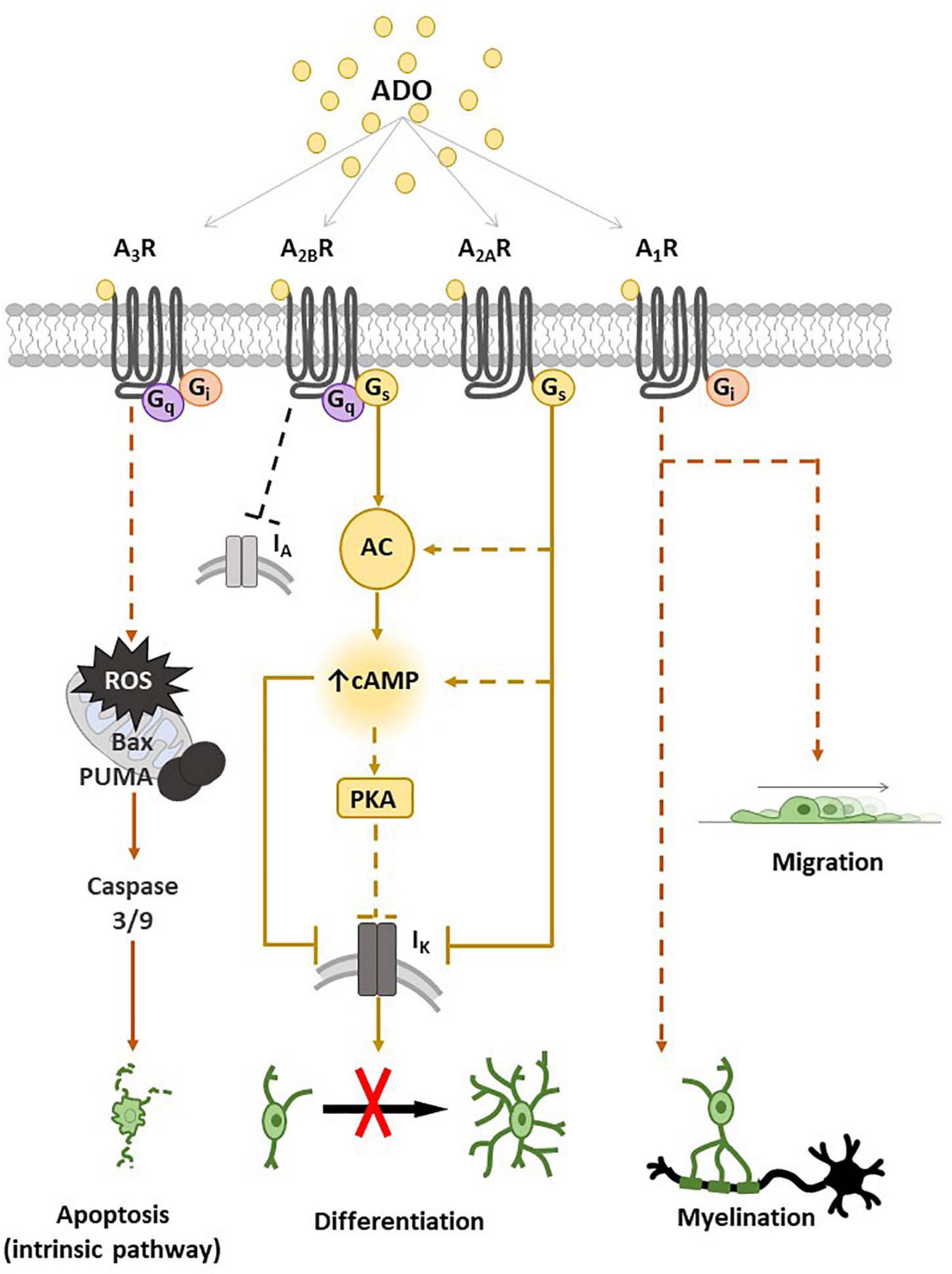
Figure 1. Adenosine receptor expression and main transduction pathways activated in oligodendrocyte progenitor cells (OPCs) and oligodendrocytes (OLs). Schematic representation of A1, A2A, A2B and A3 receptor (A1R, A2AR, A2BR and A3R) effects on OPCs and main intracellular pathways involved. The activation of A1R by adenosine (ADO) or other selective receptor agonists promotes myelination and migration. The stimulation of Gs-coupled A2AR and/or A2BR leads to adenylyl cyclase (AC) activation with a consequent increase in intracellular cyclic adenosine monophosphate (cAMP), which closes IK channels and inhibits OPC differentiation, probably by a mechanism involving protein kinase A (PKA). A3R stimulation induces OPC apoptosis by the activation of an intrinsic pathway, i.e., through reactive oxygen species (ROS) production and activation of Bcl-2-associated X (Bax), p53-upregulated modulator of apoptosis (PUMA) and caspase 3/9. Dotted lines are used when the intracellular pathway/s have not been described.
It has been demonstrated that tonic electrical stimulation of co-cultures of OPCs with dorsal root ganglion neurons induces the release of adenosine that inhibits OPC proliferation and promotes their maturation toward pre-myelinating OLs, an effect blocked by a cocktail of A1R, A2AR and A3R antagonists (Stevens et al., 2002), suggesting that endogenous adenosine released in response to impulse activity promotes oligodendrocyte development and myelination. Furthermore, A1R agonists have been reported to stimulate OPC migration (Figure 1; Othman et al., 2003).
Concerning the A2AR subtype, our group of research demonstrated that the selective A2AR agonist CGS21680 inhibits TEA- sensitive IK currents in cultured OPCs and delays in vitro cell differentiation without affecting neither cell viability nor proliferation (Coppi et al., 2013a). These effects were completely prevented in the presence of the selective A2AR antagonist SCH58261 (Coppi et al., 2013a). In keeping with data demonstrating that TEA impairs OPC maturation (Attali et al., 1997; Gallo et al., 1996; Coppi et al., 2013b) and blocks myelin deposition in the embryonic spinal cord (Shrager and Novakovic, 1995) and ovine OPCs (Soliven et al., 1988), it appears that the Gs-coupled A2AR inhibits OPC differentiation by reducing IK currents. In line with this assumption is the observation that selective activation of GPR17, a Gi-coupled P2Y-like receptor, enhances TEA-sensitive IK and improves OPC differentiation (Coppi et al., 2013b).
The less known adenosine receptor in OPCs is the A3R subtype. The only paper available in the literature demonstrates that the A3R agonist 2-CI-IB-MECA induces apoptosis of cultured oligodendroglial cells isolated from rat optic nerve (Gonzalez-Fernandez et al., 2014) and induces myelin loss in an ex vivo preparation of optic nerve, an effect prevented by the A3R antagonist MRS1220 (Gonzalez-Fernandez et al., 2014). Moreover MRS1220 also prevented optic nerve demyelination induced by in vitro ischemic-like conditions, i.e., OGD (Gonzalez-Fernandez et al., 2014). Thus, data suggest that adenosine, via activation of A3Rs, triggers oligodendrocyte death (Figure 1) and contributes to white matter ischemic damage.
Role of A2BRs in OPCs and Oligodendrogliogenesis
Current research in the field of adenosine is ongoing thanks to the growing interest on this receptor subtype. The pharmacological and physiological characterization of A2BRs has long been precluded by the lack of suitable ligands (Popoli and Pepponi, 2012).
Very few data are available to date about the role of A2BRs in oligodendroglial cells, as this adenosine receptor subtype is somewhat the most enigmatic and less studied among the four P1 receptors. We recently found that A2BRs are crucially involved in OPC maturation by demonstrating that the selective A2BR agonist BAY60-6583, and its recently synthetized analog P453 (Betti et al., 2018), inhibited in vitro OPC differentiation, as demonstrated by the reduced expression of myelin-related proteins such as MBP or MAG in primary purified OPC cultures (Coppi et al., 2020a). We also demonstrated that A2BR activation reversibly inhibits TEA- sensitive, sustained IK, and 4-amynopyridine- (4-AP) sensitive, transient IA, conductances (Coppi et al., 2020a). As mentioned above, IK are necessary to OPC maturation (Gallo et al., 1996), so this could be one of the mechanisms by which A2BRs inhibit myelin production. The AC activator forskolin mimicked BAY60-6583-mediated effect as it decreased IK currents, in line with previous data (Soliven et al., 1988). Of note, a further application of BAY60-6583 in the presence of forskolin was devoid of effect, thus indicating that A2BR activation inhibits IK by increasing intracellular cAMP levels (Coppi et al., 2020a).
Data about an inhibitory role of A2BR in myelin formation are consistent with recent findings from Manalo et al. (2020) who demonstrated that elevated cochlear adenosine levels in ADA–/– mice is associated with sensorineural hearing loss (SNHL) due to cochlear nerve fiber demyelination and mild hair cell loss. Intriguingly, A2BR-specific antagonists administered in ADA–/– mice significantly restored auditory capacity, nerve fiber density and myelin compaction. The same authors also provided genetic evidence for A2BR upregulation not only in ADA–/– hear-impaired mice but also in age-related SNHL.
Adenosine and Multiple Sclerosis
Multiple sclerosis is a chronic demyelinating disease of the CNS that leads to progressive neurological disability (Courtney et al., 2009). Immune system and inflammatory niche react against the myelin sheath that covers axon fibers altering neuronal transmission leading to the onset of a permanent condition associated with nerve decline. Since the extent and severity of nerve damage is heterogeneous, MS clinical symptoms may differ upon patients depending on the type and amount of the affected nerves. The treatments available for MS are almost limited aiming at reducing relapsing frequency or increasing the remitting speed of the disease with the major aim to manage symptoms.
Adenosine receptors are involved in inflammation and oligodendrogliogenesis, as reported above, and may represent potential therapeutic targets in the treatment of MS (Johnston et al., 2001; Stevens et al., 2002; Coppi et al., 2015; Cherchi et al., 2021). Compounds used in the treatment of MS, such as methotrexate and cladribine, have been shown to act as ligands at adenosine receptors, to exert their anti-inflammatory activities (Montesinos et al., 2000). Therefore, the beneficial actions of these drugs may also involve their ability to activate adenosine A2R and A3R in a compensatory manner to regulate cytokine expression (Johnston et al., 2001).
Experimental autoimmune encephalomyelitis (EAE) is the most frequently used animal model to study the immunopathogenesis of MS and to test the therapeutic efficacy of novel agents for preclinical study (Constantinescu et al., 2011). EAE can be induced by inoculating the animal with whole myelin or defined myelin protein, as myelin oligodendrocyte glycoprotein (MOG), with adjuvants, which lead to activation of autoreactive peripheral CD4 T-cells and their subsequent trafficking to the CNS by crossing the blood brain barrier (BBB).
The A1R is expressed on cells of the monocyte/macrophage lineage and a downregulation of A1R was found in both blood and brain from MS patients (Mayne et al., 1999; Johnston et al., 2001). Accordingly, activation of A1R is reported to protect from EAE damage (Chen et al., 2010; Wang et al., 2014; Liu G. P. et al., 2018) and A1R knockout (A1R–/–) exacerbates MOG-induced EAE pathology by increasing demyelination, axonal injury and neuroinflammation (Tsutsui et al., 2004). Finally, A1R activation improves myelin repair by recruiting OPCs in an experimental model of rat optic nerve demyelination (Asghari et al., 2013).
The role of A2ARs in MS is controversial. This receptor subtype is upregulated in human lymphocytes (Vincenzi et al., 2013) and in the CNS (Rissanen et al., 2013) of MS patients, thus suggesting an involvement in demyelinating pathologies. However, A2AR overexpression does not correlate with different forms of the disease nor is affected by MS pharmacological treatments (Vincenzi et al., 2013). Surprisingly, A2AR–/– mice developed more severe EAE than wild type animals (Mills et al., 2012; Yao et al., 2012) and the adoptive transfer of peripheral blood cells lacking the A2AR into wild type animals induced more severe EAE than both wild type and A2AR–/– mice (Mills et al., 2012). This suggests that A2AR–/– mice are susceptible to a severe acute form of EAE due to the lack of A2AR-mediated anti-inflammatory effects. Indeed, in human lymphocytes, A2AR agonist inhibits the release of proinflammatory cytokines, cell proliferation, the expression of the adhesion molecule VLA-4, and the activation of the transcription factor NF-κB; these effects were more evident in lymphocytes from MS patients in comparison to healthy subjects, in line with upregulation of A2ARs in MS lymphocytes (Vincenzi et al., 2013). Consistent with this, the activation of A2AR signaling by selective agonist inhibits the EAE progression by suppressing the specific lymphocyte proliferation, reducing the infiltration of CD4+ T lymphocytes, increasing intracellular Ca2+ levels (Liu et al., 2016), and reducing the effects of Th1 stimulation on the BBB permeability (Liu Y. et al., 2018). Additional evidence demonstrates that A2AR agonism in EAE leads to prevention of the disease when used in early disease stage (Ingwersen et al., 2016) whereas in late-stage EAE the number of foci with marked amount of myelin debris was higher in A2AR–/– mice than wild type (Ingwersen et al., 2016). These findings led to hypothesise that the relevance of A2AR for the pathogenesis of chronic autoimmune neuroinflammation may depend on the time point or the compartment, i.e., the systemic immune response vs. the CNS, an issue that has been elegantly addressed by Rajasundaram in a recent review (Rajasundaram, 2018).
Little is known about the role of A2BR in MS or EAE. Similarly to A2ARs, A2BR expression increases in peripheral lymphoid tissues of EAE mice. However, up to now, evidences converge on indicating that A2BR blockade is protective in EAE models. Indeed, Wei and colleagues demonstrated that A2BR-specific antagonists or genetic ablation of the receptor attenuated the clinical signs of EAE and protect the CNS from immune damage, probably by eliminating adenosine-mediated IL-6 production (Wei et al., 2013). Furthermore, the A2BR agonist BAY60-6583 reversed mesenchymal stem cells-induced downregulation of AQP4 expression in cultured astrocytes, that is protective for maintaining the integrity of BBB in EAE (Liu et al., 2020).
Sphingosine 1-Phoshate and Its Metabolism
Sphingosine 1-phosphate (S1P) is a natural sphingolipid present in plasma and tissues (Ksiazek et al., 2015) that acts as a modulator of different physiological and pathological processes, such as angiogenesis, vascular stability and permeability, T- and B-cell trafficking, as well as tumorigenesis (Hla et al., 2008). Circulating S1P is mainly produced and released by erythrocytes together with platelets and endothelial cells; it is mainly transported in association with apolipoprotein M (apoM) in high-density lipoprotein (HDL), while a small fraction is transported bound to albumin. ApoM can be considered a S1P chaperone protein (Obinata et al., 2019), which carries the sphingolipid in blood and interstitial fluids, facilitates S1P signaling in tissues and is responsible for physio-pathological effects different from that elicited by albumin-bound S1P. ApoM-bound S1P is able to exert multiple effects such as reduction of vascular inflammation, improvement of endothelial barrier function, inhibition of oxidized low-density lipoprotein- (ox-LDL-) induced inflammation, modification of BBB permeability and protection from bacteria-induced inflammatory responses (Christoffersen et al., 2011; Galvani et al., 2015; Christensen et al., 2016; Zheng et al., 2019). Endothelial cells embracing the BBB are able to secrete S1P into the blood as well as into brain compartments (Hajny and Christoffersen, 2017). S1P concentration is much higher in blood and lymph at μM range than in interstitial tissues due to the activity of S1P degrading enzymes. This concentration gradient, termed the “vascular S1P gradient” causes lymphocyte and hematic cell trafficking from lymph organs or bone marrow to the circulation (Schwab et al., 2005; Pappu et al., 2007; Hla et al., 2008; Cyster and Schwab, 2012). Enhanced vascular permeability, which occurs especially during inflammation, induces a burst of S1P that becomes available in the extravascular milieu, suggesting that the vascular S1P gradient may contribute to physiological and pathological conditions.
The catabolic pathway of plasma membrane-derived complex sphingolipids, mainly sphingomyelin, gives rise to different bioactive molecules, among others S1P. The first event is the sphingomyelinase-dependent hydrolysis of sphingomyelin, that leads to the production of the pro-apoptotic ceramide (Proia and Hla, 2015). Ceramidase deacylates ceramide to sphingosine, which in turn is converted into S1P by ATP-dependent phosphorylation catalyzed by two isoforms of the enzyme sphingosine kinase (SphK), namely SphK1 and 2 (Figure 2). These isozymes have been cloned and characterized, and their encoding genes are localized in different chromosomes, 17 and 19 respectively, known to produce multiple splicing variants (Imamura et al., 2001; Alemany et al., 2007). SphK1 and SphK2 differ for subcellular localization, showing partial overlapping but sometimes different biological functions (Liu et al., 2002; Pyne et al., 2016). In this regard, although knockout mouse models for either SphK1 or SphK2 have no gross phenotypic alterations, the double null mutation is lethal to embryos due to alterations in vasculogenesis and hemorrhages (Allende et al., 2004; Mizugishi et al., 2005; Michaud et al., 2006). SphK1 and SphK2 have structural homologies and share five conserved catalytic domains including an ATP-binding motif related to that of the diacylglycerol kinase family (Pitson et al., 2000; Liu et al., 2002). However, the sequence of SphK2 shows additional regions at the N-terminal and central proline-rich sequences which are absent in SphK1 (Igarashi et al., 2003; Gao et al., 2012). A nuclear localization sequence (NLS) and a nuclear exporting sequence (NES) are responsible for SphK2 major localization in the nucleus, since overexpression of SphK2 or SphK1 fused with NLS, but not wild-type SphK1, causes an inhibition of DNA synthesis (Igarashi et al., 2003). Indeed, it has been demonstrated that SphK2 has a role in epigenetic regulation of gene expression being involved in the suppression of histone deacetylase (HDAC) activity (Hait et al., 2009). Moreover, SphK2 is present in five different splicing variants that have been reported to localize into other different intracellular compartments such as the cytosol, mitochondria and the endoplasmic reticulum (ER) (Maceyka et al., 2005; Okada et al., 2005; Strub et al., 2011).
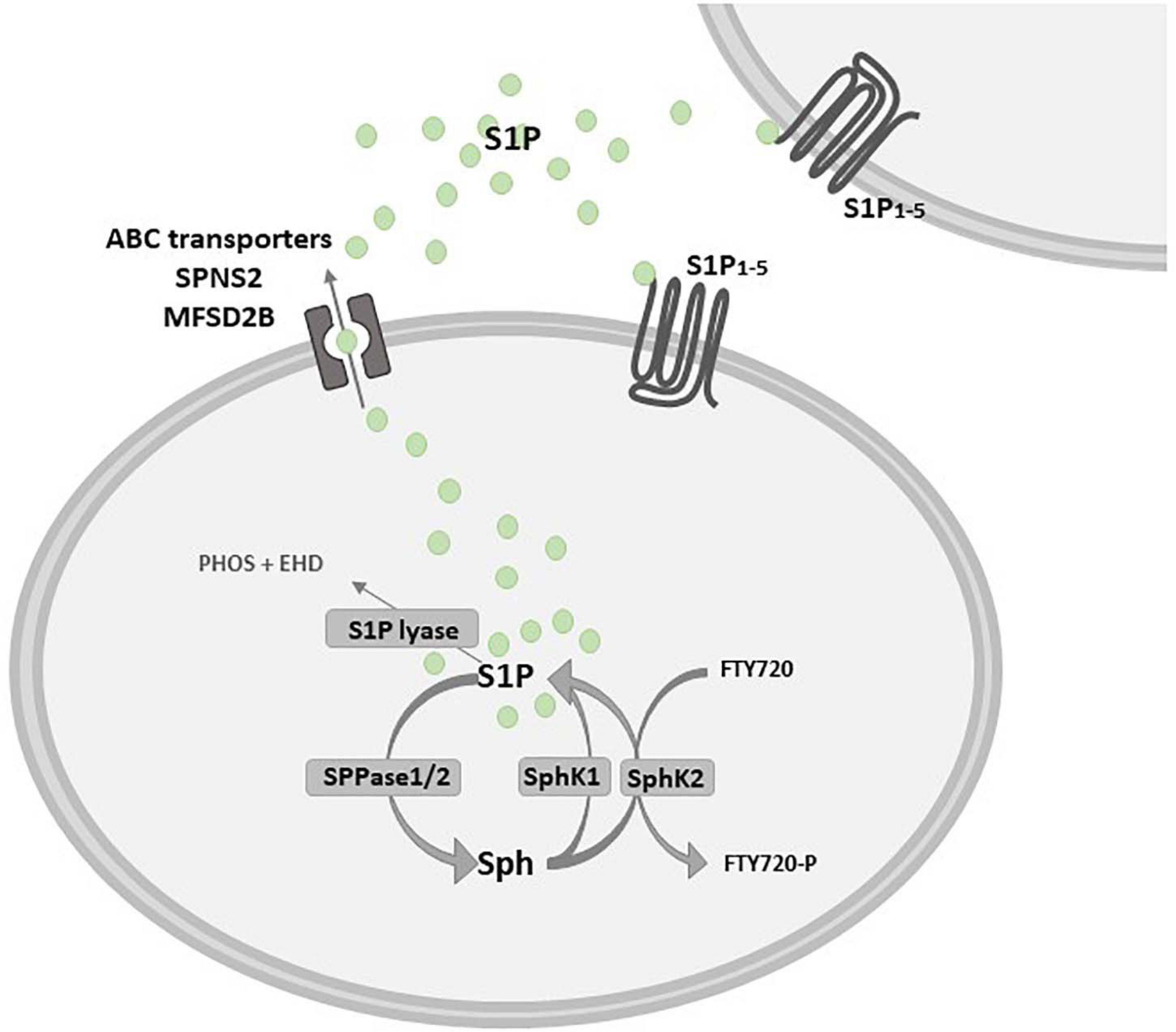
Figure 2. Schematic diagram describing the inside-out signaling of S1P. Sphingosine (Sph) kinase 1 and/or 2 (SphK1/SphK2) activation leads to sphingosine 1-phosphate (S1P) production that can act as an intracellular mediator or is extracellularly released to bind specific S1P receptors (S1P1–5). S1P release in the extracellular milieu depends on specific and/or unspecific transporters, such as the S1P transporter spinster homolog 2 (SPNS2) and the major facilitator superfamily transporter 2b (MFSD2B), as well as some ATP-binding cassette (ABC) family members, that are critically implicated in the so called S1P “inside-out signaling”. S1P is dephosphorylated by sphingosine 1-phosphate phosphatase 1 or 2 (SPPase1/2) or it is irreversibly degraded by S1P lyase in phosphoethanolamine (PHOS) and hexadecanal (EHD). Fingolimod (FTY720) is a structural analog of Sph that is rapidly phosphorylated by SphK2 in FTY720-P.
Intriguingly, SphK2 displays paradoxical effect on cell survival. Indeed, SphK2 shows a BH3 putative pro-apoptotic domain, although apoptosis is induced via SphK2-Bcl-xL-interaction after overexpression of the kinase (Liu et al., 2003). Moreover, knockdown of SphK2 also affects apoptosis induced by transforming growth factor beta (TGFβ) in C2C12 myoblasts (Cencetti et al., 2013). Alternately, SphK2 is significantly elevated in a broad range of human cancers, including bladder, melanoma, breast, neuroblastoma and leukemia (Neubauer et al., 2016; Bruno et al., 2020); in agreement to SphK2 expression in cancer, SphK2 down-regulation has been demonstrated to decrease the proliferation of cancer cells (Van Brocklyn et al., 2005; Hait et al., 2005), and SphK2-deficient xenografts show a significantly delayed growth (Weigert et al., 2009), pointing at a crucial role of the kinase in carcinogenesis.
Concerning SphK1, its up-regulation is tumorigenic whereas down-regulation results in anti-cancer effects (Sarkar et al., 2005; Taha et al., 2005). The localization of SphK1 is mainly in the cytosol, but upon activation by several stimuli the kinase is recruited at the plasma membrane (Johnson et al., 2002). Moreover, although both SphKs can phosphorylate the sphingosine analog, immunomodulatory drug FTY720 (Fingolimod), SphK2 appears to be more efficient in this activity than SphK1 (Billich et al., 2003; Paugh et al., 2003). Indeed, in mice lacking SphK2, but not SphK1, lymphopenia and lymphocyte retention into lymphoid organs induced by FTY720 are completely lost (Allende et al., 2004; Kharel et al., 2005).
The levels of S1P are tightly regulated by modulation of both anabolic and catabolic pathways. Breakdown of S1P to sphingosine is reversibly triggered by S1P phosphatases (SPP1 and 2), as well as unspecific lipid phosphate phosphatase (LPP), otherwise S1P is irreversibly degraded to hexadecenal and phosphoethanolamine by S1P lyase (Hannun and Obeid, 2008; Figure 2). De novo sphingolipids pathway alternatively accounts for the production of ceramide, which is the main hub of the sphingolipid metabolism (Hannun and Obeid, 2008): this anabolic pathway is initiated by serine palmitoyl transferase that catalyzes the condensation of serine with palmitoyl-CoA to produce 3-keto-dihydrosphingosine, which is consequently converted into dihydrosphingosine (Hanada, 2003). Ceramide synthases catalyze the acylation of dihydrosphingosine in dihydroceramide and finally dihydroceramide desaturase is involved in the final step of the de novo synthesis by producing ceramide; besides representing a substrate for sphingomyelin synthase giving rise to sphingomyelin and for other complex sphingolipid biosynthetic pathways, also de novo synthesized ceramide can fuel the activity of ceramidases and then S1P production. Ceramide is then transported to the Golgi complex, where it serves as substrate for production of complex sphingolipids (Gault et al., 2010).
Sphingosine 1-Phosphate Signaling
Extracellular stimuli including growth factors and cytokines such as epidermal growth factor (EGF), PDGF, vascular endothelial growth factor (VEGF), insulin-like growth factor- 1 (IGF-1), TNFα and TGFβ can induce the activation of SphK1 that translocates from cytosol to the plasma membrane to produce S1P (Donati et al., 2007; Bernacchioni et al., 2012; Bernacchioni et al., 2021). The bioactive sphingolipid can act in an autocrine or paracrine manner after its release in the extracellular milieu (called “inside-out signaling”), depending on specific and/or unspecific transporters, such as the S1P transporter spinster homolog 2 (SPNS2) (Hisano et al., 2012; Spiegel et al., 2019), the major facilitator superfamily transporter 2b (MFSD2B) (Kobayashi et al., 2018) and some, unspecific, ATP-binding cassette (ABC) transporters (Mitra et al., 2006; Figure 2).
The bioactive S1P selectively binds to high affinity cell surface G protein-coupled receptors (GPCRs; S1PRs), named S1P1, S1P2, S1P3, S1P4, and S1P5 (Ishii et al., 2004) that have been involved in the majority of physiological and pathological actions evoked by S1P such as immune response, cardiovascular functions, cancer, atherosclerosis (Cartier and Hla, 2019) as well as MS (Strub et al., 2010; Maceyka et al., 2012). While S1P1, S1P2 and S1P3 show broad tissue expression, S1P4 displays quite selective localization in immune system and S1P5 is primarily expressed in the spleen, on natural killer cells and other lymphocytes, and in CNS, mainly in oligodendrocytes (Mutoh et al., 2012). S1PRs exhibit a distinct capacity to couple to different G proteins thus activating different patterns of intracellular signaling cascades (Figure 3). S1P1 exclusively activates members of the Gi family, whereas S1P2 and S1P3 have a broader coupling profile and not only activate Gi but also Gq and G12/13. Moreover, activation of Rho, Rac and other small GTPases induces downstream signaling pathways including MAPK, phosphoinositide 3-kinase/Akt, Rho-associated protein kinase (ROCK). Further downstream effectors of S1PRs include AC, PLC, PKC and intracellularCa2+ transients (Windh et al., 1999; Maceyka et al., 2012; Figure 3). In addition, several lines of evidence show that S1P can also act as an intracellular messenger (Kohno et al., 2006; Olivera and Spiegel, 2001; Strub et al., 2010; Maceyka et al., 2012) regulating fundamental biological processes, such as gene expression, mitochondrial functions and inflammation by interacting with intracellular targets, including HDACs, E3 ubiquitin ligases and prohibitin 2. Indeed, it has been shown that SphK2 is associated with histone H3, and regulates histone tail acetylation via S1P-medated inhibition of HDAC, thus inducing chromatin remodeling and gene transcription (Hait et al., 2009). Interestingly, TNFα-induced activation of NF-κB requires SphK1 activity and S1P production. In particular, pro-survival TNF signaling requires TRADD-mediated recruitment of TNF receptor-associated factor 2 (TRAF2), which is the prototypical member of E3 ubiquitin ligase (Alvarez et al., 2010). Recruitment of TRAF2 to the TNFα-induced signaling complex results in the polyubiquitination of receptor interacting protein 1 (RIP1) preventing procaspase 8 cleavage that leads to apoptosis. Instead, polyubiquitinated RIP1 is capable of binding and activating IκB kinase (IKK), that phosphorylates the inhibitor of NF-κB (IκB), thus releasing the NF-κB dimer that translocates to the nucleus to exert transcriptional control. The RING domain of E3 ubiquitin ligase TRAF2 binds and activates SphK1, responsible for increased S1P levels essential for NF-κB-dependent p21/cip1 and c-fos gene expression that counteract caspase-8 activation and apoptosis induction. This peculiar molecular mechanism that accounts for S1P pro-survival effects relies exclusively on intracellular effects, since agonists of S1PR (such as dihydro S1P) unable to interact with TRAF2 do not possess anti-apoptotic effects. Moreover, S1P is able to specifically bind to prohibitin 2 (PHB2), regulating mitochondrial respiratory chain assembly. PHB2 is a highly conserved, ubiquitously expressed protein that forms large complexes in the inner mitochondrial membrane composed of heterodimers of PHB2/PHB1. SphK2 located in the mitochondria produces S1P that interacts with PHB2 and affects the assembly of complex IV (COX) respiratory chain activity and oxygen consumption (Strub et al., 2010). Finally, it was reported that both pharmacological inhibition/knockdown of SphK2, and overexpression of S1P lyase/SPP1 in neuroblastoma cells abrogate BACE1-mediated Aβ production (Takasugi et al., 2011) whereas exogenous S1P failed to increase amyloid-beta (Aβ) production. On the other hand, SphK2 overexpression increased the amount of secreted Aβ by producing S1P that interacts with BACE1, suggesting that SphK2-generated S1P regulates Aβ production via BACE1 activation.
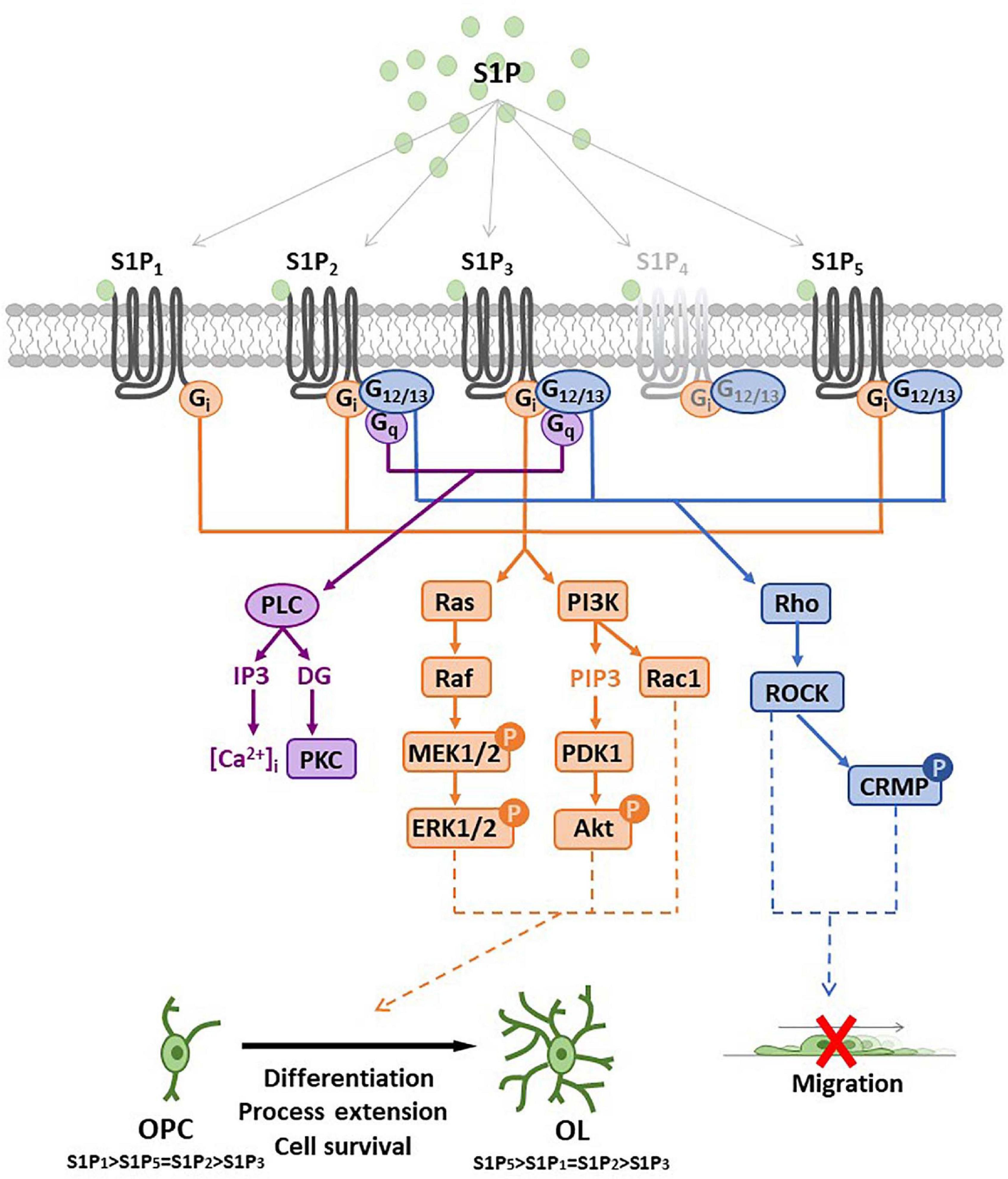
Figure 3. Sphingosine 1-phosphate receptor (S1PR) expression and main signal transduction pathways activated in oligodendrocyte progenitor cells (OPCs) and oligodendrocytes (OLs). Schematic representation of sphingosine 1-phosphate (S1P) G-protein coupled receptors (S1P1–5) and main signaling pathways involved after receptor activation. All S1P1–5 are coupled to Gi protein that activates Ras/PI3K pathways (in orange) promoting oligodendrocyte differentiation, process extension or cell survival. S1P2/5 are also coupled to G12/13 protein that activates Rho pathway (in blue) and reduces OPC migration or induces process retraction. S1P2/3 are coupled also with Gq protein that activates PLC leading to intracellular calcium increase and PKC activation. Abbreviation: mitogen-activated protein kinase (MEK); extracellular signal-regulated kinases (ERK); phosphatidylinositol 3-kinase (PI3K); phosphatidylinositol (3,4,5)-trisphosphate (PIP3); 3-phosphoinositide dependent protein kinase-1 (PDK1); protein kinase B (Akt); phospholipase C (PLC); protein kinase C (PKC); Rho-associated protein kinase 1 (ROCK); collapsin response mediator protein (CRMP); inositol triphosphate (IP3); diacylglycerol (DG).
Nevertheless, it has been extensively demonstrated that S1PRs have a major role in S1P-dependent biological effects. Importantly depending on the expressed profile of S1PRs, S1P appears capable of differently affecting key cellular events such as proliferation, survival, motility and differentiation in different cell types (Chun et al., 2000; Spiegel and Milstien, 2003; Blaho and Hla, 2011; Soliven et al., 2011). Indeed, S1PR have a wide variety of biological effects in multiple organs and tissues, such as immune, cardiovascular and respiratory systems, as well as in the CNS. Experimental evidence has further highlighted S1P receptors as a potential targets for the regulation of vascular permeability and neuroprotection in different conditions such as pain, stroke and demyelinating diseases (Fyrst and Saba, 2010).
Multiple Sclerosis and Fingolimod
Myriocin-derivative FTY720 (fingolimod), the active substance of Gilenya®, has been authorized by Food and Drug Administration (FDA) and European Medicines Agency (EMA) as the first oral treatment for relapsing-remitting MS, based on extensive clinical trials (Kappos et al., 2010; Cohen et al., 2010). FTY720 is a sphingosine analog prodrug phosphorylated by SphK2 isozyme to produce the active form FTY720-phosphate (FTY720-P) (Paugh et al., 2003; Billich et al., 2003; Zemann et al., 2006; Figure 2), that in turn activates four out of five S1PR receptor subtypes, except S1P2, in the range of sub-nanomolar concentrations (Brinkmann and Lynch, 2002; Mandala et al., 2002). As initially reported, FTY720 was found to act as immunomodulator capable of depleting mature T cells in allograft models (Chiba et al., 2005). Although early studies addressed FTY720 as a low-efficacy suppressor of transplantation rejection (Budde et al., 2006), its successful employment in EAE supported the therapeutic action for MS treatment (Brinkmann and Lynch, 2002; Fujino et al., 2003; Webb et al., 2004; Kataoka et al., 2005; Papadopoulos et al., 2010).
The main recognized mechanism by which FTY720 improves MS disease is by affecting immune responses, specifically regulating lymphocyte trafficking. Circulating T lymphocytes express S1P1 and lower levels of S1P3/4 (Graeler and Goetzl, 2002) receptors, and the interaction of exogenous S1P with S1P1 is capable of initiating lymphocyte egress from lymph nodes by overcoming retention signals (Brinkmann et al., 2010). Although acute administration of FTY720-P activates S1P1 (Camm et al., 2014), chronic exposure to the S1P analogue leads to irreversible receptor internalization resulting in ‘functional antagonism’ of S1P1 signaling (Graler and Goetzl, 2004; Brinkmann, 2009; Gergely et al., 2012). FTY720 has a selective mechanism of action, targeting specific subclasses of lymphocytes: FTY720 treatment negatively modulates S1P1, thus causing a retention of circulating pathogenic lymphocytes (naive and central memory T cells positive for the chemokine receptor 7, the CCR7) back into the lymph nodes (Matloubian et al., 2004), thereby preventing their infiltration into the CNS, where they exert pathological effects (Cohen and Chun, 2011). However, FTY720 does not significantly affect activation or proliferation of redistributed naïve and central memory T cells and does not block the egress of CCR7-negative effector memory T cells from lymph nodes, preserving immunosurveillance (Mehling et al., 2008).
Intriguingly, differently from FTY720, apoM-S1P is unessential for lymphocyte trafficking although limits lymphopoiesis by activating the S1P1 receptor on lymphocyte progenitors. Indeed, the effect exerted by apoM-bound S1P has been investigated in EAE mice lacking apoM, who developed more severe disease with increased lymphocytes in the CNS and breakdown of the BBB. Moreover, apoM-bound S1P, but not albumin-, inhibits lymphopoiesis in vitro and overexpression of apoM in rodents decreases endothelial inflammation and EAE manifestation exerting a protective function against autoimmune inflammation (Blaho et al., 2015; Wang et al., 2019).
In addition to immunological actions, FTY720 can penetrate the BBB acting on different S1P receptors that are expressed in brain resident cells, such as astrocytes and oligodendrocytes, claiming the possibility that FTY720 may elicit direct actions on CNS. Indeed, after the treatment with the pro-drug FTY720, FTY720-P has been detected in the cerebrospinal fluid at sub-nanomolar levels (Foster et al., 2007), which are sufficient to modulate human CNS cell properties in vitro (Miron et al., 2008c). This approach is consistent with multiple actions of the lysosphingolipid in the CNS, in line with growing literature describing direct effects of S1P signaling on brain cells. Using S1P receptor specific agonists and antagonists, S1P3 and S1P5 were involved in FTY720-induced effects for the remyelination and astrogliosis, whereas S1P1 and S1P5 affected microgliosis (Miron et al., 2010). Therapeutic interventions that affect oligodendrocyte remyelination processes could be critical factors for long-term functional recovery in MS. In particular, in organotypic cerebellar slices (Miron et al., 2010), the S1P analog was found to enhance remyelination which occurs subsequently to a demyelinating insult, this event being mediated at least in part by OPC differentiating into myelinating OLs.
Although FTY720 treatment has no positive impact on myelin content under basal conditions, FTY720 administration increases both remyelination score subsequent to lysolecithin-induced damage, and the number of endogenous OPCs within the lesion by facilitating migration, recruitment and proliferation of these cells. Furthermore, it enhances OPC process extension and differentiation into mature OLs (Yazdi et al., 2015), as well as amplifies the number of axons with remyelinated myelin sheaths. Despite these findings, in cuprizone model of MS, FTY720 treatment do not enhance remyelination while decreasing severity of demyelination and promoting OPC proliferation (Kim et al., 2011; Alme et al., 2015). However, in this MS model, fingolimod induces higher number of mature OLs near demyelinated areas, indicating a potential effect on differentiation and/or migration without apparent effects on remyelination (Hu et al., 2011; Alme et al., 2015; Nystad et al., 2020). Nevertheless, another research group reported that fingolimod treatment (0.3 mg/kg) causes remyelination in acute cuprizone-induced demyelination model (Slowik et al., 2015). Hypothesis on such inconsistencies can be made regarding the concentration of fingolimod as well as time-dependence that may cause different effects in cuprizone model. Fingolimod (1 mg/kg) treatment after 3 days from cuprizone administration induces survival of mature OLs whereas later administration of the drug (started at 10 days after cuprizone treatment) lacks cytoprotective effect (Kim et al., 2018), claiming the possibility that early intervention appears to be required to prevent demyelination. In cuprizone model of demyelination, FTY720 (0.3 mg/kg), co-administered with transplanted neural progenitor cells derived from induced pluripotent cells (iPS-NPC), increases OPC survival and proliferation. Moreover, the authors assess that differentiation of transplanted iPS-NPC into oligodendroglial lineage may occur (Yazdi et al., 2015).
Treatment with FTY720 at the onset of EAE reduces clinical symptoms and decreases demyelination by blocking the Akt/mTOR signaling pathway (Hou et al., 2016). In later stage of EAE disease, FTY720 treatment increases MBP level and promotes the appearance of newly generated myelinating OLs, via Sonic Hedgehog signaling pathway, decreases EAE clinical manifestation, and improves neurological functions (Zhang et al., 2015). Furthermore, FTY720 administration (1 mg/kg) during EAE induction in female mice reduces demyelinated area, axonal damage, brain atrophy while increasing brain-derived neurotrophic factor (BDNF) level and clinical scores (Fukumoto et al., 2014; Smith et al., 2018), underling the positive effect of FTY720 on regeneration in different types of demyelination models.
Finally, conditional knockout of S1P1 in neural lineages have been used to identify a key role for astrocytes in reducing the severity of pathological changes in EAE. Indeed, the efficacy of fingolimod is lost by astrocytic deletion of S1P1, highlighting that the main protective effect of this compound in EAE comprises the modulation of astrocyte function by S1P1 (Choi et al., 2011).
Sphingosine 1-Phosphate Receptor Signaling in Oligodendrocytes and Interaction With A2BRs
OPC availability, recruitment and differentiation into mature oligodendrocytes are pivotal aspects involved in remyelination. Experimental evidence demonstrates that S1PRs display different biological effects depending on oligodendrocyte differentiation state. Oligodendroglial cells express four out of five S1PRs, S1P1, S1P2, S1P3, and S1P5, whose expression profiles change and are selectively controlled during oligodendrogenesis. Indeed, the predominant isoform in immature OPC is S1P1 whereas S1P2, S1P3 and S1P5 are present at much lower levels (Im et al., 2000; Yu N. C. et al., 2004; Jaillard et al., 2005; Novgorodov et al., 2007). During differentiation from OPC into mature OLs, S1P5 and S1P1 are reciprocally regulated, the first being augmented while the latter decreases (Jung et al., 2007; Coelho et al., 2007; Miron et al., 2008c; van Doom et al., 2012). Indeed, human mature OLs express S1PR transcripts in relative abundance of S1P5 > S1P3 > S1P1 (Miron et al., 2008a). In particular, the modulation of S1PR expression seems to be mediated by PDGF that is produced by OPCs. Indeed, S1PRs are differentially modulated by PDGF resulting in downregulation of S1P5 and upregulation of S1P1 in OPCs. Downregulation of S1P1 by RNA interference affects PDGF-induced proliferation of OPCs (Jung et al., 2007) whereas, in mature OLs, S1P signaling promotes cell survival, suggesting that S1PRs may exert different functions during oligodendroglial development (Okada et al., 2009). The effects of fingolimod on oligodendrocyte process dynamics depended on both dose and duration of treatment, since S1PR activation by fingolimod induces process retraction in O4-positive pre-OLs, that appears to be transient and restricted to immature cells, not observed at later and mature developmental stages (Miron et al., 2008b). Process retraction is mediated by S1P5 via a ROCK/collapsin response-mediated signaling pathway, whereas the effect is abrogated in S1P5–/– mice derived oligodendrocytes. Prolonged treatment with higher doses of fingolimod induces process extension associated with Rac1-linked cytoskeletal signaling cascades mimicked by a S1P1 agonist SEW2871 (Miron et al., 2008b). Similarly, these results can be corroborated by the fact that, depending on the dose, FTY720-P exerts opposite actions in rat OPC cultures (Jung et al., 2007). Indeed, high concentration of FTY720-P (1 μM) inhibits, OPC differentiation, whereas low concentration (10 nM) enhances both the percentage of mature OLs and MBP expression.
Furthermore, S1P-induced survival of mature OLs is mediated through a pertussis toxin- (PTx) sensitive, Akt-mediated pathway, since the molecular mechanism activated by S1PRs in oligodendrocytes specifically involves the phosphorylation of ERK1/2 and Akt, that subsequently promotes cell survival (Jaillard et al., 2005; van Doom et al., 2012). Noteworthy, the pro-survival effect of S1PR in human mature OLs is mimicked by the administration of S1P5 agonist. Interestingly, S1PR agonists affect oligodendrocytes differentiation stages depending on the molecular mechanisms evoked and in a concentration-dependent manner. In particular, human OPC cultures treated with low nanomolar concentrations of either FTY720-P or S1P present enhanced differentiation into both pre-OLs (O4-positive) and mature (GC-positive) OLs. Of note, ERK1/2 pathway is involved in the differentiation of OPC into O4 positive-cells, since inhibition of MAPK pathway by U0126 prevented pre-OLs generation. However, the transition to mature OLs is mediated by p38MAPK signaling, since PD169316 administration blocks the progression of O4 positive into mature stages of differentiation (Cui et al., 2014).
Notably, experimental evidences highlight that activation of S1P5 induces process retraction whereas activation of S1P1 enhances process extension of OPCs (Miron et al., 2008b), and coherently during early myelination S1P1 abrogation in the oligodendroglial cells delays OPC differentiation into mature OLs (Kim et al., 2011). In agreement, S1P1–/– mice show decreased myelin protein expression, thinner myelin and susceptibility to demyelination induced by cuprizone, that is conceivable with the major expression of S1P1 in OPCs that could play a pivotal role in early OPC differentiation stages. On the contrary, abnormalities in myelination are not evident in S1P5–/– mice; oligodendrocytes display a higher expression of S1P5 compared to other S1PRs where it may be involved in late myelination processes. Although S1P5 is expressed at lower levels in OPCs compared to OLs and OPCs are devoid of pro-survival effects of S1P5, this receptor subtype is activated by S1P to arrest OPC migration (Novgorodov et al., 2007). Indeed, S1P-induced decrease of chemiotaxis is completely prevented by the specific downregulation of S1P5, but not S1P2, and is insensitive to PTx, suggesting that S1P5-initiated signaling is not mediated by the Gαi-protein coupled pathway. The molecular mechanism that is responsible for the impairment of migratory capacity after S1P administration involves G12/13 that stimulates the Rho/ROCK signaling pathway (Novgorodov et al., 2007). The authors suggest that glutamate treatment of OPC may increase S1P export and S1P extracellular levels to modulate OPC motility and claim the possibility for S1P to be a part of the neuron-oligodendroglial communication network in developing brain, that could have a role also during remyelination processes. These data are also consistent with the physiological effect of S1P5 in OPCs during differentiation postulating that changes in receptor coupling with heterotrimeric G-proteins may occur, thus leading to the activation of different signaling pathways.
Finally, SphK1 has a protective role against apoptosis in OPC cultures (Saini et al., 2005). In particular, Neurotrophin 3 (NT-3) accounts for SphK1 translocation to plasma membrane and activation to exert its pro-survival effect in these cells. Remarkably, down-regulation of SphK1 negatively affects the capacity of NT-3 to protect oligodendrocyte progenitors from apoptosis. In agreement with these findings, analysis of plaques from MS brains shows reduced levels of S1P and increased sphingosine and C16/18-ceramide (Qin et al., 2010).
In the light of above results, we recently performed a study aimed to merge current knowledge on S1P pathway with A2BR signaling. Namely, by using either or both pharmacological approach and receptor silencing, we demonstrated that an interplay occurs between A2BRs and SphK/S1P axis in OPCs (Figure 4). Indeed, SphK1 enzyme is activated when OPCs are cultured in the presence of the A2BR selective agonist BAY60-6583, thus rising S1P production, whereas its silencing by small interference RNA (siRNA) increases the expression of S1P lyase, promoting S1P removal (Coppi et al., 2020a). This observation led to hypothesize that the anti-differentiating effect exerted by A2BR activation in OPCs is mediated by an increase in S1P intracellular levels (Figure 4). This hypothesis was confirmed by findings that the SphK inhibitors VPC96047 or VPC96091 markedly increased MAG and MBP expression in OPC cultures, indicating enhanced cell maturation, and also significantly increased IK currents, necessary to OPC differentiation (Coppi et al., 2020a). Thus, it appears that an increase in S1P production possibly accounts for the anti-differentiating effect of BAY60-6583 in OPCs, whereas A2BR silencing, by promoting S1P removal through the activation of S1P lyase, facilitates OPC maturation. An additional proof that A2BRs and S1P signaling are interconnected in OPCs resides in the fact that this receptor subtype is upregulated during cell differentiation, an effect that is completely prevented when cells are differentiated in the presence of the pan SphK inhibitor VPC96047 (Coppi et al., 2020a).
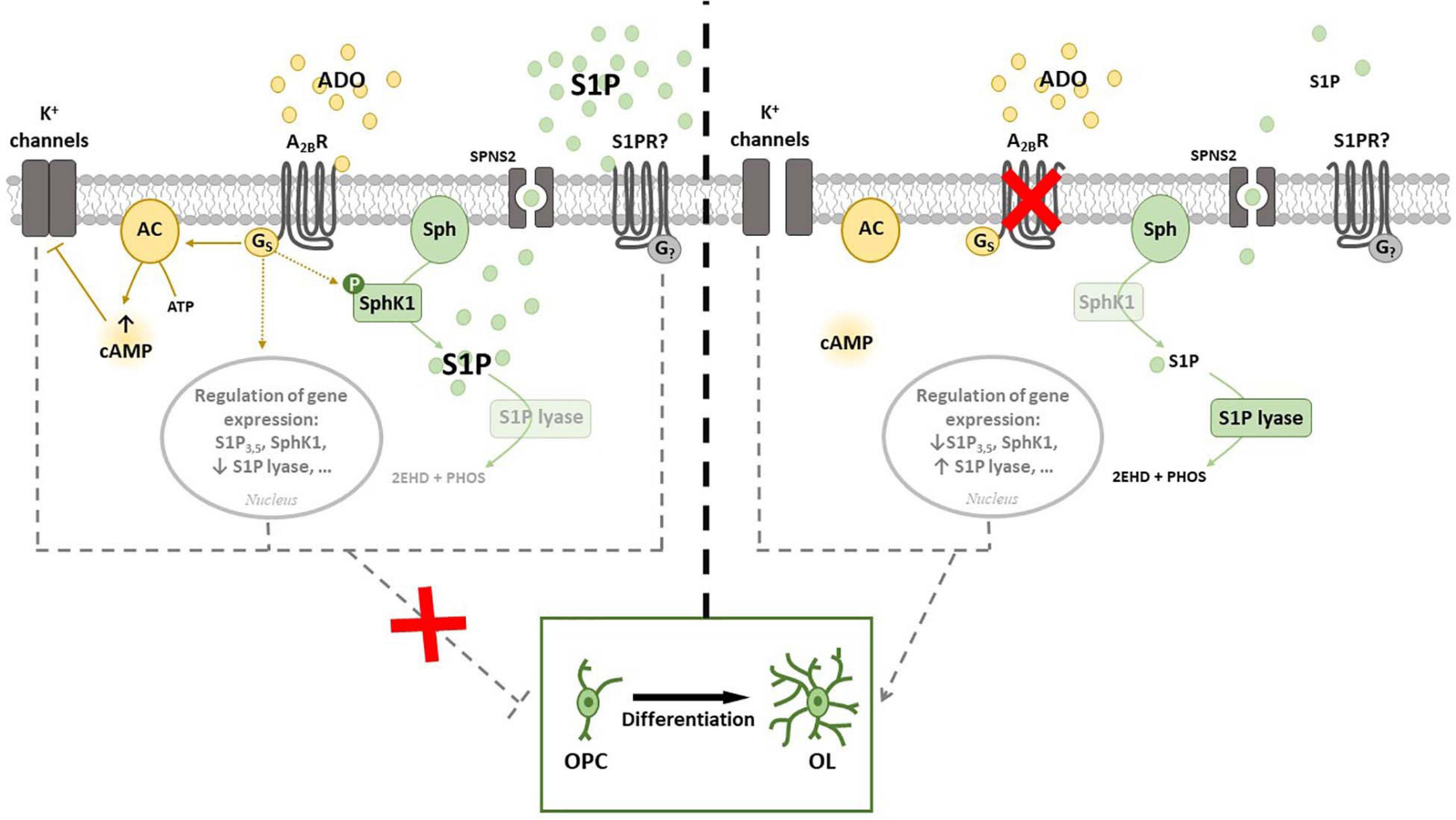
Figure 4. Cross-talk between adenosine A2B receptor (A2BR) and Sphingosine 1-phosphate (S1P). (Left panel) A2BR throughout Gs activation leads to an increase of intracellular cAMP that inhibits K+ channels. The activation of A2BR regulates gene expression of proteins involved in S1P signaling and metabolism and increases the phosphorylation of SphK1. All these events prevent OPC differentiation in vitro. (Right panel) A2BR silencing allows K+ channels to be opened and strikingly increases expression of S1P lyase, that irreversible catalyzes S1P and blocks S1P degradation signaling. In this conditions OPC differentiation is facilitated. Abbreviations: Adenosine (ADO); Sphingosine (Sph) kinase 1 (SphK1); specific spinster homolog 2 (SPNS2); adenylyl cyclase (AC) (Coppi et al., 2020a,b).
We also found that FTY720-P differently affects BAY60-6583-mediated K+ current inhibition depending upon the concentration applied. When administered at 1 μM, it mimicked and partially occluded the effect of a subsequent BAY60-6583 application on voltage-dependent K+ currents. This confirms, again, that S1P and A2BR pathways converge. On the other hand, the effect of BAY60-6583 on ramp currents was significantly enhanced in the presence of low (10 nM) FTY720-P (Coppi et al., 2020a). Similarly, 10 nM FTY720-P increased, whereas 1 μM decreased, MAG expression after 7 days of OPC maturation. Contrasting effects of this compound depending on the concentration used have been previously reported by others, the effects of fingolimod depending on both concentration and treatment duration (Jung et al., 2007; Miron et al., 2008c).
Differently from FTY720-P, when S1P is used as a ligand, the effect on K+ currents was not observed (Coppi et al., 2020a). This apparent discrepancy may be ascribed to the fact that receptor ligation by FTY720-P is restricted to all S1P receptors except S1P2, which however is activated by S1P. Moreover, the functional outcome induced by S1PR modulators could differ from one ligand to another since it could differently affect receptor fate. Indeed, it has been shown that FTY720-P can induce S1P1 receptor degradation, whereas S1P affects receptor recycling. Finally the lack of effect exerted by exogenous S1P on IK is in agreement with previous data in different cellular models, such as skeletal muscle cells, where agonist-induced S1P intracellular production and activity, called inside-out signaling, has different, even sometimes opposite, actions compared to exogenous S1P (Donati et al., 2004; Cencetti et al., 2010; Cencetti et al., 2013). This effect can be explained by a localized release of bioactive lipid in membrane microdomain where the availability of certain receptor subtypes is limited. The spatial regulation of S1P biosynthesis within the cell, together with its localized partitioning into plasma membrane domains, determines the subset of engaged S1PRs and thus the biological outcome (Donati et al., 2013).
Conclusion
Multiple evidences indicate that adenosine may interact with S1P signaling in orchestrating the processes concurring to OPC maturation and thus to the remodeling of brain development and/or repair after a demyelinating insult.
In particular, adenosine A2BR appears to play a critical role in oligodendrogliogenesis since its agonism activates SphK1 and reduces OPC differentiation. These data are in agreement with the fact that, on one hand, SphK inhibition decreases A2BR expression leading to an increase in OPC differentiation, and on the other, specific down-regulation of A2BR reduces SphK1 and potently induces S1P lyase expression thus pushing S1P toward catabolism, and thereby facilitating OPC maturation. On the whole, the available results suggest that A2BR antagonism represents a possible co-adjuvant strategy to improve remyelination promoted by the prodrug sphingosine analog, FTY720 (Fingolimod) for the treatment of MS, as confirmed by previous findings in the literature reporting a protective role of A2BR block in EAE models (Wei et al., 2013; Liu et al., 2020).
Author Contributions
EC, FP, PB, AP, and FCe: conceptualization. FP and PB validation. EC, AP, PB, FP, FCe, and CD: resources. EC, FCe, FCh, and AP: writing–original draft preparation. MV, FCh, FCe, and CD writing–review and editing. All authors contributed to the article and approved the submitted version.
Funding
The present work was supported by the University of Florence (Fondi Ateneo, AP); PRIN 2015E8EMCM_002 (AP); Fondazione Italiana Sclerosi Multipla (FISM): 2019/R-Single/036 (AP and EC); and EC was supported by Fondazione Umberto Veronesi FUV2020-3299.
Conflict of Interest
The authors declare that the research was conducted in the absence of any commercial or financial relationships that could be construed as a potential conflict of interest.
References
Alemany, R., van Koppen, C. J., Danneberg, K., ter Braak, M., and Heringdorf, D. M. Z. (2007). Regulation and functional roles of sphingosine kinases. Naunyn Schmiedeb. Arch. Pharmacol. 374, 413–428. doi: 10.1007/s00210-007-0132-3
Allende, M. L., Sasaki, T., Kawai, H., Olivera, A., Mi, Y., van Echten-Deckert, G., et al. (2004). Mice deficient in sphingosine kinase 1 are rendered lymphopenic by FTY720. J. Biol. Chem. 279, 52487–52492. doi: 10.1074/jbc.m406512200
Alme, M. N., Nystad, A. E., Bo, L., Myhr, K. M., Vedeler, C. A., Wergeland, S., et al. (2015). Fingolimod does not enhance cerebellar remyelination in the cuprizone model. J. Neuroimmunol. 285, 180–186. doi: 10.1016/j.jneuroim.2015.06.006
Alvarez, S. E., Harikumar, K. B., Hait, N. C., Allegood, J., Strub, M. G., Kim, Y. E., et al. (2010). Sphingosine-1-phosphate is a missing cofactor for the E3 ubiquitin ligase TRAF2. Nature 465, 1084–U1149.
Antonioli, L., Blandizzi, C., Pacher, P., and Hasko, G. (2013). Immunity, inflammation and cancer: a leading role for adenosine. Nat. Rev. Cancer 13, 842–857. doi: 10.1038/nrc3613
Antonioli, L., Blandizzi, C., Pacher, P., and Hasko, G. (2019). The purinergic system as a pharmacological target for the treatment of immune-mediated inflammatory diseases. Pharmacol. Rev. 71, 345–382. doi: 10.1124/pr.117.014878
Asghari, A. A., Azarnia, M., Mirnajafi-Zadeh, J., and Javan, M. (2013). Adenosine A1 receptor agonist, N6-cyclohexyladenosine, protects myelin and induces remyelination in an experimental model of rat optic chiasm demyelination; electrophysiological and histopathological studies. J. Neurol. Sci. 325, 22–28. doi: 10.1016/j.jns.2012.11.008
Attali, B., Wang, N., Kolot, A., Sobko, A., Cherepanov, V., and Soliven, B. (1997). Characterization of delayed rectifier Kv channels in oligodendrocytes and progenitor cells. J. Neurosci. 17, 8234–8245. doi: 10.1523/jneurosci.17-21-08234.1997
Barateiro, A., and Fernandes, A. (2014). Temporal oligodendrocyte lineage progression: in vitro models of proliferation, differentiation and myelination. Biochim. Biophys. Acta 1843, 1917–1929. doi: 10.1016/j.bbamcr.2014.04.018
Barres, B. A., Koroshetz, W. J., Swartz, K. J., Chun, L. L. Y., and Corey, D. P. (1990). Ion channel expression by white matter glia - the o-2a glial progenitor-cell. Neuron 4, 507–524. doi: 10.1016/0896-6273(90)90109-s
Bergles, D. E., and Richardson, W. D. (2016). Oligodendrocyte development and plasticity. Cold Spring Harb. Perspect. Biol. 8:a020453. doi: 10.1101/cshperspect.a020453
Bernacchioni, C., Cencetti, F., Blescia, S., Donati, C., and Bruni, P. (2012). Sphingosine kinase/sphingosine 1-phosphate axis: a new player for insulin-like growth factor-1-induced myoblast differentiation. Skelet. Muscle 2:15. doi: 10.1186/2044-5040-2-15
Bernacchioni, C., Ghini, V., Squecco, R., Idrizaj, E., Garella, R., Puliti, E., et al. (2021). Role of sphingosine 1-phosphate signalling axis in muscle atrophy induced by TNF alpha in C2C12 myotubes. Int. J. Mol. Sci. 22:1280. doi: 10.3390/ijms22031280
Betti, M., Catarzi, D., Varano, F., Falsini, M., Varani, K., Vincenzi, F., et al. (2018). The aminopyridine-3,5-dicarbonitrile core for the design of new non-nucleoside-like agonists of the human adenosine A(2B) receptor. Eur. J. Med. Chem. 150, 127–139. doi: 10.1016/j.ejmech.2018.02.081
Billich, A., Bornancin, F., Devay, P., Mechtcheriakova, D., Urtz, N., and Baumruker, T. (2003). Phosphorylation of the immunomodulatory drug FTY720 by sphingosine kinases. J. Biol. Chem. 278, 47408–47415. doi: 10.1074/jbc.m307687200
Blaho, V. A., Galvani, S., Engelbrecht, E., Liu, C., Swendeman, S. L., Kono, M., et al. (2015). HDL-bound sphingosine-1-phosphate restrains lymphopoiesis and neuroinflammation. Nature 523:342. doi: 10.1038/nature14462
Blaho, V. A., and Hla, T. (2011). Regulation of mammalian physiology, development, and disease by the Sphingosine 1-Phosphate and Lysophosphatidic acid receptors. Chem. Rev. 111, 6299–6320. doi: 10.1021/cr200273u
Bonetto, G., Kamen, Y., Evans, K. A., and Karadottir, R. T. (2020). Unraveling myelin plasticity. Front. Cell. Neurosci. 14:156. doi: 10.3389/fncel.2020.00156
Bortoluzzi, A., Vincenzi, F., Govoni, M., Padovan, M., Ravani, A., Borea, P. A., et al. (2016). A(2A) adenosine receptor upregulation correlates with disease activity in patients with systemic lupus erythematosus. Arthrit. Res. Ther. 18:192.
Brazel, C. Y., Rosti, R. T., Boyce, S., Rothstein, R. P., and Levison, S. W. (2004). Perinatal hypoxia/ischemia damages and depletes progenitors from the mouse subventricular zone. Dev. Neurosci. 26, 266–274. doi: 10.1159/000082143
Brinkmann, V. (2009). FTY720 (fingolimod) in multiple sclerosis: therapeutic effects in the immune and the central nervous system. Br. J. Pharmacol. 158, 1173–1182. doi: 10.1111/j.1476-5381.2009.00451.x
Brinkmann, V., Billich, A., Baumruker, T., Heining, P., Schmouder, R., Francis, G., et al. (2010). Fingolimod (FTY720): discovery and development of an oral drug to treat multiple sclerosis. Nat. Rev. Drug Discov. 9, 883–897. doi: 10.1038/nrd3248
Brinkmann, V., and Lynch, K. R. (2002). FTY720: targeting G-protein-coupled receptors for sphingosine 1-phosphate in transplantation and autoimmunity. Curr. Opin. Immunol. 14, 569–575. doi: 10.1016/s0952-7915(02)00374-6
Bruno, G., Cencetti, F., Pini, A., Tondo, A., Cuzzubbo, D., Fontant, F., et al. (2020). beta 3-adrenoreceptor blockade reduces tumor growth and increases neuronal differentiation in neuroblastoma via SK2/S1P(2) modulation. Oncogene 39, 368–384. doi: 10.1038/s41388-019-0993-1
Budde, K., Schutz, M., Glander, P., Peters, H., Waiser, J., Liefeldt, L., et al. (2006). FTY720 (fingolimod) in renal transplantation. Clin. Transplant. 20, 17–24. doi: 10.1111/j.1399-0012.2006.00596.x
Burnstock, G., Fredholm, B. B., and Verkhratsky, A. (2011). Adenosine and ATP receptors in the brain. Curr. Top. Med. Chem. 11, 973–1011. doi: 10.2174/156802611795347627
Camm, J., Hla, T., Bakshi, R., and Brinkmann, V. (2014). Cardiac and vascular effects of fingolimod: mechanistic basis and clinical implications. Am. Heart J. 168, 632–644. doi: 10.1016/j.ahj.2014.06.028
Cartier, A., and Hla, T. (2019). Sphingosine 1-phosphate: lipid signaling in pathology and therapy. Science 366:323.
Cencetti, F., Bernacchioni, C., Nincheri, P., Donati, C., and Bruni, P. (2010). Transforming growth factor-beta 1 induces transdifferentiation of myoblasts into myofibroblasts via up-regulation of sphingosine Kinase-1/S1P(3) axis. Mol. Biol. Cell 21, 1111–1124. doi: 10.1091/mbc.e09-09-0812
Cencetti, F., Bernacchioni, C., Tonelli, F., Roberts, E., Donati, C., and Bruni, P. (2013). TGF beta 1 evokes myoblast apoptotic response via a novel signaling pathway involving S1P(4) transactivation upstream of Rho-kinase-2 activation. FASEB J. 27, 4532–4546. doi: 10.1096/fj.13-228528
Chandrasekaran, B., Samarneh, S., Jaber, A. M. Y., Kassab, G., and Agrawal, N. (2019). Therapeutic potentials of A(2B) adenosine receptor ligands: current status and perspectives. Curr. Pharm. Des. 25, 2741–2771. doi: 10.2174/1381612825666190717105834
Chang, A., Tourtellotte, W. W., Rudick, R., and Trapp, B. D. (2002). Premyelinating oligodendrocytes in chronic lesions of multiple sclerosis. N. Engl. J. Med. 346, 165–173. doi: 10.1056/nejmoa010994
Chen, G. Q., Chen, Y. Y., Wang, X. S., Wu, Z. S., Yang, M. H., Xu, H. Q., et al. (2010). Chronic caffeine treatment attenuates experimental autoimmune encephalomyelitis induced by guinea pig spinal cord homogenates in Wistar rats. Brain Res. 1309, 116–125. doi: 10.1016/j.brainres.2009.10.054
Chen, J. F., Eltzschig, H. K., and Fredholm, B. B. (2013). Adenosine receptors as drug targets - what are the challenges? Nat. Rev. Drug Discov. 12, 265–286. doi: 10.1038/nrd3955
Cherchi, F., Pugliese, A. M., and Coppi, E. (2021). Oligodendrocyte precursor cell maturation: role of adenosine receptors. Neural Regen. Res. 16, 1686–1692. doi: 10.4103/1673-5374.306058
Chiba, K., Hoshino, Y., Ohtsuki, M., Kataoka, H., Maeda, Y., Matsuyuki, H., et al. (2005). Immunosuppressive activity of FTY720, sphingosine 1-phosphate receptor agonist: I. prevention of allograft rejection in rats and dogs by FTY720 and FTY720-phosphate. Transplant. Proc. 37, 102–106. doi: 10.1016/j.transproceed.2004.12.286
Chittajallu, R., Aguirre, A. A., and Gallo, V. (2005). Downregulation of platelet-derived growth factor-alpha receptor-mediated tyrosine kinase activity as a cellular mechanism for K+ channel regulation during oligodendrocyte development in situ. J. Neurosci. 25, 8601–8610. doi: 10.1523/jneurosci.2122-05.2005
Choi, J. W., Gardell, S. E., Herr, D. R., Rivera, R., Lee, C., Noguchi, K., et al. (2011). FTY720 (fingolimod) efficacy in an animal model of multiple sclerosis requires astrocyte sphingosine 1-phosphate receptor 1 (S1P(1)) modulation. Proc. Natl. Acad. Sci. U.S.A. 108, 751–756. doi: 10.1073/pnas.1014154108
Christensen, P. M., Liu, C. H., Swendeman, S. L., Obinata, H., Qvortrup, K., Nielsen, L. B., et al. (2016). Impaired endothelial barrier function in apolipoprotein M-deficient mice is dependent on sphingosine-1-phosphate receptor 1. FASEB J. 30, 2351–2359. doi: 10.1096/fj.201500064
Christoffersen, C., Obinata, H., Kumaraswamy, S. B., Galvani, S., Ahnström, J., Sevvana, M., et al. (2011). Endothelium-protective sphingosine-1-phosphate provided by HDL-associated apolipoprotein M. Proc. Natl. Acad. Sci. U.S.A. 108, 9613–9618. doi: 10.1073/pnas.1103187108
Chun, J., Weiner, J. A., Fukushima, N., Contos, J. J., Zhang, G., Kimura, Y., et al. (2000). Neurobiology of receptor-mediated lysophospholipid signaling - From the first lysophospholipid receptor to roles in nervous system function and development. Ann. N. Y. Acad. Sci. 905, 110–117. doi: 10.1111/j.1749-6632.2000.tb06543.x
Ciana, P., Fumagalli, M., Trincavelli, M. L., Verderio, C., Rosa, P., Lecca, D., et al. (2006). The orphan receptor GPR17 identified as a new dual uracil nucleotides/cysteinyl-leukotrienes receptor. EMBO J. 25, 4615–4627. doi: 10.1038/sj.emboj.7601341
Coelho, R. P., Payne, S. G., Bittman, R., Spiegel, S., and Sato-Bigbee, C. (2007). The immunomodulator FTY720 has a direct cytoprotective effect in oligodendrocyte progenitors. J. Pharmacol. Exp. Ther. 323, 626–635. doi: 10.1124/jpet.107.123927
Cohen, J. A., Barkhof, F., Comi, G., Hartung, H., Khatri, O. B., Montalban, X., et al. (2010). Oral fingolimod or intramuscular interferon for relapsing multiple sclerosis. N. Engl. J. Med. 362, 402–415.
Cohen, J. A., and Chun, J. (2011). Mechanisms of Fingolimod’s efficacy and adverse effects in multiple sclerosis. Ann. Neurol. 69, 759–777. doi: 10.1002/ana.22426
Colotta, V., Lenzi, O., Catarzi, D., Varano, F., Squarcialupi, L., Costagli, C., et al. (2012). 3-Hydroxy-1H-quinazoline-2,4-dione derivatives as new antagonists at ionotropic glutamate receptors: molecular modeling and pharmacological studies. Eur. J. Med. Chem. 54, 470–482. doi: 10.1016/j.ejmech.2012.05.036
Constantinescu, C. S., Farooqi, N., O’Brien, K., and Gran, B. (2011). Experimental autoimmune encephalomyelitis (EAE) as a model for multiple sclerosis (MS). Br. J. Pharmacol. 164, 1079–1106. doi: 10.1111/j.1476-5381.2011.01302.x
Coppi, E., Cellai, L., Maraula, G., Dettori, I., Melani, A., Pugliese, A. M., et al. (2015). Role of adenosine in oligodendrocyte precursor maturation. Front. Cell. Neurosci. 9:155. doi: 10.3389/fncel.2015.00155
Coppi, E., Cellai, L., Maraula, G., Pugliese, A. M., and Pedata, F. (2013a). Adenosine A(2A) receptors inhibit delayed rectifier potassium currents and cell differentiation in primary purified oligodendrocyte cultures. Neuropharmacology 73, 301–310. doi: 10.1016/j.neuropharm.2013.05.035
Coppi, E., Maraula, G., Fumagalli, M., Failli, P., Cellai, L., Bonfanti, E., et al. (2013b). UDP-glucose enhances outward K plus currents necessary for cell differentiation and stimulates cell migration by activating the GPR17 receptor in oligodendrocyte precursors. Glia 61, 1155–1171. doi: 10.1002/glia.22506
Coppi, E., Cherchi, F., Fusco, I., Dettori, I., Gaviano, L., Magni, G., et al. (2020a). Adenosine A(2B) receptors inhibit K+ currents and cell differentiation in cultured oligodendrocyte precursor cells and modulate sphingosine-1-phosphate signaling pathway. Biochem. Pharmacol. 177:113956. doi: 10.1016/j.bcp.2020.113956
Coppi, E., Dettori, I., Cherchi, F., Bulli, I., Venturini, M., Lana, D., et al. (2020b). A(2B) adenosine receptors: when outsiders may become an attractive target to treat brain ischemia or Demyelination. Int. J. Mol. Sci. 21:9697. doi: 10.3390/ijms21249697
Coppi, E., Dettori, I., Cherchi, F., Bulli, I., Venturini, M., Pedata, F., et al. (2021). New insight into the role of adenosine in demyelination, stroke and neuropathic pain. Front. Pharmacol. 11:625662. doi: 10.3389/fphar.2020.625662
Coppi, E., Pedata, F., and Gibb, A. J. (2012). P2Y(1) receptor modulation of Ca2+-activated K+ currents in medium-sized neurons from neonatal rat striatal slices. J. Neurophysiol. 107, 1009–1021. doi: 10.1152/jn.00816.2009
Corradetti, R., Lo Conte, G., Moroni, F., Passani, B. M., and Pepeu, G. (1984). Adenosine decreases aspartate and glutamate release from rat hippocampal slices. Eur. J. Pharmacol. 104, 19–26. doi: 10.1016/0014-2999(84)90364-9
Courtney, A. M., Treadaway, K., Remington, G., and Frohman, E. (2009). Multiple sclerosis. Med. Clin. North Am. 93, 451–476.
Cui, Q. L., Fang, J., Kennedy, T. E., Almazan, G., and Antel, J. P. (2014). Role of p38MAPK in S1P receptor-mediated differentiation of human oligodendrocyte progenitors. Glia 62, 1361–1375. doi: 10.1002/glia.22688
Cyster, J. G., and Schwab, S. R. (2012). Sphingosine-1-phosphate and lymphocyte egress from lymphoid organs. Ann. Rev. Immunol. 30, 69–94. doi: 10.1146/annurev-immunol-020711-075011
Dawson, M. R. L., Polito, A., Levine, J. M., and Reynolds, R. (2003). NG2-expressing glial progenitor cells: an abundant and widespread population of cycling cells in the adult rat CNS. Mol. Cell. Neurosci. 24, 476–488. doi: 10.1016/s1044-7431(03)00210-0
de Castro, F., and Bribian, A. (2005). The molecular orchestra of the migration of oligodendrocyte precursors during development. Brain Res. Rev. 49, 227–241. doi: 10.1016/j.brainresrev.2004.12.034
Donati, C., Cencetti, F., and Bruni, P. (2013). New insights into the role of sphingosine 1-phosphate and lysophosphatidic acid in the regulation of skeletal muscle cell biology. Biochim. Biophys. Acta 1831, 176–184. doi: 10.1016/j.bbalip.2012.06.013
Donati, C., Meacci, E., Nuti, F., Becciolini, L., Farnararo, M., and Bruni, P. (2004). Sphingosine 1-phosphate regulates myogenic differentiation: a major role for S1P(2) receptor. FASEB J. 18, 449–451.
Donati, C., Nincheri, P., Cencetti, F., Rapizzi, E., Farnararo, M., and Bruni, P. (2007). Tumor necrosis factor-alpha exerts pro-myogenic action in C2C12 myoblasts dvia sphingosine kinase/S1P(2) signaling. FEBS Lett. 581, 4384–4388. doi: 10.1016/j.febslet.2007.08.007
Dunwiddie, T. V. (1984). Interactions between the effects of adenosine and calcium on synaptic responses in rat hippocampus in vitro. J. Physiol. 350, 545–559. doi: 10.1113/jphysiol.1984.sp015217
Eckle, T., Faigle, M., Grenz, A., Laucher, S., Thompson, L. F., and Eltzschig, H. K. (2008). A2B adenosine receptor dampens hypoxia-induced vascular leak. Blood 111, 2024–2035. doi: 10.1182/blood-2007-10-117044
Emery, B. (2010). Regulation of oligodendrocyte differentiation and Myelination. Science 330, 779–782. doi: 10.1126/science.1190927
Feoktistov, I., Polosa, R., Holgate, S. T., and Biaggioni, I. (1998). Adenosine A(2B) receptors: a novel therapeutic target in asthma? Trends Pharmacol. Sci. 19, 148–153. doi: 10.1016/s0165-6147(98)01179-1
Fields, R. D. (2004). Volume transmission in activity-dependent regulation of myelinating glia. Neurochem. Int. 45, 503–509. doi: 10.1016/j.neuint.2003.11.015
Fields, R. D., and Burnstock, G. (2006). Purinergic signalling in neuron-glia interactions. Nat. Rev. Neurosci. 7, 423–436.
Fields, R. D., and Stevens-Graham, B. (2002). Neuroscience - new insights into neuron-glia communication. Science 298, 556–562. doi: 10.1126/science.298.5593.556
Foster, C. A., Howard, L. M., Schweitzer, A., Persohn, E., Hiestand, P. C., Balatoni, B., et al. (2007). Brain penetraGessiion of the oral immunomodulatory drug FTY720 and its phosphorylation in the central nervous system during experimental autoimmune encephalomyelitis: consequences for mode of action in multiple sclerosis. J. Pharmacol. Exp. Ther. 323, 469–476. doi: 10.1124/jpet.107.127183
Franklin, R. J. M., and Ffrench-Constant, C. (2017). Regenerating CNS myelin - from mechanisms to experimental medicines. Nat. Rev. Neurosci. 18, 753–769. doi: 10.1038/nrn.2017.136
Fredholm, B. B., Ijzerman, A. P., Jacobson, K. A., Linden, J., and Muller, C. E. (2011). International union of basic and clinical pharmacology. LXXXI. nomenclature and classification of adenosine receptors-an update. Pharmacol. Rev. 63, 1–34. doi: 10.1124/pr.110.003285
Fujino, M., Funeshima, N., Kitazawa, Y., Kimura, H., Amemiya, H., Suzuki, S., et al. (2003). Amelioration of experimental autoimmune encephalomyelitis in lewis rats by FTY720 treatment. J. Pharmacol. Exp. Ther. 305, 70–77. doi: 10.1124/jpet.102.045658
Fukumoto, K., Mizoguchi, H., Takeuchi, H., Horiuchi, H., Kawanokuchi, J., Jin, S. J., et al. (2014). Fingolimod increases brain-derived neurotrophic factor levels and ameliorates amyloid beta-induced memory impairment. Behav. Brain Res. 268, 88–93. doi: 10.1016/j.bbr.2014.03.046
Fumagalli, M., Daniele, S., Lecca, D., Lee, P. R., Parravicini, C., Fields, D. R., et al. (2011). Phenotypic changes, signaling pathway, and functional correlates of GPR17-expressing neural precursor cells during oligodendrocyte differentiation. J. Biol. Chem. 286, 10593–10604. doi: 10.1074/jbc.m110.162867
Fusco, I., Cherchi, F., Catarzi, D., Colotta, V., Varano, F., Pedata, F., et al. (2019). Functional characterization of a novel adenosine A(2B) receptor agonist on short-term plasticity and synaptic inhibition during oxygen and glucose deprivation in the rat CA1 hippocampus. Brain Res. Bull. 151, 174–180. doi: 10.1016/j.brainresbull.2019.05.018
Fusco, I., Ugolini, F., Lana, D., Coppi, E., Dettori, I., Gaviano, L., et al. (2018). The selective antagonism of adenosine A(2B) receptors reduces the synaptic failure and neuronal death induced by oxygen and glucose deprivation in rat CA1 hippocampus in vitro. Front. Pharmacol. 9:399. doi: 10.3389/fphar.2018.00399
Fyrst, H., and Saba, J. D. (2010). An update on sphingosine-1-phosphate and other sphingolipid mediators. Nat. Chem. Biol. 6, 489–497. doi: 10.1038/nchembio.392
Gallo, V., Zhou, J. M., McBain, C. J., Wright, P., Knutson, P. L., and Armstrong, R. C. (1996). Oligodendrocyte progenitor cell proliferation and lineage progression are regulated by glutamate receptor-mediated K+ channel block. J. Neurosci. 16, 2659–2670. doi: 10.1523/jneurosci.16-08-02659.1996
Galvani, S., Sanson, M., Blaho, V. A., Swendeman, S. L., Obinata, H., Conger, H., et al. (2015). HDL-bound sphingosine 1-phosphate acts as a biased agonist for the endothelial cell receptor S1P(1) to limit vascular inflammation. Sci. Signal. 8:ra79. doi: 10.1126/scisignal.aaa2581
Gao, P., Peterson, Y. K., Smith, R. A., and Smith, C. D. (2012). Characterization of isoenzyme-selective inhibitors of human sphingosine kinases. PLoS One 7:e011543. doi: 10.1371/journal.pone.0044543
Gard, A. L., and Pfeiffer, S. E. (1990). 2 proliferative stages of the oligodendrocyte lineage (a2b5+o4- and o4+galc-) under different mitogenic control. Neuron 5, 615–625. doi: 10.1016/0896-6273(90)90216-3
Gault, C. R., Obeid, L. M., and Hannun, Y. A. (2010). An overview of sphingolipid metabolism: from synthesis to breakdown. Adv. Exp. Med. Biol. 688, 1–23. doi: 10.1007/978-1-4419-6741-1_1
Gautier, H. O. B., Evans, K. A., Volbracht, K., James, R., Sitnikov, S., Lundgaard, I., et al. (2015). Neuronal activity regulates remyelination via glutamate signalling to oligodendrocyte progenitors. Nat. Commun. 6:8518.
Gergely, P., Nuesslein-Hildesheim, B., Guerini, D., Brinkmann, V., Traebert, M., Bruns, C., et al. (2012). The selective sphingosine 1-phosphate receptor modulator BAF312 redirects lymphocyte distribution and has species-specific effects on heart rate. Br. J. Pharmacol. 167, 1035–1047. doi: 10.1111/j.1476-5381.2012.02061.x
Gessi, S., Varani, K., Merighi, S., Cattabriga, E., Pancaldi, C., Szabadkai, Y., et al. (2005). Expression, pharmacological profile, and functional coupling of A(2B) receptors in a recombinant system and in peripheral blood cells using a novel selective antagonist radioligand, H-3 MRE 2029-F20. Mol. Pharmacol. 67, 2137–2147. doi: 10.1124/mol.104.009225
Gessiaa, S., Merighi, S., Varani, K., Leung, E., Mac Lennan, S., and Borea, P. A. (2008). The A(3) adenosine receptor: an enigmatic player in cell biology. Pharmacol. Ther. 117, 123–140. doi: 10.1016/j.pharmthera.2007.09.002
Goncalves, F. Q., Pires, J., Pliassova, A., Beleza, R., Lemos, C., Marques, J. M., et al. (2015). Adenosine A(2b) receptors control A(1) receptor-mediated inhibition of synaptic transmission in the mouse hippocampus. Eur. J. Neurosci. 41, 876–886.
Goncalves, M. L., and Ribeiro, J. A. (1996). Adenosine A(2) receptor activation facilitates Ca-45(2+) uptake by rat brain synaptosomes. Eur. J. Pharmacol. 310, 257–261. doi: 10.1016/0014-2999(96)00383-4
Gonzalez-Fernandez, E., Sanchez-Gomez, M. V., Perez-Samartin, A., Arellano, R. O., and Matute, C. (2014). A(3) Adenosine receptors mediate oligodendrocyte death and ischemic damage to optic nerve. Glia 62, 199–216. doi: 10.1002/glia.22599
Graeler, M., and Goetzl, E. J. (2002). Activation-regulated expression and chemotactic function of sphingosine 1-phosphate receptors in mouse splenic T cells. FASEB J. 16, 1874–1878. doi: 10.1096/fj.02-0548com
Graler, M. H., and Goetzl, E. J. (2004). The immunosuppressant FTY720 down-regulates sphingosine 1-phosphate G protein-coupled receptors. FASEB J. 18, 551–553. doi: 10.1096/fj.03-0910fje
Grinspan, J. (2002). Cells and signaling in oligodendrocyte development. J. Neuropathol. Exp. Neurol. 61, 297–306. doi: 10.1093/jnen/61.4.297
Gutman, G. A., Chandy, K. G., Grissmer, S., Lazdunski, M., McKinnon, D., Pardo, L. A., et al. (2005). International Union of Pharmacology. LIII. Nomenclature and molecular relationships of voltage-gated potassium channels. Pharmacol. Rev. 57, 473–508. doi: 10.1124/pr.57.4.10
Hait, N. C., Allegood, J., Maceyka, M., Strub, G. M., Harikumar, K. B., Singh, S. K., et al. (2009). Regulation of Histone acetylation in the nucleus by sphingosine-1-phosphate. Science 325, 1254–1257. doi: 10.1126/science.1176709
Hait, N. C., Sarkar, S., Le Stunff, H., Mikami, A., Maceyka, M., Milstien, S., et al. (2005). Role of sphingosine kinase 2 in cell migration toward epidermal growth factor. J. Biol. Chem. 280, 29462–29469. doi: 10.1074/jbc.m502922200
Hajny, S., and Christoffersen, C. (2017). A novel perspective on the ApoM-S1P Axis, highlighting the metabolism of ApoM and its role in liver fibrosis and neuroinflammation. Int. J. Mol. Sci. 18:1636. doi: 10.3390/ijms18081636
Hanada, K. (2003). Serine palmitoyltransferase, a key enzyme of sphingolipid metabolism. Bioch. Biophys. Acta. 1632, 16–30. doi: 10.1016/s1388-1981(03)00059-3
Hannun, Y. A., and Obeid, L. M. (2008). Principles of bioactive lipid signalling: lessons from sphingolipids. Nat. Rev. Mol. Cell Biol. 9, 139–150. doi: 10.1038/nrm2329
Hasko, G., Linden, J., Cronstein, B., and Pacher, P. (2008). Adenosine receptors: therapeutic aspects for inflammatory and immune diseases. Nat. Rev. Drug Discov. 7, 759–770.
Hisano, Y., Kobayashi, N., Yamaguchi, A., and Nishi, T. (2012). Mouse SPNS2 functions as a sphingosine-1-phosphate transporter in vascular endothelial cells. Plos One 7:e38941. doi: 10.1371/journal.pone.0038941
Hla, T., Venkataraman, K., and Michaud, J. (2008). The vascular S1P gradient - cellular sources and biological significance. Biochim. Biophys. Acta 1781, 477–482. doi: 10.1016/j.bbalip.2008.07.003
Hou, H. Q., Cao, R. J., Miao, J., Sun, Y. F., Liu, X. Q., Song, X. J., et al. (2016). Fingolimod ameliorates the development of experimental autoimmune encephalomyelitis by inhibiting Akt-mTOR axis in mice. Int. Immunopharmacol. 30, 171–178. doi: 10.1016/j.intimp.2015.11.024
Hu, Y. H., Lee, X. H., Ji, B. X., Guckian, K., Apicco, D., Pepinsky, R. B., et al. (2011). Sphingosine 1-phosphate receptor modulator fingolimod (FTY720) does not promote remyelination in vivo. Mol. Cell. Neurosci. 48, 72–81. doi: 10.1016/j.mcn.2011.06.007
Igarashi, N., Okada, T., Hayashi, S., Fujita, T., Jahangeer, S., and Nakamura, S. (2003). Sphingosine kinase 2 is a nuclear protein and inhibits DNA synthesis. J. Biol. Chem. 278, 46832–46839. doi: 10.1074/jbc.m306577200
Im, D. S., Heise, C. E., Ancellin, N., O’Dowd, B. F., Shei, G. J., Heavens, R. P., et al. (2000). Characterization of a novel sphingosine 1-phosphate receptor, Edg-8. J. Biol. Chem. 275, 14281–14286. doi: 10.1074/jbc.275.19.14281
Imamura, T., Ohgane, J., Ito, S., Ogawa, T., Hattori, N., Tanaka, S., et al. (2001). CpG island of rat sphingosine kinase-1 gene: tissue-dependent DNA methylation status and multiple alternative first exons. Genomics 76, 117–125. doi: 10.1006/geno.2001.6607
Ingwersen, J., Wingerath, B., Graf, J., Lepka, K., Hofrichter, M., Schröter, F., et al. (2016). Dual roles of the adenosine A2a receptor in autoimmune neuroinflammation. J. Neuroinflam. 13:48.
Inoue, K. (2017). Molecular basis of nucleobase transport systems in mammals. Biol. Pharm. Bull. 40, 1130–1138. doi: 10.1248/bpb.b17-00374
Ishii, I., Fukushima, N., Ye, X. Q., and Chun, J. (2004). Lysophospholipid receptors: signaling and biology. Annu. Rev. Biochem. 73, 321–354.
Jacobson, K. A., and Gao, Z. G. (2006). Adenosine receptors as therapeutic targets. Nat. Rev. Drug Discov. 5, 247–264.
Jaillard, C., Harrison, S., Stankoff, B., Aigrot, M. S., Calver, A. R., Duddy, G., et al. (2005). Edg8/S1P5: an oligodendroglial receptor with dual function on process retraction and cell survival. J. Neurosci. 25, 1459–1469. doi: 10.1523/jneurosci.4645-04.2005
Johnson, K. R., Becker, K. P., Facchinetti, M. M., Hannun, Y. A., and Obeid, L. M. (2002). PKC-dependent activation of sphingosine kinase 1 and translocation to the plasma membrane - Extracellular release of sphingosine-1-phosphate induced by phorbol 12-myristate 13-acetate (PMA). J. Biol. Chem. 277, 35257–35262.
Johnston, J. B., Silva, C., Gonzalez, G., Holden, J., Warren, K. G., Metz, L. M., et al. (2001). Diminished adenosine A1 receptor expression on macrophages in brain and blood of patients with multiple sclerosis. Ann. Neurol. 49, 650–658. doi: 10.1002/ana.1007
Jung, C. G., Kim, H. J., Miron, V. E., Cook, S., Kennedy, T. E., Foster, C. A., et al. (2007). Functional consequences of S1P receptor modulation in rat oligodendroglial lineage cells. Glia 55, 1656–1667. doi: 10.1002/glia.20576
Jung, M., Sommer, I., Schachner, M., and Nave, K. A. (1996). Monoclonal antibody O10 defines a conformationally sensitive cell-surface epitope of proteolipid protein (PLP): evidence that PLP misfolding underlies dysmyelination in mutant mice. J. Neurosci. 16, 7920–7929. doi: 10.1523/jneurosci.16-24-07920.1996
Kappos, L., Radue, E. W., O’Connor, P., Polman, C., Hohlfeld, R., Calabresi, P., et al. (2010). A Placebo-controlled trial of oral fingolimod in relapsing multiple sclerosis. N. Engl. J. Med. 362, 387–401.
Karadottir, R., Hamilton, N. B., Bakiri, Y., and Attwell, D. (2008). Spiking and nonspiking classes of oligodendrocyte precursor glia in CNS white matter. Nat. Neurosci. 11, 450–456. doi: 10.1038/nn2060
Kataoka, H., Sugahara, K., Shimano, K., Teshima, K., Koyama, M., Fukunari, A., et al. (2005). FTY720, Sphingosine 1-phosphate receptor modulator, ameliorates experimental autoimmune encephalomyelitis by inhibition of T cell infiltration. Cell. Mol. Immunol. 2, 439–448.
Kettenmann, H., Blankenfeld, G. V., and Trotter, J. (1991). Physiological properties of oligodendrocytes during development. Ann. N. Y. Acad. Sci. 633, 64–77. doi: 10.1111/j.1749-6632.1991.tb15596.x
Kharel, Y., Lee, S., Snyder, A. H., Heasley-O’neill, S. L., Morris, M. A., Setiady, Y., et al. (2005). Sphingosine kinase 2 is required for modulation of lymphocyte traffic by FTY720. J. Biol. Chem. 280, 36865–36872. doi: 10.1074/jbc.m506293200
Kim, H. J., Miron, V. E., Dukala, D., Proia, R. L., Ludwin, S. K., Traka, M., et al. (2011). Neurobiological effects of sphingosine 1-phosphate receptor modulation in the cuprizone model. FASEB J. 25, 1509–1518. doi: 10.1096/fj.10-173203
Kim, S., Bielawski, J., Yang, H., Kong, Y., Zhou, B. Y., and Li, J. R. (2018). Functional antagonism of sphingosine-1-phosphate receptor 1 prevents cuprizone-induced demyelination. Glia 66, 654–669. doi: 10.1002/glia.23272
Knutson, P., Ghiani, C. A., Zhou, J. M., Gallo, V., and McBain, C. J. (1997). K+ channel expression and cell proliferation are regulated by intracellular sodium and membrane depolarization in oligodendrocyte progenitor cells. J. Neurosci. 17, 2669–2682. doi: 10.1523/jneurosci.17-08-02669.1997
Kobayashi, N., Kawasaki-Nishi, S., Otsuka, M., Hisano, Y., Yamaguchi, A., and Nishi, T. (2018). MFSD2B is a sphingosine 1-phosphate transporter in erythroid cells. Sci. Rep. 8:4969.
Kohno, M., Momoi, M., Oo, M. L., Paik, J. H., Lee, Y. M., Venkataraman, K., et al. (2006). Intracellular role for sphingosine kinase 1 in intestinal adenoma cell proliferation. Mol. Cell. Biol. 26, 7211–7223. doi: 10.1128/mcb.02341-05
Koscso, B., Csoka, B., Pacher, P., and Hasko, G. (2011). Investigational A(3) adenosine receptor targeting agents. Expert Opin. Investig. Drugs 20, 757–768. doi: 10.1517/13543784.2011.573785
Ksiazek, M., Chacinska, M., Chabowski, A., and Baranowski, M. (2015). Sources, metabolism, and regulation of circulating sphingosine-1-phosphate. J. Lipid Res. 56, 1271–1281. doi: 10.1194/jlr.r059543
Latini, S., Bordoni, F., Pedata, F., and Corradetti, R. (1999). Extracellular adenosine concentrations during in vitro ischaemia in rat hippocampal slices. Br. J. Pharmacol. 127, 729–739. doi: 10.1038/sj.bjp.0702591
Latini, S., and Pedata, F. (2001). Adenosine in the central nervous system: release mechanisms and extracellular concentrations. J. Neurochem. 79, 463–484. doi: 10.1046/j.1471-4159.2001.00607.x
Lecca, D., Trincavelli, M. L., Gelosa, P., Sironi, L., Ciana, P., Fumagalli, M., et al. (2008). The recently identified P2Y-Like receptor GPR17 is a sensor of brain damage and a new target for brain repair. PLoS One 3:e3579. doi: 10.1371/journal.pone.0003579
Levi, G., Gallo, V., and Ciotti, M. T. (1986). Bipotential precursors of putative fibrous astrocytes and oligodendrocytes in rat cerebellar cultures express distinct surface-features and neuron-like gamma-aminobutyric-acid transport. Proc. Natl. Acad. Sci. U.S.A. 83, 1504–1508. doi: 10.1073/pnas.83.5.1504
Levine, J. M., Reynolds, R., and Fawcett, J. W. (2001). The oligodendrocyte precursor cell in health and disease. Trends Neurosci. 24, 39–47. doi: 10.1016/s0166-2236(00)01691-x
Ligon, K. L., Kesari, S., Kitada, M., Sun, T., Arnett, H. A., Alberta, J. A., et al. (2006). Development of NG2 neural progenitor cells requires Olig gene function. Proc. Natl. Acad. Sci. U.S.A. 103, 7853–7858. doi: 10.1073/pnas.0511001103
Liu, G. P., Zhang, W., Guo, J., Kong, F. Q., Zhou, S. M., Chen, S., et al. (2018). Adenosine binds predominantly to adenosine receptor A1 subtype in astrocytes and mediates an immunosuppressive effect. Brain Res. 1700, 47–55. doi: 10.1016/j.brainres.2018.06.021
Liu, Y., Alahiri, M., Ulloa, B., Xie, B. X., and Sadiq, S. A. (2018). Adenosine A2A receptor agonist ameliorates EAE and correlates with Th1 cytokine-induced blood brain barrier dysfunction via suppression of MLCK signaling pathway. Immun. Inflamm. Dis. 6, 72–80. doi: 10.1002/iid3.187
Liu, H., Chakravarty, D., Maceyka, M., Milstien, S., and Spiegel, S. (2002). Sphingosine kinases: a novel family of lipid kinases. Prog. Nucleic Acid Res. Mol. Biol. 71, 493–511. doi: 10.1016/s0079-6603(02)71049-0
Liu, H., Toman, R. E., Goparaju, S. K., Maceyka, M., Nava, V. E., Sankala, H., et al. (2003). Sphingosine kinase type 2 is a putative BH3-only protein that induces apoptosis. J. Biol. Chem. 278, 40330–40336. doi: 10.1074/jbc.m304455200
Liu, H., Zhang, Y. J., Wu, H. Y., D’Alessandro, A., Yegutkin, G. G., Song, A., et al. (2016). Beneficial role of erythrocyte adenosine A2B receptor-mediated AMP-activated protein kinase activation in high-altitude hypoxia. Circulation 134, 405–421. doi: 10.1161/circulationaha.116.021311
Liu, Y. Q., Ma, Y. Y., Du, B. Y., Wang, Y. T., Yang, G. Y., and Bi, X. Y. (2020). Mesenchymal stem cells attenuated blood-brain barrier disruption via downregulation of aquaporin-4 expression in EAE mice. Mol. Neurobiol. 57, 3891–3901. doi: 10.1007/s12035-020-01998-z
Lopes, L. V., Cunha, R. A., Kull, B., Fredholm, B. B., and Ribeiro, J. A. (2002). Adenosine A(2A) receptor facilitation of hippocampal synaptic transmission is dependent on tonic A(1) receptor inhibition. Neuroscience 112, 319–329. doi: 10.1016/s0306-4522(02)00080-5
Lundgaard, I., Luzhynskaya, A., Stockley, J. H., Wang, Z., Evans, K. A., Swire, M., et al. (2013). Neuregulin and BDNF induce a switch to NMDA receptor-dependent Myelination by Oligodendrocytes. Plos Biol. 11:e1001743. doi: 10.1371/journal.pbio.1001743
Maceyka, M., Harikumar, K. B., Milstien, S., and Spiegel, S. (2012). Sphingosine-1-phosphate signaling and its role in disease. Tren. Cell Biol. 22, 50–60. doi: 10.1016/j.tcb.2011.09.003
Maceyka, M., Sankala, H., Hait, N. C., Le Stunff, H., Liu, H., Toman, R., et al. (2005). SphK1 and SphK2, sphingosine kinase isoenzymes with opposing functions in sphingolipid metabolism. J. Biol. Chem. 280, 37118–37129. doi: 10.1074/jbc.m502207200
Malerba, F., Paoletti, F., Ercole, B. B., Materazzi, S., Nassini, R., Coppi, E., et al. (2015). Functional characterization of human ProNGF and NGF mutants: identification of NGF P61SR100E as a “Painless” lead investigational candidate for therapeutic applications. PLoS One 10:e0136425. doi: 10.1371/journal.pone.0136425
Manalo, J. M., Liu, H., Ding, D. L., Hicks, J., Sun, H., Salvi, R., et al. (2020). Adenosine A2B receptor: a pathogenic factor and a therapeutic target for sensorineural hearing loss. FASEB J. 34, 15771–15787. doi: 10.1096/fj.202000939r
Mandala, S., Hajdu, R., Bergstrom, J., Quackenbush, E., Xie, J., Milligan, J., et al. (2002). Alteration of lymphocyte trafficking by sphingosine-1-phosphate receptor agonists. Science 296, 346–349. doi: 10.1126/science.1070238
Maraula, G., Lana, D., Coppi, E., Gentile, F., Mello, T., Melani, A., et al. (2014). The selective antagonism of P2X7 and P2Y1 receptors prevents synaptic failure and affects cell proliferation induced by oxygen and glucose deprivation in rat dentate gyrus. PLoS One 9:e115273. doi: 10.1371/journal.pone.0115273
Maraula, G., Traini, C., Mello, T., Coppi, E., Galli, A., Pedata, F., et al. (2013). Effects of oxygen and glucose deprivation on synaptic transmission in rat dentate gyrus: role of A(2A) adenosine receptors. Neuropharmacology 67, 511–520. doi: 10.1016/j.neuropharm.2012.12.002
Matloubian, M., Lo, C. G., Cinamon, G., Lesneski, M. J., Xu, Y., Brinkmann, V., et al. (2004). Lymphocyte egress from thymus and peripheral lymphoid organs is dependent on S1P receptor 1. Nature 427, 355–360. doi: 10.1038/nature02284
Mayne, M., Shepel, P. N., Jiang, Y., Geiger, J. D., and Power, C. (1999). Dysregulation of adenosine A(1) receptor-mediated cytokine expression in peripheral blood mononuclear cells from multiple sclerosis patients. Ann. Neurol. 45, 633–639. doi: 10.1002/1531-8249(199905)45:5<633::aid-ana12>3.0.co;2-x
Mehling, M., Brinkmann, V., Antel, J., Bar-Or, A., Goebels, N., Vedrine, C., et al. (2008). FTY720 therapy exerts differential effects on T cell subsets in multiple sclerosis. Neurology 71, 1261–1267. doi: 10.1212/01.wnl.0000327609.57688.ea
Melani, A., Cipriani, S., Vannucchi, M. G., Nosi, D., Donati, C., Bruni, P., et al. (2009). Selective adenosine A(2a) receptor antagonism reduces JNK activation in oligodendrocytes after cerebral ischaemia. Brain 132, 1480–1495. doi: 10.1093/brain/awp076
Michaud, J., Kohno, M., Proia, R. L., and Hla, T. (2006). Normal acute and chronic inflammatory responses in sphingosine kinase 1 knockout mice. FEBS Lett. 580, 4607–4612. doi: 10.1016/j.febslet.2006.07.035
Mills, J. H., Alabanza, L. M., Mahamed, D. A., and Bynoe, M. S. (2012). Extracellular adenosine signaling induces CX3CL1 expression in the brain to promote experimental autoimmune encephalomyelitis. J. Neuroinflamm. 9:193.
Miron, V. E., Hall, J. A., Kennedy, T. E., Soliven, B., and Antel, J. P. (2008a). Cyclical and dose-dependent responses of adult human mature oligodendrocytes to fingolimod. Am. J. Pathol. 173, 1143–1152. doi: 10.2353/ajpath.2008.080478
Miron, V. E., Jung, C. G., Kim, H. J., Kennedy, T. E., Soliven, B., and Antel, J. P. (2008b). FTY720 modulates human oligodendrocyte progenitor process extension and survival. Ann. Neurol. 63, 61–71. doi: 10.1002/ana.21227
Miron, V. E., Schubart, A., and Antel, J. P. (2008c). Central nervous system-directed effects of FTY720 (fingolimod). J. Neurol. Sci. 274, 13–17. doi: 10.1016/j.jns.2008.06.031
Miron, V. E., Ludwin, S. K., Darlington, P. J., Jarjour, A. A., Soliven, B., Kennedy, T. E., et al. (2010). Fingolimod (FTY720) enhances remyelination following demyelination of organotypic cerebellar slices. Am. J. Pathol. 176, 2682–2694. doi: 10.2353/ajpath.2010.091234
Mitra, P., Oskeritzian, C. A., Payne, S. G., Beaven, M. A., Milstien, S., and Spiegel, S. (2006). Role of ABCO in export of sphingosine-1-phosphate from mast cells. Proc. Natl. Acad. Sci. U.S.A. 103, 16394–16399. doi: 10.1073/pnas.0603734103
Mizugishi, K., Yamashita, T., Olivera, A., Miller, G. F., Spiegel, S., and Proia, R. L. (2005). Essential role for sphingosine kinases in neural and vascular development. Mol. Cell. Biol. 25, 11113–11121. doi: 10.1128/mcb.25.24.11113-11121.2005
Montesinos, M. C., Yap, J. S., Desai, A., Posadas, I., McCrary, C. T., and Cronstein, B. N. (2000). Reversal of the antiinflammatory effects of methotrexate by the nonselective adenosine receptor antagonists theophylline and caffeine - Evidence that the antiinflammatory effects of methotrexate are mediated via multiple adenosine receptors in rat adjuvant arthritis. Arthrit. Rheum. 43, 656–663. doi: 10.1002/1529-0131(200003)43:3<656::aid-anr23>3.0.co;2-h
Mutoh, T., Rivera, R., and Chun, J. (2012). Insights into the pharmacological relevance of lysophospholipid receptors. Br. J. Pharmacol. 165, 829–844. doi: 10.1111/j.1476-5381.2011.01622.x
Muzzi, M., Coppi, E., Pugliese, A. M., and Chiarugi, A. (2013). Anticonvulsant effect of AMP by direct activation of adenosine A1 receptor. Exp. Neurol. 250, 189–193. doi: 10.1016/j.expneurol.2013.09.010
Nave, K. A. (2010). Myelination and support of axonal integrity by glia. Nature 468, 244–252. doi: 10.1038/nature09614
Neubauer, H. A., Pham, D. H., Zebol, J. R., Moretti, P. A., Peterson, A. L., Leclercq, T. M., et al. (2016). An oncogenic role for sphingosine kinase 2. Oncotarget 7, 64886–64899.
Neumann, B., Segel, M., Chalut, K. J., and Franklin, R. J. M. (2019). Remyelination and ageing: Reversing the ravages of time. Mult. Scler. J. 25, 1835–1841. doi: 10.1177/1352458519884006
Novgorodov, A. S., El-Alwani, M., Bielawski, J., Obeid, L. M., and Gudz, T. I. (2007). Activation of sphingosine-1-phosphate receptor S1P5 inhibits oligodendrocyte progenitor migration. FASEB J. 21, 1503–1514. doi: 10.1096/fj.06-7420com
Nystad, A. E., Lereim, R. R., Wergeland, S., Oveland, E., Myhr, K. M., Bo, L., et al. (2020). Fingolimod downregulates brain sphingosine-1-phosphate receptor 1 levels but does not promote remyelination or neuroprotection in the cuprizone model. J. Neuroimmunol. 339:577091. doi: 10.1016/j.jneuroim.2019.577091
Obinata, H., Kuo, A., Wada, Y., Swendeman, S., Liu, C. H., Blaho, V. A., et al. (2019). Identification of ApoA4 as a sphingosine 1-phosphate chaperone in ApoM- and albumin-deficient mice S. J. Lipid Res. 60, 1912–1921. doi: 10.1194/jlr.ra119000277
Okada, T., Ding, G., Sonoda, H., Kajimoto, T., Haga, Y., Khosrowbeygi, A., et al. (2005). Involvement of N-terminal-extended form of sphingosine kinase 2 in serum-dependent regulation of cell proliferation and apoptosis. J. Biol. Chem. 280, 36318–36325. doi: 10.1074/jbc.m504507200
Okada, T., Kajimoto, T., Jahangeer, S., and Nakamura, S. (2009). Sphingosine kinase/sphingosine 1-phosphate signalling in central nervous system. Cell. Signal. 21, 7–13. doi: 10.1016/j.cellsig.2008.07.011
Olivera, A., and Spiegel, S. (2001). Sphingosine kinase: a mediator of vital cellular functions. Prostaglandins Other Lipid Mediat. 64, 123–134. doi: 10.1016/s0090-6980(01)00108-3
Othman, T., Yan, H. L., and Rrvkees, S. A. (2003). Oligodendrocytes express functional A1 adenosine receptors that stimulate cellular migration. Glia 44, 166–172. doi: 10.1002/glia.10281
Papadopoulos, D., Rundle, J., Patel, R., Marshall, I., Stretton, J., Eaton, R., et al. (2010). FTY720 Ameliorates MOG-induced experimental autoimmune encephalomyelitis by suppressing both cellular and humoral immune responses. J. Neurosci. Res. 88, 346–359. doi: 10.1002/jnr.22196
Pappu, R., Schwab, S. R., Cornelissen, I., Pereira, J. P., Regard, J. B., Xu, Y., et al. (2007). Promotion of lymphocyte egress into blood and lymph by distinct sources of sphingosine-1-phosphate. Science 316, 295–298. doi: 10.1126/science.1139221
Patel, J. R., and Klein, R. S. (2011). Mediators of oligodendrocyte differentiation during remyelination. FEBS Lett. 585, 3730–3737. doi: 10.1016/j.febslet.2011.04.037
Paugh, S. W., Payne, S. G., Barbour, S. E., Milstien, S., and Spiegel, S. (2003). The immunosuppressant FTY720 is phosphorylated by sphingosine kinase type 2. FEBS Lett. 554, 189–193. doi: 10.1016/s0014-5793(03)01168-2
Pedata, F., Melani, A., Pugliese, A. M., Coppi, E., Cipriani, S., and Traini, C. (2007). The role of ATP and adenosine in the brain under normoxic and ischemic conditions. Purinergic Signal. 3, 299–310. doi: 10.1007/s11302-007-9085-8
Pedata, F., Pugliese, A. M., Coppi, E., Dettori, I., Maraula, G., Cellai, L., et al. (2014). Adenosine A(2A) receptors modulate acute injury and neuroinflammation in brain ischemia. Med. Inflamm. 2014:805198.
Peterfreund, R. A., MacCollin, M., Gusella, J., and Fink, J. S. (1996). Characterization and expression of the human A2a adenosine receptor gene. J. Neurochem. 66, 362–368. doi: 10.1046/j.1471-4159.1996.66010362.x
Pitson, S. M., Moretti, P. A. B., Zebol, J. R., Xia, P., Gamble, J. R., Vadas, M. A., et al. (2000). Expression of a catalytically inactive sphingosine kinase mutant blocks agonist-induced sphingosine kinase activation - A dominant-negative sphingosine kinase. J. Biol. Chem. 275, 33945–33950. doi: 10.1074/jbc.m006176200
Popoli, P., and Pepponi, R. (2012). Potential therapeutic relevance of adenosine A(2B) and A(2A) receptors in the central nervous system. Cns Neurol. Disord. Drug Targets 11, 664–674. doi: 10.2174/187152712803581100
Pringle, N. P., Mudhar, H. S., Collarini, E. J., and Richardson, W. D. (1992). Pdgf receptors in the rat cns - during late neurogenesis, pdgf alpha-receptor expression appears to be restricted to glial-cells of the oligodendrocyte lineage. Development 115, 535–551. doi: 10.1242/dev.115.2.535
Proia, R. L., and Hla, T. (2015). Emerging biology of sphingosine-1-phosphate: its role in pathogenesis and therapy. J. Clin. Invest. 125, 1379–1387. doi: 10.1172/jci76369
Pyne, S., Adams, D. R., and Pyne, N. J. (2016). Sphingosine 1-phosphate and sphingosine kinases in health and disease: recent advances. Prog. Lipid Res. 62, 93–106. doi: 10.1016/j.plipres.2016.03.001
Qin, J. D., Berdyshev, E., Goya, J., Natarajan, V., and Dawson, G. (2010). Neurons and oligodendrocytes recycle Sphingosine 1-phosphate to ceramide significance for apoptosis and multiple sclerosis. J. Biol. Chem. 285, 14134–14143. doi: 10.1074/jbc.m109.076810
Rajasundaram, S. (2018). Adenosine A2A receptor signaling in the immunopathogenesis of experimental autoimmune encephalomyelitis. Front. Immunol. 9:402. doi: 10.3389/fimmu.2018.00402
Ramkumar, V., Stiles, G. L., Beaven, M. A., and Ali, H. (1993). The a(3) adenosine receptor is the unique adenosine receptor which facilitates release of allergic mediators in mast-cells. J. Biol. Chem. 268, 16887–16890. doi: 10.1016/s0021-9258(19)85277-8
Rissanen, E., Virta, J. R., Paavilainen, T., Tuisku, J., Helin, S., Luoto, P., et al. (2013). Adenosine A2A receptors in secondary progressive multiple sclerosis: a C-11 TMSX brain PET study. J. Cereb. Blood Flow Metab. 33, 1394–1401. doi: 10.1038/jcbfm.2013.85
Romanelli, E., Merkler, D., Mezydlo, A., Weil, M. T., Weber, M. S., Nikić, I., et al. (2016). Myelinosome formation represents an early stage of oligodendrocyte damage in multiple sclerosis and its animal model. Nat. Commun. 7:13275.
Saab, A. S., Tzvetavona, I. D., Trevisiol, A., Baltan, S., Dibaj, P., Kusch, K., et al. (2016). Oligodendroglial NMDA receptors regulate glucose import and axonal energy metabolism. Neuron 91, 119–132. doi: 10.1016/j.neuron.2016.05.016
Saini, H. S., Coelho, R. P., Goparaju, S. K., Jolly, P. S., Maceyka, M., Spiegel, S., et al. (2005). Novel role of sphingosine kinase 1 as a mediator of neurotrophin-3 action in oligodendrocyte progenitors. J. Neurochem. 95, 1298–1310. doi: 10.1111/j.1471-4159.2005.03451.x
Sarkar, S., Maceyka, M., Hait, N. C., Paugh, S. W., Sankala, H., Milstien, S., et al. (2005). Sphingosine kinase 1 is required for migration, proliferation and survival of MCF-7 human breast cancer cells. FEBS Lett. 579, 5313–5317. doi: 10.1016/j.febslet.2005.08.055
Schwab, S. R., Pereira, J. P., Matloubian, M., Xu, Y., Huang, Y., and Cyster, J. G. (2005). Lymphocyte sequestration through S1P lyase inhibition and disruption of S1P gradients. Science 309, 1735–1739. doi: 10.1126/science.1113640
Scolding, N. J., Frith, S., Linington, C., Morgan, B. P., Campbell, A. K., and Compston, D. A. S. (1989). Myelin-oligodendrocyte glycoprotein (mog) is a surface marker of oligodendrocyte maturation. J. Neuroimmunol. 22, 169–176. doi: 10.1016/0165-5728(89)90014-3
Shrager, P., and Novakovic, S. D. (1995). Control of myelination, axonal growth, and synapse formation in spinal-cord explants by ion channels and electrical-activity. Dev. Brain res. 88, 68–78. doi: 10.1016/0165-3806(95)00081-n
Simon, C., Gotz, M., and Dimou, L. (2011). Progenitors in the adult cerebral cortex: cell cycle properties and regulation by physiological stimuli and injury. Glia 59, 869–881. doi: 10.1002/glia.21156
Slowik, A., Schmidt, T., Beyer, C., Amor, S., Clarner, T., and Kipp, M. (2015). The sphingosine 1-phosphate receptor agonist FTY720 is neuroprotective after cuprizone-induced CNS demyelination. Br. J. Pharmacol. 172, 80–92. doi: 10.1111/bph.12938
Smith, P. A., Schmid, C., Zurbruegg, S., Jivkov, M., Doelemeyer, A., Theil, D., et al. (2018). Fingolimod inhibits brain atrophy and promotes brain-derived neurotrophic factor in an animal model of multiple sclerosis. J. Neuroimmunol. 318, 103–113. doi: 10.1016/j.jneuroim.2018.02.016
Soliven, B., Miron, V., and Chun, J. (2011). The neurobiology of sphingosine 1-phosphate signaling and sphingosine 1-phosphate receptor modulators. Neurology 76, S9–S14.
Soliven, B., Szuchet, S., Arnason, B. G. W., and Nelson, D. J. (1988). Forskolin and phorbol esters decrease the same k+ conductance in cultured oligodendrocytes. J. Memb. Biol. 105, 177–186. doi: 10.1007/bf02009170
Sontheimer, H., and Kettenmann, H. (1988). Heterogeneity of potassium currents in cultured oligodendrocytes. Glia 1, 415–420. doi: 10.1002/glia.440010609
Sontheimer, H., Trotter, J., Schachner, M., and Kettenmann, H. (1989). Channel expression correlates with differentiation stage during the development of oligodendrocytes from their precursor cells in culture. Neuron 2, 1135–1145. doi: 10.1016/0896-6273(89)90180-3
Spiegel, S., Maczis, M. A., Maceyka, M., and Milstien, S. (2019). New insights into functions of the sphingosine-1-phosphate transporter SPNS2. J. Lipid Res. 60, 484–489. doi: 10.1194/jlr.s091959
Spiegel, S., and Milstien, S. (2003). Sphingosine-1-phosphate: an enigmatic signalling lipid. Nat. Rev. Mol. Cell Biol. 4, 397–407. doi: 10.1038/nrm1103
Spitzer, S. O., Sitnikov, S., Kamen, Y., Evans, K. A., Kronenberg-Versteeg, D., Dietmann, S., et al. (2019). Oligodendrocyte progenitor cells become regionally diverse and heterogeneous with age. Neuron 101:459. doi: 10.1016/j.neuron.2018.12.020
Stellwagen, D., and Malenka, R. C. (2006). Synaptic scaling mediated by glial TNF-alpha. Nature 440, 1054–1059. doi: 10.1038/nature04671
Stevens, B., Porta, S., Haak, L. L., Gallo, V., and Fields, R. D. (2002). Adenosine: a neuron-glial transmitter promoting myelination in the CNS in response to action potentials. Neuron 36, 855–868.
Strub, G. M., Maceyka, M., Hait, N. C., Milstien, S., and Spiegel, S. (2010). Extracellular and intracellular actions of sphingosine-1-Phosphate. Adv. Exp. Med. Biols. 688, 141–155. doi: 10.1007/978-1-4419-6741-1_10
Strub, G. M., Paillard, M., Liang, J., Gomez, L., Allegood, J. C., Hait, N. C., et al. (2011). Sphingosine-1-phosphate produced by sphingosine kinase 2 in mitochondria interacts with prohibitin 2 to regulate complex IV assembly and respiration. FASEB J. 25, 600–612. doi: 10.1096/fj.10-167502
Szuchet, S., Nielsen, J. A., Lovas, G., Domowicz, M. S., de Velasco, J. M., Maric, D., et al. (2011). The genetic signature of perineuronal oligodendrocytes reveals their unique phenotype. Eur. J. Neurosci. 34, 1906–1922. doi: 10.1111/j.1460-9568.2011.07922.x
Taha, T. A., Kitatani, K., El-Alwani, M., Bielawski, J., Hannun, Y. A., and Obeid, L. M. (2005). Loss of sphingosine kinase-1 activates the intrinsic pathway of programmed cell death: modulation of sphingolipid levels and the induction of apoptosis. FASEB J. 19:482. doi: 10.1096/fj.05-4412fje
Takasugi, N., Sasaki, T., Suzuki, K., Osawa, S., Isshiki, H., Hori, Y., et al. (2011). BACE1 activity is modulated by cell-associated sphingosine-1-Phosphate. J. Neurosci. 31, 6850–6857. doi: 10.1523/jneurosci.6467-10.2011
Tsutsui, S., Schnermann, J., Noorbakhsh, F., Henry, S., Yong, V. W., Winston, B. W., et al. (2004). A1 adenosine receptor upregulation and activation attenuates neuroinflammation and demyelination in a model of multiple sclerosis. J. Neurosci. 24, 1521–1529. doi: 10.1523/jneurosci.4271-03.2004
Van Brocklyn, J. R., Jackson, C. A., Pearl, D. K., Kotur, M. S., Snyder, P. J., and Prior, T. W. (2005). Sphingosine kinase-1 expression correlates with poor survival of patients with glioblastoma multiforme: Roles of sphingosine kinase isoforms in growth of glioblastoma cell lines. J. Neuropathol. Exp. Neurol. 64, 695–705. doi: 10.1097/01.jnen.0000175329.59092.2c
van Doom, R., Pinheiro, M. A. L., Kooij, G., Lakeman, K., van het Hof, B., and van der Pol, S. M. (2012). Sphingosine 1-phosphate receptor 5 mediates the immune quiescence of the human brain endothelial barrier. J. Neuroinflamm. 9:133.
Varani, K., Padovan, M., Vincenzi, F., Targa, M., Trotta, F., Govoni, M., et al. (2011). A(2A) and A(3) adenosine receptor expression in rheumatoid arthritis: upregulation, inverse correlation with disease activity score and suppression of inflammatory cytokine and metalloproteinase release. Arthrit. Res. Ther. 13:R197.
Verkhratsky, A., and Burnstock, G. (2014). Biology of purinergic signalling: its ancient evolutionary roots, its omnipresence and its multiple functional significance. Bioessays 36, 697–705. doi: 10.1002/bies.201400024
Verkhratsky, A. N., Trotter, J., and Kettenmann, H. (1990). Cultured glial precursor cells from mouse cortex express 2 types of calcium currents. Neurosci. Lett. 112, 194–198. doi: 10.1016/0304-3940(90)90202-k
Vincenzi, F., Corciulo, C., Targa, M., Merighi, S., Gessi, S., Casetta, I., et al. (2013). Multiple sclerosis lymphocytes upregulate A(2A) adenosine receptors that are antiinflammatory when stimulated. Eur. J. Immunol. 43, 2206–2216. doi: 10.1002/eji.201343314
Vincenzi, F., Ravani, A., Pasquini, S., Merighi, S., Gessi, S., Romagnoli, R., et al. (2016). Positive allosteric modulation of A(1) adenosine receptors as a novel and promising therapeutic strategy for anxiety. Neuropharmacology 111, 283–292. doi: 10.1016/j.neuropharm.2016.09.015
Wakai, A., Wang, J. H., Winter, D. C., Street, J. T., O’Sullivan, R. G., and Redmond, H. P. (2001). Adenosine inhibits neutrophil vascular endothelial growth factor release and transendothelial migration via A(2B) receptor activation. Shock 15, 297–301. doi: 10.1097/00024382-200115040-00008
Wang, M., Luo, G. H., Liu, H., Zhang, Y. P., Wang, B., Di, D. M., et al. (2019). Apolipoprotein M induces inhibition of inflammatory responses via the S1PR1 and DHCR24 pathways. Mol. Med. Rep. 19, 1272–1283.
Wang, T., Xi, N. N., Chen, Y., Shang, X. F., Hu, Q., Chen, J. F., et al. (2014). Chronic caffeine treatment protects against experimental autoimmune encephalomyelitis in mice: therapeutic window and receptor subtype mechanism. Neuropharmacology 86, 203–211. doi: 10.1016/j.neuropharm.2014.06.029
Warrington, A. E., Barbarese, E., and Pfeiffer, S. E. (1992). Stage specific, (o4+galc-) isolated oligodendrocyte progenitors produce mbp+myelin invivo. Dev. Neurosci. 14, 93–97. doi: 10.1159/000111652
Webb, M., Tharn, C. S., Lin, F. F., Lariosa-Willingham, K., Yu, N. C., Hale, J., et al. (2004). Sphingosine 1-phosphate receptor agonists attenuate relapsing-remitting experimental autoimmune encephalitis in SJL mice. J. Neuroimm. 153, 108–121. doi: 10.1016/j.jneuroim.2004.04.015
Wei, W., Du, C. S., Lv, J., Zhao, G. X., Li, Z. X., Wu, Z. Y., et al. (2013). Blocking A(2B) adenosine receptor alleviates pathogenesis of experimental autoimmune encephalomyelitis via inhibition of IL-6 production and Th17 differentiation. J. Immunol. 190, 138–146. doi: 10.4049/jimmunol.1103721
Weigert, A., Schiffmann, S., Sekar, D., Ley, S., Menrad, H., Werno, C., et al. (2009). Sphingosine kinase 2 deficient tumor xenografts show impaired growth and fail to polarize macrophages towards an anti-inflammatory phenotype. Int. J. Cancer 125, 2114–2121. doi: 10.1002/ijc.24594
Williamson, A. V., Compston, D. A. S., and Randall, A. D. (1997). Analysis of the ion channel complement of the rat oligodendrocyte progenitor in a commonly studied in vitro preparation. Eur. J. Neurosci. 9, 706–720. doi: 10.1111/j.1460-9568.1997.tb01419.x
Windh, R. T., Lee, M. J., Hla, T., An, S. Z., Barr, A. J., and Manning, D. R. (1999). Differential coupling of the sphingosine 1-phosphate receptors Edg-1, Edg-3, and H218/Edg-5 to the G(i), G(q), and G(12) families of heterotrimeric G proteins. J. Biol. Chem. 274, 27351–27358. doi: 10.1074/jbc.274.39.27351
Yang, D., Zhang, Y., Nguyen, H. G., Koupenova, M., Chauhan, A. K., Makitalo, M., et al. (2006). The A(2B) adenosine receptor protects against inflammation and excessive vascular adhesion. J. Clin. Invest. 116, 1913–1923. doi: 10.1172/jci27933
Yao, S. Q., Li, Z. Z., Huang, Q. Y., Li, F., Wang, Z. W., Augusto, E., et al. (2012). Genetic inactivation of the adenosine A2A receptor exacerbates brain damage in mice with experimental autoimmune encephalomyelitis. J. Neurochem. 123, 100–112. doi: 10.1111/j.1471-4159.2012.07807.x
Yazdi, A., Baharvand, H., and Javan, M. (2015). Enhanced remyelination following lysolecithin-induced demyelination in mice under treatment with fingolimod (FTY720). Neuroscience 311, 34–44. doi: 10.1016/j.neuroscience.2015.10.013
Yu, L. Q., Frith, M. C., Suzuki, Y., Peterfreund, R. A., Gearan, T., Sugano, S., et al. (2004). Characterization of genomic organization of the adenosine A(2A) receptor gene by molecular and bioinformatics analyses. Brain Res. 1000, 156–173. doi: 10.1016/j.brainres.2003.11.072
Yu, N. C., Lariosa-Willingham, K. D., Lin, F. F., Webb, M., and Rao, T. S. (2004). Characterization of lysophosphatidic acid and sphingosine-1-phosphate-mediated signal transduction in rat cortical oligodendrocytes. Glia 45, 17–27. doi: 10.1002/glia.10297
Yu, W. P., Collarini, E. J., Pringle, N. P., and Richardson, W. D. (1994). Embryonic expression of myelin genes - evidence for a focal source of oligodendrocyte precursors in the ventricular zone of the neurol tube. Neuron. 12, 1353–1362. doi: 10.1016/0896-6273(94)90450-2
Zemann, B., Kinzel, B., Muller, M., Reuschel, R., Mechtcheriakova, D., Urtz, N., et al. (2006). Sphingosine kinase type 2 is essential for lymphopenia induced by the immunomodulatory drug FTY720. Blood 107, 1454–1458. doi: 10.1182/blood-2005-07-2628
Zhang, J., Zhang, Z. G., Li, Y., Ding, X. S., Shang, X., Lu, M., et al. (2015). Fingolimod treatment promotes proliferation and differentiation of oligodendrocyte progenitor cells in mice with experimental autoimmune encephalomyelitis. Neurobiol. Dis. 76, 57–66. doi: 10.1016/j.nbd.2015.01.006
Zhang, S. C. (2001). Defining glial cells during CNS development. Nat. Rev. Neurosci. 2, 840–843. doi: 10.1038/35097593
Zheng, Z., Zeng, Y. Z., Zhu, X., Tan, Y., Li, Y., Li, Q., et al. (2019). ApoM-S1P modulates Ox-LDL-induced inflammation through the PI3K/Akt signaling pathway in HUVECs. Inflammation 42, 606–617. doi: 10.1007/s10753-018-0918-0
Zonouzi, M., Renzi, M., Farrant, M., and Cull-Candy, S. G. (2011). Bidirectional plasticity of calcium-permeable AMPA receptors in oligodendrocyte lineage cells. Nat. Neurosci. 14, 1430–U1103.
Keywords: adenosine, sphingosine kinase (SphK), remyelination, K+ channels, oligodendrocyte differentiation, sphingosine-1-phosphate, oligodendrocyte progenitor cells (OPCs), A2B receptors
Citation: Coppi E, Cencetti F, Cherchi F, Venturini M, Donati C, Bruni P, Pedata F and Pugliese AM (2021) A2B Adenosine Receptors and Sphingosine 1-Phosphate Signaling Cross-Talk in Oligodendrogliogenesis. Front. Neurosci. 15:677988. doi: 10.3389/fnins.2021.677988
Received: 08 March 2021; Accepted: 22 April 2021;
Published: 26 May 2021.
Edited by:
Francisney Pinto Nascimento, Universidade Federal da Integração Latino-Americana, BrazilReviewed by:
Pavel Katsel, Icahn School of Medicine at Mount Sinai, United StatesMarco Milanese, University of Genoa, Italy
Copyright © 2021 Coppi, Cencetti, Cherchi, Venturini, Donati, Bruni, Pedata and Pugliese. This is an open-access article distributed under the terms of the Creative Commons Attribution License (CC BY). The use, distribution or reproduction in other forums is permitted, provided the original author(s) and the copyright owner(s) are credited and that the original publication in this journal is cited, in accordance with accepted academic practice. No use, distribution or reproduction is permitted which does not comply with these terms.
*Correspondence: Elisabetta Coppi, ZWxpc2FiZXR0YS5jb3BwaUB1bmlmaS5pdA==