- Department of Neuroscience and Experimental Therapeutics, College of Medicine, Texas A&M University, Bryan, TX, United States
Currently there are approximately 291,000 people suffering from a spinal cord injury (SCI) in the United States. SCI is associated with traumatic changes in mobility and neuralgia, as well as many other long-term chronic health complications, including metabolic disorders, diabetes mellitus, non-alcoholic steatohepatitis, osteoporosis, and elevated inflammatory markers. Due to medical advances, patients with SCI survive much longer than previously. This increase in life expectancy exposes them to novel neurological complications such as memory loss, cognitive decline, depression, and Alzheimer’s disease. In fact, these usually age-associated disorders are more prevalent in people living with SCI. A common factor of these disorders is the reduction in hippocampal neurogenesis. Inflammation, which is elevated after SCI, plays a major role in modulating hippocampal neurogenesis. While there is no clear consensus on the mechanism of the decline in hippocampal neurogenesis and cognition after SCI, we will examine in this review how SCI-induced inflammation could modulate hippocampal neurogenesis and provoke age-associated neurological disorders. Thereafter, we will discuss possible therapeutic options which may mitigate the influence of SCI associated complications on hippocampal neurogenesis.
Introduction
Spinal Cord Injury (SCI) is defined as trauma to the spinal cord leading to complete or incomplete injuries and represents the second most common cause of paralysis behind stroke (Armour et al., 2016). According to the National Spinal Cord Injury Statistical Center, there are over 17,000 new cases each year in the United States alone with motor vehicle accidents being the most common cause (Singh et al., 2014), 77.1% of victims being male (Devivo, 2012). The average lifetime medical costs for a SCI victim 25 years of age at time of injury is $1.5-4.7 million depending on severity (Christopher & Dana Reeve Foundation, 2020). Besides locomotion impairment, people living with SCI suffer from a wide variety of health complications, leading to a reduction in life expectancy and quality of life (Figure 1). The location of injury determines pathologies such as loss of respiratory, bowel and bladder control which, along with the severity of trauma, determines the probability of recovery (Alizadeh et al., 2019; Christopher & Dana Reeve Foundation, 2020; Shepherd Center, 2020; SpinalCord.Com, 2020).
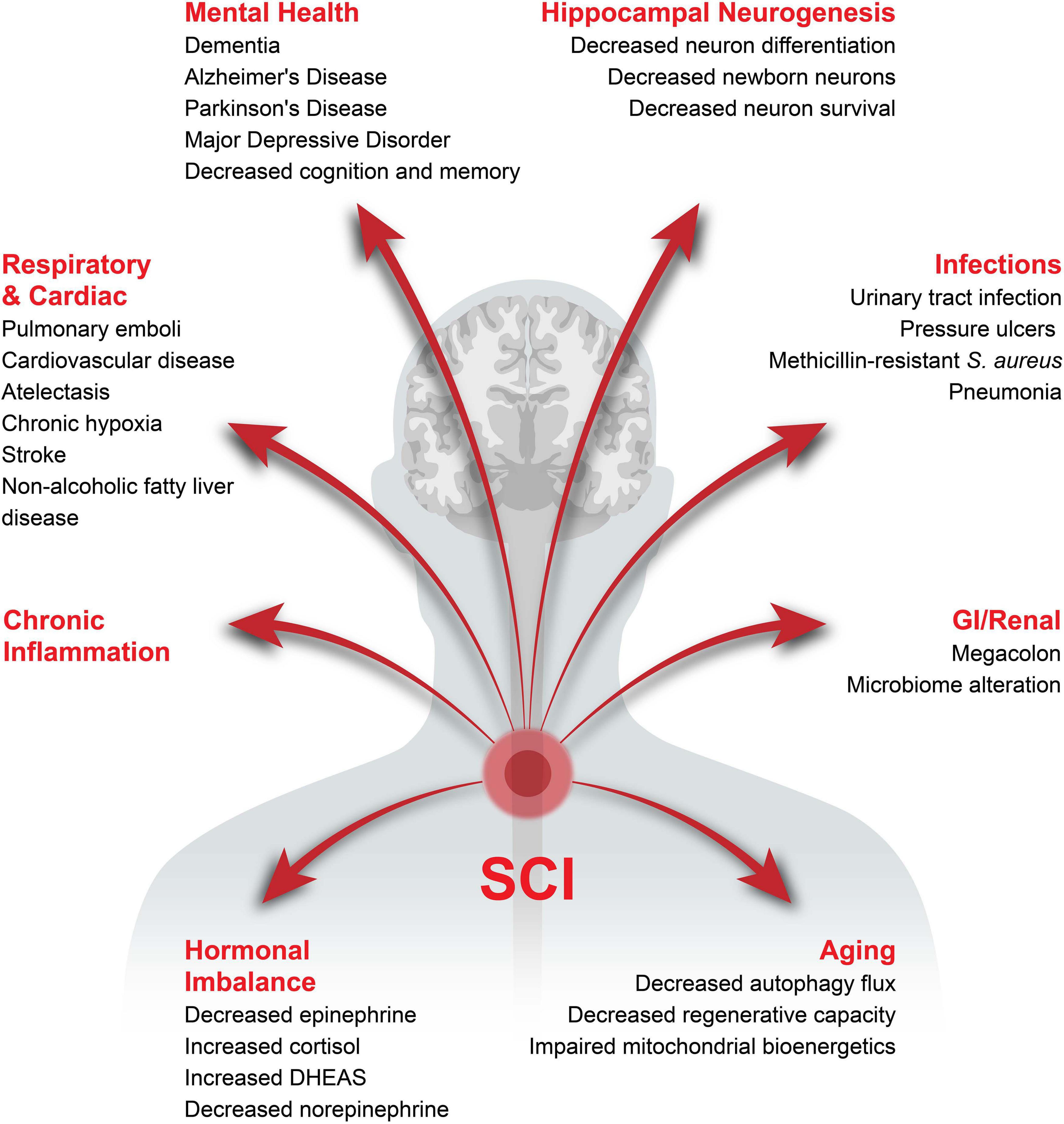
Figure 1. Health complications associated with chronic SCI. The potential health complications developing after SCI have been categorized into major sections with the specific complications in the respective category listed underneath.
One understudied area in the field of SCI is the development of neurological and psychological disorders, cognitive deficits and memory impairments. However, these issues associated with SCI are becoming more apparent since the demographic of the SCI population has shifted in the last few decades. Indeed, the average age at injury is increasing, from 29 in the 1970s to 43 today (National Spinal Cord Injury Statistical Center, 2019), with a peak in the 17-29 group and a second in an older population (> 70 years) (James and Alice, 2019). Additionally, the average age of persons living with SCI is continuously increasing, with and average age of 48 and around 80% being over 40 years old (Christopher & Dana Reeve Foundation, 2021). The rates of incidence of neurological and psychological disorders is increased in people with chronic SCI (Anderson et al., 2007; Huang et al., 2017). Similarly, people with SCI have a 13-fold higher probability of cognitive impairments (Craig et al., 2017), specifically, an impaired ability to learn new tasks and create new memories with a decrease in information processing speed, verbal fluency (Chiaravalloti et al., 2020), visual memory, and perceptual reasoning (Macciocchi et al., 2013). The reasons behind these cognitive declines after SCI are unknown. Memory and cognitive impairments are associated with the reduction of hippocampal neurogenesis in non-SCI models (Bruel-Jungerman et al., 2005; Aimone et al., 2009; Costa et al., 2015). Therefore, one hypothesis is that the cognitive deficits and memory decline in people with SCI, compared to healthy age-matched controls, might be due to decreased hippocampal neurogenesis. In this review, we will focus on the impact of SCI on hippocampal neurogenesis, while it is noteworthy that other age-related processes might also induce these cognitive changes such as loss of both gray and white matter (Resnick et al., 2003) and reduction in synaptic density (Terry and Katzman, 2001).
There are only a handful of reports associated SCI with the reduction of hippocampal neurogenesis in pre-clinical models (Figure 2 and Table 1). Some reports associated inflammation with the reduction of neurogenesis while the direct causative effect has not been determined (Wu et al., 2014). However, reduction in hippocampal neurogenesis and SCI have a common denominator, inflammation. Indeed, in non-SCI models, inflammatory response is heavily involved in reducing hippocampal neurogenesis (Wu et al., 2013). Induced inflammatory response after SCI is a well-studied field in both the acute and chronic phases (Sato et al., 2012; Herman et al., 2018). How these SCI-induced inflammatory responses might alter hippocampal neurogenesis is unknown.
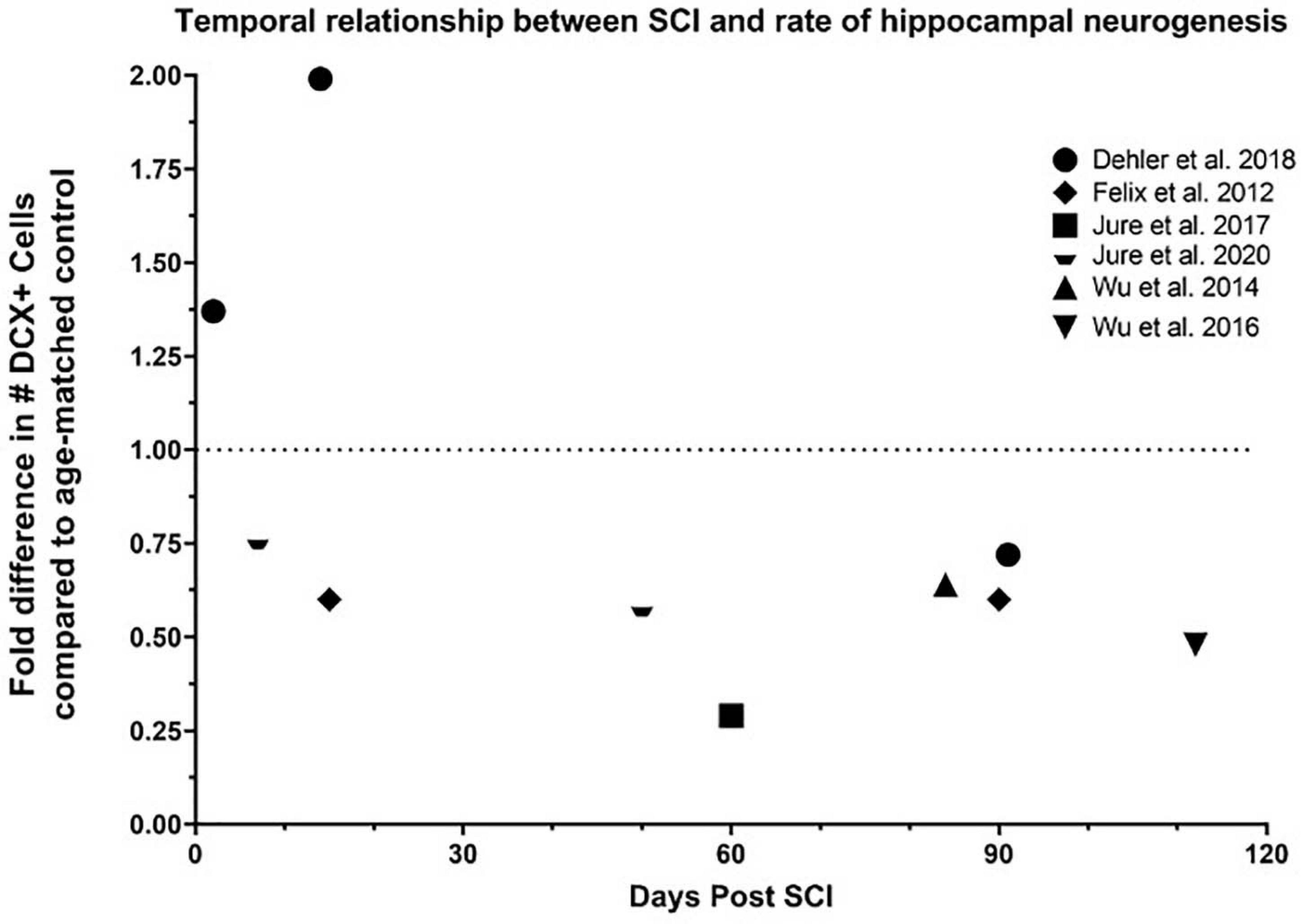
Figure 2. Temporal relationship between SCI and rate of hippocampal neurogenesis. Dehler et al. presented a 37% increase 2 days post injury (dpi), 99% increase 14 dpi, and 28% decrease 91 dpi in the number of DCX+ neurons in the dentate gyrus in a T8 80% transection model on 12-week-old mice (Dehler et al., 2018). Felix et al. reported a 40% decrease 15 and 90 dpi in the number of BrdU+/DCX+ neurons in the dentate gyrus in a C2 left transection in 16-week-old rats (Felix et al., 2012). Jure et al. illustrated a 71% decrease in the number of DCX+ neurons in the dentate gyrus 60 days after severe T8 compression on 8-week-old rats (Jure et al., 2017), and 24% and 42% decrease in the number of DCX+ neurons in the dentate gyrus 7 and 50 days after moderate T8 compression, respectively, on 8-week-old mice. Wu et al. demonstrated a 36% and 52% reduction in the number of DCX+ neurons in the dentate gyrus 84 and 112 days after a moderate T9 contusion in 20-26g mice, respectively (Wu et al., 2014, 2016).
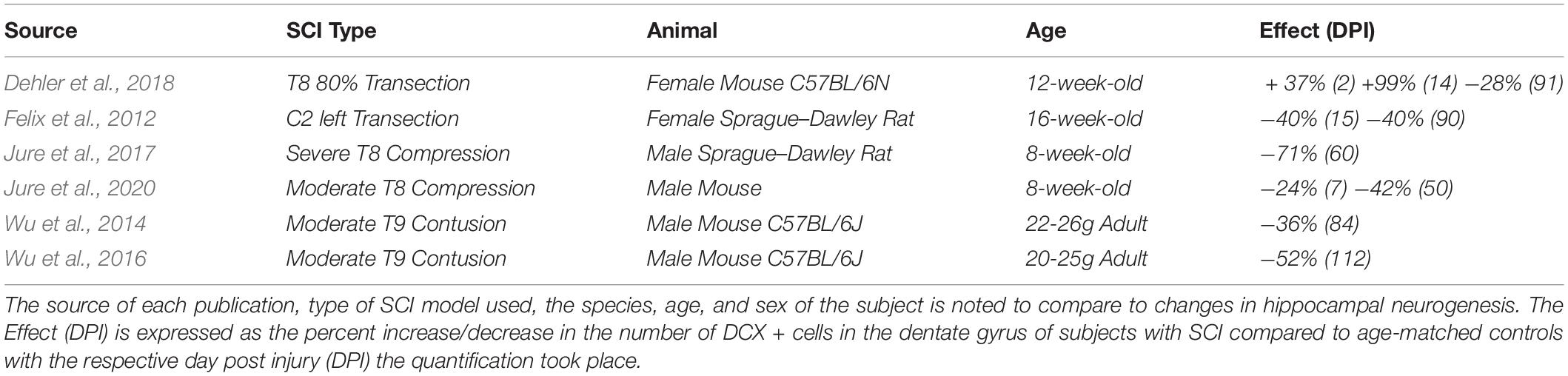
Table 1. Relationship between spinal cord injury (SCI) and rate of hippocampal neurogenesis from different sources.
In this review, we will first discuss the associations between hippocampal neurogenesis and neurological diseases, and neurogenesis after SCI. Next, we will explain how acute inflammation after SCI has potential to either reduce or possibly enhance neurogenesis. A major part of this review will be describing how SCI-induced chronic inflammation might reduce hippocampal neurogenesis and induce cognitive and memory disorders, addressing changes in inflammation associated with aging and several health-issues induced by SCI, including infection, gastrointestinal dysfunctions, respiratory complications and hormonal imbalance (Figure 1 and Table 2). We will finish by proposing therapeutic options that might be of interest to increase hippocampal neurogenesis via the reduction in local and systemic inflammation (Figure 3). It is of high interest to better understand how SCI can reduce hippocampal neurogenesis and contributes to the increased prevalence of cognitive decline and neurodegenerative diseases. Only then will it be conceivable to use the modulation of hippocampal neurogenesis as a tool to reduce the rate of cognitive decline, memory loss, and associated neurodegenerative diseases people with SCI suffer.
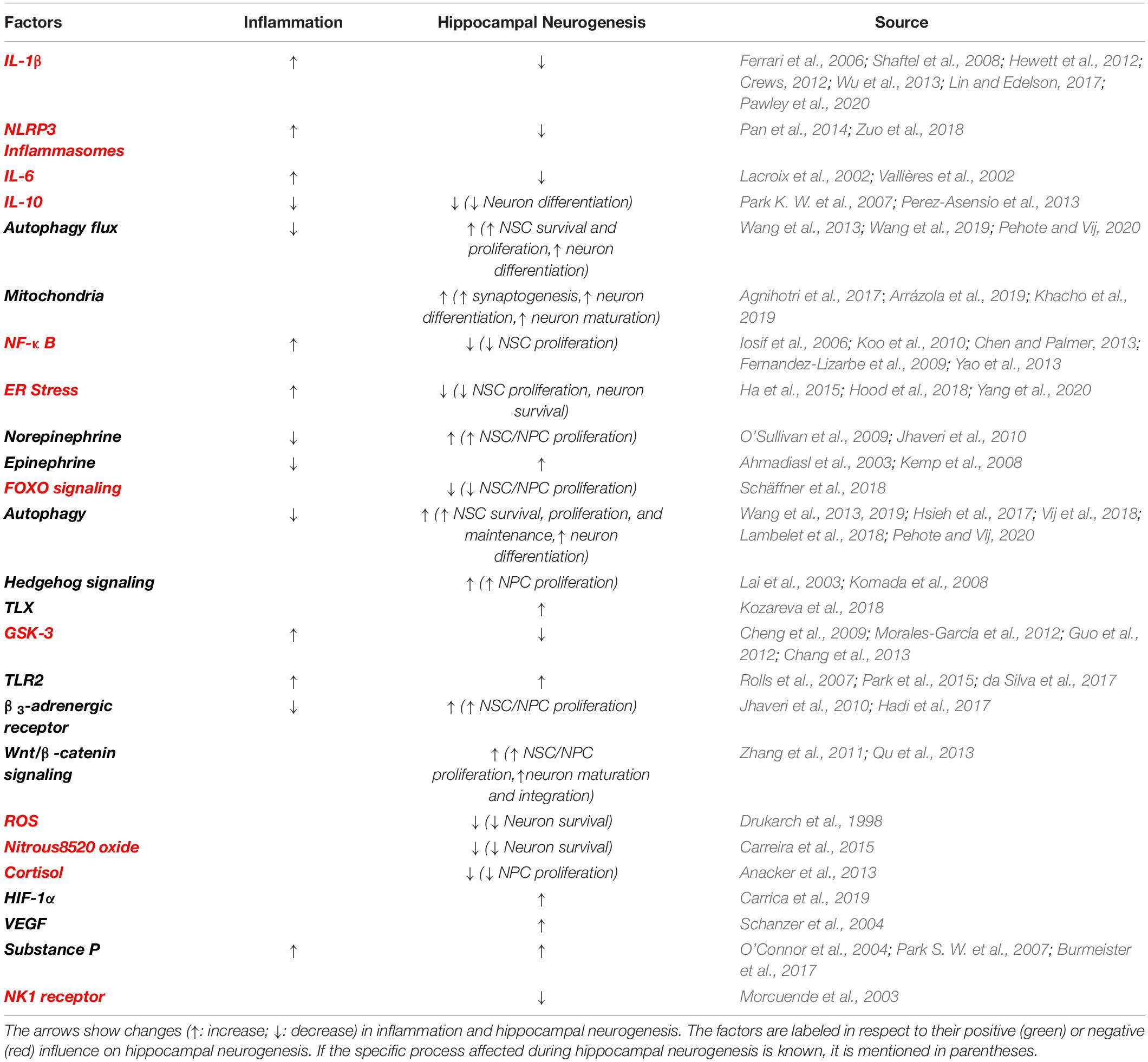
Table 2. Factors, signaling molecules, hormones and activities following SCI that impact hippocampal neurogenesis and inflammation in some cases.
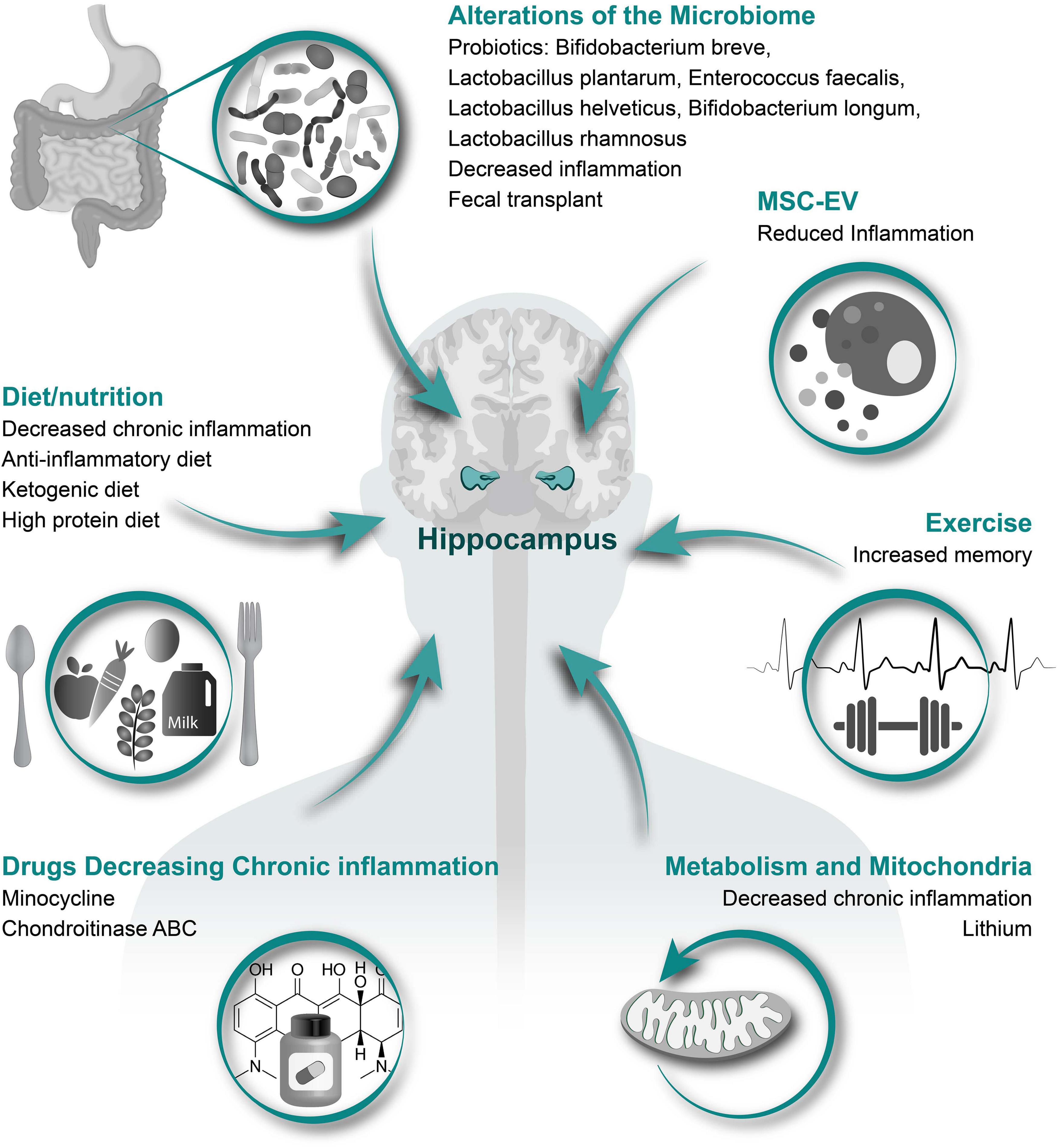
Figure 3. Therapeutic options to increase hippocampal neurogenesis. Illustrated here are several different categories of therapeutic options that have potential to alleviate the SCI induced reduction in hippocampal neurogenesis with specific treatment options and potential affects listed underneath each category.
Associations Between Hippocampal Neurogenesis and Neuropathogenesis
Hippocampal neurogenesis occurs in a region called the subgranular zone (SGZ), producing granule cells in the dentate gyrus (Toni and Schinder, 2015) throughout a human’s lifetime (Eriksson et al., 1998; Boldrini et al., 2018; Moreno-Jimenez et al., 2019), although its presence in humans during adulthood has been the center of much controversy for decades (Lima and Gomes-Leal, 2019) due to the substantial reduction of hippocampal neurogenesis with aging (Sorrells et al., 2018) causing great difficulty in finding and analyzing in aged subjects (Moreno-Jiménez et al., 2021). Furthermore, the doublecortin (DCX) used to label immature neurons is also expressed in adult entorhinal, amygdala, and parahippocampal neurons (Sorrells et al., 2021) which may artificially increase the calculated rate of neurogenesis. Hippocampal neurogenesis is the process of which neural stem cell (NSC, radial glia-like type 1 cells) transitioning through transiently amplifying neural progenitor cells (NPC) types 2 and 3, transform into the early postmitotic maturation phase where it is decided if the newborn neurons will go through apoptosis or mature. 30–70% of newborn hippocampal neurons and neuroblasts undergo apoptosis within the first month (Dayer et al., 2003; Ryu et al., 2016). Notably, the largest population of newborn cells undergoing apoptosis in the hippocampus are amplifying NPC that are transitioning into neuroblasts (Sierra et al., 2010). Next, the neurons undergo neuritogenesis and synaptogenesis as they mature through the late postmitotic maturation phase, migrate into the inner granule cell layer as they form complex dendritic processes to the molecular layer and an elongated axon connecting to the CA3, integrating into the neural network (Song et al., 2012; Kempermann et al., 2015).
Roles of Hippocampal Neurogenesis
The role of hippocampal neurogenesis in memory and learning is prominent. There is evidence implicating hippocampal neurogenesis’ role in influencing the rate of recovery of memory capacity, maintaining memory capacity throughout adulthood (Alam et al., 2018) and long-term memory formation in rats (Bruel-Jungerman et al., 2005) and new memories (Aimone et al., 2009). Hippocampal neurogenesis in mice has been credited with providing cognitive flexibility (Burghardt et al., 2012; Anacker and Hen, 2017) and pattern separation (Sahay et al., 2011). The rate of hippocampal neurogenesis is positively correlated with the recovery of memory capacity (Alam et al., 2018) and spatial memory performance in rats (Drapeau et al., 2003), and ability to retain new memory in mice (Kempermann and Gage, 2002). Conditional ablation of hippocampus neurogenesis in APPswe/PS1ΔE9 transgenic mice induced deficits in pattern separation and contextual fear conditioning (Hollands et al., 2017). Increasing hippocampal neurogenesis enhances pattern separation abilities in mice (Sahay et al., 2011). Focal X-irradiation of hippocampal neurogenesis in mice lead to a reduction in contextual fear conditioning with no effect on spatial memory (Saxe et al., 2006). Clelland et al. demonstrated with 2 distinctive methods, X-irradiation and genetic manipulation, that ablation of hippocampal neurogenesis in mice decreases spatial discrimination for only similar spatial locations but not distinct spatial locations (Clelland et al., 2009). Notably, radiation-induced brain injuries provoke a strong inflammatory response in the brain (Greene-Schloesser et al., 2013) which may impact surrounding regions as well.
There are many associations between improving hippocampal neurogenesis, either through exercise (van Praag et al., 1999; Trinchero et al., 2019) or estrogen (Tanapat et al., 1999), and increase in cognitive performance and memory (Daniel et al., 1997; Packard and Teather, 1997; Chang and Etnier, 2009). Increasing hippocampal neurogenesis in mice lead to increased inhibitory activity in the trisynaptic circuit, an acute increase in spatial memory and allocentric navigation, and maintained procedural and contextual memories in aged mice (Berdugo-Vega et al., 2020). The process of learning directly induces a 32% increase in neurogenesis (Leuner et al., 2004) and a 94% increase in number of bromodeoxyuridine (BrdU) + cells in the rat dentate gyrus (Gould et al., 1999) demonstrating a positive correlation between learning and the rate of hippocampal neurogenesis. Some studies suggest the increased prevalence of immature neurons is due to increased new neuron cell survival rather than increased cell proliferation rate (Tashiro et al., 2007; Anderson et al., 2011). Tashiro et al. illustrated increased survival of NeuN + /BrdU + cells in mice born ≤ 3 weeks prior to enrichment with the greatest increase in neurons born 1–2 weeks prior to enrichment (Tashiro et al., 2007). Epp et al. observed a higher number of BrdU + cells in rats regardless of learning type when BrdU was injected 6-10 days prior to learning, and an increase in the percentage of NeuN/BrdU + cells when BrdU was injected 1–5 days prior to learning, compared to rats that did not undergo learning. Since these neurons were born before learning took place, the data suggest an increase in neuron survival rather than in neurogenesis. Learning type also matters, as place-trained rats had higher number of BrdU + cells in the granular cell layer when BrdU was injected 6–10 days prior to learning compared to cue-trained rats (Epp et al., 2007). Further experimentation is needed to determine how specific learning activities affect the survival of each cell type and if the mitigation of increased neuron survival will impact cognitive processes. Due to the difficulties of measuring changes in hippocampal neurogenesis in live subjects, experimentation is usually done on small rodents. There is a possibility that the mechanism of learning and memory formation in humans differs significantly.
Connection Between SCI, Neurological Diseases and Hippocampal Neurogenesis
Mental wellbeing is an important factor for healthy aging; mental disorders decrease life expectancy by 10.1 years on average (Insel, 2015). People living with SCI are 2.14 times as likely to develop dementia (Huang et al., 2017), 1.71 times as likely to develop Alzheimer’s disease (Yeh et al., 2018), and 1.65 times as likely to develop Parkinson’s disease (Yeh et al., 2016). Unfortunately, people living with SCI are much more likely to commit suicide with reports in England showing a 5-fold increase in per capita suicide rates (Savic et al., 2018). Furthermore, 28% of rats with T12 contusion SCI suffered from depression (Brakel et al., 2019) which was associated with increased serum levels of interleukin (IL)-6 and IL-1α before and IL-6 and tumor necrosis factor (TNF)-α after injury (Brakel et al., 2021). These findings imply predisposition to depression even before injury occurs if systemic increases in inflammation induces depression (Lee and Giuliani, 2019). 49.3% of persons with SCI suffer mild to severe depression (Khazaeipour et al., 2015), 4.3-fold greater than the national average for Major Depressive Disorder (Pedersen et al., 2014). Mental health is also dependent on SCI severity; individuals with high severity SCI are 1.85 times as likely to develop depression than those suffering from less severe SCI (Lim et al., 2017). Furthermore, those suffering from urinary incontinence have a 78% higher probability of developing schizophrenia (Hsu et al., 2017); urinary incontinence is positively correlated with increasing severity of SCI (Elmelund et al., 2018). Therefore, SCI-induced mental disorders are common disruptions of healthy aging and longevity. However, a direct link between SCI and the development of any of these neurological diseases is missing. We propose here that this link might be the reduction in hippocampal neurogenesis.
Indeed, many neuropathological disorders leading to cognitive and memory impairments are associated with impaired hippocampal neurogenesis (Costa et al., 2015; Han et al., 2016). In Alzheimer’s disease, there is a significant reduction in hippocampal neurogenesis (Haughey et al., 2002), the results of several mechanisms, including β-amyloid (Aβ) plaque (Haughey et al., 2002) and Aβ42 assemblies (Sun et al., 2009). Parkinson’s disease, the second most common neurodegenerative disease (Bertram and Tanzi, 2005) which is also associated with cognitive impairment in a subset of patients (Watson and Leverenz, 2010), can be caused by α-Synuclein (α-syn) accumulation around dopaminergic neurons (Masliah et al., 2000). Not only does overexpression and aberrant α-syn accumulation perturb hippocampal neurogenesis (Winner et al., 2012; Winner and Winkler, 2015), but passive immunization against α-syn reverses cognitive and memory impairments (Masliah et al., 2011) which are hippocampus associated functions. The size of the hippocampus of humans with schizophrenia are reduced (Steen et al., 2006) with a reduction in the number of Ki67 + cells, although no changes in the number of NeuN + were noted (Reif et al., 2006; Allen et al., 2016). Rats with depression had decreased number of DCX + cells in the suprapyramidal blade of the dentate gyrus (Brakel et al., 2021). Increasing hippocampal neurogenesis through genetic manipulation was able to rescue mice from depressive like behaviors induced by chronic corticosterone administration (Hill et al., 2015).
Inflammation and infections, which have potential in inducing neurodegenerative diseases such as Parkinson’s disease (Caggiu et al., 2019), also influence hippocampal neurogenesis by reducing the number of BrdU/NeuN double positive cells in the dentate gyrus of rats (Ekdahl et al., 2003). In these neuropathologies, some of the patient’s symptoms, such as impaired cognitive function and memory loss, may be due to the decreased levels of hippocampal neurogenesis. Thus, it is hypothesized that reversing changes to hippocampal neurogenesis has clinical significance in reversing cognitive decline and memory loss (Peng and Bonaguidi, 2018). Hippocampal neurogenesis is also implicated in helping preserve the dentate gyrus after a prion infection in mice (Gomez-Nicola et al., 2014). Nonetheless, the direct mechanism of cognitive decline induced by neurodegenerative diseases and the direct influence of hippocampal neurogenesis on mental and neurological wellbeing has yet to be elucidated.
Hippocampal Neurogenesis After SCI
Only a handful of studies have assessed the impact of SCI on hippocampal neurogenesis (Figure 3 and Table 1). SCI-induced modulation of hippocampal neurogenesis is time-, severity-, age- and model-dependent. The rate of hippocampal neurogenesis (assessed by the quantification of either DCX + or DCX/BrdU + cells in the dentate gyrus) significantly declines at 7 days post injury (dpi), 50 dpi (Jure et al., 2020), 60 dpi (Jure et al., 2017), 84 dpi (Wu et al., 2014), and 112 dpi (Wu et al., 2016) after moderate to severe thoracic SCI while the mild SCI model in these studies showed no significant changes. Felix et al. using a left transection SCI model found decreased hippocampal neurogenesis just 15 dpi (in coherence with Jure et al.) which remained significantly decreased during the entire 3-month study after cervical transection (Felix et al., 2012). This is contradictory to another study which reported a significant increase in DCX + /BrdU + cells 2 and 14 dpi in an 80% thoracic transection model. This was accompanied with a significant decline in hippocampal neurogenesis (28% below baseline) at a chronic time point (91 dpi) (Dehler et al., 2018). Major differences between studies were the location of injury (cervical vs thoracic) and animal species used (mouse vs. rat). The location of injury does generally impact both motor control and organ dysfunction differently (Alizadeh et al., 2019) which might also influence hippocampal neurogenesis differently. Subject species might also impact neurogenesis differently since rats and mice have different inflammatory reactions to SCI. Mice have delayed T-cell entry and less, if any, influx of dendritic cells into the injury site compared to rats (Sroga et al., 2003). Dendritic cells are antigen-presenting cells that initiate adaptive immune function (Martin-Gayo and Yu, 2019). Nevertheless, Jure et al. confirmed Felix’s findings of decreased hippocampal neurogenesis at 7 dpi using the same species and location as Dehler et al. (mice, T8); although, unlike Felix and Dehler, Jure et al. used a compression model which may impact hippocampal neurogenesis and systemic inflammation differently. Transection and contusion SCI models can invoke different inflammatory changes. After transection, there is damage to the meninges which support neuroinflammation (Rua and McGavern, 2018). Leaking cerebral spinal fluid may lead to the reduction in microglia around the injury site of rats with spinal transections (Watanabe et al., 1999). Contusions also induce greater rostrocaudal spread of pathologies (Hausmann, 2003). Furthermore, Jure observed no change and Wu observed a smaller decrease in neurogenesis in less severe (mild) SCI models. We hypothesized that milder SCI perturb hippocampal neurogenesis less than severe SCI due to being associated with less severe inflammation and smaller inflammatory responses compared to severe injuries (Yang et al., 2005). BrdU injections for the last 4 weeks of a 4-month (using a T9 contusion model) demonstrated a significant decrease in BrdU/NeuN + and%BrdU/NeuN + cells and a downward trend in %BrdU/DCX + cells. These data are implicative of both a decrease in neuron differentiation and survival of newborn neurons during chronic SCI (Wu et al., 2016). Altogether, injury type and severity are likely to influence neurogenesis patterns. It may explain why the 2 largest decreases in hippocampal neurogenesis are seen in studies using the compression and contusion model (Wu et al., 2016; Jure et al., 2017). Therefore, a more careful and systemic characterization of the impact of SCI on neurogenesis in relationship to time after injury, severity of injury, age, sex, subject specie, and injury type is needed to further elucidate the discrepancies found in these studies.
Relation Between SCI, Acute Inflammation, and Hippocampal Neurogenesis
Spinal Cord Injury activates various inflammatory pathways. Throughout recovery, there are dynamic changes in both the severity and polarization of inflammation. These modulations have potential to influence hippocampal neurogenesis and associated processes.
Acute Inflammatory Changes in the Spinal Cord Following SCI
Spinal Cord Injury leads to necrosis, activating the immune system (van Den Hauwe et al., 2020) leading to a rapid increase in macrophages, activated microglia, and CD45 + leukocytes at the injury site (Okada, 2016). Within 30 min of SCI, IL-1β and TNF-α signaling across the entire spinal cord increases followed by IL-6 expression increasing in both spinal astrocytes and microglia and leukemia inhibitory factor (LIF) expression increasing only in spinal astrocytes at the injury site within 3 h (Pineau and Lacroix, 2007; Sutherland et al., 2017). Since the permeability of the blood spinal cord barrier is significantly elevated immediately after SCI (Cohen et al., 2009) while the blood brain barrier permeability is rapidly increased for the first 24 h after SCI (Donnelly and Popovich, 2008), there is potential for inflammatory biological agents to be quickly transported from the injury site to the hippocampus through the cardiovascular system. Another possible mechanism for rapid systemic inflammatory changes following SCI is changes in the extracellular vesicle (EV) profile being released into the bloodstream (Dutta et al., 2021). After SCI in mice, there is an increased proportion of CD81 + EVs found in the plasma for the first 7 dpi. Intracranial injections of CD81 + EVs collected after SCI increased astrocyte IL-1β and IL-1α cytokine levels and astrocyte reactivity gene expressions suggesting CD81 + EVs have potential to modulate the reactivity of astrocytes even in regions distant from the injury site (Khan et al., 2021). CD81 has been implicated in increasing the migration of activated T lymphocytes (Hasezaki et al., 2020) and inducing some of the neurological pathologies associated with experimental autoimmune encephalomyelitis (EAE) (Dijkstra et al., 2008). To determine the direct influence of inflammatory cytokines on recovery, IL-6 was injected into the lesion core causing a 6-fold and 2-fold increase in neutrophil and microglial presence, respectively, while increasing the size of the damaged lesion core (Lacroix et al., 2002). Therefore, proper modulation of the inflammatory system is necessary to mitigate tissue trauma.
In response to SCI, p38 MAPK is activated in reactive spinal microglia producing IL-1, IL-6, TNF-α, and prostaglandin (PD)E2 (Ji and Suter, 2007; Sato et al., 2012). Notably, microglia also produce anti-inflammatory cytokines like IL-10 (Park K. W. et al., 2007). The physical penetration of foreign objects into the spinal cord increases the probability of a pathogenic infection. Lipopolysaccharides (LPS) from those pathogens induce signal transducer and activator of transcription (STAT)1 signaling which promotes pro-inflammatory microglial phenotype (Liu et al., 2012) and stimulates the release of reactive oxygen species (ROS) and pro-inflammatory cytokines from microglia (Butturini et al., 2019). COX-1 expressing spinal microglia also increased for up to 4 weeks after SCI (Schwab et al., 2000a). Although unclear how COX-1 can impact persons with SCI, COX-1 is highly associated with other neurological disorders including neuroinflammation (Choi et al., 2008; Aid et al., 2010; Choi et al., 2010), Alzheimer’s disease (Yermakova et al., 1999), and ischemia (Schwab et al., 2000b). Microglia are vital neuroprotective components of our central nervous system (CNS) (Streit, 2005), preventing neuron and oligodendrocyte death (Bellver-Landete et al., 2019), stripping synapses (Chen and Trapp, 2016), and increasing spinal cord repairs (Fu et al., 2020; Li et al., 2020a). Microglia can also secrete Interferon (IFN)-β to remove axon and myelin debris from the injury site (Kocur et al., 2015), increase axon regenerative potential, and improve functional locomotor recovery (Tanaka et al., 2009).
After SCI, there is an elevated level of astrocyte activation for at least the first week after injury (Schnell et al., 1999). These reactive astrocytes in the lesion penumbra, long thought to inhibit axon regeneration (Rudge and Silver, 1990) have also been shown to improve healing and locomotor functional recover while acting as a neuroprotectant (Bush et al., 1999; Faulkner et al., 2004). Astrocytes also protect and maintain the blood brain barrier (Cabezas et al., 2014) which becomes significantly more permeable after SCI (Donnelly and Popovich, 2008). Reactive astrocytes become scar-forming (Okada et al., 2018) and produce TNF-α, IL-1β, interferon gamma-induced protein (IP)-10, macrophage inflammatory protein (MIP)-1α, and C-C chemokine ligand (CCL)5 (Choi et al., 2014). Overall, reactive astrocytes are essential in recovery after SCI, mitigating cell death and loss of locomotor function (Faulkner et al., 2004).
SCI-Induced Acute Inflammation May Modulate Hippocampal Neurogenesis
There are several inflammatory molecules that are altered acutely after SCI which could positively or negatively impact hippocampal neurogenesis. Unless the changes were analyzed in the hippocampus, it is unknown if changes only occurring in the spinal cord can impact hippocampal processes. Quickly after SCI, several pro-inflammatory molecules, such as TNF-α and IL-1β, are locally secreted in the spinal cord. These factors are known to reduce hippocampal neurogenesis in non-SCI settings (Lan et al., 2012; Wu et al., 2013; Donzis and Tronson, 2014). Jure et al. reported a reduction of newborn hippocampal neurons during the acute stages of SCI, which is associated with increased astrocytic and microglial activation in the hippocampus (Felix et al., 2012; Jure et al., 2020). At 8 dpi, IL-6 expression is elevated in the hippocampus, although, TNF-α, iNOS, and CD68 expressions are not significantly increased (Wu et al., 2014). Therefore, SCI has potential to directly alter cytokine levels in the hippocampus. SCI also induces a significant increase in glucocorticoid expression at the injury site during the first 24 h after injury (Yan et al., 1999). Glucocorticoid expression in the hippocampus, although dose dependent, can reduce NPC proliferation and DCX + neuroblasts while favoring astrocyte differentiation (Anacker et al., 2013). Therefore, it is tempting to speculate that SCI-induced glucocorticoids might directly reduce neurogenesis in the hippocampus. Nevertheless, further experimentation is required to determine the changes in both hippocampal glucocorticoid and cytokine levels immediately after SCI and how these SCI-induced changes directly impact neurogenesis during the acute stages of SCI.
On the other hand, Dehler et al. observed a significant increase in neurogenesis 7 days post SCI (Dehler et al., 2018). Several factors secreted at the lesion site could participate in such increase. Retinoic acid is elevated in the spinal cord 4–14 days post SCI with its decline 21 days post injury (Schrage et al., 2006), and has been shown to increase NSC and NPC proliferation through the activation of hypoxia-inducible factor (HIF)-1α (Mishra et al., 2018). HIF-1α is required for Notch signal activation (Li J. et al., 2018), learning and hippocampal neurogenesis (Carrica et al., 2019). Interestingly, HIF-1α is significantly increased in the spinal cord within 12-24 hours post injury, reaching maximal levels 3-7 days post SCI with a gradual decrease thereafter (Ju et al., 2002; Xiaowei et al., 2006). Vascular endothelial growth factor (VEGF), which is induced by HIF-1α (Lin et al., 2004) and upregulated alongside HIF-1α after SCI (Chen et al., 2017), also stimulates hippocampal neurogenesis (Schanzer et al., 2004) and even reverses age-associated decline in cognition (Han et al., 2017) and hippocampal neurogenesis (Licht et al., 2016). Therefore, one mechanism of increased hippocampal neurogenesis immediately after SCI may be through the increase of VEGF expression following the retinoic acid-induced activation of the HIF-1α, which would activate neurogenesis away from the injury site. Alternatively, retinoic acid might travel to the hippocampus and directly activate HIF-1α in the hippocampal NSC/NPCs.
To the contrary of many inflammatory molecules increased after SCI, substance P, typically secreted by inflammatory cells (O’Connor et al., 2004), is drastically decreased in the spinal cord during the first 4 weeks after injury (Leonard et al., 2014). Substance P activates neurokinin (NK)1 receptor (Schank and Heilig, 2017), and NK1 receptor deletion increases both hippocampal neurogenesis and BDNF levels (Morcuende et al., 2003). Substance P injections into lateral ventricles increased the number of DCX + neurons in the hippocampus by 12% (Park S. W. et al., 2007). Substance P also mediates the LPS-induced production of IL-6 from astrocytes (Burmeister et al., 2017) and has pro-inflammatory effects (O’Connor et al., 2004) which has potential to decrease hippocampal neurogenesis. Altogether, these data suggest the potential role of substance P and NK1 receptor in participating in the modulation of neurogenesis. How the reduction of substance P at the injury site can be linked to acute changes in hippocampal neurogenesis after SCI remains to be determined.
We want to emphasize that the potential beneficial effects of acute inflammation after SCI on neurogenesis are under debate. While it is conceivable that this early increase in neurogenesis is a potential attempt to protect from a future decline in neurogenesis, increasing cell proliferation in the hippocampus immediately after injury will not be of clinical interest if the newborn neurons are not capable of surviving, maturing, and integrating into the hippocampal network. A possible therapeutic avenue may be to mimic the neurogenesis promoting processes induced by acute inflammation mentioned above during the chronic injury stages of SCI. It is hypothesized that the beneficial impact of acute inflammation is time dependent. Therefore, prolonged exposures to such systemic changes must be further elucidated.
SCI-Induced Chronic Inflammation Neuropathologies and Reduction of Hippocampal Neurogenesis
While the exact acute effects of SCI, and presumably of acute inflammation, on hippocampal neurogenesis is still under debate, all the studies agree that neurogenesis is severely reduced in chronic models of SCI. Aberrant immune behavior in chronic SCI leads to autoimmunity, chronic inflammation and immune deficiency. SCI-induced changes are systemic, almost every organ and system are differently impacted by SCI depending on severity of injury. Ischemia, edema and oxidative damage are increased at the site of injury accumulating further strong inflammatory responses (Carlson et al., 1998). People living with SCI are more susceptible to infections and cardiometabolic dysfunctions (Bauman and Spungen, 2001; Phillips and Krassioukov, 2015). Other dysfunctions commonly occurring after SCI are splenic atrophy, neurogenic bowel dysfunction, inflammation-induced muscle atrophy, osteoporosis, and neurogenic heterotopic ossification (Sun X. et al., 2016). These statistics vary greatly between countries depending on the healthcare and economic status of the country (Singh et al., 2014), 12% of SCI patients die from infections, 21.4% from respiratory disease, and 4.9% from gastrointestinal-renal complications (National Spinal Cord Injury Statistical Center, 2014, 2019). This translates to a 539% increase in infection, 280% increase in respiratory disease, and 139% increase in gastrointestinal-renal related fatalities per capita for people living with SCI relative to the average per capita fatality rates in the United States (National Spinal Cord Injury Statistical Center, 2014; Centers for Disease Control and Prevention, 2017; National Spinal Cord Injury Statistical Center, 2019).
SCI-Induced Inflammation May Cause Neuropathologies
Several pro-inflammatory and pro-infectious molecules are associated with both SCI and different neuropathologies. Increased IL-1 production as a defense mechanism stimulates β-amyloid precursor protein production (Goldgaber et al., 1989); treatment of hippocampal neurons with IL-6 induces hyperphosphorylation of tau (Quintanilla et al., 2004). Collectively, both IL-1 and IL-6 increase the likelihood of developing Alzheimer’s disease (Iqbal et al., 2010; Hansen et al., 2018; Kinney et al., 2018). IL-1β-induced production of IL-6 (Chen et al., 2005; Trompet et al., 2008) has not only been shown to increase age-associated cognitive decline (Trompet et al., 2008), but also lead to depression (Capuron et al., 2008; Raison and Miller, 2011; Lee and Giuliani, 2019). Elevated IL-6 serum levels are strongly correlated with schizophrenia (Schmitt et al., 2005; Neelamekam et al., 2014). Maternal infections during pregnancy that increased IL-1 β, IL-6, and TNF-α levels in their offspring, hypothesized to be LPS-induced, increased susceptibility to schizophrenia (Gilmore et al., 2004). Similarly, LPS from bacterial species such as Pseudomonas aeruginosa, commonly found in people living with SCI, induces Parkinson’s disease in animal models (Dutta et al., 2008; Wang et al., 2015; Huang et al., 2018). Other molecules known to activate inflammatory cytokines, such as chondroitin sulfate proteoglycans (CSPGs) (Stephenson and Yong, 2018), are increased after SCI (Iaci et al., 2007; Dyck and Karimi-Abdolrezaee, 2018), and are involved in Alzheimer’s disease (DeWitt et al., 1993) and neurodegeneration (Galtrey and Fawcett, 2007). SCI-induced inflammation and LPS accumulation may directly cause the increased prevalence of Alzheimer’s disease, Parkinson’s disease, schizophrenia, and other neurological disorders common among persons with SCI. Nonetheless, the direct effects of SCI-induced changes in cytokine levels on hippocampal neurogenesis and neuropathologies have yet to be elucidated. Notably, some cytokines levels are elevated only at the injury site which may not affect hippocampal neurogenesis, although can cause systemic changes since blood vessel permeability is proportional to the level of inflammation (Park et al., 2018) and the permeability of the blood spinal cord barrier is elevated for at least the first 8 weeks after injury (Cohen et al., 2009).
Relationship Between SCI-Induced Health Complications and Neurogenesis
SCI-Induced Infections and Their Effects on Neurogenesis
Spinal Cord Injury patients have much higher rates of infection, in part due to their compromised immune system and their inability to expel excretion fluids (Vyas et al., 2015). Short-term local inflammatory responses should not have prolonged effects on hippocampal neurogenesis, yet systemic infections directly increase both TNF-α and IL-1β levels in the brain (Püntener et al., 2012) which do impact hippocampal processes. Pseudomonas aeruginosa associated bloodstream infections were more fatal in SCI patients than in patients on immunosuppressant therapy (Saliba et al., 2017) suggesting SCI has devasting impacts on immune function. Over 74% of SCI patients are infected with methicillin-resistant Staphylococcus aureus (MRSA) at one point, a systemic infection that activates GSK-3β leading to increased TNF-α expression, nitric oxide (NO) production (Cheng et al., 2009), NF-κB activation and iNOS expression (Chang et al., 2013). It is estimated that 5–15% of SCI patients will develop pneumonia (Montgomerie, 1997) which is most commonly caused by Streptococcus pneumonia (Claudia Antonieta Nieves Prado, 2018). The cell wall of Streptococcus pneumonia contains lipoteichoic acid (LTA), which not only induces meningitis, inflammation of brain and spinal cord meninges, but also impairs hippocampal neurogenesis (Hoffmann et al., 2007) by inducing apoptosis of hippocampal NSC and NPC (Hofer et al., 2011). LTA is also found in Staphylococcus aureus which interacts with toll-like receptor 2 (TLR2) (Schroder et al., 2003) to activate pro-inflammatory macrophages through a CD14 mediated manner (da Silva et al., 2017). Reduction of pro-inflammatory macrophage activation improved hippocampal neurogenesis (Zhang et al., 2017). Notably, many argue the existence of more than 2 macrophage phenotypes as the role of each phenotype is complex and time-dependent (Sato, 2015). Although TLR2 is required for pro-inflammatory macrophage activation and its over activation affects neural development (Du et al., 2011), TLR2 is also required for hippocampal neurogenesis (Rolls et al., 2007; Park et al., 2015). Therefore, total inhibition of an inflammatory pathway does often come with unattended harmful consequences.
Urinary tract infection (UTI) inducing pathogens are potentially strong contributors to the impaired hippocampal neurogenesis patterns in people with chronic SCI. Pseudomonas aeruginosa activates TLR4 and TLR5 in mice (Sun et al., 2010), contributing to the activation of NLR Family CARD Domain Containing 4 (NLRC4) and NLR Family Pyrin Domain Containing 3 (NLRP3) inflammasomes (Lin and Kazmierczak, 2017) similarly to Klebsiella pneumoniae (Codo et al., 2018). TLR4 activation directly in the brain has been implicated to inhibit hippocampal neurogenesis in TBI (Ye et al., 2017), development (Rolls et al., 2007) and alcohol addiction (Vetreno et al., 2018) models. Furthermore, systemic inhibition of NLRP3 inflammasomes through a heat shock protein (HSP)90 inhibitor increased BDNF levels and hippocampal neurogenesis (Zuo et al., 2018). Moreover, NLRP3 inflammasomes increase IL-1β production in macrophages and pulmonary inflammation (Kang et al., 2017), increasing susceptibility to further respiratory infections such as pneumonia (Mizgerd, 2018). This suggests the potential for UTI to reduce hippocampal neurogenesis. Indeed, UTI infections will mostly impact local regions around and in urinary tracts and may not affect hippocampal processes. Although, chronic inflammation in one region of the body still has potential to induce systemic changes over time, especially since inflammation increases blood vessel permeability (Park et al., 2018). Persons with SCI are more prone to both local infections, such as UTIs, and systemic infections, such as MRSA. Therefore, the systemic and chronic infections caused by SCI have potential to influence neurological processes, including hippocampal neurogenesis. Further experimentation must be conducted to determine the direct effect of each infection type on hippocampal neurogenesis in both an acute and chronic manner.
Role of Cardiometabolic Disorders in Neurogenesis
The prevalence of diabetes mellitus, stroke, and heart disease in person’s with SCI are 3-fold, 3.7-fold, and 2.7-fold higher, respectively, than that of the general population (Lavela et al., 2006; Cragg et al., 2013; Wan and Krassioukov, 2014; Evonuk et al., 2017; Sharif and Hou, 2017). Diabetes in mice increases IL-6, TNFα, GFAP, and Iba-1 expression in the hippocampus (Bhusal et al., 2019). Diabetes impacts hippocampus function, reducing cell proliferation in the dentate gyrus (Stranahan et al., 2008), number of neurons in the hilus (Beauquis et al., 2006) and total number of hippocampal neurons (Bhusal et al., 2019). Diabetes is associated with a reduction in learning efficiency, memory, and spatial cognition in mice (Alvarez et al., 2009; Bhusal et al., 2019) and verbal fluency in women (Kanaya et al., 2004). Stroke (transient global ischemia) in rats, increases astrocyte mediated iNOS production in the hippocampus (Endoh et al., 1994). Stroke in mice impairs hippocampus-dependent spatial memory hypothesized to be caused by aberrant neurogenesis failing to integrate newborn neurons properly (Woitke et al., 2017). Strokes that induced hippocampal infarction in humans led to problems in recognition and long-term memory (Szabo et al., 2009). 7 days after SCI, the death of hepatocytes increase TNF-α and IL-1β expression more than 10-fold in the liver. These changes progress liver dysfunction (Sauerbeck et al., 2015) and non-alcoholic steatohepatitis, leading to metabolic dysfunction during the chronic phases (Goodus and McTigue, 2020). SCI impairs liver function, causing lipid accumulation (Sauerbeck et al., 2015) leading to a 2- fold higher rate of non-alcoholic fatty acid liver disease (NAFLD). NAFLD leads to brain hypoperfusion and reduces both cognitive (Pinçon et al., 2019) and memory capacity (Ross et al., 2012). Those with NAFLD also had diminished testosterone levels (Barbonetti et al., 2016) which is essential for the survival of newborn hippocampal neurons (Spritzer and Galea, 2007)and neuroplasticity (Wainwright et al., 2011). 58.9% of individuals with SCI have low high density lipoprotein cholesterol (HDL-C) (Vichiansiri et al., 2012) which is common in women with severe depression (Ramachandran Pillai et al., 2018) and is associated with memory deficits in humans (Singh-Manoux et al., 2008). Reduction in HDL-C in humans is also associated with reduced gray matter in the parahippocampul region (Ward et al., 2010) which is involved in spatial memory in humans (Bohbot et al., 2015). Cardiometabolic disorders are undoubtedly associated with chronic inflammation (Lopez-Candales et al., 2017) and can influence hippocampal neurogenesis, although, the direct effects of cardiometabolic disorders caused by SCI on neurogenesis have yet to be evaluated.
Gastrointestinal-Renal Disorders and Neurogenesis
People living with SCI commonly have major bowel dysfunctions. In fact, 18-73% of them develop megacolon (Harari and Minaker, 2000; Faaborg et al., 2011) which is often associated with inflammatory bowel disease (IBD) and hypothesized to be caused by infections (Hommes et al., 2004; Autenrieth and Baumgart, 2012). IBD quickly increases IL-6, TNFα, IL-10, and IL-1β levels in the hippocampus, resulting in a short-term increase in hippocampal neurogenesis. In the chronic stages, IBD decreases overall proliferation (Zonis et al., 2015), migration, and integration of newborn hippocampal neurons (Gampierakis et al., 2020). Mice with SCI have gut dysbiosis associated with both activation and phenotypical alteration of the immune cells in gut-associated lymphoid tissues (Kigerl et al., 2016). Clostridium saccharogumia is depleted in the intestines of rats with SCI (O’Connor et al., 2018). Clostridium saccharogumia produces enterolactone in the gut of rats (Woting et al., 2010) which is associated with reducing the risk of cardiovascular disease which might indirectly improve hippocampal neurogenesis. Several studies have analyzed SCI-induced changes in the microbiota (Kigerl et al., 2018; Bannerman et al., 2021; Du et al., 2021), although, it is unclear how these changes can impact hippocampal neurogenesis. Determining the influence of SCI-induced changes to the microbiota and altering it to increase hippocampal neurogenesis is a developing area of research (Jogia and Ruitenberg, 2020; Tang et al., 2021). SCI induces the dysfunction of many peripheral organs which can all participate in the reduction of neurogenesis, although, the direct influence of SCI-induced peripheral organ dysfunction on neurogenesis needs further evaluation.
Respiratory Disease and Neurogenesis
Respiratory disease is a major problem in the SCI community; persons with SCI have a 46.9-fold and 37.1-fold higher probability of dying from pulmonary emboli and pneumonia, respectively (DeVivo et al., 1993). Inability to cough can prevent the clearing of contaminants from the lungs, further increasing infection rate. Atelectasis, commonly occuring in SCI patients, decreases areation, coupled with the loss of neurological control of breathing and coughing transforms the lungs into a breeding ground for pathogens causing pnemonia and further activating inflammatory pathways (Berlowitz et al., 2016). Decreased lung and chest wall compliance may cause the reduction in vital capacity seen in SCI patients (Brown et al., 2006) lessening oxygen consumption efficiency by 46% (Manning et al., 1992). Chronic hypoxia, common among SCI patients, degenerates neural cells (Hernandez-Gerez et al., 2019). Persons with SCI have higher rates of sleep apnea (Khuu et al., 2019) and significantly lower oxygen saturation levels (Fuller et al., 2013), which perturbs hippocampal neurogenesis and synaptic plasticity (Khuu et al., 2019). Furthermore, lipogenesis, which depends on oxygen intake, is required for the proliferation of NSC/NPC and hippocampal neurons (Knobloch et al., 2017) and may also contribute to the 69.8% increase in prevalence of metabolic syndrome in adolescents with chronic SCI (Nelson et al., 2007) creating yet another positive feedback loop. It would be interesting to assess how different regimens of intermittent hypoxia, which increases hippocampal HIF-1α levels (Tregub et al., 2020), could be used to enhance hippocampal neurogenesis. Current research shows intermittent, but not chronic, hypoxia can promote NSC/NPC proliferation and increase BDNF expression, hippocampal neurogenesis, and cognition (Zhu et al., 2005; Zhu et al., 2010; Meng et al., 2020).
Hormone Imbalance and Neurogenesis
Hormonal imbalance, regardless of the cause, contributes to a dysfunctional immune system and influences hippocampal neurogenesis. Endocrine disorders are common in people with SCI (Bauman et al., 2017; Gater et al., 2019). Epinephrine and norepinephrine are significantly lower in SCI patients (Schmid et al., 1998). Epinephrine counteracts anaphylaxis (Kemp et al., 2008), consequently, the probability of allergic reactions increase, increasing inflammation in the brain (Sarlus et al., 2012). It is hypothesized that the increase in hippocampal neurogenesis after exercise is mediated through epinephrine (Ahmadiasl et al., 2003), therefore, any reduction in epinephrine production may decrease the beneficial effects of exercise (Petersen and Pedersen, 2005). Norepinephrine is required for antibody production (Kohm and Sanders, 1999), therefore, a lack thereof may also relate to a dysfunctional immune system and increased prevalence of infections. Norepinephrine reuptake inhibitors reduce neuroinflammation (O’Sullivan et al., 2009) and stimulate NSC/NPC proliferation meditated by the β3-adrenergic receptor (Jhaveri et al., 2010). The stimulation of the β3-adrenergic receptor not only inhibits the LPS/TLR4-induced inflammatory response, but also induces anti-inflammatory effects in macrophages (Hadi et al., 2017). Elevated levels of cortisol and dehydroepiandrosterone sulfate (DHEAS) are present during chronic SCI (Campagnolo et al., 1999; Popovich et al., 2001; Prüss et al., 2017). Like many of the other signaling molecules, the effects of both cortisol and DHEAS are dose dependent. DHEAS increases hippocampal plasticity in the CA1 region, spatial learning and memory only at low doses (Diamond, 2004). Low dose treatment of cortisol increased NPC proliferation, reducing DCX + neuroblasts and favoring astrocyte differentiation, while high dose cortisol treatment impairs both NPC proliferation and hedgehog signaling while increasing FoxO3A signaling (Anacker et al., 2013). Sonic hedgehog signaling increases NPC proliferation (Lai et al., 2003) and neurogenesis during development (Komada et al., 2008). FoxO deletion in adults increases NSC/NPC proliferation but impairs the autophagy flux (Schäffner et al., 2018). Therefore, abolishing cortisol levels may induce more inflammatory dysregulation while low cortisol levels seen in healthy adults may still provide the benefits of FoxO-induced autophagy flux. This provides another example of the need to properly modulate signaling pathways rather than eradicate them.
Compounding Effects of SCI and Aging
Aging is highly associated with reduced hippocampal neurogenesis (Knoth et al., 2010) and cognitive capacities such as memory, attention, and executive cognitive functions (Murman, 2015). SCI increases the prevalence of age-associated disorders, such as neurodegenerative diseases (Huang et al., 2017) and cognitive deficits (Craig et al., 2017). We hypothesize that older individuals with SCI are more susceptible to aberrant immune function leading to greater incidence rates for disorders associated with hippocampal function. With improvement in modern medicine, we are seeing an unprecedented increase in life expectancy; an increase of 5.5 years just from the year 2000 (World Health Organization, 2020). Although there is a notable increase in life expectancy for people living with SCI, the increase in lifespan has not been comparable to the general population (Groah et al., 2012). Chronic inflammatory diseases are increasingly the most common cause for mortality in the overall population (Remington and Brownson, 2011). Aging with chronic inflammation, as seen in persons with SCI, decreases our ability to regenerate damaged tissue, increasing susceptibility to fatal infections and injuries (Xia et al., 2016). Healthy aging is usually associated with elevated IL-10 and reduced IL-6 levels; however, people requiring increased resistance to infections, such as those with SCI, generally have elevated IL-6 and decreased IL-10 expression instead (Caruso et al., 2004). When comparing healthy uninjured ∼ 7-, ∼ 13-, and ∼23-month-old rats, no significant changes were found in the expression of TNF-α, CXCL10, VEGF, IL-1α, IL-1β, and IL-2/4/6/10/17/18 hippocampal cytokine levels (Scheinert et al., 2015). Therefore, increased pro-inflammatory cytokines production seen in those suffering from chronic SCI is likely directly induced by SCI rather than aging itself. Furthermore, age diminishes microglial sensitivity to IL-4, a pleiotropic anti-inflammatory cytokine associated with polarization toward anti-inflammatory and wound-resolution microglial phenotypes (Chatterjee et al., 2014), which reduces a person’s ability to recovery from SCI (Fenn et al., 2014). Therefore, the age factor exacerbates the inflammatory pattern increasing susceptibility to inflammatory diseases such as diabetes, cardiovascular disease, rheumatoid arthritis, respiratory infections, and gastrointestinal-renal disorders. In conclusion, aging itself does not lead to inflammatory diseases yet aging makes one less tolerant to fluctuations in homeostasis; therefore, aging persons with SCI are more likely to succumb to inflammatory diseases which leads to greater reductions in hippocampal neurogenesis.
Aging itself does lead to a great variety of cellular processes such as more senescent cells (Rea et al., 2018) and an age-dependent decline in autophagy efficiency, increasing the probability of cytoplasmic protein aggregation leading to a higher prevalence of neurodegenerative diseases (Nah et al., 2015). Furthermore, aging decreases mitochondrial enzyme efficiency, respiratory capacity, and phosphocreatine recovery time (Sun N. et al., 2016). Mitochondria undergo morphological changes during neurogenesis, shifting from glycolysis to oxidative phosphorylation as the newly differentiated neurons lose glycolysis associated genes (Shin et al., 2015). The impairment of mitochondrial bioenergetics prohibits the differentiation of NPCs into neurons (Lorenz et al., 2017), reduces NSC/NPC proliferation and newborn hippocampal neurons, leading to cognitive deficits (Voloboueva and Giffard, 2011; Khacho et al., 2017). SCI also impairs mitochondrial bioenergetics in the spinal cord (Sullivan et al., 2007). It has yet to be determined if SCI induces mitochondrial impairments in the hippocampus. Since both the mental health of an individual and newborn neurons are reliant on oxidative phosphorylation, aging SCI patients often endure many neurological and psychiatric symptoms at higher rates (Son and Han, 2018). We hypothesize that the age-induced decline in mitochondrial function and efficiency is compounded by the effects of SCI induced mitochondrial dysfunction leading to greater reductions in adult hippocampal neurogenesis resulting in higher rates of cognitive decline during chronic SCI. This theory remains to be tested; however, if correct, targeting mitochondrial activity will potentially enhance neurogenesis and reduce the SCI-induced memory and cognitive dysfunctions.
Potential Mechanisms Modulating Neurogenesis After Chronic SCI
There currently is no consensus on the exact mechanism for the reduction of neurogenesis after SCI and very few articles investigated this matter. Some potential pathways altering neurogenesis in other diseases and associated with SCI, include the increased pro-inflammatory cytokine production, glial cell activation and reduced autophagy. Here, we will discuss SCI-induced changes that have potential to modulate hippocampal neurogenesis.
Impact of Pro-inflammatory Cytokines Associated With SCI on Neurogenesis
Spinal Cord Injury is associated with increased pro-inflammatory cytokine expression in the brain, such as TNF-α, IL-6 (Wu et al., 2014), and IL-1β (Jure et al., 2020), which have potential to reduce adult hippocampal neurogenesis. In numerous pathologies and diseases leading to a reduction in hippocampal neurogenesis, pro-inflammatory cytokines have been implicated as the main culprit. Chronic IL-1β expression leads to neurodegeneration, impaired memory, and EAE (Ferrari et al., 2006; Shaftel et al., 2008; Hewett et al., 2012; Lin and Edelson, 2017) by activating STAT3-induced astrogliosis inhibiting neuronal differentiation (Chen et al., 2013). While exercise is a potent activator of hippocampal neurogenesis (Rhodes et al., 2003; Liu and Nusslock, 2018; Trinchero et al., 2019), IL-1β can prevent the exercise-induced rescue of hippocampal neurogenesis (Wu et al., 2012). Sustained overexpression of IL-1β in the hippocampus directly impedes hippocampal neurogenesis (Wu et al., 2013; Pawley et al., 2020) and mediates the decrease of adult hippocampal neurogenesis after ethanol exposure (Crews, 2012).
IL-1β expression is also increased by GSK-3β which inhibits Wnt signaling (Badimon et al., 2019) and orphan nuclear receptor tailless homolog (TLX) expression involved in NSC maintenance and fate determination (Zhao et al., 2009; Islam and Zhang, 2015). GSK-3β inhibition, which in of itself increases hippocampal neurogenesis (Guo et al., 2012; Morales-Garcia et al., 2012), significantly reduces the effects of IL-1β mediated inhibition of TLX which also increases hippocampal neurogenesis (Green and Nolan, 2012; Ryan et al., 2013). The deletion of TLX not only decreases hippocampal neurogenesis (Kozareva et al., 2018), but may also increase susceptibility to microglial-induced hippocampal inflammation (Kozareva et al., 2019) which can further impede neurogenesis. GSK-3β is also an autophagy inhibitor mediated by mammalian target of rapamycin (mTOR) complex 1 (Mancinelli et al., 2017) and its inhibition successfully increased autophagy (Weikel et al., 2016). Therefore, GSK-3β is a vital target for recovery, as impairing autophagy leads to a dysfunctional immune system (Wang et al., 2019; Pehote and Vij, 2020).
Chronic NF-κB activation, mediated by neuronal NOS (Zhu et al., 2018), suppresses hippocampal neurogenesis (Koo et al., 2010). Chronic IL-6 has also shown to impair hippocampal neurogenesis (Vallières et al., 2002) although its effects are time- and context-dependent. During development, bursts of IL-6 production increase the NSC population while long-term IL-6 exposure reverses its effects on NSCs (Storer et al., 2018). The dramatic increase in IL-6 production after focal cerebral ischemia is hypothesized to prevent neuronal death (Loddick et al., 1998). TNF-α activates NF-κB signaling, decreasing NSC proliferation (Chen and Palmer, 2013) and hippocampal neurogenesis (Iosif et al., 2006). TNF-α expression also significantly reduces the neurite length and branching points of neurons (Neumann et al., 2002). Therefore, prolonged exposure to pro-inflammatory cytokines induced by SCI is likely to inhibit different stages of the hippocampal neurogenesis. Consequently, each cytokine might reduce hippocampal neurogenesis differently, and therefore impact neurological functions differently. It will be interesting to determine the exact mechanisms of each cytokines, and the precise stage of neurogenesis they might interfere with.
Hippocampal Microglia and Neurogenesis
Microglia are the resident immune cells of the CNS, responsible for phagocytosis, nurturing neural cells, and are neuroprotective during infections and injury (Rock et al., 2004; Lenz and Nelson, 2018). Just 1-week post SCI, the number of activated microglia in the injury site increases ∼9-fold and remains elevated by ∼4-fold 4-weeks post injury (Zhou et al., 2018). In humans, increased activation of microglia has been found in the injury site months following SCI (Fleming et al., 2006). In the hippocampus, microglia are essential for hippocampal neurogenesis and neuronal differentiation (Appel et al., 2018). Microglial depletion after SCI alleviated depression, cognitive dysfunction, and neuron death in the hippocampus (Li et al., 2020b). Yet, activated microglia during chronic inflammation secrete cytokines to induce apoptosis before phagocytizing hippocampal neurons (Ekdahl et al., 2003), inhibiting neuronal differentiation and favoring astrocytic differentiation (Diaz-Aparicio et al., 2020). Hippocampal microglia activation is known to impair NPC proliferation and hippocampal neurogenesis in non-SCI models (Stefani et al., 2018). After SCI, the number of activated and total microglia in the hippocampus of rats increase in a severity-dependent manner at 60 dpi while the number of ramified microglial remain unchanged (Jure et al., 2017). However in mice, at 50 dpi, IL-1β, IL-18 and TNF-α expression are all significantly increased in the hippocampus while the number of microglial cells in the hippocampus are reduced (Jure et al., 2020). Possible reasonings for the increase in cytokine production in mice during the decrease in microglial cells are that these cytokines are being produced by other neural cells, such as astrocytes (Cekanaviciute and Buckwalter, 2016), or the hippocampal microglia are overactive and produce more cytokines locally, or a combination of the two. There are several reasons for the discrepancy between Jure’s (2017, 2020) study. Jure’s (2020) study only analyzed the brains of mice with moderate SCI yet Jure’s (2017) study analyzed the brains of rats with SCI of different severities. As previously mentioned, mice and rats have different inflammatory responses to SCI (Sroga et al., 2003). The moderate injury in the 2020 study might not have evoked enough of a response to elevate the number of microglia in the hippocampus of mice at 50 dpi. The 2020 study used Iba-1 (cytoplasm protein) marker in contrary to the Ox42 (membrane protein) marker used in the 2017 study. The 2017 study is expected to see a larger increase in microglia compared to the 2020 study even though microglial response between studies should not be compared due to changes in specie models which evoke different inflammatory responses (Sroga et al., 2003). Even at 90 dpi, IL-6, TNF-α, and iNOS expression remains elevated in the hippocampus. IL-10 expression in the hippocampus is reduced at 8 dpi while being elevated at 90 dpi (Wu et al., 2014); although anti-inflammatory, IL-10 reduces neuron differentiation and neurogenesis (Perez-Asensio et al., 2013).
Chronic overactivation of microglia in the hippocampus might also be due to the increased prevalence of infections in persons with SCI. Bacterial infections activating the TLR4 receptor on microglia through NF-κB signaling activate microglial production of TNF-α, IL-1β, iNOS, ROS, and NO, increasing neuroinflammation (Fernandez-Lizarbe et al., 2009; Yao et al., 2013). In short duration, NO promotes hippocampal NSC proliferation, but long-term exposure to NO is detrimental to newborn neuron survival (Carreira et al., 2015). ROS are generated in small quantities during normal adult hippocampal neurogenesis (Walton et al., 2012), but can be toxic to neurons in large quantities, causing DNA damage (Hemnani and Parihar, 1998) and increasing susceptibility to neurodegenerative disorders (Gilgun-Sherki et al., 2001). Microglia are activated for neuroprotection, yet, similar to other inflammatory pathways, overactivation leads to aberrant behavior resulting in declined hippocampal neurogenesis and is associated with cognitive deficits (Wu et al., 2014).
Microglia also have a great impact on the activity and proliferation of other neural cells, such as astrocytes, regardless of the pathology or location. Chronically activated microglia mediate astrogliosis (Zhang et al., 2010), a transformation of astrocytes to an activated state (Sofroniew, 2014). Microglial production of lymphotoxin, TNF, and IL-6 are mitogenic for astrocytes (Barna et al., 1990; Selmaj et al., 1990), while production of prostaglandin (PG)D2 and IL-1β mediates astrogliosis (Herx and Yong, 2001; Mohri et al., 2006). The role of astrocytes in hippocampal neurogenesis is far-reaching. Astrocytes assist in integrating newborn hippocampal neurons into the existing neuron network (Krzisch et al., 2015) and are essential in preserving the integrity of the blood brain barrier (Lien et al., 2012). After SCI, the population of reactive astrocytes is increased across the hippocampus at both 7 and 50 dpi (Jure et al., 2020). It is not clear if this astrocytic response is the cause or the consequence of the increase in the microglial activation and if the local increase of pro-inflammatory molecules from astrocytes and microglia in the hippocampus are reducing neurogenesis. Indeed, an alternative hypothesis would be that molecules transported systemically from the spinal lesion site to the hippocampus directly reduce neurogenesis and increase the activation of astrocytes and microglia. As mentioned above, another possibility is that systemic inflammation induces hippocampal microglia activation and astrogliosis, which in turn secrete local molecules reducing hippocampal neurogenesis. However, whether these beneficial effects are the direct results of microglia depletion in the hippocampus, or indirectly through changes in astrocyte reactivity remains to be determined.
Alteration of Debris Removal, Autophagy, and Intracellular Processes After Chronic SCI
Autoimmunity is a significant threat to longevity, causing aberrant and chronic inflammatory responses. After SCI, microglia participate in the phagocytosis of cell debris (Green et al., 2019) and prune neurons through NF-κB activated astroglial release of complement C3 (Stevens et al., 2007; Chu et al., 2010). Defective clearance of apoptotic cells in any region has been attributed to the progression of autoimmune disorders (Schwab et al., 2014). The debris from the apoptotic cells acts as a survival signal for B cells while the left over nucleic acids mimic viral infections (Munoz et al., 2010). This aberrant B-cell activation leads to dysregulation of antibody production, and autoimmune reactions to CNS proteins (Ankeny et al., 2006). Reduction of debris removal in the spinal cord after chronic SCI is likely to participate in the chronic inflammation state, indirectly reducing hippocampal neurogenesis. Alternatively, if SCI induces debris accumulation in the hippocampus during chronic SCI, it might participate in the higher prevalence of neurodegeneration seen in persons with SCI (Amanat et al., 2019). However, the increase in the debris accumulation in the hippocampus after SCI and respective mechanisms remain to be demonstrated.
Autophagy flux is the process of degradation of cytoplasmic proteins and organelles (du Toit et al., 2018) which is vital for proper oligodendrocyte function (Bankston et al., 2019), minimizing neuronal damage (Tang et al., 2014), and essential for improving locomotor function (Saraswat Ohri et al., 2018). Autophagosomes’ ability to degrade LPS containing pathogens (Bah and Vergne, 2017; Hagio-Izaki et al., 2018) and decrease IL-1β secretion (Harris et al., 2011; Iula et al., 2018) should modulate the activity of the innate immune system. Although autophagosomes accumulate after SCI (Zhang et al., 2014; Muñoz-Galdeano et al., 2018), autophagy flux in the spinal cord is impaired (Liu S. et al., 2018). Impaired autophagy flux, which can induce neurodegeneration (Hara et al., 2006; Komatsu et al., 2006; Liu S. et al., 2015), has been implicated to cause inflammation and autoimmune disorders in many disease models (Hsieh et al., 2017; Lambelet et al., 2018; Vij et al., 2018), especially in neurogenerative diseases (Nixon, 2013). Using the FIP200 knockdown model for studying autophagy, Wang et al. found autophagy improves NSC survival, proliferation and differentiation into neurons, assists with NSC maintenance, and overall increases adult neurogenesis (Wang et al., 2013). Further experimentation is required to determine how SCI directly influences autophagy in the hippocampus.
Spinal Cord Injury patients, on average 28 years after injury, displayed a 525% increase in IL-6, 115.9% increase in circulating vascular adhesion molecule (sVCAM)-1, and 44.4%increase in endothelin-1 (Wang et al., 2007). This may be due to SCI-induced alterations in gene expression, as patients over a year after injury had natural killer (NK) related genes under expressed while TLR, TNF-α, epidermal growth factor (EGF), IL, insulin, CXCR4, and PDGF pathways were over expressed (da Silva Alves et al., 2013; Herman et al., 2018). These significant changes may be due to SCI-induced sympathetic nervous system dysfunction (Campagnolo et al., 1994) or through a perpetual cycle of chronic inflammation, infections, and dysfunctional immune system. NK and its constituent, 12/15-lipoxygenase, responsible for destroying and phagocytizing viral particles, are significantly downregulated even several years after SCI (Campagnolo et al., 2008; Uderhardt et al., 2012). NLRP3 inflammasomes and associated pro-inflammatory pathways are further activated, followed by dysfunctional autophagosomes increasing the presence of bacterial LPS (Mouasni et al., 2019) while the prevalence of infections increases (Evans et al., 2008; Tofte et al., 2017). This predicament creates a simultaneous increase in inflammatory cytokine production and infection rate. Overactivation of the NLRP3 inflammasome pathway can be harmful as it induces microglia mediated IL-1β secretion (Pan et al., 2014) which is already elevated due to the lack of inhibition from the dysfunctional autophagosomes and increased LPS presence. This is compounded by diminished response to newer threats as antibody production and CD8 + T cell response are both impaired, increasing the probability of people with SCI succumbing to otherwise non-fatal infections (Bracchi-Ricard et al., 2016). This creates a positive feedback loop where autophagy disfunction increases the prevalence of both autoimmune inducing debris formation and infection inducing pathogens, both increasing pro-inflammatory pathways which further increases the prevalence of inflammation inducing pathogenesis; altogether, increasing the probability of impaired hippocampal neurogenesis. Altogether, changes in debris removal, autophagy, and intracellular processes in the chronic phases of SCI, either at the spinal cord lesion site or in the hippocampus, via systemic or direct effects on the neurogenic niche, may play a complex and important role in the modulation of neurogenesis and must be further evaluated in the context of SCI.
SCI-Induced Endoplasmic Reticulum Stress in the Hippocampus and Neurogenesis
Endoplasmic reticulum (ER) is an essential part of all cells for sorting, packaging, and modifying proteins vital for cell survival. ER stress is associated with several neurodegenerative diseases, ischemia, diabetes, bipolar disorder and can lead to cell death (Raghubir et al., 2011). GRP78, a marker for ER stress (Lee, 2005), is still elevated in the hippocampus 4 months post SCI in a linear relationship to the severity of the SCI (Wu et al., 2016) implicating SCI causes chronic ER stress. Chronic ER stress has been shown to elevate inflammatory markers, CXCL3 and CXCL10 (Ha et al., 2015), induce neuronal death (Hood et al., 2018), reduce NSC proliferation (Yang et al., 2020), and impair autophagy (Ogata et al., 2006). JNK, which is dysregulated by chronic inflammation (Lessel et al., 2017), mediates the ER stress-induced impairment of autophagy, and inhibits the maturation of newborn hippocampal neurons (Mohammad et al., 2018). CCL21, which is also elevated 4 months post SCI in the hippocampus (Wu et al., 2016), is mediated by CXCL3 alongside CXCL10 to activate microglia (Rappert et al., 2002; Lessel et al., 2017). The continuous CCL21 accumulation in the hippocampus after SCI may lead to chronic microglial activation and is hypothesized to be the reason there is chronic microglial activation in areas distant from the injury site (de Jong et al., 2005) such as the hippocampus. ER stress and associated processes in hippocampal cells can be one mechanism of reduced hippocampal neurogenesis during chronic SCI. The demonstration that SCI-induced ER stress directly reduces neurogenesis has not been established yet.
Conclusion
Spinal Cord Injury-induced inflammation, whether directly or indirectly induced, has a tremendous impact on longevity and quality of life of persons living with SCI. Inflammation also has vital roles in maintaining a defense system and helping repair and regenerate tissue. The intensity, timing and duration of the inflammatory response is crucial for determining its all-encompassing effects. SCI leads to increased prevalence of many secondary pathologies, such as impaired autophagy, ER stress, chronic pro-inflammatory cytokine production, infections and many others. Consequently, the constantly activated endogenous inflammatory system starts behaving aberrantly in a self-destructive manner which not only decreases the lifespan of persons with SCI, but also substantially reduces their quality of life. These pathologies lead to a perpetual positive feedback loop which generate further complications such as neurodegenerative, gastrointestinal and cardiometabolic disorders which compound the effects of the initial pathologies. As such, many researchers are targeting the immune system. Modulating inflammation appropriately has therapeutic potential; although, modulation has also negatively impacted functional locomotor recovery when incorporated incorrectly (Beck et al., 2010). Therefore, it is vital to holistically study and understand the long-term impact of immune modulation before reaching clinical trials for anti-inflammatory therapeutics.
Therapeutic Options
We will discuss below potential options to treat or prevent the SCI-induced reduction in hippocampal neurogenesis and mitigate cognitive dysfunction and memory impairments in people with SCI. The treatment options discussed herein are intended to either act directly on hippocampal processes or indirectly in a holistic manner to counteract the many secondary pathologies induced by inflammation (Figure 2). Many of the treatment options discussed herein have not been tested to increase neurogenesis in preclinical SCI models or persons living with SCI. Therefore, the efficacy of said treatments are speculative based on their mechanism of action.
Exercise
Exercise has been shown to enhance hippocampal neurogenesis (Rhodes et al., 2003) and increase memory capacity (Loprinzi et al., 2018). Therefore, exercise is a potential treatment option alleviating the reduction of neurogenesis after SCI via increased hippocampal BDNF levels (Hötting et al., 2016), decreased pro-inflammatory cytokine expression, and increased anti-inflammatory cytokine expression (Petersen and Pedersen, 2005; Flynn et al., 2007; da Silva Alves et al., 2013). As previously mentioned, exercise can also increase hippocampal neurogenesis through epinephrine (Ahmadiasl et al., 2003), therefore, impaired epinephrine production (such as in the context of SCI) may decrease the anti-inflammatory effects of exercise. Additionally, exercise is known to reduce susceptibility to depression (Stanton and Reaburn, 2014) and improve psychological wellbeing (Callaghan et al., 2011). Exercising after SCI is a challenge and may not always be an option especially for persons with severe or high cervical injuries. It would be of great clinical interest to test whether new technologies can stimulate muscles to provide some of the benefits associated with exercise (Ragnarsson, 2008). Alternatively, passive range of motion exercises with a partner/caregiver may produce the same impact as regular exercise; although, its effects on inflammation and hippocampal neurogenesis have yet to be elucidated.
Nutrition
Dietary choices have also shown to have a profound impact on the overall inflammatory state of an individual, with or without SCI. As discussed above, we hypothesized that reducing inflammation after SCI is an attractive strategy to target hippocampal neurogenesis. However, how an anti-inflammatory diet might slow down or reverse hippocampal neurogenesis after SCI has yet to be test. A 12-week anti-inflammatory diet (foods with anti-inflammatory effects such as berries, fish, and broccoli) not only decreased pro-inflammatory cytokines, IL-2, and IFN-γ, but also reduced SCI-induced neuropathic pain (Allison et al., 2016). A high-protein diet given to people living with SCI decreases TNF-α levels with improved insulin sensitivity (Li Y. et al., 2018) which increases hippocampal neurogenesis and plasticity (Spinelli et al., 2019). Moreover, mice with SCI on the ketogenic diet (high fat, moderate protein, low carbohydrate) present a reduction in NF-κB, TNF-α, IL-1β, IFN-γ, and oxidative stress levels, activation of Nrf2 (Lu et al., 2018), and improved forelimb motor control (Streijger et al., 2013). Nrf2 activation has anti-oxidative effects vital for aging brains to maintain their rate of hippocampal neurogenesis, specifically, inducing NSC/NPC proliferation. Nrf2 also increases survival and integration of transplanted NSC/NPC (Robledinos-Antón et al., 2017; Ray et al., 2018). In humans, the increased uptake of ω-3 fatty acids mitigates the age-induced cognitive decline (van Gelder et al., 2007). Vitamin B/D/E, flavonoids, and curcumin are also of great interest to target cognitive decline (Gómez-Pinilla, 2008). Specific studies are necessary to demonstrate the direct influence of diet on hippocampal neurogenesis and cognition in persons with chronic SCI.
Alterations of the Microbiome
The use of microbiome alterations for therapeutic purposes has become a rapidly evolving field as gut inflammasomes can influence brain pathology through the gut-brain axis (Rutsch et al., 2020). Recent advancements suggest microbiome alterations have the potential to help persons with SCI by improving locomotor function and immune health (Kigerl et al., 2018). Bacteria are known to directly influence not just our inflammatory pathways, but also neurogenesis and neurological disorders. Therefore, reducing systemic inflammation through modulation of the microbiome might be a strategy to increase neurogenesis. A recent study showed that alteration of the gut microbiome alters hippocampal neurogenesis in both an age- and sex-dependent manner, with young male germ-free mice showing decreased hippocampal neurogenesis and increased neuron apoptosis (Scott et al., 2020). Gut microbiome from 2-year-old mice transplanted into 6-week-old germ-free mice increased the number of newborn hippocampal neurons and local BDNF expression in the short-term while the transplantation of microbiome from 6-week-old mice had no effect (Kundu et al., 2019). It is unclear if this effect is induced by acute inflammation or as a response to a pathology caused by the microbiome transplantation from older mice. Therefore, the contents of, sensitivity to, and effects of microbiome transplantation are age dependent. Specific bacterial strains have shown unique therapeutic potential, although none of the following strains have been tested in pre-clinical models of SCI as way to modulate inflammation, recovery, or hippocampal neurogenesis. Enterococcus faecalis, although inflammasome inducing and frequently found in the pressure ulcers of SCI patients, has shown therapeutic potential to reverse the suppression of inflammatory bowel disease-induced impairment of hippocampal neurogenesis (Takahashi et al., 2019). Another strain from the same genus, Enterococcus durans, significantly decreases IL-1β, IL-17, IFN-γ, IL-6, and CXCL1 expression in the Peyer’s patches of treated mice while supporting local Faecalibacterium prausnitzii populations (Carasi et al., 2017). Faecalibacterium prausnitzii decreases TNF-α and IL-12 expression while increasing IL-10 expression in the colons of mice with Crohn’s disease (Sokol et al., 2008). Bifidobacterium breve has shown promise in increasing hippocampal BDNF levels (O’Sullivan et al., 2011) and suppressing hippocampal inflammation (Kobayashi et al., 2017). A combinational therapy of Lactobacillus helveticus and Bifidobacterium longum attenuates stress-induced suppression of hippocampal neurogenesis (Ait-Belgnaoui et al., 2014). Lactobacillus rhamnosus modulates GABA receptor mRNA expression and provides protection against stress (Bravo et al., 2011; McVey Neufeld et al., 2019). Heat−killed Lactobacillus brevis enhances hippocampal neuron survival and improves memory (Ishikawa et al., 2019). Lastly, Lactobacillus plantarum increases BDNF, serotonin, serotonin transporter, and neurotrophin expression in the brain while elevating intestinal serotonin concentrations with potential antidepressant properties (Choi et al., 2019; Ranuh et al., 2019; Rudzki et al., 2019). Interestingly, fecal transplantation in rats after SCI reduces gut dysbiosis, and the development of anxiety and depression (Schmidt et al., 2020). Both depression and anxiety are associated with the reduction in hippocampal neurogenesis in non-SCI models (Revest et al., 2009; Eisch and Petrik, 2012; Hill et al., 2015). Therefore, an attractive theory would be that fecal transplantation reduces the loss of neurogenesis, via changes in inflammation, ameliorating anxiety/depression in rats with SCI. Altogether, these data suggest a complex relationship between microbiome, inflammation, and modulation of hippocampal neurogenesis. Personalized medicine is likely to be appropriate here to create special blends of probiotic supplements based on the beneficial strains missing from the patient’s gut microbiome. While direct applications to SCI models and hippocampal neurogenesis remain to be tested, the translational potential of probiotic treatment after SCI is of interest to potentially reduce systemic inflammation and ameliorate neurogenesis, memory, and cognition.
Anti-inflammatory Therapeutics
As discussed, we hypothesize that chronic inflammation after SCI is responsible for the reduction in neurogenesis. Suppressing aberrant inflammatory processes during chronic SCI is vital to improving longevity and overall health as many of the secondary pathologies following SCI are chronic inflammatory diseases which generally have a negative impact on hippocampal neurogenesis. Several anti-inflammatory therapeutics have been shown to improve hippocampal neurogenesis and would therefore be potential candidates to minimize secondary pathologies while mitigating the decline in hippocampal neurogenesis. Methylprednisolone perturbs the cytotoxic effects of pro-inflammatory cytokines, such as TNF-α and IL-6, and impedes the activation of T cells while increasing the apoptosis rate of activated immune cells (Sloka and Stefanelli, 2005). After transient cerebral ischemia, acute methylprednisolone treatment increases both the number and migration rate of newborn neurons (Jing et al., 2012) which are essential for the survival and integration of newborn neurons. However, based on 13 clinical studies, acute methylprednisolone treatment has less than a modest effect on neurological function (Hugenholtz et al., 2002) while increasing an SCI patient’s risk of pneumonia 2.6-fold (Gerndt et al., 1997). Erythropoietin, which hinders the actions of pro-inflammatory cytokines (Peng et al., 2020), not only increases locomotor recovery after SCI, but also the number of newborn hippocampal neurons (Zhang et al., 2018). Acute treatment with minocycline, an anti-inflammatory and anti-biotic agent, provides neuroprotection and enhances behavioral recovery, hindlimb function, and strength in mice with SCI (Wells et al., 2003; Elewa et al., 2006). Minocycline also mitigates age-induced spatial learning deficits and increases the number of newborn neurons in the hippocampus of non-injured 3-month old mice (Kohman et al., 2013). Chondroitinase ABC, which degrades pro-inflammatory CSPGs, promotes plasticity in the spinal cord (Barritt et al., 2006) and brain (Chen et al., 2014), augments locomotor recovery following SCI (García-Alías et al., 2011), is neuroprotective (Chen et al., 2014), and prevents social defeat–induced persistent stress memory loss (Riga et al., 2017), all of which can positively impacts hippocampal neurogenesis. Importantly, any treatment improving locomotor function will increase physical activity, thereby likely improving hippocampal neurogenesis and cognitive function. Notably, proper functioning inflammatory systems, especially during development, are vital components of neurogenesis (Storer et al., 2018). Therefore, anti-inflammatory therapeutics must be given to younger patients with extreme caution. All of these anti-inflammatory therapeutic options show promise in enhancing hippocampal function or related cognitive function. None of the SCI patients have received these treatments to directly enhance their cognitive capacity or hippocampal neurogenesis, therefore, more experimentation is required in the context of SCI.
Metabolism and Mitochondria
There is evidence of mitochondrial dysfunction in the spinal cord, with impaired mitochondrial bioenergetics as early as 12 h after SCI (Sullivan et al., 2007). Mitochondria modulate synaptogenesis, fuel hippocampal neurogenesis and assist with cognitive development and memory formation (Arrázola et al., 2019; Khacho et al., 2019). Inducing mitochondrial dysfunction inhibits NSC growth and differentiation into neurons and impairs immature hippocampal neuron maturation (Agnihotri et al., 2017). Therefore, cellular energy capacity can be a limiting factor in hippocampal neurogenesis. Mitochondria have recently been a target of interest for repair strategies, although its specific effects on hippocampal neurogenesis in the context of SCI have yet to be tested. Lithium increases electron transport chain activity, theoretically increasing respiratory capacity of mitochondria. Lithium is hypothesized to reverse the impaired energy generation in the brains’ of bipolar disorder patients (Maurer et al., 2009). Lithium tested on animal SCI models showed inhibition of GSK-3β while doubling the effects of chondroitinase ABC-induced increase in axon regeneration (Yick et al., 2004). Therefore, increasing mitochondrial bioenergetics does have potential in impeding chronic inflammation and improving locomotor function. As previously noted, inhibition of GSK-3β can increase hippocampal neurogenesis (Guo et al., 2012; Morales-Garcia et al., 2012). Cyclosporin A, an immunosuppressant that enhances mitochondrial function through inhibition of mitochondrial permeability transition pore (Scholpa and Schnellmann, 2017), enhances hippocampal neurogenesis by increasing NPC survival and number of newborn neurons (Hunt et al., 2010; Chow and Morshead, 2016).
However, mitochondrial function is not analogous to immunosuppression; mitochondria are integral parts of an active immune system from activating inflammation during infection to pro-inflammatory signaling (Missiroli et al., 2020). Intravenous mitochondria injections not only increased endurance in mice, but also increased energy production and ATP levels in the brain while decreasing neural ROS levels in a Parkinson’s disease model (Shi et al., 2017); ROS generally decreases neuron survival (Drukarch et al., 1998). Recently, mitochondrial transplantation in the spinal cord has been tested in an SCI model, and despite not promoting functional recovery, showed encouraging results for maintaining bioenergetics (Gollihue et al., 2018). Mitochondrial transplantation in a stroke model can mitigate neuron death and ROS and increase neurogenesis in the subventricular zone (Zhang et al., 2019). Altogether, mitochondria-based therapeutics show promise for promoting hippocampal neurogenesis although its mechanism of action is complex and must be further elucidated in the context of SCI.
Extracellular Vesicles
Another promising therapeutic option for increasing hippocampal neurogenesis, reducing inflammation, and improving locomotor function is the use of mesenchymal stem cell derived extracellular vesicles (MSC-EV). MSC-EVs improve functional locomotor recovery after SCI via suppression of the activation of neurotoxic reactive astrocytes and shift microglial polarization from pro-inflammatory to anti-inflammatory profiles (Liu W. et al., 2018; Liu et al., 2020). MSC-EVs effectively decrease astrocyte reactivity and microglial activation after TBI as well (Yang et al., 2017). MSC-EVs mitigate apoptosis of neurons and increase locomotor recovery through the Wnt/β-catenin signaling pathway (Li et al., 2019). The Wnt/β-catenin signaling pathway is essential to hippocampal neurogenesis as it supports the proliferation of NSC/NPC and the maturation and integration of newborn neurons (Zhang et al., 2011; Qu et al., 2013). MSC-EVs promote hippocampal neurogenesis (Reza-Zaldivar et al., 2019; Chen et al., 2020), prevent memory dysfunction after status epilepticus (Long et al., 2017), and improve cognitive impairments in diabetes (Nakano et al., 2016) and Alzheimer’s disease (Nakano et al., 2020) models. MSC-EVs can even be administered intranasally to target forebrain and hippocampal neurons while preserving functional activity preventing undesirable delivery to other regions of the CNS (Kodali et al., 2019). MSC-EVs are currently in a phase 2 clinical trial in stroke patients (Yazdani, 2019); testing in humans in the context of SCI remains to be performed.
Alternative Pathway for SCI-Induced Modulation of Hippocampal Neurogenesis
Although many of the hippocampal changes induced by inflammation is hypothesized to be caused by systemic changes, the hippocampus might also interact with motor neurons possibly providing an alternative pathway for SCI-induced modulation of hippocampal neurogenesis. This hypothesis has yet to be evaluated. The axons of cortical layer V pyramidal cells descend down the corticospinal tract to control locomotion (Fitzpatrick, 2001). SCI-induced damage to these axons causes degeneration (Mittal et al., 2016; Hassannejad et al., 2019) which can modify the overall health of the cortical neurons and may eventually lead to neuronal death (Hu et al., 2012). The neocortex interacts with the hippocampus through axonal projections into the perirhinal cortex (van Strien et al., 2009) which projects to the entorhinal cortex (de Curtis and Paré, 2004). The entorhinal cortex then projects neurons onto area CA1 and the subiculum of the hippocampus (Witter et al., 2017). Neurons from the entorhinal cortex also project axons to the dentate gyrus (Andersen et al., 1969; van Groen et al., 2003). The direct pathway connecting the upper motor neurons to the hippocampus has yet to be fully elucidated. We hypothesize that the cellular responses of the cortical neurons after SCI, the changes in their connectivity and plasticity in the brain, and eventually their death, might influence hippocampal neurogenesis. Furthermore, the voluntary movement of limbs does activate the hippocampus even when learning or memory are not required (Burman, 2019), therefore, there is evidence for constant cross-talk between these regions. Nonetheless, current research has not determined the direct physical connection or communication between the hippocampus and motor cortex and how degeneration in one area can affect the other.
Conclusion
Beyond the evident effect of causing paralysis, spinal cord injuries induce a plethora of health complications due to the dysfunction of peripheral organs. These include, but are not limited to, gastrointestinal, renal, respiratory, hepatic, and cardiometabolic issues. In addition, new evidence demonstrates that SCI also leads to cognitive and memory impairments and neurodegenerative and psychological disorders. One possible reason may be the SCI-induced accelerated decline in the rate of hippocampal neurogenesis compared to identically aged able-bodied individuals. This decline in hippocampal neurogenesis might be caused by the high rate of infections present in people living with SCI, detrimental effects of local and systemic chronic inflammation, hormonal imbalance, autophagy flux and ER impairments, or dysfunction of microglia and astrocytes in the hippocampus. A better understanding of the mechanisms of action of the SCI-induced reduction of neurogenesis at the cellular and molecular level is necessary. Current research is showing promising results in battling against chronic inflammation and neurodegeneration. Diet, exercise, alteration of the microbiome, and anti-inflammatory compounds are therapeutic tools that might be of interest in promoting neurogenesis and reducing SCI-induced neurological disorders. Yet their efficacy and direct effects on hippocampal neurogenesis after SCI remain to be tested. Furthermore, the mechanism of which SCI induces these neurological disorders, and the therapeutic benefits of augmenting hippocampal neurogenesis will have to be determined. This will require a collaboration between researchers, clinicians, and chemists as well as day-to-day caregivers, dieticians, and kinesiologists to develop and test new techniques, compounds, and procedures to help alleviate the harmful effects of chronic inflammation on neurological function while restoring proper immune function to mitigate further secondary pathologies.
Author Contributions
AS and CG contributed equally to the work and approved it for publication.
Funding
CG is a TIRR Foundation Fellow, and is supported by Mission Connect, Craig H. Neilsen Foundation (650332).
Conflict of Interest
The authors declare that the research was conducted in the absence of any commercial or financial relationships that could be construed as a potential conflict of interest.
Acknowledgments
We thank Theresa Sutherland for helping to edit the manuscript. We also thank Graphit Science and Art for preparing the illustrations used in the figures.
References
Agnihotri, S. K., Shen, R., Li, J., Gao, X., and Bueler, H. (2017). Loss of PINK1 leads to metabolic deficits in adult neural stem cells and impedes differentiation of newborn neurons in the mouse hippocampus. Faseb. J. 31, 2839–2853. doi: 10.1096/fj.201600960rr
Ahmadiasl, N., Alaei, H., and Hänninen, O. (2003). Effect of exercise on learning, memory and levels of epinephrine in rats’ hippocampus. J. Sports Sci. Med. 2, 106–109.
Aid, S., Silva, A. C., Candelario-Jalil, E., Choi, S. H., Rosenberg, G. A., and Bosetti, F. (2010). Cyclooxygenase-1 and -2 differentially modulate lipopolysaccharide-induced blood-brain barrier disruption through matrix metalloproteinase activity. J. Cereb. Blood Flow Metab. 30, 370–380. doi: 10.1038/jcbfm.2009.223
Aimone, J. B., Wiles, J., and Gage, F. H. (2009). Computational influence of adult neurogenesis on memory encoding. Neuron 61, 187–202. doi: 10.1016/j.neuron.2008.11.026
Ait-Belgnaoui, A., Colom, A., Braniste, V., Ramalho, L., Marrot, A., Cartier, C., et al. (2014). Probiotic gut effect prevents the chronic psychological stress-induced brain activity abnormality in mice. Neurogastroenterol. Motil. 26, 510–520. doi: 10.1111/nmo.12295
Alam, M. J., Kitamura, T., Saitoh, Y., Ohkawa, N., Kondo, T., and Inokuchi, K. (2018). Adult neurogenesis conserves hippocampal memory capacity. J. Neurosci. 38:6854. doi: 10.1523/jneurosci.2976-17.2018
Alizadeh, A., Dyck, S. M., and Karimi-Abdolrezaee, S. (2019). Traumatic spinal cord injury: an overview of pathophysiology, models and acute injury mechanisms. Front. Neurol. 10:282. doi: 10.3389/fneur.2019.00282
Allen, K. M., Fung, S. J., and Weickert, C. S. (2016). Cell proliferation is reduced in the hippocampus in schizophrenia. Aust. N. Zeal. J. Psychiatry 50, 473–480. doi: 10.1177/0004867415589793
Allison, D. J., Thomas, A., Beaudry, K., and Ditor, D. S. (2016). Targeting inflammation as a treatment modality for neuropathic pain in spinal cord injury: a randomized clinical trial. J. Neuroinflammation 13:152.
Alvarez, E. O., Beauquis, J., Revsin, Y., Banzan, A. M., Roig, P., De Nicola, A. F., et al. (2009). Cognitive dysfunction and hippocampal changes in experimental type 1 diabetes. Behav. Brain Res. 198, 224–230. doi: 10.1016/j.bbr.2008.11.001
Amanat, M., Vaccaro, A. R., Salehi, M., and Rahimi-Movaghar, V. (2019). Neurological conditions associated with spinal cord injury. Inform. Med. Unlocked 16:100245.
Anacker, C., Cattaneo, A., Luoni, A., Musaelyan, K., Zunszain, P. A., Milanesi, E., et al. (2013). Glucocorticoid-related molecular signaling pathways regulating hippocampal neurogenesis. Neuropsychopharmacology 38, 872–883. doi: 10.1038/npp.2012.253
Anacker, C., and Hen, R. (2017). Adult hippocampal neurogenesis and cognitive flexibility — linking memory and mood. Nat. Rev. Neurosci. 18, 335–346. doi: 10.1038/nrn.2017.45
Andersen, P., Bliss, T. V., Lomo, T., Olsen, L. I., and Skrede, K. K. (1969). Lamellar organization of hippocampal excitatory pathways. Acta Physiol. Scand. 76, 4a–5a.
Anderson, C. J., Vogel, L. C., Chlan, K. M., Betz, R. R., and Mcdonald, C. M. (2007). Depression in adults who sustained spinal cord injuries as children or adolescents. J. Spinal. Cord Med. (30 Suppl. 1), S76–S82.
Anderson, M. L., Sisti, H. M., Curlik, D. M. II, and Shors, T. J. (2011). Associative learning increases adult neurogenesis during a critical period. Eur. J. Neurosci. 33, 175–181. doi: 10.1111/j.1460-9568.2010.07486.x
Ankeny, D. P., Lucin, K. M., Sanders, V. M., Mcgaughy, V. M., and Popovich, P. G. (2006). Spinal cord injury triggers systemic autoimmunity: evidence for chronic B lymphocyte activation and lupus-like autoantibody synthesis. J. Neurochem. 99, 1073–1087. doi: 10.1111/j.1471-4159.2006.04147.x
Appel, J. R., Ye, S., Tang, F., Sun, D., Zhang, H., Mei, L., et al. (2018). Increased microglial activity, impaired adult hippocampal neurogenesis, and depressive-like behavior in microglial VPS35-depleted mice. J. Neurosci. 38, 5949–5968. doi: 10.1523/jneurosci.3621-17.2018
Armour, B. S., Courtney-Long, E. A., Fox, M. H., Fredine, H., and Cahill, A. (2016). Prevalence and causes of paralysis-United States, 2013. Am. J. Public Health 106, 1855–1857. doi: 10.2105/ajph.2016.303270
Arrázola, M. S., Andraini, T., Szelechowski, M., Mouledous, L., Arnauné-Pelloquin, L., Davezac, N., et al. (2019). Mitochondria in developmental and adult neurogenesis. Neurotox Res. 36, 257–267.
Badimon, L., Casani, L., Camino-Lopez, S., Juan-Babot, O., and Borrell-Pages, M. (2019). GSK3beta inhibition and canonical Wnt signaling in mice hearts after myocardial ischemic damage. PLoS One 14:e0218098. doi: 10.1371/journal.pone.0218098
Bah, A., and Vergne, I. (2017). Macrophage autophagy and bacterial infections. Front. Immunol. 8:1483. doi: 10.3389/fimmu.2017.01483
Bankston, A. N., Forston, M. D., Howard, R. M., Andres, K. R., Smith, A. E., Ohri, S. S., et al. (2019). Autophagy is essential for oligodendrocyte differentiation, survival, and proper myelination. Glia 67, 1745–1759.
Bannerman, C. A., Douchant, K., Sheth, P. M., and Ghasemlou, N. (2021). The gut-brain axis and beyond: microbiome control of spinal cord injury pain in humans and rodents. Neurobiol. Pain 9:100059. doi: 10.1016/j.ynpai.2020.100059
Barbonetti, A., Caterina Vassallo, M. R., Cotugno, M., Felzani, G., Francavilla, S., and Francavilla, F. (2016). Low testosterone and non-alcoholic fatty liver disease: Evidence for their independent association in men with chronic spinal cord injury. J. Spinal Cord Med. 39, 443–449. doi: 10.1179/2045772314y.0000000288
Barna, B. P., Estes, M. L., Jacobs, B. S., Hudson, S., and Ransohoff, R. M. (1990). Human astrocytes proliferate in response to tumor necrosis factor alpha. J. Neuroimmunol. 30, 239–243. doi: 10.1016/0165-5728(90)90108-y
Barritt, A. W., Davies, M., Marchand, F., Hartley, R., Grist, J., Yip, P., et al. (2006). Chondroitinase ABC promotes sprouting of intact and injured spinal systems after spinal cord injury. J. Neurosci. 26, 10856–10867. doi: 10.1523/jneurosci.2980-06.2006
Bauman, W. A., and Spungen, A. M. (2001). Carbohydrate and lipid metabolism in chronic spinal cord injury. J. Spinal Cord Med. 24, 266–277. doi: 10.1080/10790268.2001.11753584
Bauman, W. A., Wecht, J. M., and Biering-Sørensen, F. (2017). International spinal cord injury endocrine and metabolic extended data set. Spinal Cord 55, 466–477. doi: 10.1038/sc.2016.164
Beauquis, J., Roig, P., Homo-Delarche, F., De Nicola, A., and Saravia, F. (2006). Reduced hippocampal neurogenesis and number of hilar neurones in streptozotocin-induced diabetic mice: reversion by antidepressant treatment. Eur. J. Neurosci. 23, 1539–1546. doi: 10.1111/j.1460-9568.2006.04691.x
Beck, K. D., Nguyen, H. X., Galvan, M. D., Salazar, D. L., Woodruff, T. M., and Anderson, A. J. (2010). Quantitative analysis of cellular inflammation after traumatic spinal cord injury: evidence for a multiphasic inflammatory response in the acute to chronic environment. Brain 133, 433–447. doi: 10.1093/brain/awp322
Bellver-Landete, V., Bretheau, F., Mailhot, B., Vallières, N., Lessard, M., Janelle, M.-E., et al. (2019). Microglia are an essential component of the neuroprotective scar that forms after spinal cord injury. Nat. Commun. 10:518.
Berdugo-Vega, G., Arias-Gil, G., López-Fernández, A., Artegiani, B., Wasielewska, J. M., Lee, C.-C., et al. (2020). Increasing neurogenesis refines hippocampal activity rejuvenating navigational learning strategies and contextual memory throughout life. Nat. Commun. 11:135.
Berlowitz, D. J., Wadsworth, B., and Ross, J. (2016). Respiratory problems and management in people with spinal cord injury. Breathe (Sheff) 12, 328–340. doi: 10.1183/20734735.012616
Bertram, L., and Tanzi, R. E. (2005). The genetic epidemiology of neurodegenerative disease. J. Clin. Invest. 115, 1449–1457. doi: 10.1172/jci24761
Bhusal, A., Rahman, M. H., Lee, I.-K., and Suk, K. (2019). Role of hippocampal lipocalin-2 in experimental diabetic encephalopathy. Front. Endocrinol. 10:25. doi: 10.3389/fendo.2019.00025
Bohbot, V. D., Allen, J. J. B., Dagher, A., Dumoulin, S. O., Evans, A. C., Petrides, M., et al. (2015). Role of the parahippocampal cortex in memory for the configuration but not the identity of objects: converging evidence from patients with selective thermal lesions and fMRI. Front. Hum. Neurosci. 9:431. doi: 10.3389/fnhum.2015.00431
Boldrini, M., Fulmore, C. A., Tartt, A. N., Simeon, L. R., Pavlova, I., Poposka, V., et al. (2018). Human hippocampal neurogenesis persists throughout aging. Cell stem cell 22, 589–599.e585.
Bracchi-Ricard, V., Zha, J., Smith, A., Lopez-Rodriguez, D. M., Bethea, J. R., and Andreansky, S. (2016). Chronic spinal cord injury attenuates influenza virus-specific antiviral immunity. J. Neuroinflam. 13, 125–125.
Brakel, K., Aceves, A. R., Aceves, M., Hierholzer, A., Nguyen, Q. N., and Hook, M. A. (2019). Depression-like behavior corresponds with cardiac changes in a rodent model of spinal cord injury. Exp. Neurol. 320:112969. doi: 10.1016/j.expneurol.2019.112969
Brakel, K., Aceves, M., Garza, A., Yoo, C., Escobedo, G., Panchani, N., et al. (2021). Inflammation increases the development of depression behaviors in male rats after spinal cord injury. Brain Behav. Immun. Health 14:100258. doi: 10.1016/j.bbih.2021.100258
Bravo, J. A., Forsythe, P., Chew, M. V., Escaravage, E., Savignac, H. M., Dinan, T. G., et al. (2011). Ingestion of Lactobacillus strain regulates emotional behavior and central GABA receptor expression in a mouse via the vagus nerve. Proc. Natl. Acad. Sci. U.S.A. 108, 16050–16055. doi: 10.1073/pnas.1102999108
Brown, R., Dimarco, A. F., Hoit, J. D., and Garshick, E. (2006). Respiratory dysfunction and management in spinal cord injury. Respir. Care 51, 853–870.
Bruel-Jungerman, E., Laroche, S., and Rampon, C. (2005). New neurons in the dentate gyrus are involved in the expression of enhanced long-term memory following environmental enrichment. Eur. J. Neurosci. 21, 513–521. doi: 10.1111/j.1460-9568.2005.03875.x
Burghardt, N. S., Park, E. H., Hen, R., and Fenton, A. A. (2012). Adult-born hippocampal neurons promote cognitive flexibility in mice. Hippocampus 22, 1795–1808. doi: 10.1002/hipo.22013
Burman, D. D. (2019). Hippocampal connectivity with sensorimotor cortex during volitional finger movements: laterality and relationship to motor learning. PLoS One 14:e0222064. doi: 10.1371/journal.pone.0222064
Burmeister, A. R., Johnson, M. B., Chauhan, V. S., Moerdyk-Schauwecker, M. J., Young, A. D., Cooley, I. D., et al. (2017). Human microglia and astrocytes constitutively express the neurokinin-1 receptor and functionally respond to substance P. J. Neuroinflam. 14:245.
Bush, T. G., Puvanachandra, N., Horner, C. H., Polito, A., Ostenfeld, T., Svendsen, C. N., et al. (1999). Leukocyte infiltration, neuronal degeneration, and neurite outgrowth after ablation of scar-forming, reactive astrocytes in adult transgenic mice. Neuron 23, 297–308. doi: 10.1016/s0896-6273(00)80781-3
Butturini, E., Boriero, D., Carcereri De Prati, A., and Mariotto, S. (2019). STAT1 drives M1 microglia activation and neuroinflammation under hypoxia. Arch. Biochem. Biophys. 669, 22–30. doi: 10.1016/j.abb.2019.05.011
Cabezas, R., Avila, M., Gonzalez, J., El-Bachá, R. S., Báez, E., García-Segura, L. M., et al. (2014). Astrocytic modulation of blood brain barrier: perspectives on Parkinson’s disease. Front. Cell. Neurosci. 8:211. doi: 10.3389/fncel.2014.00211
Caggiu, E., Arru, G., Hosseini, S., Niegowska, M., Sechi, G., Zarbo, I. R., et al. (2019). Inflammation, infectious triggers, and Parkinson’s Disease. Front. Neurol. 10:122. doi: 10.3389/fneur.2019.00122
Callaghan, P., Khalil, E., Morres, I., and Carter, T. (2011). Pragmatic randomised controlled trial of preferred intensity exercise in women living with depression. BMC Public Health 11:465. doi: 10.1186/1471-2458-11-465
Campagnolo, D. I., Bartlett, J. A., Chatterton, R. Jr., and Keller, S. E. (1999). Adrenal and pituitary hormone patterns after spinal cord injury. Am. J. Phys. Med. Rehabil. 78, 361–366. doi: 10.1097/00002060-199907000-00013
Campagnolo, D. I., Dixon, D., Schwartz, J., Bartlett, J. A., and Keller, S. E. (2008). Altered innate immunity following spinal cord injury. Spinal Cord 46, 477–481. doi: 10.1038/sc.2008.4
Campagnolo, D. I., Keller, S. E., Delisa, J. A., Glick, T. J., Sipski, M. L., and Schleifer, S. J. (1994). Alteration of immune system function in tetraplegics. A pilot study. Am. J. Phys. Med. Rehabil. 73, 387–393. doi: 10.1097/00002060-199411000-00003
Capuron, L., Su, S., Miller, A. H., Bremner, J. D., Goldberg, J., Vogt, G. J., et al. (2008). Depressive symptoms and metabolic syndrome: is inflammation the underlying link? Biol. Psychiatry 64, 896–900. doi: 10.1016/j.biopsych.2008.05.019
Carasi, P., Racedo, S. M., Jacquot, C., Elie, A. M., and Serradell, M. D. L. Á, and Urdaci, M. C. (2017). Enterococcus durans EP1 a promising anti-inflammatory probiotic able to stimulate sIgA and to increase Faecalibacterium prausnitzii abundance. Front. Immunol. 8:88. doi: 10.3389/fimmu.2017.00088
Carlson, S. L., Parrish, M. E., Springer, J. E., Doty, K., and Dossett, L. (1998). Acute inflammatory response in spinal cord following impact injury. Exp. Neurol. 151, 77–88. doi: 10.1006/exnr.1998.6785
Carreira, B. P., Santos, D. F., Santos, A. I., Carvalho, C. M., and Araújo, I. M. (2015). Nitric oxide regulates neurogenesis in the hippocampus following seizures. Oxid. Med. Cell. Longev. 2015, 451512–451512.
Carrica, L., Li, L., Newville, J., Kenton, J., Gustus, K., Brigman, J., et al. (2019). Genetic inactivation of hypoxia inducible factor 1-alpha (HIF-1α) in adult hippocampal progenitors impairs neurogenesis and pattern discrimination learning. Neurobiol. Learn. Memory 157, 79–85. doi: 10.1016/j.nlm.2018.12.002
Caruso, C., Lio, D., Cavallone, L., and Franceschi, C. (2004). Aging, longevity, inflammation, and cancer. Ann. N. Y. Acad. Sci. 1028, 1–13. doi: 10.1155/2012/321653
Cekanaviciute, E., and Buckwalter, M. S. (2016). Astrocytes: integrative regulators of neuroinflammation in stroke and other neurological diseases. Neurotherapeutics 13, 685–701. doi: 10.1007/s13311-016-0477-8
Centers for Disease Control and Prevention (2017). Table 19. Leading Causes of Death and Numbers of Deaths, by Sex, Race, and Hispanic Origin: United States, 1980 and 2016 [Online]. Available online at: https://www.cdc.gov/nchs/data/hus/2017/019.pdf
Chang, Y. K., and Etnier, J. L. (2009). Exploring the dose-response relationship between resistance exercise intensity and cognitive function. J. Sport Exerc. Psychol. 31, 640–656. doi: 10.1123/jsep.31.5.640
Chang, Y. T., Chen, C. L., Lin, C. F., Lu, S. L., Cheng, M. H., Kuo, C. F., et al. (2013). Regulatory role of GSK-3 beta on NF- kappa B, nitric oxide, and TNF- alpha in group A streptococcal infection. Mediators Inflamm. 2013:720689.
Chatterjee, P., Chiasson, V. L., Bounds, K. R., and Mitchell, B. M. (2014). Regulation of the anti-inflammatory cytokines interleukin-4 and interleukin-10 during pregnancy. Front. Immunol. 5:253. doi: 10.3389/fimmu.2014.00253
Chen, B., Tsui, S., and Smith, T. J. (2005). IL-1 beta induces IL-6 expression in human orbital fibroblasts: identification of an anatomic-site specific phenotypic attribute relevant to thyroid-associated ophthalmopathy. J. Immunol. 175, 1310–1319. doi: 10.4049/jimmunol.175.2.1310
Chen, E., Xu, D., Lan, X., Jia, B., Sun, L., Zheng, J. C., et al. (2013). A novel role of the STAT3 pathway in brain inflammation-induced human neural progenitor cell differentiation. Curr. Mol. Med. 13, 1474–1484. doi: 10.2174/15665240113139990076
Chen, H., Li, J., Liang, S., Lin, B., Peng, Q., Zhao, P., et al. (2017). Effect of hypoxia-inducible factor-1/vascular endothelial growth factor signaling pathway on spinal cord injury in rats. Exp. Ther. Med. 13, 861–866. doi: 10.3892/etm.2017.4049
Chen, S.-Y., Lin, M.-C., Tsai, J.-S., He, P.-L., Luo, W.-T., Chiu, I.-M., et al. (2020). Exosomal 2’,3’-CNP from mesenchymal stem cells promotes hippocampus CA1 neurogenesis/neuritogenesis and contributes to rescue of cognition/learning deficiencies of damaged brain. Stem Cells Transl. Med. 9, 499–517. doi: 10.1002/sctm.19-0174
Chen, X.-R., Liao, S.-J., Ye, L.-X., Gong, Q., Ding, Q., Zeng, J.-S., et al. (2014). Neuroprotective effect of chondroitinase ABC on primary and secondary brain injury after stroke in hypertensive rats. Brain Res. 1543, 324–333. doi: 10.1016/j.brainres.2013.12.002
Chen, Z., and Palmer, T. D. (2013). Differential roles of TNFR1 and TNFR2 signaling in adult hippocampal neurogenesis. Brain Behavior Immun. 30, 45–53. doi: 10.1016/j.bbi.2013.01.083
Chen, Z., and Trapp, B. D. (2016). Microglia and neuroprotection. J. Neurochem. 136(Suppl. 1), 10–17. doi: 10.1111/jnc.13062
Cheng, Y.-L., Wang, C.-Y., Huang, W.-C., Tsai, C.-C., Chen, C.-L., Shen, C.-F., et al. (2009). Staphylococcus aureus induces microglial inflammation via a glycogen synthase kinase 3beta-regulated pathway. Infect. Immun. 77, 4002–4008. doi: 10.1128/iai.00176-09
Chiaravalloti, N. D., Weber, E., Wylie, G., Dyson-Hudson, T., and Wecht, J. M. (2020). Patterns of cognitive deficits in persons with spinal cord injury as compared with both age-matched and older individuals without spinal cord injury. J. Spinal Cord Med. 43, 88–97. doi: 10.1080/10790268.2018.1543103
Choi, J., Kim, Y.-K., and Han, P.-L. (2019). Extracellular vesicles derived from lactobacillus plantarum increase BDNF expression in cultured hippocampal neurons and produce antidepressant-like effects in mice. Exp. Neurobiol. 28, 158–171. doi: 10.5607/en.2019.28.2.158
Choi, S. H., Aid, S., Choi, U., and Bosetti, F. (2010). Cyclooxygenases-1 and -2 differentially modulate leukocyte recruitment into the inflamed brain. Pharmacogenomics J. 10, 448–457. doi: 10.1038/tpj.2009.68
Choi, S. H., Langenbach, R., and Bosetti, F. (2008). Genetic deletion or pharmacological inhibition of cyclooxygenase-1 attenuate lipopolysaccharide-induced inflammatory response and brain injury. Faseb. J. 22, 1491–1501. doi: 10.1096/fj.07-9411com
Choi, S. S., Lee, H. J., Lim, I., Satoh, J., and Kim, S. U. (2014). Human astrocytes: secretome profiles of cytokines and chemokines. PLoS One 9:e92325. doi: 10.1371/journal.pone.0092325
Chow, A., and Morshead, C. M. (2016). Cyclosporin A enhances neurogenesis in the dentate gyrus of the hippocampus. Stem Cell Res. 16, 79–87. doi: 10.1016/j.scr.2015.12.007
Christopher & Dana Reeve Foundation (2020). Costs of Living With SCI [Online]. Available online at: https://www.christopherreeve.org/living-with-paralysis/costs-and-insurance/costs-of-living-with-spinal-cord-injury
Christopher & Dana Reeve Foundation (2021). One Degree of Separation, Paralysis and Spinal Cord Injury in the United States [Online]. Available online at: http://s3.amazonaws.com/reeve-assets-production/8112REPTFINAL.PDF
Chu, Y., Jin, X., Parada, I., Pesic, A., Stevens, B., Barres, B., et al. (2010). Enhanced synaptic connectivity and epilepsy in C1q knockout mice. Proc. Natl. Acad. Sci. U.S.A. 107, 7975–7980. doi: 10.1073/pnas.0913449107
Claudia Antonieta Nieves Prado, S. P. (2018). Pneumococcal Infections (Streptococcus Pneumoniae) [Online]. Available online at: https://emedicine.medscape.com/article/225811-overview
Clelland, C. D., Choi, M., Romberg, C., Clemenson, G. D. Jr., Fragniere, A., Tyers, P., et al. (2009). A functional role for adult hippocampal neurogenesis in spatial pattern separation. Science (New York, N.Y.) 325, 210–213. doi: 10.1126/science.1173215
Codo, A. C., Saraiva, A. C., Dos Santos, L. L., Visconde, M. F., Gales, A. C., Zamboni, D. S., et al. (2018). Inhibition of inflammasome activation by a clinical strain of Klebsiella pneumoniae impairs efferocytosis and leads to bacterial dissemination. Cell Death Disease 9, 1182–1182.
Cohen, D. M., Patel, C. B., Ahobila-Vajjula, P., Sundberg, L. M., Chacko, T., Liu, S.-J., et al. (2009). Blood-spinal cord barrier permeability in experimental spinal cord injury: dynamic contrast-enhanced MRI. NMR Biomed. 22, 332–341. doi: 10.1002/nbm.1343
Costa, V., Lugert, S., and Jagasia, R. (2015). Role of adult hippocampal neurogenesis in cognition in physiology and disease: pharmacological targets and biomarkers. Handb. Exp. Pharmacol. 228, 99–155. doi: 10.1007/978-3-319-16522-6_4
Cragg, J. J., Noonan, V. K., Krassioukov, A., and Borisoff, J. (2013). Cardiovascular disease and spinal cord injury: results from a national population health survey. Neurology 81, 723–728. doi: 10.1212/wnl.0b013e3182a1aa68
Craig, A., Guest, R., Tran, Y., and Middleton, J. (2017). Cognitive impairment and mood states after spinal cord injury. J. Neurotrauma 34, 1156–1163. doi: 10.1089/neu.2016.4632
Crews, F. (2012). Inflammasome-IL-1β signaling mediates ethanol inhibition of hippocampal neurogenesis. Front. Neurosci. 6:77. doi: 10.3389/fnins.2012.00077
da Silva Alves, E., De Aquino Lemos, V., Ruiz Da Silva, F., Lira, F. S., Dos Santos, R. V. T., et al. (2013). Low-grade inflammation and spinal cord injury: exercise as therapy? Mediators Inflamm. 2013:971841.
da Silva, T. A., Zorzetto-Fernandes, A. L. V., Cecílio, N. T., Sardinha-Silva, A., Fernandes, F. F., and Roque-Barreira, M. C. (2017). CD14 is critical for TLR2-mediated M1 macrophage activation triggered by N-glycan recognition. Sci. Rep. 7:7083.
Daniel, J. M., Fader, A. J., Spencer, A. L., and Dohanich, G. P. (1997). Estrogen enhances performance of female rats during acquisition of a radial arm maze. Horm. Behav. 32, 217–225. doi: 10.1006/hbeh.1997.1433
Dayer, A. G., Ford, A. A., Cleaver, K. M., Yassaee, M., and Cameron, H. A. (2003). Short-term and long-term survival of new neurons in the rat dentate gyrus. J. Comp. Neurol. 460, 563–572. doi: 10.1002/cne.10675
de Curtis, M., and Paré, D. (2004). The rhinal cortices: a wall of inhibition between the neocortex and the hippocampus. Prog. Neurobiol. 74, 101–110. doi: 10.1016/j.pneurobio.2004.08.005
de Jong, E. K., Dijkstra, I. M., Hensens, M., Brouwer, N., Van Amerongen, M., Liem, R. S. B., et al. (2005). Vesicle-mediated transport and release of CCL21 in endangered neurons: a possible explanation for microglia activation remote from a primary lesion. J. Neurosci. 25:7548. doi: 10.1523/jneurosci.1019-05.2005
Dehler, S., Lou, W. P.-K., Gao, L., Skabkin, M., Dällenbach, S., Neumann, A., et al. (2018). An immune-CNS axis activates remote hippocampal stem cells following spinal transection injury. Front. Mol. Neurosci. 11:443.
Devivo, M. J. (2012). Epidemiology of traumatic spinal cord injury: trends and future implications. Spinal Cord 50, 365–372. doi: 10.1038/sc.2011.178
DeVivo, M. J., Black, K. J., and Stover, S. L. (1993). Causes of death during the first 12 years after spinal cord injury. Arch. Phys. Med. Rehabil. 74, 248–254.
DeWitt, D. A., Silver, J., Canning, D. R., and Perry, G. (1993). Chondroitin sulfate proteoglycans are associated with the lesions of Alzheimer’s disease. Exp. Neurol. 121, 149–152. doi: 10.1006/exnr.1993.1081
Diamond, D. M. (2004). Enhancement of cognitive and electrophysiological measures of hippocampal functioning in rats by a low, but not high, dose of dehydroepiandrosterone sulfate (DHEAS). Nonlinearity Biol. Toxicol. Med. 2, 371–377.
Diaz-Aparicio, I., Paris, I., Sierra-Torre, V., Plaza-Zabala, A., Rodríguez-Iglesias, N., Márquez-Ropero, M., et al. (2020). Microglia actively remodel adult hippocampal neurogenesis through the phagocytosis secretome. J. Neurosci. 40, 1453–1482. doi: 10.1523/jneurosci.0993-19.2019
Dijkstra, S., Kooij, G., Verbeek, R., Van Der Pol, S. M., Amor, S., and Geisert, E. E. Jr., et al. (2008). Targeting the tetraspanin CD81 blocks monocyte transmigration and ameliorates EAE. Neurobiol. Dis. 31, 413–421. doi: 10.1016/j.nbd.2008.05.018
Donnelly, D. J., and Popovich, P. G. (2008). Inflammation and its role in neuroprotection, axonal regeneration and functional recovery after spinal cord injury. Exp. Neurol. 209, 378–388. doi: 10.1016/j.expneurol.2007.06.009
Donzis, E. J., and Tronson, N. C. (2014). Modulation of learning and memory by cytokines: signaling mechanisms and long term consequences. Neurobiol. Learn. Mem. 115, 68–77. doi: 10.1016/j.nlm.2014.08.008
Drapeau, E., Mayo, W., Aurousseau, C., Le Moal, M., Piazza, P.-V., and Abrous, D. N. (2003). Spatial memory performances of aged rats in the water maze predict levels of hippocampal neurogenesis. Proc. Natl. Acad. Sci. U.S.A. 100, 14385–14390. doi: 10.1073/pnas.2334169100
Drukarch, B., Schepens, E., Stoof, J. C., Langeveld, C. H., and Van Muiswinkel, F. L. (1998). Astrocyte-enhanced neuronal survival is mediated by scavenging of extracellular reactive oxygen species. Free Radic. Biol. Med. 25, 217–220. doi: 10.1016/s0891-5849(98)00050-1
Du, J., Zayed, A. A., Kigerl, K. A., Zane, K., Sullivan, M. B., and Popovich, P. G. (2021). Spinal cord injury changes the structure and functional potential of gut bacterial and viral communities. mSystems 6:e01356–20.
Du, X., Fleiss, B., Li, H., D’angelo, B., Sun, Y., Zhu, C., et al. (2011). Systemic stimulation of TLR2 impairs neonatal mouse brain development. PLoS One 6:e19583. doi: 10.1371/journal.pone.0019583
du Toit, A., Hofmeyr, J.-H. S., Gniadek, T. J., and Loos, B. (2018). Measuring autophagosome flux. Autophagy 14, 1060–1071.
Dutta, D., Khan, N., Wu, J., and Jay, S. M. (2021). Extracellular vesicles as an emerging frontier in spinal cord injury pathobiology and therapy. Trends Neurosci. (in press). doi: 10.1016/j.tins.2021.01.003
Dutta, G., Zhang, P., and Liu, B. (2008). The lipopolysaccharide Parkinson’s disease animal model: mechanistic studies and drug discovery. Fundam. Clin. Pharmacol. 22, 453–464. doi: 10.1111/j.1472-8206.2008.00616.x
Dyck, S. M., and Karimi-Abdolrezaee, S. (2018). Role of chondroitin sulfate proteoglycan signaling in regulating neuroinflammation following spinal cord injury. Neural Regen. Res. 13, 2080–2082. doi: 10.4103/1673-5374.241452
Eisch, A. J., and Petrik, D. (2012). Depression and hippocampal neurogenesis: a road to remission? Science (New York, N.Y.) 338, 72–75. doi: 10.1126/science.1222941
Ekdahl, C. T., Claasen, J.-H., Bonde, S., Kokaia, Z., and Lindvall, O. (2003). Inflammation is detrimental for neurogenesis in adult brain. Proc. National Acad. Sci. U.S.A. 100, 13632–13637.
Elewa, H. F., Hilali, H., Hess, D. C., Machado, L. S., and Fagan, S. C. (2006). Minocycline for short-term neuroprotection. Pharmacotherapy 26, 515–521. doi: 10.1592/phco.26.4.515
Elmelund, M., Klarskov, N., and Biering-Sorensen, F. (2018). Prevalence of urinary incontinence in women with spinal cord injury. Spinal Cord 56, 1124–1133. doi: 10.1038/s41393-018-0157-0
Endoh, M., Maiese, K., and Wagner, J. (1994). Expression of the inducible form of nitric oxide synthase by reactive astrocytes after transient global ischemia. Brain Res. 651, 92–100. doi: 10.1016/0006-8993(94)90683-1
Epp, J. R., Spritzer, M. D., and Galea, L. A. (2007). Hippocampus-dependent learning promotes survival of new neurons in the dentate gyrus at a specific time during cell maturation. Neuroscience 149, 273–285. doi: 10.1016/j.neuroscience.2007.07.046
Eriksson, P. S., Perfilieva, E., Bjork-Eriksson, T., Alborn, A. M., Nordborg, C., Peterson, D. A., et al. (1998). Neurogenesis in the adult human hippocampus. Nat. Med. 4, 1313–1317.
Evans, C. T., Lavela, S. L., Weaver, F. M., Priebe, M., Sandford, P., Niemiec, P., et al. (2008). Epidemiology of hospital-acquired infections in veterans with spinal cord injury and disorder. Infect. Control Hosp. Epidemiol. 29, 234–242. doi: 10.1086/527509
Evonuk, K. S., Prabhu, S. D., Young, M. E., and Desilva, T. M. (2017). Myocardial ischemia/reperfusion impairs neurogenesis and hippocampal-dependent learning and memory. Brain Behavior Immun. 61, 266–273. doi: 10.1016/j.bbi.2016.09.001
Faaborg, P. M., Christensen, P., Rosenkilde, M., Laurberg, S., and Krogh, K. (2011). Do gastrointestinal transit times and colonic dimensions change with time since spinal cord injury? Spinal Cord 49, 549–553. doi: 10.1038/sc.2010.162
Faulkner, J. R., Herrmann, J. E., Woo, M. J., Tansey, K. E., Doan, N. B., and Sofroniew, M. V. (2004). Reactive astrocytes protect tissue and preserve function after spinal cord injury. J. Neurosci. 24, 2143–2155. doi: 10.1523/jneurosci.3547-03.2004
Felix, M.-S., Popa, N., Djelloul, M., Boucraut, J., Gauthier, P., Bauer, S., et al. (2012). Alteration of forebrain neurogenesis after cervical spinal cord injury in the adult rat. Front. Neurosci. 6:45. doi: 10.3389/fnins.2012.00045
Fenn, A. M., Hall, J. C., Gensel, J. C., Popovich, P. G., and Godbout, J. P. (2014). IL-4 signaling drives a unique arginase+/IL-1beta+ microglia phenotype and recruits macrophages to the inflammatory CNS: consequences of age-related deficits in IL-4Ralpha after traumatic spinal cord injury. J. Neurosci. 34, 8904–8917. doi: 10.1523/jneurosci.1146-14.2014
Fernandez-Lizarbe, S., Pascual, M., and Guerri, C. (2009). Critical role of TLR4 response in the activation of microglia induced by ethanol. J. Immunol. 183:4733. doi: 10.4049/jimmunol.0803590
Ferrari, C. C., Pott Godoy, M. C., Tarelli, R., Chertoff, M., Depino, A. M., and Pitossi, F. J. (2006). Progressive neurodegeneration and motor disabilities induced by chronic expression of IL-1beta in the substantia nigra. Neurobiol. Dis. 24, 183–193. doi: 10.1016/j.nbd.2006.06.013
Fitzpatrick, P. A. (2001). “The Primary motor cortex: upper motor neurons that initiate complex voluntary movements,” in Neuroscience, 2 Edn, eds D. Purves, G. J. Augustine, and D. Fitzpatrick (Sunderland: Sinauer Associates).
Fleming, J. C., Norenberg, M. D., Ramsay, D. A., Dekaban, G. A., Marcillo, A. E., Saenz, A. D., et al. (2006). The cellular inflammatory response in human spinal cords after injury. Brain 129, 3249–3269. doi: 10.1093/brain/awl296
Flynn, M. G., Mcfarlin, B. K., and Markofski, M. M. (2007). The anti-inflammatory actions of exercise training. Am. J. Lifestyle Med. 1, 220–235.
Fu, H., Zhao, Y., Hu, D., Wang, S., Yu, T., and Zhang, L. (2020). Depletion of microglia exacerbates injury and impairs function recovery after spinal cord injury in mice. Cell Death Dis. 11:528.
Fuller, D. D., Lee, K.-Z., and Tester, N. J. (2013). The impact of spinal cord injury on breathing during sleep. Respir. Physiol. Neurobiol. 188, 344–354. doi: 10.1016/j.resp.2013.06.009
Galtrey, C. M., and Fawcett, J. W. (2007). The role of chondroitin sulfate proteoglycans in regeneration and plasticity in the central nervous system. Brain Res. Rev. 54, 1–18. doi: 10.1016/j.brainresrev.2006.09.006
Gampierakis, I.-A., Koutmani, Y., Semitekolou, M., Morianos, I., Polissidis, A., Katsouda, A., et al. (2020). Hippocampal neural stem cells and microglia response to experimental inflammatory bowel disease (IBD). Mol. Psychiatry 26, 1248–1263. doi: 10.1038/s41380-020-0651-6
García-Alías, G., Petrosyan, H. A., Schnell, L., Horner, P. J., Bowers, W. J., Mendell, L. M., et al. (2011). Chondroitinase ABC combined with neurotrophin NT-3 secretion and NR2D expression promotes axonal plasticity and functional recovery in rats with lateral hemisection of the spinal cord. J. Neurosci. 31, 17788–17799. doi: 10.1523/jneurosci.4308-11.2011
Gater, D. R. Jr., Farkas, G. J., Berg, A. S., and Castillo, C. (2019). Prevalence of metabolic syndrome in veterans with spinal cord injury. J. Spinal Cord Med. 42, 86–93. doi: 10.1080/10790268.2017.1423266
Gerndt, S. J., Rodriguez, J. L., Pawlik, J. W., Taheri, P. A., Wahl, W. L., Micheals, A. J., et al. (1997). Consequences of high-dose steroid therapy for acute spinal cord injury. J. Trauma 42, 279–284. doi: 10.1097/00005373-199702000-00017
Gilgun-Sherki, Y., Melamed, E., and Offen, D. (2001). Oxidative stress induced-neurodegenerative diseases: the need for antioxidants that penetrate the blood brain barrier. Neuropharmacology 40, 959–975. doi: 10.1016/s0028-3908(01)00019-3
Gilmore, J. H., Fredrik Jarskog, L., Vadlamudi, S., and Lauder, J. M. (2004). Prenatal infection and risk for schizophrenia: IL-1beta, IL-6, and TNFalpha inhibit cortical neuron dendrite development. Neuropsychopharmacology 29, 1221–1229. doi: 10.1038/sj.npp.1300446
Goldgaber, D., Harris, H. W., Hla, T., Maciag, T., Donnelly, R. J., Jacobsen, J. S., et al. (1989). Interleukin 1 regulates synthesis of amyloid beta-protein precursor mRNA in human endothelial cells. Proc. Natl. Acad. Sci. U.S.A. 86, 7606–7610. doi: 10.1073/pnas.86.19.7606
Gollihue, J. L., Patel, S. P., Eldahan, K. C., Cox, D. H., Donahue, R. R., Taylor, B. K., et al. (2018). Effects of mitochondrial transplantation on bioenergetics, cellular incorporation, and functional recovery after spinal cord injury. J. Neurotrauma 35, 1800–1818. doi: 10.1089/neu.2017.5605
Gomez-Nicola, D., Suzzi, S., Vargas-Caballero, M., Fransen, N. L., Al-Malki, H., Cebrian-Silla, A., et al. (2014). Temporal dynamics of hippocampal neurogenesis in chronic neurodegeneration. Brain 137, 2312–2328. doi: 10.1093/brain/awu155
Gómez-Pinilla, F. (2008). Brain foods: the effects of nutrients on brain function. Nat. Rev. Neurosci. 9, 568–578. doi: 10.1038/nrn2421
Goodus, M. T., and McTigue, D. M. (2020). Hepatic dysfunction after spinal cord injury: a vicious cycle of central and peripheral pathology? Exp. Neurol. 325:113160. doi: 10.1016/j.expneurol.2019.113160
Gould, E., Beylin, A., Tanapat, P., Reeves, A., and Shors, T. J. (1999). Learning enhances adult neurogenesis in the hippocampal formation. Nat. Neurosci. 2, 260–265. doi: 10.1038/6365
Green, H. F., and Nolan, Y. M. (2012). Unlocking mechanisms in interleukin-1β-induced changes in hippocampal neurogenesis—a role for GSK-3β and TLX. Transl. Psychiatry 2:e194. doi: 10.1038/tp.2012.117
Green, L. A., Nebiolo, J. C., and Smith, C. J. (2019). Microglia exit the CNS in spinal root avulsion. PLoS Biol. 17:e3000159. doi: 10.1371/journal.pbio.3000159
Greene-Schloesser, D., Moore, E., and Robbins, M. E. (2013). Molecular pathways: radiation-induced cognitive impairment. Clin. Cancer Res. 19, 2294–2300. doi: 10.1158/1078-0432.ccr-11-2903
Groah, S. L., Charlifue, S., Tate, D., Jensen, M. P., Molton, I. R., Forchheimer, M., et al. (2012). Spinal cord injury and aging: challenges and recommendations for future research. Am. J. Phys. Med. Rehabil. 91, 80–93.
Guo, W., Murthy, A. C., Zhang, L., Johnson, E. B., Schaller, E. G., Allan, A. M., et al. (2012). Inhibition of GSK3β improves hippocampus-dependent learning and rescues neurogenesis in a mouse model of fragile X syndrome. Hum. Mol. Genet. 21, 681–691. doi: 10.1093/hmg/ddr501
Ha, Y., Liu, H., Xu, Z., Yokota, H., Narayanan, S. P., Lemtalsi, T., et al. (2015). Endoplasmic reticulum stress-regulated CXCR3 pathway mediates inflammation and neuronal injury in acute glaucoma. Cell Death Dis. 6:e1900. doi: 10.1038/cddis.2015.281
Hadi, T., Douhard, R., Dias, A. M. M., Wendremaire, M., Pezzè, M., Bardou, M., et al. (2017). Beta3 adrenergic receptor stimulation in human macrophages inhibits NADPHoxidase activity and induces catalase expression via PPARγ activation. Biochim. Biophys. Acta (BBA) Mol. Cell Res. 1864, 1769–1784. doi: 10.1016/j.bbamcr.2017.07.003
Hagio-Izaki, K., Yasunaga, M., Yamaguchi, M., Kajiya, H., Morita, H., Yoneda, M., et al. (2018). Lipopolysaccharide induces bacterial autophagy in epithelial keratinocytes of the gingival sulcus. BMC Cell Biol. 19:18. doi: 10.1186/s12860-018-0168-x
Han, M.-H., Lee, E.-H., and Koh, S.-H. (2016). Current opinion on the role of neurogenesis in the therapeutic strategies for Alzheimer Disease, Parkinson Disease, and Ischemic Stroke; considering neuronal voiding function. Int. Neurourol. J. 20, 276–287. doi: 10.5213/inj.1632776.388
Han, W., Song, X., He, R., Li, T., Cheng, L., Xie, L., et al. (2017). VEGF regulates hippocampal neurogenesis and reverses cognitive deficits in immature rats after status epilepticus through the VEGF R2 signaling pathway. Epilepsy Behav. 68, 159–167. doi: 10.1016/j.yebeh.2016.12.007
Hansen, D. V., Hanson, J. E., and Sheng, M. (2018). Microglia in Alzheimer’s disease. J. Cell Biol. 217, 459–472.
Hara, T., Nakamura, K., Matsui, M., Yamamoto, A., Nakahara, Y., Suzuki-Migishima, R., et al. (2006). Suppression of basal autophagy in neural cells causes neurodegenerative disease in mice. Nature 441, 885–889. doi: 10.1038/nature04724
Harari, D., and Minaker, K. L. (2000). Megacolon in patients with chronic spinal cord injury. Spinal Cord 38, 331–339. doi: 10.1038/sj.sc.3101010
Harris, J., Hartman, M., Roche, C., Zeng, S. G., O’shea, A., Sharp, F. A., et al. (2011). Autophagy controls IL-1beta secretion by targeting pro-IL-1beta for degradation. J. Biol. Chem. 286, 9587–9597.
Hasezaki, T., Yoshima, T., and Mine, Y. (2020). Anti-CD81 antibodies reduce migration of activated T lymphocytes and attenuate mouse experimental colitis. Sci. Rep. 10:6969.
Hassannejad, Z., Yousefifard, M., Azizi, Y., Zadegan, S. A., Sajadi, K., Sharif-Alhoseini, M., et al. (2019). Axonal degeneration and demyelination following traumatic spinal cord injury: a systematic review and meta-analysis. J. Chem. Neuroanat. 97, 9–22. doi: 10.1016/j.jchemneu.2019.01.009
Haughey, N. J., Nath, A., Chan, S. L., Borchard, A. C., Rao, M. S., and Mattson, M. P. (2002). Disruption of neurogenesis by amyloid beta-peptide, and perturbed neural progenitor cell homeostasis, in models of Alzheimer’s disease. J. Neurochem. 83, 1509–1524. doi: 10.1046/j.1471-4159.2002.01267.x
Hausmann, O. N. (2003). Post-traumatic inflammation following spinal cord injury. Spinal Cord 41, 369–378. doi: 10.1038/sj.sc.3101483
Hemnani, T., and Parihar, M. S. (1998). Reactive oxygen species and oxidative DNA damage. Indian J. Physiol. Pharmacol. 42, 440–452.
Herman, P., Stein, A., Gibbs, K., Korsunsky, I., Gregersen, P., and Bloom, O. (2018). Persons with chronic spinal cord injury have decreased natural killer cell and increased toll-like receptor/inflammatory gene expression. J. Neurotrauma 35, 1819–1829. doi: 10.1089/neu.2017.5519
Hernandez-Gerez, E., Fleming, I. N., and Parson, S. H. (2019). A role for spinal cord hypoxia in neurodegeneration. Cell Death Dis. 10:861.
Herx, L. M., and Yong, V. W. (2001). Interleukin-1 beta is required for the early evolution of reactive astrogliosis following CNS lesion. J. Neuropathol Exp. Neurol. 60, 961–971. doi: 10.1093/jnen/60.10.961
Hewett, S. J., Jackman, N. A., and Claycomb, R. J. (2012). Interleukin-1β in central nervous system injury and repair. Eur. J. Neurodegener. Dis. 1, 195–211. doi: 10.1007/978-4-431-77922-3_47
Hill, A. S., Sahay, A., and Hen, R. (2015). Increasing adult hippocampal neurogenesis is sufficient to reduce anxiety and depression-like behaviors. Neuropsychopharmacology 40, 2368–2378. doi: 10.1038/npp.2015.85
Hofer, S., Grandgirard, D., Burri, D., Frohlich, T. K., and Leib, S. L. (2011). Bacterial meningitis impairs hippocampal neurogenesis. J. Neuropathol. Exp. Neurol. 70, 890–899. doi: 10.1097/nen.0b013e3182303f31
Hoffmann, O., Mahrhofer, C., Rueter, N., Freyer, D., Bert, B., Fink, H., et al. (2007). Pneumococcal cell wall-induced meningitis impairs adult hippocampal neurogenesis. Infect. Immun. 75, 4289–4297. doi: 10.1128/iai.01679-06
Hollands, C., Tobin, M. K., Hsu, M., Musaraca, K., Yu, T.-S., Mishra, R., et al. (2017). Depletion of adult neurogenesis exacerbates cognitive deficits in Alzheimer’s disease by compromising hippocampal inhibition. Mol. Neurodegener. 12, 64–64.
Hommes, D. W., Sterringa, G., Van Deventer, S. J., Tytgat, G. N., and Weel, J. (2004). The pathogenicity of cytomegalovirus in inflammatory bowel disease: a systematic review and evidence-based recommendations for future research. Inflamm. Bowel Dis. 10, 245–250. doi: 10.1097/00054725-200405000-00011
Hood, K. N., Zhao, J., Redell, J. B., Hylin, M. J., Harris, B., Perez, A., et al. (2018). Endoplasmic reticulum stress contributes to the loss of newborn hippocampal neurons after traumatic brain injury. J. Neurosci. 38:2372. doi: 10.1523/jneurosci.1756-17.2018
Hötting, K., Schickert, N., Kaiser, J., Röder, B., and Schmidt-Kassow, M. (2016). The effects of acute physical exercise on memory, peripheral BDNF, and cortisol in young adults. Neural Plasticity 2016:6860573.
Hsieh, C.-W., Chang, C.-Y., Chen, Y.-M., Chen, H.-H., Hung, W.-T., Gung, N.-R., et al. (2017). Impaired autophagic flux and its related inflammation in patients with adult-onset Still’s disease. Oncotarget 9, 110–121. doi: 10.18632/oncotarget.23098
Hsu, W. Y., Muo, C. H., Ma, S. P., and Kao, C. H. (2017). Association between schizophrenia and urinary incontinence: a population-based study. Psychiatry Res. 248, 35–39. doi: 10.1016/j.psychres.2016.12.012
Hu, Y., Park, K. K., Yang, L., Wei, X., Yang, Q., Cho, K.-S., et al. (2012). Differential effects of unfolded protein response pathways on axon injury-induced death of retinal ganglion cells. Neuron 73, 445–452. doi: 10.1016/j.neuron.2011.11.026
Huang, B., Liu, J., Meng, T., Li, Y., He, D., Ran, X., et al. (2018). Polydatin prevents lipopolysaccharide (LPS)-induced Parkinson’s Disease via regulation of the AKT/GSK3β-Nrf2/NF-κB signaling axis. Front. Immunol. 9:2527. doi: 10.3389/fimmu.2018.02527
Huang, S. W., Wang, W. T., Chou, L. C., Liou, T. H., and Lin, H. W. (2017). Risk of dementia in patients with spinal cord injury: a nationwide population-based cohort study. J. Neurotrauma 34, 615–622. doi: 10.1089/neu.2016.4525
Hugenholtz, H., Cass, D. E., Dvorak, M. F., Fewer, D. H., Fox, R. J., Izukawa, D. M., et al. (2002). High-dose methylprednisolone for acute closed spinal cord injury–only a treatment option. Can. J. Neurol. Sci. 29, 227–235. doi: 10.1017/s0317167100001992
Hunt, J., Cheng, A., Hoyles, A., Jervis, E., and Morshead, C. M. (2010). Cyclosporin a has direct effects on adult neural precursor cells. J. Neurosci. 30, 2888–2896. doi: 10.1523/jneurosci.5991-09.2010
Iaci, J. F., Vecchione, A. M., Zimber, M. P., and Caggiano, A. O. (2007). Chondroitin sulfate proteoglycans in spinal cord contusion injury and the effects of chondroitinase treatment. J. Neurotrauma 24, 1743–1759. doi: 10.1089/neu.2007.0366
Insel, T. (2015). Post by Former NIMH Director Thomas Insel: Mortality and Mental Disorders [Online]. Available online at: https://www.nimh.nih.gov/about/directors/thomas-insel/blog/2015/mortality-and-mental-disorders.shtml
Iosif, R. E., Ekdahl, C. T., Ahlenius, H., Pronk, C. J. H., Bonde, S., Kokaia, Z., et al. (2006). Tumor necrosis factor receptor 1 is a negative regulator of progenitor proliferation in adult hippocampal neurogenesis. J. Neurosci. 26:9703. doi: 10.1523/jneurosci.2723-06.2006
Iqbal, K., Liu, F., Gong, C. X., and Grundke-Iqbal, I. (2010). Tau in Alzheimer disease and related tauopathies. Curr. Alzheimer Res. 7, 656–664. doi: 10.2174/156720510793611592
Ishikawa, R., Fukushima, H., Nakakita, Y., Kado, H., and Kida, S. (2019). Dietary heat-killed Lactobacillus brevis SBC8803 (SBL88TM) improves hippocampus-dependent memory performance and adult hippocampal neurogenesis. Neuropsychopharmacol. Rep. 39, 140–145. doi: 10.1002/npr2.12054
Islam, M. M., and Zhang, C.-L. (2015). TLX: a master regulator for neural stem cell maintenance and neurogenesis. Biochim. Biophys. Acta 1849, 210–216. doi: 10.1016/j.bbagrm.2014.06.001
Iula, L., Keitelman, I. A., Sabbione, F., Fuentes, F., Guzman, M., Galletti, J. G., et al. (2018). Autophagy mediates interleukin-1β secretion in human neutrophils. Front. Immunol. 9:269. doi: 10.3389/fimmu.2018.00269
James, S. L., and Alice, T. (2019). Global, regional, and national burden of traumatic brain injury and spinal cord injury, 1990-2016: a systematic analysis for the Global Burden of Disease Study 2016. Lancet Neurol. 18, 56–87.
Jhaveri, D. J., Mackay, E. W., Hamlin, A. S., Marathe, S. V., Nandam, L. S., Vaidya, V. A., et al. (2010). Norepinephrine directly activates adult hippocampal precursors via beta3-adrenergic receptors. J. Neurosci. 30, 2795–2806. doi: 10.1523/jneurosci.3780-09.2010
Ji, R.-R., and Suter, M. R. (2007). p38 MAPK, microglial signaling, and neuropathic pain. Mol. Pain 3, 33–33.
Jing, Y., Hou, Y., Song, Y., and Yin, J. (2012). Methylprednisolone improves the survival of new neurons following transient cerebral ischemia in rats. Acta Neurobiol. Exp. (Wars) 72, 240–252.
Jogia, T., and Ruitenberg, M. J. (2020). Traumatic spinal cord injury and the gut microbiota: current insights and future challenges. Front. Immunol. 11:704. doi: 10.3389/fimmu.2020.00704
Ju, Y., He, M., Mao, B., and Gao, L. (2002). [Sequential changes of hypoxia-inducible factor 1 alpha in experimental spinal cord injury and its significance]. Hua Xi Yi Ke Da Xue Xue Bao 33, 43–45.
Jure, I., De Nicola, A. F., Encinas, J. M., and Labombarda, F. (2020). Spinal cord injury leads to hippocampal glial alterations and neural stem cell inactivation. Cell. Mol. Neurobiol. (in press). doi: 10.1007/s10571-020-00900-8
Jure, I., Pietranera, L., De Nicola, A. F., and Labombarda, F. (2017). Spinal cord injury impairs neurogenesis and induces glial reactivity in the hippocampus. Neurochem. Res. 42, 2178–2190. doi: 10.1007/s11064-017-2225-9
Kanaya, A. M., Barrett-Connor, E., Gildengorin, G., and Yaffe, K. (2004). Change in cognitive function by glucose tolerance status in older adults: a 4-year prospective study of the Rancho Bernardo study cohort. Arch. Intern. Med. 164, 1327–1333. doi: 10.1001/archinte.164.12.1327
Kang, M. J., Jo, S. G., Kim, D. J., and Park, J. H. (2017). NLRP3 inflammasome mediates interleukin-1beta production in immune cells in response to Acinetobacter baumannii and contributes to pulmonary inflammation in mice. Immunology 150, 495–505. doi: 10.1111/imm.12704
Kemp, S. F., Lockey, R. F., Simons, F. E. R., and World Allergy Organization ad hoc Committee on Epinephrine in Anaphylaxis. (2008). Epinephrine: the drug of choice for anaphylaxis-a statement of the world allergy organization. World Allergy Organ. J. 1, S18–S26.
Kempermann, G., and Gage, F. H. (2002). Genetic determinants of adult hippocampal neurogenesis correlate with acquisition, but not probe trial performance, in the water maze task. Eur. J. Neurosci. 16, 129–136. doi: 10.1046/j.1460-9568.2002.02042.x
Kempermann, G., Song, H., and Gage, F. H. (2015). Neurogenesis in the adult hippocampus. Cold Spring Harb. Perspect. Biol. 7:a018812.
Khacho, M., Clark, A., Svoboda, D. S., Maclaurin, J. G., Lagace, D. C., Park, D. S., et al. (2017). Mitochondrial dysfunction underlies cognitive defects as a result of neural stem cell depletion and impaired neurogenesis. Hum. Mol. Genet. 26, 3327–3341. doi: 10.1093/hmg/ddx217
Khacho, M., Harris, R., and Slack, R. S. (2019). Mitochondria as central regulators of neural stem cell fate and cognitive function. Nat. Rev. Neurosci. 20, 34–48. doi: 10.1038/s41583-018-0091-3
Khan, N. Z., Cao, T., He, J., Ritzel, R. M., Li, Y., Henry, R. J., et al. (2021). Spinal cord injury alters microRNA and CD81+ exosome levels in plasma extracellular nanoparticles with neuroinflammatory potential. Brain Behavior Immun. 92, 165–183. doi: 10.1016/j.bbi.2020.12.007
Khazaeipour, Z., Taheri-Otaghsara, S.-M., and Naghdi, M. (2015). Depression following spinal cord injury: its relationship to demographic and socioeconomic indicators. Top. Spinal Cord Injury Rehabil. 21, 149–155. doi: 10.1310/sci2102-149
Khuu, M. A., Pagan, C. M., Nallamothu, T., Hevner, R. F., Hodge, R. D., Ramirez, J.-M., et al. (2019). Intermittent hypoxia disrupts adult neurogenesis and synaptic plasticity in the dentate gyrus. J. Neurosci. 39, 1320–1331. doi: 10.1523/jneurosci.1359-18.2018
Kigerl, K. A., Hall, J. C. E., Wang, L., Mo, X., Yu, Z., and Popovich, P. G. (2016). Gut dysbiosis impairs recovery after spinal cord injury. J. Exp. Med. 213, 2603–2620. doi: 10.1084/jem.20151345
Kigerl, K. A., Mostacada, K., and Popovich, P. G. (2018). Gut microbiota are disease-modifying factors after traumatic spinal cord injury. Neurotherapeutics 15, 60–67. doi: 10.1007/s13311-017-0583-2
Kinney, J. W., Bemiller, S. M., Murtishaw, A. S., Leisgang, A. M., Salazar, A. M., and Lamb, B. T. (2018). Inflammation as a central mechanism in Alzheimer’s disease. Alzheimers Dement. (New York, N. Y.) 4, 575–590.
Knobloch, M., Pilz, G.-A., Ghesquière, B., Kovacs, W. J., Wegleiter, T., Moore, D. L., et al. (2017). A fatty acid oxidation-dependent metabolic shift regulates adult neural stem cell activity. Cell Rep. 20, 2144–2155. doi: 10.1016/j.celrep.2017.08.029
Knoth, R., Singec, I., Ditter, M., Pantazis, G., Capetian, P., Meyer, R. P., et al. (2010). Murine features of neurogenesis in the human hippocampus across the lifespan from 0 to 100 years. PLoS One 5:e8809. doi: 10.1371/journal.pone.0008809
Kobayashi, Y., Sugahara, H., Shimada, K., Mitsuyama, E., Kuhara, T., Yasuoka, A., et al. (2017). Therapeutic potential of Bifidobacterium breve strain A1 for preventing cognitive impairment in Alzheimer’s disease. Sci. Rep. 7:13510.
Kocur, M., Schneider, R., Pulm, A.-K., Bauer, J., Kropp, S., Gliem, M., et al. (2015). IFNβ secreted by microglia mediates clearance of myelin debris in CNS autoimmunity. Acta Neuropathol. Commun. 3:20.
Kodali, M., Castro, O. W., Kim, D.-K., Thomas, A., Shuai, B., Attaluri, S., et al. (2019). Intranasally administered human MSC-derived extracellular vesicles pervasively incorporate into neurons and microglia in both intact and status epilepticus injured forebrain. Int. J. Mol. Sci. 21:181. doi: 10.3390/ijms21010181
Kohm, A. P., and Sanders, V. M. (1999). Suppression of antigen-specific Th2 cell-dependent IgM and IgG1 production following norepinephrine depletion in vivo. J. Immunol. 162, 5299–5308.
Kohman, R. A., Bhattacharya, T. K., Kilby, C., Bucko, P., and Rhodes, J. S. (2013). Effects of minocycline on spatial learning, hippocampal neurogenesis and microglia in aged and adult mice. Behav. Brain Res. 242, 17–24. doi: 10.1016/j.bbr.2012.12.032
Komada, M., Saitsu, H., Kinboshi, M., Miura, T., Shiota, K., and Ishibashi, M. (2008). Hedgehog signaling is involved in development of the neocortex. Development 135, 2717–2727. doi: 10.1242/dev.015891
Komatsu, M., Waguri, S., Chiba, T., Murata, S., Iwata, J., Tanida, I., et al. (2006). Loss of autophagy in the central nervous system causes neurodegeneration in mice. Nature 441, 880–884. doi: 10.1038/nature04723
Koo, J. W., Russo, S. J., Ferguson, D., Nestler, E. J., and Duman, R. S. (2010). Nuclear factor-κB is a critical mediator of stress-impaired neurogenesis and depressive behavior. Proc. Natl. Acad. Sci.U.S.A. 107, 2669–2674. doi: 10.1073/pnas.0910658107
Kozareva, D. A., Hueston, C. M., Ó’léime, C. S., Crotty, S., Dockery, P., Cryan, J. F., et al. (2019). Absence of the neurogenesis-dependent nuclear receptor TLX induces inflammation in the hippocampus. J. Neuroimmunol. 331, 87–96. doi: 10.1016/j.jneuroim.2017.08.008
Kozareva, D. A., O’leary, O. F., Cryan, J. F., and Nolan, Y. M. (2018). Deletion of TLX and social isolation impairs exercise-induced neurogenesis in the adolescent hippocampus. Hippocampus 28, 3–11. doi: 10.1002/hipo.22805
Krzisch, M., Temprana, S. G., Mongiat, L. A., Armida, J., Schmutz, V., Virtanen, M. A., et al. (2015). Pre-existing astrocytes form functional perisynaptic processes on neurons generated in the adult hippocampus. Brain Struct. Funct. 220, 2027–2042. doi: 10.1007/s00429-014-0768-y
Kundu, P., Lee, H. U., Garcia-Perez, I., Tay, E. X. Y., Kim, H., Faylon, L. E., et al. (2019). Neurogenesis and prolongevity signaling in young germ-free mice transplanted with the gut microbiota of old mice. Sci. Transl. Med. 11:eaau4760. doi: 10.1126/scitranslmed.aau4760
Lacroix, S., Chang, L., Rose-John, S., and Tuszynski, M. H. (2002). Delivery of hyper-interleukin-6 to the injured spinal cord increases neutrophil and macrophage infiltration and inhibits axonal growth. J. Comp. Neurol. 454, 213–228. doi: 10.1002/cne.10407
Lai, K., Kaspar, B. K., Gage, F. H., and Schaffer, D. V. (2003). Sonic hedgehog regulates adult neural progenitor proliferation in vitro and in vivo. Nat. Neurosci. 6, 21–27. doi: 10.1038/nn983
Lambelet, M., Terra, L. F., Fukaya, M., Meyerovich, K., Labriola, L., Cardozo, A. K., et al. (2018). Dysfunctional autophagy following exposure to pro-inflammatory cytokines contributes to pancreatic β-cell apoptosis. Cell Death Dis. 9:96.
Lan, X., Chen, Q., Wang, Y., Jia, B., Sun, L., Zheng, J., et al. (2012). TNF-α affects human cortical neural progenitor cell differentiation through the autocrine secretion of leukemia inhibitory factor. PLoS One 7:e50783. doi: 10.1371/journal.pone.0050783
Lavela, S. L., Weaver, F. M., Goldstein, B., Chen, K., Miskevics, S., Rajan, S., et al. (2006). Diabetes mellitus in individuals with spinal cord injury or disorder. J. Spinal Cord Med. 29, 387–395. doi: 10.1080/10790268.2006.11753887
Lee, A. S. (2005). The ER chaperone and signaling regulator GRP78/BiP as a monitor of endoplasmic reticulum stress. Methods 35, 373–381. doi: 10.1016/j.ymeth.2004.10.010
Lee, C.-H., and Giuliani, F. (2019). The role of inflammation in depression and fatigue. Front. Immunol. 10:1696.
Lenz, K. M., and Nelson, L. H. (2018). Microglia and beyond: innate immune cells as regulators of brain development and behavioral function. Front. Immunol. 9:698. doi: 10.3389/fimmu.2018.00698
Leonard, A. V., Manavis, J., Blumbergs, P. C., and Vink, R. (2014). Changes in substance P and NK1 receptor immunohistochemistry following human spinal cord injury. Spinal Cord 52, 17–23. doi: 10.1038/sc.2013.136
Lessel, W., Silver, A., Jechorek, D., Guenther, T., Roehl, F. W., Kalinski, T., et al. (2017). Inactivation of JNK2 as carcinogenic factor in colitis-associated and sporadic colorectal carcinogenesis. Carcinogenesis 38, 559–569. doi: 10.1093/carcin/bgx032
Leuner, B., Mendolia-Loffredo, S., Kozorovitskiy, Y., Samburg, D., Gould, E., and Shors, T. J. (2004). Learning enhances the survival of new neurons beyond the time when the hippocampus is required for memory. J. Neurosci. 24, 7477–7481. doi: 10.1523/jneurosci.0204-04.2004
Li, C., Jiao, G., Wu, W., Wang, H., Ren, S., Zhang, L., et al. (2019). Exosomes from bone marrow mesenchymal stem cells inhibit neuronal apoptosis and promote motor function recovery via the Wnt/β-catenin signaling pathway. Cell Transpl. 28, 1373–1383. doi: 10.1177/0963689719870999
Li, J., Polston, K. F. L., Eraslan, M., Bickel, C. S., Windham, S. T., Mclain, A. B., et al. (2018). A high-protein diet or combination exercise training to improve metabolic health in individuals with long-standing spinal cord injury: a pilot randomized study. Physiol. Rep. 6:e13813. doi: 10.14814/phy2.13813
Li, Y., He, X., Kawaguchi, R., Zhang, Y., Wang, Q., Monavarfeshani, A., et al. (2020a). Microglia-organized scar-free spinal cord repair in neonatal mice. Nature 587, 613–618. doi: 10.1038/s41586-020-2795-6
Li, Y., Ritzel, R. M., Khan, N., Cao, T., He, J., Lei, Z., et al. (2020b). Delayed microglial depletion after spinal cord injury reduces chronic inflammation and neurodegeneration in the brain and improves neurological recovery in male mice. Theranostics 10, 11376–11403. doi: 10.7150/thno.49199
Li, Y., Wu, L., Yu, M., Yang, F., Wu, B., Lu, S., et al. (2018). HIF-1alpha is critical for the activation of notch signaling in neurogenesis during acute epilepsy. Neuroscience 394, 206–219. doi: 10.1016/j.neuroscience.2018.10.037
Licht, T., Rothe, G., Kreisel, T., Wolf, B., Benny, O., Rooney, A. G., et al. (2016). VEGF preconditioning leads to stem cell remodeling and attenuates age-related decay of adult hippocampal neurogenesis. Proc. Natl. Acad. Sci.U.S.A. 113, E7828–E7836.
Lien, C. F., Mohanta, S. K., Frontczak-Baniewicz, M., Swinny, J. D., Zablocka, B., and Gorecki, D. C. (2012). Absence of glial alpha-dystrobrevin causes abnormalities of the blood-brain barrier and progressive brain edema. J. Biol. Chem. 287, 41374–41385. doi: 10.1074/jbc.m112.400044
Lim, S.-W., Shiue, Y.-L., Ho, C.-H., Yu, S.-C., Kao, P.-H., Wang, J.-J., et al. (2017). Anxiety and depression in patients with traumatic spinal cord injury: a nationwide population-based cohort study. PLoS One 12:e0169623. doi: 10.1371/journal.pone.0169623
Lima, S. M. A., and Gomes-Leal, W. (2019). Neurogenesis in the hippocampus of adult humans: controversy “fixed” at last. Neural Regener. Res. 14, 1917–1918. doi: 10.4103/1673-5374.259616
Lin, C., Mcgough, R., Aswad, B., Block, J. A., and Terek, R. (2004). Hypoxia induces HIF-1alpha and VEGF expression in chondrosarcoma cells and chondrocytes. J. Orthop. Res. 22, 1175–1181. doi: 10.1016/j.orthres.2004.03.002
Lin, C.-C., and Edelson, B. T. (2017). New insights into the role of IL-1β in experimental autoimmune encephalomyelitis and multiple sclerosis. J. Immuno. (Baltimore, Md. : 1950) 198, 4553–4560. doi: 10.4049/jimmunol.1700263
Lin, C. K., and Kazmierczak, B. I. (2017). Inflammation: a double-edged sword in the response to Pseudomonas aeruginosa infection. J. Innate Immun. 9, 250–261. doi: 10.1159/000455857
Liu, P. W., Chen, M. F., Tsai, A. P., and Lee, T. J. (2012). STAT1 mediates oroxylin a inhibition of iNOS and pro-inflammatory cytokines expression in microglial BV-2 cells. PLoS One 7:e50363. doi: 10.1371/journal.pone.0050363
Liu, P. Z., and Nusslock, R. (2018). Exercise-mediated neurogenesis in the hippocampus via BDNF. Front. Neurosci. 12:52. doi: 10.3389/fnins.2018.00052
Liu, S., Li, Y., Choi, H. M. C., Sarkar, C., Koh, E. Y., Wu, J., et al. (2018). Lysosomal damage after spinal cord injury causes accumulation of RIPK1 and RIPK3 proteins and potentiation of necroptosis. Cell Death Dis. 9, 476–476.
Liu, S., Sarkar, C., Dinizo, M., Faden, A. I., Koh, E. Y., Lipinski, M. M., et al. (2015). Disrupted autophagy after spinal cord injury is associated with ER stress and neuronal cell death. Cell Death Dis. 6:e1582. doi: 10.1038/cddis.2014.527
Liu, W., Rong, Y., Wang, J., Zhou, Z., Ge, X., Ji, C., et al. (2020). Exosome-shuttled miR-216a-5p from hypoxic preconditioned mesenchymal stem cells repair traumatic spinal cord injury by shifting microglial M1/M2 polarization. J. Neuroinflamm. 17:47.
Liu, W., Wang, Y., Gong, F., Rong, Y., Luo, Y., Tang, P., et al. (2018). Exosomes derived from bone mesenchymal stem cells repair traumatic spinal cord injury by suppressing the activation of A1 neurotoxic reactive astrocytes. J. Neurotrauma 36, 469–484. doi: 10.1089/neu.2018.5835
Loddick, S. A., Turnbull, A. V., and Rothwell, N. J. (1998). Cerebral interleukin-6 is neuroprotective during permanent focal cerebral ischemia in the rat. J. Cereb Blood Flow Metab. 18, 176–179. doi: 10.1097/00004647-199802000-00008
Long, Q., Upadhya, D., Hattiangady, B., Kim, D. K., An, S. Y., Shuai, B., et al. (2017). Intranasal MSC-derived A1-exosomes ease inflammation, and prevent abnormal neurogenesis and memory dysfunction after status epilepticus. Proc. Natl. Acad. Sci. U.S.A. 114, E3536–E3545.
Lopez-Candales, A., Hernández Burgos, P. M., Hernandez-Suarez, D. F., and Harris, D. (2017). Linking chronic inflammation with cardiovascular disease: from normal aging to the metabolic syndrome. J. Nat. Sci. 3:e341.
Loprinzi, P. D., Frith, E., Edwards, M. K., Sng, E., and Ashpole, N. (2018). The effects of exercise on memory function among young to middle-aged adults: systematic review and recommendations for future research. Am. J. Health Promot. 32, 691–704. doi: 10.1177/0890117117737409
Lorenz, C., Lesimple, P., Bukowiecki, R., Zink, A., Inak, G., Mlody, B., et al. (2017). Human iPSC-derived neural progenitors are an effective drug discovery model for neurological MtDNA disorders. Cell Stem Cell 20, 659–674.e659.
Lu, Y., Yang, Y. Y., Zhou, M. W., Liu, N., Xing, H. Y., Liu, X. X., et al. (2018). Ketogenic diet attenuates oxidative stress and inflammation after spinal cord injury by activating Nrf2 and suppressing the NF-kappaB signaling pathways. Neurosci. Lett. 683, 13–18. doi: 10.1016/j.neulet.2018.06.016
Macciocchi, S. N., Seel, R. T., and Thompson, N. (2013). The impact of mild traumatic brain injury on cognitive functioning following co-occurring spinal cord injury. Arch. Clin. Neuropsychol. 28, 684–691. doi: 10.1093/arclin/act049
Mancinelli, R., Carpino, G., Petrungaro, S., Mammola, C. L., Tomaipitinca, L., Filippini, A., et al. (2017). Multifaceted roles of GSK-3 in cancer and autophagy-related diseases. Oxid. Med. Cell. Longev. 2017:4629495.
Manning, H., Mccool, F. D., Scharf, S. M., Garshick, E., and Brown, R. (1992). Oxygen cost of resistive-loaded breathing in quadriplegia. J. Appl. Physiol. 73, 825–831. doi: 10.1152/jappl.1992.73.3.825
Martin-Gayo, E., and Yu, X. G. (2019). Role of Dendritic cells in natural immune control of HIV-1 infection. Front. Immunol. 10:1306. doi: 10.3389/fimmu.2019.01306
Masliah, E., Rockenstein, E., Mante, M., Crews, L., Spencer, B., Adame, A., et al. (2011). Passive immunization reduces behavioral and neuropathological deficits in an alpha-synuclein transgenic model of Lewy body disease. PLoS One 6:e19338. doi: 10.1371/journal.pone.0019338
Masliah, E., Rockenstein, E., Veinbergs, I., Mallory, M., Hashimoto, M., Takeda, A., et al. (2000). Dopaminergic loss and inclusion body formation in alpha-synuclein mice: implications for neurodegenerative disorders. Science 287, 1265–1269. doi: 10.1126/science.287.5456.1265
Maurer, I. C., Schippel, P., and Volz, H. P. (2009). Lithium-induced enhancement of mitochondrial oxidative phosphorylation in human brain tissue. Bipolar Disord. 11, 515–522. doi: 10.1111/j.1399-5618.2009.00729.x
McVey Neufeld, K. A., O’mahony, S. M., Hoban, A. E., Waworuntu, R. V., Berg, B. M., Dinan, T. G., et al. (2019). Neurobehavioural effects of Lactobacillus rhamnosus GG alone and in combination with prebiotics polydextrose and galactooligosaccharide in male rats exposed to early-life stress. Nutr. Neurosci. 22, 425–434. doi: 10.1080/1028415x.2017.1397875
Meng, S. X., Wang, B., and Li, W. T. (2020). Intermittent hypoxia improves cognition and reduces anxiety-related behavior in APP/PS1 mice. Brain Behav. 10:e01513.
Mishra, S., Kelly, K. K., Rumian, N. L., and Siegenthaler, J. A. (2018). Retinoic acid is required for neural stem and progenitor cell proliferation in the adult hippocampus. Stem Cell Rep. 10, 1705–1720. doi: 10.1016/j.stemcr.2018.04.024
Missiroli, S., Genovese, I., Perrone, M., Vezzani, B., Vitto, V. A. M., and Giorgi, C. (2020). The role of mitochondria in inflammation: from cancer to neurodegenerative disorders. J. Clin. Med. 9:740. doi: 10.3390/jcm9030740
Mittal, P., Gupta, R., Mittal, A., and Mittal, K. (2016). MRI findings in a case of spinal cord Wallerian degeneration following trauma. Neurosciences (Riyadh, Saudi Arabia) 21, 372–373. doi: 10.17712/nsj.2016.4.20160278
Mizgerd, J. P. (2018). Inflammation and pneumonia: why are some more susceptible than others? Clin. Chest Med. 39, 669–676.
Mohammad, H., Marchisella, F., Ortega-Martinez, S., Hollos, P., Eerola, K., Komulainen, E., et al. (2018). JNK1 controls adult hippocampal neurogenesis and imposes cell-autonomous control of anxiety behaviour from the neurogenic niche. Mol. Psychiatry 23, 362–374. doi: 10.1038/mp.2016.203
Mohri, I., Taniike, M., Taniguchi, H., Kanekiyo, T., Aritake, K., Inui, T., et al. (2006). Prostaglandin D2-mediated microglia/astrocyte interaction enhances astrogliosis and demyelination in twitcher. J. Neurosci. 26, 4383–4393. doi: 10.1523/jneurosci.4531-05.2006
Montgomerie, J. Z. (1997). Infections in patients with spinal cord injuries. Clin Infect Dis 1285-1290:quiz1291–2.
Morales-Garcia, J. A., Luna-Medina, R., Alonso-Gil, S., Sanz-Sancristobal, M., Palomo, V., Gil, C., et al. (2012). Glycogen synthase kinase 3 inhibition promotes adult hippocampal neurogenesis in vitro and in vivo. ACS Chem. Neurosci. 3, 963–971. doi: 10.1021/cn300110c
Morcuende, S., Gadd, C. A., Peters, M., Moss, A., Harris, E. A., Sheasby, A., et al. (2003). Increased neurogenesis and brain-derived neurotrophic factor in neurokinin-1 receptor gene knockout mice. Eur. J. Neurosci. 18, 1828–1836. doi: 10.1046/j.1460-9568.2003.02911.x
Moreno-Jimenez, E. P., Flor-Garcia, M., Terreros-Roncal, J., Rabano, A., Cafini, F., Pallas-Bazarra, N., et al. (2019). Adult hippocampal neurogenesis is abundant in neurologically healthy subjects and drops sharply in patients with Alzheimer’s disease. Nat. Med. 25, 554–560. doi: 10.1038/s41591-019-0375-9
Moreno-Jiménez, E. P., Terreros-Roncal, J., Flor-García, M., Rábano, A., and Llorens-Martín, M. (2021). Evidences for adult hippocampal neurogenesis in humans. J. Neurosci. 41, 2541–2553. doi: 10.1523/jneurosci.0675-20.2020
Mouasni, S., Gonzalez, V., Schmitt, A., Bennana, E., Guillonneau, F., Mistou, S., et al. (2019). The classical NLRP3 inflammasome controls FADD unconventional secretion through microvesicle shedding. Cell Death Dis. 10:190.
Munoz, L. E., Lauber, K., Schiller, M., Manfredi, A. A., and Herrmann, M. (2010). The role of defective clearance of apoptotic cells in systemic autoimmunity. Nat. Rev. Rheumatol. 6, 280–289. doi: 10.1038/nrrheum.2010.46
Muñoz-Galdeano, T., Reigada, D., Del Águila, Á, Velez, I., Caballero-López, M. J., Maza, R. M., et al. (2018). Cell specific changes of autophagy in a mouse model of contusive spinal cord injury. Front. Cell. Neurosci. 12:164. doi: 10.3389/fncel.2018.00164
Murman, D. L. (2015). The impact of age on cognition. Semin. Hear. 36, 111–121. doi: 10.1055/s-0035-1555115
Nah, J., Yuan, J., and Jung, Y.-K. (2015). Autophagy in neurodegenerative diseases: from mechanism to therapeutic approach. Mol. Cells 38, 381–389. doi: 10.14348/molcells.2015.0034
Nakano, M., Kubota, K., Kobayashi, E., Chikenji, T. S., Saito, Y., Konari, N., et al. (2020). Bone marrow-derived mesenchymal stem cells improve cognitive impairment in an Alzheimer’s disease model by increasing the expression of microRNA-146a in hippocampus. Sci. Rep. 10:10772.
Nakano, M., Nagaishi, K., Konari, N., Saito, Y., Chikenji, T., Mizue, Y., et al. (2016). Bone marrow-derived mesenchymal stem cells improve diabetes-induced cognitive impairment by exosome transfer into damaged neurons and astrocytes. Sci. Rep. 6:24805.
National Spinal Cord Injury Statistical Center (2014). Spinal Cord Injury Model Systems 2014 Annual Report [Online]. Available online at: https://www.nscisc.uab.edu/PublicDocuments/reports/pdf/2014%20NSCISC%20Annual%20Statistical%20Report%20Complete%20Public%20Version.pdf
National Spinal Cord Injury Statistical Center (2019). Annual Statistical Report 2019 [Online]. nscisc.uab.edu: National Spinal Cord Injury Statistical Center. Available online at: https://www.nscisc.uab.edu/public/2019%20Annual%20Report%20-%20Complete%20Public%20Version.pdf
Neelamekam, S., Nurjono, M., and Lee, J. (2014). Regulation of interleukin-6 and leptin in schizophrenia patients: a preliminary analysis. Clin. Psychopharmacol. Neurosci. 12, 209–214. doi: 10.9758/cpn.2014.12.3.209
Nelson, M. D., Widman, L. M., Abresch, R. T., Stanhope, K., Havel, P. J., Styne, D. M., et al. (2007). Metabolic syndrome in adolescents with spinal cord dysfunction. J. Spinal Cord Med. 30(Suppl. 1), S127–S139.
Neumann, H., Schweigreiter, R., Yamashita, T., Rosenkranz, K., Wekerle, H., and Barde, Y.-A. (2002). Tumor necrosis factor inhibits neurite outgrowth and branching of hippocampal neurons by a rho-dependent mechanism. J. Neurosci. 22, 854–862. doi: 10.1523/jneurosci.22-03-00854.2002
Nixon, R. A. (2013). The role of autophagy in neurodegenerative disease. Nat. Med. 19, 983–997. doi: 10.1038/nm.3232
O’Connor, G., Jeffrey, E., Madorma, D., Marcillo, A., Abreu, M. T., Deo, S. K., et al. (2018). Investigation of microbiota alterations and intestinal inflammation post-spinal cord injury in rat model. J. Neurotrauma 35, 2159–2166. doi: 10.1089/neu.2017.5349
O’Connor, T. M., O’connell, J., O’brien, D. I., Goode, T., Bredin, C. P., and Shanahan, F. (2004). The role of substance P in inflammatory disease. J. Cell Physiol. 201, 167–180. doi: 10.1002/jcp.20061
Ogata, M., Hino, S.-I., Saito, A., Morikawa, K., Kondo, S., Kanemoto, S., et al. (2006). Autophagy is activated for cell survival after endoplasmic reticulum stress. Mol. Cell. Biol. 26, 9220–9231.
Okada, S. (2016). The pathophysiological role of acute inflammation after spinal cord injury. Inflam. Regener. 36, 20–20.
Okada, S., Hara, M., Kobayakawa, K., Matsumoto, Y., and Nakashima, Y. (2018). Astrocyte reactivity and astrogliosis after spinal cord injury. Neurosci. Res. 126, 39–43. doi: 10.1016/j.neures.2017.10.004
O’Sullivan, E., Barrett, E., Grenham, S., Fitzgerald, P., Stanton, C., Ross, R. P., et al. (2011). BDNF expression in the hippocampus of maternally separated rats: does Bifidobacterium breve 6330 alter BDNF levels? Benef Microbes 2, 199–207. doi: 10.3920/bm2011.0015
O’Sullivan, J. B., Ryan, K. M., Curtin, N. M., Harkin, A., and Connor, T. J. (2009). Noradrenaline reuptake inhibitors limit neuroinflammation in rat cortex following a systemic inflammatory challenge: implications for depression and neurodegeneration. Int. J. Neuropsychopharmacol. 12, 687–699. doi: 10.1017/s146114570800967x
Packard, M. G., and Teather, L. A. (1997). Posttraining estradiol injections enhance memory in ovariectomized rats: cholinergic blockade and synergism. Neurobiol. Learn. Mem. 68, 172–188. doi: 10.1006/nlme.1997.3785
Pan, Y., Chen, X. Y., Zhang, Q. Y., and Kong, L. D. (2014). Microglial NLRP3 inflammasome activation mediates IL-1beta-related inflammation in prefrontal cortex of depressive rats. Brain Behav. Immun. 41, 90–100. doi: 10.1016/j.bbi.2014.04.007
Park, K. W., Lee, H. G., Jin, B. K., and Lee, Y. B. (2007). Interleukin-10 endogenously expressed in microglia prevents lipopolysaccharide-induced neurodegeneration in the rat cerebral cortex in vivo. Exp. Mol. Med. 39, 812–819. doi: 10.1038/emm.2007.88
Park, S. A., Jeong, S., Choe, Y. H., and Hyun, Y.-M. (2018). Sensing of vascular permeability in inflamed vessel of live animal. J. Anal. Methods Chem. 2018, 5797152.
Park, S. J., Lee, J. Y., Kim, S. J., Choi, S.-Y., Yune, T. Y., and Ryu, J. H. (2015). Toll-like receptor-2 deficiency induces schizophrenia-like behaviors in mice. Sci. Rep. 5:8502.
Park, S. W., Yan, Y. P., Satriotomo, I., Vemuganti, R., and Dempsey, R. J. (2007). Substance P is a promoter of adult neural progenitor cell proliferation under normal and ischemic conditions. J. Neurosurg. 107, 593–599. doi: 10.3171/jns-07/09/0593
Pawley, L. C., Hueston, C. M., O’leary, J. D., Kozareva, D. A., Cryan, J. F., O’leary, O. F., et al. (2020). Chronic intrahippocampal interleukin-1β overexpression in adolescence impairs hippocampal neurogenesis but not neurogenesis-associated cognition. Brain Behavior Immun. 83, 172–179. doi: 10.1016/j.bbi.2019.10.007
Pedersen, C. B., Mors, O., Bertelsen, A., Waltoft, B. L., Agerbo, E., Mcgrath, J. J., et al. (2014). A comprehensive nationwide study of the incidence rate and lifetime risk for treated mental disorders. JAMA Psychiatry 71, 573–581. doi: 10.1001/jamapsychiatry.2014.16
Pehote, G., and Vij, N. (2020). Autophagy augmentation to alleviate immune response dysfunction, and resolve respiratory and COVID-19 exacerbations. Cells 9:1952. doi: 10.3390/cells9091952
Peng, B., Kong, G., Yang, C., and Ming, Y. (2020). Erythropoietin and its derivatives: from tissue protection to immune regulation. Cell Death Dis. 11:79.
Peng, L., and Bonaguidi, M. A. (2018). Function and dysfunction of adult hippocampal neurogenesis in regeneration and disease. Am. J. Pathol. 188, 23–28. doi: 10.1016/j.ajpath.2017.09.004
Perez-Asensio, F. J., Perpiñá, U., Planas, A. M., and Pozas, E. (2013). Interleukin-10 regulates progenitor differentiation and modulates neurogenesis in adult brain. J. Cell Sci. 126, 4208–4219.
Petersen, A. M., and Pedersen, B. K. (2005). The anti-inflammatory effect of exercise. J. Appl. Physiol. 98, 1154–1162.
Phillips, A. A., and Krassioukov, A. V. (2015). Contemporary cardiovascular concerns after spinal cord injury: mechanisms, maladaptations, and management. J. Neurotrauma 32, 1927–1942. doi: 10.1089/neu.2015.3903
Pinçon, A., De Montgolfier, O., Akkoyunlu, N., Daneault, C., Pouliot, P., Villeneuve, L., et al. (2019). Non-Alcoholic fatty liver disease, and the underlying altered fatty acid metabolism, reveals brain hypoperfusion and contributes to the cognitive decline in APP/PS1 mice. Metabolites 9:104. doi: 10.3390/metabo9050104
Pineau, I., and Lacroix, S. (2007). Proinflammatory cytokine synthesis in the injured mouse spinal cord: multiphasic expression pattern and identification of the cell types involved. J. Comp. Neurol. 500, 267–285. doi: 10.1002/cne.21149
Popovich, P. G., Stuckman, S., Gienapp, I. E., and Whitacre, C. C. (2001). Alterations in immune cell phenotype and function after experimental spinal cord injury. J. Neurotrauma 18, 957–966. doi: 10.1089/089771501750451866
Prüss, H., Tedeschi, A., Thiriot, A., Lynch, L., Loughhead, S. M., Stutte, S., et al. (2017). Spinal cord injury-induced immunodeficiency is mediated by a sympathetic-neuroendocrine adrenal reflex. Nat. Neurosci. 20, 1549–1559. doi: 10.1038/nn.4643
Püntener, U., Booth, S. G., Perry, V. H., and Teeling, J. L. (2012). Long-term impact of systemic bacterial infection on the cerebral vasculature and microglia. J. Neuroinflamm. 9:146.
Qu, Q., Sun, G., Murai, K., Ye, P., Li, W., Asuelime, G., et al. (2013). Wnt7a regulates multiple steps of neurogenesis. Mol. Cell. Biol. 33, 2551–2559. doi: 10.1128/mcb.00325-13
Quintanilla, R. A., Orellana, D. I., Gonzalez-Billault, C., and Maccioni, R. B. (2004). Interleukin-6 induces Alzheimer-type phosphorylation of tau protein by deregulating the cdk5/p35 pathway. Exp. Cell Res. 295, 245–257. doi: 10.1016/j.yexcr.2004.01.002
Raghubir, R., Nakka, V. P., and Mehta, S. L. (2011). Endoplasmic reticulum stress in brain damage. Methods Enzymol. 489, 259–275. doi: 10.1016/b978-0-12-385116-1.00015-7
Ragnarsson, K. T. (2008). Functional electrical stimulation after spinal cord injury: current use, therapeutic effects and future directions. Spinal Cord 46, 255–274. doi: 10.1038/sj.sc.3102091
Raison, C. L., and Miller, A. H. (2011). Is depression an inflammatory disorder? Curr. Psychiatry Rep. 13, 467–475. doi: 10.1007/s11920-011-0232-0
Ramachandran Pillai, R., Wilson, A. B., Premkumar, N. R., Kattimani, S., Sagili, H., and Rajendiran, S. (2018). Low serum levels of high-density lipoprotein cholesterol (HDL-c) as an indicator for the development of severe postpartum depressive symptoms. PLoS One 13:e0192811. doi: 10.1371/journal.pone.0192811
Ranuh, R., Athiyyah, A. F., Darma, A., Risky, V. P., Riawan, W., Surono, I. S., et al. (2019). Effect of the probiotic Lactobacillus plantarum IS-10506 on BDNF and 5HT stimulation: role of intestinal microbiota on the gut-brain axis. Iran. J. Microbiol. 11, 145–150.
Rappert, A., Biber, K., Nolte, C., Lipp, M., Schubel, A., Lu, B., et al. (2002). Secondary lymphoid tissue chemokine (CCL21) activates CXCR3 to trigger a Cl- current and chemotaxis in murine microglia. J. Immunol. 168, 3221–3226. doi: 10.4049/jimmunol.168.7.3221
Ray, S., Corenblum, M. J., Anandhan, A., Reed, A., Ortiz, F. O., Zhang, D. D., et al. (2018). A role for Nrf2 expression in defining the aging of hippocampal neural stem cells. Cell Transpl. 27, 589–606. doi: 10.1177/0963689718774030
Rea, I. M., Gibson, D. S., Mcgilligan, V., Mcnerlan, S. E., Alexander, H. D., and Ross, O. A. (2018). Age and age-related diseases: role of inflammation triggers and cytokines. Front. Immunol. 9:586. doi: 10.3389/fimmu.2018.00586
Reif, A., Fritzen, S., Finger, M., Strobel, A., Lauer, M., Schmitt, A., et al. (2006). Neural stem cell proliferation is decreased in schizophrenia, but not in depression. Mol. Psychiatry 11, 514–522. doi: 10.1038/sj.mp.4001791
Remington, P. L., and Brownson, R. C. (2011). Fifty years of progress in chronic disease epidemiology and control. MMWR Suppl. 60, 70–77.
Resnick, S. M., Pham, D. L., Kraut, M. A., Zonderman, A. B., and Davatzikos, C. (2003). Longitudinal magnetic resonance imaging studies of older adults: a shrinking brain. J. Neurosci. 23, 3295–3301. doi: 10.1523/jneurosci.23-08-03295.2003
Revest, J. M., Dupret, D., Koehl, M., Funk-Reiter, C., Grosjean, N., Piazza, P. V., et al. (2009). Adult hippocampal neurogenesis is involved in anxiety-related behaviors. Mol. Psychiatry 14, 959–967. doi: 10.1038/mp.2009.15
Reza-Zaldivar, E. E., Hernández-Sapiéns, M. A., Gutiérrez-Mercado, Y. K., Sandoval-Ávila, S., Gomez-Pinedo, U., Márquez-Aguirre, A. L., et al. (2019). Mesenchymal stem cell-derived exosomes promote neurogenesis and cognitive function recovery in a mouse model of Alzheimer’s disease. Neural Regener. Res. 14, 1626–1634. doi: 10.4103/1673-5374.255978
Rhodes, J. S., Van Praag, H., Jeffrey, S., Girard, I., Mitchell, G. S., and Garland, T. Jr., et al. (2003). Exercise increases hippocampal neurogenesis to high levels but does not improve spatial learning in mice bred for increased voluntary wheel running. Behav. Neurosci. 117, 1006–1016. doi: 10.1037/0735-7044.117.5.1006
Riga, D., Kramvis, I., Koskinen, M. K., Van Bokhoven, P., Van Der Harst, J. E., Heistek, T. S., et al. (2017). Hippocampal extracellular matrix alterations contribute to cognitive impairment associated with a chronic depressive-like state in rats. Sci. Transl. Med. 9:eaai8753. doi: 10.1126/scitranslmed.aai8753
Robledinos-Antón, N., Rojo, A. I., Ferreiro, E., Núñez, Á, Krause, K.-H., Jaquet, V., et al. (2017). Transcription factor NRF2 controls the fate of neural stem cells in the subgranular zone of the hippocampus. Redox Biol. 13, 393–401. doi: 10.1016/j.redox.2017.06.010
Rock, R. B., Gekker, G., Hu, S., Sheng, W. S., Cheeran, M., Lokensgard, J. R., et al. (2004). Role of microglia in central nervous system infections. Clin. Microbiol. Rev. 17, 942–964. doi: 10.1128/cmr.17.4.942-964.2004
Rolls, A., Shechter, R., London, A., Ziv, Y., Ronen, A., Levy, R., et al. (2007). Toll-like receptors modulate adult hippocampal neurogenesis. Nat. Cell Biol. 9, 1081–1088. doi: 10.1038/ncb1629
Ross, A. P., Bruggeman, E. C., Kasumu, A. W., Mielke, J. G., and Parent, M. B. (2012). Non-alcoholic fatty liver disease impairs hippocampal-dependent memory in male rats. Physiol. Behav. 106, 133–141. doi: 10.1016/j.physbeh.2012.01.008
Rua, R., and McGavern, D. B. (2018). Advances in meningeal immunity. Trends Mol. Med. 24, 542–559. doi: 10.1016/j.molmed.2018.04.003
Rudge, J. S., and Silver, J. (1990). Inhibition of neurite outgrowth on astroglial scars in vitro. J. Neurosci. 10, 3594–3603. doi: 10.1523/jneurosci.10-11-03594.1990
Rudzki, L., Ostrowska, L., Pawlak, D., Malus, A., Pawlak, K., Waszkiewicz, N., et al. (2019). Probiotic Lactobacillus plantarum 299v decreases kynurenine concentration and improves cognitive functions in patients with major depression: a double-blind, randomized, placebo controlled study. Psychoneuroendocrinology 100, 213–222. doi: 10.1016/j.psyneuen.2018.10.010
Rutsch, A., Kantsjö, J. B., and Ronchi, F. (2020). The gut-brain axis: how microbiota and host inflammasome influence brain physiology and pathology. Front. Immunol. 11:604179. doi: 10.3389/fimmu.2020.604179
Ryan, S. M., O’keeffe, G. W., O’connor, C., Keeshan, K., and Nolan, Y. M. (2013). Negative regulation of TLX by IL-1β correlates with an inhibition of adult hippocampal neural precursor cell proliferation. Brain Behavior Immun. 33, 7–13. doi: 10.1016/j.bbi.2013.03.005
Ryu, J. R., Hong, C. J., Kim, J. Y., Kim, E.-K., Sun, W., and Yu, S.-W. (2016). Control of adult neurogenesis by programmed cell death in the mammalian brain. Mol. Brain 9:43.
Sahay, A., Scobie, K. N., Hill, A. S., O’carroll, C. M., Kheirbek, M. A., Burghardt, N. S., et al. (2011). Increasing adult hippocampal neurogenesis is sufficient to improve pattern separation. Nature 472, 466–470. doi: 10.1038/nature09817
Saliba, M., Saadeh, D., Bouchand, F., Davido, B., Duran, C., Clair, B., et al. (2017). Outcome of bloodstream infections among spinal cord injury patients and impact of multidrug-resistant organisms. Spinal Cord 55, 148–154. doi: 10.1038/sc.2016.176
Saraswat Ohri, S., Bankston, A. N., Mullins, S. A., Liu, Y., Andres, K. R., Beare, J. E., et al. (2018). Blocking autophagy in oligodendrocytes limits functional recovery after spinal cord injury. J. Neurosci. 38, 5900–5912. doi: 10.1523/jneurosci.0679-17.2018
Sarlus, H., Höglund, C. O., Karshikoff, B., Wang, X., Lekander, M., Schultzberg, M., et al. (2012). Allergy influences the inflammatory status of the brain and enhances tau-phosphorylation. J. Cell. Mol. Med. 16, 2401–2412. doi: 10.1111/j.1582-4934.2012.01556.x
Sato, A., Ohtaki, H., Tsumuraya, T., Song, D., Ohara, K., Asano, M., et al. (2012). Interleukin-1 participates in the classical and alternative activation of microglia/macrophages after spinal cord injury. J. Neuroinflamm. 9, 65–65.
Sauerbeck, A. D., Laws, J. L., Bandaru, V. V., Popovich, P. G., Haughey, N. J., and Mctigue, D. M. (2015). Spinal cord injury causes chronic liver pathology in rats. J. Neurotrauma 32, 159–169. doi: 10.1089/neu.2014.3497
Savic, G., Devivo, M. J., Frankel, H. L., Jamous, M. A., Soni, B. M., and Charlifue, S. (2018). Suicide and traumatic spinal cord injury—a cohort study. Spinal Cord 56, 2–6. doi: 10.1038/sc.2017.98
Saxe, M. D., Battaglia, F., Wang, J.-W., Malleret, G., David, D. J., Monckton, J. E., et al. (2006). Ablation of hippocampal neurogenesis impairs contextual fear conditioning and synaptic plasticity in the dentate gyrus. Proc. Natl. Acad. Sci. U.S.A. 103, 17501–17506. doi: 10.1073/pnas.0607207103
Schäffner, I., Minakaki, G., Khan, M. A., Balta, E.-A., Schlötzer-Schrehardt, U., Schwarz, T. J., et al. (2018). FoxO function is essential for maintenance of autophagic flux and neuronal morphogenesis in adult neurogenesis. Neuron 99, 1188–1203.e1186.
Schank, J. R., and Heilig, M. (2017). Substance P and the neurokinin-1 receptor: the new CRF. Int. Rev. Neurobiol. 136, 151–175. doi: 10.1016/bs.irn.2017.06.008
Schanzer, A., Wachs, F. P., Wilhelm, D., Acker, T., Cooper-Kuhn, C., Beck, H., et al. (2004). Direct stimulation of adult neural stem cells in vitro and neurogenesis in vivo by vascular endothelial growth factor. Brain Pathol. 14, 237–248. doi: 10.1111/j.1750-3639.2004.tb00060.x
Scheinert, R. B., Asokan, A., Rani, A., Kumar, A., Foster, T. C., and Ormerod, B. K. (2015). Some hormone, cytokine and chemokine levels that change across lifespan vary by cognitive status in male Fischer 344 rats. Brain Behav. Immun. 49, 216–232. doi: 10.1016/j.bbi.2015.06.005
Schmid, A., Huonker, M., Stahl, F., Barturen, J. M., Konig, D., Heim, M., et al. (1998). Free plasma catecholamines in spinal cord injured persons with different injury levels at rest and during exercise. J. Auton. Nerv. Syst. 68, 96–100. doi: 10.1016/s0165-1838(97)00127-6
Schmidt, E. K. A., Torres-Espin, A., Raposo, P. J. F., Madsen, K. L., Kigerl, K. A., Popovich, P. G., et al. (2020). Fecal transplant prevents gut dysbiosis and anxiety-like behaviour after spinal cord injury in rats. PLoS One 15:e0226128. doi: 10.1371/journal.pone.0226128
Schmitt, A., Bertsch, T., Tost, H., Bergmann, A., Henning, U., Klimke, A., et al. (2005). Increased serum interleukin-1beta and interleukin-6 in elderly, chronic schizophrenic patients on stable antipsychotic medication. Neuropsychiatric Dis. Treat. 1, 171–177. doi: 10.2147/nedt.1.2.171.61048
Schnell, L., Fearn, S., Klassen, H., Schwab, M. E., and Perry, V. H. (1999). Acute inflammatory responses to mechanical lesions in the CNS: differences between brain and spinal cord. Eur. J Neurosci. 11, 3648–3658. doi: 10.1046/j.1460-9568.1999.00792.x
Scholpa, N. E., and Schnellmann, R. G. (2017). Mitochondrial-based therapeutics for the treatment of spinal cord injury: mitochondrial biogenesis as a potential pharmacological target. J. Pharmacol. Exp. Ther. 363, 303–313. doi: 10.1124/jpet.117.244806
Schrage, K., Koopmans, G., Joosten, E. A., and Mey, J. (2006). Macrophages and neurons are targets of retinoic acid signaling after spinal cord contusion injury. Eur. J. Neurosci. 23, 285–295. doi: 10.1111/j.1460-9568.2005.04534.x
Schroder, N. W., Morath, S., Alexander, C., Hamann, L., Hartung, T., Zahringer, U., et al. (2003). Lipoteichoic acid (LTA) of Streptococcus pneumoniae and Staphylococcus aureus activates immune cells via Toll-like receptor (TLR)-2, lipopolysaccharide-binding protein (LBP), and CD14, whereas TLR-4 and MD-2 are not involved. J. Biol. Chem 278, 15587–15594. doi: 10.1074/jbc.m212829200
Schwab, J. M., Brechtel, K., Nguyen, T. D., and Schluesener, H. J. (2000a). Persistent accumulation of cyclooxygenase-1 (COX-1) expressing microglia/macrophages and upregulation by endothelium following spinal cord injury. J. Neuroimmunol. 111, 122–130. doi: 10.1016/s0165-5728(00)00372-6
Schwab, J. M., Nguyen, T. D., Postler, E., Meyermann, R., and Schluesener, H. J. (2000b). Selective accumulation of cyclooxygenase-1-expressing microglial cells/macrophages in lesions of human focal cerebral ischemia. Acta Neuropathol. 99, 609–614. doi: 10.1007/s004010051170
Schwab, J. M., Zhang, Y., Kopp, M. A., Brommer, B., and Popovich, P. G. (2014). The paradox of chronic neuroinflammation, systemic immune suppression, autoimmunity after traumatic chronic spinal cord injury. Exp. Neurol. 258, 121–129. doi: 10.1016/j.expneurol.2014.04.023
Scott, G. A., Terstege, D. J., Vu, A. P., Law, S., Evans, A., and Epp, J. R. (2020). Disrupted neurogenesis in germ-free mice: effects of age and sex. Front. Cell Dev. Biol. 8:407. doi: 10.3389/fcell.2020.00407
Selmaj, K. W., Farooq, M., Norton, W. T., Raine, C. S., and Brosnan, C. F. (1990). Proliferation of astrocytes in vitro in response to cytokines. A primary role for tumor necrosis factor. J. Immunol. 144, 129–135.
Shaftel, S. S., Griffin, W. S. T., and O’banion, M. K. (2008). The role of interleukin-1 in neuroinflammation and Alzheimer disease: an evolving perspective. J. Neuroinflamm. 5:7. doi: 10.1186/1742-2094-5-7
Sharif, H., and Hou, S. (2017). Autonomic dysreflexia: a cardiovascular disorder following spinal cord injury. Neural Regener. Res. 12, 1390–1400. doi: 10.4103/1673-5374.215241
Shepherd Center (2020). Understanding Spinal Cord Injury [Online]. Available online at: http://www.spinalinjury101.org/details/levels-of-injury
Shi, X., Zhao, M., Fu, C., and Fu, A. (2017). Intravenous administration of mitochondria for treating experimental Parkinson’s disease. Mitochondrion 34, 91–100. doi: 10.1016/j.mito.2017.02.005
Shin, J., Berg, D. A., Zhu, Y., Shin, J. Y., Song, J., Bonaguidi, M. A., et al. (2015). Single-Cell RNA-Seq with waterfall reveals molecular cascades underlying adult neurogenesis. Cell Stem Cell 17, 360–372. doi: 10.1016/j.stem.2015.07.013
Sierra, A., Encinas, J. M., Deudero, J. J. P., Chancey, J. H., Enikolopov, G., Overstreet-Wadiche, L. S., et al. (2010). Microglia shape adult hippocampal neurogenesis through apoptosis-coupled phagocytosis. Cell Stem Cell 7, 483–495. doi: 10.1016/j.stem.2010.08.014
Singh, A., Tetreault, L., Kalsi-Ryan, S., Nouri, A., and Fehlings, M. G. (2014). Global prevalence and incidence of traumatic spinal cord injury. Clin. Epidemiol. 6, 309–331. doi: 10.2147/clep.s68889
Singh-Manoux, A., Gimeno, D., Kivimaki, M., Brunner, E., and Marmot, M. G. (2008). Low HDL cholesterol is a risk factor for deficit and decline in memory in midlife: the Whitehall II study. Arterioscler. Thromb. Vasc. Biol. 28, 1556–1562. doi: 10.1161/atvbaha.108.163998
Sloka, J. S., and Stefanelli, M. (2005). The mechanism of action of methylprednisolone in the treatment of multiple sclerosis. Mult. Scler. J. 11, 425–432. doi: 10.1191/1352458505ms1190oa
Sokol, H., Pigneur, B., Watterlot, L., Lakhdari, O., Bermúdez-Humarán, L. G., Gratadoux, J.-J., et al. (2008). Faecalibacterium prausnitzii is an anti-inflammatory commensal bacterium identified by gut microbiota analysis of Crohn disease patients. Proc. Natl. Acad. Sci. U.S.A. 105, 16731–16736. doi: 10.1073/pnas.0804812105
Son, G., and Han, J. (2018). Roles of mitochondria in neuronal development. BMB Rep. 51, 549–556. doi: 10.5483/bmbrep.2018.51.11.226
Song, J., Christian, K. M., Ming, G.-L., and Song, H. (2012). Modification of hippocampal circuitry by adult neurogenesis. Dev. Neurobiol. 72, 1032–1043. doi: 10.1002/dneu.22014
Sorrells, S. F., Paredes, M. F., Cebrian-Silla, A., Sandoval, K., Qi, D., Kelley, K. W., et al. (2018). Human hippocampal neurogenesis drops sharply in children to undetectable levels in adults. Nature 555, 377–381. doi: 10.1038/nature25975
Sorrells, S. F., Paredes, M. F., Zhang, Z., Kang, G., Pastor-Alonso, O., Biagiotti, S., et al. (2021). Positive controls in adults and children support that very few, if any, new neurons are born in the adult human hippocampus. J. Neurosci. 41, 2554–2565. doi: 10.1523/jneurosci.0676-20.2020
SpinalCord.Com (2020). Spinal Cord Injury Types [Online]. Available online at: https://www.spinalcord.com/types-of-spinal-cord-injuries
Spinelli, M., Fusco, S., and Grassi, C. (2019). Brain insulin resistance and hippocampal plasticity: mechanisms and biomarkers of cognitive decline. Front. Neurosci. 13:788. doi: 10.3389/fnins.2019.00788
Spritzer, M. D., and Galea, L. A. (2007). Testosterone and dihydrotestosterone, but not estradiol, enhance survival of new hippocampal neurons in adult male rats. Dev. Neurobiol. 67, 1321–1333. doi: 10.1002/dneu.20457
Sroga, J. M., Jones, T. B., Kigerl, K. A., Mcgaughy, V. M., and Popovich, P. G. (2003). Rats and mice exhibit distinct inflammatory reactions after spinal cord injury. J. Comp. Neurol. 462, 223–240. doi: 10.1002/cne.10736
Stanton, R., and Reaburn, P. (2014). Exercise and the treatment of depression: a review of the exercise program variables. J. Sci. Med. Sport 17, 177–182. doi: 10.1016/j.jsams.2013.03.010
Steen, R. G., Mull, C., Mcclure, R., Hamer, R. M., and Lieberman, J. A. (2006). Brain volume in first-episode schizophrenia: systematic review and meta-analysis of magnetic resonance imaging studies. Br. J. Psychiatry 188, 510–518. doi: 10.1192/bjp.188.6.510
Stefani, J., Tschesnokowa, O., Parrilla, M., Robaye, B., Boeynaems, J.-M., Acker-Palmer, A., et al. (2018). Disruption of the microglial ADP receptor P2Y(13) enhances adult hippocampal neurogenesis. Front. Cell. Neurosci. 12:134.
Stephenson, E. L., and Yong, V. W. (2018). Pro-inflammatory roles of chondroitin sulfate proteoglycans in disorders of the central nervous system. Matrix Biol. 71-72, 432–442. doi: 10.1016/j.matbio.2018.04.010
Stevens, B., Allen, N. J., Vazquez, L. E., Howell, G. R., Christopherson, K. S., Nouri, N., et al. (2007). The classical complement cascade mediates CNS synapse elimination. Cell 131, 1164–1178. doi: 10.1016/j.cell.2007.10.036
Storer, M. A., Gallagher, D., Fatt, M. P., Simonetta, J. V., Kaplan, D. R., and Miller, F. D. (2018). Interleukin-6 regulates adult neural stem cell numbers during normal and abnormal post-natal development. Stem Cell Rep. 10, 1464–1480. doi: 10.1016/j.stemcr.2018.03.008
Stranahan, A. M., Arumugam, T. V., Cutler, R. G., Lee, K., Egan, J. M., and Mattson, M. P. (2008). Diabetes impairs hippocampal function through glucocorticoid-mediated effects on new and mature neurons. Nat. Neurosci. 11, 309–317. doi: 10.1038/nn2055
Streijger, F., Plunet, W. T., Lee, J. H. T., Liu, J., Lam, C. K., Park, S., et al. (2013). Ketogenic diet improves forelimb motor function after spinal cord injury in rodents. PLoS One 8:e78765. doi: 10.1371/journal.pone.0078765
Streit, W. J. (2005). Microglia and neuroprotection: implications for Alzheimer’s disease. Brain Res. Brain Res. Rev. 48, 234–239. doi: 10.1016/j.brainresrev.2004.12.013
Sullivan, P. G., Krishnamurthy, S., Patel, S. P., Pandya, J. D., and Rabchevsky, A. G. (2007). Temporal characterization of mitochondrial bioenergetics after spinal cord injury. J. Neurotrauma 24, 991–999. doi: 10.1089/neu.2006.0242
Sun, B., Halabisky, B., Zhou, Y., Palop, J. J., Yu, G., Mucke, L., et al. (2009). Imbalance between GABAergic and glutamatergic transmission impairs adult neurogenesis in an animal model of Alzheimer’s Disease. Cell Stem Cell 5, 624–633. doi: 10.1016/j.stem.2009.10.003
Sun, N., Youle, R. J., and Finkel, T. (2016). The mitochondrial basis of aging. Mol. Cell 61, 654–666. doi: 10.1016/j.molcel.2016.01.028
Sun, X., Jones, Z. B., Chen, X.-M., Zhou, L., So, K.-F., and Ren, Y. (2016). Multiple organ dysfunction and systemic inflammation after spinal cord injury: a complex relationship. J. Neuroinflamm. 13:260.
Sun, Y., Karmakar, M., Roy, S., Ramadan, R. T., Williams, S. R., Howell, S., et al. (2010). TLR4 and TLR5 on corneal macrophages regulate Pseudomonas aeruginosa keratitis by signaling through MyD88-dependent and -independent pathways. J. Immunol. 185, 4272–4283. doi: 10.4049/jimmunol.1000874
Sutherland, T. C., Mathews, K. J., Mao, Y., Nguyen, T., and Gorrie, C. A. (2017). Differences in the cellular response to acute spinal cord injury between developing and mature rats highlights the potential significance of the inflammatory response. Front. Cell. Neurosci. 10:310. doi: 10.3389/fncel.2016.00310
Szabo, K., Förster, A., Jäger, T., Kern, R., Griebe, M., Hennerici, M. G., et al. (2009). Hippocampal lesion patterns in acute posterior cerebral artery stroke: clinical and MRI findings. Stroke 40, 2042–2045. doi: 10.1161/strokeaha.108.536144
Takahashi, K., Nakagawasai, O., Nemoto, W., Odaira, T., Sakuma, W., Onogi, H., et al. (2019). Effect of Enterococcus faecalis 2001 on colitis and depressive-like behavior in dextran sulfate sodium-treated mice: involvement of the brain-gut axis. J. Neuroinflamm. 16, 201–201.
Tanaka, T., Ueno, M., and Yamashita, T. (2009). Engulfment of axon debris by microglia requires p38 MAPK activity. J. Biol. Chem. 284, 21626–21636. doi: 10.1074/jbc.m109.005603
Tanapat, P., Hastings, N. B., Reeves, A. J., and Gould, E. (1999). Estrogen stimulates a transient increase in the number of new neurons in the dentate gyrus of the adult female rat. J. Neurosci. 19, 5792–5801. doi: 10.1523/jneurosci.19-14-05792.1999
Tang, P., Hou, H., Zhang, L., Lan, X., Mao, Z., Liu, D., et al. (2014). Autophagy reduces neuronal damage and promotes locomotor recovery via inhibition of apoptosis after spinal cord injury in rats. Mol. Neurobiol. 49, 276–287. doi: 10.1007/s12035-013-8518-3
Tang, W., Meng, Z., Li, N., Liu, Y., Li, L., Chen, D., et al. (2021). Roles of gut microbiota in the regulation of hippocampal plasticity, inflammation, and hippocampus-dependent behaviors. Front. Cell. Infect. Microbiol. 10:611014. doi: 10.3389/fcimb.2020.611014
Tashiro, A., Makino, H., and Gage, F. H. (2007). Experience-specific functional modification of the dentate gyrus through adult neurogenesis: a critical period during an immature stage. J. Neurosci. 27, 3252–3259. doi: 10.1523/jneurosci.4941-06.2007
Terry, R. D., and Katzman, R. (2001). Life span and synapses: will there be a primary senile dementia? Neurobiol. Aging 22, 347–348. doi: 10.1016/s0197-4580(00)00250-5
Tofte, N., Nielsen, A. C., Trostrup, H., Andersen, C. B., Von Linstow, M., Hansen, B., et al. (2017). Chronic urinary tract infections in patients with spinal cord lesions - biofilm infection with need for long-term antibiotic treatment. Apmis 125, 385–391. doi: 10.1111/apm.12685
Toni, N., and Schinder, A. F. (2015). Maturation and functional integration of new granule cells into the adult hippocampus. Cold Spring Harb. Perspect. Biol. 8:a018903. doi: 10.1101/cshperspect.a018903
Tregub, P. P., Malinovskaya, N. A., Morgun, A. V., Osipova, E. D., Kulikov, V. P., Kuzovkov, D. A., et al. (2020). Hypercapnia potentiates HIF-1α activation in the brain of rats exposed to intermittent hypoxia. Respir. Physiol. Neurobiol. 278:103442. doi: 10.1016/j.resp.2020.103442
Trinchero, M. F., Herrero, M., and Schinder, A. F. (2019). Rejuvenating the brain with chronic exercise through adult neurogenesis. Front. Neurosci. 13:1000. doi: 10.3389/fnins.2019.01000
Trompet, S., De Craen, A. J., Slagboom, P., Shepherd, J., Blauw, G. J., Murphy, M. B., et al. (2008). Genetic variation in the interleukin-1 beta-converting enzyme associates with cognitive function. The PROSPER study. Brain 131, 1069–1077. doi: 10.1093/brain/awn023
Uderhardt, S., Herrmann, M., Oskolkova, O. V., Aschermann, S., Bicker, W., Ipseiz, N., et al. (2012). 12/15-lipoxygenase orchestrates the clearance of apoptotic cells and maintains immunologic tolerance. Immunity 36, 834–846. doi: 10.1016/j.immuni.2012.03.010
Vallières, L., Campbell, I. L., Gage, F. H., and Sawchenko, P. E. (2002). Reduced hippocampal neurogenesis in adult transgenic mice with chronic astrocytic production of interleukin-6. J. Neurosci. 22, 486–492. doi: 10.1523/jneurosci.22-02-00486.2002
van Den Hauwe, L., Sundgren, P. C., and Flanders, A. E. (2020). “Spinal trauma and spinal cord injury (SCI),” in Diseases of the Brain, Head and Neck, Spine 2020–2023: Diagnostic Imaging, eds J. Hodler, R. A. Kubik-Huch, and G. K. Von Schulthess (Cham: Springer International Publishing), 231–240.
van Gelder, B. M., Tijhuis, M., Kalmijn, S., and Kromhout, D. (2007). Fish consumption, n-3 fatty acids, and subsequent 5-y cognitive decline in elderly men: the Zutphen Elderly Study. Am. J. Clin. Nutr. 85, 1142–1147. doi: 10.1093/ajcn/85.4.1142
van Groen, T., Miettinen, P., and Kadish, I. (2003). The entorhinal cortex of the mouse: organization of the projection to the hippocampal formation. Hippocampus 13, 133–149. doi: 10.1002/hipo.10037
van Praag, H., Christie, B. R., Sejnowski, T. J., and Gage, F. H. (1999). Running enhances neurogenesis, learning, and long-term potentiation in mice. Proc. Natl. Acad. Sci. U.S.A. 96, 13427–13431. doi: 10.1073/pnas.96.23.13427
van Strien, N. M., Cappaert, N. L. M., and Witter, M. P. (2009). The anatomy of memory: an interactive overview of the parahippocampal–hippocampal network. Nat. Rev. Neurosci. 10, 272–282. doi: 10.1038/nrn2614
Vetreno, R. P., Lawrimore, C. J., Rowsey, P. J., and Crews, F. T. (2018). Persistent adult neuroimmune activation and loss of hippocampal neurogenesis following adolescent ethanol exposure: blockade by exercise and the anti-inflammatory drug indomethacin. Front. Neurosci. 12:200. doi: 10.3389/fnins.2018.00200
Vichiansiri, R., Saengsuwan, J., Manimmanakorn, N., Patpiya, S., Preeda, A., Samerduen, K., et al. (2012). The prevalence of dyslipidemia in patients with spinal cord lesion in Thailand. Cholesterol 2012:847462.
Vij, N., Chandramani-Shivalingappa, P., Van Westphal, C., Hole, R., and Bodas, M. (2018). Cigarette smoke-induced autophagy impairment accelerates lung aging, COPD-emphysema exacerbations and pathogenesis. Am. J. Physiol. Cell Physiol. 314, C73–C87.
Voloboueva, L. A., and Giffard, R. G. (2011). Inflammation, mitochondria, and the inhibition of adult neurogenesis. J. Neurosci. Res. 89, 1989–1996. doi: 10.1002/jnr.22768
Vyas, S., Varshney, D., Sharma, P., Juyal, R., Nautiyal, V., and Shrotriya, V. (2015). An overview of the predictors of symptomatic urinary tract infection among nursing students. Ann. Med. Health Sci. Res. 5, 54–58. doi: 10.4103/2141-9248.149790
Wainwright, S. R., Lieblich, S. E., and Galea, L. A. M. (2011). Hypogonadism predisposes males to the development of behavioural and neuroplastic depressive phenotypes. Psychoneuroendocrinology 36, 1327–1341. doi: 10.1016/j.psyneuen.2011.03.004
Walton, N. M., Shin, R., Tajinda, K., Heusner, C. L., Kogan, J. H., Miyake, S., et al. (2012). Adult neurogenesis transiently generates oxidative stress. PLoS One 7:e35264. doi: 10.1371/journal.pone.0035264
Wan, D., and Krassioukov, A. V. (2014). Life-threatening outcomes associated with autonomic dysreflexia: a clinical review. J. Spinal Cord Med. 37, 2–10. doi: 10.1179/2045772313y.0000000098
Wang, C., Liang, C.-C., Bian, Z. C., Zhu, Y., and Guan, J.-L. (2013). FIP200 is required for maintenance and differentiation of postnatal neural stem cells. Nat. Neurosci. 16, 532–542. doi: 10.1038/nn.3365
Wang, K., Chen, Y., Zhang, P., Lin, P., Xie, N., and Wu, M. (2019). Protective features of autophagy in pulmonary infection and inflammatory diseases. Cells 8:123. doi: 10.3390/cells8020123
Wang, Q., Liu, Y., and Zhou, J. (2015). Neuroinflammation in Parkinson’s disease and its potential as therapeutic target. Transl. Neurodegener. 4:19.
Wang, T. D., Wang, Y. H., Huang, T. S., Su, T. C., Pan, S. L., and Chen, S. Y. (2007). Circulating levels of markers of inflammation and endothelial activation are increased in men with chronic spinal cord injury. J. Formos Med. Assoc. 106, 919–928. doi: 10.1016/s0929-6646(08)60062-5
Ward, M., Bendlin, B., Mclaren, D., Hess, T., Callagher, C., Kastman, E., et al. (2010). Low HDL cholesterol is associated with lower gray matter volume in cognitively healthy adults. Front. Aging Neurosci. 2:29. doi: 10.3389/fnagi.2010.00029
Watanabe, T., Yamamoto, T., Abe, Y., Saito, N., Kumagai, T., and Kayama, H. (1999). Differential activation of microglia after experimental spinal cord injury. J. Neurotrauma 16, 255–265. doi: 10.1089/neu.1999.16.255
Watson, G. S., and Leverenz, J. B. (2010). Profile of cognitive impairment in Parkinson’s disease. Brain Pathol. (Zurich, Switzerland) 20, 640–645.
Weikel, K. A., Cacicedo, J. M., Ruderman, N. B., and Ido, Y. (2016). Knockdown of GSK3β increases basal autophagy and AMPK signalling in nutrient-laden human aortic endothelial cells. Biosci. Rep. 36:e00382.
Wells, J. E., Hurlbert, R. J., Fehlings, M. G., and Yong, V. W. (2003). Neuroprotection by minocycline facilitates significant recovery from spinal cord injury in mice. Brain 126, 1628–1637. doi: 10.1093/brain/awg178
Winner, B., Regensburger, M., Schreglmann, S., Boyer, L., Prots, I., Rockenstein, E., et al. (2012). Role of α-synuclein in adult neurogenesis and neuronal maturation in the dentate gyrus. J. Neurosci. 32, 16906–16916. doi: 10.1523/jneurosci.2723-12.2012
Winner, B., and Winkler, J. (2015). Adult neurogenesis in neurodegenerative diseases. Cold Spring Harb. Perspect. Biol. 7:a021287.
Witter, M. P., Doan, T. P., Jacobsen, B., Nilssen, E. S., and Ohara, S. (2017). Architecture of the entorhinal cortex a review of entorhinal anatomy in rodents with some comparative notes. Front. Syst. Neurosci. 11:46. doi: 10.3389/fnsys.2017.00046
Woitke, F., Ceanga, M., Rudolph, M., Niv, F., Witte, O. W., Redecker, C., et al. (2017). Adult hippocampal neurogenesis poststroke: more new granule cells but aberrant morphology and impaired spatial memory. PLoS One 12:e0183463. doi: 10.1371/journal.pone.0183463
World Health Organization (2020). Global Health Observatory (GHO) data – Life expectancy [Online]. Available online at: https://www.who.int/gho/mortality_burden_disease/life_tables/situation_trends_text/en/
Woting, A., Clavel, T., Loh, G., and Blaut, M. (2010). Bacterial transformation of dietary lignans in gnotobiotic rats. FEMS Microbiol. Ecol. 72, 507–514. doi: 10.1111/j.1574-6941.2010.00863.x
Wu, J., Zhao, Z., Kumar, A., Lipinski, M. M., Loane, D. J., Stoica, B. A., et al. (2016). Endoplasmic reticulum stress and disrupted neurogenesis in the brain are associated with cognitive impairment and depressive-like behavior after spinal cord injury. J. Neurotrauma 33, 1919–1935. doi: 10.1089/neu.2015.4348
Wu, J., Zhao, Z., Sabirzhanov, B., Stoica, B. A., Kumar, A., Luo, T., et al. (2014). Spinal cord injury causes brain inflammation associated with cognitive and affective changes: role of cell cycle pathways. J. Neurosci. 34, 10989–11006. doi: 10.1523/jneurosci.5110-13.2014
Wu, M. D., Hein, A. M., Moravan, M. J., Shaftel, S. S., Olschowka, J. A., and O’banion, M. K. (2012). Adult murine hippocampal neurogenesis is inhibited by sustained IL-1β and not rescued by voluntary running. Brain Behav. Immun. 26, 292–300. doi: 10.1016/j.bbi.2011.09.012
Wu, M. D., Montgomery, S. L., Rivera-Escalera, F., Olschowka, J. A., and O’banion, M. K. (2013). Sustained IL-1β expression impairs adult hippocampal neurogenesis independent of IL-1 signaling in nestin+ neural precursor cells. Brain Behav. Immun. 32, 9–18. doi: 10.1016/j.bbi.2013.03.003
Xia, S., Zhang, X., Zheng, S., Khanabdali, R., Kalionis, B., Wu, J., et al. (2016). An update on inflamm-aging: mechanisms, prevention, and treatment. J. Immunol. Res. 2016:8426874.
Xiaowei, H., Ninghui, Z., Wei, X., Yiping, T., and Linfeng, X. (2006). The experimental study of hypoxia-inducible factor-1alpha and its target genes in spinal cord injury. Spinal Cord 44, 35–43. doi: 10.1038/sj.sc.3101813
Yan, P., Xu, J., Li, Q., Chen, S., Kim, G. M., Hsu, C. Y., et al. (1999). Glucocorticoid receptor expression in the spinal cord after traumatic injury in adult rats. J. Neurosci. 19, 9355–9363. doi: 10.1523/jneurosci.19-21-09355.1999
Yang, L., Jones, N. R., Blumbergs, P. C., Van Den Heuvel, C., Moore, E. J., Manavis, J., et al. (2005). Severity-dependent expression of pro-inflammatory cytokines in traumatic spinal cord injury in the rat. J. Clin. Neurosci. 12, 276–284. doi: 10.1016/j.jocn.2004.06.011
Yang, Y., Ye, Y., Su, X., He, J., Bai, W., and He, X. (2017). MSCs-derived exosomes and neuroinflammation, neurogenesis and therapy of traumatic brain injury. Front. Cell. Neurosci. 11:55. doi: 10.3389/fncel.2017.00055
Yang, Z., Shao, Y., Zhao, Y., Li, Q., Li, R., Xiao, H., et al. (2020). Endoplasmic reticulum stress-related neuroinflammation and neural stem cells decrease in mice exposure to paraquat. Sci. Rep. 10:17757.
Yao, L., Kan, E. M., Lu, J., Hao, A., Dheen, S. T., Kaur, C., et al. (2013). Toll-like receptor 4 mediates microglial activation and production of inflammatory mediators in neonatal rat brain following hypoxia: role of TLR4 in hypoxic microglia. J. Neuroinflamm. 10:785.
Yazdani, S. O. (2019). Allogenic Mesenchymal Stem Cell Derived Exosome in Patients With Acute Ischemic Stroke. Isfahan: Isfahan University of Medical Sciences. (ClinicalTrials.gov).
Ye, Y., Yang, Y., Chen, C., Li, Z., Jia, Y., Su, X., et al. (2017). Electroacupuncture improved hippocampal neurogenesis following traumatic brain injury in mice through inhibition of TLR4 signaling pathway. Stem Cells Int. 2017, 5841814.
Yeh, T.-S., Ho, Y.-C., Hsu, C.-L., and Pan, S.-L. (2018). Spinal cord injury and Alzheimer’s disease risk: a population-based, retrospective cohort study. Spinal Cord 56, 151–157.
Yeh, T. S., Huang, Y. P., Wang, H. I., and Pan, S. L. (2016). Spinal cord injury and Parkinson’s disease: a population-based, propensity score-matched, longitudinal follow-up study. Spinal Cord 54, 1215–1219.
Yermakova, A. V., Rollins, J., Callahan, L. M., Rogers, J., and O’banion, M. K. (1999). Cyclooxygenase-1 in human Alzheimer and control brain: quantitative analysis of expression by microglia and CA3 hippocampal neurons. J. Neuropathol. Exp. Neurol. 58, 1135–1146. doi: 10.1097/00005072-199911000-00003
Yick, L. W., So, K. F., Cheung, P. T., and Wu, W. T. (2004). Lithium chloride reinforces the regeneration-promoting effect of chondroitinase ABC on rubrospinal neurons after spinal cord injury. J. Neurotrauma 21, 932–943. doi: 10.1089/0897715041526221
Zhang, D., Hu, X., Qian, L., O’callaghan, J. P., and Hong, J.-S. (2010). Astrogliosis in CNS pathologies: is there a role for microglia? Mol. Neurobiol. 41, 232–241. doi: 10.1007/s12035-010-8098-4
Zhang, H., Fang, X., Huang, D., Luo, Q., Zheng, M., Wang, K., et al. (2018). Erythropoietin signaling increases neurogenesis and oligodendrogenesis of endogenous neural stem cells following spinal cord injury both in vivo and in vitro. Mol. Med. Rep. 17, 264–272.
Zhang, J., Xie, X., Tang, M., Zhang, J., Zhang, B., Zhao, Q., et al. (2017). Salvianolic acid B promotes microglial M2-polarization and rescues neurogenesis in stress-exposed mice. Brain Behav. Immun. 66, 111–124. doi: 10.1016/j.bbi.2017.07.012
Zhang, L., Yang, X., Yang, S., and Zhang, J. (2011). The Wnt?/β-catenin signaling pathway in the adult neurogenesis. Eur. J. Neurosci. 33, 1–8. doi: 10.1111/j.1460-9568.2010.7483.x
Zhang, Q., Huang, C., Meng, B., Tang, T. S., and Yang, H. L. (2014). Changes in autophagy proteins in a rat model of spinal cord injury. Chin. J. Traumatol. 17, 193–197.
Zhang, Z., Ma, Z., Yan, C., Pu, K., Wu, M., Bai, J., et al. (2019). Muscle-derived autologous mitochondrial transplantation: a novel strategy for treating cerebral ischemic injury. Behav. Brain Res. 356, 322–331.
Zhao, C., Sun, G., Li, S., and Shi, Y. (2009). A feedback regulatory loop involving microRNA-9 and nuclear receptor TLX in neural stem cell fate determination. Nat. Struct. Mol. Biol. 16, 365–371. doi: 10.1038/nsmb.1576
Zhou, Y., Li, N., Zhu, L., Lin, Y., and Cheng, H. (2018). The microglial activation profile and associated factors after experimental spinal cord injury in rats. Neuropsychiatr. Dis. Treat. 14, 2401–2413. doi: 10.2147/ndt.s169940
Zhu, L.-J., Ni, H.-Y., Chen, R., Chang, L., Shi, H.-J., Qiu, D., et al. (2018). Hippocampal nuclear factor kappa B accounts for stress-induced anxiety behaviors via enhancing neuronal nitric oxide synthase (nNOS)-carboxy-terminal PDZ ligand of nNOS-Dexras1 coupling. J. Neurochem. 146, 598–612. doi: 10.1111/jnc.14478
Zhu, L. L., Zhao, T., Li, H. S., Zhao, H., Wu, L. Y., Ding, A. S., et al. (2005). Neurogenesis in the adult rat brain after intermittent hypoxia. Brain Res. 1055, 1–6. doi: 10.1016/j.brainres.2005.04.075
Zhu, X.-H., Yan, H.-C., Zhang, J., Qu, H.-D., Qiu, X.-S., Chen, L., et al. (2010). Intermittent hypoxia promotes hippocampal neurogenesis and produces antidepressant-like effects in adult rats. J. Neurosci. 30, 12653–12663. doi: 10.1523/jneurosci.6414-09.2010
Zonis, S., Pechnick, R. N., Ljubimov, V. A., Mahgerefteh, M., Wawrowsky, K., Michelsen, K. S., et al. (2015). Chronic intestinal inflammation alters hippocampal neurogenesis. J. Neuroinflamm. 12:65.
Zuo, Y., Wang, J., Liao, F., Yan, X., Li, J., Huang, L., et al. (2018). Inhibition of heat shock protein 90 by 17-AAG reduces inflammation via P2X7 Receptor/NLRP3 inflammasome pathway and increases neurogenesis after subarachnoid hemorrhage in mice. Front. Mol. Neurosci. 11:401. doi: 10.3389/fnmol.2018.00401
Keywords: neurogenesis, spinal cord injury, inflammation, memory and cognitive impairment, therapeutics
Citation: Sefiani A and Geoffroy CG (2021) The Potential Role of Inflammation in Modulating Endogenous Hippocampal Neurogenesis After Spinal Cord Injury. Front. Neurosci. 15:682259. doi: 10.3389/fnins.2021.682259
Received: 18 March 2021; Accepted: 17 May 2021;
Published: 18 June 2021.
Edited by:
Angélica Zepeda, National Autonomous University of Mexico, MexicoReviewed by:
Junfang Wu, University of Maryland School of Medicine, United StatesFlorencia Labombarda, CONICET Instituto de Biología y Medicina Experimental (IBYME), Argentina
Jorge Valero, University of Salamanca, Spain
Enric Llorens, Karolinska Institutet (KI), Sweden
Hedong Li, Augusta University, United States
Copyright © 2021 Sefiani and Geoffroy. This is an open-access article distributed under the terms of the Creative Commons Attribution License (CC BY). The use, distribution or reproduction in other forums is permitted, provided the original author(s) and the copyright owner(s) are credited and that the original publication in this journal is cited, in accordance with accepted academic practice. No use, distribution or reproduction is permitted which does not comply with these terms.
*Correspondence: Cédric G. Geoffroy, Z2VvZmZyb3lAdGFtdS5lZHU=
†These authors have contributed equally to this work