- 1Middleton High School, Middleton, WI, United States
- 2Department of Pathology and Laboratory Medicine, Perelman School of Medicine, University of Pennsylvania, Philadelphia, PA, United States
- 3Center for Neurodegenerative Disease Research, Perelman School of Medicine at the University of Pennsylvania, Philadelphia, PA, United States
- 4Department of Neuroscience, Genentech, Inc., South San Francisco, CA, United States
Several studies investigating the pathogenesis of Alzheimer’s disease have identified various interdependent constituents contributing to the exacerbation of the disease, including Aβ plaque formation, tau protein hyperphosphorylation, neurofibrillary tangle accumulation, glial inflammation, and the eventual loss of proper neural plasticity. Recently, using various models and human patients, another key factor has been established as an influential determinant in brain homeostasis: the gut–brain axis. The implications of a rapidly aging population and the absence of a definitive cure for Alzheimer’s disease have prompted a search for non-pharmaceutical tools, of which gut-modulatory therapies targeting the gut–brain axis have shown promise. Yet multiple recent studies examining changes in human gut flora in response to various probiotics and environmental factors are limited and difficult to generalize; whether the state of the gut microbiota in Alzheimer’s disease is a cause of the disease, a result of the disease, or both through numerous feedback loops in the gut–brain axis, remains unclear. However, preliminary findings of longitudinal studies conducted over the past decades have highlighted dietary interventions, especially Mediterranean diets, as preventative measures for Alzheimer’s disease by reversing neuroinflammation, modifying the intestinal and blood–brain barrier (BBB), and addressing gut dysbiosis. Conversely, the consumption of Western diets intensifies the progression of Alzheimer’s disease through genetic alterations, impaired barrier function, and chronic inflammation. This review aims to support the growing body of experimental and clinical data highlighting specific probiotic strains and particular dietary components in preventing Alzheimer’s disease via the gut–brain axis.
Introduction
Lifestyle changes, particularly one’s diet, have emerged as a topic of significant interest in epidemiological research due to the capacity to modulate molecular mechanisms in the gastrointestinal tract, which has been associated with gastrointestinal and extra-gastrointestinal diseases (Vijay and Valdes, 2022). Recently, numerous studies have established the bidirectional communication between the gut microbiota and the central nervous system (CNS), described as the microbiota–gut–brain axis (GBA), and its potential effects in the context of neurological diseases, a reasonable connection to be made considering probiotics’ ability to restore gut homeostasis and serve as a host ameliorative factor (Yan and Polk, 2020; Socała et al., 2021). The rising interest in potential mediators between the gut microbiota and the pathogenesis of chronic neurodegenerative diseases, especially Alzheimer’s disease (AD), is associated with the public health and socioeconomic problem represented by neurodegenerative diseases, a concern exacerbated by a lack of concrete knowledge on the subject (Bredesen, 2016; Amato et al., 2019).
Dementia has been broadly defined as a loss of cognitive and behavioral functioning combined with an acquired memory impairment to an extent where a person’s ability to address daily living activities is diminished (Eschweiler et al., 2010; Zmily and Abu-Saymeh, 2013; Jithesh et al., 2020). The rapidly aging population and the consequent increase in the prevalence of dementia cases (Dua et al., 2017) are expected to present both immediate and long-term socioeconomic implications in the next few decades if no treatments are developed within that timeframe (Alzheimer’s Dementia, 2020).
At the center of focus is AD, a progressive disease beginning with mild memory loss to severe cognitive decline, accounting for 60–80% of all dementia cases (Garre-Olmo, 2018). AD is characterized by a complex neuropsychological profile consisting of interactions between different conditions (Ngandu et al., 2015), including age and the impairment of physiological barriers (Hebert et al., 2013), the inheritance of the APOE-4 gene (Seshadri et al., 1995; Farrer et al., 1997; Green et al., 2002), amyloid β (Aβ) peptide deposition (Fagan et al., 2006; Tapiola et al., 2009; Lee et al., 2019), intracellular neurofibrillary tangles (NFTs) (Franzmeier et al., 2020; Mattsson-Carlgren et al., 2020; Johansson et al., 2021), and external risk factors or conditions (Reitz et al., 2011; Beydoun et al., 2014). The public costs and consequences of the Alzheimer’s epidemic have been underestimated (Eschweiler et al., 2010), and major social trends will have direct and adverse effects on our ability to deal with this inevitable crisis in the years ahead.
Despite advances in our understanding of the pathological factors of AD at the cellular level, there have been no novel disease-modifying clinical therapies that have successfully addressed these identified mechanisms or that have effectively reversed the progression of AD. This includes Biogen’s anti-Aβ monoclonal antibody aducanumab, which was recently approved by the US Food and Drug Administration, aiming to remove amyloid protein depositions from the brains of patients at the expense of potential adverse effects (Ferrero et al., 2016; Yiannopoulou and Papageorgiou, 2020; Crosson et al., 2021; Hershey and Tarawneh, 2021). The evidence for any clinical benefit from aducanumab and previous drugs directed at amyloid deposits has been highly speculative based on both analyses of conflicting clinical trial data (Nicoll et al., 2019; Crosson et al., 2021; Knopman et al., 2021; McCleery and Quinn, 2021; Richard et al., 2021) and the compromised validity of the evidence presented due to the decision to approve the drug based on a surrogate endpoint, specifically Aβ reductions, whose status as a likely predictor of clinical benefit is also highly tentative (McCleery and Quinn, 2021). As most AD cases are late-onset and appear to have relatively long asymptomatic prodromal phases, novel therapies targeting the predicted inducers of AD may have a limited effect, especially when treatment is initiated in a patient who is already exhibiting symptoms (Golde, 2009). Thus, due to aducanumab’s inability to establish efficacy (Howard and Liu, 2020) and the persisting linear, amyloid-centric approach to AD research (Mullane and Williams, 2013), we must focus on new approaches that effectively target the disease trigger.
It is crucial to concentrate research efforts on identifying the precise stages of the complex pathological cascade that result in neuronal death in AD and, ideally, determining the relative sequence and timing of those events within that cascade (Golde, 2009). Subsequently, we will need to determine which of these steps are potentially targetable, develop novel therapies that interfere with these steps while considering the extent of disease progression (Lee et al., 2019), and continuously demonstrate that these therapies are effective in slowing or reversing AD (Golde, 2009; Sahoo et al., 2018; Salloway et al., 2020).
Despite the extensive research dedicated to deciphering AD pathogenesis and discovering novel drug treatments (Lee et al., 2019), the physiological complications established by a dynamic, selective barrier known as the blood–brain barrier (BBB) make it difficult to assess the therapeutic effectiveness of these methods due to restrictions on drug transportation (Fong, 2015; Pardridge, 2020), inadequate AD drug delivery models (Wan et al., 2009; de Lange and Hammarlund-Udenaes, 2015), and uncertainty of intracellular entry levels of the agent when measured through cerebrospinal fluid (CSF) (de Lange and Hammarlund-Udenaes, 2015). Furthermore, it has become clear that the involvement of pathological proteins in AD is incredibly intricate (Spires-Jones et al., 2017), and the complexity of clinical trial design has further complicated the consideration of combination therapies (Salloway et al., 2020). As a result, the gut microbiome has attracted attention as an alternative targetable area due to its ability to be manipulated through various nutritional therapies and indirectly interact with the BBB (Suganya and Koo, 2020). Several microbial-derived neurochemicals involved in the gut–microbiota–brain crosstalk appear to be implicated in the basis of neurodegeneration (Parker et al., 2020). This review aims to assist in the growing recognition of pathways in the GBA and their potential usefulness in addressing AD among medical communities. The exploration of this bidirectional communication, as well as the overall modulation process of one’s diet, will reveal new scenarios in chronic neurodegeneration research.
Alzheimer’s disease neuroimmunology
AD is a neurodegenerative brain disorder characterized by a progressive decline in cognitive functions and reduced autonomy through the deterioration of neuronal cells and their networks (Kodis et al., 2018; Theofilas et al., 2018; Angelucci et al., 2019). While the pathophysiological conditions of AD are still relatively unclear, the disease has been attributed to extracellular accumulations of abnormally folded amyloid β (Aβ) proteins that polymerize and aggregate into plaques (Koffie et al., 2009; Wei et al., 2010; Hong et al., 2016; Jack et al., 2018; Jutten et al., 2019; Tiwari et al., 2019) through the alteration of APP (Herms et al., 2004; Ashley et al., 2005), as well as the hyperphosphorylation of the microtubule-associated protein tau into paired helical filaments and neuropil threads (Weingarten et al., 1975; Ballatore et al., 2007; Alavi Naini and Soussi-Yanicostas, 2015; Murray et al., 2015). This polymerization of proteins results in cytoskeleton disorganization in the axonal process (Himmelstein et al., 2012; Kolarova et al., 2012) and is thought to activate microglial cells, which further contributes to neurotoxicity (Rajmohan and Reddy, 2017; Ismail et al., 2020). In addition to genetic predispositions (Chakrabarti et al., 2015; van der Lee et al., 2018; Lee et al., 2020), environmental factors likely help trigger the onset of AD cases (Killin et al., 2016; Armstrong, 2019).
The two-peak hypothesis
Despite the wide variation in clinicopathologic study designs and disagreement regarding amyloid pathology metrics, several ubiquitous findings identify a rather weak correlation between the density of amyloid plaques and the severity of cognitive decline compared to NFTs, especially in late prodromal phases (Markesbery et al., 2006; Jack et al., 2009; Nelson et al., 2009; Ismail et al., 2020). These observations indicate that simply suppressing Aβ peptide aggregation does not serve as a protective measure against AD, and alternate physiological aberrations caused by crosstalk between external systems (namely the GBA) may play an influential role.
Cell-to-cell transmission of tau
Understanding tau dysfunctions and aggregation mechanisms are critical for developing new therapeutic strategies, especially considering the spread of tau toxicity through cell–to–cell transmission properties reported between anatomically connected brain regions (Uemura et al., 2020). To date, the exact mechanisms involved in the transmission of tau remain obscure, but it appears that misfolded forms of tau can serve as a template for the misfolding of normally structured tau (Jucker and Walker, 2013; Vijay and Morris, 2014) and spread trans-synaptically through afferent connections in correspondence with intrinsic connectivity (rather than proximity) (Ahmed et al., 2014), as well as in a manner reminiscent of prion spreading to induce the spread of filamentous tau (Hoenig et al., 2018; Schwarz et al., 2018). Macropinocytosis has been proposed as the most likely mechanism of tau uptake (Kerr and Teasdale, 2009; Holmes et al., 2013), delivering pathological tau in an exosome-dependent manner to unaffected cells and becoming internalized through the facilitation of microglia (Baker et al., 2016; Wang et al., 2017a), astrocytes, and oligodendrocytes (Martini-Stoica et al., 2018; Perea et al., 2019).
Similar to tau transmission throughout the CNS, tau could be delivered between different organs through neuronal connections (Zaccai et al., 2008). Considering the similar putative mechanisms of tau and α-synuclein (αSyn) transmission (Xu et al., 2014), whose pathology may be triggered by inflammatory agents in the enteric nervous system and spreads to the brain via the vagus nerve (VN) (Holmqvist et al., 2014; Sampson et al., 2016), tau pathology may be induced outside of the CNS. Notably, several tau isoforms were found to readily and bidirectionally cross the BBB in mice (Hidalgo et al., 2018), and tau was found in exosomes isolated from CSF (Saman et al., 2012) and the blood of patients with AD (Fiandaca et al., 2015). Thus, the cell–to–cell transmission of abnormal proteins that occur within the GBA deserves special attention in order to identify new targetable areas for AD therapies.
Neuroinflammation
Research indicates that chronic inflammation through the build-up of ineffective glial cells and mediators from potentially long-distance paths of transmission (Jiang and Bhaskar, 2020) are implicated in AD pathogenesis. There are four types of glial cells in the CNS, two of which are focused on in this review as neuroinflammatory mediators between the gut and the brain: (1) microglia, which are phagocytic scavenger cells similar to macrophages in the nervous system (Keren-Shaul et al., 2017), and (2) astrocytes, which are starlike cells that comprise the most abundant proportion of glial cells in the brain (Jo et al., 2014).
The role of microglia in Alzheimer’s disease
Microglia, the first line of cellular defense, serve to dynamically survey the environment, sculpt neuronal circuits, and coordinate the communication between the innate and adaptive immune systems (Keren-Shaul et al., 2017). However, microglia-mediated responses are commonly described as “double-edged” because of their harmful side effects (Trotta et al., 2018), including the increase in localized cytokine concentrations (Keren-Shaul et al., 2017; Kwon and Koh, 2020), downregulation of Aβ and APP phagocytosis receptors (Ghosh et al., 2013; Brosseron et al., 2014; Kwon and Koh, 2020), and the tendency to sustain toxic recruitment around plaques. Furthermore, in conditions of gut dysbiosis, pathogenic compounds activate soluble myeloid cells 2 (TREM2), which are highly expressed in microglia (Lill et al., 2015; Reigstad et al., 2015; Piccio et al., 2016; Kwon and Koh, 2020), to impair bidirectional communication between the brain and the blood, the VN, or the glymphatic system (Natale et al., 2019). Thus, further studies are warranted to investigate the clinical benefits of targeting the microglial state at specific periods along the inflammatory cascade between the gut and brain.
The role of astrocytes in Alzheimer’s disease
In a healthy CNS, astrocytes are fundamental in maintaining homeostasis by clearing debris, protecting against oxidative stress, and presenting antigens for T cells (Constantinescu et al., 2005; Perea et al., 2009; González-Reyes et al., 2017). Astrocytes also play a key function in preserving the integrity of the BBB, a highly selective semipermeable border that regulates the movement of molecules, ions, and cells between the blood and the CNS (Daneman and Prat, 2015). Astrogliosis (Zamanian et al., 2012; Batarseh et al., 2016; González-Reyes et al., 2017; Liddelow et al., 2017; Fang et al., 2018; Guttenplan et al., 2020) stimulates the release of vasoactive endothelial growth factors, which increases BBB permeability and encourages the extravasation of leukocytes (Bush et al., 1999; Forman et al., 2004; Haseloff et al., 2005; Argaw et al., 2012; Cabezas et al., 2014; Sofroniew, 2015). NF-κB and complement signaling are also activated (Liao et al., 2004; Kawahara et al., 2009; Lian et al., 2015; Turillazzi et al., 2016; Jones and Kounatidis, 2017), impeding communication between neurons and glial cells through calcium and glutamate dysregulation (Rothstein et al., 1996; Berliocchi et al., 2005; Dabir et al., 2006; Friedman, 2006; Shigetomi et al., 2008; Ndountse and Chan, 2009; Sompol et al., 2017; Mahmoud et al., 2019), and producing inflammatory mediators in response to scavenger receptors ligands (Murgas et al., 2012) and LPS (Forloni et al., 1997), highlighting the gut microbiota’s influence on the immune system.
Perspectives on glial cell recruitment
Extensive review has established the positive feedback loop between the functional changes in the Aβ-clearing mechanisms of both microglia and astrocytes as well as the promotion of neuroinflammatory mediators (Meda et al., 1995; Flint et al., 2008; Oksanen et al., 2017). The actions of several interwoven factors in AD pathophysiology are outlined in Figure 1. However, further studies are necessary to investigate why Aβ keeps accumulating despite the initial recruitment of supposedly protective cells in the context of external stresses on the GBA and to better understand the dynamic relationships between microglia, astrocytes, and the BBB.
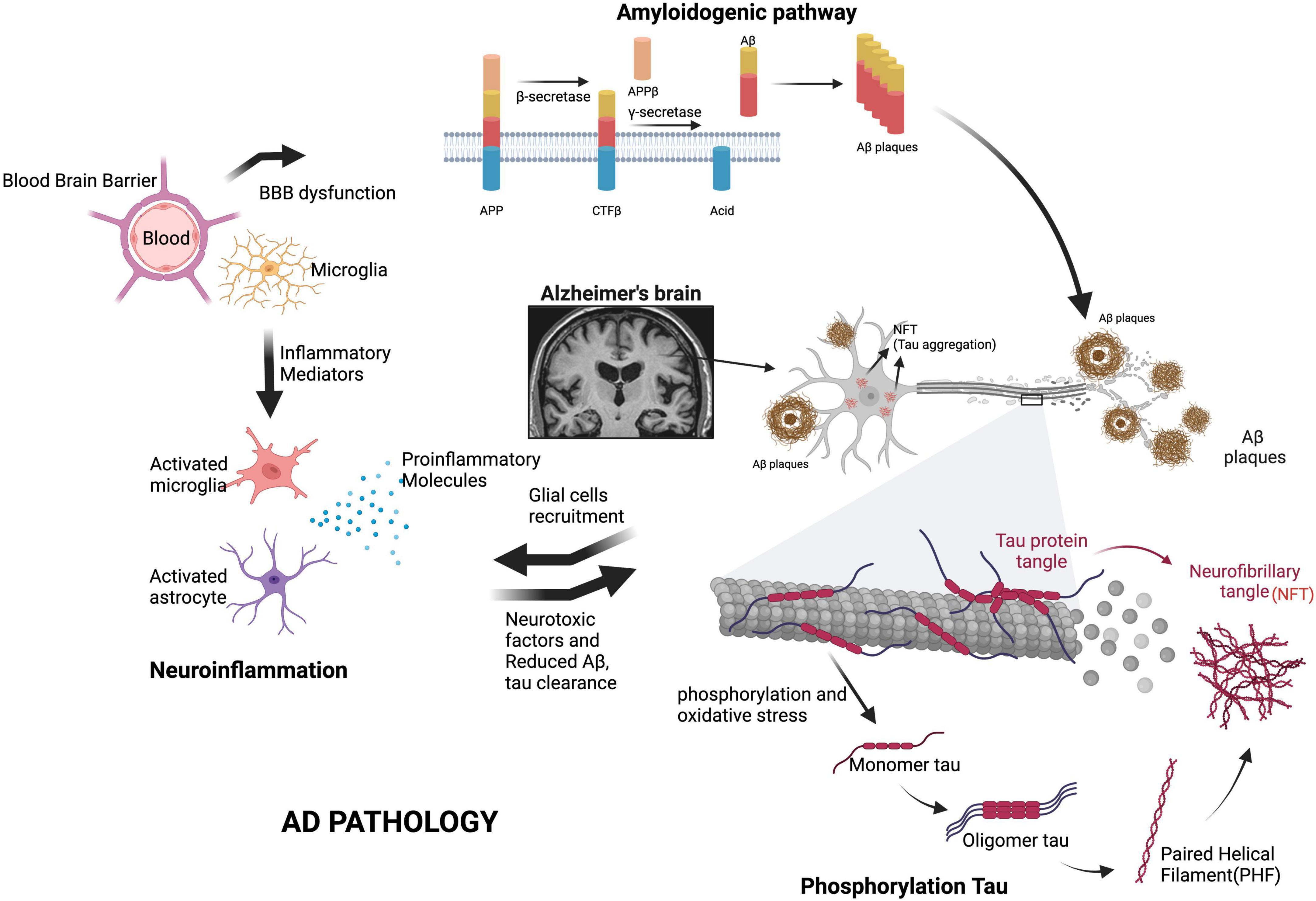
Figure 1. Key factors in AD pathogenesis. The formation of amyloid plaques through the overexpression of APP, tau hyperphosphorylation and NFT pathology, neuroinflammation, mitochondrial dysfunction and oxidative stress, translocation of harmful gut substances through a permeable BBB, and the eventual loss of synaptic function are the main causes of AD. Additional glial cell recruitment by proinflammatory mediators dysregulates Aβ and tau clearance, further contributing to neurodegeneration. Aβ, amyloid beta; APP, amyloid precursor protein; CTFβ, neurotoxic C-terminal fragment; BBB, blood-brain barrier. We have obtained the appropriate licenses from BioRender for this figure.
Mediators between gut dysbiosis and the brain in Alzheimer’s disease
The gut microbiota
The human gastrointestinal (GI) tract accommodates a complex and dynamic community of microorganisms that regulate several aspects of host physiology and interactions with the environment (Thursby and Juge, 2017). The vast estimate of bacterial cells in the GI tract replaces various functions of the host, including the reinforcement of gut integrity, immunomodulation, vitamin synthesis, fermentation of food components into absorbable metabolites, and protection against pathogens (Heintz-Buschart and Wilmes, 2018). Thus, a shift in the bacterial composition produces adverse yet foreseeable results, including the pathogenesis of several notable inflammatories and neuropsychiatric disorders (Kho and Lal, 2018). Furthermore, dysbiosis of the gut microbiota is associated not only with intestinal disorders but also with numerous extraintestinal diseases, such as neurological disorders (Brandscheid et al., 2017), including AD, which is the focus of this review.
The composition of the microbiota is constantly being modified by the host and environmental factors, therefore, a method for regulating exposure to the host immune system is required. To fulfill this condition, the GI tract has developed a dynamic intestinal barrier consisting of several factors that rely on the gut microbiota, including physical (the epithelial and mucus layers), biochemical (antimicrobial enzymes), and immunological (Immunoglobulin A) components (Hooper and Macpherson, 2010; Thursby and Juge, 2017; Ghosh et al., 2021). Of the several bacterial phyla present in the gut microbiota, Firmicutes and Bacteroidetes account for 90%, with the rest composed of the subdominant phyla Proteobacteria, Actinobacteria, Fusobacteria, and Verrucomicrobia (Hill J.M. et al., 2014; Rinninella et al., 2019).
The Firmicutes phylum, which consists of mostly Gram-positive bacteria and makes up the largest portion of bacteria in the gut, is composed of over 200 different genera, such as Lactobacillus, Eubacterium, Enterococcus, Ruminococcus, and most notably Clostridium (Rinninella et al., 2019). Bacteroidetes are the second most abundant phyla of Gram-negative bacteria and consist of predominant genera, including Bacteroides, Prevotella, and Xylanibacter (Sánchez-Tapia et al., 2019). Other relevant genera present in the gut microbiota include Escherichia and Desulfovibrio from the Proteobacteria phylum and Actinobacteria (Pushpanathan et al., 2019). The unique gut microbiota profile of enterotypes (Rinninella et al., 2019) will remain relatively stable after reaching a homeostatic climax composition of appropriate diversity, but is heavily affected by age, in which the microbiota composition becomes less diverse due to the characterization of higher Firmicutes: Bacteroidetes ratio, an increase in Proteobacteria and a decrease in Bifidobacterium (Ottman et al., 2012). Similar to age, prominent metabolic diseases establish a similar association between reduced microbial diversity and poor health (Valdes et al., 2018). Thus, diversity seems to be a good indicator of a healthy gut, accentuating the role of dietary patterns in cognitive health.
Gram-positive bacteria
Studies on the metagenomic sequencing of the human distal gut microbiome have revealed that a healthy gut microbiome is predominantly composed of Clostridium and Lactobacillus species with antimicrobial characteristics (Sweeney and Morton, 2013). Bacteria with a Gram-positive cell wall structure, such as Lactobacillus Rhamnosus and Bifidobacterium, can produce a favorable assortment of short-chain fatty acids (SCFA), most notably butyrate, by fermenting nondigestible carbohydrates and dietary fibers (Huang et al., 2018; Dalile et al., 2019).
Short-chain fatty acids
SCFAs are involved in plant-based food consumption and are associated with mucus production, the promotion of intestinal barrier integrity, and limits the translocation of bacterial proinflammatory molecules (Fukuda et al., 2011; Chambers et al., 2018; Silva et al., 2020). SCFAs are thought to play a critical role in the gut–brain crosstalk through brain uptake and modulation (Duscha et al., 2020), the inducement of morphological changes in glial cells, providing energy for colonocytes, and supporting the integrity of the BBB (den Besten et al., 2013; Silva et al., 2020). SCFA absorption by colonic epithelial cells also modifies the luminal pH levels in the colon, preventing pH-sensitive pathogenic bacteria associated with AD (Yatsunenko et al., 2012; Hill J.M. et al., 2014; Chakraborti, 2015). Intelligent pharmacological approaches consisting of anti-inflammatory drugs (Hill J.M. et al., 2014) in combination with dietary strategies that encourage the adequate production of SCFAs could be useful in the clinical management of AD.
Gram-negative bacteria
Bacteroidetes is a phylum of Gram-negative rod-shaped bacteria that account for about 25% of the anaerobes residing in the human colon (Wexler, 2007; Johnson et al., 2017). Sequence-based studies of gut bacterial diversity have revealed that Prevotella is more common in a plant-rich diet and has been associated with vegetarianism while the Bacteroides genus constitutes diets rich in animal protein and saturated fats (Tomova et al., 2019).
Bacteroidales and Bacteroides are by far the most abundant contributors to the biosynthesis of lipopolysaccharides (LPS), which are lipid-soluble outer-membrane complexes of Gram-negative bacteria (d’Hennezel et al., 2017; Tomova et al., 2019). Such endotoxins contribute to oxidative stress and the overproduction of inflammatory cytokines/chemokines through multiple structural components (Huszczynski et al., 2020) that initiate downstream inflammatory signaling, such as the NF-κB signaling pathway (Guo et al., 2013; Lin et al., 2020).
The translocation of endotoxins into the blood is accomplished by the breakdown of the intestinal epithelial barrier by pathogenic bacteria, specifically through interruptions in the paracellular, semipermeable barriers between individual epithelial cells composed of tight junction proteins (Ghosh et al., 2020; Hollander and Kaunitz, 2020). Increased paracellular transport of luminal bacteria and associated metabolites into the Lamina Propria and systemic circulation promotes proinflammatory interactions between bacterial products, epithelial cells, and immune cells activated through the TLR4/FAK/MyD88 signal transduction axis and auxiliary molecules associated with intracellular signaling (Guo et al., 2015; Lykhmus et al., 2016; Ghosh et al., 2020; Hollander and Kaunitz, 2020). Endotoxemia may be the basis for neurological complications in AD due to its role in gut dysbiosis and chronic inflammation.
The firmicutes: Bacteroidetes ratio
The relationship between Firmicutes and Bacteroidetes has become an auspicious target for the nutritional and therapeutic treatment of various pathological conditions affecting the GI tract, host energy metabolism, the immune system, and the CNS (Magne et al., 2020). Though the relative abundance of Firmicutes and Bacteroidetes varies between humans, abnormal alterations to the Firmicutes: Bacteroidetes ratio leads to gut dysbiosis and vice versa. Harach et al. (2017). have determined that there is a negative correlation between Firmicutes and Bacteroidetes: as one increases, the other decreases. Gut dysbiosis observed in this relationship specifically occurs either through the low-grade inflammation resulting from the release of lipid A (a component of LPS) by a surplus of Gram-negative bacteria or the increased efficiency of fermentation and metabolism of carbohydrates and lipids by an overabundance of Firmicutes bacteria (Bostanciklio, 2019; Stojanov et al., 2020).
Dysbiosis
Substantial studies have presented compelling evidence connecting impaired gut microbial diversity and abnormal Firmicutes: Bacteroidetes ratio to AD. In a study of 5XFAD mice with severe amyloid pathology (Oakley et al., 2006), it was noted that the abundance of Firmicutes greatly increased while the abundance of Bacteroidetes decreased, perhaps through the presence of metabolites belonging to other pathogenic bacteria (Brandscheid et al., 2017). Aβ and hyperphosphorylated tau levels in APPPS1 mice increased in correlation with age, thereby increasing neurodegeneration and reducing the abundance of Firmicutes, Verrucomicrobia, Proteobacteria, and Actinobacteria (Harach et al., 2017). Other studies that evaluated the diversity of Firmicutes and Bacteroidetes in patients with brain amyloidosis have determined reduced richness and diversity of bacterial taxa (Vogt et al., 2017) and a significant reduction in Eubacterium rectale and Bacteroides fragilis (Cattaneo et al., 2017), both of which have anti-inflammatory properties (Troy and Kasper, 2010; Islam et al., 2021). Furthermore, Zhuang et al. (2018) discovered a lower level of Bacteroidetes in patients with AD, while an increase in the Ruminococcus species, a Gram-positive bacterium with the potential to degrade mucus through the expression of intramolecular trans-sialidases (Mazloom et al., 2019).
Compared to cognitively normal controls, patients with AD had an altered gut microbiota composition due to differences in Bacteroidales, Ruminococcaceae, Selenomonadales, and Lachnoclostridium (Zhuang et al., 2018). Restoring and diversifying the Firmicutes: Bacteroidetes ratio with the proper probiotics and diet choices, especially through the introduction to a plant-based diet and probiotics, such as Lactobacillus (Mazloom et al., 2019), can help suppress inflammatory responses, enhance intestinal barrier function, and inhibit pathogenic bacterial adhesion, a strategy that has already shown promise in some patients (Tang et al., 2016; d’Hennezel et al., 2017). However, contradictory results concerning bacterial relationships remain (Chenoll et al., 2011), and further research is required to better understand these two dominant bacterial populations to choose the most appropriate probiotic strains or mixtures for optimal health outcomes.
Other prominent phlya
The population of Proteobacteria, a major phylum of Gram-negative bacteria, and Actinobacteria, a phylum of Gram-positive bacteria, typically constitute the remaining proportion of bacterial organisms in the gut microbiota (Khanna and Tosh, 2014). Actinobacteria, specifically Bifidocterium, are fundamental in the regulation of gut homeostasis and potentially improve memory function via hormonal signaling in the GBA (Mazloom et al., 2019). The effectiveness of such applications is possible due to the beneficial properties of Bifidobacterium, including the stimulation of dendritic cells to regulate intestinal immune homeostasis, the establishment of immune-protective measures while suppressing proinflammatory cytokines, and the overall improvement of GI barrier function (Mazloom et al., 2019).
Certain Bifidobacteria, in conjunction with Lactobacilli, have been observed to produce health-promoting conjugated linoleic acid and small antimicrobial peptides, which ward off pathogenic bacteria, such as E. coli, Salmonella, H. pylori, Listeria monocytogenes, and Rotavirus (Chenoll et al., 2011). In contrast, the increased abundance of Proteobacteria in the gut has been linked to gut dysbiosis and the pathogenesis of the disease (Rizzatti et al., 2017; Ruotolo et al., 2020). Although the connection between the prevalence of Proteobacteria in the gut during disease is not fully understood, it has been hypothesized that a combination of decreased oxygen levels by colonocytes in the lumen through beta-oxidation processes and the increased production of nitrate in the case of intestinal inflammation creates an anaerobic environment in which Proteobacteria can flourish (Rizzatti et al., 2017). Similar to Bacteroidetes, Proteobacteria also contain LPS in their outer membrane due to their Gram-negative staining, which induces low-grade inflammation and metabolic disorders (Azad et al., 2018).
Microbiome analysis
The microbial composition is thought to be influential in AD etiology and has attracted significant attention (He et al., 2020). Studies on the gut microbiota in patients with AD using fecal DNA samples revealed an overall decreased abundance of Firmicutes and Actinobacteria and an increase in Bacteroidetes and Proteobacteria (Rizzatti et al., 2017). Interestingly, the study identified potentially protective bacterial taxa in non-AD patients and observed a positive correlation between CSF inflammation biomarkers and an increased abundance of Bacteroides and Clostridiaceae, supporting a link between altered gut microbial composition and glial activation in AD (Rizzatti et al., 2017).
Similarly, a different study reported that a peripheral inflammatory state in AD patients with brain amyloidosis may be associated with an increase in proinflammatory Escherichia/Shigella and a decrease in anti-inflammatory E. rectale (Cattaneo et al., 2017). Although not as reliable, postmortem human studies do highlight the presence of various other pathogens, including H. pylori, Chlamydophila pneumoniae, and other spirochetes (Kowalski and Mulak, 2019). Additional studies on the microbiome of AP5P/PS1 transgenic mice revealed a general increase in Helicobacteraceae, Desulfovibrionaceae, Rikenellaceae, Odoribacter, and most notably Proteobacteria (typically from the Betaproteobacteria class) while showing a decrease in Prevotella (Biagi et al., 2010; Bäuerl et al., 2018). Mice, which are carriers of the APOE4 genotype, were associated with a loss of SCFAs due to a failure to replace decreased levels of butyrate-producing bacteria, such as Prevotellaceae and Ruminococcaceae (Tran et al., 2019).
The role of the gut–brain axis in Alzheimer’s disease
It is crucial to identify the main mechanisms of communication between the brain and the gut to understand how modifications to the gut microbiota can affect the nervous system in the context of AD. The GBA describes a complex system of bidirectional communication between the cognitive centers of the brain, intestinal functions and permeability, immune activation, enteric reflex, and enteroendocrine signaling (Carabotti et al., 2015). The GBA provides various metabolic pathways by which each respective system can influence the other, through mechanisms, such as the immune system, microbial metabolites, tryptophan metabolism, the VN, and the enteric nervous system, as well as the hypothalamus–pituitary–adrenal (HPA) axis (Ait-Belgnaoui et al., 2012; Cryan et al., 2019). The regulation of not only these mechanisms but also the permeability of the BBB and the intestinal barrier ensure efficient communication between the gut microbiota and the brain, which is essential for protecting the CNS (Daneman and Prat, 2015).
The general disruption of any neural, hormonal, and immunological pathways that define the GBA will affect intestinal motility and secretion, resulting in visceral hypersensitivity and cellular alterations of the immune and enteroendocrine systems (Appleton, 2018). Functional GI disruptions have pathological consequences associated with gut-related metabolic disorders, stress-related psychiatric disorders, and brain-related disorders (Appleton, 2018). Considering the bidirectional influence of the GBA, as well as emerging evidence advocating the use of probiotics to alleviate conditions exacerbated by gut dysbiosis, alterations to the gut microbiota may improve AD conditions.
Regulators of the gut–brain crosstalk
For communication between the CNS and the gut microbiome to occur, the passage through the intestinal barrier and the BBB must be well-regulated (Zhu et al., 2020). A layer of epithelial cells and mucus protects the intestinal barrier, facilitating the translocation of microbes, bacterial products, and other metabolites into the systemic circulation and lymphatic tissue (Zheng et al., 2020). Prominent bacterial molecules, such as SCFAs and LPS are major influencers of this semipermeable barrier by either promoting or inhibiting the translocation of proinflammatory cytokines, affecting the body’s immune response (Jergens et al., 2021).
Blood-brain barrier
The BBB is a highly specialized barrier of endothelial cells and intercellular junctions that line cerebral microvessels, regulating the entry of plasma components, red blood cells, leukocytes, and potentially neurotoxic molecules from the plasma to CSF in the CNS (Fung et al., 2017). The breakdown of the BBB may occur due to aging, disease, infection, or genetic factors that may drive fibrin and CNS amyloid deposition through dysfunctional Aβ transport (i.e., decreased activity of Aβ clearance mechanisms while an increase in Aβ40 and RAGE activity) (Rothhammer et al., 2016), but the role of circulating immune system cells in AD and the mechanisms that regulate this breakdown of the BBB are still poorly understood (Zenaro et al., 2017). However, BBB dysfunction is a major cause of chronic neuroinflammation through reduced Aβ clearance and endothelial transport, impairment of pericyte functions, decreased integrity of tight junctions, accumulation of toxins in the CNS, and the recruitment of glial cells and leukocytes in the brain (Fung et al., 2017). Further insight into leukocyte-endothelial interactions in AD, adhesion molecules, and bacterial-linked low-grade inflammation in both the intestinal and the BBB may offer valuable biomarkers of AD.
The immune system
The immune system is one of the main pathways through which microbes of the gut microbiota can interact with the GBA and the CNS (Appleton, 2018). The immune system consists of a complex network of innate components that can adapt and respond to various microbial and environmental challenges, a system that has largely evolved to maintain the symbiotic relationship of the host with the highly diverse microbes of the gut (Zenaro et al., 2017). Most host-microbe interactions occur at the luminal-mucosal interface secreted by the single-cell layer of the gut, in which the exchange of molecules through the mucous layer and epithelium serves to facilitate communication between the gut and the immune system through the recognition of antigens (Profaci et al., 2020).
Along with the stimulation and activation of the innate and adaptive immune systems, emerging evidence indicates that several commensal bacteria metabolites, including neuromodulators, bacteriocins, bile acids, choline, and SCFAs, are immunomodulatory and produce neurotransmitters, neuropeptides, endocrine messengers, and microbial by-products that can enter the blood and lymphatic systems, influencing neural messages through afferent neurons (Profaci et al., 2020). Likewise, the increased expression of proinflammatory cytokines and circulating bacterial endotoxins (LPS) due to gut dysbiosis can modulate the GBA by increasing intestinal permeability and triggering chronic low-grade inflammation in extraintestinal areas of the body (Appleton, 2018). Based on this influence, the colonization of the gut microbiota with a specific balance of anti-inflammatory bacteria, such as Bacteroides fragilis, Bifidobacterium, and Lactobacillus, as well as pro-inflammatory bacteria, including, E. coli, Enterobacteriaceae, and other segmented filamentous bacteria may encourage varied immunological responses (Fung et al., 2017).
Microbial metabolites
Tryptophan
Microbial metabolites have demonstrated an active role in directly governing CNS physiology through neurotransmitters and can influence the function and maturation of microglial cells (Belkaid and Hand Timothy, 2014), as well as astrocytes, which are affected by tryptophan metabolism (Wang Y. et al., 2018). The gut microbiota can influence astrocytes by activating receptors through microbial metabolites, such as Type 1 interferon and indoxyl-3-sulfate, which display anti-inflammatory properties and improve symptoms in experimental autoimmune encephalomyelitis (Rothhammer et al., 2016; Fung et al., 2017). Gut microbes that metabolize the essential amino acid tryptophan may interfere with tryptophan metabolism, limiting tryptophan availability for the host, which affects serotonergic neurotransmission, the function of central and enteric nervous systems, and may result in impaired cognitive functions (Kaur et al., 2019). Additionally, tryptophan can be diverted away from serotonin production and into the kynurenine/quinolinate pathways (metabolites of tryptophan), which has been proposed to disturb neurological functions and cause depression-like symptoms due to the reduction of tryptophan concentrations in the blood, thus limiting the production of important neurotransmitters in the brain, such as serotonin (Kaur et al., 2019). The significance of tryptophan metabolism in the GBA and its corresponding bacterial pathways indicates the importance of neuroactive compounds in host-microbiota interaction.
Neuroactive compounds
The gut microbiota encourages the production, modulation, and release of essential neurotransmitters that influence immune cells and alter brain functioning. Various Lactobacillus (Strandwitz, 2018), Bifidobacterium, and Escherichia species can synthesize Gamma-aminobutyric acid (GABA), the major inhibitory neurotransmitter of the CNS that is distributed throughout the host (Cryan and Dinan, 2012). GABA secretion decreases intracellular pH via the glutamtic acid resistance system, and its production has been linked to insulin sensitivity and reduced sensitivity to visceral pain in rat models (Strandwitz, 2018). Additionally, GABA levels in the cortices and the hippocampal regions in the brain are thought to be involved in controlling cortical excitability and working memory performance, and the functional enhancement of GABA inhibitory interneurons may help establish a resistance to Aβ deposition (Mandal et al., 2017; Xu Y. et al., 2020b). Other examples of species that can produce neurotransmitters include Candida, Streptococcus, and Escherichia in the production of serotonin, Saccharomyces in the production of noradrenaline, Lactobacillus in the production of acetylcholine, and Bacillus in the production of dopamine (Halverson and Alagiakrishnan, 2020). Bacterial production of SCFAs, such as butyric acid, propionic acid, and acetic acid, also affects the release of mucosal serotonin by stimulating the sympathetic nervous system, demonstrating the broader influence microbial metabolites have on cognitive processes (Reigstad et al., 2015; Maltz et al., 2018).
Microbial amyloids
Microbial-generated amyloids may play a crucial role in neuroinflammation and the formation of plaques (Fulop et al., 2018). Fungal surface structures and amyloidogenic fungal proteins, E. coli and curli fibers, and various Gram-negative bacteria with their respective amyloid systems were discovered to facilitate surface adhesion and biofilm development (a matrix of extracellular polymeric amyloids and other lipoproteins), which placed a tremendous systemic amyloid burden on the host when the intestinal barrier and the BBB were restructured (Hill and Lukiw, 2015). Biofilm-associated amyloids and lipoproteins produced by Firmicutes, Bacteroidetes, and Proteobacteria are recognized by microglia, which subsequently induce the production of cytokines that drive NF-kB signaling, phagocytosis, and innate immune defense responses that are repeatedly recognized to exacerbate the inflammatory response through Aβ peptide production (Miller A. L. et al., 2021).
Other than contributions to the autoimmune process, it has been hypothesized that bacterial amyloids may indirectly induce the aggregation of human amyloidogenic proteins (Friedland, 2015; Chen et al., 2016; Miraglia and Colla, 2019). Bacterial amyloids, such as curli, share structural and physical properties with human pathogenic amyloids and activate the same toll-like receptors that recognize Aβ and αSyn, which are further propagated from the gut to the brain by bacterial amyloids in biofilm and may cause motor deficits in AD and PD (Kim et al., 2019). Notably, it has been argued that microbial products and signals from the gut, such as αSyn, reach the brain via the VN rather than the circulatory system and the BBB, as corroborated in some mice models (Challis et al., 2020). Regardless of how exactly bacterial amyloids affect the CNS in AD, probiotics and other gut-modulatory therapies have been proposed as precautionary measures to reduce the pathological amyloid content of both the gut and the brain and reverse amyloid-induced neuroinflammation (Naomi et al., 2021).
The vagus nerve
A key mechanism of communication in the GBA involves the VN, which is the longest cranial nerve in the body and the main component of the parasympathetic nervous system that sends bidirectional information through afferent and efferent fibers (Breit et al., 2018). Because of its role in interoceptive awareness, the VN can sense microbiota metabolites through its vagal afferents, which can detect chemicals absorbed across the epithelial layer or released by epithelial cells in response to luminal stimuli (Xu Y. et al., 2020a). In turn, this gut information is then transmitted to the CNS, where it is integrated into the central autonomic network and relayed in the brainstem, where gut vagal afferents mostly synapse onto neurons in the solitary tract (Bonaz et al., 2018; Suarez et al., 2018).
The stimulation of vagal afferent fibers in the gut influences monoaminergic brain systems and engages the hypothalamus and limbic system, designating the VN as an important coordinator between neural, behavioral, and endocrine responses as well as a key target for the treatment of psychiatric, neurological, and inflammatory diseases (Breit et al., 2018). Conversely, the CNS can influence the intestines via the VN and efferent fibers (Alfonsetti et al., 2022). A cholinergic anti-inflammatory pathway that can dampen peripheral inflammation and decrease intestinal permeability has been identified in the VN’s fibers, thus playing an indirect but significant role in the alteration of microbiota composition (Bonaz et al., 2018). Descending projections from brain systems that have been activated due to stress inhibit the VN, resulting in deleterious effects on the GI tract and the autonomic activities of the gut that are observed in gastrointestinal disorders (Bonaz et al., 2018). Considering the extent of the VN’s role in the modulation of inflammation, the regulation of nutrition, and overall microbiota–brain communication, it may be advantageous to monitor vagal tones through VN stimulation, nutritional approaches, and psychotropic drugs to address gut dysbiosis (Breit et al., 2018).
The hypothalamus–pituitary–adrenal axis
Afferent spinal and vagal sensory neurons in the VN carry signals from the intestinal end to the brain stem, which engages the hypothalamus system (Breit et al., 2018). The HPA axis is a major neuroendocrine system that responds to psychological and physical stressors by releasing corticotropin-releasing factor (CRF) and, subsequently, adrenocorticotropic hormone (ACTH), which elevates cortisol levels (Farzi et al., 2018).
The neuroendocrine processes of the HPA axis in the context of AD can result in the continued activation of the immune system and the HPA axis itself, which encourages further alterations to intestinal permeability and motility when combined with a microbiota-driven proinflammatory state and increased exposure to LPS (Farzi et al., 2018). The direct consequence of early dysregulation of the HPA axis in patients with AD resulted in glucocorticoid over-secretion, which was discovered to be highly toxic in limbic structures and increased AD biomarkers (Canet et al., 2019). The hyperactivity of the HPA axis, elevated serum cortisol levels, and the dysregulation of the negative corticosteroid feedback system can increase the vulnerability of cerebral neurons and disrupt critical neurotrophic functions (Carabotti et al., 2015), indicating that the cascade of glucocorticoids may be the major mechanism responsible for behavioral alterations during AD progression (Ahmad et al., 2019). In addition to hypercortisolemia, the dysfunction of the hypothalamic–pituitary–gonadal axis may affect hormones with hippocampal receptors, resulting in the dysregulation of neuronal development and function in AD (Ahmad et al., 2019).
The gut microbiota’s involvement in the HPA axis response to stress, and ultimately AD, is a prime target for therapeutic strategies aiming to reverse stress-related conditions of AD. Studies that observed exaggerated HPA axis responses to stress in GF mice and reduced responses in GF mice colonized with specific Bifidobacterium species suggest that exposure to continuous stress can alter the organism’s microbiota composition and that the introduction of specific microbial populations can influence an organism’s stress responsiveness (Foster et al., 2017). The role of a gut-influenced HPA axis in the reversal of AD remains to be elucidated, and more detailed studies are necessary to establish the relationship between the hypersecretion of adrenal cortisol and amyloid pathology (Ahmad et al., 2019).
Perspectives on the gut–brain axis
The complexities of the GBA are not completely understood. However, changes in the gut–brain crosstalk that are induced by AD pathogenesis are generally associated with neurological complications and vice versa, as depicted in Figure 2. Considering that gut microbial disruptions induce alterations in the brain, it is within reason to expect a reversal of those modifications if the gut microbiota can be restored to its appropriate state (Boehme et al., 2020). Much of the observable influence of probiotics, fermented foods, and plant-based dietary patterns on cognitive status can be attributed to their ability to use the GBA communication systems to positively impact both the brain and the gut (O’Callaghan and van Sinderen, 2016). As a result, the use of dietary therapies to influence changes in neurological disorders is a compelling and imperative field of research.
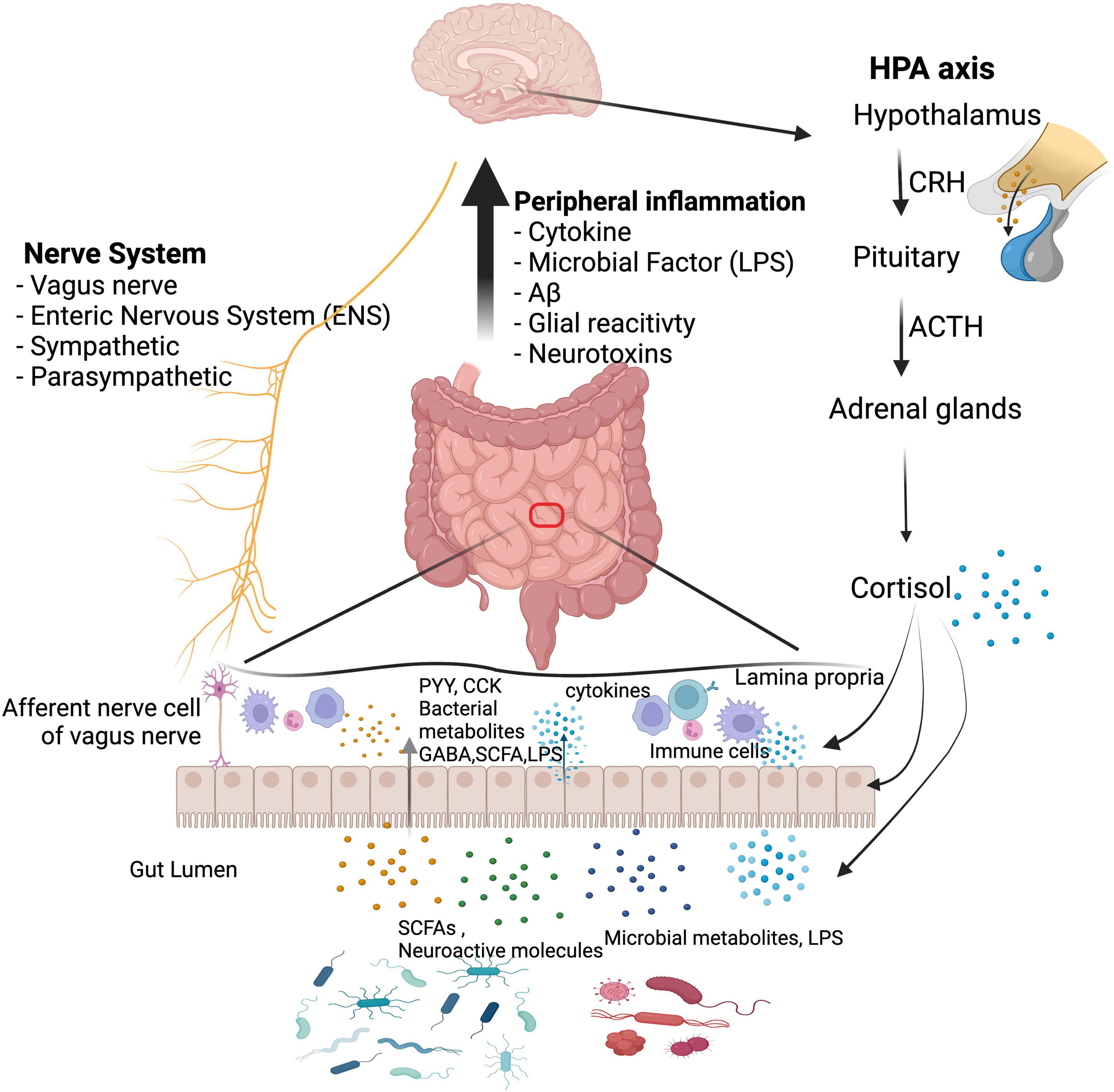
Figure 2. Communication networks between the gut microbiota and the brain. Communication pathways include immunological, neural, and hormonal pathways, which can function collectively: (1) MAMPs can activate both the peripheral and the central immune system; (2) microbial metabolites reach the brain to regulate neurological health; (3) a bidirectional influence is established between the CNS and the gut microbiota through the vagus nerve and the enteric nervous system; (4) interactions with the HPA axis regulate brain function and gut microbiota composition. Gut dysbiosis decreases the presence of beneficial substances (e.g., SCFAs) and increases toxic substances (e.g., amyloids and LPS), which cause the intestinal mucosal barrier and BBB to become more permeable, exacerbating inflammatory conditions. CRH, corticotropin-releasing hormone; ACTH, adrenocorticotropic hormone; PYY, peptide tyrosine tyrosine; CCK, cholecystokinin; GABA, gamma-aminobutyric acid; SCFA, short-chain fatty acid; LPS, lipopolysaccharide. We have obtained the appropriate licenses from BioRender for this figure.
The effects of food on the gut–brain axis
Although associations between diet, psychosocial stress, and neurodegenerative diseases have been reported, determining causal relationships in human studies is difficult. Nonetheless, numerous studies on AD models in recent years have supported nutritional therapies and dietary interventions, especially when subjects adhere to “AD-protective” diets, such as the Mediterranean-DASH Intervention for Neurodegenerative Delay (MIND) diet, which is a hybrid of the Mediterranean and Dietary Approaches to Stop Hypertension (DASH) diets (van den Brink et al., 2019). Conversely, Western dietary patterns, which typically consist of processed foods, convenience products, snacks, and sugary soft drinks, are deficient in essential nutrients, such as fibers, vitamins, and minerals, that are essential for preventing chronic metabolic inflammation (Christ et al., 2019). Several studies have discovered that such dietary patterns consisting of large amounts of processed carbohydrates, saturated fats, and so-called “empty” calories (Gush et al., 2021) exacerbate the inflammatory conditions associated with AD.
Another issue of interest is the role of probiotics and fermented foods in the prevention of AD through bioactive metabolites, an overall process that may be achievable through neuroendocrine/neuroactive compounds, gut microbiota diversification, and other neurological effects (Xiang et al., 2022). Though nutritional therapies have not been established as a preemptive measure for preventing or reversing AD, various studies suggest that future research should be conducted to explore the pathophysiological process and the subsequent development of efficacious, nonpharmacological strategies for AD prevention.
Probiotics and fermented foods
Probiotics are live microorganisms that provide a health benefit to the host by supporting a healthy digestive tract when applied to the body (Hill C. et al., 2014). Fermented foods, which are defined as “foods made through desired microbial growth and enzymatic conversions of food components,” are related to this process (Marco et al., 2021). Various fermented foods, most notably kimchi, yogurt, kefir, kombucha, sourdough bread, and soybean products, contain live cultures necessary for fermentation that have the potential to provide probiotic functions by modulating the gut microbiota, though human clinical studies are limited (Mota de Carvalho et al., 2018).
Common probiotic strains found in fermented foods include yeasts and a group of Gram-positive, non-spore-forming bacteria known as lactic acid bacteria (LAB) (Mokoena, 2017), typically consisting of Lactobacillus (Heller, 2001), Streptococcus, Leuconostoc, and Bifidobacterium (Rezac et al., 2018). Probiotic functions of LAB include inhibiting pathogenic bacteria colonization through competition and antimicrobial compounds, regulating intestinal transit, enhancing the intestinal barrier through anti-inflammatory cytokines and intestinal/epithelial cell growth factors, and regulating immune responses (Wang J. et al., 2018).
Probiotics also support the host by enhancing the production of nutritive compounds, such as antioxidant enzymes (Wang et al., 2017b), vitamin C, phenolic compounds, conjugated linoleic acid, folate (B9), and carotenoids (Peng et al., 2020; Xu Y. et al., 2020a; Pourbaba et al., 2021). LAB, Bifidobacterium, and other commensal bacteria provide additional vitamins, including riboflavin, vitamin 12, and vitamin K, which are essential to body functions, as well as SCFAs through carbohydrate fermentation (LeBlanc et al., 2017). Neuroactive compounds produced by probiotics may be significant to cognitive health, including serotonin, GABA, histamine, arachidonic acid, and adrenaline (Ma et al., 2020). Thus, certain fermented foods and probiotics may play crucial roles in neuroprotection through their interactions with the immune, endocrine, and nervous systems, the intestinal barrier, and the BBB.
The effect of probiotics on the gut–brain axis
One overarching theme linking specific probiotics to the prevention of neurodegeneration is potent anti-inflammatory action through the microbial modulation of antigen-presenting innate immune cells (macrophages, dendritic cells, and B-cells) in the subepithelial lamina propria tissue (Mann and Li, 2014) which positions the immune system in close proximity to the gut microbiota, antigens, and any pathogens that cross the epithelial barrier, allowing Toll-like receptors (TLRs) on these immune cells to recognize microbe-associated molecular patterns (MAMPs) and pathogenic ligands that initiate signaling cascades (Hug et al., 2018). Additionally, commensal microbiota produces a variety of secondary neuroactive molecules that interact with molecular signaling cascades (Morgan et al., 2007; Thomas et al., 2012; Tomaro-Duchesneau et al., 2012; Gim et al., 2013), which all play an extensive role in endocrine, lipogenesis, and apoptosis mechanisms that co-regulate key CNS processes with the brain (Clarke et al., 2014).
Neurotransmitter precursors
Some probiotics encode genes for specific enzymes that convert dietary substrates into corresponding neurotransmitters or precursors (Serrano-Pozo et al., 2011) while others act as signaling molecules to induce this process and stimulate enteroendocrine cells (Yano et al., 2015; Ceppa et al., 2020). Generally, neurotransmitters (e.g., glutamate, dopamine, serotonin, GABA) must be synthesized in the brain from local pools of amino acids and acted upon by corresponding neurotransmitter-producing cells and intermediate host enzymes, as functional neurotransmitters themselves cannot penetrate the BBB (Zaragozá, 2020; Chem et al., 2021). For example, enterochromaffin cells take up tryptophan from dietary protein to synthesize serotonin, a process that is regulated by the bacterial kynurenine synthesis pathway (Bailey and Cryan, 2017; de et al., 2018), where spore-forming probiotics (predominantly Clostridia) can promote the biosynthesis of serotonin and decarboxylation of the precursor 5-hydroxytryptophan (Yano et al., 2015; Luqman et al., 2018). Furthermore, colonic 5-HT production is influenced by various other probiotic metabolites (SCFAs) by promoting stimulatory activities on enterochromaffin cells (Reigstad et al., 2015; Yano et al., 2015). Accordingly, the dietary origins of these precursors enable the modified intestinal microbiota to influence host behavior by regulating the metabolism of these neurotransmitter precursors.
In vivo studies
Lactobacillus
Extensive studies in recent years have tried to establish the connection between Gram-positive bacteria, especially from the order Latobacillales, and the prevention of microglia-mediated neuroinflammation (Saez-Lara et al., 2015; Morshedi et al., 2019). A study on the spatial memory of rats with Aβ1–40 intrahippocampal injection used a probiotic mixture of Lactobacillus reuteri, Lactobacillus rhamnosus, and Bifidobacterium infantis to demonstrate decreased Aβ aggregation in the hippocampus, augmented antioxidant enzymatic activity, reduced oxidative stress markers, reduced levels of inflammation (namely IL-1β, IL-6, and TNF-α), and an increase in anti-inflammatory responses (IL-10) (Mehrabadi and Sadr, 2020). A follow-up study that compared blood and stool specimens of AD patients discovered that using a probiotic treatment significantly decreased IL-6 levels and increased IL-10 levels (Mombelli et al., 2020). A different study comparing similar serum and stool specimens of AD patients after a similar probiotic treatment of Lactobacillus, Lactococcus, and Bifidobacterium strains reported a protective adjustment to inflammation as well as an increase in kynurenine serum levels and tryptophan breakdown (Leblhuber et al., 2018).
Additionally, the gut status of a Drosophila melanogaster AD model before and after Lactobacillus plantarum DR7 administration revealed an overall reduction in Wolbachia and an increase in Acetobacter populations (Tan et al., 2020) while Abraham et al. (2019) determined that the supplementation of Lactobacillus acidophilus, Bifidobacterium longum, and various vitamins increased butyrogenesis and spatial memory, suggesting that gut dysbiosis accompanying AD can be reversed. While its role in cognitive function and the progression of AD has not been elucidated, bacteria from the Latobacillales order exhibit immunomodulatory and gut-modulatory effects.
Bifidobacterium
Bifidobacterium is another probiotic that has been identified as a beneficial modulator of the GBA by maintaining bodily functions and deterring pathogens (Unno et al., 2003). A study aimed at investigating the prevalence of AD pathophysiology after Bifidobacterium Lactis Probio-M8 intake quantified the number and size of Aβ plaques in APP/PS1 mice, and the researchers concluded that the specific probiotic treatment attenuated cognitive impairment by restoring the richness of a gut microbiota lacking in Lactobacillus and Streptococcus, resembling the gut diversity of a pre-treatment younger status (Cao et al., 2021). Interestingly, Kobayashi et al. (2017) discovered another strain of Bifidobacterium that did not affect the gut microbiota of AD model mice to a great extent at phylum levels but significantly increased the population of Actinobacteria and family Bifidobacteriaceae and may have even suppressed Aβ-induced changes by modulating genes associated with immune response in the hippocampus. The use of probiotics may be a strategy to promote Aβ clearance by regulating immune-reactive genes induced by Aβ (Tang et al., 2021) and not exclusively through the suppression of proinflammatory biomarkers.
Other probiotic strains
Other intestinal commensal bacteria with probiotic characteristics include Bacillales strains from the Firmicutes phylum and certain Clostridium clusters, although there are risks associated with some Clostridium species due to exotoxin release (Guo et al., 2020). A study reported that β-cell mass, insulin resistance, and Abeta deposition were normalized in AD rats fed a diet consisting of probiotic-infused chungkookjang (fermented soybeans), indicating that Bacillus licheniformis stimulated key pathways in glucose metabolism (Yang et al., 2015). Resistance to brain insulin signaling and impaired glucose metabolism are common features of AD, obesity, and diabetes, implying the existence of common mechanisms underlying these disorders (Ferreira et al., 2018). The decreased levels of insulin-like growth factors-I and –II displayed in AD brains accompanied by the attenuation of insulin-phosphoinositide 3-kinase-Akt signaling activates GSK-3b (a major kinase that increases tau phosphorylation) and decreases glucose and Aβ uptake by astrocytes (Yang et al., 2015; Ferreira et al., 2018). Similarly, Sun et al. (2020) discovered that Clostridium butyricum treatment ameliorated cognitive deficits and decreased Aβ oligomerization in APP/PS1 mice by reversing abnormal butyrate levels and gut microbiota alterations, which helped to suppress microglial activation. The comparative effects of probiotic formulations are further characterized in Table 1.
Conflicting evidence
Although there is substantial evidence for the abilities of probiotics and enhanced gut diversity to address gut inflammation and delay neurodegeneration, several other studies do not report such an anti-inflammatory effect on the brain, or at least a significant one. Agahi et al. (2018) reported that neither the cognitive function nor antioxidant biomarkers in AD patients were affected by probiotic supplementation consisting of Lactobacillus acidophilus, Lactobacillus casei, Lactobacillus fermentum, and Bifidobacterium bifidum, Similarly, a meta-analysis concluded that there were no forms of probiotic/prebiotic intervention that had a significant effect on global or specific domains of cognition, including fermented foods (Marx et al., 2020).
However, common reasons for these inconsistencies include the disease severity or stage, varying formulations of probiotic bacteria, supplement exposure time, the use of different cognitive-related inflammatory biomarkers and cognitive test designs, heterogeneity regarding the populations and sizes used, and an overall limited number of statistically powered studies (Agahi et al., 2018; Marx et al., 2020). Differences in the severity of the disease stage, as well as duration and timing of supplement administration, may be particularly crucial, as the loss of synapses and NFT development are irreversible pathological changes in later stages of AD and act as general thresholds of irreversible neurotoxicity (contributing to the failure of many anti-Aβ drugs) (Husna Ibrahim et al., 2020). In fact, Akbari et al. (2016) and Marx et al. (2020) conducted another clinical trial on people with multiple sclerosis using the same probiotic supplementation and observed a positive effect on motor behavior and gene expression of biochemical factors, indicating that the probiotics initially used have modulatory capabilities and that different factors specific to AD are negating the effects of probiotic interventions.
Thus, inconsistent effects of supplements on neurodegeneration should not be construed as paradoxical results, as factors, including age and stage of the disease, must still be considered (Agahi et al., 2018). Future studies are required to identify the most effective probiotic strains in the inhibition of AD progression, as well as the necessary timing for such therapies within a complex neurotoxic cascade.
Mediterranean and Mediterranean-DASH intervention for neurodegenerative delay diets
The Mediterranean diet pattern is renowned for being one of the healthiest diets in the world, consisting of vegetables, fruits, whole grain, legumes, nuts, vegetable oils, fish, shellfish, white meat, eggs, and dairy products, which provides a healthy profile of dietary fiber, low glycemic index, anti-inflammatory effects, and various antioxidants (Castro-Quezada et al., 2014). The Mediterranean-style diet (MeDi) lowers the risk of deficiencies in micronutrient intake and balances the intake of certain macronutrients, as it supplies high amounts of B group vitamins, antioxidant vitamins (vitamins E and C), and carotenes while increasing monounsaturated fatty acid (MUFA) consumption (Castro-Quezada et al., 2014; Akbari et al., 2016).
The Mediterranean diet and associated lifestyle habits can improve cardiovascular (Martínez-González et al., 2019) and cognitive health (Karstens et al., 2019) by providing better qualities of dietary fat, bioactive compounds, and overall nutrition, prompting the organization of health promotion strategies incorporating this diet, especially in populations with significant micronutrient deficiencies.
Dietary fiber
Dietary fibers are carbohydrates that are not hydrolyzed by the endogenous enzymes in the small intestine of humans (Berding et al., 2021). Prebiotic oligosaccharide isolates, or dietary carbohydrates that are selectively fermented by gut microbiota to confer health benefits to the host, are a well-known type of dietary fiber that reach the site of action in the colon and are fermented by saccharolytic microbes, such as Bifidobacteria (Kelly et al., 2021). To note, while several murine studies have demonstrated the psychophysiological effects of prebiotics, there are very few studies that have examined the targeting of the GBA in humans (Peterson, 2020).
The metabolization of various dietary fibers with complex chemical structures, such as starch or cellulose (Dhingra et al., 2012), has been associated with an increase in microbial diversity ranging in strain specificities and enzymatic capacities (Flint et al., 2008; Martínez et al., 2010; Alfa et al., 2018) that usually highlight several beneficial microbes, such as Bifidobacteria, Lactobacilli, Ruminococcus, and Akkermansia muciniphila (Berding et al., 2021), as well as the inhibition of pathogens (e.g. some Clostridium species, Enterococcus, Escherichia (So et al., 2018; Tangestani et al., 2020)). Independently of the gut microbiota, dietary fiber can interact locally with enterocytes, dendritic cells, macrophages, and monocytes and support epithelial barrier function by promoting the assembly of tight junction proteins or intestinal epithelial cell proliferation through AMP-activated protein kinase (AMPK), epidermal growth factor receptors, or TLR mechanisms (Bindels et al., 2017; Cai et al., 2020). Besides local effects, the growth of beneficial gut commensals and their metabolites associated with high fiber intake could be more relevant to the more distant brain-modulating properties.
The role of short-chain fatty acids in the gut–brain axis
SCFAs, the most obvious route of communication between the gut and brain that is mediated by dietary fibers, are absorbed into the portal circulation and mediate neurological signals principally via interactions with orphan G protein-coupled receptors/free fatty acid receptors and the inhibition of histone deacetylases (HDACs) (Offermanns, 2014; Koh et al., 2016). Through free fatty acid receptors, propionate initiates gluconeogenesis in the gut lumen through afferent circuits (Grompone et al., 2012), such as the dorsal motor nucleus of the vagus nerve (Lacassagne and Kessler, 2000), which accentuates central signaling processes (Sjögren et al., 2002). Importantly, the vagus nerve can be activated by fiber-responsive bacteria such as L. rhamnosus to stimulate BDNF expression (Berding et al., 2021).
Apart from direct vagal stimulation, SCFAs can act as endocrine signaling molecules that can easily be transported across the BBB by monocarboxylate transporters (Morgan and Zheng-gang, 2010) to influence brain biochemistry and longevity such as the Foxo gene locus (Braniste et al., 2014; Pino et al., 2014; Vidovic et al., 2018) by the HDAC inhibitory activity of butyrate (Chriett et al., 2019). Dietary fibers can mediate the production of glucagon-peptide 1, PYY, and ghrelin, gut-derived hormones that can cross the BBB and reach the brain, as such messengers can be stimulated by both gut microbes and SCFAs (Bauer et al., 2016).
Additionally, SCFAs modulate the expression of several neurotransmitter mediators, including the production of catecholamines through tyrosine hydroxylase expression (Fukumoto et al., 2003), stimulation of serotonin receptors on the vagal sensory fibers (Shah et al., 2006), and GABA receptors (Nankova et al., 2014). Although functional neurotransmitters cannot directly be produced from dietary fiber, bacterial species such as Lactobacillus, which are known neurotransmitter producers, respond to dietary fiber (Kato-Kataoka et al., 2016; Mika et al., 2018). Besides protecting the BBB against neurotoxic factors, SCFAs and consequently dietary fibers modulate the kynurenine pathway, which can cross the BBB and metabolize into kynurenic acid (Westfall et al., 2017). Thus, it is certain that there are many underlying avenues of the fiber-brain crosstalk that have been identified and will surely emerge.
Fatty acids
Monounsaturated fatty acids
MUFAs include one double bond in the fatty acid chain with the remainder single-bonded (Hammad et al., 2016). The alleviation of endothelial dysfunction and reduced insulin concentrations by the consumption of the MeDi may be linked to high consumption of MUFAs such as oleic acid that are derived from extra virgin olive oil (Ye et al., 2021), which enhances the gut microbiota diversity of models under risk of metabolic syndrome (Pu et al., 2016; Hidalgo et al., 2018; Lang et al., 2018) by raising the Firmicutes: Bacteroidetes ratio, Bifidobacterium (Actinobacteria) populations in humans (Machate et al., 2020), and Bifidobacteria to E. coli ratios (Miller C.B. et al., 2021). Indeed, further studies suggest that replacing SFA-enriched diets with MUFA such as the MeDi had a positive impact on butyrate-producing bacterial populations, notably the Bifdobacteriaceae family, Prevotella, Turicibacter, Roseburia, Oscillospira, and Verrucomicrobia (Wolters et al., 2019).
Thus, MUFAs may alter gut microbiota structure to favor SCFA-producing organisms, and an anti-inflammatory profile has been described (Michielsen et al., 2019). Studies comparing mice raised on a MUFA-rich diet versus a SFA-rich diet show higher circulating levels of anti-inflammatory markers, including IL-4, IL-10, and PPARγ, as well as lower levels of pro-inflammatory markers such as IL-6, IL-8, MCP-1, IL-1β, CRP, and TNF-α (Tamer et al., 2020). In correlation, MUFAs decrease M1 macrophage infiltration and stimulates M2 macrophage polarization (Ravaut et al., 2021), especially through adiponectin expression (Lovren et al., 2010) and the treatment of immune cells with palmitoleate (Chan et al., 2015). Palmitoleate decreases NF-κB nuclear translocation via the stimulation of PPARγ and the phosphorylation of AMPK, which increases MGL2, IL-10, TGFβ1, and MRC1 (Yang et al., 2010; Chan et al., 2015). Another prominent MUFA is oleate, which inhibits LPS-induced IL-1β maturation (Finucane et al., 2015), reverses cell death pathways activated by SFAs (Sieber et al., 2010), and protects various cells from palmitic-mediated insulin resistance (Coll et al., 2008). Though there is a lack of substantial studies so far, the addition of MUFA in diets may be a nutraceutical avenue to ameliorate the general metabolic profile of AD.
Polyunsaturated fatty acids
Omega-3 (n-3) long-chain polyunsaturated fatty acids (n3 LC-PUFA), which are also highlighted in the MeDi, have demonstrated the ability to increase SCFA-producing organisms, such as Bifidobacterium and Lactobacillus (Ghosh et al., 2013), and suppress mediators of mucosal inflammation such as Helicobacter, Enterobacteria, and Firmicutes (Yu et al., 2014), which reduces impaired cytokine/chemokine induction (Ghosh et al., 2013), suppresses LPS-producing bacteria to address endotoxemia conditions (Kaliannan et al., 2015), and enhancing intestinal wall integrity (Costantini et al., 2017). Furthermore, n3 LC-PUFAs could modulate intestinal epithelial barrier integrity and overall enteral health through the regulation of intestinal alkaline phosphatase to modify intestinal membrane pH, as proven by eicosapentaenoic acid (EPA), docosahexaenoic acid (DHA), and α-Linolenic acid (Costantini et al., 2017). These essential fatty acids could attenuate the decrease of the Firmicutes: Bacteroides ratio (Liu et al., 2012), detoxify LPS (Kaliannan et al., 2015), and be incorporated into microbiota membranes, which modifies adherence to the intestinal membrane (Ye et al., 2021). The resolution of inflammation has also been observed, where DHA could inhibit TLR4 activation and address LPS levels (Hwang et al., 2016) while EPA could increase TGF-β synthesis (Liu et al., 2012). Of interest is the n3/n6 PUGAs ratio, which may be implicated in the promotion of anti-inflammatory effects (Simopoulos, 2016) and the modulation of endocannabinoid signaling, which is involved in brain development, cytokine release from microglia, neurotransmitter release, and synaptic plasticity (Cristino et al., 2020).
Antioxidants
Vitamins
The nutrients having antioxidant potential can both stop the excess production of free radicals and promote scavenging of prevailing free radicals in the host body (Eke et al., 2017), which supports cellular and humoral immune responses (Crump et al., 2013). Vitamins are associated with various microbial synthesis pathways, being produced by and influencing the gut microbiota composition (Sun, 2010) and reducing inflammatory markers such as IL-6 and TNF-α (Shirpoor et al., 2016). In addition, vitamin E such as α-tocopherol and γ-tocopherol-rich tocopherols seem to be implicated in the mitigation of mucosal tissue damage via altering the composition of gut microbiota in colitis-induced mice (Liu et al., 2021), as well as exhibiting powerful antioxidant properties on lipoproteins and cell membranes (Hasegawa et al., 2001). Moreover, vitamin E was associated with the capacity to modulate the Firmicutes: Bacteroidetes ratio at the phylum level (Yang et al., 2020), lower inflammatory mediators from the Proteobacteria and Firmicutes phylum (Mandal et al., 2016), and increase butyrate-producing bacteria, such as Roseburia (Tang et al., 2016). The redox state of vitamin C, another essential antioxidant, was also found to restore Lactobacillus, Bifidobacterium, and Coriobacteriaceae populations as well as decreasing Bacteroidetes and E. coli (Xu et al., 2014). Furthermore, vitamin C impacts the chemotaxis process in neutrophils and the phagocytosis process of microbes by protecting cells from oxidative explosions (Cerullo et al., 2020).
Polyphenols
Polyphenols sequester the ROS and RNS to prevent the formation of toxic Aβ oligomers and NFTs (Ruotolo et al., 2020). Polyphenols that can selectively detoxify Aβ oligomers are generally capable of crossing the BBB (Hwang and Yen, 2008) such as hesperetin, hesperidin, neohersperidin, citrus flavanones, and various aryl-γ-valerolactone and arylvaleric acid derivatives, which are major compounds of derived from the bacterial metabolization of flavan-3-ols (Unno et al., 2003; Reddy et al., 2020). Further metabolism of the valerolactones results in the formation of bioavailable phenolic degradation products and secondary polyphenolic metabolites, which have demonstrated greater BBB permeability in vivo and can attenuate neuroinflammation (Carregosa et al., 2020). Additionally, dietary polyphenolic compounds undergo extensive catabolism in the colon to form small polyphenolic compounds such as urolithins and pyrogallol, which are effective as antioxidant agents (Verzelloni et al., 2011) and can attenuate the neuroinflammation in BV2 microglia through the NF-κB, MAPK, and Akt signaling pathways (Xu et al., 2018). Indeed, Taxifolin, a naturally occurring flavonoid polyphenolic compound, exhibited neuroprotective effects in mice models by reducing the production of TREM2 (Reddy et al., 2020).
Carotenoids
Besides exerting various antioxidant properties through the neutralization of ROS, several carotenoids have showcased anti-neuroinflammatory properties that address LPS-induced mechanisms in microglia, such as lutein through the inhibition of NF-κB and lipid peroxidation (Kim et al., 2008; Wu et al., 2015) and Fucoxanthin through the inhibition of NF-κB, protein kinase B, and MAPK signaling and subsequently TNF-α, ROS, IL-6 production (Lee et al., 2021). Crocetin and crocin were found to inhibit LPS-induced nitric oxide and cytokine formation in microglia and block the effects of LPS on hippocampus cell death (Nam et al., 2010), while Ilycopene decreased LPS-induced expression of IL-1β, heme oxygenase-1, IL-6, and TNF-α throughout the plasma and brain in murine models (Sachdeva and Chopra, 2015; Zhang et al., 2016). Collectively, such findings suggest that carotenoids act as strong anti-inflammatory agents in the CNS. Additionally, β-carotene is a precursor to vitamin A, which could increase the abundance of Lactobacillus to protect against norovirus infections (Rackerby et al., 2020) and increase other bacteria, such as Allobaculum, Akkermansia, and Bifidobacterium (Lee and Ko, 2016), promoting intestinal barrier function. The restorative function of vitamin A on the Firmicutes: Bacteroidetes ratio is also an interesting area of study (Nan et al., 2021).
Clinical studies
Mediterranean diet
Studies on the MeDi form a clearer consensus regarding the relationship between human gut modulation and cognitive performance compared to the exclusive use of probiotics and/or AD models. A study consisting of middle-aged adults discovered that lower MeDi adherence correlated with higher rates of Aβ deposition, hypometabolism, hypertension, and a greater number of AD biomarker abnormalities (e.g., higher C-Pittsburgh compound B PET deposition), while higher MeDi adherence was estimated to provide up to 3.5 years of protection against AD due to the normalization of the aforementioned observations (Berti et al., 2018) and could potentially alleviate prodromal AD symptoms (Ballarini et al., 2021). Notably, the MeDi has higher scores of monounsaturated/saturated fat ratios, whose phenolic components have been associated with reduced AD pathology in animal models (Qosa et al., 2015; Román et al., 2019) and a lower risk of mild cognitive impairment (Martinez-Lapiscina et al., 2013; Ballarini et al., 2021; Tzekaki et al., 2021).
Additionally, a modified Mediterranean-ketogenic diet is thought to stimulate intestinal SCFA production and reduce the abundance of genetic pathways associated with bacterial toxins, energy balance, and inflammation (Nagpal et al., 2019; Rusek et al., 2019). Due to the production of ketone bodies through such diets and their ability to reverse energy hypometabolism, regulate glutamate release, and promote Aβ clearance across the BBB (Versele et al., 2020), Mediterranean diets enriched with isocaloric coconut oil are considered an alternative to glucose for brain metabolism and sustainment of neurogenesis through the assembly of a larger number of medium-chain triglycerides (de la Rubia Ortí et al., 2018; Chatterjee et al., 2020).
Mediterranean-DASH intervention for neurodegenerative delay diet
The concept of a combination of modified diets has been extended to Mediterranean and DASH diets, producing a hybrid form known as the MIND diet (Castro-Quezada et al., 2014). Multiple cross-sectional, longitudinal, and intervention studies on the elderly with an increased risk for AD support the mitigation of cognitive deficits in AD based on a higher MIND diet adherence score (Castro-Quezada et al., 2014; Tangney et al., 2014; Morris et al., 2015; Mosconi et al., 2018; van den Brink et al., 2019; Wesselman et al., 2021). These strong associations between dietary patterns and cognitive domains may have been mediated by effects on medial temporal atrophy and cerebral amyloid pathology through the gut microbiota and immune system modulation (Ballarini et al., 2021; Wiȩckowska-Gacek et al., 2021). In some cases, the recently developed MIND diet was discovered to be more effective compared to the independent use of either the Mediterranean or DASH diets, revealing a stronger inverse association with AD pathogenesis (Castro-Quezada et al., 2014; Hosking et al., 2019) and premature death in the highest tertile of adherence (Corley, 2020). The superior effectiveness of the MIND diet in recent studies may be explained by the consumption of nuts, the synergistic action of additional dietary components specific to neuroprotection excluded in the MeDi (including unique categories of green leafy vegetables and berries providing potent polyphenols), as well as the restriction of particular less-healthy foods used in the calculation of the MIND score and not usually accounted for in the MeDi (Hosking et al., 2019; Corley, 2020). Longitudinal and cross-sectional studies investigating the association between adherence to the MeDi/MIND diet and cognitive health are outlined in Table 2.
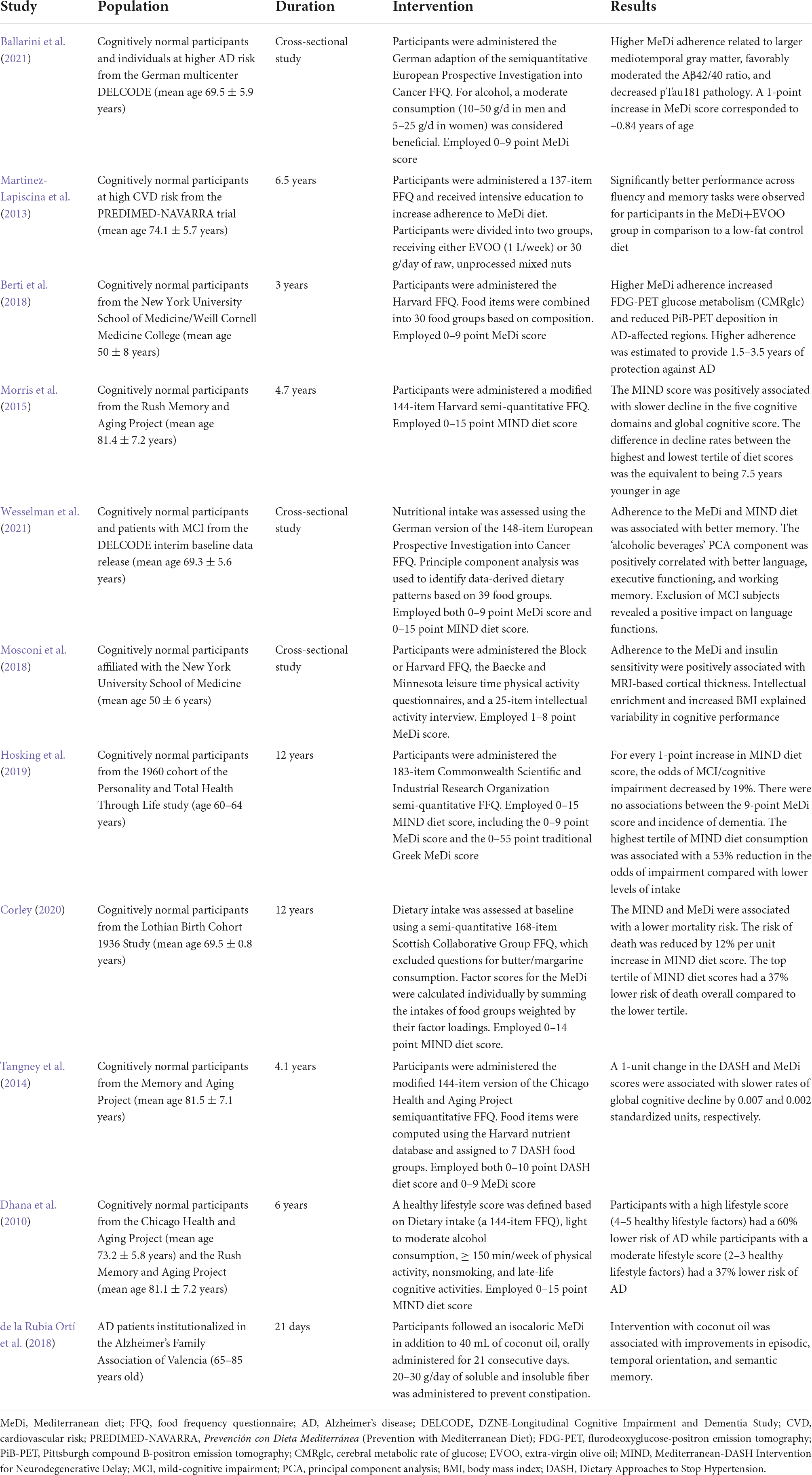
Table 2. Results of included studies investigating the relationship between MeDi/MIND diet adherence and cognitive performance.
Perspectives on Mediterranean dietary patterns
While clinical applications of potential dietary pathways are yet to be fully justified, several studies indicate that healthier diets focusing on neuroprotection through specific nutrients and vitamins (e.g., docosahexaenoic acid and other omega-3 fatty acids, vitamins B complex and E, and a lower sodium consumption), physical exercise, and a healthy lifestyle profile (Smith, 2019) could initiate a cascade of metabolic alterations in the gut microbiota, which suppress inflammation and oxidative stress (Sano et al., 1997; Dhana et al., 2010; Yurko-Mauro et al., 2010; Kim et al., 2013; Morris et al., 2017; Moore et al., 2018; Rocaspana-García et al., 2018; Faraco et al., 2019) as depicted in Figure 3. As these lifestyle factors are easily modifiable by the individual, it is imperative to promote feasible strategies consisting of healthy, nutrient-rich diets and lifestyle behaviors among older adults to delay or prevent AD. Other studies with larger samples and longer longitudinal follow-ups are needed to replicate these research findings in community-based samples with more diverse economic, medical, and social backgrounds, as well as to determine whether AD biomarker changes are predictive of AD onset in relation to healthy diet adherence and exposure time (Berti et al., 2018).
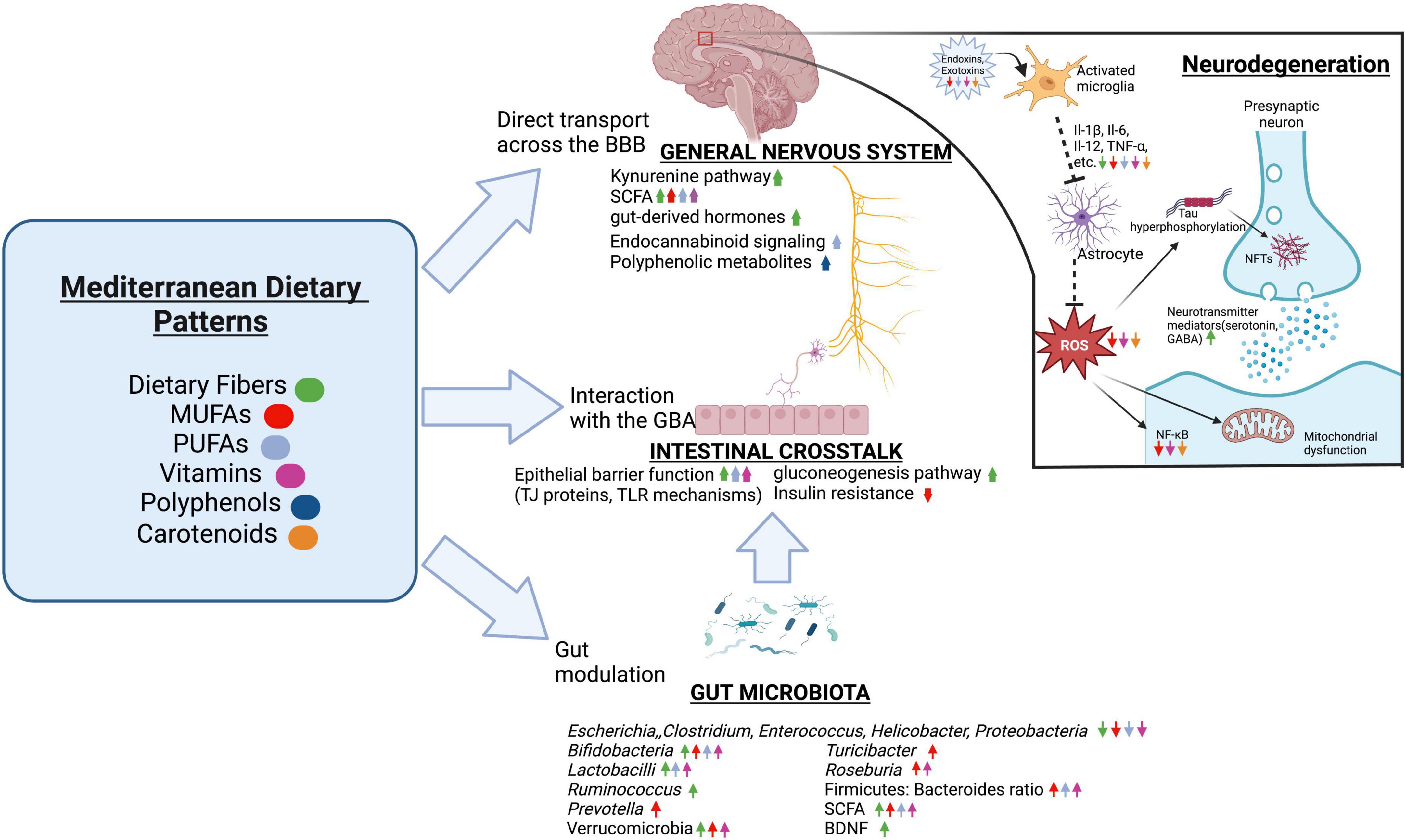
Figure 3. The effects of Mediterranean dietary components on the GBA. Dietary fibers, MUFAs, PUFAs, vitamins, polyphenols, carotenoids, and other bioactive compounds have been found to stimulate SCFA-producing organisms while inhibiting pathogenic bacteria, reducing endotoxemia and inflammatory pathways such as NF-κB, protecting gut barrier integrity independently of the gut microbiota, and promoting the production and translocation of neuroactive molecules across the tightly regulated BBB to directly influence the CNS. Additionally, many components of the Mediterranean dietary pattern neutralize oxidative stress and protect against apoptosis. The color of the arrows correspond to one of the six dietary components, where an up arrow represents the promotion of a factor of the GBA while a down arrow represents the inhibition of said factor. SCFA, short-chain fatty acid; BBB, blood-brain barrier; BDNF, brain-derived neurotrophic factor; TJ, tight junction; TLR, toll-like receptor; MUFAs, monounsaturated fatty acids; PUFAs, polyunsaturated fatty acids; LPS, lipopolysaccharide; IL-1β, Interleukin 1-beta; TNF-α, tumor necrosis factor-alpha; NO, nitric oxide; ROS, reactive oxygen species; GABA, gamma aminobutyric acid; NF-κB, Nuclear factor-κB; GBA, gut-brain axis. We have obtained the appropriate licenses from BioRender for this figure.
High-fat diets
In contrast to probiotics, fermented foods, MIND/MeDi diets, and other plant-based dietary patterns, the Western diet (WD) is known to promote diverse forms of inflammation and disease through gut modulation (Nagpal et al., 2019; Rusek et al., 2019). The WD is characterized by a high protein content from fatty domesticated and processed meats, saturated fats, refined grains, sugar, alcohol, salt, and corn-derived fructose syrup obtained from common foods (Nagpal et al., 2019; Versele et al., 2020). Major hallmarks of the WD include the ultra-processing of foods and the breakage of cell walls in fiber-rich foods, the use of food additives and noncaloric artificial sweeteners by the masses (emulsifiers have pro-inflammatory potential, while lecithin has the opposite effect, prompting inquiry regarding the role of food additives), and increased amounts of readily accessible acellular nutrients in the small intestine and the colon, which facilitates the growth of certain bacteria specialized in metabolizing simple sugars and promoting pathogen-associated molecular patterns (Zinöcker and Lindseth, 2018). Due to increased territorial competition, the tendency to favor foods providing microbial and human cells with more easily digestible substrates usually implies the reduced intake of cellular plant-based foods and, consequently, the diminished growth of bacteria that degrade fiber and produce beneficial metabolites (e.g., SCFAs) (Statovci et al., 2017). These observations indicate that diet-induced dysbiosis, metabolic disorders, and neuroinflammation are interconnected.
Alzheimer’s disease biomarkers
In cross-sectional and longitudinal studies, higher intake of fruit, vegetables, whole grains, oily fish, low-fat dairy, and relevant vitamin supplements (e.g., B12, folate, D) was associated with a reduction in AD biomarkers, such as Aβ40 and Aβ42, tau levels in the CSF, and chemical compounds used in PET imaging, while a diet consisting of high glycemic, high saturated fat foods was associated with an increase in the said biomarker burdens (Hill et al., 2019). Additionally, the WD may augment tau phosphorylation. After treating C57BL/6NHsd mice with a high-fat diet, Kothari et al. (2017) observed an increased expression of the active form of GSK-3β, hyperactivation of Akt and AMPKSer485 through insulin resistance (which inhibits tau dephosphorylation), and a significant decrease in Activity Regulated Cytoskeleton-associated protein, which is crucial in long-term memory consolidation. In turn, the loss of synaptic plasticity through the formation of Aβ plaques and NFTs promotes neuroanatomical changes. Interestingly, adherence to the WD may increase gray matter volume and cortical thicknesses in temporoparietal regions, supporting mounting evidence that early, preclinical increases in cortical volumes foreshadow the pathogenesis of AD later in life because such developments may indicate neuroinflammation (Frye et al., 2021). The mechanisms underlying increased cortical volumes in the context of AD progression require further study to better differentiate between resilient phenotypes and signs of vulnerability, allowing intervention to occur before mild-cognitive impairment (Christensen et al., 2013; Mancuso et al., 2014; Frye et al., 2021).
Endotoxins
The WD exhibits an adverse effect on the CNS by upsetting barrier integrity and the balance of gut metabolites, causing chronic activation of glial cells and increasing AD biomarkers (Naja et al., 2015; Statovci et al., 2017). Studies comparing the effects of high-fat WD against plant-based MeDi on AD models (i.e., C56BL/6J mice, APPswe mice, and APP21 transgenic rats) treated with LPS and/or subjected to contextual fear conditioning discovered overall significant differences in brain neuroinflammation status and cognitive testing results between diet groups, with mice consuming the WD exhibiting more cognitive decline during open-field testing (Ivanova et al., 2020; Munster, 2020; Wiȩckowska-Gacek et al., 2021). These findings reveal that mice fed MeDi were better protected against cognitive deficits and AD-related pathologies induced by LPS treatment in the gut and the presence of human APP, including the strong enhancement of full-length APP levels and cleavage of its C-terminal forms, the increased presence of soluble Aβ42, WD-induced hippocampal astrogliosis, and widespread white matter microgliosis, than mice fed WD (Kahn et al., 2012; Ivanova et al., 2020; Wiȩckowska-Gacek et al., 2021).
Genetic alterations
Persistent adherence to a high-fat diet aggravated AD-like phenotypes and TREM2 levels in APP23 mice, principally through the altered expression of genes involved in immune responses, nervous system development, synaptic transmission, mitochondrial biogenesis, and apoptosis regulation (Nam et al., 2017). The complementary effects of these gut-modulatory processes relating to mitochondrial dysfunction, enhanced inflammatory responses, increased deposition of Aβ, and the reduced expression of genes related to neuronal projection contribute to the neurotoxic presence of Aβ plaques, which induces behavioral changes reflected in AD models (Nam et al., 2017; Ivanova et al., 2020).
Perspectives on western dietary patterns
Ultimately, diets can be considered a nonpharmaceutical tool to accentuate cognitive performance in racially diverse populations (Nutaitis et al., 2019). However, diets lacking fermentable fibers in favor of foods high in saturated fats and virulence potential have become alarmingly commonplace (Rakhra et al., 2020). In addition to metabolic syndrome, which is found to be contemporaneous with the progression of neurodegeneration in AD models, WD strongly accelerates the AD pathological processes through LPS translocation, BBB impairment, intensification of the amyloidogenic APP cleavage path, and chronic activation of glial cells with impaired phagocytic abilities (Ivanova et al., 2020). Thus, the WD should be considered a vital modifiable risk factor of AD development. Accordingly, society must aim to provide better dietary advice on how to better nourish both the gut microbiota and the host.
Discussion
It is unclear whether most established physiological dysfunctions are causative factors of AD, results of AD, or both. According to the existing body of literature, AD pathogenesis is triggered by the interdependent reverberations of Aβ plaque formation, hyperphosphorylation of tau protein, NFT accumulation, glial inflammation, and the ultimate loss of neural plasticity and proper neuron function. The search for nonpharmaceutical tools to relieve AD symptoms sparked interest in gut-modulatory therapies, which are expected to have a considerable influence on brain homeostasis through the GBA.
The GBA allows microbes, metabolites, and neuroactive compounds to communicate via a complex network of neural, hormonal, and immunological pathways. As a result, dietary interventions help reverse increased oxidation levels, impaired neuroactive pathways, and damage to the HPA axis, conferring health benefits that could prove helpful in managing AD. Fixing diet-induced dysbiosis is an especially intriguing first step toward addressing the AD epidemic, as current FDA-approved medications have proven to be ineffective and emphasize the need for alternative approaches. Indeed, several researchers speak of the promising potential of gut microbiota-modifying therapies, such as probiotics, fermented foods, and plant-based dietary patterns. Inevitably, eliminating Western foods and unhealthy habits may also reverse the progression of neurodegenerative diseases.
Yet, while research attention has focused on the potential ameliorating role of diet in neurodegeneration, there has been little evidence underscoring both disease-modifying/total reversal capabilities as well as the practicality of an all-encompassing diet therapy for the clinical management of AD, especially in late-onset stages. Thus, significant lifestyle changes should be considered as purely a preventative measure for AD in the healthy population, and this quality could be made a priority during stages of mild cognitive impairment to help alleviate stresses on the GBA. This is especially vital considering the feasibility of simple dietary switches before and during the early stages of AD. Research on cases of severe cognitive decline should review the plausibility of dietary adherence in the context of medication side effects, a decline in appetite, the need to shift focus to the prevention of malnutrition (which entails a high-calorie diet rather than specific dietary adherence), and the consideration that aggressive nutritional support may not be appropriate once significant neurodegeneration has occurred. These concerns raise the need to identify the exact period in late-stage AD when it becomes medically futile to take dietary precautionary measures.
Future studies should continue to compare the longitudinal effectiveness of different dietary patterns as a strategy to reverse AD during progressive stages, identify the relative sequence and timing of such dietary interventions within a very complex cascade of physiological events with the assistance of preclinical biomarkers, and help establish future dietary guidelines that promote neuroprotective foods and augment brain function.
Author contributions
DL, VL, and SH: conceptualization. DL: writing—original draft preparation. DL and SH: writing—review and editing. VL and SH: funding acquisition. All authors have read and agreed to the published version of the manuscript.
Funding
This research was funded by the National Institute on Aging (grant no. T32AG000255).
Acknowledgments
We thank John Q. Trojanowski for valuable discussion and feedback.
Conflict of interest
Author SH was employed by Genetech, Inc.
The remaining authors declare that the research was conducted in the absence of any commercial or financial relationships that could be construed as a potential conflict of interest.
Publisher’s note
All claims expressed in this article are solely those of the authors and do not necessarily represent those of their affiliated organizations, or those of the publisher, the editors and the reviewers. Any product that may be evaluated in this article, or claim that may be made by its manufacturer, is not guaranteed or endorsed by the publisher.
References
Abraham, D., Feher, J., Scuderi, G. L., Szabo, D., Dobolyi, A., Cservenak, M., et al. (2019). Exercise and probiotics attenuate the development of Alzheimer’s disease in transgenic mice: role of microbiome. Exp. Gerontol. 115, 122–131. doi: 10.1016/j.exger.2018.12.005
Agahi, A., Hamidi, G. A., Daneshvar, R., Hamdieh, M., Soheili, M., Alinaghipour, A., et al. (2018). Does severity of Alzheimer’s disease contribute to its responsiveness to modifying gut microbiota? a double blind clinical trial. Front. Neurol. 9:662. doi: 10.3389/fneur.2018.00662
Ahmad, M. H., Fatima, M., and Mondal, A. C. (2019). Role of hypothalamic-pituitary-adrenal axis, hypothalamic-pituitary-gonadal axis and insulin signaling in the pathophysiology of Alzheimer’s disease. Neuropsychobiology 77, 197–205. doi: 10.1159/000495521
Ahmed, Z., Cooper, J., Murray, T. K., Garn, K., McNaughton, E., Clarke, H., et al. (2014). A novel in vivo model of tau propagation with rapid and progressive neurofibrillary tangle pathology: the pattern of spread is determined by connectivity, not proximity. Acta Neuropathol. 127, 667–683. doi: 10.1007/s00401-014-1254-6
Ait-Belgnaoui, A., Durand, H., Cartier, C., Chaumaz, G., Eutamene, H., Ferrier, L., et al. (2012). Prevention of gut leakiness by a probiotic treatment leads to attenuated HPA response to an acute psychological stress in rats. Psychoneuroendocrinology 37, 1885–1895. doi: 10.1016/j.psyneuen.2012.03.024
Akbari, E., Asemi, Z., Kakhaki, R. D., Bahmani, F., Kouchaki, E., Tamtaji, O. R., et al. (2016). Effect of probiotic supplementation on cognitive function and metabolic status in Alzheimer’s disease: a randomized, double-blind and controlled trial. Front. Aging Nuerosci. 8:256. doi: 10.3389/fnagi.2016.00256
Alavi Naini, S. M., and Soussi-Yanicostas, N. (2015). Tau hyperphosphorylation and oxidative stress, a critical vicious circle in neurodegenerative tauopathies? Oxid. Med. Cell. Long. 2015:151979. doi: 10.1155/2015/151979
Alfa, M. J., Strang, D., Tappia, P. S., Graham, M., Van Domselaar, G., Forbes, J. D., et al. (2018). A randomized trial to determine the impact of a digestion resistant starch composition on the gut microbiome in older and mid-age adults. Clin. Nutr. 37, 797–807. doi: 10.1016/j.clnu.2017.03.025
Alfonsetti, M., Castelli, V., and d’Angelo, M. (2022). Are we what we eat? impact of diet on the gut-brain axis in Parkinson’s disease. Nutrients 14:380. doi: 10.3390/nu14020380
Amato, A., Terzo, S., and Mulè, F. (2019). Natural compounds as beneficial antioxidant agents in neurodegenerative disorders: a focus on Alzheimer’s disease. Antioxidants 8:608. doi: 10.3390/antiox8120608
Angelucci, F., Cechova, K., Amlerova, J., and Hort, J. (2019). Antibiotics, gut microbiota, and Alzheimer’s disease. J. Neuroinflamm. 16:108. doi: 10.1186/s12974-019-1494-4
Appleton, J. (2018). The gut-brain axis: influence of microbiota on mood and mental health. Integr. Med. 17, 28–32.
Argaw, A. T., Asp, L., Zhang, J., Navrazhina, K., Pham, T., Mariani, J. N., et al. (2012). Astrocyte-derived VEGF-A drives blood-brain barrier disruption in CNS inflammatory disease. J. Clin. Invest. 122, 2454–2468. doi: 10.1172/jci60842
Armstrong, R. A. (2019). Risk factors for Alzheimer’s disease. Folia Neuropathol. 57, 87–105. doi: 10.5114/fn.2019.85929
Ashley, J., Packard, M., Ataman, B., and Budnik, V. (2005). Fasciclin II signals new synapse formation through amyloid precursor protein and the scaffolding protein dX11/Mint. J. Neurosci. 25, 5943–5955. doi: 10.1523/jneurosci.1144-05.2005
Azad, M. A. K., Sarker, M., Li, T., and Yin, J. (2018). Probiotic species in the modulation of gut microbiota: an overview. BioMed Res. Int. 2018:9478630. doi: 10.1155/2018/9478630
Bailey, M. T., and Cryan, J. F. (2017). The microbiome as a key regulator of brain, behavior and immunity: commentary on the 2017 named series. Brain Behav. Immun. 66, 18–22. doi: 10.1016/j.bbi.2017.08.017
Baker, S., Polanco, J. C., and Götz, J. (2016). Extracellular vesicles containing P301L mutant tau accelerate pathological tau phosphorylation and oligomer formation but do not seed mature neurofibrillary tangles in ALZ17 mice. J. Alzheimer’s Dis. 54, 1207–1217. doi: 10.3233/JAD-160371
Ballarini, T., Melo, van Lent, D., Brunner, J., Schröder, A., Wolfsgruber, S., et al. (2021). Mediterranean diet, Alzheimer disease biomarkers, and brain atrophy in old age. Neurology 96, e2920–e2932. doi: 10.1212/wnl.0000000000012067
Ballatore, C., Lee, V. M. Y., and Trojanowski, J. Q. (2007). Tau-mediated neurodegeneration in Alzheimer’s disease and related disorders. Nat. Rev. Neurosci. 8, 663–672. doi: 10.1038/nrn2194
Batarseh, Y. S., Duong, Q.-V., Mousa, Y. M., Al Rihani, S. B., Elfakhri, K., and Kaddoumi, A. (2016). Amyloid-β and astrocytes interplay in Amyloid-β related disorders. Int. J. Mol. Sci. 17:338. doi: 10.3390/ijms17030338
Bauer, P. V., Hamr, S. C., and Duca, F. A. (2016). Regulation of energy balance by a gut-brain axis and involvement of the gut microbiota. Cell Mol. Life. Sci. 73, 737–755. doi: 10.1007/s00018-015-2083-z
Bäuerl, C., Collado, M. C., Diaz Cuevas, A., Viña, J., and Pérez Martínez, G. (2018). Shifts in gut microbiota composition in an APP/PSS1 transgenic mouse model of Alzheimer’s disease during lifespan. Lett. Appl. Microbiol. 66, 464–471. doi: 10.1111/lam.12882
Belkaid, Y., and Hand Timothy, W. (2014). Role of the microbiota in immunity and inflammation. Cell 157, 121–141. doi: 10.1016/j.cell.2014.03.011
Berding, K., Carbia, C., and Cryan, J. F. (2021). Going with the grain: fiber, cognition, and the microbiota-gut-brain-axis. Exp. Biol. Med. 246, 796–811. doi: 10.1177/1535370221995785
Berliocchi, L., Bano, D., and Nicotera, P. (2005). Ca2+ signals and death programmes in neurons. Philos. Trans. R. Soc. B: Biol. Sci. 360, 2255–2258. doi: 10.1098/rstb.2005.1765
Berti, V., Walters, M., Sterling, J., Quinn, C. G., Logue, M., Andrews, R., et al. (2018). Mediterranean diet and 3-year Alzheimer brain biomarker changes in middle-aged adults. Neurology 90, e1789–e1798. doi: 10.1212/wnl.0000000000005527
Beydoun, M. A., Beydoun, H. A., Gamaldo, A. A., Teel, A., Zonderman, A. B., and Wang, Y. (2014). Epidemiologic studies of modifiable factors associated with cognition and dementia: systematic review and meta-analysis. BMC Public Health 14:643. doi: 10.1186/1471-2458-14-643
Biagi, E., Nylund, L., Candela, M., Ostan, R., Bucci, L., Pini, E., et al. (2010). Through ageing, and beyond: gut microbiota and inflammatory status in seniors and centenarians. PLoS One 5:e10667. doi: 10.1371/journal.pone.0010667
Bindels, L. B., Segura Munoz, R. R., Gomes-Neto, J. C., Mutemberezi, V., Martínez, I., Salazar, N., et al. (2017). Resistant starch can improve insulin sensitivity independently of the gut microbiota. Microbiome 5:12. doi: 10.1186/s40168-017-0230-5
Boehme, M., van de Wouw, M., Bastiaanssen, T. F. S., Olavarría-Ramírez, L., Lyons, K., Fouhy, F., et al. (2020). Mid-life microbiota crises: middle age is associated with pervasive neuroimmune alterations that are reversed by targeting the gut microbiome. Mol. Psychiatry 25, 2567–2583. doi: 10.1038/s41380-019-0425-1
Bonaz, B., Bazin, T., and Pellissier, S. (2018). The vagus nerve at the interface of the microbiota-gut-brain axis. Front. Neurosci. 12:49. doi: 10.3389/fnins.2018.00049
Bostanciklioğlu, M. (2019). The role of gut microbiota in pathogenesis of Alzheimer’s disease. J. Appl. Microbiol. 127, 954–967. doi: 10.1111/jam.14264
Brandscheid, C., Schuck, F., Reinhardt, S., Schäfer, K.-H., Pietrzik, C. U., Grimm, M., et al. (2017). Altered gut microbiome composition and tryptic activity of the 5xFAD Alzheimer’s mouse model. J. Alzheimer’s Dis. 56, 775–788. doi: 10.3233/JAD-160926
Braniste, V., Al-Asmakh, M., Kowal, C., Anuar, F., Abbaspour, A., Tóth, M., et al. (2014). The gut microbiota influences blood-brain barrier permeability in mice. Sci. Transl. Med. 6:263ra158. doi: 10.1126/scitranslmed.3009759
Bredesen, D. E. (2016). Inhalational Alzheimer’s disease: an unrecognized - and treatable - epidemic. Aging 8, 304–313. doi: 10.18632/aging.100896
Breit, S., Kupferberg, A., Rogler, G., and Hasler, G. (2018). Vagus nerve as modulator of the brain-gut axis in psychiatric and inflammatory disorders. Front. Psychiatry 9:44. doi: 10.3389/fpsyt.2018.00044
Brosseron, F., Krauthausen, M., Kummer, M., and Heneka, M. T. (2014). Body fluid cytokine levels in mild cognitive impairment and Alzheimer’s disease: a comparative overview. Mol. Neurobiol. 50, 534–544. doi: 10.1007/s12035-014-8657-1
Bush, T. G., Puvanachandra, N., Horner, C. H., Polito, A., Ostenfeld, T., Svendsen, C. N., et al. (1999). Leukocyte infiltration, neuronal degeneration, and neurite outgrowth after ablation of scar-forming, reactive astrocytes in adult transgenic mice. Neuron 23, 297–308. doi: 10.1016/S0896-6273(00)80781-3
Cabezas, R., Ávila, M., Gonzalez, J., El-Bachá, R. S., Báez, E., García-Segura, L. M., et al. (2014). Astrocytic modulation of blood brain barrier: perspectives on Parkinson’s disease. Front. Cell. Neurosci. 8:211. doi: 10.3389/fncel.2014.00211
Cai, Y., Folkerts, J., Folkerts, G., Maurer, M., and Braber, S. (2020). Microbiota-dependent and-independent effects of dietary fibre on human health. Br. J. Pharmacol. 177, 1363–1381. doi: 10.1111/bph.14871
Canet, G., Hernandez, C., Zussy, C., Chevallier, N., Desrumaux, C., and Givalois, L. (2019). Is AD a stress-related disorder? focus on the HPA axis and its promising therapeutic targets. Front. Aging Neurosci. 11:269. doi: 10.3389/fnagi.2019.00269
Cao, J., Amakye, W. K., Qi, C., Liu, X., Ma, J., and Ren, J. (2021). Bifidobacterium lactis Probio-M8 regulates gut microbiota to alleviate Alzheimer’s disease in the APP/PS1 mouse model. Eur. J. Nutr. 60, 3757–3769. doi: 10.1007/s00394-021-02543-x
Carabotti, M., Scirocco, A., Maselli, M. A., and Severi, C. (2015). The gut-brain axis: interactions between enteric microbiota, central and enteric nervous systems. Ann. Gastroenterol. 28, 203–209.
Carregosa, D., Carecho, R., Figueira, N. I, and Santos, C. (2020). Low-Molecular weight metabolites from polyphenols as effectors for attenuating neuroinflammation. J. Agric. Food Chem. 68, 1790–1807. doi: 10.1021/acs.jafc.9b02155
Castro-Quezada, I., Román-Viñas, B., and Serra-Majem, L. (2014). The mediterranean diet and nutritional adequacy: a review. Nutrients 6, 231–248. doi: 10.3390/nu6010231
Cattaneo, A., Cattane, N., Galluzzi, S., Provasi, S., Lopizzo, N., Festari, C., et al. (2017). Association of brain amyloidosis with pro-inflammatory gut bacterial taxa and peripheral inflammation markers in cognitively impaired elderly. Neurobiol. Aging 49, 60–68. doi: 10.1016/j.neurobiolaging.2016.08.019
Ceppa, F. A., Izzo, L., Sardelli, L., Raimondi, I., Tunesi, M., Albani, D., et al. (2020). Human gut-microbiota interaction in neurodegenerative disorders and current engineered tools for its modeling. Front. Cell. Infect. Microbiol. 10:297. doi: 10.3389/fcimb.2020.00297
Cerullo, G., Negro, M., Parimbelli, M., Pecoraro, M., Perna, S., Liguori, G., et al. (2020). The long history of Vitamin C: from prevention of the common cold to potential aid in the treatment of COVID-19. Front. Immunol. 11:574029. doi: 10.3389/fimmu.2020.574029
Chakrabarti, S., Khemka, V. K., Banerjee, A., Chatterjee, G., Ganguly, A., and Biswas, A. (2015). Metabolic risk factors of sporadic Alzheimer’s disease: implications in the pathology. pathogenesis and treatment. Aging Dis. 6, 282–299. doi: 10.14336/ad.2014.002
Chakraborti, C. K. (2015). New-found link between microbiota and obesity. World J. Gastrointest Pathophysiol. 6, 110–119. doi: 10.4291/wjgp.v6.i4.110
Challis, C., Hori, A., Sampson, T. R., Yoo, B. B., Challis, R. C., Hamilton, A. M., et al. (2020). Gut-seeded α-synuclein fibrils promote gut dysfunction and brain pathology specifically in aged mice. Nat. Neurosci. 23, 327–336. doi: 10.1038/s41593-020-0589-7
Chambers, E. S., Preston, T., Frost, G., and Morrison, D. J. (2018). Role of gut microbiota-generated short-chain fatty acids in metabolic and cardiovascular health. Curr. Nutrition Rep. 7, 198–206. doi: 10.1007/s13668-018-0248-8
Chan, K. L., Pillon, N. J., Sivaloganathan, D. M., Costford, S. R., Liu, Z., Théret, M., et al. (2015). Palmitoleate reverses high fat-induced proinflammatory macrophage polarization via AMP-activated protein kinase (AMPK). J. Biol. Chem. 290, 16979–16988. doi: 10.1074/jbc.M115.646992
Chatterjee, P., Fernando, M., Fernando, B., Dias, C. B., Shah, T., Silva, R., et al. (2020). Potential of coconut oil and medium chain triglycerides in the prevention and treatment of Alzheimer’s disease. Mech. Ageing Dev. 186:111209. doi: 10.1016/j.mad.2020.111209
Chem, Y., Xu, J., and Chen, Y. (2021). Regulation of neurotransmitters by the gut microbiota and effects on cognition in neurological disorders. Nutrients 13:2099. doi: 10.3390/nu13062099
Chen, S. G., Stribinskis, V., Rane, M. J., Demuth, D. R., Gozal, E., Roberts, A. M., et al. (2016). Exposure to the functional bacterial amyloid protein curli enhances alpha-synuclein aggregation in aged fischer 344 rats and Caenorhabditis elegans. Sci. Rep. 6:34477. doi: 10.1038/srep34477
Chenoll, E., Casinos, B., Bataller, E., Astals, P., Echevarría, J., Iglesias, J. R., et al. (2011). Novel probiotic bifidobacterium bifidum CECT 7366 strain active against the pathogenic bacterium Helicobacter pylori. Appl. Environ. Microbiol. 77, 1335–1343. doi: 10.1128/AEM.01820-10
Chriett, S., Dąbek, A., Wojtala, M., Vidal, H., Balcerczyk, A., and Pirola, L. (2019). Prominent action of butyrate over β-hydroxybutyrate as histone deacetylase inhibitor, transcriptional modulator and anti-inflammatory molecule. Sci. Rep. 9:742. doi: 10.1038/s41598-018-36941-9
Christ, A., Lauterbach, M., and Latz, E. (2019). Western diet and the immune system: an inflammatory connection. Immunity 51, 794–811. doi: 10.1016/j.immuni.2019.09.020
Christensen, E. G., Licht, T. R., Kristensen, M., and Bahl, M. I. (2013). Bifidogenic effect of whole-grain wheat during a 12-week energy-restricted dietary intervention in postmenopausal women. Eur. J. Clin. Nutr. 67, 1316–1321. doi: 10.1038/ejcn.2013.207
Clarke, G., Stilling, R. M., Kennedy, P. J., Stanton, C., Cryan, J. F., and Dinan, T. G. (2014). Minireview: gut microbiota: the neglected endocrine organ. Mol. Endocrinol. 28, 1221–1238. doi: 10.1210/me.2014-1108
Coll, T., Eyre, E., Rodríguez-Calvo, R., Palomer, X., Sánchez, R. M., Merlos, M., et al. (2008). Oleate reverses palmitate-induced insulin resistance and inflammation in skeletal muscle cells. J. Biol. Chem. 283, 11107–11116. doi: 10.1074/jbc.M708700200
Constantinescu, C. S., Tani, M., Ransohoff, R. M., Wysocka, M., Hilliard, B., Fujioka, T., et al. (2005). Astrocytes as antigen-presenting cells: expression of IL-12/IL-23. J. Neurochem. 95, 331–340. doi: 10.1111/j.1471-4159.2005.03368.x
Corley, J. (2020). Adherence to the MIND diet is associated with 12-year all-cause mortality in older adults. Public Health Nutrition 25, 358–367. doi: 10.1017/S1368980020002979
Costantini, L., Molinari, R., Farinon, B., and Merendino, N. (2017). Impact of Omega-3 fatty acids on the gut microbiota. Int. J. Mol. Sci. 18:2645. doi: 10.3390/ijms18122645
Cristino, L., Bisogno, T., and Di Marzo, V. (2020). Cannabinoids and the expanded endocannabinoid system in neurological disorders. Nat. Rev. Neurol. 16, 9–29. doi: 10.1038/s41582-019-0284-z
Crosson, F. J., Covinsky, K., and Redberg, R. F. (2021). Medicare and the shocking US food and drug administration approval of aducanumab: crisis or opportunity? JAMA Intern. Med. 181, 1278–1280. doi: 10.1001/jamainternmed.2021.4610
Crump, K. E., Langston, P. K., Rajkarnikar, S., and Grayson, J. M. (2013). Antioxidant treatment regulates the humoral immune response during acute viral infection. J. Virol. 87, 2577–2586. doi: 10.1128/JVI.02714-12
Cryan, J. F., and Dinan, T. G. (2012). Mind-altering microorganisms: the impact of the gut microbiota on brain and behaviour. Nat. Rev. Neurosci. 13, 701–712. doi: 10.1038/nrn3346
Cryan, J. F., O’Riordan, K. J., Cowan, C. S. M., Sandhu, K. V., Bastiaanssen, T. F. S., Boehme, M., et al. (2019). The microbiota-gut-brain axis. Physiol. Rev. 99, 1877–2013. doi: 10.1152/physrev.00018.2018
Dabir, D. V., Robinson, M. B., Swanson, E., Zhang, B., Trojanowski, J. Q., Lee, V. M.-Y., et al. (2006). Impaired glutamate transport in a mouse model of tau pathology in astrocytes. J. Neurosci. 26, 644–654. doi: 10.1523/jneurosci.3861-05.2006
Dalile, B., Van Oudenhove, L., Vervliet, B., and Verbeke, K. (2019). The role of short-chain fatty acids in microbiota-gut-brain communication. Nat. Rev. Gastroenterol. Hepatol. 16, 461–478. doi: 10.1038/s41575-019-0157-3
Daneman, R., and Prat, A. (2015). The blood-brain barrier. Cold Spring Harb. Perspect. Biol. 7:a020412. doi: 10.1101/cshperspect.a020412
de, J. R., De-Paula, V., Forlenza, A. S., and Forlenza, O. V. (2018). Reevance of gutmicrobiota in cognition. beavious and Alzheimer’s disease. Parmacol. Res. 136, 29–34. doi: 10.1016/j.phrs.2018.07.007
de la Rubia Ortí, J. E., García-Pardo, M. P., Drehmer, E., Sancho Cantus, D., Julián Rochina, M., Aguilar, M. A., et al. (2018). Improvement of main cognitive functions in patients with Alzheimer’s disease after treatment with coconut oil enriched mediterranean diet: a pilot study. J. Alzheimer’s Dis. 65, 577–587. doi: 10.3233/JAD-180184
de Lange, E., and Hammarlund-Udenaes, M. (2015). Translational aspects of blood-brain barrier transport and central nervous system effects of drugs: from discovery to patients. Clin. Pharmacol. Therapeutics 97, 380–394. doi: 10.1002/cpt.76
den Besten, G., van Eunen, K., Groen, A. K., Venema, K., Reijngoud, D.-J., and Bakker, B. M. (2013). The role of short-chain fatty acids in the interplay between diet, gut microbiota, and host energy metabolism. J. Lipid Res. 54, 2325–2340. doi: 10.1194/jlr.R036012
Dhana, K., Evans, D. A., Rajan, K. B., Bennett, D. A., and Morris, M. C. (2010). Healthy lifestyle and the risk of alzheimer dementia. Neurology 95, e374–e383. doi: 10.1212/wnl.0000000000009816
d’Hennezel, E., Abubucker, S., Murphy, L. O., Cullen, T. W., and Lozupone, C. (2017). Total lipopolysaccharide from the human gut microbiome silences toll-like receptor signaling. mSystems 2:e00046-17. doi: 10.1128/mSystems.00046-17
Dhingra, D., Michael, M., Rajput, H., and Patil, R. T. (2012). Dietary fibre in foods: a review. J. Food Sci. Technol. 49, 255–266. doi: 10.1007/s13197-011-0365-5
Dua, T., Seeher, K. M., Sivananthan, S., Chowdhary, N., Pot, A. M., and Saxena, S. (2017). World health organization’s global action plan on the public health response to dementia 2017-2025. Alzheimer’s & Dementia 13, 1450–1451. doi: 10.1016/j.jalz.2017.07.758
Duscha, A., Gisevius, B., Hirschberg, S., Yissachar, N., Stangl, G. I., Eilers, E., et al. (2020). Propionic acid shapes the multiple sclerosis disease course by an immunomodulatory mechanism. Cell 180, 1067–80.e16. doi: 10.1016/j.cell.2020.02.035.
Eke, B. C., Jibiri, N. N., Bede, E. N., Anusionwu, B. C., Orji, C. E., and Alisi, C. S. (2017). Effect of ingestion of microwaved foods on serum anti-oxidant enzymes and vitamins of albino rats. J. Radiat. Res. Appl. Sci. 10, 148–151. doi: 10.1016/j.jrras.2017.03.001
Eschweiler, G. W., Leyhe, T., Klöppel, S., and Hüll, M. (2010). New developments in the diagnosis of dementia. Dtsch. Arztebl. Int. 107, 677–683. doi: 10.3238/arztebl.2010.0677
Fagan, A. M., Mintun, M. A., Mach, R. H., Lee, S.-Y., Dence, C. S., Shah, A. R., et al. (2006). Inverse relation between in vivo amyloid imaging load and cerebrospinal fluid Aβ42 in humans. Ann. Neurol. 59, 512–519. doi: 10.1002/ana.20730
Fang, F., Yu, Q., Arancio, O., Chen, D., Gore, S. S., Yan, S. S., et al. (2018). RAGE mediates Aβ accumulation in a mouse model of Alzheimer’s disease via modulation of β- and γ-secretase activity. Hum. Mol. Genet. 27, 1002–1014. doi: 10.1093/hmg/ddy017
Faraco, G., Hochrainer, K., Segarra, S. G., Schaeffer, S., Santisteban, M. M., Menon, A., et al. (2019). Dietary salt promotes cognitive impairment through tau phosphorylation. Nature 574, 686–690. doi: 10.1038/s41586-019-1688-z
Farrer, L. A., Cupples, L. A., Haines, J. L., Hyman, B., Kukull, W. A., Mayeux, R., et al. (1997). Effects of age, sex, and ethnicity on the association between apolipoprotein e genotype and Alzheimer disease: a meta-analysis. JAMA 278, 1349–1356. doi: 10.1001/jama.1997.03550160069041
Farzi, A., Fröhlich, E. E., and Holzer, P. (2018). Gut microbiota and the neuroendocrine system. Neurotherapeutics 15, 5–22. doi: 10.1007/s13311-017-0600-5
Ferreira, L. S. S., Fernandes, C. S., Vieira, M. N. N., and De Felice, F. G. (2018). Insulin resistance in Alzheimer’s disease. Front. Neurosci. 12:830. doi: 10.3389/fnins.2018.00830
Ferrero, J., Williams, L., Stella, H., Leitermann, K., Mikulskis, A., O’Gorman, J., et al. (2016). First-in-human, double-blind, placebo-controlled, single-dose escalation study of aducanumab (BIIB037) in mild-to-moderate Alzheimer’s disease. Alzheimers Dement 2, 169–176. doi: 10.1016/j.trci.2016.06.002
Fiandaca, M. S., Kapogiannis, D., Mapstone, M., Boxer, A., Eitan, E., Schwartz, J. B., et al. (2015). Identification of preclinical Alzheimer’s disease by a profile of pathogenic proteins in neurally derived blood exosomes: a case-control study. Alzheimer’s Dementia 11, 600–607.e1. doi: 10.1016/j.jalz.2014.06.008.
Finucane, O. M., Lyons, C. L., Murphy, A. M., Reynolds, C. M., Klinger, R., Healy, N. P., et al. (2015). Monounsaturated fatty acid-enriched high-fat diets impede adipose NLRP3 inflammasome-mediated IL-1beta secretion and insulin resistance despite obesity. Diab. Metab. Res. Rev. 64, 2116–2128. doi: 10.2337/db14-1098
Flint, H. J., Bayer, E. A., Rincon, M. T., Lamed, R., and White, B. A. (2008). Polysaccharide utilization by gut bacteria: potential for new insights from genomic analysis. Nat. Rev. Microbiol. 6, 121–131. doi: 10.1038/nrmicro1817
Fong, C. W. (2015). Permeability of the blood-brain barrier: molecular mechanism of transport of drugs and physiologically important compounds. J. Membr. Biol. 248, 651–669. doi: 10.1007/s00232-015-9778-9
Forloni, G., Mangiarotti, F., Angeretti, N., Lucca, E., and De Simoni, M. G. (1997). B -Amyloid fragment potentiates IL-6 And TNF-A secretion By LPS in astrocytes but not in microglia. Cytokine 9, 759–762. doi: 10.1006/cyto.1997.0232
Forman, M. S., Lal, D., Zhang, B., Dabir, D., Lee, V. M., and Trojanowski, J. Q. (2004). O1-04-01 Transgenic mouse models of TAU pathology in astrocytes leading to nervous system degeneration. Neurobiol. Aging 25:S16. doi: 10.1016/S0197-4580(04)80052-6
Foster, J. A., Rinaman, L., and Cryan, J. F. (2017). Stress & the gut-brain axis: regulation by the microbiome. Neurobiol. Stress 7, 124–136. doi: 10.1016/j.ynstr.2017.03.001
Franzmeier, N., Neitzel, J., Rubinski, A., Smith, R., Strandberg, O., Ossenkoppele, R., et al. (2020). Functional brain architecture is associated with the rate of tau accumulation in Alzheimer’s disease. Nat. Commun. 11:347. doi: 10.1038/s41467-019-14159-1
Friedland, R. P. (2015). Mechanisms of molecular mimicry involving the microbiota in neurodegeneration. J. Alzheimer’s Dis. 45, 349–362. doi: 10.3233/JAD-142841
Friedman, L. K. (2006). Calcium: a role for Neuroproduction and sustained adaptation. Mol. Interventions 6, 315–329. doi: 10.1124/mi.6.6.5
Frye, B. M., Craft, S., Register, T. C., Andrews, R. N., Appt, S. E., Vitolins, M. Z., et al. (2021). Diet, psychosocial stress, and Alzheimer’s disease-related neuroanatomy in female nonhuman primates. Alzheimers Dement 17, 733–744. doi: 10.1002/alz.12232
Fukuda, S., Toh, H., Hase, K., Oshima, K., Nakanishi, Y., Yoshimura, K., et al. (2011). Bifidobacteria can protect from enteropathogenic infection through production of acetate. Nature 469, 543–547. doi: 10.1038/nature09646
Fukumoto, S., Tatewaki, M., Yamada, T., Fujimiya, M., Mantyh, C., Voss, M., et al. (2003). Short-chain fatty acids stimulate colonic transit via intraluminal 5-HT release in rats. Am. J. Physiol. Regul. Integr. Comp. Physiol. 284, R1269–R1276. doi: 10.1152/ajpregu.00442.2002
Fulop, T., Witkowski, J. M., Bourgade, K., Khalil, A., Zerif, E., Larbi, A., et al. (2018). Can an infection hypothesis explain the beta amyloid hypothesis of Alzheimer’s disease? Front. Aging Neurosci. 10:224. doi: 10.3389/fnagi.2018.00224
Fung, T. C., Olson, C. A., and Hsiao, E. Y. (2017). Interactions between the microbiota, immune and nervous systems in health and disease. Nat. Neurosci. 20, 145–155. doi: 10.1038/nn.4476
Garre-Olmo, J. (2018). [Epidemiology of Alzheimer’s disease and other dementias]. Rev. Neurol. 66, 377–386.
Ghosh, S., DeCoffe, D., Brown, K., Rajendiran, E., Estaki, M., Dai, C., et al. (2013). Fish oil attenuates omega-6 polyunsaturated fatty acid-induced dysbiosis and infectious colitis but impairs LPS dephosphorylation activity causing sepsis. PLoS One 8:e55468. doi: 10.1371/journal.pone.0055468
Ghosh, S., Whitley, C. S., Haribabu, B., and Jala, V. R. (2021). Regulation of intestinal barrier function by microbial metabolites. Cell. Mol. Gastroenterol. Hepatol. 11, 1463–1482. doi: 10.1016/j.jcmgh.2021.02.007
Ghosh, S. S., Wang, J., Yannie, P. J., and Ghosh, S. (2020). Intestinal barrier dysfunction, LPS translocation, and disease development. J. Endocrine Soc. 4:bvz039. doi: 10.1210/jendso/bvz039
Gim, S. A., Sung, J. H., Shah, F. A., Kim, M. O., and Koh, P. O. (2013). Ferulic acid regulates the AKT/GSK-3β/CRMP-2 signaling pathway in a middle cerebral artery occlusion animal model. Lab. Animal Res. 29, 63–69. doi: 10.5625/lar.2013.29.2.63
Golde, T. E. (2009). The therapeutic importance of understanding mechanisms of neuronal cell death in neurodegenerative disease. Mol. Neurodegeneration 4:8. doi: 10.1186/1750-1326-4-8
González-Reyes, R. E., Nava-Mesa, M. O., Vargas-Sánchez, K., Ariza-Salamanca, D., and Mora-Muñoz, L. (2017). Involvement of astrocytes in Alzheimer’s disease from a neuroinflammatory and oxidative stress perspective. Front. Mol. Neurosci. 10:427. doi: 10.3389/fnmol.2017.00427
Green, R. C., Cupples, L. A., Go, R., Benke, K. S., Edeki, T., Griffith, P. A., et al. (2002). Risk of dementia among white and african american relatives of patients with Alzheimer disease. JAMA 287, 329–336. doi: 10.1001/jama.287.3.329
Grompone, G., Martorell, P., Llopis, S., González, N., Genovés, S., Mulet, A. P., et al. (2012). Anti-inflammatory Lactobacillus rhamnosus CNCM I-3690 strain protects against oxidative stress and increases lifespan in Caenorhabditis elegans. PLoS One 7:e52493. doi: 10.1371/journal.pone.0052493
Guo, P., Zhang, K., Ma, X., and He, P. (2020). Clostridium species as probiotics: potentials and challenges. J. Animal Sci. Biotechnol. 11:24. doi: 10.1186/s40104-019-0402-1
Guo, S., Al-Sadi, R., Said, H. M., and Ma, T. Y. (2013). Lipopolysaccharide causes an increase in intestinal tight junction permeability in vitro and in vivo by inducing enterocyte membrane expression and localization of TLR-4 and CD14. Am. J. Pathol. 182, 375–387. doi: 10.1016/j.ajpath.2012.10.014
Guo, S., Nighot, M., Al-Sadi, R., Alhmoud, T., Nighot, P., and Ma, T. Y. (2015). Lipopolysaccharide regulation of intestinal tight junction permeability is mediated by TLR4 signal transduction pathway activation of FAK and MyD88. J. Immunol. 195, 4999–5010. doi: 10.4049/jimmunol.1402598
Gush, L., Shah, S., and Gilani, F. (2021). Chapter 23 - Macronutrients and Micronutrients. A Prescription for Healthy Living. Cambridge, MA: Academic Press.
Guttenplan, K. A., Weigel, M. K., Adler, D. I., Couthouis, J., Liddelow, S. A., Gitler, A. D., et al. (2020). Knockout of reactive astrocyte activating factors slows disease progression in an ALS mouse model. Nat. Commun. 11:3753. doi: 10.1038/s41467-020-17514-9
Halverson, T., and Alagiakrishnan, K. (2020). Gut microbes in neurocognitive and mental health disorders. Ann. Med. 52, 423–443. doi: 10.1080/07853890.2020.1808239
Hammad, S., Pu, S., and Jones, P. J. (2016). Current evidence supporting the link between dietary fatty acids and Cardiovascular disease. Lipids 51, 507–517. doi: 10.1007/s11745-015-4113-x
Harach, T., Marungruang, N., Duthilleul, N., Cheatham, V., Mc Coy, K. D., Frisoni, G., et al. (2017). Reduction of abeta amyloid pathology in APPPS1 transgenic mice in the absence of gut microbiota. Sci. Rep. 7:41802. doi: 10.1038/srep41802
Hasegawa, T., Yoneda, M., Nakamura, K., Makino, I., and Terano, A. (2001). Plasma transforming growth factor-beta1 level and efficacy of alpha-tocopherol in patients with non-alcoholic steatohepatitis: a pilot study. Aliment. Pharmacol. Ther. 15, 1667–1672. doi: 10.1046/j.1365-2036.2001.01083.x
Haseloff, R. F., Blasig, I. E., Bauer, H. C., and Bauer, H. (2005). In search of the astrocytic factor(s) modulating blood-brain barrier functions in brain capillary endothelial cells in vitro. Cell. Mol. Neurobiol. 25, 25–39. doi: 10.1007/s10571-004-1375-x
He, Y., Li, B., Sun, D., and Chen, S. (2020). Gut microbiota: implications in Alzheimer’s disease. J. Clin. Med. 9:2042. doi: 10.3390/jcm9072042
Hebert, L. E., Weuve, J., Scherr, P. A., and Evans, D. A. (2013). Alzheimer disease in the United States (2010-2050) estimated using the 2010 census. Neurology 80, 1778–1783. doi: 10.1212/WNL.0b013e31828726f5
Heintz-Buschart, A., and Wilmes, P. (2018). Human gut microbiome: function matters. Trends Microbiol. 26, 563–574. doi: 10.1016/j.tim.2017.11.002
Heller, K. J. (2001). Probiotic bacteria in fermented foods: product characteristics and starter organisms. Am. J. Clin. Nutr. 73, 374s–379s. doi: 10.1093/ajcn/73.2.374s
Herms, J., Anliker, B., Heber, S., Ring, S., Fuhrmann, M., Kretzschmar, H., et al. (2004). Cortical dysplasia resembling human type 2 lissencephaly in mice lacking all three APP family members. EMBO J. 23, 4106–4115. doi: 10.1038/sj.emboj.7600390
Hershey, L. A., and Tarawneh, R. (2021). Clinical efficacy, drug safety, and surrogate endpoints: has aducanumab met all of its expectations? Neurology 97, 517–518. doi: 10.1212/WNL.0000000000012453
Hidalgo, M., Prieto, I., Abriouel, H., Villarejo, A. B., Ramírez-Sánchez, M., Cobo, A., et al. (2018). Changes in gut microbiota linked to a reduction in systolic blood pressure in spontaneously hypertensive rats fed an extra virgin olive oil-enriched diet. Plant Foods Hum. Nutr. 73, 1–6. doi: 10.1007/s11130-017-0650-1
Hill, C., Guarner, F., Reid, G., Gibson, G. R., Merenstein, D. J., Pot, B., et al. (2014). The international scientific association for probiotics and prebiotics consensus statement on the scope and appropriate use of the term probiotic. Nat. Rev. Gastroenterol. Hepatol. 11, 506–514. doi: 10.1038/nrgastro.2014.66
Hill, E., Goodwill, A. M., Gorelik, A., and Szoeke, C. (2019). Diet and biomarkers of Alzheimer’s disease: a systematic review and meta-analysis. Neurobiol. Aging 76, 45–52. doi: 10.1016/j.neurobiolaging.2018.12.008
Hill, J. M., Clement, C., Pogue, A. I., Bhattacharjee, S., Zhao, Y., and Lukiw, W. J. (2014). Pathogenic microbes, the microbiome, and Alzheimer’s disease (AD). Front. Aging Neurosci. 6:127. doi: 10.3389/fnagi.2014.00127
Hill, J. M., and Lukiw, W. J. (2015). Microbial-generated amyloids and Alzheimer’s disease (AD). Front. Aging Neurosci. 7:9. doi: 10.3389/fnagi.2015.00009
Himmelstein, D. S., Ward, S. M., Lancia, J. K., Patterson, K. R., and Binder, L. I. (2012). Tau as a therapeutic target in neurodegenerative disease. Pharmacol. Therapeutics 136, 8–22. doi: 10.1016/j.pharmthera.2012.07.001
Hoenig, M. C., Bischof, G. N., Seemiller, J., Hammes, J., Kukolja, J., and Onur, et al. (2018). Networks of tau distribution in Alzheimer’s disease. Brain 141, 568–581. doi: 10.1093/brain/awx353
Hollander, D., and Kaunitz, J. D. (2020). The “Leaky Gut”: tight junctions but loose associations? Digestive Dis. Sci. 65, 1277–1287. doi: 10.1007/s10620-019-05777-2
Holmes, B. B., DeVos, S. L., Kfoury, N., Li, M., Jacks, R., Yanamandra, K., et al. (2013). Heparan sulfate proteoglycans mediate internalization and propagation of specific proteopathic seeds. Proc. Natl. Acad. Sci. U S A. 110, E3138–E3147. doi: 10.1073/pnas.1301440110
Holmqvist, S., Chutna, O., Bousset, L., Aldrin-Kirk, P., Li, W., Björklund, T., et al. (2014). Direct evidence of Parkinson pathology spread from the gastrointestinal tract to the brain in rats. Acta Neuropathol. 128, 805–820. doi: 10.1007/s00401-014-1343-6
Hong, S., Beja-Glasser, V. F., Nfonoyim, B. M., Frouin, A., Li, S., Ramakrishnan, S., et al. (2016). Complement and microglia mediate early synapse loss in Alzheimer mouse models. Science 352, 712–716. doi: 10.1126/science.aad8373
Hooper, L. V., and Macpherson, A. J. (2010). Immune adaptations that maintain homeostasis with the intestinal microbiota. Nat. Rev. Immunol. 10, 159–169. doi: 10.1038/nri2710
Hosking, D. E., Eramudugolla, R., Cherbuin, N., and Anstey, K. J. (2019). MIND not Mediterranean diet related to 12-year incidence of cognitive impairment in an Australian longitudinal cohort study. Alzheimer’s Dementia 15, 581–589. doi: 10.1016/j.jalz.2018.12.011
Howard, R., and Liu, K. Y. (2020). Questions EMERGE as biogen claims aducanumab turnaround. Nat. Rev. Neurol. 16, 63–64. doi: 10.1038/s41582-019-0295-9
Huang, Y., Shi, X., Li, Z., Shen, Y., Shi, X., Wang, L., et al. (2018). Possible association of Firmicutes in the gut microbiota of patients with major depressive disorder. Neuropsychiatric Dis. Treatment 14, 3329–3337. doi: 10.2147/NDT.S188340
Hug, H., Mohajeri, M. H., and La Fata, G. (2018). Toll-Like receptors: regulators of the immune response in the human gut. Nutrients 10:203. doi: 10.3390/nu10020203
Husna Ibrahim, N., Yahaya, M. F., Mohamed, W., Teoh, S. L., Hui, C. K., and Kumar, J. (2020). Pharmacotherapy of Alzheimer’s disease: seeking clarity in a time of uncertainty. Front. Pharmacol. 11:261. doi: 10.3389/fphar.2020.00261
Huszczynski, S. M., Lam, J. S., and Khursigara, C. M. (2020). The role of Pseudomonas aeruginosa lipopolysaccharide in bacterial pathogenesis and physiology. Pathogens 9:6. doi: 10.3390/pathogens9010006
Hwang, D. H., Kim, J. A., and Lee, J. Y. (2016). Mechanisms for the activation of Toll-like receptor 2/4 by saturated fatty acids and inhibition by docosahexaenoic acid. Eur. J. Pharmacol. 785, 24–35. doi: 10.1016/j.ejphar.2016.04.024
Hwang, S. L., and Yen, G. C. (2008). Neuroprotective effects of the citrus flavanones against H2O2-induced cytotoxicity in PC12 cells. J. Agric. Food Chem. 56, 859–864. doi: 10.1021/jf072826r
Islam, S. M. S., Ryu, H.-M., Sayeed, H. M., Byun, H.-O., Jung, J.-Y., Kim, H.-A., et al. (2021). Eubacterium rectale attenuates HSV-1 induced systemic inflammation in mice by inhibiting CD83. Front. Immunol. 12:712312. doi: 10.3389/fimmu.2021.712312
Ismail, R., Parbo, P., Madsen, L. S., Hansen, A. K., Hansen, K. V., Schaldemose, J. L., et al. (2020). The relationships between neuroinflammation, beta-amyloid and tau deposition in Alzheimer’s disease: a longitudinal PET study. J. Neuroinflamm. 17:151. doi: 10.1186/s12974-020-01820-6
Ivanova, N., Liu, Q., Agca, C., Agca, Y., Noble, E. G., Whitehead, S. N., et al. (2020). White matter inflammation and cognitive function in a co-morbid metabolic syndrome and prodromal Alzheimer’s disease rat model. J. Neuroinflamm. 17:29. doi: 10.1186/s12974-020-1698-7
Jack, C. R., Lowe, V. J., Weigand, S. D., Wiste, H. J., Senjem, M. L., Knopman, D. S., et al. (2009). Serial PIB and MRI in normal, mild cognitive impairment and Alzheimer’s disease: implications for sequence of pathological events in Alzheimer’s disease. Brain 132, 1355–1365. doi: 10.1093/brain/awp062
Jack, C. R., Wiste, H. J., Schwarz, C. G., Lowe, V. J., Senjem, M. L., Vemuri, P., et al. (2018). Longitudinal tau PET in ageing and Alzheimer’s disease. Brain 141, 1517–1528. doi: 10.1093/brain/awy059
Jergens, A. E., Parvinroo, S., Kopper, J., and Wannemuehler, M. J. (2021). Rules of engagement: epithelial-microbe interactions and inflammatory bowel disease. Front. Med. 8:669913. doi: 10.3389/fmed.2021.669913
Jiang, S., and Bhaskar, K. (2020). Degradation and transmission of tau by autophagic-endolysosomal networks and potential therapeutic targets for tauopathy. Front. Mol. Neurosci. 13:586731. doi: 10.3389/fnmol.2020.586731
Jithesh, M., Anagha, P., Vinod, R., and Aparna, P. M. (2020). Dementia associated with seizure disorder - a case report. J. Ayurveda Int. Med. Sci. 5, 282–287.
Jo, S., Yarishkin, O., Hwang, Y. J., Chun, Y. E., Park, M., Woo, D. H., et al. (2014). GABA from reactive astrocytes impairs memory in mouse models of Alzheimer’s disease. Nat. Med. 20, 886–896. doi: 10.1038/nm.3639
Johansson, M., Stomrud, E., Insel, P. S., Leuzy, A., Johansson, P. M., Smith, R., et al. (2021). Mild behavioral impairment and its relation to tau pathology in preclinical Alzheimer’s disease. Trans. Psychiatry 11:76. doi: 10.1038/s41398-021-01206-z
Johnson, E. L., Heaver, S. L., Walters, W. A., and Ley, R. E. (2017). Microbiome and metabolic disease: revisiting the bacterial phylum Bacteroidetes. J. Mol. Med. 95, 1–8. doi: 10.1007/s00109-016-1492-2
Jones, S. V., and Kounatidis, I. (2017). Nuclear factor-kappa B and Alzheimer disease, unifying genetic and environmental risk factors from cell to humans. Front. Immunol. 8:1805. doi: 10.3389/fimmu.2017.01805
Jucker, M., and Walker, L. C. (2013). Self-propagation of pathogenic protein aggregates in neurodegenerative diseases. Nature 501, 45–51. doi: 10.1038/nature12481
Jutten, R. J., Dicks, E., Vermaat, L., Barkhof, F., Scheltens, P., Tijms, B. M., et al. (2019). Impairment in complex activities of daily living is related to neurodegeneration in Alzheimer’s disease-specific regions. Neurobiol. Aging 75, 109–116. doi: 10.1016/j.neurobiolaging.2018.11.018
Kahn, M. S., Kranjac, D., Alonzo, C. A., Haase, J. H., Cedillos, R. O., McLinden, K. A., et al. (2012). Prolonged elevation in hippocampal Aβ and cognitive deficits following repeated endotoxin exposure in the mouse. Behav. Brain Res. 229, 176–184. doi: 10.1016/j.bbr.2012.01.010
Kaliannan, K., Wang, B., Li, X. Y., Kim, K. J., and Kang, J. X. (2015). A host-microbiome interaction mediates the opposing effects of omega-6 and omega-3 fatty acids on metabolic endotoxemia. Sci. Rep. 5:11276. doi: 10.1038/srep11276
Karstens, A. J., Tussing-Humphreys, L., Zhan, L., Rajendran, N., Cohen, J., Dion, C., et al. (2019). Associations of the Mediterranean diet with cognitive and neuroimaging phenotypes of dementia in healthy older adults. Am. J. Clin. Nutr. 109, 361–368. doi: 10.1093/ajcn/nqy275
Kato-Kataoka, A., Nishida, K., Takada, M., Suda, K., Kawai, M., Shimizu, K., et al. (2016). Fermented milk containing Lactobacillus casei strain Shirota prevents the onset of physical symptoms in medical students under academic examination stress. Benef Microbes 7, 153–156. doi: 10.3920/BM2015.0100
Kaur, H., Bose, C., and Mande, S. S. (2019). Tryptophan metabolism by gut microbiome and gut-brain-axis: an in silico analysis. Front. Neurosci. 13:1365. doi: 10.3389/fnins.2019.01365
Kawahara, T. L. A., Michishita, E., Adler, A. S., Damian, M., Berber, E., Lin, M., et al. (2009). SIRT6 links histone H3 lysine 9 deacetylation to NF-κB-dependent gene expression and organismal life span. Cell 136, 62–74. doi: 10.1016/j.cell.2008.10.052
Kelly, S. M., Munoz-Munoz, J., and van Sinderen, D. (2021). Plant glycan metabolism by bifidobacteria. Front. Microbiol. 12:609418. doi: 10.3389/fmicb.2021.609418
Keren-Shaul, H., Spinrad, A., Weiner, A., Matcovitch-Natan, O., Dvir-Szternfeld, R., Ulland, T. K., et al. (2017). A unique microglia type associated with restricting development of Alzheimer’s disease. Cell 169, 1276–1290.e17. doi: 10.1016/j.cell.2017.05.018.
Kerr, M. C., and Teasdale, R. D. (2009). Defining macropinocytosis. Traffic 10, 364–371. doi: 10.1111/j.1600-0854.2009.00878.x
Khanna, S., and Tosh, P. K. (2014). A clinician’s primer on the role of the microbiome in human health and disease. Mayo Clin. Proc. 89, 107–114. doi: 10.1016/j.mayocp.2013.10.011
Kho, Z. Y., and Lal, S. K. (2018). The human gut microbiome - a potential controller of wellness and disease. Front. Microbiol. 9:1835. doi: 10.3389/fmicb.2018.01835
Killin, L. O. J., Starr, J. M., Shiue, I. J., and Russ, T. C. (2016). Environmental risk factors for dementia: a systematic review. BMC Geriatrics 16:175. doi: 10.1186/s12877-016-0342-y
Kim, D. H., Grodstein, F., Rosner, B., Kang, J. H., Cook, N. R., Manson, J. E., et al. (2013). Seafood types and age-related cognitive decline in the women’s health study. J. Gerontol. Series A 68, 1255–1262. doi: 10.1093/gerona/glt037
Kim, J. H., Na, H. J., Kim, C. K., Kim, J. Y., Ha, K. S., Lee, H., et al. (2008). The non-provitamin A carotenoid, lutein, inhibits NF-kappaB-dependent gene expression through redox-based regulation of the phosphatidylinositol 3-kinase/PTEN/Akt and NF-kappaB-inducing kinase pathways: role of H(2)O(2) in NF-kappaB activation. Free Radic Biol. Med. 45, 885–896. doi: 10.1016/j.freeradbiomed.2008.06.019
Kim, S., Kwon, S.-H., Kam, T.-I., Panicker, N., Karuppagounder, S. S., Lee, S., et al. (2019). Transneuronal propagation of pathologic α-synuclein from the gut to the brain models Parkinson’s disease. Neuron 103, 627–641.e7. doi: 10.1016/j.neuron.2019.05.035.
Knopman, D. S., Jones, D. T., and Greicius, M. D. (2021). Failure to demonstrate efficacy of aducanumab: an analysis of the EMERGE and ENGAGE trials as reported by Biogen, December 2019. Alzheimers Dement 17, 696–701. doi: 10.1002/alz.12213
Kobayashi, Y., Sugahara, H., Shimada, K., Mitsuyama, E., Kuhara, T., Yasuoka, A., et al. (2017). Therapeutic potential of Bifidobacterium breve strain A1 for preventing cognitive impairment in Alzheimer’s disease. Sci. Rep. 7:13510. doi: 10.1038/s41598-017-13368-2
Kodis, E. J., Choi, S., Swanson, E., Ferreira, G., and Bloom, G. S. (2018). N-methyl-D-aspartate receptor-mediated calcium influx connects amyloid-β oligomers to ectopic neuronal cell cycle reentry in Alzheimer’s disease. Alzheimer’s Dementia 14, 1302–1312. doi: 10.1016/j.jalz.2018.05.017
Koffie, R. M., Meyer-Luehmann, M., Hashimoto, T., Adams, K. W., Mielke, M. L., Garcia-Alloza, M., et al. (2009). Oligomeric amyloid β associates with postsynaptic densities and correlates with excitatory synapse loss near senile plaques. Proc. Natl. Acad. Sci. U S A. 106, 4012–4017. doi: 10.1073/pnas.0811698106
Koh, A., De Vadder, F., Kovatcheva-Datchary, P., and Bäckhed, F. (2016). From dietary fiber to host physiology: short-chain fatty acids as key bacterial metabolites. Cell 165, 1332–1345. doi: 10.1016/j.cell.2016.05.041
Kolarova, M., García-Sierra, F., Bartos, A., Ricny, J., and Ripova, D. (2012). Structure and pathology of Tau Protein in Alzheimer disease. Int. J. Alzheimer’s Dis. 2012:731526. doi: 10.1155/2012/731526
Kothari, V., Luo, Y., Tornabene, T., O’Neill, A. M., Greene, M. W., Geetha, T., et al. (2017). High fat diet induces brain insulin resistance and cognitive impairment in mice. Biochimica Biophys. Acta (BBA) - Mol. Basis Dis. 1863, 499–508. doi: 10.1016/j.bbadis.2016.10.006
Kowalski, K., and Mulak, A. (2019). Brain-Gut-Microbiota axis in Alzheimer’s disease. J. Neurogastroenterol. Motil. 25, 48–60. doi: 10.5056/jnm18087
Kwon, H. S., and Koh, S.-H. (2020). Neuroinflammation in neurodegenerative disorders: the roles of microglia and astrocytes. Trans. Neurodegeneration 9:42. doi: 10.1186/s40035-020-00221-2
Lacassagne, O., and Kessler, J. P. (2000). Cellular and subcellular distribution of the amino-3-hydroxy-5-methyl-4-isoxazole propionate receptor subunit GluR2 in the rat dorsal vagal complex. Neuroscience 99, 557–563. doi: 10.1016/s0306-4522(00)00204-9
Lang, J. M., Pan, C., Cantor, R. M., Wilson Tang, W. H., Garcia-Garcia, J. C., Kurtz, I., et al. (2018). Impact of individual traits, saturated fat, and protein source on the gut microbiome. mBio 9:e01604-18. doi: 10.1128/mBio.01604-18
LeBlanc, J. G., Chain, F., Martín, R., Bermúdez-Humarán, L. G., Courau, S., and Langella, P. (2017). Beneficial effects on host energy metabolism of short-chain fatty acids and vitamins produced by commensal and probiotic bacteria. Microbial Cell Factories 16:79. doi: 10.1186/s12934-017-0691-z
Leblhuber, F., Steiner, K., Schuetz, B., Fuchs, D., and Gostner, M. J. (2018). Probiotic supplementation in patients with Alzheimer’s dementia - an explorative intervention study. Curr. Alzheimer Res. 15, 1106–1113. doi: 10.2174/1389200219666180813144834
Lee, A. H., Shin, H. Y., Park, J. H., Koo, S. Y., Kim, S. M., and Yang, S. H. (2021). Fucoxanthin from microalgae Phaeodactylum tricornutum inhibits pro-inflammatory cytokines by regulating both NF-κB and NLRP3 inflammasome activation. Sci. Rep. 11:543. doi: 10.1038/s41598-020-80748-6
Lee, E.-G., Tulloch, J., Chen, S., Leong, L., Saxton, A. D., Kraemer, B., et al. (2020). Redefining transcriptional regulation of the APOE gene and its association with Alzheimer’s disease. PLoS One 15:e0227667. doi: 10.1371/journal.pone.0227667
Lee, H., and Ko, G. (2016). Antiviral effect of vitamin A on norovirus infection via modulation of the gut microbiome. Sci. Rep. 6:25835. doi: 10.1038/srep25835
Lee, J. C., Kim, S. J., Hong, S., and Kim, Y. (2019). Diagnosis of Alzheimer’s disease utilizing amyloid and tau as fluid biomarkers. Exp. Mol. Med. 51, 1–10. doi: 10.1038/s12276-019-0250-2
Lian, H., Yang, L., Cole, A., Sun, L., Chiang Angie, C. A., Fowler Stephanie, W., et al. (2015). NFκB-Activated astroglial release of complement C3 compromises neuronal morphology and function associated with Alzheimer’s disease. Neuron 85, 101–115. doi: 10.1016/j.neuron.2014.11.018
Liao, Y.-F., Wang, B.-J., Cheng, H.-T., Kuo, L.-H., and Wolfe, M. S. (2004). Tumor necrosis factor-alpha, interleukin-1beta, and interferon-gamma stimulate gamma-secretase-mediated cleavage of amyloid precursor protein through a JNK-dependent MAPK pathway. J. Biol. Chem. 279, 49523–49532. doi: 10.1074/jbc.M402034200
Liddelow, S. A., Guttenplan, K. A., Clarke, L. E., Bennett, F. C., Bohlen, C. J., Schirmer, L., et al. (2017). Neurotoxic reactive astrocytes are induced by activated microglia. Nature 541, 481–487. doi: 10.1038/nature21029
Lill, C. M., Rengmark, A., Pihlstrøm, L., Fogh, I., Shatunov, A., Sleiman, P. M., et al. (2015). The role of TREM2 R47H as a risk factor for Alzheimer’s disease, frontotemporal lobar degeneration, amyotrophic lateral sclerosis, and Parkinson’s disease. Alzheimer’s Dementia 11, 1407–1416. doi: 10.1016/j.jalz.2014.12.009
Lin, T.-L., Shu, C.-C., Chen, Y.-M., Lu, J.-J., Wu, T.-S., Lai, W.-F., et al. (2020). Like cures like: pharmacological activity of anti-inflammatory lipopolysaccharides from gut microbiome. Front. Pharmacol. 11:554. doi: 10.3389/fphar.2020.00554
Liu, K. Y., Nakatsu, C. H., Jones-Hall, Y., Kozik, A., and Jiang, Q. (2021). Vitamin E alpha- and gamma-tocopherol mitigate colitis, protect intestinal barrier function and modulate the gut microbiota in mice. Free Radic Biol. Med. 163, 180–189. doi: 10.1016/j.freeradbiomed.2020.12.017
Liu, T., Hougen, H., Vollmer, A. C., and Hiebert, S. M. (2012). Gut bacteria profiles of Mus musculus at the phylum and family levels are influenced by saturation of dietary fatty acids. Anaerobe 18, 331–337. doi: 10.1016/j.anaerobe.2012.02.004
Lovren, F., Pan, Y., Quan, A., Singh, K. K., Shukla, P. C., Gupta, M., et al. (2010). Adiponectin primes human monocytes into alternative anti-inflammatory M2 macrophages. Am. J. Physiol. Heart Circ. Physiol. 299, H656–H663. doi: 10.1152/ajpheart.00115.2010
Luqman, A., Nega, M., Nguyen, M. T., Ebner, P., and Götz, F. (2018). SadA-Expressing Staphylococci in the human gut show increased cell adherence and internalization. Cell Rep. 22, 535–545. doi: 10.1016/j.celrep.2017.12.058
Lykhmus, O., Mishra, N., Koval, L., Kalashnyk, O., Gergalova, G., Uspenska, K., et al. (2016). Molecular mechanisms regulating LPS-induced inflammation in the brain. Front. Mol. Neurosci. 9:19. doi: 10.3389/fnmol.2016.00019
Ma, T., Jin, H., Kwok, L.-Y., Sun, Z., Liong, M.-T., and Zhang, H. (2020). Probiotic consumption relieved human stress and anxiety symptoms via modulating the gut microbiota and neuroactive potential. bioRxiv [preprint] doi: 10.1101/2020.08.05.237776
Machate, D. J., Figueiredo, P. S., Marcelino, G., Guimarães, RdCA, Hiane, P. A., et al. (2020). Fatty acid diets: regulation of gut microbiota composition and obesity and its related metabolic dysbiosis. Int. J. Mol. Sci. 21:4093. doi: 10.3390/ijms21114093
Magne, F., Gotteland, M., Gauthier, L., Zazueta, A., Pesoa, S., Navarrete, P., et al. (2020). The firmicutes/bacteroidetes ratio: a relevant marker of gut dysbiosis in obese patients? Nutrients 12:1474. doi: 10.3390/nu12051474
Mahmoud, S., Gharagozloo, M., Simard, C., and Gris, D. (2019). Astrocytes maintain glutamate homeostasis in the CNS by controlling the balance between glutamate uptake and release. Cells 8:184. doi: 10.3390/cells8020184
Maltz, R. M., Keirsey, J., Kim, S. C., Mackos, A. R., Gharaibeh, R. Z., Moore, C. C., et al. (2018). Prolonged restraint stressor exposure in outbred CD-1 mice impacts microbiota, colonic inflammation, and short chain fatty acids. PLoS One 13:e0196961. doi: 10.1371/journal.pone.0196961
Mancuso, R., Baglio, F., Cabinio, M., Calabrese, E., Hernis, A., Nemni, R., et al. (2014). Titers of herpes simplex virus type 1 antibodies positively correlate with grey matter volumes in Alzheimer’s disease. J. Alzheimer’s Dis. 38, 741–745. doi: 10.3233/JAD-130977
Mandal, P. K., Kansara, K., and Dabas, A. (2017). The GABA-Working memory relationship in Alzheimer’s disease. J. Alzheimer’s Dis. Rep. 1, 43–45. doi: 10.3233/ADR-170003
Mandal, S., Godfrey, K. M., McDonald, D., Treuren, W. V., Bjørnholt, J. V., Midtvedt, T., et al. (2016). Fat and vitamin intakes during pregnancy have stronger relations with a pro-inflammatory maternal microbiota than does carbohydrate intake. Microbiome 4:55. doi: 10.1186/s40168-016-0200-3
Mann, E. R., and Li, X. (2014). Intestinal antigen-presenting cells in mucosal immune homeostasis: crosstalk between dendritic cells, macrophages and B-cells. World J. Gastroenterol. 20, 9653–9664. doi: 10.3748/wjg.v20.i29.9653
Marco, M. L., Sanders, M. E., Gänzle, M., Arrieta, M. C., Cotter, P. D., De Vuyst, L., et al. (2021). The International Scientific Association for Probiotics and Prebiotics (ISAPP) consensus statement on fermented foods. Nat. Rev. Gastroenterol. Hepatol. 18, 196–208. doi: 10.1038/s41575-020-00390-5
Markesbery, W. R., Schmitt, F. A., Kryscio, R. J., Davis, D. G., Smith, C. D., and Wekstein, D. R. (2006). Neuropathologic substrate of mild cognitive impairment. Arch. Neurol. 63, 38–46. doi: 10.1001/archneur.63.1.38
Martínez, I., Kim, J., Duffy, P. R., Schlegel, V. L., and Walter, J. (2010). Resistant starches types 2 and 4 have differential effects on the composition of the fecal microbiota in human subjects. PLoS One 5:e15046. doi: 10.1371/journal.pone.0015046
Martínez-González, M. A., Gea, A., and Ruiz-Canela, M. (2019). The mediterranean diet and cardiovascular health. Circ. Res. 124, 779–798. doi: 10.1161/CIRCRESAHA.118.313348
Martinez-Lapiscina, E. H., Clavero, P., Toledo, E., San Julian, B., Sanchez-Tainta, A., Corella, D., et al. (2013). Virgin olive oil supplementation and long-term cognition: the predimed-navarra randomized, trial. J. Nutrition Health Aging 17, 544–552. doi: 10.1007/s12603-013-0027-6
Martini-Stoica, H., Cole, A. L., Swartzlander, D. B., Chen, F., Wan, Y.-W., Bajaj, L., et al. (2018). TFEB enhances astroglial uptake of extracellular tau species and reduces tau spreading. J. Exp. Med. 215, 2355–2377. doi: 10.1084/jem.20172158
Marx, W., Scholey, A., Firth, J., D’Cunha, N. M., Lane, M., Hockey, M., et al. (2020). Prebiotics, probiotics, fermented foods and cognitive outcomes: a meta-analysis of randomized controlled trials. Neurosci. Biobehav. Rev. 118, 472–484. doi: 10.1016/j.neubiorev.2020.07.036
Mattsson-Carlgren, N., Andersson, E., Janelidze, S., Ossenkoppele, R., Insel, P., Strandberg, O., et al. (2020). Aβ deposition is associated with increases in soluble and phosphorylated tau that precede a positive Tau PET in Alzheimer’s disease. Sci. Adv. 6:eaaz2387. doi: 10.1126/sciadv.aaz2387
Mazloom, K., Siddiqi, I., and Covasa, M. (2019). Probiotics: how effective are they in the fight against obesity? Nutrients 11:258. doi: 10.3390/nu11020258
McCleery, J., and Quinn, T. J. (2021). Aducanumab and the certainty of evidence. Age Ageing 50, 1899–1900. doi: 10.1093/ageing/afab167
Meda, L., Cassatella, M. A., Szendrei, G. I., Otvos, L., Baron, P., Villalba, M., et al. (1995). Activation of microglial cells by β-amyloid protein and interferon-γ. Nature 374, 647–650. doi: 10.1038/374647a0
Mehrabadi, S., and Sadr, S. S. (2020). Assessment of probiotics mixture on memory function, inflammation markers, and oxidative stress in an alzheimer’s disease model of rats. Iranian Biomed. J. 24, 220–228. doi: 10.29252/ibj.24.4.220
Michielsen, C. C. J. R., Hangelbroek, R. W. J., Feskens, E. J. M., and Afman, L. A. (2019). Disentangling the effects of monounsaturated fatty acids from other components of a mediterranean diet on serum metabolite profiles: a randomized fully controlled dietary intervention in healthy subjects at risk of the metabolic syndrome. Mol. Nutr. Food Res. 63:e1801095. doi: 10.1002/mnfr.201801095
Mika, A., Gaffney, M., Roller, R., Hills, A., Bouchet, C. A., Hulen, K. A., et al. (2018). Feeding the developing brain: juvenile rats fed diet rich in prebiotics and bioactive milk fractions exhibit reduced anxiety-related behavior and modified gene expression in emotion circuits. Neurosci. Lett. 677, 103–109. doi: 10.1016/j.neulet.2018.01.052
Miller, A. L., Bessho, S., Grando, K., and Tükel, Ç (2021). Microbiome or infections: amyloid-containing biofilms as a trigger for complex human diseases. Front. Immunol. 12:638867. doi: 10.3389/fimmu.2021.638867
Miller, C. B., Benny, P., Riel, J., Boushey, C., Perez, R., Khadka, V., et al. (2021). Adherence to Mediterranean diet impacts gastrointestinal microbial diversity throughout pregnancy. BMC Pregnancy Childbirth 21:558. doi: 10.1186/s12884-021-04033-8
Miraglia, F., and Colla, E. (2019). Microbiome. Parkinson’s disease and molecular mimicry. Cells 8:222. doi: 10.3390/cell8030222
Mokoena, M. P. (2017). Lactic acid bacteria and their bacteriocins: classification, biosynthesis and applications against uropathogens: a mini-review. Molecules 22:1255. doi: 10.3390/molecules22081255
Mombelli, E., Lopizzo, N., Marizzoni, M., Mirabelli, P., Luongo, D., Salvatore, M., et al. (2020). Effect of a probiotic administration on inflammatory profile and clinical features in patients with Alzheimer’s disease. Alzheimer’s Dementia 16:e042737. doi: 10.1002/alz.042737
Moore, K., Hughes, C. F., Ward, M., Hoey, L., and McNulty, H. (2018). Diet, nutrition and the ageing brain: current evidence and new directions. Proc. Nutrition Soc. 77, 152–163. doi: 10.1017/S0029665117004177
Morgan, M. J., and Zheng-gang, L. (2010). Crosstalk of reactive oxygen species and NF-K B signaling. Cell Res. 21, 103–115. doi: 10.1038/cr.2010.178
Morgan, R. K., McAllister, B., Cross, L., Green, D. S., Kornfeld, H., Center, D. M., et al. (2007). Histamine 4 receptor activation induces recruitment of FoxP3+ T cells and inhibits allergic asthma in a murine model. J. Immunol. 178, 8081–8089. doi: 10.4049/jimmunol.178.12.8081
Morris, J. K., Vidoni, Johnson, D. K., Van Sciver, A., Mahnken, J. D., Honea, R. A., et al. (2017). Aerobic exercise for Alzheimer’s disease: a randomized controlled pilot trial. PLoS One 12:e0170547. doi: 10.1371/journal.pone.0170547
Morris, M. C., Tangney, C. C., Wang, Y., Sacks, F. M., Barnes, L. L., Bennett, D. A., et al. (2015). MIND diet slows cognitive decline with aging. Alzheimer’s Dementia 11, 1015–1022. doi: 10.1016/j.jalz.2015.04.011
Morshedi, M., Hashemi, R., Moazzen, S., Sahebkar, A., and Hosseinifard, E. S. (2019). Immunomodulatory and anti-inflammatory effects of probiotics in multiple sclerosis: a systematic review. J. Neuroinflamm. 16:231. doi: 10.1186/s12974-019-1611-4
Mosconi, L., Walters, M., Sterling, J., Quinn, C., McHugh, P., Andrews, R. E., et al. (2018). Lifestyle and vascular risk effects on MRI-based biomarkers of Alzheimer’s disease: a cross-sectional study of middle-aged adults from the broader New York City area. BMJ Open 8:e019362. doi: 10.1136/bmjopen-2017-019362
Mota de Carvalho, N., Costa, E. M., Silva, S., Pimentel, L., Fernandes, T. H., et al. (2018). Fermented foods and beverages in human diet and their influence on gut microbiota and health. Fermentation 4:90. doi: 10.3390/fermentation4040090
Mullane, K., and Williams, M. (2013). Alzheimer’s therapeutics: continued clinical failures question the validity of the amyloid hypothesis—but what lies beyond? Biochem. Pharmacol. 85, 289–305. doi: 10.1016/j.bcp.2012.11.014
Munster, C. (2020). The Effect of High-fat, Western Diet vs. Plant-based, Mediterranean Diet on Alzheimer’s Pathology in Mice thesis, Departmental Honors Fort Worth, TX: Texas Christian University.
Murgas, P., Godoy, B., and von Bernhardi, R. (2012). Aβ potentiates inflammatory activation of glial cells induced by scavenger receptor ligands and inflammatory mediators in culture. Neurotox. Res. 22, 69–78. doi: 10.1007/s12640-011-9306-3
Murray, M. E., Lowe, V. J., Graff-Radford, N. R., Liesinger, A. M., Cannon, A., Przybelski, S. A., et al. (2015). Clinicopathologic and 11C-Pittsburgh compound B implications of Thal amyloid phase across the Alzheimer’s disease spectrum. Brain 138, 1370–1381. doi: 10.1093/brain/awv050
Nagpal, R., Neth, B. J., Wang, S., Craft, S., and Yadav, H. (2019). Modified Mediterranean-ketogenic diet modulates gut microbiome and short-chain fatty acids in association with Alzheimer’s disease markers in subjects with mild cognitive impairment. EBioMedicine 47, 529–542. doi: 10.1016/j.ebiom.2019.08.032
Naja, F., Hwalla, N., Itani, L., Karam, S., Mehio Sibai, A., and Nasreddine, L. (2015). A Western dietary pattern is associated with overweight and obesity in a national sample of Lebanese adolescents (13-19 years): a cross-sectional study. Br. J. Nutrition 114, 1909–1919. doi: 10.1017/S0007114515003657
Nam, K. N., Mounier, A., Wolfe, C. M., Fitz, N. F., Carter, A. Y., Castranio, E. L., et al. (2017). Effect of high fat diet on phenotype, brain transcriptome and lipidome in Alzheimer’s model mice. Sci. Rep. 7:4307. doi: 10.1038/s41598-017-04412-2
Nam, K. N., Park, Y. M., Jung, H. J., Lee, J. Y., Min, B. D., Park, S. U., et al. (2010). Anti-inflammatory effects of crocin and crocetin in rat brain microglial cells. Eur. J. Pharmacol. 648, 110–116. doi: 10.1016/j.ejphar.2010.09.003
Nan, W., Si, H., Yang, Q., Shi, H., Zhang, T., Shi, Q., et al. (2021). Effect of vitamin a supplementation on growth performance, serum biochemical parameters, intestinal immunity response and gut microbiota in american mink (Neovison vison). Animals (Basel) 11:1577. doi: 10.3390/ani11061577
Nankova, B. B., Agarwal, R., MacFabe, D. F., and La Gamma, E. F. (2014). Enteric bacterial metabolites propionic and butyric acid modulate gene expression, including CREB-dependent catecholaminergic neurotransmission, in PC12 cells–possible relevance to autism spectrum disorders. PLoS One 9:e103740. doi: 10.1371/journal.pone.0103740
Naomi, R., Embong, H., Othman, F., Ghazi, H. F., Maruthey, N., and Bahari, H. (2021). Probiotics for Alzheimer’s disease: a systematic review. Nutrients 14:20. doi: 10.3390/nu14010020
Natale, G., Biagioni, F., Busceti, C. L., Gambardella, S., Limanaqi, F., and Fornai, F. (2019). TREM receptors connecting bowel inflammation to neurodegenerative disorders. Cells 8:1124. doi: 10.3390/cells8101124
Ndountse, L. T., and Chan, H. M. (2009). Role of N-methyl-D-aspartate receptors in polychlorinated biphenyl mediated neurotoxicity. Toxicol. Lett. 184, 50–55. doi: 10.1016/j.toxlet.2008.10.013
Nelson, P. T., Braak, H., and Markesbery, W. R. (2009). Neuropathology and cognitive impairment in Alzheimer disease: a complex but coherent relationship. J. Neuropathol. Exp. Neurol. 68, 1–14. doi: 10.1097/NEN.0b013e3181919a48
Ngandu, T., Lehtisalo, J., Solomon, A., Levälahti, E., Ahtiluoto, S., Antikainen, R., et al. (2015). A 2 year multidomain intervention of diet, exercise, cognitive training, and vascular risk monitoring versus control to prevent cognitive decline in at-risk elderly people (FINGER): a randomised controlled trial. Lancet 385, 2255–2263. doi: 10.1016/S0140-6736(15)60461-5
Nicoll, J. A. R., Buckland, G. R., Harrison, C. H., Page, A., Harris, S., Love, S., et al. (2019). Persistent neuropathological effects 14 years following amyloid-β immunization in Alzheimer’s disease. Brain 142, 2113–2126. doi: 10.1093/brain/awz142
Alzheimer’s Dementia (2020). 2020 Alzheimer’s disease facts and figures. Alzheimer’s Dementia 16, 391–460. doi: 10.1002/alz.12068
Nutaitis, A. C., Tharwani, S. D., Serra, M. C., Goldstein, F. C., Zhao, L., Sher, S. S., et al. (2019). Diet as a risk factor for cognitive decline in african americans and caucasians with a parental history of Alzheimer’s disease: a cross-sectional pilot study dietary patterns. J. Prevention Alzheimers Dis. 6, 50–55. doi: 10.14283/jpad.2018.44
Oakley, H., Cole, S. L., Logan, S., Maus, E., Shao, P., Craft, J., et al. (2006). Intraneuronal β-Amyloid aggregates, neurodegeneration, and neuron loss in transgenic mice with five familial Alzheimer’s disease mutations: potential factors in amyloid plaque formation. J. Neurosci. 26, 10129–10140. doi: 10.1523/jneurosci.1202-06.2006
O’Callaghan, A., and van Sinderen, D. (2016). Bifidobacteria and their role as members of the human gut microbiota. Front. Microbiol. 7:925. doi: 10.3389/fmicb.2016.00925
Offermanns, S. (2014). Free fatty acid [FFA) and hydroxy carboxylic acid [HCA) receptors. Annu. Rev. Pharmacol. Toxicol. 54, 407–434.
Oksanen, M., Petersen, A. J., Naumenko, N., Puttonen, K., Lehtonen, Š, Gubert Olivé, M., et al. (2017). PSEN1 mutant iPSC-Derived model reveals severe astrocyte pathology in Alzheimer’s disease. Stem Cell Rep. 9, 1885–1897. doi: 10.1016/j.stemcr.2017.10.016
Ottman, N., Smidt, H., de Vos, W., and Belzer, C. (2012). The function of our microbiota: who is out there and what do they do? Front. Cell. Infect. Microbiol. 2:104. doi: 10.3389/fcimb.2012.00104
Pardridge, W. M. (2020). Blood-Brain barrier and delivery of protein and gene therapeutics to brain. Front. Aging Neurosci. 11:373. doi: 10.3389/fnagi.2019.00373
Parker, A., Fonseca, S., and Carding, S. R. (2020). Gut microbes and metabolites as modulators of blood-brain barrier integrity and brain health. Gut Microbes 11, 135–157. doi: 10.1080/19490976.2019.1638722
Peng, M., Tabashsum, Z., Patel, P., Bernhardt, C., Biswas, C., Meng, J., et al. (2020). Prevention of enteric bacterial infections and modulation of gut microbiota with conjugated linoleic acids producing Lactobacillus in mice. Gut Microbes 11, 433–452. doi: 10.1080/19490976.2019.1638724
Perea, G., Navarrete, M., and Araque, A. (2009). Tripartite synapses: astrocytes process and control synaptic information. Trends Neurosci. 32, 421–431.
Perea, J. R., López, E., Díez-Ballesteros, J. C., Ávila, J., Hernández, F., and Bolós, M. (2019). Extracellular monomeric tau is internalized by astrocytes. Front. Neurosci. 13:442. doi: 10.3389/fnins.2019.00442
Peterson, C. T. (2020). Dysfunction of the microbiota-gut-brain axis in neurodegenerative disease: the promise of therapeutic modulation with prebiotics, medicinal herbs, probiotics, and synbiotics. J. Evidence-based Int. Med. 25:2515690X20957225. doi: 10.1177/2515690X20957225
Piccio, L., Deming, Y., Del-Águila, J. L., Ghezzi, L., Holtzman, D. M., Fagan, A. M., et al. (2016). Cerebrospinal fluid soluble TREM2 is higher in Alzheimer disease and associated with mutation status. Acta Neuropathol. 131, 925–933. doi: 10.1007/s00401-016-1533-5
Pino, E., Amamoto, R., Zheng, L., Cacquevel, M., Sarria, J. C., Knott, G. W., et al. (2014). FOXO3 determines the accumulation of α-synuclein and controls the fate of dopaminergic neurons in the substantia nigra. Hum. Mol. Genet. 23, 1435–1452. doi: 10.1093/hmg/ddt530
Pourbaba, H., Anvar, A., Pourahmad, R., and Ahari, H. (2021). Increase in conjugated linoleic acid content and improvement in microbial and physicochemical properties of a novel kefir stored at refrigerated temperature using complementary probiotics and prebiotic. Food Sci. Technol. 41, 254–266. doi: 10.1590/fst.61520
Profaci, C. P., Munji, R. N., Pulido, R. S., and Daneman, R. (2020). The blood-brain barrier in health and disease: important unanswered questions. J. Exp. Med. 217:e20190062. doi: 10.1084/jem.20190062
Pu, S., Khazanehei, H., Jones, P. J., and Khafipour, E. (2016). Interactions between obesity status and dietary intake of monounsaturated and polyunsaturated oils on human gut microbiome profiles in the Canola Oil Multicenter Intervention Trial (COMIT). Front. Microbiol. 7:1612.
Pushpanathan, P., Mathew, G. S., Selvarajan, S., Seshadri, K. G., and Srikanth, P. (2019). Gut microbiota and its mysteries. Indian J. Med. Microbiol. 37, 268–277. doi: 10.4103/ijmm.IJMM_19_373
Qosa, H., Mohamed, L. A., Batarseh, Y. S., Alqahtani, S., Ibrahim, B., LeVine, H., et al. (2015). Extra-virgin olive oil attenuates amyloid-β and tau pathologies in the brains of TgSwDI mice. J. Nutr. Biochem. 26, 1479–1490. doi: 10.1016/j.jnutbio.2015.07.022
Rackerby, B., Kim, H. J., Dallas, D. C., and Park, S. H. (2020). Understanding the effects of dietary components on the gut microbiome and human health. Food Sci. Biotechnol. 29, 1463–1474. doi: 10.1007/s10068-020-00811-w
Rajmohan, R., and Reddy, P. H. (2017). Amyloid-Beta and phosphorylated tau accumulations cause abnormalities at synapses of Alzheimer’s disease neurons. J. Alzheimer’s Dis. 57, 975–999.
Rakhra, V., Galappaththy, S. L., Bulchandani, S., and Cabandugama, P. K. (2020). Obesity and the western diet: how we got here. Mo. Med. 117, 536–538.
Ravaut, G., Légiot, A., Bergeron, K.-F., and Mounier, C. (2021). Monounsaturated fatty acids in obesity-related inflammation. Int. J. Mol. Sci. 22:330. doi: 10.3390/ijms22010330
Reddy, V. P., Aryal, P., Robinson, S., Rafiu, R., Obrenovich, M., and Perry, G. (2020). Polyphenols in Alzheimer’s disease and in the gut-brain axis. Microorganisms 8:199. doi: 10.3390/microorganisms8020199
Reigstad, C. S., Salmonson, C. E., Rainey, J. F. I. I. I., Szurszewski, J. H., Linden, D. R., Sonnenburg, J. L., et al. (2015). Gut microbes promote colonic serotonin production through an effect of short-chain fatty acids on enterochromaffin cells. FASEB J. 29, 1395–1403. doi: 10.1096/fj.14-259598
Reitz, C., Brayne, C., and Mayeux, R. (2011). Epidemiology of Alzheimer disease. Nat. Rev. Neurol. 7, 137–152.
Rezac, S., Kok, C. R., Heermann, M., and Hutkins, R. (2018). Fermented foods as a dietary source of live organisms. Front. Microbiol. 9:1785. doi: 10.3389/fmicb.2018.01785
Richard, E., den Brok, M. G. H. E., and van Gool, W. A. (2021). Bayes analysis supports null hypothesis of anti-amyloid beta therapy in Alzheimer’s disease. Alzheimer’s Dementia 17, 1051–1055. doi: 10.1002/alz.12379
Rinninella, E., Raoul, P., Cintoni, M., Franceschi, F., Miggiano, G. A. D., Gasbarrini, A., et al. (2019). What is the healthy gut microbiota composition? a changing ecosystem across age, environment, diet, and diseases. Microorganisms 7:14. doi: 10.3390/microorganisms7010014
Rizzatti, G., Lopetuso, L. R., Gibiino, G., Binda, C., and Gasbarrini, A. (2017). Proteobacteria: a common factor in Human diseases. BioMed Res. Int. 2017:9351507. doi: 10.1155/2017/9351507
Rocaspana-García, M., Blanco-Blanco, J., Arias-Pastor, A., Gea-Sánchez, M., and Piñol-Ripoll, G. (2018). Study of community-living Alzheimer’s patients’ adherence to the Mediterranean diet and risks of malnutrition at different disease stages. PeerJ 6:e5150. doi: 10.7717/peerj.5150
Román, G. C., Jackson, R. E., Reis, J., Román, A. N., Toledo, J. B., and Toledo, E. (2019). Extra-virgin olive oil for potential prevention of Alzheimer disease. Revue Neurologique 175, 705–723. doi: 10.1016/j.neurol.2019.07.017
Rothhammer, V., Mascanfroni, I. D., Bunse, L., Takenaka, M. C., Kenison, J. E., Mayo, L., et al. (2016). Type I interferons and microbial metabolites of tryptophan modulate astrocyte activity and central nervous system inflammation via the aryl hydrocarbon receptor. Nat. Med. 22, 586–597. doi: 10.1038/nm.4106
Rothstein, J. D., Dykes-Hoberg, M., Pardo, C. A., Bristol, L. A., Jin, L., Kuncl, R. W., et al. (1996). Knockout of glutamate transporters reveals a major role for astroglial transport in excitotoxicity and clearance of glutamate. Neuron 16, 675–686. doi: 10.1016/S0896-6273(00)80086-0
Ruotolo, R., Minato, I., La Vitola, P., Artioli, L., Curti, C., Franceschi, V., et al. (2020). Flavonoid-Derived human Phenyl-γ-Valerolactone metabolites selectively detoxify Amyloid-β oligomers and prevent memory impairment in a mouse model of Alzheimer’s disease. Mol. Nutr. Food Res. 64:e1900890. doi: 10.1002/mnfr.201900890
Rusek, M., Pluta, R., Ułamek-Kozioł, M., and Czuczwar, S. J. (2019). Ketogenic diet in Alzheimer’s disease. Int. J. Mol. Sci. 20:3892. doi: 10.3390/ijms20163892
Sachdeva, A. K., and Chopra, K. (2015). Lycopene abrogates Aβ(1-42)-mediated neuroinflammatory cascade in an experimental model of Alzheimer’s disease. J. Nutr. Biochem. 26, 736–744. doi: 10.1016/j.jnutbio.2015.01.012
Saez-Lara, M. J., Gomez-Llorente, C., Plaza-Diaz, J., and Gil, A. (2015). The role of probiotic lactic acid bacteria and bifidobacteria in the prevention and treatment of inflammatory bowel disease and other related diseases: a systematic review of randomized human clinical trials. Biomed. Res. Int. 2015:505878. doi: 10.1155/2015/505878
Sahoo, A. K., Dandapat, J., Dash, U. C., and Kanhar, S. (2018). Features and outcomes of drugs for combination therapy as multi-targets strategy to combat Alzheimer’s disease. J. Ethnopharmacol. 215, 42–73. doi: 10.1016/j.jep.2017.12.015
Salloway, S. P., Sevingy, J., Budur, K., Pederson, J. T., DeMattos, R. B., Von Rosenstiel, P., et al. (2020). Advancing combination therapy for Alzheimer’s disease. Alzheimer’s Dementia: Trans. Res. Clin. Interventions 6:e12073. doi: 10.1002/trc2.12073
Saman, S., Kim, W., Raya, M., Visnick, Y., Miro, S., Saman, S., et al. (2012). Exosome-associated tau is secreted in tauopathy models and is selectively phosphorylated in cerebrospinal fluid in early Alzheimer disease. J. Biol. Chem. 287, 3842–3849. doi: 10.1074/jbc.M111.277061
Sampson, T. R., Debelius, J. W., Thron, T., Janssen, S., Shastri, G. G., Ilhan, Z. E., et al. (2016). Gut microbiota regulate motor deficits and neuroinflammation in a model of Parkinson’s disease. Cell 167, 1469–1480.e12.
Sánchez-Tapia, M., Tovar, A. R., and Torres, N. (2019). Diet as regulator of gut microbiota and its role in health and disease. Arch. Med. Res. 50, 259–268. doi: 10.1016/j.arcmed.2019.09.004
Sano, M., Ernesto, C., Thomas, R. G., Klauber, M. R., Schafer, K., Grundman, M., et al. (1997). A controlled trial of selegiline, alpha-tocopherol, or both as treatment for Alzheimer’s disease. New England J. Med. 336, 1216–1222. doi: 10.1056/nejm199704243361704
Schwarz, A. J., Shcherbinin, S., Slieker, L. J., Risacher, S. L., Charil, A., Irizarry, M. C., et al. (2018). Topographic staging of tau positron emission tomography images. Alzheimer’s Dementia: Diagnosis Assess. Dis. Monitor. 10, 221–231. doi: 10.1016/j.dadm.2018.01.006
Serrano-Pozo, A., Frosch, M. P., Masliah, E., and Hyman, B. T. (2011). Neuropathological alterations in Alzheimer disease. Cold Spring Harb. Perspect. Med. 1:a006189. doi: 10.1101/cshperspect.a006189
Seshadri, S., Drachman, D. A., and Lippa, C. F. (1995). Apolipoprotein E ε4 allele and the lifetime risk of Alzheimer’s disease: what physicians know, and what they should know. Arch. Neurol. 52, 1074–1079. doi: 10.1001/archneur.1995.00540350068018
Shah, P., Nankova, B. B., Parab, S., and La Gamma, E. F. (2006). Short chain fatty acids induce TH gene expression via ERK-dependent phosphorylation of CREB protein. Brain Res. 1107, 13–23. doi: 10.1016/j.brainres.2006.05.097
Shigetomi, E., Bowser, D. N., Sofroniew, M. V., and Khakh, B. S. (2008). Two forms of astrocyte calcium excitability have distinct effects on NMDA receptor-mediated slow inward currents in pyramidal neurons. J. Neurosci. 28, 6659–6663. doi: 10.1523/jneurosci.1717-08.2008
Shirpoor, A., Barmaki, H., Khadem Ansari, M., Lkhanizadeh, B., and Barmaki, H. (2016). Protective effect of vitamin E against ethanol-induced small intestine damage in rats. Biomed. Pharmacother. 78, 150–155. doi: 10.1016/j.biopha.2016.01.015
Sieber, J., Lindenmeyer, M. T., Kampe, K., Campbell, K. N., Cohen, C. D., Hopfer, H., et al. (2010). Regulation of podocyte survival and endoplasmic reticulum stress by fatty acids. Am. J Physiol Ren Physiol. 299, F821–F829. doi: 10.1152/ajprenal.00196.2010
Silva, Y. P., Bernardi, A., and Frozza, R. L. (2020). The role of short-chain fatty acids from gut microbiota in gut-brain communication. Front. Endocrinol. 11:25. doi: 10.3389/fendo.2020.00025
Simopoulos, A. P. (2016). An increase in the Omega-6/Omega-3 fatty acid ratio increases the risk for obesity. Nutrients 8:128. doi: 10.3390/nu8030128
Sjögren, M. J., Hellström, P. T., Jonsson, M. A., Runnerstam, M., Silander, H. C., and Ben-Menachem, E. (2002). Cognition-enhancing effect of vagus nerve stimulation in patients with Alzheimer’s disease: a pilot study. J. Clin. Psychiatry 63, 972–980. doi: 10.4088/jcp.v63n1103
Smith, P. J. (2019). Pathways of prevention: a scoping review of dietary and exercise interventions for neurocognition. Brain Plasticity 5, 3–38. doi: 10.3233/BPL-190083
So, D., Whelan, K., Rossi, M., Morrison, M., Holtmann, G., Kelly, J. T., et al. (2018). Dietary fiber intervention on gut microbiota composition in healthy adults: a systematic review and meta-analysis. Am. J. Clin. Nutr. 107, 965–983. doi: 10.1093/ajcn/nqy041
Socała, K., Doboszewska, U., Szopa, A., Serefko, A., Włodarczyk, M., Zieliñska, A., et al. (2021). The role of microbiota-gut-brain axis in neuropsychiatric and neurological disorders. Pharmacol. Res. 172:105840. doi: 10.1016/j.phrs.2021e.105840
Sofroniew, M. V. (2015). Astrocyte barriers to neurotoxic inflammation. Nat. Rev. Neurosci. 16, 249–263. doi: 10.1038/nrn3898
Sompol, P., Furman, J. L., Pleiss, M. M., Kraner, S. D., Artiushin, I. A., Batten, S. R., et al. (2017). Calcineurin/NFAT signaling in activated astrocytes drives network hyperexcitability in Aβ-Bearing mice. J. Neurosci. 37, 6132–6148. doi: 10.1523/jneurosci.0877-17.2017
Spires-Jones, T. L., Attems, J., and Thal, D. R. (2017). Interactions of pathological proteins in neurodegenerative diseases. Acta Neuropathol. 134, 187–205. doi: 10.1007/s00401-017-1709-7
Statovci, D., Aguilera, M., MacSharry, J., and Melgar, S. (2017). The impact of western diet and nutrients on the microbiota and immune response at mucosal interfaces. Front. Immunol. 8:838. doi: 10.3389/fimmu.2017.00838
Stojanov, S., Berlec, A., and Štrukelj, B. (2020). The influence of probiotics on the firmicutes/bacteroidetes ratio in the treatment of obesity and inflammatory Bowel disease. Microorganisms 8:1715. doi: 10.3390/microorganisms8111715
Strandwitz, P. (2018). Neurotransmitter modulation by the gut microbiota. Brain Res. 1693, 128–133. doi: 10.1016/j.brainres.2018.03.015
Suarez, A. N., Hsu, T. M., Liu, C. M., Noble, E. E., Cortella, A. M., Nakamoto, E. M., et al. (2018). Gut vagal sensory signaling regulates hippocampus function through multi-order pathways. Nat. Commun. 9:2181. doi: 10.1038/s41467-018-04639-1
Suganya, K., and Koo, B.-S. (2020). Gut-Brain axis: role of gut microbiota on neurological disorders and how probiotics/prebiotics beneficially modulate microbial and immune pathways to improve brain functions. Int. J. Mol. Sci. 21:7551. doi: 10.3390/ijms21207551
Sun, J. (2010). Vitamin D and mucosal immune function. Curr. Opin. Gastroenterol. 26, 591–595. doi: 10.1097/MOG.0b013e32833d4b9f
Sun, J., Xu, J., Yang, B., Chen, K., Kong, Y., Fang, N., et al. (2020). Effect of Clostridium butyricum against microglia-mediated neuroinflammation in Alzheimer’s disease via regulating gut microbiota and metabolites butyrate. Mol. Nutrition Food Res. 64:1900636. doi: 10.1002/mnfr.201900636
Sweeney, T. E., and Morton, J. M. (2013). The human gut microbiome: a review of the effect of obesity and surgically induced weight loss. JAMA Surgery 148, 563–569. doi: 10.1001/jamasurg.2013.5
Tamer, F., Ulug, E., Akyol, A., and Nergiz-Unal, R. (2020). The potential efficacy of dietary fatty acids and fructose induced inflammation and oxidative stress on the insulin signaling and fat accumulation in mice. Food Chem. Toxicol. 135:110914. doi: 10.1016/j.fct.2019.110914
Tan, F. H. P., Liu, G., Lau, S. A., Jaafar, M. H., Park, Y. H., Azzam, G., et al. (2020). Lactobacillus probiotics improved the gut microbiota profile of a Drosophila melanogaster Alzheimer’s disease model and alleviated neurodegeneration in the eye. Benef. Microbes 11, 79–89. doi: 10.3920/bm2019.0086
Tang, M., Frank, D. N., Sherlock, L., Ir, D., Robertson, C. E., and Krebs, N. F. (2016). Effect of Vitamin E with therapeutic iron supplementation on iron repletion and gut microbiome in US iron deficient infants and toddlers. J. Pediatr. Gastroenterol. Nutr. 63, 379–385. doi: 10.1097/MPG.0000000000001154
Tang, W., Meng, Z., Li, N., Liu, Y., Li, L., Chen, D., et al. (2021). Roles of gut microbiota in the regulation of hippocampal plasticity, inflammation, and hippocampus-dependent behaviors. Front. Cell. Infect. Microbiol. 10:611014. doi: 10.3389/fcimb.2020.611014
Tangestani, H., Emamat, H., Ghalandari, H., and Shab-Bidar, S. (2020). Whole grains, dietary fibers and the human gut microbiota: a systematic review of existing literature. Recent Pat. Food Nutr. Agric. 11, 235–248. doi: 10.2174/2212798411666200316152252
Tangney, C. C., Li, H., Wang, Y., Barnes, L., Schneider, J. A., Bennett, D. A., et al. (2014). Relation of DASH- and mediterranean-like dietary patterns to cognitive decline in older persons. Neurology 83, 1410–1416. doi: 10.1212/wnl.0000000000000884
Tapiola, T., Alafuzoff, I., Herukka, S.-K., Parkkinen, L., Hartikainen, P., Soininen, H., et al. (2009). Cerebrospinal fluid β-Amyloid 42 and tau proteins as biomarkers of alzheimer-type pathologic changes in the brain. Arch. Neurol. 66, 382–389. doi: 10.1001/archneurol.2008.596
Theofilas, P., Ehrenberg, A. J., Nguy, A., Thackrey, J. M., Dunlop, S., Mejia, M. B., et al. (2018). Probing the correlation of neuronal loss, neurofibrillary tangles, and cell death markers across the Alzheimer’s disease Braak stages: a quantitative study in humans. Neurobiol. Aging 61, 1–12. doi: 10.1016/j.neurobiolaging.2017.09.007
Thomas, C. M., Hong, T., van Pijkeren, J. P., Hemarajata, P., Trinh, D. V., Hu, W., et al. (2012). Histamine derived from probiotic Lactobacillus reuteri suppresses TNF via modulation of PKA and ERK signaling. PLoS One 7:e31951. doi: 10.1371/journal.pone.0031951
Thursby, E., and Juge, N. (2017). Introduction to the human gut microbiota. Biochem. J. 474, 1823–1836. doi: 10.1042/bcj20160510
Tiwari, S., Atluri, V., Kaushik, A., Yndart, A., and Nair, M. (2019). Alzheimer’s disease: pathogenesis, diagnostics, and therapeutics. Int. J. Nanomed. 14, 5541–5554. doi: 10.2147/IJN.S200490
Tomaro-Duchesneau, C., Saha, S., Malhotra, M., Coussa-Charley, M., Kahouli, I., Jones, M. L., et al. (2012). Probiotic ferulic acid esterase active Lactobacillus fermentum NCIMB 5221 APA microcapsules for oral delivery: preparation and in vitro characterization. Pharmaceuticals 5, 236–248. doi: 10.3390/ph5020236
Tomova, A., Bukovsky, I., Rembert, E., Yonas, W., Alwarith, J., Barnard, N. D., et al. (2019). The effects of vegetarian and vegan diets on gut microbiota. Front. Nutrition 6:47. doi: 10.3389/fnut.2019.00047
Tran, T. T. T., Corsini, S., Kellingray, L., Hegarty, C., Le Gall, G., Narbad, A., et al. (2019). APOE genotype influences the gut microbiome structure and function in humans and mice: relevance for Alzheimer’s disease pathophysiology. FASEB J. 33, 8221–8231. doi: 10.1096/fj.201900071R
Trotta, T., Antonietta Panaro, M., Cianciulli, A., Mori, G., Di Benedetto, A., and Porro, C. (2018). Microglia-derived extracellular vesicles in Alzheimer’s disease: a double-edged sword. Biochem. Pharmacol. 148, 184–192. doi: 10.1016/j.bcp.2017.12.020
Troy, E. B., and Kasper, D. L. (2010). Beneficial effects of Bacteroides fragilis polysaccharides on the immune system. Front. Bioscience-Landmark 15, 25–34. doi: 10.2741/3603
Turillazzi, E., Neri, M., Cerretani, D., Cantatore, S., Frati, P., Moltoni, L., et al. (2016). Lipid peroxidation and apoptotic response in rat brain areas induced by long-term administration of nandrolone: the mutual crosstalk between ROS and NF-kB. J. Cell Mol. Med. 20, 601–612. doi: 10.1111/jcmm.12748
Tzekaki, E. E., Papaspyropoulos, A., Tsolaki, M., Lazarou, E., Kozori, M., and Pantazaki, A A. (2021). Restoration of BMI1 levels after the administration of early harvest extra virgin olive oil as a therapeutic strategy against alzheimer’s disease. Exp. Gerontol. 144:111178. doi: 10.1016/j.exger.2020.111178
Uemura, N., Uemura, M. T., Luk, K. C., Lee, V. M. Y., and Trojanowski, J. Q. (2020). Cell-to-Cell transmission of tau and α-Synuclein. Trends Mol. Med. 26, 936–952. doi: 10.1016/j.molmed.2020.03.012
Unno, T., Tamemoto, K., Yayabe, F., and Kakuda, T. (2003). Urinary excretion of 5-(3’,4’-dihydroxyphenyl)-gamma-valerolactone, a ring-fission metabolite of (-)-epicatechin, in rats and its in vitro antioxidant activity. J. Agric. Food Chem. 51, 6893–6898. doi: 10.1021/jf034578e
Valdes, A. M., Walter, J., Segal, E., and Spector, T. D. (2018). Role of the gut microbiota in nutrition and health. BMJ 361:k2179. doi: 10.1136/bmj.k2179
van den Brink, A. C., Brouwer-Brolsma, E. M., Berendsen, A. A. M., and van de Rest, O. (2019). The mediterranean, dietary approaches to stop hypertension (DASH), and Mediterranean-DASH intervention for neurodegenerative delay (MIND) diets are associated with less cognitive decline and a lower risk of alzheimer’s disease—a review. Adv. Nutrition 10, 1040–1065. doi: 10.1093/advances/nmz054
van der Lee, S. J., Wolters, F. J., Ikram, M. K., Hofman, A., Ikram, M. A., Amin, N., et al. (2018). The effect of APOE and other common genetic variants on the onset of Alzheimer’s disease and dementia: a community-based cohort study. Lancet Neurol. 17, 434–444. doi: 10.1016/S1474-4422(18)30053-X
Versele, R., Corsi, M., Fuso, A., Sevin, E., Businaro, R., Gosselet, F., et al. (2020). Ketone bodies promote Amyloid-β1-40 clearance in a human in vitro blood-brain barrier model. Int. J. Mol. Sci. 21:934. doi: 10.3390/ijms21030934
Verzelloni, E., Pellacani, C., Tagliazucchi, D., Tagliaferri, S., Calani, L., Costa, L. G., et al. (2011). Antiglycative and neuroprotective activity of colon-derived polyphenol catabolites. Mol. Nutr. Food Res. 55, (Suppl. 1), S35–S43. doi: 10.1002/mnfr.201000525
Vidovic, D., Davila, R. A., Gronostajski, R. M., Harvey, T. J., and Piper, M. (2018). Transcriptional regulation of ependymal cell maturation within the postnatal brain. Neural Dev. 13:2. doi: 10.1186/s13064-018-0099-4
Vijay, A., and Valdes, A. M. (2022). Role of the gut microbiome in chronic diseases: a narrative review - european journal of clinical nutrition (2022). Eur. J. Clin. Nutr. 76, 489–501. doi: 10.1038/s41430-021-00991-6
Vijay, N., and Morris, M. E. (2014). Role of monocarboxylate transporters in drug delivery to the brain. Curr. Pharm. Des. 20, 1487–1498. doi: 10.2174/13816128113199990462
Vogt, N. M., Kerby, R. L., Dill-McFarland, K. A., Harding, S. J., Merluzzi, A. P., Johnson, S. C., et al. (2017). Gut microbiome alterations in Alzheimer’s disease. Sci. Rep. 7:13537. doi: 10.1038/s41598-017-13601-y
Wan, H. I., Jacobsen, J. S., Rutkowski, J. L., and Feuerstein, G. Z. (2009). Translational medicine lessons from flurizan’s failure in Alzheimer’s Disease (AD) trial: implication for future drug discovery and development for AD. Clin. Trans. Sci. 2, 242–247. doi: 10.1111/j.1752-8062.2009.00121.x
Wang, J., Ji, H., Wang, S., Liu, H., Zhang, W., Zhang, D., et al. (2018). Probiotic Lactobacillus plantarum promotes intestinal barrier function by strengthening the epithelium and modulating gut microbiota. Front. Microbiol. 9:1953. doi: 10.3389/fmicb.2018.01953
Wang, Y., Balaji, V., Kaniyappan, S., Krüger, L., Irsen, S., Tepper, K., et al. (2017a). The release and trans-synaptic transmission of Tau via exosomes. Mol. Neurodegeneration 12:5. doi: 10.1186/s13024-016-0143-y
Wang, Y., Wang, Z., Wang, Y., Li, F., Jia, J., Song, X., et al. (2018). The gut-microglia connection: implications for central nervous system diseases. Front. Immunol. 9:2325. doi: 10.3389/fimmu.2018.02325
Wang, Y., Wu, Y., Wang, Y., Xu, H., Mei, X., Yu, D., et al. (2017b). Antioxidant properties of probiotic bacteria. Nutrients 9:521. doi: 10.3390/nu9050521
Wei, W., Nguyen, L. N., Kessels, H. W., Hagiwara, H., Sisodia, S., and Malinow, R. (2010). Amyloid beta from axons and dendrites reduces local spine number and plasticity. Nat. Neurosci. 13, 190–196. doi: 10.1038/nn.2476
Weingarten, M. D., Lockwood, A. H., Hwo, S. Y., and Kirschner, M. W. (1975). A protein factor essential for microtubule assembly. Proc. Natl. Acad. Sci. U S A. 72, 1858–1862. doi: 10.1073/pnas.72.5.1858
Wesselman, L. M. P., van Lent, D. M., Schröder, A., van de Rest, O., Peters, O., Menne, F., et al. (2021). Dietary patterns are related to cognitive functioning in elderly enriched with individuals at increased risk for Alzheimer’s disease. Eur. J. Nutr. 60, 849–860. doi: 10.1007/s00394-020-02257-6
Westfall, S., Lomis, N., Kahouli, I., Dia, S. Y., Singh, S. P., and Prakash, S. (2017). Microbiome, probiotics and neurodegenerative diseases: deciphering the gut brain axis. Cell Mol. Life. Sci. 74, 3769–3787. doi: 10.1007/s00018-017-2550-9
Wexler, H. M. (2007). Bacteroides: the good, the bad, and the nitty-gritty. Clin. Microbiol. Rev. 20, 593–621. doi: 10.1128/CMR.00008-07
Wiȩckowska-Gacek, A., Mietelska-Porowska, A., Chutorañski, D., Wydrych, M., Długosz, J., and Wojda, U. (2021). Western diet induces impairment of liver-brain axis accelerating neuroinflammation and amyloid pathology in Alzheimer’s disease. Front. Aging Neurosci. 13:654509. doi: 10.3389/fnagi.2021.654509
Wolters, M., Ahrens, J., Romaní-Pérez, M., Watkins, C., Sanz, Y., Benítez-Páez, A., et al. (2019). Dietary fat, the gut microbiota, and metabolic health - a systematic review conducted within the MyNewGut project. Clin. Nutr. 38, 2504–2520. doi: 10.1016/j.clnu.2018.12.024
Wu, W., Li, Y., Wu, Y., Zhang, Y., Wang, Z., and Liu, X. (2015). Lutein suppresses inflammatory responses through Nrf2 activation and NF-κB inactivation in lipopolysaccharide-stimulated BV-2 microglia. Mol. Nutr. Food Res. 59, 1663–1673. doi: 10.1002/mnfr.201500109
Xiang, S., Ji, J. L., Li, S., Cao, X. P., Xu, W., Tan, L., et al. (2022). Efficacy and safety of probiotics for the treatment of Alzheimer’s disease, mild cognitive impairment, and parkinson’s disease: a systematic review and meta-analysis. Front. Aging Neurosci. 14:730036. doi: 10.3389/fnagi.2022.730036
Xu, J., Xu, C., Chen, X., Cai, X., Yang, S., Sheng, Y., et al. (2014). Regulation of an antioxidant blend on intestinal redox status and major microbiota in early weaned piglets. Nutrition 30, 584–589. doi: 10.1016/j.nut.2013.10.018
Xu, J., Yuan, C., Wang, G., Luo, J., Ma, H., Xu, L., et al. (2018). Urolithins attenuate LPS-Induced neuroinflammation in BV2Microglia via MAPK, Akt, and NF-κB signaling pathways. J. Agric. Food Chem. 66, 571–580. doi: 10.1021/acs.jafc.7b03285
Xu, Y., Hlaing, M. M., Glagovskaia, O., Augustin, M. A., and Terefe, N. S. (2020a). Fermentation by probiotic Lactobacillus gasseri strains enhances the carotenoid and fibre contents of carrot juice. Foods 9:1803. doi: 10.3390/foods9121803
Xu, Y., Zhao, M., Han, Y., and Zhang, H. (2020b). GABAergic inhibitory interneuron deficits in Alzheimer’s disease: implications for treatment. Front. Neurosci. 14:660. doi: 10.3389/fnins.2020.00660
Yan, F., and Polk, D. B. (2020). Probiotics and probiotic-derived functional factors-mechanistic insights into applications for intestinal homeostasis. Front. Immunol. 11:1248. doi: 10.3389/fimmu.2020.01428
Yang, H. J., Kwon, D. Y., Kim, H. J., Kim, M. J., Jung, D. Y., Kang, H. J., et al. (2015). Fermenting soybeans with Bacillus licheniformis potentiates their capacity to improve cognitive function and glucose homeostaisis in diabetic rats with experimental Alzheimer’s type dementia. Eur. J. Nutr. 54, 77–88. doi: 10.1007/s00394-014-0687-y
Yang, Q., Liang, Q., Balakrishnan, B., Belobrajdic, D. P., Feng, Q. J., and Zhang, W. (2020). Role of dietary nutrients in the modulation of gut microbiota: a narrative review. Nutrients 12:381. doi: 10.3390/nu12020381
Yang, Z., Kahn, B. B., Shi, H., and Xue, B. Z. (2010). Macrophage alpha1 AMP-activated protein kinase (alpha1AMPK) antagonizes fatty acid-induced inflammation through SIRT1. J. Biol. Chem. 285, 19051–19059. doi: 10.1074/jbc.M110.123620
Yano, J. M., Yu, K., and Donaldson, G. P. (2015). Indigenous bacteria from the gut microbiota regulate host serotonin biosynthesis. Cell 161, 264–276. doi: 10.1016/j.cell.2015.02.047
Yatsunenko, T., Rey, F. E., Manary, M. J., Trehan, I., Dominguez-Bello, M. G., Contreras, M., et al. (2012). Human gut microbiome viewed across age and geography. Nature 486, 222–227. doi: 10.1038/nature11053
Ye, Z., Xu, Y. J., and Liu, Y. (2021). Influences of dietary oils and fats, and the accompanied minor content of components on the gut microbiota. Trends Food Sci. Technol. 113, 255–276. doi: 10.1016/j.tifs.2021.05.001
Yiannopoulou, K. G., and Papageorgiou, S. G. (2020). Current and future treatments in alzheimer disease: an update. J. Cent. Nerv. Syst. Dis. 12:1179573520907397. doi: 10.1177/1179573520907397
Yu, H. N., Zhu, J., Pan, W. S., Shen, S. R., Shan, W. G., and Das, U. N. (2014). Effects of fish oil with a high content of n-3 polyunsaturated fatty acids on mouse gut microbiota. Arch. Med. Res. 45, 195–202. doi: 10.1016/j.arcmed.2014.03.008
Yurko-Mauro, K., McCarthy, D., Rom, D., Nelson, E. B., Ryan, A. S., Blackwell, A., et al. (2010). Beneficial effects of docosahexaenoic acid on cognition in age-related cognitive decline. Alzheimer’s Dementia 6, 456–464. doi: 10.1016/j.jalz.2010.01.013
Zaccai, J., Brayne, C., McKeith, I., Matthews, F., and Ince, P. G. (2008). On behalf of the MRC cognitive function ANS. patterns and stages of α-synucleinopathy. Relevance Population-based Cohort 70, 1042–1048. doi: 10.1212/01.wnl.0000306697.48738.b6
Zamanian, J. L., Xu, L., Foo, L. C., Nouri, N., Zhou, L., Giffard, R. G., et al. (2012). Genomic analysis of reactive astrogliosis. J. Neurosci. 32, 6391–6410. doi: 10.1523/jneurosci.6221-11.2012
Zaragozá, R. (2020). Transport of amino acids across the blood-brain barrier. Front. Physiol. 11:973. doi: 10.3389/fphys.2020.00973
Zenaro, E., Piacentino, G., and Constantin, G. (2017). The blood-brain barrier in Alzheimer’s disease. Neurobiol. Dis. 107, 41–56. doi: 10.1016/j.nbd.2016.07.007
Zhang, F., Fu, Y., Zhou, X., Pan, W., Shi, Y., Wang, M., et al. (2016). Depression-like behaviors and heme oxygenase-1 are regulated by Lycopene in lipopolysaccharide-induced neuroinflammation. J. Neuroimmunol. 298, 1–8. doi: 10.1016/j.jneuroim.2016.06.001
Zheng, D., Liwinski, T., and Elinav, E. (2020). Interaction between microbiota and immunity in health and disease. Cell Res. 30, 492–506. doi: 10.1038/s41422-020-0332-7
Zhu, S., Jiang, Y., Xu, K., Cui, M., Ye, W., Zhao, G., et al. (2020). The progress of gut microbiome research related to brain disorders. J. Neuroinflamm. 17:25. doi: 10.1186/s12974-020-1705-z
Zhuang, Z.-Q., Shen, L.-L., Li, W.-W., Fu, X., Zeng, F., Gui, L., et al. (2018). Gut microbiota is altered in patients with Alzheimer’s disease. J. Alzheimer’s Dis. 63, 1337–1346. doi: 10.3233/JAD-180176
Zinöcker, M. K., and Lindseth, I. A. (2018). The western diet-microbiome-host interaction and its role in metabolic disease. Nutrients 10:365.
Keywords: Alzheimer’s disease, tau, inflammation, gut–brain axis, probiotic, Mediterranean diet, Western diet
Citation: Lee D, Lee VM and Hur SK (2022) Manipulation of the diet–microbiota–brain axis in Alzheimer’s disease. Front. Neurosci. 16:1042865. doi: 10.3389/fnins.2022.1042865
Received: 13 September 2022; Accepted: 14 October 2022;
Published: 04 November 2022.
Edited by:
Mohd Farooq Shaikh, Monash University, MalaysiaReviewed by:
Thaarvena Retinasamy, Monash University Malaysia, MalaysiaValentina Cecarini, University of Camerino, Italy
Copyright © 2022 Lee, Lee and Hur. This is an open-access article distributed under the terms of the Creative Commons Attribution License (CC BY). The use, distribution or reproduction in other forums is permitted, provided the original author(s) and the copyright owner(s) are credited and that the original publication in this journal is cited, in accordance with accepted academic practice. No use, distribution or reproduction is permitted which does not comply with these terms.
*Correspondence: Seong Kwon Hur, aHVyLnNlb25nQGdlbmUuY29t