- Department of Biology, The College of Idaho, Caldwell, ID, United States
The pteropod mollusk, Clione limacina, is a useful model system for understanding the neural basis of behavior. Of particular interest are the unique swimming behavior and neural circuitry that underlies this swimming behavior. The swimming system of Clione has been studied by two primary groups—one in Russia and one in the United States of America—for more than four decades. The neural circuitry, the cellular properties, and ion channels that create and change the swimming locomotor rhythm of Clione—particularly mechanisms that contribute to swimming acceleration—are presented in this review.
Introduction
Many animals, by virtue of neural circuits called central pattern generators (CPGs) in their central nervous system, can create and control rhythmic movements (Delcomyn, 1980; Guertin, 2013; Bucher et al., 2015). These movements may be visceral in nature, controlling for example, heartbeat and digestive movement (Selverston et al., 1976; Arbas and Calabrese, 1984), or somatic, controlling body movements related to locomotion (Getting, 1981; Grillner and Wallén, 1985; Pearson and Wolf, 1987; Brodfuehrer et al., 1995; Arshavsky et al., 1998; Jing and Gillette, 1999; Thompson and Watson, 2005; Guertin, 2009; Fetcho and McLean, 2010; Zhong et al., 2012; Stein, 2018). This review summarizes the circuit-level and cellular properties of CPG interneurons of the marine pteropod mollusk, Clione limacina, that create and control the rhythmic movement of the animal’s wing-like parapodia. An added emphasis is on the mechanisms contributing to an increase in Clione locomotor speed.
Clione, its major internal organs, and its central nervous system along with some peripheral nerves is shown in Figure 1. The Clione depicted in Figure 1A. is representative of the animal in its slow swimming mode in which the long axis of the body is oriented vertically in the water column with the head (containing the buccal cones, BC, and buccal mass, BM) pointing upward toward the water’s surface and tail (T) pointing directly downward toward the ocean bottom. Within the head are the prey capturing BC and feeding structures of the BM. There are three laterally placed BC on each side of Clione’s head that rapidly inflate to capture its prey, the shelled thecosome pteropod mollusk, Limacina helicina. The BM contains a feeding apparatus that includes chitinous hooks and radula, along with associated musculature. The hooks and radula grasp the Limacina and pull it from the shell to be swallowed. Other organs depicted in Figure 1 include organs of the reproductive system, the ovotestis (OT), organs of the digestive system, the esophagus (E) and digestive gland (DG), and the circulatory system, the heart (H; Wagner, 1885; Lalli and Gilmer, 1989). Because protraction of the prey capturing BC, and an increase in heartbeat occur with swimming acceleration, these structures are relevant to the discussion of the control of Clione swimming speed and are addressed in this review (Arshavsky et al., 1990; Norekian and Satterlie, 1995).
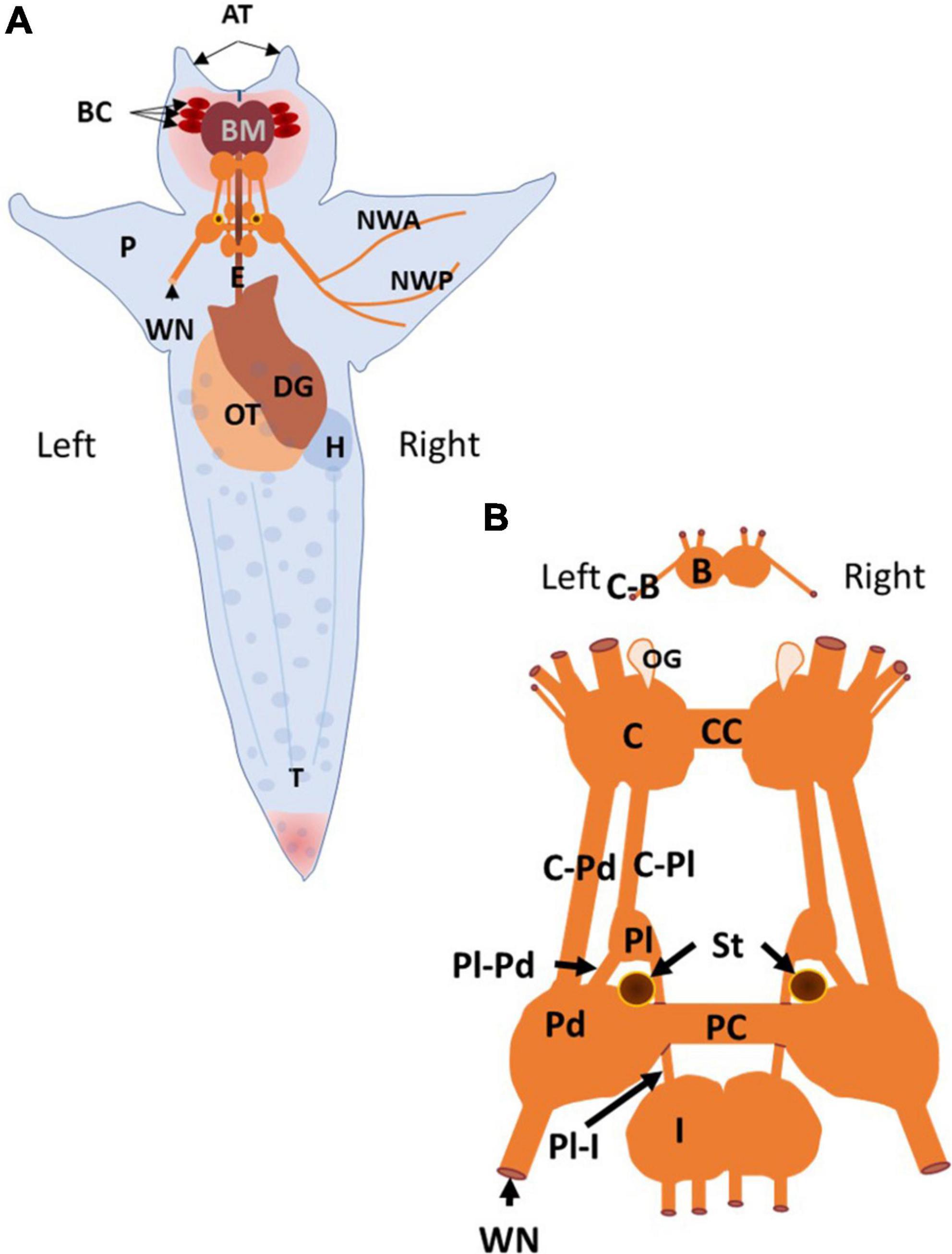
Figure 1. The anatomy of Clione and the organization of its central nervous system and related structures are illustrated. (A) The dorsal surface of Clione is depicted. Clione is typically engaged in slow, hovering swimming in which the long axis of the body is oriented perpendicularly to the ocean surface and ocean bottom with the head pointing upward and the tail pointing downward as depicted. The structures that are identified include retracted buccal cones (BC) and buccal mass (BM) found in the head and are important in prey capture and feeding. The buccal cones participate in prey capture and the buccal mass includes the radula and as set of chitinous hooks with associated musculature for extracting the prey, Limacina helicina, from its shell. Additionally, there are two laterally placed cephalic appendages, the anterior tentacles (AT) connected with the head of Clione. Between the anterior tentacles is slit-like mouth flanked by lips. Limacina is swallowed whole and passes down the esophagus (E) that is encircled by the central nervous system (CNS) composed of interconnected ganglia. Other organs identified include reproductive organs, the ovotestis (OT), digestive organs, the digestive gland (DG), and cardiovascular organs, the heart (H). Additionally, the major nerve innervating the wing-like parapodia (P), the wing nerve (WN) and its two primary branches (NWA and NWP) are identified. (B) The Clione CNS consists of paired ganglia that include the buccal ganglia (B), the cerebral ganglia (C), the pleural ganglia (Pl), the pedal ganglia (Pd), and intestinal ganglia (I). The buccal ganglia are connected to the cerebral ganglia via cerebro-buccal connectives (C-B; cut), the left and right cerebral ganglia are connected to each other by a commissure (CC) and connected to the ipsilateral pleural ganglia and ipsilateral pedal ganglia via the cerebro-pleural connective (C-Pl) and the cerebro-pedal connective (C-Pd), respectively. The pleural ganglia are connected to the ipsilateral pedal and intestinal ganglia via the pleuro-pedal and pleuro-intestinal connectives, respectively. The left and right pedal ganglia are connected to each other by the pedal commissure. The wing nerve is also connected to the pedal ganglia and carries afferent and efferent information related to the wing-like parapodia (P). Other structures identified include the sensory structures labeled OG, the olfactory organ, and ST, the statocysts (Wagner, 1885; Arshavsky et al., 1985a,b,c, 1990; Lalli and Gilmer, 1989; Hermans and Satterlie, 1992; Satterlie et al., 1995; Moroz et al., 2000; Zelenin and Panchin, 2000).
The central nervous system of Clione, shown in Figure 1B, like that of other gastropod mollusks, consists of pairs of connected ganglia that encircle the esophagus (E in Figure 1A) as a circumesophageal ring. From anterior to posterior, the ganglion of Clione includes paired buccal ganglia (B), cerebral ganglia (C), pleural ganglia (Pl), pedal ganglia (Pd), and intestinal ganglia (I). The right and left paired ganglia are connected by commissures, such as the right and left pedal ganglia that are connected via a pedal commissure (PC), or are fused together, such as the intestinal ganglia. There is no connecting commissure between the right and left pleural ganglia. Also shown in Figure 1B are the statocysts (St) that make up the vestibular or statomotor system of Clione, which contribute to the ability of Clione to orient its body within the water column and, during slow swimming, maintain the vertical body orientation as depicted in Figure 1B (Satterlie et al., 1985; Satterlie and Spencer, 1985; Panchin et al., 1995a). The wing nerve (WN) carries axons that innervate the parapodia and branches into two main branches, the anterior and posterior branches (NWA and NWP, respectively; Zelenin and Panchin, 2000).
Swimming locomotion in Clione is produced by the dorsal-ventral movement of its parapodia (P in Figure 1A; also referred to as wings). Parapodial movement is controlled by swimming musculature that is in turn controlled by motoneurons whose rhythmic activity is produced and controlled by a circuit of swim interneurons making up the animal’s swim CPG (Arshavsky et al., 1985a,b,c; Satterlie, 1985; Satterlie and Spencer, 1985). A simple schematic diagram of the Clione swim CPG is illustrated in Figure 2. This diagram is a classic example of the half-center model first proposed by Brown (1911) and shows that the Clione swim CPG consists of two groups of swim interneurons. One group of swim interneurons controls the dorsal (or upstroke) movement of the parapodia, and the other group of swim interneurons controls the ventral (or downstroke) movement of the parapodia. Dorsal and ventral swim interneurons reciprocally inhibit each other so that when one group is active it simultaneously causes an inhibitory postsynaptic potential (IPSP) in the other group, thus inhibiting the other group. This reciprocal inhibition creates the alternating dorsal-ventral movement of the parapodia, and the duration of the IPSP is a principal variable that controls Clione swimming frequency (Arshavsky et al., 1993a; Satterlie et al., 2000). Furthermore, each swim interneuron produces a single broad action potential that conveys information to control synergistic motoneurons whose activity then controls the corresponding parapodial muscle contractions. Thus, there is a one-to-one correspondence between the activity of the interneuron, its synergistic motoneurons, and the parapodial muscle contraction produced by motoneuron innervation (Arshavsky et al., 1985b; Satterlie and Spencer, 1985).
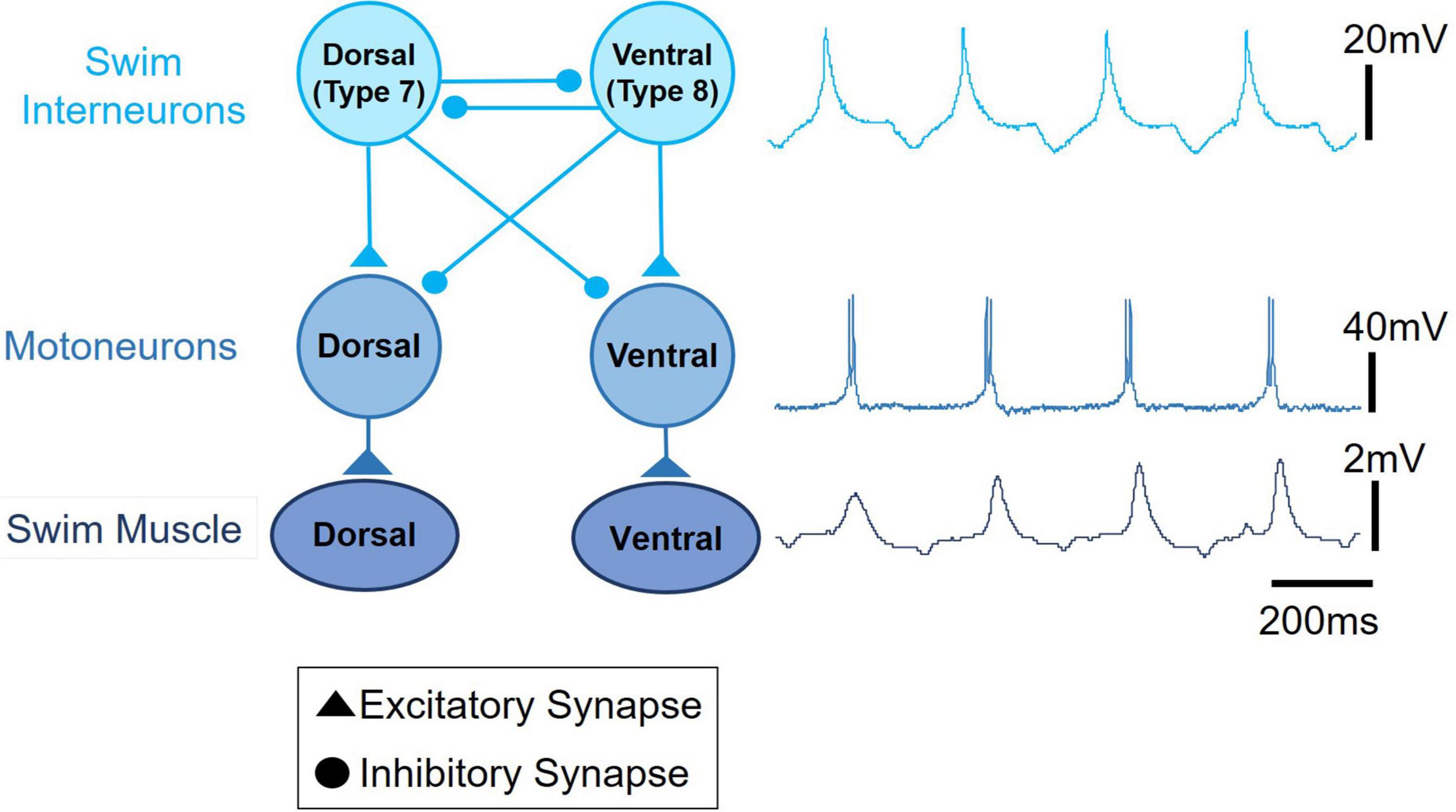
Figure 2. A schematic circuit representation of the Clione swim central pattern generator (swim CPG) network. The single broad action potential recorded from a dorsal swim interneuron, the burst of action potentials recorded from a synergistic dorsal small motoneuron, and the uncalibrated movement of the parapodia are displayed on the right next to the circuit diagram. Excitatory synaptic communication is shown by filled triangles and inhibitory synaptic communication is shown by closed circles. An important network feature of the Clione swim CPG is the reciprocal inhibitory synaptic communication between antagonistic groups of swim interneurons.
The interneurons that compose the Clione swim CPG, the motoneurons that the CPG controls, and the neurons that contribute to the variability of locomotor activity and other related behaviors such as feeding and heartbeat regulation are illustrated in Figures 3A–E.
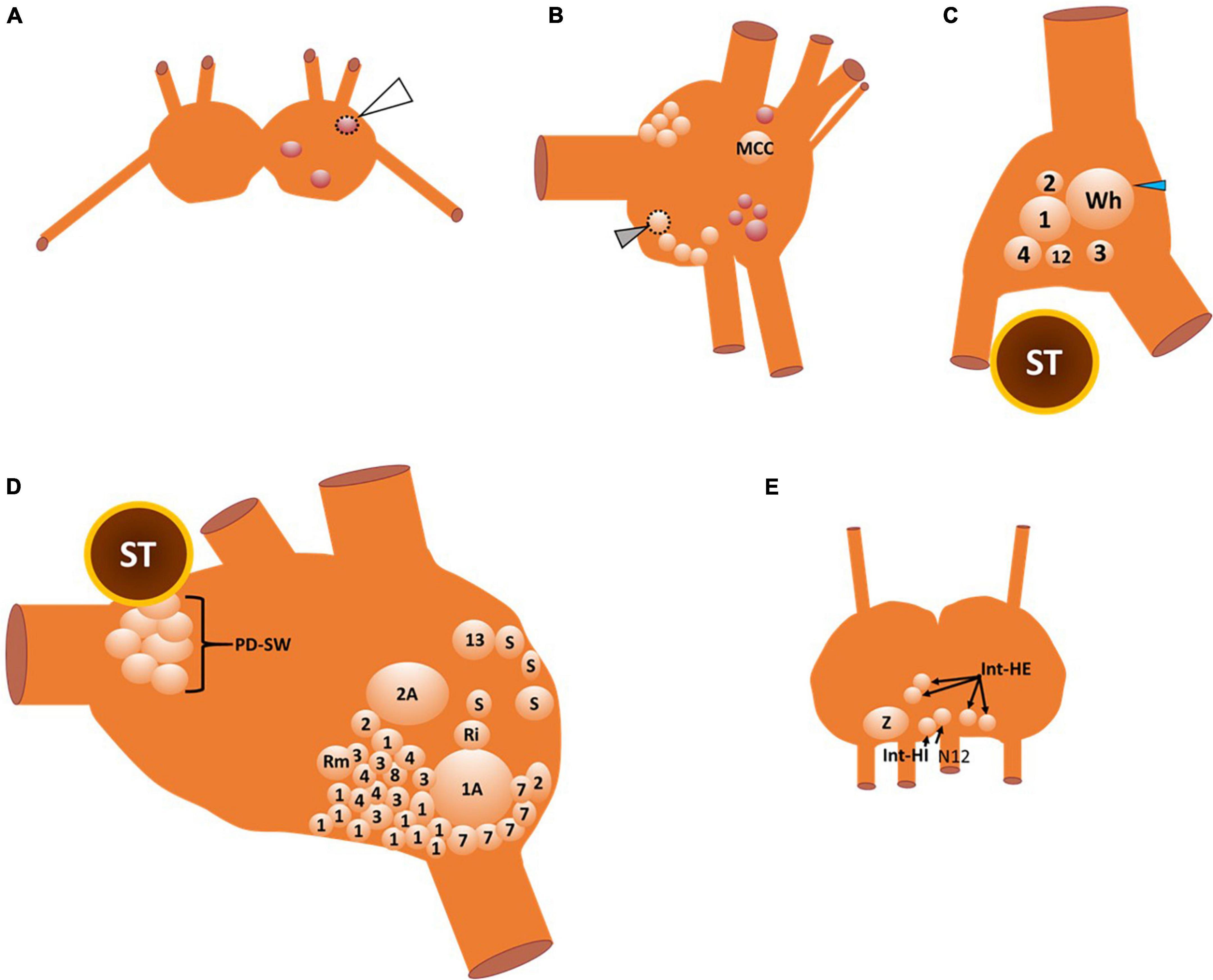
Figure 3. A map of the neuronal somas of the buccal ganglia (A), right cerebral ganglion (B), right pleural ganglion (C), right pedal ganglion (D), and intestinal ganglia (E). Cells that are outlined by a dashed line are located on the ventral surface, otherwise all other somas are located on the dorsal surface. There are three neurons in the buccal ganglia (A) that are all GABAergic (see also Figure 4D). The one buccal ganglia soma that is outlined by the dotted line and indicated by the white arrowhead is located on the ventral surface of the buccal ganglia. The neurons of the cerebral ganglia (B) include the large serotonergic metacerebral cell that participates in feeding behaviors. The cluster of neuronal somas that occur posteromedial (including the ventral cell that is outlined with a dotted line and indicated by the gray arrowhead) and anteromedial near the cerebral commissure (cut) are serotonergic cells that evoke locomotor speed acceleration when stimulated. GABAergic neurons that are shown in red are important elements of the control of feeding behavior. In the right pleural ganglia (C) there are six neuronal somas (all located on the dorsal surface) identified that participate in swimming, whole-body withdrawal, and reproduction. The neuronal soma labeled 12 is the type 12 interneuron that is recruited into the Clione swim CPG during swim acceleration. Neuronal somas 1–4 participate in the whole-body withdrawal response that promotes wing and buccal cone retraction and inhibit swimming. The large soma labeled Wh and indicated by the blue arrowhead is the asymmetric pleural white cell that is only located in the right pleural ganglia. Wh participates in reproduction and egg laying. The neuronal somas of the right pedal ganglia (D) include interneurons that compose the Clione swim CPG, motoneurons, neurons that control the strength of parapodial movement, and neurons related to the parapodial (wing) withdrawal reflex. Odd numbers designate neurons that control the dorsal flexion of the parapodia while even numbers designate neurons that control the ventral flexion of the parapodia during swimming. The neurons labeled 7 and 8 are swim interneurons that compose the Clione swim CPG. The neurons labeled 1, 2, 3, and 4 are small motoneurons whose activity controls the slow-twitch swim muscle fibers within restricted innervation fields of the ipsilateral parapodia. Neurons 1A and 2A are large motoneurons that are also referred to as general excitor motoneurons (GEMN or GE) that innervate the entire expanse of the parapodia and communicate to both slow-twitch and fast-twitch swim muscle fibers in the parapodia. Neuron 13 is dopaminergic and controls swim inhibition. Neurons designated S, Ri, and Rm are sensory, interneuron, and motoneurons, respectively, that control the wing retraction reflex. Neuronal somas identified in the intestinal ganglia (E) control the heartbeat (all on the dorsal surface of the ganglia). These neurons include the intestinal heart excitor cells (Int-HE), Z cell (Z), and intestinal heart inhibitory cells (Int-HI). Axons of these neurons exit the median nerve, N12 (Arshavsky et al., 1985a,b,c, 1989, 1990; Satterlie, 1985, 1995a; Satterlie and Spencer, 1985; Norekyan, 1989; Huang and Satterlie, 1990; Norekian and Satterlie, 1996a; Malyshev et al., 2008).
The buccal ganglia are shown in Figure 3A. The three neuronal somas (colored red) in the buccal ganglia shown in Figure 3A are GABAergic (see also Figure 4D) and control aspects of feeding behavior. The GABAergic neuron indicated by the white arrowhead and outlined with a dashed line is located on the ventral surface of the buccal ganglion. Within the cerebral ganglia shown in Figure 3B, are serotonergic neurons (orange) that are important mediators of swim acceleration. One of these serotonergic neurons is found on the ventral surface of the cerebral ganglion (gray arrowhead and outlined with a dashed line). Additionally, shown in red and located laterally and caudally near the cerebro-pedal connective are four GABAergic neurons (see also Figure 4D) that participate in feeding behavior (Norekian, 1999). Within the pleural ganglia (Figure 3C) are neurons that include swim interneuron 12 that is recruited into the CPG circuit during swim acceleration (Arshavsky et al., 1985d,1989; Pirtle and Satterlie, 2006). Additionally, the neurons controlling whole body withdrawal and inhibition of swimming are in the pleural ganglia and include Pl-W neurons labeled 1–4 (Norekyan, 1989; Norekian and Satterlie, 1996a). The large asymmetrical white cell (blue arrowhead in Figure 3C) found in the right pleural ganglia mediates egg laying (Norekian and Satterlie, 2001). The neurons of the pedal ganglia include the swim interneurons composing the Clione swim CPG and the swim motoneurons controlled by the CPG circuit. Swim interneurons and the synergistic swim motoneurons that control the dorsal flexion of the parapodia are designated by odd numbers while the swim interneurons and the synergistic swim motoneurons that control the ventral flexion of the parapodia are designated by even numbers. Swim interneurons include the dorsal swim interneurons, 7, and ventral swim interneurons, 8 (see also Figure 2). There are swim motoneurons with relatively small soma called small motoneurons labeled 1, 2, 3, and 4. These small motoneurons have restricted innervation fields and control only the slow-twitch swim muscle of the parapodia. Two neurons labeled 1A and 2A are large motoneurons (referred to as general excitor motoneurons; GEMN or GE) that have an expansive innervation field covering the entire parapodia and control the contraction of both slow-twitch and fast-twitch swim muscle fibers (Arshavsky et al., 1985b; Satterlie and Spencer, 1985; Satterlie, 1993). Located in the medial part of the pedal ganglia near the pedal commissure are a cluster of serotonergic neurons called Pd-SW neurons that control the contractile force of parapodial slow-twitch muscle fibers (Satterlie, 1995b; Plyler and Satterlie, 2020). A single asymmetric serotonergic neuron in the left pedal ganglia is the heart excitor neuron (HE) that controls the heartbeat (Figure 4A; Arshavsky et al., 1990; Satterlie et al., 1995; Malyshev et al., 2008). The remaining neurons labeled in the pedal ganglion are the neurons that control the wing withdrawal reflex when the parapodia (wings) receive tactile stimulation. These neurons include sensory neurons (S), wing-retraction interneurons (Ri), and wing-retraction motoneurons (Rm; Huang and Satterlie, 1990). Interneuron 13 is dopaminergic and mediates swim inhibition (Norekyan, 1989). The neuronal somas in the intestinal ganglia shown in Figure 3E control the heart rate and include heart excitors that increase the heart rate, Int-HE and Z cell, and heart inhibitors, Int-HI, that decrease heart rate (Arshavsky et al., 1990; Malyshev et al., 2008).
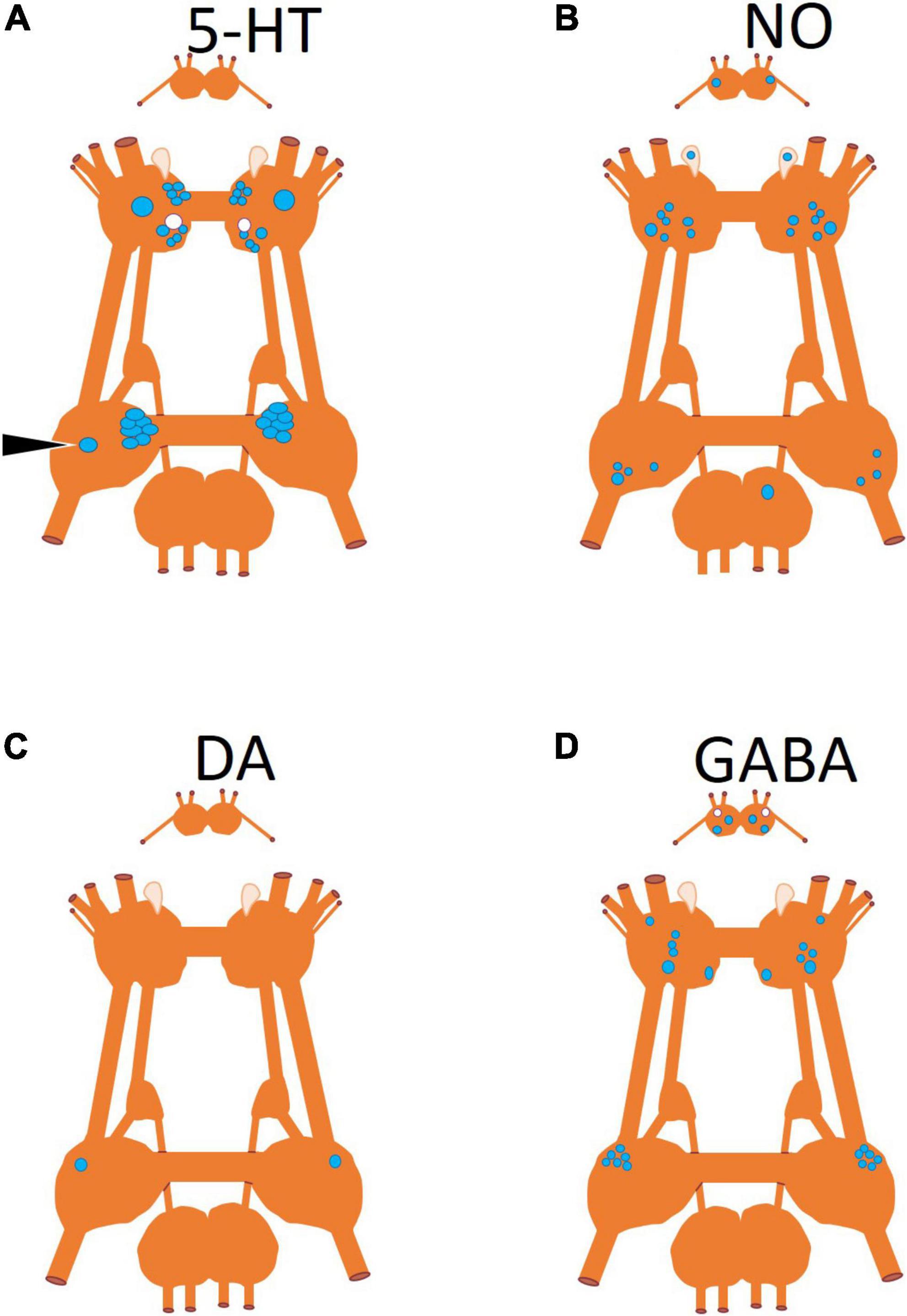
Figure 4. Neuronal somas mapped according to neurotransmitter produced by the neuron. (A) Serotonin (5-HT) identified by immunohistochemistry (Satterlie et al., 1995), (B) nitric oxide (NO) identified by nicotinamide adenine dinucleotide phosphate diaphorase (NADPH-d) histochemistry (Moroz et al., 2000), (C) dopamine identified through pharmacological antagonist blocking its physiological effect (Norekyan, 1989), and (D) gamma-aminobutyric acid (GABA) identified by immunohistochemistry (Norekian, 1999; Norekian and Malyshev, 2006). Somas that are blue are located on the dorsal surface of the ganglion and somas in white are located on the ventral surface of the ganglion.
In addition to the anatomical locations of neurons that control the behaviors of Clione, Figure 4 shows the neurotransmitters characteristic of these neurons (Figures 4A–D). The neurotransmitters, serotonin and GABA, were localized by immunohistochemistry (Norekian, 1999). Nitric oxide (NO) was localized using nicotinamide adenine dinucleotide phosphate diaphorase (NADPH-d) histochemistry (Moroz et al., 2000). The single pedal, neuron 13 (Figure 4) was identified as dopaminergic through pharmacological methods (Norekyan, 1989).
The neurons contained within the ganglia as described above were characterized by two different research groups—one in the United States of America (Satterlie and Norekian) and one in Russia (Arshavsky). Hence, the names of the same neurons are often different. Table 1 summarizes the names and other aspects of neurons to aid the reader in identifying the various names of neurons characterized by both Satterlie and Arshavsky research groups.
Two cellular properties of Clione swim interneurons that contribute to locomotor pattern generation are the sag potential and postinhibitory rebound (Pirtle and Satterlie, 2007, 2021; Pirtle et al., 2010). The sag potential, which relates to the current called Ih mediated by hyperpolarization-cyclic nucleotide-gated ion channels (HCN channels) will be addressed later in this review. Postinhibitory rebound is a depolarization that occurs following inhibition of swim interneurons. Thus, postinhibitory rebound plays a crucial role in locomotor pattern generation by allowing inhibited swim interneurons to quickly rebound to reach the threshold and produce an action potential (Satterlie, 1985; Arshavsky et al., 1993a; Satterlie et al., 2000; Pirtle and Satterlie, 2004, 2007). Figure 5 shows how postinhibitory rebound produced in a ventral swim interneuron by applying a−1 nA current injection can quickly bring about swimming in Clione during a state of swimming cessation. Following the −1 nA current injection, the ventral swim interneuron produces an action potential that in turn inhibits the dorsal interneurons. A simultaneous recording of membrane potential from the dorsal swim interneuron shows that it also generates a postinhibitory rebound-induced action potential that inhibits the ventral swim interneuron. This alternating pattern of activity continues as a brief bout of fictive swimming that lasts approximately 4 s.
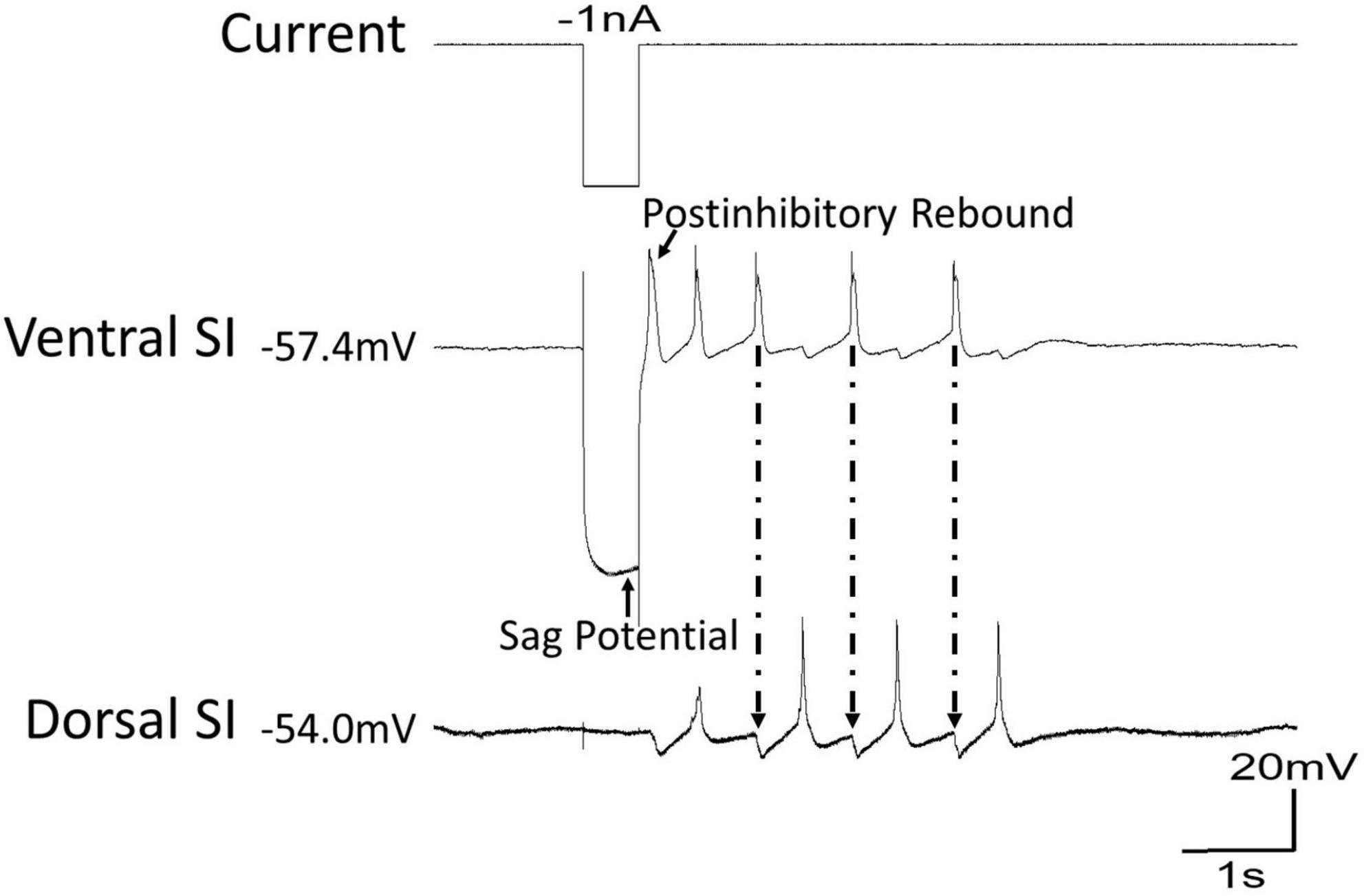
Figure 5. During a time of swim cessation, a hyperpolarizing current of –1 nA injected into the ventral swim interneuron of Clione evokes a sag potential during the hyperpolarization followed by a rebound in action potential activity creating a short bout of swimming. Arrows with dashed lines show the inhibitory postsynaptic potentials (IPSPs) in the dorsal swim interneuron produced by the action potentials of the ventral swim interneuron.
Several molluscan species have contributed to our understanding of locomotor CPGs and their modulation. Among these are Tritonia diomedea (Getting, 1981), Pleurobranchaea californica (Jing and Gillette, 1999), Aplysia brasiliana (Gamkrelidze et al., 1995), Melibe leonina (Thompson and Watson, 2005), and C. limacina (Arshavsky et al., 1985a,b,c; Satterlie, 1985; Satterlie and Spencer, 1985). All these molluscan species are useful model systems for elucidating mechanisms of locomotor control because, compared to the more complex vertebrate animals, they all have simple neural circuits and large re-identifiable (i.e., neurons that can be identified in the same ganglion location between individual animals) neurons whose electrical properties can be studied in reduced semi-intact preparations. Additionally, mollusks show complex behaviors related to locomotion within an environmental context.
Advantages among the molluscan species used as a model for studying locomotor CPGs stem from their differences in ecological niche and related behaviors. Clione is unique among the related mollusks used in the study of locomotor CPGs for two major reasons. First, Clione occupies the midwater realm of the ocean where it keeps its position within the water column by virtually constantly swimming (Mackie and Mills, 1983; Arshavsky et al., 1985a; Mackie, 1985; Satterlie et al., 1985). Clione begins to sink if it ceases to swim, and it is hypothesized that Clione migrates up and down in the water column (Mackie, 1985) through the modulation of swimming behavior—Clione moves upward towards the surface of the water column by swimming and moves down the water column by inhibiting its swimming behavior (Arshavsky et al., 1992; Satterlie et al., 1985). Because Clione typically keeps its position within the water column by hovering locomotion, it is virtually in a constant state of motion. Thus, one advantage of Clione is that there is little need to activate the swim CPG interneurons to produce fictive swimming behavior by virtue of the animal being in a state of near-continuous swimming (Satterlie et al., 1985). Second, Clione can change its locomotor speed in response to feeding and predator avoidance (Arshavsky et al., 1985a). Thus, Clione is an invaluable model system for assessing the mechanisms that underlie locomotor speed change. Much of the research done on the Clione swim CPG has focused on mechanisms contributing to swim acceleration at both the circuit-level and cellular level within the swim CPG (Arshavsky et al., 1989; Kabotyanskii and Sakharov, 1991; Satterlie, 1991; Norekian and Satterlie, 1996a; Panchin et al., 1996; Satterlie and Norekian, 1996; Satterlie et al., 2000; Pirtle and Satterlie, 2021).
Clione is holoplanktonic and, as previously said, lives in the midwater where it keeps its position in the water column by engaging in active swimming (Lalli, 1967; Mackie and Mills, 1983; Mackie, 1985; Lalli and Gilmer, 1989). However, Clione can stop and restart locomotion depending on behavioral contexts. For example, Clione will accelerate swimming when hunting and when escaping potential predators (Arshavsky et al., 1985a,1992; Satterlie and Spencer, 1985). Similarly, tactile stimuli to the animal’s anterior region or parapodia elicit a behavioral response that consists of retraction of the head, the parapodia, and the tail leading to inhibition of swimming—a “whole-body withdrawal” response (Norekian and Satterlie, 1996a). Therefore, neural circuitry that controls Clione swimming behavior must be malleable to alter the locomotor speed.
Animals, including Clione, can accelerate locomotor speed by increasing the rate of locomotor appendage displacement, increasing the applied force of the locomotor appendage, or a combination of both increasing the rate of locomotor appendage displacement and increasing the applied force of the locomotor appendage (Satterlie, 1995a,b). Additionally, Clione can alter their locomotor speed through mechanical changes in their parapodia that include changing the stiffness of the parapodia and changing the angle of attack of the parapodia (Szymik and Satterlie, 2011, 2017). Stimulation of cerebral serotonergic neurons in Clione accelerates swimming locomotion at the circuit level through reconfiguration of the animal’s CPG and recruitment of more neurons.
Stimulation of serotonergic neurons found in the anterior and posterior portions of the cerebral ganglia (Cr-SA and Cr-SP neurons, respectively) and a serotonergic neuron on the ventral surface of the cerebral ganglia (Cr-SV) in Clione modulates swimming behavior by increasing locomotor speed through circuit level and cellular level mechanisms (Panchin et al., 1995b,1996; Satterlie, 1995b; Satterlie et al., 1995; Satterlie and Norekian, 1995, 1996). From a non-modulated state of slow swimming, serotonergic modulation can initiate swim acceleration in Clione by one of three general mechanisms: (1) by increasing parapodial contractility without a change in the frequency of parapodial movement (peripheral modulation only), (2) by increasing the frequency of parapodial movement without a change in parapodial contractility (central modulation only), or (3) by increasing both the parapodial contractility and by increasing the frequency of parapodial movement (both peripheral and central modulation; Satterlie and Norekian, 1996).
Central and peripheral serotonergic modulation of Clione locomotor activity involves changes at the circuit and cellular levels. At the circuit level, serotonin reconfigures the CPG circuit by recruiting several neurons that include 1A and 2A motoneurons, also called general excitor motoneurons (GEMN), in the pedal ganglia, pedal serotonergic neurons (Pd-SW) in the pedal ganglia, type 12 interneurons in the pleural ganglia, and type 8e interneurons of the pedal ganglion (Satterlie and Norekian, 1996; Arshavsky et al., 1998). These circuit-level changes that recruit neurons are shown in Figures 6–10.
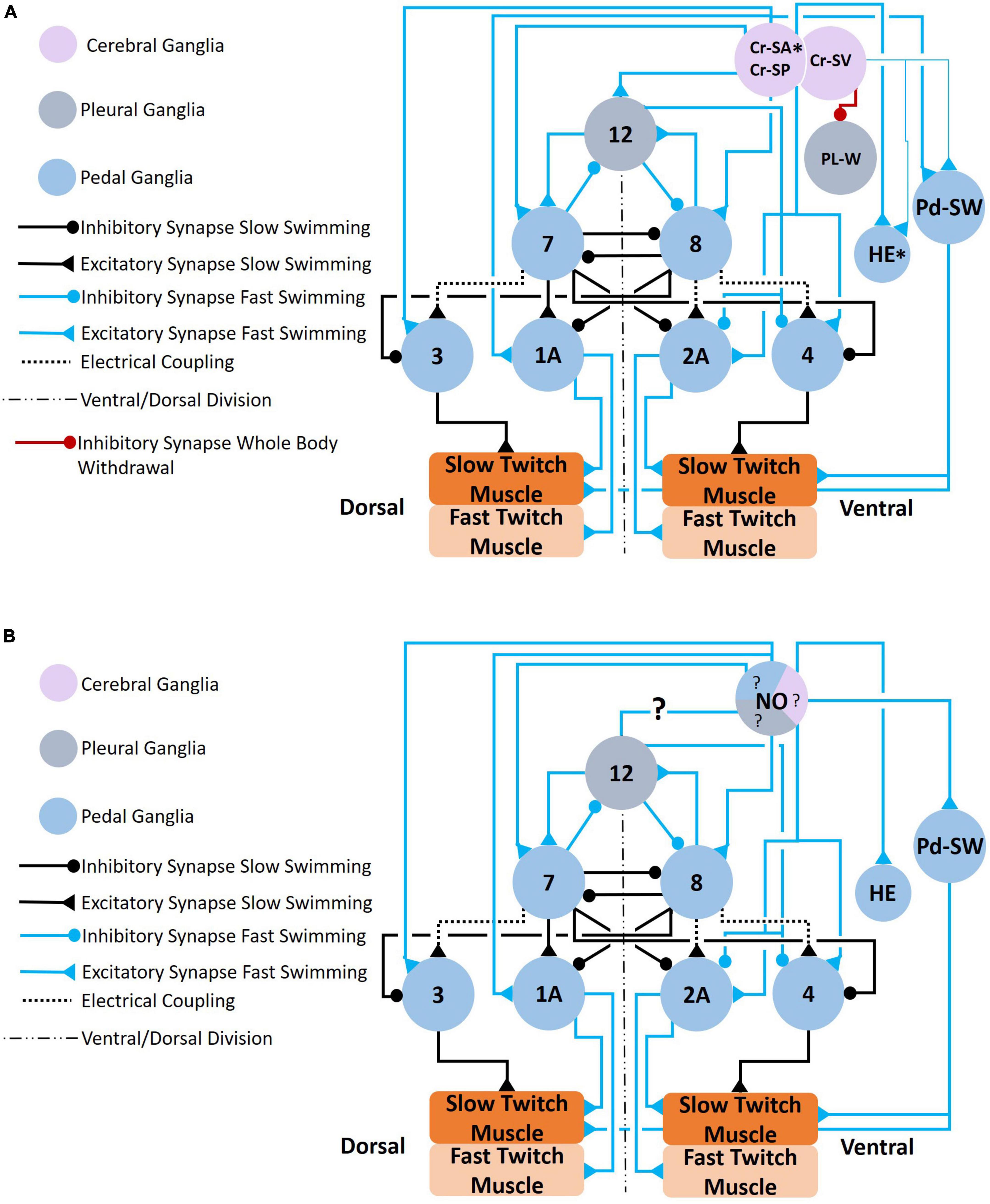
Figure 6. (A) Circuit mediating locomotor acceleration through the recruitment of neurons (blue connections). Three groups of cerebral serotonergic neurons, Cr-SA, Cr-SP, and Cr-SV, control recruitment of both interneurons and motoneurons in the swim system during swim acceleration. The Cr-SA and Cr-SP neurons (all on the dorsal surface of the cerebral ganglia) have overlapping functions—they recruit the type 12 interneuron into the Clione swim CPG, recruit large 1A and 2A (GEMNs), and recruit serotonergic neurons, Pd-SW neurons (that control force of contraction of slow-twitch swim muscle). The Cr-SA neurons are the only member of the Cr-SA/Cr-SP group to stimulate the asymmetric heart excitor (HE) neuron of the left pedal ganglia, which is indicated by the asterisk. The Cr-SV neuron is found on the ventral surface of the cerebral ganglia and weakly excite the HE and Pd-SW neurons as indicated by the thinner blue lines. The Cr-SV neuron also simultaneously inhibits the pleural withdrawal neurons, Pl-W, that participate in the competing whole-body withdrawal response. (B) Circuit mediating swim acceleration by NO. Nitrergic neurons are found in the cerebral (purple), pleural (gray), and pedal (blue) ganglia. However, it is not known which specific nitrergic neurons participate in swim acceleration. The responses of the elements of the swim system to NO were determined experimentally by bath application of NO-donors. The effect that NO has on the type 12 interneuron of the pleural ganglia has not been established (Norekian and Satterlie, 1996b,2001; Satterlie and Norekian, 1996; Moroz et al., 2000; Pirtle and Satterlie, 2021).
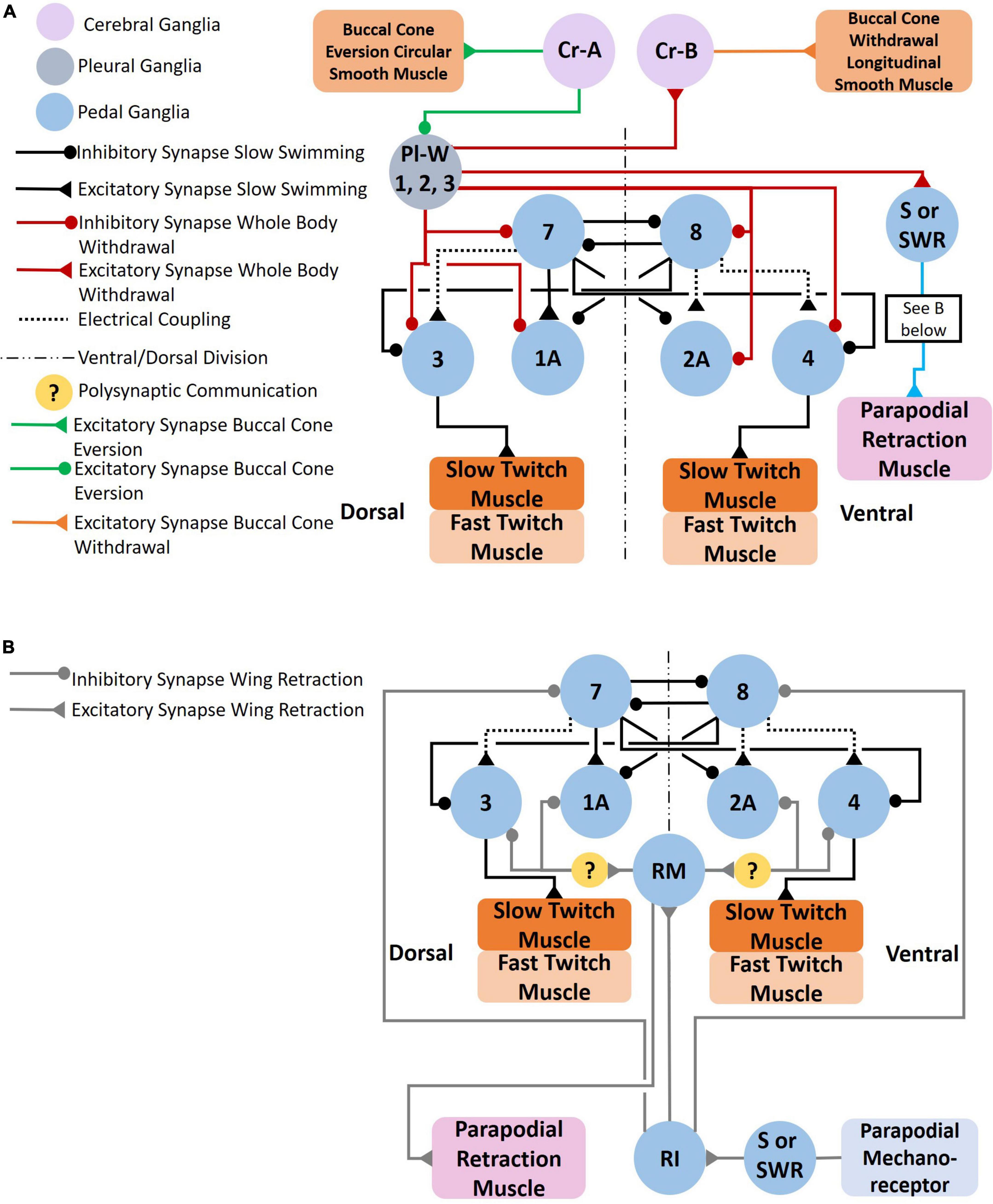
Figure 7. (A) Circuit mediating the Clione whole-body withdrawal response and buccal cone eversion and withdrawal. Stimulus to the head of Clione elicits the passive defensive withdrawal behavior through the activation of Pl-W neurons. Pl-W neurons in the pleural ganglia promote a passive defensive withdrawal behavior by inhibiting the swim system and promoting the withdrawal of buccal cones through the activation of Cr-B neurons that control the longitudinal smooth muscle of the buccal cones consequently leading to buccal cone withdrawal. The Pl-W neurons not only inhibit the swim CPG interneurons and swim motoneurons, but also work in concert with pedal neurons mediating the wing retraction reflex (B) by stimulating the wing mechanosensory neurons in the pedal ganglia (labeled S in Figure 3D) that mediate the wing retraction reflex. Furthermore, Pl-W neurons stimulate intestinal heart inhibitory neurons (Int-HI; see Figure 8) to inhibit the heartbeat. Feeding behavior overrides the withdrawal reflex. When feeding, Cr-A neurons simultaneously promote buccal cone eversion (see also Figures 9A, B) and inhibit Pl-W neurons (Norekyan, 1989; Huang and Satterlie, 1990; Norekian and Satterlie, 1996a; Malyshev et al., 2008).
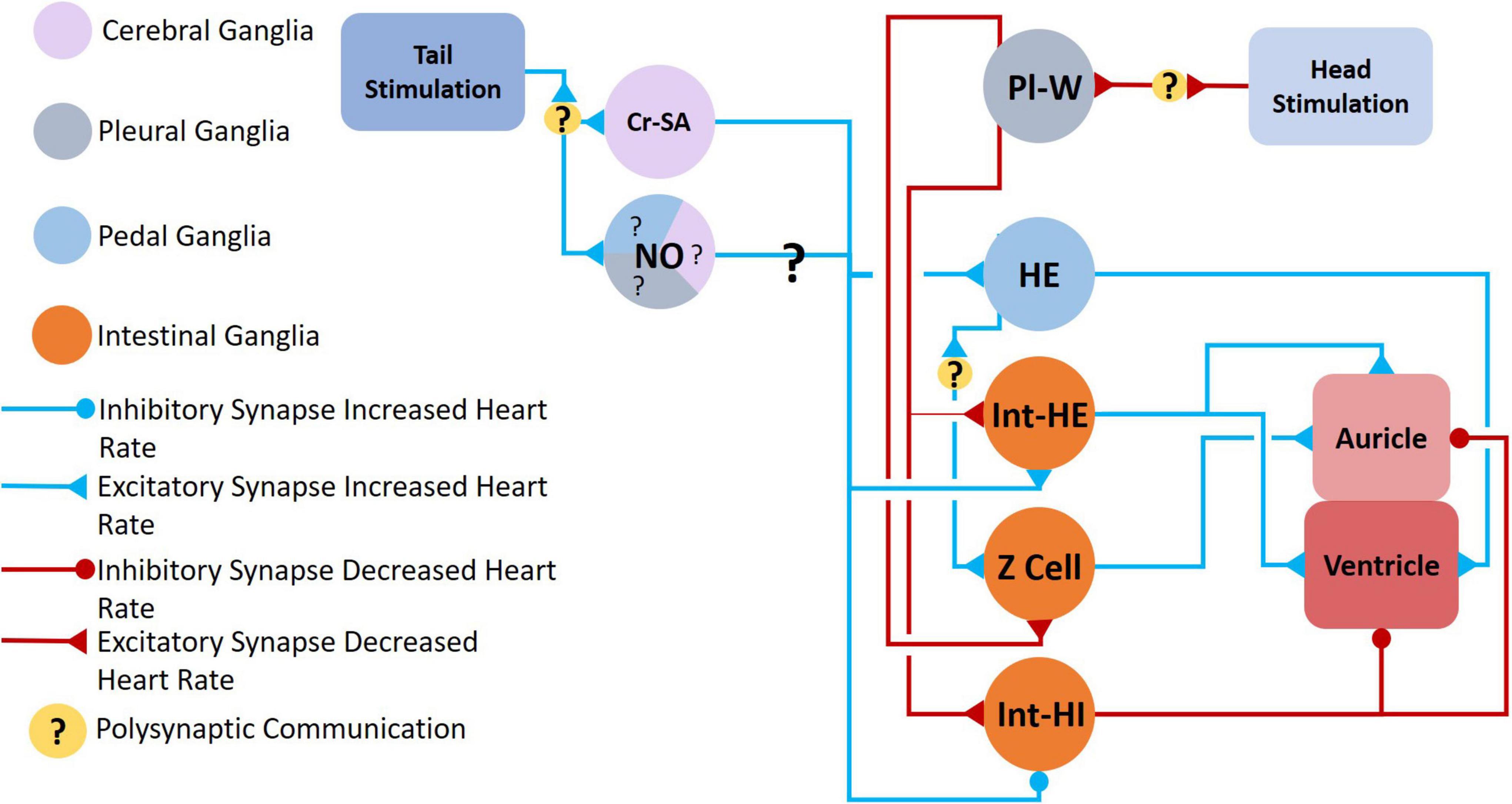
Figure 8. Neural circuit mediating changes in heartbeat. Clione heart rate increases during swim acceleration and is controlled by serotonergic Cr-SA neurons and NO, both of which also mediate swim acceleration. The exact nitrergic neurons involved is unknown (hence, the question marks—?—for NO). Tail stimulation accelerates swimming locomotion and increases heart rate through the excitation of serotonergic heart excitor (HE) neurons and neurons of the intestinal ganglia, Int-HE and Z cell. Head stimulation produces the opposite response—a decrease in heart rate—through stimulation of heart inhibitory neurons in the intestinal ganglia, Int-HI. Polysynaptic communication is indicated (Arshavsky et al., 1990; Satterlie and Norekian, 1995; Malyshev et al., 2008).
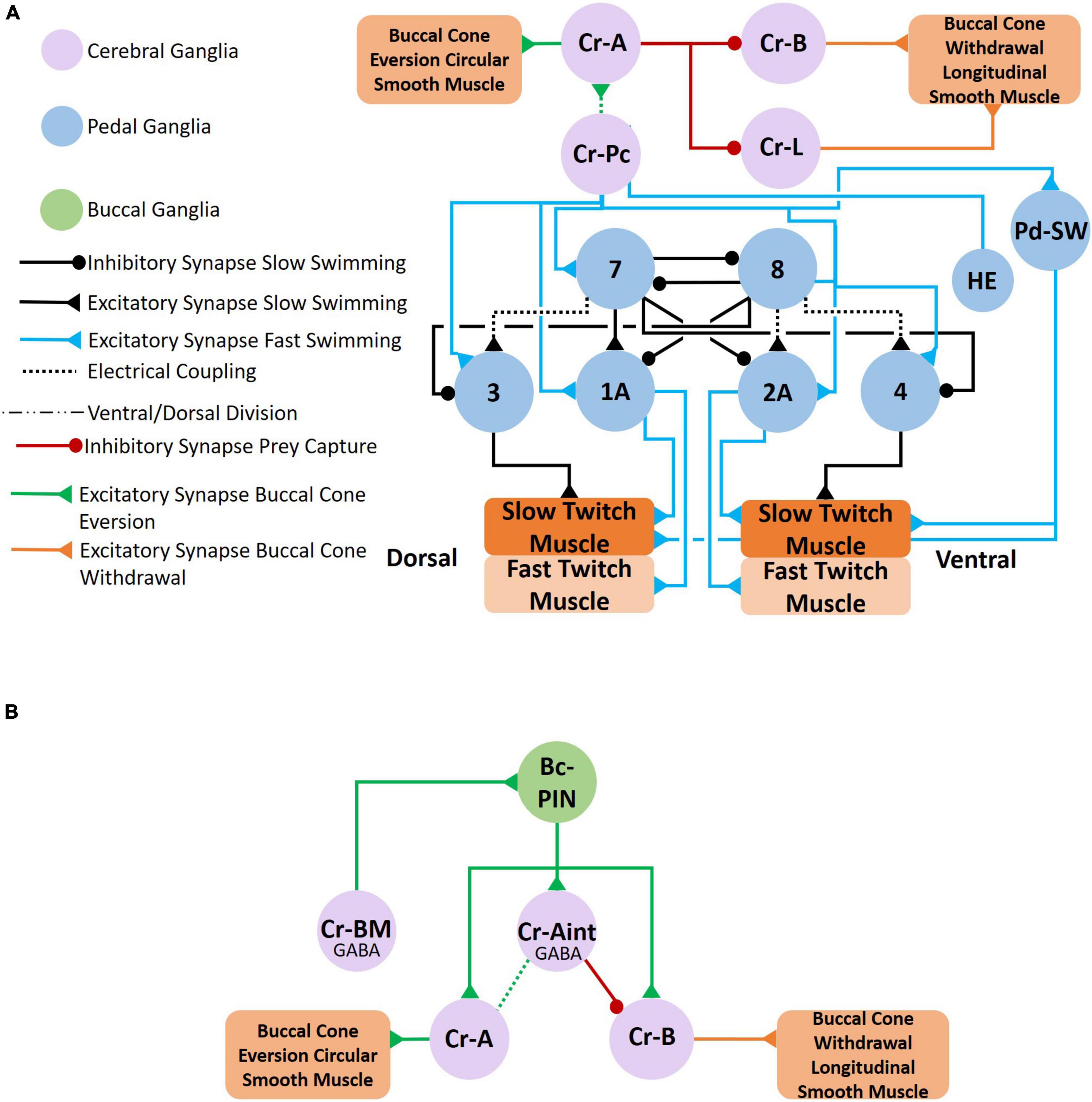
Figure 9. Feeding behavior is evoked when Clione encounters its prey, Limacina helicina. Feeding behavior involves the eversion of prey capture appendages—the buccal cones—and results in swim acceleration. (A) Cr-Pc (prey capture neurons) in the cerebral ganglia coordinate both feeding behavior and locomotor acceleration. First, Cr-Pc neurons activate Cr-A neurons through chemical synaptic communication and electrical coupling. The Cr-A neurons in turn activate circular smooth muscle in the buccal cones to facilitate buccal cone eversion during prey capture. Cr-Pc neurons also stimulate swim interneurons of the Clione swim CPG, stimulate motoneurons (including 1A and 2A motoneurons), Pd-SW neurons and the HE neuron. Thus, Cr-Pc neurons promote swim acceleration and increased heart rate, the later contributing to hydraulic inflation of the buccal cones during prey capture. In addition to promoting buccal cone eversion, Cr-A neurons simultaneously inhibit the competing buccal cone withdrawal by inhibiting Cr-B and Cr-L neurons that stimulate the buccal cone’s longitudinal smooth muscle fibers. (B) The cerebral GABAergic neurons, Cr-BM and Cr-Aint, mediate co-activation of motoneurons that control both buccal cone eversion and buccal cone withdrawal. Cr-BM excites the Bc-PIN interneuron of the buccal ganglia. The Bc-PIN in turn excites the Cr-Aint and simultaneously excites competing motoneurons, Cr-A motoneurons that control the buccal cone circular smooth muscle and promote buccal cone eversion and Cr-B motoneurons the control the buccal cone longitudinal smooth muscle and promote buccal cone withdrawal. Co-activation of Cr-A and Cr-B motoneurons occur during removal of the prey, L. helicina, from its shell (Arshavsky et al., 1993a,b; Norekian and Satterlie, 1993, 1995; Norekian, 1995; Norekian and Malyshev, 2005, 2006).
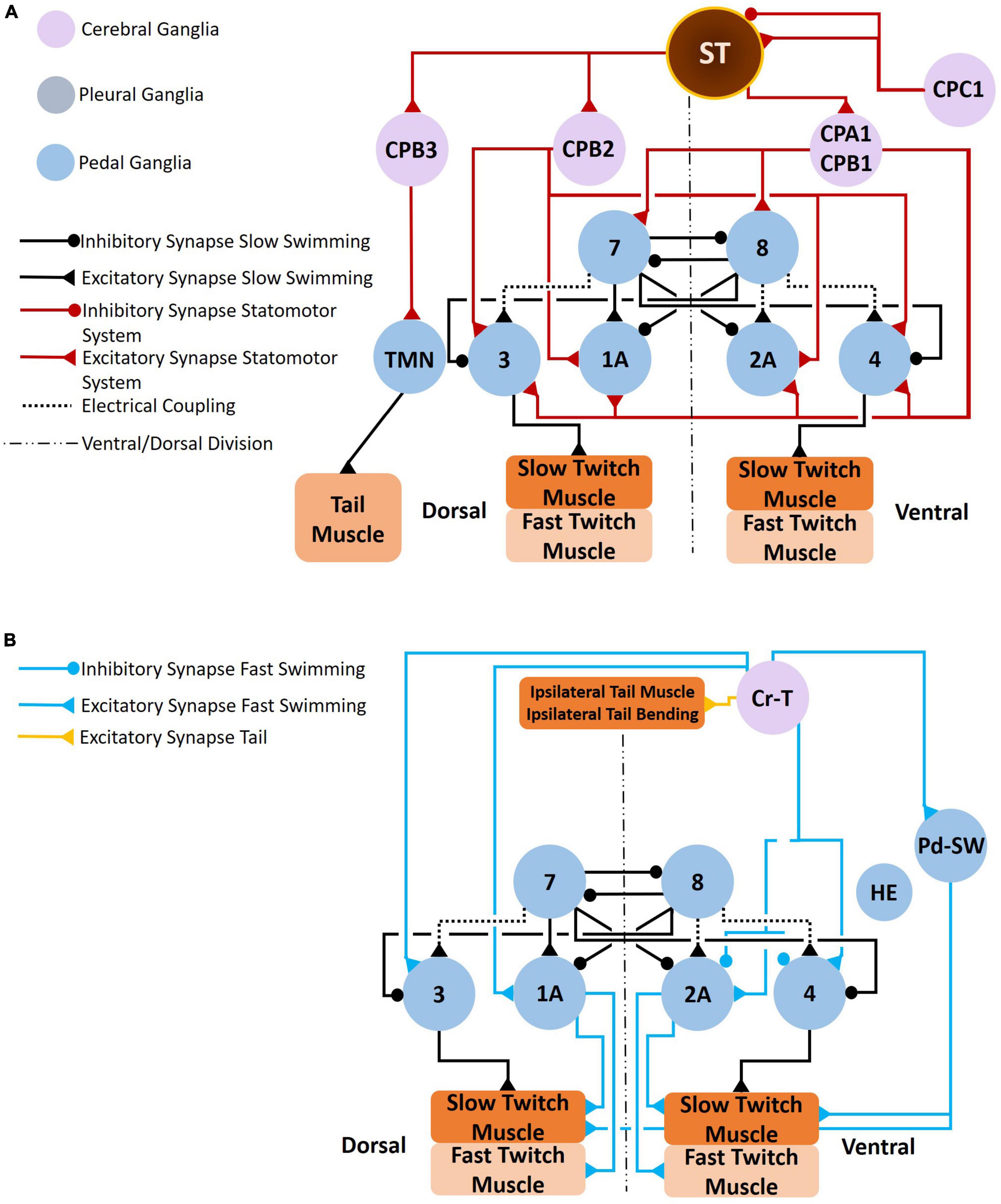
Figure 10. Clione postural responses, which involve tail movements, change in response to swim acceleration and feeding behavior. The circuit that coordinate tail movements to swim acceleration are illustrated in A,B. As shown in A, the statocyst receives input from both gravity and CPC1 neurons. CPC1 neurons have differential affects on the statocyst receptor cells (SRCs) depending on SRCs innervated. The SRCs of the statocysts send excitatory signals to CPA1/CPA2 neurons, CPB2 neurons, and CPB3 neurons. The CPA1/CPB1 neurons stimulate interneurons of the Clione locomotor CPG and stimulate swim motoneurons (including the large 1A and 2A motoneurons). The CPB2 neurons stimulate only motoneurons (including the large 1A and 2A motoneurons). Thus, the SRCs promote swim acceleration. Furthermore, SRCs stimulate CPB3 neurons that control tail motoneurons (TMN) that in turn control the bending of the Clione tail (T in Figure 1A). Tail bending acts like a rudder to steer Clione in circular and spiraling movements that are characteristic of feeding behavior. Coordinated tail bending is also achieved through the activation of Cr-T neurons (B). Cr-T neurons stimulate Pd-SW neurons to enhance the force of parapodial contraction, stimulate motoneurons (including the large 1A and 2A motoneurons), and stimulate the tail musculature to bend the tail in a coordinated fashion (Norekian, 1995; Panchin et al., 1995a,b,c).
Circuit-level reconfiguration of the Clione swim system: Type 1A and 2A motoneurons (general excitor motoneurons), pedal serotonergic neurons, and type 12 interneurons
Stimulation of cerebral serotonergic neurons, Cr-SA and Cr-SP neurons (Figure 6A) reconfigures the Clione swim system by recruiting large motoneurons 1A and 2A (GEMN) motoneurons in the pedal ganglia (Arshavsky et al., 1985b; Satterlie, 1993; Norekian and Satterlie, 1996b,2001). These large motoneurons (80 μm diameter somas) innervate the entire parapodial muscular system—both slow-twitch fatigue resistant and fast-twitch fatigable muscle fibers—via the wing nerve. These large GEMNs contrast with the small motoneurons that innervate limited areas of the parapodial muscular system and only innervate the slow-twitch muscle fibers of the parapodia. Thus, when active, GEMNs increase the frequency and contractility of both the slow-twitch muscle and fast-twitch muscle fibers throughout the entire parapodia to increase the locomotor speed of Clione (Satterlie, 1993, 1995a).
Anterior and posterior portions of the cerebral ganglia excite the Pd-SW serotonergic neurons in the pedal ganglia and the asymmetric HE neuron in the left pedal ganglia. Additionally, Cr-V neurons weakly stimulate Pd-SW and HE neurons while inhibiting Pl-W neurons that will be described later (Figure 6A; Norekian and Satterlie, 1996b,2001). Both the right and the left pedal ganglia have in their antero-medial regions an agglomeration of serotonergic neurons called Pd-SW neurons (Satterlie, 1995b). Plyler and Satterlie (2020) describe each group of Pd-SW neurons as consisting of 5–9 somas that range from 20 to 80 μm with no difference in number of somas in the right and the left pedal ganglia. Furthermore, Plyler and Satterlie (2020) describe two types of Pd-SW neurons that show different innervation patterns and electrophysiological properties. These findings suggest that one group of Pd-SW neurons innervates the slow-twitch muscles fibers of the parapodia via the wing-nerve and plays a role in increasing locomotor speed by increasing the force of parapodial contractions during either slow swimming or fast swimming modes.
Stimulation of Cr-SA and Cr-SP evokes plateau potentials in type 12 interneurons that are in the pleural ganglia. A simultaneous recording of swim interneuron and type 12 interneuron during slow fictive swimming is shown in Figure 3. There is one type 12 interneuron soma in each of the two pleural ganglia whose axons exit each pleural ganglia via the pleural-pedal connective entering the ipsilateral pedal ganglia and traversing to the contralateral pedal ganglia via the pedal commissure. Type 12 interneurons are functionally incorporated into the Clione swim CPG when stimulated to activity by cerebral serotonergic neurons. Thus, the inclusion of type 12 interneurons into the CPG is one of the types of circuit-level reconfiguration that occurs to change the swimming locomotor speed in Clione (Figure 6A; Arshavsky et al., 1985d,1989; Satterlie and Norekian, 1995, 1996; Pirtle and Satterlie, 2006).
The morphology of the type 12 interneuron in relationship to the anatomy of the ganglia has permitted Pirtle and Satterlie (2006) to show the relative contribution of the type 12 interneuron to the acceleration of Clione swimming. In these experiments, the pleural-pedal connective was cut and the dissected preparation of Clione (consisting of the isolated ganglia and parapodia) was subjected to a 48-hour refrigerated (4–6°C) incubation period. Thus, these experiments effectively prevent the contribution of the type 12 interneurons from influencing the locomotor CPG and therefore swim acceleration through feedback inhibition. Pirtle and Satterlie (2006) show that the type 12 interneurons cannot, by themselves, produce the swim acceleration of Clione, but work simultaneously with cellular changes to swim interneurons and swim motoneurons that are produced by serotonin.
Experiments by Pirtle and Satterlie (2006) suggest that type 12 interneurons may contribute to locomotor speed in several ways. First, type 12 interneurons may help produce swimming stability by enhancing synaptic communication among the CPG interneurons. Several cellular changes occur to swim CPG interneurons. One of these cellular changes is a decrease in the duration of swim CPG interneurons, also referred to as spike narrowing (Satterlie et al., 2000). This spike narrowing is hypothesized to reduce the synaptic efficacy among the swim CPG swim interneurons. Thus, one of the possible roles of type 12 interneurons is to supply enhanced synaptic efficacy to counter the loss of such synaptic efficacy because of spike narrowing of the component swim interneurons of the Clione swim CPG.
Second, the feedback provided by type 12 interneurons may be a source of parapodial synchronization during fast swimming in Clione. As previously shown, the type 12 interneurons extend their axon through the pleural-pedal connective to the ipsilateral pedal ganglia. However, an extension of the type 12 interneuron axon also crosses to the opposite side via the pedal commissure to the contralateral pedal ganglia. Thus, a type 12 interneuron on one side will synaptically communicate with CPG swim interneurons found in both the right and left pedal ganglia. Therefore, the type 12 interneurons may influence the timing of swim interneuron activity by causing the right and left swim interneuron activity to better coincide in time. Furthermore, active plateau potentials of type 12 interneurons only reduce the cycle period of Clione CPG interneuron firing between the second to the third and between the third to the fourth cycle of the swim rhythm. Thus, the role of the type 12 interneurons may be to boost locomotor acceleration prior to the contribution of serotonergic alteration of endogenous cellular properties of the swim interneurons (Pirtle and Satterlie, 2006).
The effect of the nitrergic system on swimming locomotor regulation is shown in Figure 6B. NO has been shown (through bath application of NO donors) to activate many of the same neural elements of the swim system including swim interneurons, swim motoneurons, and Pd-SW neurons (Moroz et al., 2000; Pirtle and Satterlie, 2021). However, the effect of NO on type 12 neurons and their recruitment into the swim CPG has not been demonstrated—hence the ?s in Figure 6B. There are several locations for nitrergic neurons in the ganglia of Clione illustrated in Figure 4B. However, the exact nitrergic neurons that stimulate Pd-SW neurons remains unknown.
The serotonergic and nitrergic neurons accelerate Clione swimming locomotion. On the other hand, the Pl-W neurons (Norekian and Satterlie, 1996a), pedal swim inhibitory neuron 13 (Norekyan, 1989), and the wing retraction reflex (Huang and Satterlie, 1990), inhibit swimming locomotion (Figures 7A, B). Also shown in Figure 7A are the Cr-A neurons that promote buccal cone eversion during prey capture by inhibiting Pl-W neurons. The Pl-W neuron prevents the extrusion of the BC—appendages that engage in prey capture during fast swimming—and promote their withdrawal by stimulating Cr-B neurons (Figure 7A). Dopamine has been shown to inhibit swimming locomotion in Clione (Kabotyanski and Sakharov, 1988), but the only confirmed dopaminergic neuron mediating withdrawal and hence swim inhibition is cell 13 in the pedal ganglion (Figures 3D, 4C; Norekyan, 1989). Additionally, Huang and Satterlie (1990), describe a wing retraction reflex in which tactile stimuli to the parapodia evoke a parapodial (wing) retraction and inhibit swimming (Figures 3D, 7B).
Swim acceleration and whole-body withdrawal/wing retraction are competing behaviors—when one behavior is active the other behavior is suppressed. Thus, swim acceleration and whole-body withdrawal/wing retraction are said to be “mutually exclusive” (Norekian and Satterlie, 1996a). Additionally, Norekian and Satterlie (1996a) describe the Clione swim and passive defensive withdrawal behaviors in terms of a hierarchy in which one behavior takes precedence over the other. In their analysis, slow swimming represents the base behavior and whole-body withdrawal supersedes slow swimming. On the other hand, swim acceleration as occurs during hunting and feeding, supersedes the whole-body withdrawal behavior. Thus, the whole-body withdrawal circuit inhibits the swimming circuit during slow swimming. Contrastingly, hunting and feeding behavior excites the swim circuit while simultaneously inhibiting the whole-body withdrawal circuit. Figure 6A shows the serotonergic Cr-SV neurons inhibit the Pl-W neurons during swim acceleration (Norekian and Satterlie, 1996a,b; Satterlie and Norekian, 2001).
Reconfiguration of the swim CPG and recruitment of neurons during the swim acceleration also activates circuits regulating the heartbeat, feeding apparatus, and tail and statomotor system
The heartbeat of Clione increases when tactile stimuli are applied to the tail and is directly influenced by neurons of the pedal and intestinal ganglia (Arshavsky et al., 1990; Malyshev et al., 2008). When engaged in fast swimming, a circuit of neurons favors an increase in the heart rate (Figure 8). The Cr-SA serotonergic neurons contribute to an increase in heart rate by exciting the asymmetric heart excitor (HE) neuron found in the dorsal surface of the left pedal ganglion. HE in turn stimulates contraction of the ventricle (Satterlie and Norekian, 1995; Malyshev et al., 2008). The HE neuron also stimulates the Z cell found in the left intestinal ganglia, and the Z cell subsequently stimulates auricle contraction. Another finding by Malyshev et al. (2008) is that tail stimulation excites intestinal heart excitor neurons (Int-HE neurons) and these neurons stimulate the contraction of both the ventricle and the auricle. While NO stimulates the HE neuron (Moroz et al., 2000) it is unknown which nitrergic neurons participate in this response (Figures 4B, 6B).
Decreased heart rate is favored when the Pl-W neurons of the pleural ganglion’s whole-body withdrawal system excites Int-HI neurons. The Int-HI neurons subsequently inhibit both ventricular and auricle contraction (Malyshev et al., 2008). A somewhat contradictory finding by Malyshev et al. (2008) is that the Pl-W neurons weakly stimulate Int-HE cells and Z cells. Malyshev et al. (2008) Suggest that this action of Pl-W neurons is to help prime the heart (i.e., “fill the ventricle”) so that upon resumed swimming the heart is poised to efficiently “re-inflate” the flaccid Clione body.
Clione has an open circulatory system and a hydrostatic skeleton. The increased heart rate that occurs during tail stimulation or during feeding may contribute to swim acceleration and facilitate hydraulic inflation of the BC (Arshavsky et al., 1990). Szymik and Satterlie (2017) have shown that fluid in Clione’s hemocoel is distributed to the parapodia and head and that constriction of the neck of Clione occurs during buccal cone eversion. Additionally, the distribution of hemocoelic fluid to the wings may control wing stiffness to contribute to swimming speed (Szymik and Satterlie, 2011).
The coordination of locomotor acceleration and feeding is accomplished in part by a cerebral neuron referred to as a cerebral prey capture neuron (Cr-Pc; Norekian and Satterlie, 1995) illustrated in Figures 9A, B. Cr-Pc neurons excite the swim system, heart, and neurons that control buccal cone eversion. Thus, Cr-Pc neurons coordinate locomotor and feeding circuits when Clione is engaged in feeding on its prey, L. helicina. As already mentioned, coordination of locomotion and heartbeat are important aspects of feeding behavior. In addition to activating elements of Clione’s swim system and the HE neuron, Cr-Pc neurons are responsible for controlling the circular smooth muscle in the BC that promote buccal cone eversion while simultaneously inhibiting neurons Cr-B and Cr-L that control buccal cone withdrawal (Figure 9A; Norekian and Satterlie, 1995). The antagonistic neurons, Cr-A and Cr-B, that produce opposite effects on the BC are also coordinated during feeding such that both neurons are activated simultaneously during the late phase of feeding behavior. This coordination is achieved by the recruitment of the GABAergic Cr-Aint neurons as illustrated in Figure 9B (Norekian and Satterlie, 1993; Norekian and Malyshev, 2006).
In the circuit illustrated in Figure 9B, GABAergic Cr-BM neurons mediate the co-activation of both Cr-A and Cr-B neurons through a buccal ganglion interneuron, Bc-PIN. The GABAergic Cr-BM neurons excite Bc-PIN interneurons that then communicate with three neurons in the cerebral ganglion that control buccal cone eversion and withdrawal—the Cr-Aint, Cr-A and Cr-B neurons. The Bc-PIN interneurons are the linkage that co-activates both Cr-A and Cr-B neurons during feeding behavior. Functionally, the recruitment of Cr-BM neurons may allow differential movement of the BC during different phases of feeding behavior (Norekian and Malyshev, 2006; Norekian et al., 2019).
During slow swimming, Clione maintains a relatively stable vertical position in the water column. However, during feeding Clione may make circular and spiraling movements as it hunts and captures its prey. Therefore, reconfiguration of the Clione swim system also coordinates the swim system to tail movements that control the posture of Clione. Control of tail movements has been described by Norekian (1995) and Panchin et al. (1995a). The circuits controlling the tail movements of Clione are illustrated in Figures 10A, B.
A key component of postural control in Clione are the paired statocysts that reside on the dorsal surface of the pedal ganglia. The statocysts have been described by Tsirulis (1974) and consist of 9–11 receptor cells (statocyst receptor cells, SRCs) forming a spherical structure with a cavity in which resides a statolith. The SRCs respond to gravity and receive differential input from CPC1 neurons located in the cerebral ganglia. Some SRCs receive excitatory input from CPC1 neurons while others receive inhibitory input from CPC1 neurons (Figure 10A). Panchin et al., 1995a,b propose that the differential inputs to SRCs from CPC1 neurons modify the output of SRCs during behavioral responses. The SRCs excite several cerebral neurons that control swimming and movement of the tail—these include the CPA1/CPB1, CPB2, and CPB3 neurons.
CPA1/CPB1 neurons are likely serotonergic (Panchin et al., 1995b) and correspond to Cr-SA and Cr-SP neurons respectively as described by Satterlie and Norekian (1995). The CPA1/CPB1 neurons excite both swim interneurons composing the swim CPG and swim motoneurons. Thus, SRCs can alter the locomotor speed of Clione through this part of the statocyst circuit. CPB2 neurons are excited by the SRCs and in turn excite swim motoneurons. The CPB3 neurons are excited by the SRCs and control tail movement through the excitation of tail motoneurons in the pedal ganglion (Figure 10A; Panchin et al., 1995a).
In addition to the neurons described above by Panchin et al. (1995a), there are neurons identified by Norekian (1995) that control Clione tail movements. These neurons are designated cerebral T neurons (Cr-T in Figure 10B). Cr-T neurons occur in pairs on the ventral side in each of the cerebral ganglia (both right and left cerebral ganglia). One member of the Cr-T pair, Cr-T1, has a soma of 80 μm and is positioned near the emergence of the cerebral commissure while the other member of the Cr-T pair, Cr-T2, has a soma of 60 μm and is positioned immediately anterior to Cr-T1. The axons of Cr-T1 and Cr-T2 neurons ultimately exit the ipsilateral pedal ganglion to innervate the ipsilateral dorsolateral tail muscles in the body wall.
Stimulation of Cr-T1 and Cr-T2 neurons produce tail bending in a coordinated fashion. When a single Cr-T1 neuron is stimulated it will produce ipsilateral bending of the tail such that the tail bends to the ipsilateral direction. However, when a left and right Cr-T1 neurons are stimulated together, the tail bends in a dorsal direction without deviating from the midline of the long body axis. There are no synaptic connections between Cr-T neurons and the neurons previously described that control buccal cone eversion (i.e., Cr-A neurons). Currently, there is also no evidence that the Cr-T neurons interact with SRCs or the other neurons described in Figure 10A. However, Cr-T neurons do monosynaptically excite swim motoneurons (both small motoneuron and large 1A/2A, general excitor motoneurons; Norekian, 1995).
Nitric oxide participates in locomotor and feeding circuits and excites the HE neuron to affect heart rate (Moroz et al., 2000). Additionally, NO may participate in the statomotor system (Panchin et al., 1995c; Moroz et al., 2000). However, the exact role that the specific nitrergic neurons play in modulation of locomotor behavior, feeding behavior, heartbeat regulation, and body orientation in Clione remains unclear—hence the question marks in Figure 6B that indicates that it is currently unknown what nitrergic neurons are involved. Nitrergic modulation at the circuit level may be a redundant system to that of serotonin because serotonergic neurons participate (e.g., Cr-SA and CPA1/CPB1 neurons). On the other hand, nitrergic modulation may work in separately or more subtly in combination with the serotonergic system in controlling locomotor speed, heartbeat, feeding, and body orientation at the circuit level. Further research of the circuit level role of NO is required to confirm these hypotheses.
Cellular mechanisms and ion channels modulating Clione locomotor speed
Neuromodulation involves the release of substances (i.e., neuromodulators) that change ongoing activity in neural circuits such that the output of the neural circuit is changed. Neuromodulation may affect synaptic communication among neurons forming a CPG, may affect the electrical characteristics of neurons forming a CPG, or may affect both synaptic communication and electrical characteristics of neurons forming a CPG. The change in neural circuit output commonly results when the neuromodulator binds to a receptor of one or more neurons in the circuit to start a signal transduction cascade in these target neurons that ultimately change their synaptic communication, electrical properties, or both synaptic communication and electrical properties. Neuromodulation may involve enhancement or depression of the neural circuit output to adjust behavior to suit changing environmental conditions (Kaczmarek and Levitan, 1987; Marder and Thirumalai, 2002; Dickinson, 2006; Harris-Warrick, 2011; Bucher and Marder, 2013; Nadim and Bucher, 2014; Golowasch, 2019). Moreover, the complexity and diversity of neuromodulatory effects may involve interconnected elements that are extrinsic to the CPG circuitry or that are an intrinsic part of the CPG circuitry (Katz, 1998).
In Clione, serotonin and NO are two neuromodulator substances that enhance swim CPG output and thus contribute to locomotor acceleration. Both serotonin and NO are extrinsic to the Clione swim CPG. Furthermore, both serotonin and NO modulate Clione swimming locomotion by eliciting changes in swim interneurons comprising the Clione swim CPG circuit (Satterlie and Norekian, 1995; Moroz et al., 2000; Satterlie et al., 2000). Nitric oxide’s ability to alter Clione swimming locomotion is mediated by soluble guanylyl cyclase (sGC), the production of cyclic guanosine monophosphate (cGMP) by sGC, and the activation of cGMP-dependent protein kinase activity (Pirtle and Satterlie, 2021). Less is known about the signal transduction mechanisms that mediate the similar acceleration of swimming locomotion by serotonin. However, Pirtle and Satterlie (2021) have shown that the ability of serotonin to increase Clione locomotor speed is not dependent on cGMP while the effect of NO on increasing locomotor speed is dependent on cGMP.
Both serotonin and NO-cGMP signaling cause the acceleration of Clione swimming through a common set of cellular mechanisms that involve modification of the electrical properties of swim interneurons. These cellular mechanisms include (1) spike-narrowing, (2) baseline depolarization, (3) an enhanced sag potential, and (4) enhanced postinhibitory rebound (Satterlie and Norekian, 1995, 2001; Satterlie et al., 2000; Pirtle and Satterlie, 2021). These cellular mechanisms are dependent on the involvement of ion channels and their corresponding ion conductance. Knowledge of ion channel operation within CPG circuits is essential for understanding how CPGs create rhythmic output and how this rhythmic output is modulated (Grillner, 1999; Brocard, 2019). The ion channels that are involved in the Clione swim CPG have been described with some difficulty.
The ionic currents characteristic of Clione swim CPG interneurons is challenging to study for several reasons. First, these swim interneurons must be found electrophysiologically by impaling them with microelectrodes. A second problem arises because the electrical coupling between other synergistic swim interneurons and between synergistic motoneurons creates space clamp issues when recording membrane currents of swim interneurons in single electrode voltage clamp experiments. To overcome the space clamp problem, an electrically identified swim interneuron must be physically isolated by using the recording electrode to pull the identified swim CPG interneuron out of the pedal ganglia. This isolation process is done by slowly moving the microelectrode impaling the swim CPG interneuron via a micromanipulator to gradually wrench the interneuron soma away from surrounding cells within the ganglia without rupturing its plasma membrane (Figure 11).
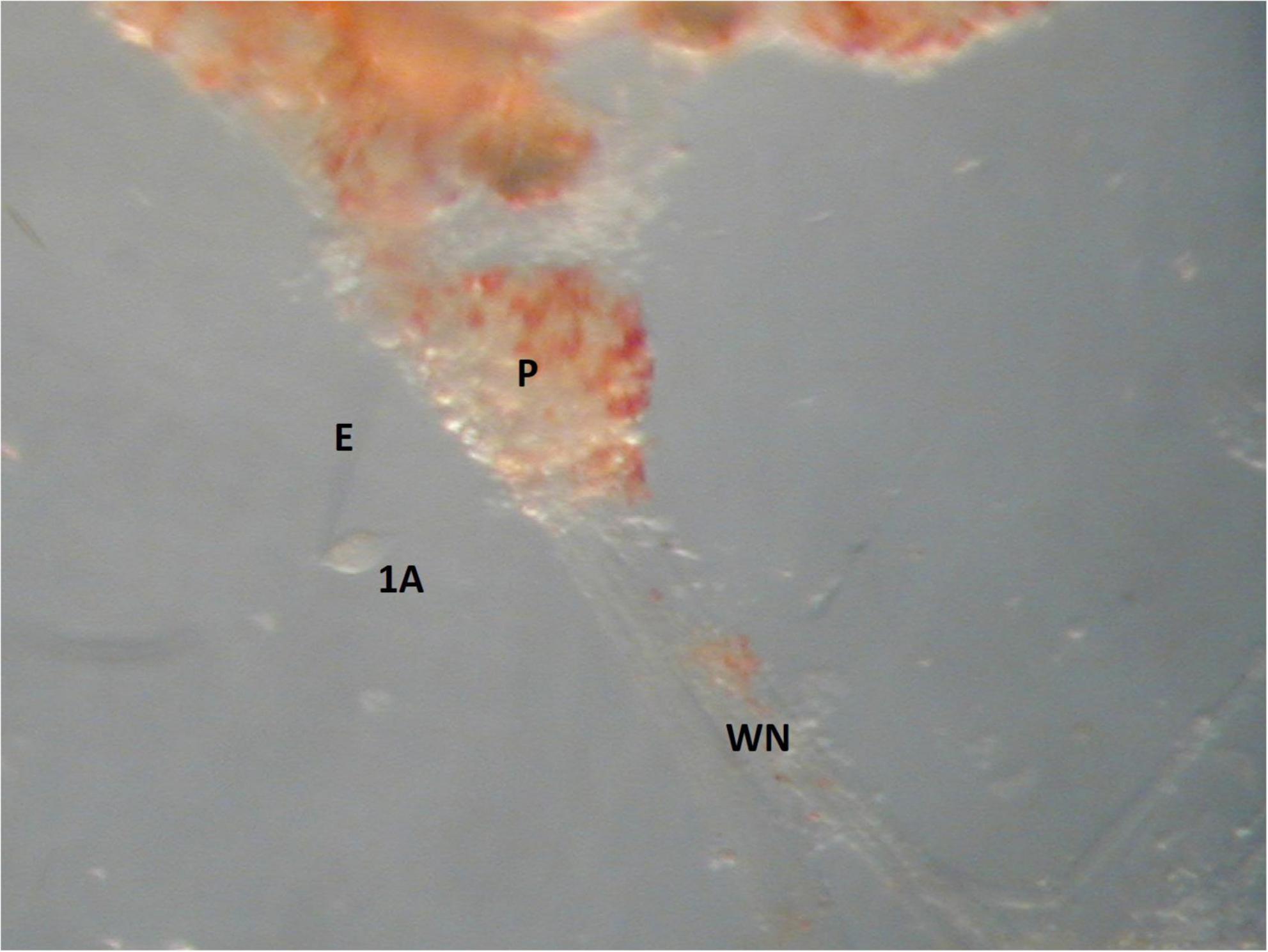
Figure 11. An image of the cell isolation method used in single electrode voltage clamp experiments. The soma of a large motoneuron, 1A, is isolated by the recording electrode E. The width of the 1A motoneuron is 30 μm and the width of the pedal ganglia, P, is 350 μm.
Ionic currents have been characterized in Clione swim interneurons using this method of physically isolating the swim interneuron in single-electrode voltage clamp experiments (Pirtle and Satterlie, 2004). Figure 12A shows the intracellular recording of a type 7 swim interneuron before isolation. One current of particular interest is the hyperpolarization-activated cyclic nucleotide-gated cation current, Ih, mediated by HCN ion channels). Ih is evoked in single electrode voltage clamp experiments with hyperpolarizing voltage steps that are applied from a holding potential of −40 mV. Ih is characterized by a slowly developing inward current that is mediated by an increase in both Na+ and K+ conductance (Pape, 1996; Lüthi and McCormick, 1998). In intracellular—or current clamp—recordings, the Ih current manifests as a slow depolarizing drift in the membrane potential during the application of hyperpolarizing current injection. This slow depolarizing drift in membrane potential is called a sag potential. Much of the information about Ih in Clione swim interneurons is inferred from changes in the sag potential produced by applying hyperpolarizing current to these cells during the intracellular recording of membrane potential in these cells (Figures 12B, C; Pirtle et al., 2010; Pirtle and Satterlie, 2021).
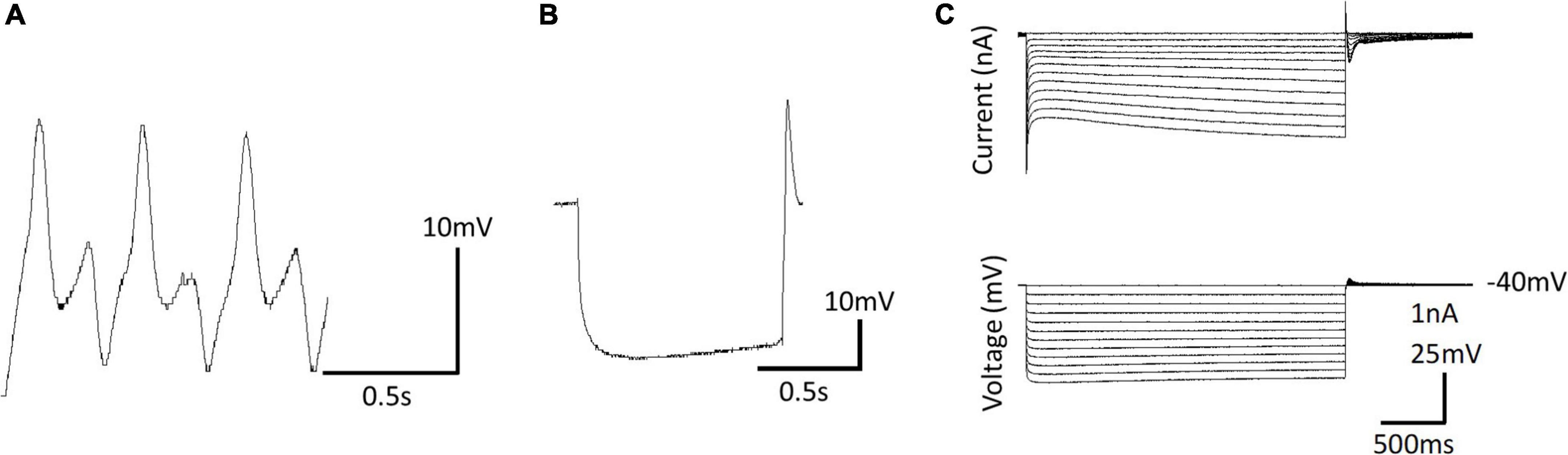
Figure 12. (A) Intracellular recording of a dorsal swim interneuron (type 7 swim interneuron) before physically isolating the cell. (B) Postinhibitory rebound and sag potential evoked by injecting a –1 nA, 1 s duration current pulse into the isolated cell identified in A. (C) Single electrode voltage clamp of the isolated cell (A) showing the development of slow inward current evoked by hyperpolarizing voltage steps from a holding potential of –40 mV—the hyperpolarization-activated cyclic nucleotide-gated inward current, Ih.
Intracellular recording of the sag potential requires isolating cells, not because of space clamp issues as associated with single electrode voltage clamp, but because these cells receive synaptic input that interferes with the recording of individual cellular properties of swim interneurons. Synaptic blockade using the muscarinic acetylcholine receptor antagonists, atropine, the glutamate receptor antagonist, 6-cyano-7-nitroquinoxaline-2,3-dione disodium (CNQX), and the sodium ion channel blocker, tetrodotoxin is used to thus chemically isolate Clione swim interneurons for intracellular recordings (Pirtle and Satterlie, 2004). The sag potential of swim interneurons can change with the application of the neuromodulator serotonin and the second messenger, cGMP. The addition of serotonin or cGMP increases fictive swim frequency as recorded intracellular from Clione swim CPG interneurons. Furthermore, serotonin and cGMP enhance the sag potential of Clione swim CPG interneurons by making the trajectory of the sag potential reach a more depolarized level faster. Thus, the enhancement of the sag contributes to increased frequency of swim interneuron action potentials and therefore an increase of the locomotor speed of Clione (Pirtle et al., 2010; Pirtle and Satterlie, 2021).
Because both serotonin and NO contribute to the enhancement of the sag potential, both serotonergic and nitrergic modulatory inputs converge to affect the activity of a common ion channel—the HCN ion channel that produces Ih and corresponding sag potential—in the same swim interneurons comprising the Clione swim CPG. In this respect, the Clione swim CPG is like the noradrenergic and serotonergic modulation of Ih in thalamic relay neurons where both noradrenalin and serotonin enhance Ih (McCormick and Pape, 1990). Kintos et al. (2016) indicate that the “functional consequences” of the convergence are “less clear.” Kintos et al. (2016) use a model of the crab gastric mill to demonstrate that the convergence of two neuromodulators onto a single ion channel is not merely redundant but may produce “distinct effects.” It is possible that the serotonergic and nitrergic modulation of the Clione swim CPG are not just redundant systems that affect the locomotor speed in the same way—or additive way. Rather, it may be that serotonergic and nitrergic effects are subtly different in their effects.
It is known with certainty that NO produces swim acceleration in Clione through cGMP-mediated changes in the cellular properties of neurons forming the Clione swim locomotor CPG (Moroz et al., 2000; Pirtle and Satterlie, 2021). However, it is not known what signal transduction mechanism mediates the serotonergic modulation of Clione swimming speed. One possible candidate is that serotonin binds to a G-protein coupled receptor that starts a cAMP signal transduction cascade. This is a likely candidate because both cAMP (through binding of epinephrine to beta-1 adrenergic receptors and subsequent activation of adenylyl cyclase) and cGMP (through NO stimulation of sGC) modulate If—Ih associated with the vertebrate sinoatrial node pacemaker cells (Musialek et al., 1997; Accili et al., 2002; Biel et al., 2009; Hennis et al., 2022) by binding to the intracellular C-terminal cyclic nucleotide binding domain (CNBD) of the HCN channels to promote their opening (Biel et al., 2002; Hennis et al., 2022). While the binding of cAMP to the CNBD is greater than cGMP in mammalian HCN channels, the effect of both cAMP and cGMP is to increase heart rate (Accili et al., 2002; Craven and Zagotta, 2006). Additionally, the crayfish swimmeret motoneurons respond to both cAMP and cGMP in cooperative way to increase the frequency of rhythmic bursting in these motoneurons. In the crayfish swimmeret system, cAMP increases locomotor frequency while cGMP (mediated by NO) is facilitatory (Mita et al., 2014). Similar signal transduction mechanisms involving cAMP and cGMP may function in Clione swim interneurons to modulate swimming locomotor speed through an increase in CPG interneuron excitability.
There are several metabotropic 5-HT receptor subtypes in invertebrates, including mollusks (Tierney, 2001) and some of these molluscan 5-HT receptors are linked to cAMP production via activation of adenylyl cyclase (Cohen et al., 2003). However, preliminary experimental results indicate that cAMP inhibits swimming locomotion in Clione. When the membrane permeable cAMP analog, 8-bromo-cAMP is administered, a decrease in fictive locomotor activity is recorded from Clione swim interneurons (Pirtle, unpublished data). Thus, cAMP does not contribute to swimming acceleration in Clione, but rather inhibits swimming. These unpublished data are consistent with the findings that the HCN ion channel cloned from the closely related marine mollusk, Aplysia californica, is more sensitive to cGMP than to cAMP (Yang et al., 2015) and that in Clione, the CNBD of HCN channels may preferentially bind cGMP. Cyclic AMP also disrupts swimming locomotion in the escape swim CPG of Tritonia (Clemens et al., 2007). Clemens et al. (2007) hypothesize that fluctuating concentrations of cAMP and intracellular Ca2+ may be involved in controlling the timing of the swimming activity in Tritonia—especially the termination of swimming. Levels of cGMP and cAMP in Clione swim interneurons may work similarly. In Clione, cGMP works as an accelerator (Pirtle and Satterlie, 2021) and cAMP (unpublished data) works as a brake. The balance of cGMP and cAMP may be critical for determining the frequency of action potentials generated in Clione swim interneurons and thus influence locomotor speed.
Discussion
Modulation of swimming behavior in Clione involves both circuit-level and cellular changes. At the circuit level, recruitment of interneurons (i.e., type 12 interneurons) to reconfigure the swim CPG and recruitment of motoneurons (i.e., general excitor motoneurons—or 1A and 2A motoneurons and Pd-SW neurons) are essential components to Clione swim acceleration. Swimming in Clione involves a two-geared system and modulation of swimming speed can occur by a change of gears or a change within gears—the former requiring a change in the frequency output of the Clione swim CPG and the later requiring changes in muscle contractility (Satterlie et al., 1990; Satterlie, 1991, 1993; Plyler and Satterlie, 2020). Cellular changes that occur during swim acceleration addressed in this review focus on how the hyperpolarization-activated cyclic nucleotide channel current, Ih, contributes to swim acceleration in Clione.
Experiments by Pirtle and Satterlie (2006) suggest that the role of type 12 interneuron may involve more than providing feedback inhibition as originally suggested (Arshavsky et al., 1985d,1993a). The involvement of type 12 interneurons to synaptic efficacy and synchronization of the locomotor appendages, the parapodia, represent critical gaps in the current understanding of how an interneuron’s recruitment into a CPG contributes to reconfiguration locomotor output (Pirtle and Satterlie, 2006). Additionally, while it is known that serotonin recruits Clione type 12 interneurons it is not known how NO affects these cells. Additional, experiments to identify how NO may contribute to type 12 interneuron recruitment are necessary.
There are several unanswered questions regarding motoneuron recruitment in Clione—particularly the recruitment of Pd-SW cells. It is clear that one group of Pd-SW cells is responsible for increasing the strength of parapodial movement through the modulation of slow-twitch muscle fibers (Satterlie, 1995b; Plyler and Satterlie, 2020). However, the role of Pd-SW neurons in modulating Clione swimming speed within the slow swimming gear is unclear. Furthermore, the contribution of one group of Pd-SW cells that are hypothesized to control the body wall musculature during fast swimming remains unresolved primarily due to the lack of morphological data on the distribution of these neurons to the body wall. The Pd-SW neurons to the body wall may work in concert with serotonergic modulation of heartbeat to distribute hemolymph to the wings resulting in enhanced wing stiffness as a mechanism to increase locomotor speed (Arshavsky et al., 1990; Szymik and Satterlie, 2017; Plyler and Satterlie, 2020). Because NO also modulates both locomotor behavior and heartbeat in Clione (Moroz et al., 2000; Pirtle and Satterlie, 2021), the coordination and relative contribution of serotonergic and nitrergic systems in controlling this possible mechanism requires attention.
At the cellular level, the HCN ion channels contribute to modulation of swimming in Clione. HCN ion channels produce the Ih current and corresponding sag potential. Blocking Ih with ZD7288 slows but does not completely abolish swimming in Clione. However, blocking Ih with ZD7288 does prevent serotonin-induced swim acceleration in Clione (Pirtle et al., 2010). Therefore, Ih is a modulatory target for serotonin but is not essential for rhythm generation. Ih is also a target for NO-cGMP modulation of Clione swimming (Pirtle and Satterlie, 2021). Other possible roles for Ih may include modulation of synaptic communication. Ih has been shown by Yang et al. (2015) to be found in siphon motoneurons of Aplysia, and in this regard, Ih contributes to classical conditioning of the siphon withdrawal reflex through a NO signaling pathway and synaptic facilitation. Additionally, Ih has been shown to modulate synaptic transmission and synaptic strength through serotonin-induced cAMP production in the neuromuscular junction of crayfish (Beaumont and Zucker, 2000). Therefore, there may be a similar role of Ih in Clione. Ih in Clione swim interneurons may respond to a NO-cGMP signal to enhance transmitter release during fast swimming speed. This possible mechanism may work in concert with type 12 interneuron recruitment to help strengthen synaptic communication to oppose the decreased synaptic efficacy associated with swim interneuron spike narrowing during swim acceleration (Satterlie et al., 2000; Pirtle and Satterlie, 2007).
Finally, the effect of both serotonin and NO on swim acceleration are similar—both serotonergic and nitrergic modulatory systems accelerate Clione swimming through common mechanisms that involve Ih (Satterlie and Norekian, 1996; Satterlie et al., 2000; Pirtle et al., 2010; Pirtle and Satterlie, 2021). Currently, the signal transduction mechanism and second messengers that mediate the serotonergic response in Clione swim interneurons is unknown. Serotonin and NO may be redundant neuromodulators that work separately or together. Additionally, there may be cross communication between serotonergic and nitrergic neuromodulatory systems, however, there may also be subtle differences in the modulatory effects of serotonergic and nitrergic modulation in the Clione swim system. Identification of the second messengers that mediate serotonergic modulation of Clione will be essential to elucidate these possibilities.
The modulation of the Clione swim CPG shares many common features with that of other locomotor systems. Two modulatory paradigms that function in the Clione swim CPG are (1) motoneuron and interneuron recruitment and (2) modification of cellular properties and ion channels. Further research of the Clione swim system may reveal more fundamental similarities and differences with other invertebrate and vertebrate locomotor CPGs. An important part of the locomotor control mechanism in Clione that needs further clarification is the role of second messengers. Signal transduction involving second messengers is critical for determining how locomotor CPG circuits are modulated (Clemens and Katz, 2001; Clemens et al., 2007). A complete analysis of the role of cyclic nucleotides in changing locomotor speed in Clione promises to yield significant information regarding how locomotor CPGs are modulated.
The evolution of locomotor activity in Clione in relationship to other marine gastropod mollusks should be addressed. Several other molluscan species exhibit locomotor activity that has evolved repeatedly and involves the gastropod foot. In Clione, the parapodia are derived from the foot and it is the dorsal-ventral flexion of the parapodia that propel Clione in swimming locomotion. In other marine gastropods, the dorsal-ventral bending of the body wall (e.g., Tritonia and Pleurobranchaea) and lateral bending of the body wall (e.g., Melibe) produces swimming locomotor activity and, in both instances, is associated with muscles of the foot. The differences in locomotor abilities among the various marine gastropods likely reflects adaptive radiation as the different species occupy different ecological niches. For example, Clione is holoplanktonic and is typically in a state of continuous swimming to maintain its position in the water column. This contrast with, for example, the benthic Tritonia that crawls on the ocean bottom and only swims as a means of predatory avoidance (Katz et al., 2001; Willows, 2001). Despite the different modes of swimming among the different marine gastropods, the neural circuitry in each share a common ancestry. For example, the cerebral serotonergic neurons, the Clione Cr-SP group, are homologs of the Tritonia serotonergic dorsal swim interneurons (DSIs). One of the unique differences in evolution that has occurred is the different roles that these homologous neurons take in terms of swimming behavior. In Tritonia, the serotonergic DSIs are both part of the Tritonia CPG and modulate swimming behavior. The DSIs are therefore considered to participate in intrinsic neuromodulation of Tritonia swim behavior. In Clione, these homologs, the Cr-SP neurons, are extrinsic neuromodulators (Katz et al., 2001). These evolutionary differences, as previously stated, are principally because Tritonia and Clione locomotor behavior are specific for the ecological niche that these two species occupy.
Additionally, the closely related Clione antarctica of the southern hemisphere shows significant differences in its locomotor system when compared to the northern hemisphere congener, C. limacina (Rosenthal et al., 2009; Dymowska et al., 2012). Foremost of the differences between C. antarctica and C. limacina is the absence of fast swimming in C. antarctica and associated loss of the neural circuitry (1A and 2A motoneurons) and fast-twitch musculature that produces fast swimming. Rosenthal et al. (2009) suggest that the loss of fast swimming in C. antarctica is an evolutionary adaptive tradeoff in which the species lost locomotor acceleration in favor of increased aerobic metabolism in response to living in a much colder environment. The evolutionary differences of C. limacina and C. antarctica evoke important questions. For example, both C. limacina and C. antarctica are feeding specialist that prey on L. helicina (Dymowska et al., 2012). The neural circuitry underlying locomotion, feeding, and statomotor systems are linked in C. limacina. The connections, or lack thereof, between locomotor, feeding, and statomotor systems in C. antarctica remain unknown. Additionally, similarities and differences in the neuromodulatory serotonergic and nitrergic systems between C. limacina and C. antarctica need to elucidated.
Author contributions
The author confirms being the sole contributor of this work and has approved it for publication.
Funding
Funding for this publication was provided by the College of Idaho Jolley Fund. There is no associated grant number for this funding source as this funding source is an internal funding source provided by donations to The College of Idaho.
Acknowledgments
This work was not possible without the continued support of Friday Harbor Laboratories, Friday Harbor, Washington. The author thanks the College of Idaho Jolley Fund for covering the publishing costs for this review.
Conflict of interest
The author declares that the research was conducted in the absence of any commercial or financial relationships that could be construed as a potential conflict of interest.
Publisher’s note
All claims expressed in this article are solely those of the authors and do not necessarily represent those of their affiliated organizations, or those of the publisher, the editors and the reviewers. Any product that may be evaluated in this article, or claim that may be made by its manufacturer, is not guaranteed or endorsed by the publisher.
References
Accili, E. A., Proenza, C., Baruscotti, M., and DiFrancesco, D. (2002). From funny current to HCN channels: 20 years of excitation. News Physiol. Sci. 17, 32–37. doi: 10.1152/physiologyonline.2002.17.1.32
Arbas, E. A., and Calabrese, R. L. (1984). Rate modification in the heartbeat central pattern generator of the medicinal leech. J. Comp. Physiol. 155, 783–794. doi: 10.1007/BF00611595
Arshavsky, Y. I., Deliagina, T. G., Orlovsky, G. N., Panchin, Y. V., Popova, L. B., and Sadreyev, R. I. (1998). Analysis of the central pattern generator for swimming in the mollusk Clione. Ann. NY Acad. Sci. 16, 51–69. doi: 10.1111/j.1749-6632.1998.tb09038.x
Arshavsky, Y., Orlovsky, G., Panchin, Y., Roberts, A., and Soffe, S. (1993a). Neuronal control of swimming locomotion: Analysis of the pteropod mollusc Clione and embryos of the amphibian Xenopus. Trends Neurosci. 16, 227–233. doi: 10.1016/0166-2236(93)90161-e
Arshavsky, Y. I., Deliagina, T. G., Gamkrelidze, G. N., Orlovsky, G. N., Panchin, Y. V., and Popova, L. B. (1993b). Pharmacologically induced elements of the hunting and feeding behavior in the pteropod mollusk Clione limacina. II. Effects of physostigmine. J. Neurophyiol. 69, 522–532. doi: 10.1152/jn.1993.69.2.522
Arshavsky, Y. I., Beloozerova, I. N., Orlovsky, G. N., Panchin, Y. V., and Pavlova, G. A. (1985a). Control of locomotion in marine mollusc Clione limacina I. Efferent activity during actual and fictitious swimming. Exp. Brain Res. 58, 255–262. doi: 10.1007/BF00235307
Arshavsky, Y. I., Beloozerova, I. N., Orlovsky, G. N., Panchin, Y. V., and Pavlova, G. A. (1985b). Control of locomotion in marine mollusc Clione limacina II. Rhythmic neurons of pedal ganglion. Exp. Brain Res. 58, 263–272. doi: 10.1007/BF00235308
Arshavsky, Y. I., Beloozerova, I. N., Orlovsky, G. N., Panchin, Y. V., and Pavlova, G. A. (1985c). Control of locomotion in marine mollusc Clione limacina III. On the origin of locomotory rhythm. Exp. Brain Res. 58, 273–284. doi: 10.1007/BF00235309
Arshavsky, Y. I., Beloozerova, I. N., Orlovsky, G. N., Panchin, Y. V., and Pavlova, G. A. (1985d). Control of locomotion in marine mollusc Clione limacina IV. Role of type 12 interneurons. Exp. Brain Res. 58, 285–293. doi: 10.1007/BF00235310
Arshavsky, Y. I., Deliagina, T. G., Gelfand, I. M., Orlovsky, G. N., Panchin, Y. V., Pavlova, G. A., et al. (1990). Neural control of heart beat in the pteropod mollusc Clione limacina: Coordination of circulatory and locomotory systems. J. Exp. Biol. 148, 461–475. doi: 10.1242/jeb.148.1.461
Arshavsky, Y. I., Deliagina, T. G., Orlovsky, G. N., Panchin, Y. V., and Papova, L. B. (1992). Interneurones mediating the escape reaction of the marine mollusc Clione limacina. J. Exp. Biol. 164, 307–314. doi: 10.1242/jeb.164.1.307
Arshavsky, Y. I., Orlavsky, G. N., Panchin, Y. V., and Pavlova, G. A. (1989). Control of locomotion in marine mollusc Clione limacina. VII reexamination of type 12 interneurons. Exp. Brain Res. 78, 398–406. doi: 10.1007/BF00228912
Beaumont, V., and Zucker, R. S. (2000). Enhancement of synaptic transmission by cyclic AMP modulation of presynaptic Ih channels. Nat. Neurosci. 3, 133–141. doi: 10.1038/72072
Biel, M., Schneider, A., and Wahl, C. (2002). Cardiac HCN channels: Structure, function, and modulation. Trends Cardiovasc. Med. 12, 206–213. doi: 10.1016/S1050-1738(02)00162-7
Biel, M., Wahl-Schott, C., Michalakis, S., and Zong, X. (2009). Hyperpolarization-activated cation channels: From genes to function. Physiol. Rev. 89, 847–885. doi: 10.1152/physrev.00029.2008
Brocard, F. (2019). New channel lineup in spinal circuits governing locomotion. Curr. Opin. Physiol. 8, 14–22. doi: 10.1016/j.cophys.2018.11.009
Brodfuehrer, P. D., Debski, E. A., O’Gara, B. A., and Friesen, W. O. (1995). Neural control of leech swimming. J. Neurobiol. 27, 403–428. doi: 10.1002/neu.480270312
Brown, T. G. (1911). The intrinsic factors in the act of progression in the mammal. Proc. R. Soc. Lond. 84, 308–319. doi: 10.1098/rspb.1911.0077
Bucher, D., Haspel, G., Golowasch, J., and Nadim, F. (2015). “Central pattern generators,” in eLS (Chichester: John Wiley & Sons, Ltd.), 1–12. doi: 10.1002/9780470015902.a0000032.pub2
Clemens, S., Calin-Jageman, R., Sakurai, A., and Katz, P. S. (2007). Altering cAMP levels within a central pattern generator modifies or disrupts rhythmic motor output. J. Comp. Physiol. A Neuroethol. Sens. Neural Behav. Physiol. 193, 1265–1271. doi: 10.1007/s00359-007-0280-4
Clemens, S., and Katz, P. S. (2001). G protein signaling in a neuronal network is necessary for rhythmic motor pattern production. J. Neurophysiol. 89, 762–772. doi: 10.1152/jn.00765.2002
Cohen, J. E., Onyike, C. U., McElroy, E., Lin, A. H., and Abrams, T. W. (2003). Pharmacological characterization of an adenylyl cyclase-coupled 5-HT receptor in Aplysia: Comparison with mammalian 5-HT receptors. J. Neurophysiol. 89, 1440–1455. doi: 10.1152/jn.01004.2002
Craven, K. B., and Zagotta, W. N. (2006). CNG and HCN channels: Two peas, one pod. Annu. Rev. Physiol. 68, 375–401. doi: 10.1146/annurev.physiol.68.040104.134728
Delcomyn, F. (1980). Neural basis of rhythmic behavior in animals. Science 210, 492–498. doi: 10.1126/science.7423199
Dickinson, P. S. (2006). Neuromodulation of central pattern generators in invertebrates and vertebrates. Curr. Opin. Neurobiol. 16, 604–614. doi: 10.1016/j.conb.2006.10.007
Dymowska, A. K., Manfredi, T., Rosenthal, J. J. C., and Seibel, B. A. (2012). Temperature compensation of aerobic capacity and performance in the Antarctic pteropod, Clione antarctica, compared with its northern congener, C. limacina. J. Exp. Biol. 215, 3370–3378. doi: 10.1242/jeb.07060
Fetcho, J. R., and McLean, D. L. (2010). Some principles of organization of spinal neurons underlying locomotion in zebrafish and their implications. Ann. N. Y. Acad. Sci. 1198, 94–104. doi: 10.1111/j.1749-6632.2010.05539.x
Gamkrelidze, G. N., Laurienti, P. J., and Blankenship, J. E. (1995). Identification and characterization of cerebral ganglion neurons that induce swimming and modulate swim-related pedal ganglion neurons in Aplysia brasiliana. J. Neurophysiol. 74, 1444–1462. doi: 10.1152/jn.1995.74.4.1444
Getting, P. A. (1981). Mechanisms of pattern generation underlying swimming in Tritonia. I. neural network formed by monosynaptic connections. J. Neurophysiol. 46, 65–79. doi: 10.1152/jn.1981.46.1.65
Golowasch, J. (2019). Neuromodulation of central pattern generators and its role in the functional recovery of pattern generator activity. J. Neurophysiol. 122, 300–315.
Grillner, S. (1999). Bridging the gap—from ion channels to networks and behavior. Curr. Opin. Neurobiol. 9, 663–669. doi: 10.1016/S0959-4388(99)00036-7
Grillner, S., and Wallén, P. (1985). Central pattern generators for locomotion, with special reference to vertebrates. Ann. Rev. Neurosci. 8, 233–261. doi: 10.1146/annurev.ne.08.030185.001313
Guertin, P. A. (2009). The mammalian central pattern generator. Brain Res. Rev. 62, 45–56. doi: 10.1016/j.brainresrev.2009.08.002
Guertin, P. A. (2013). Central pattern generators for locomotion: Anatomical, physiological, and pathological considerations. Front. Neurol. 3:183. doi: 10.3389/fneur.2012.00183
Harris-Warrick, R. M. (2011). Neuromodulation and flexibility in central pattern generator networks. Curr. Opin. Neurobiol. 21, 685–692. doi: 10.1016/j.conb.2011.05.011
Hennis, K., Biel, M., Fenske, S., and Wahl-Schott, C. (2022). Paradigm shift: New concepts for HCN4 function in cardiac pacemaking. Pflugers Arch. 474, 649–663. doi: 10.1007/s00424-022-02698-4
Hermans, C. O., and Satterlie, R. A. (1992). Fast-strike feeding behavior in a pteropod mollusk, Clione limacina phipps. Biol. Bull. 182, 1–7. doi: 10.2307/1542175
Huang, Z., and Satterlie, R. A. (1990). Neuronal mechanisms underlying behavioral switching in a pteropod mollusc. J. Comp. Physiol. A Neuroethol. Sens. Neural. Behav. Physiol. 166, 875–887. doi: 10.1007/BF00187335
Jing, J., and Gillette, R. (1999). Central pattern generator for escape swimming in the notapsid sea slug Pleurobranchaea californica. J. Neurophysiol. 81, 654–667. doi: 10.1152/jn.1999.81.2.654
Kabotyanski, E. A., and Sakharov, D. A. (1988). Monoamine-dependent behavioral states in the pteropod mollusc Clione limacina. Symp. Biol. Hung. 36, 463–477.
Kabotyanskii, E. A., and Sakharov, D. A. (1991). Neuronal correlates of the serotonin-dependent behavior of the pteropod mollusc Clione limacina. Neurosci. Behav. Physiol. 21, 422–435. doi: 10.1007/BF01200277
Kaczmarek, L. K., and Levitan, I. B. (1987). What is neuromodulation? Neuromodulation the biochemical control of neuronal excitability, Chap. New York, NY. Oxford University Press, 3–17.
Katz, P. S. (1998). Comparison of extrinsic and intrinsic neuromodulation in two central pattern generator circuits in invertebrates. Exp. Physiol. 83, 281–292. doi: 10.1113/expphysiol.1998.sp004113
Katz, P. S., Fickbohm, D. J., and Lynn-Bullock, C. P. (2001). Evidence that the central pattern generator for swimming in Tritonia arose from a non-rhythmic neuromodulatory arousal system: Implication for the evolution of specialized behavior. Am. Zool. 41, 962–975. doi: 10.1093/icb/41.4.962
Kintos, N., Nusbaum, M. P., and Nadim, F. (2016). Convergent neuromodulation onto a network neuron can have divergent effects at the network level. J. Comput. Neurosci. 40, 113–135. doi: 10.1007/s10827-015-0587-z
Lalli, C. M. (1967). Studies on the structure and biology of two gymnostomatous pteropods, Clione limacina Agersborg and Curcibranchaea machrochira (Meisenheimer). Ph.D. thesis. Seattle, WA: University of Washington.
Lalli, C. M., and Gilmer, R. W. (1989). Pelagic snails: The biology of holoplanktonic gastropod mollusks. Stanford: Standford University Press.
Lüthi, A., and McCormick, D. A. (1998). H-current: Properties of a neuronal and network pacemaker. Neuron 21, 9–12.
Mackie, G. O. (1985). Midwater macroplankton of British Columbia studied by submersible PISCES IV. J. Plankton Res. 7, 753–777.
Mackie, G. O., and Mills, C. E. (1983). Use of the Pisces IV submersible for zooplankton studies in coastal waters of British Columbia. Can. J. Fish Aquat. Sci. 40, 763–776.
Malyshev, A. Y., Norekian, T. P., and Balaban, P. M. (2008). Neural control of heartbeat during two antagonistic behaviors: Whole body withdrawal and escape swimming in the mollusk Clione limacina. J. Comp. Physiol. A Neuroethol. Sens. Neural Behav. Physiol. 194, 899–906. doi: 10.1007/s00359-008-0362-y
Malyshev, A. Y., Norekian, T. P., and Willows, A. O. D. (1999). Differential effects of serotonergic and peptidergic cardioexcitatory neurons on the heart activity in the pteropod mollusc, Clione limacina. J. Comp. Physiol. A 185, 551–560. doi: 10.1007/s003590050415
Marder, E., and Thirumalai, V. (2002). Cellular, synaptic and network effects of neuromodulation. Neural Netw. 15, 479–493. doi: 10.1016/s0893-6080(02)00043-6
McCormick, D. A., and Pape, H. C. (1990). Noradrenergic and serotonergic modulation of a hyperpolarization-activated cation current in thalamic relay neurons. J. Physiol. 431, 319–342. doi: 10.1113/jphysiol.1990.sp018332
Mita, A., Yoshida, M., and Nagayama, T. (2014). Nitric oxide modulates a swimmeret beating rhythm in the crayfish. J. Exp. Biol. 217(Pt. 24), 4423–4431. doi: 10.1242/jeb.110551
Moroz, L. L., Norekian, T. P., Pirtle, T. J., Robertson, K. J., and Satterlie, R. A. (2000). Distribution of NADPH-diaphorase reactivity and effects of nitric oxide on feeding and locomotory circuitry in the pteropod mollusc, Clione limacina. J. Comp. Neurol. 427, 247–284. doi: 10.1002/1096-9861(20001113)427:2<274::AID-CNE8<3.0.CO;2-#
Musialek, P., Lei, M., Brown, H. F., Paterson, D. J., and Casadei, B. (1997). Nitric oxide can increase heart rate by stimulating the hyperpolarization-activated inward current, I(f). Circ. Res. 81, 60–68. doi: 10.1161/01.RES.81.1.60
Nadim, F., and Bucher, D. (2014). Neuromodulation of neurons and synapses. Curr. Opin. Neurobiol. 29, 48–56.
Norekian, T. P. (1995). Prey capture phase of feeding behavior in the pteropod mollusc, Clione limacina: Neural mechanisms. J. Comp. Physiol. A 177, 41–53. doi: 10.1007/BF00243397
Norekian, T. P. (1999). GABAergic excitatory synapses and electrical coupling sustain prolonged discharges in the prey capture neural network of Clione limacina. J. Neurosci. 19, 1863–1875. doi: 10.1523/JNEUROSCI.19-05-01863.1999
Norekian, T. P., Hermans, C. O., and Satterlie, R. A. (2019). Organization of buccal cone musculature in the pteropod mollusc Clione limacina. Biol. Bull. 273, 36–47. doi: 10.1086/704737
Norekian, T. P., and Malyshev, A. Y. (2005). Coordinated excitatory effect of GABAergic interneurons on three feeding motor programs in the mollusk Clione limacina. J. Neurophysiol. 93, 305–315. doi: 10.1152/jn.00722.2004
Norekian, T. P., and Malyshev, A. Y. (2006). Neural mechanisms underlying co-activation of functionally antagonistic motoneurons during a Clione feeding behavior. J. Neurophysiol. 95, 2560–2569. doi: 10.1152/jn.01174.2005
Norekian, T. P., and Satterlie, R. A. (1993). Cerebral neurons underlying prey capture movements in the pteropod mollusc, Clione limacina I. Physiology, morphology. J. Comp. Physiol. A 172, 153–169. doi: 10.1007/BF00189393
Norekian, T. P., and Satterlie, R. A. (1995). An identified cerebral interneuron initiates different elements of prey capture behavior in the pteropod mollusc, Clione limacina. Invertebr. Neurosci. 1, 235–248. doi: 10.1007/BF02211025
Norekian, T. P., and Satterlie, R. A. (1996a). Whole body withdrawal circuit and its involvement in the behavioral hierarchy of the mollusk Clione limacina. J. Neurophysiol. 75, 529–537. doi: 10.1152/jn.1996.75.2.529
Norekian, T. P., and Satterlie, R. A. (1996b). Cerebral serotonergic neurons reciprocally modulate swim and withdrawal neural networks in the mollusk Clione limacina. J. Neurophysiol. 75, 538–546. doi: 10.1152/jn.1996.75.2.538
Norekian, T. P., and Satterlie, R. A. (2001). Serotonergic neural system not only activates swimming but also inhibits competing neural centers in a pteropod mollusc. Am. Zool. 41, 993–1000. doi: 10.1093/icb/41.4.993
Norekyan, T. P. (1989). Neurons determining the passive defensive response in the pteropod mollusk Clione limacina. Neurophysiology 21, 495–502.
Panchin, Y. V., Arshavsky, Y. I., Deliagina, T. G., Orlovsky, G. N., Popova, L. B., and Selverston, A. I. (1996). Control of locomotion in the marine mollusc Clione limacina. XI. Effects of serotonin. Exp. Brain Res. 109, 361–365. doi: 10.1007/BF00231794
Panchin, Y. V., Arshavsky, Y. I., Deliagina, T. G., Popova, L. B., and Orlovsky, G. N. (1995a). Control of locomotion in marine mollusk Clione limacina IX. Neuronal mechanisms of spatial orientation. J. Neurophysiol. 73, 1924–1937. doi: 10.1152/jn.1995.73.5.1924
Panchin, Y. V., Popova, L. B., Deliagina, T. G., Orlovsky, G. N., and Arshavsky, Y. I. (1995b). Control of locomotion in marine mollusk Clione limacina. VIII. cerebropedal neurons. J. Neurophysiol. 73, 1912–1923. doi: 10.1152/jn.1995.73.5.1912
Panchin, Y. V., and Sadreyev, R. I. (1997). Effects of acetylcholine and glutamate on isolated neurons of locomotory network of Clione. Neuroreporat 8, 2897–2901. doi: 10.1097/00001756-199709080-00019
Panchin, Y. V., Sadreyev, R. I., and Arshavsky, Y. I. (1995c). Statomotor system in the marine mollusk Clione limacina. J. Neurophysiol. 73, 407–410. doi: 10.1152/jn.1995.73.1.407
Pape, H.-C. (1996). Queer current and pacemaker: The hyperpolarization-activated cation curren in neurones. Ann. Rev. Physiol. 58, 299–327. doi: 10.1146/annurev.ph.58.030196.001503
Pearson, K. C., and Wolf, H. (1987). Comparison of motor patterns in the intact and deafferented flight system of the locust I. electromyographic analysis. J. Comp. Physiol. A 160, 259–268. doi: 10.1007/BF00609731
Pirtle, T. J., and Satterlie, R. A. (2021). Cyclic guanosine monophosphate modulates locomotor acceleration induced by nitric oxide but not serotonin in Clione limacina central pattern generator swim interneurons. Integr. Org. Biol. 3:obaa045. doi: 10.1093/iob/obaa045
Pirtle, T. P., and Satterlie, R. A. (2004). Cellular mechanisms underlying swim acceleration in the pteropod mollusk Clione limacina. Integr. Comp. Biol. 44, 37–46. doi: 10.1093/icb/44.1.37
Pirtle, T. P., and Satterlie, R. A. (2006). The contribution of the pleural type 12 interneurons to swim acceleration in Clione limacina. Invert. Neurosci. 6, 161–168. doi: 10.1007/s10158-006-0029-8
Pirtle, T. P., and Satterlie, R. A. (2007). The role of postinhibitory rebound in the locomotor central-pattern generator of Clione limacina. Integr. Comp. Biol. 47, 451–456. doi: 10.1093/icb/icm085
Pirtle, T. P., Willingham, K., and Satterlie, R. A. (2010). A hyperpolarization-activated inward current alters swim frequency of the pteropod mollusk Clione limacina. Comp. Biochem. Physiol. A Mol. Integr. Physiol. 157, 319–327. doi: 10.1016/j.cbpa.2010.07.025
Plyler, J. B., and Satterlie, R. A. (2020). Pedal serotonergic neuron clusters of the pteropod mollusc, Clione limacina, contain two morphological subtypes with different innervation targets. Invert. Neurosci. 20:21. doi: 10.1007/s10158-020-00256-0
Rosenthal, J. J. C., Seibel, B. A., Dymowska, A., and Benzanilla, F. (2009). Trade-off between aerobic capacity and locomotor capability in an Antarctic pteropod. Proc. Natl. Acad. Sci. U. S. A. 106, 6192–6196. doi: 10.1073/pnas.0901321106
Satterlie, R. A. (1985). Reciprocal inhibition and postinhibitory rebound produce reverberation in a locomotor pattern generator. Science 229, 402–404. doi: 10.1126/science.229.4711.4
Satterlie, R. A. (1991). Neural control of speed changes in an opisthobranch locomotory system. Biol. Bull. 180, 228–233. doi: 10.2307/1542392
Satterlie, R. A. (1993). Neuromuscular organization in the pteropod mollusc Clione limacina. J. Exp. Biol. 181, 119–140. doi: 10.1242/jeb.181.1.119
Satterlie, R. A. (1995b). Serotonergic modulation of swimming speed in the pteropod mollusc Clione limacina. II. Peripheral modulatory neurons. J. Exp. Biol. 198, 905–916. doi: 10.1242/jeb.198.4.905
Satterlie, R. A. (1995a). Peripheral variability in the locomotory system of the pteropod mollusc Clione limacina. Am. Zool. 35, 542–547. doi: 10.1093/icb/35.6.542
Satterlie, R. A., and Courtney, C. (2008). Hexamethonium sensitivity of the swim musculature of the pteropod mollusc, Clione limacina. Invert. Neurosci. 8, 157–166. doi: 10.1007/s10158-008-0079-1
Satterlie, R. A., Goslow, G. E., and Reyes, A. (1990). Two types of striated muscle suggest two-geared swimming in the pteropod mollusc Clione limacina. Exp. Biol. 255, 131–140. doi: 10.1002/jez.1402550202
Satterlie, R. A., Labarbera, M., and Spencer, A. N. (1985). Swimming in the pteropod mollusc, Clione limacina: I. Behaviour and morphology. J. Exp. Biol. 116, 189–204. doi: 10.1242/jeb.116.1.189
Satterlie, R. A., and Norekian, T. P. (1995). Serotonergic modulation of swimming speed in the pteropod mollusc Clione limacina. III. Cerebral neurons. J. Exp. Biol. 198, 917–930. doi: 10.1242/jeb.198.4.917
Satterlie, R. A., and Norekian, T. P. (1996). Modulation of swimming in the pteropod mollusc, Clione limacina: Role of a compartmental serotonergic system. Invert. Neurosci. 2, 157–165. doi: 10.1007/BF02214171
Satterlie, R. A., and Norekian, T. P. (2001). Mechanisms of locomotory speed change: The pteropod solution. Am. Zool. 41, 1001–1008. doi: 10.1093/icb/41.4.1001
Satterlie, R. A., Norekian, T. P., Jordan, S., and Kazilek, C. J. (1995). Serotonergic modulation of swimming speed in the pteropod mollusc Clione limacina. I. Serotonin immunoreactivity in the central nervous system and wings. J. Exp. Biol. 98, 895–904. doi: 10.1242/jeb.198.4.895
Satterlie, R. A., Norekian, T. P., and Pirtle, T. J. (2000). Serotonin-induced spike narrowing in a locomotor pattern generator permits increases in cycle frequency during accelerations. J. Nuerophysiol. 83, 2163–2170. doi: 10.1152/jn.2000.83.4.2163
Satterlie, R. A., and Spencer, A. N. (1985). Swimming in the pteropod mollusc, Clione limacina: II. Physiology. J. Exp. Biol. 116, 205–222. doi: 10.1242/jeb.116.1.205
Selverston, A. I., Russell, D. F., Miller, J. P., and King, D. G. (1976). The stomatogastric nervous system: Structure and function of a small neural network. Prog. Neurobiol. 7, 215–290. doi: 10.1016/0301-0082(76)90008-3
Stein, P. S. (2018). Central pattern generators in the turtle spinal cord: Selection among the forms of motor behaviors. J. Neurophysiol. 119, 422–440. doi: 10.1152/jn.00602.2017
Szymik, B. G., and Satterlie, R. A. (2011). Changes in wingstroke kinematics associated with a change in swimming in a pteropod mollusk, Clione limacina. J. Exp. Biol. 214, 3935–3947. doi: 10.1242/jeb.058461
Szymik, B. G., and Satterlie, R. A. (2017). Circulation of hemocoelic fluid during slow and fast swimming in the pteropod mollusc Clione limacina. Invert. Biol. 136, 290–300. doi: 10.1111/IVB.12182
Thompson, S., and Watson, W. H. III. (2005). Central pattern generator for swimming in Melibe. J. Exp. Biol. 208, 1347–1361. doi: 10.1242/jeb.01500
Tierney, A. J. (2001). Structue and function of invertebrate 5-HT receptors: A review. Comp. Biochem. Physiol. A Mol. Integr. Physiol. 128, 791–804.
Tsirulis, T. P. (1974). The fine structure of the statocyst in the pteropod mollusc Clione limacina. J. Evol. Biochem. Physiol. 10, 181–189.
Wagner, N. (1885). Die wirbellosen des weissen meeres: Zoologische forschungen an der kuste des solowetzkischen meerbusens in den sommermonaten der jahre 1877, 1878, 1879 und 1882. Leipzig: Verlag von Wilhelm Engelmann.
Willows, A. O. D. (2001). Costs and benefits of opisthobranch swimming and neurobehavioral mechanisms. Am. Zool. 41, 943–951. doi: 10.1093/icb/41.4.943
Yang, Q., Kuzyk, P., Antonov, I., Bostwick, C. J., Kohn, A. B., Moroz, L. L., et al. (2015). Hyperpolarization-activated, cyclic nucleotide-gated cation channels in Aplysia: Contribution to classical conditioning. Proc. Natl. Acad. Sci. U. S. A. 112, 16030–16035. doi: 10.1073/pnas.1501731113
Zelenin, P. V., and Panchin, Y. V. (2000). Projection pattern and target selection of Clione limacina motoneurons sprouting within an intact environment. J. Comp. Neurol. 423, 220–226. doi: 10.1002/1096-9861(20000724)423:2<220::AID-CNE3<3.0.CO;2-M
Zhong, G., Shevtsova, N. A., Rybak, I. A., and Harris-Warrick, R. M. (2012). Neural activity in the isolated mouse spinal cord during spontaneous deletions in fictive locomotion: Insights into locomotor central pattern generator organization. J. Physiol. 590, 4735–4759. doi: 10.1113/jphysiol.2012.240895
Keywords: Clione, CPG, locomotion, modulation, HCN, statocyst function, feeding behavior, neural circuit
Citation: Pirtle TJ (2022) A review of the circuit-level and cellular mechanisms contributing to locomotor acceleration in the marine mollusk Clione limacina. Front. Neurosci. 16:1072974. doi: 10.3389/fnins.2022.1072974
Received: 18 October 2022; Accepted: 05 December 2022;
Published: 22 December 2022.
Edited by:
James Newcomb, New England College, United StatesReviewed by:
Leonid L. Moroz, University of Florida, United StatesBrett Szymik, Augusta University/University of Georgia Medical Partnership, Georgia
Copyright © 2022 Pirtle. This is an open-access article distributed under the terms of the Creative Commons Attribution License (CC BY). The use, distribution or reproduction in other forums is permitted, provided the original author(s) and the copyright owner(s) are credited and that the original publication in this journal is cited, in accordance with accepted academic practice. No use, distribution or reproduction is permitted which does not comply with these terms.
*Correspondence: Thomas J. Pirtle, ✉ dHBpcnRsZUBjb2xsZWdlb2ZpZGFoby5lZHU=