- 1Center of Cyclotron and PET Radiopharmaceuticals, Department of Nuclear Medicine and PET/CT-MRI Center, The First Affiliated Hospital of Jinan University, Guangzhou, China
- 2Department of Rehabilitation Medicine, The First Affiliated Hospital of Jinan University, Jinan University, Guangzhou, China
- 3Guangdong-Hongkong-Macau Institute of CNS Regeneration, Ministry of Education CNS Regeneration Collaborative Joint Laboratory, Jinan University, Guangzhou, China
Autism spectrum disorder (ASD) is a basket term for neurodevelopmental disorders characterized by marked impairments in social interactions, repetitive and stereotypical behaviors, and restricted interests and activities. Subtypes include (A) disorders with known genetic abnormalities including fragile X syndrome, Rett syndrome, and tuberous sclerosis and (B) idiopathic ASD, conditions with unknown etiologies. Positron emission tomography (PET) is a molecular imaging technology that can be utilized in vivo for dynamic and quantitative research, and is a valuable tool for exploring pathophysiological mechanisms, evaluating therapeutic efficacy, and accelerating drug development in ASD. Recently, several imaging studies on ASD have been published and physiological changes during ASD progression was disclosed by PET. This paper reviews the specific radioligands for PET imaging of critical biomarkers in ASD, and summarizes and discusses the similar and different discoveries in outcomes of previous studies. It is of great importance to identify general physiological changes in cerebral glucose metabolism, cerebral blood flow perfusion, abnormalities in neurotransmitter systems, and inflammation in the central nervous system in ASD, which may provide excellent points for further ASD research.
Autism Spectrum Disorder
Autism spectrum disorder (ASD) is a pervasive developmental disorder characterized by persistently impaired social interactions and interpersonal communication, limited, repetitive, and stereotyped behaviors, and a significantly limited range of interests and activities. In 2013, the fifth edition of the Diagnostic and Statistical Manual of Mental Disorders (DSM-5) revolutionized the diagnostic criteria for ASD (American Psychiatric Association, 2013). In this manual, four categories previously defined as pervasive developmental disorders were identified: autistic disorder, Asperger syndrome (AS), pervasive developmental disorder not otherwise specified (PDD-NOS), and childhood disintegrative syndrome (also known as Heller Syndrome). These disorders are now collectively referred to as ASD. The diagnostic criteria were reduced from three items in DSM-IV to two in DSM-5: social dysfunction, repetitive and stereotypical behavior. Both verbal and non-verbal communication disorders are incorporated into social disorders. These shifts in diagnostic criteria have changed the diagnosis and treatment of different subtypes of pervasive developmental disorders, and affected the consistency and comparability of previous imaging studies. To avoid concept confusion, each cited document will be described with “Autism/AS/PDD/ASD” according to its diagnostic criteria in addition to ASD collectively.
The etiology of idiopathic ASD remains unclear and available evidence suggests the ASD phenotype is driven by complex interactions between genetic and environmental factors that affect brain maturation, synaptic function, and cortical networks. Environmental influences in early childhood development may play a key role in the development of ASD and obstetric complications may predispose vulnerable offspring to ASD (Brasic and Holland, 2006, 2007). At the same time, children who experience obstetric complications are also more likely to exhibit genetic causation of the disease (Katsanis, 2016). The latest large gene study has identified 102 risk genes associated with ASD in humans (Satterstrom et al., 2020). Most risk genes are expressed early in brain development and play a role in the regulation of gene expression or neuronal communication, thus having neurodevelopmental and neurophysiological affects. In cerebral cortex cells, risk genes are highly expressed in the spectrum of excitatory and inhibitory neurons, which is consistent with excitation/inhibition imbalances in multiple ASD-associated pathways.
At present, a diagnosis of ASD is mainly based on the medical history of patients and their families and clinical evaluation of patients. The two medical history data collection tools considered the gold standard for clinical diagnosis of ASD are: Autism Diagnostic Interview Revised (ADI-R), which is a structured interview for the family members of the patient (Le Couteur et al., 2003), and The Autism Diagnostic Observation Schedule (ADOS), which is a play-based interview with children and their families or with high-functioning children, adolescents, or adults (Lord et al., 2012). Clinical evaluation of patients with ASD includes physical and neurological examinations (Brasić et al., 2004). In addition, neuroimaging is also an effective evaluation tool for the diagnosis and treatment of ASD. Although there are currently no specific biomarkers available to diagnose ASD subtypes (i.e., without any known genetic or environmental causes), neuroimaging techniques have great potential for explaining the complexity of symptoms and signs observed in multiple ASD subtypes (Walton et al., 2013). In past decades, neuroimaging techniques have been used to explore the pathophysiologic mechanisms of ASD-related abnormalities in brain glucose metabolism, blood perfusion, and neurotransmitter systems. Neuroimaging techniques will play an increasingly important role in early diagnosis, treatment, and efficacy evaluation of ASD.
Neuroimaging
Neuroimaging technology is used to visualize the structural and functional changes of the central nervous system. Structural imaging, including X-rays, computed tomography (CT) and magnetic resonance imaging (MRI), enables high-resolution visualization of anatomical structures. Functional imaging, including nuclear neuroimaging techniques such as positron emission tomography (PET) and single photon emission computed tomography (SPECT), as well as functional magnetic resonance (fMRI) techniques, help visualize and quantify functional and molecular-level changes in the nervous system (Wong et al., 2007). Non-invasive neuroimaging techniques provide dynamic and quantitative technical support for in vivo neuropsychiatric disorder studies, and are important means of studying structural, functional, and neurochemical molecule differences in the brains of ASD patients. The imaging techniques widely used in ASD research are MRI, SPECT, and PET.
Magnetic Resonance Imaging
The combination of structural and functional MRI has significant advantages in the central nervous system. The main applied technologies in ASD research include magnetic resonance spectroscopy (MRS), blood oxygen-level dependent (BOLD) and arterial spin labeling (ASL). MRS can determine the molecular composition and spatial configuration of tissues by detecting the chemical shift of metabolites in magnetic resonance imaging. It can be applied to extract qualitative and quantitative information on various metabolites in the brain to characterize pathophysiological changes associated with brain diseases. The chemical shifts differ for different metabolites, which is a reflection of the pathological and physiological changes in different types of energy metabolism, including n-acetylaspartic acid (NAA), creatine (Cr) and creatine phosphate (Cr/PCr), adenosine diphosphate and triphosphate (ATP/ADP), choline (Cho), inositol (ml), glutamino-glutamate complex (Glx), and lactic acid (Wagner et al., 1985; Barker, 2001; Stanley, 2002; Page et al., 2006). BOLD and ASL can non-invasively detect changes in cerebral hemodynamics and brain function. It can display the activation state of cerebral cortex functional areas under task stimulation, which is of great value in locating the activated cortex and evaluating the brain function status, drug efficacy and prognosis of patients (Mash et al., 2019). fMRI has been widely used in animal experiments (Durieux et al., 2015; Janik et al., 2016; Mudd et al., 2017) and clinical trials (Tebartz van Elst et al., 2014; Goji et al., 2017) for ASD research.
Single Photon Emission Computed Tomography
Single photon emission computed tomography (SPECT) are realized visualization by detecting the metabolism and distribution of single-photon radionuclide-labeled functional probes in the body, thus reflecting specific physiological changes. Probes with longer half-lives (T1/2) are commonly used clinically, such as 123I (T1/2 ∼13 h) and 99mTc (T1/2 ∼24 h). Due to the high sensitivity of SPECT functional imaging and high resolution of CT structural imaging, SPECT/CT is an effective tool for in vivo disease diagnosis and new drug research (Van Audenhaege et al., 2015), and plays an important role in the neuroimaging of ASD (Makkonen et al., 2008; Bjørklund et al., 2018). Since SPECT and SPECT/CT are often available in nuclear medical departments of general hospitals, SPECT and SPECT/CT can typically be performed in community hospitals without cyclotrons.
Positron Emission Tomography
Positron emission tomography (PET) detects processes associated with the metabolism and distribution of positron emitting radionuclide-labeled probes in vivo. Commonly used radionuclides include 11C (T1/2 ∼20.4 min), 18F (T1/2 ∼109.8 min), 13N (T1/2 ∼10.0 min) and 15O (T1/2 ∼2.0 min). It should be noted that radionuclides with short half-lives, such as 11C (T1/2 ∼20.4 min) and 15O (T1/2 ∼2.0 min), can only be conducted at tertiary medical centers with onsite cyclotrons. Simultaneously with metabolic distribution of the probe in the body, nuclear decay occurs and positrons emits. The positrons collide with surrounding electrons, and produce two gamma photons (511 keV each) in 180° reverse motion, which is recorded by multiple pairs of detectors located in opposite positions. Computer software then deal with the detected gamma photon signals to create three-dimensional images (Volkow et al., 1988). Compared to MRI and SPECT, PET is significantly more advantageous for the absolutely quantitative determination of parameters (such as glucose metabolic rate, protein synthesis rate, gene expression, enzyme activity and receptor density) related to specific biochemical pathways due to its advantages in sensitivity, spatial resolution and time efficiency.
Positron emission tomography imaging technology based on radioactive probe has unique technical advantages in tracer and quantitative detection of the distribution and change of various metabolites, receptors, enzymes, transporters, and study of drug absorption, excretion and other metabolic laws. Given the advantage of microdose, PET tracers can be used to conduct preclinical studies and early clinical trials (i.e., Phase 0 or early Phase I) to obtain target occupancy, pharmacokinetic and pharmacodynamic information, and preliminarily understand various physiological performance of drugs in vivo, providing a favorable basis for later clinical trials (McCluskey et al., 2020). PET probe is not only a diagnostic clinical drug, but also can be used as an evaluation tool for clinical translational research of other drugs. Over the course of the development of PET technology, more than 1,000 PET probes have been produced with many more being continuously and rapidly developed for disease research. There are a number of types of probes, including those used for imaging of metabolic substrates or analogs, such as glucose, amino acids, and fatty acids (Shiue and Welch, 2004), receptors using protein-binding probes, such as receptors and transporters (Smith et al., 2003; Gjedde et al., 2005), genes, immunological features, apoptosis, angiogenesis, hypoxia, and signal transduction (MacLaren et al., 2000; Jain and Batra, 2003). PET probes that have good brain penetration, high binding affinity and specificity, and a good metabolic stability and distribution volume are effective tools for neuroimaging. The main research targets of ASD and their corresponding PET probes are shown in Table 1.
Positron Emission Tomography Molecular Imaging in Autism Spectrum Disorder
The development of PET has provided non-invasive and dynamic technical support for the study of ASD in vivo (Syed and Brasic, 2019). Although the etiology and pathology of ASD are complex and unclear, PET has been used by researchers over past decades to explore the pathophysiological changes in ASD from various angles, resulting in a large amount of evidence being obtained and new research directions opening up (Schifter et al., 1994; Rumsey and Ernst, 2000; Chugani, 2012; Zürcher et al., 2015; Hwang et al., 2017; Wolff et al., 2018; Girault and Piven, 2020; Meyer et al., 2020; Kowalewska et al., 2021).
The neuroimaging studies on ASD have been comprehensively summarized in several literatures. Due to the difference of time scale and research angle, the contents and focus in each review are different. Early published literature, such as the comprehensive systematic review by Zürcher et al. (2015), provided us with valuable references, but now a large number of new research results need to be supplemented. Some of the reviews published in recent years have focused on summarizing the state of ASD imaging research in general. For example, McPartland et al. (2021) recently published a review about “Looking Back at the Next 40 Years of ASD Neuroscience Research,” which discussed the most comprehensive analysis of the five most common neuroscience modalities employed in ASD research. Kowalewska et al. (2021) only stated consistent results from ASD studies by screening journal with high impact factors. Others, while covering several neuroimaging techniques, tend to focus on the application results of a specific neuroimaging technique in detail, such as Li et al. (2021) discuss more about MRI content.
As the foundation work of ASD PET probe development, and the need to constantly update and summarize the existing research results. The authors conducted a PubMed, EMBASE, Scopus and PsychINFO search of ASD PET literature published before 2022.01.01 using various combinations of “ASD,” “autism spectrum disorder” and “PET.” In this paper, we discuss cerebral glucose metabolism, cerebral blood perfusion, neurotransmitter system (precursors, transporters and receptors) and some new research targets, such as neuroinflammation related targets and arginine vasopressin receptor targets. By summarizing the clear pathophysiological mechanism of ASD and stating the unclear research conclusions, the application status and prospect of PET technology in ASD research were discussed. It provides a more comprehensive and detailed literature review for researchers in the field of ASD PET probe development, which is helpful for readers to grasp the status quo of ASD PET research more intuitively and conveniently, and hopefully provides some enlightenment for readers to explore new research directions.
Cerebral Glucose Metabolism
Glucose is the main energy source of brain cells and thus the state of glucose metabolism partly reflects changes in brain function. As a glucose analog, [18F]FDG can be taken up by brain cells that utilize high levels of glucose and undergo specific intracellular phosphorylation. Prior to radioactive decay, [18F]FDG is unable to complete intracellular glycolysis due to the loss of oxygen at site 2 and, therefore, imaging using [18F]FDG is a good reflection of glucose uptake and phosphorylation distribution in brain cells (Fowler and Ido, 2002). [18F]FDG is very valuable in ASD PET research due to its excellent imaging characteristics, including a long half-life, short scanning time, mature preparation process, and relatively simple scanning process (Zürcher et al., 2015).
The subject of brain glucose metabolism changes is among the most widely studied in ASD PET. The majority of experimental studies focus on differences in brain glucose metabolism between ASD patients and normal subjects. However, due to the differences in experimental subjects and design, there remains no consensus on changes of FDG metabolism in individuals with ASD. As early as 1985, Rumsey et al. (1985) conducted a brain [18F]FDG PET study on 10 adult males with autism and 15 typical developing controls, and found the cerebral glucose metabolism of the patients with autism was more diffuse. However, most [18F]FDG PET studies since then have found brain glucose metabolism in ASD is typically reduced in local brain tissue compared to typical developing controls; however, this is not true for specific brain regions (Buchsbaum et al., 1992; Chugani et al., 1996; Haznedar et al., 1997, 2000, 2006; Deriaz et al., 2012; Dilber et al., 2013; Manglunia and Puranik, 2016). In these cases, Chugani et al. (2007) conducted a [18F]FDG PET study on four autistic children with wine spotting, and decreased glucose metabolism in the bilateral medial temporal regions, anterior cingulate gyrus, frontal cortex, right temporal cortex, and cerebellum was observed. The frontal temporal lobe glucose metabolism asymmetry was opposite that of the typical child with autism. In a few studies, patients with ASD displayed both decreased and increased glucose metabolism in different brain regions (Siegel et al., 1992; Asano et al., 2001; Hazlett et al., 2004; Anil Kumar et al., 2017; Mitelman et al., 2018; Kadwa et al., 2019). In addition, earlier studies also reported no significant difference in cerebral glucose metabolism between the autism and control subjects (De Volder et al., 1987; Herold et al., 1988; Heh et al., 1989). As summarized in Table 2, there is no consensus on decreases or increases in glucose metabolism in specific brain regions in patients with ASD, however, the most common discoveries in brain glucose metabolism patterns in patients with ASD were decreases in glucose metabolism in the temporal lobe and abnormal metabolism in areas highly connected to the temporal lobe, such as the frontal lobe, parietal lobe, and adjacent limbic cortex. This is consistent with the anatomical connectivity between the supratemporal and associative cortexes (Seltzer and Pandya, 1978; Pandya and Yeterian, 1985; Sitoh and Tien, 1997) and is supported by findings on the brain functional network for glucose metabolism (Lee et al., 2011a,b, 2012).
[18F]FDG PET is not only used in the study of glucose metabolism changes in specific brain regions in patients with ASD, but also in evaluation by neuroimaging before and after ASD treatment. An [18F]FDG PET study conducted on five adult patients with autism and one with AS before and after fluoxetine treatment found the glucose metabolic rate in the right frontal lobe, especially in the anterior cingulate gyrus and orbitofrontal cortex, was significantly higher after treatment than before. In addition, patients who presented with higher glucose metabolic rates in the medial frontal lobe and anterior cingulate before treatment were more likely to respond well to fluoxetine, suggesting responses to fluoxetine can be predicted based on baseline cingulate metabolism (Buchsbaum et al., 2001). However, cerebral glucose metabolism after ASD treatment does not always show an increase in glucose metabolism. A [18F]FDG PET study in a 14-year-old boy with ASD assessing before and after bilateral deep brain stimulation (DBS) in the nucleus accumbens (NAc) found the patient’s clinical symptoms improved significantly after 2 years of treatment with NAc DBS. Brain [18F]FDG PET results showed glucose metabolism in the prefrontal, frontal, and occipital cortexes was significantly lower than before treatment (Park et al., 2017). Another [18F]FDG PET study assessing before and after treatment with a ketogenic diet (KD), which is a mature therapy for refractory epilepsy, in a 6-year-old child with ASD revealed bilateral local reductions in glucose metabolism in the proximal meso-temporal lobe, basal ganglia region, and cerebellum in patients with ASD. After 12 months on a KD, the patient’s glucose metabolism had decreased significantly and diffusely across the entire cerebral cortex compared to before KD (Żarnowska et al., 2018). The underlying reason for this result is the conversion of the main energy source of the brain cells from glucose to ketone bodies (Frye and Rossignol, 2014; Courchesne-Loyer et al., 2017). These findings suggest ASD therapy can improve clinical symptoms and alter the state of cerebral glucose metabolism in patients with ASD. The use of [18F]FDG PET to assess glucose metabolism levels before and after ASD treatment can not only help in effectively screening treatment subjects before clinical treatment, but also provide support for the evaluation of efficacy after clinical treatment. In addition, the effects of ASD drugs acting on 5-hydroxytryptamine, dopamine, gamma-aminobutyric acid, and other neurotransmitter systems with influence on brain glucose metabolism should be further explored.
Cerebral Blood Flow
The [15O]CO2 steady-state inhalation technique was used in the early stages of PET imaging to study cerebral perfusion, but its reliability is insufficient. Imaging with probes that have a small relative molecular weight and are uncharged and fat-soluble (mainly [15O]H2O) are now more preferable. These probes can enter brain cells through the blood–brain barrier, where the amount of probe entering brain cells is positively correlated with regional cerebral blood flow (rCBF). Because rCBF generally directly correlates with metabolism associated with local brain function, cerebral perfusion imaging can also reflect the state of local brain function to a certain extent. To measure rCBF, it is necessary to record the dynamic intracranial distribution of the probe after the probe enters the human body. Meanwhile, blood samples of the subjects are continuously collected to evaluate carotid artery input function. With the help of image processing, radiation concentration-time curves are obtained to calculate the rCBF value of the corresponding brain region (Joseph-Mathurin et al., 2018).
In early experiments, cerebral perfusion imaging was performed on six patients with autism and eight normal controls after nasal inhalation of [15O]CO2 and no difference in rCBF was found (Herold et al., 1988). These negative results may be due to the influence of [15O]CO2 absorption on cerebral perfusion imaging quality or limitations of early low-resolution PET cameras. High-resolution PET combined with statistical parameter mapping was able to find some local abnormalities which the low-resolution PET failed to detect (Boddaert et al., 2002). In subsequent experiments, intravenous injection of [15O]H2O was used for cerebral perfusion imaging on ASD patients. These researches conducted cerebral perfusion imaging while patients carried out various social functions with related tasks (such as emotional processing, theory of mind, and listening and speaking skills), and then image data analysis based on the statistical parameters of voxel was used to compare perfusion in distinct brain regions in stimulated patients with ASD and normal controls (Happé et al., 1996; Müller et al., 1998; Hall et al., 2003). According to different activation tasks, differences in the activation of regions based on the cerebral perfusion imaging results were usually related to behavioral functional control regions of the human brain. As the ability of patients with ASD to perform tasks varies greatly due to individual factors, such as age and IQ, the results of different experiments were not comparable (Table 3). However, some continuous studies have revealed rules for changes in brain function and rCBF in ASD. When [15O]H2O PET was used to study differences in rCBF in four adult patients with autism and five typical developing controls during a verbal task (Müller et al., 1998), the right dentate nucleus and left frontal region 46 were activated less during speech, hearing, and expressive language, and more during motor speech function in those with autism. The intergroup differences in the thalamus were consistent with those in region 46 during speech expression. In 1999, [15O]H2O PET was used to explore differences in rCBF between five highly functioning adult patients with autism and five typical developing controls during a verbal task (Müller et al., 1999). The results partially support atypical (diminished or reversed) hemispheric dominance of receptive language. In the patient group, hearing was associated with a significant reversal of normal left hemisphere dominance, while decreases in the bilateral superior temporal gyrus and cerebellar rCBF in those with autism suggests a decrease in cerebellar involvement in non-verbal auditory perception and possible expression of language. These results may indicate atypical functional specialization of the dentate-thalamo-cortical pathway and are consistent with a regional-specific biochemical disorder model of the brain in development of autism. This is in line with previous findings that dys-serotonin synthesis affects the dentate-thalamo-cortical pathway in boys with autism (Chugani et al., 1997). Three experiments using [15O]H2O to evaluate differences in rCBF between patients with autism and normal controls during a language task confirmed abnormal functioning of the temporal lobe region with decreased rCBF (mainly in the left temporal region) in those with autism, which is consistent with changes in the glucose metabolism pattern in brains with autism (Boddaert et al., 2002, 2003, 2004).
For task-state cerebral perfusion imaging, functional magnetic resonance such as arterial spin labeling (ASL) sequence has unique advantages (Saitovitch et al., 2019). In the future, joint imaging or comparative studies with PET and fMRI will have more widespread application prospects.
5-Hydroxytryptamine System
5-Hydroxytryptamine (5-HT), also known as serotonin, is an inhibitory neurotransmitter that regulates neuronal migration and synaptogenesis during brain development (Yang et al., 2014). Abnormalities of the 5-HT system in autism were first reported in 1961 (Schain and Freedman, 1961). Whole blood tests in 23 children with autism and severe mental disability showed 5-HT was significantly increased, as well as slightly increased in seven children with severe mental disability without autism. Four non-autistic and non-mentally handicapped children and 12 children with borderline or mild mental retardation had normal whole blood 5-HT concentrations. Follow-up studies confirmed the elevated whole blood 5-HT levels in patients with autism, and found that immediate family members of the patient also had elevated whole blood 5-HT levels (Hwang et al., 2017). In addition, 5-HT antagonists have been shown to lead to some improvement in autism-related symptoms, although not the core symptoms associated with autism (McDougle et al., 1996; Croen et al., 2011; Hollander et al., 2012). Given the overwhelming evidence of the association between the 5-HT system and ASD, 5-HT has become the most common neurotransmitter system assessed in studies of ASD. To summarize the current ASD-related 5-HT PET imaging studies, abnormalities of the 5-HT system in patients with ASD mainly manifest as an increase in 5-HT concentration in peripheral blood, disorder of 5-HT synthesis in the brain, a decrease in 5-HT transporters in the brain, and abnormal functioning of 5-HT receptors in the brain.
5-Hydroxytryptamine Precursor
Tryptophan hydroxylase is a precursor of 5-HT and α-methyl-L-tryptophan can specifically bind to tryptophan hydroxylase. Therefore, radio-labeled [11C]AMT has been widely used as a specific probe to measure 5-HT synthesis (Chakraborty et al., 1996; Chugani and Muzik, 2000). Unilateral changes in 5-HT synthesis in the dentate-thalamo-cortical pathway in boys with autism were analyzed with [11C]AMT PET (Chugani et al., 1997). Asymmetries in 5-HT synthesis were found in the frontal cortex, thalamus, and cerebellar dentate nucleus in all seven boys tested, but not in a girl with autism. Five of the boys with autism had reduced 5-HT synthesis in the left frontal cortex and thalamus, while the other two had reduced 5-HT synthesis in the right frontal cortex and thalamus. In all seven patients, elevated 5-HT synthesis was observed in the contralateral dentate nucleus. In addition, Chugani et al. (1999) also used [11C]AMT PET to measure 5-HT synthesis in 30 children of different ages with autism, 8 of their non-autistic siblings, and 16 non-autistic children with epilepsy and the mechanism underlying abnormal 5-HT synthesis in autism was further explored. The results showed that 5-HT synthesis in a non-autistic child before the age of 5 was more than twice that of an adult and then decreased to adult levels after the age of 5. However, whole-brain 5-HT synthesis decreased during childhood in children with autism, but increased gradually between the ages of 2 and 15 years, where it reached 1.5 times the normal adult value and showed no gender difference. There were significant age-dependent differences in 5-HT synthesis between the autism and epileptic groups, and the autism and sibling groups. Chandana et al. (2005) used [11C]AMT PET to explore the association between global and local abnormalities in serotonin synthesis and handedness and language function in children with autism. By measuring cerebral 5-HT synthesis in 117 children with autism, multiple abnormal cortical involvement patterns, including in the right cortex, left cortex, and non-lateralization, were observed. Children with autism who have reduced AMT binding in the left cortex showed greater severe language impairment, while there was a higher autism prevalence with reduced AMT binding in the right cortex among those that were left-handed and ambidextrous. Abnormal asymmetrical development of the serotonin system both globally and locally may result in faulty neural circuitry that specifies hemispheric specialization.
5-Hydroxytryptamine Transporter
The mechanism by which the human body regulates the 5-HT concentrations outside of brain cells is mainly through 5-hydroxytryptamine transporter (5-HTT), also known as serotonin transporter (SERT), which actively transfers 5-HT back to 5-hydroxytryptamergic neurons. Dysregulation of the 5-HT system in patients with ASD is also accompanied by dysregulation of 5-HTT, but the exact relationship between 5-HTT changes and ASD remains to be determined. Currently, PET probes used in the study of ASD-related 5-HTT anomalies mainly include [11C](+)McN5652, [11C]DASB, [18F]Fmener-D2, and [11C]MADAM.
[11C](+)McN5652 was the first probe used to successfully image 5-HTT in the human body despite the relatively low signal-to-noise ratio and has a leading role in the field of 5-HTT imaging in vivo (Suehiro et al., 1993). Nakamura et al. (2010) explored the differences in 5-HTT between 20 adult males with high-functioning autism and 20 typical developing controls by using [11C](+)McN5652 PET. The results showed 5-HTT levels in the whole brain of men with autism were lower than in normal men. Compared to [11C](+)McN5652, [11C]DASB is more widely used and accepted due to its high repeatability and reliability that are a result of its high-affinity reversible binding with 5-HTT (Wilson et al., 2002). However, studies using [11C]DASB to detect 5-HTT in ASD have yielded different results. Girgis et al. (2011) conducted a [11C]DASB PET study on 17 adult patients with ASD and 17 typical developing controls, and found no statistically significant differences in 5-HTT between groups. More recently, Andersson et al. (2020) used [11C]MADAM PET to study 15 adult patients with autism and 15 typical developing controls, and found 5-HTT availability was significantly lower in the gray matter, brain stem, and nine other gray matter subregions in the group with autism. The results further support previous research suggesting that 5-HTT density is reduced in autism (Lundberg et al., 2006). Differences in conclusions of various studies may be a result of the heterogeneity of study subjects, which also suggests there may be differences in the molecular imaging manifestations in patients with different ASD subtypes.
In addition, some probes targeting 5-HTT (such as [11C]ADAM, [11C]DAPA, and [11C]AFM) have demonstrated their effectiveness in animal and human studies, which would promote the exploration of the relationship between 5-HTT and ASD, and improve our understanding of the pathophysiological mechanisms of ASD (Huang et al., 2001, 2002).
5-Hydroxytryptamine Receptor
5-Hydroxytryptamine produces different physiological effects by stimulating different 5-HT receptor (5-HTR) subtypes. At present, 14 5-HTR subtypes have been found. The clear correlation between density changes in 5-HTR and ASD has not been clarified. By now, only the 5-HTR subtypes 5-HT2AR and 5-HT1AR have been used in ASD PET studies. The probes targeting 5-HT2AR include [11C]MDL100907, [18F]Setoperone, and [18F]Altanserin, and the probes targeting 5-HT1AR mainly include [18F]MPPF and [18F]F13714.
[18F]Setoperone is a reliable probe with high specificity for 5-HT2AR (Blin et al., 1990). Beversdorf et al. (2012) used [18F]Setoperone PET to study six patients with autism and 10 typical developing controls, and found there was less [18F]Setoperone binding in the thalamus of patients than in the control group with no significant differences in other regions. Goldberg et al. (2009) used [18F]Setoperone PET to study 19 parents of children with autism and 17 typical developing controls. The results showed that the cortical 5-HT2AR binding potential (BPND) of the parents of the child with autism was significantly lower than that of the control group. Platelet 5-HT levels were significantly negatively correlated with the cortical 5-HT2AR BPND in the parents of the child with autism. The parents of the child with autism had low cortical 5-HT2AR density, consistent with reports of reduced 5-HT2AR expression and function in patients with ASD. It also further verified the pathophysiological mechanism of elevated 5-HT level in peripheral blood of ASD patients. In another study, the probe [11C]MDL100907 was used to characterize 5-HT2AR in AS. Imaging of 17 adult patients with AS and 17 typical developing controls showed that there was no statistical difference in regional [11C]MDL100907 BPND (Girgis et al., 2011).
To explore the relationship between 5-HT1AR binding potential, gray matter volume (GMV), and social personality, a PET study was performed on 18 adult patients with ASD (7 with Autism and 11 with AS) and 24 typical developing controls using a 5-HT1AR-specific probe [18F]MPPF (Lefevre et al., 2020). 5-HT1AR BPND had a regional negative correlation with GMV, although no difference in 5-HT1AR density was observed between the two groups. However, there were specific associations between 5-HT1AR, GMV, and social personality scores in the striatum of the control group not found in the ASD group. This suggests that there is a 5-HT system disturbance associated with 5-HT1AR density changes in the striatum of patients with ASD.
In addition to 5-HT2AR and 5-HT1AR, the application of PET probes targeting other subtypes of 5-HT receptors in ASD research can also be explored. Further probe development and quantitative imaging studies of 5-HTR will help improve our understanding of the pathogenesis of ASD (Zürcher et al., 2015).
Oxytocin
Oxytocin (OXT) is a neuropeptide that plays an important role in the regulation of social behavior in mammals by interacting with oxytocin receptor (OTR). Previous studies have found abnormal OXT levels in patients with autism (Modahl et al., 1998). In addition, a large number of studies has confirmed intranasal OXT treatment can improve social behavior in autism. A study by Guastella et al. (2010) showed OXT treatment through intranasal inhalation could improve performance in an eye-mind task in those with autism. FMRI studies on children and adult patients with autism also found several brain regions, including the striatum, showed improved responses to stimuli after OXT treatment (Domes et al., 2013; Gordon et al., 2013).
Unfortunately, PET probes targeting OTR satisfactorily have yet to be successfully developed. The probes [11C]EMPA (Wang et al., 2013) and [11C]PF-3274167 (Vidal et al., 2017) have not been under continued use due to unsatisfactory physical and chemical properties. As OXT and 5-HT are structurally and functionally integrated, they collectively mediate emotion-based behavior. The amygdala is the center of OXT regulation of 5-HT. OXT can inhibit amygdala activity and decrease anxiety, while high amygdala activity and dysregulation of 5-HT are associated with increased anxiety. Therefore, some investigations about the mechanisms by which OXT plays a role in ASD by measuring changes in the human 5-HT system before and after OXT treatment were conducted. Mottolese et al. (2014) used [18F]MPPF PET to detect changes in 5-HT1AR in the brains of 24 healthy subjects taking OXT or a placebo, and found OXT increased [18F]MPPF BPND in the dorsal raphe nucleus (the core region of 5-HT synthesis), amygdala/hippocampus complex, insula, and orbitofrontal cortex. In a [18F]MPPF PET study of 18 adult patients with ASD and 24 typical developing controls, Lefevre et al. (2018) found no difference in the concentration and distribution of 5-HT1AR between patients with ASD and controls. However, OXT significantly increased [18F]MPPF BPND in some brain regions in the control group, but not in the ASD group. Hirosawa et al. (2017) imaged 10 adult males with autism men before and after OXT treatment using [11C]DASB PET. A significant increase in [11C]DASB BPND in the left inferior frontal gyrus was observed after OXT treatment and extended to the left middle frontal gyrus in those with autism. However, there was no significant correlation between changes in clinical symptoms and [11C]DASB BPND. These studies revealed a partial role for OXT in inhibition of 5-HT signaling, as well as a partial relationship between changes in the serotonergic system and prosociality after OXT therapy. Further studies are needed to verify the underlying relationship between OXT and ASD.
Dopamine System
As the most abundant catecholamine neurotransmitter in the brain, dopamine (DA) is involved in the regulation of various physiological functions of the central nervous system, including social reward and social motivation. A number of genetic studies on patients with autism have reported an association between DA transporter and receptor-associated gene mutations and ASD-associated clinical symptoms (Gadow et al., 2008; Staal et al., 2012; Hamilton et al., 2013). In addition, the efficacy of psychotherapeutic agents targeting the DA system in improving clinical symptoms in patients with ASD suggests that the DA system plays a role in mediating behavior in these patients. The DA system is an important focus of ASD neuroimaging research. There have been many PET studies on the DA system, but there is no consensus on the exact changes that occur for DA precursor, transporters, and receptors in the brains of patients with ASD.
Dopamine Precursor
Dopa is the precursor of DA, and can be absorbed, metabolized, and stored by dopaminergic endings. Imaging with [18F]FDOPA reflects DA synthesis levels in the brain. Ernst et al. (1997) studied the differences in brain DA synthesis between 14 children with autism and 10 neurotypical children using [18F]FDOPA PET. The DA ratios in the caudate nucleus, putamen, midbrain, and lateral and medial prefrontal regions (areas rich in dopaminergic endings) compared to the occipital cortex (area lacking dopaminergic endings) were calculated. The DA ratio in anterior medial prefrontal cortex/occipital cortex displayed a 39% decrease in those with autism compared with the neurotypical children. However, there were no significant differences in other measured areas. When [18F]FDOPA PET was used in eight adult patients with AS and five typical developing controls, the [18F]FDOPA inflow value (Ki) in the striatum and frontal cortex in those with AS was increased compared the normal control group (Nieminen-von Wendt et al., 2004). However, a recent study comparing 44 adult patients with ASD and 22 typical developing controls using [18F]FDOPA PET did not find significant inter-group differences in the striatum’s ability to produce dopamine (Schalbroeck et al., 2021a,b). The results of these studies are inconsistent, which is most likely related to the heterogeneity of the study subjects.
Dopamine Transporter
In a previously mentioned study of 20 high-functioning adult patients with autism and 20 typical developing controls, DAT was measured using [11C]WIN-35,428 in addition to imaging 5-HTT using [11C]McN-5652. The results showed that patients with autism had significantly higher DAT binding in the orbital prefrontal cortex. In addition, DAT binding in the orbitofrontal cortex was inversely correlated with 5-HTT binding in those with autism (Nakamura et al., 2010).
Dopamine Receptor
Fernell et al. (1997) conducted a PET study on six children with autism before and after treatment with R-BH4, a cofactor of tyrosine hydroxylase in the catecholamine and serotonin biosynthesis pathways, using [11C]NMS. With improvement in symptoms, dopamine receptor 2 (D2R) in the caudate and putamen of children with autism was decreased by approximately 10% after R-BH4 treatment from abnormally high pre-treatment levels to normal levels. Fujino et al. (2020) investigated differences in the dopamine receptor 1 (D1R) between adults with autism and typical developing controls using [11C]SCH23390 PET, and found D1R binding in the striatum, anterior cingulate cortex, and temporal cortex was significantly negatively correlated with ASD detail attention scores and significantly positively correlated with emotional perception scores in those with autism. However, there was no significant intergroup differences in D1R binding in the striatum, anterior cingulate cortex, and temporal cortex. The latest study compared 10 adult patients with ASD with 12 typical developing controls using [11C]raclopride PET (Zürcher et al., 2021). Compared with the control group, the ASD group showed reduced D2R/D3R binding in bilateral putamen and left caudate nucleus.
Amino Acid Neurotransmitters
Protein synthesis is necessary for many important processes in the brain, including synaptic plasticity, long-term memory, and experience-dependent development. Based in part on the association between ASD and single-gene mutations, such as in glial 3/4, neuroprotein 1, and shank 3, atypical synapse protein synthesis is thought to play a role in neurodevelopmental disorders, including ASD (Kelleher and Bear, 2008). Since amino acids are the basic components of proteins, the quantitative study of amino acids and its receptors has revealed some features of abnormal protein synthesis in ASD.
Gamma-Aminobutyric Acid
Gamma-aminobutyric acid (GABA) is a major inhibitory neurotransmitter in the brain and plays a role in synaptic pruning and regulation of development. GABA dysfunction may lead to an imbalance in excitation/inhibition of the nervous system, a phenomenon associated with ASD (Pizzarelli and Cherubini, 2011). Previous human genetics studies found mutations in the GABAA receptor gene and genes related to GABA synthesis in those with autism (Cook et al., 1998; Coghlan et al., 2012). Autoradiography studies revealed reduced GABAA in the hippocampus and anterior cingulate cortex of patients with autism (Blatt et al., 2001; Oblak et al., 2009). Studies on rodent models of ASD have found prolonged neuronal excitation caused by early GABA inhibition may be an underlying mechanism in ASD (Tyzio et al., 2014). Although a large body of evidence suggests changes in GABA may be associated with ASD, there are currently very few PET studies on GABA.
Mendez et al. (2013) used [11C]RO15-4513 PET to measure GABAA receptor α1 and α5 subtype levels in three high-functioning adult patients with autism and three typical developing controls, and found that binding of [11C]RO15-4513 was significantly reduced in the brains of those with autism. Region of interest analysis also revealed significant reductions in the bilateral amygdala and NAc, a result associated with low levels of the GABAA α5 subtype. These results provide preliminary evidence for the deletion of GABAA α5 in autism and support the further study of ASD-related GABA system abnormalities. However, when [18F]FMZ was used in 15 adult patients with ASD and 15 typical developing controls, and [11C]RO15-4513 in 12 adult patients with ASD and 16 typical developing controls for PET, there were no differences found in the availability of GABAA receptors or GABAA α5 subtype in any region of the brain in adults with or without ASD (Horder et al., 2018). Fung et al. (2020) measured total GABAA receptor densities in 28 adults patients with ASD and 29 typical developing controls using [18F]FMZ PET and GABA concentrations using 1H-MRS. Based on the bilateral thalamus and left dorsolateral prefrontal cortex (DLPFC) as the study areas, [18F]FMZ PET revealed no differences in GABAA receptor density between the ASD and control groups. However, 1H-MRS measurements showed the GABA/Water ratio (GABA normalized to Water signal) in the left DLPFC of patients with ASD was significantly higher than in the control group. When it comes to the role of GABA in ASD pathogenesis, PET research still has a broad area to explore.
Glutamate
Based on the theory that there is an imbalance in brain excitation/inhibition in individuals with ASD, changes in glutamate are also an important focus of ASD neuroimaging research (Rubenstein and Merzenich, 2003). Currently, there are some probes with satisfactory radiochemical properties that can be applied to ASD studies on glutamate receptors, such as [18F]FPEB, [11C]ITMM, and [11C]MMPIP, which binds specifically to metabolic glutamate receptor 5 (mGluR5), metabolic glutamate receptor 1 (mGluR1), and metabolic glutamate receptor 7 (mGluR7), respectively (Barret et al., 2010; Sullivan et al., 2013; Toyohara et al., 2013; Wong et al., 2013). However, only [18F]FPEB has been used in clinical ASD PET research.
Metabolic glutamate receptor 5 visualization and animal model studies based on [18F]FPEB PET have confirmed the association between ASD and glutamate system imbalance. [18F]FPEB PET imaging of a Shank3 complete knockout mouse model (Shank3B-/-) showed that BPND of mGluR5 was significantly increased in the hippocampus, thalamus, striatum, and amygdala in Shank3B-/- mice compared with normal mice (Cai et al., 2019). Fatemi et al. (2018) used [18F]FPEB PET to study six adult patients with autism and three typical developing controls and found there were significantly higher [18F]FPEB BPND in the posterior central gyri and cerebellum of those with autism. There was a significant negative correlation between age and [18F]FPEB BPND in the cerebellum, but no significant negative correlation with the posterior central gyrus. Precuneus [18F]FPEB BPND was positively correlated with the sleepiness scale score on the Abnormal Behavior Checklist (ABC), while cerebellar [18F]FPEB BPND was negatively correlated with ABC total score, ABC hyperactivity subscale, and ABC inappropriate speech subscale. These new findings provide the first evidence that mGluR5 binding is altered in key regions of brains of those with autism, suggesting abnormal glutamate signaling in these regions. The correlations between [18F]FPEB binding potential changes in the cerebellum and precuneus suggest some ASD symptoms may be influenced by abnormal glutamate signaling. In a recent study, Brašić et al. (2021) used [18F]FPEB PET to compare the density and distribution of mGluR5 in the brains of patients with idiopathic autism spectrum disorder (IASD), fragile X syndrome (FXS), and TD. The results showed that the expression of mGluR5 in cortical regions of IASD patients was significantly increased compared with TD patients, while the expression of mGluR5 in all regions of FXS patients was significantly decreased compared with TD patients.
In addition, probes targeting mGluR1 and mGluR7 with good kinetic properties are currently under development and future imaging studies on ASD will reveal more about the pathophysiological mechanisms of glutamate’s role in ASD.
Leucine
Leucine content affects the rate of protein synthesis in neurons and has a physiologically significant role in regulation of dendritic spines, where defects in the latter are associated with neuropsychiatric diseases (Shih and Hsueh, 2018). Shandal et al. (2011) used [11C]Leucine PET to study overall brain protein synthesis in stunted children with and without PDD and measured the involvement of leucine in protein synthesis. It was determined that protein synthesis in the temporal lobe language region of stunted children with PDD was increased. Notably, previous cerebral perfusion imaging studies on patients with ASD also suggested there are abnormalities in the temporal lobe region and abnormal protein synthesis in the language region of children with developmental delay and PDD may be related to widespread symptoms. However, a new study used [11C] Leucine PET to measure cerebral protein synthesis in nine young patients with fragile X syndrome and 14 typical developing controls, no significant differences were found between the two groups (Schmidt et al., 2020). In addition, a robust radiolabeled assay was applied to measure rate of protein synthesis in peripheral blood mononuclear cells (PMCS) and platelets in 13 patients with fragile X syndrome and 14 typical developing controls by Dionne et al. (2021). The results showed that the protein synthesis rate was decreased in patients with fragile X syndrome. These findings challenge the reports of increased protein synthesis in fragile X syndrome. It indicates that more research is needed to explore the leucine abnormality associated with ASD.
Acetylcholine
Acetylcholinesterase (AChE) inhibitors have been reported to reduce aggression and inattention, as well as overall ASD symptoms in pharmacological studies and animal models of ASD (Hardan and Handen, 2002; Nicolson et al., 2006; Karvat and Kimchi, 2014). These studies provide evidence for the critical role of the cholinergic system in the etiology of ASD. However, there is currently only one PET study applied to these patients. When the acetylcholine analog [11C]MP4A was used to measure acetylcholinesterase activity, the main finding was a lack of cholinergic innervation in the fusiform gyri in adult patients with autism (Suzuki et al., 2011). Additional targeted PET studies in the future will help clarify the role of cholinergic dysfunction in the pathogenesis of ASD.
Neuroinflammation
Immune-mediated mechanisms are considered to be among the pathophysiological factors leading to ASD (Stigler et al., 2009). Imaging studies, specimen studies, and animal models have revealed central nervous system inflammation, including microglial activation and underlying microglial pathology, in patients with neuropsychiatric disorders such as ASD, depression, and schizophrenia. In addition, various psychotropic drugs are thought to have a direct effect on microglia. However, the relationship between microglia, neurotransmitters, and neuropsychiatric disorders has not been fully elucidated to date (Kato et al., 2013).
Microglia play an important role in the growth and development of the central nervous system and immunity. Activated microglia overexpress the translocator protein (TSPO), which is the most common imaging biomarker for neuroinflammation. TSPO probes have been continuously in development, with the first generation being [11C]PK11195, the second generation [11C]DPA713, [18F]FEPPA, and [11C]PBR28, and the third generation [11C]ER176 and [18F]GE180 (Best et al., 2019; Werry et al., 2019). However, the use of these probes in ASD studies has been rarely reported. Using the first-generation TSPO probe [11C]PK11195, Suzuki et al. (2013) studied differences in microglia activation between 20 18- to 31-year-old high-functioning patients with ASD and 20 age and IQ matched controls. Increased binding of [11C]PK11195 in the fusiform gyrus, prefrontal cortex, cingulate cortex, midbrain and cerebellum was also reported in ASD patients. When 15 adult patients with ASD were compared with 18 typical developing controls using the second-generation TSPO probe [11C]PBR28, those with ASD were found to have lower regional TSPO expression in multiple brain regions, including the bilateral insular cortex, bilateral precuneus/posterior cingulate gyrus, bilateral temporal gyrus, angular gyrus, and superior limbic gyrus (Zürcher et al., 2020). The results of these two studies are inconsistent, which might be due to the heterogeneity of ASD patients, such as age, gender, IQ and subtype, and the influence of TSPO gene polymorphism in patients should also be considered. The efficacy of other second- and third-generation TSPO probes has been tested in studies of other neurological and psychiatric disorders and future imaging studies of patients with ASD will help to enrich our understanding of the pathophysiological mechanisms of ASD and understand disparities between previous studies (Owen et al., 2010; Fujita et al., 2017; Hafizi et al., 2017; Kobayashi et al., 2018).
In addition, due to the importance of neuroinflammation imaging, some promising new targets and corresponding probes are being explored. For example, monoamine oxidase B (MAO-B) and its specific probe [11C]SL25.1188, cyclooxygenase (COX)-1 and its specific probe [11C]PS13, cyclooxygenase (COX)-2 and its specific probe [11C]MC1, colony-stimulating factor 1 receptor (CSF1R) and its specific probe [11C]CPPC, and purinergic P2X7 receptor (P2X7R) and its specific probes [11C]JNJ-54173717 (JNJ-717), [18F]JNJ-64413739, and [11C]SMW139 (Figure 1). The key roles of these targets in neuroinflammation and the effectiveness of imaging using these probes have been demonstrated in previous experiments and their application will open up new directions in the study of neuroimaging of ASD (Meyer et al., 2020).
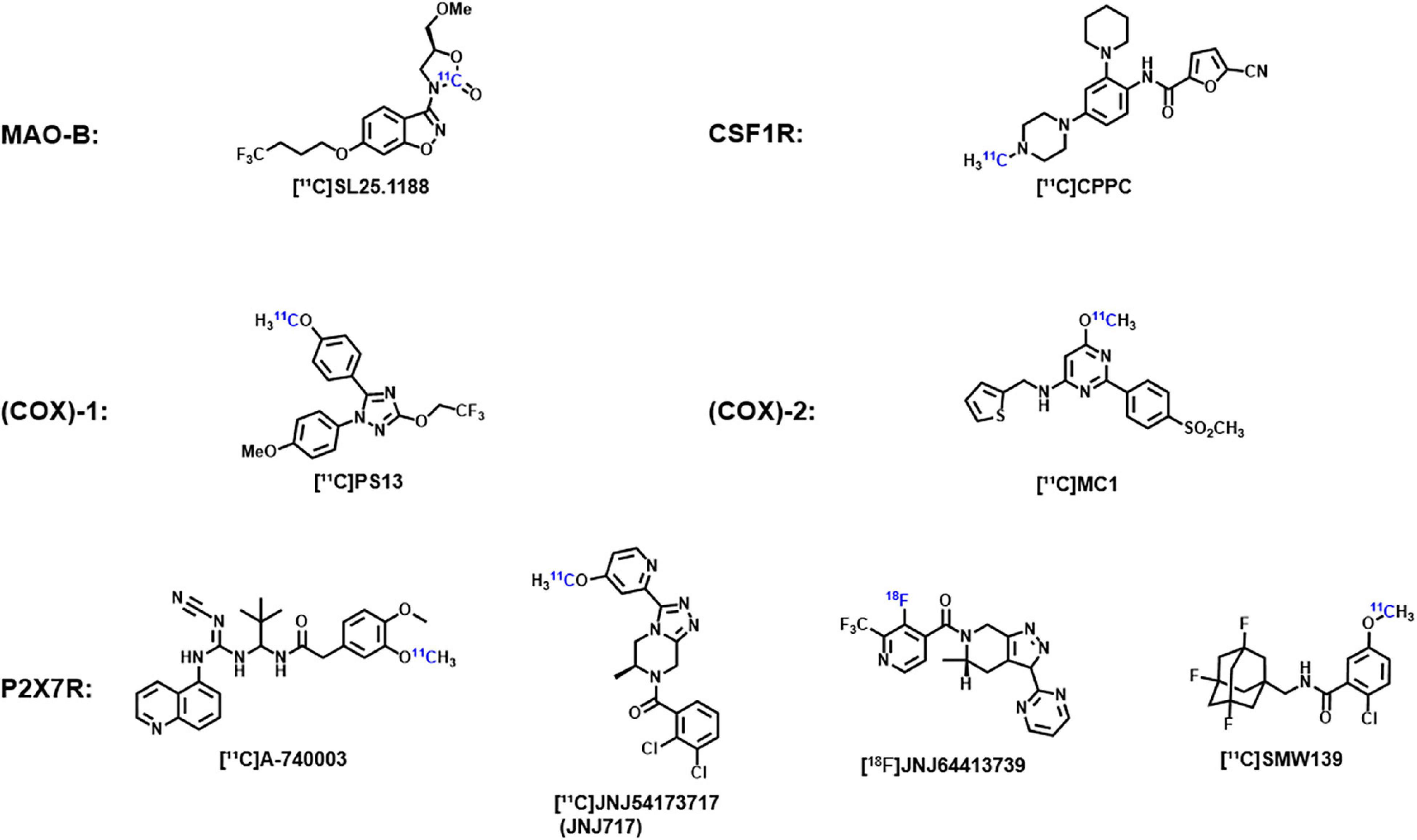
Figure 1. Chemical structures of the corresponding probes of monoamine oxidase B, cyclooxygenase-1, cyclooxygenase-2, colony-stimulating factor 1 receptors, and purinable P2X7 receptors.
Arginine Vasopressin
Arginine vasopressin (AVP) is a non-peptide neuroendocrine hormone synthesized by neurons in the hypothalamus. AVP interacts with the three G-protein-coupled receptors V1a/V1b/V2, and plays a variety of physiological roles in mammals including being an antidiuretic, increasing blood pressure, releasing adrenal corticosteroid, and regulating social behavior (Koob and Bloom, 1982; Jard, 1998). Since early animal studies have found AVP plays an important role in regulating biosocial behaviors, including mating, parentage, sociability and aggression, an increasing number of studies have focused on the correlation between AVP and ASD (Goodson and Thompson, 2010; Kelly and Goodson, 2014; Caldwell, 2017). Although no consistent conclusions have been reached regarding the differences in AVP concentrations between patients with ASD and normal controls, multiple human studies on the correlation between AVP and ASD suggest that AVP and its signaling pathways have a promising future in supporting ASD diagnosis and drug development (Miller et al., 2013; Shou et al., 2017; Parker et al., 2018; Wilczyński et al., 2019). Some recent studies have shown V1a receptor antagonists can modulate the action of AVP, thereby affecting the core symptoms of ASD. Balovaptan, a novel V1a receptor antagonist developed by Roche, showed improvement on the Vineland-II Adaptive Behavior Scale (including secondary endpoints of communication, social interaction and daily living skills) in a 12-week Phase 2 study of adults with ASD (Bolognani et al., 2019). The drug was therefore approved by the U.S. Food and Drug Administration in January 2018 for phase III clinical trials in adults with ASD and completed in July 2020. However, as of June 2020, balovaptan was discontinued in phase II clinical trials of ASD treatment in children and adolescents due to its ineffectiveness in this population (Schnider et al., 2020). Although the V1a receptor has been a new focus of ASD research, only [18F]SRX246 and [11C]SRX246 (Fabio et al., 2012), [11C](1S,5R)-1 (Naik et al., 2017), [11C]PF-184563 (Haider et al., 2021) PET probes targeting the V1a receptor have been recently developed (Figure 2). These PET probes have demonstrated their radiochemical properties in autoradiography and animal experiments, but have not yet been utilized in human experiments. The subsequent development of PET probes that can be applied to ASD studies will further reveal the role of AVP in the pathophysiological mechanisms of ASD.
Conclusion
Autism spectrum disorder is a disease caused by multiple factors, both heredity and environmental, and its core cause remains unclear. Early diagnosis is very important for the treatment of ASD. Earlier and clearer diagnosis and intervention are conducive to improving the clinical symptoms of ASD in patients, which is an important influencer on treatment efficacy. Application of PET can dynamically and quantitatively evaluate resting and task states, cerebral glucose metabolism before and after treatment, cerebral blood flow perfusion, and neurotransmitter system biomarkers. It can also aid exploration of the pathophysiology of ASD and auxiliary of ASD diagnosis and treatment, and promote drug research and development. The discovery of ASD biomarkers and development of corresponding PET probes is the key to ASD PET research, which plays an important role in clinical diagnosis, drug development, and efficacy evaluation. Currently, studies have found biomarkers, such as those involved in glucose metabolism, cerebral blood perfusion, neurotransmitter systems (Table 4), and neuroinflammation, associated with ASD. However, ASD is a general designation of a group of neurodevelopmental disorders. Differences in subjects (e.g., age, gender, and IQ), experimental design (e.g., awake/sleep/anesthesia, resting-state/task-state, and imaging conditions), and different ASD subtypes may lead to contradictory conclusions among different studies (Lombardo et al., 2019). Although the clinical diagnostic criteria for autism, Asperger’s, atypical autism/unclassified pervasive developmental disorder, and disintegrated disorder in children are grouped into ASD for unified diagnosis and treatment based on behavioral definitions, the results of existing PET imaging studies suggest there may be differences in the pathophysiological mechanisms at the molecular level. For example, in PET studies of 5-HT and DA systems, imaging results of patients with AS are often inconsistent with those of patients with autism. This is a limitation of current cross-sectional studies on ASD, which may cause unexpected problems in subsequent research. Therefore, future ASD PET studies may be required to explore whether there are differences in the pathophysiological mechanisms in different subtypes of ASD at the molecular level. The results will serve as valuable references for clinical diagnosis and treatment of ASD, and will further guide the design and selection of experimental subjects, and maintain consistency in experimental methods in subsequent studies (Hong et al., 2020).
In this paper, we summarize several targets, including cerebral glucose metabolism, cerebral blood perfusion, neurotransmitter system, neuroinflammation, arginine vasopressin receptor, as well as the corresponding positron probe and existing research results, which can be applied in ASD imaging studies. It can be seen that due to the repeated emphasis on the heterogeneity of ASD patients, PET studies using different probes can find some common results, while PET studies using the same probe will also produce different results. Just as TSPO gene polymorphism affects the imaging efficiency of different PET probes, the heterogeneity of ASD patients also strongly affects the consistency of probe imaging results for each target (Owen et al., 2012). Therefore, although it is important to explore new biomarkers and develop PET probes for ASD research, it is difficult to evaluate which probes have more application value in the existing system. Rather than using a probe to look for common results within the broad basket of ASD, it is more valuable to look for and establish a specific association between a biomarker, a probe, and a specific subtype of ASD at the molecular level would be more valuable (McPartland et al., 2020). This is the biggest challenge for ASD positron probe development and a more valuable exploration direction.
In-depth studies based on PET molecular imaging can provide a new perspective for the diagnosis and treatment of ASD, but there are also some difficulties. PET/CT and PET/MR imaging require patients to lie on a machine for scanning after injection of the probe. The whole imaging process requires protection from some radiation, long preparation and scanning period, and high coordination of patients. ASD usually presents during childhood, besides, patients have a large age span and significant differences in IQ. To carry out resting or task state PET imaging research, it is often necessary for professional technicians to carry out targeted training on patients, including on drug injection, adaptation to the scanning environment, and familiarity with the task process and, if necessary, anesthesia operation. These problems are not necessary to overcome for ASD experimental research, but also for clinical practice. It requires the joint efforts of doctors, technicians, nurses, and researchers in nuclear medicine, neuropsychiatry, rehabilitation, and other specialties to promote continuous advancements in ASD diagnosis and treatment research.
Limitations
This paper mainly focused on the PET imaging research in neuroimaging of ASD, including the targets and corresponding PET probes. However, ASD is a general term for a group of developmental disorders. The time span of literature research is long, the difference in diagnostic criteria is large, and the heterogeneity of research objects is obvious. These factors lead to the poor comparability of the conclusions from the relevant imaging studies. In addition, the reliability of PET methodology and imaging efficiency of various PET probes (i.e., time window for data acquisition, in vivo specificity, test–retest reliability) are also important factors leading to the confusion about the current ASD research results. In the future, categorizing and analyzing the sample size, age, gender, IQ, ASD subtype and other influencing factors of subjects by single factor control will be conducive to better understanding the research outcomes and exploring new research ideas. In addition, the research results only relying on one neuroimaging technique are limited, and the combination of multiple imaging modalities is a more valuable direction.
Author Contributions
ZT, HW, and XS contributed to the writing of the manuscript. WM and JY collect and sort out literatures. WY and XL contributed to the English language editing. LH and SZ constructed the figures and tables. SY provided constructive suggestions. HX and LW conceived the project and modified the manuscript. All authors contributed to the article and approved the submitted version.
Funding
This work was financially supported by the National Natural Science Foundation of China (Grant No. 82071974) and the Science and Technology Program of Guangzhou, China (202206010106).
Conflict of Interest
The authors declare that the research was conducted in the absence of any commercial or financial relationships that could be construed as a potential conflict of interest.
Publisher’s Note
All claims expressed in this article are solely those of the authors and do not necessarily represent those of their affiliated organizations, or those of the publisher, the editors and the reviewers. Any product that may be evaluated in this article, or claim that may be made by its manufacturer, is not guaranteed or endorsed by the publisher.
Acknowledgments
We gratefully acknowledge the support of K.C. Wong Education Foundation (China).
References
American Psychiatric Association (2013). Diagnostic and Statistical Manual of Mental Disorders (DSM-5), 5th Edn. Arlington, VA: American Psychiatric Association.
Andersson, M., Tangen, Ä, Farde, L., Bölte, S., Halldin, C., Borg, J., et al. (2020). Serotonin transporter availability in adults with autism-a positron emission tomography study. Mol. Psychiatry 26, 1647–1658. doi: 10.1038/s41380-020-00868-3
Anil Kumar, B. N., Malhotra, S., Bhattacharya, A., Grover, S., and Batra, Y. K. (2017). Regional cerebral glucose metabolism and its association with phenotype and cognitive functioning in patients with Autism. Indian J. Psychol. Med. 39, 262–270. doi: 10.4103/0253-7176.207344
Asano, E., Chugani, D. C., Muzik, O., Behen, M., Janisse, J., Rothermel, R., et al. (2001). Autism in tuberous sclerosis complex is related to both cortical and subcortical dysfunction. Neurology 57, 1269–1277. doi: 10.1212/wnl.57.7.1269
Barret, O., Tamagnan, G., Batis, J., Jennings, D., Zubal, G., Russel, D., et al. (2010). Quantitation of glutamate mGluR5 receptor with 18F-FPEB PET in humans. NeuroImage 52, S202. doi: 10.1016/j.neuroimage.2010.04.164
Best, L., Ghadery, C., Pavese, N., Tai, Y. F., and Strafella, A. P. (2019). New and Old TSPO PET radioligands for imaging brain microglial activation in neurodegenerative disease. Curr. Neurol. Neurosci. Rep. 19:24. doi: 10.1007/s11910-019-0934-y
Beversdorf, D. Q., Nordgren, R. E., Bonab, A. A., Fischman, A. J., Weise, S. B., Dougherty, D. D., et al. (2012). 5-HT2 receptor distribution shown by [18F] setoperone PET in high-functioning autistic adults. J. Neuropsychiatry Clin. Neurosci. 24, 191–197. doi: 10.1176/appi.neuropsych.11080202
Bjørklund, G., Kern, J. K., Urbina, M. A., Saad, K., El-Houfey, A. A., Geier, D. A., et al. (2018). Cerebral hypoperfusion in autism spectrum disorder. Acta Neurobiol. Exp. (Wars) 78, 21–29.
Blatt, G. J., Fitzgerald, C. M., Guptill, J. T., Booker, A. B., Kemper, T. L., and Bauman, M. L. (2001). Density and distribution of hippocampal neurotransmitter receptors in autism: an autoradiographic study. J. Autism Dev. Disord. 31, 537–543. doi: 10.1023/a:1013238809666
Blin, J., Sette, G., Fiorelli, M., Bletry, O., Elghozi, J. L., Crouzel, C., et al. (1990). A method for the in vivo investigation of the serotonergic 5-HT2 receptors in the human cerebral cortex using positron emission tomography and 18F-labeled setoperone. J. Neurochem. 54, 1744–1754. doi: 10.1111/j.1471-4159.1990.tb01229.x
Boddaert, N., Barthélémy, C., Poline, J. B., Samson, Y., Brunelle, F., and Zilbovicius, M. (2005). Autism: functional brain mapping of exceptional calendar capacity. Br. J. Psychiatry 187, 83–86. doi: 10.1192/bjp.187.1.83
Boddaert, N., Belin, P., Chabane, N., Poline, J. B., Barthélémy, C., Mouren-Simeoni, M. C., et al. (2003). Perception of complex sounds: abnormal pattern of cortical activation in autism. Am. J. Psychiatry 160, 2057–2060. doi: 10.1176/appi.ajp.160.11.2057
Boddaert, N., Chabane, N., Barthélemy, C., Bourgeois, M., Poline, J. B., Brunelle, F., et al. (2002). Bitemporal lobe dysfonction in infantile autism: positron emission tomography study. J. Radiol. 83(12 Pt. 1), 1829–1833.
Boddaert, N., Chabane, N., Belin, P., Bourgeois, M., Royer, V., Barthelemy, C., et al. (2004). Perception of complex sounds in autism: abnormal auditory cortical processing in children. Am. J. Psychiatry 161, 2117–2120. doi: 10.1176/appi.ajp.161.11.2117
Bolognani, F., Rubido, M. d. V., Squassante, L., Wandel, C., Derks, M., Murtagh, L., et al. (2019). A phase 2 clinical trial of a vasopressin V1a receptor antagonist shows improved adaptive behaviors in men with autism spectrum disorder. Sci. Transl. Med. 11:eaat7838. doi: 10.1126/scitranslmed.aat7838
Brasic, J. R., and Holland, J. A. (2006). Reliable classification of case-control studies of autistic disorder and obstetric complications. J. Dev. Phys. Disabil. 18, 355–381. doi: 10.1007/s10882-006-9021-9
Brasic, J. R., and Holland, J. A. (2007). A qualitative and quantitative review of obstetric complications and autistic disorder. J. Dev. Phys. Disabil. 19, 337–364. doi: 10.1007/s10882-007-9054-8
Brasić, J. R., Barnett, J. Y., Kowalik, S., Tsaltas, M. O., and Ahmad, R. (2004). Neurobehavioral assessment of children and adolescents attending a developmental disabilities clinic. Psychol. Rep. 95(3 Pt. 2), 1079–1086. doi: 10.2466/pr0.95.3f.1079-1086
Brašić, J. R., Nandi, A., Russell, D. S., Jennings, D., Barret, O., Martin, S. D., et al. (2021). Cerebral expression of metabotropic glutamate receptor subtype 5 in idiopathic autism spectrum disorder and fragile X syndrome: a pilot study. Int. J. Mol. Sci. 22:2863. doi: 10.3390/ijms22062863
Buchsbaum, M. S., Hollander, E., Haznedar, M. M., Tang, C., Spiegel-Cohen, J., Wei, T. C., et al. (2001). Effect of fluoxetine on regional cerebral metabolism in autistic spectrum disorders: a pilot study. Int. J. Neuropsychopharmacol. 4, 119–125. doi: 10.1017/s1461145701002280
Buchsbaum, M. S., Siegel, B. V. Jr., Wu, J. C., Hazlett, E., Sicotte, N., Haier, R., et al. (1992). Brief report: attention performance in autism and regional brain metabolic rate assessed by positron emission tomography. J. Autism Dev. Disord. 22, 115–125. doi: 10.1007/bf01046407
Cai, G., Wang, M., Wang, S., Liu, Y., Zhao, Y., Zhu, Y., et al. (2019). Brain mGluR5 in Shank3B(-/-) mice studied with in vivo [(18)F]FPEB PET imaging and ex vivo immunoblotting. Front. Psychiatry 10:38. doi: 10.3389/fpsyt.2019.00038
Caldwell, H. K. (2017). Oxytocin and vasopressin: powerful regulators of social behavior. Neuroscientist 23, 517–528. doi: 10.1177/1073858417708284
Castelli, F., Frith, C., Happé, F., and Frith, U. (2002). Autism, Asperger syndrome and brain mechanisms for the attribution of mental states to animated shapes. Brain 125(Pt 8), 1839–1849. doi: 10.1093/brain/awf189
Chakraborty, P. K., Mangner, T. J., Chugani, D. C., Muzik, O., and Chugani, H. T. (1996). A high-yield and simplified procedure for the synthesis of alpha-[11C]methyl-L-tryptophan. Nucl. Med. Biol. 23, 1005–1008. doi: 10.1016/s0969-8051(96)00127-8
Chandana, S. R., Behen, M. E., Juhász, C., Muzik, O., Rothermel, R. D., Mangner, T. J., et al. (2005). Significance of abnormalities in developmental trajectory and asymmetry of cortical serotonin synthesis in autism. Int. J. Dev. Neurosci. 23, 171–182. doi: 10.1016/j.ijdevneu.2004.08.002
Chugani, D. C. (2012). Neuroimaging and neurochemistry of autism. Pediatr. Clin. North Am. 59, 63–73. doi: 10.1016/j.pcl.2011.10.002, x.,
Chugani, D. C., and Muzik, O. (2000). Alpha[C-11]methyl-L-tryptophan PET maps brain serotonin synthesis and kynurenine pathway metabolism. J. Cereb. Blood Flow Metab. 20, 2–9. doi: 10.1097/00004647-200001000-00002
Chugani, D. C., Muzik, O., Behen, M., Rothermel, R., Janisse, J. J., Lee, J., et al. (1999). Developmental changes in brain serotonin synthesis capacity in autistic and nonautistic children. Ann. Neurol. 45, 287–295.
Chugani, D. C., Muzik, O., Rothermel, R., Behen, M., Chakraborty, P., Mangner, T., et al. (1997). Altered serotonin synthesis in the dentatothalamocortical pathway in autistic boys. Ann. Neurol. 42, 666–669. doi: 10.1002/ana.410420420
Chugani, H. T., Da Silva, E., and Chugani, D. C. (1996). Infantile spasms: III. Prognostic implications of bitemporal hypometabolism on positron emission tomography. Ann. Neurol. 39, 643–649. doi: 10.1002/ana.410390514
Chugani, H. T., Juhász, C., Behen, M. E., Ondersma, R., and Muzik, O. (2007). Autism with facial port-wine stain: a new syndrome? Pediatr. Neurol. 37, 192–199. doi: 10.1016/j.pediatrneurol.2007.05.005
Coghlan, S., Horder, J., Inkster, B., Mendez, M. A., Murphy, D. G., and Nutt, D. J. (2012). GABA system dysfunction in autism and related disorders: from synapse to symptoms. Neurosci. Biobehav. Rev. 36, 2044–2055. doi: 10.1016/j.neubiorev.2012.07.005
Cook, E. H. Jr., Courchesne, R. Y., Cox, N. J., Lord, C., Gonen, D., Guter, S. J., et al. (1998). Linkage-disequilibrium mapping of autistic disorder, with 15q11-13 markers. Am. J. Hum. Genet. 62, 1077–1083. doi: 10.1086/301832
Courchesne-Loyer, A., Croteau, E., Castellano, C. A., St-Pierre, V., Hennebelle, M., and Cunnane, S. C. (2017). Inverse relationship between brain glucose and ketone metabolism in adults during short-term moderate dietary ketosis: a dual tracer quantitative positron emission tomography study. J. Cereb. Blood Flow Metab. 37, 2485–2493. doi: 10.1177/0271678x16669366
Croen, L. A., Grether, J. K., Yoshida, C. K., Odouli, R., and Hendrick, V. (2011). Antidepressant use during pregnancy and childhood autism spectrum disorders. Arch. Gen. Psychiatry 68, 1104–1112. doi: 10.1001/archgenpsychiatry.2011.73
De Volder, A., Bol, A., Michel, C., Congneau, M., and Goffinet, A. M. (1987). Brain glucose metabolism in children with the autistic syndrome: positron tomography analysis. Brain Dev. 9, 581–587. doi: 10.1016/s0387-7604(87)80089-x
Deriaz, N., Willi, J. P., Orihuela-Flores, M., Galli Carminati, G., and Ratib, O. (2012). Treatment with levetiracetam in a patient with pervasive developmental disorders, severe intellectual disability, self-injurious behavior, and seizures: a case report. Neurocase 18, 386–391. doi: 10.1080/13554794.2011.627336
Dilber, C., Calışkan, M., Sönmezoğlu, K., Nişli, S., Mukaddes, N. M., Tatlı, B., et al. (2013). Positron emission tomography findings in children with infantile spasms and autism. J. Clin. Neurosci. 20, 373–376. doi: 10.1016/j.jocn.2012.03.034
Dionne, O., Lortie, A., Gagnon, F., and Corbin, F. (2021). Rates of protein synthesis are reduced in peripheral blood mononuclear cells (PBMCs) from fragile X individuals. PLoS One 16:e0251367. doi: 10.1371/journal.pone.0251367
Domes, G., Heinrichs, M., Kumbier, E., Grossmann, A., Hauenstein, K., and Herpertz, S. C. (2013). Effects of intranasal oxytocin on the neural basis of face processing in autism spectrum disorder. Biol. Psychiatry 74, 164–171. doi: 10.1016/j.biopsych.2013.02.007
Duchesnay, E., Cachia, A., Boddaert, N., Chabane, N., Mangin, J. F., Martinot, J. L., et al. (2011). Feature selection and classification of imbalanced datasets: application to PET images of children with autistic spectrum disorders. Neuroimage 57, 1003–1014. doi: 10.1016/j.neuroimage.2011.05.011
Durieux, A. M., Fernandes, C., Murphy, D., Labouesse, M. A., Giovanoli, S., Meyer, U., et al. (2015). Targeting glia with N-acetylcysteine modulates brain glutamate and behaviors relevant to neurodevelopmental disorders in C57BL/6J mice. Front. Behav. Neurosci. 9:343. doi: 10.3389/fnbeh.2015.00343
Ernst, M., Zametkin, A. J., Matochik, J. A., Pascualvaca, D., and Cohen, R. M. (1997). Low medial prefrontal dopaminergic activity in autistic children. Lancet 350:638. doi: 10.1016/s0140-6736(05)63326-0
Fabio, K., Guillon, C., Lacey, C. J., Lu, S. F., Heindel, N. D., Ferris, C. F., et al. (2012). Synthesis and evaluation of potent and selective human V1a receptor antagonists as potential ligands for PET or SPECT imaging. Bioorg. Med. Chem. 20, 1337–1345. doi: 10.1016/j.bmc.2011.12.013
Fatemi, S. H., Wong, D. F., Brašić, J. R., Kuwabara, H., Mathur, A., Folsom, T. D., et al. (2018). Metabotropic glutamate receptor 5 tracer [(18)F]-FPEB displays increased binding potential in postcentral gyrus and cerebellum of male individuals with autism: a pilot PET study. Cerebellum Ataxias 5:3. doi: 10.1186/s40673-018-0082-1
Fernell, E., Watanabe, Y., Adolfsson, I., Tani, Y., Bergström, M., Hartvig, P., et al. (1997). Possible effects of tetrahydrobiopterin treatment in six children with autism–clinical and positron emission tomography data: a pilot study. Dev. Med. Child Neurol. 39, 313–318. doi: 10.1111/j.1469-8749.1997.tb07437.x
Fowler, J. S., and Ido, T. (2002). Initial and subsequent approach for the synthesis of 18FDG. Semin. Nucl. Med. 32, 6–12. doi: 10.1053/snuc.2002.29270
Frye, R. E., and Rossignol, D. A. (2014). Treatments for biomedical abnormalities associated with autism spectrum disorder. Front. Pediatr. 2:66. doi: 10.3389/fped.2014.00066
Fujino, J., Tei, S., Takahata, K., Matsuoka, K., Tagai, K., Sano, Y., et al. (2020). Binding of dopamine D1 receptor and noradrenaline transporter in individuals with autism spectrum disorder: a PET study. Cereb. Cortex 30, 6458–6468. doi: 10.1093/cercor/bhaa211
Fujita, M., Kobayashi, M., Ikawa, M., Gunn, R. N., Rabiner, E. A., Owen, D. R., et al. (2017). Comparison of four (11)C-labeled PET ligands to quantify translocator protein 18 kDa (TSPO) in human brain: (R)-PK11195, PBR28, DPA-713, and ER176-based on recent publications that measured specific-to-non-displaceable ratios. EJNMMI Res. 7:84. doi: 10.1186/s13550-017-0334-8
Fung, L. K., Flores, R. E., Gu, M., Sun, K. L., James, D., Schuck, R. K., et al. (2020). Thalamic and prefrontal GABA concentrations but not GABA(A) receptor densities are altered in high-functioning adults with autism spectrum disorder. Mol. Psychiatry 26, 1634–1646. doi: 10.1038/s41380-020-0756-y
Gadow, K. D., Roohi, J., DeVincent, C. J., and Hatchwell, E. (2008). Association of ADHD, tics, and anxiety with dopamine transporter (DAT1) genotype in autism spectrum disorder. J. Child Psychol. Psychiatry 49, 1331–1338. doi: 10.1111/j.1469-7610.2008.01952.x
Gendry Meresse, I., Zilbovicius, M., Boddaert, N., Robel, L., Philippe, A., Sfaello, I., et al. (2005). Autism severity and temporal lobe functional abnormalities. Ann. Neurol. 58, 466–469. doi: 10.1002/ana.20597
Girault, J. B., and Piven, J. (2020). The neurodevelopment of autism from infancy through toddlerhood. Neuroimaging Clin. N. Am. 30, 97–114. doi: 10.1016/j.nic.2019.09.009
Girgis, R. R., Slifstein, M., Xu, X., Frankle, W. G., Anagnostou, E., Wasserman, S., et al. (2011). The 5-HT(2A) receptor and serotonin transporter in Asperger’s disorder: a PET study with [11C]MDL 100907 and [11C]DASB. Psychiatry Res. 194, 230–234. doi: 10.1016/j.pscychresns.2011.04.007
Gjedde, A., Wong, D. F., Rosa-Neto, P., and Cumming, P. (2005). Mapping neuroreceptors at work: on the definition and interpretation of binding potentials after 20 years of progress. Int. Rev. Neurobiol. 63, 1–20. doi: 10.1016/s0074-7742(05)63001-2
Goji, A., Ito, H., Mori, K., Harada, M., Hisaoka, S., Toda, Y., et al. (2017). Assessment of anterior cingulate cortex (ACC) and left cerebellar metabolism in asperger’s syndrome with proton magnetic resonance spectroscopy (MRS). PLoS One 12:e0169288. doi: 10.1371/journal.pone.0169288
Goldberg, J., Anderson, G. M., Zwaigenbaum, L., Hall, G. B., Nahmias, C., Thompson, A., et al. (2009). Cortical serotonin type-2 receptor density in parents of children with autism spectrum disorders. J. Autism Dev. Disord. 39, 97–104. doi: 10.1007/s10803-008-0604-4
Goodson, J. L., and Thompson, R. R. (2010). Nonapeptide mechanisms of social cognition, behavior and species-specific social systems. Curr. Opin. Neurobiol. 20, 784–794. doi: 10.1016/j.conb.2010.08.020
Gordon, I., Vander Wyk, B. C., Bennett, R. H., Cordeaux, C., Lucas, M. V., Eilbott, J. A., et al. (2013). Oxytocin enhances brain function in children with autism. Proc. Natl. Acad. Sci. U.S.A. 110, 20953–20958. doi: 10.1073/pnas.1312857110
Guastella, A. J., Einfeld, S. L., Gray, K. M., Rinehart, N. J., Tonge, B. J., Lambert, T. J., et al. (2010). Intranasal oxytocin improves emotion recognition for youth with autism spectrum disorders. Biol. Psychiatry 67, 692–694. doi: 10.1016/j.biopsych.2009.09.020
Hafizi, S., Tseng, H. H., Rao, N., Selvanathan, T., Kenk, M., Bazinet, R. P., et al. (2017). Imaging microglial activation in untreated first-episode psychosis: a PET study with [(18)F]FEPPA. Am. J. Psychiatry 174, 118–124. doi: 10.1176/appi.ajp.2016.16020171
Haider, A., Xiao, Z., Xia, X., Chen, J., Van, R. S., Kuang, S., et al. (2021). Development of a triazolobenzodiazepine-based PET probe for subtype-selective vasopressin 1A receptor imaging. Pharmacol. Res. 173:105886. doi: 10.1016/j.phrs.2021.105886
Hall, G. B., Szechtman, H., and Nahmias, C. (2003). Enhanced salience and emotion recognition in Autism: a PET study. Am. J. Psychiatry 160, 1439–1441. doi: 10.1176/appi.ajp.160.8.1439
Hamilton, P. J., Campbell, N. G., Sharma, S., Erreger, K., Herborg Hansen, F., Saunders, C., et al. (2013). De novo mutation in the dopamine transporter gene associates dopamine dysfunction with autism spectrum disorder. Mol. Psychiatry 18, 1315–1323. doi: 10.1038/mp.2013.102
Happé, F., Ehlers, S., Fletcher, P., Frith, U., Johansson, M., Gillberg, C., et al. (1996). ’Theory of mind’ in the brain. Evidence from a PET scan study of Asperger syndrome. Neuroreport 8, 197–201. doi: 10.1097/00001756-199612200-00040
Hardan, A. Y., and Handen, B. L. (2002). A retrospective open trial of adjunctive donepezil in children and adolescents with autistic disorder. J. Child Adolesc. Psychopharmacol. 12, 237–241. doi: 10.1089/104454602760386923
Hazlett, E. A., Buchsbaum, M. S., Hsieh, P., Haznedar, M. M., Platholi, J., LiCalzi, E. M., et al. (2004). Regional glucose metabolism within cortical Brodmann areas in healthy individuals and autistic patients. Neuropsychobiology 49, 115–125. doi: 10.1159/000076719
Haznedar, M. M., Buchsbaum, M. S., Hazlett, E. A., LiCalzi, E. M., Cartwright, C., and Hollander, E. (2006). Volumetric analysis and three-dimensional glucose metabolic mapping of the striatum and thalamus in patients with autism spectrum disorders. Am. J. Psychiatry 163, 1252–1263. doi: 10.1176/appi.ajp.163.7.1252
Haznedar, M. M., Buchsbaum, M. S., Metzger, M., Solimando, A., Spiegel-Cohen, J., and Hollander, E. (1997). Anterior cingulate gyrus volume and glucose metabolism in autistic disorder. Am. J. Psychiatry 154, 1047–1050. doi: 10.1176/ajp.154.8.1047
Haznedar, M. M., Buchsbaum, M. S., Wei, T. C., Hof, P. R., Cartwright, C., Bienstock, C. A., et al. (2000). Limbic circuitry in patients with autism spectrum disorders studied with positron emission tomography and magnetic resonance imaging. Am. J. Psychiatry 157, 1994–2001. doi: 10.1176/appi.ajp.157.12.1994
Heh, C. W., Smith, R., Wu, J., Hazlett, E., Russell, A., Asarnow, R., et al. (1989). Positron emission tomography of the cerebellum in autism. Am. J. Psychiatry 146, 242–245. doi: 10.1176/ajp.146.2.242
Herold, S., Frackowiak, R. S., Le Couteur, A., Rutter, M., and Howlin, P. (1988). Cerebral blood flow and metabolism of oxygen and glucose in young autistic adults. Psychol. Med. 18, 823–831. doi: 10.1017/s0033291700009752
Hirosawa, T., Kikuchi, M., Ouchi, Y., Takahashi, T., Yoshimura, Y., Kosaka, H., et al. (2017). A pilot study of serotonergic modulation after long-term administration of oxytocin in autism spectrum disorder. Autism Res. 10, 821–828. doi: 10.1002/aur.1761
Hollander, E., Soorya, L., Chaplin, W., Anagnostou, E., Taylor, B. P., Ferretti, C. J., et al. (2012). A double-blind placebo-controlled trial of fluoxetine for repetitive behaviors and global severity in adult autism spectrum disorders. Am. J. Psychiatry 169, 292–299. doi: 10.1176/appi.ajp.2011.10050764
Hong, S. J., Vogelstein, J. T., Gozzi, A., Bernhardt, B. C., Yeo, B. T. T., Milham, M. P., et al. (2020). Toward neurosubtypes in autism. Biol. Psychiatry 88, 111–128. doi: 10.1016/j.biopsych.2020.03.022
Horder, J., Andersson, M., Mendez, M. A., Singh, N., Tangen, Ä, Lundberg, J., et al. (2018). GABA(A) receptor availability is not altered in adults with autism spectrum disorder or in mouse models. Sci. Transl. Med. 10:eaam8434. doi: 10.1126/scitranslmed.aam8434
Horwitz, B., Rumsey, J. M., Grady, C. L., and Rapoport, S. I. (1988). The cerebral metabolic landscape in autism. Intercorrelations of regional glucose utilization. Arch. Neurol. 45, 749–755. doi: 10.1001/archneur.1988.00520310055018
Huang, Y., Bae, S.-A., Zhu, Z., Guo, N., Hwang, D.-R., and Laruelle, M. (2001). Fluorinated analogues of ADAM as new PET radioligands for the serotonin transporter: synthesis and pharmacological evaluation. J. Labelled Compd. Radiopharm. 44, S18–S20. doi: 10.1002/jlcr.2580440107
Huang, Y., Hwang, D. R., Narendran, R., Sudo, Y., Chatterjee, R., Bae, S. A., et al. (2002). Comparative evaluation in nonhuman primates of five PET radiotracers for imaging the serotonin transporters: [11C]McN 5652, [11C]ADAM, [11C]DASB, [11C]DAPA, and [11C]AFM. J. Cereb. Blood Flow Metab. 22, 1377–1398. doi: 10.1097/01.Wcb.0000040948.67415.05
Hwang, B. J., Mohamed, M. A., and Brašić, J. R. (2017). Molecular imaging of autism spectrum disorder. Int. Rev. Psychiatry 29, 530–554. doi: 10.1080/09540261.2017.1397606
Jain, M., and Batra, S. K. (2003). Genetically engineered antibody fragments and PET imaging: a new era of radioimmunodiagnosis. J. Nucl. Med. 44, 1970–1972.
Janik, R., Thomason, L. A. M., Stanisz, A. M., Forsythe, P., Bienenstock, J., and Stanisz, G. J. (2016). Magnetic resonance spectroscopy reveals oral Lactobacillus promotion of increases in brain GABA, N-acetyl aspartate and glutamate. Neuroimage 125, 988–995. doi: 10.1016/j.neuroimage.2015.11.018
Joseph-Mathurin, N., Su, Y., Blazey, T. M., Jasielec, M., Vlassenko, A., Friedrichsen, K., et al. (2018). Utility of perfusion PET measures to assess neuronal injury in Alzheimer’s disease. Alzheimers Dement. (Amst.) 10, 669–677. doi: 10.1016/j.dadm.2018.08.012
Kadwa, R. A., Sahu, J. K., Singhi, P., Malhi, P., and Mittal, B. R. (2019). Prevalence and characteristics of sensory processing abnormalities and its correlation with FDG-PET findings in children with autism. Indian J. Pediatr. 86, 1036–1042. doi: 10.1007/s12098-019-03061-9
Karvat, G., and Kimchi, T. (2014). Acetylcholine elevation relieves cognitive rigidity and social deficiency in a mouse model of autism. Neuropsychopharmacology 39, 831–840. doi: 10.1038/npp.2013.274
Kato, T. A., Yamauchi, Y., Horikawa, H., Monji, A., Mizoguchi, Y., Seki, Y., et al. (2013). Neurotransmitters, psychotropic drugs and microglia: clinical implications for psychiatry. Curr. Med. Chem. 20, 331–344. doi: 10.2174/0929867311320030003
Katsanis, N. (2016). The continuum of causality in human genetic disorders. Genome Biol. 17:233. doi: 10.1186/s13059-016-1107-9
Kelleher, R. J. III, and Bear, M. F. (2008). The autistic neuron: troubled translation? Cell 135, 401–406. doi: 10.1016/j.cell.2008.10.017
Kelly, A. M., and Goodson, J. L. (2014). Hypothalamic oxytocin and vasopressin neurons exert sex-specific effects on pair bonding, gregariousness, and aggression in finches. Proc. Natl. Acad. Sci. U.S.A. 111, 6069–6074. doi: 10.1073/pnas.1322554111
Kobayashi, M., Jiang, T., Telu, S., Zoghbi, S. S., Gunn, R. N., Rabiner, E. A., et al. (2018). (11)C-DPA-713 has much greater specific binding to translocator protein 18 kDa (TSPO) in human brain than (11)C-(R)-PK11195. J. Cereb. Blood Flow Metab. 38, 393–403. doi: 10.1177/0271678x17699223
Koob, G. F., and Bloom, F. E. (1982). Behavioral effects of neuropeptides: endorphins and vasopressin. Annu. Rev. Physiol. 44, 571–582. doi: 10.1146/annurev.ph.44.030182.003035
Kowalewska, B., Drozdz, W., and Kowalewski, L. (2021). Positron emission tomography (PET) and single-photon emission computed tomography (SPECT) in autism research: literature review. Ir. J. Psychol. Med. 1–15. doi: 10.1017/ipm.2021.15
Le Couteur, A., Lord, C., and Rutter, M. (2003). The Autism Diagnostic Interview-Revised (ADI-R). Los Angeles, CA: Western Psychological Services.
Lee, H., Chung, M. K., Kang, H., Kim, B. N., and Lee, D. S. (2011a). Computing the shape of brain networks using graph filtration and Gromov-Hausdorff metric. Med. Image Comput. Comput. Assist. Interv. 14(Pt. 2), 302–309. doi: 10.1007/978-3-642-23629-7_37
Lee, H., Kang, H., Chung, M. K., Kim, B. N., and Lee, D. S. (2012). Persistent brain network homology from the perspective of dendrogram. IEEE Trans. Med. Imaging 31, 2267–2277. doi: 10.1109/tmi.2012.2219590
Lee, H., Lee, D. S., Kang, H., Kim, B. N., and Chung, M. K. (2011b). Sparse brain network recovery under compressed sensing. IEEE Trans. Med. Imaging 30, 1154–1165. doi: 10.1109/tmi.2011.2140380
Lefevre, A., Mottolese, R., Redouté, J., Costes, N., Le Bars, D., Geoffray, M. M., et al. (2018). Oxytocin fails to recruit serotonergic neurotransmission in the autistic brain. Cereb. Cortex 28, 4169–4178. doi: 10.1093/cercor/bhx272
Lefevre, A., Richard, N., Mottolese, R., Leboyer, M., and Sirigu, A. (2020). An association between serotonin 1A receptor, gray matter volume, and sociability in healthy subjects and in autism spectrum disorder. Autism Res. 13, 1843–1855. doi: 10.1002/aur.2360
Li, X., Zhang, K., He, X., Zhou, J., Jin, C., Shen, L., et al. (2021). Structural, functional, and molecular imaging of autism spectrum disorder. Neurosci. Bull. 37, 1051–1071. doi: 10.1007/s12264-021-00673-0
Lombardo, M. V., Lai, M. C., and Baron-Cohen, S. (2019). Big data approaches to decomposing heterogeneity across the autism spectrum. Mol. Psychiatry 24, 1435–1450. doi: 10.1038/s41380-018-0321-0
Lord, C., Rutter, M., DiLavore, P. C., Risi, S., Gotham, K., and Bishop, S. (2012). Autism Diagnostic Observation Schedule: ADOS-2. Los Angeles, CA: Western Psychological Services.
Lundberg, J., Halldin, C., and Farde, L. (2006). Measurement of serotonin transporter binding with PET and [11C]MADAM: a test-retest reproducibility study. Synapse 60, 256–263. doi: 10.1002/syn.20297
MacLaren, D. C., Toyokuni, T., Cherry, S. R., Barrio, J. R., Phelps, M. E., Herschman, H. R., et al. (2000). PET imaging of transgene expression. Biol. Psychiatry 48, 337–348. doi: 10.1016/s0006-3223(00)00970-7
Makkonen, I., Riikonen, R., Kokki, H., Airaksinen, M. M., and Kuikka, J. T. (2008). Serotonin and dopamine transporter binding in children with autism determined by SPECT. Dev. Med. Child Neurol. 50, 593–597. doi: 10.1111/j.1469-8749.2008.03027.x
Manglunia, A. S., and Puranik, A. D. (2016). FDG PET/CT findings in a clinically diagnosed case of childhood autism. Indian J. Nucl. Med. 31, 138–140. doi: 10.4103/0972-3919.178302
Mash, L. E., Keehn, B., Linke, A. C., Liu, T. T., and Connectivity, R. M. J. B. (2019). Atypical relationships between spontaneous EEG and fMRI activity in autism. Brain Connect. 10, 18–28.
McCluskey, S. P., Plisson, C., Rabiner, E. A., and Howes, O. (2020). Advances in CNS PET: the state-of-the-art for new imaging targets for pathophysiology and drug development. Eur. J. Nucl. Med. Mol. Imaging 47, 451–489. doi: 10.1007/s00259-019-04488-0
McDougle, C. J., Naylor, S. T., Cohen, D. J., Volkmar, F. R., Heninger, G. R., and Price, L. H. (1996). A double-blind, placebo-controlled study of fluvoxamine in adults with autistic disorder. Arch. Gen. Psychiatry 53, 1001–1008. doi: 10.1001/archpsyc.1996.01830110037005
McPartland, J. C., Bernier, R. A., Jeste, S. S., Dawson, G., Nelson, C. A., Chawarska, K., et al. (2020). The autism biomarkers consortium for clinical trials (ABC-CT): scientific context, study design, and progress toward biomarker qualification. Front. Integr. Neurosci. 14:16. doi: 10.3389/fnint.2020.00016
McPartland, J. C., Lerner, M. D., Bhat, A., Clarkson, T., Jack, A., Koohsari, S., et al. (2021). Looking back at the next 40 years of ASD neuroscience research. J. Autism Dev. Disord. 51, 4333–4353. doi: 10.1007/s10803-021-05095-5
Mendez, M. A., Horder, J., Myers, J., Coghlan, S., Stokes, P., Erritzoe, D., et al. (2013). The brain GABA-benzodiazepine receptor alpha-5 subtype in autism spectrum disorder: a pilot [(11)C]Ro15-4513 positron emission tomography study. Neuropharmacology 68, 195–201. doi: 10.1016/j.neuropharm.2012.04.008
Meyer, J. H., Cervenka, S., Kim, M. J., Kreisl, W. C., Henter, I. D., and Innis, R. B. (2020). Neuroinflammation in psychiatric disorders: PET imaging and promising new targets. Lancet Psychiatry 7, 1064–1074. doi: 10.1016/s2215-0366(20)30255-8
Miller, M., Bales, K. L., Taylor, S. L., Yoon, J., Hostetler, C. M., Carter, C. S., et al. (2013). Oxytocin and vasopressin in children and adolescents with autism spectrum disorders: sex differences and associations with symptoms. Autism Res. 6, 91–102. doi: 10.1002/aur.1270
Mitelman, S. A., Bralet, M. C., Mehmet Haznedar, M., Hollander, E., Shihabuddin, L., Hazlett, E. A., et al. (2018). Positron emission tomography assessment of cerebral glucose metabolic rates in autism spectrum disorder and schizophrenia. Brain Imaging Behav. 12, 532–546. doi: 10.1007/s11682-017-9721-z
Modahl, C., Green, L., Fein, D., Morris, M., Waterhouse, L., Feinstein, C., et al. (1998). Plasma oxytocin levels in autistic children. Biol. Psychiatry 43, 270–277. doi: 10.1016/s0006-3223(97)00439-3
Mottolese, R., Redouté, J., Costes, N., Le Bars, D., and Sirigu, A. (2014). Switching brain serotonin with oxytocin. Proc. Natl. Acad. Sci. U.S.A. 111, 8637–8642. doi: 10.1073/pnas.1319810111
Mudd, A. T., Berding, K., Wang, M., Donovan, S. M., and Dilger, R. N. (2017). Serum cortisol mediates the relationship between fecal Ruminococcus and brain N-acetylaspartate in the young pig. Gut Microbes 8, 589–600. doi: 10.1080/19490976.2017.1353849
Müller, R. A., Behen, M. E., Rothermel, R. D., Chugani, D. C., Muzik, O., Mangner, T. J., et al. (1999). Brain mapping of language and auditory perception in high-functioning autistic adults: a PET study. J. Autism Dev. Disord. 29, 19–31. doi: 10.1023/a:1025914515203
Müller, R. A., Chugani, D. C., Behen, M. E., Rothermel, R. D., Muzik, O., Chakraborty, P. K., et al. (1998). Impairment of dentato-thalamo-cortical pathway in autistic men: language activation data from positron emission tomography. Neurosci. Lett. 245, 1–4. doi: 10.1016/s0304-3940(98)00151-7
Naik, R., Valentine, H., Hall, A., Mathews, W. B., Harris, J. C., Carter, C. S., et al. (2017). Development of a radioligand for imaging V1a vasopressin receptors with PET. Eur. J. Med. Chem. 139, 644–656. doi: 10.1016/j.ejmech.2017.08.037
Nakamura, K., Sekine, Y., Ouchi, Y., Tsujii, M., Yoshikawa, E., Futatsubashi, M., et al. (2010). Brain serotonin and dopamine transporter bindings in adults with high-functioning autism. Arch. Gen. Psychiatry 67, 59–68. doi: 10.1001/archgenpsychiatry.2009.137
Nicolson, R., Craven-Thuss, B., and Smith, J. (2006). A prospective, open-label trial of galantamine in autistic disorder. J. Child Adolesc. Psychopharmacol. 16, 621–629. doi: 10.1089/cap.2006.16.621
Nieminen-von Wendt, T., Metsähonkala, L., Kulomäki, T., Aalto, S., Autti, T., Vanhala, R., et al. (2003). Changes in cerebral blood flow in Asperger syndrome during theory of mind tasks presented by the auditory route. Eur. Child Adolesc. Psychiatry 12, 178–189. doi: 10.1007/s00787-003-0337-z
Nieminen-von Wendt, T. S., Metsähonkala, L., Kulomäki, T. A., Aalto, S., Autti, T. H., Vanhala, R., et al. (2004). Increased presynaptic dopamine function in Asperger syndrome. Neuroreport 15, 757–760. doi: 10.1097/00001756-200404090-00003
Oblak, A., Gibbs, T. T., and Blatt, G. J. (2009). Decreased GABAA receptors and benzodiazepine binding sites in the anterior cingulate cortex in autism. Autism Res. 2, 205–219. doi: 10.1002/aur.88
Owen, D. R., Howell, O. W., Tang, S. P., Wells, L. A., Bennacef, I., Bergstrom, M., et al. (2010). Two binding sites for [3H]PBR28 in human brain: implications for TSPO PET imaging of neuroinflammation. J. Cereb. Blood Flow Metab. 30, 1608–1618. doi: 10.1038/jcbfm.2010.63
Owen, D. R., Yeo, A. J., Gunn, R. N., Song, K., Wadsworth, G., Lewis, A., et al. (2012). An 18-kDa translocator protein (TSPO) polymorphism explains differences in binding affinity of the PET radioligand PBR28. J. Cereb. Blood Flow Metab. 32, 1–5. doi: 10.1038/jcbfm.2011.147
Pagani, M., Manouilenko, I., Stone-Elander, S., Odh, R., Salmaso, D., Hatherly, R., et al. (2012). Brief report: alterations in cerebral blood flow as assessed by PET/CT in adults with autism spectrum disorder with normal IQ. J. Autism Dev. Disord. 42, 313–318. doi: 10.1007/s10803-011-1240-y
Page, L. A., Daly, E., Schmitz, N., Simmons, A., Toal, F., Deeley, Q., et al. (2006). In vivo 1H-magnetic resonance spectroscopy study of amygdala-hippocampal and parietal regions in autism. Am. J. Psychiatry 163, 2189–2192. doi: 10.1176/appi.ajp.163.12.2189
Pandya, D. N., and Yeterian, E. H. (1985). “Architecture and connections of cortical association areas,” in Association and Auditory Cortices. Cerebral Cortex, Vol. 4, eds A. Peters and E. G. Jones (Boston, MA: Springer).
Park, H. R., Kim, I. H., Kang, H., Lee, D. S., Kim, B. N., Kim, D. G., et al. (2017). Nucleus accumbens deep brain stimulation for a patient with self-injurious behavior and autism spectrum disorder: functional and structural changes of the brain: report of a case and review of literature. Acta Neurochir. (Wien) 159, 137–143. doi: 10.1007/s00701-016-3002-2
Parker, K. J., Garner, J. P., Oztan, O., Tarara, E. R., Li, J., Sclafani, V., et al. (2018). Arginine vasopressin in cerebrospinal fluid is a marker of sociality in nonhuman primates. Sci. Transl. Med. 10:eaam9100. doi: 10.1126/scitranslmed.aam9100
Pizzarelli, R., and Cherubini, E. (2011). Alterations of GABAergic signaling in autism spectrum disorders. Neural Plast. 2011:297153. doi: 10.1155/2011/297153
Rubenstein, J. L., and Merzenich, M. M. (2003). Model of autism: increased ratio of excitation/inhibition in key neural systems. Genes Brain Behav. 2, 255–267. doi: 10.1034/j.1601-183x.2003.00037.x
Rumsey, J. M., and Ernst, M. (2000). Functional neuroimaging of autistic disorders. Ment. Retard. Dev. Disabil. Res. Rev. 6, 171–179.
Rumsey, J. M., Duara, R., Grady, C., Rapoport, J. L., Margolin, R. A., Rapoport, S. I., et al. (1985). Brain metabolism in autism. Resting cerebral glucose utilization rates as measured with positron emission tomography. Arch. Gen. Psychiatry 42, 448–455. doi: 10.1001/archpsyc.1985.01790280026003
Saitovitch, A., Rechtman, E., Lemaitre, H., Tacchella, J.-M., Vinçon-Leite, A., Douard, E., et al. (2019). Superior temporal sulcus hypoperfusion in children with autism spectrum disorder: an arterial spin-labeling magnetic resonance study. bioRxiv [preprint]. doi: 10.1101/771584
Satterstrom, F. K., Kosmicki, J. A., Wang, J., Breen, M. S., De Rubeis, S., An, J. Y., et al. (2020). Large-scale exome sequencing study implicates both developmental and functional changes in the neurobiology of autism. Cell 180, 568–584.e523. doi: 10.1016/j.cell.2019.12.036
Schain, R. J., and Freedman, D. X. (1961). Studies on 5-hydroxyindole metabolism in autistic and other mentally retarded children. J. Pediatr. 58, 315–320. doi: 10.1016/s0022-3476(61)80261-8
Schalbroeck, R., de Geus-Oei, L. F., Selten, J. P., Yaqub, M., Schrantee, A., van Amelsvoort, T., et al. (2021a). Cerebral [(18)F]-FDOPA uptake in autism spectrum disorder and its association with autistic traits. Diagnostics 11:2404. doi: 10.3390/diagnostics11122404
Schalbroeck, R., van Velden, F. H. P., de Geus-Oei, L. F., Yaqub, M., van Amelsvoort, T., Booij, J., et al. (2021b). Striatal dopamine synthesis capacity in autism spectrum disorder and its relation with social defeat: an [(18)F]-FDOPA PET/CT study. Transl. Psychiatry 11:47. doi: 10.1038/s41398-020-01174-w
Schifter, T., Hoffman, J. M., Hatten, H. P. Jr., Hanson, M. W., Coleman, R. E., and DeLong, G. R. (1994). Neuroimaging in infantile autism. J. Child Neurol. 9, 155–161. doi: 10.1177/088307389400900210
Schmidt, K. C., Loutaev, I., Quezado, Z., Sheeler, C., and Smith, C. B. (2020). Regional rates of brain protein synthesis are unaltered in dexmedetomidine sedated young men with fragile X syndrome: a L-[1-(11)C]leucine PET study. Neurobiol. Dis. 143:104978. doi: 10.1016/j.nbd.2020.104978
Schnider, P., Bissantz, C., Bruns, A., Dolente, C., Goetschi, E., Jakob-Roetne, R., et al. (2020). Discovery of balovaptan, a vasopressin 1a receptor antagonist for the treatment of autism spectrum disorder. J. Med. Chem. 63, 1511–1525. doi: 10.1021/acs.jmedchem.9b01478
Seltzer, B., and Pandya, D. N. (1978). Afferent cortical connections and architectonics of the superior temporal sulcus and surrounding cortex in the rhesus monkey. Brain Res. 149, 1–24. doi: 10.1016/0006-8993(78)90584-x
Shandal, V., Sundaram, S. K., Chugani, D. C., Kumar, A., Behen, M. E., and Chugani, H. T. (2011). Abnormal brain protein synthesis in language areas of children with pervasive developmental disorder: a L-[1-11C]-leucine PET study. J. Child Neurol. 26, 1347–1354. doi: 10.1177/0883073811405200
Sharma, A., Gokulchandran, N., Sane, H., Nagrajan, A., Paranjape, A., Kulkarni, P., et al. (2013). Autologous bone marrow mononuclear cell therapy for autism: an open label proof of concept study. Stem Cells Int. 2013:623875. doi: 10.1155/2013/623875
Shih, Y. T., and Hsueh, Y. P. (2018). The involvement of endoplasmic reticulum formation and protein synthesis efficiency in VCP- and ATL1-related neurological disorders. J. Biomed. Sci 25:2. doi: 10.1186/s12929-017-0403-3
Shiue, C. Y., and Welch, M. J. (2004). Update on PET radiopharmaceuticals: life beyond fluorodeoxyglucose. Radiol. Clin. North Am. 42, 1033–1053, viii. doi: 10.1016/j.rcl.2004.08.009
Shou, X. J., Xu, X. J., Zeng, X. Z., Liu, Y., Yuan, H. S., Xing, Y., et al. (2017). A volumetric and functional connectivity MRI study of brain arginine-vasopressin pathways in autistic children. Neurosci. Bull. 33, 130–142. doi: 10.1007/s12264-017-0109-2
Siegel, B. V. Jr., Asarnow, R., Tanguay, P., Call, J. D., Abel, L., Ho, A., et al. (1992). Regional cerebral glucose metabolism and attention in adults with a history of childhood autism. J. Neuropsychiatry Clin. Neurosci. 4, 406–414. doi: 10.1176/jnp.4.4.406
Siegel, B. V. Jr., Nuechterlein, K. H., Abel, L., Wu, J. C., and Buchsbaum, M. S. (1995). Glucose metabolic correlates of continuous performance test performance in adults with a history of infantile autism, schizophrenics, and controls. Schizophr. Res. 17, 85–94. doi: 10.1016/0920-9964(95)00033-i
Sitoh, Y. Y., and Tien, R. D. (1997). The limbic system. An overview of the anatomy and its development. Neuroimaging Clin. N. Am. 7, 1–10.
Smith, G. S., Koppel, J., and Goldberg, S. (2003). Applications of neuroreceptor imaging to psychiatry research. Psychopharmacol. Bull. 37, 26–65.
Staal, W. G., de Krom, M., and de Jonge, M. V. (2012). Brief report: the dopamine-3-receptor gene (DRD3) is associated with specific repetitive behavior in autism spectrum disorder (ASD). J. Autism Dev. Disord. 42, 885–888. doi: 10.1007/s10803-011-1312-z
Stanley, J. A. (2002). In vivo magnetic resonance spectroscopy and its application to neuropsychiatric disorders. Can. J. Psychiatry 47, 315–326. doi: 10.1177/070674370204700402
Stigler, K. A., Sweeten, T. L., Posey, D. J., and McDougle, C. J. (2009). Autism and immune factors: a comprehensive review. Res. Autism Spectr. Disord. 3, 840–860. doi: 10.1016/j.rasd.2009.01.007
Suehiro, M., Scheffel, U., Ravert, H. T., Dannals, R. F., and Wagner, H. N. Jr. (1993). [11C](+)McN5652 as a radiotracer for imaging serotonin uptake sites with PET. Life Sci. 53, 883–892. doi: 10.1016/0024-3205(93)90440-e
Sullivan, J. M., Lim, K., Labaree, D., Lin, S. F., McCarthy, T. J., Seibyl, J. P., et al. (2013). Kinetic analysis of the metabotropic glutamate subtype 5 tracer [(18)F]FPEB in bolus and bolus-plus-constant-infusion studies in humans. J. Cereb. Blood Flow Metab. 33, 532–541. doi: 10.1038/jcbfm.2012.195
Suzuki, K., Sugihara, G., Ouchi, Y., Nakamura, K., Futatsubashi, M., Takebayashi, K., et al. (2013). Microglial activation in young adults with autism spectrum disorder. JAMA Psychiatry 70, 49–58. doi: 10.1001/jamapsychiatry.2013.272
Suzuki, K., Sugihara, G., Ouchi, Y., Nakamura, K., Tsujii, M., Futatsubashi, M., et al. (2011). Reduced acetylcholinesterase activity in the fusiform gyrus in adults with autism spectrum disorders. Arch. Gen. Psychiatry 68, 306–313. doi: 10.1001/archgenpsychiatry.2011.4
Syed, A. B., and Brasic, J. R. (2019). Nuclear neurotransmitter molecular imaging of autism spectrum disorder. AIMS Mol. Sci. 6, 87–106. doi: 10.3934/molsci.2019.4.87
Tebartz van Elst, L., Maier, S., Fangmeier, T., Endres, D., Mueller, G. T., Nickel, K., et al. (2014). Disturbed cingulate glutamate metabolism in adults with high-functioning autism spectrum disorder: evidence in support of the excitatory/inhibitory imbalance hypothesis. Mol. Psychiatry 19, 1314–1325. doi: 10.1038/mp.2014.62
Toyohara, J., Sakata, M., Oda, K., Ishii, K., Ito, K., Hiura, M., et al. (2013). Initial human PET studies of metabotropic glutamate receptor type 1 ligand 11C-ITMM. J. Nucl. Med. 54, 1302–1307. doi: 10.2967/jnumed.113.119891
Tyzio, R., Nardou, R., Ferrari, D. C., Tsintsadze, T., Shahrokhi, A., Eftekhari, S., et al. (2014). Oxytocin-mediated GABA inhibition during delivery attenuates autism pathogenesis in rodent offspring. Science 343, 675–679. doi: 10.1126/science.1247190
Van Audenhaege, K., Van Holen, R., Vandenberghe, S., Vanhove, C., Metzler, S. D., and Moore, S. C. (2015). Review of SPECT collimator selection, optimization, and fabrication for clinical and preclinical imaging. Med. Phys. 42, 4796–4813. doi: 10.1118/1.4927061
Vidal, B., Karpenko, I. A., Liger, F., Fieux, S., Bouillot, C., Billard, T., et al. (2017). [(11)C]PF-3274167 as a PET radiotracer of oxytocin receptors: radiosynthesis and evaluation in rat brain. Nucl. Med. Biol. 55, 1–6. doi: 10.1016/j.nucmedbio.2017.07.008
Volkow, N. D., Mullani, N. A., and Bendriem, B. (1988). Positron emission tomography instrumentation: an overview. Am. J. Physiol. Imaging 3, 142–153.
Wagner, H. N. Jr., Dannals, R. F., Frost, J. J., Wong, D. F., Ravert, H. T., Wilson, A. A., et al. (1985). Imaging neuroreceptors in the human brain in health and disease. Radioisotopes 34, 103–107. doi: 10.3769/radioisotopes.34.2_103
Walton, E., Turner, J. A., and Ehrlich, S. (2013). Neuroimaging as a potential biomarker to optimize psychiatric research and treatment. Int. Rev. Psychiatry 25, 619–631. doi: 10.3109/09540261.2013.816659
Wang, C., Moseley, C. K., Carlin, S. M., Wilson, C. M., Neelamegam, R., and Hooker, J. M. (2013). Radiosynthesis and evaluation of [11C]EMPA as a potential PET tracer for orexin 2 receptors. Bioorg. Med. Chem. Lett. 23, 3389–3392. doi: 10.1016/j.bmcl.2013.03.079
Werry, E. L., Bright, F. M., Piguet, O., Ittner, L. M., Halliday, G. M., Hodges, J. R., et al. (2019). Recent developments in TSPO PET imaging as a biomarker of neuroinflammation in neurodegenerative disorders. Int. J. Mol. Sci. 20:3161. doi: 10.3390/ijms20133161
Wilczyński, K. M., Zasada, I., Siwiec, A., and Janas-Kozik, M. (2019). Differences in oxytocin and vasopressin levels in individuals suffering from the autism spectrum disorders vs general population - a systematic review. Neuropsychiatr. Dis. Treat. 15, 2613–2620. doi: 10.2147/ndt.S207580
Wilson, A. A., Ginovart, N., Hussey, D., Meyer, J., and Houle, S. (2002). In vitro and in vivo characterisation of [11C]-DASB: a probe for in vivo measurements of the serotonin transporter by positron emission tomography. Nucl. Med. Biol. 29, 509–515. doi: 10.1016/s0969-8051(02)00316-5
Wolff, J. J., Jacob, S., and Elison, J. T. (2018). The journey to autism: insights from neuroimaging studies of infants and toddlers. Dev. Psychopathol. 30, 479–495. doi: 10.1017/s0954579417000980
Wong, D. F., Gründer, G., and Brasic, J. R. (2007). Brain imaging research: does the science serve clinical practice? Int. Rev. Psychiatry 19, 541–558. doi: 10.1080/09540260701564849
Wong, D. F., Waterhouse, R., Kuwabara, H., Kim, J., Brašić, J. R., Chamroonrat, W., et al. (2013). 18F-FPEB, a PET radiopharmaceutical for quantifying metabotropic glutamate 5 receptors: a first-in-human study of radiochemical safety, biokinetics, and radiation dosimetry. J. Nucl. Med. 54, 388–396. doi: 10.2967/jnumed.112.107995
Yang, C. J., Tan, H. P., and Du, Y. J. (2014). The developmental disruptions of serotonin signaling may involved in autism during early brain development. Neuroscience 267, 1–10. doi: 10.1016/j.neuroscience.2014.02.021
Żarnowska, I., Chrapko, B., Gwizda, G., Nocuń, A., Mitosek-Szewczyk, K., and Gasior, M. (2018). Therapeutic use of carbohydrate-restricted diets in an autistic child; a case report of clinical and 18FDG PET findings. Metab. Brain Dis. 33, 1187–1192. doi: 10.1007/s11011-018-0219-1
Zilbovicius, M., Boddaert, N., Belin, P., Poline, J. B., Remy, P., Mangin, J. F., et al. (2000). Temporal lobe dysfunction in childhood autism: a PET study. Positron emission tomography. Am. J. Psychiatry 157, 1988–1993. doi: 10.1176/appi.ajp.157.12.1988
Zürcher, N. R., Bhanot, A., McDougle, C. J., and Hooker, J. M. (2015). A systematic review of molecular imaging (PET and SPECT) in autism spectrum disorder: current state and future research opportunities. Neurosci. Biobehav. Rev. 52, 56–73. doi: 10.1016/j.neubiorev.2015.02.002
Zürcher, N. R., Loggia, M. L., Mullett, J. E., Tseng, C., Bhanot, A., Richey, L., et al. (2020). [(11)C]PBR28 MR-PET imaging reveals lower regional brain expression of translocator protein (TSPO) in young adult males with autism spectrum disorder. Mol. Psychiatry 26, 1659–1669. doi: 10.1038/s41380-020-0682-z
Zürcher, N. R., Walsh, E. C., Phillips, R. D., Cernasov, P. M., Tseng, C. J., Dharanikota, A., et al. (2021). A simultaneous [(11)C]raclopride positron emission tomography and functional magnetic resonance imaging investigation of striatal dopamine binding in autism. Transl. Psychiatry 11:33. doi: 10.1038/s41398-020-01170-0
Keywords: autism spectrum disorder, ASD, neuroimaging, positron emission tomography, PET, radioligands
Citation: Tan Z, Wei H, Song X, Mai W, Yan J, Ye W, Ling X, Hou L, Zhang S, Yan S, Xu H and Wang L (2022) Positron Emission Tomography in the Neuroimaging of Autism Spectrum Disorder: A Review. Front. Neurosci. 16:806876. doi: 10.3389/fnins.2022.806876
Received: 02 November 2021; Accepted: 14 March 2022;
Published: 13 April 2022.
Edited by:
Adriaan Anthonius Lammertsma, University Medical Center Groningen, NetherlandsReviewed by:
Stephanie Ameis, University of Toronto, CanadaJames Brasic, Johns Hopkins University, United States
Christin Schifani, Campbell Family Mental Health Research Institute, Canada, contributed to the review of SA
Copyright © 2022 Tan, Wei, Song, Mai, Yan, Ye, Ling, Hou, Zhang, Yan, Xu and Wang. This is an open-access article distributed under the terms of the Creative Commons Attribution License (CC BY). The use, distribution or reproduction in other forums is permitted, provided the original author(s) and the copyright owner(s) are credited and that the original publication in this journal is cited, in accordance with accepted academic practice. No use, distribution or reproduction is permitted which does not comply with these terms.
*Correspondence: Hao Xu, dHhoQGpudS5lZHUuY24=; Lu Wang, bF93YW5nMTAwOUBqbnUuZWR1LmNu
†These authors have contributed equally to this work