- Center for Translational NeuroImaging, Northeastern University, Boston, MA, United States
There are numerous publications on methods and applications for awake functional MRI across different species, e.g., voles, rabbits, cats, dogs, and rhesus macaques. Each of these species, most obviously rhesus monkey, have general or unique attributes that provide a better understanding of the human condition. However, much of the work today is done on rodents. The growing number of small bore (≤30 cm) high field systems 7T- 11.7T favor the use of small animals. To that point, this review is primarily focused on rodents and their many applications in awake function MRI. Applications include, pharmacological MRI, drugs of abuse, sensory evoked stimuli, brain disorders, pain, social behavior, and fear.
Introduction
Between 1998 and 2000 there were three studies on awake imaging in rhesus monkeys (Dubowitz et al., 1998; Stefanacci et al., 1998; Zhang et al., 2000). These were all done in large bore human 1.5T scanners with surgical implants in the skull used to immobilize the head. My lab published the first paper on awake rat imaging in 1999 (Lahti et al., 1999). The system was a General Electric CSI-II 2.0T small bore scanner for animal research. In the early 1990s GE was essentially giving these small scanners away with any purchase of their large bore, clinical 1.5T Signa scanner to encourage researchers to develop new pulse sequences and protocols that could eventually migrate to the console of the human scanner. Coming from a Psychiatry Department, I reasoned this new field of non-invasive MRI could help understand the etiology and pathophysiology of mental illness. A normal brain with some genetic risk could be subjected to an environmental insult early in life and followed into adulthood and the onset of mental illness. Mental health professionals use the phrase “diathesis and stress” to describe this cause and effect. This type of prospective, longitudinal study inflicting early insult and following brain function with aging is one of the major advantages of animal imaging that cannot be done in the clinic.
My introduction to animal imaging was discouraging. I was told that the rat had to be anesthetized and that the radiofrequency signal would be received by a loop of copper wire placed at the top of the head limiting the image to the underlying cortex. With talented electrical and mechanical engineers, a company was started, aimed at designing and developing the first radiofrequency electronics and restraining systems for awake imaging in small animals.
Why animal imaging when human imaging should suffice? Indeed, structural brain imaging started in the 1980s and functional brain imaging followed in the 1990s (Ogawa et al., 1992) predating any animal studies. The answer is simple “greater experimental latitude”, i.e., we can do things in animals that we cannot do in humans. Some of the best examples can be found in the study of Schedule I drugs, i.e., drugs with no currently accepted medical use and a high potential for abuse, like methylenedioxymethamphetamine (ecstasy), heroine, and until recently, marijuana. The applications of awake imaging are the primary emphasis of this review. The section “Background” will briefly cover methodological issues around awake imaging using MRI.
Background
Avoiding Anesthesia
Animal imaging without anesthesia was met with some resistance, an attitude that persists today based on the many publications proposing “better” anesthetics for functional MRI studies. The contrarians argue that the reduction in stress and motion artifact using anesthesia and sedatives is better than the confound of stress when interpreting brain function. The hesitancy to adopt awake imaging is also founded in the need for specialized technology, additional costs, and time, and for some institutions, ethical issues around the care and treatment of animals. While there are multiple reasons to avoid anesthesia when studying brain function (see reviews Gao et al., 2017; Steiner et al., 2021), many obvious to the layperson, the most compelling is translation to the human condition. We do preclinical studies on brain function in animals to inform the public and guide clinical researchers in their effort to understand the human condition. This communication from bench top to clinic is made seamless if the same imaging modalities, at similar field strengths and under the same physiological conditions is performed by both. Human brain function is never studied under general anesthesia unless you are studying the effects of anesthesia. How antipsychotics, anxiolytics, analgesics, psychostimulants, drugs of abuse, etc., affect brain function cannot be studied under anesthesia. CNS active drugs affect cognition, emotion, and perception of the external and internal milieu all of which are affected by anesthesia.
Acclimation
There are many published variations on acclimation protocols for preparing different animals for awake imaging, e.g., rats (King et al., 2005; Upadhyay et al., 2011; Russo et al., 2021), mice (Ferris et al., 2014; Yoshida et al., 2016; Madularu et al., 2017b; Han et al., 2019), marmoset monkeys (Ferris and Snowdon, 2005; Silva et al., 2011; Ziegler et al., 2020), rabbits (Wyrwicz et al., 2000), dogs (Berns et al., 2012; Cuaya et al., 2016), and rhesus macaques (Goense et al., 2010). Some describe surgical methods and implants for immobilizing the head while many use non-invasive passive restraining systems. In general, animals are lightly anesthetized with a volatile anesthetic, secured in a restraining system, and allowed to recover in a virtual environment meant to mimic the scanner. This virtual simulation with head restraint is usually accompanied by background noise mimicking the actual noise associated with the pulse sequence (Almeida et al., 2021). In our first studies (King et al., 2005) rats were acclimated for 90 min every other day for eight days. Acclimation was assessed by changes in heart rate, respiratory rate, and blood levels of the stress hormone, corticosterone. Physiologic and endocrine measures of autonomic arousal decrease with acclimation. Interestingly, when rats are imaged awake without acclimation and again after several days of acclimation we found no differences in cerebral blood flow (CBF) across multiple brain regions. Essentially baseline CBF is stable in awake rats regardless of stress, a critical point when measuring changes in functional signal dependent upon blood flow. In our more recent studies, we acclimate for five consecutive days, increasing the duration of acclimation with each subsequent day (Sadaka et al., 2021).
In some cases anesthesia can be completely avoided during the acclimation procedure. Rats surgically implanted with a head post that fits into a cradle to prevent any rotational motion can be trained over 8–10 days to enter a “snuggle sack,” slide their head post into the cradle, and lay motionless (Chang P. C. et al., 2016). While time consuming, this procedure eliminates the early use of light anesthesia required for most awaking imaging set-ups and provides excellent functional imaging with a minimum of motion artifact. Silva et al. (2011) described a step-by-step procedure for acclimating marmoset monkeys for each separate phase of the set-up, e.g., magnet environment, restraint, noise, etc., without the use of anesthesia. Ziegler et al. (2020), developed a method for acclimating preadolescent common marmoset monkeys for awake imaging without ever being exposed to anesthetics or sedatives, a condition that better reflects the human imaging experience. The unique design of the head cradle eliminated the need for ear bars or bite bar, helping to minimizing discomfort. The first study on awake dogs trained two animals to remain motionless for enough time to acquire images without the use of anesthetics, sedatives or physical restraint (Berns et al., 2012). Indeed, the absence of any exposure to anesthetics and sedatives with minimum restraint has been the case for all laboratories imaging awake dogs (Andics et al., 2014; Cuaya et al., 2016; Huber and Lamm, 2017).
The purpose of acclimation is to reduce stress and minimize motion artifact. Interestingly this is not true for all animals. We discovered that prairie voles can be imaged without acclimation on their first time in the scanner (Yee et al., 2016). Efforts to acclimate only exacerbated the stress as measured by motion artifact, vocalizations, and recording heart rate/respiratory sinus arrhythmia.
Acoustic Noise
As noted above, MRI is noisy. The rapid changes in electromagnetic forces driving the gradients create the acoustic noise that is pulse sensitive and exacerbated at high field strengths (Mansfield et al., 1995). The problem has been effectively addressed in human imaging with hardware solutions, wave phase reduction, and altered pulse sequences, all of which can reduce the noise by as much as 20 dB (McJury et al., 1997; Hennel, 2001; Katsunuma et al., 2002). Most recently, Paasonen et al. (2020), addressed the problem in awake rat imaging by developing Multi-Band SWeep Imaging with Fourier Transformation (MB-SWIFT) at 9.4 T for EPI sequences. They report a reduction in acoustic noise by 20 to 30-dB as compared to standard EPI sequences accompanied by a decrease in motion artifact providing resting state connectivity data comparable to those reported standard EPI studies (Paasonen et al., 2020).
Radiofrequency Coils and Restrainers
Our first studies used a volume coil to transmit and a saddle shaped surface coil to receive radiofrequency signals. The head restrainer employed ear bars and a tooth bar, much like a stereotaxis, to immobilize the head. The body was restricted to a fitted tube. Since then, the RF electronics evolved into a single quadrature transmit/receive volume coil that was customized to the size of the rat’s head to optimize brain coverage and space filling for better signal-to-noise (SNR) and homogeneity (Figure 1). The ear bars were eliminated and replaced with a passive head restraining system to minimize the discomfort and stress (see video of rat setup for awake imaging https://www.youtube.com/watch?v=JQX1wgOV3K4). The system is ergonomic enabling setup in minutes, and completely non-invasive with no surgical implants used to immobilize the head. There have been several publications on the construction of RF coils and restraining systems for awake MRI imaging in rats (Martin et al., 2013; Chang J. B. et al., 2016; Madularu et al., 2017a; Stenroos et al., 2018; Derksen et al., 2021), mice (Ferris et al., 2014; Madularu et al., 2017b; Tsurugizawa et al., 2021), and the common marmoset monkey (Papoti et al., 2013, 2017; Schaeffer et al., 2019; Ziegler et al., 2020).
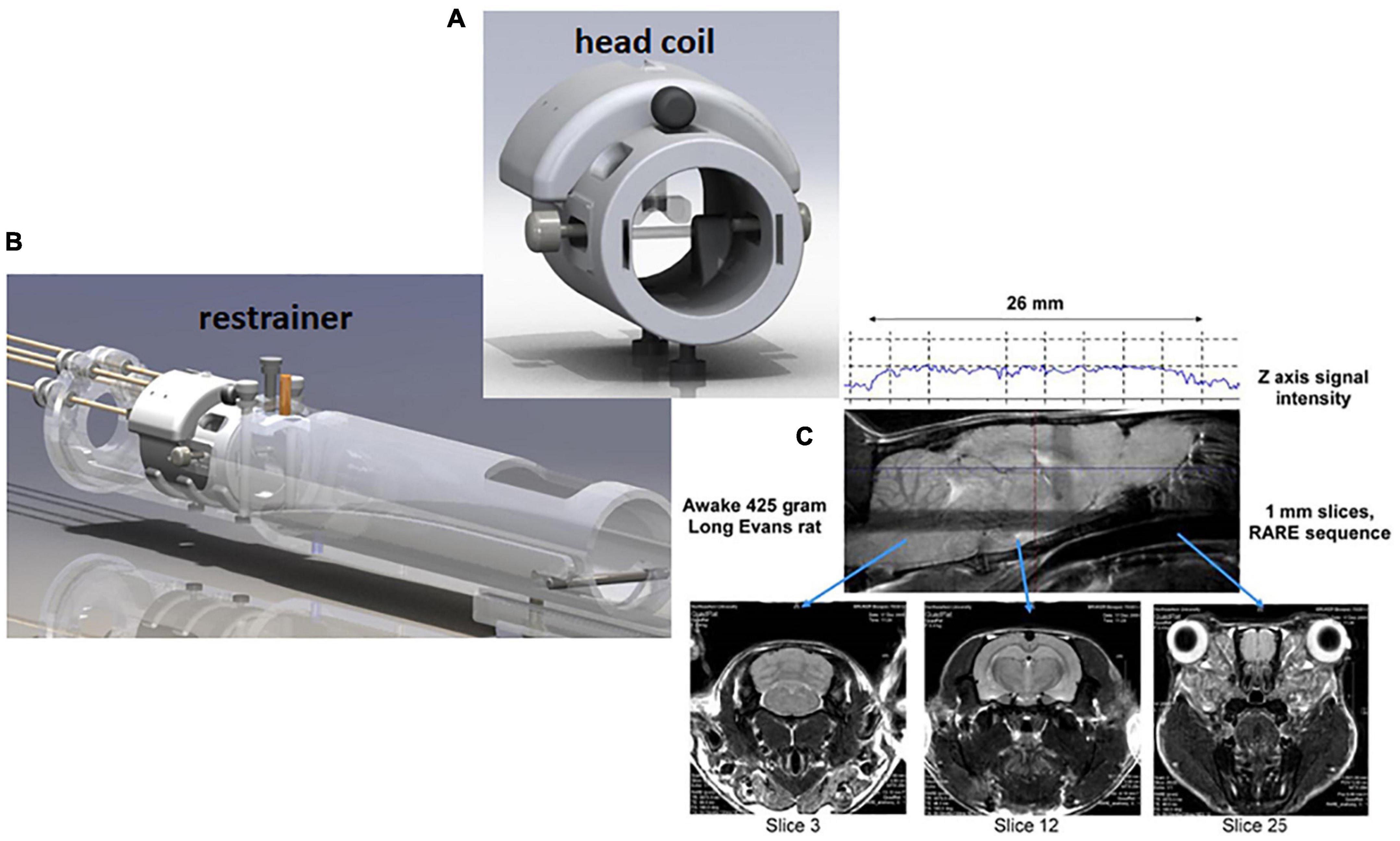
Figure 1. Rat imaging system. Shown are a radiofrequency coil (A) and restraining system (B) for awake rat imaging. A quadrature transmit/receive volume coil is built into the head restrainer to optimize space fitting, signal-to-noise, and field homogeneity. The design of coil allows for whole brain imaging from olfactory bulbs (slice 25) to cerebellum (slice 3) (C).
Event-Related Blood Oxygen Level Dependent Imaging
The common read-out for awake imaging in animals and humans is a change in blood oxygen level dependent (BOLD) signal contrast (Kim and Ogawa, 2012) as compared to a baseline obtained prior to the introduction of a drug or a general stimulus. The change in signal can occur within 3–10 s after provocation and can persist for a different duration in time dependent upon the experimental paradigm and the presence or absence of anesthesia (Martin et al., 2006). In some of the earlier studies changes in CBF and contrast enhanced cerebral blood volume (CBV) were measured as markers for functional brain activity. To optimize anatomical fidelity a spin echo pulse sequence can be used. It should also be emphasized that high neuroanatomical fidelity and spatial resolution are critical in identifying distributed neural circuits in any animal imaging study. Many brain areas in a segmented rat atlas have in-plane boundaries of less than 400 μm2 and may extend for over 1,000 μm in the rostral/caudal plane. With the development of a segmented, annotated 3D MRI atlas for rats (Ekam Solutions, Boston, MA, United States), it is now possible to localize functional imaging data to 173 precise 3D “volumes of interest” in clearly delineate brain areas. Therefore, it is critical that the functional images are a very accurate reconstruction of the original brain neuroanatomy as shown in Figure 2.
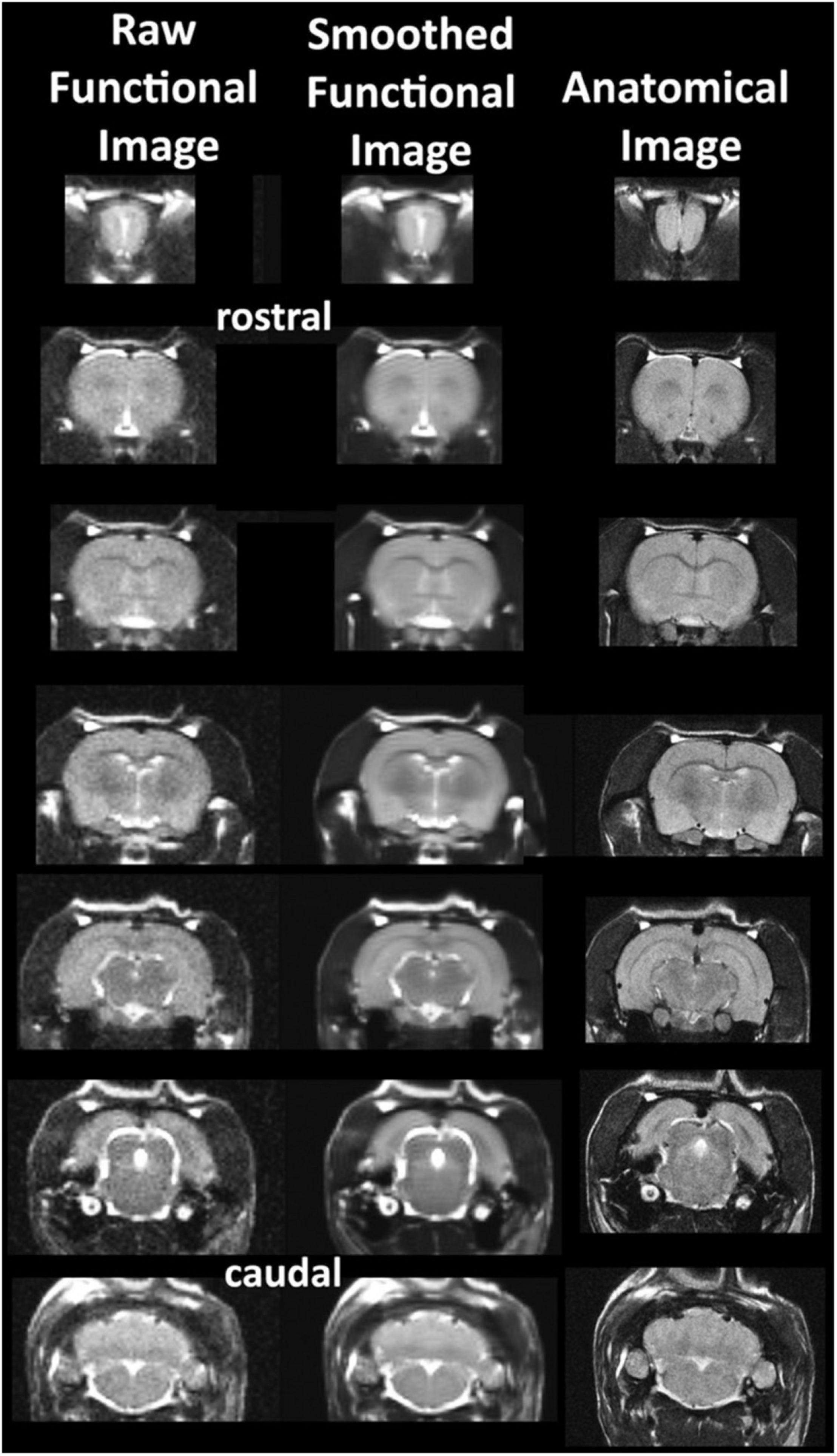
Figure 2. Anatomical fidelity. Shown are examples of brain images collected during an awake imaging session in rats using a multi-slice spin echo, RARE (rapid acquisition with relaxation enhancement) pulse sequence. The anatomical images to the right are taken at the beginning of the scanning session. The column on the left shows the same images but collected for functional analysis using HASTE, a RARE pulse sequence modified for faster acquisition time. Whole brain acquisition time is approximately six sec with a spatial resolution of 312 μm2. The images in the middle column have been smoothed during pre-processing. Note the anatomical fidelity between the functional images and their original anatomical image. The absence of any distortion is necessary when registering the data to an atlas. (Adapted with permission from Ferris et al. (2015)).
Resting State Functional Connectivity
This modality was originally described by Biswal and colleagues as connections between brain areas based on similar low frequency fluctuations in BOLD signal (Biswal et al., 1995). Realistically, resting state in awake animal imaging means no external stimulus. There is undoubtedly some level of stress associated with resting state functional connectivity (rsFC) in animals, particularly rodents. However, following different acclimation protocols, rsFC neural circuits analogous to those reported in human imaging have been identified in animals (for recent review see Pais-Roldan et al., 2021). The first method for rsFC in awake rats was published by Zhang et al. (2010) using a seed-based correlation analysis. Subsequently, studies from different labs used independent component analysis to identify functional clusters or neural circuits, e.g., default mode, salience mode, analogous to those found in humans (Becerra et al., 2011b; Liang et al., 2011; Upadhyay et al., 2011; Belcher et al., 2013; Liu et al., 2019). Tu et al. (2021) combined Designer Receptors Exclusively Activated by Designer Drugs (DREADDs) chemistry and rsFC to suppress the anterior cingulate cortex, a key circuit hub, and showed alterations in whole brain neural circuit organization and integration in the rat. Combining optogenetics with rsFC, Desai et al. (2011), showed they could activate the primary somatosensory cortex and recruit a distributed neural circuit of brain regions that included the motor cortex, striatum, and contralateral somatosensory cortex in mice. rsFC in mice has also been used to identify individual variations in the organization of the cortex that could predict differences in motor behavior (Bergmann et al., 2020). However, there is a dilemma when imaging rsFC in awake animals as noted above. How to choose between the confound of mild stress and arousal associated with the fully awake condition or the use of light anesthesia or sedation which would reflect a true “resting” condition, albeit artificial. The BOLD signal obtained with a combination of isoflurane and medetomidine, an α2 adrenergic receptor agonist, is reported to accurately reflect changes in brain activity and associated neural vascular coupling for use in rsFC (Grandjean et al., 2014; Brynildsen et al., 2017). However, there have been other studies comparing different anesthetics that do not support the use of isoflurane/medetomidine as the best choice for rsFC (Paasonen et al., 2017, 2018), a disparity possibly due to the dosing regimen of medetomidine (Kint et al., 2020).
Small Animals
Over time there have been several publications across numerous mammalian species on methods and applications for awake imaging. These include mice (Ferris et al., 2014), rats (Febo, 2011), voles (Yee et al., 2016), rabbits (Weiss et al., 2018), cats (Ma et al., 2013), dogs (Berns et al., 2012), pigeons (Behroozi et al., 2020) common marmosets monkeys (Ferris et al., 2001; Papoti et al., 2013; Silva, 2017), and rhesus macaques (Goense et al., 2010). Each of these species, most obviously rhesus monkey, have general or unique attributes that provide a better understanding of the human condition. For example the special relationship between dogs and humans and the communication between them [see seminal studies by Andics et al. (2014, 2016) and Huber and Lamm (2017), Karl et al. (2020, 2021)]. However, much of the work today is focused on rodents. From the early CSI-II 2.0T produced by GE to the ultra-high field 11.7T system developed by Bruker, the bore size was kept small to reduce the cost of the technology while pushing the limits of spatial resolution. Hence these small-bore systems (≤30 cm) favor the use of small animals. To that point, this review is primarily focused on rodents and their many applications in awake imaging.
Applications
Pharmacological MRI
One of the major applications of awake imaging in rodents has been driven by pharmaceutical companies to aid in characterizing or “fingerprinting” drug candidates in early drug discovery. Does a drug candidate get into the brain and interact with its target in a dose-dependent manner? The general protocol is simple and usually involves three different doses of drug plus a vehicle control. These are usually small molecules with specific CNS targets. Scientists from Abbott Laboratories (Abbott Park, IL, United States) pioneered the work on Pharmacological MRI (phMRI) in awake rats. Using a potent agonist for α4β2 nicotinic acetylcholine receptor they mapped the change in brain activity that fit the know localization of the receptors demonstrating target engagement and activation of neural circuitry that fit the behavioral data (Skoubis et al., 2006). In another study they used phMRI to show dose-dependent activation of neural circuitry involved in emesis as a model for predicting emetic liability of early drug candidates (Chin et al., 2006). Addressing the topic of pain regulation through cannabinoid signaling they used a non-selective CB1/CB2 and selective CB2 receptor agonists to affect BOLD signal and concluded CB1 and not CB2 was the primary target of cannabinoid signaling (Chin et al., 2008). They also mapped the BOLD activation pattern of subanesthetic doses of ketamine showing both positive and negative BOLD changes over the brain (Chin et al., 2011). These studies included drugs that would block the effects of ketamine as a potential imaging method for screening new anti-psychotics (Baker et al., 2012). Tang et al. (2018) used phMRI to map brain activation in awake rats in response to ketamine and traxoprodil, a putative antidepressant and selective GluN2B NMDA receptor antagonist. Traxoprodil produced a dose-dependent change in specific brain regions many that overlap with the activation pattern of ketamine (Tang et al., 2018).
In a just published study in mice treated with the phytocannabinoid cannabidiol (CBD) we reported a dose-dependent polarization of BOLD signal change along the rostral-caudal axis of the brain with the forebrain showing positive signal while the brainstem and cerebellum presented with negative signal (Sadaka et al., 2021). The brainstem ascending reticular activating system (ARAS) was decoupled to much of the brain but was hyperconnected to the olfactory system and prefrontal cortex. In one of the more compelling phMRI studies, Becerra et al. (2013) ran a parallel experiment between rats and humans comparing species equivalent doses of buprenorphine, a partial mu-opioid receptor agonist on circuit activation and showed comparable patterns of BOLD signal change. This study demonstrated the significance of phMRI in translating drug function between rats and humans in early drug discovery. In a subsequent study, published only a year later, this translation of buprenorphine activity across species using phMRI was also demonstrated using cynomolgus monkeys (Seah et al., 2014).
Not all small molecules with CNS targets present with a dose-dependent change in brain activity. To this point, is evidence from our lab on a study using the neuropeptide oxytocin, a neurochemical signal involved in social and emotional behavior (Carter et al., 2020). Systemically administered oxytocin failed to induce a dose-dependent change in BOLD signal in a study comparing central vs peripheral routes of oxytocin administration (Ferris et al., 2015). While a single dose of intracerebroventricular (ICV) oxytocin activated many of the brain areas with a high density of oxytocin receptors, peripheral oxytocin failed to do so. However, peripheral oxytocin had its own unique “fingerprint” affecting the olfactory bulb, cerebellum, and several brainstem areas relevant to the ARAS. The patterns of brain activity suggest that peripheral oxytocin may interact at the level of the olfactory bulb and, through sensory afferents from the autonomic nervous system, influence brain activity This raises an interesting consideration when performing any functional brain imaging study in response to a systemically administered drug. While the drug may cross the blood brain barrier and show target engagement, the final profile of BOLD activation may have contributions from the peripheral nervous system.
Drugs of Abuse
Cocaine
In the early 2000s, Febo and colleagues published a series of foundational studies on the effects of cocaine on brain function in awake rats. To avoid perturbations in cardiovascular and respiratory function they used ICV injections during the scanning session to elicit activity in dopaminergic pathways (Febo et al., 2004). To follow the neuroadaptive changes that occur with drug addiction, they treated rats for several consecutive days with cocaine followed by a washout period and cocaine challenge during awake imaging (Febo et al., 2005c). Rats with a history of cocaine exposure showed a blunted BOLD response in dopaminergic pathways as compared to drug naïve rats. Following up on the literature reporting estrogen alters behavioral sensitivity to cocaine (Becker, 1999), Febo et al. (2005a) worked with ovariectomized rats with and without estrogen treatment and chronically exposed to cocaine. Following a washout period, females with estrogen showed enhanced BOLD signal changes in dopaminergic pathways as compared to drug naïve controls exposed to cocaine in contrast to the male response reported in their previous study. The Febo lab continued to publish numerous studies on cocaine in awake rats on topics that included sexual receptivity (Febo et al., 2011), odor processing (Johnson et al., 2013; Caffrey and Febo, 2014), epigenetics (Febo et al., 2009a), and maternal care in rats addicted to cocaine prior to pregnancy (Febo and Ferris, 2007; Nephew et al., 2012).
Using a modified rat holder that enabled dams to breast feed during the imaging session (Figure 3), we asked the question “Is pup suckling more rewarding than cocaine?” (Ferris et al., 2005). Before the imaging session, 4-to-8-day old pups were physically isolated from their mother in the home cage for 2–3 h by confining them to an inverted perforated Plexiglas box. These pups were then positioned in the magnet in a cradle beneath the mother separated by a thin plastic sheet. During the imaging session the sheet could be removed exposing the hind end teats, at which time the pups would immediately start to feed. Pups sucking induced a robust positive BOLD activation of dopaminergic circuitry with little negative BOLD. In the same study, virgin females given cocaine showed the same pattern of positive BOLD activation with little negative BOLD evidence that both breast feeding and cocaine elicit similar brain mechanisms regarding motivation and reward. Interestingly, when mothers were exposed to cocaine and not their pups the pattern of brain activity was reversed - the dopaminergic reward circuitry showed primarily negative BOLD. Hence, the reproductive condition of the female appears to be a critical determinant in predicting the effect of cocaine on brain activation.
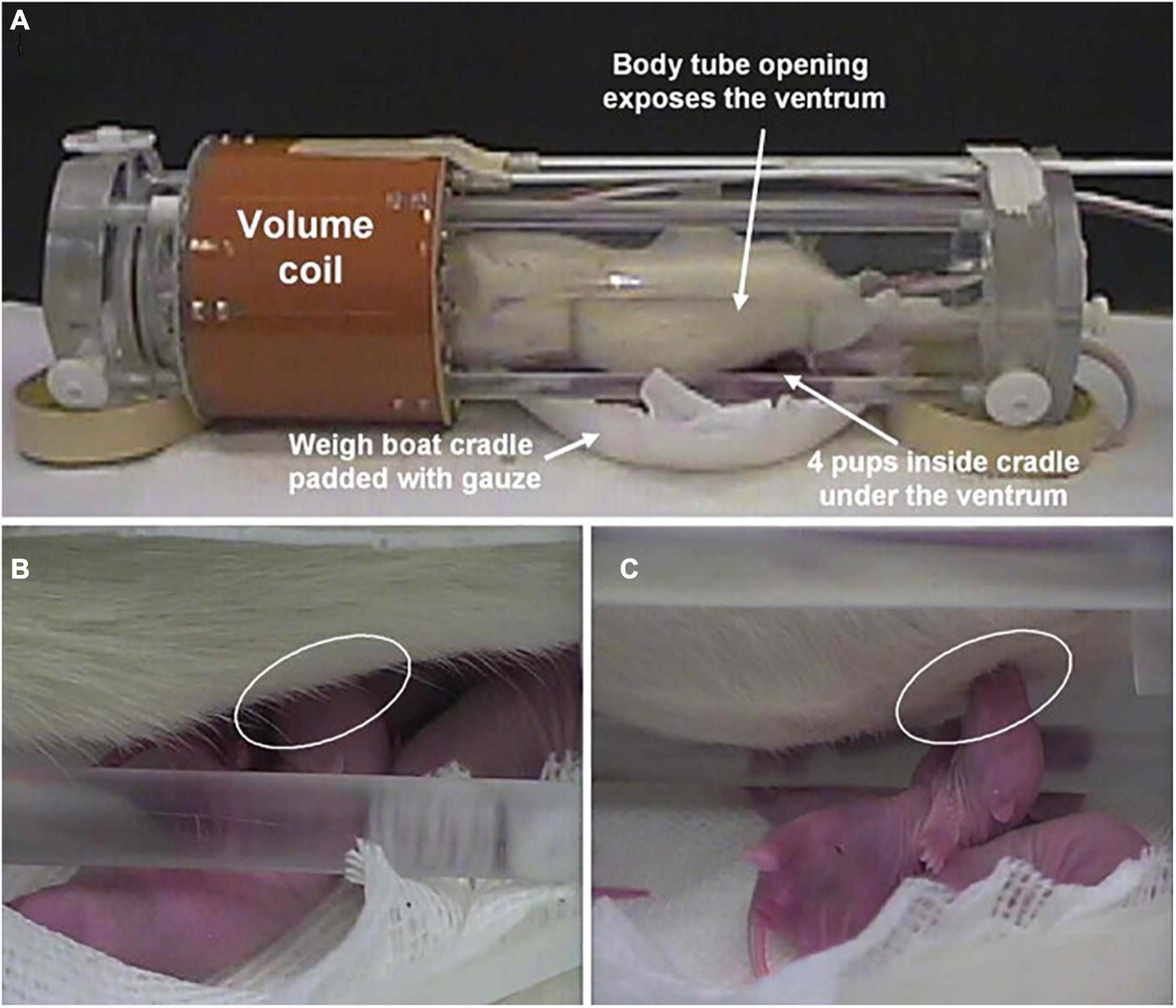
Figure 3. Setting for pup suckling in the magnet. Shown is the experimental setup for imaging pup suckling during the scanning session of awake dams. (A) The opening in the body tube allowing pups to gain access to the dam for nursing. Pups are in a cradle beneath the dam. (B) Pup suckling is established within seconds during a functional scan. (C) Side view showing a suckling pup. (Adapted with permission from Febo et al. (2005b)).
Opioids
Kaufman et al. (2013) published the first study on opioids using fentanyl a mu receptor agonists and U69,593 a kappa agonist in awake cynomolgus monkeys and showed selective activity for each drug on reward circuitry. The activity of oxycodone was characterized in a genetic mouse model devoid of the mu-opioid receptor vs wild type controls (Moore et al., 2016). The positive BOLD signal change essentially mirrored the localization of mu-opioid receptors in wild-type but was absent in the knock-out mice when mapped onto a segmented 3D MRI atlas with 122 brain areas. The pattern of activity in wild-type mice was also closely associated with key brain areas involved in pain regulation. The effects of acute and chronic exposure to oxycodone in rats were studied to follow the neuroadaptive changes that occur with opioid addiction (Iriah et al., 2019). The initial design had drug naïve rats imaged during for their first scanning session in response to systemically injected oxycodone. Rats were then treated on four consecutive days with oxycodone in a conditioned place preference chamber to demonstrate drug seeking behavior. On the following day rats were again scanned in response to vehicle control or oxycodone. The study was terminated because of severe motion artifact during the baseline acquisitions for both treatments. Interestingly, the hyperexcitability was ascribed to withdrawal symptoms as treatment with oxycodone eliminated the motion artifact that persisted with vehicle. To follow the neuroadaptive changes that occur with chronic oxycodone exposure it was necessary to use manganese enhanced imaging with general anesthesia to localize the accumulation of contrast agent in dopaminergic pathways, hippocampus, and amygdala.
Amphetamine
Madularu et al. (2015b) studied brain activity in ovariectomized female rats sensitized to amphetamine with different levels of estrogen replacement and in the face of a haloperidol treatment. Amphetamine on a background of high estrogen enhances brain activation in dopaminergic pathways (Madularu et al., 2015b). Ovariectomized rats exposed to daily treatments of the antipsychotic haloperidol together with estrogen replacement also show enhanced responsivity to amphetamine challenge in neural circuits associated with schizophrenia, e.g., hippocampus, habenula, amygdala, and alterations in whole brain and hippocampal volumes (Madularu et al., 2015a,2016). Together these studies suggest awake imaging in rats can be used to assess the interaction between ovarian steroids and the sensitivity of neuroleptics to impact dopaminergic signaling in brain areas associated with schizophrenia. Awake rats challenged with amphetamine show an increase in cerebral blood volume correlated with changes in striatal dopamine concentrations (Kashiwagi et al., 2015).
Tetrahydrocannabinol
Tetrahydrocannabinol (THC), the psychoactive ingredient in cannabis, was first tested in awake rats by systemic injection (Madularu et al., 2017c). Low dose of THC, but not vehicle or high dose THC increased positive and negative BOLD signal in areas high in CB1 receptors and in neural circuits associated with pain and cognition. Fara and coworkers ran the first and only imaging study to date on awake rodents looking at changes in brain activity in drug naïve mice exposed to vaporized cannabis (12.3% THC) during the scanning session (Farra et al., 2020). This study using inhaled cannabis was designed to achieve blood levels of THC reported in human recreational use via a route of administration that better reflects the human experience. There were robust changes in both positive and negative BOLD signal (Figure 4). The positive BOLD activation map suggests enhanced sensory processes while the negative BOLD map suggests reduced behavioral arousal.
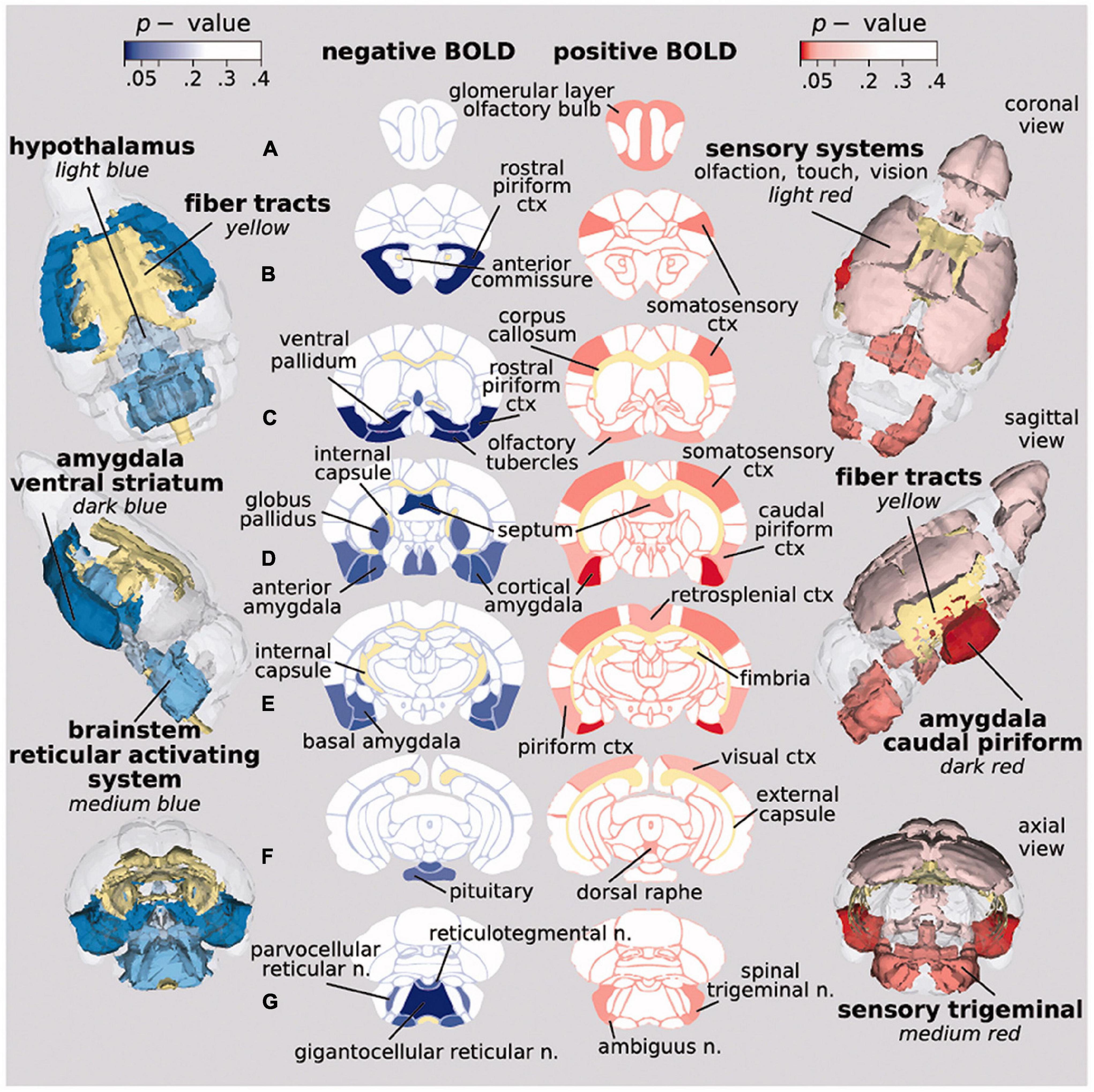
Figure 4. Activation maps on inhaled vaporized cannabis. Probability maps showing significant changes in BOLD signal in brain areas that differed in mice exposed to inhaled cannabis or placebo. Positive BOLD (red) reflects an increase in signal, while negative BOLD (blue) indicates a decrease. (Adapted with permission from Farra et al. (2020)).
MDMA
The studies on methylenedioxymethamphetamine (MDMA, ecstasy) in awake animals are few. Because MDMA is a Schedule I drug there are no studies on drug naïve humans. During the scanning session an oral dose of MDMA was given to marmosets and images acquired over 90 min (Brevard et al., 2006b; Meyer et al., 2006). The change in brain activity over time was correlate with the change in blood levels of MDMA.
Nicotine
Li et al. (2008) studied the addictive properties of nicotine in awake rats by comparing the change in BOLD signal intensity to acute and chronic drug exposure. Repeated exposure to nicotine prolongs BOLD activation in the hippocampus, reward circuitry and prefrontal cortex. In a subsequent study, varenicline, a partial agonist at the α4β2 nicotinic acetylcholine receptor used to treat nicotine addiction, was tested in awake rats, and was shown to produce a pattern of brain activity not unlike nicotine alone. However, repeated exposure to varenicline failed to cause the sensitization effect of nicotine (King et al., 2011) evidence in support of varenicline’s therapeutic efficacy. Adolescent male rats chronically exposed to nicotine with or without menthol show differences in functional connectivity (Thompson et al., 2018). Menthol combined with nicotine show enhanced functional connectivity in reward circuitry and other regions not seen with nicotine alone.
Ethanol
There are only two studies done on awake rats given ethanol during the scanning session. The first measured the change in CBV using contrast enhanced functional MRI and reported a robust increase in activity in cortex and striatum (Luo et al., 2007). The second, trained rats to respond to the conditioned stimulus of a light pulse during imaging while simultaneously giving i.p. ethanol. After several days of this conditioning procedure, the caudate-putamen, anterior insular cortex, hippocampus, ventral pallidum, and nucleus accumbens were activated by light alone (Tsurugizawa et al., 2012).
Sensory Evoked Stimuli
There have been many imaging studies on awake animals following changes in brain activity to natural stimuli like olfaction, visual cues, sound, touch, and taste. Mice given light pulses of different durations and frequencies showing activation in visual pathways (Dinh et al., 2021). The most common stimulus in rodents is the presentation of an odor. Kulkarni et al. mapped changes in brain activity to four different odors presented to rats during the scanning session (Kulkarni et al., 2012). As expected, all odors activated olfactory pathways; however, one odor, benzaldehyde, the smell of almond, increased activity in brain areas associated with emotion, memory, and reward. Indeed, mammals have genes that code for the benzaldehyde receptor in olfactory epithelium (Bozza et al., 2002). That evolution would favor the smell of almond is not surprising given its caloric density and durable structure. Hence the smell of almond elicits a positive emotional response in rats. What makes this study so interesting is that rats challenged with benzaldehyde were “odor naïve” never having been exposed to almond. The response was innate! The brain is wired to recognize benzaldehyde as a rewarding stimulus. As a validation for awake imaging in prairie voles, animals were presented with different odors and the change in brain activity mapped to a 3D MRI vole atlas (Yee et al., 2016). Zhifeng and coworkers developed an awaking imaging system in mice that allows behavioral responses, e.g., licking, to odor cues (Han et al., 2019). Using an implanted head holder, mice can be trained to lick for water during in a go/no-go conditioning paradigm driven by odor cues. This same awake mouse imaging system can also be used to follow brain activity in response to sensorimotor and auditory stimuli during the scanning session (Chen et al., 2020). Male marmoset monkeys presented with the smell of sexual receptive females show positive BOLD activation in many areas of the brain (Ferris et al., 2001) that is reversed, i.e., negative BOLD, when the stimulus comes from an ovariectomized female (Ferris et al., 2004).
With respect to marmoset monkeys, there are awake imaging studies mapping brain activity in response to touch, sound, and visual stimuli. The application of light touch by a puff of air delivered to the face, arm or leg produces cortical and subcortical maps of topographical body representation (Clery et al., 2020a). Presentation of mixed and pure tones revealed the auditory cortex has tonotopic organization for processing complex and simple stimuli (Toarmino et al., 2017). There are several studies looking at the response of the visual cortex and associated neural circuitry to various types of visual stimulation paradigms. Marmosets can be taught to carry out visual tasks during the scanning session in response to the presentations of different images, e.g., conspecific faces, conspecific body parts and general objects, resulting in the activation of visual cortex and subcortical areas (Hung et al., 2015b). The regional pattern of activity is selective for different categories of visual stimuli. For example, the occipitotemporal pathway shows the strongest preference for faces over other stimuli (Hung et al., 2015a). Objects in motion, coming toward or receding, evoke activity across a large cortical network involving frontal, parietal, temporal, and occipital areas. The activation is greatest as objects come closer suggesting a network for processing stimuli entering a peri personal space (Clery et al., 2020b). Marmosets can be imaged while watching movies to record patterns of brain activity that reflect a more naturalistic, complex stimulus. Patterns of brain activity in movie driven fMRI between marmoset monkeys and humans show similar functional correlates and circuit organization (Hori et al., 2021).
The original “vivarium” studies included the resident/intruder model (Ferris et al., 2008), pup suckling model (Ferris et al., 2005; Febo et al., 2008) (see Figure 3) and another imaging lactating dams responding to the visual presentation of their own pups with and without a male intruder (Nephew et al., 2009). This staged environmental situation using other animals to elicit changes in brain activity was recently used by Gilbert and coworkers to model social interactions between marmosets by simultaneously measuring brain activity in awake marmosets during the scanning session (Gilbert et al., 2021). Each restraining system was equipped with its own RF electronics. Each monkey was positioned facing each other providing the means for visual and olfactory communication. The activation maps generated by this social interaction were associated with visuomotor tasks.
Brain Disorders
Epilepsy
In a series of seminal studies, Ferris and Tenney (2013) used awake imaging in rats and marmoset monkeys to study the neural circuitry of absence seizures. Absence seizure or petit mal do not involve uncontrolled skeletal muscle contraction; instead, the seizures is characterized by a 3 Hz spike wave discharge on EEG accompanied by a trance-like behavior that may persist for over a min (Snead, 1995). Without the confound of motion artifact this type of epilepsy is fertile ground for awake imaging. Giving rats γ -hydroxybutyric acid (GBH), a metabolite of the inhibitory neurotransmitter GABA, Tenney et al. (2003), could triggered an absence seizure during the scanning session. Unlike humans the slow wave discharge is 6–7 Hz s; nonetheless, the pattern of brain activation was centered on corticothalamic neural circuitry fundamental to human absence seizures. In a subsequent study using the WAG/Rij rat, a genetic model of spontaneous absence seizure, Tenney et al., used the onset of the 6-7 Hz EEG signal to initiate image acquisition (Tenney et al., 2004a). This EEG-triggered BOLD imaging shows a dominant corticothalamic activation pattern similar to the GBH-induced seizure. In a final study they used the precursor molecule to GBH, γ-butyrolactone (GBL), to induce absence seizures in marmoset monkeys (Tenney et al., 2004b). In this model they were able to reproduce the 3Hz spike wave discharge, the diagnostic criteria for a human absence seizure, making this model more relevant to the human condition. Again the absence seizure is dominated by corticothalamic activation that could be blocked by treatment with the antiepileptic drug, ethosuximide. While it is not possible to image a generalized tonic-clonic seizure due to motion artifact, it is possible to image the organization of brain activity that contributes to the genesis of this type of seizure. To this end, Brevard et al. (2006a) used pentylenetetrazol (PTZ) to trigger a tonic-clonic seizure in rats during the scanning session. Within seconds of giving PTZ ICV there is an increase in BOLD signal in the thalamus, particularly anterior thalamic nucleus, retrosplenial ctx and hippocampus. These areas peak in BOLD activation just seconds before seizure onset. Pretreatment with ethosuximide blocks all activity in the anterior thalamic n. and retrosplenial cortex and prevents seizure onset.
Fragile X Syndrome
The olfactory stimulus of almond that triggers the innate circuitry involved in motivation toward a rewarding stimulus was tested in a genetic rat model of Fragile X (Kenkel et al., 2016). Fragile X syndrome is the leading genetic cause of autism, a condition noted for withdrawal, gaze aversion and disrupted social communication. The study was done with “odor naïve” wild-type and fragile X mental retardation 1 gene (fmr1) KO male rats. When exposed to the smell of almond, wild-type rats show the expected activation of olfactory and reward circuitry. KO rats on the other hand, fail to show positive BOLD activation, but instead show negative BOLD in brain areas associated with emotion and reward. These findings suggest that the disrupted social communication and avoidance behavior in autism may be due, in part, to the inability to process rewarding stimuli.
Huntington’s Disease
The presentation of almond odor has also been used to map brain activity in a mouse model of Huntington’s disease, the zQ175 knock-in mice containing a human mutant allele with the expanded CAG repeats within the native mouse huntingtin gene (Ferris et al., 2014). Changes in BOLD activity were compared between wild-type, HETzQ175, and HOMzQ175 genotypes. HOMzQ175 mice show a reduced response to almond odor as compared to the other genotypes suggesting a deficit in olfaction which is common in neurodegenerative diseases (Patino et al., 2021). The expected changes in cortex and basal ganglia that characterize Huntington’s were not observed; instead, there was gene-dose effect on brain areas high in huntingtin associated protein 1.
Alzheimer’s Disease
Sakurai et al. (2020) probed the APPswe / PSEN1dE9 double transgenic mouse model of Alzheimer’s for biomarkers of altered reward-based behavior. To do so, they developed an imaging system to follow changes on BOLD activity during licking behavior in a 14T scanner. Thirsty mice are given light cues to elicit drinking. The conditioned drinking response is associated with hyperactivity in the raphe nucleus and hippocampus, a response that may reflect the enhanced impulsivity common to Alzheimer’s.
Parkinson’s Disease
While this review is primarily focused on small rodents and marmoset monkeys, attention should be drawn to the work of Zhang et al. (2000) on their studies using awake rhesus monkeys to understand drugs that impact basal ganglia activity in normal controls, during aging (Zhang et al., 2001) and in a MPTP model of Parkinson’s disease (Zhang et al., 2006). Studies combining normal aging with aging plus MPTP and drug treatments involving the dopamine receptor agonist apomorphine and different dopamine receptor antagonists were able to parse out the altered motor dysfunction seen in Parkinson’s and aging as changes in striatum and globus pallidus, respectively (Andersen et al., 2015). The results show it is feasible to use drug provocation protocols to differentiate Parkinson’s from age-associated parkinsonism.
Pain
Studies on pain necessitate the use awaking imaging. The data collected from acute and chronic pain models provide insights into the integrated neural circuits processing nociceptive stimuli and the neuroplasticity of these circuits to chronic pain and their responsiveness to therapeutics. Becerra et al. (2011a) applied a thermal stimulus of 48°C to the hindpaw of awake rats and reported activation of cortical, subcortical and brainstem areas consistent with the know literature on pain neural pathways. These researchers also studied a unique rat model for migraine pain that entails the application of a cocktail of inflammatory agents to the dura of awake rats to activate nociceptive fibers in the meninges (Becerra et al., 2017). This inflammatory soup (IS) model was used to look at the altered sensitivity to mechanical and thermal stimulation and resting state FC during the scanning session. With this model of intracranial pain they found hypersensitivity to mechanical stimulation of the face as evidenced by an increase in BOLD signal in hypothalamus, hippocampus, and somatosensory cortex with altered rsFC. In a subsequent study sumatriptan, 5HT-1B/D-receptor agonist and naproxen sodium, a COX-1 and COX-2 inhibitor, drugs used to treat migraines were tested in the IS model and shown to alter whole brain neural circuits that included the default mode, salience, sensorimotor, and cerebellar networks (Bishop et al., 2019). The IS model has also been used by others to show altered rsFC in the periaqueductal gray and anterior cingulate to brain regions involved in the sensory and emotional aspects of migraine headaches (Jia et al., 2017, 2019). Yee et al. (2015) looked at the response to intradermal capsaicin injection into the hindpaw of rats. The specificity of the pain response to capsaicin was tested in a transgenic rat model with a deletion of the TRPV1 gene, the target of capsaicin. The putative neural circuitry of pain is activated in wild-type but not transgenic rats. In addition, wild type but not KO rats show activation of Papez (Kulkarni et al., 2012) and habenular neural circuitry both of which play important roles in emotional experience, learning and memory of aversive information. Awake imaging has been used to test and demonstrate the efficacy of a DALDA analogue, a potent mu-opioid peptide delivered as an encapsulated nanoparticle on capsaicin-induced pain (Shah et al., 2014). Chang et al. (2017) showed the development of neuropathy was associated with changes in BOLD activity in prefrontal ctx and accumbens. With respect to awake imaging and pain it should be noted that the process of acclimating animals to the stress of restraint may affect the pain response, a point of concern that should be considered when interpreting the data (Low et al., 2016).
Social Behavior and Fear
Oxytocin and Affiliation
Oxytocin and vasopressin are two phylogenetically old neuropeptides found throughout the animal kingdom and co-opted by different species for numerous functions primarily related to social behavior (Ferris, 2008). Oxytocin has been associated with affiliation and pair bonding while vasopressin with approach/avoidance and aggression. During imaging, Febo and colleagues exposed dams to pup suckling (see Figure 3 section on Drugs of Abuse) before and after ICV administration of an oxytocin receptor antagonist while another group of dams was given ICV oxytocin alone (Febo et al., 2005b). Pup sucking and exogenous oxytocin cause similar patterns of positive BOLD activation in brain areas associated with olfaction, emotions, motivation, and reward while blockade of oxytocin receptors reduces this activation. These data add to a body of literature that oxytocin may strengthen mother–infant bond formation. In a subsequent study lactating dams were tested for fear elicited by exposure to predator scent trimethylthiazoline (TMT) the smell of fox presented during the imaging session (Febo et al., 2009b). Pretreatment with oxytocin alters the innate fear response to threat of predation, enhancing brain activity in areas associated with emotion and cognition and reducing activity in areas involved in autonomic visceromotor and skeletomotor responses.
In a study testing central vs peripheral administration of oxytocin, Dumais et al. (2017) looked for differences in brain activity in awake male and female rats. Males are more responsive to both centrally and peripherally administered oxytocin than females. Centrally administered neuropeptide activates areas associated with motivation and reward. However, peripheral oxytocin modulates neural activation differently in male and female rats. The pattern and magnitude of activation are different, but not the direction, showing a sex differences depending on the route of administration. These findings highlight the need to include both sexes in basic and clinical studies to fully understand the role of oxytocin on brain function.
Vasopressin and Aggression
There are numerous studies on the role of vasopressin as a chemical signal in the brain controlling agonistic behavior (for review see Febo and Ferris, 2014). Giving vasopressin or a selective arginine vasopressin, V1a receptor antagonist can enhance or inhibit aggressive responding, respectively (Ferris et al., 1999, 2006). Similarly, there are many studies showing that serotonin (5HT) receptor agonists and reuptake inhibitors like fluoxetine reduce aggressive behavior (e.g., Ferris et al., 1997, 1999).
Since overt aggression in a laboratory setting is measured by physical bites and attacks on a conspecific it does not lend itself to awake imaging. However, aggressive motivation, i.e., the autonomic arousal preceding overt aggression can be measured. Aggressive motivation can be elicited by putting a male intruder into the home cage of a resident male and his female partner. In this context, the resident male will attack the intruder. The intruder is forewarned of the impending attack by the piloerection of fur along the back of the resident. The piloerection is an autonomic display of a centrally organized response of aggressive motivation or intent to attack. Giving the resident male an orally active, selective V1a receptor antagonist or a 5HT reuptake inhibitor will block piloerection toward an intruder. This resident/intruder paradigm with piloerection can be staged in the magnet during the scanning session (Ferris et al., 2008). This is done with a “vivarium,” a housing unit containing bedding from the home cage along with the resident’s female partner. The resident is prepared for awake imaging and positioned in the magnet. The vivarium is then positioned in the magnet centimeters away from the resident’s head. Five minutes into data acquisition, an intruder is placed into the vivarium. Within less than a min the resident will piloerect. In this study the change in brain activity was mapped to a 3D MRI rat atlas with over 100 brain volumes. The increase and decrease in activity across numerous brain areas correlates with the piloerection and is interpreted to be the neurocircuitry of aggressive motivation. Both drugs block key nodes in the known neural circuitry of aggression.
Fear
The first study on fear in awake animals was done in the Flinders Sensitive Line (FSL), a rat model of depression. Trimethylthiazoline was used to trigger the innate fear of predation in FSL and control rats causing differences in activity in the prefrontal cortex and amygdala (Huang et al., 2011). Holmes and colleagues followed with a series of studies in rats and mice using a conditioned-fear model associating a light cue with foot shock (Brydges et al., 2013; Harris et al., 2015, 2016). This conditioned-fear response was performed during the scanning session and the change in BOLD signal mapped to fear neural circuitry. This study was repeated again but with rats having a history of early life stress. Early life stress in rats produces depression-like behavior. With this model the conditioned fear response is exacerbated as compared to controls. In a subsequent study, rats deficient in brain-derived neurotrophic factor (BDNF), a chemical signal associated with psychiatric disorders, were tested for conditioned fear during imaging (Harris et al., 2016). Interestingly these rats fail to activate fear circuitry as compared to controls showing a functional relationship between BDNF and emotional regulation. Awake imaging can also be used to image condition taste aversion. During an imaging session the sweet taste of saccharine can be associated with the nauseating effect of i.p. lithium chloride. The BOLD signal response shows activation of prelimbic ctx, insular ctx and basolateral amygdala. This neural circuitry was confirmed by connectivity studies using diffusion tensor imaging and postmortem histology and c-Fos for cellular activation (Uematsu et al., 2015). Rats can also be conditioned to associate the taste of sucrose with the single exposure of a live predator, the sable ferret, during a scanning session. This initial exposure elicits the expected activation of emotional and cognitive neural circuits. However, when reimaged two weeks later the increase in brain activity in fear circuitry in response to the taste of sucrose alone is exacerbated suggesting the memory of the initial aversive experience is worse than the experience itself (Ferris et al., 2011). A single exposure to predator odor can alters rsFC in fear circuitry. Rats exposed to the collar taken from a cat vs an unworn collar, display heightened levels of anxiety one week later and alteration in connectivity between the infralimbic ctx and amygdala (Liang et al., 2014).
Any experience associated with high stress like predatory fear engages the brain-adrenal axis and the release of glucocorticoids—stress hormones. How much of the behavioral response, conditioned or innate, is affected by adrenal steroids? It is well known that glucocorticoids can have long term effects by acting like transcription factors affecting gene activity and neural plasticity (Kim and Diamond, 2002). Can stress hormones have an immediate non-genomic effect altering the behavioral response to an aversive emotional experience? To address this question, blood levels of corticosterone were measured in rats exposed to low and high stress conditions (Ferris and Stolberg, 2010). During a scanning session, adrenalectomized rats were given i.v. injections of corticosterone that elevated blood levels to those measured in intact rats. Within the first 2 min there was a significant increase in brain activity in the neural circuitry of emotion and memory to the high stress glucocorticoid injection but not the low stress injection showing that stress hormones can have immediate non genomic effects on brain activity. A thorough review on stress and functional MRI in humans and animals is provided by Dopfel and Zhang (2018).
Novel Applications
Brain Clearance
Much has been written about the glymphatic system and clearance of metabolic waste and unwanted proteins and the confound of anesthesia (Ferris, 2021). ICV injected tracer into awake mice rapidly appears in the lymphatic system and systemic circulation (Ma et al., 2017), but is retarded in the presence of anesthesia (Ma et al., 2019). When imaging is performed during the dark phase of the mouse light-dark cycle, a time when they are most active and clearance is low, ICV tracer rapidly comes to equilibrium across the brain parenchyma under awake conditions but not under anesthesia (Gakuba et al., 2018). Cai et al. (2020) published the first study on the circadian control of perivascular clearance altering the time of image acquisition between the light and dark phases of a 24 h cycle. ICV injection of MRI contrast agent in awake rats effectively moves throughout the brain parenchyma during the dark phase but not so during light when rats are at rest or sleeping. This pattern of trace accumulation indicates greater clearance during light phase than the dark phase. Mild repetitive head injury without evidence of brain damage alters perivascular clearance in the midbrain dopaminergic system weeks after insult. This was shown by awake imaging of MRI contrast agent in rats during the light phase of the circadian cycle (Cai et al., 2021). Perivascular clearance of ferumoxytol an iron oxide MRI contrast agent infused ICV in awake rats during the circadian light phase appears within minutes in the subarachnoid space, nasal cavity, nasal pharynx, and soft palate at the back of the throat (Figure 5; Leaston et al., 2021). Fluorescence quantum dots infused ICV again appear in the esophagus within minutes of injection suggesting unwanted waste from the brain may be cleared through a brain-to-gut pathway in addition to the meningeal and nasal lymphatics.
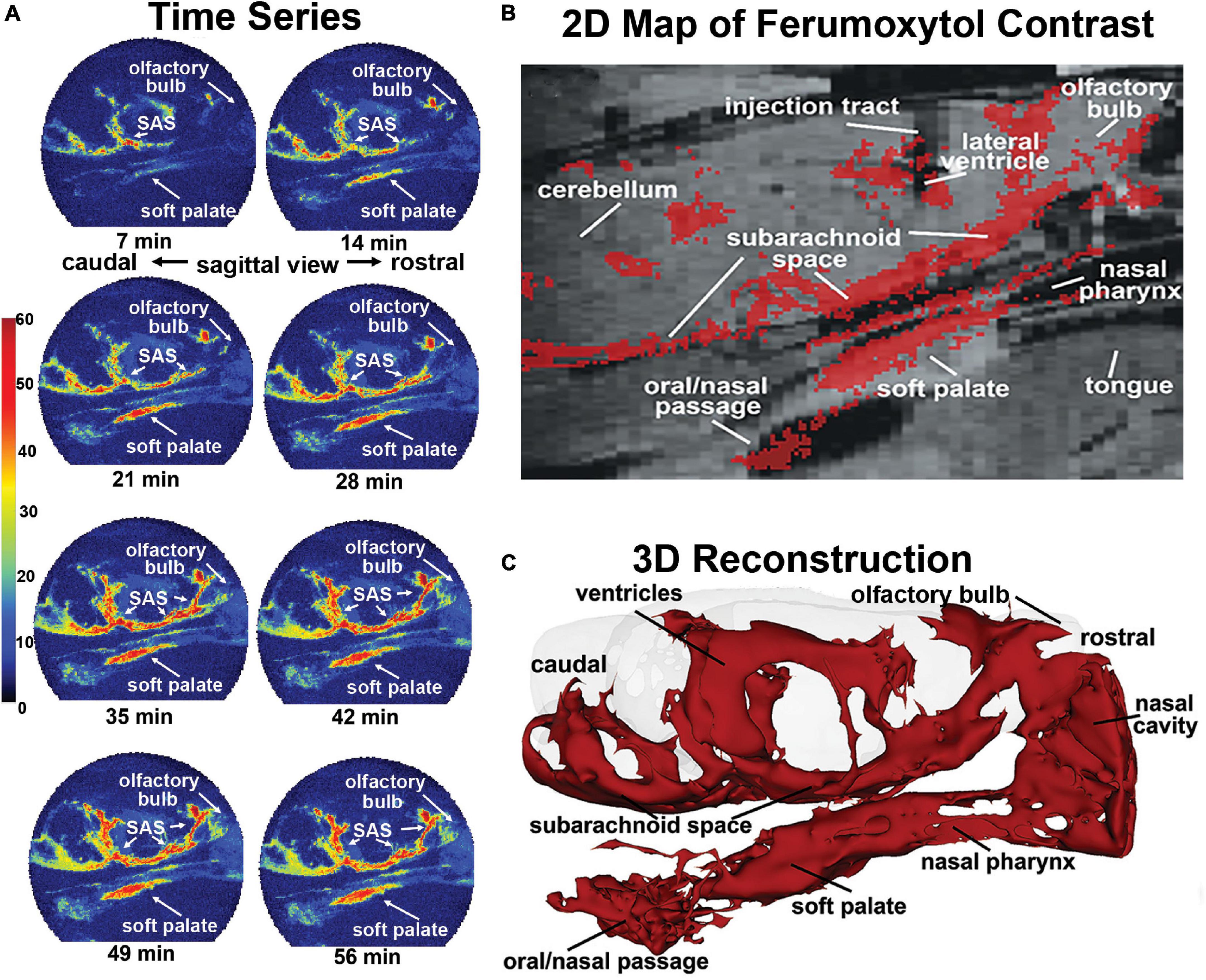
Figure 5. Mapping clearance from brain to soft palate. Shown is the movement and accumulation of ferumoxytol. The time series (A) are from a single subject, collected at 7-min intervals over 70 min long scanning session. A summary image (B) of the average CA signal from six rats is mapped onto the T2 weighted anatomy image of the brain. (C) The 3D reconstruction of CA within and outside the brain from a single subject at the end of the imaging study. (Adapted with permission from Leaston et al., 2021).
Summary
Imaging awake animals using MRI will only improve as we move forward based on better acclimation protocols and restraining systems that eliminate all surgical preparations, ear bars, and other points of irritation. Better hardware and radiofrequency electronics will optimize space filling and SNR for complete brain coverage and the highest image quality. Image registration, data analysis and data interpretation will become automated assisted by artificial intelligence. All of these improvements should be standardized.
I have done my best to find and summarize all of the applications and publications on awake MRI in mice, rats, and marmoset monkeys. These animals are the most commonly used species for medical research, involving small bore high field MRI systems. While marmosets have the greatest translational value they have become very expensive limiting their access to most researchers. However, marmoset will play a significant role going forward in visual and social neuroscience and aging research. Indeed, marmosets are short-lived and offer many advantages over other non-human primates both in and out of the magnet (Tardif et al., 2011). The future will likely focus on rodents, and then more on rats than mice. Rats are phylogenetically closer to humans than mice (Gibbs et al., 2004) and are favored for neuroscience research (Iannaccone and Jacob, 2009; Ellenbroek and Youn, 2016) not to mention they have bigger brains which is better for MRI. PhMRI studies will continue to characterize new therapeutics not only for their ability to get into the brain and engage with their target but also for their potential liability in the areas of addiction and self-harm. In this area there should be more collaboration between pharmaceutical companies and academic labs. Other than epilepsy and Parkinson’s there are very few studies on awake functional MRI in animal models of neurological disorders. This can only improve as more transgenic models of neurodegenerative disease are generated in rats. The study of drug addiction is a key future area of research. The ability to expose a drug naïve brain to a known or potential addictive compound and record the acute and neuroadaptive effects to repeated exposer across multiple integrated neural circuits is an underdeveloped application. What are the functional changes in the brain that occur with drug addiction? Indeed, these types of prospective longitudinal studies on the same animal opens up new areas of research on changes in brain function in models of cancer, diabetes, cardiovascular disease, neurotrauma and the biggest disease of all – aging.
Author Contributions
CF was the sole author and responsible for the content of this review.
Conflict of Interest
The author has a financial interest in Animal Imaging Research, the company that makes the radiofrequency electronics and holders for awake animal imaging. The author also has a partnership interest in Ekam Solutions the company that develops 3D MRI atlases for animal research.
Publisher’s Note
All claims expressed in this article are solely those of the authors and do not necessarily represent those of their affiliated organizations, or those of the publisher, the editors and the reviewers. Any product that may be evaluated in this article, or claim that may be made by its manufacturer, is not guaranteed or endorsed by the publisher.
Acknowledgments
I want to thank my colleagues Jean King, Reinhold Ludwig, John Sullivan, Marcelo Febo, Praveen Kulkarni, Charles Snowden, Nancy Shultz Darken, Toni Ziegler, and Steven Toddes for their significant contributions to the field of awake animal MRI and Febo and Dan Madularu for their help in editing this review.
References
Almeida, J., Severo, F., and Nunes, D. (2021). Impact of the sound of magnetic resonance imaging pulse sequences in awake mice. J. Appl. Anim. Welf Sci. 25, 75–88. doi: 10.1080/10888705.2021.1941023
Andersen, A. H., Hardy, P. A., Forman, E., Gerhardt, G. A., Gash, D. M., Grondin, R. C., et al. (2015). Pharmacologic MRI (phMRI) as a tool to differentiate Parkinson’s disease-related from age-related changes in basal ganglia function. Neurobiol. Aging 36, 1174–1182. doi: 10.1016/j.neurobiolaging.2014.10.014
Andics, A., Gabor, A., Gacsi, M., Farago, T., Szabo, D., and Miklosi, A. (2016). Neural mechanisms for lexical processing in dogs. Science 353, 1030–1032. doi: 10.1126/science.aaf3777
Andics, A., Gacsi, M., Farago, T., Kis, A., and Miklosi, A. (2014). Voice-sensitive regions in the dog and human brain are revealed by comparative fMRI. Curr. Biol. 24, 574–578. doi: 10.1016/j.cub.2014.01.058
Baker, S., Chin, C. L., Basso, A. M., Fox, G. B., Marek, G. J., and Day, M. (2012). Xanomeline modulation of the blood oxygenation level-dependent signal in awake rats: development of pharmacological magnetic resonance imaging as a translatable pharmacodynamic biomarker for central activity and dose selection. J. Pharmacol. Exp. Ther. 341, 263–273. doi: 10.1124/jpet.111.188797
Becerra, L., Bishop, J., Barmettler, G., Kainz, V., Burstein, R., and Borsook, D. (2017). Brain network alterations in the inflammatory soup animal model of migraine. Brain Res. 1660, 36–46. doi: 10.1016/j.brainres.2017.02.001
Becerra, L., Pendse, G., Chang, P. C., Bishop, J., and Borsook, D. (2011b). Robust reproducible resting state networks in the awake rodent brain. PLoS One 6:e25701. doi: 10.1371/journal.pone.0025701
Becerra, L., Chang, P. C., Bishop, J., and Borsook, D. (2011a). CNS activation maps in awake rats exposed to thermal stimuli to the dorsum of the hindpaw. Neuroimage 54, 1355–1366. doi: 10.1016/j.neuroimage.2010.08.056
Becerra, L., Upadhyay, J., Chang, P. C., Bishop, J., Anderson, J., Baumgartner, R., et al. (2013). Parallel buprenorphine phMRI responses in conscious rodents and healthy human subjects. J. Pharmacol. Exp. Ther. 345, 41–51. doi: 10.1124/jpet.112.201145
Becker, J. B. (1999). Gender differences in dopaminergic function in striatum and nucleus accumbens. Pharmacol. Biochem. Behav. 64, 803–812. doi: 10.1016/s0091-3057(99)00168-9
Behroozi, M., Helluy, X., Strockens, F., Gao, M., Pusch, R., Tabrik, S., et al. (2020). Event-related functional MRI of awake behaving pigeons at 7T. Nat. Commun. 11:4715. doi: 10.1038/s41467-020-18437-1
Belcher, A. M., Yen, C. C., Stepp, H., Gu, H., Lu, H., Yang, Y., et al. (2013). Large-scale brain networks in the awake, truly resting marmoset monkey. J. Neurosci. 33, 16796–16804. doi: 10.1523/JNEUROSCI.3146-13.2013
Bergmann, E., Gofman, X., Kavushansky, A., and Kahn, I. (2020). Individual variability in functional connectivity architecture of the mouse brain. Commun. Biol. 3:738. doi: 10.1038/s42003-020-01472-5
Berns, G. S., Brooks, A. M., and Spivak, M. (2012). Functional MRI in awake unrestrained dogs. PLoS One 7:e38027. doi: 10.1371/journal.pone.0038027
Bishop, J., Becerra, L., Barmettler, G., Chang, P. C., Kainz, V., Burstein, R., et al. (2019). Modulation of brain networks by sumatriptan-naproxen in the inflammatory soup migraine model. Pain 160, 2161–2171. doi: 10.1097/j.pain.0000000000001583
Biswal, B., Yetkin, F. Z., Haughton, V. M., and Hyde, J. S. (1995). Functional connectivity in the motor cortex of resting human brain using echo-planar MRI. Magn. Reson. Med. 34, 537–541. doi: 10.1002/mrm.1910340409
Bozza, T., Feinstein, P., Zheng, C., and Mombaerts, P. (2002). Odorant receptor expression defines functional units in the mouse olfactory system. J. Neurosci. 22, 3033–3043. doi: 10.1523/JNEUROSCI.22-08-03033.2002
Brevard, M. E., Meyer, J. S., Harder, J. A., and Ferris, C. F. (2006b). Imaging brain activity in conscious monkeys following oral MDMA (”ecstasy”). Magn. Reson. Imaging 24, 707–714. doi: 10.1016/j.mri.2006.03.010
Brevard, M. E., Kulkarni, P., King, J. A., and Ferris, C. F. (2006a). Imaging the neural substrates involved in the genesis of pentylenetetrazol-induced seizures. Epilepsia 47, 745–754. doi: 10.1111/j.1528-1167.2006.00502.x
Brydges, N. M., Whalley, H. C., Jansen, M. A., Merrifield, G. D., Wood, E. R., Lawrie, S. M., et al. (2013). Imaging conditioned fear circuitry using awake rodent fMRI. PLoS One 8:e54197. doi: 10.1371/journal.pone.0054197
Brynildsen, J. K., Hsu, L. M., Ross, T. J., Stein, E. A., Yang, Y., and Lu, H. (2017). Physiological characterization of a robust survival rodent fMRI method. Magn. Reson. Imaging 35, 54–60. doi: 10.1016/j.mri.2016.08.010
Caffrey, M. K., and Febo, M. (2014). Cocaine-associated odor cue re-exposure increases blood oxygenation level dependent signal in memory and reward regions of the maternal rat brain. Drug Alcohol Depend. 134, 167–177. doi: 10.1016/j.drugalcdep.2013.09.032
Cai, X., Harding, I. C., Sadaka, A. H., Colarusso, B., Kulkarni, P., Ebong, E., et al. (2021). Mild repetitive head impacts alter perivascular flow in the midbrain dopaminergic system in awake rats. Brain Commun. 3:fcab265. doi: 10.1093/braincomms/fcab265
Cai, X., Qiao, J., Kulkarni, P., Harding, I. C., Ebong, E., and Ferris, C. F. (2020). Imaging the effect of the circadian light-dark cycle on the glymphatic system in awake rats. Proc. Natl. Acad. Sci. U.S.A. 117, 668–676. doi: 10.1073/pnas.1914017117
Carter, C. S., Kenkel, W. M., MacLean, E. L., Wilson, S. R., Perkeybile, A. M., Yee, J. R., et al. (2020). Is oxytocin “nature’s medicine”? Pharmacol. Rev. 72, 829–861. doi: 10.1124/pr.120.019398
Chang, J. B., Quinnies, K. M., Realubit, R., Karan, C., Rand, J. H., and Tatonetti, N. P. (2016). A novel, rapid method to compare the therapeutic windows of oral anticoagulants using the Hill coefficient. Sci. Rep. 6:29387. doi: 10.1038/srep29387
Chang, P. C., Centeno, M. V., Procissi, D., Baria, A., and Apkarian, A. V. (2017). Brain activity for tactile allodynia: a longitudinal awake rat functional magnetic resonance imaging study tracking emergence of neuropathic pain. Pain 158, 488–497. doi: 10.1097/j.pain.0000000000000788
Chang, P. C., Procissi, D., Bao, Q., Centeno, M. V., Baria, A., and Apkarian, A. V. (2016). Novel method for functional brain imaging in awake minimally restrained rats. J. Neurophysiol. 116, 61–80. doi: 10.1152/jn.01078.2015
Chen, X., Tong, C., Han, Z., Zhang, K., Bo, B., Feng, Y., et al. (2020). Sensory evoked fMRI paradigms in awake mice. Neuroimage 204:116242. doi: 10.1016/j.neuroimage.2019.116242
Chin, C. L., Fox, G. B., Hradil, V. P., Osinski, M. A., McGaraughty, S. P., Skoubis, P. D., et al. (2006). Pharmacological MRI in awake rats reveals neural activity in area postrema and nucleus tractus solitarius: relevance as a potential biomarker for detecting drug-induced emesis. Neuroimage 33, 1152–1160. doi: 10.1016/j.neuroimage.2006.06.059
Chin, C. L., Tovcimak, A. E., Hradil, V. P., Seifert, T. R., Hollingsworth, P. R., Chandran, P., et al. (2008). Differential effects of cannabinoid receptor agonists on regional brain activity using pharmacological MRI. Br. J. Pharmacol. 153, 367–379. doi: 10.1038/sj.bjp.0707506
Chin, C. L., Upadhyay, J., Marek, G. J., Baker, S. J., Zhang, M., Mezler, M., et al. (2011). Awake rat pharmacological magnetic resonance imaging as a translational pharmacodynamic biomarker: metabotropic glutamate 2/3 agonist modulation of ketamine-induced blood oxygenation level dependence signals. J. Pharmacol. Exp. Ther 336, 709–715. doi: 10.1124/jpet.110.173880
Clery, J. C., Hori, Y., Schaeffer, D. J., Gati, J. S., Pruszynski, J. A., and Everling, S. (2020a). Whole brain mapping of somatosensory responses in awake marmosets investigated with ultra-high-field fMRI. J. Neurophysiol. 124, 1900–1913. doi: 10.1152/jn.00480.2020
Clery, J. C., Schaeffer, D. J., Hori, Y., Gilbert, K. M., Hayrynen, L. K., Gati, J. S., et al. (2020b). Looming and receding visual networks in awake marmosets investigated with fMRI. Neuroimage 215:116815. doi: 10.1016/j.neuroimage.2020.116815
Cuaya, L. V., Hernandez-Perez, R., and Concha, L. (2016). Our faces in the Dog’s brain: functional imaging reveals temporal cortex activation during perception of human faces. PLoS One 11:e0149431. doi: 10.1371/journal.pone.0149431
Derksen, M., Rhemrev, V., van der Veer, M., Jolink, L., Zuidinga, B., Mulder, T., et al. (2021). Animal studies in clinical MRI scanners: a custom setup for combined fMRI and deep-brain stimulation in awake rats. J. Neurosci. Methods 360:109240. doi: 10.1016/j.jneumeth.2021.109240
Desai, M., Kahn, I., Knoblich, U., Bernstein, J., Atallah, H., Yang, A., et al. (2011). Mapping brain networks in awake mice using combined optical neural control and fMRI. J. Neurophysiol. 105, 1393–1405. doi: 10.1152/jn.00828.2010
Dinh, T. N. A., Jung, W. B., Shim, H. J., and Kim, S. G. (2021). Characteristics of fMRI responses to visual stimulation in anesthetized vs. awake mice. Neuroimage 226:117542. doi: 10.1016/j.neuroimage.2020.117542
Dopfel, D., and Zhang, N. (2018). Mapping stress networks using functional magnetic resonance imaging in awake animals. Neurobiol. Stress 9, 251–263. doi: 10.1016/j.ynstr.2018.06.002
Dubowitz, D. J., Chen, D. Y., Atkinson, D. J., Grieve, K. L., Gillikin, B., and Bradley, W. G. Jr., et al. (1998). Functional magnetic resonance imaging in macaque cortex. Neuroreport 9, 2213–2218. doi: 10.1097/00001756-199807130-00012
Dumais, K. M., Kulkarni, P. P., Ferris, C. F., and Veenema, A. H. (2017). Sex differences in neural activation following different routes of oxytocin administration in awake adult rats. Psychoneuroendocrinology 81, 52–62. doi: 10.1016/j.psyneuen.2017.04.003
Ellenbroek, B., and Youn, J. (2016). Rodent models in neuroscience research: is it a rat race? Dis. Model. Mech. 9, 1079–1087. doi: 10.1242/dmm.026120
Farra, Y. M., Eden, M. J., Coleman, J. R., Kulkarni, P., Ferris, C. F., Oakes, J. M., et al. (2020). Acute neuroradiological, behavioral, and physiological effects of nose-only exposure to vaporized cannabis in C57BL/6 mice. Inhal. Toxicol. 32, 200–217. doi: 10.1080/08958378.2020.1767237
Febo, M. (2011). Technical and conceptual considerations for performing and interpreting functional MRI studies in awake rats. Front. Psychiatry 2:43. doi: 10.3389/fpsyt.2011.00043
Febo, M., Akbarian, S., Schroeder, F. A., and Ferris, C. F. (2009a). Cocaine-induced metabolic activation in cortico-limbic circuitry is increased after exposure to the histone deacetylase inhibitor, sodium butyrate. Neurosci. Lett. 465, 267–271. doi: 10.1016/j.neulet.2009.07.065
Febo, M., Shields, J., Ferris, C. F., and King, J. A. (2009b). Oxytocin modulates unconditioned fear response in lactating dams: an fMRI study. Brain Res. 1302, 183–193. doi: 10.1016/j.brainres.2009.09.043
Febo, M., and Ferris, C. F. (2007). Development of cocaine sensitization before pregnancy affects subsequent maternal retrieval of pups and prefrontal cortical activity during nursing. Neuroscience 148, 400–412. doi: 10.1016/j.neuroscience.2007.05.026
Febo, M., and Ferris, C. F. (2014). Oxytocin and vasopressin modulation of the neural correlates of motivation and emotion: results from functional MRI studies in awake rats. Brain Res. 1580, 8–21. doi: 10.1016/j.brainres.2014.01.019
Febo, M., Segarra, A. C., Nair, G., Schmidt, K., Duong, T. Q., and Ferris, C. F. (2005c). The neural consequences of repeated cocaine exposure revealed by functional MRI in awake rats. Neuropsychopharmacology 30, 936–943. doi: 10.1038/sj.npp.1300653
Febo, M., Ferris, C. F., and Segarra, A. C. (2005a). Estrogen influences cocaine-induced blood oxygen level-dependent signal changes in female rats. J. Neurosci. 25, 1132–1136. doi: 10.1523/JNEUROSCI.3801-04.2005
Febo, M., Numan, M., and Ferris, C. F. (2005b). Functional magnetic resonance imaging shows oxytocin activates brain regions associated with mother-pup bonding during suckling. J. Neurosci. 25, 11637–11644. doi: 10.1523/JNEUROSCI.3604-05.2005
Febo, M., Segarra, A. C., Stolberg, T. L., and Ferris, C. F. (2011). BOLD signal response to cocaine varies with sexual receptivity in female rats. Neuroreport 22, 19–22. doi: 10.1097/WNR.0b013e3283416f81
Febo, M., Segarra, A. C., Tenney, J. R., Brevard, M. E., Duong, T. Q., and Ferris, C. F. (2004). Imaging cocaine-induced changes in the mesocorticolimbic dopaminergic system of conscious rats. J. Neurosci. Methods 139, 167–176. doi: 10.1016/j.jneumeth.2004.04.028
Febo, M., Stolberg, T. L., Numan, M., Bridges, R. S., Kulkarni, P., and Ferris, C. F. (2008). Nursing stimulation is more than tactile sensation: it is a multisensory experience. Horm. Behav. 54, 330–339. doi: 10.1016/j.yhbeh.2008.02.024
Ferris, C., and Tenney, J. (2013). “Functional magnetic resonance imaging in epilepsy: methods and applications using awake animals,” in Neuronal Networks in Brain Function, CNS Disorders, and Therapeutics, eds C. Faingold and H. Blumenfeld (San Diego, CA: Elsevier Press).
Ferris, C. F. (2008). Functional magnetic resonance imaging and the neurobiology of vasopressin and oxytocin. Prog. Brain Res. 170, 305–320. doi: 10.1016/S0079-6123(08)00425-1
Ferris, C. F. (2021). Rethinking the conditions and mechanism for glymphatic clearance. Front. Neurosci. 15:624690. doi: 10.3389/fnins.2021.624690
Ferris, C. F., Kulkarni, P., Sullivan, J. M. Jr., Harder, J. A., Messenger, T. L., and Febo, M. (2005). Pup suckling is more rewarding than cocaine: evidence from functional magnetic resonance imaging and three-dimensional computational analysis. J. Neurosci. 25, 149–156. doi: 10.1523/JNEUROSCI.3156-04.2005
Ferris, C. F., Kulkarni, P., Toddes, S., Yee, J., Kenkel, W., and Nedelman, M. (2014). Studies on the Q175 knock-in model of Huntington’s disease using functional imaging in awake mice: evidence of olfactory dysfunction. Front. Neurol. 5:94. doi: 10.3389/fneur.2014.00094
Ferris, C. F., Lu, S. F., Messenger, T., Guillon, C. D., Heindel, N., Miller, M., et al. (2006). Orally active vasopressin V1a receptor antagonist, SRX251, selectively blocks aggressive behavior. Pharmacol. Biochem. Behav. 83, 169–174. doi: 10.1016/j.pbb.2006.01.001
Ferris, C. F., Melloni, R. H. Jr., Koppel, G., Perry, K. W., Fuller, R. W., and Delville, Y. (1997). Vasopressin/serotonin interactions in the anterior hypothalamus control aggressive behavior in golden hamsters. J. Neurosci. 17, 4331–4340. doi: 10.1523/JNEUROSCI.17-11-04331.1997
Ferris, C. F., Smerkers, B., Kulkarni, P., Caffrey, M., Afacan, O., Toddes, S., et al. (2011). Functional magnetic resonance imaging in awake animals. Rev. Neurosci. 22, 665–674.
Ferris, C. F., and Snowdon, C. T. (2005). “Functional magnetic resonance imaging in conscious marmoset monkeys: methods and applications in neuroscience research,” in The Laboratory Primate: Handbook of Experimental Animals, ed. S. Wolfe-Coate (Amsterdam: Elsevier Science), 353–359. doi: 10.1016/b978-012080261-6/50021-0
Ferris, C. F., Snowdon, C. T., King, J. A., Duong, T. Q., Ziegler, T. E., Ugurbil, K., et al. (2001). Functional imaging of brain activity in conscious monkeys responding to sexually arousing cues. Neuroreport 12, 2231–2236. doi: 10.1097/00001756-200107200-00037
Ferris, C. F., Snowdon, C. T., King, J. A., Sullivan, J. M. Jr., Ziegler, T. E., Olson, D. P., et al. (2004). Activation of neural pathways associated with sexual arousal in non-human primates. J. Magn. Reson. Imaging 19, 168–175. doi: 10.1002/jmri.10456
Ferris, C. F., and Stolberg, T. (2010). Imaging the immediate non-genomic effects of stress hormone on brain activity. Psychoneuroendocrinology 35, 5–14. doi: 10.1016/j.psyneuen.2009.09.003
Ferris, C. F., Stolberg, T., and Delville, Y. (1999). Serotonin regulation of aggressive behavior in male golden hamsters (Mesocricetus auratus). Behav. Neurosci. 113, 804–815. doi: 10.1037//0735-7044.113.4.804
Ferris, C. F., Stolberg, T., Kulkarni, P., Murugavel, M., Blanchard, R., Blanchard, D. C., et al. (2008). Imaging the neural circuitry and chemical control of aggressive motivation. BMC Neurosci 9:111. doi: 10.1186/1471-2202-9-111
Ferris, C. F., Yee, J. R., Kenkel, W. M., Dumais, K. M., Moore, K., Veenema, A. H., et al. (2015). Distinct BOLD activation profiles following central and peripheral oxytocin administration in awake rats. Front. Behav. Neurosci. 9:245. doi: 10.3389/fnbeh.2015.00245
Gakuba, C., Gaberel, T., Goursaud, S., Bourges, J., Di Palma, C., Quenault, A., et al. (2018). General anesthesia inhibits the activity of the “glymphatic system”. Theranostics 8, 710–722. doi: 10.7150/thno.19154
Gao, Y. R., Ma, Y., Zhang, Q., Winder, A. T., Liang, Z., Antinori, L., et al. (2017). Time to wake up: studying neurovascular coupling and brain-wide circuit function in the un-anesthetized animal. Neuroimage 153, 382–398. doi: 10.1016/j.neuroimage.2016.11.069
Gibbs, R. A., Weinstock, G. M., Metzker, M. L., Muzny, D. M., Sodergren, E. J., Scherer, S., et al. (2004). Genome sequence of the Brown Norway rat yields insights into mammalian evolution. Nature 428, 493–521. doi: 10.1038/nature02426
Gilbert, K. M., Clery, J. C., Gati, J. S., Hori, Y., Johnston, K. D., Mashkovtsev, A., et al. (2021). Simultaneous functional MRI of two awake marmosets. Nat. Commun. 12:6608. doi: 10.1038/s41467-021-26976-4
Goense, J. B., Whittingstall, K., and Logothetis, N. K. (2010). Functional magnetic resonance imaging of awake behaving macaques. Methods 50, 178–188. doi: 10.1016/j.ymeth.2009.08.003
Grandjean, J., Schroeter, A., Batata, I., and Rudin, M. (2014). Optimization of anesthesia protocol for resting-state fMRI in mice based on differential effects of anesthetics on functional connectivity patterns. Neuroimage 102(Pt 2), 838–847. doi: 10.1016/j.neuroimage.2014.08.043
Han, Z., Chen, W., Chen, X., Zhang, K., Tong, C., Zhang, X., et al. (2019). Awake and behaving mouse fMRI during Go/No-Go task. Neuroimage 188, 733–742. doi: 10.1016/j.neuroimage.2019.01.002
Harris, A. P., Lennen, R. J., Brydges, N. M., Jansen, M. A., Pernet, C. R., Whalley, H. C., et al. (2016). The role of brain-derived neurotrophic factor in learned fear processing: an awake rat fMRI study. Genes Brain Behav. 15, 221–230. doi: 10.1111/gbb.12277
Harris, A. P., Lennen, R. J., Marshall, I., Jansen, M. A., Pernet, C. R., Brydges, N. M., et al. (2015). Imaging learned fear circuitry in awake mice using fMRI. Eur. J. Neurosci. 42, 2125–2134. doi: 10.1111/ejn.12939
Hennel, F. (2001). Fast spin echo and fast gradient echo MRI with low acoustic noise. J. Magn. Reson. Imaging 13, 960–966. doi: 10.1002/jmri.1138
Hori, Y., Clery, J. C., Selvanayagam, J., Schaeffer, D. J., Johnston, K. D., Menon, R. S., et al. (2021). Interspecies activation correlations reveal functional correspondences between marmoset and human brain areas. Proc. Natl. Acad. Sci. U.S.A. 118:e2110980118. doi: 10.1073/pnas.2110980118
Huang, W., Heffernan, M. E., Li, Z., Zhang, N., Overstreet, D. H., and King, J. A. (2011). Fear induced neuronal alterations in a genetic model of depression: an fMRI study on awake animals. Neurosci. Lett. 489, 74–78. doi: 10.1016/j.neulet.2010.11.069
Huber, L., and Lamm, C. (2017). Understanding dog cognition by functional magnetic resonance imaging. Learn. Behav. 45, 101–102. doi: 10.3758/s13420-017-0261-6
Hung, C. C., Yen, C. C., Ciuchta, J. L., Papoti, D., Bock, N. A., Leopold, D. A., et al. (2015b). Functional MRI of visual responses in the awake, behaving marmoset. NeuroImage 120, 1–11. doi: 10.1016/j.neuroimage.2015.06.090
Hung, C. C., Yen, C. C., Ciuchta, J. L., Papoti, D., Bock, N. A., Leopold, D. A., et al. (2015a). Functional mapping of face-selective regions in the extrastriate visual cortex of the marmoset. J. Neurosci. 35, 1160–1172. doi: 10.1523/JNEUROSCI.2659-14.2015
Iriah, S. C., Trivedi, M., Kenkel, W., Grant, S. E., Moore, K., Yee, J. R., et al. (2019). Oxycodone exposure: a magnetic resonance imaging study in response to acute and chronic oxycodone treatment in rats. Neuroscience 398, 88–101. doi: 10.1016/j.neuroscience.2018.11.042
Jia, Z., Chen, X., Tang, W., Zhao, D., and Yu, S. (2019). Atypical functional connectivity between the anterior cingulate cortex and other brain regions in a rat model of recurrent headache. Mol. Pain 15:1744806919842483. doi: 10.1177/1744806919842483
Jia, Z., Tang, W., Zhao, D., and Yu, S. (2017). Disrupted functional connectivity between the periaqueductal gray and other brain regions in a rat model of recurrent headache. Sci. Rep. 7:3960. doi: 10.1038/s41598-017-04060-6
Johnson, T. R., Smerkers, B., Moulder, J. K., Stellar, J. R., and Febo, M. (2013). Neural processing of a cocaine-associated odor cue revealed by functional MRI in awake rats. Neurosci. Lett. 534, 160–165. doi: 10.1016/j.neulet.2012.11.054
Karl, S., Boch, M., Zamansky, A., van der Linden, D., Wagner, I. C., Volter, C. J., et al. (2020). Exploring the dog-human relationship by combining fMRI, eye-tracking and behavioural measures. Sci. Rep. 10:22273. doi: 10.1038/s41598-020-79247-5
Karl, S., Sladky, R., Lamm, C., and Huber, L. (2021). Neural responses of pet dogs witnessing their caregiver’s positive interactions with a conspecific: an fMRI study. Cereb. Cortex Commun. 2:tgab047. doi: 10.1093/texcom/tgab047
Kashiwagi, Y., Rokugawa, T., Yamada, T., Obata, A., Watabe, H., Yoshioka, Y., et al. (2015). Pharmacological MRI response to a selective dopamine transporter inhibitor, GBR12909, in awake and anesthetized rats. Synapse 69, 203–212. doi: 10.1002/syn.21803
Katsunuma, A., Takamori, H., Sakakura, Y., Hamamura, Y., Ogo, Y., and Katayama, R. (2002). Quiet MRI with novel acoustic noise reduction. MAGMA 13, 139–144. doi: 10.1007/BF02678588
Kaufman, M. J., Janes, A. C., Frederick, B., Brimson-Theberge, M., Tong, Y., McWilliams, S. B., et al. (2013). A method for conducting functional MRI studies in alert nonhuman primates: initial results with opioid agonists in male cynomolgus monkeys. Exp. Clin. Psychopharmacol. 21, 323–331. doi: 10.1037/a0033062
Kenkel, W. M., Yee, J. R., Moore, K., Madularu, D., Kulkarni, P., Gamber, K., et al. (2016). Functional magnetic resonance imaging in awake transgenic fragile X rats: evidence of dysregulation in reward processing in the mesolimbic/habenular neural circuit. Transl. Psychiatry 6:e763. doi: 10.1038/tp.2016.15
Kim, J. J., and Diamond, D. M. (2002). The stressed hippocampus, synaptic plasticity and lost memories. Nat. Rev. Neurosci. 3, 453–462. doi: 10.1038/nrn849
Kim, S. G., and Ogawa, S. (2012). Biophysical and physiological origins of blood oxygenation level-dependent fMRI signals. J. Cereb. Blood Flow Metab. 32, 1188–1206. doi: 10.1038/jcbfm.2012.23
King, J., Huang, W., Chen, W., Heffernan, M., Shields, J., Rane, P., et al. (2011). A comparison of brain and behavioral effects of varenicline and nicotine in rats. Behav. Brain Res. 223, 42–47. doi: 10.1016/j.bbr.2011.04.012
King, J. A., Garelick, T. S., Brevard, M. E., Chen, W., Messenger, T. L., Duong, T. Q., et al. (2005). Procedure for minimizing stress for fMRI studies in conscious rats. J. Neurosci. Methods 148, 154–160. doi: 10.1016/j.jneumeth.2005.04.011
Kint, L. T., Seewoo, B. J., Hyndman, T. H., Clarke, M. W., Edwards, S. H., Rodger, J., et al. (2020). The pharmacokinetics of medetomidine administered subcutaneously during isoflurane anaesthesia in sprague-dawley rats. Animals (Basel) 10:1050. doi: 10.3390/ani10061050
Kulkarni, P., Stolberg, T., Sullivanjr, J. M., and Ferris, C. F. (2012). Imaging evolutionarily conserved neural networks: preferential activation of the olfactory system by food-related odor. Behav. Brain Res. 230, 201–207. doi: 10.1016/j.bbr.2012.02.002
Lahti, K. M., Ferris, C. F., Li, F., Sotak, C. H., and King, J. A. (1999). Comparison of evoked cortical activity in conscious and propofol-anesthetized rats using functional MRI. Magn. Reson. Med. 41, 412–416. doi: 10.1002/(sici)1522-2594(199902)41:2<412::aid-mrm28>3.0.co;2-3
Leaston, J., Kulkarni, P., Gharagouzloo, C., Qiao, J., Bens, N., and Ferris, C. F. (2021). Do we swallow the waste from our brain? Front. Neurosci. 15:763780. doi: 10.3389/fnins.2021.763780
Li, Z., DiFranza, J. R., Wellman, R. J., Kulkarni, P., and King, J. A. (2008). Imaging brain activation in nicotine-sensitized rats. Brain Res. 1199, 91–99. doi: 10.1016/j.brainres.2008.01.016
Liang, Z., King, J., and Zhang, N. (2011). Uncovering intrinsic connectional architecture of functional networks in awake rat brain. J. Neurosci. 31, 3776–3783. doi: 10.1523/JNEUROSCI.4557-10.2011
Liang, Z., King, J., and Zhang, N. (2014). Neuroplasticity to a single-episode traumatic stress revealed by resting-state fMRI in awake rats. Neuroimage 103, 485–491. doi: 10.1016/j.neuroimage.2014.08.050
Liu, C., Yen, C. C., Szczupak, D., Ye, F. Q., Leopold, D. A., and Silva, A. C. (2019). Anatomical and functional investigation of the marmoset default mode network. Nat. Commun. 10:1975. doi: 10.1038/s41467-019-09813-7
Low, L. A., Bauer, L. C., Pitcher, M. H., and Bushnell, M. C. (2016). Restraint training for awake functional brain scanning of rodents can cause long-lasting changes in pain and stress responses. Pain 157, 1761–1772. doi: 10.1097/j.pain.0000000000000579
Luo, F., Li, Z., Treistman, S. N., Kim, Y. R., King, J. A., Fox, G. B., et al. (2007). Confounding effects of volatile anesthesia on CBV assessment in rodent forebrain following ethanol challenge. J. Magn. Reson. Imaging 26, 557–563. doi: 10.1002/jmri.21083
Ma, M., Qian, C., Li, Y., Zuo, Z., and Liu, Z. (2013). Setup and data analysis for functional magnetic resonance imaging of awake cat visual cortex. Neurosci. Bull. 29, 588–602. doi: 10.1007/s12264-013-1349-4
Ma, Q., Ineichen, B. V., Detmar, M., and Proulx, S. T. (2017). Outflow of cerebrospinal fluid is predominantly through lymphatic vessels and is reduced in aged mice. Nat. Commun. 8:1434. doi: 10.1038/s41467-017-01484-6
Ma, Q., Ries, M., Decker, Y., Muller, A., Riner, C., Bucker, A., et al. (2019). Rapid lymphatic efflux limits cerebrospinal fluid flow to the brain. Acta Neuropathol. 137, 151–165. doi: 10.1007/s00401-018-1916-x
Madularu, D., Yee, J. R., Kenkel, W. M., Moore, K. A., Kulkarni, P., Shams, W. M., et al. (2015b). Integration of neural networks activated by amphetamine in females with different estrogen levels: a functional imaging study in awake rats. Psychoneuroendocrinology 56, 200–212. doi: 10.1016/j.psyneuen.2015.02.022
Madularu, D., Kulkarni, P., Ferris, C. F., and Brake, W. G. (2015a). Changes in brain volume in response to estradiol levels, amphetamine sensitization and haloperidol treatment in awake female rats. Brain Res. 1618, 100–110. doi: 10.1016/j.brainres.2015.05.025
Madularu, D., Kulkarni, P., Yee, J. R., Kenkel, W. M., Shams, W. M., Ferris, C. F., et al. (2016). High estrogen and chronic haloperidol lead to greater amphetamine-induced BOLD activation in awake, amphetamine-sensitized female rats. Horm. Behav. 82, 56–63. doi: 10.1016/j.yhbeh.2016.04.007
Madularu, D., Mathieu, A. P., Kumaragamage, C., Reynolds, L. M., Near, J., Flores, C., et al. (2017b). A non-invasive restraining system for awake mouse imaging. J. Neurosci. Methods 287, 53–57. doi: 10.1016/j.jneumeth.2017.06.008
Madularu, D., Kumaragamage, C., Mathieu, A. P., Kulkarni, P., Rajah, M. N., Gratton, A. P., et al. (2017a). A chronic in situ coil system adapted for intracerebral stimulation during MRI in rats. J. Neurosci. Methods 284, 85–95. doi: 10.1016/j.jneumeth.2017.04.018
Madularu, D., Yee, J. R., Kulkarni, P., and Ferris, C. F. (2017c). System-specific activity in response to Delta9 -tetrahydrocannabinol: a functional magnetic resonance imaging study in awake male rats. Eur. J. Neurosci. 46, 2893–2900. doi: 10.1111/ejn.13754
Mansfield, P., Chapman, B. L., Bowtell, R., Glover, P., Coxon, R., and Harvey, P. R. (1995). Active acoustic screening: reduction of noise in gradient coils by Lorentz force balancing. Magn. Reson. Med. 33, 276–281. doi: 10.1002/mrm.1910330220
Martin, C., Martindale, J., Berwick, J., and Mayhew, J. (2006). Investigating neural-hemodynamic coupling and the hemodynamic response function in the awake rat. Neuroimage 32, 33–48. doi: 10.1016/j.neuroimage.2006.02.021
Martin, C. J., Kennerley, A. J., Berwick, J., Port, M., and Mayhew, J. E. (2013). Functional MRI in conscious rats using a chronically implanted surface coil. J. Magn. Reson. Imaging 38, 739–744. doi: 10.1002/jmri.23914
McJury, M., Stewart, R. W., Crawford, D., and Toma, E. (1997). The use of active noise control (ANC) to reduce acoustic noise generated during MRI scanning: some initial results. Magn. Reson. Imaging 15, 319–322. doi: 10.1016/s0730-725x(96)00337-2
Meyer, J. S., Brevard, M. E., Piper, B. J., Ali, S. F., and Ferris, C. F. (2006). Neural effects of MDMA as determined by functional magnetic resonance imaging and magnetic resonance spectroscopy in awake marmoset monkeys. Ann. N. Y. Acad. Sci. 1074, 365–376. doi: 10.1196/annals.1369.036
Moore, K., Madularu, D., Iriah, S., Yee, J. R., Kulkarni, P., Darcq, E., et al. (2016). BOLD imaging in awake wild-type and mu-opioid receptor knock-out mice reveals on-target activation maps in response to oxycodone. Front. Neurosci. 10:471. doi: 10.3389/fnins.2016.00471
Nephew, B. C., Caffrey, M. K., Felix-Ortiz, A. C., and Febo, M. (2012). Cocaine sensitization increases kyphosis and modulates neural activity in adult nulliparous rats. Brain Sci. 2, 667–683. doi: 10.3390/brainsci2040667
Nephew, B. C., Caffrey, M. K., Felix-Ortiz, A. C., Ferris, C. F., and Febo, M. (2009). Blood oxygen level-dependent signal responses in corticolimbic ‘emotions’ circuitry of lactating rats facing intruder threat to pups. Eur. J. Neurosci. 30, 934–945. doi: 10.1111/j.1460-9568.2009.06875.x
Ogawa, S., Tank, D. W., Menon, R., Ellermann, J. M., Kim, S. G., Merkle, H., et al. (1992). Intrinsic signal changes accompanying sensory stimulation: functional brain mapping with magnetic resonance imaging. Proc. Natl. Acad. Sci. U.S.A. 89, 5951–5955. doi: 10.1073/pnas.89.13.5951
Paasonen, J., Laakso, H., Pirttimaki, T., Stenroos, P., Salo, R. A., Zhurakovskaya, E., et al. (2020). Multi-band SWIFT enables quiet and artefact-free EEG-fMRI and awake fMRI studies in rat. NeuroImage 206:116338. doi: 10.1016/j.neuroimage.2019.116338
Paasonen, J., Salo, R. A., Huttunen, J. K., and Grohn, O. (2017). Resting-state functional MRI as a tool for evaluating brain hemodynamic responsiveness to external stimuli in rats. Magn. Reson. Med. 78, 1136–1146. doi: 10.1002/mrm.26496
Paasonen, J., Stenroos, P., Salo, R. A., Kiviniemi, V., and Grohn, O. (2018). Functional connectivity under six anesthesia protocols and the awake condition in rat brain. Neuroimage 172, 9–20. doi: 10.1016/j.neuroimage.2018.01.014
Pais-Roldan, P., Mateo, C., Pan, W. J., Acland, B., Kleinfeld, D., Snyder, L. H., et al. (2021). Contribution of animal models toward understanding resting state functional connectivity. Neuroimage 245:118630. doi: 10.1016/j.neuroimage.2021.118630
Papoti, D., Yen, C. C., Hung, C. C., Ciuchta, J., Leopold, D. A., and Silva, A. C. (2017). Design and implementation of embedded 8-channel receive-only arrays for whole-brain MRI and fMRI of conscious awake marmosets. Magn. Reson. Med. 78, 387–398. doi: 10.1002/mrm.26339
Papoti, D., Yen, C. C., Mackel, J. B., Merkle, H., and Silva, A. C. (2013). An embedded four-channel receive-only RF coil array for fMRI experiments of the somatosensory pathway in conscious awake marmosets. NMR Biomed. 26, 1395–1402. doi: 10.1002/nbm.2965
Patino, J., Karagas, N. E., Chandra, S., Thakur, N., and Stimming, E. F. (2021). Olfactory dysfunction in Huntington’s disease. J. Huntingtons Dis. 10, 413–422.
Russo, G., Helluy, X., Behroozi, M., and Manahan-Vaughan, D. (2021). Gradual restraint habituation for awake functional magnetic resonance imaging combined with a sparse imaging paradigm reduces motion artifacts and stress levels in rodents. Front. Neurosci. 15:805679. doi: 10.3389/fnins.2021.805679
Sadaka, A. H., Ozuna, A. G., Ortiz, R. J., Kulkarni, P., Johnson, C. T., Bradshaw, H. B., et al. (2021). Cannabidiol has a unique effect on global brain activity: a pharmacological, functional MRI study in awake mice. J. transl. Med. 19:220. doi: 10.1186/s12967-021-02891-6
Sakurai, K., Shintani, T., Jomura, N., Matsuda, T., Sumiyoshi, A., and Hisatsune, T. (2020). Hyper BOLD activation in dorsal raphe nucleus of APP/PS1 Alzheimer’s disease mouse during reward-oriented drinking test under thirsty conditions. Sci. Rep. 10:3915. doi: 10.1038/s41598-020-60894-7
Schaeffer, D. J., Gilbert, K. M., Hori, Y., Gati, J. S., Menon, R. S., and Everling, S. (2019). Integrated radiofrequency array and animal holder design for minimizing head motion during awake marmoset functional magnetic resonance imaging. Neuroimage 193, 126–138. doi: 10.1016/j.neuroimage.2019.03.023
Seah, S., Asad, A. B., Baumgartner, R., Feng, D., Williams, D. S., Manigbas, E., et al. (2014). Investigation of cross-species translatability of pharmacological MRI in awake nonhuman primate – a buprenorphine challenge study. PLoS One 9:e110432. doi: 10.1371/journal.pone.0110432
Shah, L., Kulkarni, P., Ferris, C., and Amiji, M. M. (2014). Analgesic efficacy and safety of DALDA peptide analog delivery to the brain using oil-in-water nanoemulsion formulation. Pharm. Res. 31, 2724–2734. doi: 10.1007/s11095-014-1370-y
Silva, A. C. (2017). Anatomical and functional neuroimaging in awake, behaving marmosets. Dev. Neurobiol. 77, 373–389. doi: 10.1002/dneu.22456
Silva, A. C., Liu, J. V., Hirano, Y., Leoni, R. F., Merkle, H., Mackel, J. B., et al. (2011). Longitudinal functional magnetic resonance imaging in animal models. Methods Mol. Biol. 711, 281–302. doi: 10.1007/978-1-61737-992-5_14
Skoubis, P. D., Hradil, V., Chin, C. L., Luo, Y., Fox, G. B., and McGaraughty, S. (2006). Mapping brain activity following administration of a nicotinic acetylcholine receptor agonist, ABT-594, using functional magnetic resonance imaging in awake rats. Neuroscience 137, 583–591. doi: 10.1016/j.neuroscience.2005.08.072
Snead, O. C. III (1995). Basic mechanisms of generalized absence seizures. Ann. Neurol. 37, 146–157. doi: 10.1002/ana.410370204
Stefanacci, L., Reber, P., Costanza, J., Wong, E., Buxton, R., Zola, S., et al. (1998). fMRI of monkey visual cortex. Neuron 20, 1051–1057. doi: 10.1016/s0896-6273(00)80485-7
Steiner, A. R., Rousseau-Blass, F., Schroeter, A., Hartnack, S., and Bettschart-Wolfensberger, R. (2021). Systematic review: anesthetic protocols and management as confounders in rodent blood oxygen level dependent functional magnetic resonance imaging (BOLD fMRI)-part b: effects of anesthetic agents, doses and timing. Animals (Basel) 11:199. doi: 10.3390/ani11010199
Stenroos, P., Paasonen, J., Salo, R. A., Jokivarsi, K., Shatillo, A., Tanila, H., et al. (2018). Awake rat brain functional magnetic resonance imaging using standard radio frequency coils and a 3D printed restraint kit. Front. Neurosci. 12:548.
Tang, H., Kukral, D., Li, Y. W., Fronheiser, M., Malone, H., Pena, A., et al. (2018). Mapping the central effects of (+/-)-ketamine and traxoprodil using pharmacological magnetic resonance imaging in awake rats. J. Psychopharmacol. 32, 146–155. doi: 10.1177/0269881117746901
Tardif, S. D., Mansfield, K. G., Ratnam, R., Ross, C. N., and Ziegler, T. E. (2011). The marmoset as a model of aging and age-related diseases. ILAR J. 52, 54–65. doi: 10.1093/ilar.52.1.54
Tenney, J. R., Duong, T. Q., King, J. A., and Ferris, C. F. (2004a). FMRI of brain activation in a genetic rat model of absence seizures. Epilepsia 45, 576–582. doi: 10.1111/j.0013-9580.2004.39303.x
Tenney, J. R., Marshall, P. C., King, J. A., and Ferris, C. F. (2004b). fMRI of generalized absence status epilepticus in conscious marmoset monkeys reveals corticothalamic activation. Epilepsia 45, 1240–1247. doi: 10.1111/j.0013-9580.2004.21504.x
Tenney, J. R., Duong, T. Q., King, J. A., Ludwig, R., and Ferris, C. F. (2003). Corticothalamic modulation during absence seizures in rats: a functional MRI assessment. Epilepsia 44, 1133–1140. doi: 10.1046/j.1528-1157.2003.61002.x
Thompson, M. F., Poirier, G. L., Davila-Garcia, M. I., Huang, W., Tam, K., Robidoux, M., et al. (2018). Menthol enhances nicotine-induced locomotor sensitization and in vivo functional connectivity in adolescence. J. Psychopharmacol 32, 332–343. doi: 10.1177/0269881117719265
Toarmino, C. R., Yen, C. C. C., Papoti, D., Bock, N. A., Leopold, D. A., Miller, C. T., et al. (2017). Functional magnetic resonance imaging of auditory cortical fields in awake marmosets. Neuroimage 162, 86–92. doi: 10.1016/j.neuroimage.2017.08.052
Tsurugizawa, T., Tamada, K., Debacker, C., Zalesky, A., and Takumi, T. (2021). Cranioplastic surgery and acclimation training for awake mouse fMRI. Bio Protoc. 11:e3972. doi: 10.21769/BioProtoc.3972
Tsurugizawa, T., Uematsu, A., Uneyama, H., and Torii, K. (2012). Functional brain mapping of conscious rats during reward anticipation. J. Neurosci. Methods 206, 132–137. doi: 10.1016/j.jneumeth.2012.02.014
Tu, W., Ma, Z., and Zhang, N. (2021). Brain network reorganization after targeted attack at a hub region. Neuroimage 237:118219. doi: 10.1016/j.neuroimage.2021.118219
Uematsu, A., Kitamura, A., Iwatsuki, K., Uneyama, H., and Tsurugizawa, T. (2015). Correlation between activation of the prelimbic cortex, basolateral amygdala, and agranular insular cortex during taste memory formation. Cereb. Cortex 25, 2719–2728. doi: 10.1093/cercor/bhu069
Upadhyay, J., Baker, S. J., Chandran, P., Miller, L., Lee, Y., Marek, G. J., et al. (2011). Default-mode-like network activation in awake rodents. PLoS One 6:e27839. doi: 10.1371/journal.pone.0027839
Weiss, C., Procissi, D., Power, J. M., and Disterhoft, J. F. (2018). The rabbit as a behavioral model system for magnetic resonance imaging. J. Neurosci. Methods 300, 196–205. doi: 10.1016/j.jneumeth.2017.05.021
Wyrwicz, A. M., Chen, N., Li, L., Weiss, C., and Disterhoft, J. F. (2000). fMRI of visual system activation in the conscious rabbit. Magn. Reson. Med. 44, 474–478. doi: 10.1002/1522-2594(200009)44:3<474::aid-mrm19>3.0.co;2-i
Yee, J. R., Kenkel, W., Caccaviello, J. C., Gamber, K., Simmons, P., Nedelman, M., et al. (2015). Identifying the integrated neural networks involved in capsaicin-induced pain using fMRI in awake TRPV1 knockout and wild-type rats. Front. Syst. Neurosci. 9:15. doi: 10.3389/fnsys.2015.00015
Yee, J. R., Kenkel, W. M., Kulkarni, P., Moore, K., Perkeybile, A. M., Toddes, S., et al. (2016). BOLD fMRI in awake prairie voles: a platform for translational social and affective neuroscience. Neuroimage 138, 221–232. doi: 10.1016/j.neuroimage.2016.05.046
Yoshida, K., Mimura, Y., Ishihara, R., Nishida, H., Komaki, Y., Minakuchi, T., et al. (2016). Physiological effects of a habituation procedure for functional MRI in awake mice using a cryogenic radiofrequency probe. J. Neurosci. Methods 274, 38–48. doi: 10.1016/j.jneumeth.2016.09.013
Zhang, N., Rane, P., Huang, W., Liang, Z., Kennedy, D., Frazier, J. A., et al. (2010). Mapping resting-state brain networks in conscious animals. J. Neurosci. Methods 189, 186–196. doi: 10.1016/j.jneumeth.2010.04.001
Zhang, Z., Andersen, A., Grondin, R., Barber, T., Avison, R., Gerhardt, G., et al. (2001). Pharmacological MRI mapping of age-associated changes in basal ganglia circuitry of awake rhesus monkeys. Neuroimage 14, 1159–1167. doi: 10.1006/nimg.2001.0902
Zhang, Z., Andersen, A. H., Ai, Y., Loveland, A., Hardy, P. A., Gerhardt, G. A., et al. (2006). Assessing nigrostriatal dysfunctions by pharmacological MRI in parkinsonian rhesus macaques. Neuroimage 33, 636–643. doi: 10.1016/j.neuroimage.2006.07.004
Zhang, Z., Andersen, A. H., Avison, M. J., Gerhardt, G. A., and Gash, D. M. (2000). Functional MRI of apomorphine activation of the basal ganglia in awake rhesus monkeys. Brain Res. 852, 290–296. doi: 10.1016/s0006-8993(99)02243-x
Keywords: fear, drug abuse, pain, epilepsy, phMRI (pharmacological fMRI)
Citation: Ferris CF (2022) Applications in Awake Animal Magnetic Resonance Imaging. Front. Neurosci. 16:854377. doi: 10.3389/fnins.2022.854377
Received: 13 January 2022; Accepted: 09 March 2022;
Published: 05 April 2022.
Edited by:
Annemie Van Der Linden, University of Antwerp, BelgiumReviewed by:
Sung-Ho Lee, University of North Carolina at Chapel Hill, United StatesJaakko Paasonen, University of Eastern Finland, Finland
Copyright © 2022 Ferris. This is an open-access article distributed under the terms of the Creative Commons Attribution License (CC BY). The use, distribution or reproduction in other forums is permitted, provided the original author(s) and the copyright owner(s) are credited and that the original publication in this journal is cited, in accordance with accepted academic practice. No use, distribution or reproduction is permitted which does not comply with these terms.
*Correspondence: Craig F. Ferris, Yy5mZXJyaXNAbm9ydGhlYXN0ZXJuLmVkdQ==