- 1Centre for Eye and Vision Research, Hong Kong Science Park, Hong Kong, Hong Kong SAR, China
- 2Department of Physiotherapy and Paramedicine, School of Health and Life Sciences, Glasgow Caledonian University, Glasgow, United Kingdom
- 3School of Optometry, The Hong Kong Polytechnic University, Kowloon, Hong Kong SAR, China
- 4School of Optometry and Vision Science, University of Waterloo, Waterloo, ON, Canada
Objective: Multiple studies have explored the use of visual cortex non-invasive brain stimulation (NIBS) to enhance visual function. These studies vary in sample size, outcome measures, and methodology. We conducted a systematic review and meta-analyses to assess the effects of NIBS on visual functions in human participants with normal vision.
Methods: We followed the PRISMA guidelines, and a review protocol was registered with PROSPERO before study commencement (CRD42021255882). We searched Embase, Medline, PsychInfo, PubMed, OpenGrey and Web of Science using relevant keywords. The search covered the period from 1st January 2000 until 1st September 2021. Comprehensive meta-analysis (CMA) software was used for quantitative analysis.
Results: Fifty studies were included in the systematic review. Only five studies utilized transcranial magnetic stimulation (TMS) and no TMS studies met our pre-specified criteria for meta-analysis. Nineteen transcranial electrical stimulation studies (tES, 38%) met the criteria for meta-analysis and were the focus of our review. Meta-analysis indicated acute effects (Hedges’s g = 0.232, 95% CI: 0.023–0.442, p = 0.029) and aftereffects (0.590, 95% CI: 0.182–0.998, p = 0.005) of tES on contrast sensitivity. Visual evoked potential (VEP) amplitudes were significantly enhanced immediately after tES (0.383, 95% CI: 0.110–0.665, p = 0.006). Both tES (0.563, 95% CI: 0.230–0.896, p = 0.001) and anodal-transcranial direct current stimulation (a-tDCS) alone (0.655, 95% CI: 0.273–1.038, p = 0.001) reduced crowding in peripheral vision. The effects of tES on visual acuity, motion perception and reaction time were not statistically significant.
Conclusion: There are significant effects of visual cortex tES on contrast sensitivity, VEP amplitude, an index of cortical excitability, and crowding among normally sighted individuals. Additional studies are required to enable a comparable meta-analysis of TMS effects. Future studies with robust experimental designs are needed to extend these findings to populations with vision loss.
Clinical trial registration: ClinicalTrials.gov/, identifier CRD42021255882.
1. Introduction
Non-invasive brain stimulation (NIBS) enables the modulation of neural activity in targeted, superficial areas of the human brain. There are two primary NIBS techniques: transcranial magnetic stimulation (TMS) and transcranial electrical stimulation (tES).
Transcranial magnetic stimulation utilizes electromagnetic induction to generate brief electric currents within the stimulated brain area and can be delivered as either single pulses or a string of repetitive pulses. Single pulses of TMS can generate action potentials that induce a motor or perceptual response. For example, TMS delivered to the primary motor cortex can cause peripheral muscle contraction (Pascualleone et al., 1994) and TMS of the primary visual cortex can induce a phosphene percept (Bohotin et al., 2003). Repetitive pulses of TMS (rTMS) can increase or decrease cortical excitability within the stimulated brain region and alter the regional concentration of neurotransmitters such as gamma-aminobutyric acid (GABA) and glutamate (Michael et al., 2003). The effect of rTMS on cortical excitability and local neurochemistry depends on the structure of the pulse train (Hallett, 2000). Commonly used pulse trains include 1 and 10 Hz stimulation frequencies as well as continuous and intermittent theta burst protocols (cTBS and iTBS) (Huang et al., 2005).
Transcranial electrical stimulation involves the delivery of an electrical current to the brain using head-mounted electrodes. tES stimulation protocols include transcranial direct current stimulation (tDCS), transcranial random noise stimulation (tRNS) and transcranial alternating current stimulation (tACS). tES does not induce action potentials but may alter membrane potentials [tDCS (Nitsche and Paulus, 2001; Nitsche et al., 2003), tRNS (Terney et al., 2008)], induce regional changes in neurotransmitter concentration [tDCS (Stagg et al., 2009; Bachtiar et al., 2015; Hunter et al., 2015)], alter cortical excitability [tDCS (Nitsche and Paulus, 2000, 2001), tRNS (Terney et al., 2008; Moliadze et al., 2010)], entrain patterns of neural activity [tACS (Battleday et al., 2014)] and alter the signal to noise ratio within stimulated regions [tRNS, refer to Reed (Reed and Kadosh, 2018) for a review]. NIBS has been used in multiple research contexts including the study of fundamental neurological processes, cognition (Hoy et al., 2015; Grabner et al., 2018; Guleken et al., 2020), and the development of new therapeutic interventions [e.g., depression (Martin et al., 2018; Moreno et al., 2020), neurorehabilitation (Liew et al., 2014)].
Visual brain areas are attractive targets for NIBS research because regions such as the primary visual cortex and motion sensitive extrastriate area [middle temporal (MT)] are close to the cortical surface and techniques such as visual psychophysics, electroencephalography, and magnetic resonance imaging are available to measure the effects of the stimulation on neural activity and perception (Thompson et al., 2009, 2016; Miniussi et al., 2013). In addition, NIBS is emerging as a promising tool for vision rehabilitation (Pascual-Leone et al., 1998; Thompson et al., 2008). However, the literature on NIBS of visual brain areas is diverse with a wide range of different study designs, stimulation protocols, outcome measures and population samples. The aim of this structured review and meta-analysis was to assess whether visual cortex NIBS can enhance visual perception and/or modulate visual cortex activity (measured using visual evoked potentials). We did not include studies that used NIBS to induce “virtual lesions” or impair visual function to probe fundamental neurological processes. Our original plan was to review visual cortex NIBS studies involving either healthy or clinical populations [e.g., amblyopia (Spiegel et al., 2013; Ding et al., 2016) or hemianopia (Plow et al., 2012; Olma et al., 2013)]. However, our literature search revealed that studies of clinical populations did not employ common study designs and were relatively few. We therefore limited our review to studies examining the effect of NIBS on vision enhancement in healthy participants with normal vision.
2. Materials and methods
This systematic review conforms to the Preferred Reporting Items for Systematic Reviews and Meta-Analyses 2020 (PRISMA-2020) guidelines (Page et al., 2021). We registered the review protocol with the International Prospective Register of Systematic Reviews (PROSPERO; Ref. No: CRD42021255882) in June 2021, prior to the initiation of the data extraction processes. We adopted the PICO (Participants, Intervention, Comparators and Outcome) format in generating the research question. The intervention was any form of NIBS (including tDCS, tACS, tRNS, and TMS), while the comparators included sham (placebo) NIBS. Outcomes of interest included psychophysical measures of contrast sensitivity, visual crowding, visual acuity, motion perception, visual evoked potentials (VEPs), and reaction time among others. The study conceptualization and development of the review protocol were undertaken by authors UMB, JYW, BT, and AMYC.
2.1. Search strategy
A systematic search of PubMed, Embase, PsycINFO, Web of Science, Medline and OpenGrey databases was conducted from 1st January 2000 until 1st September 2021. The search terms were grouped under two themes, namely: “Brain area,” and “NIBS.” The electronic search involved combining terms under each theme using the Boolean operator “OR.” The search themes were then combined using the Boolean “AND” (see Supplementary material for details of the search themes/terms). Citation management software (EndNote X9, Clarivate Analytics, Philadelphia, PA, USA) was used to organize the electronic search results and remove duplicates. Two of the authors (JYW and UMB) independently conducted the electronic search. Any discrepancies during the independent search process were resolved by consulting a third author (AMYC). A thorough manual search of the reference lists of the identified studies and a forward reference search (via Google scholar) were also conducted.
2.2. Study eligibility criteria
Studies were included if they: (i) assessed the effect of NIBS on enhancing visual functions among normally sighted individuals; (ii) included a sham stimulation control; (iii) were available in full text and (iv) written in English. We excluded studies that were: (i) conducted on individuals presenting with mental disorders, cognitive impairments, or visual impairments; (ii) used NIBS to disrupt or impair visual function, (iii) review protocols; (iv) systematic reviews; (v) conference abstracts and (vi) case studies.
2.3. Article screening
The identified studies via electronic search processes were sequentially screened at the title, abstract and full text phases by two of the authors (JYW and UMB). Any discrepancies identified by the two authors during the screening phases were resolved by discussion or consultation with the corresponding author (AMYC).
2.4. Data extraction
The primary data for this study quantified the effect of NIBS on enhancing visual functions. Other relevant data extracted included the study reference, year of publication, title of study, study design, NIBS method, brain area stimulated, and visual function(s) measured. Data extraction was undertaken independently by JYW and BWSC using an extraction tool designed in Microsoft Excel. Disagreements between the authors during the data extraction process were resolved by discussion or consultation with the other authors (BT and AMYC).
2.5. Data analysis
Meta-analyses were conducted using the Comprehensive Meta-Analysis (CMA) software version 3.0 (Biostat Inc., Englewood, NJ, USA). Outcomes of studies that utilized protocols from the same NIBS delivery technique (tES or TMS) and reported findings on the same visual function were pooled for meta-analyses. Therefore tDCS, tRNS, and tACS studies were pooled and rTMS and TBS studies were pooled. Similar studies with differing techniques for measuring a specific visual function could be pooled. Finally, studies with a common outcome measure were pooled. Examples include reaction time and VEP. Within each pooled group, we included all relevant studies and looked at acute (immediate, same day pre- vs. post-effects of NIBS), and after effects (same day, but at a designated time point after stimulation—i.e., 10–30 min post-stimulation). In the first instance, all related NIBS subtypes (tES or TMS) were combined for a general overview, and where there were enough studies, the stimulation protocol subtypes were analyzed separately. Studies numbering two and above that met the meta-analysis criteria were pooled in a meta-analysis, in-line with a previous recommendation (Valentine et al., 2010). Study authors were contacted via email to obtain any missing data for the included studies. Unless otherwise indicated, stimulation was applied to the occipital lobe/primary visual cortex (V1). Data presented graphically were extracted using the GetData Graph Digitizer 2.26.1 Data reported as median and range were converted to mean and standard deviation (Hozo et al., 2005). We adopted the bias-adjusted, standardized mean difference (SMD; Hedges’s g) to analyze the extracted data from the primary studies. The chi-square test (I2 statistics) was used to determine the degree of variance across studies (Higgins et al., 2003), and a random-effects model was used for all the meta-analyses due to methodological heterogeneity among the studies. A p-value of <0.05 indicated statistical significance.
2.6. Quality appraisals of the included studies
Two authors (ASYP, KWST) attempted to conduct quality ratings of the included studies using the Downs and Black quality rating tool (Downs and Black, 1998), which consisted of 27 items. Ratings were conducted independently prior to comparison. However, it was noted that 14 randomly selected studies, were all rated of “poor” quality, suggesting that perhaps this instrument might not have been the most appropriate for the types of intervention studies included in this meta-analysis.
3. Results
3.1. Characteristics of the included studies
In total, 5,266 studies were identified through the electronic database and manual searches, among which 50 met the review criteria after sequential screening of the title, abstract and the full text (Kraft et al., 2010; Fertonani et al., 2011; Zito et al., 2015; Barbieri et al., 2016; Ding et al., 2016; Reinhart et al., 2016; van Koningsbruggen et al., 2016; Battaglini et al., 2017, 2020a; Behrens et al., 2017; Bonder et al., 2018; Contemori et al., 2019; He et al., 2019; Dong et al., 2020; Nakazono et al., 2020; Raveendran et al., 2020; Wu et al., 2020; Chen et al., 2021; Lau et al., 2021). Of these studies, 19 met the criteria for meta-analysis. Supplementary material summarizes the reasons why the remaining 31 studies were not eligible for meta-analysis. Only five TMS studies were identified through the search process. Because these studies did not have common methodologies or outcomes measures, they did not meet our criteria for meta-analysis. Therefore, our meta-analysis was conducted only on tES studies.
The study flowchart detailing the search outcome and screening processes is presented in Figure 1. Overall, the included studies recruited 674 participants. For the NIBS modalities adopted in the included studies, most studies utilized tDCS (n = 14, 73.7%), then tRNS (n = 3, 15.8%) and tACS (n = 1, 5.3%). Another study utilized tRNS with tDCS (n = 1, 5.3%). The visual functions examined among the studies were contrast sensitivity (n = 7, 36.8%), reaction time (n = 6, 31.6%), VEPs (n = 4, 21.1%), motion perception (n = 3, 15.8%), crowding (n = 3, 15.8%), and visual acuity (n = 2, 10.5%)2. Table 1 presents the study characteristics.
3.2. Quantitative analysis of whether NIBS can enhance visual function
3.2.1. Contrast sensitivity
3.2.1.1. Acute effect of tES (a-tDCS, tRNS, and tACS) on contrast sensitivity
Included studies measured the same-day effects of a single NIBS session on contrast sensitvity. The pooled analysis involved six studies, among which four utilized a-tDCS (Kraft et al., 2010; Ding et al., 2016; Behrens et al., 2017; He et al., 2019), one adopted tACS (Nakazono et al., 2020) and one utilized tRNS (Battaglini et al., 2020a). In studies that measured multiple outcomes, only the contrast sensitivity results were included (Ding et al., 2016; Nakazono et al., 2020). Contrast sensitivity was measured using perimetry (Kraft et al., 2010; Behrens et al., 2017), a 10 cycles per degree (cpd) Gabor patch (Ding et al., 2016), or stimuli presented at a range of spatial frequencies (He et al., 2019; Battaglini et al., 2020a; Nakazono et al., 2020). If the study measured contrast sensitivity at more than one spatial frequency, the results for the highest spatial frequency was chosen, because the most challenging condition was expected to show the greatest NIBS-induced enhancement. Spatial frequencies selected included 9 cpd (Nakazono et al., 2020), 10 cpd (Ding et al., 2016), and both 7 cpd and 12 cpd for the study by Battaglini et al. (2020a) because the authors explicitly hypothesized that sensitivity for both higher spatial frequencies would be enhanced by the tRNS. For studies that measured contrast sensitivity at more than one retinal eccentricity, the measures for central vision were selected for meta-analysis to provide consistency across studies. Nakazono et al. (2020) compared alpha and beta tACS to a sham condition. Both stimulation frequencies were included in the meta-analysis. Similarly, Battaglini et al. (2020a) used vertical and 45° oriented Gabors for their contrast detection tasks. Both orientations were included in the meta-analysis. We pooled the effect of active stimulation against sham conditions for the analysis (Figure 2). The result indicated a statistically significant acute effect of tES stimulation (Hedges’s g = 0.232, 95% CI: 0.023–0.442, p = 0.029) on contrast sensitivity in normally sighted participants.
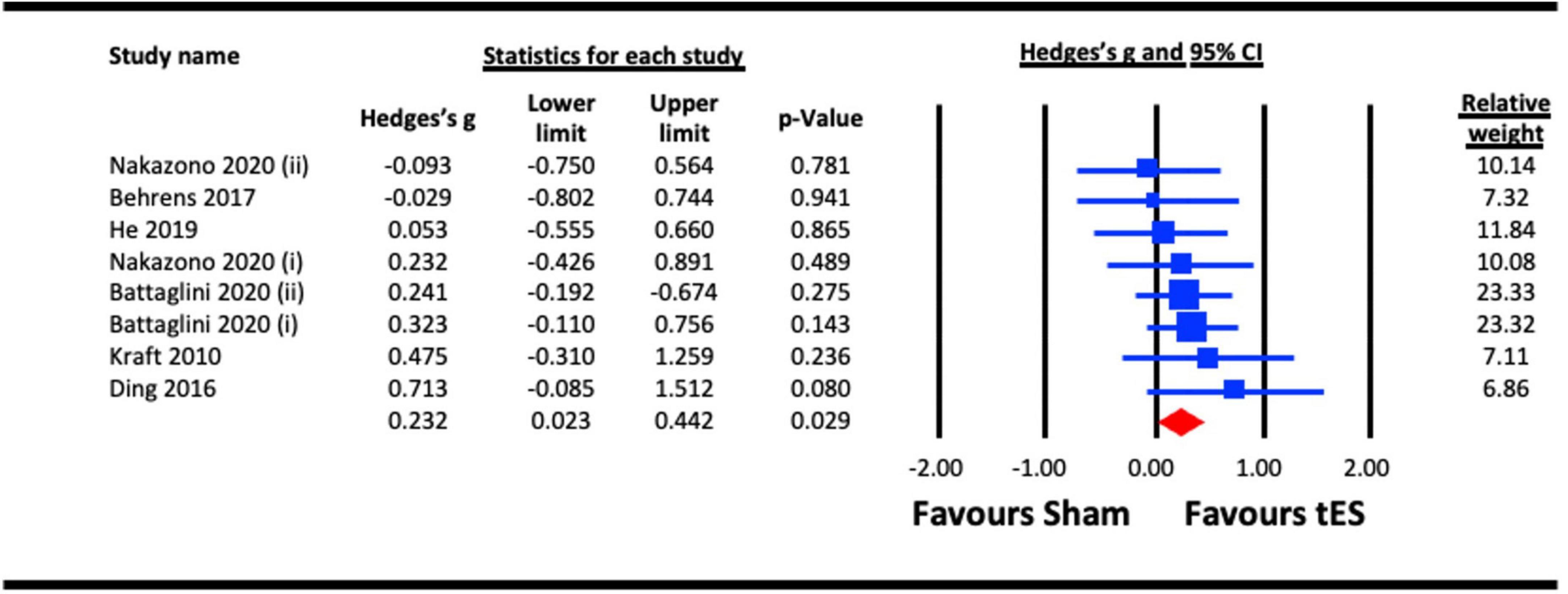
Figure 2. Acute effect of transcranial electrical stimulation (tES) [anodal-transcranial direct current stimulation (a-tDCS), transcranial random noise stimulation (tRNS), and transcranial alternating current stimulation (tACS)] on contrast sensitivity. Meta-analyses for Nakazono (2020) were separated for data on 9.0 cpd alpha (acute) and 9.0 cpd beta (acute), represented as Nakazono (2020) (i) and Nakazono (2020) (ii) Meta analyses for Battaglini (2020a) were separated for data using contrast stimuli of 45° and vertical, represented as Battaglini (2020a) (i) and Battaglini (2020a) (ii).
3.2.1.2. Acute effect of anodal tDCS (a-tDCS) on contrast sensitivity
A single session acute effect of a-tDCS on contrast sensitivity is illustrated in Figure 3. Of those studies included in section 1.1, the a-tDCs studies were pooled for the analysis (Kraft et al., 2010; Ding et al., 2016; Behrens et al., 2017; He et al., 2019). There was a trend favoring an effect of a-tDCS stimulation on contrast sensitivity as per the main analysis, but this failed to reach statistical significance (Hedges’s g = 0.262, 95% CI: −0.101 to 0.625, p = 0.158).
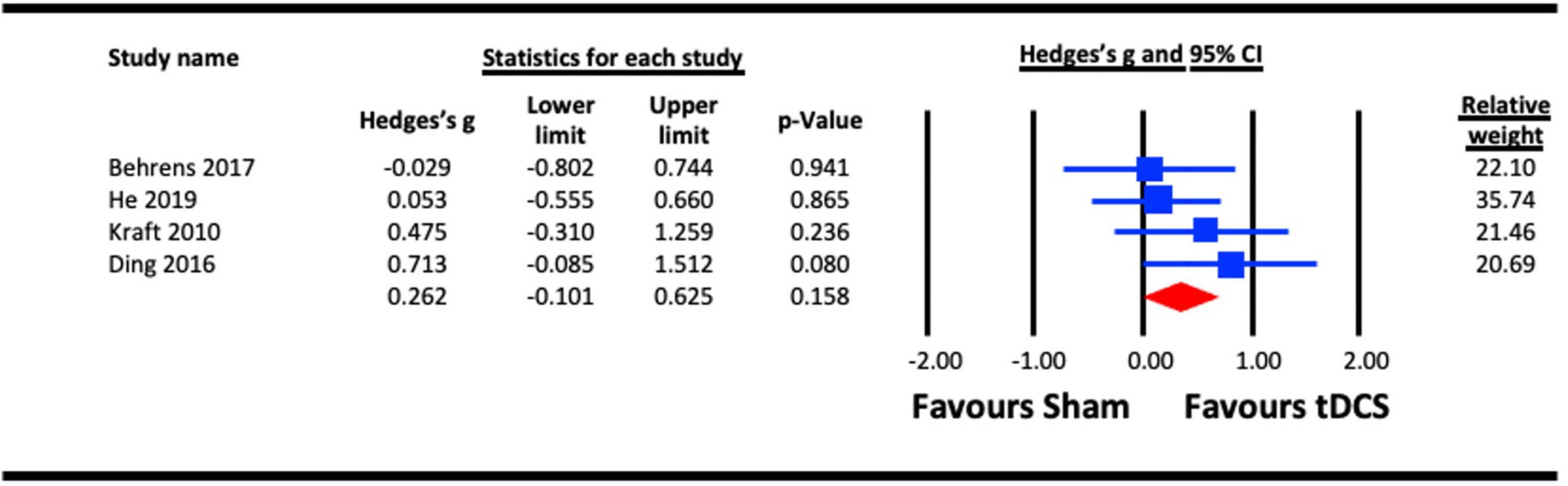
Figure 3. Acute effect of anodal-transcranial direct current stimulation (a-tDCS) on contrast sensitivity.
3.2.1.3. Aftereffect of tES (a-tDCS and tACS) on contrast sensitivity
Nakazono et al. (2020) and Ding et al. (2016) reported aftereffects of tES on contrast sensitivity measured 10- and 30 min post-stimulation, respectively (Figure 4). A meta-analysis revealed a statistically significant aftereffect of tES stimulation on contrast sensitivity (Hedges’s g = 0.590, 95% CI: 0.182–0.998, p = 0.005).
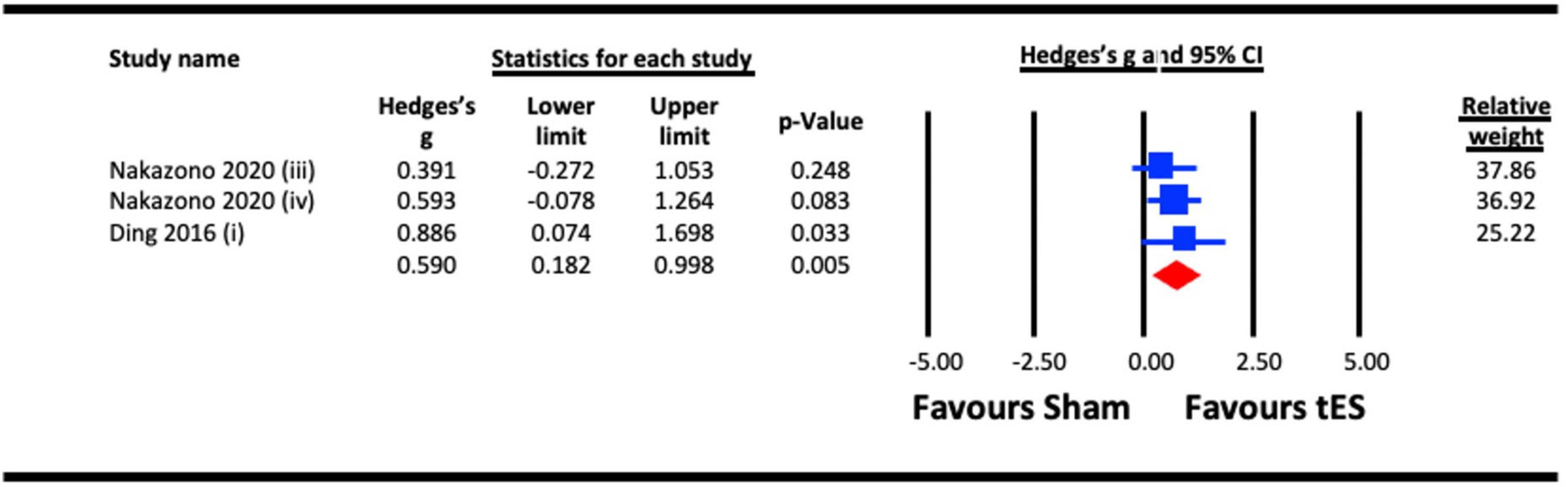
Figure 4. Aftereffect of transcranial electrical stimulation (tES) [anodal-transcranial direct current stimulation (a-tDCS) and transcranial alternating current stimulation (tACS)] on contrast sensitivity. Meta-analyses for Nakazono (2020) were separated for data on 9.0 cpd beta (at 10 min post) and 9.0 cpd alpha (at 10 min post), represented as Nakazono (2020) (iii) and Nakazono (2020) (iv).
3.2.2. Visual evoked potentials (VEPs)
3.2.2.1. Acute effect of tES (a-tDCS and tACS) on VEP amplitude
Four studies were pooled for analysis to assess the acute effect of tES on VEP amplitude, three utilized a-tDCS (Ding et al., 2016; Dong et al., 2020; Lau et al., 2021) and one adopted tACS (Nakazono et al., 2020). In studies that measured the acute effects of NIBS on different visual functions (e.g., contrast sensitivity and VEPs), the results from the VEP measure were taken (Ding et al., 2016; Nakazono et al., 2020). Different components of VEPs were estimated in the studies, including amplitude of P100-N75 (Ding et al., 2016; Nakazono et al., 2020), amplitude of the alpha activity over the parieto-occipital area (Dong et al., 2020), and N1 and P1 amplitudes (Lau et al., 2021) (both included in the analysis). Similarly, where alpha and beta tACS were utilized in a study (Nakazono et al., 2020), the effect of each stimulation condition against a sham effect was extracted for the analysis. We pooled the effect of active stimulation against sham conditions for the analysis (Figure 5). The result indicated a statistically significant increase in VEP amplitude immediately after tES at visual cortex (Hedges’s g = 0.383, 95% CI: 0.110–0.655, p = 0.006).
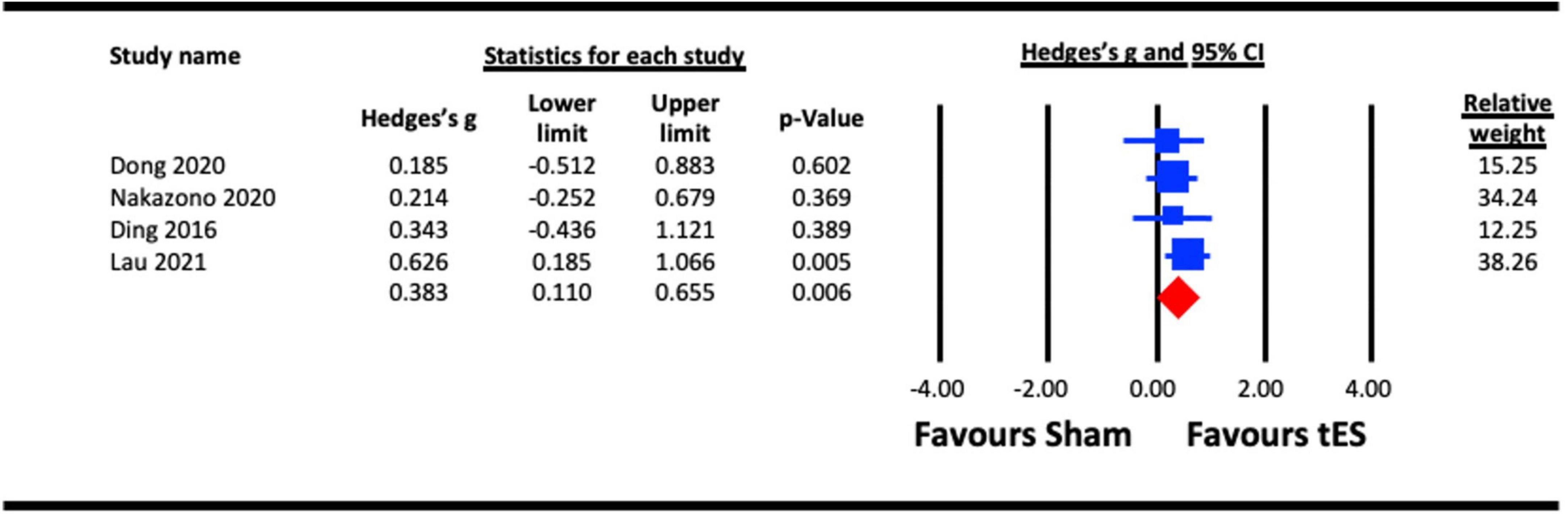
Figure 5. Acute effect of transcranial electrical stimulation (tES) [anodal-transcranial direct current stimulation (a-tDCS) and transcranial alternating current stimulation (tACS)] on visual evoked potentials (VEPs). Nakazono (2020), combined effect of alpha and beta tACS; Lau (2021), combined effect of N1 and P1 amplitudes.
3.2.3. Crowding
3.2.3.1. Acute effect of tES (a-tDCS and tRNS) on crowding
Three studies were pooled for analysis to assess the acute effect of tES on crowding, two utilized a-tDCS (Raveendran et al., 2020; Chen et al., 2021), and one adopted tRNS (Contemori et al., 2019). In the study with multiple experiments involving different groups of participants (Chen et al., 2021), data from each experiment were pooled separately in the analysis. The results for NIBS applied to the hemisphere contralateral to the presented stimuli against a sham condition were pooled in the analysis (Chen et al., 2021). The earliest effect of a-tDCS on crowding (5 min post-stimulation) reported by Raveendran et al. (2020) was pooled for analysis. The analysis (Figure 6) indicated a statistically significant effect of tES on crowding (Hedges’s g = 0.563, 95% CI: 0.230–0.896, p = 0.001).
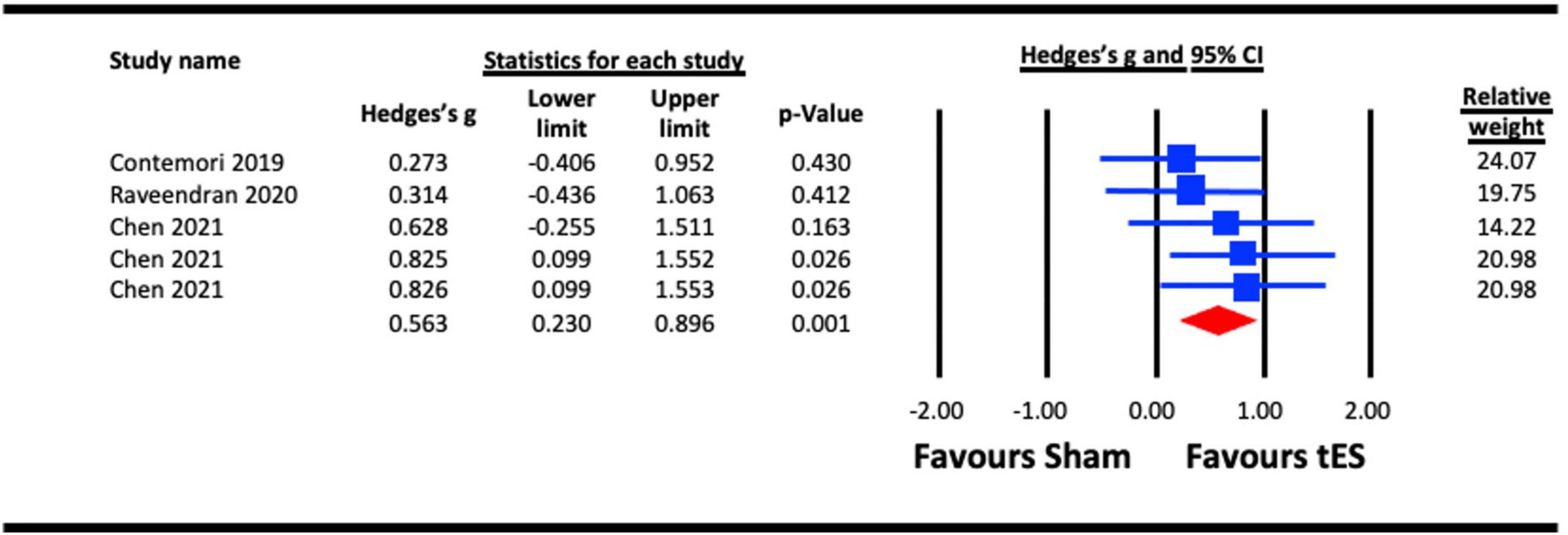
Figure 6. Acute effect of transcranial electrical stimulation (tES) [anodal-transcranial direct current stimulation (a-tDCS) and transcranial random noise stimulation (tRNS)] on crowding. Chen (2021) (each line represents the outcome of experiments 1–3).
3.2.3.2. Acute effect of a-tDCS on crowding
To assess the acute effect of a-tDCS on crowding (independent of tRNS), data from the two studies that used a-tDCS (Raveendran et al., 2020; Chen et al., 2021) were pooled for analysis. The result (Figure 7) indicated a statistically significant effect of a-tDCS on crowding (Hedges’s g = 0.655, 95% CI: 0.273–1.038, p = 0.001).
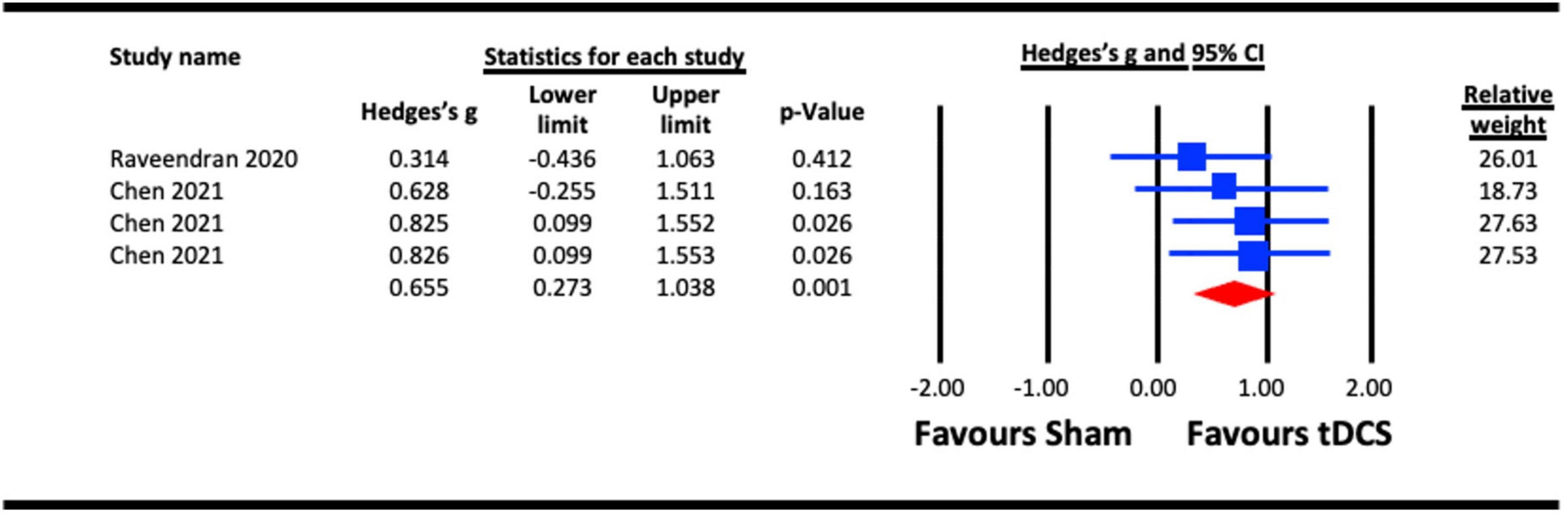
Figure 7. Acute effect of anodal-transcranial direct current stimulation (a-tDCS) on crowding. Chen (2021) (each line represents the outcome of experiments 1–3).
3.2.4. Visual acuity
3.2.4.1. Acute effect of a-tDCS on visual acuity
To assess the acute effect of a-tDCS on visual acuity, two studies (Reinhart et al., 2016; Bonder et al., 2018) were pooled for analysis. In a study that measured the acute effects of a-tDCS on different visual functions (visual acuity, contrast sensitivity and VEPs), the results from the visual acuity measure were taken (Reinhart et al., 2016). The pooled effect for the active stimulation condition in each study was compared to sham conditions (Figure 8). The result indicated a statistically non-significant effect of a-tDCS on visual acuity (Hedges’s g = 0.408, 95% CI: −0.056 to 0.872, p = 0.085).
3.2.5. Motion perception
3.2.5.1. Acute effect of a-tDCS on motion perception
The pooled analysis to assess the acute effect of a-tDCS on motion perception involved three studies (Zito et al., 2015; Battaglini et al., 2017; Wu et al., 2020). All studies stimulated extrastriate cortical area V5 (MT). In the study that measured the acute effects of a-tDCS on different visual functions (motion and shape perception), the results from the motion perception measure were taken (Zito et al., 2015). The pooled effect for each of the study were compared against sham control conditions (Figure 9). The result indicated a statistically non-significant effect of a-tDCS on motion perception (Hedges’s g = 0.802, 95% CI: −0.458 to 2.063, p = 0.212).
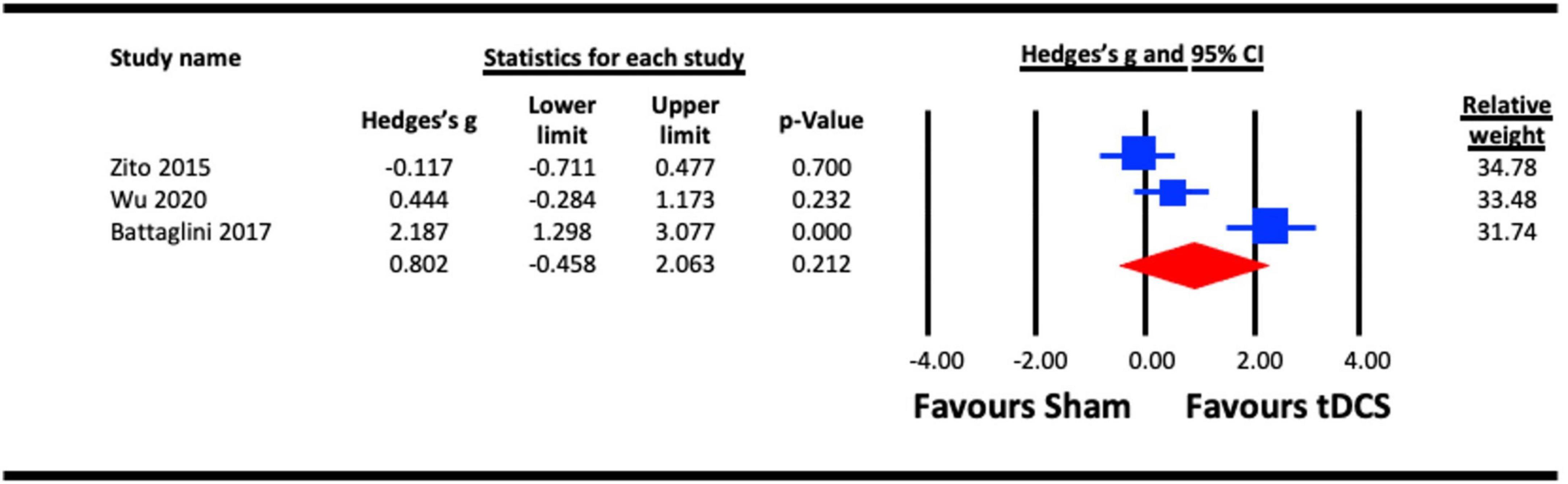
Figure 9. Acute effect of anodal-transcranial direct current stimulation (a-tDCS) on motion perception.
3.2.6. Reaction time
3.2.6.1. Acute effect of tES (tRNS and a-tDCS) on reaction time
Reaction time was analysed as a proxy of vision-related cognitive processing. Six studies were pooled for the analysis to assess the acute effect of tES on reaction time, five utilized a-tDCS (Zito et al., 2015; Barbieri et al., 2016; Reinhart et al., 2016; Bonder et al., 2018) and two adopted tRNS (Fertonani et al., 2011; van Koningsbruggen et al., 2016). In studies that measured the acute effects of NIBS on different visual functions (e.g., face/object/motion perception, visual acuity, VEPs, contrast sensitivity, attentional capture and reaction time), the results from the reaction time measure were taken (Fertonani et al., 2011; Zito et al., 2015; Barbieri et al., 2016; Reinhart et al., 2016; van Koningsbruggen et al., 2016; Bonder et al., 2018). When a study reported the effect of multiple NIBS protocols on same group of participants (for example tRNS and a-tDCS) (Fertonani et al., 2011), both effects were pooled in the tES meta-analysis (Figure 10), respectively. Similarly, in a study with dual experimental/stimulation conditions (face and object recognition) (Barbieri et al., 2016), the effects of both conditions on the reaction time were combined and pooled in the analysis. Outcome of the analysis illustrated a statistically non-significant effect of tES on reaction time (vision-related cognitive processing) (Hedges’s g = 1.001, 95% CI: −0.405 to 2.406, p = 0.163).
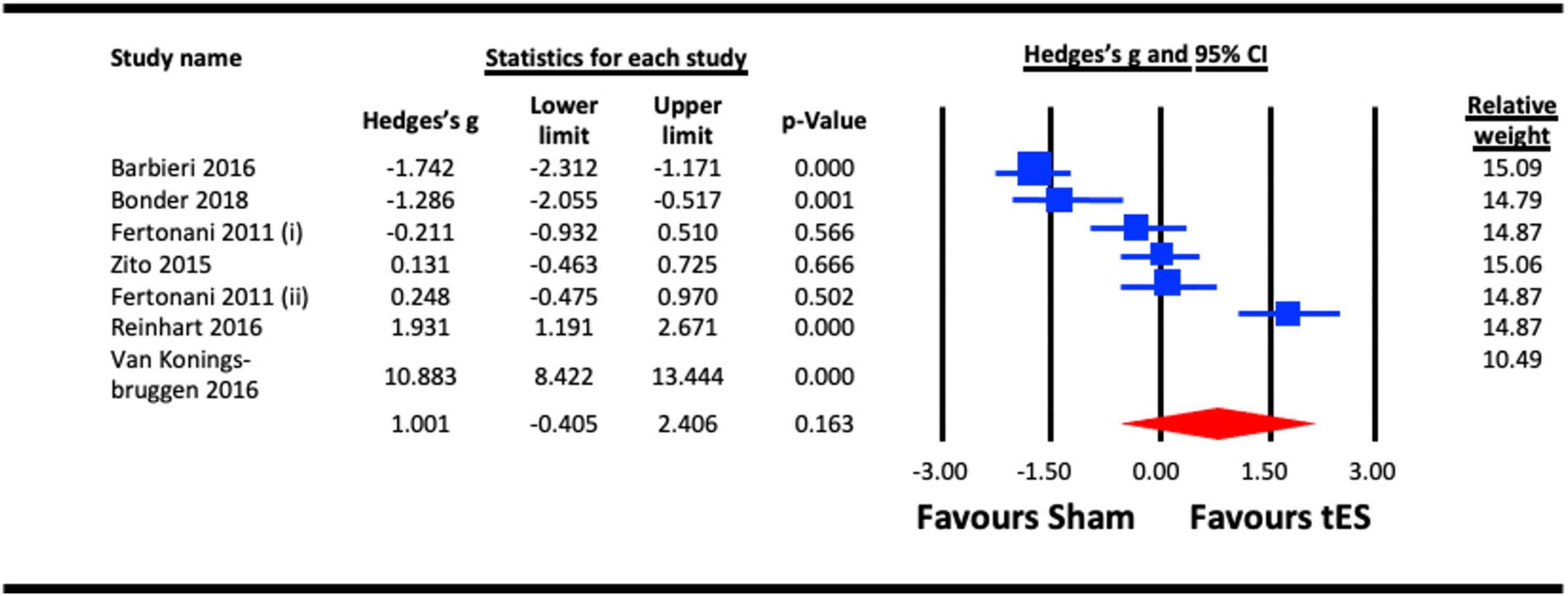
Figure 10. Acute effect of transcranial electrical stimulation (tES) [anodal-transcranial direct current stimulation (a-tDCS) and transcranial random noise stimulation (tRNS)] on reaction time. Barbieri (2016) (included data for combined effect of a-tDCS face and object tasks); Meta-analysis for Fertonani (2011) were separated for data on a-tDCS and tRNS, represented as Fertonani (2011) (i) and Fertonani (2011) (ii).
3.2.6.2. Acute effect of a-tDCS on reaction time
To assess the acute effect of a-tDCS on reaction time, data from the five studies that used a-tDCS in the sub-section 6.1 (Fertonani et al., 2011; Zito et al., 2015; Barbieri et al., 2016; Reinhart et al., 2016; Bonder et al., 2018) were pooled. The result (Figure 11) indicated a statistically non-significant effect of a-tDCS on reaction time (Hedges’s g = −0.241, 95% CI: −1.474 to 0.991, p = 0.701).
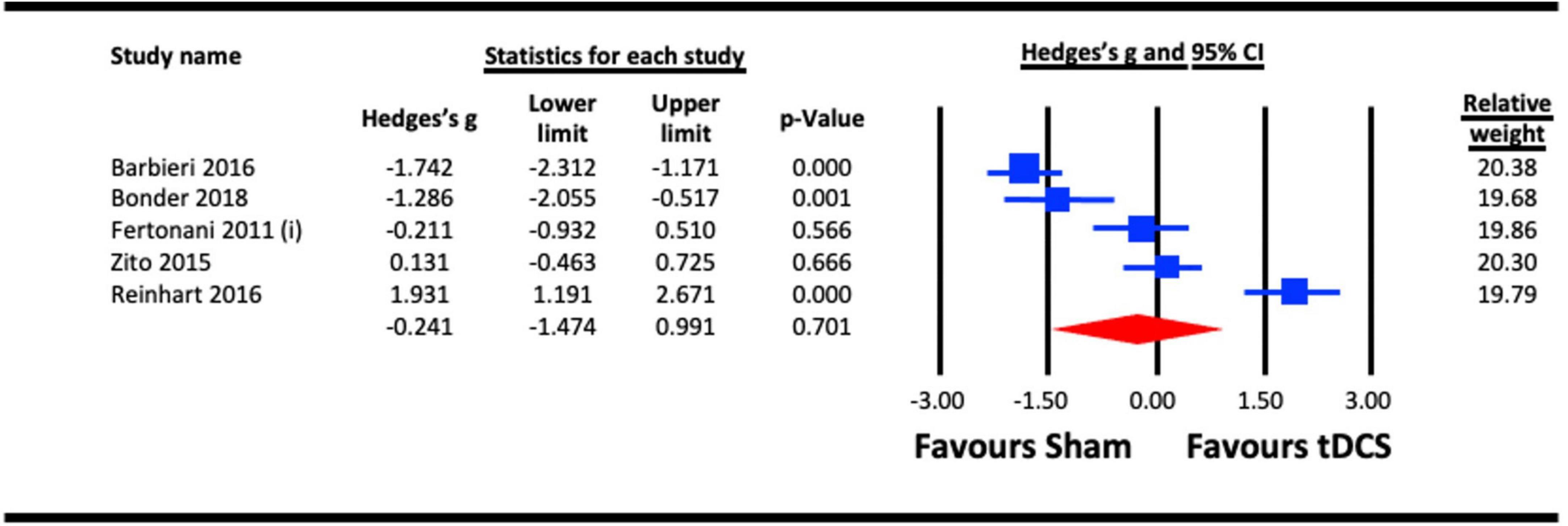
Figure 11. Acute effect of anodal-transcranial direct current stimulation (a-tDCS) on reaction time. Barbieri (2016), combined effect of a-tDCS face and object tasks; Fertonani (2011) (i), effect of a-tDCS.
4. Discussion
To recapitulate, the aim of this structured review and meta-analysis was to assess whether visual cortex NIBS could enhance visual function and/or modulate visual cortex activity. Both tES and TMS have been used as rehabilitation tools to enhance a variety of neural functions including cognition (Hara et al., 2021) and motor control (Liew et al., 2014). Unexpectedly, our literature review identified only five studies that investigated the use of TMS to enhance a specific visual function. Because these five studies used different stimulation protocols and/or different outcome measures, they could not be meta-analyzed (see Supplementary material for details). Although TMS has been widely used in vision research, it appears to have been used primarily to explore the function of targeted cortical areas or neural networks rather than a tool to enhance specific visual functions. However, it is clear that TMS does exert an effect on brain areas involved in visual processing and the five studies identified by our review reported improvements or alterations in the targeted visual function post stimulation. These effects were associated with changes in cortical excitability, neurotransmitter concentrations and signal to noise within the stimulated area. Furthermore, studies involving clinical populations have reported improvements in a variety of visual functions following visual cortex TMS (Thompson et al., 2008; Clavagnier et al., 2013). Therefore, it is likely that TMS will play a larger role in vison enhancement studies as the field continues to grow.
For the reasons described above, our meta-analysis involved only tES studies. Studies exploring the use of tES to enhance normal vision have employed a diverse range of experimental designs with time scales that range from the acute effects of a single tES session to multi-session studies that combine tES with perceptual learning. This diversity resulted in limited opportunities for meta-analyses. However, by pooling across different tES stimulation protocols and differing methodologies for assessing a common outcome measure, we were able to assess the effects of a single tES session vs. sham stimulation on contrast sensitivity, VEP amplitude, visual crowding, visual acuity, motion perception, and reaction time.
Both contrast sensitivity (Kraft et al., 2010; Ding et al., 2016; Behrens et al., 2017; He et al., 2019; Battaglini et al., 2020a; Nakazono et al., 2020) and visual crowding (Contemori et al., 2019; Raveendran et al., 2020; Chen et al., 2021) were significantly enhanced by visual cortex tES relative to sham within our meta-analyses, and were examined at different timescales relative to stimulation. Results from our meta-analyses showed beneficial acute effects of tES in enhancing contrast sensitivity (Figure 2) and reduced crowding (Figure 6). The sub-analysis of studies that only employed a-tDCS revealed improvements in crowding following stimulation (Figure 7), but not for contrast sensitivity (Figure 3). Our meta-analyses looking at later time points (i.e., aftereffects) could only be performed for contrast sensitivity as there was only one study investigating the effect of NIBS on crowding. We observed that tES was effective in modulating contrast sensitivity at a fixed time point after stimulation (Figure 4), indicating that the effects of stimulation on improving contrast sensitivity persisted beyond the stimulation period. Despite only one study measuring the aftereffects of a-tDCS on crowding (Raveendran et al., 2020) (in this case lateral-inhibition, a low-level mechanism that may contribute to crowding), the study reported a larger effect at 30 min vs. 5 min after stimulation, which is in line with the time-scale of the effects observed in the contrast sensitivity meta-analysis.
From a mechanistic perspective, the meta-analysis of changes in VEP amplitude (Ding et al., 2016; Dong et al., 2020; Nakazono et al., 2020; Lau et al., 2021) following visual cortex tES vs. sham revealed enhanced cortical excitability (i.e., larger VEP amplitudes) following tES (Figure 5). Increased cortical excitability may enhance neural sensitivity to contrast and weaken lateral inhibition mechanisms that contribute to crowding. The connection between tES and increased cortical excitability may be mediated by the relative concentration of the inhibitory neurotransmitter GABA and the excitatory neurotransmitter glutamate within the stimulated area. Reduced GABA concentration within motor cortex following a-tDCS has been reported by multiple studies (Stagg et al., 2011; Bachtiar et al., 2018) and it is possible that tES may have a similar effect when applied to the visual cortex. Within this framework, the delayed effects of tES on contrast sensitivity (Ding et al., 2016; Nakazono et al., 2020) [and perhaps crowding (Raveendran et al., 2020)] could reflect a gradual change in GABA concentration that continues for a period after the stimulation session. However, the time course of tES effects on GABA concentration remains unclear and it is also unknown whether the effects of tES on GABA concentration are the same for the motor and visual cortices. It is worth noting that indirect evidence exists suggesting that visual cortex tES does not influence GABA (Abuleil et al., 2021). Therefore, while the effects of visual cortex tES on contrast sensitivity, crowding, and VEP amplitude are supported by our meta-analyses, the underlying mechanisms require investigation.
Meta-analyses revealed no evidence for the effectiveness of tES on visual acuity, motion perception or reaction time. The visual acuity and motion perception meta-analyses included the fewest individual experiments [two for visual acuity (Reinhart et al., 2016; Bonder et al., 2018) and three for motion perception (Zito et al., 2015; Battaglini et al., 2017; Wu et al., 2020)] with considerable variations in the visual stimuli used to measure the outcomes. The small sample combined with significant protocol differences may have limited our power to detect an effect. It is possible that measures of visual acuity and motion perception differ from contrast sensitivity and crowding in their response to tES. For motion perception, it is also possible that area MT responds to tES in a way that is distinct from that of the primary visual cortex. However, additional studies are required to fully address these questions.
The reaction time meta-analysis included seven experiments and revealed high variability across studies with two reporting longer reaction times following tES (Barbieri et al., 2016; Bonder et al., 2018), three reporting no effect (Fertonani et al., 2011; Zito et al., 2015) and two reporting shorter reaction times (Reinhart et al., 2016; van Koningsbruggen et al., 2016); one with a moderate effect size (Reinhart et al., 2016) and the other with a Hedge’s G greater than five (van Koningsbruggen et al., 2016). Reaction times can be affected by multiple variables including attention, task complexity, participant instructions, and speed accuracy trade-off. The studies included in the reaction time meta-analysis differed considerably in the types of visual stimuli and tasks employed and therefore it is perhaps not surprising that tES of cortical regions responsible for early, low-level visual processing did not produce consistent effects across studies.
Our search criteria included studies involving low and high level visual functions, however, the studies eligible for meta-analysis all focused on relatively low-level visual functions. This may be because the identified studies tended to target the primary visual cortex and therefore selected outcomes measures targeting early-stages of visual processing. However, studies not included in the meta-analysis did report NIBSs effects on face perception (Barbieri et al., 2016), visuo-motor coordination (Antal et al., 2004b,c), and attention (Laczo et al., 2012). Higher level processed such as attentive search, multisensory integration and sensory decision making are potential targets for studies exploring the potential beneficial effects of NIBS on higher level sensory function and perception.
The diverse nature of the NIBS and vision literature forced us to pool across different tES protocols, visual stimuli, and experimental designs in our meta-analyses. Therefore, our results should be interpreted with caution. In particular, a non-significant meta-analysis may reflect important variations in experimental parameters rather than no effect of the stimulation itself. The tES studies included in this review varied in terms of the stimulation devices employed and specific stimulation parameters. Unfortunately, this variation combined with the different outcome measures used across studies prevented us from conducting analyses to identify optimal visual cortex stimulation protocols. However, Table 1 does provide details of device-independent parameters such as electrode size and properties of the stimulating current to enable future analyses when a pool of more uniform studies becomes available. In addition, our inclusion of multiple independent experiments from a single publication may have amplified study-specific sources of bias. As the literature on NIBS and vision continues to develop, future meta-analyses may be able to adopt more stringent analyses criteria.
5. Conclusion
Our review revealed that most vision enhancement studies involving healthy populations have employed tES rather than TMS. Meta-analyses provided evidence for the effectiveness of visual cortex tES compared to sham stimulation on modulating contrast sensitivity and crowding. These effects were accompanied by evidence for a significant increase in visual cortex excitability indexed by VEP amplitude following tES. Despite the diversity of study designs in the current tES and vision literature, the results of this review indicate that tES can enhance at least some visual functions and strengthen the foundation for the application of tES in studies of vision rehabilitation. The TMS studies identified by this review also suggest that the use of TMS to enhance visual function warrants further investigation.
Author contributions
UMB: accessing and verifying the data, conceptualization, articles’ electronic search, articles screening, meta-analysis, and draft of manuscript. JYW: accessing and verifying the data, articles screening, conceptualization, and draft of manuscript. ASYP: conceptualization, quality rating, and draft of manuscript. KWST: quality rating and draft of manuscript. BWSC: accessing and verifying the data, and data extraction. BT and AMYC: conceptualization, draft of manuscript, and project supervision. All authors had full access to the data in the study and had final responsibility for the decision to submit for publication.
Funding
This work was supported by Hong Kong Research Grants Council (Research Impact Fund R5047-19 and General Research Fund 15602821) and Government of the Hong Kong Special Administrative Region & InnoHK.
Conflict of interest
The authors declare that the research was conducted in the absence of any commercial or financial relationships that could be construed as a potential conflict of interest.
Publisher’s note
All claims expressed in this article are solely those of the authors and do not necessarily represent those of their affiliated organizations, or those of the publisher, the editors and the reviewers. Any product that may be evaluated in this article, or claim that may be made by its manufacturer, is not guaranteed or endorsed by the publisher.
Supplementary material
The Supplementary Material for this article can be found online at: https://www.frontiersin.org/articles/10.3389/fnins.2023.1119200/full#supplementary-material
Footnotes
- ^ http://getdata-graph-digitizer.com/
- ^ Note that some studies included more than one visual functions. Hence, the sum of the total percentage exceeds 100%.
References
Abuleil, D., McCulloch, D., and Thompson, B. (2021). Visual cortex cTBS increases mixed percept duration while a-tDCS has no effect on binocular rivalry. PLoS One 16:e0239349. doi: 10.1371/journal.pone.0239349
Antal, A., Kincses, T., Nitsche, M., Bartfai, O., and Paulus, W. (2004a). Excitability changes induced in the human primary visual cortex by transcranial direct current stimulation: direct electrophysiological evidence. Invest. Ophthalmol. Vis. Sci. 45, 702–707. doi: 10.1167/iovs.03-0688
Antal, A., Nitsche, M., Kincses, T., Kruse, W., Hoffmann, K., and Paulus, W. (2004b). Facilitation of visuo-motor learning by transcranial direct current stimulation of the motor and extrastriate visual areas in humans. Eur. J. Neurosci. 19, 2888–2892. doi: 10.1111/j.1460-9568.2004.03367.x
Antal, A., Nitsche, M., Kruse, W., Kincses, T., Hoffmann, K., and Paulus, W. (2004c). Direct current stimulation over V5 enhances visuomotor coordination by improving motion perception in humans. J. Cogn. Neurosci. 16, 521–527. doi: 10.1162/089892904323057263
Bachtiar, V., Johnstone, A., Berrington, A., Lemke, C., Johansen-Berg, H., Emir, U., et al. (2018). Modulating regional motor cortical excitability with noninvasive brain stimulation results in neurochemical changes in bilateral motor cortices. J. Neurosci. 38, 7327–7336. doi: 10.1523/JNEUROSCI.2853-17.2018
Bachtiar, V., Near, J., Johansen-Berg, H., and Stagg, C. (2015). Modulation of GABA and resting state functional connectivity by transcranial direct current stimulation. eLife 4:e08789. doi: 10.7554/eLife.08789
Barbieri, M., Negrini, M., Nitsche, M., and Rivolta, D. (2016). Anodal-tDCS over the human right occipital cortex enhances the perception and memory of both faces and objects. Neuropsychologia 81, 238–244. doi: 10.1016/j.neuropsychologia.2015.12.030
Battaglini, L., Contemori, G., Penzo, S., and Maniglia, M. (2020a). tRNS effects on visual contrast detection. Neurosci. Lett. 717:134696. doi: 10.1016/j.neulet.2019.134696
Battaglini, L., Mena, F., and Casco, C. (2020b). Improving motion detection via anodal transcranial direct current stimulation. Restor. Neurol. Neurosci. 38, 395–405. doi: 10.3233/RNN-201050
Battaglini, L., Mena, F., Ghiani, A., Casco, C., Melcher, D., and Ronconi, L. (2020c). The effect of alpha tACS on the temporal resolution of visual perception. Front. Psychol. 11:1765. doi: 10.3389/fpsyg.2020.01765
Battaglini, L., Noventa, S., and Casco, C. (2017). Anodal and cathodal electrical stimulation over V5 improves motion perception by signal enhancement and noise reduction. Brain Stimul. 10, 773–779. doi: 10.1016/j.brs.2017.04.128
Battleday, R., Muller, T., Clayton, M., and Cohen Kadosh, R. (2014). Mapping the mechanisms of transcranial alternating current stimulation: a pathway from network effects to cognition. Front. Psychiatry 5:162. doi: 10.3389/fpsyt.2014.00162
Behrens, J., Kraft, A., Irlbacher, K., Gerhardt, H., Olma, M., and Brandt, S. (2017). Long-lasting enhancement of visual perception with repetitive noninvasive transcranial direct current stimulation. Front. Cell Neurosci. 11:238. doi: 10.3389/fncel.2017.00238
Bocci, T., Nasini, F., Caleo, M., Restani, L., Barloscio, D., Ardolino, G., et al. (2018). Unilateral application of cathodal tDCS reduces transcallosal inhibition and improves visual acuity in amblyopic patients. Front. Behav. Neurosci. 12:109. doi: 10.3389/fnbeh.2018.00109
Bohotin, V., Fumal, A., Vandenheede, M., Bohotin, C., and Schoenen, J. (2003). Excitability of visual V1-V2 and motor cortices to single transcranial magnetic stimuli in migraine: a reappraisal using a figure-of-eight coil. Cephalalgia 23, 264–270. doi: 10.1046/j.1468-2982.2003.00475.x
Bonder, T., Gopher, D., and Yeshurun, Y. (2018). The joint effects of spatial cueing and transcranial direct current stimulation on visual acuity. Front. Psychol. 9:159. doi: 10.3389/fpsyg.2018.00159
Cabral-Calderin, Y., Schmidt-Samoa, C., and Wilke, M. (2015). Rhythmic gamma stimulation affects bistable perception. J. Cogn. Neurosci. 27, 1298–1307. doi: 10.1162/jocn_a_00781
Campana, G., Camilleri, R., Moret, B., Ghin, F., and Pavan, A. (2016). Opposite effects of high- and low-frequency transcranial random noise stimulation probed with visual motion adaptation. Sci. Rep. 6:38919. doi: 10.1038/srep38919
Chaieb, L., Antal, A., and Paulus, W. (2008). Gender-specific modulation of short-term neuroplasticity in the visual cortex induced by transcranial direct current stimulation. Vis. Neurosci. 25, 77–81. doi: 10.1017/S0952523808080097
Chen, G., Zhu, Z., He, Q., and Fang, F. (2021). Offline transcranial direct current stimulation improves the ability to perceive crowded targets. J. Vis. 21:1. doi: 10.1167/jov.21.2.1
Clavagnier, S., Thompson, B., and Hess, R. (2013). Long lasting effects of daily theta burst rTMS sessions in the human amblyopic cortex. Brain Stimul. 6, 860–867. doi: 10.1016/j.brs.2013.04.002
Contemori, G., Trotter, Y., Cottereau, B., and Maniglia, M. (2019). tRNS boosts perceptual learning in peripheral vision. Neuropsychologia 125, 129–136. doi: 10.1016/j.neuropsychologia.2019.02.001
Costa, T., Costa, M., Magalhaes, A., Rego, G., Nagy, B., Boggio, P., et al. (2015a). The role of early stages of cortical visual processing in size and distance judgment: a transcranial direct current stimulation study. Neurosci. Lett. 588, 78–82. doi: 10.1016/j.neulet.2014.12.055
Costa, T., Gualtieri, M., Barboni, M., Katayama, R., Boggio, P., and Ventura, D. (2015b). Contrasting effects of transcranial direct current stimulation on central and peripheral visual fields. Exp. Brain Res. 233, 1391–1397. doi: 10.1007/s00221-015-4213-0
Costa, T., Hamer, R., Nagy, B., Barboni, M., Gualtieri, M., Boggio, P., et al. (2015c). Transcranial direct current stimulation can selectively affect different processing channels in human visual cortex. Exp. Brain Res. 233, 1213–1223. doi: 10.1007/s00221-015-4199-7
Costa, T., Nagy, B., Barboni, M., Boggio, P., and Ventura, D. (2012). Transcranial direct current stimulation modulates human color discrimination in a pathway-specific manner. Front. Psychiatry 3:78. doi: 10.3389/fpsyt.2012.00078
Ding, Z., Li, J., Spiegel, D., Chen, Z., Chan, L., Luo, G., et al. (2016). The effect of transcranial direct current stimulation on contrast sensitivity and visual evoked potential amplitude in adults with amblyopia. Sci. Rep. 6:19280. doi: 10.1038/srep19280
Dong, G., Wang, Y., and Chen, X. (2020). Anodal occipital tDCS enhances spontaneous alpha activity. Neurosci. Lett. 721:134796. doi: 10.1016/j.neulet.2020.134796
Downs, S., and Black, N. (1998). The feasibility of creating a checklist for the assessment of the methodological quality both of randomised and non-randomised studies of health care interventions. J. Epidemiol. Commun. Health 52, 377–384. doi: 10.1136/jech.52.6.377
Fertonani, A., Pirulli, C., and Miniussi, C. (2011). Random noise stimulation omproves neuroplasticity in perceptual learning. J. Neurosci. 31, 15416–15423. doi: 10.1523/JNEUROSCI.2002-11.2011
Grabner, R., Krenn, J., Fink, A., Arendasy, M., and Benedek, M. (2018). Effects of alpha and gamma transcranial alternating current stimulation (tACS) on verbal creativity and intelligence test performance. Neuropsychologia 118, 91–98. doi: 10.1016/j.neuropsychologia.2017.10.035
Guleken, Z., Eskikurt, G., and Karamursel, S. (2020). Investigation of the effects of transcranial direct current stimulation and neurofeedback by continuous performance test. Neurosci. Lett. 716:134648. doi: 10.1016/j.neulet.2019.134648
Hallett, M. (2000). Transcranial magnetic stimulation and the human brain. Nature 406, 147–150. doi: 10.1038/35018000
Hansen, B., Richard, B., Andres, K., Johnson, A., Thompson, B., and Essock, E. A. (2015). A cortical locus for anisotropic overlay suppression of stimuli presented at fixation. Vis. Neurosci. 32:E023. doi: 10.1017/S0952523815000255
Hara, T., Shanmugalingam, A., McIntyre, A., and Burhan, A. (2021). Evidence for NIBS in facilitating rehabilitation of cognitive function after stroke. Brain Stimulation 14:1716. doi: 10.1016/j.brs.2021.10.420
He, Q., Lin, B., Zhao, J., Shi, Y., Yan, F., and Huang, C. (2019). No effects of anodal transcranial direct current stimulation on contrast sensitivity function. Restor. Neurol. Neurosci. 37, 109–118. doi: 10.3233/RNN-180881
Heinrichs-Graham, E., McDermott, T., Mills, M., Coolidge, N., and Wilson, T. (2017). Transcranial direct-current stimulation modulates offline visual oscillatory activity: a magnetoencephalography study. Cortex 88, 19–31. doi: 10.1016/j.cortex.2016.11.016
Higgins, J., Thompson, S., Deeks, J., and Altman, D. (2003). Measuring inconsistency in meta-analyses. Br. Med. J. 327, 557–560. doi: 10.1136/bmj.327.7414.557
Hoy, K., Bailey, N., Arnold, S., Windsor, K., John, J., Daskalakis, Z., et al. (2015). The effect of gamma-tACS on working memory performance in healthy controls. Brain Cogn. 101, 51–56. doi: 10.1016/j.bandc.2015.11.002
Hozo, S., Djulbegovic, B., and Hozo, I. (2005). Estimating the mean and variance from the median, range, and the size of a sample. BMC Med. Res. Methodol. 5:13. doi: 10.1186/1471-2288-5-13
Huang, Y., Edwards, M., Rounis, E., Bhatia, K., and Rothwell, J. (2005). Theta burst stimulation of the human motor cortex. Neuron 45, 201–206. doi: 10.1016/j.neuron.2004.12.033
Hunter, M., Coffman, B., Gasparovic, C., Calhoun, V., Trumbo, M., and Clark, V. (2015). Baseline effects of transcranial direct current stimulation on glutamatergic neurotransmission and large-scale network connectivity. Brain Res. 1594, 92–107. doi: 10.1016/j.brainres.2014.09.066
Kim, D., Kim, E., Lee, C., and Im, C. (2019). Can anodal transcranial direct current stimulation increase steady-state visual evoked potential responses? J. Korean Med. Sci. 34:e285. doi: 10.3346/jkms.2019.34.e285
Kraft, A., Roehmel, J., Olma, M., Schmidt, S., Irlbacher, K., and Brandt, S. (2010). Transcranial direct current stimulation affects visual perception measured by threshold perimetry. Exp. Brain Res. 207, 283–290. doi: 10.1007/s00221-010-2453-6
Laczo, B., Antal, A., Niebergall, R., Treue, S., and Paulus, W. (2012). Transcranial alternating stimulation in a high gamma frequency range applied over V1 improves contrast perception but does not modulate spatial attention. Brain Stimul. 5, 484–491. doi: 10.1016/j.brs.2011.08.008
Larcombe, S., Kennard, C., O’Shea, J., and Bridge, H. (2019). No effect of anodal transcranial direct current stimulation (tDCS) over hMT plus on motion perception learning. Front. Neurosci. 12:1044. doi: 10.3389/fnins.2018.01044
Lau, C., Tseng, L., Walsh, V., and Hsu, T. (2021). Revisiting the effects of transcranial direct current stimulation on pattern-reversal visual evoked potentials. Neurosci. Lett. 756:135983. doi: 10.1016/j.neulet.2021.135983
Liew, S., Santarnecchi, E., Buch, E., and Cohen, L. (2014). Non-invasive brain stimulation in neurorehabilitation: local and distant effects for motor recovery. Front. Hum. Neurosci. 8:378. doi: 10.3389/fnhum.2014.00378
Martin, D., Moffa, A., Nikolin, S., Bennabi, D., Brunoni, A., Flannery, W., et al. (2018). Cognitive effects of transcranial direct current stimulation treatment in patients with major depressive disorder: an individual patient data meta-analysis of randomised, sham-controlled trials. Neurosci. Biobehav. Rev. 90, 137–145. doi: 10.1016/j.neubiorev.2018.04.008
Michael, N., Gosling, M., Reutemann, M., Kersting, A., Heindel, W., Arolt, V., et al. (2003). Metabolic changes after repetitive transcranial magnetic stimulation (rTMS) of the left prefrontal cortex: a sham-controlled proton magnetic resonance spectroscopy (H-1 MRS) study of healthy brain. Eur. J. Neurosci. 17, 2462–2468. doi: 10.1046/j.1460-9568.2003.02683.x
Miniussi, C., Harris, J., and Ruzzoli, M. (2013). Modelling non-invasive brain stimulation in cognitive neuroscience. Neurosci. Biobehav. Rev. 37, 1702–1712. doi: 10.1016/j.neubiorev.2013.06.014
Moliadze, V., Antal, A., and Paulus, W. (2010). Boosting brain excitability by transcranial high frequency stimulation in the ripple range. J. Physiol. 588, 4891–4904. doi: 10.1113/jphysiol.2010.196998
Moreno, L., Senra, H., Lewis, P., Moreno, N., Linhares, J., Santana, R., et al. (2020). Cost-effectiveness of basic vision rehabilitation (The basic VRS-effect study): study protocol for a randomised controlled trial. Ophthalmic Physiol. Opt. 40, 350–364. doi: 10.1111/opo.12665
Nakazono, H., Ogata, K., Takeda, A., Yamada, E., Kimura, T., and Tobimatsu, S. (2020). Transcranial alternating current stimulation of α but not β frequency sharpens multiple visual functions. Brain Stimul. 13, 343–352. doi: 10.1016/j.brs.2019.10.022
Nitsche, M., and Paulus, W. (2000). Excitability changes induced in the human motor cortex by weak transcranial direct current stimulation. J. Physiol. 527, 633–639. doi: 10.1111/j.1469-7793.2000.t01-1-00633.x
Nitsche, M., and Paulus, W. (2001). Sustained excitability elevations induced by transcranial DC motor cortex stimulation in humans. Neurology 57, 1899–1901. doi: 10.1212/WNL.57.10.1899
Nitsche, M., Nitsche, M., Klein, C., Tergau, F., Rothwell, J., and Paulus, W. (2003). Level of action of cathodal DC polarisation induced inhibition of the human motor cortex. Clin. Neurophysiol. 114, 600–604. doi: 10.1016/S1388-2457(02)00412-1
Olma, M., Dargie, R., Behrens, J., Kraft, A., Irlbacher, K., Fahle, M., et al. (2013). Long-term effects of serial anodal tDCS on motion perception in subjects with occipital stroke measured in the unaffected visual hemifield. Front. Hum. Neurosci. 7:314. doi: 10.3389/fnhum.2013.00314
Page, M., McKenzie, J., Bossuyt, P., Boutron, I., Hoffmann, T., Mulrow, C., et al. (2021). The PRISMA 2020 statement: an updated guideline for reporting systematic reviews. Int. J. Surg. 88:105906. doi: 10.1016/j.ijsu.2021.105906
Pascual-Leone, A., Tormos, J., Keenan, J., Tarazona, F., Canete, C., and Catala, M. (1998). Study and modulation of human cortical excitability with transcranial magnetic stimulation. J. Clin. Neurophysiol. 15, 333–343. doi: 10.1097/00004691-199807000-00005
Pascualleone, A., Vallssole, J., Wassermann, E., and Hallett, M. (1994). Responses to rapid-rate transcranial magnetic stimulation of the human motor cortex. Brain 117, 847–858. doi: 10.1093/brain/117.4.847
Peters, M., Thompson, B., Merabet, L., Wu, A., and Shams, L. (2013). Anodal tDCS to V1 blocks visual perceptual learning consolidation. Neuropsychologia 51, 1234–1239. doi: 10.1016/j.neuropsychologia.2013.03.013
Pirulli, C., Fertonani, A., and Miniussi, C. (2013). The role of timing in the induction of neuromodulation in perceptual learning by transcranial electric stimulation. Brain Stimul. 6, 683–689. doi: 10.1016/j.brs.2012.12.005
Plow, E., Obretenova, S., Fregni, F., Pascual-Leone, A., and Merabet, L. (2012). Comparison of visual field training for hemianopia with active versus sham transcranial direct cortical stimulation. Neurorehabil. Neural Repair. 26, 616–626. doi: 10.1177/1545968311431963
Ranieri, F., Coppola, G., Musumeci, G., Capone, F., Di Pino, G., Parisi, V., et al. (2019). Evidence for associative plasticity in the human visual cortex. Brain Stimul. 12, 705–713. doi: 10.1016/j.brs.2019.01.021
Raveendran, R., Tsang, K., Tiwana, D., Chow, A., and Thompson, B. (2020). Anodal transcranial direct current stimulation reduces collinear lateral inhibition in normal peripheral vision. PLoS One 15:e0232276. doi: 10.1371/journal.pone.0232276
Reed, T., and Kadosh, R. (2018). Transcranial electrical stimulation (tES) mechanisms and its effects on cortical excitability and connectivity. J. Inherit. Metab. Dis. 41, 1123–1130. doi: 10.1007/s10545-018-0181-4
Reinhart, R., Xiao, W., McClenahan, L., and Woodman, G. (2016). Electrical stimulation of visual cortex can immediately improve spatial vision. Curr. Biol. 26, 1867–1872. doi: 10.1016/j.cub.2016.05.019
Richard, B., Johnson, A., Thompson, B., and Hansen, B. (2015). The effects of tDCS across the spatial frequencies and orientations that comprise the contrast sensitivity function. Front. Psychol. 6:1784. doi: 10.3389/fpsyg.2015.01784
Schaeffner, L., and Welchman, A. (2019). The mixed-polarity benefit of stereopsis arises in early visual cortex. J. Vis. 19:9. doi: 10.1167/19.2.9
Sczesny-Kaiser, M., Beckhaus, K., Dinse, H., Schwenkreis, P., Tegenthoff, M., and Hoffken, O. (2016). Repetitive transcranial direct current stimulation induced excitability changes of primary visual cortex and visual learning effects- a pilot study. Front. Behav. Neurosci. 10:116. doi: 10.3389/fnbeh.2016.00116
Somer, E., Allen, J., Brooks, J., Buttrill, V., and Javadi, A. (2020). Theta phase-dependent modulation of perception by concurrent transcranial alternating current stimulation and periodic visual stimulation. J. Cogn. Neurosci. 32, 1142–1152. doi: 10.1162/jocn_a_01539
Spiegel, D., Hansen, B., Byblow, W., and Thompson, B. (2012). Anodal transcranial direct current stimulation reduces psychophysically measured surround suppression in the human visual cortex. PLoS One 7:e36220. doi: 10.1371/journal.pone.0036220
Spiegel, D., Li, J., Hess, R., Byblow, W., Deng, D., Yu, M., et al. (2013). Transcranial direct current stimulation enhances recovery of stereopsis in adults with amblyopia. Neurotherapeutics 10, 831–839. doi: 10.1007/s13311-013-0200-y
Stagg, C., Bachtiar, V., and Johansen-Berg, H. (2011). The role of GABA in human motor learning. Curr. Biol. 21, 480–484. doi: 10.1016/j.cub.2011.01.069
Stagg, C., Best, J., Stephenson, M., O’Shea, J., Wylezinska, M., Kincses, Z., et al. (2009). Polarity-sensitive modulation of cortical neurotransmitters by transcranial stimulation. J. Neurosci. 29, 5202–5206. doi: 10.1523/JNEUROSCI.4432-08.2009
Terney, D., Chaieb, L., Moliadze, V., Antal, A., and Paulus, W. (2008). Increasing human brain excitability by transcranial high-frequency random noise stimulation. J. Neurosci. 28, 14147–14155. doi: 10.1523/JNEUROSCI.4248-08.2008
Thompson, B., Aaen-Stockdale, C., Koski, L., and Hess, R. F. (2009). A double dissociation between striate and extrastriate visual cortex for pattern motion perception revealed using rTMS. Hum. Brain Mapp. 30, 3115–3126. doi: 10.1002/hbm.20736
Thompson, B., Deblieck, C., Wu, A., Iacoboni, M., and Liu, Z. (2016). Psychophysical and rTMS evidence for the presence of motion opponency in human V5. Brain Stimul. 9, 876–881. doi: 10.1016/j.brs.2016.05.012
Thompson, B., Mansouri, B., Koski, L., and Hess, R. (2008). Brain plasticity in the adult: modulation of function in amblyopia with rTMS. Curr. Biol. 18, 1067–1071. doi: 10.1016/j.cub.2008.06.052
Valentine, J., Pigott, T., and Rothstein, H. (2010). How many studies do you need?: a primer on statistical power for meta-analysis. J. Educ. Behav. Stat. 35, 215–247. doi: 10.3102/1076998609346961
van Koningsbruggen, M., Ficarella, S., Battelli, L., and Hickey, C. (2016). Transcranial random-noise stimulation of visual cortex potentiates value-driven attentional capture. Soc. Cogn. Affect. Neurosci. 11, 1481–1488. doi: 10.1093/scan/nsw056
Van Meel, C., Daniels, N., Op de Beeck, H., and Baeck, A. (2016). Effect of tDCS on task relevant and irrelevant perceptual learning of complex objects. J. Vis. 16:13. doi: 10.1167/16.6.13
Waterston, M., and Pack, C. (2010). Improved discrimination of visual stimuli following repetitive transcranial magnetic stimulation. PLoS One 5:e10354. doi: 10.1371/journal.pone.0010354
Wu, D., Li, C., Liu, N., Xu, P., and Xiao, W. (2020). Visual motion perception improvements following direct current stimulation over V5 are dependent on initial performance. Exp. Brain Res. 238, 2409–2416. doi: 10.1007/s00221-020-05842-7
Wunder, S., Hunold, A., Fiedler, P., Schlegelmilch, F., Schellhorn, K., and Haueisen, J. (2018). Novel bifunctional cap for simultaneous electroencephalography and transcranial electrical stimulation. Sci. Rep. 8:7259. doi: 10.1038/s41598-018-25562-x
Keywords: non-invasive brain stimulation, visual function, meta-analyses, transcranial direct current stimulation, transcranial electrical stimulation, contrast sensitivity, visual evoked potentials, crowding
Citation: Bello UM, Wang J, Park ASY, Tan KWS, Cheung BWS, Thompson B and Cheong AMY (2023) Can visual cortex non-invasive brain stimulation improve normal visual function? A systematic review and meta-analysis. Front. Neurosci. 17:1119200. doi: 10.3389/fnins.2023.1119200
Received: 09 December 2022; Accepted: 13 February 2023;
Published: 02 March 2023.
Edited by:
Kevin Duffy, Dalhousie University, CanadaReviewed by:
Alexander Hunold, Technische Universität Ilmenau, GermanyTzu-Yu Hsu, Taipei Medical University, Taiwan
Copyright © 2023 Bello, Wang, Park, Tan, Cheung, Thompson and Cheong. This is an open-access article distributed under the terms of the Creative Commons Attribution License (CC BY). The use, distribution or reproduction in other forums is permitted, provided the original author(s) and the copyright owner(s) are credited and that the original publication in this journal is cited, in accordance with accepted academic practice. No use, distribution or reproduction is permitted which does not comply with these terms.
*Correspondence: Allen M. Y. Cheong, YWxsZW4ubXkuY2hlb25nQHBvbHl1LmVkdS5oaw==