- 1Department of Human Kinetics, St. Francis Xavier University, Antigonish, NS, Canada
- 2Department of Chemistry, St. Francis Xavier University, Antigonish, NS, Canada
- 3Department of Psychology, St. Francis Xavier University, Antigonish, NS, Canada
Lactate, the redox-balanced end product of glycolysis, travels within and between cells to fulfill an array of physiologic functions. While evidence for the centrality of this lactate shuttling in mammalian metabolism continues to mount, its application to physical bioenergetics remains underexplored. Lactate represents a metabolic “cul-de-sac,” as it can only re-enter metabolism by first being converted back to pyruvate by lactate dehydrogenase (LDH). Given the differential distribution of lactate producing/consuming tissues during metabolic stresses (e.g., exercise), we hypothesize that lactate shuttling vis-à-vis the exchange of extracellular lactate between tissues serves a thermoregulatory function, i.e., an allostatic strategy to mitigate the consequences of elevated metabolic heat. To explore this idea, the rates of heat and respiratory oxygen consumption in saponin-permeabilized rat cortical brain samples fed lactate or pyruvate were measured. Heat and respiratory oxygen consumption rates, and calorespirometric ratios were lower during lactate vs. pyruvate-linked respiration. These results support the hypothesis of allostatic thermoregulation in the brain with lactate.
Introduction
In mammals, most of the energy used to sustain life is derived from the oxidation of fatty acids, amino acids, and glucose by oxygen (O2) to form water and CO2 in the mitochondria. Because these oxidation reactions are exothermic, an organism will generate heat in proportion to its metabolic rate (Silva, 2006). Metabolic heat from aerobic respiration contributes to the maintenance of body temperature within a relatively narrow range (homeothermic endothermy) that is among the hallmarks of mammalian evolution (Crompton et al., 1978; Silva, 2006). However, during periods of elevated whole-body metabolic activity associated with intense or prolonged physical activity, this excess heat can contribute to the development of hyperthermia, necessitating the evolution of various heat-dissipation mechanisms to maintain thermal homeostasis (Brotherhood, 2008). In peripheral tissues such as skeletal muscles, metabolic heat can be dissipated through surface radiation and evaporative cooling (i.e., sweating), mediated largely by vascular convective heat transfer (González-Alonso, 2012). The more spherical geometry of the brain and its location within the cranial cavity makes it less conducive to dissipating heat via transcranial mechanisms (Nelson and Nunneley, 1998; Wang et al., 2014). Cerebral blood flow and volume are therefore considered the primary means of thermal regulation in the human brain (Nybo, 2012; Wang et al., 2014). However, the relatively small temperature gradient between the body core and the brain may limit the efficacy of this heat transfer mechanism during exercise in hot environments, especially in species like humans and rats which lack a carotid rete and the selective brain cooling it affords some other animals (Nybo, 2012). While some cooling is possible as blood travels along the carotid artery in humans, the transit time may be too short to allow for sufficient heat removal, even when artificial cooling is applied externally (Nybo, 2012). This lack of cooling is puzzling given that the brain is responsible for some 20% of the total oxygen consumption in humans at rest, despite constituting only 2% of body mass (Rolfe and Brown, 1997). Additionally, the brain has been shown to be very sensitive to changes in temperature; brain hyperthermia can be induced through a variety of physiological stressors such as bacterial infection/inflammation (Thompson et al., 2003) or recreational drug use (Brown and Kiyatkin, 2004; Kiyatkin et al., 2014), and has been implicated in the negative health outcomes associated with these conditions. At the same time, limiting oxygen to the brain for aerobic respiration quickly leads to brain damage or death of the organism (Sarkar et al., 2019). Given these considerations, understanding the mechanisms by which cerebral temperatures can be regulated is of particular concern to the field of neuroscience.
Some insight can be gleaned by considering the metabolic behavior of the brain itself. Conventional wisdom posits that the brain is an obligate glucose consumer under normal resting conditions (e.g., Hui et al., 2017; Dienel, 2019a). The human brain may release a small amount of lactate at rest, but during exercise, cerebral lactate uptake increases in proportion to the rise in arterial lactate concentration (Quistorff et al., 2008; Siebenmann et al., 2021), contributing to 27.8% of cerebral metabolic rate during maximal exercise (Smith and Ainslie, 2017). Thus, evidence generally supports that the brain possesses a substantial capacity to use lactate as a fuel (e.g., Smith et al., 2003; Gallagher et al., 2009; van Hall et al., 2009; Boumezbeur et al., 2010; Wyss et al., 2011; and as reviewed in Schurr, 2006). Anticipatory regulation [i.e., allostasis; (Sterling and Eyer, 1988)] is a logical strategy to guard against threats to homeostatic brain and body temperatures (Sterling, 2012). Mason (2017) convincingly argued a role for lactate and lactate shuttling in the context of neuroenergetic allostasis, allostatic load, and allostatic overload associated with injury and illness. Given the brain’s capacity to consume lactate, and the conditions in which muscle heat production and lactate levels increase (i.e., exercise), one might wonder whether lactate shuttling constitutes an allostatic means of mitigating cerebral metabolic heat production during intense or prolonged exercise in hot environments.
The early comment by Fletcher and Hopkins (1907) that “there is hardly any important fact concerning the lactic acid formation in muscle which, advanced by one observer, has not been contradicted by some other” echoes across the literature. Indeed, use of lactate as a fuel has largely supplanted its former reputation as a “dead-end” consequence of anaerobic metabolism and glycogenic precursor; and yet, prior misinterpretations of lactate metabolism carry an historical inertia against which evolving understandings must contend (e.g., Schurr, 2017; Brooks, 2018; Ferguson et al., 2018). In 1923, A.V. Hill and Otto Meyerhof split the Nobel Prize in Physiology or Medicine for discoveries relating to the production of heat in muscle (Hill), and the relationship between oxygen consumption and lactate metabolism in muscle (Meyerhof) (Barclay and Curtin, 2022). Hill’s work, carried out in isolated frog muscle, indicated that consumption of lactate by muscle cells yielded significantly less heat than would be expected for the complete combustion (oxidation) of lactate (Schurr, 2017). As noted by Schurr (2017), the prevailing interpretation of these results at the time was that most of the lactate produced during muscle contractions is utilized for glycogen resynthesis, with a minor fraction undergoing complete oxidation. This view has been largely revised for mammalian lactate metabolism vis-à-vis lactate shuttling (Brooks and Gaesser, 1980; Gaesser and Brooks, 1980; Miller et al., 2002a,b). As first conceived by Brooks (1985), a central tenet of the lactate shuttle hypothesis is that lactate travels within and between cells as an oxidizable fuel (Brooks, 2020). While evidence for the centrality of this concept of lactate-as-fuel in mammalian metabolism continues to mount (e.g., Hui et al., 2017), and the array of physiologic functions ascribed to lactate has only expanded (Brooks et al., 2022a), research examining the thermal bioenergetics of lactate oxidation remains comparatively sparse since the time of Hill and Meyerhof. The lactate shuttle may provide a pathway by which the “missing” heat noted by Hill may be reconciled by shuttling lactate out of the muscle and into the blood stream in the intact organism (Bassett Jr, 2002).
The present exploration is informed by two foundational assumptions: (1) lactate is effectively always the end-product of glycolysis (Rogatzki et al., 2015; Schurr, 2017); and (2) lactate diffuses across cell membranes via monocarboxylate transporters (MCTs) in proportion to its concentration gradient (Hertz and Dienel, 2005). Thus, we wondered if a thermoregulatory function might be ascribed to the metabolism of lactate, and measured rates of heat production and oxygen consumption in permeabilized samples of rat brain respiring with lactate versus pyruvate (+NAD+). The hypothesis being tested is summarized in Figure 1 and Tables 1, 2.
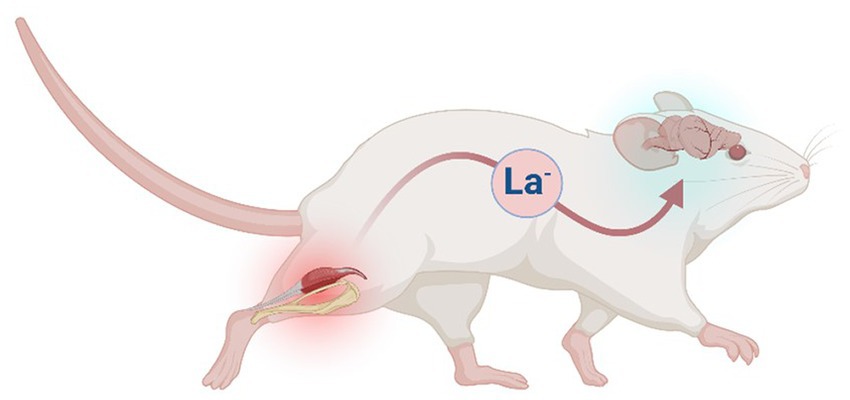
Figure 1. Hypothetical model in which the lactate shuttle serves a thermoregulatory function in mammals. In the example depicted (mouse), excess lactate (La−) released by skeletal muscle during periods of elevated glycolysis (e.g., exercise) is shuttled to the brain via circulating blood. In the model, metabolism of La− of extracellular origin is accompanied by a lower rate of heat production. Figure created with BioRender.com.
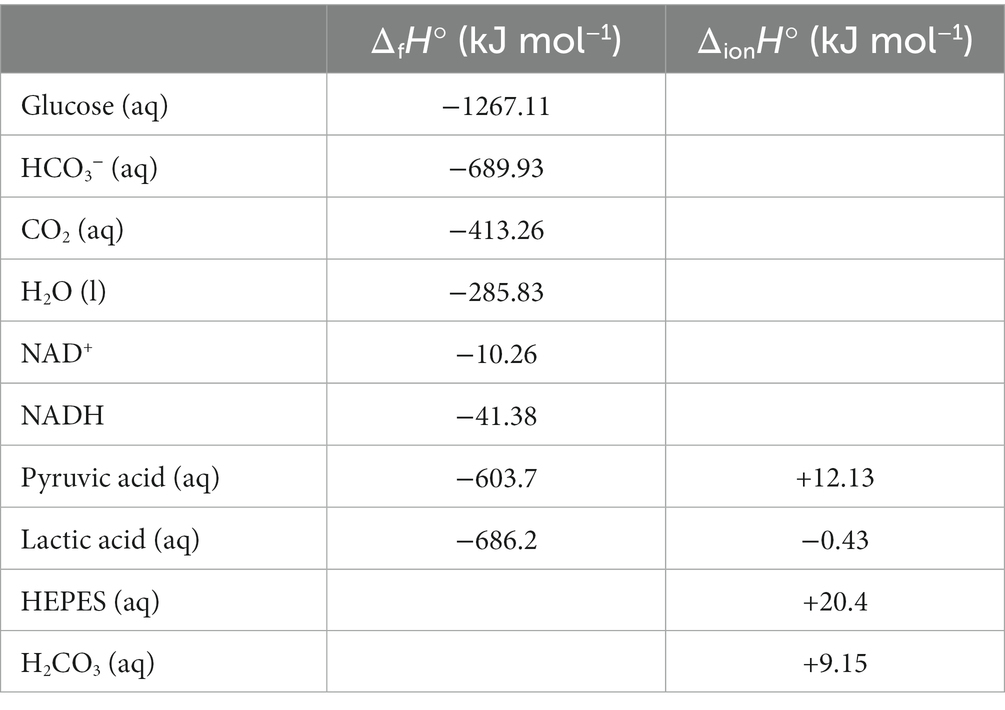
Table 1. Standard enthalpies of formation (ΔfH°) and ionization (ΔionH°) associated with complete lactate and pyruvate oxidation, as reported in Miller and Smith-Magowan (1990), Goldberg et al. (2002), and Hammes and Hammes-Schiffer (2015).
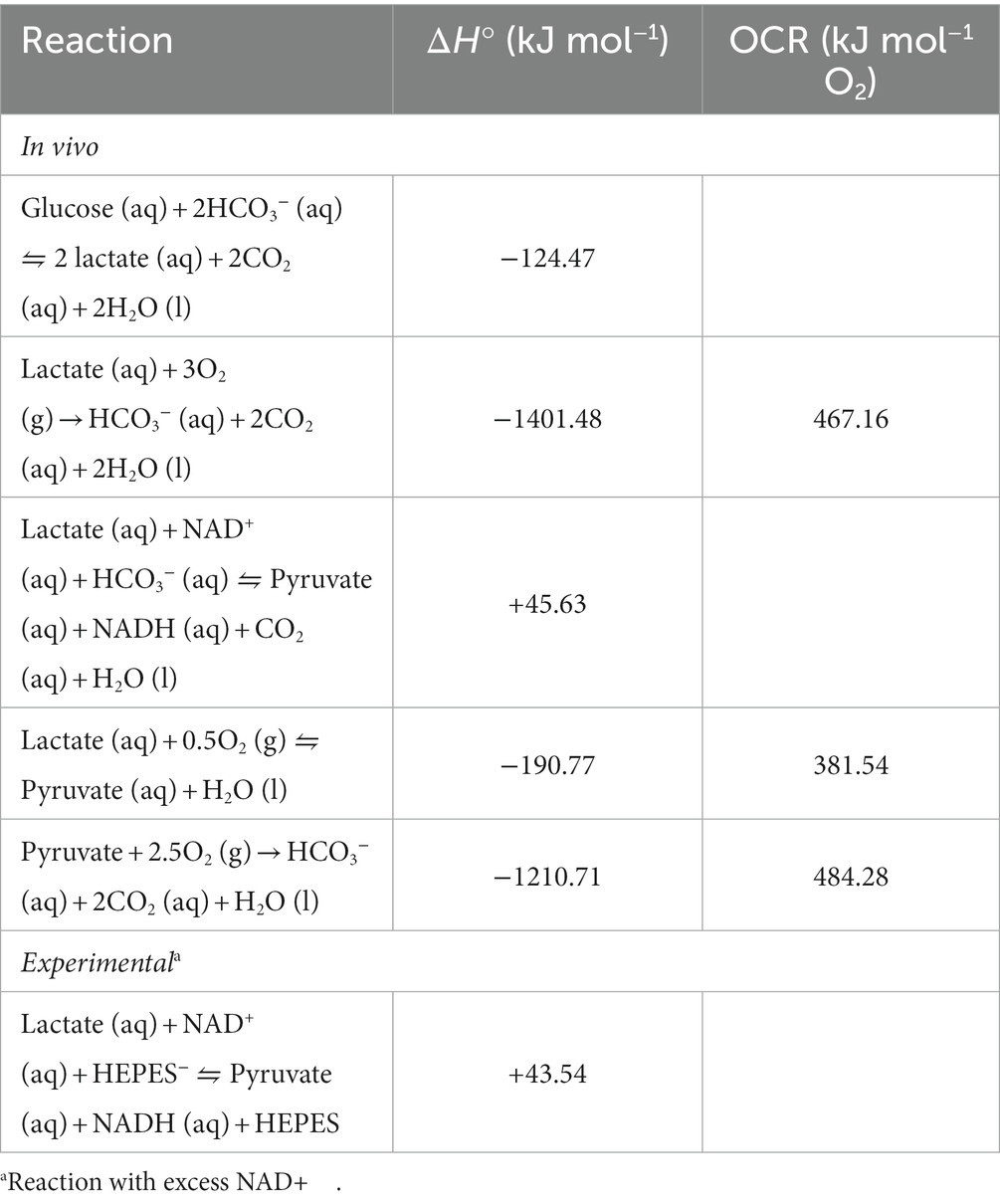
Table 2. Calculated standard reaction enthalpy changes (ΔH°) and oxycaloric ratios (OCR) for reactions in skeletal muscle and brain.
Scott and Kemp (2005) tested whether mitochondrial lactate oxidation could function as a heat sink in the manner described above. Using calorimetry and oxygen consumption measurements in parallel, they determined the calorespirometric (CR) ratio (kJ mol−1 O2) during either lactate- or pyruvate-supported respiration in permeabilized pEF TB/C3 hybridoma cells and mouse myocardial fibers. They found the CR ratio with lactate was the same as that with pyruvate (Scott and Kemp, 2005). Unfortunately, they did not report either heat or oxygen consumption rates. Also, Scott and Kemp (2005) did not include the cofactor for the L-lactate dehydrogenase (LDH) reaction, NAD+, the non-mitochondrial matrix portions of which may be lost with permeabilized tissues and cells. Here, we measured rates of heat and oxygen consumption in permeabilized samples of rat brain respiring with lactate versus pyruvate (+NAD+). In contrast to Scott and Kemp (2005), we find that the CR ratio is lower in brain tissue respiring with lactate. These results are discussed from both mechanistic and teleological perspectives.
Methods
Animals and reagents
Six male Wistar rats were purchased from Charles River Laboratories (Montreal, Canada) and acclimated for two weeks in an on-site facility with temperature control and reversed light:dark cycle prior to experiments. These rats were doubly housed in cages with access to running wheels, standard chow, and water ad libitum. On the day of the experiment, the rats were weighed (average body mass: 331 ± 36 g), and anesthetized via an intraperitoneal injection of 250 mg/ml sodium pentobarbital (Vetoquinol, QC, Canada). All other chemicals, including sodium L-lactate and sodium pyruvate were purchased from MilliporeSigma. Under deep anesthesia, the rats were decapitated, and their brains immediately dissected using blade and large rat brain anatomical matrix (1.0 mm Coronal sections, Zivic Instruments, Pittsburgh, United States). Dissected tissues were prepared for analysis as previously described (Herbst and Holloway, 2015; Herbst et al., 2018). All procedures in this study were approved by the St. Francis Xavier University Animal Care Committee, and conformed to the standards of the Canadian Council on Animal Care.
Heat production and oxygen consumption rate measurements
Following dissection, small (<35 mg) samples of frontal cortex were weighed, and then added to either the isothermal titration microcalorimeter (ITC; Model 4,200, Calorimetry Sciences Corporation), or the Oxygraph O2k high-resolution respirometer (O2k; OROBOROS Instruments, Innsbruck). The chambers of both instruments contained 1 ml (ITC) or 1.5 ml (O2k) of MiR05 mitochondrial respiration buffer (Pesta and Gnaiger, 2012), to which the following had been added: 20 mM creatine, 50 μg/mL saponin, 4 mM malate, 2 mM NAD+, and 5 mM ADP. The ITC and O2k protocols were performed in parallel, at 25°C, and with continual stirring. Following relative stabilization of the ITC heat rate trace, either L-lactate or pyruvate (10 mM final concentration) was injected into the chambers of each instrument. Due to the time required for the ITC to stabilize, measurements were made at 25°C (as opposed to 37°C, for example) and only one experimental substrate could reliably be tested per sample, due to inevitable oxygen limitations apparent at the end of each trial, as indicated in the O2k. Heat rate (dQ/dt) data were expressed as μJ s−1 mg−1 wet weight. Respiratory oxygen consumption rate (JO2) data were expressed as pmol s−1 mg−1 wet weight. dQ/dt and JO2 data were processed in parallel to give the calorespirometric (CR) ratio (kJ mol−1 O2), according to Skolik et al. (2018).
Analysis
Data are mean ± SEM and analyzed using Graphpad Prism (version 9.5.0) software. dQ/dt, JO2, and the CR ratio with lactate were compared to those with pyruvate using independent student’s t-test (α-level = 0.05).
Results
To explore the hypothesis that the metabolic heat associated with lactate versus pyruvate oxidation differs, the rates of heat (dQ/dt) and respiratory oxygen consumption (JO2) in saponin-permeabilized rat cortical brain samples fed lactate or pyruvate were measured.
The results in Figure 2 show the dQ/dt, JO2, and CR ratio in brain tissue are all significantly lower in the presence of excess lactate from an external source. The dQ/dt with lactate (Figure 2A) is lower by about a factor of 3 and the JO2 is lower by about a factor of 2 compared with the experiment with pyruvate. The measured CR ratio for pyruvate (564.1 ± 59.6 kJ mol−1 O2; Figure 2) is in reasonable agreement with its oxycaloric equivalent (484.28 kJ mol−1 O2; Table 2), but the CR ratio for lactate is markedly lower (283.7 ± 25.3 kJ mol−1 O2; Figure 2) than its oxycaloric equivalent (467.16 kJ mol−1 O2; Table 2). However, we must consider: is a similar reduction in metabolic heat rate likely to occur in a living organism, or is this an artefact of the experimental conditions? The agreement between our pyruvate experimental values and both the theoretical oxycaloric equivalent and other literature sources suggest that this is not the case.
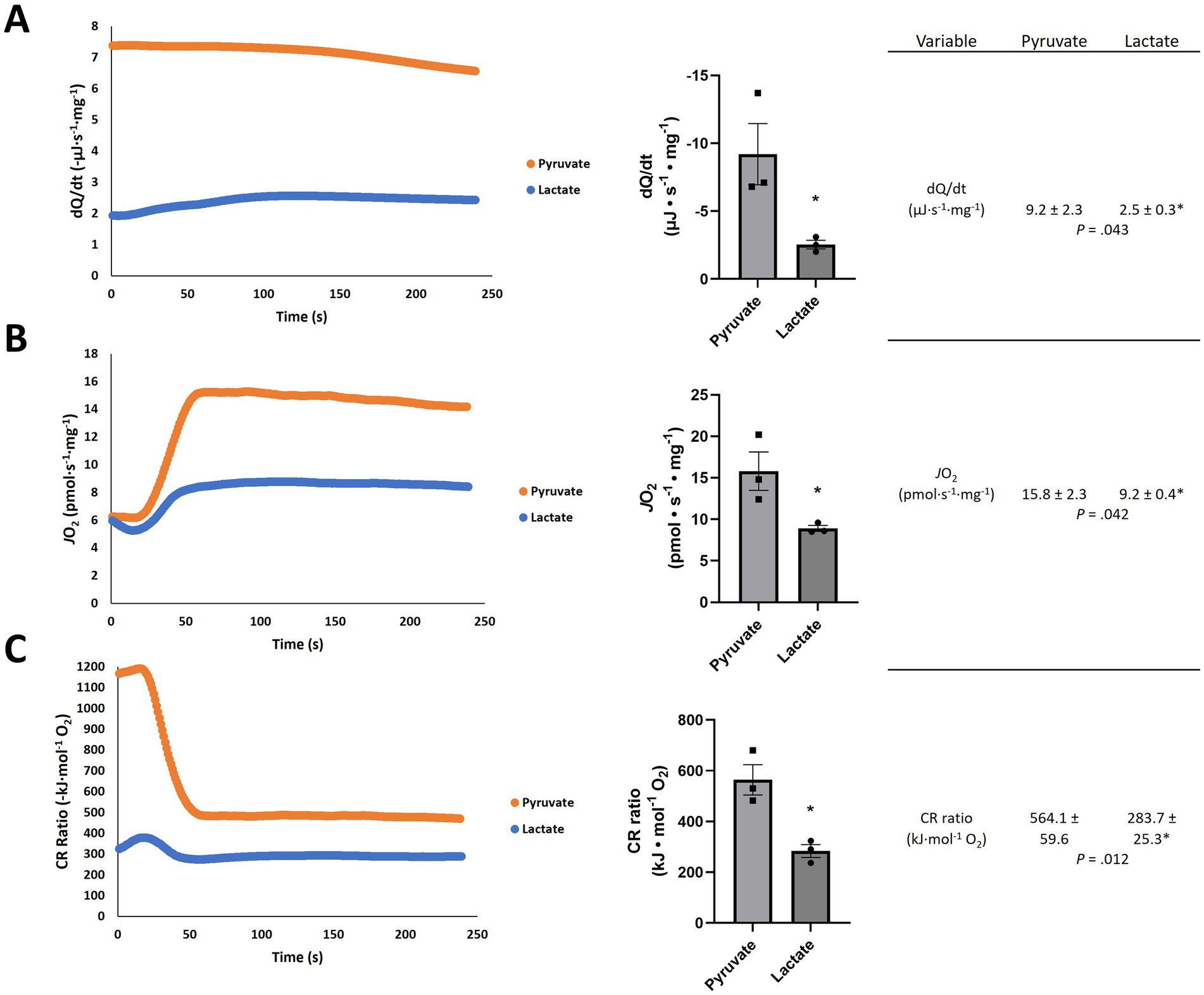
Figure 2. Representative traces of (A) heat rate (dQ/dt), (B) oxygen consumption rate (JO2) and (C) calorespirometric (CR) ratio in permeabilized rat frontal cortex samples fed pyruvate or lactate + NAD+. Numeric values are mean ± SEM; n = 3; *p-values from t-tests with 6 independent trials.
Discussion
In the present exploration, we hypothesized a lower rate of heat production associated with mitochondrial lactate vs. pyruvate oxidation in the rat brain, reflective of a larger working model in which the metabolism of lactate serves a thermoregulatory function (Figure 1). Addition of lactate elicited lower dQ/dt and JO2 per mg of rat tissue when compared to an equimolar addition of pyruvate (Figure 2). Interestingly, the CR ratio with lactate (283.7 ± 25.3 kJ mol−1 O2) fell below both the theoretical oxycaloric equivalent predicted from Tables 1, 2 (467.16 kJ mol−1 O2) and that predicted by Thornton’s rule [455 ± 15 kJ mol−1 O2 (Hansen et al., 2004)].
Thornton’s Rule states that the heat of combustion of organic compounds per mole of O2 is relatively constant (Thornton, 1917). This rule implies that as a cell system moves toward 100% aerobic metabolism, the CR ratio will approach the theoretical oxycaloric constant for the particular cell line or tissue type and substrate source (Skolik et al., 2018); published oxycaloric constants therefore tend to range between 430 to 480 kJ mol−1 (Gnaiger and Kemp, 1990; Kemp, 2000). In the context of calorespirometry in a cell catabolizing glucose under aerobic conditions, a CR ratio below the oxycaloric equivalent implies the products of glycolysis (i.e., lactate and/or pyruvate) are not fully oxidized to CO2 and water.
There are two ways to decrease metabolic heat rate: decrease the rate of the reaction(s) producing the heat, and/or alter the chemistry (e.g., by changing the conditions in which the reaction(s) occur). In our experiments, the O2 consumption rate is a direct measure of the complete oxidative metabolic rate in the permeabilized brain tissue, and the CR ratio indicates the reactions that are occurring. Using Thornton’s rule (oxycaloric equivalent; Table 2) and the measured O2 consumption rates (Figure 2), the predicted heat rate for pyruvate-fed brain tissue is 7.7 μJ s−1 mg−1 and for lactate-fed brain tissue it is 4.3 μJ s−1 mg−1. These results alone directly support the hypothesis, inasmuch as the complete oxidation of lactate, and thus predicted rate of heat is lower with lactate than pyruvate. The calculated heat rate and CR ratio for pyruvate agree with the measured heat rate and CR ratio, but the heat rate and CR ratio for lactate are lower than values predicted when applying the oxycaloric ratio (Table 2) to the oxygen consumption rate. To understand this disagreement between the observed and predicted values, and further develop the model, consider that the complete oxidation of lactate can be divided into three reactions, each with calculated standard reaction enthalpies (ΔHn; n = reaction):
Combining the three reactions (R), we can produce an overall equation describing the rate of heat generation:
where (R1), (R2), and (R3) are the rate of reactions (1)–(3), respectively. According to the principles of enzyme kinetics, the rate of reactions (1)–(3) depend on the concentrations of substrates (lactate, pyruvate, or NAD+), and the relative activities of LDH and the enzyme(s) that limit the rate of complete pyruvate and NADH oxidation. If the conversion of lactate to pyruvate (R1) is faster than oxidation removes pyruvate (R3), then pyruvate will build up and diffuse out of the cell. This gives rise to a decoupling between the rate of lactate consumption (R1), CO2 production (R3), and heat generation (Σ(R1 × ΔH1) (R2 × ΔH2) (R3 × ΔH3)). However, in the experiments carried out in the present work, if the reaction (R1) occurs outside of the matrix, it becomes:
If we assume negligible NAD+ regeneration in the experiment, the combined (R5) and (R3) is:
Because both the predicted dQ/dt (4.3 μJ s−1 mg−1) from the measured JO2 via Thornton’s Rule and the oxycaloric equivalent for (R6) (466.87 kJ mol−1 O2) exceed the measured values for both, incomplete lactate oxidation involving a decoupling of the rates of (R5) from (R3) is a plausible explanation. In the experimental conditions, if lactate is only oxidized by NAD+ to pyruvate (R5), but the NADH thus formed is reoxidized by oxygen (R2), the ΔH = −190.77 kJ mol−1 and the CR ratio is 381.54 kJ mol−1 O2. This is still well above our measured CR ratio for lactate (283.7 ± 25.3 kJ mol−1 O2). Therefore, it is reasonable to infer that appreciable lactate oxidation to pyruvate occurred (R5), with attenuated oxidation of the pyruvate (R3) and/or negligible reoxidation of NADH (R2) by oxygen. Assuming no NADH reoxidation in (R5) (i.e., 2.5O2 per lactate incompletely oxidized), the observed CR ratio with lactate (283.7 kJ mol−1 O2), ΔH3, and ΔH5 give a ratio of (R5) to (R3) equaling 11.5. In other words, the experimentally observed CR ratio with lactate is explained by a rate of (R5) exceeding (R3) by a factor of 11.5. Note that in the organism, NAD+ is only a redox cofactor in the catalysis of lactate oxidation to pyruvate, and the regeneration of NAD+ is ultimately due to O2 oxidation of NADH (R2) in vivo. Therefore, the LDH-catalyzed lactate oxidation to pyruvate in vivo (R1) would need to exceed the rates of complete pyruvate oxidation (R3) and NADH oxidation (R2) by a factor of 12.06 for the CR ratio to approach our observed 283.7 kJ mol−1 O2.
According to our hypothetical model, the dQ/dt during periods of net lactate oxidation depend on the relative activities of LDH and the enzyme(s) that limit the rate of pyruvate oxidation. If the conversion of lactate to pyruvate is faster than oxidation removes pyruvate, then pyruvate would be expected to build up and diffuse out of the cell. Note that L-lactate is likely a direct and/or indirect inhibitor of pyruvate oxidation (discussed below). Using human erythrocytes, which notably lack mitochondria, Minikami and de Verdier (1976) reported that the reaction catalyzed by LDH is the most enthalpic step in glycolysis. This led them to speculate that pyruvate, by virtue of its permeability to cell membranes, may be involved in the regulation of heat production in cell systems (Minikami and de Verdier, 1976). Here, we are suggesting that regulation of metabolic heat is accomplished via lactate of extracellular origin. However, with regard to the afore mentioned buildup of pyruvate predicted by a decoupling of (R1) and (R3), we are not aware of evidence supporting substantial cerebral pyruvate efflux in vivo, save for perhaps with hypoxia (Overgaard et al., 2012) or intravenous infusion of hypertonic sodium lactate (e.g., Bouzat et al., 2014). Another potential explanation for nonoxidative removal of pyruvate would be through carboxylation involving pyruvate carboxylase and/or malic enzyme activities (Hassel, 2000). However, we are unaware of reported enthalpies of pyruvate carboxylation. Investigating the potential role of pyruvate carboxylation in the current model are the anticipated objectives of follow-up work.
The CR ratio for pyruvate in the present work agrees with that found by Scott and Kemp (2005): 502 ± 15 kJ mol−1 O2 in permeabilized hybridoma cells and 506 ± 47 kJ mol−1 O2 in permeabilized cardiac muscle fibers. However, our CR ratio for brain tissue respiring with lactate is substantially lower than the Scott and Kemp (2005) values: 517 ± 89 kJ mol−1 O2 in permeabilized hybridoma cells and 502 ± 15 kJ mol−1 O2 in permeabilized cardiac muscle fibers for lactate oxidation. Note that both our work and Scott and Kemp (2005) used the same HEPES buffer, so that cannot account for any differences. Scott and Kemp (2005) did not include the cofactor for the LDH reaction, NAD+, which may be an issue, as lactate was likely oxidized to pyruvate by the 2 mM of NAD+ supplied in the present preparation. This reduces the ΔH from an exothermic −190.77 kJ mol−1 (ΔH2) if lactate were oxidized to pyruvate by O2 to an endothermic +43.54 kJ mol−1 (ΔH5). The agreement between the measured CR ratio and the calculated oxycaloric ratio for pyruvate shows that pyruvate was oxidized by oxygen, whereas the discrepancy between the experimental and predicted values for lactate imply that lactate may have been oxidized by NAD+ faster in the experiment. In living brain tissue, the dQ/dt is also expected to be from oxidation of lactate to pyruvate by O2 (via NADH). Thus, the measured heat rate in the present experiments may be disregarded when considering the heat rate produced by lactate oxidation to pyruvate in the brains of mammals. Instead, the fastest reaction in vivo is expected to be (R2) ( ); but an obvious consideration in vivo is lactate oxidation to pyruvate by NAD+. NADH produced by extra-matrix LDH in the oxidation of lactate would likely need to be shuttled into the mitochondria via the malate–aspartate shuttle (McKenna et al., 2006; Gellerich et al., 2012; Kane, 2014), or others for example (Passarella et al., 2021), for the expected heat of combustion with lactate to approach that of pyruvate. In the present experiments, it is doubtful that any substantial contribution by remnants of the malate–aspartate shuttle remains in the permeabilized samples tested.
Incomplete lactate oxidation implies potentially elevated NADH, which calls into question whether neurons or glia are even capable of accommodating such a redox shift under physiologic conditions. Large shifts in the NAD+/NADH ratio have been observed in neurons and astrocytes treated with high concentrations (>10 mM) of lactate (Wilhelm and Hirrlinger, 2011; Yang et al., 2014), reflecting elevated NADH, but not necessarily NAD+ concentrations in cells with multiple NAD+ producing/consuming activities. While the physiological accuracy of extremely large rises in NADH have been questioned (Dienel, 2019b), the observed increases in NADH with high lactate in brain cells suggests a capacity for these cells to accommodate redox shifts associated with incomplete lactate oxidation.
The most straightforward explanation for a low in vivo value of the CR ratio for lactate comes from the lactate shuttle. Lactate must first be converted to pyruvate prior to any further transformations. Incomplete lactate oxidation involving attenuated oxidation of the resulting NADH could, in theory, account for lower CR ratios. However, in an in vivo scenario in which the NADH formed in the oxidation of lactate to pyruvate is not reoxidized by O2 (R1), but pyruvate is completely oxidized (R3) reduces the CR ratio from complete lactate oxidation (Table 2) by only 1.13 kJ mol O2−1. Alternatively, a scenario in which lactate is only oxidized to pyruvate in vivo (R2), instead of to CO2 and water (Table 2), but the formed NADH is reoxidized by O2, reduces the heat produced by 85.62 kJ mol O2−1 consumed. And while neuronal Ca+2 levels, for example, may alter the activity of the malate–aspartate shuttle, (e.g., Contreras and Satrustegui, 2009; recently reviewed by del Arco et al., 2023), the magnitude of the reduction in lactate CR ratios observed in the current work suggests that pyruvate oxidation was affected.
Interestingly, it was reported recently that lactate partially inhibits mitochondrial respiration in permeabilized cardiac fibers from naked mole rats (Huynh and Pamenter, 2022). The inhibitory effect of lactate decreased respiration with either palmitoyl-carnitine (37%) or pyruvate (20%) (Huynh and Pamenter, 2022). On the other hand, heat acclimation has been found to increase the expression of mitochondrial LDH (LDHB; the isoform associated with the oxidation of lactate to pyruvate) in rat heart (Mreisat et al., 2020), which should, paradoxically, lead to increased pyruvate oxidation. In isolated rat brain mitochondria, L-lactate has been observed to partially inhibit (29%) mitochondrial respiration with pyruvate (Ling et al., 2012). This was suggested to possibly be the result of competitive inhibition of mitochondrial pyruvate transport, given lactate may share the same mitochondrial transporter (Ling et al., 2012), but it is noted also that Valenti et al. (2002) reported evidence of mitochondrial lactate/pyruvate antiport in rat heart mitochondria. Related to this, membrane potential-dependent enrichment of lactate in mitochondria was recently observed using a ratiometric lactate sensor (Li et al., 2023). Computational modeling of the pyruvate dehydrogenase (PDH) activity under simulated physiological conditions indicates that PDH is largely regulated through product inhibition by NADH (Jelinek and Moxley, 2021). Depending on the submitochondrial location of LDH and the activity of mitochondrial electron shuttles, the NADH formed in the LDH-catalyzed lactate oxidation to pyruvate may therefore be expected to inhibit PDH. Moreover, L-lactate-mediated release of Mg+2 from the endoplasmic reticulum and subsequent mitochondrial uptake was demonstrated in hepatocytes (Daw et al., 2020). Mitochondrial uptake of Mg+2 led to a marked inhibition of pyruvate-linked mitochondrial oxygen consumption rates (Daw et al., 2020). With low-concentration saponin-permeabilization of the plasma membrane, both the endoplasmic reticulum and mitochondrial membranes remain intact in muscle fibers (Saks et al., 1998). If a similar phenomenon occurs in our preparations (i.e., L-lactate-mediated inhibition of complete pyruvate oxidation), this could spell an appreciable divergence, or “decoupling,” in rates of LDH-catalyzed lactate oxidation to pyruvate and the complete oxidation of the resulting pyruvate. The net result of L-lactate—mediated inhibition of pyruvate oxidation with or without incompletely oxidized NADH formed via LDH is thus expected to result in a decrease in the CR ratio for lactate. Depending on the level of inhibition of complete pyruvate oxidation, oxidation of lactate to pyruvate would need to exceed the rate of mitochondrial NADH shuttling by a factor of about 11.06 (no inhibition of pyruvate oxidation), 8.11 (Ling et al., 2012), or 9.03 (Huynh and Pamenter, 2022) to reach sub-300 CR ratios in vivo.
Another potential factor worth mentioning is the experimental media component, lactobionate. It has been suggested that the 60 mM lactobionate of the standard MiR05 respiratory buffer media could inhibit mitochondrial L-lactate transport into the matrix (Passarella et al., 2014, 2021), which if true, could potentially explain our results. However, NAD+-dependent respiration with lactate has been observed in permeabilized brain, and skeletal muscle and cardiac fibers in media with (Jacobs et al., 2013; Herbst et al., 2018; Mahalingam et al., 2023) and without (Elustondo et al., 2013) lactobionate. Moreover, lactate-stimulated respiration in the absence of added NAD+ was not observed in a study using media without lactobionate to test permeabilized skeletal and cardiac fibers (Ponsot et al., 2005). In any case, we chose to recapitulate the experimental media conditions of Scott and Kemp (2005), which included 60 mM lactobionate. More recently, Lund et al. (2023) demonstrated the confounding effects of osmotic load and counterion (Na+) of sodium L-lactate in mice. We used pH-neutral aqueous sodium L-lactate in the present work but compared this to equimolar (10 mM) pH-neutral aqueous sodium pyruvate. Therefore, any potential osmolarity and/or counterion effects should be similar between the lactate and pyruvate conditions tested in the present work.
It is curious that Scott and Kemp (2005) failed to observe a difference in the CR ratios of permeabilized mouse myocardial fibers using either lactate or pyruvate substrates. In comparing the results from the present study with those of Scott and Kemp (2005) a key difference in experimental design is our inclusion of NAD+, and the tissue type investigated. Several previous studies report mitochondrial lactate oxidation in muscle, but only after adding NAD+ (Szczesna-Kaczmarek, 1990; Elustondo et al., 2013; Jacobs et al., 2013; Mahalingam et al., 2023). Added NAD+ should not be necessary for mitochondrial lactate oxidation if LDH resides in the mitochondrial matrix. The most recent version of the MitoCarta inventory of mitochondrial proteins in mouse and human lists a mitochondrial matrix location for L-lactate dehydrogenase in several tissues; the heart and skeletal muscle are not among them (http://www.broadinstitute.org/mitocarta; Rath et al., 2021). Alternatively, the mitochondrial lactate oxidation complex is proposed to directly couple the endergonic LDH-catalyzed oxidation of lactate to pyruvate outside of the matrix with the exergonic redox change in cytochrome c oxidase (complex IV of the respiratory chain) during mitochondrial electron transport (Hashimoto et al., 2006, 2008; see: Figure 1 in Hashimoto and Brooks, 2008). However, such a relationship should not influence the heats of reaction and cannot explain the current results beyond incomplete oxidation. To be clear, neither the physical nor functional submitochondrial location of LDH were explored in the current study. Recent discussion of the topic may be found elsewhere (Fulghum et al., 2019; Borst, 2020; Young et al., 2020; Glancy et al., 2021; Passarella et al., 2021; Brooks et al., 2022b). The fact that complete heats of combustion are released or dissipated as heat (i.e., catabolic steady-state) is bioenergetically independent of subcellular compartmentalization, per se. However, if subcellular compartmentalization alters the rates of reactions (e.g., by limiting substrate transport) involved in complete lactate oxidation, or alters the conditions in which the reaction(s) occurs (e.g., different buffering compounds in vs. outside the mitochondrial matrix), this would be expected to influence the heat rate. Therefore, a limitation to the current work is the use of standard enthalpies of formation and ionization to estimate reaction enthalpies, which can vary under non-standard conditions.
From a kinetic perspective, the difference in oxygen consumption rates between lactate and pyruvate suggests a level of regulation warranting further exploration in more physiological/pathophysiological contexts. Testing combined substrates, including lactate and pyruvate together in the ratios they are found in vivo for example [e.g., as with traumatic brain injury (Vespa et al., 2012)], may better define or refine the findings and ideas proffered in the current work. We thusly join Schurr (2017) in speculating about the possibility of “…controlled utilization” of lactate. Indeed, our heat and oxygen consumption data with lactate do support a more controlled utilization than equimolar pyruvate, and are hypothesized to contribute to observations in the intact organism (see Teleological Perspective below). Whether, or to what degree these phenomena contribute to physiology in vivo requires further research.
Teleological perspective
While extrapolating thermoregulatory responses in exercising rats to human thermal physiology has its potential limitations (Wanner et al., 2015), it is reasonable to expect that similar physical bioenergetics governs cerebral lactate metabolism in both species. In animals lacking a carotid rete such as rats and humans, there is no evidence for whole brain selective cooling, though whether rats possess regional selective brain cooling is controversial (Jessen, 2001). In exercising rats, coercive exercise to exhaustion in the heat can lead to death, coincident with elevated core body temperature (Fruth and Gisolfi, 1983), and core and/or brain hyperthermia appear to limit voluntary exercise with heat in rats (Fuller et al., 1998).
In humans, brain temperature is predominantly dictated by core body and arterial blood temperature (Nybo, 2012), and during prolonged exercise with hyperthermia, heat release from the brain remains inadequate (Nybo et al., 2002). While cerebral oxygenation due to blood flow increases during intense exercise (compared to rest), the cerebral metabolic ratio of O2/[glucose + ½lactate] decreases, a finding which has been interpreted to reflect declining complete oxidation, but not accumulation, of glucose and lactate taken up by the brain (Ide et al., 1999; Dalsgaard et al., 2004). Increasing non-oxidative or incomplete cerebral oxidative metabolism is therefore among the plausible explanations for these observations. Any shift towards incomplete vs. complete oxidative disposal of glucose and/or lactate in the brain would also reduce the rate of metabolic heat production. Incidentally, the rate at which exercising muscle breaks down its glycogen and releases lactate is exacerbated in hot environments (Fink et al., 1975; Febbraio et al., 1994; Parkin et al., 1999; Hargreaves, 2008); the mechanisms responsible are thought to include increased muscle temperatures and levels of circulating epinephrine (Hargreaves, 2008). Epinephrine is among the primary hormonal mediators of the stress response, and the dynamic regulation of its secretion is a central example of allostasis (McEwen, 2000). Allostasis, a term coined by Sterling and Eyer (1988) to refer to physiologic stability through variation, has been used to describe the brain-body involvement in budgeting resources in anticipation of biologic needs. Thus, allostasis serves homeostasis, a key parameter of which is stable core body temperature in homeotherms. If the release (e.g., muscle) and consumption (e.g., brain) of lactate during exercise in hot environments constitutes an allostatic means of anticipating and responding to thermal stress cues, then one would expect this to be reflected in training adaptations associated with acclimation to exercise in hot environments. Indeed, it is.
A decrease in metabolic heat production has been observed with acclimation to exercise in a hot environment (e.g., Sawka et al., 1983; Périard et al., 2015; Rivas et al., 2017). While the observed effects on metabolic heat production varies, additional metabolic adaptations may reflect the type of unevenly balanced metabolic heat production strategy hypothesized in the current work. These include a relatively small decrease in muscle glycogenolysis, and attenuated lactate accumulation (Périard et al., 2015). Because the typical increases in lactate threshold observed with endurance exercise training are thought to be more a result of augmented rates of lactate clearance (Donovan and Brooks, 1983; Donovan and Pagliassotti, 1989, 1990; MacRae et al., 1992; Phillips et al., 1995; Bergman et al., 1999; Messonnier et al., 2013), as opposed to its release, this suggests that acclimation to exercise in hot environments increases the rates of lactate clearance from circulation, in line with a metabolic thermoregulatory function for mitochondrial lactate oxidation proposed in the current work. Whether this is true, and if so, important, will require further research. It should also be noted that the most effective strategy to mitigate the hyperthermic effects of exercise in hot environments appears to be aerobic fitness itself (Alhadad et al., 2019), the development of which is well associated with increases in lactate threshold, and thus rate of lactate clearance.
The therapeutic use of intravenous (IV) infusion of lactate for type 2 diabetes, Alzheimer’s disease, cardiac disease, and traumatic brain injury (TBI) was recently reviewed (van Gemert et al., 2022). Of these, TBI patients, in particular, appear to benefit from IV lactate (Bouzat et al., 2013; Bouzat and Oddo, 2014; Gantner et al., 2014; Brooks and Martin, 2015; Carpenter et al., 2015). The mechanisms by which IV lactate improves long-term outcomes in patients with TBI are not fully understood, but it is interesting to note that systemic hyperthermia is a common occurrence after TBI and may even induce secondary brain injury (Svedung Wettervik et al., 2021). In light of the results of the current work, it is tempting to speculate about a role for IV lactate in mitigating the effects of hyperthermia associated with TBI due to the “cooler” thermo-neuroenergetics of lactate oxidation.
The export of lactate has been shown to play a vital role in tissues which require rapid activation such as fast-twitch glycolytic muscles or neurons following activation and neurotransmitter release vis-a-vis the Lactate Efflux model (Shulman et al., 2001). In both cases, glycolysis is used to meet transient spikes in energy demand. However, the TCA cycle is not initiated even in the presence of excess oxygen. A related phenomenon is observed in many cancer cells, which display an increased uptake of glucose and fermentation to lactate even in the presence of functional mitochondria and sufficient oxygen levels (i.e., the Warburg effect) (Liberti and Locasale, 2016). A recent investigation aimed to explore the Warburg effect from the perspective of metabolic thermogenesis. Using 13C-metabolic flux analysis of 12 cultured cancer cell lines combined with in silico metabolic simulations, Okahashi et al. (2023) present evidence that point to a reduction in metabolic heat production as a potential explanation for the increased reliance on glycolytic metabolism in cancer. In light of the evidence for lactate shuttling in cancer cells (e.g., Sonveaux et al., 2008; Goodwin et al., 2015) and the results of the present work, mitigating metabolic heat via intercellular lactate and/or pyruvate (Hong et al., 2016) exchange and metabolism among cancer cells with elevated demands for ATP seems plausible. It is noted that whole-body and localized-regional hyperthermia has long been used as an effective cancer treatment alone, or as an adjuvant therapy to traditional cancer treatments (Wust et al., 2002; Mallory et al., 2016), though a complete understanding of the basic mechanisms by which heat achieves its therapeutic effects is lacking (Lee et al., 2020).
In conclusion, we have presented evidence suggesting that the rate of heat production in lactate-fed mitochondria is intriguingly low in the mammalian brain. This is in accordance with a hypothetical working model in which the lactate shuttle serves a thermoregulatory function in the brain (Figure 1). To what extent this may contribute to the integrative thermoregulation of the intact organism awaits further investigation.
Data availability statement
The raw data supporting the conclusions of this article will be made available by the authors, without undue reservation.
Ethics statement
The animal study was reviewed and approved by St. Francis Xavier University Animal Care Committee.
Author contributions
DK, EN, and DM contributed to conception and design of the study. DK, AF, EN, KB, and DM were involved in data collection. DK, EN, and AF analyzed data. DK, AF and DM interpreted the results. DK and AF wrote the manuscript. KB and DM contributed to revisions. All authors contributed to the article and approved the submitted version.
Funding
This work was supported by NSERC Discovery Grant RGPIN-2015-04286 (DK) and Research NS Scotia Scholars 2022-2111 (EN).
Acknowledgments
The authors thank the helpful staff of the StFX Animal Care Facility. Our thanks also to the reviewers of this manuscript, one of whom in particular gave considerable helpful feedback and guidance.
Conflict of interest
The authors declare that the research was conducted in the absence of any commercial or financial relationships that could be construed as a potential conflict of interest.
Publisher’s note
All claims expressed in this article are solely those of the authors and do not necessarily represent those of their affiliated organizations, or those of the publisher, the editors and the reviewers. Any product that may be evaluated in this article, or claim that may be made by its manufacturer, is not guaranteed or endorsed by the publisher.
References
Alhadad, S. B., Tan, P. M., and Lee, J. K. (2019). Efficacy of heat mitigation strategies on core temperature and endurance exercise: a meta-analysis. Front. Physiol. 10:71. doi: 10.3389/fphys.2019.00071
Barclay, C. J., and Curtin, N. A. (2022). The legacy of AV Hill’s Nobel Prize winning work on muscle energetics. J. Physiol. 600, 1555–1578. doi: 10.1113/JP281556
Bassett, D. R. Jr. (2002). Scientific contributions of AV Hill: exercise physiology pioneer. J. Appl. Physiol. 93, 1567–1582. doi: 10.1152/japplphysiol.01246.2001
Bergman, B. C., Wolfel, E. E., Butterfield, G. E., Lopaschuk, G. D., Casazza, G. A., Horning, M. A., et al. (1999). Active muscle and whole body lactate kinetics after endurance training in men. J. Appl. Physiol. 87, 1684–1696. doi: 10.1152/jappl.1999.87.5.1684
Borst, P. (2020). The malate–aspartate shuttle (Borst cycle): how it started and developed into a major metabolic pathway. IUBMB Life 72, 2241–2259. doi: 10.1002/iub.2367
Boumezbeur, F., Petersen, K. F., Cline, G. W., Mason, G. F., Behar, K. L., Shulman, G. I., et al. (2010). The contribution of blood lactate to brain energy metabolism in humans measured by dynamic 13C nuclear magnetic resonance spectroscopy. J. Neurosci. 30, 13983–13991. doi: 10.1523/JNEUROSCI.2040-10.2010
Bouzat, P., and Oddo, M. (2014). Lactate and the injured brain: friend or foe? Curr. Opin. Crit. Care 20, 133–140. doi: 10.1097/MCC.0000000000000072
Bouzat, P., Sala, N., Payen, J. F., and Oddo, M. (2013). Beyond intracranial pressure: optimization of cerebral blood flow, oxygen, and substrate delivery after traumatic brain injury. Ann. Intensive Care 3, 1–9. doi: 10.1186/2110-5820-3-23
Bouzat, P., Sala, N., Suys, T., Zerlauth, J. B., Marques-Vidal, P., Feihl, F., et al. (2014). Cerebral metabolic effects of exogenous lactate supplementation on the injured human brain. Intensive Care Med. 40, 412–421. doi: 10.1007/s00134-013-3203-6
Brooks, G. A. (1985). Lactate: glycolytic end product and oxidative substrate during sustained exercise in mammals—the “lactate shuttle”. In: Circulation, Respiration, and Metabolism, pp. 208–218. Springer, Berlin, Heidelberg.
Brooks, G. A., Arevalo, J. A., Osmond, A. D., Leija, R. G., Curl, C. C., and Tovar, A. P. (2022a). Lactate in contemporary biology: a phoenix risen. J. Physiol. 600, 1229–1251. doi: 10.1113/JP280955
Brooks, G. A., Curl, C. C., Leija, R. G., Osmond, A. D., Duong, J. J., and Arevalo, J. A. (2022b). Tracing the lactate shuttle to the mitochondrial reticulum. Exp. Mol. Med. 54, 1332–1347. doi: 10.1038/s12276-022-00802-3
Brooks, G. A., and Gaesser, G. A. (1980). End points of lactate and glucose metabolism after exhausting exercise. J. Appl. Physiol. 49, 1057–1069. doi: 10.1152/jappl.1980.49.6.1057
Brooks, G. A. (2018). The science and translation of lactate shuttle theory. Cell Metab. 27, 757–785.
Brooks, G. A. (2020). The tortuous path of lactate shuttle discovery: From cinders and boards to the lab and ICU. J. Sport Health Sci. 9, 446–460.
Brooks, G. A., and Martin, N. A. (2015). Cerebral metabolism following traumatic brain injury: new discoveries with implications for treatment. Front. Neurosci. 8:408. doi: 10.3389/fnins.2014.00408
Brotherhood, J. R. (2008). Heat stress and strain in exercise and sport. J. Sci. Med. Sport 11, 6–19. doi: 10.1016/j.jsams.2007.08.017
Brown, P. L., and Kiyatkin, E. A. (2004). Brain hyperthermia induced by MDMA (‘ecstasy’): modulation by environmental conditions. Eur. J. Neurosci. 20, 51–58. doi: 10.1111/j.0953-816X.2004.03453.x
Carpenter, K. L., Jalloh, I., and Hutchinson, P. J. (2015). Glycolysis and the significance of lactate in traumatic brain injury. Front. Neurosci. 9:112. doi: 10.3389/fnins.2015.00112
Contreras, L., and Satrustegui, J. (2009). Calcium signaling in brain mitochondria. J. Biol. Chem. 284, 7091–7099. doi: 10.1074/jbc.M808066200
Crompton, A. W., Taylor, C. R., and Jagger, J. A. (1978). Evolution of homeothermy in mammals. Nature 272, 333–336. doi: 10.1038/272333a0
Dalsgaard, M. K., Quistorff, B., Danielsen, E. R., Selmer, C., Vogelsang, T., and Secher, N. H. (2004). A reduced cerebral metabolic ratio in exercise reflects metabolism and not accumulation of lactate within the human brain. J. Physiol. 554, 571–578. doi: 10.1113/jphysiol.2003.055053
Daw, C. C., Ramachandran, K., Enslow, B. T., Maity, S., Bursic, B., Novello, M. J., et al. (2020). Lactate elicits ER-mitochondrial Mg2+ dynamics to integrate cellular metabolism. Cells 554, 571–578. doi: 10.1113/jphysiol.2003.055053
del Arco, A., González-Moreno, L., Pérez-Liébana, I., Juaristi, I., González-Sánchez, P., Contreras, L., et al. (2023). Regulation of neuronal energy metabolism by calcium: role of MCU and Aralar/malate-aspartate shuttle. Biochim. Biophys. Acta Mol. Cell. Res. 1870:119468. doi: 10.1016/j.bbamcr.2023.119468
Dienel, G. A. (2019a). Brain glucose metabolism: integration of energetics with function. Physiol. Rev. 99, 949–1045. doi: 10.1152/physrev.00062.2017
Dienel, G. A. (2019b). Does shuttling of glycogen-derived lactate from astrocytes to neurons take place during neurotransmission and memory consolidation? J. Neurosci. Res. 97, 863–882. doi: 10.1002/jnr.24387
Donovan, C. M., and Brooks, G. A. (1983). Endurance training affects lactate clearance, not lactate production. Am. J. Physiol. Endocrinol. Metab. 244, E83–E92. doi: 10.1152/ajpendo.1983.244.1.E83
Donovan, C. M., and Pagliassotti, M. J. (1989). Endurance training enhances lactate clearance during hyperlactatemia. Am. J. Physiol. Endocrinol. Metab. 257, E782–E789. doi: 10.1152/ajpendo.1989.257.5.E782
Donovan, C. M., and Pagliassotti, M. J. (1990). Enhanced efficiency of lactate removal after endurance training. J. Appl. Physiol. 68, 1053–1058. doi: 10.1152/jappl.1990.68.3.1053
Elustondo, P. A., White, A. E., Hughes, M. E., Brebner, K., Pavlov, E., and Kane, D. A. (2013). Physical and functional association of lactate dehydrogenase (LDH) with skeletal muscle mitochondria. J. Biol. Chem. 288, 25309–25317. doi: 10.1074/jbc.M113.476648
Febbraio, M. A., Snow, R. J., Hargreaves, M., Stathis, C. G., Martin, I. K., and Carey, M. F. (1994). Muscle metabolism during exercise and heat stress in trained men: effect of acclimation. J. Appl. Physiol. 76, 589–597. doi: 10.1152/jappl.1994.76.2.589
Ferguson, B. S., Rogatzki, M. J., Goodwin, M. L., Kane, D. A., Rightmire, Z., and Gladden, L. B. (2018). Lactate metabolism: historical context, prior misinterpretations, and current understanding. Eur. J. Appl. Physiol. 118, 691–728. doi: 10.1007/s00421-017-3795-6
Fink, W. J., Costill, D. L., and Van Handel, P. J. (1975). Leg muscle metabolism during exercise in the heat and cold. Eur. J. Appl. Physiol. Occup. Physiol. 34, 183–190. doi: 10.1007/BF00999931
Fletcher, W. M., and Hopkins, F. G. (1907). Lactic acid in amphibian muscle. J. Physiol. 35, 247–309. doi: 10.1113/jphysiol.1907.sp001194
Fruth, J. M., and Gisolfi, C. V. (1983). Work-heat tolerance in endurance-trained rats. J. Appl. Physiol. 54, 249–253. doi: 10.1152/jappl.1983.54.1.249
Fulghum, K. L., Rood, B. R., Shang, V. O., McNally, L. A., Riggs, D. W., Zheng, Y. T., et al. (2019). Mitochondria-associated lactate dehydrogenase is not a biologically significant contributor to bioenergetic function in murine striated muscle. Redox Biol. 24:101177. doi: 10.1016/j.redox.2019.101177
Fuller, A., Carter, R. N., and Mitchell, D. (1998). Brain and abdominal temperatures at fatigue in rats exercising in the heat. J. Appl. Physiol. 84, 877–883. doi: 10.1152/jappl.1998.84.3.877
Gaesser, G. A., and Brooks, G. A. (1980). Glycogen repletion following continuous and intermittent exercise to exhaustion. J. Appl. Physiol. 49, 722–728. doi: 10.1152/jappl.1980.49.4.722
Gallagher, C. N., Carpenter, K. L., Grice, P., Howe, D. J., Mason, A., Timofeev, I., et al. (2009). The human brain utilizes lactate via the tricarboxylic acid cycle: a 13C-labelled microdialysis and high-resolution nuclear magnetic resonance study. Brain 132, 2839–2849. doi: 10.1093/brain/awp202
Gantner, D., Moore, E. M., and Cooper, D. J. (2014). Intravenous fluids in traumatic brain injury: what’s the solution? Curr. Opin. Crit. Care 20, 385–389. doi: 10.1097/MCC.0000000000000114
Gellerich, F. N., Gizatullina, Z., Trumbekaite, S., Korzeniewski, B., Gaynutdinov, T., Seppet, E., et al. (2012). Cytosolic Ca2+ regulates the energization of isolated brain mitochondria by formation of pyruvate through the malate–aspartate shuttle. Biochem. J. 443, 747–755. doi: 10.1042/BJ20110765
Glancy, B., Kane, D. A., Kavazis, A. N., Goodwin, M. L., Willis, W. T., and Gladden, L. B. (2021). Mitochondrial lactate metabolism: history and implications for exercise and disease. J. Physiol. 599, 863–888. doi: 10.1113/JP278930
Gnaiger, E., and Kemp, R. B. (1990). Anaerobic metabolism in aerobic mammalian cells: information from the ratio of calorimetric heat flux and respirometric oxygen flux. Biochim. Biophys. Acta 1016, 328–332. doi: 10.1016/0005-2728(90)90164-Y
Goldberg, R. N., Kishore, N., and Lennen, R. M. (2002). Thermodynamic quantities for the ionization reactions of buffers. J. Phys. Chem. Ref. Data 31, 231–370. doi: 10.1063/1.1416902
González-Alonso, J. (2012). Human thermoregulation and the cardiovascular system. Exp. Physiol. 97, 340–346. doi: 10.1113/expphysiol.2011.058701
Goodwin, M. L., Gladden, L. B., Nijsten, M. W., and Jones, K. B. (2015). Lactate and cancer: revisiting the Warburg effect in an era of lactate shuttling. Front. Nutr. 1:27. doi: 10.3389/fnut.2014.00027
Hammes, G. G., and Hammes-Schiffer, S. (2015). Physical Chemistry for the Biological Sciences. Hoboken, New Jersey: John Wiley & Sons, 465.
Hansen, L. D., Macfarlane, C., McKinnon, N., Smith, B. N., and Criddle, R. S. (2004). Use of calorespirometric ratios, heat per CO2 and heat per O2, to quantify metabolic paths and energetics of growing cells. Thermochim. Acta 422, 55–61. doi: 10.1016/j.tca.2004.05.033
Hargreaves, M. (2008). Physiological limits to exercise performance in the heat. J. Sci. Med. Sport 11, 66–71. doi: 10.1016/j.jsams.2007.07.002
Hashimoto, T., and Brooks, G. A. (2008). Mitochondrial lactate oxidation complex and an adaptive role for lactate production. Med. Sci. Sports Exerc. 40, 486–494. doi: 10.1249/MSS.0b013e31815fcb04
Hashimoto, T., Hussien, R., and Brooks, G. A. (2006). Colocalization of MCT1, CD147, and LDH in mitochondrial inner membrane of L6 muscle cells: evidence of a mitochondrial lactate oxidation complex. Am. J. Physiol. Endocrinol. Metab. 290, E1237–E1244. doi: 10.1152/ajpendo.00594.2005
Hashimoto, T., Hussien, R., Cho, H. S., Kaufer, D., and Brooks, G. A. (2008). Evidence for the mitochondrial lactate oxidation complex in rat neurons: demonstration of an essential component of brain lactate shuttles. PLoS One 3:e2915. doi: 10.1371/journal.pone.0002915
Hassel, B. (2000). Carboxylation and anaplerosis in neurons and glia. Mol. Neurobiol. 22, 021–040. doi: 10.1385/MN:22:1-3:021
Herbst, E. A., George, M. A., Brebner, K., Holloway, G. P., and Kane, D. A. (2018). Lactate is oxidized outside of the mitochondrial matrix in rodent brain. Appl. Physiol. Nutr. Metab. 43, 467–474. doi: 10.1139/apnm-2017-0450
Herbst, E. A., and Holloway, G. P. (2015). Permeabilization of brain tissue in situ enables multiregion analysis of mitochondrial function in a single mouse brain. J. Physiol. 593, 787–801. doi: 10.1113/jphysiol.2014.285379
Hertz, L., and Dienel, G. A. (2005). Lactate transport and transporters: general principles and functional roles in brain cells. J. Neurosci. Res. 79, 11–18. doi: 10.1002/jnr.20294
Hong, C. S., Graham, N. A., Gu, W., Camacho, C. E., Mah, V., Maresh, E. L., et al. (2016). MCT1 modulates cancer cell pyruvate export and growth of tumors that co-express MCT1 and MCT4. Cell Rep. 14, 1590–1601. doi: 10.1016/j.celrep.2016.01.057
Hui, S., Ghergurovich, J. M., Morscher, R. J., Jang, C., Teng, X., Lu, W., et al. (2017). Glucose feeds the TCA cycle via circulating lactate. Nature 551, 115–118. doi: 10.1038/nature24057
Huynh, K. W., and Pamenter, M. E. (2022). Lactate inhibits naked mole-rat cardiac mitochondrial respiration. J. Comp. Physiol. B 192, 501–511. doi: 10.1007/s00360-022-01430-z
Ide, K., Horn, A., and Secher, N. H. (1999). Cerebral metabolic response to submaximal exercise. J. Appl. Physiol. 87, 1604–1608. doi: 10.1152/jappl.1999.87.5.1604
Jacobs, R. A., Meinild, A. K., Nordsborg, N. B., and Lundby, C. (2013). Lactate oxidation in human skeletal muscle mitochondria. Am. J. Physiol. Endocrinol. Metab. 304, E686–E694. doi: 10.1152/ajpendo.00476.2012
Jelinek, B. A., and Moxley, M. A. (2021). Detailed evaluation of pyruvate dehydrogenase complex inhibition in simulated exercise conditions. Biophys. J. 120, 936–949. doi: 10.1016/j.bpj.2021.01.018
Jessen, C. (2001). Selective brain cooling in mammals and birds. Jpn. J. Physiol. 51, 291–301. doi: 10.2170/jjphysiol.51.291
Kane, D. A. (2014). Lactate oxidation at the mitochondria: a lactate-malate-aspartate shuttle at work. Front. Neurosci. 8:366. doi: 10.3389/fnins.2014.00366
Kemp, R. B. (2000). “Fire burn and cauldron bubble” (W. Shakespeare): what the calorimetric–respirometric (CR) ratio does for our understanding of cells? Thermochim. Acta 355, 115–124. doi: 10.1016/S0040-6031(00)00442-1
Kiyatkin, E. A., Kim, A. H., Wakabayashi, K. T., Baumann, M. H., and Shaham, Y. (2014). Critical role of peripheral vasoconstriction in fatal brain hyperthermia induced by MDMA (ecstasy) under conditions that mimic human drug use. J. Neurosci. 34, 7754–7762. doi: 10.1523/JNEUROSCI.0506-14.2014
Lee, S. Y., Fiorentini, G., Szasz, A. M., Szigeti, G., Szasz, A., and Minnaar, C. A. (2020). Quo vadis oncological hyperthermia (2020)? Front. Oncol. 10:1690. doi: 10.3389/fonc.2020.01690
Li, X., Zhang, Y., Xu, L., Wang, A., Zou, Y., Li, T., et al. (2023). Ultrasensitive sensors reveal the spatiotemporal landscape of lactate metabolism in physiology and disease. Cell Metab. 35, 200–211.e9. doi: 10.1016/j.cmet.2022.10.002
Liberti, M. V., and Locasale, J. W. (2016). The Warburg effect: how does it benefit cancer cells? Trends Biochem. Sci. 41, 211–218. doi: 10.1016/j.tibs.2015.12.001
Ling, B., Peng, F., Alcorn, J., Lohmann, K., Bandy, B., and Zello, G. A. (2012). D-Lactate altered mitochondrial energy production in rat brain and heart but not liver. Nutr. Metab. 9, 1–8.
Lund, J., Breum, A. W., Gil, C., Falk, S., Sass, F., Isidor, M. S., et al. (2023). The anorectic and thermogenic effects of pharmacological lactate in male mice are confounded by treatment osmolarity and co-administered counterions. Nat. Metab. 5, 1–22. doi: 10.1038/s42255-023-00780-4
MacRae, H. S., Dennis, S. C., Bosch, A. N., and Noakes, T. D. (1992). Effects of training on lactate production and removal during progressive exercise in humans. J. Appl. Physiol. 72, 1649–1656. doi: 10.1152/jappl.1992.72.5.1649
Mahalingam, S., Coulson, S. Z., Scott, G. R., and McClelland, G. B. (2023). Function of left ventricle mitochondria in highland deer mice and lowland mice. J. Comp. Physiol. B 193, 207–217. doi: 10.1007/s00360-023-01476-7
Mallory, M., Gogineni, E., Jones, G. C., Greer, L., and Simone, C. B. II (2016). Therapeutic hyperthermia: the old, the new, and the upcoming. Crit. Rev. Oncol. Hematol. 97, 56–64. doi: 10.1016/j.critrevonc.2015.08.003
Mason, S. (2017). Lactate shuttles in neuroenergetics—homeostasis, allostasis and beyond. Front. Neurosci. 11:43. doi: 10.3389/fnins.2017.00043
McEwen, B. S. (2000). Allostasis and allostatic load: implications for neuropsychopharmacology. Neuropsychopharmacology 22, 108–124. doi: 10.1016/S0893-133X(99)00129-3
McKenna, M. C., Waagepetersen, H. S., Schousboe, A., and Sonnewald, U. (2006). Neuronal and astrocytic shuttle mechanisms for cytosolic-mitochondrial transfer of reducing equivalents: current evidence and pharmacological tools. Biochem. Pharmacol. 71, 399–407. doi: 10.1016/j.bcp.2005.10.011
Messonnier, L. A., Emhoff, C. A. W., Fattor, J. A., Horning, M. A., Carlson, T. J., and Brooks, G. A. (2013). Lactate kinetics at the lactate threshold in trained and untrained men. J. Appl. Physiol. 114, 1593–1602. doi: 10.1152/japplphysiol.00043.2013
Miller, B. F., Fattor, J. A., Jacobs, K. A., Horning, M. A., Navazio, F., Lindinger, M. I., et al. (2002a). Lactate and glucose interactions during rest and exercise in men: effect of exogenous lactate infusion. J. Physiol. 544, 963–975. doi: 10.1113/jphysiol.2002.027128
Miller, B. F., Fattor, J. A., Jacobs, K. A., Horning, M. A., Suh, S. H., Navazio, F., et al. (2002b). Metabolic and cardiorespiratory responses to “the lactate clamp”. Am. J. Physiol. Endocrinol. Metab. 283, E889–E898. doi: 10.1152/ajpendo.00266.2002
Miller, S. L., and Smith‐Magowan, D. (1990). The thermodynamics of the Krebs cycle and related compounds. J. Phys. Chem. Ref. Data 19, 1049–1073.
Minikami, S., and de Verdier, C. H. (1976). Calorimetric study on human erythrocyte glycolysis: heat production in various metabolic conditions. Eur. J. Biochem. 65, 451–460. doi: 10.1111/j.1432-1033.1976.tb10360.x
Mreisat, A., Kanaani, H., Saada, A., and Horowitz, M. (2020). Heat acclimation mediated cardioprotection is controlled by mitochondrial metabolic remodeling involving HIF-1α. J. Therm. Biol. 93:102691. doi: 10.1016/j.jtherbio.2020.102691
Nelson, D. A., and Nunneley, S. A. (1998). Brain temperature and limits on transcranial cooling in humans: quantitative modeling results. Eur. J. Appl. Physiol. Occup. Physiol. 78, 353–359. doi: 10.1007/s004210050431
Nybo, L. (2012). Brain temperature and exercise performance. Exp. Physiol. 97, 333–339. doi: 10.1113/expphysiol.2011.062273
Nybo, L., Secher, N. H., and Nielsen, B. (2002). Inadequate heat release from the human brain during prolonged exercise with hyperthermia. J. Physiol. 545, 697–704. doi: 10.1113/jphysiol.2002.030023
Okahashi, N., Shima, T., Kondo, Y., Araki, C., Tsuji, S., Sawai, A., et al. (2023). Metabolic flux and flux balance analyses indicate the relevance of metabolic thermogenesis and aerobic glycolysis in cancer cells. bioRxiv. doi: 10.1101/2021.11.16.468557. [Epub ahead of preprint].
Overgaard, M., Rasmussen, P., Bohm, A. M., Seifert, T., Brassard, P., Zaar, M., et al. (2012). Hypoxia and exercise provoke both lactate release and lactate oxidation by the human brain. FASEB J. 26, 3012–3020. doi: 10.1096/fj.11-191999
Parkin, J. M., Carey, M. F., Zhao, S., and Febbraio, M. A. (1999). Effect of ambient temperature on human skeletal muscle metabolism during fatiguing submaximal exercise. J. Appl. Physiol. 86, 902–908. doi: 10.1152/jappl.1999.86.3.902
Passarella, S., Paventi, G., and Pizzuto, R. (2014). The mitochondrial L-lactate dehydrogenase affair. Front. Neurosci. 8:407. doi: 10.3389/fnins.2014.00407
Passarella, S., Schurr, A., and Portincasa, P. (2021). Mitochondrial transport in glycolysis and gluconeogenesis: achievements and perspectives. Int. J. Mol. Sci. 22:12620. doi: 10.3390/ijms222312620
Périard, J. D., Racinais, S., and Sawka, M. N. (2015). Adaptations and mechanisms of human heat acclimation: applications for competitive athletes and sports. Scand. J. Med. Sci. Sports 25, 20–38. doi: 10.1111/sms.12408
Pesta, D., and Gnaiger, E. (2012). High-resolution respirometry: OXPHOS protocols for human cells and permeabilized fibers from small biopsies of human muscle. In: Mitochondrial Bioenergetics. New York, Dordrecht Heidelberg, London: Springer, Humana Press, 25–58.
Phillips, S. M., Green, H. J., Tarnopolsky, M. A., and Grant, S. M. (1995). Increased clearance of lactate after short-term training in men. J. Appl. Physiol. 79, 1862–1869. doi: 10.1152/jappl.1995.79.6.1862
Ponsot, E., Zoll, J., N’guessan, B., Ribera, F., Lampert, E., Richard, R., et al. (2005). Mitochondrial tissue specificity of substrates utilization in rat cardiac and skeletal muscles. J. Cell. Physiol. 203, 479–486.
Quistorff, B., Secher, N. H., and Van Lieshout, J. J. (2008). Lactate fuels the human brain during exercise. FASEB J. 22, 3443–3449. doi: 10.1096/fj.08-106104
Rath, S., Sharma, R., Gupta, R., Ast, T., Chan, C., Durham, T. J., et al. (2021). MitoCarta3.0: an updated mitochondrial proteome now with sub-organelle localization and pathway annotations. Nucleic Acids Res. 49, D1541–D1547. doi: 10.1093/nar/gkaa1011
Rivas, E., Rao, M., Castleberry, T., and Ben-Ezra, V. (2017). The change in metabolic heat production is a primary mediator of heat acclimation in adults. J. Therm. Biol. 70, 69–79. doi: 10.1016/j.jtherbio.2017.10.001
Rogatzki, M. J., Ferguson, B. S., Goodwin, M. L., and Gladden, L. B. (2015). Lactate is always the end product of glycolysis. Front. Neurosci. 9:22. doi: 10.3389/fnins.2015.00022
Rolfe, D. F., and Brown, G. C. (1997). Cellular energy utilization of standard metabolic and molecular origin rate in mammals. Physiol. Rev. 77, 731–758. doi: 10.1152/physrev.1997.77.3.731
Saks, V. A., Veksler, V. I., Kuznetsov, A. V., Kay, L., Sikk, P., Tiivel, T., et al. (1998). Permeabilized cell and skinned fiber techniques in studies of mitochondrial function in vivo. Bioenergetics of the Cell: Quantitative Aspects. BV, Dordrecht: Springer-Science + Business Media, 81–100.
Sarkar, S., Chakraborty, D., Bhowmik, A., and Ghosh, M. K. (2019). Cerebral ischemic stroke: cellular fate and therapeutic opportunities. Front. Biosci. 24, 415–430. doi: 10.2741/4727
Sawka, M. N., Pandolf, K. B., Avellini, B. A., and Shapiro, Y. (1983). Does heat acclimation lower the rate of metabolism elicited by muscular exercise? Aviat. Space Environ. Med. 54, 27–31.
Schurr, A. (2006). Lactate: the ultimate cerebral oxidative energy substrate? J. Cereb. Blood Flow Metab. 26, 142–152. doi: 10.1038/sj.jcbfm.9600174
Schurr, A. (2017). “Lactate, not pyruvate, is the end product of glucose metabolism via glycolysis,” in Carbohydrate. eds. M. Caliskan, I. H. Kavakli, and G. C. Oz (InTech).
Scott, C., and Kemp, R. (2005). Direct and indirect calorimetry of lactate oxidation: implications for whole-body energy expenditure. J Sports Sci. 23, 15–19. doi: 10.1080/02640410410001716760
Shulman, R. G., Hyder, F., and Rothman, D. L. (2001). Lactate efflux and the neuroenergetic basis of brain function. NMR in Biomedicine: An International Journal Devoted to the Development and Application of Magnetic Resonance In Vivo 14, 389–396. doi: 10.1096/fj.08-106104
Siebenmann, C., Sørensen, H., Bonne, T. C., Zaar, M., Aachmann-Andersen, N. J., Nordsborg, N. B., et al. (2021). Cerebral lactate uptake during exercise is driven by the increased arterial lactate concentration. J. Appl. Physiol. 131, 1824–1830. doi: 10.1152/japplphysiol.00505.2021
Silva, J. E. (2006). Thermogenic mechanisms and their hormonal regulation. Physiol. Rev. 86, 435–464. doi: 10.1152/physrev.00009.2005
Skolik, R. A., Konkle, M. E., and Menze, M. A. (2018). Calorespirometry: a powerful, noninvasive approach to investigate cellular energy metabolism. J. Vis. Exp. 135:57724. doi: 10.3791/57724-v
Smith, K. J., and Ainslie, P. N. (2017). Regulation of cerebral blood flow and metabolism during exercise. Exp. Physiol. 102, 1356–1371. doi: 10.1113/EP086249
Smith, D., Pernet, A., Hallett, W. A., Bingham, E., Marsden, P. K., and Amiel, S. A. (2003). Lactate: a preferred fuel for human brain metabolism in vivo. J. Cereb. Blood Flow Metab. 23, 658–664. doi: 10.1097/01.WCB.0000063991.19746.11
Sonveaux, P., Végran, F., Schroeder, T., Wergin, M. C., Verrax, J., Rabbani, Z. N., et al. (2008). Targeting lactate-fueled respiration selectively kills hypoxic tumor cells in mice. J. Clin. Invest. 118, 3930–3942. doi: 10.1172/JCI36843
Sterling, P., and Eyer, J. (1988). “Allostasis: a new paradigm to explain arousal pathology” in Handbook of Life Stress, Cognition and Health. eds. S. Fisher and J. T. Reason (Chicester, NY: Wiley).
Sterling, P. (2012). Allostasis: a model of predictive regulation. Physiol. Behav. 106, 5–15. doi: 10.1016/j.physbeh.2011.06.004
Svedung Wettervik, T. M., Engquist, H., Lenell, S., Howells, T., Hillered, L., Rostami, E., et al. (2021). Systemic hyperthermia in traumatic brain injury—relation to intracranial pressure dynamics, cerebral energy metabolism, and clinical outcome. J. Neurosurg. Anesthesiol. 33, 329–336. doi: 10.1097/ANA.0000000000000695
Szczesna-Kaczmarek, A. (1990). L-lactate oxidation by skeletal muscle mitochondria. Int. J. Biochem. 22, 617–620. doi: 10.1016/0020-711X(90)90038-5
Thompson, H. J., Tkacs, N. C., Saatman, K. E., Raghupathi, R., and McIntosh, T. K. (2003). Hyperthermia following traumatic brain injury: a critical evaluation. Neurobiol. Dis. 12, 163–173. doi: 10.1016/S0969-9961(02)00030-X
Thornton, W. M. (1917). XV. The relation of oxygen to the heat of combustion of organic compounds. Lond. Edinb. Dublin Philos. Mag. J. Sci. 33, 196–203. doi: 10.1080/14786440208635627
Valenti, D., de Bari, L., Atlante, A., and Passarella, S. (2002). L-lactate transport into rat heart mitochondria and reconstruction of the L-lactate/pyruvate shuttle. Biochem. J. 364, 101–104. doi: 10.1042/bj3640101
van Gemert, L. A., de Galan, B. E., Wevers, R. A., Ter Heine, R., and Willemsen, M. A. (2022). Lactate infusion as therapeutical intervention: a scoping review. Eur. J. Pediatr. 181, 2227–2235. doi: 10.1007/s00431-022-04446-3
van Hall, G., Stømstad, M., Rasmussen, P., Jans, Ø., Zaar, M., Gam, C., et al. (2009). Blood lactate is an important energy source for the human brain. J. Cereb. Blood Flow Metab. 29, 1121–1129. doi: 10.1038/jcbfm.2009.35
Vespa, P., McArthur, D. L., Stein, N., Huang, S. C., Shao, W., Filippou, M., et al. (2012). Tight glycemic control increases metabolic distress in traumatic brain injury: a randomized controlled within-subjects trial. Crit. Care Med. 40, 1923–1929. doi: 10.1097/CCM.0b013e31824e0fcc
Wang, H., Wang, B., Normoyle, K. P., Jackson, K., Spitler, K., Sharrock, M. F., et al. (2014). Brain temperature and its fundamental properties: a review for clinical neuroscientists. Front. Neurosci. 8:307. doi: 10.3389/fnins.2014.00307
Wanner, S. P., Prímola-Gomes, T. N., Pires, W., Guimaraes, J. B., Hudson, A. S. R., Kunstetter, A. C., et al. (2015). Thermoregulatory responses in exercising rats: methodological aspects and relevance to human physiology. Temperature 2, 457–475. doi: 10.1080/23328940.2015.1119615
Wilhelm, F., and Hirrlinger, J. (2011). The NAD+/NADH redox state in astrocytes: independent control of the NAD+ and NADH content. J. Neurosci. Res. 89, 1956–1964. doi: 10.1002/jnr.22638
Wust, P., Hildebrandt, B., Sreenivasa, G., Rau, B., Gellermann, J., Riess, H., et al. (2002). Hyperthermia in combined treatment of cancer. Lancet Oncol. 3, 487–497. doi: 10.1016/S1470-2045(02)00818-5
Wyss, M. T., Jolivet, R., Buck, A., Magistretti, P. J., and Weber, B. (2011). In vivo evidence for lactate as a neuronal energy source. J. Neurosci. 31, 7477–7485. doi: 10.1523/JNEUROSCI.0415-11.2011
Yang, J., Ruchti, E., Petit, J. M., Jourdain, P., Grenningloh, G., Allaman, I., et al. (2014). Lactate promotes plasticity gene expression by potentiating NMDA signaling in neurons. Proc. Natl. Acad. Sci. 111, 12228–12233. doi: 10.1073/pnas.1322912111
Keywords: bioenergetics, brain, calorimetry, lactate, mitochondria, pyruvate, thermal physiology
Citation: Kane DA, Foo ACY, Noftall EB, Brebner K and Marangoni DG (2023) Lactate shuttling as an allostatic means of thermoregulation in the brain. Front. Neurosci. 17:1144639. doi: 10.3389/fnins.2023.1144639
Edited by:
Fahmeed Hyder, Yale University, United StatesReviewed by:
Ruoyu Yang, Shanghai University of Medicine and Health Sciences, ChinaLee Hansen, Brigham Young University, United States
George A. Brooks, University of California, Berkeley, United States
Copyright © 2023 Kane, Foo, Noftall, Brebner and Marangoni. This is an open-access article distributed under the terms of the Creative Commons Attribution License (CC BY). The use, distribution or reproduction in other forums is permitted, provided the original author(s) and the copyright owner(s) are credited and that the original publication in this journal is cited, in accordance with accepted academic practice. No use, distribution or reproduction is permitted which does not comply with these terms.
*Correspondence: Daniel A. Kane, dkane@stfx.ca