- Department of Anesthesiology and Perioperative Medicine, University of Louisville, Louisville, KY, United States
Introduction
Our understanding of neuroenergetics was drastically changed 35 years ago with the revelation that lactate, the end-product of the glycolytic pathway, can be used as a substrate of the mitochondrial oxidative phosphrylation pathway (oxphos) in the absence of glucose (Schurr et al., 1988a). Expectedly, when new scientific discoveries question a long-standing dogma, the idea that lactate is a substrate of oxphos was received with great skepticism from the majority of the scientific community in the field of cerebral energy metabolism (for details see Schurr, 2014). Unfortunately, this skepticism has led many to ignore accumulating data supporting the role of lactate as a substrate of oxphos, which is evidenced in the majority of biochemistry textbook chapters and online biochemistry classes, where pyruvate is still considered to be the end-product of aerobic glycolysis and the oxidative substrate of oxphos. Studies that indicate lactate to be the end-product of glycolysis, either in the absence or presence of oxygen, are generally ignored. Even studies that specifically investigate the role of lactate in neuroenergetics, including several review papers, tend to overlook earlier publications on the topic. Beyond the expected courtesy of citing earlier, relevant work, the omission of such citations in more recent publications contributes to unnecessary delay in advancing the knowledge and understanding of neuroenergetics, the most important biochemical process within the central nervous system (CNS). The past decade also saw multiple studies pointing at additional roles lactate may play in the CNS both as a receptor ligand and a signaling factor. However, those potential roles are a topic to be covered separately. This commentary will relate only to the role of lactate in neuroenergetics. Instead of a repeat attempt at explaining the persisting skepticism about lactate and its role in cerebral energy metabolism (Schurr, 2014), considered here are the scientific data that have placed lactate at the center of neuroenergetics, the process that assures normal and continuous brain function.
A very brief review of the history of lactate
In the beginning there was lactic acid in sour milk and from then on this monocarboxylate has been stigmatized, a reputation that remained with lactate when it was shown to be the product of muscular glucose consumption under anaerobic conditions. However, aerobically, muscles can dispose of it (Fletcher and Hopkins, 1907). Similarly, in heart muscle it was observed that under full oxygenation lactate is not produced (Locke and Rosenheim, 1907). Interestingly, in those early days it was assumed that glucose consumption leads to lactate production even in the presence of oxygen and that somehow aerobic conditions allow skeletal and heart muscle to quickly dispose of it. Casting lactate as an undesirable product of energy production via glucose metabolism continued unabated throughout the majority of the 20th century. Even a spate of research papers, published in the mid 1920s to early 1930s, on the ability of brain tissue in vitro to reduce its lactate levels by supplying it with oxygen, was still interpreted as a disposal mechanism (Holmes and Holmes, 1925a,b; Holmes and Ashford, 1930; Ashford and Holmes, 1931). Consequently, lactate continued to be marked as useless and at times the poisonous end-product of anaerobic glycolysis. This concept entails that lactate is not being produced during aerobic glycolysis, a postulate that led investigators to mark pyruvate as the end-product of aerobic glycolysis, and thus, the substrate of the mitochondrial tricarboxylic acid (TCA) cycle (Krebs and Johnson, 1937a,b; Krebs et al., 1938). Obviously, this concept has taken roots despite the fact that drawing glycolysis as a 10-reaction pathway, ending with pyruvate, rather than an 11-reaction one, ending with lactate, is contradictory to the basic thermodynamic rule of free energy change. Accordingly, lactate has been proposed as the oxidative substrate of mitochondrial oxphos (Schurr, 2006) based on cumulative indirect experimental data (Schurr et al., 1997a,b, 1999) that later were supported by direct biochemical and electrophysiological data (Schurr and Payne, 2007; Schurr and Gozal, 2011, 2015; Schurr, 2014, 2018). Additional studies showing that cerebellum mitochondria metabolize lactate have strengthened this concept (Atlante et al., 2007; Passarella et al., 2008). Nevertheless, in the multiple presentations available online today (for example: Intro Bio Lec. Columbia University; Gibbs Free Energy of Glycolysis Flashcards; Quizlet, Glycolysis, Part 2; Azimuth, https://www.wordpress.com; https://www.youtube.com/watch?v=-u-cQ7Onq_A), an explanation is missing of how the last glycolytic step (step 11), the conversion of pyruvate to lactate is arrested during aerobic conditions despite being the glycolytic step with the a large free energy change (ΔG = −6.0 Kcal) second only to the hexokinase reaction (ΔG = −8.0 Kcal). Moreover, this doctrinaire presentation ignores the fact that the eleventh glycolytic step allows for the replenishing of the NAD+ pool that is necessary to maintain the cyclical nature of glycolysis (Schurr, 2018). It is beyond remarkable that a great majority of biochemists, physiologists, neuroscientists, clinicians and science teachers are still reciting and promoting an incomplete 10-reaction glycolysis that ends with pyruvate, while ignoring the real last glycolytic step (step 11), which must take place under the very thermodynamic rule that applies to all the previous steps. The resistance to abandon the old dogma of glycolysis could be explained by habit of mind (Margolis, 1993; Schurr, 2014). As Figure 1 illustrates, glycolysis ends with lactate, independently of the conditions it proceeds under. Neither oxygen, nor mitochondria halt this outcome and those who claim otherwise have never offered a mechanism that explain how the arrest of the eleventh reaction occurs.
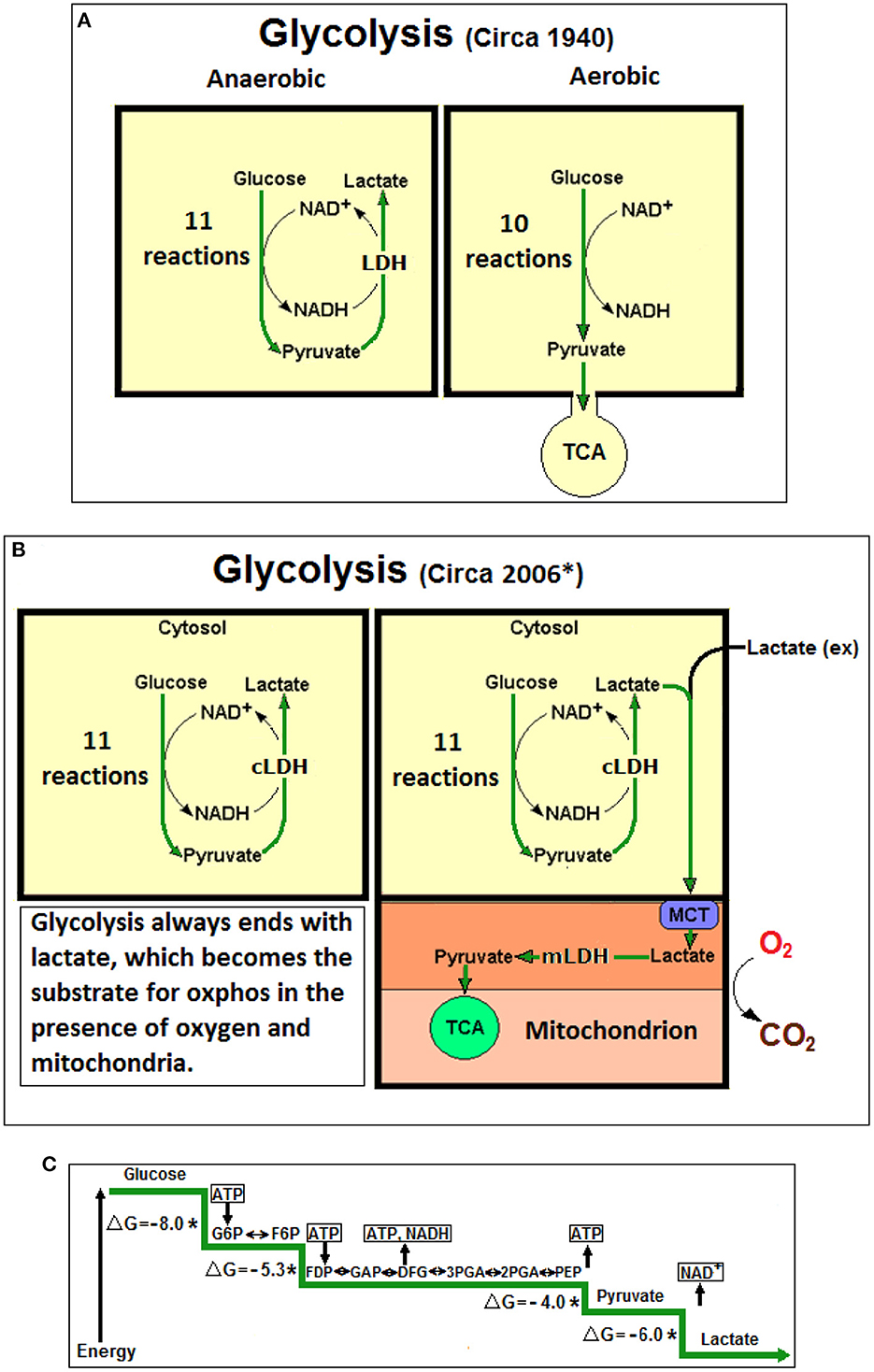
Figure 1. (A) At the beginning lactic acid (lactate) was considered to be a waste product of anaerobic glycolysis, not produced during aerobic glycolysis (glycolysis, also known as Embden-Myerhof pathway, was elucidated in 1940). The 11-reaction anaerobic glycolysis, shown on the left, ends with lactate and allows the cyclical production of NAD+ from NADH. The 10-reaction aerobic glycolysis, shown on the right, ends with pyruvate, to be used as a substrate of the tricarboxylic acid (TCA) cycle, without any reproduction of NAD+ from NADH. (B) Over six decades later (*Schurr, 2006), a unified, 11-reaction glycolytic pathway has been suggested where lactate is always its end-product regardless of the presence or absence of oxygen. (C) A schematic presentation of the glycolytic free energy change through the eleven enzymatic steps of the pathway. The first step of glycolysis, the phosphorylation of glucose to glucose-6-phosphate by hexokinase, produces the largest free energy change (ΔG = −8.0 Kcal), the last step, the conversion of pyruvate to lactate by lactate dehydrogenase (LDH) produces the second largest free energy change (ΔG = −6.0 Kcal). No mechanism has been offered in over 80 years to explain how the LDH step could be arrested, as one would expected according to the prevailing aerobic glycolysis dogma, which stands against the strongly favorable thermodynamic conditions. NAD+, nicotinamide adenine dinucleotide; NADH, nicotinamide adenine dinucleotide, reduced form; cLDH, cytosolic lactate dehydrogenase (LDH); mLDH, mitochondrial LDH; MCT, monocarboxylate transporter; Lactate (ex), external lactate; ATP, adenosine triphosphate; G6P, glucose 6-phosphate; F6P, fructose 6-phosphate; FDP, fructose 1,6-biphosphate; GAP, glyceraldehyde 3-phosphate; 3PGA, 3-phosphoglycerate; 2PGA, 2-phosphoglycerate; PEP, phosphoenolpyruvate; *Non-equilibrium reaction; ΔG, free energy change in Kcal.
As discussed elsewhere (Schurr, 2014), even four decades after the biochemical sequence of the glycolytic pathway was established, lactate (lactic acidosis) was hypothesized to be the main culprit responsible for the delayed neuronal damage observed after cerebral ischemia (Siesjo, 1981). Ironically, attempting to reproduce enhanced neuronal damage by adding lactic acid to an in vitro model of hypoxia/cerebral ischemia had indicated that lowering the pH, either with lactic acid or HCl, protected the tissue against neuronal damage (Schurr et al., 1988b). Simultaneously with the discovery that lactate is a neuronal oxidative substrate (Schurr et al., 1988a). Fox et al. (1988) claimed that glucose consumption during focal physiologic neural activity is non-oxidative. The description “non-oxidative glucose consumption” is also known as the “Warburg Effect” (Warburg et al., 1927) and “aerobic glycolysis.” Non-oxidative glucose consumption and aerobic glycolysis are terms born due to the decision to describe the glycolytic pathway as having two separate outcomes, depending on the presence or absence of oxygen namely, “aerobic” and “anaerobic” glycolysis. Accordingly, the former ends with pyruvate and the latter with lactate. In 1940 our knowledge regarding the role of mitochondria was non-existent and any hint of oxphos participation in energy metabolism was limited to the fact that phosphate participates in the metabolic reactions. The idea that the presence of oxygen somehow determines the fate of glycolytic glucose metabolism became the tenet, which still occupies both textbooks and minds.
Just glycolysis: Devoid of the misleading aerobic and anaerobic prefixes
Considering the above mentioned history, any observation of glucose metabolism not accompanied by oxygen consumption is categorized as aerobic glycolysis. Of course, red blood cells, devoid of mitochondria, but rich in oxygen, produce ATP by “aerobic” glycolysis. Proliferating cancer cells do it (Warburg et al., 1927), so does the Na/K-ATPase pump (Balaban and Bader, 1984) and, since 1988, focal neural cells during physiologic activity do it too (Fox et al., 1988). Separating glycolysis into aerobic and anaerobic pathways greatly confuses both the basic understanding of its metabolic role in the CNS and most likely in every other tissue as well as its diagnostic and medical applications. With the development and advancement of our knowledge and understanding of mitochondria and their role in respiration and ATP production, the designation of aerobic and anaerobic glycolysis was changed such that the former requires not only the presence of oxygen, but also the presence of mitochondria, while the latter requires the absence of both (Naifeh et al., 2022). Nevertheless, a mechanism by which the eleventh step of glycolysis namely, the enzymatic conversion of pyruvate to lactate by lactate dehydrogenase (LDH), is somehow “turned off” when oxygen and mitochondria are present, has never been offered or pursued, let alone resolved. This “mystery” continues to accompany every online presentation of glycolysis, in published papers and in textbooks. As indicated above, a habit of mind (Margolis, 1993; Schurr, 2014) must be responsible for the continuous promotion of a concept that is fundamentally off the mark. That the presence of oxygen has nothing to do with glycolysis ending with the tenth reaction and somehow arresting the eleventh (LDH) one from proceeding, is absolutely obvious. Similarly, no mechanism has been offered yet, to explain how the presence of mitochondria switch the glycolytic pathway from 11 to 10 reactions. Glycolysis is a pathway present in every other bodily tissue beside the CNS and multiple studies over the past several decades exhibited mitochondrial utilization of lactate as a substrate of oxphos, while other exhibited the presence of LDH intra-mitochondrially (Brooks, 1985, 1998, 2000, 2002a,b; Larrabee, 1996; Hu and Wilson, 1997; Brooks et al., 1999, 2022; Magistretti et al., 1999; Valenti et al., 2002; Mangia et al., 2003; Pellerin and Magistretti, 2003; de Bari et al., 2004, 2010; Kasischke et al., 2004; Serres et al., 2005; Hashimoto et al., 2006, 2008; Schurr and Payne, 2007; Hashimoto and Brooks, 2008; Passarella et al., 2008; Elustondo et al., 2013; Rogatzki et al., 2015; Hui et al., 2017).
Therefore, it is even more bewildering that the majority of recently published general information, both in print and online, continues to completely ignore the data proving that the glycolytic pathway, regardless of the presence of oxygen and/or mitochondria, always produces lactate as its end-product. The terminology “aerobic” and “anaerobic” glycolysis is meaningless, confusing and should be eliminated (Schurr and Passarella, 2022). Interestingly, as early as 1984 it was suggested to use the term “non-robic” glycolysis instead of the terms “aerobic” and “anaerobic” (Brooks and Fahey, 1984), a suggestion that clearly was not accepted. It is worthwhile mentioning that neuroenergetics is not the only field of research where skepticism about the roles of glycolysis and lactate persists, particularly in muscle energy metabolism and more generally in exercise physiology. Moreover, advances in treatment of neurological conditions, such as traumatic brain injury (TBI), are driving a revision in our understanding of the roles of glycolysis and lactate in these clinical settings.
A new study made use of an ultrasensitive, highly responsive, radiometric lactate sensor that enables the user to monitor subtle lactate fluctuations in living cells and animals. The investigators have definitively demonstrated that lactate is highly enriched in mammalian mitochondria and the important role it plays in cellular metabolism (Li et al., 2022). Unfortunately, the authors chose not to cite a great number of relevant studies that support their findings and could greatly strengthen their important discoveries. Nevertheless, the reported findings confirm the assertion that lactate is the glycolytic end-product and the mitochondrial substrate for the tricarboxylic acid cycle (TCA). The investigators showed that the lactate pool intra-mitochondrially in glucose-fed cell lines was up to10 times higher than that in the cytosol. Moreover, the “lactate levels are sensitive to changes in glucose levels and metabolism,” where they increased when glucose levels did and vice versa. In addition, this study found that the mitochondrial lactate enrichment was inhibited by the use of the mitochondrial uncoupler, carbonyl cyanide 3-chlorophenylhydrazone (CCCP), indicating that such enrichment is due to oxphos being coupled to the mitochondrial electron transport chain activity.
Summary
This opinion is another attempt to persuade scientists, clinicians, teachers and students alike to discontinue the use of the archaic and misleading prefixes “aerobic” and “anaerobic” always attached to the term “glycolysis.” They should be discontinued and “glycolysis” must stands alone. Since it is now clearly evident that glycolysis is a pathway that consists of eleven enzymatic reactions the last one of which always terminates with the production of lactate and NAD+, independent of the presence or absence of oxygen and mitochondria, “aerobic” and “anaerobic” glycolysis cannot be differentiated from one another. This antiquated differentiation was based on the assumption that the glycolytic pathway has two different outcomes/end products, pyruvate under aerobic and lactate under anaerobic conditions. The fact that lactate is the one and only terminal glycolytic product, any prefix assigned to “glycolysis” is, in essence, meaningless. This is especially glaring when the term “aerobic glycolysis” that is in use today means that the pathway ends with lactate as its final product without any oxygen consumption, despite the presence of oxygen (Schurr and Passarella, 2022). Consequently, pyruvate should be considered an intermediate, not different from any other glycolytic intermediate. Lactate produced in the cytosol is shuttled via monocarboxylate transporters (MCTs) intracellularly or intercellularly into mitochondria, where it is then converted to pyruvate intra-mitochondrially via a mitochondrial LDH (mLDH) to enters the TCA cycle (Figure 1B). In conclusion, the glycolytic pathway's main purpose is the production of two terminal products; lactate, the substrate of the mitochondrial TCA cycle and NAD+, a redox cofactor utilized both extra- and intra-mitochondrially.
Author contributions
The author confirms being the sole contributor of this work and has approved it for publication.
Acknowledgments
The author thanks P.E. Moore for her comments, suggestions and corrections.
Conflict of interest
The author declares that the research was conducted in the absence of any commercial or financial relationships that could be construed as a potential conflict of interest.
Publisher's note
All claims expressed in this article are solely those of the authors and do not necessarily represent those of their affiliated organizations, or those of the publisher, the editors and the reviewers. Any product that may be evaluated in this article, or claim that may be made by its manufacturer, is not guaranteed or endorsed by the publisher.
References
Ashford, C. A., and Holmes, E. G. (1931). Further observations on the oxidation of lactic acid by brain tissue. Biochem. J. 25, 2028–2049.
Atlante, A., de Bari, L., Bobba, A., Marra, E., and Passarella, S. (2007). Transport and metabolism of l-lactate occur in mitochondria from cerebellar granule cells and are modified in cells undergoing low potassium dependent apoptosis. Biochim. Biophys. Acta 1767, 1285–1299. doi: 10.1016/j.bbabio.2007.08.003
Balaban, R.S., and Bader, J.P. (1984). Studies on the relationship between glycolysis and (Na+ + K+)-ATPase in cultured cells. Biochim. Biophys. Acta 17, 419–426. doi: 10.1016/0167-4889(84)90069-7
Brooks, G.A., Curl, C.C., Leija, R.G., Osmond, A. D., Duong, J. J., and Arevalo, J. A. (2022). Tracing the lactate shuttle to the mitochondrial reticulum. Exp. Mol. Med. 54, 1332–1347. doi: 10.1038/s12276-022-00802-3
Brooks, G. A. (1985). “Lactate: glycolytic product and oxidative substrate during sustained exercise in mammals: “the lactate shuttle”” in Comparative Physiology and Biochemistry: Currecnt Topics and Trends Vol A. Respiration–Metabolism–Circulation, ed R. Gilles (Berlin: Springer-Verlag), 208–218.
Brooks, G. A. (1998). Mammalian fuel utilization during sustained exercise. Comp. Biochem. Physiol. B Biochem. Mol. Biol. 120, 89–107. doi: 10.1016/S0305-0491(98)00025-X
Brooks, G. A. (2000). Intra- and extra-cellular lactate shuttles. Med. Sci. Sports Exerc. 32, 790–799. doi: 10.1097/00005768-200004000-00011
Brooks, G. A. (2002a). Lactate shuttle: between but not within cells? J. Physiol. 541, 333. doi: 10.1113/jphysiol.2002.023705
Brooks, G. A. (2002b). Lactate shuttles in nature. Biochem. Soc. Trans. 30, 258–264. doi: 10.1042/bst0300258
Brooks, G. A., Dubouchaud, H., Brown, M., Sicurello, J. P., and Butz, C. E. (1999). Role of mitochondrial lactate dehydrogenase and lactate oxidation in the intracellular lactate shuttle. Proc. Natl. Acad. Sci. U. S. A. 96, 1129–1134. doi: 10.1073/pnas.96.3.1129
Brooks, G. A., and Fahey, T. D. (1984). Exercise Physiology: Human Bioenergetics and Its Applications. New York, NY: John Wiley and Sons, Inc., pp. 73–74.
de Bari, L., Atlante, A., Valenti, D., and Passarella, S. (2004). Partial reconstruction of in vitro gluconeogenesis arising from mitochondrial l-lactate uptake/metabolism and oxaloacetate export via novel l-lactate translocators. Biochem. J. 380, 231–242. doi: 10.1042/bj20031981
de Bari, L., Chieppa, G., Marra, E., and Passarella, S. (2010). L-lactate metabolism can occur in normal and cancer prostate cells via the novel mitochondrial L-lactate dehydrogenase. Internatl. J. Oncol. 37, 1607–1620. doi: 10.3892/ijo_00000815
Elustondo, P. A., White, A. E., Hughes, M. E., Brebner, K., Pavlov, E., and Kane, D. A. (2013). Physical and functional association of lactate dehydrogenase (LDH) with skeletal muscle mitochondria. J. Biol. Chem. 288, 25309–25317. doi: 10.1074/jbc.M113.476648
Fletcher, W. M., and Hopkins, F. G. (1907). Lactic acid in amphibian muscle. J. Physiol. 35, 247–309.
Fox, P. T., Raichle, M. E., Mintun, M. A., and Dence, C. (1988). Nonoxidative glucose consumption during focal physiologic neural activity. Science. 241, 462–464. doi: 10.1126/science.3260686
Hashimoto, T., and Brooks, G.A. (2008). Mitochondrial lactate oxidation complex and an adaptive role for lactate production. Med. Sci. Sports Exerc. 40, 486–494. doi: 10.1249/MSS.0b013e31815fcb04
Hashimoto, T., Hussien, R., and Brooks, G. A. (2006). Colocalization of MCT1, CD147, and LDH in mitochondrial inner membrane of L6 muscle cells: evidence of a mitochondrial lactate oxidation complex. Am. J. Physiol. Endocrinol. Metab. 290, E1237–E1244. doi: 10.1152/ajpendo.00594.2005
Hashimoto, T., Hussien, R., Cho, H.-S., Kaufer, D., and Brooks, G. A. (2008). Evidence for the mitochondrial lactate oxidation complex in rat neurons: demonstration of an essential component of brain lactate shuttles. PLoS ONE 3, e2915. doi: 10.1371/journal.pone.0002915
Holmes, B. E., and Holmes, E. G. (1925a). Contributions to the study of brain metabolism. I. Carbohydrate metabolism. Preliminary paper. Biochem. J. 19, 492–499.
Holmes, E. G., and Ashford, C. A. (1930). Lactic acid oxidation in brain with reference to the “Meyerof cycle.” Biochem. J. 24, 1119–1127.
Holmes, E. G., and Holmes, B. E. (1925b). Contributions to the study of brain metabolism. II. Carbohydrate metabolism. Biochem. J. 19, 836–839.
Hu, Y., and Wilson, G. S. (1997). A temporary local energy pool coupled to neuronal activity: fluctuations of extracellular lactate levels in rat brain monitored with rapid-response enzyme-based sensor. J. Neurochem. 69, 1484–1490. doi: 10.1046/j.1471-4159.1997.69041484.x
Hui, S., Ghergurovich, J., Morscher, R., Jang, C., Teng, X., Lu, W., et al. (2017). Glucose feeds the TCA cycle via circulating lactate. Nature 551, 115–118. doi: 10.1038/nature24057
Kasischke, K. A., Vishwasrao, H. D., Fisher, P. J., Zipfel, W. R., and Webb, W. W. (2004). Neural activity triggers neuronal oxidative metabolism followed by astrocytic glycolysis. Science 305, 99–103. doi: 10.1126/science.1096485
Krebs, H. A., and Johnson, W. A. (1937a). The role of citric acid in intermediary metabolism in animal tissue. Enzymologia 4, 148–156.
Krebs, H. A., and Johnson, W. A. (1937b). Metabolism of ketonic acids in animal tissues. Biochem. J. 31, 64–660.
Krebs, H. A., Salvin, E., and Johnson, W. A. (1938). The formation of citric and α-ketoglutaric acids in the mammalian body. Bichem. J. 32, 113–117.
Larrabee, M. G. (1996). Partitioning of CO2 production between glucose and lactate in excised sympathetic ganglia, with implications for brain. J. Neurochem. 67, 1726–1734. doi: 10.1046/j.1471-4159.1996.67041726.x
Li, X., Zhang, Y., Xu, L., Wang, A., Zou, Y., Li, T., et al. (2022). Ultrasensitive sensors reveal the spatiotemporal landscape of lactate metabolism in physiology and disease. Cell Metab. 34, 1–12. doi: 10.1016/j.cmet.2022.10.002
Locke, F. S., and Rosenheim, O. (1907). Contributions to the physiology of the isolated heart. The consumption of dextrose by mammalian cardiac muscle. J. Physiol. 36, 205–220.
Magistretti, P. J., Pellerin, L., Rothman, D. L., and Shulamn, R. G. (1999). Energy on demand. Science 283, 496–497. doi: 10.1126/science.283.5401.496
Mangia, S., Garreffa, G., Bianciardi, M., Giove, F., Di Salle, F., and Maraviglia, B. (2003). The aerobic brain: lactate decrease at the onset of neural activity. Neuroscience 118, 7–10. doi: 10.1016/S0306-4522(02)00792-3
Margolis, H. (1993). Paradigms and Barriers: How Habits of Mind Govern Scientific Beliefs. London: The University of Chicago Press Ltd.
Naifeh, J., Dimri, M., and Varacallo, M. (2022). Biochemistry, Aerobic Glycolysis. Treasure Island: StatPearls Publishing.
Passarella, S., de Bari, L., Valenti, D., Pizzuto, R., Paventi, G., and Altane, A. (2008). Miochondria and l-lactate metabolism. FEBS Lett. 582, 3569–3576. doi: 10.1016/j.febslet.2008.09.042
Pellerin, L., and Magistretti, P. J. (2003). Food for thought: challenging the dogmas. J. Cereb. Blood Flow Metab. 23, 1282–1286. doi: 10.1097/01.WCB.0000096064.12129.3D
Rogatzki, M.J., Ferguson, B.S., Goodwin, M.L., and Gladden, L.B. (2015). Lactate is always the end product of glycolysis. Front. Neurosci. 9, 22. doi: 10.3389/fnins.2015.00022
Schurr, A. (2006). Lactate: the ultimate cerebral oxidative energy substrate? J. Cereb. Blood Flow Metab. 26, 142–152. doi: 10.1038/sj.jcbfm.9600174
Schurr, A. (2014). Cerebral glycolysis: a century of persistent misunderstanding and misconception. Front. Neurosci. 8, 360. doi: 10.3389/fnins.2014.00360
Schurr, A. (2018). Glycolysis paradigm shift dictates a reevaluation of glucose and oxygen metabolic rates of activated neural tissue. Front. Neurosci. 12, 700. doi: 10.3389/fnins.2018.00700
Schurr, A., Dong, W-Q., Reid, K.H., West, C.A., and Rigor, B.M. (1988b). Lactic acidosis and recovery of neuronal function following cerebral hypoxia in vitro. Brain Res. 438, 311–314. doi: 10.1016/0006-8993(88)91354-6
Schurr, A., and Gozal, E. (2011). Aerobic production and utilization of lactate satisfy increased energy demands upon neuronal activation in hippocampal slices and provide neuroprotection against oxidative stress. Front. Pharmacol. 2, 96. doi: 10.3389/fphar.2011.00096
Schurr, A., and Gozal, E. (2015). Glycolysis at 75: is it time to tweak the first elucidated metabolic pathway in history? Front. Neurosci. 9, 170. doi: 10.3389/fnins.2015.00170
Schurr, A., Miller, J. J., Payne, R. S., and Rigor, B. M. (1999). An increase in lactate output by brain tissue serves to meet the energy needs of glutamate-activated neurons. J. Neurosci. 19, 34–39.
Schurr A. Passarella S. (2022) Aerobic Glycolysis: A DeOxymoron of (Neuro)Biology. Metabolites. 12, 72. 10.3390/metabo12010072
Schurr, A., and Payne, R. S. (2007). Lactate, not pyruvate, is neuronal aerobic glycolysis end product: an in vitro electrophysiological study. Neuroscience 147, 613–619. doi: 10.1016/j.neuroscience.2007.05.002
Schurr, A., Payne, R. S., Miller, J. J., and Rigor, B. M. (1997a). Brain lactate, not glucose, fuels the recovery of synaptic function from hypoxia upon reoxygenation: an in vitro study. Brain Res. 744, 105–111. doi: 10.1016/S0006-8993(96)01106-7
Schurr, A., Payne, R. S., Miller, J. J., and Rigor, B. M. (1997b). Brain lactate is an obligatory aerobic energy substrate for functional recovery after hypoxia: further in vitro validation. J. Neurochem. 69, 423–426. doi: 10.1046/j.1471-4159.1997.69010423.x
Schurr, A., West, C. A., and Rigor, B. M. (1988a). Lactate-supported synaptic function in the rat hippocampal slice preparation. Science 240, 1326–1328. doi: 10.1126/science.3375817
Serres, S., Bezancon, E., Franconi, J-M., and Merle, M. (2005). Ex vivo NMR study of lactate metabolism in rat brain under various depressed states. Neurosci. Res. 79, 1–15. doi: 10.1002/jnr.20277
Siesjo, B. K. (1981). Cell-damage in the brain: a speculative synthesis. J. Cereb. Blood Flow Metab. 1, 155–185.
Valenti, D., de Bari, L., Atlante, A., and Passarella, S. (2002). l-Lactate transport into rat heart mitochondria and reconstruction of the l-lactate/pyruvate shuttle. Biochem. J. 364, 101–104. doi: 10.1042/bj3640101
Keywords: glucose, glycolysis, lactate, lactate dehydrogenase, mitochondria, NADH (NAD+), oxydative phosphorylation (oxphos), trycarboxylic acid (TCA) cycle
Citation: Schurr A (2023) From rags to riches: Lactate ascension as a pivotal metabolite in neuroenergetics. Front. Neurosci. 17:1145358. doi: 10.3389/fnins.2023.1145358
Received: 16 January 2023; Accepted: 13 February 2023;
Published: 02 March 2023.
Edited by:
Claude Messier, University of Ottawa, CanadaReviewed by:
Baptiste Lacoste, Ottawa Hospital Research Institute (OHRI), CanadaGeorge A. Brooks, University of California, Berkeley, United States
Copyright © 2023 Schurr. This is an open-access article distributed under the terms of the Creative Commons Attribution License (CC BY). The use, distribution or reproduction in other forums is permitted, provided the original author(s) and the copyright owner(s) are credited and that the original publication in this journal is cited, in accordance with accepted academic practice. No use, distribution or reproduction is permitted which does not comply with these terms.
*Correspondence: Avital Schurr, avital.schurr@gmail.com