- School of Psychology, Faculty of Health and Behavioural Sciences, The University of Queensland, Brisbane, QLD, Australia
In the current EEG study, we used a dot-probe task in conjunction with backward masking to examine the neural activity underlying awareness and spatial processing of fearful faces and the neural processes for subsequent cued spatial targets. We presented face images under different viewing conditions (subliminal and supraliminal) and manipulated the relation between a fearful face in the pair and a subsequent target. Our mass univariate analysis showed that fearful faces elicit the N2-posterior-contralateral, indexing spatial attention capture, only when they are presented supraliminally. Consistent with this, the multivariate pattern analysis revealed a successful decoding of the location of the fearful face only in the supraliminal viewing condition. Additionally, the spatial attention capture by fearful faces modulated the processing of subsequent lateralised targets that were spatially congruent with the fearful face, in both behavioural and electrophysiological data. There was no evidence for nonconscious processing of the fearful faces in the current paradigm. We conclude that spatial attentional capture by fearful faces requires visual awareness and it is modulated by top-down task demands.
1. Introduction
Fearful expressions communicate information to other individuals regarding our perception of the environment. Specifically, we may express fear in response to dangerous events or threat. Therefore, fearful faces are usually perceived as indicators of negative events and they tend to attract our attention easily (Pourtois et al., 2004; Schupp et al., 2004). It has been reported that emotional faces including fearful faces can be detected faster and they elicit stronger neural activity, compared to neutral faces (for a review see Schindler and Bublatzky, 2020), even when people are unaware of them (Vuilleumier, 2005; Tamietto and De Gelder, 2010; Qiu et al., 2022c).
With regards to attentional capture, it has been shown that the presence of a fearful face can enhance the processing of a subsequent stimulus. Such modulatory effects of fearful faces on subsequent targets have been mainly examined using the dot-probe paradigm (e.g., Torrence and Troup, 2018). In this paradigm, a pair of face stimuli is presented before a lateralised target stimulus. The lateralised target can be presented in the same spatial location as the emotional face that precedes it (the congruent condition), or at the location opposite to the emotional face (the incongruent condition). The response to the lateralised target is measured, and the differences between congruent and incongruent conditions can be used as an index of spatial attention to the preceding faces. Previous research has repeatedly shown that a target (e.g., a dot or a letter) can be detected faster (Carlson and Reinke, 2008, 2010; Torrence et al., 2017) or discriminated more accurately (Pourtois et al., 2004) when it follows the emotional face in the spatially congruent condition, compared to when it is spatially incongruent to the emotional face (but see van Rooijen et al., 2017).
Neural imaging studies have provided supporting evidence for these behavioural observations. For example, in a dot-probe experiment using electroencephalography (EEG) recording, Carlson and Reinke (2010) presented participants with pairs of faces as the cues and a lateralised dot as the target. At the behavioural level, they found that participants’ reaction time towards dots in the congruent condition was shorter than the incongruent condition. Additionally, the neural activity, indexed by event-related potentials (ERPs), at posterior electrodes were found to be enhanced by a lateralised fearful face compared to a neutral face. The magnitude of the increase in the ERPs, in particular the face-sensitive N170, positively correlated with the reaction time difference between congruent and incongruent trials. These results were taken to suggest that fearful faces attracted spatial attention and facilitated task performance for congruent targets. However, to show that fearful faces indeed modulate attention to the subsequent targets, it would have been more compelling to show that fearful faces alter the neural response to the subsequent targets. The target-related ERPs were not reported in the study (Carlson and Reinke, 2010), leaving it an open question how attention to fearful faces modulates neural activity for the targets.
In an object-substitution masking study by Giattino et al. (2018), face and house images were used as cueing stimuli, presented subliminally (for 17 ms) and subsequently masked. Participants were required to detect a target rectangle that was either validly or invalidly cued. It was found that the participants’ ability to detect the cue stimuli was no different from chance-level guessing (Giattino et al., 2018). However, participants’ early neural activity (i.e., P1) in response to the target stimuli was enhanced when the targets were validly cued, even in trials where participants reported not being aware of the preceding cue. This cue validity effect was also found in the behavioural data such that participants localised the targets faster and more accurately in the congruent condition, even when participants were not aware of the cues (Giattino et al., 2018). Thus, in the absence of awareness of face cues, the spatial information about them was processed to a level where it modulated the neural responses to the subsequent stimuli.
In a series of backward masking experiments, we analysed ERPs for fearful faces presented in face pairs with different visibility (subliminal and supraliminal viewing conditions; Qiu et al., 2022a, 2023). We used 16 ms of presentation time in the subliminal viewing condition, as opposed to 33 ms which was used in Carlson and Reinke (2010), for a stronger impeding effect on visual awareness (Milders et al., 2008). Our results showed that fearful faces can attract spatial attention by eliciting an N2-posterior-contralateral (N2pc), only when the faces were presented above the awareness threshold (266 ms; supraliminal viewing) and when they were relevant to participants’ tasks (Qiu et al., 2022a). Although subliminal fearful faces did not elicit an N2pc, some fear-related non-spatial enhancement effect is present in the data (Qiu et al., 2022d). We then ask whether any of the fear-related effects are sustained and can modulate the neural response to stimuli presented after the faces (i.e., modulate target-related EEG signals). Importantly, we ask whether any of the effects require visual awareness, or they can occur as nonconscious processes.
To answer these questions, in the current study, we used a dot-probe task together with the backward masking technique. Specifically, pairs of faces were presented either briefly (for 16 ms) or for a longer time (166 ms) and immediately backward masked. A following lateralised dot either appeared on the same side as the fearful face (congruent) or on the side opposite to it (incongruent). In one half of the experiment, the participants were required to respond to the target dots as well as the faces that preceded them, whereas in the other half of the experiment, participants were instructed to ignore the faces.
For the face stimuli, we expected to find an N2pc for the fearful face only in the supraliminal viewing condition, replicating our previous finding (Qiu et al., 2022a). For the target dot stimuli, we expected dots in the congruent conditions to be detected faster than the incongruent condition. We predicted that the early ERPs (e.g., P1) for congruent dots would be enhanced compared to incongruent dots. Further, if fearful faces can be processed nonconsciously, such cue validity effect should be observed in both supraliminal and subliminal face presentations. Data were also analysed with a multivariate approach to examine neural patterns associated with the variables of interest (i.e., fearful face location, congruency) which may not be revealed in univariate ERP analyses.
2. Materials and methods
2.1. Participants
We determined the sample size in MorePower (Campbell and Thompson, 2012) using an effect size from a previous study with a similar design (ηp2 = 0.22; Carlson and Reinke, 2008). A minimum of 24 participants were required for a significant main effect of congruency in reaction time in a 3(fearful-face-dot congruency: congruent, incongruent, control) x 2(face-visibility: subliminal, supraliminal) x 2(face-relevancy: relevant, irrelevant) design with an effect size of 0.22 (power = 0.9, two-tailed alpha = 0.05). Thirty-one participants were recruited and were compensated with either course credits or $40 AUD. Data from five participants were excluded after data pre-processing (see below). Therefore, 26 participants constituted the final sample (Mage = 21.9, SDage = 2.1, 8 males, 18 females). This study was approved by the University of Queensland ethics committee.
2.2. Apparatus and experimental stimuli
The experiment was programmed and run in PsychoPy 3 (Peirce et al., 2019) and all stimuli were presented on a 24-inch ASUS LCD monitor (resolution: 1920 × 1,080 pixels) placed 70 cm away from the participant’s eyes.
Face stimuli were obtained from the Radboud Face Database (Langner et al., 2010). We used fearful and neutral face images from 10 different models (five females and five males). Non-face information including hair was removed by cutting the face images into oval shapes (6.5°x 5.1 in visual angle; see Figure 1A). The mask stimuli were created by scrambling the neutral face images for each model using the Scramble Filter tool1 such that each mask image consisted of 208 randomly scrambled squares (4.4 mm x 4.4 mm each), see Figure 1B. In this experiment, we used a bilateral presentation of faces and mask stimuli. Each lateralised stimulus was presented 4.1° (in visual angle) away from a central fixation on the screen. The possible face combinations included (a) fearful face on the left and neutral face on the right (fearful-face-on-left), (b) neutral face on the left and fearful face on the right (fearful-face-on-right), and (c) two neutral faces.
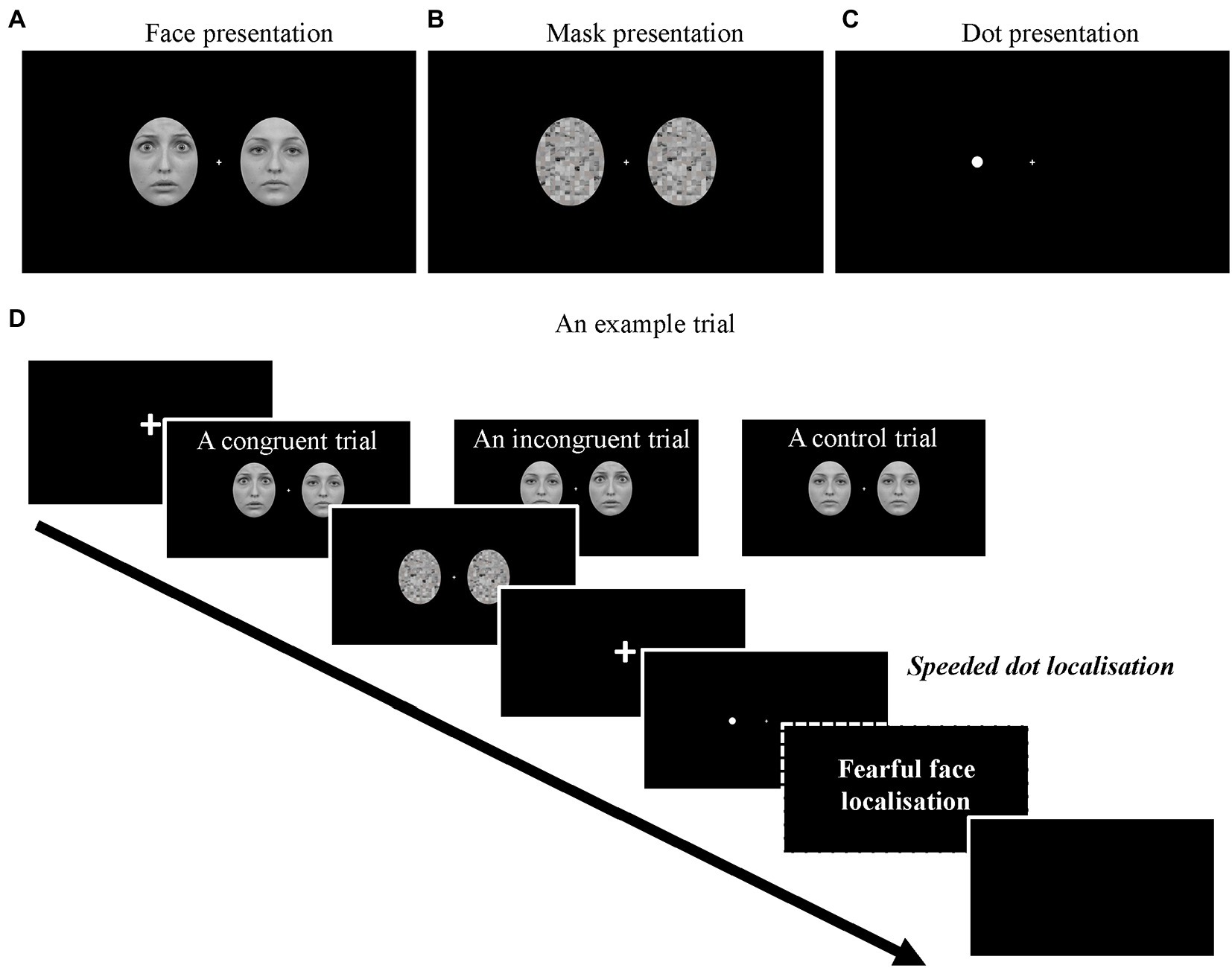
Figure 1. Examples of (A) the face images (Fearful-on-left) and (B) mask images. (C) An example of the lateralised target dot (Dot-on-left). (D) The full sequence of a trial. Note that the fearful face localisation task was only required in the face-relevant conditions.
A white disc (a dot) extending 0.25°x 0.25 in visual angle was used as the target stimulus in the current dot-probe paradigm (Figure 1C). The distance between the centre of the dot and the central fixation was 4.1° in visual angle.
All images were rendered black-and-white and were presented on a black screen. Image editing was performed in Adobe Photoshop (version 22.4.0).
2.3. Procedure
As shown in Figure 1D, at the start of each trial, a fixation screen was presented with a variable duration between 500–800 ms. Then, a pair of face stimuli appeared for either 16 ms (subliminal) or 166 ms (supraliminal), immediately followed by a pair of mask stimuli for either 166 ms or 16 ms, making the total duration of faces and masks the same across conditions. Afterwards, a fixation screen was presented for 66 ms (Torrence et al., 2017) and was followed by a lateralised dot presented either on the left or the right side of the screen for 750 ms. In a small proportion of the trials (360 trials in total), there was no dot following the mask (baseline condition). The baseline trials were introduced for us to obtain clean dot-related ERPs (see Data analysis). Upon the onset of the dot presentation (or the blank screen in the baseline condition), participants were required to correctly localise the dot as quickly as possible (left arrow key = dot on left; right arrow key = dot on right; both keys = no dot) with their right hand. If no response was made within 1,000 ms after the onset of the dot, a prompt “Too slow!” would be presented on the screen.
There were two types of blocks in the experiment: face-relevant and face-irrelevant blocks. In the face-relevant blocks, participants were instructed to first perform the dot localisation task. Then, they were required to indicate on which side of the screen they saw a fearful face (Q key = fearful face on left; E key = fearful face on right; W = no fearful face/two neutral faces) with their left hand. In the face-irrelevant blocks, participants were instructed to respond only to the dots and ignore the faces. That is, they only needed to perform the speeded dot localisation task in these blocks. Each of the face combinations was presented equiprobably in all conditions, and the dot presentations were manipulated orthogonally to the face presentations.
There were three conditions regarding the location of the fearful face and the lateralised dot in each trial: congruent condition, where the fearful face and the subsequent dot were presented on the same side of the screen, incongruent condition, where they were presented on different sides of the screen and the control condition where the two neutral faces were presented before the dot.
There were 16 blocks of 1,200 trials (including 360 baseline trials) in total with short breaks provided between blocks. Participants completed eight face-relevant blocks in either the first half or the second half of the experiment and completed the eight face-irrelevant blocks in the other half of the experiment. This order was determined randomly by the experimental programme for each participant.
2.4. EEG data recording and pre-processing
Raw continuous EEG was recorded at 1024 Hz using the BioSemi ActiveTwo system (Biosemi, Amsterdam, Netherlands). Sixty-four electrodes were placed according to the international 10–20 system location. Horizontal electrooculogram (EOG) was recorded with two bipolar electrodes. Vertical EOG was recorded with an external electrode placed below participants’ left eye. Recordings were referenced online to the CMS/DRL electrodes.
Pre-processing of the EEG data was performed with EEGLAB (Delorme and Makeig, 2004) and ERPLAB (Lopez-Calderon and Luck, 2014). We interpolated electrodes that produced noise throughout the experiment. Signals were re-sampled to 512 Hz offline, filtered from 0.1 to 30 Hz and notch-filtered at 50 Hz to remove line noise. All signals were then re-referenced to the average of all electrodes. EEG data were segmented into epochs with a time window of 600 ms from the onset of the faces and the dots, respectively for the face-related analyses and for the dot-related analyses, using a pre-stimulus baseline (−100 to 0 ms). Independent component analysis was performed on the epoched data to identify and remove eye-blink and eye-movement components in the signals. After eye-related components were removed, epochs containing other artefacts were detected and removed on a trial-by-trial basis through visual inspection. Consequently, data from five participants were excluded for further analyses due to the limited number of epochs remaining (i.e., fewer than 40 trials for each condition of interest). On average, 91% epochs were kept for the remaining participants (N = 26).
2.5. Data analysis
At the behavioural level, we examined the reaction time data from the speeded dot localisation task with a repeated-measures ANOVA. We only used reaction time data from the task-correct trials and excluded datapoints identified as outliers from an outlier cheque (i.e., beyond 3rd quartile +1.5*interquartile) for each participant. We did not analyse the accuracy data for the dot localisation task because the accuracy was near ceiling (percent correct: M = 0.96, SD = 0.03). For the fearful face localisation task, we examined the accuracy data with a paired-samples t-test. All behavioural data analyses were performed in IBM SPSS Statistics 27.
For the EEG data, we separately examined the face-related signals and dot-related signals, using both a univariate approach and a multivariate method.
2.5.1. Mass univariate analysis (ERP analysis)
We conducted ERP analyses using the factorial mass univariate analysis toolbox (Fields and Kuperberg, 2020) and the mass univariate analysis toolbox (for pairwise comparisons; Groppe et al., 2011).
Because we were interested in the early visual processing of the stimuli, posterior electrodes were selected as regions of interest. Only lateral electrodes were included in ERP analyses due to the lateralised stimulus configuration. As a result, the electrodes of interest are P3/4, P5/6, P7/8, P9/10, PO3/4, PO7/8 and O1/2. For significant difference testing, we performed the cluster-based permutation test (10,000 permutations) on all time-points within the epoch (0-600 ms), with a family-wise α level of 0.05. Electrodes were considered as spatial neighbours if adjacent electrodes were within 3.3 cm from each other (Mean spatial neighbours = 2.9; cluster inclusion p < 0.05). Follow-up comparisons were conducted using the cluster-based permutation t-tests (two-tailed family-wise α = 0.05).
For the analyses on the face-related signals, because we were interested in ERP components that are calculated as difference waves between lateral electrodes (i.e., N2pc), we collapsed the left and right electrodes and retained the information about the relation between the location of the fearful face and electrodes. Specifically, in the two fearful-face-present conditions, signals were relabelled based on whether they were contralateral or ipsilateral to the fearful face. For the neutral faces condition, the average signals between the left and right electrodes were calculated.
For the analyses on the dot-related signals, we first subtracted signals in the dot-absent baseline trials from the dot-present trials to remove effects from the preceding face stimuli. Specifically, the ERPs time-locked to the dot onset from the baseline condition would be subtracted from the ERPs from the experimental condition (i.e., dot-present) that was preceded by the same face combination (e.g., fearful-on-left). Then, we averaged signals from the left and right electrodes. Analyses were performed on the average baseline-subtracted ERPs.
2.5.2. Multivariate pattern analysis
We also examined the data with a multivariate approach using the CoSMoMVPA toolbox (Oosterhof et al., 2016) and LIBSVM (Chang and Lin, 2011). We used a radial kernel support vector machine on each time point to find the decision boundary that discriminated between patterns of two conditions of interest using signals across 16 posterior electrodes (P3/4, P5/6, P7/8, P9/10, PO3/4, PO7/8, O1/2, POz, and Oz). Then, data were spatially filtered with surface Laplacian (Kayser and Tenke, 2006) and temporally smoothed with a Gaussian-weighted running average of 20 ms. Classification was performed on each time point and 4 neighbouring time points to avoid the overfitting issue (Grootswagers et al., 2017). Single-trial data were partitioned into 10 chunks and the two classification targets were equally likely to occur in each chunk. Following a leave-one-out procedure, each classifier at each time point was trained on data from nine chunks and tested on the remaining chunk. Decoding accuracies of all iterations were then averaged at each time point for each participant. Statistical significance testing was conducted using one-sample t-tests (against chance-level decoding performance at 50%). The t statistics were corrected for multiple comparisons using Threshold-Free Cluster Enhancement (TFCE) and Monte Carlo-based permutations (Oosterhof et al., 2016). Briefly, a null distribution was acquired through flipping the sign of the statistics across time points for a random half of participants, iteratively for 10,000 times. The observed TFCE statistic at each time point was considered significant if its value was larger than the 95th percentile of the null distribution (i.e., p < 0.05 for a one-tailed test; Smith and Nichols, 2009).
For the face-related signals, we decoded the spatial location of the fearful face (fearful-face-on-left vs. fearful-face-on-right) for each condition of face-visibility (collapsing across face-relevancy), and decoded the fearful face location for each condition of face-relevancy (collapsing across face-visibility). All signals were time-locked to the face onset.
For the dot-related signals, we performed decoding of fearful-face-dot congruency (congruent vs. incongruent) across all conditions and also separately for the face-subliminal and face-supraliminal conditions. All signals were time-locked to the dot onset.
3. Results
3.1. Behavioural data
3.1.1. Fearful face localisation task
A paired-samples t-test on the accuracy data (percent correct) for the fearful face localisation task revealed that the fearful face was more accurately localised in the supraliminal condition (M = 0.74, SD = 0.19) than the subliminal condition (M = 0.34, SD = 0.02), t(25) = 10.51, p < 0.001, d = 2.06. A one-sample t-test showed that the accuracy in the subliminal condition was not different from chance-level performance (0.33), t(25) = 0.92, p = 0.368.
3.1.2. Dot localisation task
A 3(fearful-face-dot congruency) x 2(face-visibility) x 2(face-relevancy) repeated-measures ANOVA on the reaction times (in seconds) revealed a significant main effect of face-visibility (F(1, 25) = 28.05, p < 0.001, ηp2 = 0.53), a main effect of face-relevancy (F(1, 25) = 92.63, p < 0.001, ηp2 = 0.79) and a significant interaction between the two, F(1, 25) = 27.01, p < 0.001, ηp2 = 0.52. Specifically, participants were faster at localising the dot in the face-irrelevant condition (M = 0.34, SD = 0.04) than face-relevant condition (M = 0.44, SD = 0.06), and when the preceding faces were presented subliminally (M = 0.38, SD = 0.04) than when presented supraliminally (M = 0.40, SD = 0.05). The effect of face-relevancy was significant in both face-visibility conditions, ps < 0.001. However, the effect of face-visibility was significant only when the faces were task-relevant, p < 0.001. When participants did not need to attend to the faces, no difference was found between face-subliminal and face-supraliminal conditions, p = 0.295.
The interaction between face-visibility and congruency was also significant, F(2, 50) = 22.92, p = 0.008, ηp2 = 0.20. Follow-up tests showed that, the effect of congruency was non-significant in the face-subliminal condition, F(2, 50) = 1.33, p = 0.274, but was significant in the face-supraliminal condition, F(2, 50) = 3.98, p = 0.025, ηp2 = 0.14. As part of our planned comparisons, the levels of congruency were compared against each other in the face-supraliminal condition. We found that, the dot was localised significantly slower in the incongruent condition, compared to the congruent condition, p = 0.023, and the control condition, p = 0.018. The difference between congruent and control conditions was non-significant, p = 0.508. No other effect was significant, Fs < 2.25, ps > 0.116.
3.2. Mass univariate analysis
3.2.1. Face-related ERPs
For ERPs time-locked to the face stimuli, we collapsed the left and right electrodes to create the laterality variable which indicates the relation between the location of a fearful face and the electrodes. A 3(laterality based on the location of a fearful face: contralateral, ipsilateral, control) x 2(face-visibility: subliminal, supraliminal) x 2(face-relevancy: relevant, irrelevant) repeated-measures ANOVA revealed that all main effects were significant, Fs > 3.19, ps < 0.001. As shown in Figure 2A, ERPs were significantly more negative in the supraliminal compared to the subliminal condition between 113–281 ms (temporal peak: 215 ms) with a maximal effect at P9/10, and between 293–594 ms across all posterior electrodes. ERPs in the face-relevant condition were overall more negative than those in the face-irrelevant condition between 262–594 ms (temporal peak: 348 ms) with a maximal effect on P3/4, see Figure 2B. ERP waveforms are presented in Figure 2C.
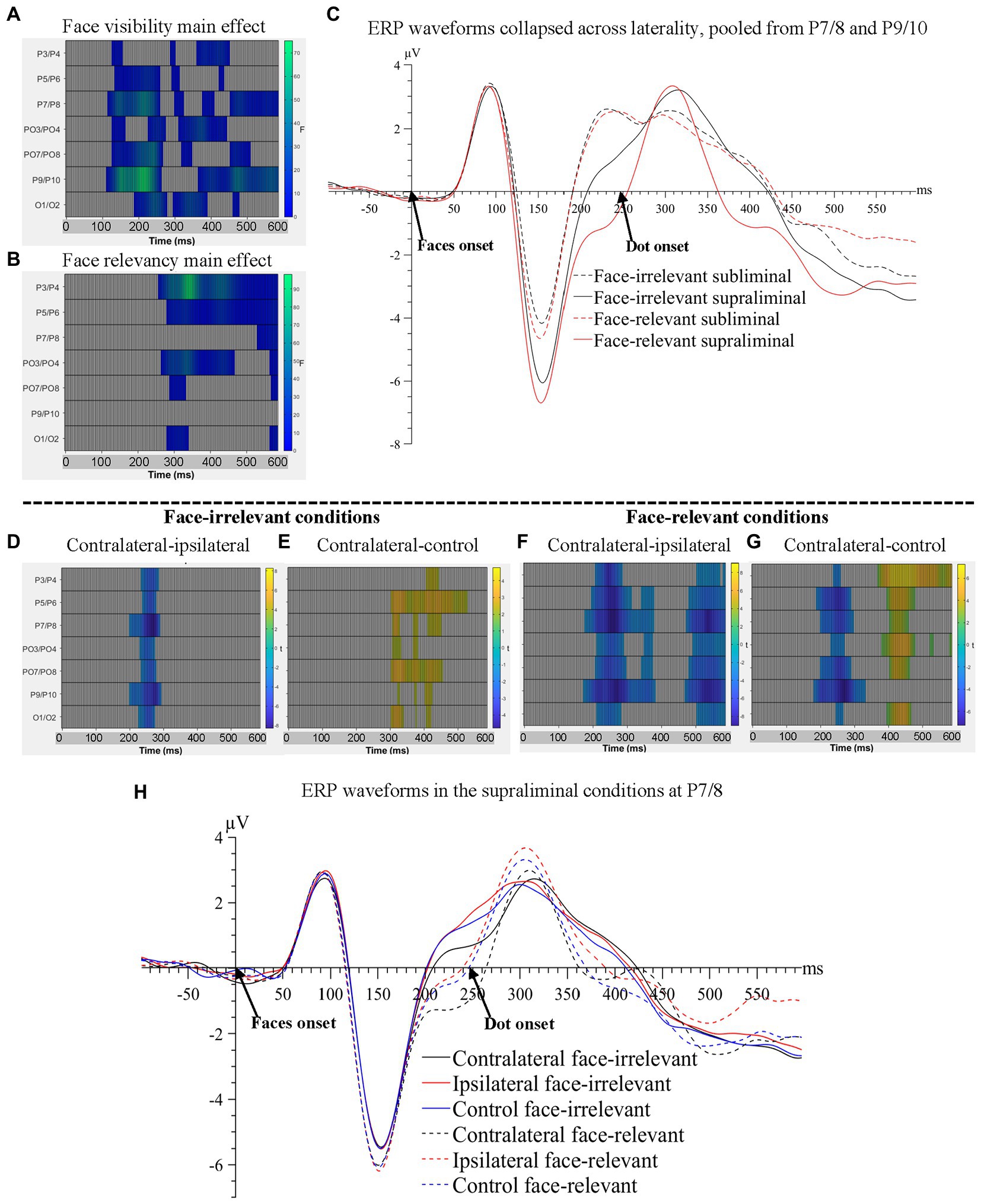
Figure 2. Raster plots for (A) the main effect of face-visibility and (B) the main effect of face-relevancy for face-related ERPs. (C) ERP waveforms collapsed across laterality conditions, pooled over P7/8 and P9/10, the two pairs of electrodes that showed the maximal main effect of face-visibility and the maximal interaction effect between face-visibility and face-relevancy. Raster plots for the contrasts between (D) contralateral and ipsilateral signals and between (E) contralateral and control signals, in the face-irrelevant conditions; between (F) contralateral and ipsilateral signals and between (G) contralateral and control signals, in the face-relevant conditions. (H) ERP waveforms for different conditions of laterality and face-relevancy in the supraliminal condition at electrodes P7/8, the pair that showed the maximal interaction effect between laterality and face-relevancy.
All interaction effects were significant including the three-way interaction between laterality, face-visibility and face-relevancy, Fs > 3.19, ps < 0.009. Follow-up tests revealed that the effect of laterality was significant only in the supraliminal conditions. Thus, we compared three levels of laterality against each other in the supraliminal condition, separately for face-relevant and face-irrelevant conditions, using the cluster-based permutation t-tests.
When the supraliminally-presented faces were task-irrelevant, signals contralateral to the fearful face were more negative than ipsilateral signals between 203–297 ms at posterior electrode sites (temporal peak: 273 ms; spatial peak: P7/8; Figure 2D), reflecting an N2pc for the fearful face. Additionally, contralateral signals were more positive than signals in the control condition in a later time window spanning from 309 to 535 ms (temporal peak: 332 ms; spatial peak: PO7/8; Figure 2E).
When the supraliminally-presented faces were task-relevant, two negative clusters at posterior electrode sites were significant when comparing contralateral against ipsilateral signals: 176-387 ms (temporal peak: 262 ms; spatial peak: P7/8), again reflecting an N2pc for the target fearful face, and a later time window, 477-600 ms (temporal peak: 543 ms; spatial peak: P7/8), see Figure 2F. Contralateral signals were also more negative than signals in the control condition between 184–336 ms (temporal peak: 273 ms; spatial peak: P9/10; Figure 2G). One additional positive cluster was found when contrasting contralateral against control conditions: 402-484 ms (temporal peak: 453 ms; spatial peak: P3/4).
Importantly, the later negativity between 477–600 ms in the contralateral-ipsilateral contrast (Figure 2F) likely reflected the sustained posterior contralateral negativity (SPCN; Luria et al., 2016), an ERP marker associated with working memory consolidation for task-relevant fearful faces. Note that the SPCN was not found in the face-irrelevant condition (Figure 2D). The ERP waveforms are plotted in Figure 2H.
To summarise, starting from 113 ms post-face-onset, significant effects of all three variables of interest (face-visibility, face-relevancy and laterality) were found on the ERPs, encompassing time windows of the N2pc and the SPCN.
3.2.2. Dot-related ERPs
For ERPs time-locked to the target dots, because we did not have a hypothesis about the contralateral and ipsilateral signal differences, we averaged signals from the left and right electrodes, regardless of the spatial relation between the target and the electrodes (for a similar procedure see Giattino et al., 2018). A 3(fearful-face-dot congruency) x 2(face-visibility) x 2(face-relevancy) repeated-measures ANOVA. As shown in Figure 3, the main effects of face visibility and face-relevancy, and the interaction between the two were significant, Fs > 4.26, ps < 0.028.
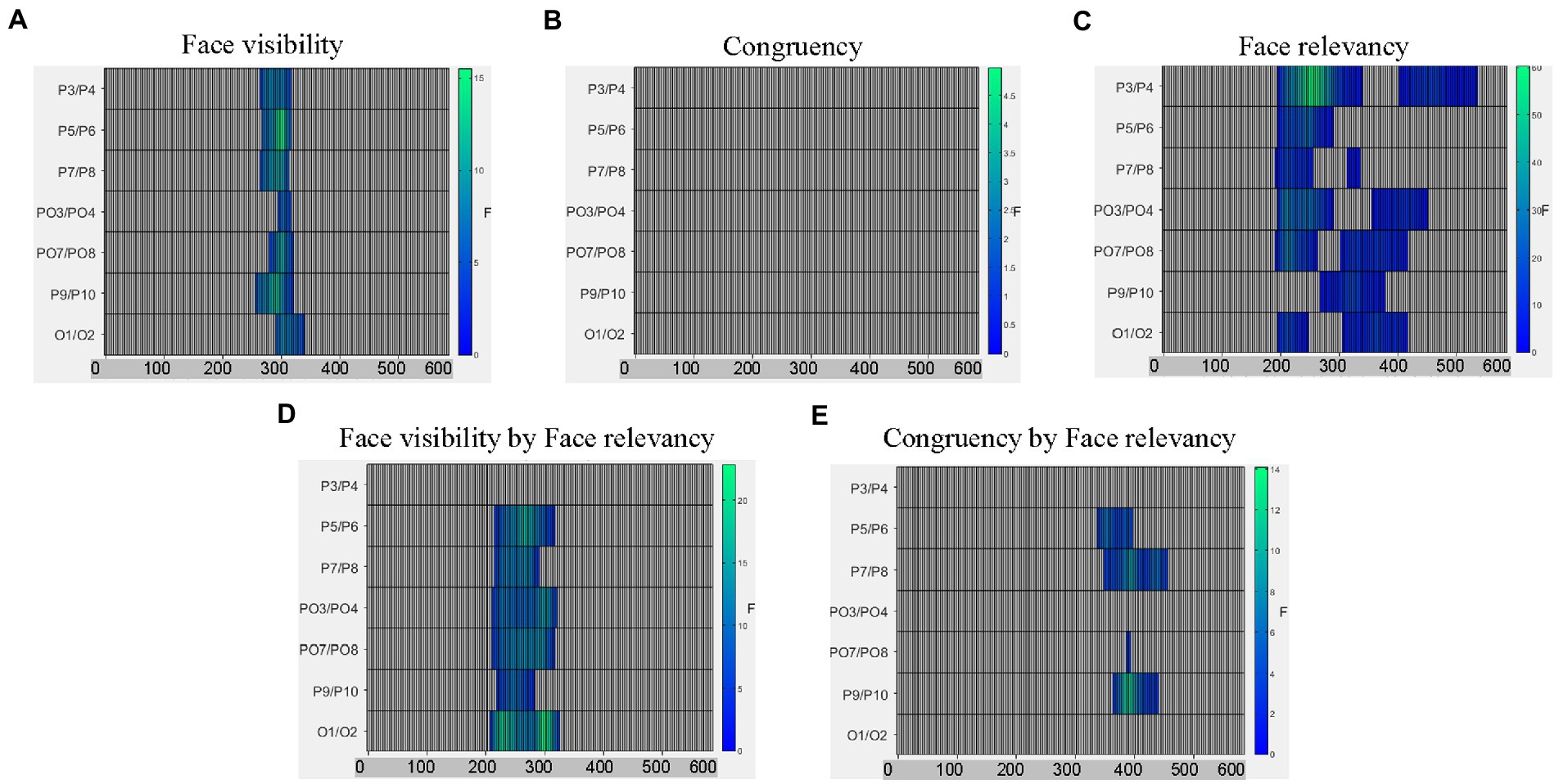
Figure 3. Raster plots for (A) the main effect of face visibility; (B) the non-significant main effect of congruency; (C) the main effect of face relevancy; (D) the interaction between face visibility and face relevancy; and (E) the interaction between congruency and face relevancy.
To follow up the interaction effect between face-visibility and face-relevancy on the dot-related ERPs, we examined the differences between the two face-relevancy conditions (face-irrelevant minus face-relevant), separately for when the faces were presented subliminally and when presented supraliminally.
When the preceding faces were presented subliminally, there was a significant negative cluster between 246–445 ms across electrodes P7/8, PO3/4, PO7/8, P9/10 and O1/2 with the maximal effect found on O1/2 (temporal peak: 316 ms), see Figure 4A. Therefore, the mid-latency N2 for dots in the face-relevant condition was smaller in this time window, compared to the face-irrelevant condition.
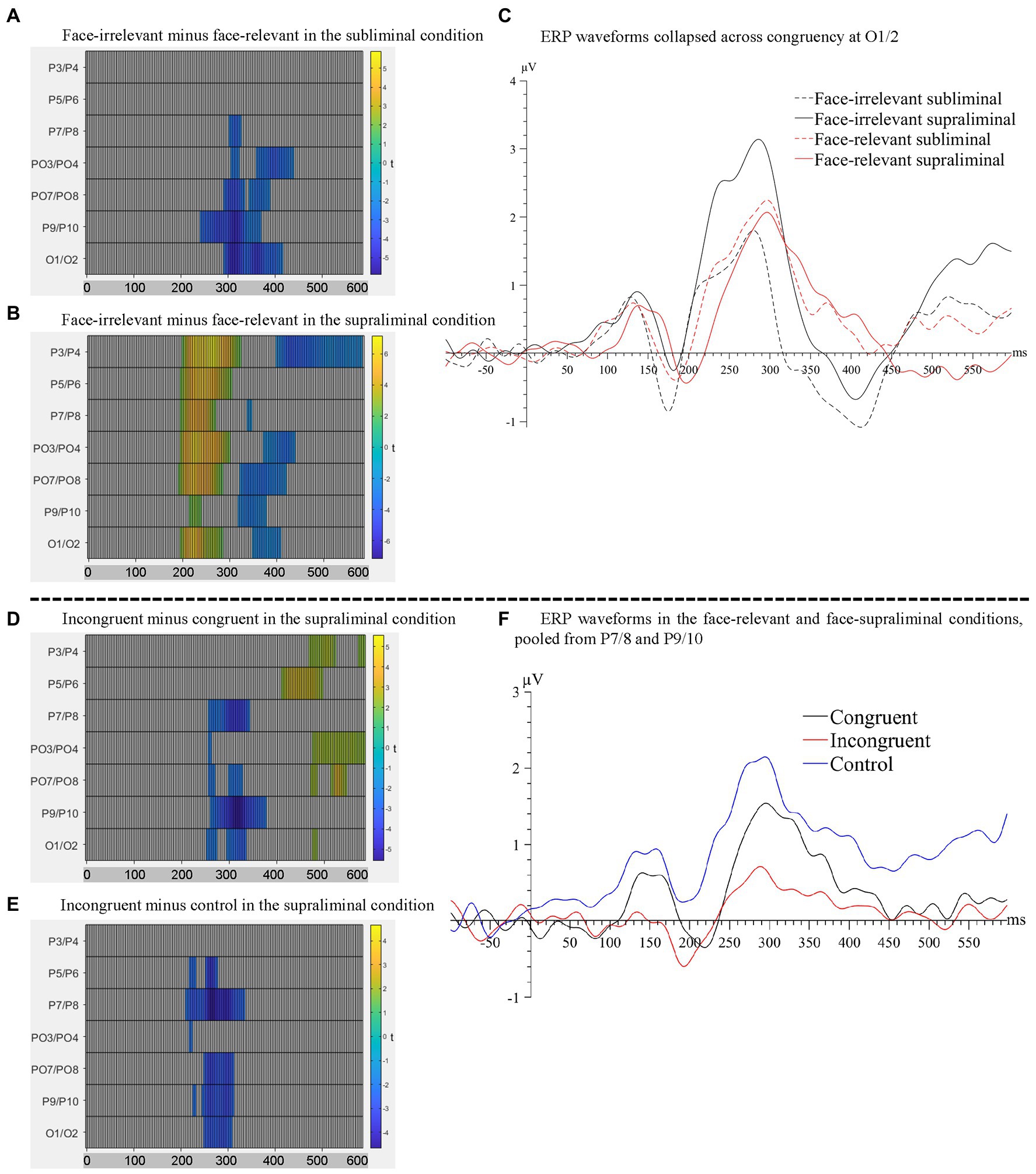
Figure 4. Raster plots for the contrasts between (A) face-irrelevant and face-relevant signals in the subliminal condition and between (B) face-irrelevant and face-relevant signals in the supraliminal condition. (C) ERP waveforms collapsed across congruency conditions at O1/2, the pair of electrodes showing the maximal interaction effect between face-relevancy and face-visibility. Raster plots for the contrasts between (D) incongruent and congruent signals and between (E) incongruent and control signals, in the supraliminal condition. (F) ERP waveforms for each congruency level in the face-relevant and face-supraliminal condition, pooled over P7/8 and P9/10.
When the preceding faces were presented supraliminally, there was a significant positive cluster between 195–328 ms across all posterior electrodes with the maximal effect found on P3/4 (temporal peak: 266 ms). In a later time window of 324-445 ms, signals in the face-relevant condition continued to be attenuated, compared to face-irrelevant condition, see Figure 4B. Combined with the ERP waveforms (Figure 4C), it appears that the mid-latency ERPs (i.e., between 195–445 ms) for dots were attenuated overall by a pair of preceding task-relevant faces, especially when they were presented supraliminally (Figure 4C). This is consistent with our behavioural finding of a slower reaction time to the target dots in the face-relevant condition, compared to face-irrelevant condition.
From the omnibus analysis on dot-related signals, the main effect of congruency was not significant. However, the interaction between congruency and face-relevancy was significant (see Figure 3), F = 3.22, p = 0.042. A simple effect test revealed that the congruency effect was significant only in the face-relevant condition, F = 3.18, p = 0.031. As part of our planned comparisons, we compared levels of congruency (congruent, incongruent, control) against each other at each level of face visibility, in the face-relevant condition.
When the faces were presented subliminally, there were no significant differences in any of the comparison pairs. However, when the faces were presented supraliminally, signals in the incongruent condition were more negative than in the congruent condition between 258–383 ms (spatial peak: P9/10; temporal peak: 320 ms), see Figure 4D. Signals in the incongruent condition were also more negative than the control condition between 215–340 ms (spatial peak: P7/8; temporal peak: 270 ms), see Figure 4E. Combined with the ERP waveforms (Figure 4F), it appears that the P2 for the dots was smaller when they were presented spatially incongruent (opposite) to the preceding fearful face, compared to when they were presented at a spatially congruent location. Thus, it appears that the processing of a target dot was impaired when it was presented at an incongruent spatial location following a task-relevant and visible fearful face, and such impaired processing was accompanied by a slower reaction to the target dot behaviourally.
To summarise, modulations of all three variables (face-visibility, face-relevancy and congruency) were found on the mid-latency ERPs. Notably, the effect of congruency was significant only in the face-visible and face-relevant condition.
3.3. Multivariate pattern analysis
3.3.1. Decoding the spatial location of fearful faces
Using MVPA, we decoded the neural activity associated with the spatial locations of the fearful faces (fearful-face-on-left vs. fearful-face-on-right) in the subliminal and supraliminal conditions, separately, using signals time-locked to the onset of the face stimuli. The decoding was successful only in the supraliminal conditions (Figure 5A, left panel), with the accuracy significantly above chance level at ~53% (SEM = 0.72) between 270–289 ms. However, the decoding accuracy was at chance-level in the subliminal conditions (Figure 5B, left panel). These results were in line with the ERP results in showing that the spatial location of fearful faces was decodable only in conditions where participants were aware of the face stimuli.
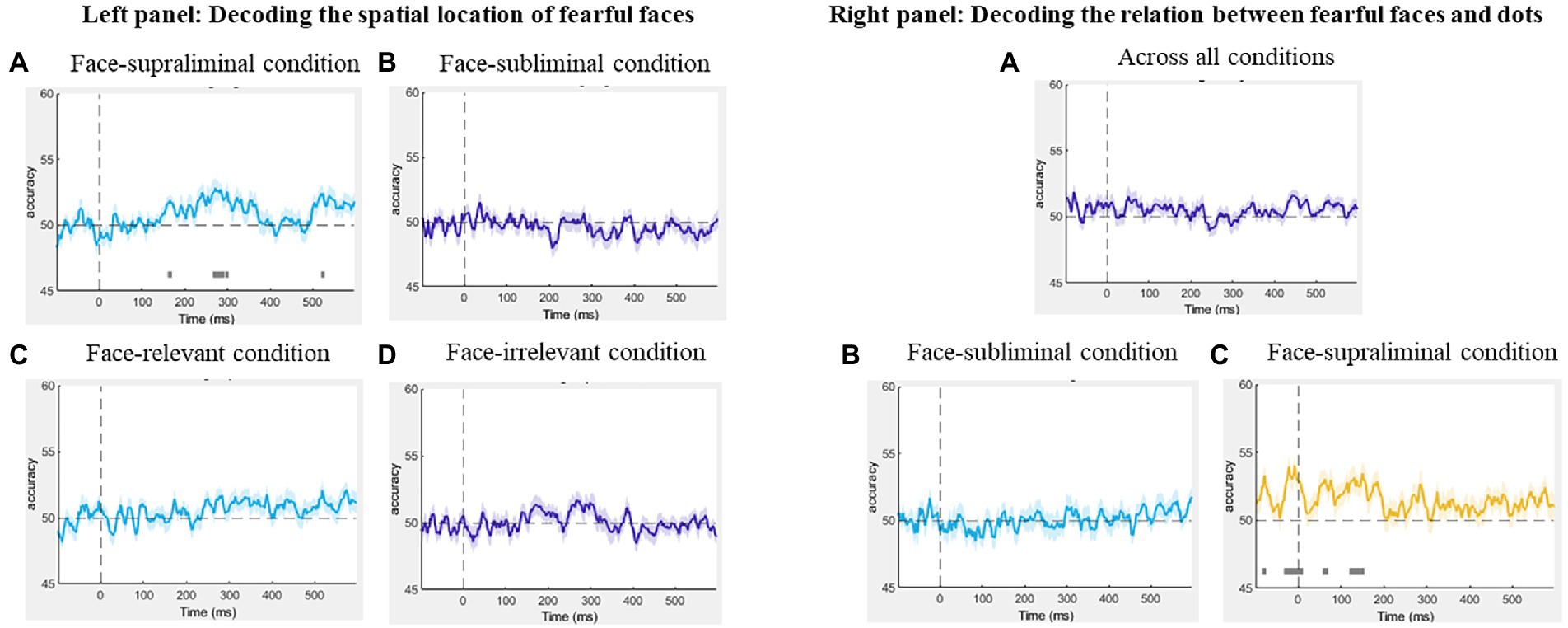
Figure 5. Left panel: Results of the decoding of spatial location of fearful faces in the (A) face-supraliminal condition, (B) face-subliminal condition, (C) face-relevant condition and (D) face-irrelevant condition. Right panel: Results of the decoding of the relation between fearful faces and the dots (A) across all conditions, (B) in the face-subliminal condition and (C) in the face-supraliminal condition.
We also decoded the spatial location of the fearful face separately in the task-relevant and task-irrelevant conditions, pooling over visibility. The decoding performance from both analyses was at chance-level throughout the entire epoch (Figures 5C,D, left panel), showing that the location of the fearful face was not decoded in either condition.
3.3.2. Decoding the relation between a fearful face and the subsequent stimulus
To examine if there was any neural pattern associated with the fearful-face-dot congruency, we decoded the neural activity between fearful-face-dot congruent and fearful-face-dot incongruent trials, using signals time-locked to the onset of the target dots. We performed the decoding first across all conditions and then separately for the face-subliminal and face-supraliminal conditions. No successful decoding of the fearful-face-dot relation was found overall (Figure 5A, right panel) or in the face-subliminal condition (Figure 5B, right panel). In the face-supraliminal condition, the decoding of congruency returned some significant results between −29 and 8 ms (Maccuracy = 49%, SEM = 0.69) and between 123 and 150 ms (Maccuracy = 50%, SEM = 0.57) (Figure 5C, right panel). Considering the near-chance decoding accuracies, we do not argue that there is very strong evidence for a successful decoding of congruency in the current analysis.
4. Discussion
Using the dot-probe paradigm with backward masked faces, we examined neural activity associated with the processing of fearful expressions, as well as subsequent visual targets.
From our mass univariate analysis on the dot-evoked signals, we found that, in the supraliminal face-relevant condition, mid-latency ERP signals were stronger for dots that appeared at the same locations as the fearful face (congruent condition), compared to when they were presented at locations opposite the fearful face (incongruent condition). Behaviourally, we found evidence for this cue validity effect, again only when the preceding face stimuli (cues) were presented supraliminally and when they were task-relevant. These results are consistent with numerous reports of the facilitatory effects of an emotional face on validly cued stimuli (for a review see Torrence and Troup, 2018). Crucially, this effect was not observed when the preceding faces were presented subliminally or when they were task-irrelevant. Therefore, the fearful face-related modulatory effect on subsequent stimuli requires conscious awareness of and top-down attention to the faces. In addition, our MVPA revealed a very low accuracy for congruency decoding. One potential reason for this could be that, in the MVPA, the baseline un-subtracted signals (dot-present ERPs) were used, and more noise introduced by neural patterns associated with the preceding face stimuli was present in the data, which resulted in a rather noisy and low decoding performance of the variable of interest (congruency).
Our second main finding is that supraliminally-presented fearful faces elicited an N2pc towards them, regardless of whether the faces were task-relevant or not. Whilst this finding is consistent to some existing research (e.g., Bar-Haim et al., 2005; Eimer and Kiss, 2007), inconsistent conclusions have been made in more recent studies including our own work (Lien et al., 2013; Zhou et al., 2020; Qiu et al., 2022a, 2023).
Although we failed to find an N2pc for task-irrelevant fearful faces in our previous experiments (Qiu et al., 2022a, 2023), our current findings do not contradict these previous findings. Specifically, task-relevancy was implemented via different methods across studies. The task-induced attentional load (Lavie et al., 2004; Lavie, 2005) varied largely between the current study and our previous ones, which could have led to two distinctly different conclusions. In our previous studies, the target non-face stimuli were superimposed onto the faces, and the onset of target stimuli was the same as the overlapping face images. Participants had to suppress the face information to accurately make a decision about the contrast-induced lines overlaid on the face images. Perhaps, the overall attentional load was higher in these previous studies, which could have prevented an N2pc from occurring (Qiu et al., 2022a, 2023). However, in the current face-irrelevant condition, the faces and the target stimuli were separated temporally by 66 ms. The competition between the faces and the targets is considered lower in the current paradigm, potentially allowing some processing of the task-irrelevant faces. As a result, an N2pc was evoked by the fearful face in the pair.
Additionally, when the faces were made task-relevant, we observed a SPCN, a marker for working memory maintenance, for the fearful face. This means that, in face-relevant conditions, the target fearful face was encoded and maintained in working memory, perhaps because this was necessary to produce a correct answer in the fearful face localisation task. However, when the requirement of attending to the fearful face was removed in the face-irrelevant condition, we no longer observed the neural processes associated with working memory (SPCN). These results are not surprising as the top-down suppression of task-irrelevant signals have been demonstrated extensively in the literature (e.g., Gaspelin and Luck, 2018; Liu et al., 2020; for a review see Luck et al., 2021). As explained, the temporal separation between the two pairs of stimuli (faces and target dots) may have allowed attention to shift to task-irrelevant fearful faces (i.e., N2pc). This is not incompatible with the “inhibitory mechanism” highlighted in this line of research where the inhibition was oftentimes exerted upon salient task-irrelevant stimuli presented simultaneously with the targets (Luck et al., 2021; for studies on face processing see Lien et al., 2013; Qiu et al., 2022a, 2023; Zhou et al., 2020). In the current data, signal suppression was perhaps present still, however it manifested as an absence of working memory consolidation for the task-irrelevant faces.
The task-relevancy of the faces also modulated the processing of the following target dot in the current paradigm. Specifically, a pair of task-relevant faces weakened the overall neural activity for the subsequent targets, regardless of the visibility of the faces. Perhaps, the dual task demands resulted in a higher attentional load in the face-relevant condition. Specifically, the task-relevant faces required a certain amount of the limited attentional or working memory resources (Xu and Chun, 2009; Ma et al., 2014) that are shared by neural processes for the target dots in close temporal proximity. Consequently, the strength of neural activity associated with the dots decreased as the preceding faces were processed more strongly in a task-relevant situation, compared to the face-irrelevant condition. Further, the decrease in dot-related ERPs was more evident when the faces were clearly visible (in the supraliminal condition) whereby the time range (200-400 ms) of this attenuation effect was larger than when the faces were presented subliminally (300-400 ms). It is likely that faces were more distracting when they were clearly visible, resulting in even stronger neural activity for the faces themselves, but further diminished ERPs for the subsequent dots. This finding is in line with our previous study using two rapid streams of visual presentations of faces, from which the amplitude of the N2pc towards a lateralised fearful face was found to decrease substantially when participants had to attend to another pair of faces presented immediately prior to it, compared to when the two face pairs were separated for longer (Qiu et al., 2022b).
Finally, and most importantly, no evidence was found for the nonconscious processing of fearful faces in the current paradigm. This was supported by both the univariate and multivariate analysis results, and through two indices of spatial attention (face-related signals and dot-related signals). Indeed, no N2pc for fearful faces was observed in the subliminal viewing condition, and the neural processes for the dots following subliminal face presentations were not modulated by the presence of a fearful face. Consistent with this, the MVPA decoding performance for the spatial location of the fearful faces was at chance-level when the faces were presented subliminally. Additionally, no successful decoding of congruency was found in the face-subliminal condition. Thus, in a bilateral presentation of face images, the spatial information about a lateralised fearful face cannot be processed without visual awareness. This finding is consistent with our recent studies (Qiu et al., 2022a, 2023) and several studies by other researchers (Koster et al., 2007; Gray et al., 2013; Hedger et al., 2019; Baier et al., 2022; Tipura and Pegna, 2022).
The majority of studies showing evidence for the nonconscious processing of fearful faces used central face presentations (e.g., Pegna et al., 2008, 2011; Del Zotto and Pegna, 2015). Faces presented laterally or more eccentric in the visual field are usually harder to detect, compared to faces presented at the centre of the visual field (Smith and Rossit, 2018; Papaioannou and Luck, 2020). Even when they are presented in a subliminal viewing condition, i.e., 16 ms (Pegna et al., 2008; Del Zotto and Pegna, 2015), some processing of fearful faces may occur for centrally presented faces, which may then result in a modulation of the ERPs. However, this modulation is not observed for lateralised faces, as shown by the current results, perhaps due to competition between the two similarly complex face stimuli in each presentation (Wirth and Wentura, 2018).
The use of bilateral presentations of faces in other studies on nonconscious emotion processing is however not rare (De Gelder et al., 2005; Koster et al., 2007; Carlson and Reinke, 2008, 2010; Bertini et al., 2013; Cecere et al., 2014; Hedger et al., 2019). For example, in Carlson and Reinke (2010), face pairs were presented for 33 ms in a backward masking experiment. It was found that the face-sensitive N170 for the masked fearful faces was enhanced, compared to masked neutral faces. However, as acknowledged by the authors themselves, there may be some conscious experience of the stimuli when they are presented for 33 ms (Carlson and Reinke, 2010; see also Pessoa et al., 2005), potentially accounting for the fear-related enhancement effect. In situations where visual awareness was more strongly impeded by either shorter presentation of faces in masking experiments (17 ms, Hedger et al., 2019; 14 ms, Koster et al., 2007) or in a continuous flash suppression procedure (Hedger et al., 2019), fearful faces did not attract spatial attention at the behavioural level. Our current EEG data complement the previous literature by showing that spatial attention was not captured by lateralised fearful faces presented subliminally (16 ms).
Another approach to investigate nonconscious emotion processing is testing patients with cortical blindness. Such patients usually experience a loss of visual awareness due to regional lesion(s) in the brain. Previous clinical studies have consistently shown that emotional faces can be processed even though the patients were incapable of detecting or reporting the stimuli (for a review see Celeghin et al., 2015). Specifically, in a bilateral presentation of face images, patients with hemifield blindness showed improved task performance on face stimuli (e.g., better emotion recognition) presented in their intact visual field when a fearful face was concurrently presented in the blind visual field, indicating some processing of the fearful face in the absence of awareness (De Gelder et al., 2005; Bertini et al., 2013; Cecere et al., 2014). This fear-related improvement on task performance was supported by electrophysical evidence (Cecere et al., 2014) as well as functional imaging evidence (De Gelder et al., 2005). However, whilst fearful faces can be processed (or “influence cognitive processing,” Koster et al., 2007) nonconsciously, they do not necessarily attract spatial attention. Supporting this, in a patient with complete destruction of the primary visual cortex, Del Zotto et al. (2013) demonstrated that, whilst the presence of emotional faces, compared to neutral faces, facilitated the patient’s task performance for subsequent sound stimuli, the spatial location of the emotional faces had no effect on the patient’s behaviour (see supplementary data in Del Zotto et al., 2013).
Taken together, we conclude that, when not consciously detected, fearful faces do not attract spatial attention and they do not affect the processing of spatially contiguous stimuli. Although consciously seen fearful faces attract spatial attention and they modulate the neural processes for following stimuli, these processes are strongly modulated by attentional load. As part of the endeavour in understanding emotional face processing, the current results point to the importance of various conditions (i.e., awareness and task-relevancy) for attentional capture by fearful faces.
Data availability statement
The datasets presented in this study can be found in online repositories. The names of the repository/repositories and accession number(s) can be found at: https://osf.io/54nw2/.
Ethics statement
The studies involving human participants were reviewed and approved by University of Queensland ethics committee. The patients/participants provided their written informed consent to participate in this study.
Author contributions
ZQ and AP contributed to conception and design of the study. ZQ and JJ collected data and performed data processing. ZQ performed data analyses and wrote the first draft of the manuscript. ZQ, SB, and AP contributed to manuscript revision. All authors contributed to the article and approved the submitted version.
Funding
ZQ was supported by UQ PhD scholarships.
Conflict of interest
The authors declare that the research was conducted in the absence of any commercial or financial relationships that could be construed as a potential conflict of interest.
Publisher’s note
All claims expressed in this article are solely those of the authors and do not necessarily represent those of their affiliated organizations, or those of the publisher, the editors and the reviewers. Any product that may be evaluated in this article, or claim that may be made by its manufacturer, is not guaranteed or endorsed by the publisher.
Footnotes
References
Baier, D., Kempkes, M., Ditye, T., and Ansorge, U. (2022). Do subliminal fearful facial expressions capture attention? Front. Psychol. 13:840746. doi: 10.3389/fpsyg.2022.840746
Bar-Haim, Y., Lamy, D., and Glickman, S. (2005). Attentional bias in anxiety: a behavioural and ERP study. Brain Cogn. 59, 11–22. doi: 10.1016/j.bandc.2005.03.005
Bertini, C., Cecere, R., and Làdavas, E. (2013). I am blind, but I “see” fear. Cortex 49, 985–993. doi: 10.1016/j.cortex.2012.02.006
Campbell, J. I., and Thompson, V. A. (2012). MorePower 6.0 for ANOVA with relational confidence intervals and Bayesian analysis. Behav. Res. Methods 44, 1255–1265. doi: 10.3758/s13428-012-0186-0
Carlson, J. M., and Reinke, K. S. (2008). Masked fearful faces modulate the orienting of covert spatial attention. Emotion 8, 522–529. doi: 10.1037/a0012653
Carlson, J. M., and Reinke, K. S. (2010). Spatial attention-related modulation of the N170 by backward masked fearful faces. Brain Cogn. 73, 20–27. doi: 10.1016/j.bandc.2010.01.007
Cecere, R., Bertini, C., Maier, M. E., and Làdavas, E. (2014). Unseen fearful faces influence face encoding: evidence from ERPs in hemianopic patients. J. Cogn. Neurosci. 26, 2564–2577. doi: 10.1162/jocn_a_00671
Celeghin, A., de Gelder, B., and Tamietto, M. (2015). From affective blindsight to emotional consciousness. Conscious. Cogn. 36, 414–425. doi: 10.1016/j.concog.2015.05.007
Chang, C.-C., and Lin, C.-J. (2011). LIBSVM: a library for support vector machines. ACM Trans. Intell. Syst. Technol. (TIST) 2, 1–27. doi: 10.1145/1961189.1961199
De Gelder, B., Morris, J. S., and Dolan, R. J. (2005). Unconscious fear influences emotional awareness of faces and voices. Proc. Natl. Acad. Sci. 102, 18682–18687. doi: 10.1073/pnas.0509179102
Del Zotto, M., Deiber, M. P., Legrand, L. B., De Gelder, B., and Pegna, A. J. (2013). Emotional expressions modulate low α and β oscillations in a cortically blind patient. Int. J. Psychophysiol. 90, 358–362. doi: 10.1016/j.ijpsycho.2013.10.007
Del Zotto, M., and Pegna, A. J. (2015). Processing of masked and unmasked emotional faces under different attentional conditions: an electrophysiological investigation. Front. Psychol. 6:1691. doi: 10.3389/fpsyg.2015.01691
Delorme, A., and Makeig, S. (2004). EEGLAB: an open source toolbox for analysis of single-trial EEG dynamics including independent component analysis. J. Neurosci. Methods 134, 9–21. doi: 10.1016/j.jneumeth.2003.10.009
Eimer, M., and Kiss, M. (2007). Attentional capture by task-irrelevant fearful faces is revealed by the N2pc component. Biol. Psychol. 74, 108–112. doi: 10.1016/j.biopsycho.2006.06.008
Fields, E. C., and Kuperberg, G. R. (2020). Having your cake and eating it too: flexibility and power with mass univariate statistics for ERP data. Psychophysiology 57:e13468. doi: 10.1111/psyp.13468
Gaspelin, N., and Luck, S. J. (2018). Combined electrophysiological and behavioural evidence for the suppression of salient distractors. J. Cogn. Neurosci. 30, 1265–1280. doi: 10.1162/jocn_a_01279
Giattino, C. M., Alam, Z. M., and Woldorff, M. G. (2018). Neural processes underlying the orienting of attention without awareness. Cortex 102, 14–25. doi: 10.1016/j.cortex.2017.07.010
Gray, K. L., Adams, W. J., Hedger, N., Newton, K. E., and Garner, M. (2013). Faces and awareness: low-level, not emotional factors determine perceptual dominance. Emotion 13, 537–544. doi: 10.1037/a0031403
Grootswagers, T., Wardle, S. G., and Carlson, T. A. (2017). Decoding dynamic brain patterns from evoked responses: a tutorial on multivariate pattern analysis applied to time series neuroimaging data. J. Cogn. Neurosci. 29, 677–697. doi: 10.1162/jocn_a_01068
Groppe, D. M., Urbach, T. P., and Kutas, M. (2011). Mass univariate analysis of event-related brain potentials/fields I: a critical tutorial review. Psychophysiology 48, 1711–1725. doi: 10.1111/j.1469-8986.2011.01273.x
Hedger, N., Garner, M., and Adams, W. J. (2019). Do emotional faces capture attention, and does this depend on awareness? Evidence from the visual probe paradigm. J. Exp. Psychol. Hum. Percept. Perform. 45, 790–802. doi: 10.1037/xhp0000640
Kayser, J., and Tenke, C. E. (2006). Principal components analysis of Laplacian waveforms as a generic method for identifying ERP generator patterns: I. evaluation with auditory oddball tasks. Clin. Neurophysiol. 117, 348–368. doi: 10.1016/j.clinph.2005.08.034
Koster, E. H., Verschuere, B., Burssens, B., Custers, R., and Crombez, G. (2007). Attention for emotional faces under restricted awareness revisited: do emotional faces automatically attract attention? Emotion 7, 285–295. doi: 10.1037/1528-3542.7.2.285
Langner, O., Dotsch, R., Bijlstra, G., Wigboldus, D. H., Hawk, S. T., and Van Knippenberg, A. D. (2010). Presentation and validation of the Radboud faces database. Cognit. Emot. 24, 1377–1388. doi: 10.1080/02699930903485076
Lavie, N. (2005). Distracted and confused?: selective attention under load. Trends Cogn. Sci. 9, 75–82. doi: 10.1016/j.tics.2004.12.004
Lavie, N., Hirst, A., De Fockert, J. W., and Viding, E. (2004). Load theory of selective attention and cognitive control. J. Exp. Psychol. Gen. 133, 339–354. doi: 10.1037/0096-3445.133.3.339
Lien, M. C., Taylor, R., and Ruthruff, E. (2013). Capture by fear revisited: an electrophysiological investigation. J. Cogn. Psychol. 25, 873–888. doi: 10.1080/20445911.2013.833933
Liu, Y., Wang, P., and Wang, G. (2020). The priority of goal-relevant information and evolutionarily threatening information in early attention processing: evidence from behavioural and ERP study. Sci. Rep. 10, 1–9. doi: 10.1038/s41598-020-65062-5
Lopez-Calderon, J., and Luck, S. J. (2014). ERPLAB: an open-source toolbox for the analysis of event-related potentials. Front. Hum. Neurosci. 8:213. doi: 10.3389/fnhum.2014.00213
Luck, S. J., Gaspelin, N., Folk, C. L., Remington, R. W., and Theeuwes, J. (2021). Progress toward resolving the attentional capture debate. Vis. Cogn. 29, 1–21. doi: 10.1080/13506285.2020.1848949
Luria, R., Balaban, H., Awh, E., and Vogel, E. K. (2016). The contralateral delay activity as a neural measure of visual working memory. Neurosci. Biobehav. Rev. 62, 100–108. doi: 10.1016/j.neubiorev.2016.01.003
Ma, W. J., Husain, M., and Bays, P. M. (2014). Changing concepts of working memory. Nat. Neurosci. 17, 347–356. doi: 10.1038/nn.3655
Milders, M., Sahraie, A., and Logan, S. (2008). Minimum presentation time for masked facial expression discrimination. Cognit. Emot. 22, 63–82. doi: 10.1080/02699930701273849
Oosterhof, N. N., Connolly, A. C., and Haxby, J. V. (2016). CoSMoMVPA: multi-modal multivariate pattern analysis of neuroimaging data in Matlab/GNU octave. Front. Neuroinform. 10:27. doi: 10.3389/fninf.2016.00027
Papaioannou, O., and Luck, S. J. (2020). Effects of eccentricity on the attention-related N2pc component of the event-related potential waveform. Psychophysiology 57:e13532. doi: 10.1111/psyp.13532
Pegna, A. J., Darque, A., Berrut, C., and Khateb, A. (2011). Early ERP modulation for task-irrelevant subliminal faces. Front. Psychol. 2:88. doi: 10.3389/fpsyg.2011.00088
Pegna, A. J., Landis, T., and Khateb, A. (2008). Electrophysiological evidence for early non-conscious processing of fearful facial expressions. Int. J. Psychophysiol. 70, 127–136. doi: 10.1016/j.ijpsycho.2008.08.007
Peirce, J. W., Gray, J. R., Simpson, S., MacAskill, M. R., Höchenberger, R., Sogo, H., et al. (2019). PsychoPy2: experiments in behaviour made easy. Behav. Res. Methods 51, 195–203. doi: 10.3758/s13428-018-01193-y
Pessoa, L., Japee, S., and Ungerleider, L. G. (2005). Visual awareness and the detection of fearful faces. Emotion 5, 243–247. doi: 10.1037/1528-3542.5.2.243
Pourtois, G., Grandjean, D., Sander, D., and Vuilleumier, P. (2004). Electrophysiological correlates of rapid spatial orienting towards fearful faces. Cereb. Cortex 14, 619–633. doi: 10.1093/cercor/bhh023
Qiu, Z., Becker, S. I., and Pegna, A. J. (2022a). Spatial attention shifting to emotional faces is contingent on awareness and task relevancy. Cortex 151, 30–48. doi: 10.1016/j.cortex.2022.02.009
Qiu, Z., Becker, S. I., and Pegna, A. J. (2022b). Spatial attention shifting to fearful faces depends on visual awareness in attentional blink: an ERP study. Neuropsychologia 172:108283. doi: 10.1016/j.neuropsychologia.2022.108283
Qiu, Z., Lei, X., Becker, S. I., and Pegna, A. J. (2022c). Neural activities during the processing of unattended and unseen emotional faces: a voxel-wise meta-analysis. Brain Imaging Behav. 16, 2426–2443. doi: 10.1007/s11682-022-00697-8
Qiu, Z., Li, X., and Pegna, A. J. (2022d). Decoding neural patterns for the processing of fearful faces under different visual awareness conditions: a multivariate pattern analysis. BioRxiv. [Preprint] doi: 10.1101/2022.12.19.520904
Qiu, Z., Zhang, J., and Pegna, A. J. (2023). Neural processing of lateralised task-irrelevant fearful faces under different awareness conditions. Conscious. Cogn. 107:103449. doi: 10.1016/j.concog.2022.103449
Schindler, S., and Bublatzky, F. (2020). Attention and emotion: an integrative review of emotional face processing as a function of attention. Cortex 130, 362–386. doi: 10.1016/j.cortex.2020.06.010
Schupp, H. T., Öhman, A., Junghöfer, M., Weike, A. I., Stockburger, J., and Hamm, A. O. (2004). The facilitated processing of threatening faces: an ERP analysis. Emotion 4, 189–200. doi: 10.1037/1528-3542.4.2.189
Smith, S. M., and Nichols, T. E. (2009). Threshold-free cluster enhancement: addressing problems of smoothing, threshold dependence and localisation in cluster inference. NeuroImage 44, 83–98. doi: 10.1016/j.neuroimage.2008.03.061
Smith, F. W., and Rossit, S. (2018). Identifying and detecting facial expressions of emotion in peripheral vision. PLoS One 13:e0197160. doi: 10.1371/journal.pone.0197160
Tamietto, M., and De Gelder, B. (2010). Neural bases of the non-conscious perception of emotional signals. Nat. Rev. Neurosci. 11, 697–709. doi: 10.1038/nrn2889
Tipura, E., and Pegna, A. J. (2022). Subliminal emotional faces do not capture attention under high attentional load in a randomized trial presentation. Vis. Cogn. 30, 280–288. doi: 10.1080/13506285.2022.2060397
Torrence, R. D., and Troup, L. J. (2018). Event-related potentials of attentional bias toward faces in the dot-probe task: a systematic review. Psychophysiology 55:e13051. doi: 10.1111/psyp.13051
Torrence, R. D., Wylie, E., and Carlson, J. M. (2017). The time-course for the capture and hold of visuospatial attention by fearful and happy faces. J. Nonverbal Behav. 41, 139–153. doi: 10.1007/s10919-016-0247-7
van Rooijen, R., Ploeger, A., and Kret, M. E. (2017). The dot-probe task to measure emotional attention: a suitable measure in comparative studies? Psychon. Bull. Rev. 24, 1686–1717. doi: 10.3758/s13423-016-1224-1
Vuilleumier, P. (2005). How brains beware: neural mechanisms of emotional attention. Trends Cogn. Sci. 9, 585–594. doi: 10.1016/j.tics.2005.10.011
Wirth, B. E., and Wentura, D. (2018). Attentional bias to threat in the general population is contingent on target competition, not on attentional control settings. Q. J. Exp. Psychol. 71, 975–988. doi: 10.1080/17470218.2017.1307864
Xu, Y., and Chun, M. M. (2009). Selecting and perceiving multiple visual objects. Trends Cogn. Sci. 13, 167–174. doi: 10.1016/j.tics.2009.01.008
Keywords: spatial attention, awareness, fearful faces, EEG, mass univariate analysis, multivariate pattern analysis
Citation: Qiu Z, Jiang J, Becker SI and Pegna AJ (2023) Attentional capture by fearful faces requires consciousness and is modulated by task-relevancy: A dot-probe EEG study. Front. Neurosci. 17:1152220. doi: 10.3389/fnins.2023.1152220
Edited by:
Qianru Xu, University of Oulu, FinlandReviewed by:
Min-Suk Kang, Sungkyunkwan University, Republic of KoreaJan Van den Stock, KU Leuven, Belgium
Copyright © 2023 Qiu, Jiang, Becker and Pegna. This is an open-access article distributed under the terms of the Creative Commons Attribution License (CC BY). The use, distribution or reproduction in other forums is permitted, provided the original author(s) and the copyright owner(s) are credited and that the original publication in this journal is cited, in accordance with accepted academic practice. No use, distribution or reproduction is permitted which does not comply with these terms.
*Correspondence: Zeguo Qiu, emVndW8ucWl1QHVxLm5ldC5hdQ==; Alan J. Pegna, YS5wZWduYUB1cS5lZHUuYXU=