- 1Departments of Biomedical Sciences, Joan C. Edwards School of Medicine, Marshall University, Huntington, WV, United States
- 2Department of Neurosurgery, The First Affiliated Hospital, Harbin Medical University, Harbin, Heilongjiang, China
- 3Departments of Clinical and Translational Sciences, Joan C. Edwards School of Medicine, Marshall University, Huntington, WV, United States
Extracellular vesicles (EVs) serve as cell-to-cell and inter-organ communicators by conveying proteins and nucleic acids with regulatory functions. Emerging evidence shows that gut microbial-released EVs play a pivotal role in the gut-brain axis, bidirectional communication, and crosstalk between the gut and the brain. Increasing pre-clinical and clinical evidence suggests that gut bacteria-released EVs are capable of eliciting distinct signaling to the brain with the ability to cross the blood–brain barrier, exerting regulatory function on brain cells such as neurons, astrocytes, and microglia, via their abundant and diversified protein and nucleic acid cargo. Conversely, EVs derived from certain species of bacteria, particularly from gut commensals with probiotic properties, have recently been shown to confer distinct therapeutic effects on various neurological disorders. Thus, gut bacterial EVs may be both a cause of and therapy for neuropathological complications. This review marshals the basic, clinical, and translational studies that significantly contributed to our up-to-date knowledge of the therapeutic potential of gut microbial-derived EVs in treating neurological disorders, including strokes, Alzheimer’s and Parkinson’s disease, and dementia. The review also discusses the newer insights in recent studies focused on developing superior therapeutic microbial EVs via genetic manipulation and/or dietary intervention.
1. Introduction
The bidirectional communication and crosstalk between the gut and the brain, termed the gut-brain axis, has been well recognized. Mounting pre-clinical data and emerging clinical evidence suggest that gut microbiota plays a pivotal role in this bidirectional communication network, leading to the more recent concept of the gut-brain-microbiota axis which has a tremendous impact on the physiological and pathophysiological processes of both the organs (Heiss and Olofsson, 2019; Osadchiy et al., 2019; Margolis et al., 2021). In this regard, extracellular vesicles (EVs) produced by gut bacteria have been implicated as a potential critical regulator of interkingdom and interorgan communication, playing a key role in normal physiology and the pathophysiology of the brain and neurological functions (Pirolli et al., 2021). Gut microbiota-derived EVs (GMEVs) are capable of eliciting distinct signaling to the brain, possibly directly via crossing the blood–brain barrier (BBB), and exerting differential effects on brain cells such as neurons, astrocytes, and microglia, via their abundant and diversified protein and small ribonucleic acid (RNA) cargo (Srivastava and Kim, 2022). GMEVs have been shown to regulate brain gene expression and induce pathology at most stages of neuroinflammation and neurodegeneration, thus playing a causative role in various diseases such as stroke, Alzheimer’s disease (AD) and Parkinson’s disease (PD), and dementia (Lee et al., 2020; Yang et al., 2022). Conversely, EVs derived from certain species of bacteria, particularly gut commensals with probiotic properties, have recently been shown to confer distinct therapeutic effects on various neurological disorders (Park and Tsunoda, 2022). Thus, GMEVs may be a cause of as well as therapy for neuropathological complications. This review compiles the fundamental, clinical, and translational studies that have made substantial contributions to our up-to-date knowledge of the therapeutic potential of GMEVs in treating neurological disorders. It also provides information regarding the underlying mechanisms of beneficial effects and the applicability of circulating GMEVs as biomarkers of various neurological disease states. This review additionally discusses the latest findings in recent studies focused on the advancement of enhanced therapeutic bacterial EVs via genetic manipulation and/or dietary intervention.
2. Role of the microbiota in gut-brain axis: microbiota-gut-brain axis
The “gut–microbiota–brain axis” refers to the network of connections involving multiple biological systems that allow bidirectional communication between gut bacteria and the brain, and is crucial in maintaining homeostasis of the gastrointestinal, central nervous, and microbial systems of animals (Morais et al., 2021). These processes may affect human health, as certain animal behaviors appear to correlate with the composition of gut bacteria, and disruptions in microbial communities have been implicated in several neurological disorders (Foster et al., 2017; Cryan et al., 2020). Emerging evidence suggests that the gut microbiota is intimately involved in the pathology of a wide range of neurological disorders and gut microbiota dysbiosis is a risk factor. Although structure and space may limit the contact between the microbiome and the intestinal epithelium, the substances secreted by the microbiome can pass through the limitations of space and serve as important factors in the function of the gut-brain axis. Various microbial products, including metabolites and secreted proteins, can cross the mucin layer to reach the host cells on the mucosal surface of the intestine. More recently, EVs have emerged as a novel communicator of the gut-microbiota-brain axis.
3. Gut microbiota-released extracellular vesicles
The gut microbiota can produce EVs in both physiological and pathological situations. Mounting evidence suggests that interkingdom crosstalk is principally mediated by EVs released by gut microbiota or by host intestinal cells (Diaz-Garrido et al., 2021).
3.1. Origin and type GMEVs
Traditionally, bacteria are categorized into two classes, according to their outer membrane nature: Gram-negative (G−) and Gram-positive (G+) bacteria (Sultan et al., 2021). The structure of G− bacteria is characterized by a double plasma membrane separated by the surrounding periplasm. The main types of EVs that are secreted come from the outer membrane and are called “outer membrane vesicles” (OMVs; Schwechheimer and Kuehn, 2015; Jan, 2017). OMVs are spherical particles consisting of the outer lobe of lipopolysaccharide (LPS) and the inner lobe of phospholipid. They originate from outer membrane bubbles, so they are enriched in the outer membrane and periplasmic biomolecules (Kulkarni and Jagannadham, 2014; Schwechheimer and Kuehn, 2015; Volgers et al., 2018). Some G− bacteria produce another type of EVs that contains fragments from the cytoplasm and periplasmic membrane and are rich in adenosine triphosphates and deoxyribonucleic acid (DNA), called inner and OMVs (Pérez-Cruz et al., 2015). In addition, recent studies have described a new type of G− bacteria-derived vesicles called “explosive outer-membrane vesicles” by the biogenesis mechanism (Toyofuku et al., 2019). The membrane of G+ bacteria has different properties from that of G− bacteria and the special thick layer of its peptidoglycan is thought to be an obstacle in the production of EVs. However, Lee EY et al. discovered EVs from Staphylococcus aureus and found that the mechanism of EVs released by G+ bacteria was also different from that of G− bacteria. EVs were produced through dilation, protease cleavage, or protein channels being pushed through thick membranes (Lee et al., 2009; Vallejo et al., 2012). Studies on a variety of G+ bacteria, such as Staphylococcus aureus, Bacillus anthracis, Listeria monocytogenes, Clostridium perfringens, Bacillus subtilis, have confirmed that the EVs derived from G+ bacteria are also spherical membrane particles with a diameter of 20–100 nm (Lee et al., 2009; Brown et al., 2015; Kim et al., 2015). Because of the lack of an outer membrane, G+ bacteria secreted EVs are called “cytoplasmic membrane vesicles” (CMVs; Toyofuku et al., 2019). EVs from G+ bacteria, despite lacking LPS and periplasmic components, carry similar types of cargo molecules to G− bacteria EVs, including peptidoglycans, lipids, proteins, and nucleic acids (Brown et al., 2015).
3.2. Diversity and complexity of molecular species in GMEVs cargo
EVs are known to mediate communication between cells, and the effects of EVs depend on the cargo they carry. The composition of the cargo varies with respect to the type of bacteria, their growth, environmental conditions, and biogenetic mechanisms. GMEVs cargo transported includes proteins, lipids, nucleic acids, and small molecules, and each plays a different role (Figure 1; Bonnington and Kuehn, 2014).
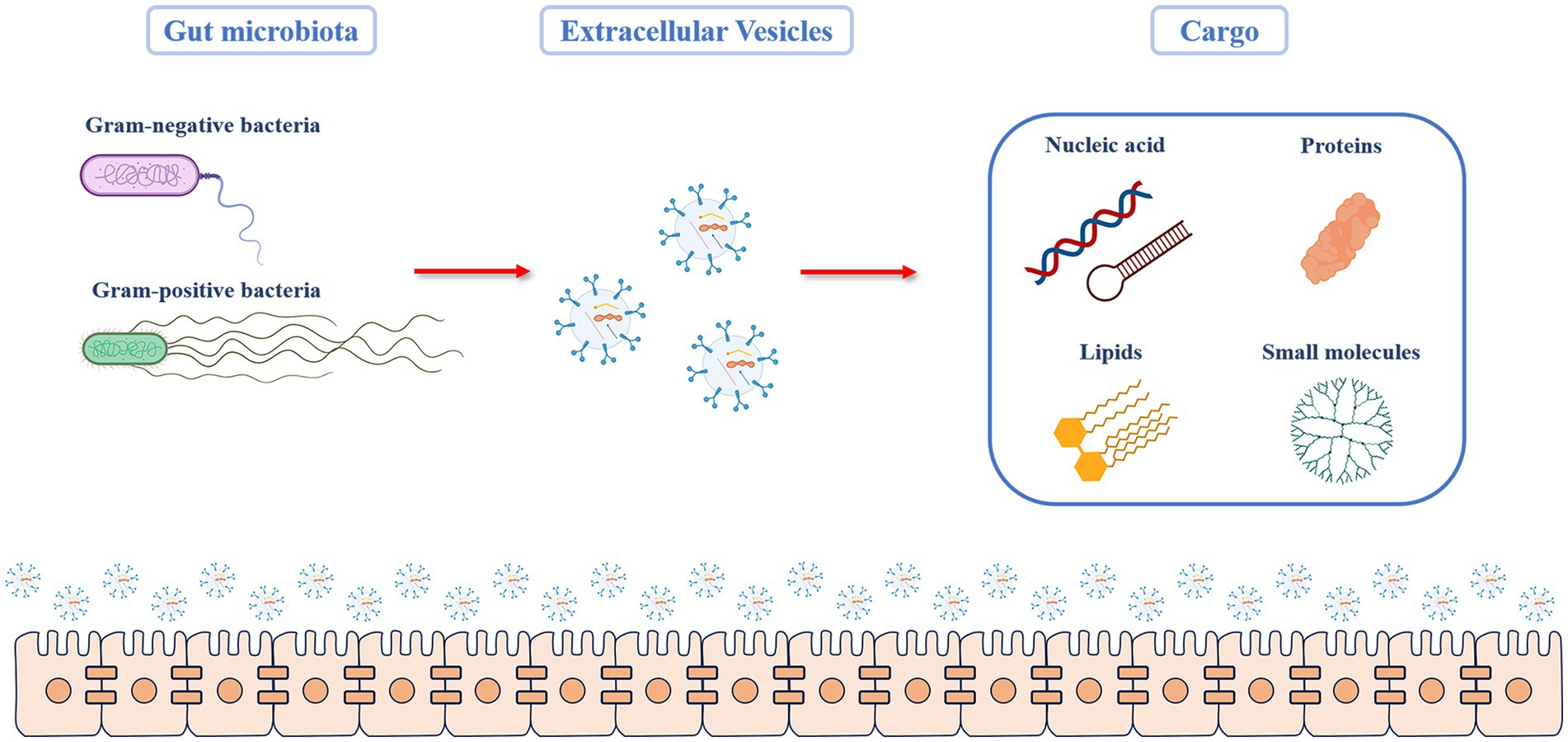
Figure 1. Cargo components of GMEVs. The Gut microbiota includes gram-positive and gram-negative bacteria, both of which can produce extracellular vesicles. The molecular components carried by the GMEV cargo can be broadly divided into four categories. (1) Proteins: they include structural proteins, porins, ion channel proteins, transporters, enzymes, and stress-related proteins. (2) Lipids: they are mainly the components of the bacterial envelope, such as lipids involved in membrane binding, such as glycerol, phospholipid, and phosphatidylglycerol (3) Nucleic acids: this includes deoxyribonucleic acid or ribonucleic acid that can mediate targeted gene transfer to other cells. (4) Other small molecules, including bacterial metabolites. GMEVs: gut microbiota-released extracellular vesicles.
3.2.1. Proteins
The proteins carried by GMEVs depend on the bacteria’s classification and specific characteristics. Currently, proteins related to many biological processes have been identified, which can be divided into structural proteins, pore proteins, ion channels, transport proteins, enzymes, and proteins related to stress response according to their functions (Lee et al., 2008). EVs released by G-bacteria are enriched in outer membrane proteins such as out membrane protein (Omp)-A, Omp-C, and Omp-F and periplasmic proteins such as AcrA and alkaline phosphatase (Choi et al., 2011; Altindis et al., 2014; Jang et al., 2014). The vesicles released by G− bacteria and symbiotic strains contain proteins that contribute to intestinal colonization, competition, and bacterial survival, as well as regulate host immune and defense responses, and some proteins are strain-specific (Lee et al., 2007; Aguilera et al., 2014; Elhenawy et al., 2014; Zakharzhevskaya et al., 2017; Hong et al., 2019). Proteins involved in vesicle biogenesis or regulating vesicles’ size, yield, and load were found in enterococcus serotype typhoid OMVs (Nevermann et al., 2019). The probiotics Escherichia coli Nissle 1917 (EcN) carry the protein encoded explicitly by the strain, most of which are adhesins and adaptive factors, which help the bacteria grow and survive in the harmful intestinal environment (Aguilera et al., 2014; Hong et al., 2019). In addition to intracellular activity, these proteins also have functions related to host interaction and tissue colonization (Jeffery, 2018).
The protein analysis of some G+ bacteria has identified membrane and cytoplasmic proteins related to several biological processes, mainly including GMEVs from pathogens, which are full of pathogenic factors and toxins, and have pathogenicity (Lee et al., 2009; Rivera et al., 2010; Olaya-Abril et al., 2014; Jeon et al., 2016; Resch et al., 2016; Surve et al., 2016; Dean et al., 2019, 2020; West et al., 2020). CMVs transporter isolated from several G+ probiotics can be responsible for their beneficial role in immune regulation and host interaction (Lee et al., 2016; Behzadi et al., 2017; Domingues and Nielsen, 2017; Li et al., 2017).
3.2.2. Lipids
The lipid is the main component of the bacterial envelope. It is generally believed that outer membrane phospholipids exist in the OMVs of G− bacteria. There is evidence that OMVs contain lipids not found in the bacterial outer membrane (Tashiro et al., 2011; Chowdhury and Jagannadham, 2013). The study of EcN showed that OMVs include lipids involved in membrane bindings, such as glycerol, phospholipids, phosphatidylglycerol, phosphatidylethanolamine, and cardiolipin. The structure of fatty acids can affect the fluidity and rigidity of lipid membranes (Barák and Muchová, 2013). LPS is a component of G− bacteria OMVs. Porphyromonas gingivalis OMVs carry LPS molecules with long sugar chains and diacyl lipid A (Haurat et al., 2011).
Similarly, diacyl lipid A accumulates in Salmonella OMVs (Elhenawy et al., 2014). In G+ bacteria, the lipid composition of CMVs varies with the type of bacteria. This may be related to the adaptation and survival of bacteria in the ecological environment (Coelho et al., 2019). In Bacillus anthracis and Streptococcus pneumoniae, vesicles are rich in C12–C16 chain saturated fatty acids (Olaya-Abril et al., 2014). Lipids containing unsaturated fatty acids are more abundant in vesicles derived from Listeria monocytogenes than in bacterial cells (Coelho et al., 2019).
3.2.3. Nucleic acid
GMEVs derived from G– and G+ bacteria are loaded with DNA and RNA. GMEVs can transfer DNA to other bacterial cells, thus mediating targeted gene transfer (Domingues and Nielsen, 2017). The nucleic acids contained in GMEVs interacts with specific intracellular receptors in host cells, thus triggering the host immune response (Gilmore et al., 2021). GMEVs contain most lumen DNA genes corresponding to specific functions related to antibiotic resistance, virulence, and stress response (Bitto and Kaparakis-Liaskos, 2017). In Clostridium perfringens, GMEVs can encode bacterial toxins, such as α-Toxin and aerolysin O (pfoA; Jiang et al., 2014). RNA in GMEVs can also regulate the host immune system and other cellular processes. Small non-coding RNA contained in GMEVs mainly regulates post-transcriptional gene expression, while RNA vectors can induce phenotypic changes in target cells (Lee, 2019). In some pathogenic bacteria, small RNA secreted by GMEVs can mediate host immune response disorder (Koeppen et al., 2016; Choi et al., 2017). Many small non-coding RNA sequences transported in GMEVs of the probiotic E. coli Nissle (EcN) are consistent with human genome regions involved in the epigenetic mechanism or gene expression regulation related to the cell-specific transcriptional control (Celluzzi and Masotti, 2016).
3.2.4. Small molecules
GMEVs are also loaded with metabolites and effectors that can regulate the function of target cells. These small molecules are selectively packaged according to the producer strains (Zakharzhevskaya et al., 2017). The effector molecules packaged in GMEVs can help bacteria survive in a specific niche. GMEVs of fragilis can be loaded with the antibacterial peptide bionic self-assembling peptide (BSAP), thus inhibiting the activity of other Bacteroides in the human intestine (Chatzidaki-Livanis et al., 2014). GMEVs can also mediate hydrophobic quorum-sensing molecules to promote communication in bacterial communities and control toxicity or biofilm formation (Brameyer et al., 2018).
3.3. The function of GMEVs: good or bad?
GMEVs involve many biological functions, varying depending on their specific cargo contents. The diversity of cargo molecules endows GMEVs to play a crucial role in bacteria-bacteria and bacteria-host interactions (Schwechheimer and Kuehn, 2015; Jan, 2017; Caruana and Walper, 2020). In terms of the interaction between bacteria and bacteria, GMEVs can help host bacteria maintain their niche by competing with or killing other bacteria. It can serve as a microbial defense mechanism to protect against harmful substances, including bacteriophages, antibiotics, reactive oxygen species, and antimicrobial peptides. It can also be used as a bait for pollutants or antibiotics against bacterial membrane to prevent harmful effects (Giordano et al., 2020). GMEVs also release antibiotic-resistant enzymes, which are beneficial to the producer strains and other susceptible bacteria in the microbial community, such as Staphylococcus aureus and Bacteroides GMEVs β-galactosidase enzyme (Lee et al., 2013; Stentz et al., 2015). GMEVs also contain hydrolytic enzymes, which catalyze the degradation of proteins and complex polysaccharides existing in the environment and help the microbial community obtain nutrients (Elhenawy et al., 2014). In addition, GMEVs nucleic acid may possess β-lactam gene, which codes for antibiotic resistance gene, and transfers at intraspecific and interspecific levels (Chatterjee et al., 2017).
In addition to the beneficial effects on bacterial communities, some GMEVs can regulate their predatory function. The gut microbiota produces EVs that carry messages of antibiotic resistance to surrounding bacteria (Schaar et al., 2014; Stentz et al., 2015). They can also carry degrading enzymes, such as murein hydrolase, peptidoglycan hydrolase, or endopeptidase, thus killing competitive beneficial bacteria (Jan, 2017). For example, GMEVs of some Micrococcus contain factors with antibacterial activity and a variety of hydrolases that lead to the cell lysis of target bacteria, thus having a predatory activity (Evans et al., 2012; Marshall and Whitworth, 2019).
4. Role of GMEVs in microbiota-host communication: inter-kingdom signaling
GMEVs interact with the host primarily through full cooperation in the host cytoplasm, activation of host receptors, and delivery of its bacterial content (O’Donoghue and Krachler, 2016). GMEVs can penetrate the eukaryotic cell membrane and intestinal cell wall (Stentz et al., 2015). It can be swallowed by immune cells in the lamina propria and has been detected in blood and urine. DNA of bacterial origin can be detected in the serum and plasma of healthy subjects (Shen et al., 2012; Gosiewski et al., 2017; Park et al., 2017). This shows that GMEVs can penetrate the intestinal epithelium and vascular endothelium and reach the distant part of the host. It is currently believed that GMEVs can pass through the intestinal wall through paracellular and transcellular pathways (Stentz et al., 2015). GMEVs can change the composition of tight junctions. Through tight junctions, the parent pathogen invades the intestinal epithelium. The vesicles of Campylobacter jejuni break down the junction proteins E-cadherin and occludin, causing the invasion of Campylobacter jejuni (Elmi et al., 2016). The vesicles of symbiotic bacteria can also increase the expression of tight junction proteins and limit the transport beside cells (Günzel and Yu, 2013). The probiotic EcN strain can produce OMVs and regulate the expression of tight junction proteins zonula occludens (ZO)-1 and ZO-2 in intestinal epithelial cells (Alvarez et al., 2016). In addition, GMEVs can also enter host cells through endocytosis, including reticulin-mediated, actin-dependent, caveolin-mediated, and reticulin-independent endocytosis (Zingl et al., 2021).
5. Role of GMEVs in gut-brain axis: inter-organ signaling
Many studies have shown that gut microbiota plays an important role in central nervous system (CNS) regulation (Heiss and Olofsson, 2019). Intestinal microbiota can attract nerve signals between the brain and intestine through the interaction between the vagus nerve and the intestinal nervous system (Goehler et al., 2008; Kunze et al., 2009; Brookes et al., 2013; Perez-Burgos et al., 2013; Bonaz et al., 2018). The host’s endocrine response can transmit the signals of intestinal microorganisms to the brain through the circulation (Clarke et al., 2013; Martin et al., 2018). Intestinal microorganisms can regulate central and peripheral immune cells, leading to changes in stress and behavioral response. Metabolites released by intestinal microorganisms, such as neurotransmitters, can be circulated through the CNS (Lyte, 2011; Sarkar et al., 2016). Currently, the understanding of how signals transfer from the gut to the brain has become a priority area of scientific investigation. Based on the functions of GMEVs we discussed, the signal transduction from the gut to the CNS could be through GMEVs by following the nervous system or circulation pathways (Figure 2). A previous study showed that the EVs of Paenalcaligenes hominins from the intestine can cause vagus-dependent cognitive impairment (Lee et al., 2020). In addition, GMEVs from Akkermansia muciniphila were reported to induce the secretion of serotonin in the colon and hippocampus of mice and the Caco-2 cell line (Yaghoubfar et al., 2020). At present, these studies provide evidence to support that GMEVs could be a signal molecule that can control CNS.
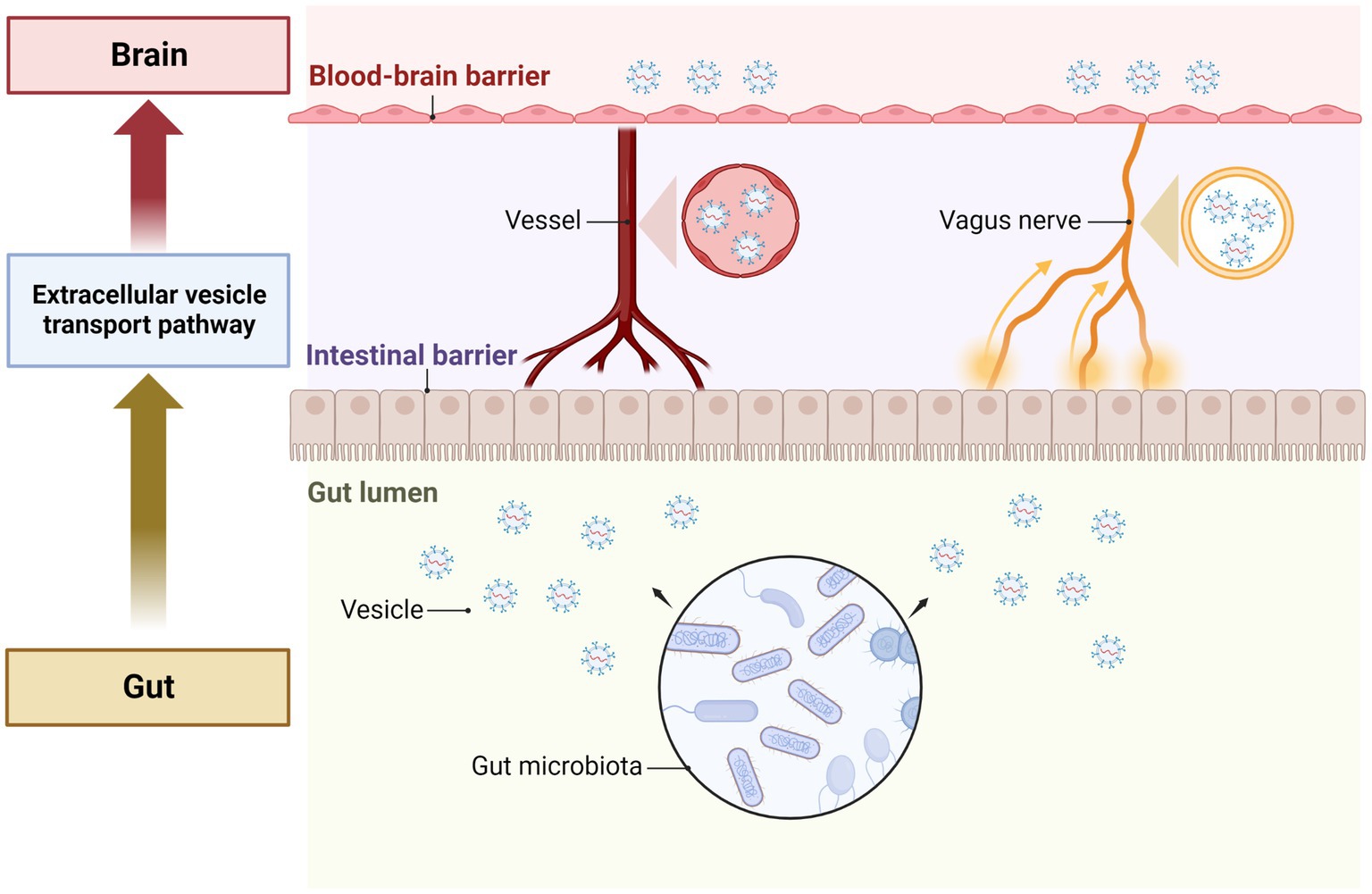
Figure 2. The role of GMEVs in mediating gut-brain communication. The gut microbiota release extracellular vesicles in the gut lumen. The vesicles pass through the cells of the intestinal epithelial layer lining the lumen and enter the blood vessels to be transported through the circulation. The vesicles then pass through the blood–brain barrier and enter the brain cells to exert their effects. In addition, vesicles can also be transported to the brain via the vagus nerves. GMEVs, gut microbiota-released extracellular vesicles.
6. Good GMEVs: probiotics-derived EVs and their potential therapeutic role in neurological disorders
Although GMEVs cannot directly cause or treat diseases, they can indirectly regulate diseases via conferring harmful and beneficial effects (Figure 3). Gut probiotics often have beneficial neurological effects, some of which could be replicated by GMEVs (Al-Nedawi et al., 2015; Choi et al., 2019; West et al., 2020). EVs from probiotics may favorably modulate microglial function, exerting beneficial effects on the nervous system (Han et al., 2019; Wei et al., 2020). GMEVs, especially probiotic EVs, have emerged as a promising tool for therapeutic applications due to their nanosized structures, cell-free systems, drug loading capacity, low toxicity, and good biocompatibility.
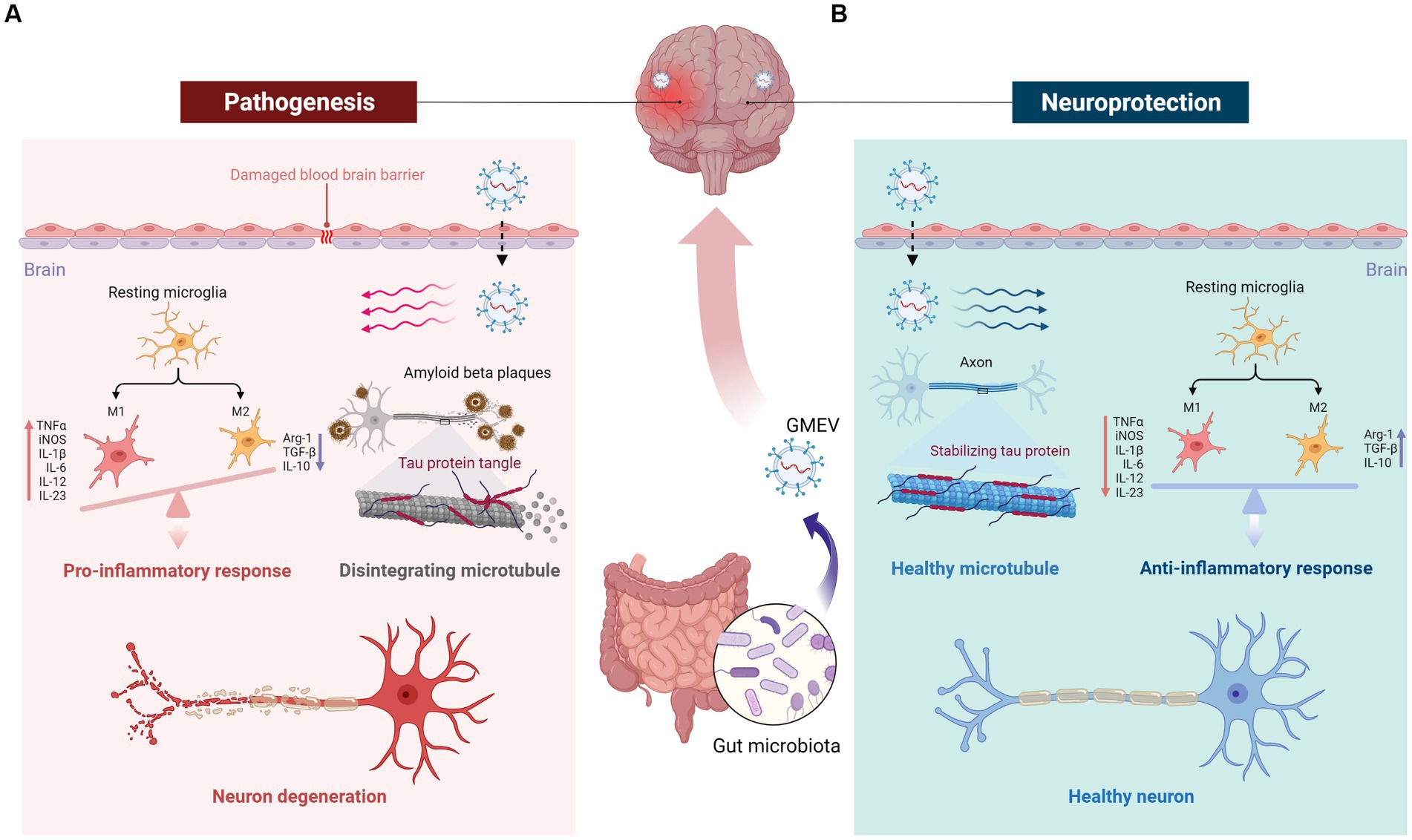
Figure 3. The double role of GMEVs in neurological disorders and the underlying mechanisms. GMEVs may aggravate or alleviate disease states indirectly via conferring harmful or beneficial effects. (A) Pathological GMEVs release their cargoes such as lipopolysaccharide that can cause disruption of the blood–brain barrier (BBB) and release of inflammatory cytokines, leading to activation of microglia and neuroinflammation. (B) The potential beneficial/therapeutic effects of GMEVs in neurological disorders could be via release protective lipids, small molecules, or microRNAs to further regulate downstream protective target proteins, thereby stabilizing the BBB, microglial balance, axon stability, and neuronal function. GMEVs, gut microbiota-released extracellular vesicles.
6.1. Strokes
Ischemic stroke (IS) is a life-threatening cerebral vascular disease accounting for high disability and mortality worldwide. Emerging recent studies suggest a potential role of EVs in the treatment of IS Indeed, in recent years, various kinds of mammalian cell-derived EVs, such as those derived from mesenchymal stem cells, neural stem cells, type 2 microglia, and brain endothelial cells have been used to treat IS by altering the expression of microRNAs (miRs) in the organism (Xu et al., 2023). However, due to the prohibitive cost of mammalian cell culture, it is difficult to obtain a culture medium for EVs extraction on a large scale in the short term, which has limited the translation of EVs research to the clinic. Therefore, an alternative source of EVs with the obvious advantages of having quicker EVs extraction, at a low cost, and in large quantities would accelerate the development of novel therapeutic modalities for IS treatment. In this regard, a recent study has shown the efficacy of Lactobacillus acidophilus-derived EVs in protecting against ischemic brain injuries (Yang et al., 2022). Lactobacillus acidophilus-derived EVs can regulate c-Fos/TGF-β1 by targeting miR101a-3p to reduce neuronal apoptosis caused by IS. For now, the direct link between GMEVs and IS still needs to be further explored. Importantly, the characterization of the bioactive factors in these probiotics-derived EVs that mediate the ameliorating effects of IS will highlight newer avenues to develop novel therapeutic modalities.
6.2. Alzheimer’s disease
AD involves various changes in the brain, including the accumulation of abnormal protein deposits, neuroinflammation, synaptic dysfunction, and neuronal loss (Cervellati and Zuliani, 2023). These changes contribute to the progressive decline in cognitive function and memory loss characteristic of AD. Epidemiological studies show that intestinal bacterial infection will increase the prevalence of AD, but most intestinal bacteria do not directly invade the CNS (Hooi et al., 2017). The OMVs derived from gut microbiota are considered to play an important role in AD (Jaeger et al., 2009; Banks and Robinson, 2010). GMEVs may be an important driver of AD pathology and play an important role in AD’s pathogenesis, development, and defense. More importantly, GMEVs may also extend the pathological range of bacteria to the whole body, causing further cascade reactions and aggravating the injury. Although there is some evidence to suggest that OMV can promote inflammatory response and exacerbate adverse effects in AD, recent studies have shown that there are also some probiotics that can alleviate disease damage after AD, and their derived GMEVs may play an important role in this process. Nutritional supplements or medications containing probiotics can improve intestinal microbiota imbalance or neurological symptoms in AD patients. These effects may be caused by the recovery of gut microbiota and the regulatory changes in GMEVs in the gut-brain axis (Pluta et al., 2020). Although there is currently limited evidence that GMEVs directly regulate AD neurological disorders, the beneficial effects of probiotics on AD are suggestive of the potential role of probiotics-derived EVs in ameliorating AD-associated complications. Meanwhile, some studies have shown that in some diseases, probiotics can act through small molecule substances such as miRs and lipids encapsulated in GMEV (Díez-Sainz et al., 2022). For example, the EVs secreted by A. actinomycetemcomitans can reach the mouse brain and play a potential role in neuroinflammatory diseases through exogenous RNA cargo (Han et al., 2019).
6.3. Parkinson’s disease
PD is the second most common neurodegenerative disease, and the incidence rate continues to rise. Pathological signs of PD include nerve inclusion bodies in the form of Lewy body and cell loss in the neurite, substantia nigra, and other brain regions (Tolosa et al., 2021). Similar to AD, epidemiological studies also show that intestinal bacterial composition will affect the status of PD (Hooi et al., 2017). There is evidence even to implicate that PD may start in the gut and then spread to the brain through the vagus nerve (Holmqvist et al., 2014). In contrast, gut microbiota holds the promise of playing a beneficial role in PD and is likely associated with GMEV. Lactobacillus plantarum is an abundant family of probiotics, and multiple subtypes are neuroprotective in PD. Lactobacillus plantarum CCFM405 alleviates rotenone-induced PD mice via regulating gut microbiota and branched-chain amino acids biosynthesis (Chu et al., 2023). Lactobacillus plantarum DP189 reduces α-synuclein aggravation in MPTP-induced PD in mice via regulating oxidative damage, inflammation, and gut microbiota disorder (Wang et al., 2022). Although the above studies did not directly indicate the protective effect of Lactobacillus plantarum-derived EVs on PD, Lactobacillus plantarum-derived EVs have been proven to have clinical effects in other diseases, such as inhibiting wrinkle formation and pigmentation, and affecting inflammatory factors in macrophages generation (Aoki-Yoshida et al., 2017; Jo et al., 2022). Interactions between probiotics and host miRs in modulating host neuropathy are rare, as miRs are one of the common cargoes of GMEVs, and this study may implicate the role of GMEVs. Recently, GMEVs produced by intestinal microorganisms have been proven to be related to the inhibition of hypothalamic energy metabolism in major depressive disorder patients (Qi et al., 2020). For example, the GMEVs extracted from Lactobacillus plantarum have been shown to exhibit antidepressant-like activity in mice (Choi et al., 2019) supporting the potential use of GMEVs as a biological therapy for neurological disorder-induced depression.
Research on the beneficial effects of GMEVs on neurological disorders is still in its early stages, warranting detailed studies to define therapeutic potential and mechanisms of GMEVs in neurological disorders. While these findings suggest promising avenues for future investigations, the application of EV-based therapies in clinical settings is still a developing field.
7. Biomarker role of circulating GMEVs in neurological disorders
EVs are abundant in all body fluids, stable and small in size, and can easily cross physiological barriers, so they are considered to be a beneficial source of circulating biomarkers (van Niel et al., 2018). Since GMEVs are enriched by molecular content (Pathan et al., 2019), GMEVs are also considered biomarkers for many neurodegenerative diseases such as AD and PD (Yuyama et al., 2015; Kapogiannis et al., 2019; Pérez et al., 2019).
The contents of GMEVs exist in different forms of origin, either as components of the parental cell or as membrane-associated particles. During GMEVs biogenesis, different cargoes (i.e., mRNA, DNA, proteins, lipids, etc.) are loaded into vesicles; this can serve as a surrogate indicator of the parental cell to provide specific cell-of-origin biomarkers (Im et al., 2014; Keerthikumar et al., 2016). The study observed a correlation between GMEVs and the development of neurological syndromes like cerebral malaria, where carbohydrase 1 and S100A8 were identified as cargoes of cerebral malaria syndrome EV by proteomic analysis (Tiberti et al., 2016). They are specifically increased during pathogenesis, strengthening the notion of these molecules as biomarkers for malaria. Therefore, further research on GMEVs as biomarkers for more neurological disorders is promising.
8. Does the type of diet influence the type of EVs produced by gut bacteria?
The efficacy of GMEVs in conferring a beneficial or harmful effect on host health is species-specific and modulated by diet. Studies in mice have shown that a high-fat diet (HFD)-induced increase in the proteobacterium Pseudomonas panaceas–derived EVs is strongly associated with the progression of metabolic disorders such as obesity, diabetes, and hypertension, the known risk factors of stroke (Choi et al., 2011). In contrast, Akkermansia muciniphila EVs are linked with alleviating HFD-induced metabolic dysfunctions (Yaghoubfar et al., 2020). Therefore, detailed mechanistic studies utilizing experimental models of stroke are warranted to select the probiotic bacterial species having beneficial effects on neuron/brain function and to unravel the critical role of their EVs in alleviating the risk factors for IS and/or the unfavorable post-stroke outcomes.
Importantly, glial cells in CNS have been proven to participate in weight homeostasis and obesity, while a HFD can usually induce obesity and metabolic syndrome, so diet has the potential to regulate the neuroinflammation (Horvath et al., 2010; García-Cáceres et al., 2012; Valdearcos et al., 2017). There is a close relationship between diet and intestinal flora, but little is known about the regulation of GMEVs by diet. Sundaram et al. developed a kind of garlic exosome-like nanoparticles (GaELNs) and administered them orally to mice on a high-calorie diet (Sundaram et al., 2022). This study’s results showed that GaELNs can preferentially ingest microglia and inhibit brain inflammation in HFD mice. GaELN phosphatidic acid forms a complex through the interaction with microglial cell brain acid soluble protein 1 and inhibits the expression of c-Myc-mediated STING, resulting in the reduction of the expression of a series of inflammatory cytokines, thus promoting neuronal differentiation and inhibiting mitochondria-mediated neuronal cell death. Although the role of GMEVs in this study is unknown, it is also an indisputable fact that EVs can regulate the neuroinflammation of mice under HFD through oral action on the intestine, which may lay a foundation for us to study the regulation of GMEVs by diet in the next step to regulate nervous system diseases.
9. Engineering bacteria to produce beneficial GMEVs
As biologically derived entities, GMEVs can be modified to achieve enhanced desirable attributes through molecular biology and bioengineering technology. Fundamentally, GMEVs may be modified via two approaches; engineering parental strain to create superior therapeutic EVs and engineering EVs after isolation to improve their functionality (Figure 4). Escherichia coli provides a broad and flexible platform for the development of bioengineered OMVs. The system contains a variety of target proteins that can be fused with OMVs-related toxin cytolysin A, including β-lactamase, organophosphate hydrolase, green fluorescent protein, and Fc antibody fragments. This system enables the display of chimeras on the OMVs’ surface, preserving target proteins and OMVs’ function (Kim et al., 2008).
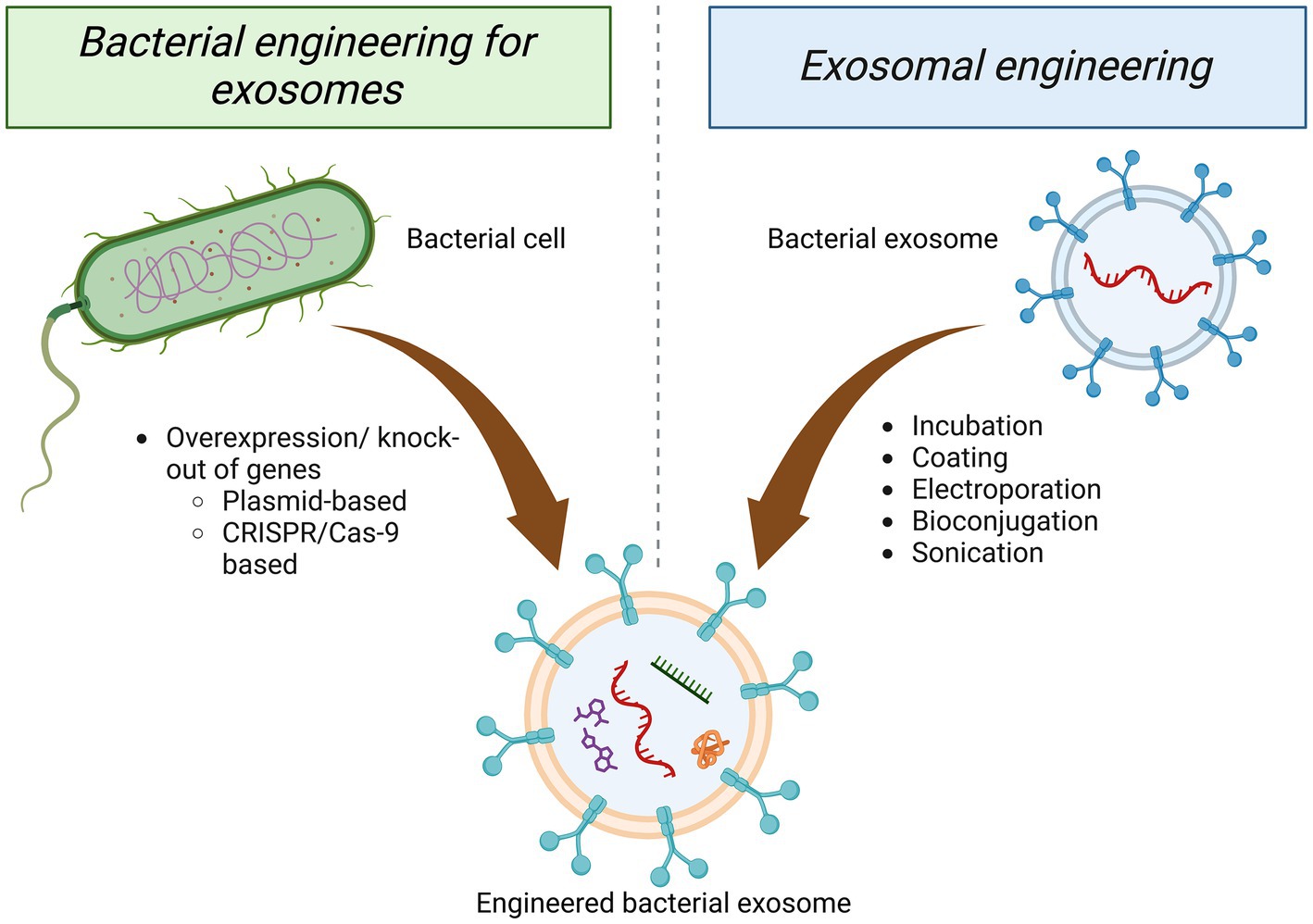
Figure 4. Approaches of engineering GMEVs. Engineering of GMEVs to confer superior functionality/beneficial effects can be achieved mainly via two approaches, either through the parental bacterial cell or isolated GMEVs. Engineering of the parental bacteria can be conducted via targeting bacterial cells for plasmid-based or CRISPR/Cas-9-based overexpression/knock-out of specific genes. The engineering of GMEVs can be achieved via various techniques such as—incubation, coating, electroporation, bioconjugation, sonication, etc. with specific genes, chemical drugs, genetic materials, etc. GMEVs, gut microbiota-released extracellular vesicles.
In addition, GMEVs can be loaded with therapeutics, and their cohesins, sulfatases, and proteases, facilitating interactions with and enter host epithelial cells, making them potentially effective drug delivery vehicles (Shen et al., 2012; Elhenawy et al., 2014; Hickey et al., 2015; Stentz et al., 2015). Importantly, drug delivery to the brain is usually limited by the BBB, and OMVs have great potential because of their ability to cross the BBB (Dong, 2018; Wei et al., 2020). Chen et al. applied a mixture of nano-gold and GMEVs to enhance the radiotherapy and immunotherapy effect against glioblastoma and prolong the survival period of mice (Chen et al., 2021). In addition, OMVs can be taken up by surrounding neutrophils and signal to microglia, and thus may be able to deliver drugs to microglia (Li et al., 2020). OMVs have been loaded with drugs or siRNA for targeted therapy in cancer treatment, and we expect to be able to target OMVs to the brain for the treatment (Shi et al., 2016; Kim et al., 2017).
Gut microbiota dysbiosis may contribute to neurological diseases, and the targeted application of GMEVs as therapeutic vaccines may be beneficial. In this case, the vaccine does not eliminate the pathogen itself but instead inhibits the active molecules released by the pathogen, leading to deleterious remodeling of the microbiota and subsequent disease onset (Pirolli et al., 2021). For example, an EcN OMVs vaccine was used to generate a broadly protective immune response to conserved microbial sugars that outperformed conventional vaccines, which have historically been costly and laborious to produce (Stevenson et al., 2018).
10. Conclusions and future perspectives
GMEVs have long been viewed as a double-edged sword for the gut-brain axis. The downside is that GMEVs can act as a causative factor to activate harmful neuroinflammation, thereby inducing the pathology of some neurodegenerative diseases, especially AD. On the plus side, the carrier role of GMEVs and their ability to cross biological barriers can amplify their specific immunomodulatory potential, which is expected to induce protective immunity and target biological barriers (such as the BBB) in a variety of diseases for drug delivery. In particular, probiotic GMEVs or OMVs vaccines can induce broad protective effects to improve microbiota dysbiosis, enhance the body’s defense mechanisms against pathogenic gut microbes, and even directly target brain therapy. At present, the value of GMEVs as a therapeutic carrier and therapeutic target for neurodegenerative diseases has been explored to some extent. But the relationship of GMEVs to genetic risk factors for neurodegeneration remains to be resolved. Furthermore, how GMEVs play a role in other neurological disorders, including IS, still needs to be explored. Brain injury has been shown to lead to intestinal chronic changes in the microbiome, which in turn may further propagate neurological damage and suggest a potential role for GMEVs in brain injury, especially IS, traumatic brain injury (Peh et al., 2022). The transportation and transmission efficiency of GMEVs-mediated signaling molecules are also lacking for further research, and how to use bioengineering techniques to decorate GMEVs to play their beneficial role also needs to be explored.
In summary, GMEVs, due to their similar composition to the gut microbiota, can replicate the deleterious and beneficial effects of pathogenic and probiotic bacteria and can directly affect neurological disorders. Moreover, due to their special carrier role, they have the potential to spread over long distances in the body, cross the BBB and induce neuroimmune responses that alter neurological function. Therefore, how to limit its bad effects, further exert its beneficial effects, and explore its role in more types of neurological diseases are the avenues for further extensive investigation.
Author contributions
BS and HS searched the literature and drafted the manuscript. AB and JB critically revised the manuscript. All authors contributed to the article and approved the submitted version.
Funding
This work was supported by the National Institute of Neurological Disorders and Stroke (1R01NS102720), Chronic Disease Research Program (WV-INBRE grant, P20GM103434) and West Virginia Clinical and Translational Science Institute (WV-CTSI) grant (2U54GM104942) to JB, National Institute of Allergy and Infectious Disease (AI130790-03), and National Institute of General Medical Sciences (P20 GM 121299-04) to AB.
Acknowledgments
Figures have been created with BioRender.com.
Conflict of interest
The authors declare that the research was conducted in the absence of any commercial or financial relationships that could be construed as a potential conflict of interest.
Publisher’s note
All claims expressed in this article are solely those of the authors and do not necessarily represent those of their affiliated organizations, or those of the publisher, the editors and the reviewers. Any product that may be evaluated in this article, or claim that may be made by its manufacturer, is not guaranteed or endorsed by the publisher.
References
Aguilera, L., Toloza, L., Giménez, R., Odena, A., Oliveira, E., Aguilar, J., et al. (2014). Proteomic analysis of outer membrane vesicles from the probiotic strain Escherichia coli Nissle 1917. Proteomics 14, 222–229. doi: 10.1002/pmic.201300328
Al-Nedawi, K., Mian, M. F., Hossain, N., Karimi, K., Mao, Y. K., Forsythe, P., et al. (2015). Gut commensal microvesicles reproduce parent bacterial signals to host immune and enteric nervous systems. FASEB J. 29, 684–695. doi: 10.1096/fj.14-259721
Altindis, E., Fu, Y., and Mekalanos, J. J. (2014). Proteomic analysis of Vibrio cholerae outer membrane vesicles. Proc. Natl. Acad. Sci. U. S. A. 111, E1548–E1556. doi: 10.1073/pnas.1403683111
Alvarez, C. S., Badia, J., Bosch, M., Giménez, R., and Baldomà, L. (2016). Outer membrane vesicles and soluble factors released by probiotic Escherichia coli Nissle 1917 and commensal ECOR63 enhance barrier function by regulating expression of tight junction proteins in intestinal epithelial cells. Front. Microbiol. 7:1981. doi: 10.3389/fmicb.2016.01981
Aoki-Yoshida, A., Saito, S., Tsuruta, T., Ohsumi, A., Tsunoda, H., and Sonoyama, K. (2017). Exosomes isolated from sera of mice fed Lactobacillus strains affect inflammatory cytokine production in macrophages in vitro. Biochem. Biophys. Res. Commun. 489, 248–254. doi: 10.1016/j.bbrc.2017.05.152
Banks, W. A., and Robinson, S. M. (2010). Minimal penetration of lipopolysaccharide across the murine blood-brain barrier. Brain Behav. Immun. 24, 102–109. doi: 10.1016/j.bbi.2009.09.001
Barák, I., and Muchová, K. (2013). The role of lipid domains in bacterial cell processes. Int. J. Mol. Sci. 14, 4050–4065. doi: 10.3390/ijms14024050
Behzadi, E., Mahmoodzadeh Hosseini, H., and Imani Fooladi, A. A. (2017). The inhibitory impacts of Lactobacillus rhamnosus GG-derived extracellular vesicles on the growth of hepatic cancer cells. Microb. Pathog. 110, 1–6. doi: 10.1016/j.micpath.2017.06.016
Bitto, N. J., and Kaparakis-Liaskos, M. (2017). The therapeutic benefit of bacterial membrane vesicles. Int. J. Mol. Sci. 18:1287. doi: 10.3390/ijms18061287
Bonaz, B., Bazin, T., and Pellissier, S. (2018). The Vagus nerve at the Interface of the microbiota-gut-brain Axis. Front. Neurosci. 12:49. doi: 10.3389/fnins.2018.00049
Bonnington, K. E., and Kuehn, M. J. (2014). Protein selection and export via outer membrane vesicles. Biochim. Biophys. Acta 1843, 1612–1619. doi: 10.1016/j.bbamcr.2013.12.011
Brameyer, S., Plener, L., Müller, A., Klingl, A., Wanner, G., and Jung, K. (2018). Outer membrane vesicles facilitate trafficking of the hydrophobic signaling molecule CAI-1 between Vibrio harveyi cells. J. Bacteriol. 200:17. doi: 10.1128/JB.00740-17
Brookes, S. J., Spencer, N. J., Costa, M., and Zagorodnyuk, V. P. (2013). Extrinsic primary afferent signalling in the gut. Nat. Rev. Gastroenterol. Hepatol. 10, 286–296. doi: 10.1038/nrgastro.2013.29
Brown, L., Wolf, J. M., Prados-Rosales, R., and Casadevall, A. (2015). Through the wall: extracellular vesicles in gram-positive bacteria, mycobacteria and fungi. Nat. Rev. Microbiol. 13, 620–630. doi: 10.1038/nrmicro3480
Caruana, J. C., and Walper, S. A. (2020). Bacterial membrane vesicles as mediators of microbe —microbe and microbe—host community interactions. Front. Microbiol. 11:432. doi: 10.3389/fmicb.2020.00432
Celluzzi, A., and Masotti, A. (2016). How our other genome controls our epi-genome. Trends Microbiol. 24, 777–787. doi: 10.1016/j.tim.2016.05.005
Cervellati, C., and Zuliani, G. (2023). Frontier on Alzheimer's disease. Int. J. Mol. Sci. 24:7748. doi: 10.3390/ijms24097748
Chatterjee, S., Mondal, A., Mitra, S., and Basu, S. (2017). Acinetobacter baumannii transfers the blaNDM-1 gene via outer membrane vesicles. J. Antimicrob. Chemother. 72, 2201–2207. doi: 10.1093/jac/dkx131
Chatzidaki-Livanis, M., Coyne, M. J., and Comstock, L. E. (2014). An antimicrobial protein of the gut symbiont Bacteroides fragilis with a MACPF domain of host immune proteins. Mol. Microbiol. 94, 1361–1374. doi: 10.1111/mmi.12839
Chen, M. H., Liu, T. Y., Chen, Y. C., and Chen, M. H. (2021). Combining augmented radiotherapy and immunotherapy through a Nano-gold and bacterial outer-membrane vesicle complex for the treatment of glioblastoma. Nanomaterials 11:1661. doi: 10.3390/nano11071661
Choi, D. S., Kim, D. K., Choi, S. J., Lee, J., Choi, J. P., Rho, S., et al. (2011). Proteomic analysis of outer membrane vesicles derived from Pseudomonas aeruginosa. Proteomics 11, 3424–3429. doi: 10.1002/pmic.201000212
Choi, J., Kim, Y. K., and Han, P. L. (2019). Extracellular vesicles derived from Lactobacillus plantarum increase BDNF expression in cultured hippocampal neurons and produce antidepressant-like effects in mice. Exp Neurobiol 28, 158–171. doi: 10.5607/en.2019.28.2.158
Choi, J. W., Um, J. H., Cho, J. H., and Lee, H. J. (2017). Tiny RNAs and their voyage via extracellular vesicles: secretion of bacterial small RNA and eukaryotic microRNA. Exp. Biol. Med. 242, 1475–1481. doi: 10.1177/1535370217723166
Chowdhury, C., and Jagannadham, M. V. (2013). Virulence factors are released in association with outer membrane vesicles of Pseudomonas syringae pv. Tomato T1 during normal growth. Biochim. Biophys. Acta 1834, 231–239. doi: 10.1016/j.bbapap.2012.09.015
Chu, C., Yu, L., Li, Y., Guo, H., Zhai, Q., Chen, W., et al. (2023). Lactobacillus plantarum CCFM405 against rotenone-induced Parkinson's disease mice via regulating gut microbiota and branched-chain amino acids biosynthesis. Nutrients 15:1737. doi: 10.3390/nu15071737
Clarke, G., Grenham, S., Scully, P., Fitzgerald, P., Moloney, R. D., Shanahan, F., et al. (2013). The microbiome-gut-brain axis during early life regulates the hippocampal serotonergic system in a sex-dependent manner. Mol. Psychiatry 18, 666–673. doi: 10.1038/mp.2012.77
Coelho, C., Brown, L., Maryam, M., Vij, R., Smith, D. F. Q., Burnet, M. C., et al. (2019). Listeria monocytogenes virulence factors, including listeriolysin O, are secreted in biologically active extracellular vesicles. J. Biol. Chem. 294, 1202–1217. doi: 10.1074/jbc.RA118.006472
Cryan, J. F., O'riordan, K. J., Sandhu, K., Peterson, V., and Dinan, T. G. (2020). The gut microbiome in neurological disorders. Lancet Neurol. 19, 179–194. doi: 10.1016/S1474-4422(19)30356-4
Dean, S. N., Leary, D. H., Sullivan, C. J., Oh, E., and Walper, S. A. (2019). Isolation and characterization of Lactobacillus-derived membrane vesicles. Sci. Rep. 9:877. doi: 10.1038/s41598-018-37120-6
Dean, S. N., Rimmer, M. A., Turner, K. B., Phillips, D. A., Caruana, J. C., Hervey, W. J. T., et al. (2020). Lactobacillus acidophilus membrane vesicles as a vehicle of Bacteriocin delivery. Front. Microbiol. 11:710. doi: 10.3389/fmicb.2020.00710
Diaz-Garrido, N., Cordero, C., Olivo-Martinez, Y., Badia, J., and Baldomà, L. (2021). Cell-to-cell communication by host-released extracellular vesicles in the gut: implications in health and disease. Int. J. Mol. Sci. 22:2213. doi: 10.3390/ijms22042213
Díez-Sainz, E., Milagro, F. I., Riezu-Boj, J. I., and Lorente-Cebrián, S. (2022). Effects of gut microbiota-derived extracellular vesicles on obesity and diabetes and their potential modulation through diet. J. Physiol. Biochem. 78, 485–499. doi: 10.1007/s13105-021-00837-6
Domingues, S., and Nielsen, K. M. (2017). Membrane vesicles and horizontal gene transfer in prokaryotes. Curr. Opin. Microbiol. 38, 16–21. doi: 10.1016/j.mib.2017.03.012
Dong, X. (2018). Current strategies for brain drug delivery. Theranostics 8, 1481–1493. doi: 10.7150/thno.21254
Elhenawy, W., Debelyy, M. O., and Feldman, M. F. (2014). Preferential packing of acidic glycosidases and proteases into Bacteroides outer membrane vesicles. MBio 5, e00909–e00914. doi: 10.1128/mBio.00909-14
Elmi, A., Nasher, F., Jagatia, H., Gundogdu, O., Bajaj-Elliott, M., Wren, B., et al. (2016). Campylobacter jejuni outer membrane vesicle-associated proteolytic activity promotes bacterial invasion by mediating cleavage of intestinal epithelial cell E-cadherin and occludin. Cell. Microbiol. 18, 561–572. doi: 10.1111/cmi.12534
Evans, A. G. L., Davey, H. M., Cookson, A., Currinn, H., Cooke-Fox, G., Stanczyk, P. J., et al. (2012). Predatory activity of Myxococcus xanthus outer-membrane vesicles and properties of their hydrolase cargo. Microbiology 158, 2742–2752. doi: 10.1099/mic.0.060343-0
Foster, J. A., Rinaman, L., and Cryan, J. F. (2017). Stress & the gut-brain axis: regulation by the microbiome. Neurobiol Stress 7, 124–136. doi: 10.1016/j.ynstr.2017.03.001
García-Cáceres, C., Fuente-Martín, E., Argente, J., and Chowen, J. A. (2012). Emerging role of glial cells in the control of body weight. Mol Metab 1, 37–46. doi: 10.1016/j.molmet.2012.07.001
Gilmore, W. J., Johnston, E. L., Zavan, L., Bitto, N. J., and Kaparakis-Liaskos, M. (2021). Immunomodulatory roles and novel applications of bacterial membrane vesicles. Mol. Immunol. 134, 72–85. doi: 10.1016/j.molimm.2021.02.027
Giordano, N. P., Cian, M. B., and Dalebroux, Z. D. (2020). Outer membrane lipid secretion and the innate immune response to gram-negative Bacteria. Infect. Immun. 88, e00920–19. doi: 10.1128/IAI.00920-19
Goehler, L. E., Park, S. M., Opitz, N., Lyte, M., and Gaykema, R. P. (2008). Campylobacter jejuni infection increases anxiety-like behavior in the holeboard: possible anatomical substrates for viscerosensory modulation of exploratory behavior. Brain Behav. Immun. 22, 354–366. doi: 10.1016/j.bbi.2007.08.009
Gosiewski, T., Ludwig-Galezowska, A. H., Huminska, K., Sroka-Oleksiak, A., Radkowski, P., Salamon, D., et al. (2017). Comprehensive detection and identification of bacterial DNA in the blood of patients with sepsis and healthy volunteers using next-generation sequencing method—the observation of DNAemia. Eur. J. Clin. Microbiol. Infect. Dis. 36, 329–336. doi: 10.1007/s10096-016-2805-7
Günzel, D., and Yu, A. S. (2013). Claudins and the modulation of tight junction permeability. Physiol. Rev. 93, 525–569. doi: 10.1152/physrev.00019.2012
Han, E. C., Choi, S. Y., Lee, Y., Park, J. W., Hong, S. H., and Lee, H. J. (2019). Extracellular RNAs in periodontopathogenic outer membrane vesicles promote TNF-α production in human macrophages and cross the blood-brain barrier in mice. FASEB J. 33, 13412–13422. doi: 10.1096/fj.201901575R
Haurat, M. F., Aduse-Opoku, J., Rangarajan, M., Dorobantu, L., Gray, M. R., Curtis, M. A., et al. (2011). Selective sorting of cargo proteins into bacterial membrane vesicles. J. Biol. Chem. 286, 1269–1276. doi: 10.1074/jbc.M110.185744
Heiss, C. N., and Olofsson, L. E. (2019). The role of the gut microbiota in development, function and disorders of the central nervous system and the enteric nervous system. J. Neuroendocrinol. 31:e12684. doi: 10.1111/jne.12684
Hickey, C. A., Kuhn, K. A., Donermeyer, D. L., Porter, N. T., Jin, C., Cameron, E. A., et al. (2015). Colitogenic Bacteroides thetaiotaomicron antigens access host immune cells in a sulfatase-dependent manner via outer membrane vesicles. Cell Host Microbe 17, 672–680. doi: 10.1016/j.chom.2015.04.002
Holmqvist, S., Chutna, O., Bousset, L., Aldrin-Kirk, P., Li, W., Björklund, T., et al. (2014). Direct evidence of Parkinson pathology spread from the gastrointestinal tract to the brain in rats. Acta Neuropathol. 128, 805–820. doi: 10.1007/s00401-014-1343-6
Hong, J., Dauros-Singorenko, P., Whitcombe, A., Payne, L., Blenkiron, C., Phillips, A., et al. (2019). Analysis of the Escherichia coli extracellular vesicle proteome identifies markers of purity and culture conditions. J Extracell Vesicles 8:1632099. doi: 10.1080/20013078.2019.1632099
Hooi, J. K. Y., Lai, W. Y., Ng, W. K., Suen, M. M. Y., Underwood, F. E., Tanyingoh, D., et al. (2017). Global prevalence of Helicobacter pylori infection: systematic review and Meta-analysis. Gastroenterology 153, 420–429. doi: 10.1053/j.gastro.2017.04.022
Horvath, T. L., Sarman, B., García-Cáceres, C., Enriori, P. J., Sotonyi, P., Shanabrough, M., et al. (2010). Synaptic input organization of the melanocortin system predicts diet-induced hypothalamic reactive gliosis and obesity. Proc. Natl. Acad. Sci. U. S. A. 107, 14875–14880. doi: 10.1073/pnas.1004282107
Im, H., Shao, H., Park, Y. I., Peterson, V. M., Castro, C. M., Weissleder, R., et al. (2014). Label-free detection and molecular profiling of exosomes with a nano-plasmonic sensor. Nat. Biotechnol. 32, 490–495. doi: 10.1038/nbt.2886
Jaeger, L. B., Dohgu, S., Sultana, R., Lynch, J. L., Owen, J. B., Erickson, M. A., et al. (2009). Lipopolysaccharide alters the blood-brain barrier transport of amyloid beta protein: a mechanism for inflammation in the progression of Alzheimer's disease. Brain Behav. Immun. 23, 507–517. doi: 10.1016/j.bbi.2009.01.017
Jan, A. T. (2017). Outer membrane vesicles (OMVs) of gram-negative Bacteria: a perspective update. Front. Microbiol. 8:1053. doi: 10.3389/fmicb.2017.01053
Jang, K. S., Sweredoski, M. J., Graham, R. L., Hess, S., and Clemons, W. M. Jr. (2014). Comprehensive proteomic profiling of outer membrane vesicles from Campylobacter jejuni. J. Proteomics 98, 90–98. doi: 10.1016/j.jprot.2013.12.014
Jeffery, C. J. (2018). Protein moonlighting: what is it, and why is it important? Philos. Trans. R. Soc. Lond. B Biol. Sci. 373:20160523. doi: 10.1098/rstb.2016.0523
Jeon, H., Oh, M. H., Jun, S. H., Kim, S. I., Choi, C. W., Kwon, H. I., et al. (2016). Variation among Staphylococcus aureus membrane vesicle proteomes affects cytotoxicity of host cells. Microb. Pathog. 93, 185–193. doi: 10.1016/j.micpath.2016.02.014
Jiang, Y., Kong, Q., Roland, K. L., and Curtiss, R. (2014). Membrane vesicles of Clostridium perfringens type a strains induce innate and adaptive immunity. Int. J. Med. Microbiol. 304, 431–443. doi: 10.1016/j.ijmm.2014.02.006
Jo, C. S., Myung, C. H., Yoon, Y. C., Ahn, B. H., Min, J. W., Seo, W. S., et al. (2022). The effect of Lactobacillus plantarum extracellular vesicles from Korean women in their 20s on skin aging. Curr. Issues Mol. Biol. 44, 526–540. doi: 10.3390/cimb44020036
Kapogiannis, D., Mustapic, M., Shardell, M. D., Berkowitz, S. T., Diehl, T. C., Spangler, R. D., et al. (2019). Association of Extracellular Vesicle Biomarkers with Alzheimer Disease in the Baltimore longitudinal study of aging. JAMA Neurol. 76, 1340–1351. doi: 10.1001/jamaneurol.2019.2462
Keerthikumar, S., Chisanga, D., Ariyaratne, D., Al Saffar, H., Anand, S., Zhao, K., et al. (2016). ExoCarta: a web-based compendium of Exosomal cargo. J. Mol. Biol. 428, 688–692. doi: 10.1016/j.jmb.2015.09.019
Kim, O. Y., Dinh, N. T., Park, H. T., Choi, S. J., Hong, K., and Gho, Y. S. (2017). Bacterial protoplast-derived nanovesicles for tumor targeted delivery of chemotherapeutics. Biomaterials 113, 68–79. doi: 10.1016/j.biomaterials.2016.10.037
Kim, J. Y., Doody, A. M., Chen, D. J., Cremona, G. H., Shuler, M. L., Putnam, D., et al. (2008). Engineered bacterial outer membrane vesicles with enhanced functionality. J. Mol. Biol. 380, 51–66. doi: 10.1016/j.jmb.2008.03.076
Kim, J. H., Lee, J., Park, J., and Gho, Y. S. (2015). Gram-negative and gram-positive bacterial extracellular vesicles. Semin. Cell Dev. Biol. 40, 97–104. doi: 10.1016/j.semcdb.2015.02.006
Koeppen, K., Hampton, T. H., Jarek, M., Scharfe, M., Gerber, S. A., Mielcarz, D. W., et al. (2016). A novel mechanism of host-pathogen interaction through sRNA in bacterial outer membrane vesicles. PLoS Pathog. 12:e1005672. doi: 10.1371/journal.ppat.1005672
Kulkarni, H. M., and Jagannadham, M. V. (2014). Biogenesis and multifaceted roles of outer membrane vesicles from gram-negative bacteria. Microbiology 160, 2109–2121. doi: 10.1099/mic.0.079400-0
Kunze, W. A., Mao, Y. K., Wang, B., Huizinga, J. D., Ma, X., Forsythe, P., et al. (2009). Lactobacillus reuteri enhances excitability of colonic AH neurons by inhibiting calcium-dependent potassium channel opening. J. Cell. Mol. Med. 13, 2261–2270. doi: 10.1111/j.1582-4934.2009.00686.x
Lee, H. J. (2019). Microbe-host communication by small RNAs in extracellular vesicles: vehicles for Transkingdom RNA transportation. Int. J. Mol. Sci. 20:1487. doi: 10.3390/ijms20061487
Lee, E. Y., Bang, J. Y., Park, G. W., Choi, D. S., Kang, J. S., Kim, H. J., et al. (2007). Global proteomic profiling of native outer membrane vesicles derived from Escherichia coli. Proteomics 7, 3143–3153. doi: 10.1002/pmic.200700196
Lee, E. Y., Choi, D. S., Kim, K. P., and Gho, Y. S. (2008). Proteomics in gram-negative bacterial outer membrane vesicles. Mass Spectrom. Rev. 27, 535–555. doi: 10.1002/mas.20175
Lee, E. Y., Choi, D. Y., Kim, D. K., Kim, J. W., Park, J. O., Kim, S., et al. (2009). Gram-positive bacteria produce membrane vesicles: proteomics-based characterization of Staphylococcus aureus-derived membrane vesicles. Proteomics 9, 5425–5436. doi: 10.1002/pmic.200900338
Lee, J. H., Choi, C. W., Lee, T., Kim, S. I., Lee, J. C., and Shin, J. H. (2013). Transcription factor σB plays an important role in the production of extracellular membrane-derived vesicles in Listeria monocytogenes. PLoS One 8:e73196. doi: 10.1371/journal.pone.0073196
Lee, J., Kim, O. Y., and Gho, Y. S. (2016). Proteomic profiling of gram-negative bacterial outer membrane vesicles: current perspectives. Proteomics Clin. Appl. 10, 897–909. doi: 10.1002/prca.201600032
Lee, K. E., Kim, J. K., Han, S. K., Lee, D. Y., Lee, H. J., Yim, S. V., et al. (2020). The extracellular vesicle of gut microbial Paenalcaligenes hominis is a risk factor for vagus nerve-mediated cognitive impairment. Microbiome 8:107. doi: 10.1186/s40168-020-00881-2
Li, M., Lee, K., Hsu, M., Nau, G., Mylonakis, E., and Ramratnam, B. (2017). Lactobacillus-derived extracellular vesicles enhance host immune responses against vancomycin-resistant enterococci. BMC Microbiol. 17:66. doi: 10.1186/s12866-017-0977-7
Li, M., Li, S., Zhou, H., Tang, X., Wu, Y., Jiang, W., et al. (2020). Chemotaxis-driven delivery of nano-pathogenoids for complete eradication of tumors post-phototherapy. Nat. Commun. 11:1126. doi: 10.1038/s41467-020-14963-0
Lyte, M. (2011). Probiotics function mechanistically as delivery vehicles for neuroactive compounds: microbial endocrinology in the design and use of probiotics. Bioessays 33, 574–581. doi: 10.1002/bies.201100024
Margolis, K. G., Cryan, J. F., and Mayer, E. A. (2021). The microbiota-gut-brain Axis: from motility to mood. Gastroenterology 160, 1486–1501. doi: 10.1053/j.gastro.2020.10.066
Marshall, R. C., and Whitworth, D. E. (2019). Is "Wolf-pack" predation by antimicrobial Bacteria cooperative? Cell behaviour and predatory mechanisms indicate profound selfishness, even when working alongside kin. Bioessays 41:e1800247. doi: 10.1002/bies.201800247
Martin, C. R., Osadchiy, V., Kalani, A., and Mayer, E. A. (2018). The brain-gut-microbiome Axis. Cell. Mol. Gastroenterol. Hepatol. 6, 133–148. doi: 10.1016/j.jcmgh.2018.04.003
Morais, L. H., Schreiber, H. L. T., and Mazmanian, S. K. (2021). The gut microbiota-brain axis in behaviour and brain disorders. Nat. Rev. Microbiol. 19, 241–255. doi: 10.1038/s41579-020-00460-0
Nevermann, J., Silva, A., Otero, C., Oyarzún, D. P., Barrera, B., Gil, F., et al. (2019). Identification of genes involved in biogenesis of outer membrane vesicles (OMVs) in Salmonella enterica Serovar Typhi. Front. Microbiol. 10:104. doi: 10.3389/fmicb.2019.00104
O’Donoghue, E. J., and Anne, M. K. (2016). Mechanisms of outer membrane vesicle entry into host cells.. Cellular microbiology 18, 1508–1517. doi: 10.1111/cmi.12655
Olaya-Abril, A., Prados-Rosales, R., Mcconnell, M. J., Martín-Peña, R., González-Reyes, J. A., Jiménez-Munguía, I., et al. (2014). Characterization of protective extracellular membrane-derived vesicles produced by Streptococcus pneumoniae. J. Proteomics 106, 46–60. doi: 10.1016/j.jprot.2014.04.023
Osadchiy, V., Martin, C. R., and Mayer, E. A. (2019). The gut-brain Axis and the microbiome: mechanisms and clinical implications. Clin. Gastroenterol. Hepatol. 17, 322–332. doi: 10.1016/j.cgh.2018.10.002
Park, J. Y., Choi, J., Lee, Y., Lee, J. E., Lee, E. H., Kwon, H. J., et al. (2017). Metagenome analysis of bodily microbiota in a mouse model of Alzheimer disease using Bacteria-derived membrane vesicles in blood. Exp Neurobiol 26, 369–379. doi: 10.5607/en.2017.26.6.369
Park, A. M., and Tsunoda, I. (2022). Helicobacter pylori infection in the stomach induces neuroinflammation: the potential roles of bacterial outer membrane vesicles in an animal model of Alzheimer's disease. Inflamm Regen 42:39. doi: 10.1186/s41232-022-00224-8
Peh, A., O’Donnell, J. A., Broughton, B. R. S., and Marques, F. Z. (2022). Gut Microbiota and Their Metabolites in Stroke: A Double-Edged Sword. Stroke. 53, 1788–1801. doi: 10.1161/STROKEAHA.121.036800
Pathan, M., Fonseka, P., Chitti, S. V., Kang, T., Sanwlani, R., Van Deun, J., et al. (2019). Vesiclepedia 2019: a compendium of RNA, proteins, lipids and metabolites in extracellular vesicles. Nucleic Acids Res. 47, D516–d519. doi: 10.1093/nar/gky1029
Pérez, M., Avila, J., and Hernández, F. (2019). Propagation of tau via extracellular vesicles. Front. Neurosci. 13:698. doi: 10.3389/fnins.2019.00698
Perez-Burgos, A., Wang, B., Mao, Y. K., Mistry, B., Mcvey Neufeld, K. A., Bienenstock, J., et al. (2013). Psychoactive bacteria Lactobacillus rhamnosus (JB-1) elicits rapid frequency facilitation in vagal afferents. Am. J. Physiol. Gastrointest. Liver Physiol. 304, G211–G220. doi: 10.1152/ajpgi.00128.2012
Pérez-Cruz, C., Delgado, L., López-Iglesias, C., and Mercade, E. (2015). Outer-inner membrane vesicles naturally secreted by gram-negative pathogenic bacteria. PLoS One 10:e0116896. doi: 10.1371/journal.pone.0116896
Pirolli, N. H., Bentley, W. E., and Jay, S. M. (2021). Bacterial extracellular vesicles and the gut-microbiota brain Axis: emerging roles in communication and potential as therapeutics. Adv Biol 5:e2000540. doi: 10.1002/adbi.202000540
Pluta, R., Ułamek-Kozioł, M., Januszewski, S., and Czuczwar, S. J. (2020). Gut microbiota and pro/prebiotics in Alzheimer's disease. Aging 12, 5539–5550. doi: 10.18632/aging.102930
Qi, X., Zhong, X., Xu, S., Zeng, B., Chen, J., Zang, G., et al. (2020). Extracellular matrix and oxidative phosphorylation: important role in the regulation of hypothalamic function by gut microbiota. Front. Genet. 11:520. doi: 10.3389/fgene.2020.00520
Resch, U., Tsatsaronis, J. A., Le Rhun, A., Stübiger, G., Rohde, M., Kasvandik, S., et al. (2016). A two-component regulatory system impacts extracellular membrane-derived vesicle production in group a Streptococcus. MBio 7, e00207–16. doi: 10.1128/mBio.00207-16
Rivera, J., Cordero, R. J., Nakouzi, A. S., Frases, S., Nicola, A., and Casadevall, A. (2010). Bacillus anthracis produces membrane-derived vesicles containing biologically active toxins. Proc. Natl. Acad. Sci. U. S. A. 107, 19002–19007. doi: 10.1073/pnas.1008843107
Sarkar, A., Lehto, S. M., Harty, S., Dinan, T. G., Cryan, J. F., and Burnet, P. W. J. (2016). Psychobiotics and the manipulation of Bacteria-gut-brain signals. Trends Neurosci. 39, 763–781. doi: 10.1016/j.tins.2016.09.002
Schaar, V., Uddbäck, I., Nordström, T., and Riesbeck, K. (2014). Group a streptococci are protected from amoxicillin-mediated killing by vesicles containing β-lactamase derived from Haemophilus influenzae. J. Antimicrob. Chemother. 69, 117–120. doi: 10.1093/jac/dkt307
Schwechheimer, C., and Kuehn, M. J. (2015). Outer-membrane vesicles from gram-negative bacteria: biogenesis and functions. Nat. Rev. Microbiol. 13, 605–619. doi: 10.1038/nrmicro3525
Shen, Y., Giardino Torchia, M. L., Lawson, G. W., Karp, C. L., Ashwell, J. D., and Mazmanian, S. K. (2012). Outer membrane vesicles of a human commensal mediate immune regulation and disease protection. Cell Host Microbe 12, 509–520. doi: 10.1016/j.chom.2012.08.004
Shi, L. Z., Fu, T., Guan, B., Chen, J., Blando, J. M., Allison, J. P., et al. (2016). Interdependent IL-7 and IFN-γ signalling in T-cell controls tumour eradication by combined α-CTLA-4+α-PD-1 therapy. Nat. Commun. 7:12335. doi: 10.1038/ncomms12335
Srivastava, P., and Kim, K. S. (2022). Membrane vesicles derived from gut microbiota and probiotics: cutting-edge therapeutic approaches for multidrug-resistant superbugs linked to neurological anomalies. Pharmaceutics 14:12370. doi: 10.3390/pharmaceutics14112370
Stentz, R., Horn, N., Cross, K., Salt, L., Brearley, C., Livermore, D. M., et al. (2015). Cephalosporinases associated with outer membrane vesicles released by Bacteroides spp. protect gut pathogens and commensals against β-lactam antibiotics. J. Antimicrob. Chemother. 70, 701–709. doi: 10.1093/jac/dku466
Stevenson, T. C., Cywes-Bentley, C., Moeller, T. D., Weyant, K. B., Putnam, D., Chang, Y. F., et al. (2018). Immunization with outer membrane vesicles displaying conserved surface polysaccharide antigen elicits broadly antimicrobial antibodies. Proc. Natl. Acad. Sci. U. S. A. 115, E3106–e3115. doi: 10.1073/pnas.1718341115
Sultan, S., Mottawea, W., Yeo, J., and Hammami, R. (2021). Gut microbiota extracellular vesicles as signaling molecules mediating host-microbiota communications. Int. J. Mol. Sci. 22:13166. doi: 10.3390/ijms222313166
Sundaram, K., Mu, J., Kumar, A., Behera, J., Lei, C., Sriwastva, M. K., et al. (2022). Garlic exosome-like nanoparticles reverse high-fat diet induced obesity via the gut/brain axis. Theranostics 12, 1220–1246. doi: 10.7150/thno.65427
Surve, M. V., Anil, A., Kamath, K. G., Bhutda, S., Sthanam, L. K., Pradhan, A., et al. (2016). Membrane vesicles of group B Streptococcus disrupt Feto-maternal barrier leading to preterm birth. PLoS Pathog. 12:e1005816. doi: 10.1371/journal.ppat.1005816
Tashiro, Y., Inagaki, A., Shimizu, M., Ichikawa, S., Takaya, N., Nakajima-Kambe, T., et al. (2011). Characterization of phospholipids in membrane vesicles derived from Pseudomonas aeruginosa. Biosci. Biotechnol. Biochem. 75, 605–607. doi: 10.1271/bbb.100754
Tiberti, N., Latham, S. L., Bush, S., Cohen, A., Opoka, R. O., John, C. C., et al. (2016). Exploring experimental cerebral malaria pathogenesis through the characterisation of host-derived plasma microparticle protein content. Sci. Rep. 6:37871. doi: 10.1038/srep37871
Tolosa, E., Garrido, A., Scholz, S. W., and Poewe, W. (2021). Challenges in the diagnosis of Parkinson's disease. Lancet Neurol. 20, 385–397. doi: 10.1016/S1474-4422(21)00030-2
Toyofuku, M., Nomura, N., and Eberl, L. (2019). Types and origins of bacterial membrane vesicles. Nat. Rev. Microbiol. 17, 13–24. doi: 10.1038/s41579-018-0112-2
Valdearcos, M., Douglass, J. D., Robblee, M. M., Dorfman, M. D., Stifler, D. R., Bennett, M. L., et al. (2017). Microglial inflammatory signaling orchestrates the hypothalamic immune response to dietary excess and mediates obesity susceptibility. Cell Metab. 26, 185–197.e3. doi: 10.1016/j.cmet.2017.05.015
Vallejo, M. C., Nakayasu, E. S., Longo, L. V., Ganiko, L., Lopes, F. G., Matsuo, A. L., et al. (2012). Lipidomic analysis of extracellular vesicles from the pathogenic phase of Paracoccidioides brasiliensis. PLoS One 7:e39463. doi: 10.1371/journal.pone.0039463
Van Niel, G., D'angelo, G., and Raposo, G. (2018). Shedding light on the cell biology of extracellular vesicles. Nat. Rev. Mol. Cell Biol. 19, 213–228. doi: 10.1038/nrm.2017.125
Volgers, C., Savelkoul, P. H. M., and Stassen, F. R. M. (2018). Gram-negative bacterial membrane vesicle release in response to the host-environment: different threats, same trick? Crit. Rev. Microbiol. 44, 258–273. doi: 10.1080/1040841X.2017.1353949
Wang, L., Zhao, Z., Zhao, L., Zhao, Y., Yang, G., Wang, C., et al. (2022). Lactobacillus plantarum DP189 reduces α-SYN aggravation in MPTP-induced Parkinson's disease mice via regulating oxidative damage, inflammation, and gut microbiota disorder. J. Agric. Food Chem. 70, 1163–1173. doi: 10.1021/acs.jafc.1c07711
Wei, S., Peng, W., Mai, Y., Li, K., Wei, W., Hu, L., et al. (2020). Outer membrane vesicles enhance tau phosphorylation and contribute to cognitive impairment. J. Cell. Physiol. 235, 4843–4855. doi: 10.1002/jcp.29362
West, C. L., Stanisz, A. M., Mao, Y. K., Champagne-Jorgensen, K., Bienenstock, J., and Kunze, W. A. (2020). Microvesicles from Lactobacillus reuteri (DSM-17938) completely reproduce modulation of gut motility by bacteria in mice. PLoS One 15:e0225481. doi: 10.1371/journal.pone.0225481
Xu, X., Zhang, H., Li, J., Chen, Y., Zhong, W., Chen, Y., et al. (2023). Combination of EPC-EXs and NPC-EXs with miR-126 and miR-210 overexpression produces better therapeutic effects on ischemic stroke by protecting neurons through the Nox2/ROS and BDNF/TrkB pathways. Exp. Neurol. 359:114235. doi: 10.1016/j.expneurol.2022.114235
Yaghoubfar, R., Behrouzi, A., Ashrafian, F., Shahryari, A., Moradi, H. R., Choopani, S., et al. (2020). Modulation of serotonin signaling/metabolism by Akkermansia muciniphila and its extracellular vesicles through the gut-brain axis in mice. Sci. Rep. 10:22119. doi: 10.1038/s41598-020-79171-8
Yang, Z., Gao, Z., Yang, Z., Zhang, Y., Chen, H., Yang, X., et al. (2022). Lactobacillus plantarum-derived extracellular vesicles protect against ischemic brain injury via the microRNA-101a-3p/c-Fos/TGF-β axis. Pharmacol. Res. 182:106332. doi: 10.1016/j.phrs.2022.106332
Yuyama, K., Sun, H., Usuki, S., Sakai, S., Hanamatsu, H., Mioka, T., et al. (2015). A potential function for neuronal exosomes: sequestering intracerebral amyloid-β peptide. FEBS Lett. 589, 84–88. doi: 10.1016/j.febslet.2014.11.027
Zakharzhevskaya, N. B., Vanyushkina, A. A., Altukhov, I. A., Shavarda, A. L., Butenko, I. O., Rakitina, D. V., et al. (2017). Outer membrane vesicles secreted by pathogenic and nonpathogenic Bacteroides fragilis represent different metabolic activities. Sci. Rep. 7:5008. doi: 10.1038/s41598-017-05264-6
Keywords: gut-brain axis, gut bacteria, microbiota, extracellular vesicle, neurological disorder
Citation: Sun B, Sawant H, Borthakur A and Bihl JC (2023) Emerging therapeutic role of gut microbial extracellular vesicles in neurological disorders. Front. Neurosci. 17:1241418. doi: 10.3389/fnins.2023.1241418
Edited by:
Daniele Lana, University of Florence, ItalyReviewed by:
Omkar Indari, St. Jude Children's Research Hospital, United StatesShweta Jakhmola, University of California, San Diego, United States
Copyright © 2023 Sun, Sawant, Borthakur and Bihl. This is an open-access article distributed under the terms of the Creative Commons Attribution License (CC BY). The use, distribution or reproduction in other forums is permitted, provided the original author(s) and the copyright owner(s) are credited and that the original publication in this journal is cited, in accordance with accepted academic practice. No use, distribution or reproduction is permitted which does not comply with these terms.
*Correspondence: Alip Borthakur, borthakur@marshall.edu; Ji Chen Bihl, bihlj@marshall.edu
†These authors have contributed equally to this work