- 1Institute for Nanobiotechnology, Johns Hopkins University, Baltimore, MD, United States
- 2Department of Biomedical Engineering, Johns Hopkins University, Baltimore, MD, United States
- 3Department of Chemical and Biomolecular Engineering, Johns Hopkins University, Baltimore, MD, United States
- 4Department of Materials Science and Engineering, Johns Hopkins University, Baltimore, MD, United States
The blood-brain barrier (BBB) is located at the interface between the vascular system and the brain parenchyma, and is responsible for communication with systemic circulation and peripheral tissues. During life, the BBB can be subjected to a wide range of perturbations or stresses that may be endogenous or exogenous, pathological or therapeutic, or intended or unintended. The risk factors for many diseases of the brain are multifactorial and involve perturbations that may occur simultaneously (e.g., two-hit model for Alzheimer’s disease) and result in different outcomes. Therefore, it is important to understand the influence of individual perturbations on BBB function in isolation. Here we review the effects of eight perturbations: mechanical forces, temperature, electromagnetic radiation, hypoxia, endogenous factors, exogenous factors, chemical factors, and pathogens. While some perturbations may result in acute or chronic BBB disruption, many are also exploited for diagnostic or therapeutic purposes. The resultant outcome on BBB function depends on the dose (or magnitude) and duration of the perturbation. Homeostasis may be restored by self-repair, for example, via processes such as proliferation of affected cells or angiogenesis to create new vasculature. Transient or sustained BBB dysfunction may result in acute or pathological symptoms, for example, microhemorrhages or hypoperfusion. In more extreme cases, perturbations may lead to cytotoxicity and cell death, for example, through exposure to cytotoxic plaques.
1. Introduction
It is increasingly evident that blood-brain barrier (BBB) health is important in promoting brain health, and that BBB dysfunction is a biomarker for various brain pathologies. BBB dysfunction is usually considered in the context of disease, however, during life, the BBB can be subjected to a wide range of perturbations or stresses (Figure 1). These perturbations may be endogenous or exogenous, pathological or therapeutic, or intended or unintended. The resultant outcomes are dependent on the dose and duration, and the combinatorial or cumulative effects of multiple perturbations. To establish the relationships between BBB health and brain health, it is essential to understand how different perturbations influence outcomes. Here we review the effects of eight perturbations on the BBB: mechanical forces, temperature, electromagnetic radiation, hypoxia, endogenous factors, exogenous factors, biochemical factors, and pathogens.
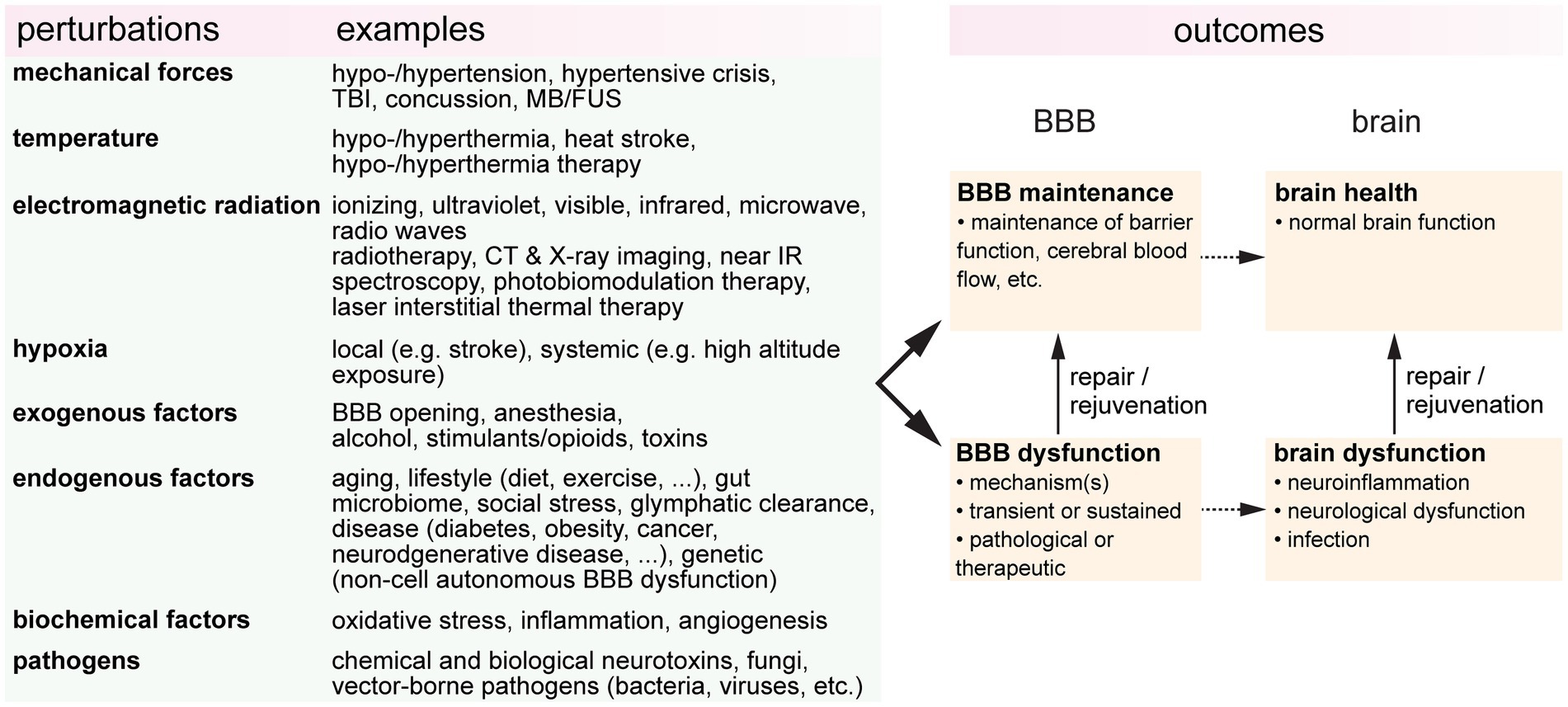
Figure 1. A wide range of perturbations can modulate blood-brain barrier (BBB function). These perturbations may be transient or sustained, endogenous or exogenous, pathological or therapeutic, or intended or unintended. TBI, traumatic brain injury; FUS, focused ultrasound; CT, computerized tomography; IR, infrared; BBBO, blood-brain barrier opening.
1.1. The blood-brain barrier
The neurovascular unit (NVU) is the functional building block of the blood-brain barrier (BBB) and includes brain microvascular endothelial cells (BMECs), supporting cells (e.g., pericytes and astrocytes), and the endothelial and parenchymal basement membranes (BMs) (Figure 2). Deletion/knock-out and functional studies have been used to infer the role of specific genes on BBB function, providing an expanding knowledge base of NVU components along the arterio-venous axis (Sweeney et al., 2019). The highly specialized BMECs provide the physical barrier between circulation and the brain. Recent transcriptomic atlases for mouse and human brain have highlighted differences in gene expression of cells in the NVU along the arterio-venous axis (Vanlandewijck et al., 2018; Garcia et al., 2022; Jeong et al., 2022; Yang A. C. et al., 2022; Crouch et al., 2023; Sun et al., 2023). For details of BBB structure readers are referred to these and other reviews.
The formation of tight junctions between BMECs effectively blocks paracellular transport of larger molecules and hence passage into and out of the brain relies on a variety of transport systems (Table 1). Passive transport is limited to small lipophilic molecules and gasses (O2 and CO2). Carrier mediated transport utilizes solute carriers (SLCs) for transport of nutrients and other essential molecules to the brain. Efflux pumps (e.g., ATP binding cassette (ABCs)) return many of the small lipophilic molecules capable of passive diffusion across the luminal membrane back into circulation (Loscher and Potschka, 2005). Vesicular trafficking via receptor mediated transport or adsorption mediated transport regulates transport of proteins and peptides into and out of the brain. Receptor-mediated transport involves ligand binding, most commonly via clathrin-mediated endocytosis. Adsorption mediated transport is initiated by electrostatic interactions between a ligand and the glycocalyx and usually involves caveolae-mediated transcytosis. Non-specific vesicular trafficking is generally suppressed in the healthy brain. Ion transporters are also important for regulating electrolyte balance. As a result of the high energy demand for maintenance of these systems, BMECs have more mitochondria compared ECs in other tissues (Oldendorf et al., 1977). For details of BBB structure in health and disease, readers are referred to recent reviews (Wong et al., 2013; Sweeney et al., 2018, 2019; Banks et al., 2021; Schaeffer and Iadecola, 2021).
1.2. BBB dysfunction
BBB dysfunction can include any gain or loss of function that has the potential to induce pathological changes in the brain. In general, BBB dysfunction can be broadly classified as: (1) processes that directly or indirectly increase paracellular transport of small molecules, macromolecules, or cells, (2) processes that result in dysfunction of transcellular transport, and (3) perturbations that result in disruption of normal signaling between BMECs and supporting cells, or in the secretion of soluble factors that can modulate the brain microenvironment (Table 1). Increased paracellular transport can result in entry of blood components, including hormones, proteins, and immune cells, into the brain and is sometimes referred to as “leaky brain” by analogy to leaky gut syndrome (Martinez-Ramirez et al., 2014; Haller et al., 2018). While increased paracellular transport is associated with loss of tight junctions, the rate of transport into the brain is also dependent on the glycocalyx and the basement membranes (Kutuzov et al., 2018). Overall, dysfunction can lead to changes in paracellular permeability, transport of nutrients and other essential molecules, immune cell transport, trafficking of pathogens into the brain, and loss of BMECs and/or supporting cells, which ultimately result in neuroinflammation, oxidative stress, and neurotoxicity (Hawkins and Davis, 2005; Engelhardt, 2008a,b; Abbott et al., 2010; Neuwelt et al., 2011). In general perturbations may be initiated from systemic circulation (e.g., endogenous or exogenous blood components), the brain parenchyma (e.g., neuroinflammation, toxic plaques, trauma, etc.), or intrinsically (e.g., mutations acquired by BMECs or supporting cells).
2. Mechanical forces
BMECs are constantly exposed to mechanical stimuli under physiological conditions. The hydrostatic pressure generated by the heart in pumping blood throughout the circulatory system exerts circumferential (hoop) and axial stresses on the endothelium, and blood flow results in a wall shear stress (Campinho et al., 2020). Furthermore, BMECs in arterioles and, to a lesser extent in capillaries, are exposed to cyclic strain due to pulsatile blood flow. BMECs can sense and transduce these mechanical cues resulting in a range of cellular responses.
2.1. Hypo−/hypertension
The brain is one of the most energy intensive organs in the human body; consuming 15–20% of oxygen in circulation (Bogorad et al., 2019). Blood supply to the brain is driven by the cerebral perfusion pressure (CPP), the mean arterial pressure (MAP), and the intercranial pressure (ICP, typically ~10 mm Hg). Due to the rigidity of the skull, there is a relatively low tolerance for changes in volume induced by changes in the volume of blood or cerebrospinal fluid (Monro-Kellie hypothesis). Cerebral blood flow (CBF) autoregulation is the process by which the brain regulates changes in cerebral blood flow and hence changes in blood volume that could increase ICP. Autoregulation maintains a relatively constant blood flow (~50 mL/min/100 g of brain tissue) over a range of CPPs from about 50–150 mm Hg (Figure 3A; Lassen, 1959; Claassen et al., 2021). However, transient changes in blood pressure (milliseconds to seconds) over this range can lead to fluctuations in CBF, where reductions in CBF of more than 30–60% (the reserve capacity) result in ischemic conditions (Baron, 2001; Lidington et al., 2021).
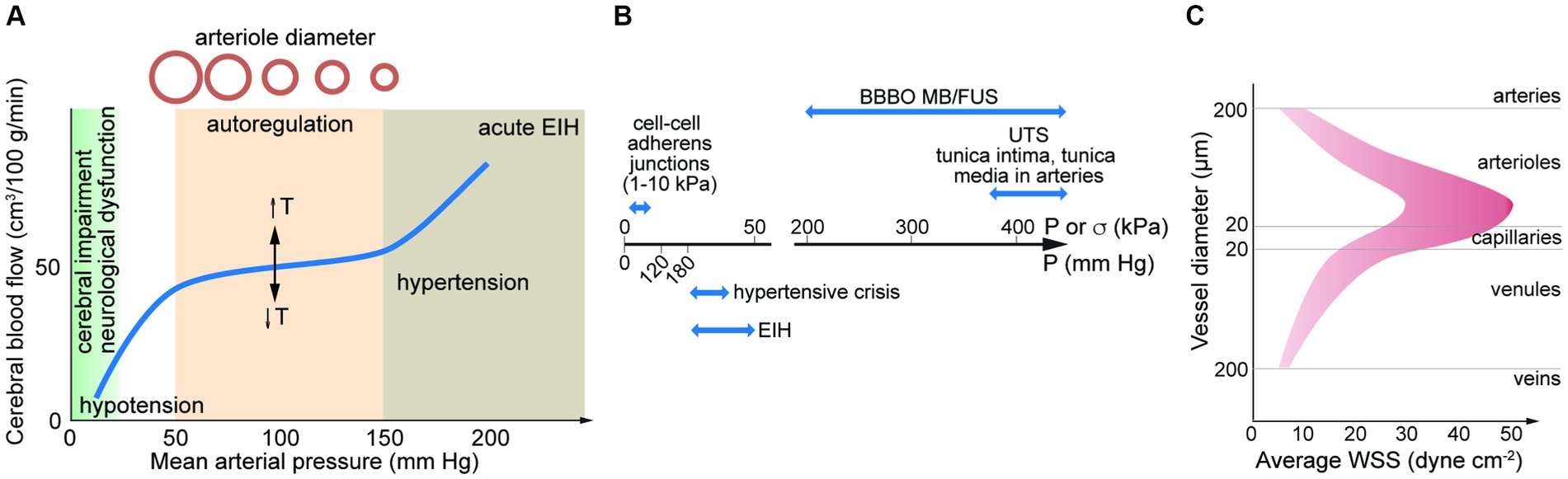
Figure 3. (A) Autoregulation results in a relatively constant cerebral blood flow over a range of mean arterial pressures (MAPs). The set-point is dependent on temperature (T). MAP can be estimated from MAP = DP + 1/3(SP – DP). SP, systolic pressure; DP, diastolic pressure. Elevated blood pressure (BP) and hypertension is defined by a BP > 180 mm Hg systolic and/or 120 mm Hg diastolic. Exercise induced hypertension (EIH) can lead to transient increases in systolic BP higher than 300 mm Hg. (B) Pressure associated with hypertensive crisis and EIH, and ultimate tensile stress for adherens junctions and arteries. Also shown is the force generated in MB/FUS. (C) Average wall shear stress along the arterio-venous axis. Data from direct measurements of flow rate and vessel diameter in the retina. UTS, ultimate tensile strength; BBBO, blood-brain barrier opening; EIH, exercise induced hypertension; MB/FUS, focused ultrasound with microbubbles.
Normal blood pressure (BP) is defined as 120/80 mm Hg (systolic/diastolic) (Flack and Adekola, 2020), although measured values vary with many factors including circadian clock, season, age, etc. (Schutte et al., 2022). Hypotension is usually defined as a BP < 90/60 mm Hg and can be caused by various factors including blood loss, dehydration, coronary artery disease, and medication. In the brain, hypotension can become dangerous if the CPP is too low to provide sufficient oxygen and glucose. Orthostatic hypotension is a reduction in BP on rising from a supine position, and is normally compensated by the autonomic nervous system. Orthostatic hypotension is characterized by a drop in BP of ≥20 mm Hg systolic and ≥ 10 mm Hg diastolic within 3 min of standing up (Joseph et al., 2017).
Increases in CPP above the range for autoregulation can result in increased ICP and increased mechanical forces on the cells of the NVU. According to current American Heart Association guidelines, elevated blood pressure is defined as 120–129 mm Hg systolic and < 80 mm Hg diastolic, stage 1 hypertension as 130–139 mm Hg systolic and/or 80–89 mm Hg diastolic, and stage 2 hypertension as >140 mm Hg systolic and/or > 90 mm Hg diastolic (Flack and Adekola, 2020). Hypertensive crisis is defined by a BP > 180 mm Hg systolic and/or 120 mm Hg diastolic (Whelton et al., 2018). Systolic BP normally increases monotonically with exercise intensity due to increased cardiac output, however, in some individuals may exhibit a hypertensive response to exercise (HRE) (Shilton et al., 2017; Moore et al., 2021). Exercise-induced hypertension (EIH) is defined as a resting BP of <140/90 mm Hg and a maximal exercise systolic BP of >210 mm Hg for males, or > 190 mm Hg for females (Schultz et al., 2013). A study of 5 power lifters reported an average peak BP of 320/250 mm Hg (MacDougall et al., 1985). However, there is no evidence for BBB dysfunction in EIH (De Silva et al., 2016).
Acute hypertensive crisis can occur under physiological (physical and psychological stress) (Kulkarni et al., 1998) and pathological conditions (e.g., TBI, seizures, and eclampsia) where autoregulation is disrupted (Schultz et al., 2013; Kim et al., 2020; Labrecque et al., 2021; Muacevic et al., 2021). Acute hypertension in healthy rats resulted in permeability to Evans blue/albumin and IgG within seconds upon increasing the MAP by ~60 mm Hg above baseline, and was reversible when the pressure returned to normal (Johansson and Martinsson, 1980; Kuang et al., 2004). Studies in animal models of acute hypertension have found BBB dysfunction occurs preferentially in post-capillary venules (De Silva et al., 2016). Studies in various animal models of chronic hypertension have found increased BBB permeability, oxidative stress, inflammation, cerebrovascular remodeling, and impairment of neurovascular coupling (Fredriksson et al., 1987; Ueno et al., 2004; De Silva et al., 2016; Setiadi et al., 2018). Increased BBB permeability has been reported to be zonation specific. For example, in a mouse model of angiotensin II hypertension, increased permeability of 3 kDa dextran was found in arterioles and venules >10 μm in diameter but not in capillaries (Santisteban et al., 2020). Some studies have suggested that the increased permeability is due to both TJ remodeling and transcytosis (Santisteban et al., 2020).
2.2. Focused ultrasound
Focused ultrasound has been explored for a wide range of purposes. High intensity focused ultrasound (HIFUS), with relatively long pulses (~ 1 s) at high acoustic pressures (typically 1 to 10 MPa) have been used for soft tissue ablation. HIFUS is used to ablate a wide range of solid tumors, including glioblastomas (Izadifar et al., 2020). In addition to destroying brain tissue, HIFUS can reversibly or irreversibly disrupt the BBB through disruption of cell–cell junctions (Mesiwala et al., 2002; McMahon et al., 2018). Very short pulses (~ 1 ms) at ≥20 MPa can induce cavitation, resulting in mechanical ablation of tissue.
Low energy pulsed focused ultrasound (pFUS) in conjunction with microbubbles (MBs) is used for reversible opening of the BBB. Energy from the acoustic waves induces stable (or non-inertial) cavitation which results in expansion and contraction of the MBs. These oscillations create local forces which can disrupt the endothelium, either forming pores in cell membranes or disrupting cell–cell junctions. The increase in permeability has been used in clinical trials to deliver therapeutics to treat diseases of the brain (Lipsman et al., 2018; Idbaih et al., 2019; Mainprize et al., 2019). The magnitude of the effect is dependent on ultrasound frequency, excitation pressure, and pulse duration (typically <1 ms). The acoustic pressure needed for BBB opening by pFUS/MB is typically in the range 100–500 kPa. This is approximately 10-fold higher than systolic blood pressures (120 mm Hg = 16 kPa) and the threshold for hypertensive crisis (180 mm Hg = 24 kPa) (Figure 3B).
The MBs are gas-filled, lipid coated microbubbles, similar to liposomes, and are typically 2–5 μm in diameter, slightly smaller than the diameter of capillaries in the brain (about 7–8 μm in humans) (Song et al., 2018). To enhance stability, MBs are filled with a high molecular weight gas with low aqueous solubility, such as perfluorocarbons. Under FUS excitation, the increase in MB diameter can be up to about 10-fold, depending on the diameter and the stiffness of the lipid shell and the viscosity of the fluid (Roovers et al., 2019). pFUS/MB results in transient pore formation in cell membranes and disruption of cell–cell junctions, both of which can result in changes in normal cell function, although these effects have not been widely studied.
In capillaries, BMECs exhibit a bamboo-like structure, and hence disruption of cell–cell junctions is more likely to occur when a bubble is close to one of the junctions. Consequently, the increase in permeability is thought to be associated with focal leaks that are randomly located in the region where the ultrasound is focused (Roovers et al., 2019). Dependent on the conditions, the defects at cell–cell junctions may allow entry of entities from small molecules, such as trypan blue, to large molecules, such as antibodies and plasmid vectors (Kinoshita et al., 2006; Kovacs et al., 2017; McMahon and Hynynen, 2017). Higher doses can result in entry of cells from circulation, as evidenced by local hemorrhage (McDannold et al., 2008; McMahon and Hynynen, 2017). Imaging of gadolinium leakage into the brain has shown that BBB opening occurs immediately after pFUS treatment (Hynynen et al., 2005) and persists for a few hours to a few days (Kovacs et al., 2017; Song et al., 2018) depending on the size of microbubbles. Studies have also shown that pFUS treatment can induce inflammation and immune responses that persist for at least 6 days post-sonication (Kovacs et al., 2017; McMahon and Hynynen, 2017). Whether pFUS-induced barrier changes and inflammation/immune responses act synergistically or antagonistically to treat brain diseases is not yet fully understood.
2.3. Traumatic brain injury and concussion
Traumatic Brain Injury (TBI) can be caused by direct impact, sudden or rapid acceleration and deceleration, penetrating injury, or blast injury. In animal TBI/concussion models, brain damage occurs with mechanical stresses from 100–500 kPa (Courtney and Courtney, 2007; Courtney and Courtney, 2015; Guley et al., 2016). In TBI, the impact of brain tissue on the inside of the skull can cause swelling, contusions, diffuse axonal injury, and disruption of blood vessels. The primary physical impact can cause significant displacement between different structures in the brain leading to shear, tensile, and compressive forces (Sahyouni et al., 2017), and resulting in structural damage to blood vessels and microhemorrhage. TBI and concussion can lead to disruption of the BBB via the primary physical injury as well as secondary responses such as inflammation and oxidative stress (Shlosberg et al., 2010; Sahyouni et al., 2017). In animal models of concussion/TBI, BBB disruption is typically biphasic with an early acute phase at 4–6 h and a delayed chronic phase starting 3–7 days after injury (Baskaya et al., 1997; Shlosberg et al., 2010; Xiong et al., 2013). Neovascularization is typically observed within 48 h after TBI and vascular remodeling is seen after 4 days (Prakash and Carmichael, 2015). Functional changes in transport have also been observed: in a rat TBI model, increased endothelial caveolae-mediated transcytosis was observed during the first 4 days following TBI with a subsequent decrease in expression of claudin-5 (Nag et al., 2009).
In humans, BBB opening associated with concussion can result in leakage of plasma proteins, such as fibrinogen and IgG (Johnson et al., 2018). MRI studies have found correlations between the amount of BBB leakage and serum levels of matrix metalloproteinase-7 (MMP-7) and S100B (O’Keeffe et al., 2020; Nichols et al., 2021). BBB leakage may persist for days to weeks, and long lasting effects of TBI include increased risk of neurodegenerative disease (Shlosberg et al., 2010; Prakash and Carmichael, 2015). BBB disruption is also a hallmark of Chronic Traumatic Encephalopathy (CTE), the consequence of repeated TBI, a brain trauma common in contact sports such as boxing and American football. Patients with CTE exhibit cerebral edema and enlarged perivascular spaces (Sweeney et al., 2018).
2.4. Wall shear stress
Blood flow results in a wall shear stress (WSS) on endothelial cells. WSS is dependent on the flow rate, blood viscosity, and vessel diameter. From Poiseuille’s law, the WSS () in straight sections of cylindrical vessels under laminar flow is given by:
where Q is the flow rate, is the blood viscosity (dependent on shear rate but typically 2–4 mPa·s), and d is the diameter of the vessel lumen.
Accurate data on flow rate in the human cerebrovasculature is limited. From measurements of vessel diameter and blood flow in microvessels in the human brain using MRI or in the human retina or conjunctiva using optical techniques, the WSS can be estimated from Poiseuille’s Law. While viscosity increases with increasing shear rate, we assume 2.2 mPa·s (cP). From these studies, the mean WSS is determined to be ~10–50 dyne cm−2 in arterioles (20–180 μm diameter), 10–30 dyne cm−2 in capillaries (9–19 μm diameter), and 5–20 dyne cm−2 in venules (30–300 μm diameter) (Figure 3C; Riva et al., 1985; Koutsiaris et al., 2007; Wang et al., 2007, 2009; Shahidi et al., 2010). A meta study of hemodynamics in the human common carotid artery reported a mean WSS of 11–13 dyne cm−2 (Reneman et al., 2006). These values should be considered approximate based on the limited applicability of Poiseuille’s Law. Average values of WSS in this range are important for maintenance of EC quiescence and NVU homeostasis. In arteries, values below this range along with disrupted flow patterns can lead to a range of pathological responses. High mean WSS (> 25 dyne cm−2) is less well studied, but is also considered to be pathological. From in vitro studies of monolayers in 2D, ECs undergo a morphological transformation from cobblestone to spindle-like under WSS of 5–20 dyne cm−2 (DeStefano et al., 2017).
2.5. Cellular level responses to mechanical stress
At the cellular level, the circumferential and axial stresses exert a tensile force on the cell–cell junctions. The ultimate tensile strength for cell–cell junctions between two isolated cells is in the range 1–10 kPa (Charras and Yap, 2018). However, the supporting cells, extracellular matrix (ECM), and basement membrane in tissues significantly increase the strength of the vessel wall. The ultimate tensile strength of the tunica intima and tunica media of large arteries is around 400 kPa, and for the tunica externa is >1,000 kPa (Camasao and Mantovani, 2021; Mishani et al., 2021). Changes in circumferential stress can arise from physical perturbations (e.g., acute/chronic high blood pressure, microbubble distortion in FUS/MB, TBI), or from exposure to biochemical perturbations that result in increased tensile stress on cell–cell junctions (e.g., osmosis) or cell loss from the lumen.
If the circumferential stress on the endothelium is sufficiently large, then junction disruption can lead to an increase in paracellular transport (Figure 4A). Since there is expected to be a range of TJ tensile strengths in a monolayer (Figure 4B), the weakest cell–cell junctions are more likely to be disrupted, leading to spatially localized focal leaks. In contrast, biochemical stress can result in a shift in the distribution of TJ strength that results in junction disruption at physiological pressures (Figure 4B). For example, a biochemical stress can cause a decrease in cell viability and hence loss of BMECs that is not immediately compensated by the remaining cells in the endothelium, resulting in local increases in paracellular permeability. If the decrease in viability is small, the endothelium may be repaired. In another example, biochemical stress (e.g., osmotic stress) may cause cells to shrink, resulting in both a decrease in cell viability and increased tensile stress on the cell–cell junctions. Indeed, chemical methods for reversible blood-brain barrier opening rely on the delicate balance between biological stress and recovery.
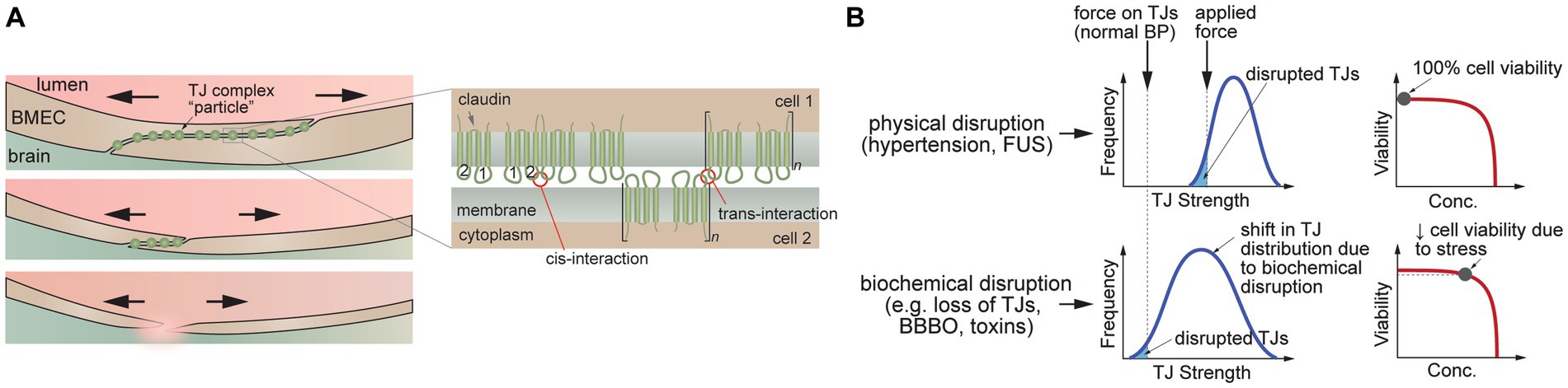
Figure 4. Disruption of tight junctions (TJs). (A) Schematic illustration of TJ disruption. Tensile forces result in disruption of the cell–cell junction and reduction in the number of TJs. (B) TJ disruption can be induced by tensile forces at cell–cell junctions (e.g., hypertension, FUS) or downregulation of TJ proteins leading to a decrease in the number of pinning points at the cell–cell junction (e.g., decrease in heterotypic interactions between claudin-5 extracellular domains). (C) Schematic illustration of trans-interactions between of claudin-5 extracellular domains that contribute to TJs.
3. Temperature
The average core body temperature (Tc) of healthy individuals is around 36°C (Mackowiak et al., 1992; Mackowiak and Worden, 1994; Sund-Levander and Grodzinsky, 2009; Protsiv et al., 2020). The average temperature of the brain is typically 1–2°C higher than Tc due to its high metabolic rate (Bain et al., 2015). Recent studies suggest that brain temperature varies with brain region and age, with temperatures as high as 40°C measured in the thalamus of healthy adults (Rzechorzek et al., 2022). The average brain temperature shows diurnal cycles, with the lowest temperature at night when CBF is highest, although these cycles are compromised with aging (Rzechorzek et al., 2022).
Common causes of elevated temperature include fever, passive heating, and exercise. Heat stress is usually defined by an increase in Tc up to 40°C (Figure 5; Bain et al., 2015), and heat stroke is associated with core temperatures above 40.5°C (Bouchama and Knochel, 2002). Heat stroke can cause symptoms including delirium, convulsions, and coma and has been defined as hyperthermia with a systemic inflammatory response leading to multi-organ failure and encephalopathy (Bouchama and Knochel, 2002). Heat stroke results in elevated systemic levels of the inflammatory cytokines TNF-α, and IL-1β along with IL-6 and IL-10 (Bouchama and Knochel, 2002).
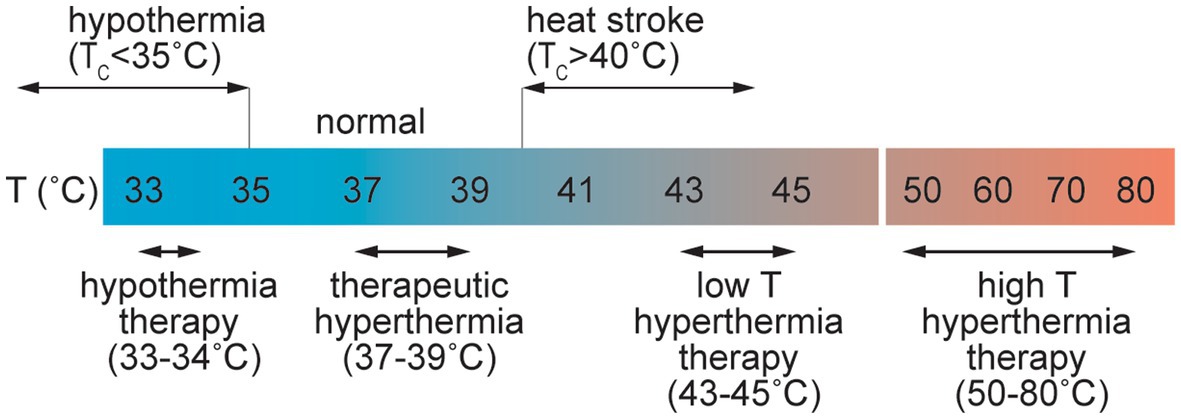
Figure 5. Schematic illustration of the range of perturbations in temperature associated with pathological and therapeutic conditions.
Core temperatures of 46–60°C are associated with irreversible cellular damage where the level of damage is often proportional to the exposure time. At the cellular level, in vitro studies have shown that cell death can occur at temperatures above 42°C. In many cases cell viability decreases approximately exponentially with time at a given temperature but is dependent on the cell type (Harmon et al., 1990; Thomsen, 1991; Dewhirst et al., 2003). Protein unfolding, which occurs at 50–90°C, also results in irreversible cell damage. Temperatures in excess of 100°C are associated with water vaporization, and temperatures greater than around 300°C result in tissue ablation and vaporization (Thomsen, 1991).
Therapeutic hyperthermia and hyperthermia therapy exploit systemic or local increases in temperature for treatment of various diseases. Therapeutic hyperthermia is usually associated with increases in Tc of 1–1.5°C. Small increases in temperature result in increased blood flow and is a potential tool for management and prevention of cardiovascular disease (CVD) (Tinken et al., 2009). However, higher temperatures can induce increased BBB permeability. In a rat model of whole body hyperthermia, young animals exposed to 38°C for 4 h showed BBB disruption and microhemorrhage (Sharma and Dey, 1986). Brain penetration of Evans blue/albumin was observed in regions of the cortex, cerebellum, hypothalamus, and thalamus, providing evidence for increased paracellular transport (Sharma and Dey, 1986). No disruption was observed following shorter exposure, and only mild disruption was found in adult mice demonstrating that both age and dose are important in the response to hyperthermia. BBB dysfunction was associated with increases in Tc of 3.51 ± 0.41°C. Exposure of rats to temperatures ≥39°C, or Tc values in excess of 42°C, frequently resulted in animal death (Sharma and Hoopes, 2003).
Various methods of local heating (laser, ultrasound, radio frequency, microwave) are used in clinical practice, usually for treatment of solid tumors (Thomsen, 1991; Dewhirst et al., 2003; Rieke and Butts Pauly, 2008). Hyperthermia therapy is usually associated with local induction of cell death and hence a local increase in BBB permeability is a consequence of endothelial cell loss. In the low temperature regime of hyperthermia therapy, local exposure to temperatures of 43–48°C for 10–60 min can induce cell death or sensitize cells to chemotherapy or radiation therapy. In the high temperature regime, short exposures to temperatures from 50–80°C induces tissue necrosis. Laser interstitial thermal therapy (LITT) is used for treatment of various types of brain tumors and epilepsy (Patel and Kim, 2020). Local heating in the brain can also result in increased permeability of the BBB, which has been exploited in enhanced drug delivery for treatment of brain tumors. In a monkey model, extravasation of Evans blue/albumin was observed in a region of the brain heated to 43°C after 60 min. Minimal leakage was observed 3 days after treatment (Nakagawa et al., 1994).
Hypothermia is associated with core body temperatures lower than 35°C. Nontherapeutic or accidental hypothermia is most commonly associated with trauma patients and is potentially fatal (Rosli et al., 2020). Therapeutic hypothermia therapy (THT) has been studied for treatment of various brain injuries including ischemic encephalopathy and stroke (Yenari and Hemmen, 2010; Kuczynski et al., 2020). THT for brain injury can be achieved through systemic cooling or selective cooling of the head by surface cooling or intra-arterial infusion of cold saline, and has been extensively studied for protection against acute ischemic stroke. Animal studies suggest that inducing temperatures of 32–34°C for 12–24 h can provide neuroprotection through increased BBB function, decreased inflammatory responses, reduced apoptosis, and decreased cerebral metabolism (Busto et al., 1987; Dumitrascu et al., 2016; Caroff et al., 2020; Kuczynski et al., 2020; Wu L. F. et al., 2020). Trials in humans have demonstrated selective cooling in the brain by inter-arterial perfusion of hypothermic saline at 4–17°C (Choi et al., 2020; Wu D. et al., 2020).
4. Electromagnetic radiation
To interact with brain tissue and impact BBB function, EM radiation must penetrate the skin, skull, and meninges. Damage to brain tissue is dependent on the wavelength and amount of energy absorbed, as well as the consequences of absorption in cells or other tissue components (e.g., heating, induced electronic transitions, generation of toxic species) (Figure 6). Damage to the BBB may be due to energy absorption in the cells of the BBB (heating) or a bystander effect from dysfunction induced in the surrounding brain parenchyma. Electromagnetic radiation includes ionizing (gamma ray and X-ray), ultraviolet, visible, infrared, microwave, and radio waves, spanning wavelengths from 1 pm (gamma rays) to 1 m (radio waves). These types of radiation are ubiquitous in daily life, for example solar irradiation (e.g., ultraviolet, visible and infrared) and communications (e.g., microwave or radio waves), and/or are used in specific applications, such as clinical diagnosis or therapy (e.g., infrared, gamma, or X-ray).
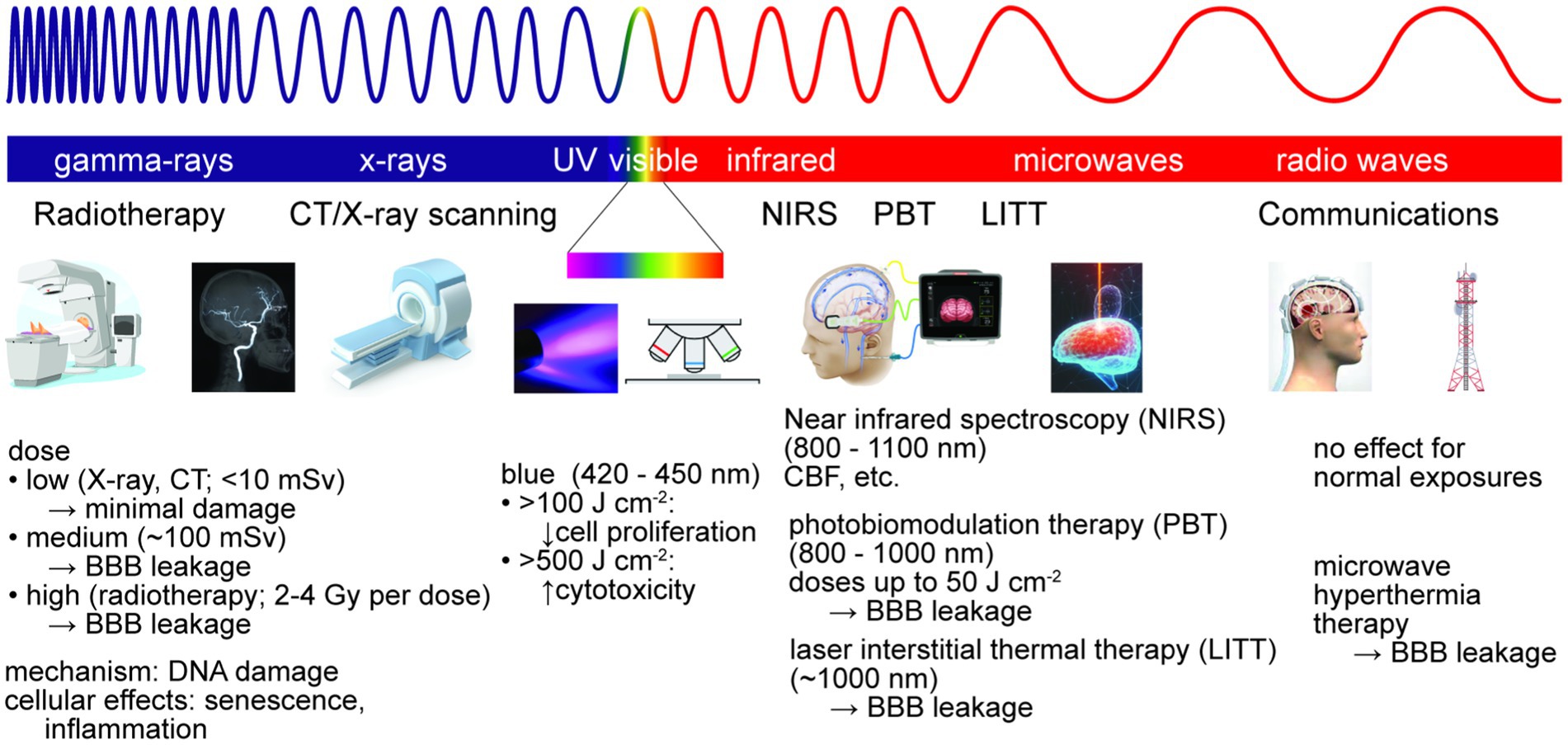
Figure 6. Electromagnetic radiation is used for brain imaging and therapy. Non-ionizing radiation is used for imaging, hyperthermia therapy, and photobiomodulation therapy (PBT). Ionizing radiation is used for imaging and radiation therapy, typically via DNA damage. At sufficiently high doses, exposure to ionizing or non-ionizing radiation can lead to reversible or irreversible BBB damage.
4.1. Ionizing radiation
The most likely sources of brain exposure to high levels of ionizing radiation are associated with clinical diagnosis or treatment (e.g., CT or X-ray scans, radiotherapy) (Lin, 2010). The SI unit for absorbed dose is the Gray (Gy), defined as energy (joules) per kilogram of tissue. The health effects of ionizing radiation are defined by the equivalent dose in sieverts (Sv), which also has units of J kg−1. A dose of 1 Sv represents a 5.5% risk of developing cancer, and an effective dose of 4–5 Sv in a short duration is considered to have a 50% risk of death within 30 days (LD50/30) (Huang et al., 2009). Doses for conventional X-ray scans vary from 0.01–1 millisieverts (mSv), whereas doses for CT scans vary from 2–10 mSv (Mettler et al., 2000; Wiest et al., 2002; Huang et al., 2009).
Whole brain radiation therapy (WBRT) is widely used to treat brain metastases, and primary brain tumors after resection (Borras et al., 2015). To minimize side effects in surrounding tissue, radiation therapy is usually administered in a series of small doses, or fractions, to improve outcomes. For example, the standard radiation therapy dose regimen for high grade gliomas is 60–65 Gy delivered in 1.8–2.0 Gy fractions over 6 weeks, and for cerebral metastases is 20–30 Gy in 3–4 Gy fractions over 2 weeks (RCR, 2019). Radiation therapy of a single metastasis may involve a single dose of 15–24 Gy.
Radiation-induced decline in cognitive function has been reported in patients who have received WBRT (Li et al., 2008; Welzel et al., 2008), as well as in animal models (Pena et al., 2000; Greene-Schloesser et al., 2012; Warrington et al., 2012, 2013; Sándor et al., 2014; Cekanaviciute et al., 2018). MRI studies of patients with either primary or metastatic brain tumors have found dose-dependent leakage of contrast agent into the brain immediately following radiation therapy (acute injury phase), indicating an increase in BBB permeability (Curnes et al., 1986; Cao et al., 2009). Although barrier function is usually restored post treatment, correlations have been found between BBB permeability and loss of cognitive function, indicating that BBB permeability may be a predictor of radiation-induced neurocognitive dysfunction (Cao et al., 2009). While transient BBB permeability suggests a route for delivery of drugs (e.g., chemotherapeutics), BBB leakage is inconsistent. For example, individuals with metastatic brain cancer, glioblastoma, and medulloblastoma display heterogeneous BBB permeability (Arvanitis et al., 2020). Triple negative and basal-type metastatic brain cancer often results in increased BBB permeability, whereas HER2-positive metastatic brain cancer is less likely to show increased BBB permeability (Yonemori et al., 2010). These differences are likely related to differences in tumor/vessel interactions in the local microenvironment (Silvestri et al., 2020).
The primary mechanism of tumor cell death in radiation therapy is via DNA damage. However, the effect on normal tissue is highly variable among patients. Several studies in animal models have shown a significant loss of spinal cord ECs and BMECs following irradiation (Ljubimova et al., 1991; Pena et al., 2000; Sándor et al., 2014). In addition, radiation doses as low as 100 mGy resulted in leakage of Evans blue/albumin into the brain in adult mice 1 week post-irradiation (Sándor et al., 2014). In vitro studies have found that irradiation of BMECs (2–8 Gy) induces cellular senescence which impairs the angiogenic response to hypoxic conditions (Ungvari et al., 2013). Based on these results, it has been proposed that ionizing radiation results in loss of BMECs leading to local tissue hypoxia and a decrease in vascular density, and that repair is inhibited by cellular senescence which impairs the angiogenic response to hypoxic conditions (Ungvari et al., 2013). This is similar to the vascular hypothesis for radiation damage in the brain, which posits that damage and loss of BMECs along with local release of inflammatory cytokines leads to necrosis and increased edema and microhemorrhage (Walker et al., 2014). Furthermore, ionizing radiation can induce an inflammatory response in BMECs, resulting in adhesion and transmigration of circulating immune cells, leading to loss of barrier function, as well as generation of reactive oxygen species (Olschowka et al., 1997; Sharp et al., 2003; Yuan et al., 2003; Lumniczky et al., 2017).
4.2. Space radiation
On Earth, humans are exposed to about 3 mSv of radiation a year from natural sources and the low level of cosmic rays that penetrate the atmosphere (Shea and Smart, 2000). In space, humans are exposed to cosmic ionizing radiation at levels substantially higher than on Earth (Nelson, 2016). For astronauts on the International Space Station, exposure is around 300 mSv per year, and exposure in interplanetary space can be more than 700 mSv per year (Cucinotta and Durante, 2006). In spaceflight, radiation exposure is coupled with microgravity, which is known to influence brain structure and function, resulting in increased intercranial pressure and altered cerebral blood flow. Therefore, the combined effects of radiation exposure and microgravity may be compounded. Despite the importance for human health in space, very little is known about the combined influence of microgravity and cosmic radiation on BBB function (Cekanaviciute et al., 2018).
4.3. Ultraviolet, visible, and infrared radiation
Humans are exposed to UV, visible, and infrared radiation during normal daily life. Levels of exposure to solar irradiation are not sufficient to penetrate the skull, and hence exposure is usually not an issue for brain health. The average solar irradiance at sea level is about 100 mW cm−2, corresponding to an energy deposition of 100 mJ cm−2 for a 1 s exposure (Solanki et al., 2013).
Studies in cadaver skulls and in vitro models of brain tissue have shown significant transcranial deposition of infrared energy at certain wavelengths in the range from 810–1,100 nm (Jagdeo et al., 2012; Cassano et al., 2019). Transcranial near infrared spectroscopy (NIRS) relies on the penetration of near IR radiation from an array of LEDs to detect changes in blood volume, blood oxygenation, and cerebral blood flow in cortical tissue (Chen W. L. et al., 2020). Due to the strong absorption in water and hemoglobin, the penetration depth is typically limited to about 2 cm. The LEDs commonly used for in NIRS typically have incident power densities of 10–20 mW cm−2 and use relatively short pulses (< 1 s).
Although the brain is not usually exposed to visible and ultraviolet radiation, absorption of visible light in tissues can generate heat or toxic molecules. Therefore, visible and UV radiation is a potential source of cell damage when imaging in vitro BBB models. Not surprisingly, most of our knowledge is based on exposure of skin cells to visible and ultraviolet irradiation. Exposure of human keratinocytes or dermal microvascular endothelial cells to red and infrared light (632–940 nm) had no effect on cell viability, whereas exposure to blue light (412–426 nm) at high fluences (66–100 J cm−2) or at 453 nm with very high fluences (>500 J cm−2) resulted in cytotoxicity (Liebmann et al., 2010). Exposure of endothelial cells or keratinocytes to blue light at nontoxic fluences resulted in a reduction of proliferation of up to 50% in a dose dependent manner (Liebmann et al., 2010).
4.4. Laser therapy
Brain photobiomodulation (PBM) therapy involves exposure to near-infrared (NIR) light (800–1,000 nm) (Salehpour et al., 2018). LEDs with power densities of 10–20 mW cm−2 are used to irradiate the brain for durations of minutes to hours, corresponding to doses of up to 50 J cm−2 (Hamblin, 2016). Near-IR radiation absorbed by the cytochrome c oxidase (COX) complex within mitochondria stimulates the electron transport chain which produces ATP. PBM can also produce NO and modulate ROS production. Studies have shown that PBM can increase gamma brain oscillations (Gonzalez-Lima and Barrett, 2014). Other regions of the brain, such as the prefrontal cortex, can absorb IR radiation via intranasal illumination (Pitzschke et al., 2015).
High energy laser irradiation can produce high temperatures in tissues, resulting in cell apoptosis and tissue necrosis. MRI-guided laser interstitial thermal therapy (LITT) is an emerging minimally invasive technique to treat primary and metastatic brain tumors and focal epilepsy (Holste and Orringer, 2020; Patel and Kim, 2020). A laser catheter is surgically guided into the tumor and wavelengths around 1,000 nm are used to generate local temperatures of 43–45°C. Guided by MRI or other imaging techniques, laser thermal therapy it can be used to kill cancer cells, but since the resolution is limited, can also induce disruption of the BBB resulting in cerebral edema and intracranial hemorrhage (Hawasli et al., 2014; Holste and Orringer, 2020). The main limitation of this method is the difficulty in controlling the temperature and avoiding adverse effects beyond the target zone. Studies in mouse models have shown that laser thermal therapy can induce solute extravasation or vessel occlusion dependent on the energy and doses (Nishimura et al., 2006). A clinical study of individuals with recurrent glioblastomas treated with LITT found that BBB permeability of MRI contrast agent peaked within 1–2 weeks after therapy and resolved within 4–6 weeks (Leuthardt et al., 2016). This increase in BBB permeability can be used to deliver drugs and other therapeutics alongside laser ablation of cancer cells, enabling combination therapy that may increase treatment efficacy (Patel et al., 2020; Patel and Kim, 2020; Lerner et al., 2022).
4.5. Microwaves and radio waves
Microwaves (300 MHz – 300 GHz) and radio waves (3 kHz – 300 MHz) are ubiquitous in communications and appliances, however, the energy of electromagnetic radiation in this frequency range is relatively low and cannot directly cause DNA damage. There is no conclusive evidence that normal daily exposures cause cancer or other pathologies, however, the effect of exposure in tissue is dependent on wavelength, power, and duration. The primary effect of tissue absorption of electromagnetic radiation in this frequency range is heating. At sufficiently high doses, microwaves and radio waves can induce tissue damage. For example, microwave hyperthermia therapy is used in the treatment of several cancers including brain cancer.
5. Hypoxia
Hypoxia is a condition in which oxygen demand in tissues or organs exceeds supply. At sea level, the partial pressure of oxygen (PO2) is about 160 mm Hg (Figure 7). Oxygen is supplied to organs via circulation, where the normal arterial oxygen partial pressure is in the range 75–100 mm Hg, decreasing to 30–40 mm Hg in veins (Ortiz-Prado et al., 2019). The average oxygen partial pressure in healthy human brain tissue is reported to be in the range 30–50 mm Hg (Carreau et al., 2011; Ortiz-Prado et al., 2019). However, tissue oxygen partial pressure in the cortex can increase to 140–350 mm Hg for subjects breathing 100% O2 (Meixensberger et al., 1993). Reduced O2 levels in the brain can occur due to decreased CBF or CPP, decreased partial pressure of oxygen in circulation (hypoxemia), decreased hemoglobin, increased metabolism, or local occlusion of vessels (e.g., ischemia).
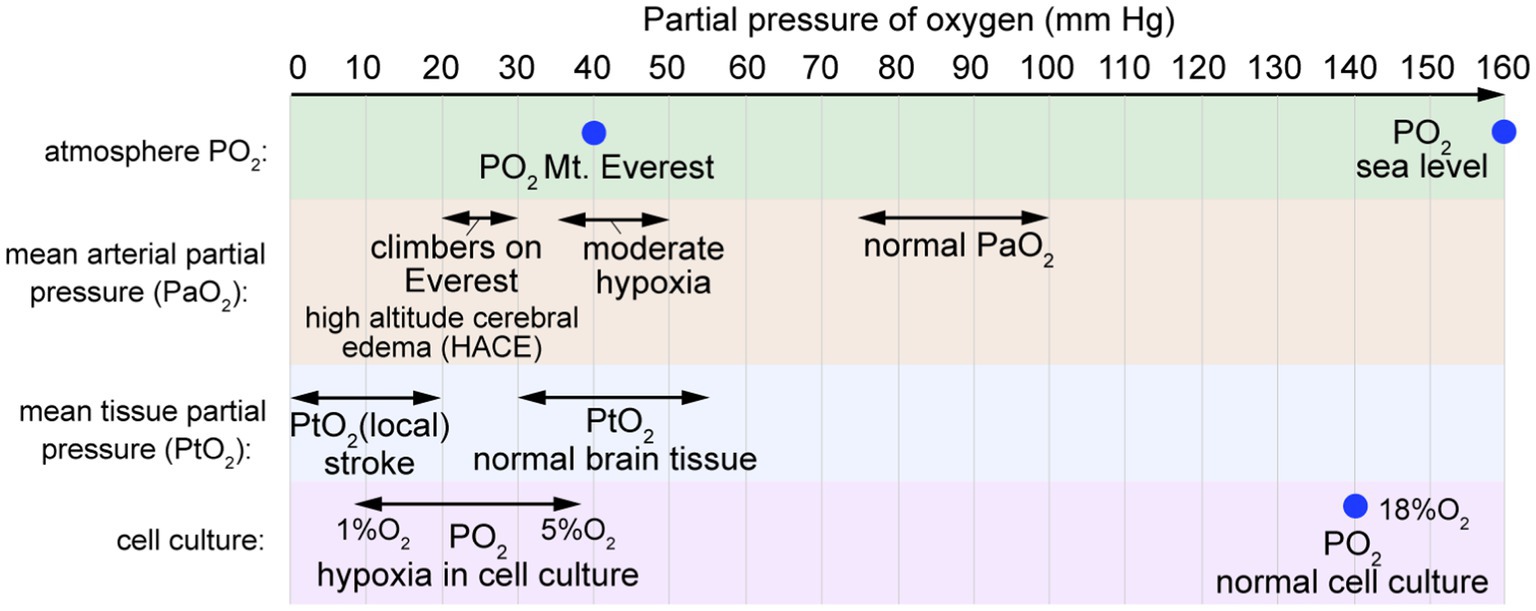
Figure 7. Partial pressure of oxygen. The mean arterial partial pressure of oxygen (PaO2) is typically 75–100 mm Hg. The mean arterial partial pressure of oxygen in brain tissue (PtO2) is typically 30–55 mm Hg. In normal cell culture, cells are maintained in humidified atmospheric air at 37°C with 5% CO2, resulting in PO2 values of 18–19% O2 (~140 mm Hg).
Following ischemic stroke, blood flow is reduced to a local region of the brain, usually due to an occlusion. Ischemia initiates a cascade of pathological events, including neuronal cell death, production of reactive oxygen species (ROS), metabolic changes, inflammation, BBB dysfunction, and neuronal cell death (Durukan and Tatlisumak, 2007; Krueger et al., 2015; Prakash and Carmichael, 2015). While the timing remains somewhat controversial, BBB dysfunction includes an increase in paracellular permeability by cell loss or loss of tight junctions, and/or an increase in transcellular transport by upregulating transcytosis (Plateel et al., 1997; Fischer et al., 2000). Claudin-5 and occludin have been shown to be degraded by MMP-9, which is highly expressed during ischemia (Bauer et al., 2010). Recent studies have found an increased prevalence of endocytic vesicles at early time points after ischemia, which suggests that transcellular transport may contribute to early BBB dysfunction (Haley and Lawrence, 2017).
In vitro studies of BMECs exposed to oxygen/glucose deprivation (OGD) have demonstrated phosphorylation and delocalization of TJ proteins, including claudin-5 and occludin. These changes appear to be downstream of hypoxia-inducible factor-1 (HIF-1) and may be mediated in part by increased secretion of VEGF (Engelhardt et al., 2014; Kokubu et al., 2017; Page et al., 2019). While astrocytes and pericytes appear to be more resilient than BMECs to OGD-induced apoptosis, these cells have been shown to respond to OGD by secreting MMPs (Lu et al., 2009) or by modulating BMEC barrier function through the secretion of growth factors, such as VEGF (Schmid-Brunclik et al., 2008), or cytokines, including IL-1β (Argaw et al., 2006). Astrocytes and pericytes also undergo structural changes following ischemic stroke, with swelling and detachment of astrocyte end-feet, and pericytes either migrating away from the endothelium, or constricting and preventing reperfusion (Liu et al., 2012).
While hypoxia is a key BBB stressor during ischemic stroke, it is difficult to distinguish BBB dysfunction caused by hypoxia from other stressors including glucose depletion, loss of shear stress, and the accumulation of waste products, each of which can independently contribute to BBB dysfunction (Pearigen et al., 1996).
At sea level, PO2 is approximately 160 mm Hg, whereas at the summit of Mount Everest (8,848 m), PO2 is 43 mm Hg (Figure 8). Arterial PO2 values of 20–30 mm Hg have been reported in climbers at 8,400 m on Mt. Everest (Grocott et al., 2009). Acute mountain sickness (AMS) affects up to 40 percent of hikers rapidly ascending to 3,000 m (9,800 ft) (Hackett and Roach, 2001), causing symptoms including headache, nausea, vomiting, fatigue, and sleep disruption (Baneke, 2010). After 3–5 days of acclimatization, cerebral blood flow returns to normal and AMS symptoms resolve. Severe cases can lead to high altitude cerebral edema (HACE) or high altitude pulmonary edema (HAPE), either of which can be life threatening. In severe AMS cases that progress to HACE, cytotoxic edema causes brain swelling, intracranial pressure increases further, and BBB permeability may increase (Baneke, 2010; Lawley et al., 2014; Davis and Hackett, 2017). Acute exposure to severely reduced PO2 results in loss of consciousness (e.g., depressurization of an aircraft), however, more gradual changes result in adaptations such as increased vascular density that can occur over several weeks (Bogorad et al., 2019).
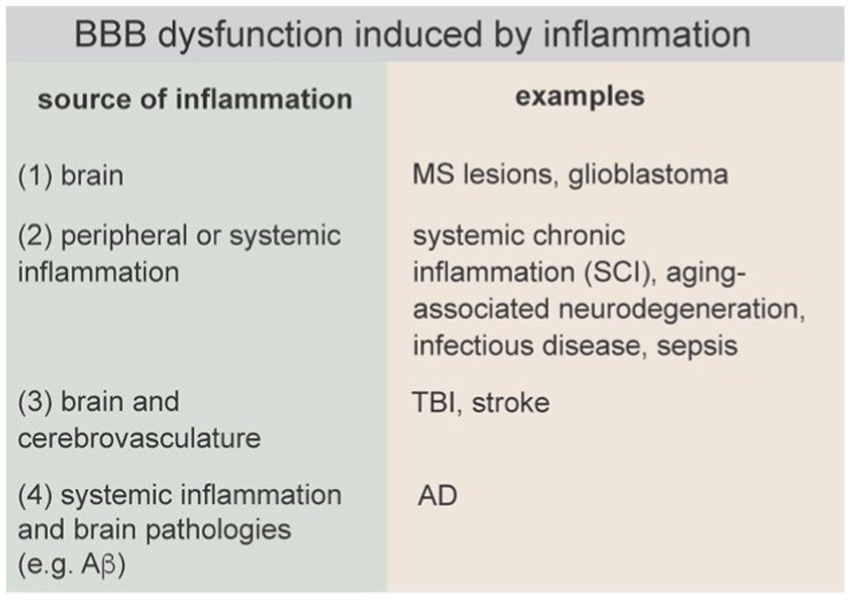
Figure 8. BBB dysfunction induced by inflammation can be initiated in the brain, by peripheral or systemic inflammation, simultaneously in the brain and cerebrovasculature, or systemically and amplified by brain pathologies.
6. Exogenous factors
Exogenous compounds that can induce BBB dysfunction include those administered to enhance drug delivery (e.g., hyperosmotic agents, vasoactive compounds, alkylglycerols (AKGs), sodium caprate, and membrane active peptides), those consumed recreationally (e.g., alcohol), and those associated with unintentional exposure (e.g., toxins) (Figure 1). These compounds display diverse mechanisms by which BBB dysfunction can occur following acute and/or chronic exposure.
6.1. Chemical blood-brain barrier opening
Hyperosmolarity causes a wide range of cellular changes including cell shrinkage, induction of apoptosis, DNA damage, and heat shock response (Alfieri and Petronini, 2007). Systemic intravenous (IV) administration of hyperosmotic agents, particularly mannitol, is widely used in the clinic to reduce cerebral edema. However, exposure to mannitol at higher concentrations can induce BBB opening (BBBO) (Rapoport et al., 1972, 1980; Rapoport, 2000; Linville et al., 2019). Since the threshold is near the solubility limit, BBBO is achieved by intra-arterial (IA) delivery (Rapoport et al., 1972, 1980; Rapoport, 2000). The mechanism of mannitol-induced BBBO is related to the increased tensile stress on cell–cell junctions induced by cell shrinkage and vasodilation (Rapoport et al., 1972, 1980; Rapoport, 2000). Recent work suggests that it is the weakest junctions that are susceptible to disruption, leading to transient focal leaks in the endothelium (Linville et al., 2019).
BBBO involves inducing sufficient cell stress to disrupt local cell–cell junctions but not the extent that the process is irreversible. Therefore, BBBO is a dynamic process with a spatio-temporal response that depends on dose and location in the brain. Increased paracellular transport is almost instantaneous after dosing (Chu et al., 2018), and hence therapeutics are commonly administered intra-arterially immediately following hyperosmotic agents as a bolus dose. Depending on administration conditions, barrier function can be restored within 5–10 min after administration (Rapoport et al., 1980; Houthoff et al., 1982). Osmotic BBBO has been used for delivery of chemotherapeutics, antibodies, nanoparticles, and gene vectors into the brain (Muldoon et al., 1995; Doolittle et al., 2000; Burkhardt et al., 2012; Lesniak et al., 2019), but has not achieved widespread clinical use in humans, at least partially due to challenges with reproducibility.
Various vasoactive compounds have been explored for transient BBBO, including bradykinin (an agonist for bradykinin receptor B2), bradykinin analogs (labradimil, retro-inverso bradykinin), adenosines (agonists for adenosine receptors) and adenosine analogs (e.g., regadenoson), endothelin-1 (an agonist for endothelin receptors A and B, ETA and ETB), C type natriuretic peptide (CNP) (an agonist for natriuretic peptide receptors B and C (NPR-B and NPR-C)), among others. Of these, bradykinin receptor is the most well-studied. Bradykinin and its synthetic analog (labradimil) are vasoactive peptides that activate β2 receptors constitutively expressed on BMECs (Borlongan and Emerich, 2003). Bradykinin is an endogenous peptide which at physiological concentrations does not disrupt the BBB. However, intracarotid injection of 4 μM labradimil induced leakage of fluorescein into the cortex in a cat model, but not 20 kDa dextran or albumin (Unterberg et al., 1984). Injection of the β-receptor agonist isoproterenol into the brain parenchyma reduced fluorescein leakage, confirming the role of receptor mediated uptake in inducing BBB leakage. EM analysis of vessels following intrajugular injection of labradimil in mice found significant tight junction penetration of lanthanum tracer but negligible changes in the number of vesicles or endocytic pits, supporting paracellular leakage due to disruption of tight junctions (Sanovich et al., 1995). In addition, in vitro studies have shown that activation of β2 receptors on rat BMECs causes calcium influx (Doctrow et al., 1994). IV administration of labradimil along with an analgesic in a rat model resulted in a two-fold increase in analgesic effect (Emerich et al., 1998). Subsequent studies of labradimil administration for transient BBBO and delivery of chemotherapeutics for treatment of brain cancer in animal models (Inamura and Black, 1994; Fike et al., 1998; Emerich et al., 1999, 2000, 2001) led to a clinical trial in humans (Cloughesy et al., 1999; Gregor et al., 1999; Prados et al., 2003). However, therapeutic effects have been inconsistent, resulting in less interest in vasoactive peptides as a means for BBBO.
Membrane active peptides (MAPs) are a new classes of compounds and have potential to induce BBBO (Sarkar et al., 2014; Meng et al., 2017; Aasen et al., 2019). Compared to hyperosmotic agents, which have low molecular weights, peptide-based strategies have customizable physiochemical properties (i.e., charge, hydrophobicity, amphipathicity) (Komin et al., 2017). For example, melittin, a MAP present in bee venom, induced reversible BBBO following intra-arterial injection in a mouse model (Linville et al., 2021).
6.2. Alcohol
Alcohol is completely miscible with water and has a moderate lipophilicity (logPoct ≈ −0.1) resulting in rapid passive diffusion across the BBB. Based on uptake studies in red blood cells, the permeability of ethanol is estimated to be around 10−3 cm s−1 (Brahm, 1982), much higher than most other small molecule drugs (Summerfield et al., 2007).
While early reports were inconclusive [summarized in Pan et al. (2008)], recent studies have found that chronic alcohol consumption causes BBB breakdown. Post-mortem brain tissue from individuals with alcoholism displays loss of basement membrane and tight junctions, increased MMP activity, and immune cell infiltration (Rubio-Araiz et al., 2017). In animal models of chronic alcohol consumption, leakage of blood components into the brain is observed, which may exacerbate prior neurodegeneration (Rubio-Araiz et al., 2017; Wei et al., 2019). In vitro studies suggest that ethanol-induced BBB dysfunction is mediated by oxidative damage to BMECs, causing loss of basement membrane, disrupted tight junctions, and increased immune cell infiltration (Haorah et al., 2005, 2008). Studies in mouse models of fetal alcohol spectrum disorders (FASD) suggest alcohol exposure can result in BBB dysfunction (Popova et al., 2023). Two photon imaging in the parietal lobe of 7–8 week male offspring revealed BBB leakage of 10 kDa and 70 kDa dextran in the FASD model but not in healthy controls (Tufa et al., 2017). Alcohol consumption can increase cerebral blood flow, even in low doses (Stendel et al., 2006), which may increase risk and worsen prognosis following hemorrhagic stroke (Liew et al., 2016). A side effect of chronic alcohol consumption is hyperammonemia, which results in increased levels of ammonia in circulation. Since the pKA for the NH4+/NH3 equilibrium is 9.24, the concentration of NH3 in blood is very low. However, NH3 can passively diffuse across the BBB, where the NH4+/NH3 equilibrium is reestablished, resulting in accumulation of NH4+ in the brain. NH4+ is toxic to neurons and blood concentrations greater than 60 μM can be clinically significant with increasing concentrations resulting in coma or death (Cohn and Roth, 2004).
6.3. Stimulants and opioids
Acute and chronic exposure to stimulants such as methamphetamines or cocaine can result in compromised BBB function (Kousik et al., 2012; Sajja et al., 2016). Methamphetamines induce hyperthermia, hypertension, brain edema, seizures, and BBB breakdown causing severe neurotoxicity (Bowyer and Ali, 2006; Bowyer et al., 2018). Extravasation of blood components into the brain parenchyma is attributed to severe oxidative damage to BMECs (Ramirez et al., 2009), which causes downregulation/loss of tight junctions (Ramirez et al., 2009; Martins et al., 2011) and MMP-9 mediated degradation of the basement membrane (Martins et al., 2011; Urrutia et al., 2013). This toxicity is strongly linked to brain/body temperature, with the BBB intact in the absence of hyperthermia (Bowyer and Ali, 2006). Upregulation of TNF-α, increased immune cell infiltration (which promotes HIV-1 neuroinvasion), downregulated glucose transporter activity, and altered efflux pump expression (Ramirez et al., 2009; Coelho-Santos et al., 2015; Sajja et al., 2016), and may further exacerbate BBB damage and neurotoxicity. Therapies which protect from BBB disruption include antioxidant treatment, TNF-α inhibition, and MMP inhibition (Ramirez et al., 2009; Martins et al., 2011; Urrutia et al., 2013; Coelho-Santos et al., 2015). Cocaine exposure results in BBB disruption through similar mechanisms (Kousik et al., 2012; Sajja et al., 2016). In a study of opioids, BBB dysfunction was associated with withdrawal rather than acute consumption (Zhu et al., 2023). In individuals with opioid use disorder, the withdrawal score was correlated with the concentration of fragile-like regulatory T cells (Tregs). In a mouse model, opioids caused BBB disruption via downregulation of astrocyte-derived fatty-acid-binding protein 7 (Fabp7), resulting in Treg transmigration into the nucleus accumbens (NAc) and ultimately promoting withdrawal symptoms.
6.4. Anesthesia
General anesthesia and surgery can induce cognitive impairments in animal models, with effects in humans still controversial (Vutskits and Xie, 2016). Multiple studies have linked anesthesia-induced cognitive impairments to BBB disruption (Tetrault et al., 2008; Yang et al., 2017; Cao et al., 2018). Interestingly, opening of the BBB from anesthesia exhibits hallmarks of osmotic disruption, including brain edema, leakage of blood components, and heterogeneity of opening across brain regions (Tetrault et al., 2008). In addition, due to the range of anesthetics and doses, different dynamics of BBBO are observed (Tetrault et al., 2008; Acharya et al., 2015; Yang et al., 2017; Cao et al., 2018). Isoflurane, a common inhaled anesthetic, is the most widely studied in relation to BBB damage and results in dose-dependent effects in animal models (Tetrault et al., 2008). Upregulation of inflammatory cytokines, angiogenic stimuli (VEGF and HIF-1α), and MMPs have been implicated in anesthesia-induced BBB disruption, along with downregulation of TJs which leads to increased paracellular permeability (Wu et al., 2012; Cao et al., 2015, 2018; Yang et al., 2017). Aged mice are particularly vulnerable to anesthesia-induced BBB disruption (Acharya et al., 2015; Yang et al., 2017), which has been linked to increased risk of neurodegeneration and dementia (Vutskits and Xie, 2016).
7. Endogenous factors
7.1. Microbiome and gut – brain axis
The gut – brain axis (GBA) enables bidirectional communication between the gastrointestinal tract and the brain either directly, via the vagus nerve, or indirectly, via soluble factors in circulation, which implicates the BBB (Parker et al., 2020; Tang et al., 2020). The gut microbiome transforms dietary components into a diverse range of molecules, including short chain fatty acids (SCFAs, e.g., butyrate, acetate, propionate), neurotransmitters, neuropeptides, and serotonin. Changes in the gut microbiome can result in dysfunction in pathways along the gut – brain axis, ultimately resulting in BBB dysfunction and neuroinflammation (secretion of TNF-α, INF-γ, IL-6). In a comparison of germ-free and pathogen-free mice, the permeability to Evans blue/albumin was higher in germ-free mice and was associated with a decrease in occludin and claudin-5 in BMECs (Braniste et al., 2014). Rescue studies found that colonization of germ-free mice with flora from pathogen-free mice resulted in decreased BBB permeability and upregulated the expression of tight junction proteins. Gavage with bacterial strains that produce butyrate, acetate, and propionate had a similar effect.
Changes in the gut microbiome can result in increased intestinal permeability (leaky gut syndrome), often due to factors such as diet, lifestyle, chronic stress, and antibiotic treatment. Leaky gut is associated with chronic systemic inflammation, and in the hygiene hypothesis, is one of several factors that contribute to chronic inflammatory diseases (Fasano, 2020).
7.2. Diet
With expanding knowledge of the gut-brain axis, diet is emerging as a key endogenous factor that influences brain and cerebrovascular health. Body mass index and obesity are correlated with risk of BBB disruption in humans (Gustafson et al., 2007). Several studies have found increased BBB permeability in the hippocampus in animal models in response to high fat or high cholesterol diets (Chen et al., 2008; Kanoski et al., 2010; de Aquino et al., 2018).
A wide array of dietary supplements have been investigated for their promotion of BBB health. Vitamin D, vitamin B (1, 12, 5, 9), Mg, and omega 3 fatty acids are thought to be neuroprotective and may directly or indirectly promote BBB health (Roy et al., 2022). Antioxidant or anti-inflammatory molecules, such as curcumin, resveratrol, sulforaphane, may have a similar effect. In patients with mild cognitive impairment, orally administered vitamin B12/B6 reduced the ratio of tau protein to albumin in CSF, implying improved barrier function (Lehmann et al., 2003). In an in vitro BBB model with a monolayer of primary BMECs in astrocyte conditioned media, Mg increased TEER and reduced permeability, and increased the transcytosis of amyloid beta (Aβ) (Zhu et al., 2018). In an in vitro multiple sclerosis (MS) model, vitamin D reduced the effects of TNF-α on BMEC barrier function (Takahashi et al., 2017). Studies in mice and humans have shown that omega-3 fatty acids reduce BBB disruption associated with aging and surgery-induced impairment (Barnes et al., 2021; Yang T. et al., 2022).
7.3. Aging
Aging is associated with accumulation of cell damage, including oxidative stress, epigenetic changes, dysregulation of cell signaling and inflammatory responses, and senescence. While healthy aging is not well defined, it can be considered as age-related changes that result in minimal decline in cognitive function (Menassa et al., 2023). MRI imaging studies suggest small but measurable increases in BBB permeability in healthy aging, although the magnitude varies considerably between individuals (Montagne et al., 2015). Transporters such as Glut-1, LAT-1, P-gp, and LRP-1 are also downregulated in aging. LRP-1 is important for brain-to-blood transport of Aβ peptide and hence its downregulation can lead to subsequent Aβ accumulation (Jaeger et al., 2009). Aβ is also a P-gp substrate, and hence downregulation of this efflux pump can also contribute to accumulation (Hartz et al., 2010). The downregulation of P-gp is also thought to increase sensitivity to CNS-active molecules that are efflux substrates (Banks et al., 2021). Transcriptomic studies in mice have found age related increases in the fraction of BMECs that express senescence-related genes (Kiss et al., 2020), and downregulation of the senescence regulator sirtuin-1 is associated with increased BBB permeability (Stamatovic et al., 2019).
Studies in animal models and post-mortem human tissue have shown a wide array of structural and functional changes during healthy aging. Changes include a decrease in microvascular density (~10–30% in the prefrontal cortex and hippocampus), a decrease in brain arteriole density (~40% in the cerebral cortex), thickening of the basement membrane, loss of SMCs, pericyte degeneration/loss, swelling of astrocytic end-feet, defects in tight junctions, increased vesicular transport, and increased permeability (Sonntag et al., 2007; Brown and Thore, 2011; Murugesan et al., 2012; Montagne et al., 2015; Erdo et al., 2017; Goodall et al., 2018; Reagan et al., 2018; Yang et al., 2020). Based on these observations, it is apparent that some level of BBB dysfunction can be tolerated with minimal changes in cognitive function. However, co-occurring pathologies, such as inflammation, can result in more advanced pathological symptoms. This has been demonstrated in mouse models, where BBB function is diminished in healthy aged mice, but cognitive decline is only apparent after an inflammation challenge (Wen et al., 2020). This is analogous to the “two-hit” vascular hypothesis for Alzheimer’s disease.
Aging is associated with changes in protein composition in circulation. Several studies have shown that infusion of plasma from young mice improves cognitive function in aged mice (Wyss-Coray, 2016). Transcriptomic studies in mice have shown significant differences in aged mice compared to young mice, with upregulation of pathways associated with innate immunity and oxidative stress response (Chen M. B. et al., 2020). These effects were most pronounced in capillaries compared to arterioles and venules. These changes were replicated in young mice 4 days after infusion of plasma from aged mice. Furthermore, infusion of plasma from young mice into aged mice resulted in rejuvenation on the transcriptome. In particular, Mfsd2a was downregulated in BMECs in aged mice, resulting in increased protein levels of caveolin-1 and implying increased non-specific vesicular transport.
7.4. Social stress
Studies in a male mouse model of social stress showed ultrastructural defects and decreased levels of claudin-5 in BMECs in capillaries in the nucleus accumbens compared to unstressed mice (Menard et al., 2017). The nucleus accumbens plays an important role in stress responses and mood disorders. In MRI imaging, increased levels of Gd contrast agent were observed in the nucleus accumbens and hippocampus of stressed mice. Treatment for 35 days with the antidepressant imipramine rescued these changes in the BBB. Conditional knockdown of CLDN5 in the nucleus accumbens did not induce depression behaviors but did induce depression following microdefeat at levels below the threshold to induce depression compared to mice without CLDN5 knockdown. Increased levels of IL-6 were observed in blood and the nucleus accumbens (but not hippocampus) of stressed mice. Reduced CLDN5 expression was also observed in post-mortem tissue of unmedicated individuals with major depressive disorder compared to healthy controls. These results provide evidence that social stress increases paracellular permeability in the nucleus accumbens resulting in accumulation of inflammatory cytokines (e.g., IL-6) and the development of depression-like behaviors (Menard et al., 2017). Similar experiments showed leaky BBB (10 kDa dextran) in the prefrontal cortex (but not nucleus accumbens) of stressed female mice (Dion-Albert et al., 2022). The pre-frontal cortex is responsible for executive function and decision making. Decreased claudin-5 was observed in microvessels of stressed mice compared to controls. Loss of claudin-5 was also observed in the pre-frontal cortex of post-mortem tissue from females who died by suicide compared to nonpsychiatric controls (Dion-Albert et al., 2022). In a panel of biomarkers for vascular health, soluble E-selectin was increased in stressed mice.
7.5. Glymphatic clearance
Fluid and solute exchange across the BBB is highly restricted and hence waste products and debris produced in the brain are: (1) transported across BMECs into circulation by passive diffusion (e.g., CO2), (2) effluxed across BMECs into circulation (e.g., soluble Ab, tau), (3) recycled by cells in the brain (e.g., microglia), or (4) transported out of the brain into the lymphatic system. In the glymphatic system model, removal of waste products into the lymphatic system occurs via fluid flow from the CSF along the perivascular spaces (PVS) around penetrating arteries (mediated by aquaporin-4 (AQP4) water channels in astrocyte end-feet), transport via interstitial flow (e.g., white matter tracts), efflux along the perivascular space around venules, and finally drainage into meningeal lymphatic vessels (Bohr et al., 2022). Two-photon microscopy studies with tracers have identified a fluid filled perivascular space around penetrating arterioles between the smooth muscle cells and astrocyte end-feet (Mestre et al., 2020). In post-mortem imaging the PVS collapses and hence the region between SMCs and astrocyte end-feet is only occupied by basement membrane. Whether there is a continuous PVS along the arterio-venous axis enabling a direct route for transport remains to be firmly established (Hannocks et al., 2018; Ferris, 2021).
While sleep and exercise promote efficient transport, perturbations that reduce the efficiency of the glymphatic system have the potential to induce BBB dysfunction. For example, reduction of the perivascular space can reduce glymphatic flow and hence result in accumulation of waste products or toxins (Bohr et al., 2022). It has been speculated that conditions such as hypertension and inflammation can impact the PVS and hence impair glymphatic clearance. For example, MRI imaging has shown that small vessel disease (SVD) is associated with microbleeds and enlarged PVS around arterioles which is thought to be due to downstream accumulation of waste products reducing flow (Brown et al., 2018). SVD is associated with accumulation of advanced glycation end products (AGEs) and activation of the corresponding receptor (RAGE). Accumulation of RAGE is associated with hypertension, aging, inflammation, and oxidative stress (Brown et al., 2018). Similarly, Since the glymphatic system plays a role, along with efflux pumps, in the removal of soluble Aβ, disruption of glymphatic clearance can lead to accumulation of Aβ plaques in the basement membrane.
8. Biochemical factors
8.1. Oxidative stress
Oxidative stress arises from an excess production of free radicals such as reactive oxygen species (ROS) and reactive nitrogen species (RNS). Free radicals are intermediates in cellular energy production and function as second messengers in various signaling pathways (Forman et al., 2004). Accumulation of free radicals, for example under hypoxic conditions, is counterbalanced by endogenous anti-oxidant mechanisms. However, under pathological conditions free radical production can overwhelm the antioxidant capacity resulting in accumulation of oxidized proteins, lipids, and nucleic acids. Free radicals and cellular oxidative stress can be triggered by an array of physical and biological processes including changes in metabolism with cell age, inflammation and immune responses, environmental factors such as smoking and air pollution, and exposure to UV or ionizing radiation (Golden et al., 2002; Auten and Davis, 2009; Al-Gubory, 2014; Carraro et al., 2018). For example, ROS and RNS are produced by phagocytic cells in response to infection. Secreted ROS and RNS can induce oxidative stress and tissue damage if not consumed by antioxidant mechanisms. The brain is particularly sensitive to oxidative stress due to its high metabolic demand, the involvement of ROS and other free radicals in some neuronal signaling pathways, ROS generation by activated microglia and astrocytes, and reduced antioxidant capacity compared to other tissues (Cobley et al., 2018). BMECs are susceptible to oxidative stress due to their high mitochondrial density (Cobley et al., 2018).
Oxidative stress is a key component in the etiology of many neurodegenerative pathologies, including Alzheimer’s disease (AD), MS, stroke, and TBI (Rodriguez-Rodriguez et al., 2014; Rao et al., 2018). Several mechanisms can promote downregulation of TJs, including ROS-induced cleavage of cysteine residues in claudin-5 and occludin, and the degradation of basement membrane through downstream production of MMPs (Rempe et al., 2016; van Leeuwen et al., 2020). These and other studies suggest a correlation between oxidative stress, loss of barrier function, and downstream neurodegeneration and disease. Since oxidative stress is a balance between production and antioxidant capacity, the impact on BBB dysfunction involves common intrinsic mechanisms including inflammation and angiogenesis. These pathways are activated across many diverse perturbations. As a result of the role of ROS in normal cell signaling, the impact of free radical production is highly dose-dependent. At low concentrations (< 1 μM), hydrogen peroxide (H2O2), a common ROS, promotes angiogenic activity in brain microvascular endothelial cells, while higher doses (> 100 μM) result in increased permeability of cell monolayers. Very high doses (> 10 mM) result in apoptosis (Anasooya Shaji et al., 2019; Chung et al., 2022).
While the generation of excess ROS in the brain represents a pathological perturbation on the BBB, various strategies have been explored for improving antioxidant capacity, particularly in stroke. Antioxidant therapies include inhibition of ROS producing enzymes, free radical scavengers (Tirilazad), increasing concentrations of endogenous free radical antioxidants (e.g., superoxide dismutase), and antioxidant supplementation (e.g., vitamin C, vitamin E, N-acetylcysteine (NAC), etc.).
8.2. Inflammation
An inflammatory response by the innate immune system involves interplay between the initial insult (infection or injury), the local tissue microenvironment, and the peripheral immune system. A successful inflammatory response results in elimination of pathogens and promotes tissue remodeling and repair. Neuroinflammation can be caused by a wide range of perturbations including pathogens, injury (e.g., TBI, stroke), endogenous factors (e.g., mutations or protein aggregation), autoimmune disease, systemic chronic inflammation, mental stress, metabolic disorders (e.g., diabetes, obesity), lifestyle factors (e.g., gut-brain axis), and environmental factors (Figure 8) (Sun et al., 2022). Sustained neuroinflammation can inhibit repair and ultimately lead to neurodegeneration and cognitive impairment.
The healthy BBB limits interactions between cells of the peripheral immune system and the brain. Evidence suggests that the machinery of immune cell recruitment and transmigration in BMECs specifically allows a subset of activated T cells in circulation to migrate across the healthy BBB (in the absence of inflammation) into the perivascular space (PVS) surrounding post-capillary venules or into the subarachnoid space (Engelhardt et al., 2017; Mapunda et al., 2022). Post-capillary venules sustain a low wall shear stress increasing the probability of capture and adhesion. Current models suggest that reactivation of these T cells following interactions with antigen presenting cells in the PVS promotes migration across the glia-limitans into the brain parenchyma (Mapunda et al., 2022; Aydin et al., 2023). Microglia, the primary resident immune cells in the brain, along with astrocytes, are responsible for many functions in the brain including the response to infection or injury.
There are four general causes of inflammation in the brain that can lead to BBB dysfunction: (1) neuroinflammation initiated in the brain parenchyma, which subsequently causes BBB dysfunction (e.g., MS), (2) neuroinflammation induced by systemic inflammation (e.g., sepsis), and (3) neuroinflammation caused by injury to the brain that results in simultaneous BBB disruption and neuroinflammation (e.g., TBI), and (4) neuroinflammation initiated by systemic inflammation and brain pathologies (e.g., Ab plaques, tau tangles) that together drive BBB dysfunction. These causes of inflammation can occur simultaneously, for example, familial mutations associated with neurodegenerative diseases (NDDs) result in production of toxic proteins both peripherally and in the brain. A current challenge is in identifying conditions or thresholds that prevent the resolution of the inflammatory response and lead to neurodegeneration, and how these thresholds may be impacted with other endogenous or exogenous perturbations such as oxidative stress, age, or lifestyle factors.
Brain hypoperfusion and ischemia lead to a cascade of events including generation of ROS, downregulation of TJs, loss of contact of astrocytic end-feet, loss of basement membrane, activation of astrocytes and microglia, and release of inflammatory cytokines, which together can result in entry of blood components into the brain. Reperfusion enables extravasation of leukocytes in circulation, further increasing release of inflammatory cytokines. Therefore, in MS, TBI, and stroke, the primary injury is followed by inflammation which leads to secondary neurodegeneration. In other diseases, including Alzheimer’s disease, amyotrophic lateral sclerosis, Parkinson’s and Huntington’s disease (HD), inflammation amplifies the pathological response and may play an important role in initiation.
The influence of systemic inflammation on BBB function is dependent on the concentration of pro-and anti-inflammatory factors, the identity of immune cells in circulation, and the duration of inflammation. At a sufficiently high level, inflammation can induce loss of barrier function, increased paracellular permeability, increased lymphocyte trafficking, and immune cell infiltration. For example, several studies in mouse models have shown a threshold concentration of LPS of around 3 mg/kg is required to observe increased solute permeability into the brain (Banks et al., 2015). Studies of Lucifer yellow permeability in rat pial capillaries (13–19 μm diameter) showed a 10-fold increase in permeability from 2 × 10−7 to 2.4 × 10−6 cm s−1 during perfusion via the carotid artery with 10 μM histamine (Easton et al., 1997). In addition, retrospective human studies have found that patients with an “abnormally inflammatory” CSF—as indicated by lymphocyte count, C-reactive protein, and erythrocyte sedimentation rate, among others—consistently had high albumin accumulation in the brain, indicating measurable disruption of the BBB (Elwood et al., 2017). Assessment of exposure to systemic inflammation is complicated by the fact that several cytokines including IL-1, IL-6, TNF-α, INF-γ, along with growth factors BDNF, EGF, and FGF are transported across the healthy BBB into the brain (Banks et al., 2009). Growth factors can also contribute to BBB leakage by promoting angiogenesis (Argaw et al., 2009, 2012).
Sepsis, an extreme reaction to infection, can lead to neuroinflammation and sepsis-associated encephalopathy (SAE), which is a common neurological complication. In animal models of exacerbated immune response using cecal ligation and punction or IP injection of LPS (10 mg/kg), increased BBB permeability (Evans blue, Gd contrast agent, etc.) is observed 12–24 h following the procedure (Gao and Hernandes, 2021). For example, in a cecal ligation and punction model in rats, immune cell adhesion in the cerebrovasculature was observed 6 h following induction (Comim et al., 2011; Barichello et al., 2021). Increased levels of MMP-2 and MMP-9 in the hippocampus and cortex at 12 h preceded BBB breakdown and increased levels of IL-1β, TNF-α, and IL-6. Persistent activation of microglia and release of ROS and RNS further increased cytotoxicity. In humans, brain tissue samples obtained from deceased sepsis patients revealed a significant downregulation of the TJ proteins occludin, claudin-5, and zonula occludens-1 (ZO-1) in microvascular endothelial cells (9) (Erikson et al., 2020), suggesting impaired BBB function.
While biomarkers such as TNF-α, IL-1β, IL-6, and CRP are associated with acute inflammation there are no recognized biomarkers for chronic inflammation (Furman et al., 2019). Systemic chronic inflammation (SCI) can be caused by various environmental and lifestyle factors and can lead to a wide range of diseases including hypertension, cardiovascular disease, and neurodegenerative disorders (Furman et al., 2019). In support of the link between SCI and neurodegenerative disease, individuals with rheumatoid arthritis treated with a TNF-α inhibitor had significantly lower incidence of AD (Chou et al., 2016). Furthermore, SCI increases with aging and is thought to be caused by cellular senescence and the development of a senescence-associated secretory phenotype (SASP) (Furman et al., 2019). Risk factors for SASP include intrinsic factors (DNA damage, oxidative stress) and extrinsic environmental and lifestyle factors (diet, gut microbiome, chronic infections, exposure to toxins, etc.).
8.3. Angiogenesis
The vasculature in the adult brain is largely quiescent, however, angiogenesis is associated with stroke (Croll and Wiegand, 2001; Brown et al., 2007; Jiang et al., 2018), brain cancer (Jain et al., 2007), TBI (Shlosberg et al., 2010; Salehi et al., 2017), exercise (Swain et al., 2003; Pereira et al., 2007), and high altitude adaptation (Figure 9; Park et al., 2016). In individuals with Huntington’s disease (HD), microvascular density is increased up to two-fold in the putamen compared to age-matched healthy controls, implying disease-associated angiogenesis (Drouin-Ouellet et al., 2015). In contrast, a loss in vascular density is associated with AD implying vessel loss and vascular pruning, although little is known about the impact of these processes on BBB function. Despite its importance in response to these perturbations, very little is known about the mechanisms of angiogenesis in the mature brain. A better understanding of the mechanistic details of angiogenesis in the mature brain is generally thought to be key to developing improved therapies for vascular repair.
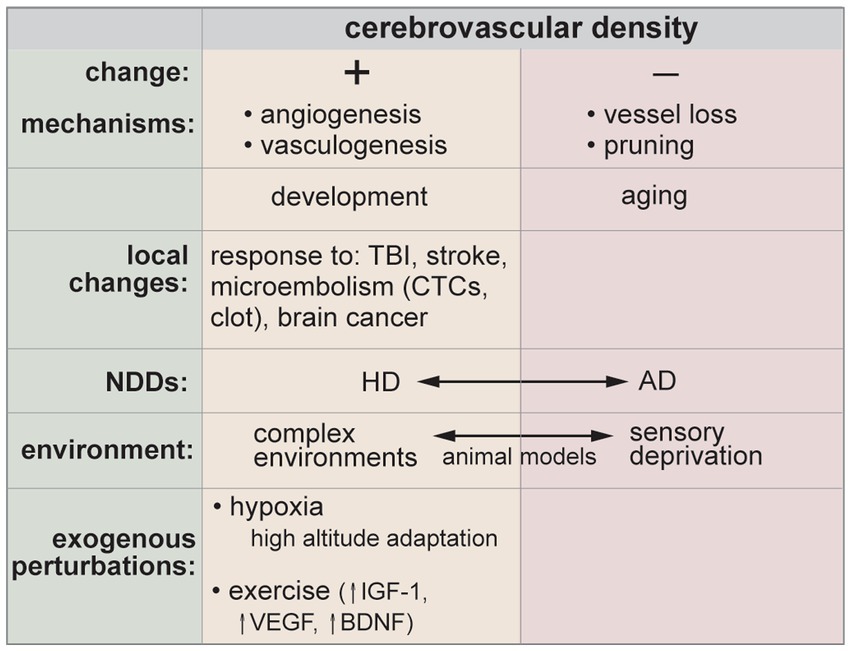
Figure 9. In the adult brain there is little change in cerebrovascular density, however, various injuries, pathological conditions, or environmental factors can promote angiogenesis or vessel loss and pruning. CTCs, circulating tumor cells. NDDs, neurodegenerative diseases. HD, Huntington’s disease. AD, Alzheimer’s disease.
In the classical model of sprouting angiogenesis in capillaries or venules in the brain, the local production of angiogenic factors activates BMECs, resulting in degradation of basement membrane and detachment of pericytes and other supporting cells (Conway et al., 2001; Carmeliet and Jain, 2011). Endothelial tip cells guide sprout growth toward another vessel, ultimately resulting in anastomosis and perfusion. Maturation of the sprout involves recruitment of pericytes (or other mural cells) and generation of new basement membrane. While the classical model depicts pericytes as passive bystanders, some studies have shown that pericytes can play an active role in angiogenesis, leading sprout growth and promoting the migration of stalk cells (Coelho-Santos et al., 2021; Zhao et al., 2023).
A wide range of growth factors are thought to regulate angiogenesis in the brain, including VEGF-A, bFGF, platelet derived growth factor (PDGF), insulin-like growth factor, Wnt, brain-derived neurotrophic factor, among others. The most widely studied are VEGF, bFGF, and PDGF, which are expressed by many diverse cell types. VEGF and bFGF promote angiogenesis in a wide range of tissues, including brain (Eelen et al., 2020; Trembath et al., 2020). PDGF-BB, secreted mainly by endothelial cells, plays an important role in recruiting pericytes to newly formed blood vessels and maintaining BBB integrity (Zhao et al., 2015). In stroke, hypoxia is thought to be the master regulator of angiogenesis with low O2 levels upregulating hypoxia inducible factors (HIFs), which in turn upregulates VEGF.
Many growth factors involved in angiogenesis have been shown to affect barrier function. VEGF downregulates TJs leading to increased BBB permeability (Argaw et al., 2012). In a mouse model, injection of 3 μL of 20 ng μL−1 VEGF-A into the brain resulted in decreased claudin-5 and occludin expression in BMECs and increased entry of albumin 24 h after injection, although no differences were detectable after 7 days, indicating that the disruption was reversible (Argaw et al., 2009).
Pathways associated with angiogenesis remain the targets for the development of pro-and anti-angiogenic therapies (Carmeliet and Jain, 2011; Durand et al., 2017; Eelen et al., 2020). Therapeutic angiogenesis, largely based on the prototypical growth factor VEGF, has been widely explored for treatment of non-brain ischemia and ischemic stroke (Semenza, 2006; Moccia et al., 2020). However, a consequence of therapeutic angiogenesis in the brain is increased paracellular permeability during vascular remodeling.
9. Pathogens
A broad range of pathogens including bacteria, fungi, and viruses can enter the brain leading to BBB dysfunction and increased risk of CNS infection and neuroinflammation. Despite the fact that these pathogens can enter the brain, the BBB remains a limitation in treating the infection.
Some of the most common bacterial species that cause CNS infection include Escherichia coli, Streptococcus pneumoniae, Haemophilus influenzae, and Neisseria meningitidis (Kim, 2006). Infection by these pathogens lead to bacterial meningitis which is inflammation of the protective membrane surrounding the brain and spinal cord. Although there is an incomplete understanding of how bacteria penetrate the BBB to cause meningitis, several factors are linked to successful invasion, including high levels of bacteria in circulation, adhesion to BMECs, and translocation across the BBB (Moxon and Ostrow, 1977; Bell et al., 1985; Kim, 2001, 2002, 2003). Currently, the main therapeutic strategies for treating bacterial meningitis include administering antibiotics as well as reducing inflammatory response using adjunctive drugs such as dexamethasone (De Gans and Van de Beek, 2002; Van de Beek et al., 2012).
Another example of bacterial infection in the CNS is Lyme neuroborreliosis, which is caused by the spirochete Borrelia burgdorferi (Bb) (Rupprecht et al., 2008; Kullberg et al., 2020). Lyme neuroborreliosis is reported in up to 12% of patients with Lyme disease, a tick-borne disease that is prevalent throughout North America, Europe, and Asia (Koedel et al., 2015). Common symptoms of Lyme neuroborreliosis include headaches, cranial nerve palsy, numbness and tingling, and fatigue (Pachner and Steiner, 2007; Schwenkenbecher et al., 2017). The exact method in which Bb are able to cross the BBB remains unclear (Comstock and Thomas, 1989; Szczepanski et al., 1990). However, in contrast with E. coli and S. pneumoniae, the concentration of Bb in human blood during infection is very low (≤ 0.1 per mL), indicating that the mechanism of infection is not consistent across bacterial species (Wormser et al., 2001; Pritt et al., 2016).
The incidence of fungal infections has increased as a result of an increase in the use of immunosuppressive drugs, autoimmune diseases, and invasive medical interventions (Lionakis and Levitz, 2018). Fungi can enter the brain either by direct inoculation (TBI, surgical intervention) or by crossing the BBB from circulation. Receptors for fungi (e.g., TLR, CLR) on astrocytes and microglia can initiate clearance or recruit immune cells via expression of cytokines (Snarr et al., 2020). However, if the infection is not cleared, neuroinflammation can lead to encephalitis and neuronal damage. Prevalent fungal species known to cause CNS infections include Cryptococcus neoformans, Candida albicans, Aspergillus fumigatus, and Zycomycetes (Lionakis and Levitz, 2018; Snarr et al., 2020). The WHO fungal priority list identifies Cryptococcus neoformans, Candida albicans, and Aspergillus fumigatus as “critical” for R&D and public health action (WHO fungal priority pathogens list to guide research, 2022). While Candida and Aspergillus are common fungal pathogens, CNS infections are relatively rare. However, for individuals with CNS infection the prognosis is poor. Cryptococcus infection in the brain has a very high mortality rate if untreated. While relatively little is known about the exact mechanisms of transport across the BBB, evidence suggests that Cryptococcus can enter the brain via infected monocytes or T cells in post-capillary venules, or by paracellular transport in capillaries (Snarr et al., 2020).
Common viruses that are known to infect the brain and disrupt the BBB include human immunodeficiency virus-1 (HIV-1), West Nile virus (WNV), herpes simplex virus-1 (HSV-1), and rabies virus (Spindler and Hsu, 2012). In the brain, the virus can replicate in various cell types leading to infection unless it is cleared by an immune response. Viral infection can lead to encephalitis and other pathological conditions such as meningitis (Klein et al., 2019). There are a variety of mechanisms by which viruses can enter the brain. The main route of entry for HIV-1 and WNV is via infected monocytes or T cells resulting in replication and infection of microglia (Wahl and Al-Harthi, 2023). As described previously, T cell migration into the perivascular space surrounding post-capillary venules is part of the limited immune surveillance in the brain. HIV invades the brain within ~2 weeks following infection (Wahl and Al-Harthi, 2023), where replication in the brain can lead to neuroinflammation and HIV-Associated Neurocognitive Disorder (HAND). HSV-1 and rabies, on the other hand, are thought to invade the brain through retrograde axonal transport (Schnell et al., 2010; Spindler and Hsu, 2012). In each of these cases, there is an increase in BBB permeability as well as inflammation (Toneatto et al., 1999; Wang et al., 2004; Phares et al., 2006). While some viruses, such as SV40, EV1, and cholera toxin B can cross endothelial barriers via caveola-mediated transcytosis, the suppression of this mode of transport in the healthy brain likely limits neuroinvasion (Klein et al., 2019). Several recent studies have found a correlation between exposure to viral infections (e.g., Epstein–Barr, viral encephalitis, influenza/pneumonia) and subsequent risk for neurodegenerative disease (Bjornevik et al., 2022; Levine et al., 2023).
10. Conclusion
BBB dysfunction is usually considered in the context of disease. Here we consider BBB dysfunction in the context of eight perturbations associated with BBB and brain health: mechanical forces, temperature, electromagnetic radiation, hypoxia, endogenous factors, exogenous factors, chemical factors, and pathogens. These perturbations may be endogenous or exogenous, pathological or therapeutic, or intended or unintended. The resultant outcome on BBB function depends on the dose (or magnitude) and duration of the perturbation. Understanding the influence of these perturbations is key to understanding the mechanisms and pathologies associated with BBB dysfunction and in developing new therapeutic strategies to treat diseases of the brain.
Author contributions
NZ: Writing – original draft, Writing – review & editing. TC: Writing – original draft, Writing – review & editing. ZG: Writing – original draft, Writing – review & editing. JJ: Writing – original draft, Writing – review & editing. LL: Writing – original draft, Writing – review & editing. RL: Writing – original draft, Writing – review & editing. AP: Writing – original draft, Writing – review & editing. LW: Writing – original draft, Writing – review & editing. PS: Conceptualization, Funding acquisition, Writing – original draft, Writing – review & editing.
Funding
The author(s) declare financial support was received for the research, authorship, and/or publication of this article. The work of PS is supported by the National Institutes of Health Grants R01NS106008, R61/33 HL154252, and R21NS131831.
Acknowledgments
AP acknowledges support from an NSF graduate fellowship (DGE2139757).
Conflict of interest
The authors declare that the research was conducted in the absence of any commercial or financial relationships that could be construed as a potential conflict of interest.
Publisher’s note
All claims expressed in this article are solely those of the authors and do not necessarily represent those of their affiliated organizations, or those of the publisher, the editors and the reviewers. Any product that may be evaluated in this article, or claim that may be made by its manufacturer, is not guaranteed or endorsed by the publisher.
References
Aasen, S. N., Espedal, H., Holte, C. F., Keunen, O., Karlsen, T. V., Tenstad, O., et al. (2019). Improved drug delivery to brain metastases by peptide-mediated Permeabilization of the blood-brain barrier. Mol. Cancer Ther. 18, 2171–2181. doi: 10.1158/1535-7163.MCT-19-0160
Abbott, N. J., Patabendige, A. A., Dolman, D. E., Yusof, S. R., and Begley, D. J. (2010). Structure and function of the blood-brain barrier. Neurobiol. Dis. 37, 13–25. doi: 10.1016/j.nbd.2009.07.030
Acharya, N. K., Goldwaser, E. L., Forsberg, M. M., Godsey, G. A., Johnson, C. A., Sarkar, A., et al. (2015). Sevoflurane and isoflurane induce structural changes in brain vascular endothelial cells and increase blood-brain barrier permeability: possible link to postoperative delirium and cognitive decline. Brain Res. 1620, 29–41. doi: 10.1016/j.brainres.2015.04.054
Alfieri, R. R., and Petronini, P. G. (2007). Hyperosmotic stress response: comparison with other cellular stresses. Pflugers Arch. 454, 173–185. doi: 10.1007/s00424-006-0195-x
Al-Gubory, K. H. (2014). Environmental pollutants and lifestyle factors induce oxidative stress and poor prenatal development. Reproduct. Bio Med. 29, 17–31. doi: 10.1016/j.rbmo.2014.03.002
Anasooya Shaji, C., Robinson, B. D., Yeager, A., Beeram, M. R., Davis, M. L., Isbell, C. L., et al. (2019). The tri-phasic role of hydrogen peroxide in blood-brain barrier endothelial cells. Sci. Rep. 9:36769. doi: 10.1038/s41598-018-36769-3
Argaw, A. T., Asp, L., Zhang, J., Navrazhina, K., Pham, T., Mariani, J. N., et al. (2012). Astrocyte-derived VEGF-A drives blood-brain barrier disruption in CNS inflammatory disease. J. Clin. Invest. 122, 2454–2468. doi: 10.1172/JCI60842
Argaw, A. T., Gurfein, B. T., Zhang, Y., Zameer, A., and John, G. R. (2009). VEGF-mediated disruption of endothelial CLN-5 promotes blood-brain barrier breakdown. Proc. Natl. Acad. Sci. U. S. A. 106, 1977–1982. doi: 10.1073/pnas.0808698106
Argaw, A. T., Zhang, Y., Snyder, B. J., Zhao, M.-L., Kopp, N., Lee, S. C., et al. (2006). IL-1β regulates blood-brain barrier permeability via reactivation of the hypoxia-angiogenesis program. J. Immunol. 177, 5574–5584. doi: 10.4049/jimmunol.177.8.5574
Arvanitis, C. D., Ferraro, G. B., and Jain, R. K. (2020). The blood-brain barrier and blood-tumour barrier in brain tumours and metastases. Nat. Rev. Cancer 20, 26–41. doi: 10.1038/s41568-019-0205-x
Auten, R. L., and Davis, J. M. (2009). Oxygen toxicity and reactive oxygen species: the devil is in the details. Pediatr. Res. 66, 121–127. doi: 10.1203/PDR.0b013e3181a9eafb
Aydin, S., Pareja, J., Schallenberg, V. M., Klopstein, A., Gruber, T., Page, N., et al. (2023). Antigen recognition detains CD8(+) T cells at the blood-brain barrier and contributes to its breakdown. Nat. Commun. 14:3106. doi: 10.3389/fimmu.2023.1233870
Bain, A. R., Nybo, L., and Ainslie, P. N. (2015). Cerebral vascular control and metabolism in heat stress. Compr. Physiol. 5, 1345–1380. doi: 10.1002/cphy.c140066
Baneke, A. (2010). What role does the blood brain barrier play in acute mountain sickness? Travel Med. Infect. Dis. 8, 257–262. doi: 10.1016/j.tmaid.2010.04.006
Banks, W. A., Gray, A. M., Erickson, M. A., Salameh, T. S., Damodarasamy, M., Sheibani, N., et al. (2015). Lipopolysaccharide-induced blood-brain barrier disruption: roles of cyclooxygenase, oxidative stress, neuroinflammation, and elements of the neurovascular unit. J. Neuroinflammation 12:223. doi: 10.1186/s12974-015-0434-1
Banks, W. A., Lynch, J. L., and Price, T. O. (2009). “Cytokines and the blood-brain barrier” in The Neuroimmunological basis of behavior and mental disorders. ed. A. S. A. S. S. Zalcman (New York, NY: Springer).
Banks, W. A., Reed, M. J., Logsdon, A. F., Rhea, E. M., and Erickson, M. A. (2021). Healthy aging and the blood-brain barrier. Nature Aging 1, 243–254. doi: 10.1038/s43587-021-00043-5
Barichello, T., Generoso, J. S., Collodel, A., Petronilho, F., and Dal-Pizzol, F. (2021). The blood-brain barrier dysfunction in sepsis. Tissue Barriers 9:1840912. doi: 10.1080/21688370.2020.1840912
Barnes, S., Chowdhury, S., Gatto, N. M., Fraser, G. E., and Lee, G. J. (2021). Omega-3 fatty acids are associated with blood-brain barrier integrity in a healthy aging population. Brain Behav. 11:2273. doi: 10.1002/brb3.2273
Baron, J. C. (2001). Perfusion thresholds in human cerebral ischemia: historical perspective and therapeutic implications. Cerebrovasc. Dis. 11, 2–8. doi: 10.1159/000049119
Baskaya, M. K., Rao, A. M., Dogan, A., Donaldson, D., and Dempsey, R. J. (1997). The biphasic opening of the blood-brain barrier in the cortex and hippocampus after traumatic brain injury in rats. Neurosci. Lett. 226, 33–36. doi: 10.1016/S0304-3940(97)00239-5
Bauer, A. T., Bürgers, H. F., Rabie, T., and Marti, H. H. (2010). Matrix Metalloproteinase-9 mediates hypoxia-induced vascular leakage in the brain via tight junction rearrangement. J. Cereb. Blood Flow Metab. 30, 837–848. doi: 10.1038/jcbfm.2009.248
Bell, L. M., Alpert, G., Campos, J. M., and Plotkin, S. A. (1985). Routine quantitative blood cultures in children with Haemophilus influenzae or Streptococcus pneumoniae bacteremia. Pediatrics 76, 901–904. doi: 10.1542/peds.76.6.901
Bjornevik, K., Cortese, M., Healy, B. C., Kuhle, J., Mina, M. J., Leng, Y., et al. (2022). Longitudinal analysis reveals high prevalence of Epstein-Barr virus associated with multiple sclerosis. Science 375, 296–301. doi: 10.1126/science.abj8222
Bogorad, M. I., DeStefano, J. G., Linville, R. M., Wong, A. D., and Searson, P. C. (2019). Cerebrovascular plasticity: processes that lead to changes in the architecture of brain microvessels. J. Cereb. Blood Flow Metab. 39, 1413–1432. doi: 10.1177/0271678X19855875
Bohr, T., Hjorth, P. G., Holst, S. C., Hrabetova, S., Kiviniemi, V., Lilius, T., et al. (2022). The glymphatic system: current understanding and modeling. iScience 25:104987. doi: 10.1016/j.isci.2022.104987
Borlongan, C. V., and Emerich, D. F. (2003). Facilitation of drug entry into the CNS via transient permeation of blood brain barrier: laboratory and preliminary clinical evidence from bradykinin receptor agonist, Cereport. Brain Res. Bull 60, 297–306. doi: 10.1016/S0361-9230(03)00043-1
Borras, J. M., Lievens, Y., Dunscombe, P., Coffey, M., Malicki, J., Corral, J., et al. (2015). The optimal utilization proportion of external beam radiotherapy in European countries: an ESTRO-HERO analysis. Radiother. Oncol. 116, 38–44. doi: 10.1016/j.radonc.2015.04.018
Bouchama, A., and Knochel, J. P. (2002). Heat stroke. N. Engl. J. Med. 346, 1978–1988. doi: 10.1056/NEJMra011089
Bowyer, J. F., and Ali, S. (2006). High doses of methamphetamine that cause disruption of the blood-brain barrier in limbic regions produce extensive neuronal degeneration in mouse hippocampus. Synapse 60, 521–532. doi: 10.1002/syn.20324
Bowyer, J. F., Tranter, K. M., Robinson, B. L., Hanig, J. P., Faubion, M. G., and Sarkar, S. (2018). The time course of blood brain barrier leakage and its implications on the progression of methamphetamine-induced seizures. Neurotoxicology 69, 130–140. doi: 10.1016/j.neuro.2018.09.008
Brahm, J. (1982). Diffusional water permeability of human-erythrocytes and their ghosts. J. Gen. Physiol. 79, 791–819. doi: 10.1085/jgp.79.5.791
Braniste, V., Al-Asmakh, M., Kowal, C., Anuar, F., Abbaspour, A., Toth, M., et al. (2014). The gut microbiota influences blood-brain barrier permeability in mice. Sci. Transl. Med. 6:9759. doi: 10.1126/scitranslmed.3009759
Brown, R., Benveniste, H., Black, S. E., Charpak, S., Dichgans, M., Joutel, A., et al. (2018). Understanding the role of the perivascular space in cerebral small vessel disease. Cardiovasc. Res. 114, 1462–1473. doi: 10.1093/cvr/cvy113
Brown, C. E., Li, P., Boyd, J. D., Delaney, K. R., and Murphy, T. H. (2007). Extensive turnover of dendritic spines and vascular remodeling in cortical tissues recovering from stroke. J. Neurosci. 27, 4101–4109. doi: 10.1523/JNEUROSCI.4295-06.2007
Brown, W. R., and Thore, C. R. (2011). Review: cerebral microvascular pathology in ageing and neurodegeneration. Neuropathol. Appl. Neurobiol. 37, 56–74. doi: 10.1111/j.1365-2990.2010.01139.x
Burkhardt, J. K., Riina, H., Shin, B. J., Christos, P., Kesavabhotla, K., Hofstetter, C. P., et al. (2012). Intra-arterial delivery of bevacizumab after blood-brain barrier disruption for the treatment of recurrent glioblastoma: progression-free survival and overall survival. World Neurosurg. 77, 130–134. doi: 10.1016/j.wneu.2011.05.056
Busto, R., Dietrich, W. D., Globus, M. Y. T., Valdes, I., Scheinberg, P., and Ginsberg, M. D. (1987). Small differences in Intraischemic brain temperature critically determine the extent of ischemic neuronal injury. J. Cereb. Blood Flow Metab. 7, 729–738. doi: 10.1038/jcbfm.1987.127
Camasao, D. B., and Mantovani, D. (2021). The mechanical characterization of blood vessels and their substitutes in the continuous quest for physiological-relevant performances. A critical review. Materials Today Bio 10:106. doi: 10.1016/j.mtbio.2021.100106
Campinho, P., Vilfan, A., and Vermot, J. (2020). Blood flow forces in shaping the vascular system: a focus on endothelial cell behavior. Front. Physiol. 11:552. doi: 10.3389/fphys.2020.00552
Cao, Y., Li, Z., Li, H., Ni, C., Li, L., Yang, N., et al. (2018). Hypoxia-inducible factor-1alpha is involved in isoflurane-induced blood-brain barrier disruption in aged rats model of POCD. Behav. Brain Res. 339, 39–46. doi: 10.1016/j.bbr.2017.09.004
Cao, Y., Ni, C., Li, Z., Li, L., Liu, Y., Wang, C., et al. (2015). Isoflurane anesthesia results in reversible ultrastructure and occludin tight junction protein expression changes in hippocampal blood-brain barrier in aged rats. Neurosci. Lett. 587, 51–56. doi: 10.1016/j.neulet.2014.12.018
Cao, Y., Tsien, C. I., Sundgren, P. C., Nagesh, V., Normolle, D., Buchtel, H., et al. (2009). Dynamic contrast-enhanced magnetic resonance imaging as a biomarker for prediction of radiation-induced neurocognitive dysfunction. Clin. Cancer Res. 15, 1747–1754. doi: 10.1158/1078-0432.CCR-08-1420
Carmeliet, P., and Jain, R. K. (2011). Molecular mechanisms and clinical applications of angiogenesis. Nature 473, 298–307. doi: 10.1038/nature10144
Caroff, J., King, R. M., Mitchell, J. E., Marosfoi, M., Licwinko, J. R., Gray-Edwards, H. L., et al. (2020). Focal cooling of brain parenchyma in a transient large vessel occlusion model: proof-of-concept. J. Neuro Intervent. Surg. 12, 209–213. doi: 10.1136/neurintsurg-2019-015179
Carraro, E., Schilirò, T., Biorci, F., Romanazzi, V., Degan, R., Buonocore, D., et al. (2018). Physical activity, lifestyle factors and oxidative stress in middle age healthy subjects. Int. J. Environ. Res. Public Health 15:1152. doi: 10.3390/ijerph15061152
Carreau, A., Hafny-Rahbi, B. E., Matejuk, A., Grillon, C., and Kieda, C. (2011). Why is the partial oxygen pressure of human tissues a crucial parameter? Small molecules and hypoxia. J. Cell. Mol. Med. 15, 1239–1253. doi: 10.1111/j.1582-4934.2011.01258.x
Cassano, P., Tran, A. P., Katnani, H., Bleier, B. S., Hamblin, M. R., Yuan, Y., et al. (2019). Selective photobiomodulation for emotion regulation: model-based dosimetry study. Neurophotonics 6:015004. doi: 10.1117/1.NPh.6.1.015004
Cekanaviciute, E., Rosi, S., and Costes, S. V. (2018). Central nervous system responses to simulated galactic cosmic rays. Int. J. Mol. Sci. 19. doi: 10.3390/ijms19113669
Charras, G., and Yap, A. S. (2018). Tensile forces and Mechanotransduction at cell-cell junctions. Curr. Biol. 28, R445–R457. doi: 10.1016/j.cub.2018.02.003
Chen, X., Gawryluk, J. W., Wagener, J. F., Ghribi, O., and Geiger, J. D. (2008). Caffeine blocks disruption of blood brain barrier in a rabbit model of Alzheimer’s disease. J. Neuroinflammation 5:12. doi: 10.1186/s12974-023-02725-w
Chen, W. L., Wagner, J., Heugel, N., Sugar, J., Lee, Y. W., Conant, L., et al. (2020). Functional near-infrared spectroscopy and its clinical application in the field of neuroscience: advances and future directions. Front. Neurosci. 14:724. doi: 10.3389/fnins.2020.00724
Chen, M. B., Yang, A. C., Yousef, H., Lee, D., Chen, W., Schaum, N., et al. (2020). Brain endothelial cells are exquisite sensors of age-related circulatory cues. Cell Rep. 30, 4418–4432.e4. doi: 10.1016/j.celrep.2020.03.012
Choi, J. H., Poli, S., Chen, M., Nguyen, T. N., Saver, J. L., Matouk, C., et al. (2020). Selective brain hypothermia in acute ischemic stroke: reperfusion without reperfusion injury. Front. Neurol. 11:594289. doi: 10.3389/fneur.2020.594289
Chou, R. C., Kane, M., Ghimire, S., Gautam, S., and Gui, J. (2016). Treatment for rheumatoid arthritis and risk of Alzheimer’s disease: a nested case-control analysis. CNS Drugs 30, 1111–1120. doi: 10.1007/s40263-016-0374-z
Chu, C., Liu, G., Janowski, M., Bulte, J. W. M., Li, S., Pearl, M., et al. (2018). Real-time MRI guidance for reproducible hyperosmolar opening of the blood-brain barrier in mice. Front. Neurol. 9:921. doi: 10.3389/fneur.2018.00921
Chung, T. D., Linville, R. M., Guo, Z., Ye, R., Jha, R., Grifno, G. N., et al. (2022). Effects of acute and chronic oxidative stress on the blood-brain barrier in 2D and 3D in vitro models. Fluids Barriers CNS 19:33. doi: 10.1186/s12987-022-00327-x
Claassen, J., Thijssen, D. H. J., Panerai, R. B., and Faraci, F. M. (2021). Regulation of cerebral blood flow in humans: physiology and clinical implications of autoregulation. Physiol. Rev. 101, 1487–1559. doi: 10.1152/physrev.00022.2020
Cloughesy, T. F., Black, K. L., Gobin, Y. P., Farahani, K., Nelson, G., Villablanca, P., et al. (1999). Intra-arterial Cereport (RMP-7) and carboplatin: a dose escalation study for recurrent malignant gliomas. Neurosurgery 44, 270–278; discussion 278-9. doi: 10.1097/00006123-199902000-00015
Cobley, J. N., Fiorello, M. L., and Bailey, D. M. (2018). 13 reasons why the brain is susceptible to oxidative stress. Redox Biol. 15, 490–503. doi: 10.1016/j.redox.2018.01.008
Coelho-Santos, V., Berthiaume, A.-A., Ornelas, S., Stuhlmann, H., and Shih, A. Y. (2021). Imaging the construction of capillary networks in the neonatal mouse brain. Proc. Natl. Acad. Sci. 118:e2100866118. doi: 10.1073/pnas.2100866118
Coelho-Santos, V., Leitao, R. A., Cardoso, F. L., Palmela, I., Rito, M., Barbosa, M., et al. (2015). The TNF-alpha/NF-kappaB signaling pathway has a key role in methamphetamine-induced blood-brain barrier dysfunction. J. Cereb. Blood Flow Metab. 35, 1260–1271. doi: 10.1038/jcbfm.2015.59
Cohn, R. M., and Roth, K. S. (2004). Hyperammonemia, bane of the brain. Clin. Pediatr. 43, 683–689. doi: 10.1177/000992280404300801
Comim, C. M., Vilela, M. C., Constantino, L. S., Petronilho, F., Vuolo, F., Lacerda-Queiroz, N., et al. (2011). Traffic of leukocytes and cytokine up-regulation in the central nervous system in sepsis. Intensive Care Med. 37, 711–718. doi: 10.1007/s00134-011-2151-2
Comstock, L. E., and Thomas, D. D. (1989). Penetration of endothelial cell monolayers by Borrelia burgdorferi. Infect. Immun. 57, 1626–1628. doi: 10.1128/iai.57.5.1626-1628.1989
Conway, E. M., Collen, D., and Carmeliet, P. (2001). Molecular mechanisms of blood vessel growth. Cardiovasc. Res. 49, 507–521. doi: 10.1016/S0008-6363(00)00281-9
Courtney, A., and Courtney, M. (2007). Links between traumatic brain injury and ballistic pressure waves originating in the thoracic cavity and extremities. Brain Inj. 21, 657–662. doi: 10.1080/02699050701481571
Courtney, A., and Courtney, M. (2015). The complexity of biomechanics causing primary blast-induced traumatic brain injury: a review of potential mechanisms. Front. Neurol. 6:221. doi: 10.3389/fneur.2015.00221
Croll, S. D., and Wiegand, S. J. (2001). Vascular growth factors in cerebral ischemia. Mol. Neurobiol. 23, 121–135. doi: 10.1385/MN:23:2-3:121
Crouch, E. E., Joseph, T., Marsan, E., and Huang, E. J. (2023). Disentangling brain vasculature in neurogenesis and neurodegeneration using single-cell transcriptomics. Trends Neurosci. 46, 551–565. doi: 10.1016/j.tins.2023.04.007
Cucinotta, F. A., and Durante, M. (2006). Cancer risk from exposure to galactic cosmic rays: implications for space exploration by human beings. Lancet Oncol. 7, 431–435. doi: 10.1016/S1470-2045(06)70695-7
Curnes, J. T., Laster, D. W., Ball, M. R., Moody, D. M., and Witcofski, R. L. (1986). MRI of radiation injury to the brain. Am. J. Roentgenol. 147, 119–124. doi: 10.2214/ajr.147.1.119
Davis, C., and Hackett, P. (2017). Advances in the prevention and treatment of high altitude illness. Emerg. Med. Clin. North Am. 35, 241–260. doi: 10.1016/j.emc.2017.01.002
de Aquino, C. C., Leitao, R. A., Oliveira Alves, L. A., Coelho-Santos, V., Guerrant, R. L., Ribeiro, C. F., et al. (2018). Effect of Hypoproteic and high-fat diets on hippocampal blood-brain barrier permeability and oxidative stress. Front. Nutr. 5:131. doi: 10.3389/fnut.2018.00131
De Gans, J., and Van de Beek, D. (2002). Dexamethasone in adults with bacterial meningitis. N. Engl. J. Med. 347, 1549–1556. doi: 10.1056/NEJMoa021334
De Silva, T. M., Peña Silva, R. A., and Faraci, F. M. (2016). “Endothelium, the blood-brain barrier, and hypertension” in Hypertension and the brain as an end-organ target. ed. H. Girouard (New York: Springer).
DeStefano, J. G., Xu, Z. S., Williams, A. J., Yimam, N., and Searson, P. C. (2017). Effect of shear stress on iPSC-derived human brain microvascular endothelial cells (dhBMECs). Fluids Barriers CNS 14. doi: 10.1186/s12987-017-0068-z
Dewhirst, M. W., Viglianti, B. L., Lora-Michiels, M., Hanson, M., and Hoopes, P. J. (2003). Basic principles of thermal dosimetry and thermal thresholds for tissue damage from hyperthermia. Int. J. Hyperth. 19, 267–294. doi: 10.1080/0265673031000119006
Dion-Albert, L., Cadoret, A., Doney, E., Kaufmann, F. N., Dudek, K. A., Daigle, B., et al. (2022). Vascular and blood-brain barrier-related changes underlie stress responses and resilience in female mice and depression in human tissue. Nat. Commun. 13:164. doi: 10.1038/s41467-021-27604-x
Doctrow, S. R., Abelleira, S. M., Curry, L. A., Heller-Harrison, R., Kozarich, J. W., Malfroy, B., et al. (1994). The bradykinin analog RMP-7 increases intracellular free calcium levels in rat brain microvascular endothelial cells. J. Pharmacol. Exp. Ther. 271, 229–237.
Doolittle, N. D., Miner, M. E., Hall, W. A., Siegal, T., Jerome, E., Osztie, E., et al. (2000). Safety and efficacy of a multicenter study using intraarterial chemotherapy in conjunction with osmotic opening of the blood-brain barrier for the treatment of patients with malignant brain tumors. Cancer 88, 637–647.
Drouin-Ouellet, J., Sawiak, S. J., Cisbani, G., Lagacé, M., Kuan, W.-L., Saint-Pierre, M., et al. (2015). Cerebrovascular and blood-brain barrier impairments in Huntington’s disease: potential implications for its pathophysiology. Ann. Neurol. 78, 160–177. doi: 10.1002/ana.24406
Dumitrascu, O. M., Lamb, J., and Lyden, P. D. (2016). Still cooling after all these years: meta-analysis of pre-clinical trials of therapeutic hypothermia for acute ischemic stroke. J. Cereb. Blood Flow Metab. 36, 1157–1164. doi: 10.1177/0271678X16645112
Durand, M. J., Ait-Aissa, K., and Gutterman, D. D. (2017). Regenerative angiogenesis: quality over quantity. Circ. Res. 120, 1379–1380. doi: 10.1161/CIRCRESAHA.117.310918
Durukan, A., and Tatlisumak, T. (2007). Acute ischemic stroke: overview of major experimental rodent models, pathophysiology, and therapy of focal cerebral ischemia. Pharmacol. Biochem. Behav. 87, 179–197. doi: 10.1016/j.pbb.2007.04.015
Easton, A. S., Sarker, M. H., and Fraser, P. A. (1997). Two components of blood-brain barrier disruption in the rat. J. Physiol. 503, 613–623.
Eelen, G., Treps, L., Li, X., and Carmeliet, P. (2020). Basic and therapeutic aspects of angiogenesis updated. Circ. Res. 127, 310–329. doi: 10.1161/CIRCRESAHA.120.316851
Elwood, E., Lim, Z., Naveed, H., and Galea, I. (2017). The effect of systemic inflammation on human brain barrier function. Brain Behav. Immun. 62, 35–40. doi: 10.1016/j.bbi.2016.10.020
Emerich, D. F., Dean, R. L., Marsh, J., Pink, M., Lafreniere, D., Snodgrass, P., et al. (2000). Intravenous cereport (RMP-7) enhances delivery of hydrophilic chemotherapeutics and increases survival in rats with metastatic tumors in the brain. Pharm. Res. 17, 1212–1219. doi: 10.1023/A:1026462629438
Emerich, D. F., Dean, R. L., Osborn, C., and Bartus, R. T. (2001). The development of the bradykinin agonist labradimil as a means to increase the permeability of the blood-brain barrier: from concept to clinical evaluation. Clin. Pharmacokinet. 40, 105–123. doi: 10.2165/00003088-200140020-00003
Emerich, D. F., Snodgrass, P., Dean, R., Agostino, M., Hasler, B., Pink, M., et al. (1999). Enhanced delivery of carboplatin into brain tumours with intravenous Cereport (RMP-7): dramatic differences and insight gained from dosing parameters. Br. J. Cancer 80, 964–970. doi: 10.1038/sj.bjc.6690450
Emerich, D. F., Snodgrass, P., Pink, M., Bloom, F., and Bartus, R. T. (1998). Central analgesic actions of loperamide following transient permeation of the blood brain barrier with Cereport (RMP-7). Brain Res. 801, 259–266. doi: 10.1016/S0006-8993(98)00571-X
Engelhardt, B. (2008a). Immune cell entry into the central nervous system: involvement of adhesion molecules and chemokines. J. Neurol. Sci. 274, 23–26. doi: 10.1016/j.jns.2008.05.019
Engelhardt, B. (2008b). The blood-central nervous system barriers actively control immune cell entry into the central nervous system. Curr. Pharm. Des. 14, 1555–1565. doi: 10.2174/138161208784705432
Engelhardt, S., Al-Ahmad, A. J., Gassmann, M., and Ogunshola, O. O. (2014). Hypoxia selectively disrupts brain microvascular endothelial tight junction complexes through a hypoxia-inducible Factor-1 (HIF-1) dependent mechanism. J. Cell. Physiol. 229, 1096–1105. doi: 10.1002/jcp.24544
Engelhardt, B., Vajkoczy, P., and Weller, R. O. (2017). The movers and shapers in immune privilege of the CNS. Nat. Immunol. 18, 123–131. doi: 10.1038/ni.3666
Erdo, F., Denes, L., and de Lange, E. (2017). Age-associated physiological and pathological changes at the blood-brain barrier: a review. J. Cereb. Blood Flow Metab. 37, 4–24. doi: 10.1177/0271678X16679420
Erikson, K., Tuominen, H., Vakkala, M., Liisanantti, J. H., Karttunen, T., Syrjala, H., et al. (2020). Brain tight junction protein expression in sepsis in an autopsy series. Crit. Care 24:385. doi: 10.1186/s13054-020-03101-3
Fasano, A. (2020). All disease begins in the (leaky) gut: role of zonulin-mediated gut permeability in the pathogenesis of some chronic inflammatory diseases. F1000Res 9:20510. doi: 10.12688/f1000research.20510.1
Ferris, C. F. (2021). Rethinking the conditions and mechanism for Glymphatic clearance. Front. Neurosci. 15:624690. doi: 10.3389/fnins.2021.624690
Fike, J. R., Gobbel, G. T., Mesiwala, A. H., Shin, H. J., Nakagawa, M., Lamborn, K. R., et al. (1998). Cerebrovascular effects of the bradykinin analog RMP-7 in normal and irradiated dog brain. J. Neuro-Oncol. 37, 199–215.
Fischer, S., Wobben, M., Kleinstück, J., Renz, D., and Schaper, W. (2000). Effect of astroglial cells on hypoxia-induced permeability in PBMEC cells. Am. J. Phys. Cell Phys. 279, C935–C944. doi: 10.1152/ajpcell.2000.279.4.C935
Flack, J. M., and Adekola, B. (2020). Blood pressure and the new ACC/AHA hypertension guidelines. Trends Cardiovasc. Med. 30, 160–164. doi: 10.1016/j.tcm.2019.05.003
Forman, H. J., Fukuto, J. M., and Torres, M. (2004). Redox signaling: thiol chemistry defines which reactive oxygen and nitrogen species can act as second messengers. Am. J. Physiol. Cell Physiol. 287, C246–C256. doi: 10.1152/ajpcell.00516.2003
Fredriksson, K., Kalimo, H., Westergren, I., Kahrstrom, J., and Johansson, B. B. (1987). Blood-brain barrier leakage and brain edema in stroke-prone spontaneously hypertensive rats. Effect of chronic sympathectomy and low protein/high salt diet. Acta Neuropathol. 74, 259–268. doi: 10.1007/BF00688190
Furman, D., Campisi, J., Verdin, E., Carrera-Bastos, P., Targ, S., Franceschi, C., et al. (2019). Chronic inflammation in the etiology of disease across the life span. Nat. Med. 25, 1822–1832. doi: 10.1038/s41591-019-0675-0
Gao, Q., and Hernandes, M. S. (2021). Sepsis-associated encephalopathy and blood-brain barrier dysfunction. Inflammation 44, 2143–2150. doi: 10.1007/s10753-021-01501-3
Garcia, F. J., Sun, N., Lee, H., Godlewski, B., Mathys, H., Galani, K., et al. (2022). Single-cell dissection of the human brain vasculature. Nature 603, 893–899. doi: 10.1038/s41586-022-04521-7
Golden, T. R., Hinerfeld, D. A., and Melov, S. (2002). Oxidative stress and aging: beyond correlation. Aging Cell 1, 117–123. doi: 10.1046/j.1474-9728.2002.00015.x
Gonzalez-Lima, F., and Barrett, D. W. (2014). Augmentation of cognitive brain functions with transcranial lasers. Front. Syst. Neurosci. 8:36. doi: 10.3389/fnsys.2014.00036
Goodall, E. F., Wang, C., Simpson, J. E., Baker, D. J., Drew, D. R., Heath, P. R., et al. (2018). Age-associated changes in the blood-brain barrier: comparative studies in human and mouse. Neuropathol. Appl. Neurobiol. 44, 328–340. doi: 10.1111/nan.12408
Greene-Schloesser, D., Robbins, M. E., Peiffer, A. M., Shaw, E. G., Wheeler, K. T., and Chan, M. D. (2012). Radiation-induced brain injury: a review. Front. Oncol. 2:73. doi: 10.3389/fonc.2012.00073
Gregor, A., Lind, M., Newman, H., Grant, R., Hadley, D. M., Barton, T., et al. (1999). Phase II studies of RMP-7 and carboplatin in the treatment of recurrent high grade glioma. RMP-7 European study group. J. Neuro-Oncol. 44, 137–145.
Grocott, M. P. W., Martin, D. S., Levett, D. Z. H., McMorrow, R., Windsor, J., Montgomery, H. E., et al. (2009). Arterial blood gases and oxygen content in climbers on Mount Everest. N. Engl. J. Med. 360, 140–149. doi: 10.1056/NEJMoa0801581
Guley, N. H., Rogers, J. T., Del Mar, N. A., Deng, Y., Islam, R. M., D’Surney, L., et al. (2016). A novel closed-head model of mild traumatic brain injury using focal primary overpressure blast to the cranium in mice. J. Neurotrauma 33, 403–422. doi: 10.1089/neu.2015.3886
Gustafson, D. R., Karlsson, C., Skoog, I., Rosengren, L., Lissner, L., and Blennow, K. (2007). Mid-life adiposity factors relate to blood-brain barrier integrity in late life. J. Intern. Med. 262, 643–650. doi: 10.1111/j.1365-2796.2007.01869.x
Hackett, P. H., and Roach, R. C. (2001). High-Altitude Illness. N. Engl. J. Med. 345, 107–114. doi: 10.1056/NEJM200107123450206
Haley, M. J., and Lawrence, C. B. (2017). The blood-brain barrier after stroke: structural studies and the role of transcytotic vesicles, the blood-brain barrier after stroke: structural studies and the role of transcytotic vesicles. J. Cereb. Blood Flow Metab. 37, 456–470. doi: 10.1177/0271678X16629976
Haller, S., Vernooij, M. W., Kuijer, J. P. A., Larsson, E. M., Jager, H. R., and Barkhof, F. (2018). Cerebral microbleeds: imaging and clinical significance. Radiology 287, 11–28. doi: 10.1148/radiol.2018170803
Hamblin, M. R. (2016). Shining light on the head: Photobiomodulation for brain disorders. BBA Clin 6, 113–124. doi: 10.1016/j.bbacli.2016.09.002
Hannocks, M. J., Pizzo, M. E., Huppert, J., Deshpande, T., Abbott, N. J., Thorne, R. G., et al. (2018). Molecular characterization of perivascular drainage pathways in the murine brain. J. Cereb. Blood Flow Metab. 38, 669–686. doi: 10.1177/0271678X17749689
Haorah, J., Knipe, B., Leibhart, J., Ghorpade, A., and Persidsky, Y. (2005). Alcohol-induced oxidative stress in brain endothelial cells causes blood-brain barrier dysfunction. J. Leukoc. Biol. 78, 1223–1232. doi: 10.1189/jlb.0605340
Haorah, J., Schall, K., Ramirez, S. H., and Persidsky, Y. (2008). Activation of protein tyrosine kinases and matrix metalloproteinases causes blood-brain barrier injury: novel mechanism for neurodegeneration associated with alcohol abuse. Glia 56, 78–88. doi: 10.1002/glia.20596
Harmon, B., Corder, A., Collins, R., Gobe, G., Allen, J., Allan, D., et al. (1990). Cell death induced in a murine mastocytoma by 42–47 C heating in vitro: evidence that the form of death changes from apoptosis to necrosis above a critical heat load. Int. J. Radiat. Biol. 58, 845–858.
Hartz, A. M. S., Miller, D. S., and Bauer, B. (2010). Restoring blood-brain barrier P-glycoprotein reduces brain amyloid-beta in a mouse model of Alzheimer’s disease. Mol. Pharmacol. 77, 715–723. doi: 10.1124/mol.109.061754
Hawasli, A. H., Kim, A. H., Dunn, G. P., Tran, D. D., and Leuthardt, E. C. (2014). Stereotactic laser ablation of high-grade gliomas. Neurosurg. Focus. 37:E1. doi: 10.3171/2014.9.FOCUS14471
Hawkins, B. T., and Davis, T. P. (2005). The blood-brain barrier/neurovascular unit in health and disease. Pharmacol. Rev. 57, 173–185. doi: 10.1124/pr.57.2.4
Holste, K. G., and Orringer, D. A. (2020). Laser interstitial thermal therapy. Neurooncol. Adv. 2:vdz035. doi: 10.1093/noajnl/vdz035
Houthoff, H. J., Go, K. G., and Gerrits, P. O. (1982). The mechanisms of blood-brain barrier impairment by hyperosmolar perfusion. An electron cytochemical study comparing exogenous HRP and endogenous antibody to HRP as tracers. Acta Neuropathol. 56, 99–112. doi: 10.1007/BF00690580
Huang, B., Law, M. W.-M., and Khong, P.-L. (2009). Whole-body PET/CT scanning: estimation of radiation dose and cancer risk. Radiology 251, 166–174. doi: 10.1148/radiol.2511081300
Hynynen, K., McDannold, N., Sheikov, N. A., Jolesz, F. A., and Vykhodtseva, N. (2005). Local and reversible blood-brain barrier disruption by noninvasive focused ultrasound at frequencies suitable for trans-skull sonications. NeuroImage 24, 12–20. doi: 10.1016/j.neuroimage.2004.06.046
Idbaih, A., Canney, M., Belin, L., Desseaux, C., Vignot, A., Bouchoux, G., et al. (2019). Safety and feasibility of repeated and transient blood-brain barrier disruption by pulsed ultrasound in patients with recurrent glioblastoma. Clin. Cancer Res. 25, 3793–3801. doi: 10.1158/1078-0432.CCR-18-3643
Inamura, T., and Black, K. L. (1994). Bradykinin selectively opens blood-tumor barrier in experimental brain tumors. J. Cereb. Blood Flow Metab. 14, 862–870. doi: 10.1038/jcbfm.1994.108
Izadifar, Z., Izadifar, Z., Chapman, D., and Babyn, P. (2020). An introduction to high intensity focused ultrasound: systematic review on principles, devices, and clinical applications. J. Clin. Med. 9:460. doi: 10.3390/jcm9020460
Jaeger, L. B., Dohgu, S., Hwang, M. C., Farr, S. A., Murphy, M. P., Fleegal-DeMotta, M. A., et al. (2009). Testing the neurovascular hypothesis of Alzheimer’s disease: LRP-1 antisense reduces blood-brain barrier clearance, increases brain levels of amyloid-beta protein, and impairs cognition. J. Alzheimers Dis. 17, 553–570. doi: 10.3233/JAD-2009-1074
Jagdeo, J. R., Adams, L. E., Brody, N. I., and Siegel, D. M. (2012). Transcranial red and near infrared light transmission in a cadaveric model. PLoS One 7:e47460. doi: 10.1371/journal.pone.0047460
Jain, R. K., Di Tomaso, E., Duda, D. G., Loeffler, J. S., Sorensen, A. G., and Batchelor, T. T. (2007). Angiogenesis in brain tumours. Nat. Rev. Neurosci. 8, 610–622. doi: 10.1038/nrn2175
Jeong, H. W., Dieguez-Hurtado, R., Arf, H., Song, J., Park, H., Kruse, K., et al. (2022). Single-cell transcriptomics reveals functionally specialized vascular endothelium in brain. elife 11:57520. doi: 10.7554/eLife.57520
Jiang, X., Andjelkovic, A. V., Zhu, L., Yang, T., Bennett, M. V., Chen, J., et al. (2018). Blood-brain barrier dysfunction and recovery after ischemic stroke. Prog. Neurobiol. 163, 144–171. doi: 10.1016/j.pneurobio.2017.10.001
Johansson, B. B., and Martinsson, L. (1980). The blood-brain barrier in adrenaline-induced hypertension. Circadian variations and modification by beta-adrenoreceptor antagonists. Acta Neurol. Scand. 62, 96–102. doi: 10.1111/j.1600-0404.1980.tb03009.x
Johnson, V. E., Weber, M. T., Xiao, R., Cullen, D. K., Meaney, D. F., Stewart, W., et al. (2018). Mechanical disruption of the blood-brain barrier following experimental concussion. Acta Neuropathol. 135, 711–726. doi: 10.1007/s00401-018-1824-0
Joseph, A., Wanono, R., Flamant, M., and Vidal-Petiot, E. (2017). Orthostatic hypotension: a review. Nephrol. Therap. 13, S55–S67. doi: 10.1016/j.nephro.2017.01.003
Kanoski, S. E., Zhang, Y., Zheng, W., and Davidson, T. L. (2010). The effects of a high-energy diet on hippocampal function and blood-brain barrier integrity in the rat. J. Alzheimers Dis. 21, 207–219. doi: 10.3233/JAD-2010-091414
Kim, K. S. (2001). Escherichia coli translocation at the blood-brain barrier. Infect. Immun. 69, 5217–5222. doi: 10.1128/IAI.69.9.5217-5222.2001
Kim, K. S. (2002). Strategy of Escherichia coli for crossing the blood-brain barrier. J. Infect. Dis. 186, S220–S224. doi: 10.1086/344284
Kim, K. S. (2003). Pathogenesis of bacterial meningitis: from bacteraemia to neuronal injury. Nat. Rev. Neurosci. 4, 376–385. doi: 10.1038/nrn1103
Kim, K. S. (2006). Microbial translocation of the blood-brain barrier. Int. J. Parasitol. 36, 607–614. doi: 10.1016/j.ijpara.2006.01.013
Kim, C.-H., Park, Y., Chun, M. Y., and Kim, Y.-J. (2020). Exercise-induced hypertension can increase the prevalence of coronary artery plaque among middle-aged male marathon runners. Medicine 99:23789. doi: 10.1097/MD.0000000000023789
Kinoshita, M., McDannold, N., Jolesz, F. A., and Hynynen, K. (2006). Noninvasive localized delivery of Herceptin to the mouse brain by MRI-guided focused ultrasound-induced blood-brain barrier disruption. Proc. Natl. Acad. Sci. U. S. A. 103, 11719–11723. doi: 10.1073/pnas.0604318103
Kiss, T., Nyul-Toth, A., Balasubramanian, P., Tarantini, S., Ahire, C., DelFavero, J., et al. (2020). Single-cell RNA sequencing identifies senescent cerebromicrovascular endothelial cells in the aged mouse brain. Geroscience 42, 429–444. doi: 10.1007/s11357-020-00177-1
Klein, R. S., Garber, C., Funk, K. E., Salimi, H., Soung, A., Kanmogne, M., et al. (2019). Neuroinflammation during RNA viral infections. Annu. Rev. Immunol. 37, 73–95. doi: 10.1146/annurev-immunol-042718-041417
Koedel, U., Fingerle, V., and Pfister, H.-W. (2015). Lyme neuroborreliosis—epidemiology, diagnosis and management. Nat. Rev. Neurol. 11, 446–456. doi: 10.1038/nrneurol.2015.121
Kokubu, Y., Yamaguchi, T., and Kawabata, K. (2017). In vitro model of cerebral ischemia by using brain microvascular endothelial cells derived from human induced pluripotent stem cells. Biochem. Biophys. Res. Commun. 486, 577–583. doi: 10.1016/j.bbrc.2017.03.092
Komin, A., Russell, L. M., Hristova, K. A., and Searson, P. C. (2017). Peptide-based strategies for enhanced cell uptake, transcellular transport, and circulation: mechanisms and challenges. Adv. Drug Deliv. Rev. 110-111, 52–64. doi: 10.1016/j.addr.2016.06.002
Kousik, S. M., Napier, T. C., and Carvey, P. M. (2012). The effects of psychostimulant drugs on blood brain barrier function and neuroinflammation. Front. Pharmacol. 3:121. doi: 10.3389/fphar.2012.00121
Koutsiaris, A. G., Tachmitzi, S. V., Batis, N., Kotoula, M. G., Karabatsas, C. H., Tsironi, E., et al. (2007). Volume flow and wall shear stress quantification in the human conjunctival capillaries and post-capillary venules in vivo. Biorheology 44, 375–386.
Kovacs, Z. I., Kim, S., Jikaria, N., Qureshi, F., Milo, B., Lewis, B. K., et al. (2017). Disrupting the blood-brain barrier by focused ultrasound induces sterile inflammation. Proc. Natl. Acad. Sci. U. S. A. 114, E75–E84. doi: 10.1073/pnas.1614777114
Krueger, M., Bechmann, I., Immig, K., Reichenbach, A., Härtig, W., and Michalski, D. (2015). Blood-brain barrier breakdown involves four distinct stages of vascular damage in various models of experimental focal cerebral ischemia. J. Cereb. Blood Flow Metab. 35, 292–303. doi: 10.1038/jcbfm.2014.199
Kuang, F., Wang, B. R., Zhang, P., Fei, L. L., Jia, Y., Duan, X. L., et al. (2004). Extravasation of blood-borne immunoglobulin G through blood-brain barrier during adrenaline-induced transient hypertension in the rat. Int. J. Neurosci. 114, 575–591. doi: 10.1080/00207450490422731
Kuczynski, A. M., Marzoughi, S., Al Sultan, A. S., Colbourne, F., Menon, B. K., van Es, A. C., et al. (2020). Therapeutic hypothermia in acute ischemic stroke—a systematic review and meta-analysis. Curr. Neurol. Neurosci. Rep. 20, 1–10. doi: 10.1007/s11910-020-01029-3
Kulkarni, S., O’Farrell, I., Erasi, M., and Kochar, M. S. (1998). Stress and hypertension. WMJ 97, 34–38.
Kullberg, B. J., Vrijmoeth, H. D., van de Schoor, F., and Hovius, J. W. (2020). Lyme borreliosis: diagnosis and management. BMJ 369:1041. doi: 10.1136/bmj.m1041
Kutuzov, N., Flyvbjerg, H., and Lauritzen, M. (2018). Contributions of the glycocalyx, endothelium, and extravascular compartment to the blood-brain barrier. Proc. Natl. Acad. Sci. U. S. A. 115, E9429–E9438. doi: 10.1073/pnas.1802155115
Labrecque, L., Smirl, J. D., and Brassard, P. (2021). Utilization of the repeated squat-stand model for studying the directional sensitivity of the cerebral pressure-flow relationship. J. Appl. Physiol. 131, 927–936. doi: 10.1152/japplphysiol.00269.2021
Lassen, N. A. (1959). Cerebral blood flow and oxygen consumption in man. Physiol. Rev. 39, 183–238. doi: 10.1152/physrev.1959.39.2.183
Lawley, J. S., Alperin, N., Bagci, A. M., Lee, S. H., Mullins, P. G., Oliver, S. J., et al. (2014). Normobaric hypoxia and symptoms of acute mountain sickness: elevated brain volume and intracranial hypertension. Ann. Neurol. 75, 890–898. doi: 10.1002/ana.24171
Lehmann, M., Regland, B., Blennow, K., and Gottfries, C. G. (2003). Vitamin B12-B6-folate treatment improves blood-brain barrier function in patients with Hyperhomocysteinaemia and mild cognitive impairment. Dement. Geriatr. Cogn. Disord. 16, 145–150. doi: 10.1159/000071002
Lerner, E. C., Edwards, R. M., Wilkinson, D. S., and Fecci, P. E. (2022). Laser ablation: heating up the anti-tumor response in the intracranial compartment. Adv. Drug Deliv. Rev. 185:114311. doi: 10.1016/j.addr.2022.114311
Lesniak, W. G., Chu, C., Jablonska, A., Du, Y., Pomper, M. G., Walczak, P., et al. (2019). A distinct advantage to Intraarterial delivery of (89)Zr-bevacizumab in PET imaging of mice with and without osmotic opening of the blood-brain barrier. J. Nucl. Med. 60, 617–622. doi: 10.2967/jnumed.118.218792
Leuthardt, E. C., Duan, C., Kim, M. J., Campian, J. L., Kim, A. H., Miller-Thomas, M. M., et al. (2016). Hyperthermic laser ablation of recurrent glioblastoma leads to temporary disruption of the peritumoral blood brain barrier. PLoS One 11:e0148613. doi: 10.1371/journal.pone.0148613
Levine, K. S., Leonard, H. L., Blauwendraat, C., Iwaki, H., Johnson, N., Bandres-Ciga, S., et al. (2023). Virus exposure and neurodegenerative disease risk across national biobanks. Neuron 111, 1086–1093 e2. doi: 10.1016/j.neuron.2022.12.029
Li, J., Bentzen, S. M., Li, J., Renschler, M., and Mehta, M. P. (2008). Relationship between neurocognitive function and quality of life after whole-brain radiotherapy in patients with brain metastasis. Int. J. Radiat. Oncol. Biol. Phys. 71, 64–70. doi: 10.1016/j.ijrobp.2007.09.059
Lidington, D., Wan, H. Y., and Bolz, S. S. (2021). Cerebral autoregulation in subarachnoid hemorrhage. Front. Neurol. 12:688362. doi: 10.3389/fneur.2021.688362
Liebmann, J., Born, M., and Kolb-Bachofen, V. (2010). Blue-light irradiation regulates proliferation and differentiation in human skin cells. J. Invest. Dermatol. 130, 259–269. doi: 10.1038/jid.2009.194
Liew, H. K., Cheng, H. Y., Huang, L. C., Li, K. W., Peng, H. F., Yang, H. I., et al. (2016). Acute alcohol intoxication aggravates brain injury caused by intracerebral hemorrhage in rats. J. Stroke Cerebrovasc. Dis. 25, 15–25. doi: 10.1016/j.jstrokecerebrovasdis.2015.08.027
Lin, E. C. (2010). Radiation risk from medical imaging. Mayo Clin. Proc. 85, 1142–1146; quiz 1146. doi: 10.4065/mcp.2010.0260
Linville, R. M., DeStefano, J. G., Sklar, M. B., Chu, C., Walczak, P., and Searson, P. C. (2019). Modeling hyperosmotic blood-brain barrier opening within human tissue-engineered in vitro brain microvessels. J. Cereb. Blood Flow Metab. 40, 1517–1532. doi: 10.1177/0271678X19867980
Linville, R. M., Komin, A., Lan, X. Y., DeStefano, J. G., Chu, C. Y., Liu, G. S., et al. (2021). Reversible blood-brain barrier opening utilizing the membrane active peptide melittin in vitro and in vivo. Biomaterials 275:120942. doi: 10.1016/j.biomaterials.2021.120942
Lionakis, M. S., and Levitz, S. M. (2018). Host control of fungal infections: lessons from basic studies and human cohorts. Annu. Rev. Immunol. 36, 157–191. doi: 10.1146/annurev-immunol-042617-053318
Lipsman, N., Meng, Y., Bethune, A. J., Huang, Y. X., Lam, B., Masellis, M., et al. (2018). Blood-brain barrier opening in Alzheimer’s disease using MR-guided focused ultrasound. Nat. Commun. 9:4529. doi: 10.1038/s41467-018-04529-6
Liu, S., Agalliu, D., Yu, C., and Fisher, M. (2012). The role of Pericytes in blood-brain barrier function and stroke. Curr. Pharm. Des. 18, 3653–3662. doi: 10.2174/138161212802002706
Ljubimova, N. V., Levitman, M. K., Plotnikova, E. D., and Eidus, L. (1991). Endothelial cell population dynamics in rat brain after local irradiation. Br. J. Radiol. 64, 934–940. doi: 10.1259/0007-1285-64-766-934
Loscher, W., and Potschka, H. (2005). Drug resistance in brain diseases and the role of drug efflux transporters. Nat. Rev. Neurosci. 6, 591–602. doi: 10.1038/nrn1728
Lu, D.-Y., Yu, W.-H., Yeh, W.-L., Tang, C.-H., Leung, Y.-M., Wong, K.-L., et al. (2009). Hypoxia-induced matrix metalloproteinase-13 expression in astrocytes enhances permeability of brain endothelial cells. J. Cell. Physiol. 220, 163–173. doi: 10.1002/jcp.21746
Lumniczky, K., Szatmári, T., and Sáfrány, G. (2017). Ionizing radiation-induced immune and inflammatory reactions in the brain. Front. Immunol. 8:517. doi: 10.3389/fimmu.2017.00517
MacDougall, J., Tuxen, D., Sale, D., Moroz, J., and Sutton, J. (1985). Arterial blood pressure response to heavy resistance exercise. J. Appl. Physiol. 58, 785–790. doi: 10.1152/jappl.1985.58.3.785
Mackowiak, P. A., Wasserman, S. S., and Levine, M. M. (1992). A critical appraisal of 98.6 degrees F, the upper limit of the normal body temperature, and other legacies of Carl Reinhold august Wunderlich. JAMA 268, 1578–1580. doi: 10.1001/jama.1992.03490120092034
Mackowiak, P. A., and Worden, G. (1994). Carl Reinhold august Wunderlich and the evolution of clinical thermometry. Clin. Infect. Dis. 18, 458–467. doi: 10.1093/clinids/18.3.458
Mainprize, T., Lipsman, N., Huang, Y. X., Meng, Y., Bethune, A., Ironside, S., et al. (2019). Blood-brain barrier opening in primary brain tumors with non-invasive MR-guided focused ultrasound: a clinical safety and feasibility study. Sci. Rep. 9:36340. doi: 10.1038/s41598-018-36340-0
Mapunda, J. A., Tibar, H., Regragui, W., and Engelhardt, B. (2022). How does the immune system enter the brain? Front. Immunol. 13:805657. doi: 10.3389/fimmu.2022.805657
Martinez-Ramirez, S., Greenberg, S. M., and Viswanathan, A. (2014). Cerebral microbleeds: overview and implications in cognitive impairment. Alzheimers Res. Ther. 6:33. doi: 10.1186/alzrt263
Martins, T., Baptista, S., Goncalves, J., Leal, E., Milhazes, N., Borges, F., et al. (2011). Methamphetamine transiently increases the blood-brain barrier permeability in the hippocampus: role of tight junction proteins and matrix metalloproteinase-9. Brain Res. 1411, 28–40. doi: 10.1016/j.brainres.2011.07.013
McDannold, N., Vykhodtseva, N., and Hynynen, K. (2008). Blood-brain barrier disruption induced by focused ultrasound and circulating preformed microbubbles appears to be characterized by the mechanical index. Ultrasound Med. Biol. 34, 834–840. doi: 10.1016/j.ultrasmedbio.2007.10.016
McMahon, D., and Hynynen, K. (2017). Acute inflammatory response following increased blood-brain barrier permeability induced by focused ultrasound is dependent on microbubble dose. Theranostics 7, 3989–4000. doi: 10.7150/thno.21630
McMahon, D., Poon, C., and Hynynen, K. (2018). “Increasing BBB permeability via focused ultrasound: Current methods in preclinical research” in Blood-brain barrier neuromethods. ed. T. Barichello (New York, NY: Humana Press)
Meixensberger, J., Dings, J., Kuhnigk, H., and Roosen, K. (1993). Studies of tissue PO2 in Normal and pathological human brain cortex. Acta Neurochir., 58–63.
Menard, C., Pfau, M. L., Hodes, G. E., Kana, V., Wang, V. X., Bouchard, S., et al. (2017). Social stress induces neurovascular pathology promoting depression. Nat. Neurosci. 20, 1752–1760. doi: 10.1038/s41593-017-0010-3
Menassa, M., Stronks, K., Khatmi, F., Roa Diaz, Z. M., Espinola, O. P., Gamba, M., et al. (2023). Concepts and definitions of healthy ageing: a systematic review and synthesis of theoretical models. EClinicalMedicine 56:101821. doi: 10.1016/j.eclinm.2022.101821
Meng, Y., Wiseman, J. A., Nemtsova, Y., Moore, D. F., Guevarra, J., Reuhl, K., et al. (2017). A basic ApoE-based peptide mediator to deliver proteins across the blood-brain barrier: long-term efficacy, toxicity, and mechanism. Mol. Ther. 25, 1531–1543. doi: 10.1016/j.ymthe.2017.03.037
Mesiwala, A. H., Farrell, L., Wenzel, H. J., Silbergeld, D. L., Crum, L. A., Winn, H. R., et al. (2002). High-intensity focused ultrasound selectively disrupts the blood-brain barrier in vivo. Ultrasound Med. Biol. 28, 389–400. doi: 10.1016/S0301-5629(01)00521-X
Mestre, H., Mori, Y., and Nedergaard, M. (2020). The Brain’s Glymphatic system: current controversies. Trends Neurosci. 43, 458–466. doi: 10.1016/j.tins.2020.04.003
Mettler, F. A. Jr., Wiest, P. W., Locken, J. A., and Kelsey, C. A. (2000). CT scanning: patterns of use and dose. J. Radiol. Prot. 20, 353–359. doi: 10.1088/0952-4746/20/4/301
Mishani, S., Belhoul-Fakir, H., Lagat, C., Jansen, S., Evans, B., and Lawrence-Brown, M. (2021). Stress distribution in the walls of major arteries: implications for atherogenesis. Quant. Imaging Med. Surg. 11, 3494–3505. doi: 10.21037/qims-20-614
Moccia, F., Antognazza, M. R., and Lodola, F. (2020). Towards novel Geneless approaches for therapeutic angiogenesis. Front. Physiol. 11:616189. doi: 10.3389/fphys.2020.616189
Montagne, A., Barnes, S. R., Sweeney, M. D., Halliday, M. R., Sagare, A. P., Zhao, Z., et al. (2015). Blood-brain barrier breakdown in the aging human hippocampus. Neuron 85, 296–302. doi: 10.1016/j.neuron.2014.12.032
Moore, M., Climie, R., Otahal, P., Sharman, J., and Schultz, M. (2021). A hypertensive response to exercise is associated with an alterated cardiovascular risk profile: a systematic review and meta-analysis. J. Hypertens. 39, E161–E162. doi: 10.1097/01.hjh.0000746172.54495.a1
Moxon, E. R., and Ostrow, P. T. (1977). Haemophilus influenzae meningitis in infant rats: role of bacteremia in pathogenesis of age-dependent inflammatory responses in cerebrospinal fluid. J. Infect. Dis. 135, 303–307. doi: 10.1093/infdis/135.2.303
Muacevic, A., Adler, J., Mohammed, L., Dhavale, M., Abdelaal, M., Alam, A., et al. (2021). Exercise-induced hypertension in healthy individuals and athletes: is it an alarming sign? Cureus 12:11988. doi: 10.7759/cureus.11988
Muldoon, L. L., Nilaver, G., Kroll, R. A., Pagel, M. A., Breakefield, X. O., Chiocca, E. A., et al. (1995). Comparison of intracerebral inoculation and osmotic blood-brain barrier disruption for delivery of adenovirus, herpesvirus, and iron oxide particles to normal rat brain. Am. J. Pathol. 147, 1840–1851.
Murugesan, N., Demarest, T. G., Madri, J. A., and Pachter, J. S. (2012). Brain regional angiogenic potential at the neurovascular unit during normal aging. Neurobiol. Aging 33, e1–e16. doi: 10.1016/j.neurobiolaging.2011.09.022
Nag, S., Manias, J. L., and Stewart, D. J. (2009). Expression of endothelial phosphorylated caveolin-1 is increased in brain injury. Neuropathol. Appl. Neurobiol. 35, 417–426. doi: 10.1111/j.1365-2990.2008.01009.x
Nakagawa, M., Matsumoto, K., Higashi, H., Furuta, T., and Ohmoto, T. (1994). Acute effects of interstitial hyperthermia on normal monkey brain--magnetic resonance imaging appearance and effects on blood-brain barrier. Neurol. Med. Chir. (Tokyo) 34, 668–675. doi: 10.2176/nmc.34.668
Nelson, G. A. (2016). Space radiation and human exposures, a primer. Radiat. Res. 185, 349–358. doi: 10.1667/RR14311.1
Neuwelt, E. A., Bauer, B., Fahlke, C., Fricker, G., Iadecola, C., Janigro, D., et al. (2011). Engaging neuroscience to advance translational research in brain barrier biology. Nat. Rev. Neurosci. 12, 169–182. doi: 10.1038/nrn2995
Nichols, P., Urriola, J., Miller, S., Bjorkman, T., Mahady, K., Vegh, V., et al. (2021). Blood-brain barrier dysfunction significantly correlates with serum matrix metalloproteinase-7 (MMP-7) following traumatic brain injury. Neuroimage Clin. 31:102741. doi: 10.1016/j.nicl.2021.102741
Nishimura, N., Schaffer, C. B., Friedman, B., Tsai, P. S., Lyden, P. D., and Kleinfeld, D. (2006). Targeted insult to subsurface cortical blood vessels using ultrashort laser pulses: three models of stroke. Nat. Methods 3, 99–108. doi: 10.1038/nmeth844
O’Keeffe, E., Kelly, E., Liu, Y., Giordano, C., Wallace, E., Hynes, M., et al. (2020). Dynamic blood-brain barrier regulation in mild traumatic brain injury. J. Neurotrauma 37, 347–356. doi: 10.1089/neu.2019.6483
Oldendorf, W. H., Cornford, M. E., and Brown, W. J. (1977). Large apparent work capability of blood-brain-barrier-study of mitochondrial content of capillary endothelial cells in brain and other tissues of rat. Ann. Neurol. 1, 409–417. doi: 10.1002/ana.410010502
Olschowka, J. A., Kyrkanides, S., Harvey, B. K., O’banion, M. K., Williams, J. P., Rubin, P., et al. (1997). ICAM-1 induction in the mouse CNS following irradiation. Brain Behav. Immun. 11, 273–285. doi: 10.1006/brbi.1997.0506
Ortiz-Prado, E., Dunn, J. F., Vasconez, J., Castillo, D., and Viscor, G. (2019). Partial pressure of oxygen in the human body: a general review. Am. J. Blood Res. 9, 1–14.
Pachner, A. R., and Steiner, I. (2007). Lyme neuroborreliosis: infection, immunity, and inflammation. Lancet Neurol. 6, 544–552. doi: 10.1016/S1474-4422(07)70128-X
Page, S., Raut, S., and Al-Ahmad, A. (2019). Oxygen-glucose deprivation/Reoxygenation-induced barrier disruption at the human blood-brain barrier is partially mediated through the HIF-1 pathway. Neuro Mol. Med. 21, 414–431. doi: 10.1007/s12017-019-08531-z
Pan, W., Barron, M., Hsuchou, H., Tu, H., and Kastin, A. J. (2008). Increased leptin permeation across the blood-brain barrier after chronic alcohol ingestion. Neuropsychopharmacology 33, 859–866. doi: 10.1038/sj.npp.1301452
Park, H. Y., Hwang, H., Park, J., Lee, S., and Lim, K. (2016). The effects of altitude/hypoxic training on oxygen delivery capacity of the blood and aerobic exercise capacity in elite athletes – a meta-analysis. J. Exerc. Nutr. Biochem. 20, 15–22. doi: 10.20463/jenb.2016.03.20.1.3
Parker, A., Fonseca, S., and Carding, S. R. (2020). Gut microbes and metabolites as modulators of blood-brain barrier integrity and brain health. Gut Microbes 11, 135–157. doi: 10.1080/19490976.2019.1638722
Patel, B., Yang, P. H., and Kim, A. H. (2020). The effect of thermal therapy on the blood-brain barrier and blood-tumor barrier. Int. J. Hyperth. 37, 35–43. doi: 10.1080/02656736.2020.1783461
Pearigen, P., Gwinn, R., and Simon, R. P. (1996). The effects in vivo of hypoxia on brain injury. Brain Res. 725, 184–191. doi: 10.1016/0006-8993(96)00215-6
Pena, L. A., Fuks, Z., and Kolesnick, R. N. (2000). Radiation-induced apoptosis of endothelial cells in the murine central nervous system: protection by fibroblast growth factor and sphingomyelinase deficiency. Cancer Res. 60, 321–327.
Pereira, A. C., Huddleston, D. E., Brickman, A. M., Sosunov, A. A., Hen, R., McKhann, G. M., et al. (2007). An in vivo correlate of exercise-induced neurogenesis in the adult dentate gyrus. Proc. Natl. Acad. Sci. 104, 5638–5643. doi: 10.1073/pnas.0611721104
Phares, T. W., Kean, R. B., Mikheeva, T., and Hooper, D. C. (2006). Regional differences in blood-brain barrier permeability changes and inflammation in the apathogenic clearance of virus from the central nervous system. J. Immunol. 176, 7666–7675. doi: 10.4049/jimmunol.176.12.7666
Pitzschke, A., Lovisa, B., Seydoux, O., Zellweger, M., Pfleiderer, M., Tardy, Y., et al. (2015). Red and NIR light dosimetry in the human deep brain. Phys. Med. Biol. 60, 2921–2937. doi: 10.1088/0031-9155/60/7/2921
Plateel, M., Teissier, E., and Cecchelli, R. (1997). Hypoxia dramatically increases the nonspecific transport of blood-borne proteins to the brain. J. Neurochem. 68, 874–877. doi: 10.1046/j.1471-4159.1997.68020874.x
Popova, S., Charness, M. E., Burd, L., Crawford, A., Hoyme, H. E., Mukherjee, R. A. S., et al. (2023). Fetal alcohol spectrum disorders. Nat. Rev. Dis. Primers. 9:11. doi: 10.1038/s41572-023-00420-x
Prados, M. D., Schold, S. C. Jr., Fine, H. A., Jaeckle, K., Hochberg, F., Mechtler, L., et al. (2003). A randomized, double-blind, placebo-controlled, phase 2 study of RMP-7 in combination with carboplatin administered intravenously for the treatment of recurrent malignant glioma. Neuro-Oncology 5, 96–103. doi: 10.1093/neuonc/5.2.96
Prakash, R., and Carmichael, S. T. (2015). Blood-brain barrier breakdown and neovascularization processes after stroke and traumatic brain injury. Curr. Opin. Neurol. 28, 556–564. doi: 10.1097/WCO.0000000000000248
Pritt, B. S., Mead, P. S., Johnson, D. K. H., Neitzel, D. F., Respicio-Kingry, L. B., Davis, J. P., et al. (2016). Identification of a novel pathogenic Borrelia species causing Lyme borreliosis with unusually high spirochaetaemia: a descriptive study. Lancet Infect. Dis. 16, 556–564. doi: 10.1016/S1473-3099(15)00464-8
Protsiv, M., Ley, C., Lankester, J., Hastie, T., and Parsonnet, J. (2020). Decreasing human body temperature in the United States since the industrial revolution. elife 9:49555. doi: 10.7554/eLife.49555
Ramirez, S. H., Potula, R., Fan, S., Eidem, T., Papugani, A., Reichenbach, N., et al. (2009). Methamphetamine disrupts blood-brain barrier function by induction of oxidative stress in brain endothelial cells. J. Cereb. Blood Flow Metab. 29, 1933–1945. doi: 10.1038/jcbfm.2009.112
Rao, X., Zhong, J., Brook, R. D., and Rajagopalan, S. (2018). Effect of particulate matter air pollution on cardiovascular oxidative stress pathways. Antioxid. Redox Signal. 28, 797–818. doi: 10.1089/ars.2017.7394
Rapoport, S. I. (2000). Osmotic opening of the blood-brain barrier: principles, mechanism, and therapeutic applications. Cell. Mol. Neurobiol. 20, 217–230. doi: 10.1023/A:1007049806660
Rapoport, S. I., Fredericks, W. R., Ohno, K., and Pettigrew, K. D. (1980). Quantitative aspects of reversible osmotic opening of the blood-brain barrier. Am. J. Phys. 238, R421–R431. doi: 10.1152/ajpregu.1980.238.5.R421
Rapoport, S. I., Hori, M., and Klatzo, I. (1972). Testing of a hypothesis for osmotic opening of the blood-brain barrier. Am. J. Phys. 223, 323–331.
Reagan, A. M., Gu, X. W., Paudel, S., Ashpole, N. M., Zalles, M., Sonntag, W. E., et al. (2018). Age-related focal loss of contractile vascular smooth muscle cells in retinal arterioles is accelerated by caveolin-1 deficiency. Neurobiol. Aging 71, 1–12. doi: 10.1016/j.neurobiolaging.2018.06.039
Rempe, R. G., Hartz, A. M. S., and Bauer, B. (2016). Matrix metalloproteinases in the brain and blood-brain barrier: versatile breakers and makers. J. Cereb. Blood Flow Metab. 36, 1481–1507. doi: 10.1177/0271678X16655551
Reneman, R. S., Arts, T., and Hoeks, A. P. (2006). Wall shear stress--an important determinant of endothelial cell function and structure--in the arterial system in vivo. Discrepancies with theory. J. Vasc. Res. 43, 251–269. doi: 10.1159/000091648
Rieke, V., and Butts Pauly, K. (2008). MR thermometry. J. Magn. Reson. Imaging 27, 376–390. doi: 10.1002/jmri.21265
Riva, C. E., Grunwald, J. E., Sinclair, S. H., and Petrig, B. L. (1985). Blood velocity and volumetric flow-rate in human retinal-vessels. Invest. Ophthalmol. Vis. Sci. 26, 1124–1132.
Rodriguez-Rodriguez, A., Egea-Guerrero, J., Murillo-Cabezas, F., and Carrillo-Vico, A. (2014). Oxidative stress in traumatic brain injury. Curr. Med. Chem. 21, 1201–1211. doi: 10.2174/0929867321666131217153310
Roovers, S., Segers, T., Lajoinie, G., Deprez, J., Versluis, M., De Smedt, S. C., et al. (2019). The role of ultrasound-driven microbubble dynamics in drug delivery: from microbubble fundamentals to clinical translation. Langmuir 35, 10173–10191. doi: 10.1021/acs.langmuir.8b03779
Rosli, D., Schnuriger, B., Candinas, D., and Haltmeier, T. (2020). The impact of accidental hypothermia on mortality in trauma patients overall and patients with traumatic brain injury specifically: a systematic review and meta-analysis. World J. Surg. 44, 4106–4117. doi: 10.1007/s00268-020-05750-5
Roy, A., Das, S., Chatterjee, I., Roy, S., and Chakraborty, R. (2022). “Anti-inflammatory effects of different dietary antioxidants” in Plant Antioxidants and Health. Reference Series in Phytochemistry. eds. H. M. Ekiert, K. G. Ramawat, and J. Arora (New York: Springer), 573–597.
Rubio-Araiz, A., Porcu, F., Perez-Hernandez, M., Garcia-Gutierrez, M. S., Aracil-Fernandez, M. A., Gutierrez-Lopez, M. D., et al. (2017). Disruption of blood-brain barrier integrity in postmortem alcoholic brain: preclinical evidence of TLR4 involvement from a binge-like drinking model. Addict. Biol. 22, 1103–1116. doi: 10.1111/adb.12376
Rupprecht, T. A., Koedel, U., Fingerle, V., and Pfister, H.-W. (2008). The pathogenesis of Lyme neuroborreliosis: from infection to inflammation. Mol. Med. 14, 205–212. doi: 10.2119/2007-00091.Rupprecht
Rzechorzek, N. M., Thrippleton, M. J., Chappell, F. M., Mair, G., Ercole, A., Cabeleira, M., et al. (2022). A daily temperature rhythm in the human brain predicts survival after brain injury. Brain 145, 2031–2048. doi: 10.1093/brain/awab466
Sahyouni, R., Gutierrez, P., Gold, E., Robertson, R. T., and Cummings, B. J. (2017). Effects of concussion on the blood-brain barrier in humans and rodents. J. Concussion 1:4518. doi: 10.1177/2059700216684518
Sajja, R. K., Rahman, S., and Cucullo, L. (2016). Drugs of abuse and blood-brain barrier endothelial dysfunction: a focus on the role of oxidative stress. J. Cereb. Blood Flow Metab. 36, 539–554. doi: 10.1177/0271678X15616978
Salehi, A., Zhang, J. H., and Obenaus, A. (2017). Response of the cerebral vasculature following traumatic brain injury. J. Cereb. Blood Flow Metab. 37, 2320–2339. doi: 10.1177/0271678X17701460
Salehpour, F., Mahmoudi, J., Kamari, F., Sadigh-Eteghad, S., Rasta, S. H., and Hamblin, M. R. (2018). Brain Photobiomodulation therapy: a narrative review. Mol. Neurobiol. 55, 6601–6636. doi: 10.1007/s12035-017-0852-4
Sándor, N., Walter, F. R., Bocsik, A., Sántha, P., Schilling-Tóth, B., Léner, V., et al. (2014). Low dose cranial irradiation-induced cerebrovascular damage is reversible in mice. PLoS One 9:e112397. doi: 10.1371/journal.pone.0112397
Sanovich, E., Bartus, R. T., Friden, P. M., Dean, R. L., Le, H. Q., and Brightman, M. W. (1995). Pathway across blood-brain barrier opened by the bradykinin agonist, RMP-7. Brain Res. 705, 125–135. doi: 10.1016/0006-8993(95)01143-9
Santisteban, M. M., Ahn, S. J., Lane, D., Faraco, G., Garcia-Bonilla, L., Racchumi, G., et al. (2020). Endothelium-macrophage crosstalk mediates blood-brain barrier dysfunction in hypertension. Hypertension 76, 795–807. doi: 10.1161/HYPERTENSIONAHA.120.15581
Sarkar, G., Curran, G. L., Sarkaria, J. N., Lowe, V. J., and Jenkins, R. B. (2014). Peptide carrier-mediated non-covalent delivery of unmodified cisplatin, methotrexate and other agents via intravenous route to the brain. PLoS One 9:e97655. doi: 10.1371/journal.pone.0097655
Schaeffer, S., and Iadecola, C. (2021). Revisiting the neurovascular unit. Nat. Neurosci. 24, 1198–1209. doi: 10.1038/s41593-021-00904-7
Schmid-Brunclik, N., Bürgi-Taboada, C., Antoniou, X., Gassmann, M., and Ogunshola, O. O. (2008). Astrocyte responses to injury: VEGF simultaneously modulates cell death and proliferation. Am. J. Phys. Regul. Integr. Comp. Phys. 295, R864–R873. doi: 10.1152/ajpregu.00536.2007
Schnell, M. J., McGettigan, J. P., Wirblich, C., and Papaneri, A. (2010). The cell biology of rabies virus: using stealth to reach the brain. Nat. Rev. Microbiol. 8, 51–61. doi: 10.1038/nrmicro2260
Schultz, M. G., Otahal, P., Cleland, V. J., Blizzard, L., Marwick, T. H., and Sharman, J. E. (2013). Exercise-induced hypertension, cardiovascular events, and mortality in patients undergoing exercise stress testing: a systematic review and meta-analysis. Am. J. Hypertens. 26, 357–366. doi: 10.1093/ajh/hps053
Schutte, A. E., Kollias, A., and Stergiou, G. S. (2022). Blood pressure and its variability: classic and novel measurement techniques. Nat. Rev. Cardiol. 19, 643–654. doi: 10.1038/s41569-022-00690-0
Schwenkenbecher, P., Pul, R., Wurster, U., Conzen, J., Pars, K., Hartmann, H., et al. (2017). Common and uncommon neurological manifestations of neuroborreliosis leading to hospitalization. BMC Infect. Dis. 17, 1–10. doi: 10.1186/s12879-016-2112-z
Semenza, G. L. (2006). Therapeutic angiogenesis: another passing phase? Circ. Res. 98, 1115–1116. doi: 10.1161/01.RES.0000223485.43020.9e
Setiadi, A., Korim, W. S., Elsaafien, K., and Yao, S. T. (2018). The role of the blood-brain barrier in hypertension. Exp. Physiol. 103, 337–342. doi: 10.1113/EP086434
Shahidi, M., Wanek, J., Gaynes, B., and Wu, T. (2010). Quantitative assessment of conjunctival microvascular circulation of the human eye. Microvasc. Res. 79, 109–113. doi: 10.1016/j.mvr.2009.12.003
Sharma, H. S., and Dey, P. K. (1986). Probable involvement of 5-hydroxytryptamine in increased permeability of blood-brain barrier under heat stress in young rats. Neuropharmacology 25, 161–167. doi: 10.1016/0028-3908(86)90037-7
Sharma, H. S., and Hoopes, P. J. (2003). Hyperthermia induced pathophysiology of the central nervous system. Int. J. Hyperth. 19, 325–354. doi: 10.1080/0265673021000054621
Sharp, C. D., Jawahar, A., Warren, A. C., Elrod, J. W., Nanda, A., and Alexander, J. S. (2003). Gamma knife irradiation increases cerebral endothelial expression of intercellular adhesion molecule 1 and E-selectin. Neurosurgery 53, 154–161. doi: 10.1227/01.NEU.0000068840.84484.DA
Shea, M., and Smart, D. (2000). Cosmic ray implications for human health. Space Sci. Rev. 93, 187–205. doi: 10.1023/A:1026544528473
Shilton, A. L., Laycock, R., and Crewther, S. G. (2017). The Maastricht acute stress test (MAST): physiological and subjective responses in anticipation, and post-stress. Front. Psychol. 8:567. doi: 10.3389/fpsyg.2017.00567
Shlosberg, D., Benifla, M., Kaufer, D., and Friedman, A. (2010). Blood-brain barrier breakdown as a therapeutic target in traumatic brain injury. Nat. Rev. Neurol. 6, 393–403. doi: 10.1038/nrneurol.2010.74
Silvestri, V. L., Henriet, E., Linville, R. M., Wong, A. D., Searson, P. C., and Ewald, A. J. (2020). A tissue-engineered 3D microvessel model reveals the dynamics of mosaic vessel formation in breast Cancer. Cancer Res. 80, 4288–4301. doi: 10.1158/0008-5472.CAN-19-1564
Snarr, B. D., Drummond, R. A., and Lionakis, M. S. (2020). It’s all in your head: antifungal immunity in the brain. Curr. Opin. Microbiol. 58, 41–46. doi: 10.1016/j.mib.2020.07.011
Solanki, S. K., Krivova, N. A., and Haigh, J. D. (2013). Solar irradiance variability and climate. Annu. Rev. Astron. Astrophys. 51, 311–351. doi: 10.1146/annurev-astro-082812-141007
Song, K. H., Harvey, B. K., and Borden, M. A. (2018). State-of-the-art of microbubble-assisted blood-brain barrier disruption. Theranostics 8, 4393–4408. doi: 10.7150/thno.26869
Sonntag, W. E., Eckman, D. M., Ingraham, J., and Riddle, D. R. (2007). “Regulation of cerebrovascular aging” in Brain aging: Models, methods, and mechanisms. ed. D. R. Riddle (Boca Raton, FL: CRC Press/Taylor & Francis)
Spindler, K. R., and Hsu, T.-H. (2012). Viral disruption of the blood-brain barrier. Trends Microbiol. 20, 282–290. doi: 10.1016/j.tim.2012.03.009
Stamatovic, S. M., Martinez-Revollar, G., Hu, A., Choi, J., Keep, R. F., and Andjelkovic, A. V. (2019). Decline in Sirtuin-1 expression and activity plays a critical role in blood-brain barrier permeability in aging. Neurobiol. Dis. 126, 105–116. doi: 10.1016/j.nbd.2018.09.006
Stendel, R., Irnich, B., al Hassan, A. A., Heidenreich, J., and Pietilae, T. (2006). The influence of ethanol on blood flow velocity in major cerebral vessels. A prospective and controlled study. Alcohol 38, 139–146. doi: 10.1016/j.alcohol.2006.06.005
Summerfield, S. G., Read, K., Begley, D. J., Obradovic, T., Hidalgo, I. J., Coggon, S., et al. (2007). Central nervous system drug disposition: the relationship between in situ brain permeability and brain free fraction. J. Pharmacol. Exp. Ther. 322, 205–213. doi: 10.1124/jpet.107.121525
Sun, N., Akay, L. A., Murdock, M. H., Park, Y., Galiana-Melendez, F., Bubnys, A., et al. (2023). Single-nucleus multiregion transcriptomic analysis of brain vasculature in Alzheimer’s disease. Nat. Neurosci. 26, 970–982. doi: 10.1038/s41593-023-01334-3
Sun, Y., Koyama, Y., and Shimada, S. (2022). Inflammation from peripheral organs to the brain: how does systemic inflammation cause Neuroinflammation? Front. Aging Neurosci. 14:903455. doi: 10.3389/fnagi.2022.903455
Sund-Levander, M., and Grodzinsky, E. (2009). Time for a change to assess and evaluate body temperature in clinical practice. Int. J. Nurs. Pract. 15, 241–249. doi: 10.1111/j.1440-172X.2009.01756.x
Swain, R. A., Harris, A. B., Wiener, E. C., Dutka, M. V., Morris, H. D., Theien, B. E., et al. (2003). Prolonged exercise induces angiogenesis and increases cerebral blood volume in primary motor cortex of the rat. Neuroscience 117, 1037–1046. doi: 10.1016/S0306-4522(02)00664-4
Sweeney, M. D., Sagare, A. P., and Zlokovic, B. V. (2018). Blood-brain barrier breakdown in Alzheimer disease and other neurodegenerative disorders. Nat. Rev. Neurol. 14, 133–150. doi: 10.1038/nrneurol.2017.188
Sweeney, M. D., Zhao, Z., Montagne, A., Nelson, A. R., and Zlokovic, B. V. (2019). Blood-brain barrier: from physiology to disease and Back. Physiol. Rev. 99, 21–78. doi: 10.1152/physrev.00050.2017
Szczepanski, A., Furie, M. B., Benach, J. L., Lane, B. P., and Fleit, H. B. (1990). Interaction between Borrelia burgdorferi and endothelium in vitro. J. Clin. Invest. 85, 1637–1647. doi: 10.1172/JCI114615
Takahashi, S., Maeda, T., Sano, Y., Nishihara, H., Takeshita, Y., Shimizu, F., et al. (2017). Takahashi. Clin. Exp. Neuroimmunol. 8, 244–254. doi: 10.1111/cen3.12398
Tang, W., Zhu, H., Feng, Y., Guo, R., and Wan, D. (2020). The impact of gut microbiota disorders on the blood-brain barrier. Infect. Drug. Resist. 13, 3351–3363. doi: 10.2147/IDR.S254403
Tetrault, S., Chever, O., Sik, A., and Amzica, F. (2008). Opening of the blood-brain barrier during isoflurane anaesthesia. Eur. J. Neurosci. 28, 1330–1341. doi: 10.1111/j.1460-9568.2008.06443.x
Thomsen, S. (1991). Pathologic analysis of photothermal and photomechanical effects of laser-tissue interactions. Photochem. Photobiol. 53, 825–835. doi: 10.1111/j.1751-1097.1991.tb09897.x
Tinken, T. M., Thijssen, D. H., Hopkins, N., Black, M. A., Dawson, E. A., Minson, C. T., et al. (2009). Impact of shear rate modulation on vascular function in humans. Hypertension 54, 278–285. doi: 10.1161/HYPERTENSIONAHA.109.134361
Toneatto, S., Finco, O., van der Putten, H., Abrignani, S., and Annunziata, P. (1999). Evidence of blood-brain barrier alteration and activation in HIV-1 gp120 transgenic mice. AIDS 13, 2343–2348. doi: 10.1097/00002030-199912030-00005
Trembath, D. G., Davis, E. S., Rao, S., Bradler, E., Saada, A. F., Midkiff, B. R., et al. (2020). Brain tumor microenvironment and angiogenesis in melanoma brain metastases. Front. Oncol. 10:604213. doi: 10.3389/fonc.2020.604213
Tufa, U., Ringuette, D., Wang, X. F., Levi, O., and Carlen, P. Two-photon microscopy imaging of blood brain barrier leakage in fetal alcohol disorder mice. Optics in the Life Sciences. Washington, DC: Optica. (2017).
Ueno, M., Sakamoto, H., Tomimoto, H., Akiguchi, I., Onodera, M., Huang, C. L., et al. (2004). Blood-brain barrier is impaired in the hippocampus of young adult spontaneously hypertensive rats. Acta Neuropathol. 107, 532–538. doi: 10.1007/s00401-004-0845-z
Ungvari, Z., Podlutsky, A., Sosnowska, D., Tucsek, Z., Toth, P., Deak, F., et al. (2013). Ionizing radiation promotes the acquisition of a senescence-associated secretory phenotype and impairs angiogenic capacity in cerebromicrovascular endothelial cells: role of increased DNA damage and decreased DNA repair capacity in microvascular radiosensitivity. J. Gerontol. Series A 68, 1443–1457. doi: 10.1093/gerona/glt057
Unterberg, A., Wahl, M., and Baethmann, A. (1984). Effects of bradykinin on permeability and diameter of pial vessels in vivo. J. Cereb. Blood Flow Metab. 4, 574–585. doi: 10.1038/jcbfm.1984.82
Urrutia, A., Rubio-Araiz, A., Gutierrez-Lopez, M. D., ElAli, A., Hermann, D. M., O’Shea, E., et al. (2013). A study on the effect of JNK inhibitor, SP600125, on the disruption of blood-brain barrier induced by methamphetamine. Neurobiol. Dis. 50, 49–58. doi: 10.1016/j.nbd.2012.10.006
Van de Beek, D., Brouwer, M. C., Thwaites, G. E., and Tunkel, A. R. (2012). Advances in treatment of bacterial meningitis. Lancet 380, 1693–1702. doi: 10.1016/S0140-6736(12)61186-6
van Leeuwen, E., Hampton, M. B., and Smyth, L. C. D. (2020). Redox signalling and regulation of the blood-brain barrier. Int. J. Biochem. Cell Biol. 125:105794. doi: 10.1016/j.biocel.2020.105794
Vanlandewijck, M., He, L., Mae, M. A., Andrae, J., Ando, K., Del Gaudio, F., et al. (2018). A molecular atlas of cell types and zonation in the brain vasculature. Nature 554, 475–480. doi: 10.1038/nature25739
Vutskits, L., and Xie, Z. (2016). Lasting impact of general anaesthesia on the brain: mechanisms and relevance. Nat. Rev. Neurosci. 17, 705–717. doi: 10.1038/nrn.2016.128
Wahl, A., and Al-Harthi, L. (2023). HIV infection of non-classical cells in the brain. Retrovirology 20:1. doi: 10.1186/s12977-023-00616-9
Walker, A. J., Ruzevick, J., Malayeri, A. A., Rigamonti, D., Lim, M., Redmond, K. J., et al. (2014). Postradiation imaging changes in the CNS: how can we differentiate between treatment effect and disease progression? Future Oncol. 10, 1277–1297. doi: 10.2217/fon.13.271
Wang, Y., Bower, B. A., Izatt, J. A., Tan, O., and Huang, D. (2007). In vivo total retinal blood flow measurement by Fourier domain Doppler optical coherence tomography. J. Biomed. Opt. 12:041215. doi: 10.1117/1.2772871
Wang, Y., Lu, A., Gil-Flamer, J., Tan, O., Izatt, J. A., and Huang, D. (2009). Measurement of total blood flow in the normal human retina using Doppler Fourier-domain optical coherence tomography. Br. J. Ophthalmol. 93, 634–637. doi: 10.1136/bjo.2008.150276
Wang, T., Town, T., Alexopoulou, L., Anderson, J. F., Fikrig, E., and Flavell, R. A. (2004). Toll-like receptor 3 mediates West Nile virus entry into the brain causing lethal encephalitis. Nat. Med. 10, 1366–1373. doi: 10.1038/nm1140
Warrington, J. P., Ashpole, N., Csiszar, A., Lee, Y. W., Ungvari, Z., and Sonntag, W. E. (2013). Whole brain radiation-induced vascular cognitive impairment: mechanisms and implications. J. Vasc. Res. 50, 445–457. doi: 10.1159/000354227
Warrington, J. P., Csiszar, A., Mitschelen, M., Lee, Y. W., and Sonntag, W. E. (2012). Whole brain radiation-induced impairments in learning and memory are time-sensitive and reversible by systemic hypoxia. PLoS One 7:e30444. doi: 10.1371/journal.pone.0030444
Wei, J., Qin, L., Fu, Y., Dai, Y., Wen, Y., and Xu, S. (2019). Long-term consumption of alcohol exacerbates neural lesions by destroying the functional integrity of the blood-brain barrier. Drug Chem. Toxicol. 45, 231–238. doi: 10.1080/01480545.2019.1681444
Welzel, G., Fleckenstein, K., Mai, S. K., Hermann, B., Kraus-Tiefenbacher, U., and Wenz, F. (2008). Acute neurocognitive impairment during cranial radiation therapy in patients with intracranial tumors. Strahlenther. Onkol. 184, 647–654. doi: 10.1007/s00066-008-1830-6
Wen, J., Ding, Y., Wang, L., and Xiao, Y. (2020). Gut microbiome improves postoperative cognitive function by decreasing permeability of the blood-brain barrier in aged mice. Brain Res. Bull. 164, 249–256. doi: 10.1016/j.brainresbull.2020.08.017
Whelton, P. K., Carey, R., Aronow, W., Casey, D., Collins, K., Dennison Himmelfarb, C., et al. (2018). Guideline for the prevention, detection, evaluation, and management of high blood pressure in adults: executive summary: a report of the American College of Cardiology/American Heart Association task force on clinical practice guidelines. Hypertension 71, 1269–1324. doi: 10.1161/HYP.0000000000000066
WHO. WHO fungal priority pathogens list to guide research, Development and public health action. Geneva: World Health Organization (2022).
Wiest, P. W., Locken, J. A., Heintz, P. H., and Mettler, F. A. (2002). CT scanning: a major source of radiation exposure. Semin. Ultrasound Ct MRI 23, 402–410. doi: 10.1016/S0887-2171(02)90011-9
Wong, A. D., Ye, M., Levy, A. F., Rothstein, J. D., Bergles, D. E., and Searson, P. C. (2013). The blood-brain barrier: an engineering perspective. Front. Neuroeng. 6:7. doi: 10.3389/fneng.2013.00007
Wormser, G. P., Bittker, S., Cooper, D., Nowakowski, J., Nadelman, R. B., and Pavia, C. (2001). Yield of large-volume blood cultures in patients with early Lyme disease. J. Infect. Dis. 184, 1070–1072. doi: 10.1086/323424
Wu, D., Chen, J., Hussain, M., Wu, L. F., Shi, J. F., Wu, C. J., et al. (2020). Selective intra-arterial brain cooling improves long-term outcomes in a non-human primate model of embolic stroke: efficacy depending on reperfusion status. J. Cereb. Blood Flow Metab. 40, 1415–1426. doi: 10.1177/0271678X20903697
Wu, X., Lu, Y., Dong, Y., Zhang, G., Zhang, Y., Xu, Z., et al. (2012). The inhalation anesthetic isoflurane increases levels of proinflammatory TNF-alpha, IL-6, and IL-1beta. Neurobiol. Aging 33, 1364–1378. doi: 10.1016/j.neurobiolaging.2010.11.002
Wu, L. F., Wu, D., Yang, T., Xu, J., Chen, J., Wang, L. L., et al. (2020). Hypothermic neuroprotection against acute ischemic stroke: the 2019 update. J. Cereb. Blood Flow Metab. 40, 461–481. doi: 10.1177/0271678X19894869
Wyss-Coray, T. (2016). Ageing, neurodegeneration and brain rejuvenation. Nature 539, 180–186. doi: 10.1038/nature20411
Xiong, Y., Mahmood, A., and Chopp, M. (2013). Animal models of traumatic brain injury. Nat. Rev. Neurosci. 14, 128–142. doi: 10.1038/nrn3407
Yang, S., Gu, C., Mandeville, E. T., Dong, Y., Esposito, E., Zhang, Y., et al. (2017). Anesthesia and surgery impair blood-brain barrier and cognitive function in mice. Front. Immunol. 8:902. doi: 10.3389/fimmu.2017.00902
Yang, A. C., Stevens, M. Y., Chen, M. B., Lee, D. P., Stähli, D., Gate, D., et al. (2020). Physiological blood-brain transport is impaired with age by a shift in transcytosis. Nature 583, 425–430. doi: 10.1038/s41586-020-2453-z
Yang, T., Velagapudi, R., Kong, C., Ko, U., Kumar, V., Brown, P., et al. (2022). Protective effects of omega-3 fatty acids in a blood-brain barrier-on-chip model and on postoperative delirium-like behaviour in mice. Br. J. Anaesth. 130, e370–e380. doi: 10.1016/j.bja.2022.05.025
Yang, A. C., Vest, R. T., Kern, F., Lee, D. P., Agam, M., Maat, C. A., et al. (2022). A human brain vascular atlas reveals diverse mediators of Alzheimer’s risk. Nature 603, 885–892. doi: 10.1038/s41586-021-04369-3
Yenari, M. A., and Hemmen, T. M. (2010). Therapeutic hypothermia for brain ischemia: where have we come and where do we go? Stroke 41, S72–S74. doi: 10.1161/STROKEAHA.110.595371
Yonemori, K., Tsuta, K., Ono, M., Shimizu, C., Hirakawa, A., Hasegawa, T., et al. (2010). Disruption of the blood brain barrier by brain metastases of triple-negative and basal-type breast cancer but not HER2/neu-positive breast cancer. Cancer 116, 302–308. doi: 10.1002/cncr.24735
Yuan, H., Gaber, M. W., McColgan, T., Naimark, M. D., Kiani, M. F., and Merchant, T. E. (2003). Radiation-induced permeability and leukocyte adhesion in the rat blood-brain barrier: modulation with anti-ICAM-1 antibodies. Brain Res. 969, 59–69. doi: 10.1016/S0006-8993(03)02278-9
Zhao, N., Kulkarni, S., Zhang, S. P., Linville, R. M., Chung, T. D., Guo, Z. B., et al. (2023). Modeling angiogenesis in the human brain in a tissue-engineered post-capillary venule. Angiogenesis 26, 203–216. doi: 10.1007/s10456-023-09868-7
Zhao, Z., Nelson, A. R., Betsholtz, C., and Zlokovic, B. V. (2015). Establishment and dysfunction of the blood-brain barrier. Cells 163, 1064–1078. doi: 10.1016/j.cell.2015.10.067
Zhu, D., Su, Y., Fu, B., and Xu, H. (2018). Magnesium reduces blood-brain barrier permeability and regulates amyloid-β transcytosis. Mol. Neurobiol. 55, 7118–7131. doi: 10.1007/s12035-018-0896-0
Keywords: blood-brain barrier, perturbations, dysfunction, brain health, neurovascular unit, brain pathologies
Citation: Zhao N, Chung TD, Guo Z, Jamieson JJ, Liang L, Linville RM, Pessell AF, Wang L and Searson PC (2023) The influence of physiological and pathological perturbations on blood-brain barrier function. Front. Neurosci. 17:1289894. doi: 10.3389/fnins.2023.1289894
Edited by:
Anuska V. Andjelkovic, University of Michigan, United StatesReviewed by:
Malgorzata Burek, Julius Maximilian University of Würzburg, GermanySvetlana M. Stamatovic, University of Michigan, United States
Copyright © 2023 Zhao, Chung, Guo, Jamieson, Liang, Linville, Pessell, Wang and Searson. This is an open-access article distributed under the terms of the Creative Commons Attribution License (CC BY). The use, distribution or reproduction in other forums is permitted, provided the original author(s) and the copyright owner(s) are credited and that the original publication in this journal is cited, in accordance with accepted academic practice. No use, distribution or reproduction is permitted which does not comply with these terms.
*Correspondence: Peter C. Searson, c2VhcnNvbkBqaHUuZWR1
†These authors have contributed equally to this work and share first authorship