- 1Department of Anesthesiology and Perioperative Medicine, University of Pittsburgh, Pittsburgh, PA, United States
- 2Department of Medicine, Division of Cardiology, University of Pittsburgh Medical Center, Pittsburgh, PA, United States
- 3Cardionomic, Incorporated, New Brighton, MN, United States
Introduction
Acute decompensated heart failure (ADHF) carries a significant burden of mortality and morbidity, with few effective treatments available (Heidenreich et al., 2022; Greene et al., 2023). Cardiopulmonary nerve stimulation (CPNS), which involves targeted electrical stimulation of specific nerves that innervate the heart, is an innovative emerging therapeutic ADHF management strategy (Petru et al., 2020; Goedeke et al., 2022; Emani et al., 2023). In a recent study, Emani et al. (2023) used the CPNS using a low-level stimulation which enhanced cardiac inotropy, decreased energy consumption, and improved patients' symptoms, function, and quality of life (Emani et al., 2023). The mechanism of action of CPNS that drove these promising results was not described by Emani et al. (2023).
This commentary aims to explore the mechanisms of CPNS, focusing on its neuromodulatory effects on the cardiac autonomic nervous system (CANS) and its potential to revolutionize ADHF therapy.
Cardiac autonomic nervous system
The CANS is a complex network of multiple layers of neural control, each hierarchically arranged to regulate cardiac function (Armour and Ardell, 2004; Hadaya and Ardell, 2020). CANS functionality is underpinned by several key layers: (1) the intrinsic cardiac nervous system (ICNS) for local control, (2) intrathoracic extracardiac ganglia, including sympathetic and superior cervical ganglia, for intrathoracic regulation, (3) the spinal cord for processing cardiac sensory information and directing sympathetic outflow, (4) the brainstem for integrating sensory information and modulating autonomic output, and (5) the higher brain centers like the cortex for overarching control (Figure 1).
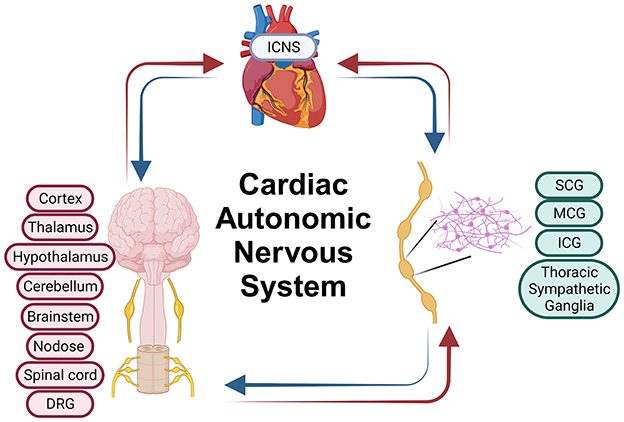
Figure 1. Cardiac autonomic nervous system. ICNS, Intrinsic Cardiac Nervous System; SCG, Superior Cervical Ganglia; MCG, Middle Cervical Ganglia; ICG, Inferior Cervical Ganglia; DRG, Dorsal Root Ganglia. Parts of this figure were created with BioRender.com.
Neuromodulation of the CANS can be achieved by altering the activity of cardiac neurons or nerves at various levels within the system, each of which can directly or indirectly influence cardiac function.
CANS stimulation
Sympathetic nerve stimulation
Activating the sympathetic nervous system releases norepinephrine (NE) in the heart, enhancing contractility, blood pressure, and heart rate. The effect varies depending on the heart region innervated. For example, stimulating the stellate ganglion, which is the fusion of the inferior cervical ganglia and thoracic 1(T1) sympathetic ganglia, on the left side primarily increases left ventricular contractility without significantly affecting heart rate, whereas right stellate ganglion stimulation predominantly elevates heart rate (Ajijola et al., 2015; Dacey et al., 2022). The ability of sympathetic nerve stimulation in altering hemodynamic parameters such as heart rate and contractility is influenced by the stimulation settings, the state of the heart, and the overall condition of the CANS. In heart failure, CANS dysfunction may alter expected responses, with the degree of response variability tied to the extent of CANS remodeling (Tjeerdsma et al., 2001; Campelo and Abreu-Lima, 2004; Hoyer et al., 2008; Kardesoglu et al., 2011; Kishi, 2012; Phillips, 2012; Ali et al., 2018; Hadaya and Ardell, 2020).
Parasympathetic nerve stimulation
Activating parasympathetic nerve fibers typically leads to bradycardia and may also lower blood pressure. The choice of stimulation parameters and the specific stimulation location are crucial, as they greatly influence the outcomes. For example, stimulating the vagus nerve, a key element of the parasympathetic nervous system, at both the cervical and heart levels leads to bradycardia. However, cervical vagus nerve stimulation is linked to various off-target effects that are absent when only the cardiac branch of the vagus nerve is stimulated (Fitchett et al., 2021). As with sympathetic stimulation, the extent of bradycardia and hemodynamic shifts induced by parasympathetic stimulation is contingent upon the heart's condition and the functional state of the CANS. This variability underscores the complex interplay between heart health and autonomic regulation.
Afferent nerve stimulation
Direct
Stimulating afferent fibers directly can yield various outcomes, dependent on the specific fiber type and stimulation intensity. These effects range from potentially cardioprotective impacts (Fallen, 2005), to adverse reactions like pain, arrhythmias, bradycardia, tachycardia, and other hemodynamic shifts. The diversity of these effects illustrates the complexity and sensitivity of afferent nerve responses to direct stimulation (Schwartz and Foreman, 1991; Chandler et al., 1993).
Indirect
The nuanced effects of indirectly modulating afferent neurons are often overlooked in neuromodulation therapies. It's crucial to recognize that the CANS operates as a closed-loop system. As such, sympathetic or parasympathetic stimulation affects more than just contractility, blood pressure, or heart rate; it also alters cardiac sensory neurons. These neurons are responsible for detecting mechanical and chemical changes in cardiac tissues and relay this sensory information throughout the CANS hierarchy for further processing (Armour, 2004; Armour and Ardell, 2004). Upon processing this sensory data, the CANS can undergo a complete state alteration. This underscores the profound impact of sensory input on the autonomic system's overall functioning, highlighting the intricate feedback mechanisms within the CANS (Armour, 2004; Armour and Ardell, 2004).
Stimulation parameters
In electrical stimulation, parameters such as frequency, amplitude, pulse width, duty cycle, polarity, burst time, and ramp time are critical in shaping therapeutic outcomes. Different stimulation parameters can result in markedly different effects. For example, low-intensity parasympathetic stimulation may offer cardioprotective benefits, whereas high-intensity stimulation of the parasympathetic nerve can precipitate arrhythmias (Ardell et al., 2017; Wang et al., 2019; Kharbanda et al., 2022). Identifying optimal stimulation parameters for maximal therapeutic effects in various cardiac diseases presents a significant challenge. There is a need for extensive translational research involving large animal models to determine these optimal parameters and to thoroughly understand the mechanisms behind a neuromodulation therapy's effectiveness.
Ganglionated plexi stimulation
The stimulation of cardiac ganglionated plexi (GPs) can lead to diverse outcomes, largely dependent on the types of neurons and nerve fibers present within these GPs. Activation of GPs containing sympathetic, parasympathetic, or local circuit neurons can result in various cardiac responses, including bradycardia, tachycardia, a combination of both, atrioventricular (AV) block, and atrial arrhythmias. This underscores the critical need for precision in targeting specific neuronal GPs in cardiac neuromodulation (Butler et al., 1990; Cardinal et al., 2009; Lim et al., 2011a,b). Stimulating multiple neuron types simultaneously within the GPs may lead to inconsistent and undesirable effects across different subjects. This variability highlights the complexity and challenges in achieving uniform therapeutic responses through GP neuromodulation (Butler et al., 1990; Cardinal et al., 2009).
CANS imbalance in HF patients
In early HF, the sympathetic nervous system becomes overly active as a compensatory response to maintain cardiac output, driven by heightened sensory signaling from cardiac afferent neurons. As HF progresses without resolution, these neurons continuously signal the CANS for increased sympathetic and reduced parasympathetic activity, leading to autonomic imbalance. This ongoing sympathetic dominance and parasympathetic dysfunction contribute to the worsening of HF, increased arrhythmias, decompensation, and mortality risk (Grassi et al., 1995; Schnabel and Bohm, 1999; Noble, 2000; Floras, 2003, 2009; Borovac et al., 2020; Gronda et al., 2022).
Cardiopulmonary nerve stimulation
Location
In human clinical studies, CPNS was delivered through a catheter equipped with a 16-electrode anchoring nitinol braid and placed within the right pulmonary artery (RPA; Figure 2). This specific location facilitated targeted stimulation of cardiac nerves in the mid- RPA region (Petru et al., 2020; Goedeke et al., 2022). The RPA, targeted in CPNS, is characterized by a complex mix of nerve structures, including various sympathetic, parasympathetic, and afferent nerves, alongside GPs. This area encompasses a variety of nerves such as the right dorsal medial cardiopulmonary nerve (CPN), right dorsal lateral CPN, right stellate CPN, CPN plexus, both superficial and deep cardiac plexus, vagal cardiac branches, inferior cervical cardiac nerves, and RPA GP (Janes et al., 1986; Murphy and Armour, 1992; Chiou et al., 1997; Kawashima, 2005; Niu et al., 2007; Wang et al., 2010; Wink et al., 2020; Zandstra et al., 2021).
CPNS effect
CPNS therapy, applied in areas densely populated with sympathetic, parasympathetic, and afferent nerves, leverages the intricate neural network of the target region to modulate cardiac function (Janes et al., 1986; Murphy and Armour, 1992; Kawashima, 2005; Wink et al., 2020; Zandstra et al., 2021). In addition, the target region for CPNS contains GPs (Chiou et al., 1997; Niu et al., 2007; Wang et al., 2010), which are home to a diverse array of neurons, including those forming local circuits (Armour, 1991; Armour et al., 1997). This variety of neurons within the GPs contributes to the nuanced and multifaceted effects of CPNS therapy. The effectiveness of CPNS is influenced by several factors, including the precise location of the electrode, stimulation parameters, patients's RPA neural anatomy, and the stage of CANS remodeling in HF patients. The positioning of the electrode in relation to specific nerves, especially those near the RPA like cardiac vagal nerves, plays a crucial role. For instance, if CPNS electrodes near cardiac vagal nerves are used, the therapy could induce bradycardia (Murphy and Armour, 1992). A multitude of afferent fibers are also present near the RPA which branch out toward either the dorsal root ganglia (DRG) or the nodose ganglia. This proximity of afferent fibers to the RPA underscores their potential involvement in the responses elicited by CPNS therapy (Janes et al., 1986; Murphy and Armour, 1992; Chiou et al., 1997; Kawashima, 2005; Niu et al., 2007; Wang et al., 2010; Wink et al., 2020; Zandstra et al., 2021). In this region, the presence of the RPA-GP or the superior vena cava-aorta (SVC-Ao) GP is notable. Stimulation of these GPs typically results in a deceleration of the sinus rate and atrioventricular conduction, demonstrating the profound impact of GP stimulation on cardiac rhythm (Cooper et al., 1980; Mick et al., 1992; Chiou et al., 1997; Lu et al., 2010). Simultaneously stimulating sympathetic, parasympathetic, and afferent fibers in CPNS can lead to non-specific CANS modulation, potentially causing issues like pain from direct nociceptive afferent stimulation or autonomic conflicts. This highlights the complexity and potential risks of CPNS, underscoring the need for precise modulation to avoid such adverse effects (Schwartz and Foreman, 1991; Chandler et al., 1993; Foreman et al., 2015; Eickholt et al., 2018; Winter et al., 2018).
The use of a catheter with multiple electrodes and the assessment of the hemodynamic response of stimulation seems to be essential in CPNS therapy for the accurate targeting of specific nerves. By selecting the electrodes adjacent to the targeted nerves, CPNS can precisely modulate nerve activity, thereby enhancing the therapy's effect and reducing the risk of non-specific modulation and related complications.
Potential mechanism of action
It has been shown that low-level CPNS could provide significant benefits to ADHF patients (Emani et al., 2023). In this commentary article, we try to discuss the potential mechanism of action of CPNS, however, to determine the exact mechanism of action of CPNS, mechanistic animal studies are needed. In the recent CPNS clinical trial, the low-intensity CPNS was performed at a cardiopulmonary sympathetic nerve which was identified prior to initiation of CPNS therapy. By this method, the CANS is stimulated at a low level which does not cause a clinically relevant acute hemodynamic response. Although the low-level CANS modulation might not cause a large acute hemodynamic response, it can cause a profound protective effect by indirect modulation of the afferent pathway (Armour and Ardell, 2004). We hypothesize that during CPNS therapy, afferent signaling is modulated either directly by direct stimulation of afferent nerve fibers or indirectly by reacting to the low-level CPNS sympathetic stimulation. The CANS controls the sympathetic and parasympathetic outflow based on the afferent/sensory input and therefore afferent modulation could cause a significant change in the whole CANS (Chen et al., 2015; van Weperen and Vaseghi, 2023).
Chronic effect
Neuromodulation therapies can provide therapeutic effects that last longer than the stimulation period as seen in the vagus nerve neuromodulation therapy in the setting of atrial fibrillation in which a 3 min vagus nerve stimulation provided more than 20 min of cardioprotective effect (Salavatian et al., 2016). The main reason for this prolonged therapeutic effect is that neuromodulation therapies aim to target the CANS imbalance, which is one of the root causes of HF progression.
Neuromodulation therapies, such as CPNS, have the potential to help the CANS exit the maladaptive state present during HF progression. This can lead to a more functional state that does not accelerate HF worsening and may even partially reverse it. The CANS is capable of adapting/changing its neural network to improve its processing function which ultimately results in an improvement of cardiac function. If the CANS, with the help of acute or chronic neuromodulation therapy, starts its own adaptive reverse remodeling process to reach a more functional state, cardiac function, and patient outcome can improve dramatically even without continuous neuromodulation therapy.
Discussion
The malfunctioning CANS is heavily remodeled in cardiac diseases such as myocardial ischemia, myocardial infarction, heart failure, and arrhythmias (Francis and Cohn, 1986; Tjeerdsma et al., 2001; Campelo and Abreu-Lima, 2004; Piepoli and Capucci, 2007; Hoyer et al., 2008; Kardesoglu et al., 2011; Phillips, 2012; van Bilsen et al., 2017; Ali et al., 2018; Hadaya and Ardell, 2020; Elia and Fossati, 2023; Kumar et al., 2023). The CPNS is capable of modulating the entire state of the CANS through both direct and indirect afferent pathway modulation that is transmitted to multiple levels of the CANS. Therefore, such profound modulation of the CANS has the potential to cause a significant improvement in a patient's trajectory by restoring the autonomic balance as seen in the low level CPNS study (Abboud, 2010; Sobotka et al., 2013; Emani et al., 2023). Neuromodulation techniques can provide cardioprotective effects including, but not limited to, reduction in cardiac arrhythmia events, improvement of cardiac function, relief of symptoms like chest pain, shortness of breath, and palpitations due to cardiac disorders, and prevention of sudden cardiac death (Salavatian and Ardell, 2018; Hadaya and Ardell, 2020). By addressing one of the root causes of HF progression, this profound CANS modulation can promote long-term cardiovascular health and reduce the risk of recurrent cardiac events, rehospitalization, and mortality.
It is also important to note that the extent of the therapeutic effect of the neuromodulation therapies varies among patients and it depends on factors like underlying cardiovascular condition, CANS condition, the neuromodulation technique and its mechanism of action, and the overall health of the patient.
Author contributions
SS: Conceptualization, Investigation, Supervision, Visualization, Writing—original draft, Writing—review & editing. JCS: Conceptualization, Funding acquisition, Investigation, Writing—original draft, Writing—review & editing. JAS: Conceptualization, Visualization, Writing—original draft, Writing—review & editing. IL: Conceptualization, Writing—original draft, Writing—review & editing. AM: Conceptualization, Writing—original draft, Writing—review & editing. JA: Conceptualization, Investigation, Writing—original draft, Writing—review & editing.
Funding
The author(s) declare financial support was received for the research, authorship, and/or publication of this article.
Conflict of interest
SS and IL have received consulting fees from Cardionomic, Inc. JCS and JAS are salaried employees with stock options of Cardionomic, Inc.
The remaining authors declare that the research was conducted in the absence of any commercial or financial relationships that could be construed as a potential conflict of interest.
The authors declare that this study received funding from Cardionomic, Inc. The funder had the following involvement in the study: providing the publication fee.
Publisher's note
All claims expressed in this article are solely those of the authors and do not necessarily represent those of their affiliated organizations, or those of the publisher, the editors and the reviewers. Any product that may be evaluated in this article, or claim that may be made by its manufacturer, is not guaranteed or endorsed by the publisher.
References
Abboud, F. M. (2010). The Walter B. Cannon Memorial Award Lecture, 2009. Physiology in perspective: the wisdom of the body. In search of autonomic balance: the good, the bad, and the ugly. Am. J. Physiol. Regul. Integr. Comp. Physiol. 298, 1449–1467. doi: 10.1152/ajpregu.00130.2010
Ajijola, O. A., Howard-Quijano, K., Scovotti, J., Vaseghi, M., Lee, C., Mahajan, A., et al. (2015). Augmentation of cardiac sympathetic tone by percutaneous low-level stellate ganglion stimulation in humans: a feasibility study. Physiol. Rep. 3:12328. doi: 10.14814/phy2.12328
Ali, A., Holm, H., Molvin, J., Bachus, E., Tasevska-Dinevska, G., Fedorowski, A., et al. (2018). Autonomic dysfunction is associated with cardiac remodelling in heart failure patients. ESC Heart Fail 5, 46–52. doi: 10.1002/ehf2.12223
Ardell, J. L., Nier, H., Hammer, M., Southerland, E. M., Ardell, C. L., Beaumont, E., et al. (2017). Defining the neural fulcrum for chronic vagus nerve stimulation: implications for integrated cardiac control. J. Physiol. 595, 6887–6903. doi: 10.1113/J.P.274678
Armour, J. A. (1991). Intrinsic cardiac neurons. J. Cardiovasc. Electrophysiol. 2, 331–341. doi: 10.1111/j.1540-8167.1991.tb01330.x
Armour, J. A. (2004). Cardiac neuronal hierarchy in health and disease. Am. J. Physiol. Regul. Integr. Comp. Physiol. 287, 262–271. doi: 10.1152/ajpregu.00183.2004
Armour, J. A., and Ardell, J. L. (2004). Basic and Clinical Neurocardiology. Oxford: Oxford University Press.
Armour, J. A., Murphy, D. A., Yuan, B. X., Macdonald, S., and Hopkins, D. A. (1997). Gross and microscopic anatomy of the human intrinsic cardiac nervous system. Anat. Rec. 247, 289–298. doi: 10.1002/(SICI)1097-0185(199702)247:2<289::AID-AR15>3.0.CO;2-L
Borovac, J. A., D'Amario, D., Bozic, J., and Glavas, D. (2020). Sympathetic nervous system activation and heart failure: current state of evidence and the pathophysiology in the light of novel biomarkers. World J. Cardiol 12, 373–408. doi: 10.4330/wjc.v12.i8.373
Butler, C. K., Smith, F. M., Cardinal, R., Murphy, D. A., Hopkins, D. A., and Armour, J. A. (1990). Cardiac responses to electrical stimulation of discrete loci in canine atrial and ventricular ganglionated plexi. Am. J. Physiol. 259, 1365–1373. doi: 10.1152/ajpheart.1990.259.5.H1365
Campelo, M., and Abreu-Lima, C. (2004). Autonomic nervous system in heart failure. Rev. Port. Cardiol. 23(Suppl.2), 49–59.
Cardinal, R., Page, P., Vermeulen, M., Ardell, J. L., and Armour, J. A. (2009). Spatially divergent cardiac responses to nicotinic stimulation of ganglionated plexus neurons in the canine heart. Auton. Neurosci. 145, 55–62. doi: 10.1016/j.autneu.2008.11.007
Chandler, M., Brennan, T., Garrison, D., Kim, K., Schwartz, P., and Foreman, R. (1993). A mechanism of cardiac pain suppression by spinal cord stimulation: implications for patients with angina pectoris. Eur. Heart J. 14, 96–105. doi: 10.1093/eurheartj/14.1.96
Chen, W. W., Xiong, X. Q., Chen, Q., Li, Y. H., Kang, Y. M., and Zhu, G. Q. (2015). Cardiac sympathetic afferent reflex and its implications for sympathetic activation in chronic heart failure and hypertension. Acta Physiol. 213, 778–794. doi: 10.1111/apha.12447
Chiou, C. W., Eble, J. N., and Zipes, D. P. (1997). Efferent vagal innervation of the canine atria and sinus and atrioventricular nodes. The third fat pad. Circulation 95, 2573–2584. doi: 10.1161/01.CIR.95.11.2573
Cooper, T. B., Hageman, G. R., James, T. N., and Waldo, A. L. (1980). Neural effects on sinus rate and atrioventricular conduction produced by electrical stimulation from a transvenous electrode catheter in the canine right pulmonary artery. Circ. Res. 46, 48–57. doi: 10.1161/01.RES.46.1.48
Dacey, M., Salahudeen, O., Swid, M. A., Carlson, C., Shivkumar, K., and Ardell, J. L. (2022). Structural and function organization of intrathoracic extracardiac autonomic projections to the porcine heart: implications for targeted neuromodulation therapy. Heart Rhythm 19, 975–983. doi: 10.1016/j.hrthm.2022.01.033
Eickholt, C., Jungen, C., Drexel, T., Alken, F., Kuklik, P., Muehlsteff, J., et al. (2018). Sympathetic and parasympathetic coactivation induces perturbed heart rate dynamics in patients with paroxysmal atrial fibrillation. Med. Sci. Monit. 24, 2164–2172. doi: 10.12659/MSM.905209
Elia, A., and Fossati, S. (2023). Autonomic nervous system and cardiac neuro-signaling pathway modulation in cardiovascular disorders and Alzheimer's disease. Front. Physiol. 14:1060666. doi: 10.3389/fphys.2023.1060666
Emani, S., Civitello, A., Tahirkheli, N. K., Edes, I. F., Libbus, I., and Spinelli, J. C. (2023). The effect of cardiac pulmonary nerve stimulation on cardiac inotropy and quality of life in patients with acute decompensated heart failure. Circulation 148(Suppl.1), 15569. doi: 10.1161/circ.148.suppl_1.15569
Fallen, E. L. (2005). Vagal afferent stimulation as a cardioprotective strategy? Introducing the concept. Ann. Noninvas. Electrocardiol. 10, 441–446. doi: 10.1111/j.1542-474X.2005.00065.x
Fitchett, A., Mastitskaya, S., and Aristovich, K. (2021). Selective neuromodulation of the vagus nerve. Front. Neurosci. 15:685872. doi: 10.3389/fnins.2021.685872
Floras, J. S. (2003). Sympathetic activation in human heart failure: diverse mechanisms, therapeutic opportunities. Acta Physiol. Scand. 177, 391–398. doi: 10.1046/j.1365-201X.2003.01087.x
Floras, J. S. (2009). Sympathetic nervous system activation in human heart failure: clinical implications of an updated model. J. Am. Coll. Cardiol. 54, 375–385. doi: 10.1016/j.jacc.2009.03.061
Foreman, R. D., Garrett, K. M., and Blair, R. W. (2015). Mechanisms of cardiac pain. Compr. Physiol. 5, 929–960. doi: 10.1002/cphy.c140032
Francis, G. S., and Cohn, J. N. (1986). The autonomic nervous system in congestive heart failure. Annu. Rev. Med. 37, 235–247. doi: 10.1146/annurev.me.37.020186.001315
Goedeke, S., Emani, S., Abraham, W. T., Brandt, M. M., and Schaefer, J. A. (2022). Cardiac pulmonary nerve stimulation [CPNS (TM)]: a novel treatment for acute decompensated heart failure. JACC Basic Transl. Sci. 7, 324–325. doi: 10.1016/j.jacbts.2022.02.015
Grassi, G., Seravalle, G., Cattaneo, B. M., Lanfranchi, A., Vailati, S., Giannattasio, C., et al. (1995). Sympathetic activation and loss of reflex sympathetic control in mild congestive heart failure. Circulation 92, 3206–3211. doi: 10.1161/01.CIR.92.11.3206
Greene, S. J., Bauersachs, J., Brugts, J. J., Ezekowitz, J. A., Lam, C. S. P., Lund, L. H., et al. (2023). Worsening heart failure: nomenclature, epidemiology, and future directions: JACC review topic of the week. J. Am. Coll. Cardiol. 81, 413–424. doi: 10.1016/j.jacc.2022.11.023
Gronda, E., Dusi, V., D'Elia, E., Iacoviello, M., Benvenuto, E., and Vanoli, E. (2022). Sympathetic activation in heart failure. Eur. Heart J. Suppl. 24, 4–11. doi: 10.1093/eurheartjsupp/suac030
Hadaya, J., and Ardell, J. L. (2020). Autonomic modulation for cardiovascular disease. Front. Physiol. 11:617459. doi: 10.3389/fphys.2020.617459
Heidenreich, P. A., Bozkurt, B., Aguilar, D., Allen, L. A., Byun, J. J., Colvin, M. M., et al. (2022). 2022 AHA/ACC/HFSA guideline for the management of heart failure: executive summary: a report of the American College of Cardiology/American Heart Association Joint Committee on Clinical Practice Guidelines. J. Am. Coll. Cardiol. 79, 1757–1780. doi: 10.1016/j.jacc.2021.12.011
Hoyer, D., Maestri, R., La Rovere, M. T., and Pinna, G. D. (2008). Autonomic response to cardiac dysfunction in chronic heart failure: a risk predictor based on autonomic information flow. Pacing Clin. Electrophysiol. 31, 214–220. doi: 10.1111/j.1540-8159.2007.00971.x
Janes, R. D., Brandys, J. C., Hopkins, D. A., Johnstone, D. E., Murphy, D. A., and Armour, J. A. (1986). Anatomy of human extrinsic cardiac nerves and ganglia. Am. J. Cardiol. 57, 299–309. doi: 10.1016/0002-9149(86)90908-2
Kardesoglu, E., Isilak, Z., Yalcin, M., and Celik, T. (2011). Autonomic nervous system in heart failure: an endless area of research/the preserved autonomic functions may provide the asymptomatic clinical status in heart failure despite advanced left ventricular systolic dysfunction. Anadolu. Kardiyol. Derg. 11:373. doi: 10.5152/akd.2011.093
Kawashima, T. (2005). The autonomic nervous system of the human heart with special reference to its origin, course, and peripheral distribution. Anat. Embryol. 209, 425–438. doi: 10.1007/s00429-005-0462-1
Kharbanda, R. K., van der Does, W. F. B., van Staveren, L. N., Taverne, Y., Bogers, A., and de Groot, N. M. S. (2022). Vagus nerve stimulation and atrial fibrillation: revealing the paradox. Neuromodulation 25, 356–365. doi: 10.1016/j.neurom.2022.01.008
Kishi, T. (2012). Heart failure as an autonomic nervous system dysfunction. J. Cardiol. 59, 117–122. doi: 10.1016/j.jjcc.2011.12.006
Kumar, H. U., Nearing, B. D., Mittal, S., Premchand, R. K., Libbus, I., DiCarlo, L. A., et al. (2023). Autonomic regulation therapy in chronic heart failure with preserved/mildly reduced ejection fraction: ANTHEM-HFpEF study results. Int. J. Cardiol. 381, 37–44. doi: 10.1016/j.ijcard.2023.03.030
Lim, P. B., Malcolme-Lawes, L. C., Stuber, T., Kojodjojo, P., Wright, I. J., Francis, D. P., et al. (2011a). Stimulation of the intrinsic cardiac autonomic nervous system results in a gradient of fibrillatory cycle length shortening across the atria during atrial fibrillation in humans. J. Cardiovasc. Electrophysiol. 22, 1224–1231. doi: 10.1111/j.1540-8167.2011.02097.x
Lim, P. B., Malcolme-Lawes, L. C., Stuber, T., Wright, I., Francis, D. P., Davies, D. W., et al. (2011b). Intrinsic cardiac autonomic stimulation induces pulmonary vein ectopy and triggers atrial fibrillation in humans. J. Cardiovasc. Electrophysiol. 22, 638–646. doi: 10.1111/j.1540-8167.2010.01992.x
Lu, Z., Scherlag, B. J., Niu, G., Lin, J., Fung, K. M., Zhao, L., et al. (2010). Functional properties of the superior vena cava (SVC)-aorta ganglionated plexus: evidence suggesting an autonomic basis for rapid SVC firing. J. Cardiovasc. Electrophysiol. 21, 1392–1399. doi: 10.1111/j.1540-8167.2010.01787.x
Mick, J. D., Wurster, R. D., Duff, M., Weber, M., Randall, W. C., and Randall, D. C. (1992). Epicardial sites for vagal mediation of sinoatrial function. Am. J. Physiol. 262, 1401–1406. doi: 10.1152/ajpheart.1992.262.5.H1401
Murphy, D. A., and Armour, J. A. (1992). Human cardiac nerve stimulation. Ann. Thorac. Surg. 54, 502–506. doi: 10.1016/0003-4975(92)90443-8
Niu, G.-D., Scherlag, B. J., Lu, Z., Ghias, M., Lazzara, R., Jackman, W. M., et al. (2007). Inducibility of atrial fibrillation in the myocardial sleeve of the superior vena cava is mediated by the ganglionated plexi on the right pulmonary artery. Am. Heart Assoc. 16:140. doi: 10.1161/circ.116.suppl_16.II_140-c
Noble, M. I. (2000). Sympathetic activation in chronic heart failure. Int. J. Cardiol. 73, 163–164. doi: 10.1016/S0167-5273(00)00212-6
Petru, J., Malek, F., Stylos, L., Goedeke, S., and Neuzil, P. (2020). Novel neuromodulation approach to improve left ventricular contractility in heart failure: a first-in-human proof-of-concept study. Circ. Arrhythm Electrophysiol. 13:e008407. doi: 10.1161/CIRCEP.120.008407
Phillips, J. K. (2012). Autonomic dysfunction in heart failure and renal disease. Front. Physiol. 3:219. doi: 10.3389/fphys.2012.00219
Piepoli, M. F., and Capucci, A. (2007). Autonomic nervous system in the genesis of arrhythmias in chronic heart failure: implication for risk stratification. Minerva Cardioangiol. 55, 325–333.
Salavatian, S., and Ardell, J. L. (2018). Neuromodulation therapies for cardiac disease. Neuromodulation 7, 1519–1530. doi: 10.1016/B978-0-12-805353-9.00129-7
Salavatian, S., Beaumont, E., Longpre, J. P., Armour, J. A., Vinet, A., Jacquemet, V., et al. (2016). Vagal stimulation targets select populations of intrinsic cardiac neurons to control neurally induced atrial fibrillation. Am. J. Physiol. Heart Circ. Physiol. 311, 1311–1320. doi: 10.1152/ajpheart.00443.2016
Schnabel, P., and Bohm, M. (1999). Sympathetic activation in heart failure: a target of therapeutic approaches. Z Kardiol 88(Suppl.3), S005–S011. doi: 10.1001/archinte.159.3.225
Schwartz, P. J., and Foreman, R. D. (1991). Cardiac pain, sympathetic afferents, and life-threatening arrhythmias. J. Cardiovasc. Electrophysiol. 2, 100–113. doi: 10.1111/j.1540-8167.1991.tb01360.x
Sobotka, P. A., Osborn, J. W., and Paton, J. F. (2013). Restoring autonomic balance: future therapeutic targets. EuroIntervention 9(Suppl.R), 140–148. doi: 10.4244/EIJV9SRA24
Tjeerdsma, G., Szabo, B. M., van Wijk, L. M., Brouwer, J., Tio, R. A., Crijns, H. J., et al. (2001). Autonomic dysfunction in patients with mild heart failure and coronary artery disease and the effects of add-on beta-blockade. Eur. J. Heart Fail 3, 33–39. doi: 10.1016/S.1388-9842(00)00119-7
van Bilsen, M., Patel, H. C., Bauersachs, J., Bohm, M., Borggrefe, M., Brutsaert, D., et al. (2017). The autonomic nervous system as a therapeutic target in heart failure: a scientific position statement from the Translational Research Committee of the Heart Failure Association of the European Society of Cardiology. Eur. J. Heart Fail 19, 1361–1378. doi: 10.1002/ejhf.921
van Weperen, V. Y. H., and Vaseghi, M. (2023). Cardiac vagal afferent neurotransmission in health and disease: review and knowledge gaps. Front. Neurosci. 17:1192188. doi: 10.3389/fnins.2023.1192188
Wang, H., Li, J., Hong, C., Liu, X., Shang, F., He, Y., et al. (2010). Epicardial ablation of right pulmonary artery ganglionated plexi for the prevention of atrial fibrillation originating in the pulmonary veins. J. Electrocardiol. 43, 367–372. doi: 10.1016/j.jelectrocard.2010.03.007
Wang, Y., Po, S. S., Scherlag, B. J., Yu, L., and Jiang, H. (2019). The role of low-level vagus nerve stimulation in cardiac therapy. Exp. Rev. Med. Dev. 16, 675–682. doi: 10.1080/17434440.2019.1643234
Wink, J., van Delft, R., Notenboom, R. G. E., Wouters, P. F., DeRuiter, M. C., Plevier, J. W. M., et al. (2020). Human adult cardiac autonomic innervation: controversies in anatomical knowledge and relevance for cardiac neuromodulation. Auton. Neurosci. 227:102674. doi: 10.1016/j.autneu.2020.102674
Winter, J., Tipton, M. J., and Shattock, M. J. (2018). Autonomic conflict exacerbates long QT associated ventricular arrhythmias. J. Mol. Cell Cardiol. 116, 145–154. doi: 10.1016/j.yjmcc.2018.02.001
Keywords: cardiopulmonary nerve stimulation, neuromodulation therapy, heart failure, cardiac autonomic nervous system, autonomic imbalance
Citation: Salavatian S, Spinelli JC, Schaefer JA, Libbus I, Mahajan A and Armour JA (2024) Cardiopulmonary nerve stimulation as a novel therapy for cardiac autonomic nervous system modulation. Front. Neurosci. 18:1377171. doi: 10.3389/fnins.2024.1377171
Received: 26 January 2024; Accepted: 16 February 2024;
Published: 28 February 2024.
Edited by:
Stephen Lewis, Case Western Reserve University, United StatesReviewed by:
Donald B. Hoover, East Tennessee State University, United StatesCopyright © 2024 Salavatian, Spinelli, Schaefer, Libbus, Mahajan and Armour. This is an open-access article distributed under the terms of the Creative Commons Attribution License (CC BY). The use, distribution or reproduction in other forums is permitted, provided the original author(s) and the copyright owner(s) are credited and that the original publication in this journal is cited, in accordance with accepted academic practice. No use, distribution or reproduction is permitted which does not comply with these terms.
*Correspondence: Siamak Salavatian, salavatian@pitt.edu