- 1Centre for Environment, Fisheries and Aquaculture Science, Lowestoft, United Kingdom
- 2TropWater, James Cook University, Townsville, QLD, Australia
- 3Collaborative Centre for Sustainable Use of the Seas (CCSUS), University of East Anglia, Norwich, United Kingdom
- 4Weymouth Laboratory, Centre for Environment, Fisheries and Aquaculture Science (Cefas), Weymouth, United Kingdom
- 5Marine Directorate of the Scottish Government, Science, Evidence, Digital and Data Portfolio, Aberdeen, United Kingdom
- 6Marine Conservation Research Group, University of Plymouth, Drake Circus, Plymouth, United Kingdom
- 7Scottish Association for Marine Science, Oban, Scotland, United Kingdom
- 8Environment Agency, Quay House, Fletton Quays, Peterborough, United Kingdom
The assessment of water quality, and in particular, of eutrophication, has been a core activity to establish, disseminate, and communicate the impact of anthropogenic influences on coastal and marine waters in the United Kingdom (UK) and globally. To date, the UK assessments of eutrophication have focused heavily on indicators, either singularly or in combination, associated with a numerical threshold, with supporting science concentrating on defining relevant thresholds and relating exceedances to management actions. However, as our understanding of the complexity of estuarine and coastal zone processes in terms of variability, time lags, ecological interactions and climate resilience has evolved, so too must the structure of our water quality assessments. This paper presents a review of existing UK eutrophication assessments, identifying what has worked and where gaps still exist, particularly as our ecosystems face rapid changes. From the gap analysis, we present a series of recommendations for future eutrophication assessments, assessing the feasibility of implementing those recommendations through consideration of effort, complexity and costs. This work presents a set of headline activities offering a renewed and revised approach to the structure of UK eutrophication assessments that will progress complex data flows, achieve enhanced alignment between directives, embed new indicators, greater understanding of ecosystem impacts and consideration of the shifting climate baseline.
1 Introduction
Excess nutrients from fertilizer application, pollution discharge, and sewage are transported from lands to oceans, impacting on coastal water quality and ecosystem health (Devlin and Brodie, 2023; Devlin et al., 2023; OSPAR, 2023; Paerl and Piehler, 2008; Painting et al., 2007; Carstensen et al., 2011). Terrestrial runoff of waters polluted with excess nutrients (primarily nitrogen and phosphorus compounds) from point sources, such as sewage treatment works (STW) discharges and aquaculture, and diffuse sources such as fertilizer losses via river discharges, have had devastating adverse effects in coastal and marine ecosystems globally (Ngatia et al., 2019; Ryther and Dunstan, 1971; Smith, 2003). Biomass production of plant matter in coastal waters is often naturally limited by the availability of nitrogen and/or phosphorus and increased anthropogenic inputs of these substances can lead to increased biomass that disturbs the natural ecological balance in marine ecosystems (de Raús Maúre et al., 2021). This disturbance, the process of eutrophication, is seen globally as one of the biggest threats to marine ecosystem health. Eutrophication, like climate change, is a cross country, cross sectoral issue with coastal regions throughout the world being impacted through the input of national and transboundary elevated nutrients (Laurent et al., 2018; Meier et al., 2019).
Eutrophication has a substantial impact on our coastal and marine systems and can limit access to ecosystem services by acting as a pressure on biodiversity and wider ecosystem approaches and industries such as shellfish harvesting and fisheries (Rhodes et al., 2017; Kermagoret et al., 2019). Even at a low level, increased nutrient loads and changing proportions of nutrients can result in changes in phytoplankton biomass and communities which can affect higher trophic level species (Duarte, 2009; Duarte et al., 2009; Carstensen et al., 2011; Frenken et al., 2023; Ibáñez et al., 2023; Dory et al., 2024). Species shifts are frequently characterized by high biomass bloom events which can have significant economic impacts as they reduce attractiveness and amenity value of coastal waters resulting in societal upset (Willis et al., 2018; Andersen et al., 2019). Increased phytoplankton biomass reduces light penetration which in turn causes habitat loss by limiting areas where seaweeds and seagrasses can grow (Carolina, 2002; Foden et al., 2005). These habitats are important for maintaining fish nursery populations and biodiverse benthic organisms. More serious eutrophication impacts involve hypoxic events which harm many organisms but are particularly damaging to sessile benthic fauna, whose loss again affects the food web and biotic water quality regulation. Extreme hypoxia and anoxia lead to a loss of both biotic and abiotic water quality regulation, as previously sequestered nutrients re-enter the water column and bacterial denitrification processes change (Best et al., 2007; Devlin and Brodie, 2023). Well-documented adverse ecological responses of increased nutrient discharge to coastal and marine waters from across the world include harmful algal bloom events (HABs) (Paerl, 2008; Glibert and Burford, 2017), changed preponderance and dominance of certain types of fast growing plankton over other, more long-lived and structural benthic primary producers (seagrass, coral, macroalgae) (Lapointe et al., 2019, 2020), the creation of hypoxia and subsequent “dead zones” (Diaz and Rosenberg, 2008) habitat degradation, and adverse changes in aquatic food webs (Carpenter et al., 1998; Gross and Hagy, 2017).
Generally, the UK eutrophication assessment approaches focus on single metrics (“indicators”) associated with a numerical threshold which are then integrated into one outcome of overall status (Best et al., 2007; Devlin et al., 2007a,b, 2009, 2012a; Greenwood et al., 2019). Historically, work has focused on the development of those thresholds, how they relate to ecosystem function and how to guide mitigative management actions from the outcomes of the assessment (Bricker et al., 2003; Bricker and Devlin, 2011; Ferreira et al., 2011). However, effective mitigation of eutrophication requires consideration of many layers of complexity, needing multiple, often cumulative actions over large spatio-temporal scales (Thornton et al., 2013). These challenges are well known with many studies recognizing the complexity of the problem due to large variations in hydrodynamics, water supply, inputs and susceptibility (Cloern, 2001; Cloern and Jassby, 2009; Duarte, 2009; Duarte et al., 2009).
As our understanding of the complexity of the coastal zone, in terms of variability, time lags, ecological interactions and resilience has evolved, so should the structure of our eutrophication monitoring and assessment. Pauly (2019) identifies the concept of shifting baselines, the phenomenon where each generation accepts the baseline as the earliest condition it experiences, with shift in baselines typically toward degradation. Our ecological systems are much different from decades ago facing multiple pressures whilst our technology to collect vast amounts of data continues to grow. Understanding this changing baseline in a complex pressure-response system requires multiple layers of information to inform and direct our understanding of what constitutes an acceptable and sustainable level of use for the marine environment. In addition, our shifting baseline needs to consider declining climate resilience, through cumulative impacts from multiple pressures, and the interactions of these pressures with increasing global temperatures (Atkins et al., 2011b; Patrício et al., 2016; Elliott et al., 2017; Laurent et al., 2018; Meier et al., 2019). Efforts to tackle eutrophication need to address the entire land-sea continuum from catchment to coast and be supported by monitoring a range of complex interactions and impacts (Thornton et al., 2013). Future approaches to eutrophication need a re-analysis of the issues, updating our frameworks and a rethinking of the complex solutions to achieve sustainable use of the marine environment.
This paper presents the outcomes of an evidence review and prioritization exercise, identifying gaps in current UK eutrophication assessment frameworks which have not fully considered how our system is changing and what is needed in future assessments to account for the complexity of the pressures that drive the impacts and the scale of potential solutions. It presents a review of historical and current eutrophication assessments that are being implemented in UK coastal and marine waters, identifying successes and challenges. The review and national consultations with eutrophication experts provided the baseline to identify five key challenges that need to be resolved for future eutrophication monitoring. We describe those challenges and outline a series of recommendations that can help achieve future success in eutrophication monitoring and assessment.
2 Methods
Part 1 of this work describes historical and current eutrophication assessment policies, identifying the aim of the assessments, the area in which it was applied, who was responsible for implementation, the structure of the assessment, and what was achieved though the implementation. This review, alongside national consultations with UK eutrophication experts provided the basis for part 2, a quantitative analysis of future eutrophication needs which were characterized into five broad thematic areas exploring future data needs, potential for further alignment, potential for new indicators, embedding greater understanding of ecosystem impacts and consideration of climate change. Part 3 presents a prioritization of the main recommendations under each theme, considering cost, feasibility and outcomes.
2.1 Review existing eutrophication assessment frameworks
We reviewed the existing environmental policies responsible for the assessment of eutrophication in the UK's coastal and marine waters, detailing their basis and implementation, overlapping spatial extents, and their component indicators and thresholds. We explored the evolution of eutrophication policy from coastal and marine areas and identify successes of both monitoring and policy implementation associated with UK eutrophication assessments. Finally, we summarized the indicators and reporting structures that form the assessment frameworks. The focus of this review was coastal to marine and freshwater eutrophication assessments were not included.
2.2. Identify key evidence gaps in current practices
Information from the review alongside national consultation with eutrophication experts across UK agencies were discussed in terms of what has worked and where gaps still exist. Topics considered essential to improve future eutrophication monitoring and assessment were characterized into five thematic areas exploring future data needs, potential for further alignment, potential for new indicators, embedding greater understanding of ecosystem impacts and consideration of climate change within eutrophication assessments. From these consultations, we identified evidence gaps within five broad themes (“data,” “alignment,” “indicators,” “ecosystem,” and “climate”) to identify knowledge and evidence needed for better informed and effective management of eutrophication (Figure 1).
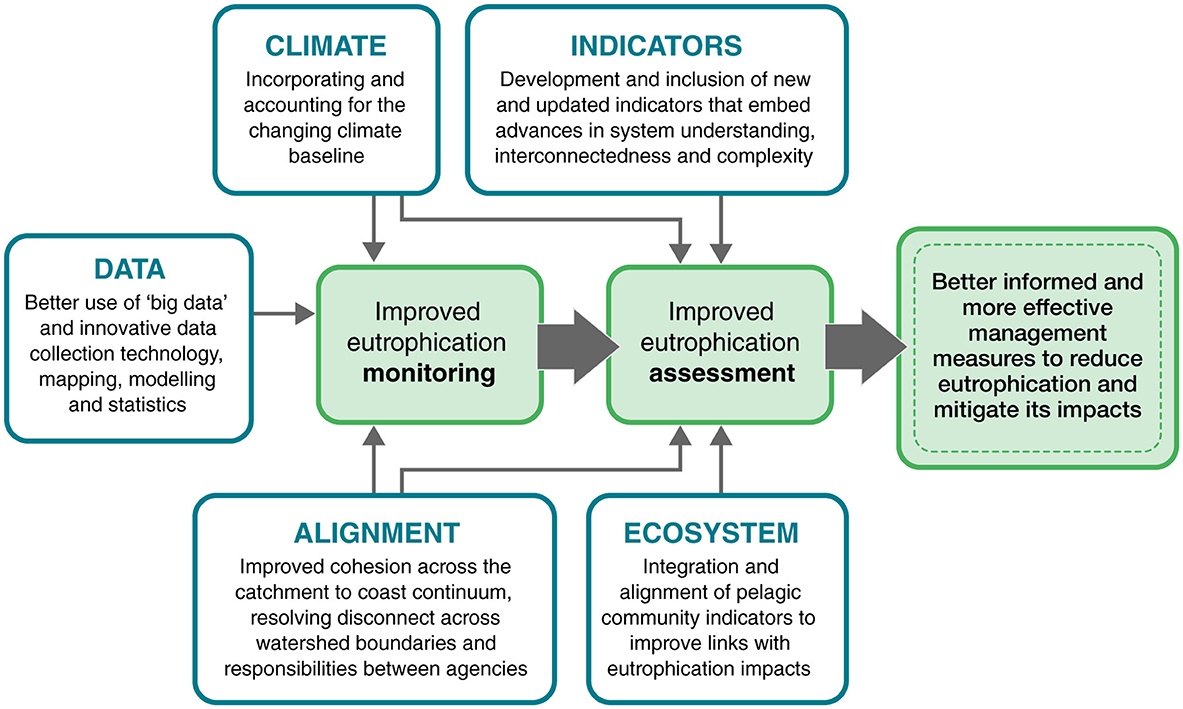
Figure 1. Five broad themes considered for the improvements of future marine eutrophication assessments.
2.3 Prioritization of activities required to fill evidence gaps
Alongside the recommendations for future eutrophication assessments, an assessment of feasibility was carried out, developed from the review and expert knowledge of what is currently occurring in UK national eutrophication monitoring programs. Feasibility of developing solutions to the key evidence gaps is reported against the effort required to achieve recommended activity, the scale of complexity and potential costs of implementation. We finish with a discussion on those recommendations and how best to achieve successful implementation of a revised, updated eutrophication monitoring and assessment strategy for coastal and marine waters.
3 Results
3.1 Review existing eutrophication assessment frameworks
3.1.1 Developing environmental directives
The design, establishment and management of eutrophication assessments in the UK have been influenced by the experience gained through the implementation of EU directives now transposed into UK law and that of the devolved administrations, and the consecutive development of monitoring to comply with the requirements of the different directives addressing eutrophication. The UK also continues to be a signatory to international conventions, in particular that of OSPAR (the Oslo and Paris Convention for the Protection of the Marine Environment of the North Atlantic). We review the application of national laws and partnerships associated with the assessment in eutrophication in UK coastal and marine waters. Figure 2 gives a timeline of the implementation of key policies and associated assessments.
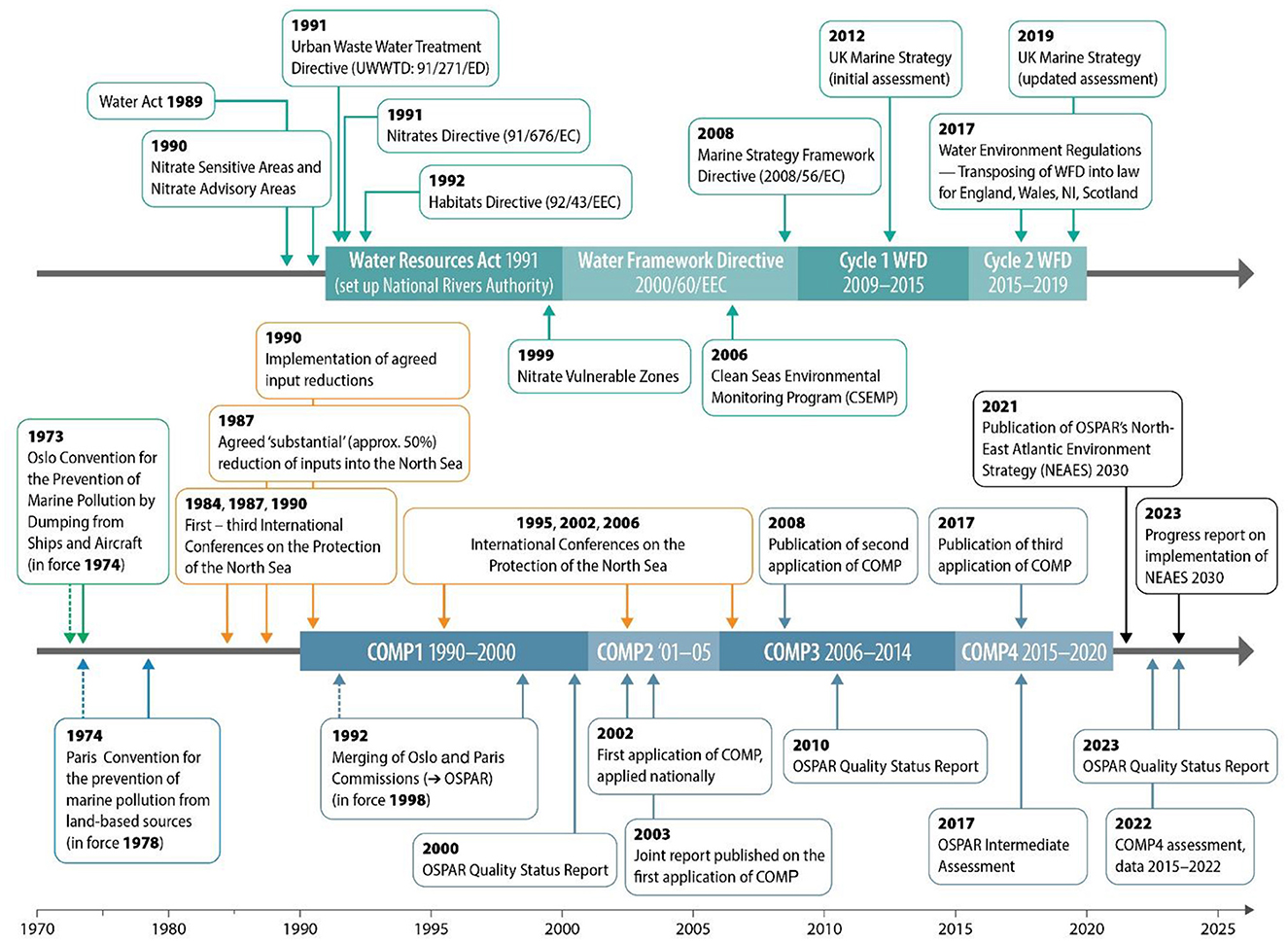
Figure 2. The evolution of eutrophication assessments for coastal and marine waters in the UK. The upper timeline focuses on the implementation of EU and national legislation with the bottom timeline showing the development of international agreements and frameworks, specifically the implementation of OSPAR.
Eutrophication emerged as a major issue in the 1970s, with concern over the input of sewage outfalls into UK rivers. Rising awareness around sewage outfalls and direct nutrient inputs meant that eutrophication from the 1970s and into the 1990s was driven by the need to mitigate point source pollution with programmes of management measures focused primarily on the reduction of phosphorus and nitrogen from sewage treatment. Much of this initial work was developed from frameworks such as the EU Urban Wastewater Treatment Directive (91/271/EEC) (European Commission, 1991a) and the EU Bathing Water Directive (2006/7/EC) (Figueras et al., 1997; European Commission, 2006). The EU Urban Wastewater Treatment Directive (91/271/ECC; hereafter UWWTD) required Member States to ensure that urban areas collect and treat wastewater which would otherwise pollute rivers, lakes and seas and succeeded at altering compliance choices and improving related national policies (Kemp, 2001). It required compliance by 1998 for wastewater treatment for all settlements of >2,000 population equivalents to have (at least) secondary treatment with more advanced treatment required for towns leading to a step change in sewage treatment from the late 1990s. Whilst the EU Bathing Water Directive was aimed at bacterial reduction, there was a positive effect of catalyzing greater nutrient reductions from STWs.
However, issues around diffuse runoff from farming and agricultural practices through the 1980s and the 1990s that were not mitigated by the UWWTD resulted in the parallel development of polices aimed at the reduction of nutrient runoff from agriculture. The implementation of European directives continued with the onset of the Nitrates Directive (91/676/ECC), which aimed to protect water quality by preventing nitrates from agricultural sources that pollute ground and surface waters and by promoting the use of good farming practices (European Commission, 1991b; Massarelli et al., 2021). The Nitrates Directive was a major step toward acknowledging and protecting water against pollution from agriculture (Tunney, 1994). The implementation of Nitrogen Vulnerable Zones (NVZs) provides an example of a catchment-based program to reduce excess groundwater nitrogen (Smith, 2000; Johnson et al., 2007; Worrall et al., 2009) where areas of elevated nitrate sensitivity were identified and, in these areas, limits on nitrate additions were imposed.
The assessment of the impact of eutrophication in the UK for estuaries and coastal waters commenced with the EU Water Framework Directive (2000/60/EC; WFD) from 2001. This covers transitional and coastal water extending for one nautical mile (1 nm) beyond the low water mark for England, Wales and Northern Ireland and 3 nm from the “coastal baseline” in Scotland (European Commission, 2000). Between 2006 and 2008, all agencies collaborated formally to develop the WFD tools and methods for the initial assessments. The EU WFD was developed as a river basin programme, connecting upstream activities with downstream ecological health. Eutrophication assessments in coastal and offshore waters (beyond the WFD waters) were also developed from the requirements of the EU Marine Strategy Framework Directive (2008/56/EC; MSFD) (European Commission, 2008). The overarching aim of the MSFD was to implement measures to achieve Good Environmental Status (GES) by 2020. First, Member States developed marine strategies consisting of an initial assessment of environmental status, along with articulating the defining characteristics, targets and indicators representing Good Environmental Status (GES). Monitoring programmes to measure progress toward GES were then established, and, where necessary, programmes of measures to achieve or maintain GES were implemented. Implementation of the WFD and MSFD resulted in shared knowledge between participating countries, development of national indicators, and a robust monitoring framework that allowed tracking of the reduction of eutrophication pressures and impacts (Borja, 2005; Devlin et al., 2007a).
Additional marine eutrophication assessments are carried out under OSPAR, the mechanism by which 15 Governments and the EU cooperate to protect the marine environment of the North-East Atlantic. OSPAR started in 1972 with the Oslo Convention against dumping and was broadened to cover land-based sources of marine pollution and the offshore industry by the Paris Convention of 1974. These two conventions were unified, updated and extended by the 1992 OSPAR Convention. Eutrophication assessments are carried out under the OSPAR “common procedure” (OSPAR COMP) (Devlin et al., 2023). The OSPAR eutrophication assessment was implemented for OSPAR COMP1 (1996–2000) and OSPAR COMP2 (2006–2014) with OSPAR COMP3 (2013–2018) providing an updated Common Assessment Criteria for the Eutrophication status of the OSPAR Marine Area. The most recent “OSPAR COMP4” has recently been concluded and provides an updated eutrophication thematic assessment (2019–2023) for 15 countries across the North-East Atlantic (Devlin et al., 2023).
Brexit and departure from EU legislation has meant several changes to the UK environmental policies which were responsible for the assessment of eutrophication in coastal and marine waters. The WFD was transposed into separate environmental legislation for England, Wales, Northern Ireland and Scotland so that supporting pieces of water legislation would continue to operate after EU exit (1 January 2021). These are the Water Environment (Water Framework Directive) (England and Wales) Regulations, the Water Environment and Water Services (Scotland) Act 2003 (WEWS Act 2017) and The Water Environment (Water Framework Directive) Regulations (Northern Ireland) 2017. For the purposes of this paper, the different regional approaches will be called WFD/WER—representing the three regional approaches for the Water Environment Regulation and Water Framework Directive across the UK, which is still the primary environmental directive that assesses transitional and coastal waters in the UK For coastal and offshore waters, the EU MSFD was transposed into national legislation through the Marine Strategy Regulations 2010 (covering England, Scotland, Wales and Northern Ireland) (DEFRA, 2012, 2019) and now sits within the UK Marine Strategy (UKMS). The DEFRA (2012) required action to achieve or maintain GES in our seas by 2020. The Regulations require the production of a “Marine Strategy” for all UK waters and that the approach is coordinated across all four UK Administrations and cooperate with other countries sharing our seas. This has resulted in the UK Marine Strategy Part One: an assessment of marine waters, objectives for GES and targets and indicators to measure progress toward GES (DEFRA, 2012, 2019) with the third report recently published (DEFRA, 2025). Eutrophication assessments under the recent UK Marine Strategy Part 1 include the coastal assessments carried out under WFD/WER and the coastal and offshore assessments carried out under the OSPAR thematic assessment (COMP4) with the two source assessments merging outcomes together but not fully integrating the source data.
3.1.2 Eutrophication indicators for UK monitoring and assessment
The type of indicators that make up a eutrophication assessment framework vary depending on the specific assessment, though many commonalities exist between eutrophication directives. The assessment of eutrophication usually includes (at least) three indicators: dissolved inorganic nutrients (nitrogen and phosphorus), phytoplankton biomass (typically measured as chlorophyll-a) and dissolved oxygen (Devlin et al., 2011, 2007a; Greenwood et al., 2019; Scavia and Bricker, 2006; Smith and Schindler, 2009; Van Beusekom et al., 2019).
Eutrophication has been defined as “the progressive enrichment of nutrients, leading to excessive plant growth,” and developed tools to classify nutrient and plant status (COM, 2017). Eutrophication may be present if one of the sensitive biological elements (particularly phytoplankton or opportunistic green algae) are moderate or worse and the supporting element of nutrients (DIN in marine waters) is also moderate or worse. These conditions should be persistent over a period of assessment cycles and investigations suggest that the causes of failure are strongly linked to nutrients. The overall eutrophication assessment is based on this evidence and other supporting parameters.
The assessment of eutrophication in the recent OSPAR eutrophication thematic assessment is based on the degree of nutrient enrichment (Category I), the direct effects of nutrient enrichment (Category II) and the indirect effects of nutrient enrichment (Category III). For Category I, the nutrients common indicator is derived from winter mean concentrations of dissolved inorganic phosphorus (DIP) and dissolved inorganic nitrogen (DIN) (Heyden and Leujak, 2022). For Category II, the Chlorophyll common indicator is derived from growing-season mean concentrations of chlorophyll (Prins and Enserink, 2022) where chlorophyll EO and water sample data are combined, weighted as a function of in-situ confidence. For Category III, the Dissolved Oxygen common indicator assesses the concentrations of dissolved oxygen near the seafloor (Devlin et al., 2022). Assessment criteria and their corresponding area-specific assessment levels as set and agreed for COMP4 are applied for each given area. The results obtained are integrated to give the classification for the given area. The overall result of the assessment depends on the outcome of the direct and indirect effects (Categories II, III), following the one-out-all-out principle (OOAO) (Devlin et al., 2011; Ferreira et al., 2011). The UK Marine Strategy is a combination of WFD/WER and OSPAR methodology (Devlin et al., 2023).
We present a comparison of the indicators across the three main UK eutrophication directives within each category in Table 1, summarizing key eutrophication indicators, assessment criteria, and geographical coverage across these frameworks. Indicators are grouped into “Category I” which relate to causative factors including nutrient loads and nutrient enrichment, “Category II” which include the direct effects of nutrient enrichment (impacts on phytoplankton biomass and community), and “Category III” which include the indirect effects of nutrient enrichment (reduction in light penetration and dissolved oxygen concentration). The WFD/WER nutrient assessment is an aggregation of two indicators (Winter DIN and Winter DIP), whilst the OSPAR assessment is based on Winter DIN and Winter DIP measured separately. The WFD/WER uses the outcome of the nutrient indicators to formally identify high risk areas for eutrophication while the nutrient indicators are not considered in the final OSPAR assessment outcome, and assessment areas that have failed nutrient indicators under the UKMS have been designated as only partially meeting GES. There are also differences between the chlorophyll and dissolved oxygen indicators and how they are used in the final assessments within WFD/WER and OSPAR. Again, these are small but significant, and result in multiple differences between the two approaches for the final assessment of eutrophication (Greenwood et al., 2019).
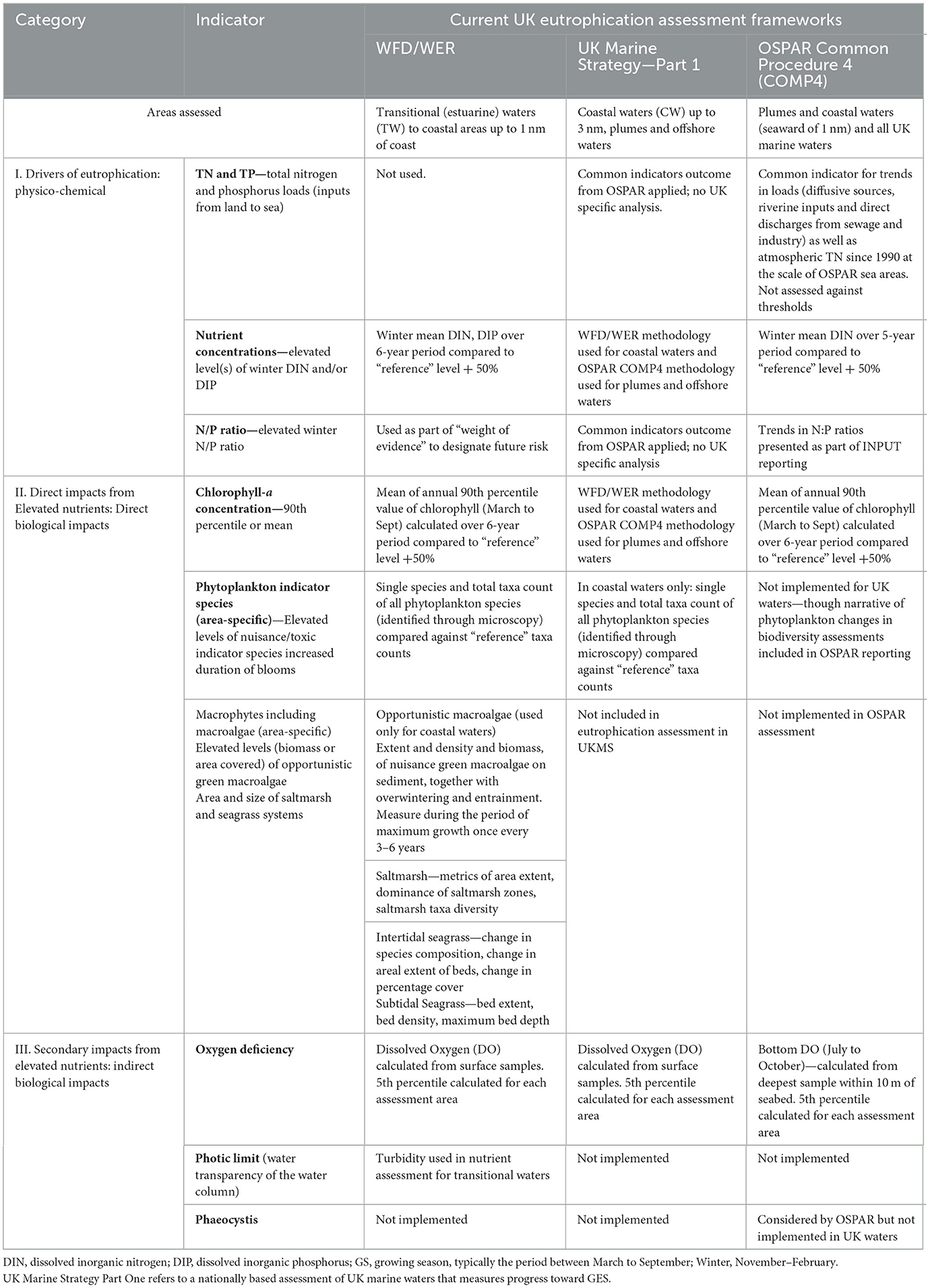
Table 1. Summary of current UK eutrophication indicators used to inform each indicator within the three UK frameworks [Water Framework, Water Framework Directive Regulations (WFD/WER for England, Wales), or Water Framework Directive (Northern Ireland, Scotland)], UK Marine Strategy and the OSPAR Common Procedure (COMP).
3.1.3 Designating eutrophication status
Indicators are assessed by comparison to their respective thresholds; however, outcomes are classified differently under each separate eutrophication frameworks. The WFD/WER eutrophication assessment includes five assessment outcome categories with “high” the most positive and “poor and bad” indicating the most perturbed, and “moderate” and “good” designating somewhat negative and somewhat positive intermediate states, respectively. Geographically specific “reference conditions” (and class boundary thresholds) for each indicator under WFD/WER were constructed based on a combination of scientific review (Borja et al., 2004), thresholds accepted under previous directives and international agreements (e.g. OSPAR, Foden et al., 2011; Devlin et al., 2007a; Painting et al., 2007), expert knowledge and investigations of outputs between water bodies at low and high risk of eutrophication (Devlin et al., 2007b). Thresholds were also validated and modified by the consensus process of the first phase of the NE Atlantic Intercalibration process (Heiskanen and Carletti, 2009).
OSPAR designates spatial assessment areas as “problem” or “non-problem” areas with respect to eutrophication. In the first three OSPAR COMPs, OSPAR Contracting Parties evaluated the eutrophication status of their national marine waters using national assessment levels (thresholds) to assess nutrients and chlorophyll a (OSPAR, 2017). For OSPAR, assessment levels that were indicative of good ecological status (non-problem area) were based on reference conditions reflecting non-eutrophic conditions with boundary between “good” and “moderate” status derived by adding a 50% deviation to the reference condition. However, OSPAR Contracting Parties used different approaches in establishing reference conditions to derive these values, leading to variable assessment levels and different outcomes of the eutrophication assessment across national borders (Malcolm et al., 2002; OSPAR, 2003, 2008a, 2017; Foden et al., 2011). OSPAR COMP4 improved this through the development of an ensemble model approach used to derive pre-eutrophic conditions (Lenhart et al., 2010; van Leeuwen et al., 2023). Nutrient loads into the NE Atlantic were estimated from rivers under reference conditions, using the European model E-HYPE and observations (Lenhart et al., 2010; Stegert et al., 2021). The chlorophyll concentrations were modeled corresponding to the estimated nutrient concentrations under reference conditions. To allow for natural variability with a “slight disturbance,” and in the absence of more specific information, the assessment level was defined as the concentration 50% above the area-specific background concentration derived from the ensemble model approach (Borja, 2005; OSPAR, 2003, 2008b). The UK Marine Strategy uses a combination of WFD/WER and OSPAR indicators and thresholds for coastal and offshore areas and applies the terminology “in/achieving GES” and “not in GES” for each indicator.
While the different directives for the assessment of eutrophication are somewhat compatible regarding the type of indicators and how they are assessed relative to thresholds, the integration frameworks, how individual indicator outcomes are brought together to provide a classification of eutrophication, status depends on what elements/indicators are used for the assessment. WFD/WER implements a “one out all out” (OOAO) process which takes the lowest value of biological elements (phytoplankton, macroalgae), while the most recent OSPAR common procedure (COMP4) used the lowest value of the chlorophyll and dissolved oxygen indicators, and the UK Marine Strategy (Part 1) combines outputs from both. Nutrients in all three directives cannot, on their own, designate a “failure” if the biological elements do not also “fail.” A failure is when a waterbody is designated as less than moderate status under the WFD/WER or not meeting good environmental status (GES) under UK Marine Strategy (Part 1) and OSPAR. However, failing nutrients can cause potential risk, and is designated as a “potential problem area” under OSPAR or high risk of future failures under the recent UK Marine Strategy (Part 1) and is identified in the WFD/WER as failing physico-chemical standards.
3.1.4 From “increased growth” to “changes to the balance of organisms”
In two cases (ECJ, 2004, 2009) the European Court of Justice ruled that the definition of eutrophication in the UWWTD must take account of “significant harmful effects of the accelerated growth of algae and higher forms of plant life resulting from discharges of urban wastewater,” that include “an undesirable disturbance of the balance of organisms present in the water.” However, because of lack of agreement amongst OSPAR signatories about adding indicators of phytoplankton community composition, the OSPAR Common Procedure has remained focused on the bulk variables, chlorophyll concentration and oxygen deficiency, as the key common indicators of the direct and indirect biological impacts associated with eutrophication of the water column.
This was also the case for the eutrophication Qualitative Descriptor (QD) of the EU Marine Strategy Framework Directive (EU MSFD), as interpreted by the European Commission (COM, 2017). Instead, what we understand as the health, good functioning, and balance of organisms of the plankton in the pelagic habitats, and which COM (2017) refers to as “the essential features and characteristics and current environmental status of marine waters” in relation to the EU MSFD's aim to achieve Good Environmental Status, was assigned (Borja et al., 2010) to the Biodiversity QD and the Food-Webs QD. In a separate process from OSPAR COMP4, Biodiversity and Food-Web indicators have also been assessed by OSPAR in a recent Quality Status Report (QSR) on the pelagic habitats (PH) of the North-East Atlantic (McQuatters-Gollop et al., 2022; Holland et al., 2023a,b). The relevant “PH” indicators PH1/FW5, based on the abundances of plankton lifeforms and Food-Webs, PH2 (also used for Food-Webs) based on biomass at each trophic level, and PH3, based on numerical biodiversity. There is continuing work to disentangle the effects of nutrient enrichment, fisheries and climate change on the PH1 indicators (McQuatters-Gollop et al., 2019; Holland et al., 2024).
There would, thus, seem to be two options for conceptualizing the changes in pelagic habitats/ecosystem state associated with eutrophication. One option is to understand eutrophication as being the increased production of organic matter and changes in the balance of organisms in pelagic habitats are seen as a consequence rather than a part of eutrophication: the view currently taken by the EU in the MSFD (Borja et al., 2010; COM, 2017) and by OSPAR, the latter having separate strategies for eutrophication and biodiversity. The other option is to see nutrient enrichment as one of several anthropogenic pressures on pelagic habitats, leading to perturbations of their normal functioning which may lead to regime shift (Tett et al., 2007, 2013; Gowen et al., 2012) as the most significant of the “undesirable disturbances” that according to the ECJ make up the final component of the eutrophication process.
3.2 Identify key evidence gaps in current practices
3.2.1 Recognizing what has worked is the first step
There have been many successes associated with the implementation of the UK eutrophication assessments, leading to positive program of measures and the reduction of nutrient inputs from both direct and diffuse sources. Starting as early as 1988, the OSPAR Contracting Parties agreed to reduce nutrient emissions to the Greater North Sea by 50%. Since then, several OSPAR Recommendations, land management initiatives and controls from regulations such as the Urban Wastewater Treatment Directive (UWWTD) (Council Directive 91/271/EEC) and WFD/WER have been taken to combat eutrophication. These include measures targeting diffuse run-off from land, atmospheric nitrogen emissions, wastewater, and other point sources. These responses have led to significant improvements in nutrient loadings to the UK marine waters since the start of monitoring in 1990. On a European scale, many measures to reduce inputs have been implemented by European Union directives covering wastewater treatment, nitrates in agriculture, industrial emissions and water and marine management with dramatic improvements in the form of atmospheric nitrogen input reductions and a reduction in fertilizer use, since 1990 (OSPAR, 2023). The four applications of the Common Procedure have revealed a steadily improving trend in the eutrophication status of OSPAR Regions II, III, and IV. Success can also be measured by the application of the eutrophication assessments, with detailed assessments completed by regulatory agencies increasing our knowledge of high-risk areas, identifying where and how programmes of measures can be implemented.
However, during the last decade, improvement trends have slowed down, with heightened concerns regarding increasing nutrient inputs from diffuse agricultural loads, combined sewage overflows and the growing marine aquaculture industry.
The application and outcomes of these assessments, whilst identifying areas that are at risk from eutrophication, can and should be improved. The original assessments were set up to define eutrophication through a set of indicators, with thresholds that were developed on historical understanding and expert knowledge. This is an evolving area, and our understanding of what is required into the future to ensure our understanding of eutrophication, to fully utilize the many emerging data sources, to look beyond simple indicators of state, and to account for climate change, requires adaptation and updating of our current methods. We present a series of recommendations around the improvement of data, alignment between different UK assessment frameworks, updating and improving eutrophication indicators, embedding ecosystem indicators within the eutrophication assessment and consideration of the interactions between climate and eutrophication (Figure 1). These recommendations set out priority areas for future assessments which are then assessed considering cost, feasibility and outcomes.
3.2.2 Improving data flows
3.2.2.1 Autonomous and remotely collected data
Our long-term monitoring datasets are the critical baselines from which we have developed and continue to develop our understanding of the changes in our coastal and marine systems. However, it is not feasible or affordable to collect all data all the time. The way we collect eutrophication assessment data is evolving, from traditional vessel-based sampling data (in-situ sampling and ex-situ analysis) to include autonomous data from in-situ sensors, satellites, and biogeochemical models (Bean et al., 2017). Furthermore, machine learning and artificial intelligence are changing the way we collect, analyse and report data within eutrophication assessments (Borja et al., 2024). There will always be a need to continue long-term, traditional in-situ sampling alongside the collection of new data types to ensure continuity of data collections for analysis and identification of trends and validation of the high frequency data sets (Addison et al., 2018; Mack et al., 2020; Holland et al., 2025), but as our technology changes, so must our approach to data streams, data repositories and assessments. We must consider data processes that can fully integrate novel and high frequency data into our statutory monitoring programs to improve understanding of complex coastal and marine processes (Dafforn et al., 2016; Addison et al., 2018) whilst ensuring robust validation of these new technologies (García-García et al., 2019; Holland et al., 2025).
High frequency and autonomous data collected and analyzed in-situ and instruments deployed from ships, continuously running on ships, and deployed on buoys is still not readily used in current assessments, despite increasing the data frequency substantively for some water quality parameters. Nutrient data rely predominantly on in-situ samples, with buoy-deployed sensors providing additional high-temporal resolution data in limited areas (Figure 3). Bottom-water oxygen concentrations also rely predominantly on in-situ data but have more recently been supplemented with ship-deployed profiler sensor data significantly increasing the spatial and temporal data coverage (Greenwood et al., 2010; Hull et al., 2020, 2021). Chlorophyll is measured from water samples as well as sensors, with increased spatial and temporal resolution resulting from “FerryBox” data: an automated flow through system which continuously measures surface water concentrations aboard the ship, and from remote sensing data (Petersen et al., 2003, 2008; Harvey et al., 2015; Bean et al., 2017; El Serafy et al., 2023).
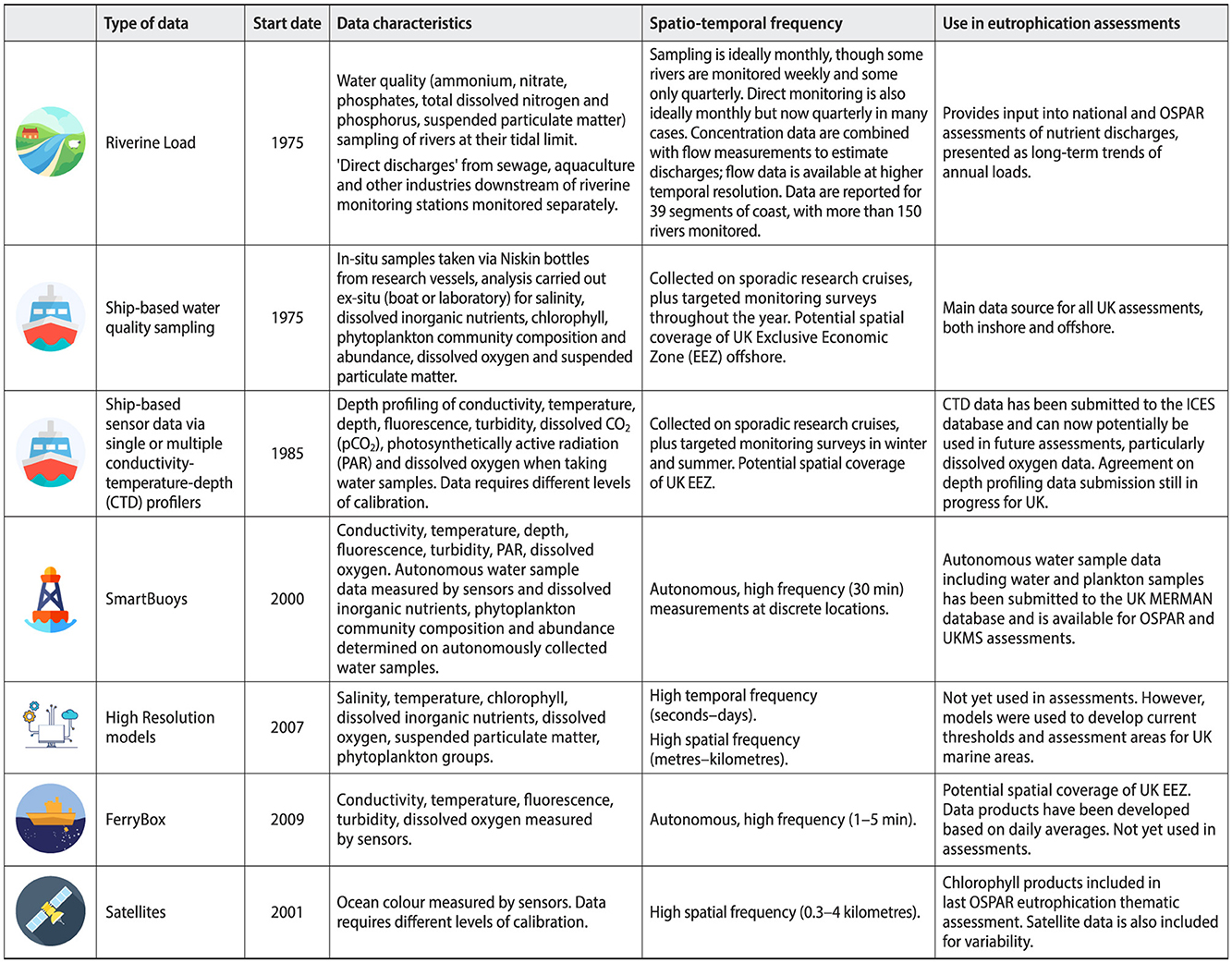
Figure 3. Different types of data collected in the UK Eutrophication monitoring programs describing the resolution, data characteristics and if the data has been used in UK Eutrophication assessments.
Remote sensing data can provide a valuable source of monitoring data, that includes chlorophyll, measures of turbidity and primary productivity and ecological health (Devlin et al., 2013, 2015; Petus et al., 2014, 2016, 2019; Capuzzo et al., 2018; Patricio-Valerio et al., 2022; Mohseni et al., 2022). Satellite earth observation data for chlorophyll were used for the first time in the recent OSPAR eutrophication thematic assessment (Devlin et al., 2023; van Leeuwen et al., 2023) combining data from in-situ sampling with higher frequency and higher spatial resolution data from remote sensing chlorophyll data (Lavigne et al., 2021) with outcomes transferred into the upcoming 2025 UK Marine Strategy assessments for marine waters (Figure 3). However, remote sensing (satellite) data has not yet been included in WFD/WER assessments due to higher uncertainties in nearshore waters, which are particularly important for eutrophication, and have observation gaps related to cloud cover (Klemas, 2011; Wei et al., 2020). Biogeochemical modeling data can also provide a potential means of supplementing limited observation data including for nutrients and oxygen, but uncertainties and model biases have prevented modeling data from being included in assessments, though some models have been invaluable for setting historical thresholds (Stegert et al., 2021; van Leeuwen et al., 2023). Full integration of high frequency data within relevant assessment areas will improve detection of when and where changes in eutrophication status are occurring.
Whilst frequency of data collection is increasing in some areas due to improved technology, some aspects of the UK's eutrophication monitoring programme are suffering significant reductions related to decreasing sampling-based monitoring in response to budget reductions. For example, the number of offshore samples taken for nutrient concentrations during the winter months which make up the eutrophication assessment period has been decreasing over the last 10 years, given the costs associated with large field programs and offshore sampling. In situ vessel sampling is resource intensive and requires ship-based sampling during logistically challenging bad weather months in winter. Sensor data for nutrients tends to be spatially limited to inshore sites and (for UK) at three autonomous sites (Mills et al., 2003, 2004). However, satellite and modeling data offers a new source of data for offshore waters and are already becoming an invaluable source of data for coastal and marine waters.
3.2.2.2 Monitoring inputs from land to sea
Accurate and timely information on nutrient concentrations and nutrient loads is integral to strategies designed to improve human wellbeing and manage the underlying drivers of water quality impairment and inform program of management measures (Joo et al., 2012; Pellerin et al., 2016). UK input monitoring for nitrogen and phosphorus has decreased in recent years, particularly in England and Scotland. For OSPAR, this monitoring consists of riverine inputs as well as “direct discharges” from sewage, industry and marine aquaculture (Axe et al., 2022) and aims to capture 90 % of inputs (Joo et al., 2012; Pellerin et al., 2016). Reductions in data frequency can impact our collective ability to understand if river systems are changing.
Recent studies have identified a common problem for many coastal waters, where abatement of phosphorus loads has occurred at a much faster rate than nitrogen abatement and mitigation (Lu and Tian, 2017; Ngatia et al., 2019; Devlin and Brodie, 2023). This has led to imbalanced nutrient ratios, where rivers and coastal systems are experiencing reductions associated with phosphorus but stabilization and/or or increases in nitrogen most likely due to increases in diffuse N from agriculture and direct sewage inputs. These nutrient imbalances can impact on plankton communities in coastal waters (Romero et al., 2012) but limited monitoring of nutrient loads and concentrations leads to a lack of understanding of the extent of this issue and coupled with changing climate and shifts in seasonality means that we may not be tracking these changes with sufficient confidence in our data.
The recent OSPAR eutrophication thematic assessment also identified issues around the monitoring of aquaculture loads, as the extent of the growth in aquaculture within the OSPAR Maritime Areas has been substantial (Axe et al., 2022; OSPAR, 2023). A gap exists concerning the agreement of minimum environmental standards for aquaculture across the OSPAR Maritime Area which is relevant to UK waters given the rise of aquaculture in many coastal areas. A more strategic approach to monitoring nutrient inputs could rationalize the collection of high-density data to focus on a few rivers, sub-catchments, and transitional and coastal waterbodies. This would require detailed analysis of existing monitoring data to identify candidate waterbodies that could best represent the types of coastal systems across the UK as well as incorporating land-use data and modeling which are not currently integrated with marine eutrophication assessment frameworks.
3.2.3 Improving the alignment between directives
3.2.3.1 Connecting the catchment to coast
Whilst the WFD/WER, UK Marine Strategy and OSPAR COMP advocate for a river basin approach, the directives are not always aligned. There is a disconnect between geographical boundaries and indicator thresholds which hinders understanding of eutrophication status across the continuum from transitional and coastal to marine waters (Foden et al., 2011). Alignment between decision making on programmes of measures and downstream impacts is not possible when there is disaggregated policy implementation across agencies, reporting to different government areas and stakeholders (Figure 4). Historically, UK terrestrial and marine environmental policies have been largely delivered in isolation despite the marine system being explicitly connected to the land with most of the marine pollution originating from terrestrial sources (Howarth, 2008). This has resulted in a disconnect between inshore and offshore assessments across the arbitrary policy line at 1 nm (3 nm in Scotland) that separates coastal water bodies under WFD/WER from the full extent of riverine plumes and the OSPAR and UK Marine Strategy assessment areas further offshore. This disjointed approach hinders our understanding of how land-based management measures are impacting our coastal systems. There is also a disconnect between eutrophication indicators and thresholds developed for OSPAR and those developed and applied under the WFD/WER, reflected in the UK Marine Strategy where the outcomes from different assessments were combined without full harmonization of data or assessment structures. More work is required to progress beyond the current method of simply combining the coastal and offshore assessment outcomes derived by different methodologies (Devlin et al., 2007a, 2023; Foden et al., 2011).
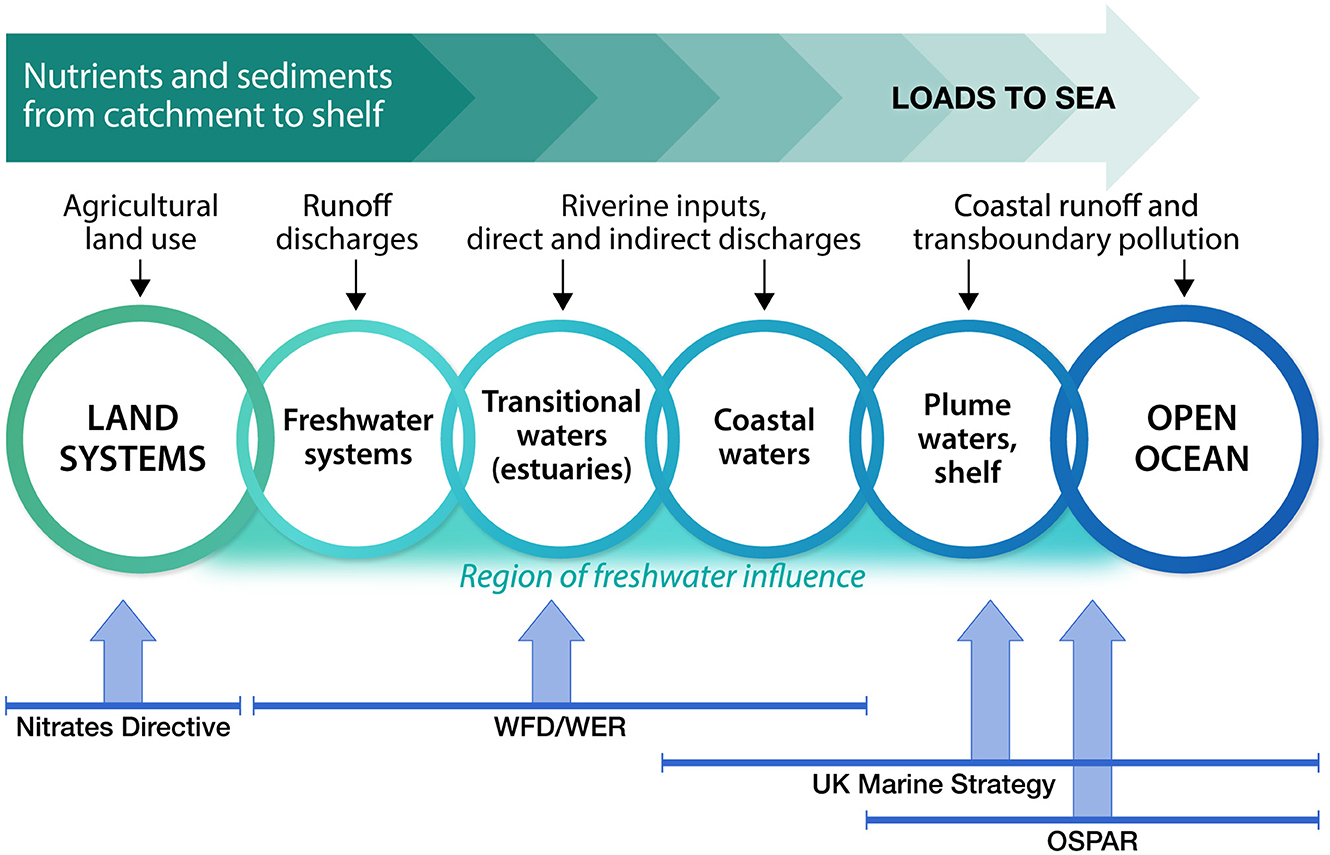
Figure 4. Eutrophication assessments span across the land to sea continuum, working across different regulations, upstream to downstream systems and a wide range of stakeholders. Different monitoring programs from across UK environment and regulatory agencies feed into the UK's national eutrophication assessment.
Future assessments should consider a fully integrated catchment to coast approach (Waterhouse et al., 2011; Brodie et al., 2012; Creighton et al., 2021) which has greater potential to change the input of terrestrial contaminants into our marine environment with subsequent positive effects on the coastal ecosystem. Managing catchments to control diffuse pollution into downstream coastal systems will also provide benefits for freshwater systems. Future eutrophication assessments should be managed more holistically, bringing diverse stakeholders together to ensure common and opposing interests are included, and to assess impacts over the ecological and hydrological boundaries.
3.2.3.2 The importance of riverine influenced areas
Until recently, eutrophication assessments carried out for the UK Marine Strategy and the OSPAR COMP have used assessment areas defined by geographical or political boundaries rather than those which are ecologically coherent and fully represent the extent of terrestrial influence in marine waters (Foden et al., 2011; van Leeuwen et al., 2015; Devlin et al., 2023). The UK coastal zone covers up to 10% of the Exclusive Economic Zone (EEZ) though that number varies dependent on the definition of coastal zone. The coastal zone is dynamic with high spatial and temporal fluctuations influenced by tides, stratification, wind and river discharges, which all influence variation in the water quality measurements. This was recognized within the initial implementation of the EU WFD (Vincent et al., 2022) which developed an approach to defining transitional and coastal typologies characterized by tidal range, mixing, salinity and depth. However, the seaward edge of the coastal assessment areas was defined by a 1 nm offshore limit for England, Wales and NI and by a 3 nm limit for Scotland, leading to abrupt delineations between the nearshore coastal areas assessed under the WFD/WER and coastal to offshore areas assessed under UK Marine Strategy and OSPAR.
Riverine freshwater plumes are the major transport mechanism for nutrients, sediments and pollutants and connect the land with the receiving coastal and marine waters. Knowledge of the variability in the extent of freshwater influence into UK marine waters is relevant for environment managers to develop strategies for improving ecosystem health and risk assessments (Schroeder et al., 2012; Devlin et al., 2012b, 2015). An approach using satellite derived suspended particulate matter (SPM) and in situ salinity provided a first estimate of the physical, chemical and biological processes (Greenwood et al., 2019) with the area of riverine influence mapped using salinity and satellite derived suspended particulate matter (Ivanov et al., 2020; Fettweis et al., 2023; Desmit et al., 2024). Sea surface salinity is the most traditional conservative tracer of freshwater discharge; however, it can be difficult to extract direct satellite-based salinity measurements with sufficient spatial resolution for coastal applications (Schroeder et al., 2012).
Although these mapping methods can improve assessments across ecologically homogeneous areas, defining river plumes seasonally and with a relatively high resolution in coastal areas is still required to assess water quality conditions across estuarine and intertidal habitats (Fronkova et al., 2022; Heal et al., 2023). Plume mapping of UK waters has now progressed to deriving and mapping the Forel Ule color scale, as determined from high resolution Sentinel-3 satellite imagery data at 1 km2 resolution for England and Wales and using the 4 km2 resolution for Scottish coastal waters (Fronkova et al., 2022). Using the relationship between ocean color and water quality parameters, recent work has defined geographically resolved assessment areas through the mapping of ocean color (Greenwood et al., 2019; Fronkova et al., 2022; Heal et al., 2023) (Figure 5). The most recent OSPAR QSR implemented a new set of ecologically relevant assessment areas (Figure 6) developed specifically for eutrophication assessment with common indicators and harmonized thresholds across national boundaries (Devlin et al., 2023; Lenhart et al., 2010; van Leeuwen et al., 2023). The new OSPAR assessment areas and thresholds, carried across for the UK Marine Strategy eutrophication assessment provides a much stronger alignment between nearshore and coastal marine waters than previous assessments, with the inclusion of plume areas and more localized coastal assessment units (Figure 5) which can be expanded into future assessments (Devlin et al., 2023).
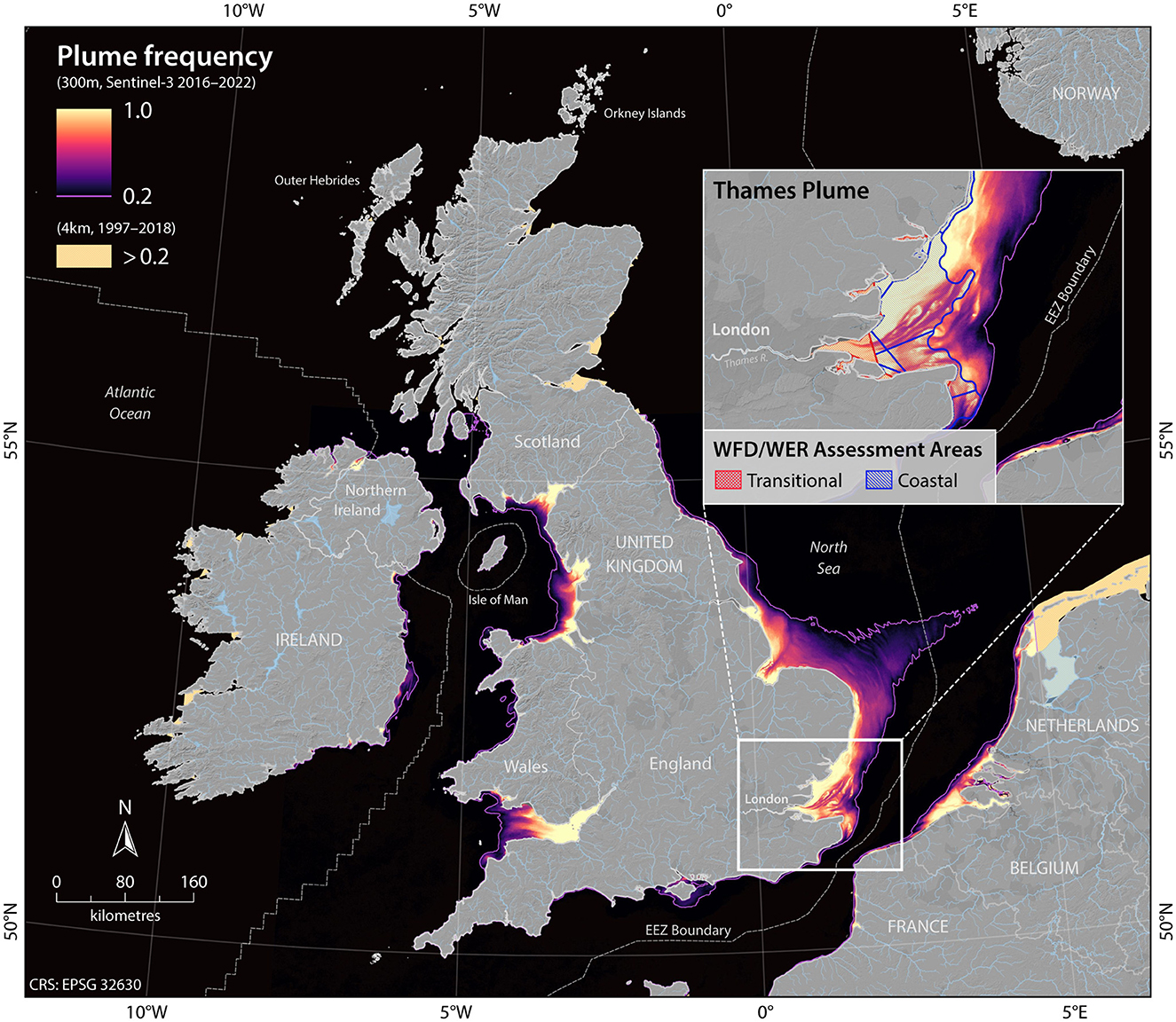
Figure 5. Riverine influenced areas for the UK are based on two different types of plume methodologies, with a higher 300 m resolution plume extent derived from the Forel Ule Index (FUI) from Sentinel-3 and lower resolution plume extent derived from Pitarch et al. (2019) 4 km FUI data. The FUI derived from Sentinel-3 has been used to map the extent of flood plumes, with FUI values of 10 or above being representative of riverine-influenced areas. The purple line designates the maximum plume extent, where the area is exposed to riverine plume at least 20% of the time between 2017–2022. Both approaches have been applied in England, Wales and Northern Ireland waters, with only the 4 km resolution imagery applied in Scotland. Development of plume imagery detailed in Fronkova et al. (2022).
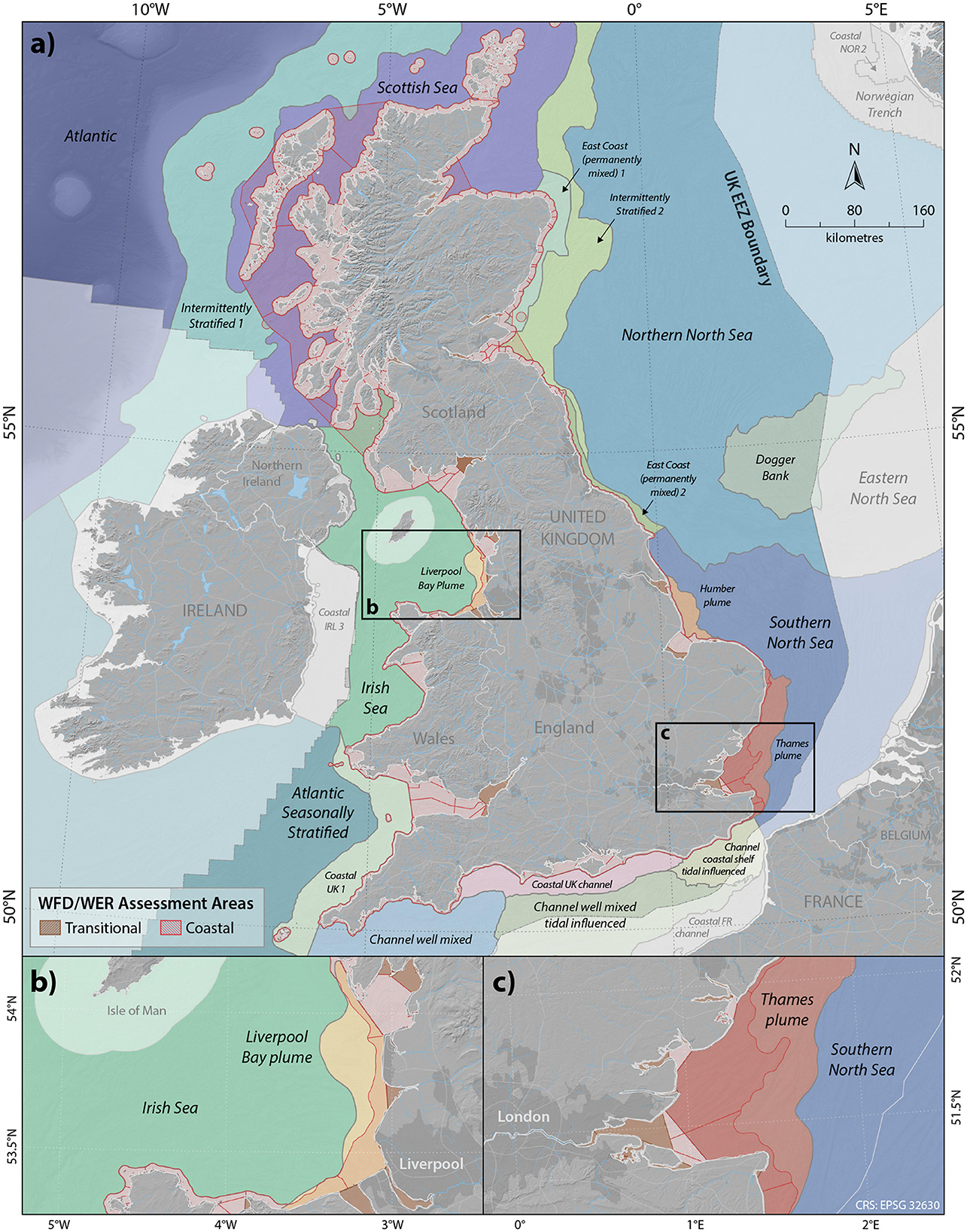
Figure 6. UK assessment areas reported under OSPAR Quality Status reporting. Note that several of these areas are shared with other OSPAR member states including Atlantic, Irish Sea, Northern North Sea and Southern North Sea, areas outside of the UK EEZ are lighter in color. WFD/WER coastal assessment units are highlighted in red. (a) Full extent of the UK assessment areas for coastal and offshore waters zoomed in to estuarine and coastal areas of (b) Liverpool Bay and (c) Thames embayment.
3.2.4 Improving our indicators of eutrophication
The eutrophication frameworks, outcomes and subsequent program of measures were based on best available information around key eutrophication indicators including nutrients, phytoplankton biomass (measured as chlorophyll) and dissolved oxygen (OSPAR, 2003, 2008a). WFD/WER assessments also use phytoplankton counts in coastal waters. These indicators are, and continue to be, highly relevant to measuring the extent and impact of eutrophication (Bricker and Devlin, 2011; Devlin et al., 2011). However, using these indicators only can limit our understanding of ecosystem impacts, and there is an urgent need to expand, both in the improvement of our current indicators and through the development of new indicators.
3.2.4.1 Indicators should consider trends and trajectory of change
Measuring nutrient loads and concentrations are important parts of understanding the trajectory of risk and potential impact of eutrophication. Reduction of diffuse nitrogen and other agricultural pollutants after the implementation of EU and UK directives, whilst initially successful, has leveled off over time. The abatement of nitrogen has not kept pace with the scale required across all catchments and diffuse nitrogen losses are now the main source of nitrogen loading into coastal and marine waters (Worrall et al., 2016; Zhang et al., 2022). At the same time, there has been an increase in the farmed fish industry in some coastal regions resulting in increasing point source introduction of nutrients to the environment that are not considered in our current assessments (Olsen et al., 2008; Holmer, 2010; Edwards, 2015). Our current assessments, using a multimetric approach incorporating several indicators, are based on status assessment (a single value aggregated over 6-year cycle), but associated trend assessments are not currently part of the quantitative assessment of eutrophication indicators. The incorporation of trend data into assessments can demonstrate the trajectory of change and help in predictions of future state. However, in the recent UK Marine Strategy and OSPAR COMP, long term changes in nutrient inputs were assessed using Mann-Kendall analysis to detect trends, quantifying (where present) monotonic trends in timeseries data, based on comparing each year's value to all preceding years (Devlin et al., 2023). This non-parametric method is useful for long term environmental data as it is not affected by any transformation of the annual data values, and it is flexible for time-series with missing data points (Bedford et al., 2020; Desmit et al., 2020). Non-parametric trend tests require only that the data be independent and can tolerate outliers (e.g., resulting from a change in analytical detection limit) and missing values in the data. Expanding the use of trend analysis such as Mann-Kendall will allow greater scrutiny of the direction of travel alongside assessment of state.
3.2.4.2 Indicators that consider susceptibility to eutrophication
Phytoplankton, through their chlorophyll cells, absorb light to provide energy for photosynthesis. Light is rarely limiting in offshore waters, but the more turbid, variable inshore waters can make it difficult for plankton to absorb enough light to grow (Devlin et al., 2008). The clarity and composition of highly dynamic estuarine and coastal waters can help inform susceptibility of the coastal and marine waters to eutrophication (Cloern, 1987, 1999, 2001). Vertical attenuation of light through the water column is attributable to the optically active components of phytoplankton, suspended particulate material (SPM) and chromophoric dissolved organic matter (CDOM). SPM is routinely measured in all UK monitoring programs but is not used as a standalone indicator. However, SPM is used in WFD/WER eutrophication assessments when nutrient concentrations exceed thresholds, and an additional (higher) threshold is applied if SPM is high (Greenwood et al., 2019). In offshore waters, CDOM originates predominantly from bacterial decomposition of phytoplankton cells, whereas in coastal waters, CDOM is dominated by humic and fulvic acids of terrestrial origin and transported to the seas through freshwater runoff from the land as well as autochthonous CDOM from salt marshes, mangroves, inter- and sub- tidal benthic microalgae, seagrasses, macroalgae and corals (Carder et al., 1989). CDOM is not routinely measured for eutrophication monitoring and assessment (Foden et al., 2008) despite being a key measurement for understanding riverine influenced plumes and light dynamics.
CDOM, ocean color, light attenuation, turbidity and SPM are all important elements in understanding the extent of the estuarine and coastal systems and the dynamics of the light conditions. Foden et al. (2008) discuss how applying a simple dose-response model of nutrient enrichment to risk of eutrophication does not consider the important role light plays in marine waters, and limits understanding of the complex interactions at play. Cloern (1999, 2001) recognizes system attributes that “filter” responses to changes in nutrient loading, including the underwater light climate, horizontal exchange, tidal mixing, grazing and biogeochemical processes. This complex response determines susceptibility, which influences the assessment of eutrophication status. The light climate is highly variable in UK waters and therefore of particular significance regarding the risk of eutrophication (Devlin et al., 2008; Foden et al., 2008, 2011).
Over the last century, the world oceans and coastal regions have experienced changes to marine lightscapes in two fundamental ways. Firstly, regions such as the Norwegian Fjords have experienced a long-term reduction in water clarity, referred to as Coastal Darkening (Aksnes et al., 2009) with large-scale drivers that are connected to effects of climate change such as more frequent and intense rainfall, increased temperatures, and other human activities that increase erosion (Dupont and Aksnes, 2013; Organelli et al., 2017; Frigstad et al., 2023). A reduction in the light availability will impact key eutrophication indicators such as phytoplankton (Capuzzo et al., 2018; Opdal et al., 2019; Wollschläger et al., 2021). Secondly, some coastal regions are experiencing a brightening of the night-time light environment linked to urbanization, on- and offshore infrastructures, fisheries, and shipping (Smyth et al., 2022; Davies et al., 2023). Knowledge of changes in natural light conditions needs to be a key part of eutrophication (and climate change) assessments into the future. The latest round of assessments show that the UK has many high nutrient transitional and coastal waters, but most of these were not deemed eutrophic due to not being able to show an “undesirable disturbance.” For example, conditions in UK coastal waters with high turbidity and large tidal systems are assumed to not support the proliferation of high biomass (Cloern, 1987; Thornton et al., 2002; Tweedley et al., 2016). However, recent work has shown that high growth can still occur under turbid conditions, with productivity remaining high despite light limitation (Gonçalves Leles et al., 2018). More work on the role of mixoplankton is required as it is now recognized that most phytoplankton and as much as half the protist-zooplankton combine both plant-like photosynthesis and animal-like consumer activity synergistically within the same single-cell (Ward and Follows, 2016; Stoecker et al., 2017; Gonçalves Leles et al., 2018; Mitra et al., 2024).
3.2.4.3 Indicators require improved understanding of natural variability
Our understanding of natural variability needs to evolve alongside our understanding of the complexity of our coastal and marine systems. The recent OSPAR eutrophication thematic assessment used an ensemble model approach to derived pre-eutrophic conditions (Lenhart et al., 2010; Stegert et al., 2021; van Leeuwen et al., 2023). Nutrient loads into the north-east Atlantic were estimated from rivers under historic conditions prior to 1900, using the European model E-HYPE and observations with historic (pre-eutrophic) conditions (Lenhart et al., 2010; Stegert et al., 2021). Reference chlorophyll concentrations were derived corresponding to the estimated nutrient concentrations under reference conditions. To account for natural variability allowing for a “slight disturbance” in the absence of more specific information, the assessment levels (thresholds) were then defined as a concentration of 50% more than the area-specific background concentration derived from the ensemble model approach (Malcolm et al., 2002; Borja, 2005; OSPAR, 2008b, 2022) which set the threshold between Non-Problem and Problem Areas. This is equivalent to the boundary setting good/moderate for the EU Water Framework Directive (WFD) and boundary setting between GES and non-GES for UK Marine Strategy and the EU MSFD (Claussen et al., 2009; Maas-Hebner et al., 2015; Topcu and Brockmann, 2021). However, when using long term monitoring data from the recent OSPAR eutrophication thematic assessment the natural variability for the common indicators (from 2015 to 2020) in UK waters varies between 24% and 56% (OSPAR, 2023). Whilst a deviation from baseline is important for all assessment processes, future assessments need to analyse “true” natural variability and look for commonalities and differences between assessment areas and different indicators.
3.2.4.4 Indicators that consider societal impacts, including environmental, social and economic drivers
Embedding environmental and societal information alongside our more traditional monitoring and assessment processes is required to fully integrate ecosystem state, pressures, stakeholders, and policy (Kristensen, 2004; Borja et al., 2006; Patrício et al., 2016). This could include quantification of economic and environmental connections, greater integration of the reporting of complex interactions between social, economic, and ecological factors, multi-disciplinary frameworks and enhanced community engagements.
One such well known framework that has achieved many positive results is the DPSIR framework, which incorporates Drivers (D), Pressures (P), State (S), Indicators (I), and Response (R). The DPSIR framework is a widely used approach to understand interconnected layers and measure the driving forces of change (Elliott et al., 2017; Kristensen, 2004; Martin et al., 2018; Patrício et al., 2016). Simple messaging and clear linkages between human-induced drivers, pressures, state, impacts, and human welfare are crucial to drive outcomes into policy implementation (Kristensen, 2004; Borja et al., 2006; Elliott et al., 2017; Martin et al., 2018). Drivers and pressures can be complex and difficult to measure and understanding the interactions between human drivers and ecological pressures is a key component to any monitoring and evaluation program, particularly one that spans from land to sea and multiple stakeholders (Oesterwind et al., 2016). The DPSIR framework has been adopted by the European Environment Agency and others (Borja et al., 2006; Atkins et al., 2011a; Patrício et al., 2016) and describes a framework for assessing the causes, consequences and responses to eutrophication in a holistic way including managing catchment to coast processes (Bowen and Riley, 2003; Langmead et al., 2007; Maccarrone et al., 2014; Le Gentil and Mongruel, 2015). DPSIR frameworks act as decision support systems which can enhance communication, knowledge transfer and interaction among scientists and policymakers, facilitating engagement among stakeholders and enhancing the legitimacy of the decision-making process (Newton and Weichselgartner, 2014).
Additionally, programs that include clear elucidation of cause and consequence, socio-economic pathways, and greater levels of engagement with communities are all attributes of successful monitoring and evaluation programs that could be applicable to UK coastal and marine systems (Figure 7). Embedding a greater understanding of natural capital into monitoring programs has been a successful way to incorporate the system flows between ecology, goods and services, and benefits to human wellbeing (Rhodes et al., 2017). Kermagoret et al. (2019) shows increasing eutrophication leads to a degradation of ecosystem service bundles, particularly for nutrient and pathogen regulation/ sequestration, or for the support of recreational and leisure activities. A cost benefit analysis on freshwater eutrophication in England and Wales, recognizing many data gaps, estimates the damage costs of freshwater eutrophication to be £75.0–114.3 million yr−1 (Pretty et al., 2002) highlighting the severe impacts of nutrient enrichment and eutrophication on many sectors of the economy. Estimates of local economic benefits for seaside recreation and waterfront property in Denmark through reduction of agricultural N losses were estimated at €35 million, with co-benefits of up to €57 million (Andersen et al., 2019). Conversely, the value of functioning coastal systems and nature based solutions to remove anthropogenic nitrogen can have wide ranging positive impacts and reduce costs of waste remediation (Watson and Beaumont, 2024). Cost accounting and reporting of natural capital benefits can be a valuable tool for positive policy implementation. Estimates of damage costs against policy response should be a critical part of any future eutrophication assessment.
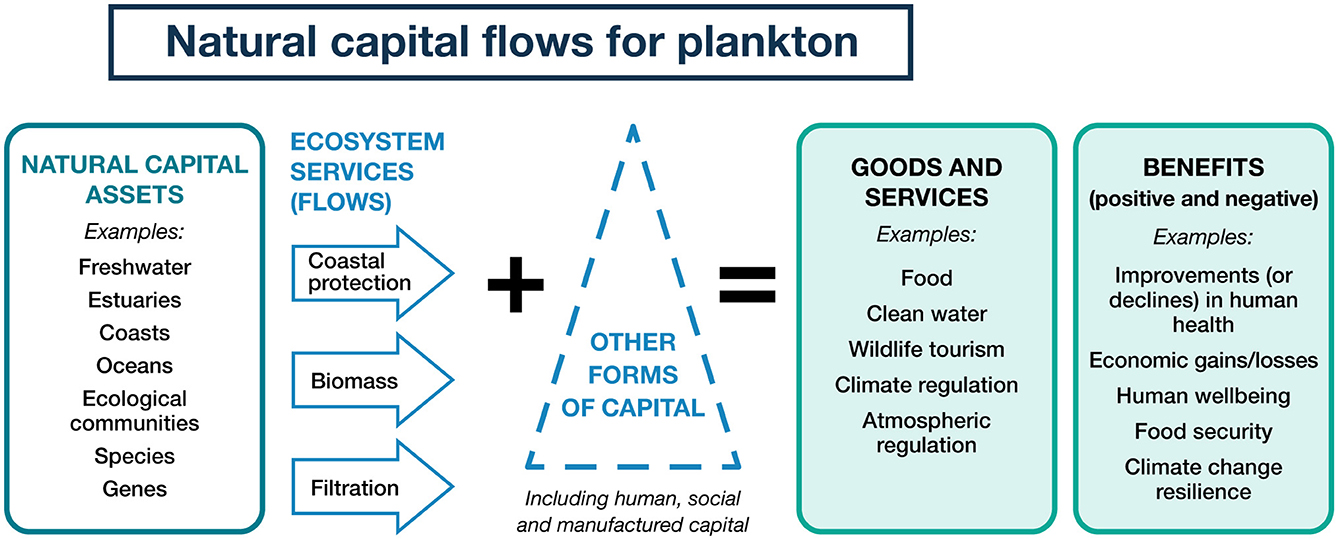
Figure 7. Schematic illustration of how natural capital represents the flow between the asset and ecosystem service into goods and services and benefits for humans. Diagram adapted from Devlin and Wenger (2024).
Integrated approaches like the cross-sectoral and transdisciplinary One Health monitoring and evaluation framework (Stentiford et al., 2020), that emphasizes the interconnections between the health of humans and ecosystems, are highly applicable to an integrated eutrophication approach. These holistic approaches recognize the benefits of programs that are relevant to a range of end-users and contribute to positive changes through management actions that engage and represent the values of a diverse range of stakeholders impacted by the decision making. This is particularly true for local and regional stakeholders but can also extend to international partnerships and frameworks.
Greater engagement of the community, not only through data collection (i.e., “citizen science”) but as an important part of the evaluation side, and by becoming embedded in decision making around policy and governance, should be a key requirement of greater success in monitoring and evaluation programs (Bischof, 2010; Cigliano et al., 2015; Darling et al., 2019). Local citizen scientists can cover important spatial gaps and when combined with long-term monitoring data from regulatory agencies, can add benefit to eutrophication monitoring programs (Loiselle et al., 2024).
3.2.4.5 Integration of indicators to multidirectional value of state
Current UK eutrophication indicators are assessed in terms of unidirectional exceedances. This approach does not fully encompass the range of conditions that support positive ecological functioning. For example, a chlorophyll threshold which, when exceeded, indicates only that increasing biomass can potentially result in undesirable disturbance to the ecosystem (Tett et al., 2007). However, recent work has also identified declining primary productivity (Capuzzo et al., 2018) due to warming waters and changing nutrient imbalances (Ryther and Dunstan, 1971; Lu and Tian, 2017; Nohe et al., 2020; Xu et al., 2020). Our eutrophication approach needs to consider the optimal range of values for each indicator, providing benchmarks for upper and lower limits. This approach would recognize that for some indicators, that there is a seasonal and temporal shape to the optimum range that needs to be considered and not just a single upper threshold (Tett et al., 2007, 2008; Bedford et al., 2020). Additionally, many of the indicators are intrinsically linked, and need to be operating in the optimum range across a wide range of interconnected ecological measurements.
Safe Space functioning is an emerging concept that is becoming more visible in our understanding of ecosystem state. Rockström et al. (2009) defined the boundaries for a “safe operating space for humanity, which outlines nine planetary goals that need to be supported together.” The underlying concept of ecosystems being dependent on various conditions, all existing in the same conceptual space, should become a tangible part of our future thinking. Eutrophication assessments could develop a range of conditions that need to be met to ensure ecological functioning and resilience (Figure 8). This could be adapted from existing indicators of nutrients, biomass and dissolved oxygen, measures of important physico-chemicals such as turbidity and expand to look at corresponding plankton community metrics focusing on changes in plankton lifeforms impacted by eutrophication and primary productivity.
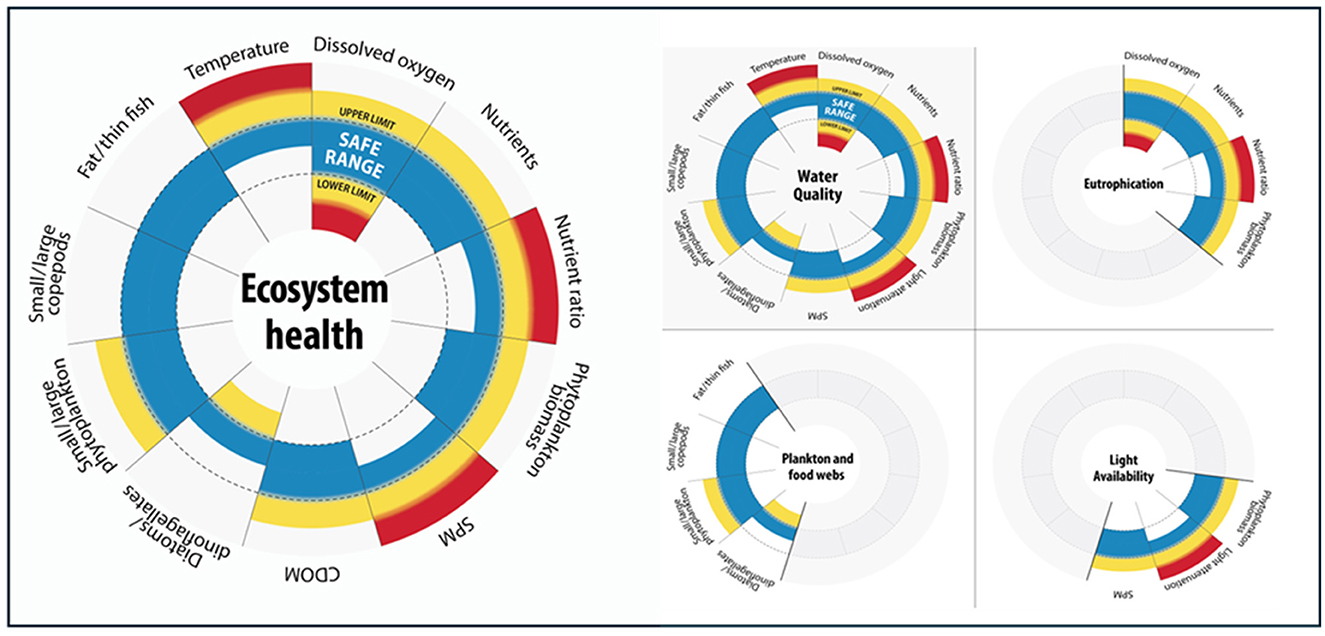
Figure 8. Visualization of the concept of “safe space” functioning, which can be adapted to represent a range of conditions that need to be supported together, identifying upper and lower limits around that condition. CDOM, chromophoric dissolved organic matter; SPM, suspended particulate matter.
3.2.5 Improving our understanding of ecosystem impacts
3.2.5.1 Eutrophication as a plankton pressure
Eutrophication is a complex process and often associated with not only changes in overall algal biomass but also with changes in plankton community structure. Common eutrophication indicators (e.g., chlorophyll a, nutrients, dissolved oxygen) are not adequate for understanding biodiversity changes, especially those associated with the proliferations of HABs (Glibert, 2017; Anderson et al., 2002).
Many coastal waters are experiencing nutrient imbalances due to the more successful mitigation of phosphorus over nitrogen and the continuing excess of nitrogen over silicon compounds. High N:Si favors non-silicified micro-algae, leading, for example, to blooms of Phaeocystis following silica depletion in the southern North Sea (Davidson et al., 2012). High N:P might favor mixotrophic nanoflagellates, which can obtain P from other micro-organisms, although this seems most relevant under oligotrophic conditions (Duhamel et al., 2019). High N:P ratios also correlate with Phaeocystis colony dominance in the southern North Sea (Lancelot et al., 2009). Many international marine waters have reportedly undergone regime shifts in phytoplankton community composition; the proportion of diatoms has decreased, (predominately in inshore, nutrient enriched coastal waters) whereas that of non-diatoms such as dinoflagellates and cyanobacteria has increased (Xiao et al., 2018; Lu et al., 2023; Chen et al., 2024). A shift in the phytoplankton community composition from diatoms, which have traditionally been dominant, to non-diatoms, can impact on the plankton community and foodwebs (Granéli and Turner, 2002; Verity et al., 2002; Lu and Tian, 2017; Chen et al., 2024). This change has several consequences, including reduced energy transfer to higher trophic levels, higher respiration rates (higher oxygen consumption and CO2 production), and accumulation of fewer economically valuable organisms damaging the regulatory, provisioning, cultural, and supporting service functions within the marine ecosystems (Yunev et al., 2017; Chen et al., 2024). The consequences of changes in diatom and dinoflagellate dynamics in the phytoplankton community is a major concern because these taxa play key roles in ecosystem processes and form the basis of many aquatic food webs (Menden-Deuer and Lessard, 2000). Dinoflagellates account for 75% of all harmful phytoplankton species (Smayda and Reynolds, 2003) which can adversely affect human health as well as marine fisheries and aquaculture (Anderson et al., 2002).
Different species of phytoplankton have different traits (Bedford et al., 2018, 2020; Graves et al., 2023; Holland et al., 2023a,b; McQuatters-Gollop et al., 2017), most notably size and shape, growth rate, life history, and behavior such as motility, that together determine their ecological niche and preferred environmental conditions, and phytoplankton are a major driver for global carbon fixation and biogeochemical cycles. Shifts in plankton species composition from diatoms to dinoflagellates may indicate a shift in the balance of organisms due to eutrophication. The composition of the phytoplankton community could be compared with area-specific reference conditions and be expressed by the ratio of diatoms to dinoflagellates. To maximize the utility of the plankton lifeform approach for informing the management of marine ecosystems, changes in the abundance of lifeforms need to be attributed to drivers of change. These drivers can include “directly manageable” anthropogenic pressures such as eutrophication caused by nutrient loading (Bedford et al., 2020; Ostle et al., 2021; Graves et al., 2023; Holland et al., 2023b).
Whilst nutrients and phytoplankton biomass are key indicators for eutrophication assessments, there has been an increasing focus on marine protection and, more generally, biodiversity protection which has led to efforts to connect eutrophication with its impacts on impact on the balance of plankton lifeforms in UK waters (Graves et al., 2023; Holland et al., 2023a; McQuatters-Gollop et al., 2024). Reanalysing historic phytoplankton data collected under the various assessments found an increase in small phytoplankton cells, relative to the decreasing large cells and an increase in the dinoflagellate group, which are generally much less nutritious and more toxic than the decreasing diatoms (Graves et al., 2023). The UK Marine Strategy provided a framework within which to include plankton in the biodiversity assessment and developed plankton tools that applied a lifeforms approach grouping plankton by their common key functional traits (McQuatters-Gollop et al., 2019; Bedford et al., 2020; Graves et al., 2023; Holland et al., 2023a). Improving our ability to track nutrient pressures against biological impact should expand on this by considering pelagic communities explicitly within the eutrophication assessments.
3.2.5.2 Nutrient imbalances and plankton health
Human-induced inputs of phosphorus (P) and particularly nitrogen (N) into the biosphere continue to be problematic for inshore waters. The ratio of N to P, which is sensitive to the relative anthropogenic inputs of the two nutrients, has emerged as a significant driver of environmental change, impacting organisms, ecosystems, and global food security (Penuelas and Sardans, 2023). Historically, P has been the main nutrient controlling upstream freshwater productivity, whereas N limitation is more prevalent in most coastal waters (Brodie et al., 2011; Devlin et al., 2023). However, controls on production and nutrient cycling in estuarine and coastal systems are physically and chemically distinct from those in freshwater counterparts, and upstream nutrient management actions (predominately P controls) have exacerbated N-limited systems further downstream (Paerl, 2008). These changing anthropogenic activities have caused imbalances in N and P loading in UK waters (Figure 9) making it difficult to control eutrophication by reducing only one nutrient. Effective management of inputs of both nutrients are needed for long-term control of eutrophication in our coastal and marine waters. There has been concern about shifting nutrient ratios for some time with increased reporting of the impact of global nutrient loads and concerns on the export of nitrogen increasing faster than phosphorus (Glibert, 2017). Changes in nutrient loading, balances and concentrations can affect plankton, their biodiversity and toxicity with unintended consequences for HABs, mixoplankton, plankton communities and the food web (Gonçalves Leles et al., 2018; Bedford et al., 2020; Graves et al., 2023; Holland et al., 2023a; Mitra et al., 2024).
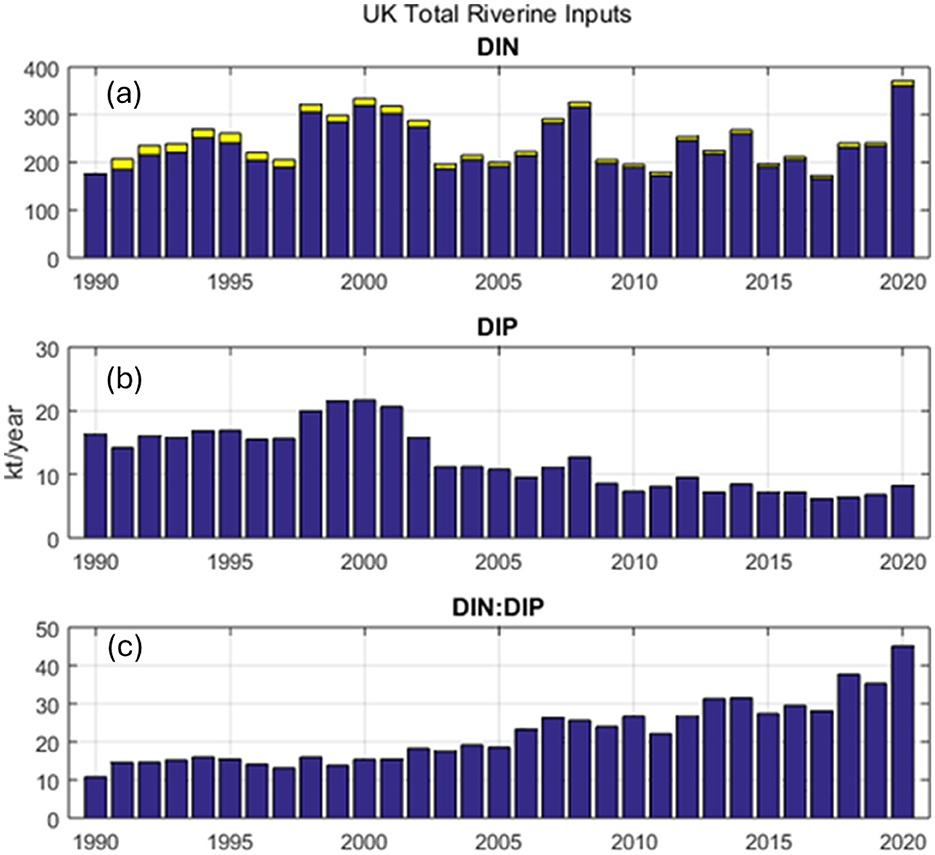
Figure 9. Long term changes in nutrient inputs from the UK including (a) Dissolved Inorganic Nitrogen (DIN), (b) Dissolved Inorganic Phosphorus (DIP) and (c) the ratio of DIN:DIP, with the increase or stabilization of DIN compared to decreasing DIP concentration leading to a five-fold increase in DIN:DIP ratio over the past 30 years.
3.2.6 Consideration of climate resilience and shifting baselines
Climate change is impacting the environmental baseline, with changes in rainfall patterns affecting the delivery of freshwater and associated nutrients and sediment to the coastal marine environment with subsequent effects on ecosystem processes. Climate change has the potential to increase nutrient run off and algal growth and modify the interactions between planktonic and pelagic organisms. Summer rainfall is predicted to decrease alongside increases in the intensity of summer and autumn rainfall events (Cotterill et al., 2023). Shifts in seasonal rainfall patterns can change both the intensity and frequency of nutrient inputs where sudden, large events can cause excessive flooding and pulses of increased inputs and sediments into the marine environment. Predicted changes in the timing and intensity of winter rainfall may lead to increased riverine inputs of nitrogen and phosphorus (Ockenden et al., 2017; Bussi et al., 2016). It is expected that climate change will result in more hydrological extremes and higher river discharges, particularly in the northern parts of the North Sea (Willems and Lloyd-Hughes, 2016). These intense events may lead to large nutrient loads entering the coastal environment, particularly if they coincide with recent agricultural applications of fertilizer or slurry and/or intense rainfall following prolonged spells of very dry weather. Changes in the frequency of such intense events may also lead to changes in discharges from combined sewer overflows (CSOs), with the potential to alter nutrient inputs to the coastal environment. The magnitude of these changes is expected to vary widely between catchments, depending on land use, type of agriculture and agricultural practices and also on future land use and socio-economic developments (Arheimer et al., 2012).
Increased water temperatures have been shown to lead to phenological shifts, biogeographical changes and changes in abundance of plankton (Brander et al., 2016; Eker-Develi et al., 2022). Temperature change and eutrophication are known to affect phytoplankton communities (McQuatters-Gollop et al., 2022), but we have limited information on the effects of interactions between simultaneous changes in temperature and nutrient loading within coastal ecosystems. Ecological time-series are critical for understanding the drivers of change, especially of the interaction between eutrophication and climate factors (Edwards et al., 2010). Such interactions have been key in driving diatom-dinoflagellate dynamics in coastal systems such as the East China Sea where studies have shown that diatoms preferred lower temperature and higher nutrient concentrations, while dinoflagellates were less sensitive to temperature and nutrient concentrations but tended to prevail at low phosphorus and high N:P ratio conditions (Xiao et al., 2018). These different traits of diatoms and dinoflagellates resulted in the observation that increasing stratification and increasing terrestrial nutrient input could promote dinoflagellates over diatoms (Xiao et al., 2018).
Increases in recycling rates of organic matter in the water column with increasing temperature have the potential to increase the availability of inorganic nutrients for phytoplankton growth during the year and therefore increase chlorophyll. Increasing storminess and intensity of rainfall have the potential to increase the turbidity of UK shelf seas. Studies have shown that turbidity in the North Sea has increased over the past 100 years as a result of changes in storminess (Capuzzo et al., 2018; Wilson and Heath, 2019). Changes in the underwater light climate due to increases in turbidity have been linked to decreases in productivity (May et al., 2003). Any increases in storminess during the stratified period could act locally to increase dissolved oxygen concentrations in bottom waters by enhancing mixing from the surface. Increased storminess could also increase resuspension of sediments from the seabed which would increase the oxygen demand for remineralisation of the organic matter, leading to a decrease in oxygen concentration (Mahaffey et al., 2023). Increases in riverine nutrient loads from altered precipitation patterns may enhance algal biomass with the potential to decrease dissolved oxygen in riverine influenced coastal waters through increased oxygen demand when the organic matter is remineralised. Being able to predict the impact of climate change and understand potential mitigation measures is key to successfully managing the marine environment and will require adjustments to our current eutrophication indicators (Table 2).
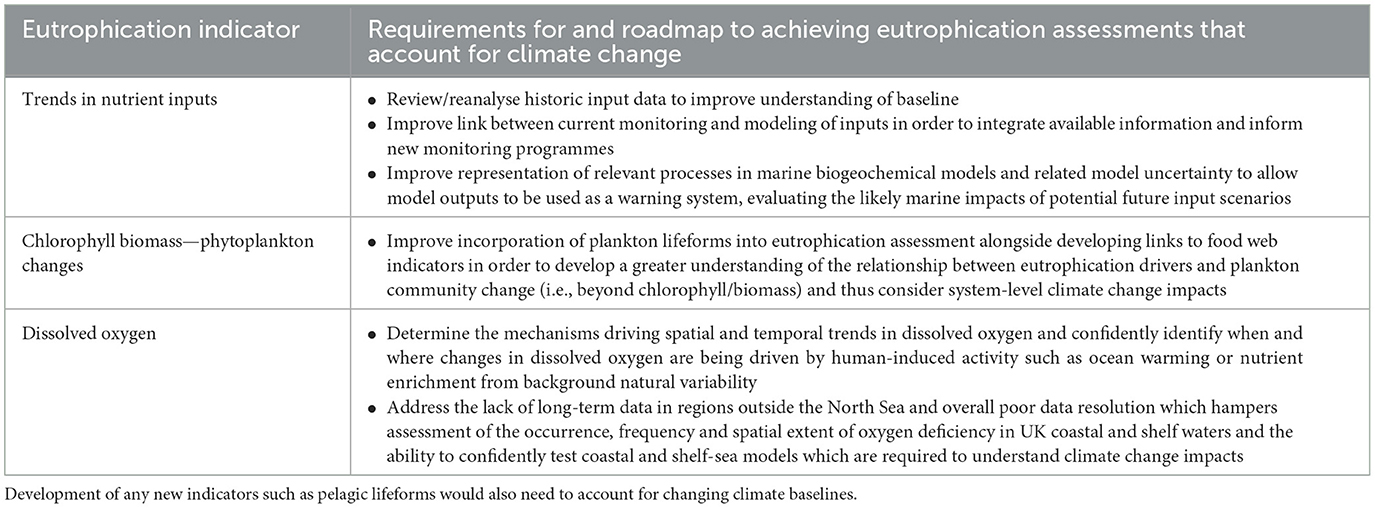
Table 2. Requirements for future eutrophication assessments to fully consider climate interactions for the three common indicators most frequently used across all eutrophication assessments.
3.3 Prioritization of solutions required to fill evidence gaps
From our review of eutrophication assessments, we have collated recommendations to improve future eutrophication monitoring programs through addressing the evidence gaps within the five themes of data, alignment, indicators, ecosystem and climate.
Following the recommendations to improve future eutrophication assessments, we next provide an assessment of feasibility, developed from the review and expert knowledge of what is currently occurring in UK national eutrophication monitoring programs.
Feasibility of developing and implementing the key evidence gaps into future monitoring and assessment is reported against the effort required to achieve the recommended activity, the scale of complexity and potential costs of implementation (Figure 10). Effort is defined as the level of agency involvement and if the work can be delivered from one or more agencies, the level of stakeholder participation required and if any components of the work has already been agreed. Complexity is a measure of the type of data required to deliver the work, with the more complex systems being more difficult to deliver and includes a consideration of how easily the data changes could be made and if those changes are dependent on cross agency, cross stakeholder agreement. Costs are a simple classification of high costs related to ongoing staff, vessel and field time against lower costs that are not dependent on implementing aspects of a field-based monitoring program.
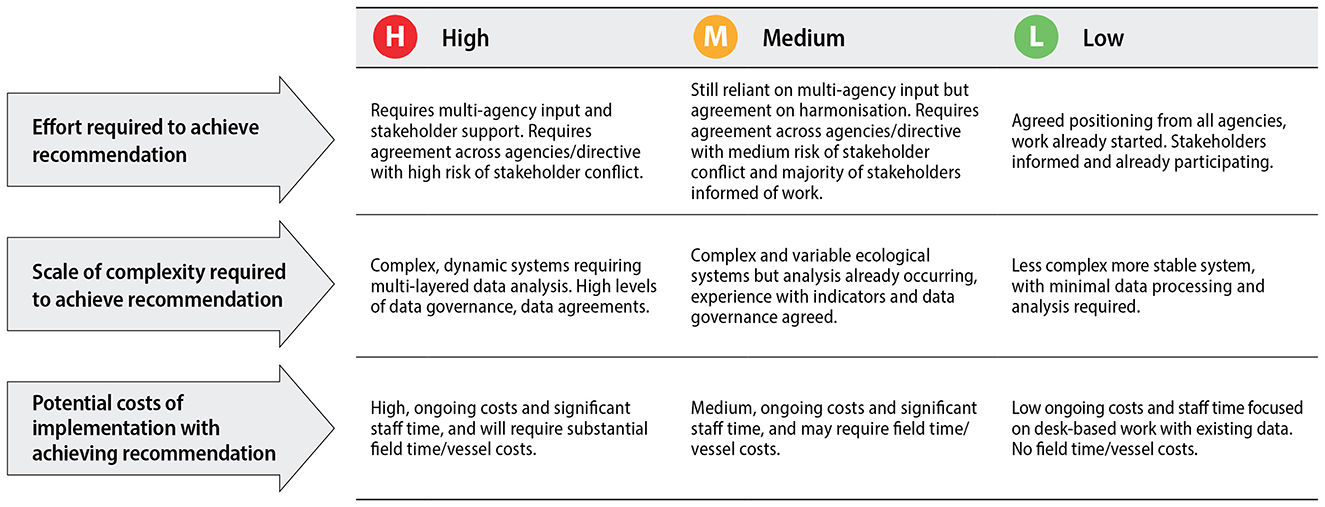
Figure 10. Qualitative ranking of different factors for measuring difficulty, complexity and cost of each recommendation for future eutrophication monitoring.
Key recommendations under each thematic area are described in Figure 11, summarizing the evidence gaps identified through the review and consultation, a list of activities that could address these evidence gaps, and then the outcomes of the feasibility assessment (effort, scale, cost). Additionally, a short narrative on impact of the improvement and progress that has already occurred is presented (Figure 11).
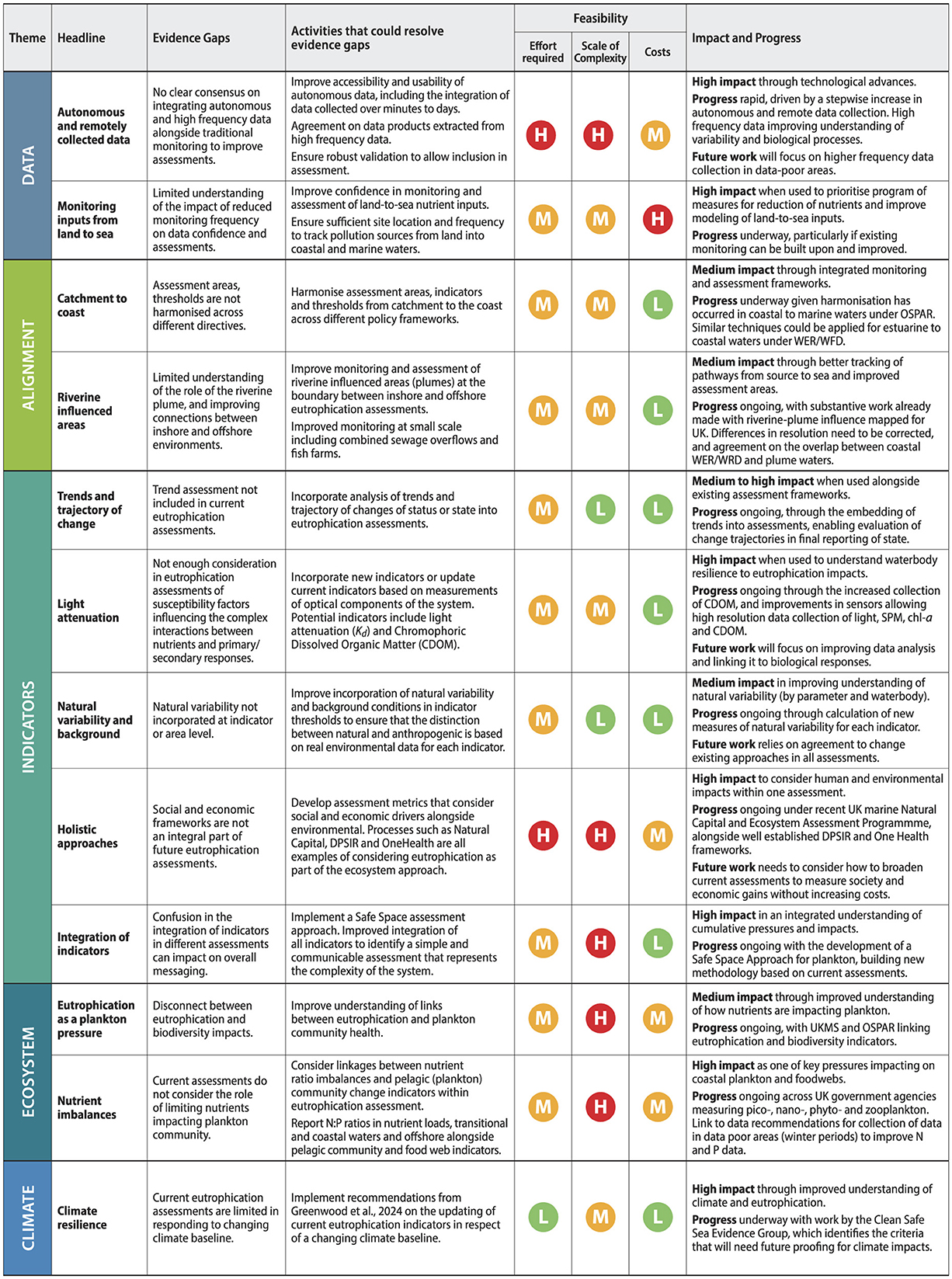
Figure 11. Recommendations for future eutrophication monitoring and assessment in UK marine waters categorized into themes of data, alignment, indicators, ecosystem and climate.
Recommendations for improved data flow require improved accessibility and usability of autonomous data, and clear processes for high level data products from autonomous and high frequency data (Figure 11). There is an urgency to restore nutrient load monitoring to enable clear pathways for a program of measures. Alignment improvements focus on the harmonization of all aspects of the different eutrophication monitoring and assessment frameworks, achieving true integration across the catchment to coast encompassing the full riverine influenced area that links coastal and offshore processes. A range of indicator improvements are presented, including more information on factors that influence susceptibility to eutrophication, refining understanding of natural variability in development of thresholds and consideration of social, economic and environmental benefits. All the indicator improvements could lead to a fully integrated assessment, aligned with the Safe Space theory of a range of conditions that support optimum benefits. Improving our understanding of the ecosystem benefits requires consideration of eutrophication as a plankton pressure, linking nutrient imbalances and impacted water quality with changes in plankton functionality. Finally, climate change and eutrophication are intrinsically linked, with the potential to exacerbate impacts, knowledge of these interactions is critical in a changing climate world.
4 Discussion
4.1 The shifting baseline of eutrophication
Etymologically, eutrophication refers to good nourishment and what was thought to be a natural process in postglacial lake evolution. However, the meaning has changed, as shown by European Court of Justice judgements (ECJ, 2004, 2009), relating to breaches of the UWWTD, that describe it as a process caused by human actions that lead to undesirable disturbance to the balance of organisms and the quality of water. Undesirable disturbance was defined as “a perturbation of a marine ecosystem that appreciably degrades the health” (Tett et al., 2013) or “threatens the sustainable human use of that ecosystem” (Tett et al., 2007, 2013; Ferreira et al., 2011). From the perspective of a natural capital approach, the term “undesirable” is a link (1) to specific concerns about the impact of anthropogenic nutrients on the ecosystem services provided by coastal seas, and (2) to the perturbations of planetary N and P fluxes that are amongst the pressures pushing Earth's biosphere outside a “safe operating space” for humanity (Rockström et al., 2009; Richardson et al., 2023).
Eutrophication is indeed a wicked problem (Thornton et al., 2013), impacting different ecosystem services and different sectors of society in different ways. For examples, changes in nutrient levels in the southern North Sea have probably contributed to changes in flatfish catches (a provisioning service) and waterbird numbers (a cultural service to birdwatchers) (Engelhard et al., 2011; Philippart et al., 2007; van Roomen et al., 2012). Use of the sea's regulating services for nutrient cycling reduces costs for householders and fish-farmers who might otherwise have to pay for removing nutrients from farm or domestic effluent (Martino et al., 2019). However, overuse of these regulating services runs the risk of harming provisioning and cultural services as a result of e.g. increasing frequency of HABs, shifts in marine food-webs that might be detrimental to commercial fisheries, and more decaying green algae on beaches.
Nutrient pollution is frequently related to diffuse sources, complicating identification and mitigation of the impacts of single sources. Le Moal et al. (2019) acknowledge these difficulties and urge a different approach to diffuse and large ranging nutrient sources where we need to address: (i) the long term cumulative impact of far reaching anthropogenic activities, (ii) the consequences of multiple, and often cumulative, actions which can be very distant both in space and time, (iii) the difficulty in disentangling past and present causes from past anthropogenic legacy (O'Higgins et al., 2014). Our future programs need to consider what Thornton et al. (2013) calls “the wicked problem of eutrophication” where multiple, often cumulative actions, remote both in space and time from the pressure, enact variable responses and trajectories along the land-sea continuum. This is compounded by complex decision-making required from multiple stakeholders with different end-user requirements, limited interactions, and government agencies reporting to different policy levers with increasing costs and reduced funding.
4.2 Contextualizing eutrophication assessments in the UK
Advances in eutrophication monitoring and successful implementation of programmes of measures has been driven by decades of environmental protection offered under policies such as the EU Urban Wastewater Treatment Directive, Nitrates Directive, Water Framework Directive, EU Marine Strategy Framework Directive, the UK Marine Strategy, and the WFD/WER and the requirements to report under the OSPAR COMP and “and for the OSPAR “Inputs of nutrients to the marine environment” indicator. This process recognizes the achievements to date under national and international environmental directives and set out a path toward improving our approach to ensure continued development of our understanding of eutrophication and all its complexities. The shift from assessments under EU environmental directives to a nationally based marine strategy has been challenging but has also acted as a catalyst to rethink how we manage our environment in respect to eutrophication. Ecosystem management requires adaptation and modification, allowing the adaptation of the environmental legislation and management to change as our understanding and the rate and scale of impact changes.
4.3 Refining our future assessments
4.3.1 Improved data flows
We require new and novel ways of collecting data to help us to understand the highly variable processes that influence the scale and extent of eutrophication. Improving our understanding of eutrophication within the national assessments will be achieved through the better use of high frequency and autonomous data and improved technology, ensuring the optimisation of the large datasets. However, the complex process of ensuring sufficient calibration of sensor data and integrating the higher resolution data into data workflows and repositories means that all available data types have not always been available for use in assessment. Ongoing investment into high-quality calibration of autonomous data will be required to allow the transition to a next-generation assessment utilizing available data alongside high-resolution data (Bean et al., 2017; Daniel et al., 2020).
4.3.2 Improved alignment
Disaggregation between the natural gradient of coast to sea does not achieve harmonized assessments. A new integrated approach will help us reveal a fuller picture of the health of our seas and oceans bringing ecosystem, economics and social criteria together to encapsulate the full value of a functioning ecosystem. Future assessments should continue to assess the continuum between estuaries and coastal systems, highlighting the importance of the riverine influenced area that lies between the UK transitional and coastal waters and further offshore assessment areas (Greenwood et al., 2019; Fronkova et al., 2022; Heal et al., 2023). Developing a catchment to coast approach should always encompass clear understanding of anthropogenic change, the technical ability to link that change to an activity or sector, transparent pathways for positive management action, recognition and incorporation of divergent societal concerns and policy tools that support sustainable outcomes for the marine environment (Devlin and Wenger, 2024). Alignment of governance strategies, integrated monitoring programs, more regular cross-agency engagement and harmonized assessments would be a first step in achieving a co-ordinated catchment to coast program.
4.3.3 Improved indicators
4.3.3.1 Trend assessments
Using long term cross-sectoral monitoring data will improve applicability for assessing changes in landscape-level environmental conditions and improve our ability to support short- and long-term management decisions (Maas-Hebner et al., 2015). The presentation of long-term data for nutrient inputs can be valuable in understanding what is changing over time, linking program of measures to reducing nutrient inputs, or conversely, showing the lack of appropriate program of measures resulting in an increase in one or more of the nutrients being measured.
4.3.3.2 Understanding “true” natural variability
Whereas our current suite of environmental indicators (including OSPAR PH1) seems reasonably complete and widely applicable in UK coastal waters, reference conditions and threshold values may need to be set locally and reflect both the local environment and local preferences for desirable conditions.
4.3.3.3 Susceptibility to eutrophication
Understanding of susceptibility and resilience needs further work, and consideration of these complexities should be considered in the development of new indicators or supporting evidence alongside the more traditional indicators.
4.3.3.4 Indicators of social, economic and environmental wellbeing
Assessment of eutrophication needs a social as well as an ecological context. Cost-benefit analysis involving ecosystem services and natural capital could assist in this but will need better models for the response of services to changes in nutrient loading, and for the preferences of different communities (or stakeholder categories) for these services. More integrated assessments such as the One Health approach, the DPSIR approach and natural capital accounting (Ross and Carter, 2013; Cork et al., 2016; Stentiford et al., 2020) explore the multiple interconnections that exist between environmental, animal and human health as a technique to incorporate multiple benefits to multiple end-users. Processes or programmes that could deliver greater social cohesion in our understanding of eutrophication need to measure environmental success through alternative indicators, such as ones that measure the protection of assets within marine natural capital, and holistic approaches that consider health between the environment and humanity as intrinsically linked in terms of water quality improvements. This will enable a safe space approach that is essential to ensuring sustainable and resilient human and environmental health.
4.3.4 Improved understanding of ecosystem impacts
Improvements in our understanding of the complex connections between nutrients and the pelagic community allow us to see how increases and imbalances in nutrients impact trophic interactions and the food web. Incorporating information on pelagic habitats will expand our eutrophication assessments from the more commonly used indicators of nutrients, chlorophyll and dissolved oxygen toward better estimates of plankton community shifts resulting from nutrient imbalances, and related impacts on ecosystem functioning. Further harmonization of data sources and assessment methods between the eutrophication and pelagic and food web assessments is expected to increase comparability and improve understanding of how nutrient loads affect ecosystem functioning and at which nutrient concentrations the ecosystem can be considered healthy (OSPAR, 2023).
4.3.5 Improved understanding of climate change and eutrophication
Climate change and eutrophication are intrinsically linked. While much is known about each individually, it is crucial to understand how they are interconnected and how climate change exacerbates marine pollution. Climate change leads, among other impacts, to floods and droughts which cause stronger variations in nutrient inputs. Future assessments need to consider the ecological effects of this greater variability and how to adequately monitor and assess this shifting baseline for the purposes of future eutrophication assessments (OSPAR, 2023).
5 Conclusion
We can improve our eutrophication assessments, despite the complexity of the drivers and impacts, and the uncertainty related to mitigation and recovery processes. Although this account has focussed on eutrophication in UK estuaries and shelf waters, much of our argument is more widely applicable. Solutions are possible, though almost never simple, and rely on a combination of long-term strategies, improved sewage and groundwater infrastructure, best management practices around agriculture and aquaculture, detailed monitoring and assessment and close partnerships between all stakeholders, public users, and government. In the UK case, improving stakeholder engagement with river trusts, conservation groups, farmers, water operators and communities needs to continue to be an integral part of the eutrophication assessment.
To enable government to continue to implement these strategies, and successfully measure their progress, UK agencies must continue to work collaboratively to innovate and improve the efficiency of monitoring programmes. This review provides an opportunity to look across national obligations and disparate approaches, identifying key evidence gaps when coastal and marine waters are continually impacted by nutrients alongside other pressures. The historical context of UK approaches to the monitoring and management of eutrophication is important when considering both the science used to inform the design of the current assessments and what science may be needed in the future to ensure robust, evidence-based decision-making. This review provides that historical context, adds the outcomes of expert discussion sessions, and identifies knowledge gaps including those involving human wellbeing and natural capital and, in doing so, sets out a framework to evolve marine eutrophication assessment and management into the future. Bringing together potentially conflicting voices to work collaboratively is the best way to progress and evolve eutrophication assessments. On the larger scale, we need to assess UK marine discharges not only for their contribution to the much-disturbed planetary N and P cycles, but also for their impact on the functioning of marine ecosystems considered as part of the biosphere.
Data availability statement
The original contributions presented in the study are included in the article/supplementary material, further inquiries can be directed to the corresponding author.
Author contributions
MD: Writing – original draft, Writing – review & editing. CG: Writing – original draft, Writing – review & editing. NG: Writing – original draft, Writing – review & editing. MA: Writing – original draft, Writing – review & editing. EB: Writing – original draft, Writing – review & editing. MH: Writing – original draft, Writing – review & editing. AM-G: Writing – original draft, Writing – review & editing. PT: Writing – original draft, Writing – review & editing. DT: Writing – original draft, Writing – review & editing. MB: Writing – original draft, Writing – review & editing.
Funding
The author(s) declare that financial support was received for the research and/or publication of this article. Funding provided though the Natural Capital and Ecosystem Assessment (NCEA) programme (NC34 Pelagic program-“PelCap”) and the UK Department for Environment, Food and Rural Affairs (Defra) through the Grant-In-Aid programme. This work was supported by the Natural Environment Research Council and the ARIES Doctoral Training Partnership (grant number NE/S007334/1).
Acknowledgments
We are grateful for the funding received from the UK Department for Environment, Food and Rural Affairs (Defra) through the marine arm of their Natural Capital and Ecosystem Assessment (NCEA) programme (NC34 Pelagic program-“PelCap”). The marine NCEA programme delivered evidence, tools and guidance to lead the way in supporting Government ambition to integrate natural capital approaches into decision making for the marine environment. Find out more at https://www.gov.uk/government/publications/natural-capital-and-ecosystem-assessment-programme. We are also grateful for the funding received from the UK Department for Environment, Food and Rural Affairs (Defra) through the Grant-in-Aid program that supports national monitoring across UK agencies, and particularly the GIA03—Water Quality (Eutrophication monitoring) led by Cefas. Thanks also to Martin Lilley for reading and vastly improving a previous version and finally thanks to the UK Eutrophication Steering Group and the UK Pelagic Habitats Evidence Group that provided many of the discussions that developed this paper.
Conflict of interest
The authors declare that the research was conducted in the absence of any commercial or financial relationships that could be construed as a potential conflict of interest.
The author(s) declared that they were an editorial board member of Frontiers, at the time of submission. This had no impact on the peer review process and the final decision.
Generative AI statement
The author(s) declare that no Gen AI was used in the creation of this manuscript.
Publisher's note
All claims expressed in this article are solely those of the authors and do not necessarily represent those of their affiliated organizations, or those of the publisher, the editors and the reviewers. Any product that may be evaluated in this article, or claim that may be made by its manufacturer, is not guaranteed or endorsed by the publisher.
References
Addison, P. F. E., Collins, D. J., Trebilco, R., Howe, S., Bax, N., Hedge, P., et al. (2018). A new wave of marine evidence-based management: emerging challenges and solutions to transform monitoring, evaluating, and reporting. ICES J. Mar. Sci. 75, 941–952. doi: 10.1093/icesjms/fsx216
Aksnes, D. L., Dupont, N., Staby, A., Fiksen, Ø., Kaartvedt, S., Aure, J., et al. (2009). Coastal water darkening and implications for mesopelagic regime shifts in Norwegian fjords. Mar. Ecol. Prog. Ser. 387, 39–49. doi: 10.3354/meps08120
Andersen, M. S., Levin, G., and Odgaard, M. V. (2019). Economic benefits of reducing agricultural N losses to coastal waters for seaside recreation and real estate value in Denmark. Mar. Pollut. Bull. 140, 146–156. doi: 10.1016/j.marpolbul.2019.01.010
Anderson, D. M., Glibert, P. M., and Burkholder, J. M. (2002). Harmful algal blooms and eutrophication: nutrient sources, composition, and consequences. Estuaries 25, 704–726. doi: 10.1007/BF02804901
Arheimer, B., Dahné, J., and Donnelly, C. (2012). Climate change impact on riverine nutrient load and land-based remedial measures of the Baltic Sea Action Plan. Ambio 41, 600–612. doi: 10.1007/s13280-012-0323-0
Atkins, J. P., Burdon, D., Elliott, M., and Gregory, A. J. (2011a). Management of the marine environment: integrating ecosystem services and societal benefits with the DPSIR framework in a systems approach. Mar. Pollut. Bull. 62, 215–226. doi: 10.1016/j.marpolbul.2010.12.012
Atkins, J. P., Gregory, A. J., Burdon, D., and Elliott, M. (2011b). Managing the marine environment: is the DPSIR framework holistic enough? Syst. Res. Behav. Sci. 28, 497–508. doi: 10.1002/sres.1111
Axe, P., Sonesten, L., Skarbövik, E., Leujak, W., and Nielsen, L. (2022). “Inputs of nutrients to the OSPAR maritime area,” in OSPAR, 2023: The 2023 Quality Status Report for the North-East Atlantic (London: OSPAR Commission). Available online at: https://oap.ospar.org/en/ospar-assessments/quality-status-reports/qsr-2023/indicator-assessments/inputs-nutrients/ (accessed April 26, 2025).
Bean, T. P., Greenwood, N., Beckett, R., Biermann, L., Bignell, J. P., Brant, J. L., et al. (2017). A review of the tools used for marine monitoring in the UK: combining historic and contemporary methods with modelling and socioeconomics to fulfil legislative needs and scientific ambitions. Front. Mar. Sci. 4:263. doi: 10.3389/fmars.2017.00263
Bedford, J., Johns, D., Greenstreet, S., and McQuatters-Gollop, A. (2018). Plankton as prevailing conditions: a surveillance role for plankton indicators within the Marine Strategy Framework Directive. Mar. Policy 89, 109–115. doi: 10.1016/j.marpol.2017.12.021
Bedford, J., Ostle, C., Johns, D. G., Atkinson, A., Best, M., Bresnan, E., et al. (2020). Lifeform indicators reveal large-scale shifts in plankton across the North-West European shelf. Glob. Change Biol. 26, 3482–3497. doi: 10.1111/gcb.15066
Best, M. A., Wither, A. W., and Coates, S. (2007). Dissolved oxygen as a physico-chemical supporting element in the Water Framework Directive. Mar. Pollut. Bull. 55, 53–64. doi: 10.1016/j.marpolbul.2006.08.037
Bischof, B. G. (2010). Negotiating uncertainty: framing attitudes, prioritizing issues, and finding consensus in the coral reef environment management “crisis.” Ocean Coast Manag. 53, 597–614. doi: 10.1016/j.ocecoaman.2010.06.020
Borja, Á. (2005). The European water framework directive: a challenge for nearshore, coastal and continental shelf research. Cont. Shelf Res. 25, 1768–1783. doi: 10.1016/j.csr.2005.05.004
Borja, A., Berg, T., Gundersen, H., Hagen, A. G., Hancke, K., Korpinen, S., et al. (2024). Innovative and practical tools for monitoring and assessing biodiversity status and impacts of multiple human pressures in marine systems. Environ. Monit. Assess. 196:694. doi: 10.1007/s10661-024-12861-2
Borja, Á., Elliott, M., Carstensen, J., Heiskanen, A. S., and van de Bund, W. (2010). Marine management - towards an integrated implementation of the European marine strategy framework and the water framework directives. Mar. Pollut. Bull. 60, 2175–2186. doi: 10.1016/j.marpolbul.2010.09.026
Borja, Á., Franco, J., Valencia, V., Bald, J., Muxika, I., Belzunce, M. J., et al. (2004). Implementation of the European water framework directive from the Basque country (northern Spain): a methodological approach. Mar. Pollut. Bull. 48, 209–218. doi: 10.1016/j.marpolbul.2003.12.001
Borja, A., Galparsoro, I., Solaun, O., Muxika, I., Tello, E. M., Uriarte, A., et al. (2006). The European Water Framework Directive and the DPSIR, a methodological approach to assess the risk of failing to achieve good ecological status. Estuar. Coast. Shelf Sci. 66, 84–96. doi: 10.1016/j.ecss.2005.07.021
Bowen, R. E., and Riley, C. (2003). Socio-economic indicators and integrated coastal management. Ocean Coast. Manag. 46, 299–312. doi: 10.1016/S0964-5691(03)00008-5
Brander, K. M., Ottersen, G., Bakker, J. P., Beaugrand, G., Herr, H., Garthe, S., et al. (2016). “Environmental impacts—marine ecosystems,” in North Sea Region Climate Change Assessment, eds. M. Quante, and F. Colijn (Cham: Springer), 241–274. doi: 10.1007/978-3-319-39745-0_8
Bricker, S., and Devlin, M. (2011). Preface: “eutrophication” issue of biogeochemistry. Biogeochemistry 106, 135–136. doi: 10.1007/s10533-011-9646-3
Bricker, S. B., Ferreira, J. G., and Simas, T. (2003). An integrated methodology for assessment of estuarine trophic status. Ecol. Modell. 169, 39–60. doi: 10.1016/S0304-3800(03)00199-6
Brodie, J., Wolanski, E., Lewis, S., and Bainbridge, Z. (2012). An assessment of residence times of land-sourced contaminants in the Great Barrier Reef lagoon and the implications for management and reef recovery. Mar. Pollut. Bull. 65, 267–279. doi: 10.1016/j.marpolbul.2011.12.011
Brodie, J. E., Devlin, M., Haynes, D., and Waterhouse, J. (2011). Assessment of the eutrophication status of the Great Barrier Reef lagoon (Australia). Biogeochemistry 106, 281–302. doi: 10.1007/s10533-010-9542-2
Bussi, G., Whitehead, P. G., Bowes, M. J., Read, D. S., Prudhomme, C., Dadson, S. J., et al. (2016). Impacts of climate change, land-use change and phosphorus reduction on phytoplankton in the River Thames (UK). Sci. Total Environ. 572, 1507–1519. doi: 10.1016/j.scitotenv.2016.02.109
Capuzzo, E., Lynam, C. P., Barry, J., Stephens, D., Forster, R. M., Greenwood, N., et al. (2018). A decline in primary production in the North Sea over 25 years, associated with reductions in zooplankton abundance and fish stock recruitment. Global Change Biol. 24, e352–e364. doi: 10.1111/gcb.13916
Carder, K. L., Steward, R. G., Harvey, G. R., and Ortner, P. B. (1989). Marine humic and fulvic acids: their effects on remote sensing of ocean chlorophyll. Limnol. Oceanogr. 34, 68–81. doi: 10.4319/lo.1989.34.1.0068
Carolina, N. (2002). Harmful algal blooms and eutrophication nutrient sources, composition, and consequences. Estuaries 25, 704–726.
Carpenter, S. R., Caraco, N. F., Correll, D. L., Howarth, R. W., and Sharpley, A. N. (1998). Nonpoint pollution of surface waters with phosphorus and nitrogen. Ecol. Appl. 8, 559–568. doi: 10.1890/1051-0761(1998)008[0559:NPOSWW]2.0.CO;2
Carstensen, J., Sánchez-Camacho, M., Duarte, C. M., Krause-Jensen, D., and Marbà, N. (2011). Connecting the dots: responses of coastal ecosystems to changing nutrient concentrations. Environ. Sci. Technol. 45, 9122–9132. doi: 10.1021/es202351y
Chen, K., Achterberg, E. P., Li, K., Zhang, J., Xin, M., Wang, X., et al. (2024). Governance pathway for coastal eutrophication based on regime shifts in diatom–dinoflagellate composition of the Bohai and Baltic Seas. Water Res. 250:121042. doi: 10.1016/j.watres.2023.121042
Cigliano, J. A., Meyer, R., Ballard, H. L., Freitag, A., Phillips, T. B., Wasser, A., et al. (2015). Making marine and coastal citizen science matter. Ocean Coast. Manag. 115, 77–87. doi: 10.1016/j.ocecoaman.2015.06.012
Claussen, U., Zevenboom, W., Brockmann, U., Topcu, D., and Bot, P. (2009). “Assessment of the eutrophication status of transitional, coastal and marine waters within OSPAR,” in Eutrophication in Coastal Ecosystems: Towards better understanding and management strategies Selected Papers from the Second International Symposium on Research and Management of Eutrophication in Coastal Ecosystems, 20–23 June 2006, Nyborg, Denmark (Cham: Springer), 49–58. doi: 10.1007/978-90-481-3385-7_5
Cloern, J. E. (1987). Turbidity as a control on phytoplankton biomass and productivity in estuaries. Cont. Shelf Res. 7, 1367–1381. doi: 10.1016/0278-4343(87)90042-2
Cloern, J. E. (1999). The relative importance of light and nutrient limitation of phytoplankton growth: a simple index of coastal ecosystem sensitivity to nutrient enrichment. Aquat. Ecol. 33, 3–15. doi: 10.1023/A:1009952125558
Cloern, J. E. (2001). Our evolving conceptual model of the coastal eutrophication problem. Mar. Ecol. Prog. Ser. 210, 223–253. doi: 10.3354/meps210223
Cloern, J. E., and Jassby, A. D. (2009). Patterns and scales of phytoplankton variability in estuarine–coastal ecosystems. Estuaries Coasts 33, 230–241. doi: 10.1007/s12237-009-9195-3
COM (2017). Commission Decision (EU) 2017/848 of 17 May 2017, laying down criteria and methodological standards on good environmental status of marine waters and specifications and standardised methods for monitoring and assessment, and repealing Decision 2010/447/EU. Official J. EU L 125, 43–74. Available online at: https://eur-lex.europa.eu/legal-content/EN/TXT/PDF/?uri=CELEX:32017D0848 (accessed April 26, 2025).
Cork, S., Hall, D., and Liljebjelke, K. (2016). One Health Case Studies: Addressing Complex Problems in a Changing World. Great Easton: 5m Books Ltd.
Cotterill, D. F., Pope, J. O., and Stott, P. A. (2023). Future extension of the UK summer and its impact on autumn precipitation. Clim. Dyn. 60, 1801–1814.
Creighton, C., Waterhouse, J., Day, J. C., and Brodie, J. (2021). Criteria for effective regional scale catchment to reef management: a case study of Australia's Great Barrier Reef. Mar. Pollut. Bull. 173:112882. doi: 10.1016/j.marpolbul.2021.112882
Dafforn, K. A., Johnston, E. L., Ferguson, A., Humphrey, C. L., Monk, W., Nichols, S. J., et al. (2016). Big data opportunities and challenges for assessing multiple stressors across scales in aquatic ecosystems. Mar. Freshw. Res. 67, 393–413. doi: 10.1071/MF15108
Daniel, A., Laës-Huon, A., Barus, C., Beaton, A. D., Blandfort, D., Guigues, N., et al. (2020). Toward a harmonization for using in situ nutrient sensors in the marine environment. Front. Mar. Sci. 6:773. doi: 10.3389/fmars.2019.00773
Darling, E. S., McClanahan, T. R., Maina, J., Gurney, G. G., Graham, N. A., Januchowski-Hartley, F., et al. (2019). Social–environmental drivers inform strategic management of coral reefs in the Anthropocene. Nat. Ecol. Evol. 3, 1341–1350. doi: 10.1038/s41559-019-0953-8
Davidson, K., Gowen, R. J., Tett, P., Bresnan, E., Harrison, P. J., McKinney, A., et al. (2012). Harmful algal blooms: how strong is the evidence that nutrient ratios and forms influence their occurrence? Estuar. Coast. Shelf Sci. 115, 399–413. doi: 10.1016/j.ecss.2012.09.019
Davies, T. W., Levy, O., Tidau, S., de Barros Marangoni, L. F., Wiedenmann, J., D'Angelo, C., et al. (2023). Global disruption of coral broadcast spawning associated with artificial light at night. Nat. Commun. 14:2511. doi: 10.1038/s41467-023-38070-y
de Raús Maúre E. Terauchi G. Ishizaka J. Clinton N. and DeWitt M. (2021). Globally consistent assessment of coastal eutrophication. Nat. Commun. 12:6142. doi: 10.1038/s41467-021-26391-9
DEFRA (2012). Marine Strategy Part One: UK Initial Assessment and Good Environmental Status. Available online at: https://assets.publishing.service.gov.uk/government/uploads/system/uploads/attachment_data/file/69632/pb13860-marine-strategy-part1-20121220.pdf (accessed March 28, 2025).
DEFRA (2019). Marine Strategy Part One: UK Updated Assessment and Good Environmental Status. Available online at: https://assets.publishing.service.gov.uk/media/5f6c8369d3bf7f7238f23151/marine-strategy-part1-october19.pdf (accessed April 26, 2025).
Desmit, X., Nohe, A., Borges, A. V., Prins, T., De Cauwer, K., and Lagring, K. (2020). Changes in chlorophyll concentration and phenology in the North Sea in relation to de-eutrophication and sea surface warming. Limnol. Oceanogr. 65, 828–847. doi: 10.1002/lno.11351
Desmit, X., Schartau, M., Riethmüller, R., Terseleer, N., Van der Zande, D., Fettweis, M., et al. (2024). The transition between coastal and offshore areas in the North Sea unraveled by suspended particle composition. Sci. Total Environ. 915:169966. doi: 10.1016/j.scitotenv.2024.169966
Devlin, M., Barry, J., and Painting, Æ. S. (2009). Extending the phytoplankton tool kit for the UK Water Framework Directive: indicators of phytoplankton community structure. Hydrobiologia 633, 151–168. doi: 10.1007/s10750-009-9879-5
Devlin, M., Best, M., Bresnan, E., Scanlan, C., and Baptie, M. (2012a). “Water Framework Directive: the development and status of phytoplankton tools for ecological assessment of coastal and transitional waters,” in Water Framework Directive–United Kingdom Technical Advisory Group (WFD-UKTAG).
Devlin, M., Best, M., and Haynes, D. (2007a). Implementation of the Water Framework Directive in European marine waters. Mar. Pollut. Bull. 55, 1–2. doi: 10.1016/j.marpolbul.2006.09.020
Devlin, M., Bricker, S., and Painting, S. (2011). Comparison of five methods for assessing impacts of nutrient enrichment using estuarine case studies. Biogeochemistry 106, 177–205. doi: 10.1007/s10533-011-9588-9
Devlin, M., and Brodie, J. (2023). “Nutrients and eutrophication,” in Marine Pollution–Monitoring, Management and Mitigation, ed. A. Reichelt-Brushett (Cham: Springer), 75–100. doi: 10.1007/978-3-031-10127-4_4
Devlin, M., Fernand, L., and Collingridge, K. (2022). “Concentrations of dissolved oxygen near the seafloor in the greater North Sea, Celtic Seas and Bay of Biscay and Iberian Coast,” in OSPAR, 2023: The 2023 Quality Status Report for the North-East Atlantic (London: OSPAR Commission). Available online at: https://oap.ospar.org/en/ospar-assessments/quality-status-reports/qsr-2023/indicator-assessments/seafloor-dissolved-oxygen/ (accessed November 18, 2024).
Devlin, M., Painting, S., and Best, M. (2007b). Setting nutrient thresholds to support an ecological assessment based on nutrient enrichment, potential primary production and undesirable disturbance. Mar. Pollut. Bull. 55, 65–73. doi: 10.1016/j.marpolbul.2006.08.030
Devlin, M., Schroeder, T., McKinna, L., Brodie, J., Brando, V., Dekker, A., et al. (2012b). “Monitoring and mapping of flood plumes in the Great Barrier Reef based on in situ and remote sensing observations,” in Environmental Remote Sensing and Systems Analysis, ed. N.-B. Chang (Boca Raton, FL: CRC Prress), 147–191.
Devlin, M., and Wenger, A. (2024). “Question 8.2 What are the key attributes of successful monitoring and evaluation programs to support coastal and marine water quality management, and what examples are there of innovative monitoring and evaluation frameworks, methods and approaches that are applicable to the Great Barrier Reef?” in 2022 Scientific Consensus Statement on land-based impacts on Great Barrier Reef water quality and ecosystem condition, eds. J. Waterhouse, M.-C. Pineda, and K. Sambrook (Townsville: C2O Consulting), 48.
Devlin, M. J., Barry, J., Mills, D. K., Gowen, R. J., Foden, J., Sivyer, D., et al. (2008). Relationships between suspended particulate material, light attenuation and Secchi depth in UK marine waters. Estuar. Coast. Shelf Sci. 79, 429–439. doi: 10.1016/j.ecss.2008.04.024
Devlin, M. J., Da Silva, E. T., Petus, C., Wenger, A., Zeh, D., and Tracey, J. (2013). Combining in-situ water quality and remotely sensed data across spatial and temporal scales to measure variability in wet season chlorophyll-a: Great Barrier Reef lagoon (Queensland, Australia). Ecol. Process. 2, 1–22. doi: 10.1186/2192-1709-2-31
Devlin, M. J., Petus, C., Da Silva, E., Tracey, D., Wolff, N. H., Waterhouse, J., et al. (2015). Water quality and river plume monitoring in the Great Barrier Reef: an overview of methods based on ocean colour satellite data. Remote Sens. 7, 12909–12941. doi: 10.3390/rs71012909
Devlin, M. J., Prins, T. C., Enserink, L., Leujak, W., Heyden, B., Axe, P. G., et al. (2023). A first ecological coherent assessment of eutrophication across the North-East Atlantic waters (2015–2020). Front. Ocean Sustain. 1:1253923. doi: 10.3389/focsu.2023.1253923
Diaz, R. J., and Rosenberg, R. (2008). Spreading dead zones and consequences for marine ecosystems. Science 321, 926–929. doi: 10.1126/science.1156401
Dory, F., Nava, V., Spreafico, M., Orlandi, V., Soler, V., Leoni, B., et al. (2024). Interaction between temperature and nutrients: how does the phytoplankton community cope with climate change? Sci. Total Environ. 906:167566. doi: 10.1016/j.scitotenv.2023.167566
Duarte, C. M. (2009). Coastal eutrophication research: a new awareness. Hydrobiologia 629, 263–269. doi: 10.1007/s10750-009-9795-8
Duarte, C. M., Conley, D. J., Carstensen, J., and Sánchez-Camacho, M. (2009). Return to Neverland: Shifting baselines affect eutrophication restoration targets. Estuaries Coasts 32, 29–36. doi: 10.1007/s12237-008-9111-2
Duhamel, S., Kim, E., Sprung, B., and Anderson, O. R. (2019). Small, pigmented eukaryotes play a major role in carbon cycling in the P-depleted western subtropical North Atlantic, which may be supported by mixotrophy. Limnol. Oceanogr. 64, 2424–2440. doi: 10.1002/lno.11193
Dupont, N., and Aksnes, D. L. (2013). Centennial changes in water clarity of the baltic sea and the north sea. Estuar. Coast. Shelf Sci. 131, 282–289. doi: 10.1016/j.ecss.2013.08.010
ECJ (2004). Commission of the European Communities v French Republic. Judgement of the European Court of Justice (2nd chamber) on 23 September 2004, in case C-280/02, concerning: Failure of a Member State to fulfil obligations - Directive 91/271/EEC - Urban wastewater treatment - Article 5(1) and (2) and Annex II - Failure to identify sensitive areas - Meaning of ‘eutrophication' - Failure to implement more stringent treatment of discharges into sensitive areas. Report of judgement. Luxembourg.
ECJ (2009). Commission of the European Communities v United Kingdom supported by Portuguese Republic. Judgement of the European Court of Justice (3rd chamber) on 10 December 2009, In Case C-390/07, under Article 226 EC for failure to fulfil obligations, pursuant to Articles 3(1) and (2) and 5(1) to (3) and (5) of, and Annex II to, Council Directive 91/271/EEC of 21 May 1991 concerning urban wastewater treatment (OJ 1991 L 135, p. 40). Report of judgement. Luxembourg.
Edwards, M., Beaugrand, G., Hays, G. C., Koslow, J. A., and Richardson, A. J. (2010). Multi-decadal oceanic ecological datasets and their application in marine policy and management. Trends Ecol. Evol. 25, 602–610. doi: 10.1016/j.tree.2010.07.007
Edwards, P. (2015). Aquaculture environment interactions: past, present and likely future trends. Aquaculture 447, 2–14. doi: 10.1016/j.aquaculture.2015.02.001
Eker-Develi, E., Kideys, A. E., Mikaelyan, A., Devlin, M. J., and Newton, A. (2022). Phytoplankton dynamics under climate change. Front. Mar. Sci. 9:869618. doi: 10.3389/fmars.2022.869618
El Serafy, G., Mészáros, L., Fernández, V., Capet, A., She, J., Sotillo, M. G., et al. (2023). EuroGOOS roadmap for operational coastal downstream services. Front. Mar. Sci. 10:1177615. doi: 10.3389/fmars.2023.1177615
Elliott, M., Burdon, D., Atkins, J. P., Borja, A., Cormier, R., De Jonge, V. N., et al. (2017). “And DPSIR begat DAPSI (W) R (M)!”-a unifying framework for marine environmental management. Mar. Pollut. Bull. 118, 27–40. doi: 10.1016/j.marpolbul.2017.03.049
Engelhard, G. H., Pinnegar, J. K., Kell, L. T., and Rijnsdorp, A. D. (2011). Nine decades of North Sea sole and plaice distribution. ICES J. Mar. Sci. 68, 1090–1104. doi: 10.1093/icesjms/fsr031
European Commission (1991a). Council Directive 91/271/EEC of 21 May 1991 concerning urban waste-water treatment. Off. J. Eur. Union L 135, 40–52.
European Commission (1991b). Council Directive 91/676/EEC of 12 December 1991 concerning the protection of waters against pollution caused by nitrates from agricultural sources. Off. J. Eur. Union L 375, 1–8.
European Commission (2000). Directive 2000/60/EC of the European Parliament and of the Council of 23 October 2000 establishing a framework for Community action in the field of water policy. Off. J. Eur. Union L 327, 1–73.
European Commission (2006). Directive 2006/7/EC of the European Parliament and of the Council of 15 February 2006 concerning the management of bathing water quality and repealing Directive 76/160/EEC. Off. J. Eur. Union L 64, 37–51.
European Commission (2008). Directive 2008/56/EC of the European Parliament and of the Council of 17 June 2008 establishing a framework for community action in the field of marine environmental policy (Marine Strategy Framework Directive) (Text with EEA relevance). Off. J. Eur. Union L 164, 19–40.
Ferreira, J. G., Andersen, J. H., Borja, A., Bricker, S. B., Camp, J., da Silva, M. C., et al. (2011). Overview of eutrophication indicators to assess environmental status within the European Marine Strategy Framework Directive. Estuar. Coast. Shelf Sci. 93, 117–131. doi: 10.1016/j.ecss.2011.03.014
Fettweis, M., Riethmüller, R., Van der Zande, D., and Desmit, X. (2023). Sample based water quality monitoring of coastal seas: how significant is the information loss in patchy time series compared to continuous ones? Sci. Total Environ. 873:162273. doi: 10.1016/j.scitotenv.2023.162273
Figueras, M. J., Polo, F., Inza, I., and Guarro, J. (1997). Past, present and future perspectives of the EU Bathing Water Directive. Mar. Pollut. Bull. 34, 148–156. doi: 10.1016/S0025-326X(97)00107-0
Foden, J., Devlin, M. J., Mills, D. K., and Malcolm, S. J. (2011). Searching for undesirable disturbance: an application of the OSPAR eutrophication assessment method to marine waters of England and Wales. Biogeochemistry 106, 157–175. doi: 10.1007/s10533-010-9475-9
Foden, J., Scanlan, C., Wells, E., Wood, P., Wilkinson, M., Best, M., et al. (2005). Opportunistic macroalgal blooms and the water framework directive. Mar. Ecol. 3:2005.
Foden, J., Sivyer, D. B., Mills, D. K., and Devlin, M. J. (2008). Spatial and temporal distribution of chromophoric dissolved organic matter (CDOM) fluorescence and its contribution to light attenuation in UK waterbodies. Estuar. Coast. Shelf Sci. 79, 707–717. doi: 10.1016/j.ecss.2008.06.015
Frenken, T., Brandenburg, K. M., and Van de Waal, D. B. (2023). Long-term nutrient load reductions and increasing lake TN : TP stoichiometry decrease phytoplankton biomass and diversity in a large shallow lake. Limnol. Oceanogr. 68:12428. doi: 10.1002/lno.12428
Frigstad, H., Andersen, G. S., Trannum, H. C., McGovern, M., Naustvoll, L. J., Kaste, Ø., et al. (2023). Three decades of change in the Skagerrak coastal ecosystem, shaped by eutrophication and coastal darkening. Estuar. Coast. Shelf Sci. 283:108193. doi: 10.1016/j.ecss.2022.108193
Fronkova, L., Greenwood, N., Martinez, R., Graham, J. A., Harrod, R., Graves, C. A., et al. (2022). Can Forel–Ule index act as a proxy of water quality in temperate waters? Application of plume mapping in Liverpool Bay, UK. Remote Sens. 14:2375. doi: 10.3390/rs14102375
García-García, L. M., Sivyer, D., Devlin, M., Painting, S., Collingridge, K., Van der Molen, J., et al. (2019). Optimizing monitoring programs: a case study based on the OSPAR eutrophication assessment for UK waters. Front. Mar. Sci. 5:503. doi: 10.3389/fmars.2018.00503
Glibert, P. M. (2017). Eutrophication, harmful algae and biodiversity — challenging paradigms in a world of complex nutrient changes. Mar. Pollut. Bull. 124, 591–606. doi: 10.1016/j.marpolbul.2017.04.027
Glibert, P. M., and Burford, M. A. (2017). Globally changing nutrient loads and harmful algal blooms: recent advances, new paradigms, and continuing challenges. Oceanography 30, 58–69. doi: 10.5670/oceanog.2017.110
Gonçalves Leles, S., Polimene, L., Bruggeman, J., Blackford, J., Ciavatta, S., Mitra, A., et al. (2018). Modelling mixotrophic functional diversity and implications for ecosystem function. J. Plankton Res. 40:fby044. doi: 10.1093/plankt/fby044
Gowen, R. J., Tett, P., and Smayda, T. J. (2012). Phytoplankton and the balance of nature: an opinion. Estuar. Coast. Shelf Sci. 113, 317–323. doi: 10.1016/j.ecss.2012.08.009
Granéli, E., and Turner, J. T. (2002). Top-down regulation in ctenophore-copepod-ciliate-diatom-phytoflagenate communities in coastal waters: a mesocosm study. Mar. Ecol. Prog. Ser. 239, 57–68. doi: 10.3354/meps239057
Graves, C. A., Best, M., Atkinson, A., Bear, B., Bresnan, E., Holland, M., et al. (2023). At what scale should we assess the health of pelagic habitats? Trade-offs between small-scale manageable pressures and the need for regional upscaling. Ecol. Indic. 154:110571. doi: 10.1016/j.ecolind.2023.110571
Greenwood, N., Devlin, M. J., Best, M., Fronkova, L., Graves, C., Milligan, A., et al. (2019). Utilising eutrophication assessment directives from freshwater to marine systems in the Thames estuary and Liverpool Bay, UK. Front. Mar. Sci. 6:116. doi: 10.3389/fmars.2019.00116
Greenwood, N., Parker, E. R., Fernand, L., Sivyer, D. B., Weston, K., Painting, S. J., et al. (2010). Detection of low bottom water oxygen concentrations in the North Sea; implications for monitoring and assessment of ecosystem health. Biogeosciences 7, 1357–1373. doi: 10.5194/bg-7-1357-2010
Gross, C. J. D., and Hagy, J. D. (2017). Attributes of successful actions to restore lakes and estuaries degraded by nutrient pollution. J. Environ. Manage. 187, 122–136. doi: 10.1016/j.jenvman.2016.11.018
Harvey, E. T., Kratzer, S., and Philipson, P. (2015). Satellite-based water quality monitoring for improved spatial and temporal retrieval of chlorophyll-a in coastal waters. Remote Sens. Environ. 158, 417–430. doi: 10.1016/j.rse.2014.11.017
Heal, R., Fronkova, L., Silva, T., Collingridge, K., Harrod, R., Greenwood, N., et al. (2023). A probabilistic approach to mapping the contribution of individual riverine discharges into liverpool bay using distance accumulation cost methods on satellite derived ocean-colour data. Remote Sens. 15:3666. doi: 10.3390/rs15143666
Heiskanen, A.-S., and Carletti, A., (eds.). (2009). Water Framework Directive intercalibration technical report. Part 3, Coastal and transitional waters. Joint Research Centre: Institute for Environment and Sustainability. Available online at: https://data.europa.eu/doi/10.2788/19561
Heyden, B., and Leujak, W. (2022). “Winter nutrient concentrations in the greater North Sea, Celtic Seas and Bay of Biscay and Iberian Coast,” in OSPAR, 2023: The 2023 Quality Status Report for the Northeast Atlantic (London: OSPAR Commission). Available online at: https://oap.ospar.org/en/ospar-assessments/quality-status-reports/qsr-2023/indicator-assessments/winter-nutrient-concentrations/ (accessed November 18, 2024).
Holland, M., Louchart, A., Artigas, L. F., and Mcquatters-Gollop, A. (2023a). “Changes in phytoplankton and zooplankton communities common indicator assessment changes in phytoplankton and zooplankton communities,” in OSPAR, 2023: The 2023 Quality Status Report for the Northeast Atlantic.
Holland, M. M., Artigas, L. F., Atkinson, A., Best, M., Bresnan, E., Devlin, M., et al. (2025). Mind the gap-the need to integrate novel plankton methods alongside ongoing long-term monitoring. Ocean Coast. Manag. 262:107542. doi: 10.1016/j.ocecoaman.2025.107542
Holland, M. M., Atkinson, A., Best, M., Bresnan, E., Devlin, M., Goberville, E., et al. (2024). Predictors of long-term variability in NE atlantic plankton communities. Sci. Total Environ. 952:175793. doi: 10.1016/j.scitotenv.2024.175793
Holland, M. M., Louchart, A., Artigas, L. F., Ostle, C., Atkinson, A., Rombouts, I., et al. (2023b). Major declines in NE Atlantic plankton contrast with more stable populations in the rapidly warming North Sea. Sci. Total Environ. 898:165505. doi: 10.1016/j.scitotenv.2023.165505
Holmer, M. (2010). Environmental issues of fish farming in offshore waters: perspectives, concerns and research needs. Aquac. Environ. Interact. 1, 57–70. doi: 10.3354/aei00007
Howarth, R. W. (2008). Coastal nitrogen pollution: a review of sources and trends globally and regionally. Harmful Algae 8, 14–20. doi: 10.1016/j.hal.2008.08.015
Hull, T., Greenwood, N., Birchill, A., Beaton, A., Palmer, M., Kaiser, J., et al. (2021). Simultaneous assessment of oxygen-and nitrate-based net community production in a temperate shelf sea from a single ocean glider. Biogeosciences 18, 6167–6180. doi: 10.5194/bg-18-6167-2021
Hull, T., Johnson, M., Greenwood, N., and Kaiser, J. (2020). Bottom mixed layer oxygen dynamics in the Celtic Sea. Biogeochemistry 149, 263–289. doi: 10.1007/s10533-020-00662-x
Ibáñez, C., Caiola, N., Barquín, J., Belmar, O., Benito-Granell, X., Casals, F., et al. (2023). Ecosystem-level effects of re-oligotrophication and N:P imbalances in rivers and estuaries on a global scale. Glob. Chang. Biol. 29:16520. doi: 10.1111/gcb.16520
Ivanov, E., Capet, A., Barth, A., Delhez, E. J. M., Soetaert, K., Grégoire, M., et al. (2020). Hydrodynamic variability in the Southern Bight of the North Sea in response to typical atmospheric and tidal regimes. Benefit of using a high resolution model. Ocean Model 154:101682. doi: 10.1016/j.ocemod.2020.101682
Johnson, D., Fletcher, S. W., and Bray, K. (2007). Integrated assessment of nitrate concentrations for the designation of groundwater Nitrate Vulnerable Zones in England and Wales. Q. J. Eng. Geol. Hydrogeol. 40, 407–415. doi: 10.1144/1470-9236/07-023
Joo, M., Raymond, M. A. A., McNeil, V. H., Huggins, R., Turner, R. D. R., Choy, S., et al. (2012). Estimates of sediment and nutrient loads in 10 major catchments draining to the Great Barrier Reef during 2006-2009. Mar. Pollut. Bull. 65, 150–166. doi: 10.1016/j.marpolbul.2012.01.002
Kemp, R. (2001). Implementation of the urban wastewater treatment directive (91/271/EEC) in Germany, the Netherlands, Spain, England and Wales. The tangible results. Eur. Environ. 11:272. doi: 10.1002/eet.272
Kermagoret, C., Claudet, J., Derolez, V., Nugues, M. M., Ouisse, V., Quillien, N., et al. (2019). How does eutrophication impact bundles of ecosystem services in multiple coastal habitats using state-and-transition models. Ocean Coast. Manag. 174, 144–153. doi: 10.1016/j.ocecoaman.2019.03.028
Klemas, V. (2011). Remote sensing techniques for studying coastal ecosystems: an overview. J. Coast Res. 27, 2–17. doi: 10.2112/JCOASTRES-D-10-00103.1
Kristensen, P. (2004). The DPSIR Framework, European Topic Centre on Water. Denmark: European Environment Agency. National Environmental Research Institute, Department of Policy Analysis, 1–10.
Lancelot, C., Rousseau, V., and Gypens, N. (2009). Ecologically based indicators for Phaeocystis disturbance in eutrophied Belgian coastal waters (Southern North Sea) based on field observations and ecological modelling. J. Sea Res. 61, 44–49. doi: 10.1016/j.seares.2008.05.010
Langmead, O., McQuatters-Gollop, A., and Mee, L. (2007). European Lifestyles and Marine Ecosystems: Exploring Challenges for Managing Europe's Seas. University of Plymouth.
Lapointe, B. E., Brewton, R. A., Herren, L. W., Porter, J. W., and Hu, C. (2019). Nitrogen enrichment, altered stoichiometry, and coral reef decline at Looe Key, Florida Keys, USA: a 3-decade study. Mar. Biol. 166:108. doi: 10.1007/s00227-019-3538-9
Lapointe, B. E., Herren, L. W., Brewton, R. A., and Alderman, P. K. (2020). Nutrient over-enrichment and light limitation of seagrass communities in the Indian River Lagoon, an urbanized subtropical estuary. Sci. Total Environ. 699:134068. doi: 10.1016/j.scitotenv.2019.134068
Laurent, A., Fennel, K., Ko, D. S., and Lehrter, J. (2018). Climate change projected to exacerbate impacts of coastal Eutrophication in the Northern Gulf of Mexico. J. Geophys. Res. Oceans 123, 3408–3426. doi: 10.1002/2017JC013583
Lavigne, H., Van der Zande, D., Ruddick, K., Cardoso Dos Santos, J. F., Gohin, F., Brotas, V., et al. (2021). Quality-control tests for OC4, OC5 and NIR-red satellite chlorophyll-a algorithms applied to coastal waters. Remote Sens. Environ. 255:112237. doi: 10.1016/j.rse.2020.112237
Le Gentil, E., and Mongruel, R. (2015). A systematic review of socio-economic assessments in support of coastal zone management (1992–2011). J. Environ. Manage. 149, 85–96. doi: 10.1016/j.jenvman.2014.10.018
Le Moal, M., Gascuel-Odoux, C., Ménesguen, A., Souchon, Y., Étrillard, C., Levain, A., et al. (2019). Eutrophication: a new wine in an old bottle? Sci. Total Environ. 651, 1–11. doi: 10.1016/j.scitotenv.2018.09.139
Lenhart, H. J., Mills, D. K., Baretta-Bekker, H., van Leeuwen, S. M., der Molen, J., van, Baretta, J. W., et al. (2010). Predicting the consequences of nutrient reduction on the eutrophication status of the North Sea. J. Mar. Syst. 81, 148–170. doi: 10.1016/j.jmarsys.2009.12.014
Loiselle, S., Bishop, I., Moorhouse, H., Pilat, C., Koelman, E., Nelson, R., et al. (2024). Citizen scientists filling knowledge gaps of phosphate pollution dynamics in rural areas. Environ. Monit. Assess. 196:20. doi: 10.1007/s10661-024-12389-5
Lu, C., and Tian, H. (2017). Global nitrogen and phosphorus fertilizer use for agriculture production in the past half century: shifted hot spots and nutrient imbalance. Earth Syst. Sci. Data 9, 181–192. doi: 10.5194/essd-9-181-2017
Lu, X., Yu, W., Chen, B., Ma, Z., Chen, G., Ge, F., et al. (2023). Imbalanced phytoplankton C, N, P and its relationship with seawater nutrients in Xiamen Bay, China. Mar. Pollut. Bull. 187:114566. doi: 10.1016/j.marpolbul.2022.114566
Maas-Hebner, K. G., Harte, M. J., Molina, N., Hughes, R. M., Schreck, C., Yeakley, J. A., et al. (2015). Combining and aggregating environmental data for status and trend assessments: challenges and approaches. Environ. Monit. Assess. 187, 1–16. doi: 10.1007/s10661-015-4504-8
Maccarrone, V., Filiciotto, F., Buffa, G., Mazzola, S., and Buscaino, G. (2014). The ICZM Balanced Scorecard: a tool for putting integrated coastal zone management into action. Mar. Policy 44, 321–334. doi: 10.1016/j.marpol.2013.09.024
Mack, L., Attila, J., Aylagas, E., Beermann, A., Borja, A., Hering, D., et al. (2020). A synthesis of marine monitoring methods with the potential to enhance the status assessment of the Baltic Sea. Front Mar. Sci. 7:552047. doi: 10.3389/fmars.2020.552047
Mahaffey, C., Hull, T., Hunter, W., Greenwood, N., Palmer, M., Sharples, J., et al. (2023). Climate change impacts on dissolved oxygen concentration in marine and coastal waters around the uk and ireland. MCCIP Sci. Rev. 2023:31.doi: 10.14465/2023.reu07.oxy
Malcolm, S., Nedwell, D., Devlin, M., Hanlon, A., Dare, S., Parker, R., et al. (2002). “First application of the OSPAR comprehensive procedure to waters around England and Wales,” in Centre for Environment, Fisheries and Aquaculture Report to DEFRA, UK (CEFAS), 1–103.
Martin, D. M., Piscopo, A. N., Chintala, M. M., Gleason, T. R., and Berry, W. (2018). Developing qualitative ecosystem service relationships with the Driver-Pressure-State-Impact-Response framework: a case study on Cape Cod, Massachusetts. Ecol. Indic. 84, 404–415. doi: 10.1016/j.ecolind.2017.08.047
Martino, L., Yan, E., and LaFreniere, L. (2019). A hybrid phytoremediation system for contaminants in groundwater. Environ. Earth Sci. 78:664. doi: 10.1007/s12665-019-8675-4
Massarelli, C., Losacco, D., Tumolo, M., Campanale, C., and Uricchio, V. F. (2021). Protection of water resources from agriculture pollution: an integrated methodological approach for the nitrates directive 91–676-EEC implementation. Int. J. Environ. Res. Public Health 18:13323. doi: 10.3390/ijerph182413323
May, C. L., Koseff, J. R., Lucas, L. V., Cloern, J. E., and Schoellhamer, D. H. (2003). Effects of spatial and temporal variability of turbidity on phytoplankton blooms. Mar. Ecol. Prog. Ser. 254, 111–128. doi: 10.3354/meps254111
McQuatters-Gollop, A., Guérin, L., Arroyo, N. L., Aubert, A., Artigas, L. F., Bedford, J., et al. (2022). Assessing the state of marine biodiversity in the Northeast Atlantic. Ecol. Indic. 141:109148. doi: 10.1016/j.ecolind.2022.109148
McQuatters-Gollop, A., Johns, D. G., Bresnan, E., Skinner, J., Rombouts, I., Stern, R., et al. (2017). From microscope to management: the critical value of plankton taxonomy to marine policy and biodiversity conservation. Mar. Policy 83, 1–10. doi: 10.1016/j.marpol.2017.05.022
McQuatters-Gollop, A., Mitchell, I., Vina-Herbon, C., Bedford, J., Addison, P. F., Lynam, C. P., et al. (2019). From science to evidence–how biodiversity indicators can be used for effective marine conservation policy and management. Front. Mar. Sci. 6:109. doi: 10.3389/fmars.2019.00109
McQuatters-Gollop, A., Stern, R. F., Atkinson, A., Best, M., Bresnan, E., Creach, V. McQuatters-Gollop, A., et al. (2024). The silent majority: pico-and nanoplankton as ecosystem health indicators for marine policy. Ecol. Indic. 159:111650. doi: 10.1016/j.ecolind.2024.111650
Meier, H. E. M., Eilola, K., Almroth-Rosell, E., Schimanke, S., Kniebusch, M., Höglund, A., et al. (2019). Disentangling the impact of nutrient load and climate changes on Baltic Sea hypoxia and eutrophication since 1850. Clim. Dyn. 53, 1145–1166. doi: 10.1007/s00382-018-4296-y
Menden-Deuer, S., and Lessard, E. J. (2000). Carbon to volume relationships for dinoflagellates, diatoms, and other protist plankton. Limnol. Oceanogr. 45, 569–579. doi: 10.4319/lo.2000.45.3.0569
Mills, D. K., Greenwood, N., Kröger, S., Devlin, M., Sivyer, D. B., Pearce, D., et al. (2004). “New approaches to improve the detection of eutrophication in UK coastal waters,” in 2004 USA-Baltic Internation Symposium (Klaipeda: IEEE), 1–7. doi: 10.1109/BALTIC.2004.7296835
Mills, D. K., Laane, R., Rees, J. M., van der Loeff, M. R., Suylen, J. M., Pearce, D. J., et al. (2003). Smartbuoy: a marine environmental monitoring buoy with a difference. Elsevier Oceanogr. Ser. 69, 311–316. doi: 10.1016/S0422-9894(03)80050-8
Mitra, A., Flynn, K. J., Stoecker, D. K., and Raven, J. A. (2024). Trait trade-offs in phagotrophic microalgae: the mixoplankton conundrum. Eur. J. Phycol. 59, 51–70. doi: 10.1080/09670262.2023.2216259
Mohseni, F., Saba, F., Mirmazloumi, S. M., Amani, M., Mokhtarzade, M., Jamali, S., et al. (2022). Ocean water quality monitoring using remote sensing techniques: a review. Mar. Environ. Res. 180:105701. doi: 10.1016/j.marenvres.2022.105701
Newton, A., and Weichselgartner, J. (2014). Hotspots of coastal vulnerability: a DPSIR analysis to find societal pathways and responses. Estuar. Coast Shelf Sci. 140, 123–133. doi: 10.1016/j.ecss.2013.10.010
Ngatia, L., Grace, M. III., J., Moriasi, D., and Taylor, R. (2019). “Nitrogen and phosphorus eutrophication in marine ecosystems,” in Monitoring of Marine Pollution, ed. H. B. Fouzia (London: IntechOpen), 77–91. doi: 10.5772/intechopen.81869
Nohe, A., Goffin, A., Tyberghein, L., Lagring, R., De Cauwer, K., Vyverman, W., et al. (2020). Marked changes in diatom and dinoflagellate biomass, composition and seasonality in the Belgian Part of the North Sea between the 1970s and 2000s. Sci. Total Environ. 716:136316. doi: 10.1016/j.scitotenv.2019.136316
Ockenden, M. C., Hollaway, M. J., Beven, K. J., Collins, A. L., Evans, R., Falloon, P. D., et al. (2017). Major agricultural changes required to mitigate phosphorus losses under climate change. Nat. Commun. 8:161. doi: 10.1038/s41467-017-00232-0
Oesterwind, D., Rau, A., and Zaiko, A. (2016). Drivers and pressures–untangling the terms commonly used in marine science and policy. J. Environ. Manage. 181, 8–15. doi: 10.1016/j.jenvman.2016.05.058
O'Higgins, T., Cooper, P., Roth, E., Newton, A., Farmer, A., Goulding, I. C., et al. (2014). Temporal constraints on ecosystem management: definitions and examples from Europe's regional seas. Ecol. Soc. 19, 45–57. doi: 10.5751/ES-06507-190446
Olsen, L. M., Holmer, M., and Olsen, Y. (2008). Perspectives of nutrient emission from fish aquaculture in coastal waters. Literature review with evaluated state of knowledge. FHF Project 542014:87.
Opdal, A. F., Lindemann, C., and Aksnes, D. L. (2019). Centennial decline in North Sea water clarity causes strong delay in phytoplankton bloom timing. Glob. Change Biol. 25:14810. doi: 10.1111/gcb.14810
Organelli, E., Nuccio, C., Lazzara, L., Uitz, J., Bricaud, A., Massi, L., et al. (2017). On the discrimination of multiple phytoplankton groups from light absorption spectra of assemblages with mixed taxonomic composition and variable light conditions. Appl. Opt. 56, 3952–3968. doi: 10.1364/AO.56.003952
OSPAR (2003). OSPAR Integrated Report 2003 on the Eutrophication Status of the OSPAR Maritime Area Based Upon the First Application of the Comprehensive Procedure, No: 189. Available online at: https://www.ospar.org/documents?d=6962 (accessed November 18, 2024).
OSPAR (2008a). Second OSPAR Integrated Report on the Eutrophication Status of the OSPAR Maritime Area, No: 372. Available online at: https://www.ospar.org/documents?d=7107 (accessed November 18, 2023).
OSPAR (2008b). Towards the 50% reduction target for nutrients Assessment of Implementation of PARCOM Recommendations 88/2 and 89/4. ISBN 978-1-905859-49-8. London: OSPARCommission.
OSPAR (2017). Third Integrated Report on the Eutrophication Status of the OSPAR Maritime Area, No: 694. Availble online at: https://www.ospar.org/documents?d=37502 (accessed November 18, 2023).
OSPAR (2022). Revision of the Common Procedure for the Identification of the Eutrophication Status of the OSPAR Maritime Area. OSPAR Agreement 2022-07. London: OSPARCommission. Available online at: https://www.ospar.org/documents?v=49366 (accessed April 26, 2025).
OSPAR (2023). “Eutrophication thematic assessment,” in OSPAR, 2023: Quality Status Report 2023 (London: OSPAR Commission). Available online at: https://oap.ospar.org/en/ospar-assessments/quality-status-reports/qsr-2023/thematic-assessments/eutrophication/ (accessed April 26, 2025).
Ostle, C., Paxman, K., Graves, C. A., Arnold, M., Artigas, F., Atkinson, A., et al. (2021). The Plankton Lifeform Extraction Tool: a digital tool to increase the discoverability and usability of plankton time-series data. Earth Syst. Sci. Data Discuss. 2021, 1–73. doi: 10.5194/essd-13-5617-2021
Paerl, H. (2008). “Nutrient and other environmental controls of harmful cyanobacterial blooms along the freshwater–marine continuum,” in Cyanobacterial Harmful Algal Blooms: State of the Science and Research Needs, ed. H. K. Hudnell (New York, NY: Springer New York), 217–237. doi: 10.1007/978-0-387-75865-7_10
Paerl, H. W., and Piehler, M. F. (2008). Nitrogen and marine eutrophication. Nitrogen Marine Environ. 2, 529–567. doi: 10.1016/B978-0-12-372522-6.00011-6
Painting, S. J., Devlin, M. J., Malcolm, S. J., Parker, E. R., Mills, D. K., Mills, C., et al. (2007). Assessing the impact of nutrient enrichment in estuaries: susceptibility to eutrophication. Mar. Pollut. Bull. 55, 74–90. doi: 10.1016/j.marpolbul.2006.08.020
Patrício, J., Elliott, M., Mazik, K., Papadopoulou, K.-N., and Smith, C. J. (2016). DPSIR—two decades of trying to develop a unifying framework for marine environmental management? Front. Mar. Sci. 3:177. doi: 10.3389/fmars.2016.00177
Patricio-Valerio, L., Schroeder, T., Devlin, M. J., Qin, Y., and Smithers, S. (2022). A machine learning algorithm for himawari-8 total suspended solids retrievals in the great barrier reef. Remote Sens. 14:3503. doi: 10.3390/rs14143503
Pauly, D. (2019). Vanishing Fish: Shifting Baselines and the Future of Global Fisheries. Vancouver, BC: Greystone Books Ltd.
Pellerin, B. A., Stauffer, B. A., Young, D. A., Sullivan, D. J., Bricker, S. B., Walbridge, M. R., et al. (2016). Emerging tools for continuous nutrient monitoring networks: sensors advancing science and water resources protection. J. Am. Water Resour. Assoc. 52:12386. doi: 10.1111/1752-1688.12386
Penuelas, J., and Sardans, J. (2023). Human-driven global nutrient imbalances increase risks to health. Eco-Environ. Health 2, 246–251. doi: 10.1016/j.eehl.2023.08.003
Petersen, W., Petschatnikov, M., Schroeder, F., and Colijn, F. (2003). FerryBox systems for monitoring coastal waters. Elsevier Oceanogr. Ser. 69, 325–333. doi: 10.1016/S0422-9894(03)80052-1
Petersen, W., Wehde, H., Krasemann, H., Colijn, F., and Schroeder, F. (2008). FerryBox and MERIS–assessment of coastal and shelf sea ecosystems by combining in situ and remotely sensed data. Estuar. Coast. Shelf Sci. 77, 296–307. doi: 10.1016/j.ecss.2007.09.023
Petus, C., Collier, C., Devlin, M., Rasheed, M., and McKenna, S. (2014). Using MODIS data for understanding changes in seagrass meadow health: a case study in the Great Barrier Reef (Australia). Mar. Environ. Res. 98, 68–85. doi: 10.1016/j.marenvres.2014.03.006
Petus, C., Devlin, M., Thompson, A., McKenzie, L., Teixeira da Silva, E., Collier, C., et al. (2016). Estimating the exposure of coral reefs and seagrass meadows to land-sourced contaminants in river flood plumes of the Great Barrier Reef: validating a simple satellite risk framework with environmental data. Remote Sens. 8:210. doi: 10.3390/rs8030210
Petus, C., Waterhouse, J., Lewis, S., Vacher, M., Tracey, D., Devlin, M., et al. (2019). A flood of information: using Sentinel-3 water colour products to assure continuity in the monitoring of water quality trends in the Great Barrier Reef (Australia). J. Environ. Manage. 248:109255. doi: 10.1016/j.jenvman.2019.07.026
Philippart, C. J., Beukema, J. J., Cadée, G. C., Dekker, R., Goedhart, P. W., van Iperen, J. M., et al. (2007). Impacts of nutrient reduction on coastal communities. Ecosystems 10, 96–119. doi: 10.1007/s10021-006-9006-7
Pitarch, J., van der Woerd, H. J., Brewin, R. J., and Zielinski, O. (2019). Optical properties of Forel-Ule water types deduced from 15 years of global satellite ocean color observations. Remote Sens. Environ. 231:111249.
Pretty, J. N., Mason, C. F., Nedwell, D. B., and Hine, R. E. (2002). A preliminary assessment of the environmental costs of the eutrophication of fresh waters in England and Wales. Essex: University of Essex. doi: 10.1021/es020793k
Prins, T., and Enserink, L. (2022). “Concentrations of chlorophyll-a in the Greater North Sea, Celtic Seas and Bay of Biscay and Iberian Coast,” in OSPAR, 2023: The 2023 Quality Status Report for the North-East Atlantic (London: OSPAR Commission). Available online at: https://oap.ospar.org/en/ospar-assessments/quality-status-reports/qsr-2023/indicator-assessments/chl-a-concentrations/ (accessed November 18, 2024).
Rhodes, C., Bingham, A., Heard, A. M., Hewitt, J., Lynch, J., Waite, R., et al. (2017). Diatoms to human uses: linking nitrogen deposition, aquatic eutrophication, and ecosystem services. Ecosphere 8:1858. doi: 10.1002/ecs2.1858
Richardson, K., Steffen, W., Lucht, W., Bendtsen, J., Cornell, S. E., Donges, J. F., et al. (2023). Earth beyond six of nine planetary boundaries. Sci. Adv. 9:eadh2458. doi: 10.1126/sciadv.adh2458
Rockström, J., Steffen, W., Noone, K., Persson, Å., Chapin, F. S., Lambin, E. F., et al. (2009). A safe operating space for humanity. Nature 461, 472–475. doi: 10.1038/461472a
Romero, E., Peters, F., and Marrasé, C. (2012). Dynamic forcing of coastal plankton by nutrient imbalances and match-mismatch between nutrients and turbulence. Mar. Ecol. Prog. Ser. 464, 69–87. doi: 10.3354/meps09846
Ross, H., and Carter, R. W. (2013). One Health: a call to environmental managers. Australas. J. Environ. Manag. 20, 83–86. doi: 10.1080/14486563.2013.804027
Ryther, J. H., and Dunstan, W. M. (1971). Nitrogen, phosphorus, and eutrophication in the coastal marine environment 1008–1013. Science 171:1008. doi: 10.1126/science.171.3975.1008
Scavia, D., and Bricker, S. B. (2006). Coastal eutrophication assessment in the United States. Biogeochemistry 79, 187–208. doi: 10.1007/978-1-4020-5517-1_9
Schroeder, T., Devlin, M. J., Brando, V. E., Dekker, A. G., Brodie, J. E., et al. (2012). Inter-annual variability of wet season freshwater plume extent into the Great Barrier Reef lagoon based on satellite coastal ocean colour observations. Mar. Pollut. Bull. 65, 210–223.
Smayda, T. J., and Reynolds, C. S. (2003). Strategies of marine dinoflagellate survival and some rules of assembly. J. Sea Res. 49, 95–106.
Smith, A. (2000). Fitting in with Brussels: implementing the urban wastewater treatment directive in England and Wales. J. Environ. Policy Plann. 2, 115–134. doi: 10.1080/714038549
Smith, V. H. (2003). Eutrophication of freshwater and coastal marine ecosystems: a global problem. Environ. Sci. Pollut. Res. 10:142. doi: 10.1065/espr2002.12.142
Smith, V. H., and Schindler, D. W. (2009). Eutrophication science: where do we go from here? Trends Ecol. Evol. 24:9. doi: 10.1016/j.tree.2008.11.009
Smyth, T. J., Wright, A. E., Edwards-Jones, A., McKee, D., Queirós, A., Rendon, O., et al. (2022). Disruption of marine habitats by artificial light at night from global coastal megacities. Elementa 10:42. doi: 10.1525/elementa.2022.00042
Stegert, C., Lenhart, H. J., Blauw, A., Friedland, R., Leujak, W., Kerimoglu, O., et al. (2021). Evaluating uncertainties in reconstructing the pre-eutrophic state of the North Sea. Front. Mar. Sci. 8:637483. doi: 10.3389/fmars.2021.637483
Stentiford, G. D., Bateman, I. J., Hinchliffe, S. J., Bass, D., Hartnell, R., Santos, E. M., et al. (2020). Sustainable aquaculture through the One Health lens. Nat. Food 1, 468–474. doi: 10.1038/s43016-020-0127-5
Stoecker, D. K., Hansen, P. J., Caron, D. A., and Mitra, A. (2017). Mixotrophy in the Marine Plankton. Ann. Rev. Mar. Sci. 9, 311–335. doi: 10.1146/annurev-marine-010816-060617
Tett, P., Carreira, C., Mills, D. K., Van Leeuwen, S., Foden, J., Bresnan, E., et al. (2008). Use of a Phytoplankton Community Index to assess the health of coastal waters. ICES J. Mar. Sci. 65, 1475–1482. doi: 10.1093/icesjms/fsn161
Tett, P., Gowen, R., Mills, D., Fernandes, T., Gilpin, L., Huxham, M., et al. (2007). Defining and detecting undesirable disturbance in the context of marine eutrophication. Mar. Pollut. Bull. 55, 282–297. doi: 10.1016/j.marpolbul.2006.08.028
Tett, P., Gowen, R. J., Painting, S. J., Elliott, M., Forster, R., Mills, D. K., et al. (2013). Framework for understanding marine ecosystem health. Mar. Ecol. Prog. Ser. 494, 1–27. doi: 10.3354/meps10539
Thornton, D. C. O., Dong, L. F., Underwood, G. J. C., and Nedwell, D. B. (2002). Factors affecting microphytobenthic biomass, species composition and production in the Colne Estuary (UK). Aquat. Microbial Ecol. 27, 285–300. doi: 10.3354/ame027285
Thornton, J. A., Harding, W. R., Dent, M., Hart, R. C., Lin, H., Rast, C. L., et al. (2013). Eutrophication as a “wicked” problem. Lakes Reserv.: Sci. Policy Manag. Sustain. Use 18, 298–316. doi: 10.1111/lre.12044
Topcu, D., and Brockmann, U. (2021). Consistency of thresholds for eutrophication assessments, examples and recommendations. Environ. Monit. Assess. 193:677. doi: 10.1007/s10661-021-09189-6
Tunney, H. (1994). Policies related to agriculture and environment in the European Community. Mar. Pollut. Bull. 29, 508–514. doi: 10.1016/0025-326X(94)90678-5
Tweedley, J. R., Warwick, R. M., and Potter, I. C. (2016). “The contrasting ecology of temperate macrotidal and microtidal estuaries,” in Oceanography and Marine Biology: An Annual Review: Volume 54 (Boca Raton, FL: CRC Press), 73–172. doi: 10.1201/9781315368597-3
Van Beusekom, J. E., Carstensen, J., Dolch, T., Grage, A., Hofmeister, R., Lenhart, H., et al. (2019). Wadden Sea Eutrophication: long-term trends and regional differences. Front. Mar. Sci. 6:370. doi: 10.3389/fmars.2019.00370
van Leeuwen, S., Tett, P., Mills, D., and van Der Molen, J. (2015). Stratified and nonstratified areas in the North Sea: Long-term variability and biological and policy implications. J. Geophys. Res. Oceans 120, 4670–4686. doi: 10.1002/2014JC010485
van Leeuwen, S. M., Lenhart, H. J., Prins, T. C., Blauw, A., Desmit, X., Fernand, L., et al. (2023). Deriving pre-eutrophic conditions from an ensemble model approach for the North-West European seas. Front. Mar. Sci. 10:1129951. doi: 10.3389/fmars.2023.1129951
van Roomen, M., Laursen, K., van Turnhout, C., van Winden, E., Blew, J., Eskildsen, K., et al. (2012). Signals from the Wadden sea: population declines dominate among waterbirds depending on intertidal mudflats. Ocean Coast. Manag. 68, 79–88. doi: 10.1016/j.ocecoaman.2012.04.004
Verity, P. G., Smetacek, V., and Smayda, T. J. (2002). Status, trends and the future of the marine pelagic ecosystem. Environ. Conserv. 29, 207–237. doi: 10.1017/S0376892902000139
Vincent, C., Heinrich, H., Edwards, A., and Haythornthwaite, J. (2022). Guidance on Typology, Reference Conditions and Classification Systems for Transitional and Coastal Waters. CIS working group. 2:119.
Ward, B. A., and Follows, M. J. (2016). Marine mixotrophy increases trophic transfer efficiency, mean organism size, and vertical carbon flux. Proc. Natl. Acad. Sci. USA. 113:1517118113. doi: 10.1073/pnas.1517118113
Waterhouse, J., Brodie, J., Lewis, S., and Mitchell, A. (2011). Quantifying the sources of pollutants in the Great Barrier Reef catchments and the relative risk to reef ecosystems. Mar. Pollut. Bull. 65, 394–406. doi: 10.1016/j.marpolbul.2011.09.031
Watson, S. C., and Beaumont, N. J. (2024). “Nutrient recycling and waste remediation as a service from estuarine and coastal ecosystems,” in Treatise on Estuarine and Coastal Science, eds. D. Baird, and M. Elliott (Amsterdam: Elsevier), 111–131 doi: 10.1016/B978-0-323-90798-9.00051-2
Wei, J., Yu, X., Lee, Z., Wang, M., and Jiang, L. (2020). Improving low-quality satellite remote sensing reflectance at blue bands over coastal and inland waters. Remote Sens. Environ. 250:112029. doi: 10.1016/j.rse.2020.112029
Willems, P., and Lloyd-Hughes, B. (2016). “Projected change—river flow and urban drainage,” in North Sea Region Climate Change Assessment, eds. M. Quante, and F. Colijn(Cham: Springer), 219–237. doi: 10.1007/978-3-319-39745-0_7
Willis, C., Papathanasopoulou, E., Russel, D., and Artioli, Y. (2018). Harmful algal blooms: the impacts on cultural ecosystem services and human well-being in a case study setting, Cornwall, UK. Mar. Policy 97, 232–238. doi: 10.1016/j.marpol.2018.06.002
Wilson, R. J., and Heath, M. R. (2019). Increasing turbidity in the North Sea during the 20th century due to changing wave climate. Ocean Sci. 15, 1615–1625. doi: 10.5194/os-15-1615-2019
Wollschläger, J., Neale, P. J., North, R. L., Striebel, M., and Zielinski, O. (2021). Editorial: Climate change and light in aquatic ecosystems: variability and ecological consequences. Front. Mar. Sci. 8:688712. doi: 10.3389/fmars.2021.688712
Worrall, F., Burt, T. P., Howden, N. J. K., and Whelan, M. J. (2016). The UK's total nitrogen budget from 1990 to 2020: a transition from source to sink? Biogeochemistry 129, 325–340. doi: 10.1007/s10533-016-0234-4
Worrall, F., Spencer, E., and Burt, T. P. (2009). The effectiveness of nitrate vulnerable zones for limiting surface water nitrate concentrations. J. Hydrol. 370, 21–28. doi: 10.1016/j.jhydrol.2009.02.036
Xiao, W., Liu, X., Irwin, A. J., Laws, E. A., Wang, L., Chen, B., et al. (2018). Warming and eutrophication combine to restructure diatoms and dinoflagellates. Water Res. 128, 206–216. doi: 10.1016/j.watres.2017.10.051
Xu, X., Lemmen, C., and Wirtz, K. W. (2020). Less nutrients but more phytoplankton: long-term ecosystem dynamics of the Southern North Sea. Front. Mar. Sci. 7:662. doi: 10.3389/fmars.2020.00662
Yunev, O. A., Velikova, V., and Carstensen, J. (2017). Effects of changing nutrient inputs on the ratio of small pelagic fish stock and phytoplankton biomass in the Black Sea. Estuar. Coast. Shelf Sci. 197, 173–184. doi: 10.1016/j.ecss.2017.08.033
Keywords: eutrophication, nutrients, pelagic community, OSPAR, UK Marine Strategy
Citation: Devlin MJ, Graves CA, Greenwood N, Allerton M, Bresnan E, Holland MM, McQuatters-Gollop A, Tett P, Tracey D and Best M (2025) Shifting sands of marine eutrophication assessments: building a future approach for UK marine waters. Front. Ocean Sustain. 3:1561741. doi: 10.3389/focsu.2025.1561741
Received: 16 January 2025; Accepted: 07 April 2025;
Published: 16 May 2025.
Edited by:
Stelios Katsanevakis, University of the Aegean, GreeceReviewed by:
Zbigniew Kowalewski, AGH University of Science and Technology, PolandPeng Zhang, Guangdong Ocean University, China
Luminita Lazar, National Institute for Marine Research and Development Grigore Antipa (INCDM), Romania
Copyright © 2025 Devlin, Graves, Greenwood, Allerton, Bresnan, Holland, McQuatters-Gollop, Tett, Tracey and Best. This is an open-access article distributed under the terms of the Creative Commons Attribution License (CC BY). The use, distribution or reproduction in other forums is permitted, provided the original author(s) and the copyright owner(s) are credited and that the original publication in this journal is cited, in accordance with accepted academic practice. No use, distribution or reproduction is permitted which does not comply with these terms.
*Correspondence: Michelle J. Devlin, bWljaGVsbGUuZGV2bGluQGNlZmFzLmdvdi51aw==