- 1Department of Aerospace Engineering, University of Illinois at Urbana-Champaign, Urbana, IL, United States
- 2Department of Mechanical Science and Engineering, University of Illinois at Urbana-Champaign, Urbana, IL, United States
Burgeoning technological advancements in practical and efficient hypersonic flight is intertwined with the research and development of airbreathing hypersonic propulsion, specifically dual-mode scramjet (DMS) engines. Due fundamentally to the lack of complete understanding and adequate modeling of the fluid dynamics and combustion processes present in DMSs, a large volume of academic works has been established towards characterizing the physical phenomena present in these engines. Significant differences in flame topologies, fluid interactions, and pressure profiles between scram and ram combustion are observed across these experimental and computational works. A focus on the dynamics responsible for combustion mode transition, choking and the propagation of the pseudoshock, is made, as is a discussion on the theoretical underpinning of the mechanisms behind flow choking and important choking thresholds. Further insight into the fundamental mechanisms and fluid and combustion physics present in DMSs may improve future designs and operability of dual-mode scramjet engines.
Introduction
Scramjets perform at an enhanced specific impulse compared to rocket-powered vehicles over a broad range of hypersonic flight Mach numbers (Curran, et al., 1996). Dual-mode scramjets (DMS), which can operate in both ram and scram combustion modes, extend the operability of a single engine platform to essentially two (Liu et al., 2019a), allowing for the design of efficient single-stage hypersonic aircraft that opens the arena for practical long-haul hypersonic transportation of goods and even passengers. Figure 1 depicts a schematic of a generic DMS flowpath.
A large volume of academic works has been established towards characterizing the physical phenomena present in these engines. The scope of this review is an overview of the investigations into the critical operational regimes of the dual mode scramjet, with a focus on the transition between ram and scram modes. The key phenomena relevant to dual mode scramjet operation can be categorized within the following overarching concepts:
1. Ram/scram combustion and mode transition
2. Choking mechanisms and thresholds
3. Combustor-isolator and pseudoshock dynamics
This overview encompasses experimental and numerical results across the literature and includes efforts to establish the theoretical underpinning for the fluid dynamics salient to mode transition.
Ram/scram combustion and mode transition
A DMSs ram mode combustion is engaged at the lower hypersonic corridor (Mach 5–7) while a smooth transition to scram is engaged as the aircraft accelerates beyond this range (Qingchun et al., 2017). While both modes serve to impart the same effect of increasing the flow total enthalpy by means of heat addition, the flow and flame topologies have been observed to be quite distinct. In general, the flame regions of scram mode combustion have been observed to be thinner and more uniformly distributed along the combustor flowpath than those of ram mode combustion (Baccarella et al., 2019). In particular, the flame front of ram mode combustion tends to be more vertical (flow transverse), with more agglomerated and spatially distinct flame regions (Fotia, et al., 2013).
In scram mode, rates of fluid mixing and chemical reaction compete against the limited residence time of the combustor throughflow. The limiting mechanism for sustained combustion in scram mode is the turbulent mixing between the injected fuel and air (Yang, et al., 2010; Liu, et al., 2020). It is observed that increasing injected fuel equivalence ratio (φ) results in a more corrugated, dynamic, and broader flame front indicative of greater levels of turbulent mixing and enhanced combustion. This can be explained by the penetration of the fuel jets; if oriented transverse with respect to the combustor flow, at higher φ, thus higher jet momentum, the fuel jet penetrates deeper into the crossflow. This allows for the mixing to occur deeper in the high momentum core combustor flow, improving the turbulent mixing which, with the higher overall fuel concentration, promotes larger and more developed turbulent flame structures, as seen in the first two images of Figure 1A (Baccarella et al., 2021).
During ram combustion and mode transition, the flow passes through a fluid structure known as a pseudoshock as coined by Matsuo et al. (1999) which is comprised of a series of shocks (and attendant shock-boundary layer interactions, or SBLI) followed by a region of viscous dissipation (Ikui, et al., 1974). A defining feature of the pseudoshock is a virtual throat that transitions the flow from supersonic to subsonic. As the Mach number decreases across this transition the static temperature and pressure increase, increasing the chemistry rates. Penetration of the fuel jets becomes more effective due to the reduction of the combustor flow momentum flux. Lastly, the reduced flow velocity results in longer flow residence times. These conditions promote more complete and localized combustion, resulting in flame structures that are fuller across the span of the flowpath. This characteristic enables unambiguous optical tracking of the pseudoshock in mode transition experiments, which can be identified by a “ball of flame” that effectively consumes the limiting combustion reactants and propagates upstream during transition. While typically mode transition due to increased equivalence ratio is understood to occur from scram to ram conditions, certain fuel compositions may yield transition and dual-mode combustion (where large regions of subsonic combustion interact with a supersonic core flow) without the clear establishment of sustained supersonic combustion, as observed by Landsberg, et al. (2021) in mode transition experiments employing endothermically cracked n-DodecDane surrogate fuel. This is attributed to deviations in ignition delay and reactivity due to differences in chemical pathways of different fuel compositions, which is supported by scram blowoff limits of different ethylene-methane mixtures experimentally determined by Nakaya, et al. (2019). It should be noted that in many experimental studies on DMSs, some level of flow vitiation may occur due to propagation of dissociated, excited, or radical species generated by the high temperatures present in the facility plenum prior to expansion. This flow vitiation can play a role in the mode transition dynamics and flame stabilization modes, where the presence of these species can dramatically influence ignition and flameholding characteristics of a fueling configuration (Hash, 2022).
Practical DMSs use wall-mounted cavity flameholders to enhance the ignition and flame stability performance (Ben-Yakar, et al., 2001; Huang et al., 2018; Liu et al., 2019b). The reactions generated in the low-speed cavity recirculation zone or cavity shear layer produce the generation of heat and combustion radicals that greatly reduce the chemical induction time for the fuel-air mixing regions further downstream in the combustor (Liu, et al., 2020). This is especially important in scram mode where the ignition delay of the fuel air mixture in the core flow can exceed the residence time of the flow. The archetypes of cavity-assisted flame stabilization are shear layer-stabilized combustion, recirculation stabilized flame, and jet-wake stabilized flame. While cavity shear layer-stabilized and recirculation zone stabilized combustion have been observed during sustained scram combustion, jet-wake stabilized flames are typically only seen in ram cases where near-jet mixing and subsonic flows provide a condition favorable for near-jet flame anchoring. In certain cases, a lifted-jet flame stabilization may occur in ram mode where φ is below that for a jet-wake stabilized combustion (Fotia, et al., 2013). An addendum to this generality is the near-jet flame anchoring of a cavity fuel injector during scram mode, where the cavity recirculation region provides the high temperatures and residence time of hot products and radicals in the vicinity of the fuel jet mixing layer necessary to induce sustained near-jet combustion (Baccarella et al., 2020). Furthermore, in a computational study employing a two-stage injection scheme, Cao, et al. (2021) were able to achieve cavity-assisted jet wake flame stabilization in an ethylene-fueled scramjet; this demonstrates a link between injection scheme, stable combustion modes and locations, and overall combustor performance.
Wall static pressure measurements can be used to indicate ram or scram DMS operation. Peak combustor pressures for ram mode can be two to three times higher than those for scram mode (O'Byrne et al., 2000; Frost et al., 2009; Liu et al., 2019a). Moreover, static pressure profiles have been observed to be steeper for ram combustion, with the pressure rise often beginning further upstream in the isolator region (Fotia, et al., 2011). In a DMS with fuel injection near the start of the combustor, the combustor pressure profile can be positively skewed towards the start of the combustor during ram (Yan, et al., 2014). In a combustor flowpath with little to no area divergence, the ram combustor pressure tends to decrease towards the end of the combustor due to the increasing Mach number of the subsonic flow; conversely, the pressure rise for scram cases typically increases more gradually and achieves the highest pressures near the exit of the combustor (Liu et al., 2019c). Large quasi-steady fluctuations in the combustor pressure profile are a feature of scram mode, since these pressure fluctuations are an indication of shock and expansion wave reflections on the flowpath walls (Shen, et al., 2020).
Choking mechanisms and thresholds
The process by which scram to ram transition occurs in a DMS is called choking, thus named due to the attendant formation of a sonic point in the flowpath. The formation and upstream propagation of the pseudoshock are identified as the primary fluid phenomena associated with choking. While the qualitative aspects of pseudoshock behavior have been characterized, the dynamics and true catalyst for choking in a DMS is not fully understood or agreed upon in the literature. The mechanisms responsible for flow choking have been identified as heat release, mass addition, friction, and flow blockage. Due to the complex and coupled effect these influences have on the flow, an accurate analytical model predicting the onset of choking has not been established.
Producing the high enthalpy flows necessary for high-speed combustion ground tests often requires a prohibitive investment in specialized facilities. Many low-enthalpy choking studies use the use of pure mass addition or actuated physical blockage (such as movable ramps or plugs) as the means of choking. Mass addition is an attractive representation of the choking mechanisms present in a combustion experiment due to the similar fluid interactions between the injection jet and crossflow (Im et al., 2016). Baccarella et al. (2021) performed a comparison between mass addition and combustion heat release induced choking for identical high enthalpy inflow conditions and found that although the two mechanisms exhibited similar isolator flow dynamics, the pseudoshock in the combustor during mode transition exhibited different qualitative characteristics, such as a more distinct shock train in the combustion case, in addition to differences in propagation dynamics.
In reacting scramjet studies, it is understood that combustion heat release plays a dominant role in the choking process (Im, et al., 2018. ; Baccarella et al., 2019). In practice, the means of initiating mode transition and controlling the advancement of the pseudoshock has been the global φ. Increasing the fueling rate drives the flow towards thermal choking (Larsson et al., 2015). At some φ, a fully-developed pseudoshock is developed and the choking/mode transition process is engaged in earnest. Further addition of heat advances the pseudoshock upstream until it has settled into the isolator and the entire combustor is subsonic, indicating ram mode (Qingchun et al., 2017). The 1-D analogy for heat release-induced choking is Rayleigh flow, whereby a choked condition is achieved at the limit of maximum heat addition for a given inflow condition. Laurence et al. (2013) compared their results for a transient combustion induced choking of the HyShot II flowpath to an unsteady Rayleigh flow analytical model. It was determined that heating beyond the Rayleigh flow choked condition could be achieved for an upstream propagating normal shock (which models the pseudoshock). However, an issue with this theoretical analysis is the requirement of a persistently propagating shock which runs counter to the establishment of a quasi-steady pseudoshock position and ram mode. It is unlikely that the true Rayleigh choking limit can be achieved in the flowpath since the choking limit would be immediately succeeded by a readjusting normal shock and subsequent inlet unstart (a critical failure mode of a DMS). This normal shock would never be realized due to the presence of the boundary layer, which serves to spread out the pressure rise. It should be noted that choking thresholds based on φ are geometry specific and dependent on mixing and combustion efficiencies, which are difficult to measure experimentally and may contribute to the departure of an experimentally validated DMS design from analytically derived predictions. Finally, as assessed by Fotia and Driscoll (2011), the effect of injection induced blockage, mass addition, and heat release are not easily decoupled and each non-negligibly contribute to the choking event.
Large flow separation regions can accompany choking induced by flow blockage (J. Wagner, 2008; H. J. Tan, 2009). Im and Do (2018) discuss the role of the flow separation as a means of flow constriction and a necessary component of non-reacting choking processes, and argue that such flow area change is unnecessary for thermal choking due to the large pressure rise associated with heat release. However, it can be shown that even cases of thermal choking the flow must also undergo fluidic confinement through the boundary layer (or some form of fluidic area change) in order to establish a thermal throat in a constant area combustor or duct. Using the 1-D relation for mass flow rate, and relating the choked conditions of the inflow and outflow conditions, the following relation is readily derived:
where subscripts 1 and 2 represent inflow and outflow conditions respectively,
Combustor area divergence is used as a means of delaying the onset of combustion-induced choking and extending the fueling range of a DMS. The effect of a diverging section in scram mode is the relief of the combustor pressure rise. This serves to delay the formation of boundary layer separation and ultimately the pseudoshock. The following differential equation derived using Shapiro’s 1-D relations (Shapiro Ascher, 1953) express the competing effect area change has with heat release, friction, and mass addition on the flow Mach number:
where
Combustor-isolator and pseudoshock dynamics
A DMS isolator shields the inlet from the combustion pressure rise and provides real estate for the shock structures. Combustor-isolator dynamics play an important role in the operation of DMS particularly in ram mode, where the propagation of the pseudoshock in the isolator produces either an ideal combustor condition or inlet unstart.
The isolator shock train, or series of reflecting oblique/normal shocks, serves as a source of compression and as a mechanism for distributing the pressure rise associated with the downstream combustion. As the combustor pressure rise increases, the shock-boundary layer system in the isolator adjusts with a thickening of the boundary layer and shock train confinement. Shock train unsteadiness in the isolator can be attributed to both local SBLI and a response to acoustic forcing via the subsonic portion of the boundary layer from pressure fluctuations in the combustor (Lin, et al., 2010). During ram, the pseudoshock resides in the isolator, and the acoustic forcing effect is especially significant due to the greater amplitude of the combustor pressure fluctuations which can be easily communicated upstream along the length of the shock train via the large subsonic regions of the pseudoshock. Recent experimental works by Hunt, et al. (2019) demonstrate the source of shock train unsteadiness to be a combination of acoustic waves generated by local separation bubble instabilities, convection of separation bubble shear layer vortices, and the acoustic feedback from the backpressure source. Backpressure feedback was observed to have a strong effect on the motion of the leading shock of the shock train. A study by Su, et al. (2016) found a close relationship between the frequency of the simulated backpressure oscillations, the location of the leading shock, and phase of the shock train motion. Kato, et al. measured a noticeable alteration of the dominant flame fluctuation frequency during ram mode with the presence of a cavity flameholder and noted the role of the cavity as an acoustic source (Kato, 2021). Le et al. (2008) experimentally observed dramatic differences in the spectral characteristics of the wall pressure measurements upstream and downstream of the leading shock, corroborating the significant role that the leading shock has on the acoustic feedback between the combustor and isolator. This study also highlights the possible use of pressure frequency history analysis as a means of unstart detection and mitigation, as opposed to more traditional pressure amplitude thresholding methods.
The length of the shock train in the isolator is governed by the overall pressure rise across the pseudoshock. Walthrup and Billig (1973) developed a model to predict shock train length as a function of pressure rise and the state of the inflow boundary layer. Emami et al. (1995) note that the isolator should be of sufficient length to contain the entire shock train during ram combustion or risk incomplete flow diffusion or inlet unstart. In a recent computational study, Fiévet and Raman (2018) observed effects of vibrational non-equilibrium on the shock train structure of a pseudoshock and found that the overall length of the shock train increased due to the latency of the N2 vibrational temperatures and corresponding pressure rise compared to a thermally perfect gas model. These factors should be considered to establish a geometric constraint to a well-designed DMS isolator for a given flight condition (Fiévet, et al., 2019).
The structure of the shock train and flow confinement is primarily dependent on the inflow Mach number (Tamaki, et al., 1969), ranging from highly confined X-type shocks at high Mach numbers (Tamaki, et al., 1971), λ-type shocks at moderate supersonic Mach numbers (Tamaki, et al., 1970), to normal shock trains with reduced boundary layer growth at low supersonic Mach numbers (Carroll, et al., 1992). The structure and large-scale dynamics of the shock train was also shown to be dependent on the cross-sectional geometry of the isolator. In a recent study by Baccarella et al. (2021) shock train dynamics were observed in an axisymmetric isolator at both high and low enthalpy conditions. Large-scale self-sustained shock train oscillations were accompanied by transitions from X-type to λ-type configurations, with mechanisms of shock train contraction, swallowing, and extension attributed to local SBLI and aerodynamic throttling/nozzling effects, shown in Figure 2B. A study by Lee et al. (2020) observed a bimodal shock train structure in a rectangular isolator that transitioned between primary and secondary X-type shock structures originating from the top/bottom walls and sidewalls of the isolator respectively, shown in Figure 2C. A power spectral analysis of the time-resolved pressure measurements of both experiments highlighted a strong peak in the frequency content of the axisymmetric shock train dynamics, while no such peak was evident in the rectangular case. It was reasoned that the SBLI and acoustic response of the shock train experienced destructive interference between the two modes of the rectangular shock train, while the dynamics were amplified in the axisymmetric case.
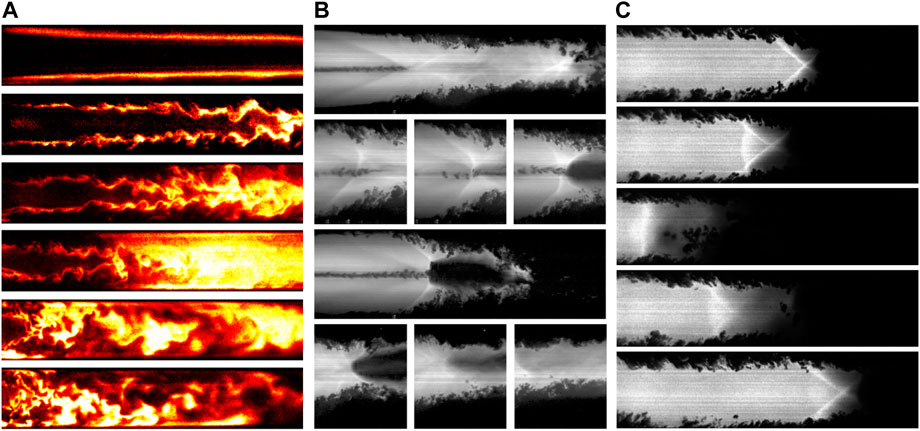
FIGURE 2. Instantaneous flow and flame visualization of DMS combustor and isolator dynamics (image sequences are top to bottom). (A) OH PLIF images of combustor choking (Baccarella et al., 2021). (B) CO2 planar laser Rayleigh scattering (PLRS) images of axisymmetric isolator shock train oscillations (Baccarella et al., 2021). (C) CO2 PLRS of rectangular shock train dynamics (Lee et al., 2020).
Conclusion
This short overview provides a contemporary snapshot of the work and understanding of the fluid and flame dynamics in DMSs across high-fidelity simulations and experiments in addition to the theoretical background that governs these physics. The qualitative distinctions between the two modes and the important thresholds and mechanisms are explored through the literature. The authors humbly hope that the current overview captures the spirit of scientific inquiry that is represented across the works presented, and that it contributes to better insight into the rich and fascinating physics that manifest in high-speed combustion and propulsion.
Author contributions
GL contributed the literature review and primary writing of the manuscript draft. TL provided important insight and expertise to improve the manuscript story. Both authors contributed to the editing of the manuscript.
Acknowledgments
This work was supported by U.S. Air Force Office of Scientific Research grant FA9550-21-1-0072 monitored by Chiping Li, and by the Office of Naval Research grant N00014-21-1-2475 monitored by Eric Marineau. The authors gratefully acknowledge these sources of support.
Conflict of interest
The authors declare that the research was conducted in the absence of any commercial or financial relationships that could be construed as a potential conflict of interest.
Publisher’s note
All claims expressed in this article are solely those of the authors and do not necessarily represent those of their affiliated organizations, or those of the publisher, the editors and the reviewers. Any product that may be evaluated in this article, or claim that may be made by its manufacturer, is not guaranteed or endorsed by the publisher.
References
Baccarella, D., Lee, G., Liu, Q., Elliott, G. S., Freund, J. B., and Lee, T. (2020). Laser-induced plasma ignition experiments in a direct-connect supersonic combustor at Mach 3 [journal]. J. Propuls. Power 36, 732–743. doi:10.2514/1.B37846
Baccarella, D., Liu, Q., McGann, B., Lee, G., and Lee, T. (2021). Isolator-combustor interactions in a circular model scramjet with thermal and non-thermal choking-induced unstart [journal]. J. Fluid Mech. 917, 1–36. doi:10.1017/jfm.2021.238
Baccarella, D., Liu, Q., McGann, B., and Lee, T. (2019). Combustion induced choking and unstart initiation in a circular constant-area supersonic flow [journal]. AIAA J. 57, 5365–5376. doi:10.2514/1.J057921
Ben-Yakar, A., and Hanson, R. K. (2001). Cavity flame-holders for ignition and flame stabilization in scramjets: An overview [journal]. J. Propuls. Power 17, 869–877. doi:10.2514/2.5818
Cao, D., Brod, H. E., Yokev, N., and Michaels, D. (2021). Flame stabilization and local combustion modes in a cavity-based scramjet using different fuel injection schemes [Journal]. Combust. Flame 233, 111562. doi:10.1016/j.combustflame.2021.111562
Carroll, B. F., and Dutton, J. C. (1992). Multiple normal shock wave/turbulent boundary-layer interactions [journal]. J. Propuls. Power 8, 441–448. doi:10.2514/3.23497
Curran, E. T., Heiser, W. H., and Pratt, D. T. (1996). Fluid phenomena in scramjet combustion systems [journal]. Annu. Rev. Fluid Mech. 28, 323–360.
Emami, S., Trexler, C. A., Auslender, A. H., and Weidner, J. P. (1995). Report. No.:L-17422 NAS 1.60:3502 NASA-TP-3502. Washington, United States: NASA.Experimental investigation of inlet-combustor isolators for a dual-mode scramjet at a Mach number of 4 [report].
Fiévet, R., Raman, V., and Auslender, A. H. (2019). Data-driven one-dimensional modeling of pseudoshocks [journal]. J. Propuls. Power 35, 313–327. doi:10.2514/1.b37175
Fiévet, R., and Raman, V. (2018). Effect of vibrational nonequilibrium on isolator shock structure [journal]. J. Propuls. Power 34, 1334–1344. doi:10.2514/1.b37108
Fotia, M. L., and Driscoll, J. F. (2011). “Assessment of isolator pseudo-shocks created by combustion with heated flow [conference],” in 17th AIAA International Space Planes and Hypersonic Systems and Technologies Conference, San Francisco, United States, April 2011, 1–24. 10.2514/6.2011-2201 .
Fotia, M. L., and Driscoll, J. F. (2013). Ram-scram transition and flame/shock-train interactions in a model scramjet experiment [journal]. J. Propuls. Power 29, 261–273. doi:10.2514/1.b34486
Frost, M. A., Gangurde, D. Y., Paull, A., and Mee, D. J. (2009). Boundary-layer separation due to combustion-induced pressure rise in supersonic flow [Journal]. AIAA J. 47, 1050–1053. doi:10.2514/1.40868
Hash, C. A., Drummond, P. M., Edwards, J. R., Kato, N., and Lee, T. (2022). Numerical simulation of stable and unstable ram-mode operation of an axisymmetric ethylene-fueled inlet-isolator-combustor configuration [Journal]. Combust. Flame 242, 112157. doi:10.1016/j.combustflame.2022.112157
Huang, W., Du, Z., Yan, L., and Moradi, R. (2018). Flame propagation and stabilization in dual-mode scramjet combustors: A survey [journal]. Prog. Aerosp. Sci. 101. doi:10.2514/1.J054974
Hunt, R. L., and Gamba, M. (2019). On the origin and propagation of perturbations that cause shock train inherent unsteadiness [Journal]. J. Fluid Mech. 861, 815–859. doi:10.1017/jfm.2018.927
Ikui, T., Matsuo, K., and Nagai, M. (1974). The mechanism of pseudo-shock waves [journal]. Bull. Jpn. Soc. Mech. Eng. 17, 731–739. doi:10.1299/jsme1958.17.731
Im, S., Baccarella, D., McGann, B., Liu, Q., Wermer, L., and Do, H. (2016). Unstart phenomena induced by mass addition and heat release in a model scramjet [journal]. J. Fluid Mech. 797, 604–629. doi:10.1017/jfm.2016.282
Im, S., and Do, H. (2018). Unstart phenomena induced by flow choking in scramjet inlet-isolators [journal]. Prog. Aerosp. Sci. 97, 1–21. doi:10.1016/J.PAEROSCI.2017.12.001
Kato, N., and Im, S. (2021). Flame dynamics under various backpressures in a model scramjet with and without a cavity flameholder [Journal]. Proc. Combust. Inst. 38, 3861–3868. doi:10.1016/j.proci.2020.07.110
Korkegi, R. H. (1975). Comparison of shock-induced two- and three- dimensional incipient turbulent seperation [journal]. AIAA J. 13, 534–535. doi:10.2514/3.49750
Landsberg, W. O., Vanyai, T., McIntyre, T. J., and Veeraragavan, A. (2021). Dual/scram-mode combustion limits of ethylene and surrogate endothermically-cracked hydrocarbon fuels at Mach 8 equivalent high-enthalpy conditions [Journal]. Proc. Combust. Inst. 38, 3835–3843. doi:10.1016/j.proci.2020.07.003
Larsson, J., Laurence, S., Bermejo-Moreno, I., Bodart, J., Karl, S., and Vicquelin, R. (2015). Incipient thermal choking and stable shock-train formation in the heat-release region of a scramjet combustor. Part II: Large eddy simulations [Journal]. Combust. Flame 162, 907–920. doi:10.1016/j.combustflame.2014.09.016
Laurence, S. J., Karl, S., Schramm, J. M., and Hannemann, K. (2013). Transient fluid-combustion phenomena in a model scramjet [Journal]. J. Fluid Mech. 722, 85–120. doi:10.1017/jfm.2013.56
Laurence, S. J., Lieber, D., Schramm, J. M., Hannemann, K., and Larsson, J. (2015). Incipient thermal choking and stable shock-train formation in the heat-release region of a scramjet combustor. Part I: Shock-tunnel experiments [Journal]. Combust. Flame 162, 921–931. doi:10.1016/j.combustflame.2014.09.016
Le, D. B., Goyne, C. P., Krauss, R., and McDaniel, J. C. (2008). Experimental study of a dual-mode scramjet isolator [journal]. Jounral Propuls. Power 24. doi:10.2514/1.32591
Lee, G., Baccarella, D., Liu, Q., Elliott, G. S., and Lee, T. (2020). “Pseudoshock dimensionality in axisymmetric and rectangular scramjets [conference],” in AIAA Scitech 2020 Forum, Orlando, United States, January 2020. 10.2514/6.2020-1610 .
Lin, K., Ma, F., Yang, V., Jackson, T. A., and Yang, V. (2010). Acoustic characterization of an ethylene-fueled scramjet combustor with a cavity flameholder flameholder [journal]. J. Propuls. Power 26, 1161–1170. doi:10.2514/1.43338
Liu, Q., Baccarella, D., Lee, G., and Lee, T. (2019c). “Influences of cavity on combustion stabilization in an axisymmetric scramjet [conference],” in AIAA Scitech Forum, 2019, San Diego, United States, January 2019. 10.2514/6.2019-1681 .
Liu, Q., Baccarella, D., McGann, B., and Lee, T. (2019b). Dual-mode operation and transition in axisymmetric scramjets [journal]. AIAA J. 57. doi:10.2514/1.J058391
Liu, Q., Baccarella, D., McGann, B. J., and Lee, T. (2019a). Combustion induced choking and unstart initiation in a circular constant-area supersonic flow [journal]. AIAA J. doi:10.2514/1.J057921
Liu, Q., Baccarella, D., and Lee, T. (2020). Review of combustion stabilization for hypersonic airbreathing propulsion [journal]. Prog. Aerosp. Sci. 119, 100636. doi:10.1016/j.paerosci.2020.100636
Matsuo, K., Miyazato, Y., and Kim, H.-D. (1999). Shock train and pseudo-shock phenomena in internal gas flows [Journal]. Prog. Aerosp. Sci. 35, 33–100. doi:10.1016/s0376-0421(98)00011-6
Nakaya, S., Kinoshita, R., Lee, J., Ishikawa, H., and Tsue, M. (2019). Analysis of supersonic combustion characteristics of ethylene/methane fuel mixture on high-speed measurements of CH* chemiluminescence [Journal]. Proc. Combust. Inst. 37, 3749–3756. doi:10.1016/j.proci.2018.09.011
O'Byrne, S., Doolan, M., Olsen, S. R., and Houwing, A. F. (2000). Analysis of Transient thermal choking processes in a model scramjet engine [Journal]. J. Propuls. Power 16, 808–814. doi:10.2514/2.5645
Ortwerth, P. J. (2001). “Scramjet flowpath integration [book section],” in Progress in astronautics and aeronautics: Scramjet propulsion. Editors E. T. Curran, and S. N. B. Murthy (Reston, Virginia: American Institute of Aeronautics and Astronautics). book auth.
Qingchun, Y., Wen, B., Khaled, C., Silong, Z., and Nicolas, G. (2017). Thermal behavior of an isolator with mode transition inducing back-pressure of a dual-mode scramjet [Journal]. Chin. J. Aeronautics 30. doi:10.1016/j.cja.2017.02.013
Shapiro Ascher, H. (1953). The dynamics and thermodynamics of compressible flow volume I [book] wiley. New York, United States: The Ronald Press Company.
Shen, W., Huang, Y., You, Y., and Yi, L. (2020). Characteristics of reaction zone in a dual-mode scramjet combustor during mode transitions [Journal]. Aerosp. Sci. Technol. 99, 105779. doi:10.1016/j.ast.2020.105779
Smart, M. K. (2015). Flow modeling of pseudoshocks in backpressured ducts [journal]. AIAA J. 53, 3577–3588. doi:10.2514/1.j054021
Su, W.-Y., Ji, Y.-X., and Chen, Y. (2016). Effects of dynamic backpressure on pseudoshock oscillations in scramjet inlet-isolator [journal]. J. Propuls. Power 32, 516–528. doi:10.2514/1.b35898
Tamaki, T., Tomita, Y., and Yamane, R. (1970). A study of pseudo-shock: 1st report, λ-type pseudo-shock [journal]. Bull. Jpn. Soc. Mech. Eng. 13, 51–58. doi:10.1299/jsme1958.13.51
Tamaki, T., Tomita, Y., and Yamane, R. (1971). A study of pseudo-shock: 2nd report, X-type pseudo-shock [journal]. Bull. Jpn. Soc. Mech. Eng. 14, 807–817. doi:10.1299/jsme1958.14.807
Tamaki, T., Tomita, Y., and Yamane, R. (1969). A study of pseudo-shock : 2nd report, X-type pseudo-shock [journal]. Bull. JSME 14, 807–817. doi:10.1299/jsme1958.14.807
Tan, H. J., Sun, S., and Lin, Z. L. (2009). Oscillatory flows of rectangular hypersonic inlet unstart caused by downstream mass-flow choking [Journal]. Journall Propuls. Power 25. doi:10.2514/1.37914
Waltrup, P. J., and Billig, F. S. (1973). Structure shock waves in cylindrical ducts [journal]. AIAA J. 11, 1404–1408. doi:10.2514/3.50600
Yan, Z., Bing, C., Gang, L., Baoxi, W., and Xu, X. (2014). Influencing factors on the mode transition in a dual-mode scramjet [Journal]. Acta Astronaut. 103, 1–15. doi:10.1016/j.actaastro.2014.06.006
Yang, V., Li, J., and Choi, J. Y. (2010). “Ignition transient in an ethylene fueled scramjet engine with air throttling; Part II: Ignition and flame development [conference],” in 48th AIAA Aerospace Sciences Meeting Including the New Horizons Forum and Aerospace Exposition, Orlando, United States, January 2010, 1–17. 10.2514/6.2010-410 .
Keywords: scramjet, hypersonics, dual mode, airbreathing engine, thermal choking, pseudoshock, supersonic combustion
Citation: Lee GS and Lee T (2023) Fluid and combustion dynamics in dual-mode scramjets. Front. Aerosp. Eng. 1:1058038. doi: 10.3389/fpace.2022.1058038
Received: 30 September 2022; Accepted: 13 December 2022;
Published: 05 January 2023.
Edited by:
Leonid Tartakovsky, Technion Israel Institute of Technology, IsraelReviewed by:
Suo Yang, University of Minnesota Twin Cities, United StatesDan Michaels, Technion Israel Institute of Technology, Israel
Matthew Oehlschlaeger, Rensselaer Polytechnic Institute, United States
Copyright © 2023 Lee and Lee. This is an open-access article distributed under the terms of the Creative Commons Attribution License (CC BY). The use, distribution or reproduction in other forums is permitted, provided the original author(s) and the copyright owner(s) are credited and that the original publication in this journal is cited, in accordance with accepted academic practice. No use, distribution or reproduction is permitted which does not comply with these terms.
*Correspondence: Tonghun Lee, dG9uZ2h1bkBpbGxpbm9pcy5lZHU=