- 1Comparative Pediatrics and Nutrition, Department of Veterinary and Animal Sciences, Faculty of Health and Medical Sciences, University of Copenhagen, Frederiksberg, Denmark
- 2Takeda, Cambridge, MA, United States
- 3Department of Pediatrics, United States Department of Agriculture/Agricultural Research Service, Children's Nutrition Research Center, Baylor College of Medicine, Houston, TX, United States
- 4Department of Neonatology, Rigshospitalet, Copenhagen, Denmark
- 5Department of Pediatrics, Odense University Hospital, Odense, Denmark
Background: Elevation of circulating insulin-like growth factor-1 (IGF-1) within normal physiological levels may alleviate several morbidities in preterm infants but safety and efficacy remain unclear. We hypothesized that IGF-1 supplementation during the first 1–2 weeks after preterm birth improves clinical outcomes and gut development, using preterm pigs as a model for infants.
Methods: Preterm pigs were given vehicle or recombinant human IGF-1/binding protein-3 (rhIGF-1, 2.25 mg/kg/d) by subcutaneous injections for 8 days (Experiment 1, n = 34), or by systemic infusion for 4 days (Experiment 2, n = 19), before collection of blood and organs for analyses.
Results: In both experiments, rhIGF-1 treatment increased plasma IGF-1 levels 3-4 fold, reaching the values reported for term suckling piglets. In Experiment 1, rhIGF-1 treatment increased spleen and intestinal weights without affecting clinical outcomes like growth, blood biochemistry (except increased sodium and gamma-glutamyltransferase levels), hematology (e.g., red and white blood cell populations), glucose homeostasis (e.g., basal and glucose-stimulated insulin and glucose levels) or systemic immunity variables (e.g., T cell subsets, neutrophil phagocytosis, LPS stimulation, bacterial translocation to bone marrow). The rhIGF-1 treatment increased gut protein synthesis (+11%, p < 0.05) and reduced the combined incidence of all-cause mortality and severe necrotizing enterocolitis (NEC, p < 0.05), but had limited effects on intestinal morphology, cell proliferation, cell apoptosis, brush-border enzyme activities, permeability and levels of cytokines (IL-1β, IL-6, IL-8). In Experiment 2, rhIGF-1 treated pigs had reduced blood creatine kinase, creatinine, potassium and aspartate aminotransferase levels, with no effects on organ weights (except increased spleen weight), blood chemistry values, clinical variables or NEC.
Conclusion: Physiological elevation of systemic IGF-1 levels for 8 days after preterm birth increased intestinal weight and protein synthesis, spleen weight and potential overall viability of pigs, without any apparent negative effects on recorded clinical parameters. The results add further preclinical support for safety and efficacy of supplemental IGF-1 to hospitalized very preterm infants.
Introduction
About 10% of all infants are born preterm [<37 weeks gestation, (1)]. In preterm infants, nutrient metabolism, immunity and many organ systems are immature which complicates their adaptation to extrauterine life. Birth is associated with a rapid fall in circulating insulin-like growth factor-1 (IGF-1) levels due to the abrupt loss of fetal or maternal IGF-1 supply. After preterm birth, the subsequent postnatal increase in IGF-1 levels occurs slowly (2). Especially very preterm infants (<32 weeks gestation) show an extended period of low postnatal IGF-1 levels with a more than 50% reduction in serum IGF-1 levels relative to term infants and gestational age-matched fetuses in utero (2–4). Low levels of circulating IGF-1 have been associated with several complications of prematurity (5), including growth restriction (6–9), systemic inflammation [early- or late-onset sepsis, (10, 11)], lung complications [bronchopulmonary dysplasia, BPD, (12)], gut inflammation [necrotizing enterocolitis, NEC, (9)], eye problems [retinopathy of prematurity, ROP, (9, 13)] and brain damage [intraventricular hemorrhage, IVH, (14, 15)]. One or more of these major neonatal morbidities occur in >60% of infants born extremely preterm [<28 weeks gestation, (16, 17)]. In a global phase 2 clinical study, supplemental recombinant human (rh) IGF-1/rhIGF binding protein-3 (rhIGF-1) reduced BPD and IVH severity in extremely preterm infants (18). A large multi-center international trial is now ongoing to verify if supplemental IGF-1, within normal physiological levels, will prevent later morbidities in extremely preterm infants (ClinicalTrials.gov registry NCT03253263).
IGF-1 is a mitogenic polypeptide with specific receptors in many tissues and organs, considered essential for growth and development during pregnancy, infancy and childhood (6–8, 19–21). Fetal and maternal IGF-1 blood levels increase with gestational age, indicating a significant role of IGF-1 in fetal development, particularly in the third trimester (4, 22, 23). In fetuses and neonates, insulin stimulates hepatic IGF-1 production and release into the bloodstream (4, 24, 25). The major part of circulating IGF-1 in children and adults is bound to IGF-binding protein (IGFBP)-3 and an acid labile subunit (ALS) that increase the half-life and regulate bioavailability (26). In addition to the liver, many tissues synthesize IGF-1 locally, facilitating paracrine and autocrine actions of IGF-1 in addition to endocrine functions (27, 28).
Colostrum contains high concentrations of IGF-1 (29, 30). The level declines rapidly during the first few days of lactation and the higher mitogenic activity of colostrum compared with mature milk may partly be attributed to the growth-promoting effects of IGF-1 (31). IGF-1 has a local effect on proliferation and maturation of the gut mucosa but is not normally absorbed intact into the circulation. Colostrum feeding reduces the incidence and severity of NEC-like lesions in preterm pigs compared to formula feeding (32–34), while studies in neonatal piglets have observed increased growth, epithelial absorption and enzyme activity in the small intestine after enteral IGF-1 supplementation (35–37). Furthermore, parenteral IGF-1 administration attenuates artificially induced intestinal damage in weaned rodents by decreasing apoptosis and increasing proliferation of epithelial cells (38–40). Preterm pigs show reduced circulating IGF-1 levels compared with term pigs (41) and our previous studies show that two daily subcutaneous injections of rhIGF-1 (1.0 mg/kg/d) reduce the severity of NEC and increase intestinal brush border peptidase activities and villus heights (42). In these studies, treatment also increased circulating neutrophil counts, thereby partly preventing postnatal neutropenia (42–44), and increased circulating IGF-1 to mean levels of ~40 ng/mL, still well below values in term suckling pigs [~70 ng/mL, (42)]. Clinical safety and efficacy of rhIGF-1 given at higher doses, near the physiological levels of term pigs, and for a longer period are not known.
IGF-1 exerts its receptor-mediated action in various organs supporting cell survival and growth, with or without effects on nutrient metabolism and glucose homeostasis. IGF-1 has insulin-like activity and increases cellular glucose uptake and utilization in the developing gut (37) and brain (45). Accordingly, IGF-1-deficient young mice show hyperinsulinemia and peripheral insulin resistance (46, 47). Clinical investigations indicate that IGF-1 may protect against development of glucose intolerance or type-2 diabetes (48) and subcutaneous IGF-1 treatment reduces insulin required to maintain normoglycemia in type-1 diabetes patients (49–51). Dysregulated glucose metabolism is a common complication of premature birth. Initially, preterm infants may suffer from hypoglycemia, which is partly related to their low glycogen stores and impaired glucogenolytic/gluconeogenic activity (52–54). Continuous parenteral glucose infusion is often required to maintain glucose levels within the first week after preterm birth and, especially in preterm pigs, periods of hypoglycemia may prevail for several weeks if fed exclusive enteral nutrition (41). Conversely, hyperglycemia (blood glucose ≥10.0 mmol/L) is also a common observation during the first postnatal weeks due to a combination of insulin resistance, pancreatic β-cell immaturity and parenteral nutrient infusion (54, 55). The risk of hyperglycemia is dependent on gestational age and birth weight and associated with increased mortality and morbidities [e.g., neurodevelopmental delay, IVH and sepsis, (54–56)]. Low serum IGF-1 levels are associated with hyperglycemia just after birth in very low birth weight infants (9). Reduced mortality, especially from sepsis, is observed after insulin treatment in critically ill adults (57) and extremely preterm infants (55) with hyperglycemia. Conversely, early insulin therapy of preterm infants without severe hyperglycemia may be harmful (58).
As a follow-up to our previous study (42), the objective of this study was to further evaluate preclinical safety and efficacy of physiological increases in circulating IGF-1 levels during the first weeks after preterm birth, when the most critical postnatal adaptation occurs. Mode and length of IGF-1 administration are crucial for clinical feasibility. Hence, we tested the effects of both longer-term, intermittent and shorter-term, continuous dosing on gut, metabolism and additional immune–related outcomes. Using the preterm pig as a model of preterm infants, we hypothesize that restoring circulating IGF-1 levels close to those in term counterparts will improve clinical and paraclinical outcomes, gut development and glucose homeostasis. To test our hypothesis, rhIGF-1 was first administered via subcutaneous (s.c.) catheters three times daily from day 1–9 after preterm birth (Experiment 1). An additional study tested the effects of a shorter protocol where rhIGF-1 was administrated continuously via parenteral nutrition from day 1–5 (Experiment 2).
Materials and methods
Animal studies were conducted in accordance with the European Communities Council Directive 2010/63/EU for the protection of animals used for experimental purposes and approved by the Danish Animal Experiments Inspectorate, Ministry of Environment and Food of Denmark. All procedures followed the ARRIVE guidelines for animal experimentation (59) and personnel participating in the studies was blinded to the treatments.
Delivery, housing and surgical procedures
The piglets (Landrace x Yorkshire x Duroc) were delivered by cesarean section at 90% of expected gestation (full term = 117 ± 2 days), housed in heated incubators and infused with parental nutrition (PN) and enteral nutrition (EN) via umbilical and orogastric catheters, respectively, as previously described (42) (Figure 1A). The EN was composed of commercially available products used for feeding infants. Formula recipe, PN and EN composition, and total nutrient supply, are shown in Supplemental Tables 1–3. Autopsy was performed on all randomized animals. However, only pigs surviving at least until day 5 in Experiment 1 and until day 4 in Experiment 2 were included in organ sampling and further laboratory analyses.
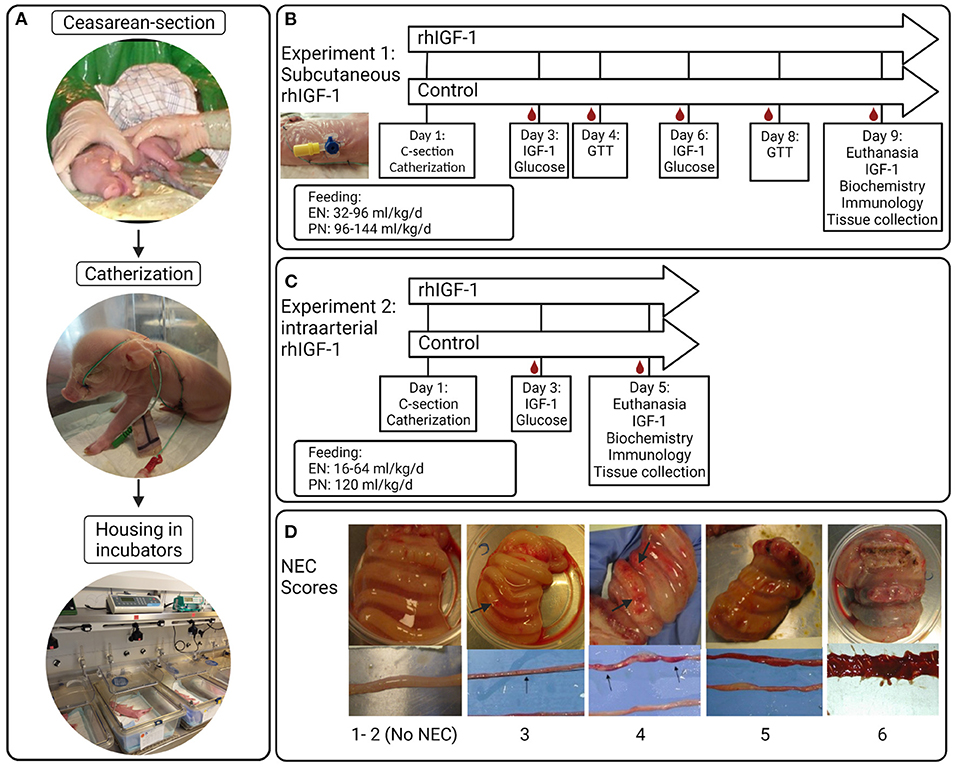
Figure 1. Overview of study procedures, experimental design and NEC scores for small intestine and colon. (A) Preterm pigs are delivered by cesarean section, resuscitated and fitted with an orogastric feeding tube and umbilical artery catheter. Pigs are supplemented parenteral nutrition to meet energy requirements and housed individually in heated incubators. (B) Blood sampling, injections and feeding regimens in Experiment 1 with subcutaneous rhIGF-1 administration. (C) Blood sampling, injections and feeding regimens in Experiment 2 with intra-arterial rhIGF-1 administration. (D) Examples of small intestinal and colonic tissues with NEC scores 1-6; 1 = absence of lesions, 2 = local hyperaemia, 3 = hyperaemia, extensive edema and local hemorrhage, 4 = extensive hemorrhage, 5 = local necrosis or pneumatosis intestinalis, 6 = extensive necrosis and pneumatosis intestinalis. Note the hemorrhagic lesions (arrows). rhIGF-1, recombinant human insulin-like growth factor-1; IGF-1, insulin-like growth factor-1; GTT, glucose tolerance test; NEC, necrotizing enterocolitis. The figure was created using BioRender.
Experiment 1: Longer-term subcutaneous rhIGF-1 administration
A 1:1 molar ratio of the non–covalent complex rhIGF-1/rhIGFBP-3 (rhIGF-1, mecasermin rinfabate) and formulation vehicle were provided by Takeda, Lexington, MA, United States. Based on literature studies in infants and pigs, we aimed for a circulating IGF-1 range of 30–110 ng/ml (3, 4, 41, 42). 73 piglets were delivered from three sows. Five piglets were euthanized before randomization due to multi-organ immature dysfunctions (mainly respiratory distress). Within each litter, pigs were block-randomized according to birth weight and gender into two groups: Pigs given 0.75 mg/kg rhIGF-1 (n = 34, male/female: n = 16/18) or equivalent volumes of vehicle (n = 34, male/female: n = 17/17) three times daily at 6.30 am, 2.30 pm and 10.30 pm via the s.c. catheter until they were euthanized on day 9. The experimental setup and placement of s.c. catheters are shown in Figure 1B. The predetermined volume of PN on individual study days (up to 144 mL/kg/d, Supplemental Table 2) was adjusted for individual pigs according to any clinical signs of dehydration, diarrhea or edema. Increasing amounts of enteral formula was provided from day 1 (32–96 mL/kg/d). Glucose concentration in the PN bags was kept at a relatively high level (140–180 g/L, Supplemental Table 1), resulting in a total supply of carbohydrate of ~10–25 g/kg/d across the study days from PN and EN (Supplemental Table 2). The purpose of the relatively high PN glucose supply was to challenge the glucose metabolic capacity of the pigs, thereby better allowing rhIGF-1 treatment to show effect on glucose clearance and insulin sensitivity.
Experiment 2: Short-term continuous systemic rhIGF-1 administration
Two sows delivered 45 piglets. Seven pigs were euthanized before randomization due to multi-organ immature dysfunctions (mainly respiratory distress). The IGF-1 product and target treatment goals were identical to those in Experiment 1. Within each litter, pigs were block-randomized according to birth weight and gender into two groups: Pigs given continuous administration of 2.25 mg/kg/day rhIGF-1 (n = 19, male/female: n = 11/8) or equivalent volumes of vehicle (n = 19, male/female: n = 11/8) via the umbilical arterial catheter, together with the PN solution, until they were euthanized on day 5. The experimental setup is shown in Figure 1C. Supply of PN was kept at 120 mL/kg/d and EN increased from day 1–5 (16–80 mL/kg/d), resulting in a carbohydrate supply of 11–13 g/kg/d (Supplemental Table 3).
Clinical observations and in vivo tests
Pig health status was evaluated daily by body weight along with clinical and fecal status twice a day using a validated scoring system (41) (except that fecal score 5 included diarrhea with visual blood). Pigs were euthanized if severe respiratory distress, sepsis, severe NEC symptoms or other severe clinical symptoms occurred after start of treatment but before the end of the experiments (denoted “all-cause mortality”). Pigs dying earlier than 12 h postnatally (on day 1) were excluded from all-cause mortality analysis. Intestinal permeability was assessed by the lactulose-mannitol method, just prior to euthanasia, as previously described (42).
Circulating IGF-1 levels
In Experiment 1, blood samples were taken via the umbilical catheter three (on day 6), five (on day 3) and eight (on day 9) hours after injection and via intracardial puncture under anesthesia just before euthanasia. In Experiment 2, blood samples were obtained on day 3 and 5 via the umbilical catheter, and via intracardial puncture under anesthesia just before euthanasia. Comparison between IGF-1 levels in samples obtained from the umbilical catheters and intracardial puncture indicated IGF-1 contamination via the umbilical catheter, resulting in falsely elevated IGF-1 measurements (Supplemental Table 4). Thus, in Experiment 2, only day 5 samples obtained via intracardial puncture were used to quantify circulating IGF-1 levels. Blood was collected in EDTA-coated tubes and plasma was separated by centrifugation at 2,000 x g for 10 min and stored at −80°C. All samples were analyzed using a human IGF-1 ELISA kit (Mediagnost GmbH, Reutlingen, Germany) in a bioanalytical testing laboratory (PPD Laboratories, Richmond, Virginia). The lower limit of quantitative detection was 20 ng/mL, but values at 10–20 ng/mL were included in the analyses to estimate low values in control animals. Values lower than 10 ng/mL were assigned a value of 5 ng/mL in the quantitative evaluations.
Blood biochemistry, hematology and systemic immune analyses
Blood was collected on the day of euthanasia in heparin vacutainers (BD Diagnostics, Oxford, United Kingdom) via the umbilical catheter or jugular vein. Biochemistry of plasma and cell counting of whole blood were analyzed using an Advia 1,800 Chemistry System and an Advia 2120i Hematology system (Siemens Healthcare, Ballerup, Denmark), respectively.
For T cell subset characterization, FACS lysing solution (BD Biosciences, Franklin Lakes, New Jersey), was added to 100 μL blood to lyse the red blood cell and the samples were washed twice in phosphate-buffered saline (PBS) containing 1% fetal bovine serum (FBS). The leukocytes were incubated with fixation/permeabilization buffer (Invitrogen, Carlsbad, California) for 30 min at 4°C in the dark, washed with permeabilization buffer [10% permeabilization concentrate (Invitrogen) in distilled water] and blocked with porcine serum (Thermo Fisher, Roskilde, Denmark) for 15 min 4°C in the dark. Subsequently, the leucocytes were stained with a mastermix containing the following monoclonal antibodies (mAbs); PE-Cy7- conjugated mouse anti-pig CD3ε (clone BB23–8E6–8C8, BD bioscience), FITC-conjugated mouse anti-pig CD4α (clone MIL17, Bio-Rad Laboratories, Hercules, California), PE-conjugated mouse anti-pig wCD8α (clone MIL12, Bio-Rad Laboratories) and APC-conjugated rat anti-mouse FOXP3 Monoclonal Antibody (clone FJK-16s, Thermo Fisher Scientific). The stained cells were washed twice in permeabilization buffer and examined using a multicolor BD Accuri C6 flow cytometer (BD Biosciences) equipped with BD FACSDiva software. The lymphocytes population was gated based on the FSC vs. SSC plot located FSClowSSClow and 10.000 CD3 + events were analyzed in each sample. T cells, T- helper cells (Th), cytotoxic T cells (Tc) and regulatory T cells (Tregs) were identified as CD3 + lymphocytes, CD3 + CD4 + CD8- lymphocytes, CD3 + CD4-CD8 + lymphocytes, and CD3 + CD4 + FoxP3 + lymphocytes, respectively.
The phagocytic activity of neutrophils against gram-negative bacteria was assessed using pHrodo Red E. coli (560/585 nm) BioParticles Phagocytosis Kits for flow cytometry (Thermo Fisher Scientific, Roskilde, Denmark). Samples were prepared as previously described (43) and analyzed on the flow cytometer. The neutrophil population was gated based on the FSC vs. SSC plot located FSChighSSChigh and 5,000 neutrophils were analyzed in each sample. The proportion of pHrodo + neutrophils in the neutrophil population indicated the phagocytic activity (%) and the median fluorescence intensity (MFI) represented the phagocytic capacity of the neutrophils.
To characterize the pigs' ability to regulate systemic inflammatory response, 294 μL whole blood was incubated with a TLR4 agonist (lipopolysaccharide, LPS, E.coli O127:B8, Sigma Aldrich, Søborg, Denmark) for 5 h at 37°C, 5% CO2. After incubation, the blood was centrifuged at 2,000 × g, 4°C for 10 min and supernatants were collected for analysis of TNF-α by ELISA (R&D Systems, Abingdon, UK), following the manufacturer's guidelines. The plasma samples were diluted 1:2.5. The lowest standard of the analyses was 31.3 pg/mL and measurements less than this were assigned a value of 15.8 pg/mL. The TNF-α response to LPS stimulation was analyzed as the percentage change in TNF-α levels in LPS-stimulated vs. unstimulated samples for each animal.
Glucose tolerance test, insulin and glucose levels
In Experiment 1, subgroups of pigs underwent an intra-arterial (i.a.) glucose tolerance test (IAGTT) on day 4 (n = 11/group) and day 8 (n = 6–7/group). After a 4 h fasting period, an i.a. bolus of 1.0 mg/kg glucose (from a 50% glucose solution) was administrated and the catheter was flushed thoroughly with saline. Then arterial blood was sampled in EDTA tubes at 0, 5, 10, 20, 40, and 60 min. Samples were centrifuged at 2,000 x g for 10 min and plasma was collected and stored at −80°C. Samples for basal insulin were obtained on day 3 and 6 in Experiment 1, and on day 3 and 5 in Experiment 2. Plasma insulin was analyzed by a commercial porcine ELISA kit (Mercodia, Salem, NC). Measurements below level of detection (2 mU/L) were assigned to 2 mU/L. Plasma glucose responses from the IAGTT was measured using a colorimetric assay (Thermo Scientific Waltham, MA), whereas basal glucose levels were measured by a glucometer (ACCU-CHEK, Roche Diagnostics, Hvidovre, Denmark) following ear vein puncture on day 3, 4, 6, and 8 in Experiment 1 and on day 3 in Experiment 2.
Tissue collection and NEC evaluation
Pigs were euthanized by intracardial administration of sodium-pentobarbital (Euthanimal, Scanvet, Denmark) on day 9 and 5, in Experiment 1 and 2, respectively. The stomach, proximal, middle and distal small intestine and colon were evaluated for macroscopic signs of NEC by two independent observers, as previously described (42, 60). NEC scores are shown in Figure 1D. “NEC” was defined as a score of at least 3 in one or more gut regions, whereas a score of at least 4 in one or more gut regions was defined as “severe NEC”. An average NEC severity score of the total gastrointestinal tract was calculated as the mean of the highest score of the small intestinal (proximal, middle or distal), colon and stomach regions. Pigs dying earlier than 12 h postnatally (on day 1) were excluded from NEC analysis. Organs were weighed and tissue from small intestine and colon was snap frozen and stored at −80°C to evaluate enzyme activities and levels of pro-inflammatory cytokines.
Gut protein synthesis, morphology, enzyme activity and inflammatory markers
Fractional rates of protein synthesis were measured in pigs euthanized day 8–9 in Experiment 1 and day 5 in Experiment 2, with a flooding dose (10 ml/kg) of L-phenylalanine (Phe) (1.50 mmol/kg) containing L-[ring-2H5]Phe at 30 mol % (0.45 mmol/kg) (Cambridge Isotope Laboratories, Tewksbury, MA) injected i.a. via the umbilical catheter 30 min prior to euthanasia. Collected distal small intestine samples were snap-frozen in liquid nitrogen and stored at −80°C. Analysis for tracer enrichment in the intestine tissue and calculation of the fractional protein synthesis rate (FSR, % protein mass synthesized/day) were performed as previously described (61). Villus height and crypt depth in the proximal small intestine were measured in paraformaldehyde-fixed slices following H&E and Alcian-blue/periodic acid-schiff staining (42). In Experiment 1, the degree of proliferation was measured with Ki67 immunohistochemical (IHC) staining in eight representative pictures of proximal small intestine sections and reported as the mean Ki67 positive area of the total nuclei area in tunica mucosa (%), as previously described (42). The degree of apoptosis was assessed by caspase-3 IHC staining and measured as mean caspase-3 positive area relative to the total mucosal cell area (%) in eight representative pictures. Brush border enzyme activities were measured in the proximal and distal small intestine in both experiments. Assays were performed using specific substrates, as described previously (62). Interleukin (IL)-1β, −6, and −8 levels in colon homogenates were analyzed using DuoSet ELISA kits (R&D systems, Minneapolis, MN) following the manufacturer's instruction. Each sample was tested in duplicate.
Statistical analysis
The statistical software package R (version 3.6.1) was used for statistical analyses. Statistical significance of difference in continuous variables was analyzed by linear models, if data were normally distributed. The model was tested for normality, data was log-transformed when required and non-parametric analysis was applied if data could not be transformed to reach normal distribution. Binary data were analyzed by a logistic regression model. Ordered categorical outcomes (e.g., NEC score) were analyzed by a proportional odds logistic regression model. Repeated measurements were analyzed by the linear mixed effect model. The above mentioned models were adjusted for possible confounders like litter, sex and birth weight. A p value < 0.05 was considered statistically significant and 0.05 ≤ p < 0.10 was noted and discussed as a tendency to an effect. Values were expressed as mean ± standard error of mean (SEM), unless otherwise specified. Levels of significance were assigned as *p < 0.05, **p < 0.01, ***p < 0.001.
Results
Experiment 1: Longer-term subcutaneous rhIGF-1 administration
Circulating IGF-1 levels
Three, five and eight h after s.c. rhIGF-1 bolus, the plasma IGF-1 levels in rhIGF-1 pigs were 66.6 ± 6.0 ng/mL (n = 20), 82.1 ± 3.4 ng/mL (n = 25) and 51.2 ± 7.8 ng/mL (n = 12), respectively (mean ± SEM, Figure 2A). One rhIGF-1 pig was excluded from all analyses because of a malfunctioning s.c. catheter, indicated by repeatedly low IGF-1 plasma levels despite the rhIGF-1 injections (Supplemental Table 5). In corresponding control pigs, the plasma IGF-1 levels were 24.6 ± 1.8 ng/mL (n = 23), 34.6 ± 2.7 ng/mL (n = 25) and 26.3 ± 3.6 ng/mL (n = 10, Figure 2A). When pigs were euthanized in a random sequence on day 9, control pigs had low IGF-1 values (19.9 ± 2.6 ng/mL, n = 15, Figure 2B), while pigs treated with rhIGF-1 had plasma IGF-1 levels within the desired physiological range at 1–6.5 h after the last injection (91.0 ± 11.8 ng/mL, n = 20, Figure 2B).
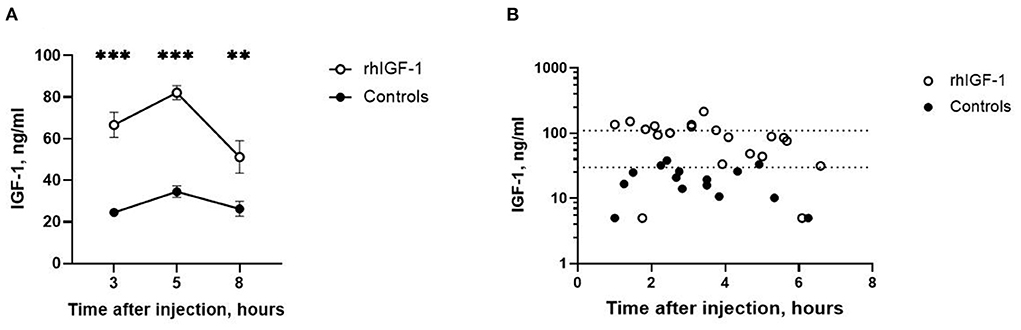
Figure 2. Circulating IGF-1 levels in Experiment 1. (A) Plasma IGF-1 levels in rhIGF-1 and control preterm pigs after a subcutaneous injection of rhIGF-1 or vehicle (mean ± SEM, n = 13–26). (B) Plasma IGF-1 levels at euthanasia 1–7 h after the last subcutaneous injection of rhIGF-1 or vehicle on day 9. Each point represent one pig. The dotted lines indicate the desired physiological range. IGF-1, insulin-like growth factor-1; rhIGF-1, recombinant human insulin-like growth factor-1. **p < 0.01, ***p < 0.001.
Clinical assessments
No differences were found between rhIGF-1 and control pigs in baseline conditions such as birth weight (945 ± 41 vs. 938 ± 41 g), rectal temperature at 1 h (37.0 ± 0.2 vs. 37.0 ± 0.2) and 24 h after birth (38.1 ± 0.2 vs. 38.5 ± 0.2). The treatment had no effect on clinical score. Fecal score increased throughout the study period with no consistent differences between groups (Supplemental Table 6).
Twelve rhIGF-1 pigs and 19 control pigs were euthanized or died ahead of the pre-determined end of the experiment at day 9 (p = 0.27). The symptoms leading to early euthanasia were respiratory failure, severe NEC symptoms or poor tissue perfusion of hind legs reflected by development of bluish/grayish tissue color (four control pigs). One rhIGF-1 pig was euthanized after blood loss due to a disconnected PN line. The remaining pigs (21 rhIGF-1 pigs and 15 control pigs) were euthanized as planned on day 9. At tissue collection, the small intestinal weight of rhIGF-1 treated pigs was 20% higher than in control pigs, with both the proximal and distal regions affected (all p < 0.05). The rhIGF-1 pigs also showed a tendency to increased small intestine length (+11%, p = 0.09) and spleen weight (+26%, p = 0.08, Table 1). Blood biochemistry showed an increase in gamma-glutamyltransferase (GGT) and sodium levels in the blood of rhIGF-1 pigs compared with control pigs (both p < 0.05, Table 2).
Hematology and immunological parameters
No differences in hematological values were observed between rhIGF-1 pigs and control pigs on day 9 (Table 3). TNF-α increases in response to LPS stimulation were highly variable and showed no difference between rhIGF-1 pigs (28.7 ± 16.9%, n = 15) and control pigs (60.2 ± 47.5%, n = 14). FACS analysis showed that the phagocytic activity and phagocytic capacity of neutrophils were similar between rhIGF-1 pigs (93.8 ± 1.1% and 3.8 ± 0.0 log (MFI), respectively, n = 19) and control pigs (94.2 ± 1.3% and 3.8 ± 0.1 log (MFI), respectively, n = 15). Also, no differences were observed between rhIGF-1 pigs (n = 19) and control pigs (n = 15) in %T cells of lymphocytes (35.4 ± 2.7 vs. 36.0 ± 4.0), %Th cells of T cells (54.5 ± 1.3 vs. 52.7 ± 2.0), %Tc cells of T cells (4.1 ± 0.4 vs. 4.4 ± 0.5) or %Treg cells of T cells (5.8 ± 0.8 vs. 5.5 ± 1.1). Consistently, no differences were observed in the total number (billions/L) of T cells (1.2 ± 0.3 vs. 1.4±0.2), Th cells (1.8 ± 0.3 vs. 2.0 ± 0.3), Tc cells (0.1 ± 0.0 vs. 0.2 ± 0.0), or Treg (0.2 ± 0.0 vs. 0.2 ± 0.0) in blood from rhIGF-1 pigs (n = 19) and control pigs (n = 13).
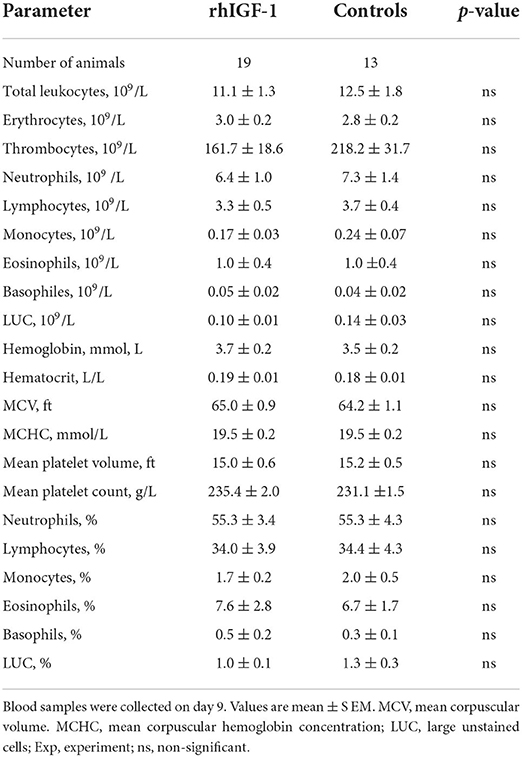
Table 3. Hematology of preterm pigs treated with rhIGF-1 or vehicle formulation subcutaneously for 8 days in Experiment 1.
Glucose tolerance test and basal insulin and glucose levels
Plasma insulin and glucose responses to IAGTT were similar among groups on day 4 and 8 (Figures 3A,B). Compared with day 4, the area under the curve of insulin levels was significantly reduced at day 8, indicating a degree of insulin resistance in the preterm pigs on day 4 (p = 0.01, Figure 3A). Basic insulin and blood glucose did not differ significantly between groups (Figures 3C,D), but a tendency toward reduced insulin levels was observed on day 3 in rhIGF-1 pigs (p = 0.08). Large individual variation in plasma insulin levels was observed, particularly on day 6, with levels ranging 13.9–502 pmol/L in the rhIGF-1 pigs and 13.9–327 pmol/L in the control pigs (Figure 3C). On days 3–4, the high-glucose PN induced a moderate hyperglycemia (mean values ~8 mmol/L) relative to normal glucose values in suckling term pigs at 4–5 mmol/L (41, 63). On day 6–8, the glucose levels decreased to normal levels (mean values ~5 mmol/L), potentially due to improved glucose tolerance in the pigs combined with the decreasing volume of PN glucose at this time of the experiment.
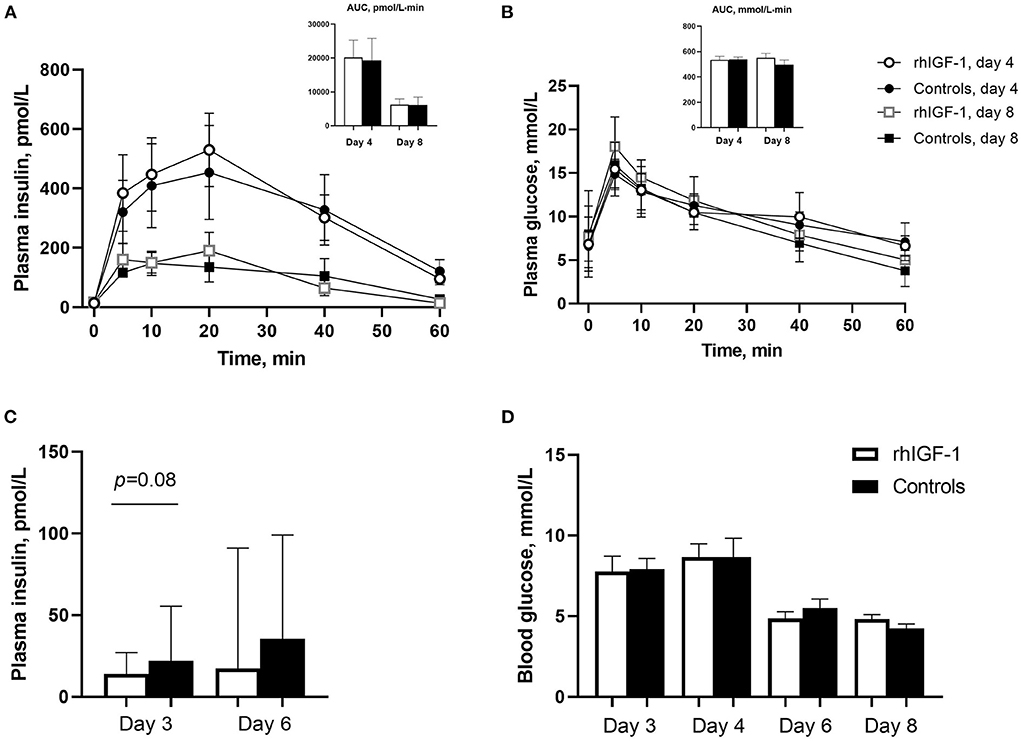
Figure 3. Blood insulin and glucose levels in Experiment 1. Plasma insulin (A) and glucose (B) levels during glucose tolerance test in rhIGF-1 and control preterm pigs day 4 (n = 11) and 8 (n = 6–7). (C) Basal plasma levels of insulin in rhIGF-1 and control preterm pigs day 3 (n = 25) and 6 (n = 20–23). (D) Blood glucose levels in rhIGF-1 and control preterm pigs day 3 (n = 24–25), 4 (n = 24–25), 6 (n = 22–23) and 8 (n = 14–18). rhIGF-1, recombinant human insulin-like growth factor-1; AUC, area under the curve. Values in (A,B,D) are presented as mean ± SEM. Values in (C) are presented as median ± IQR.
NEC lesions
Across the 2–9 day period, overall incidence of NEC (52%, 16/31 vs. 74%, 25/34, p = 0.06, Figure 4A) and the average NEC severity across the entire gut tended to be lower in rhIGF-1 pigs than in control pigs, but the differences did not reach statistical significance (1.9 ± 0.2 vs. 2.3 ± 0.2, p = 0.06, Figure 4B). The rhIGF-1 pigs showed a reduced combined incidence of all-cause mortality (day 2-8) and severe NEC on day 9 (55%, 17/31 vs. 79%, 27/34, p < 0.05, Figure 4C). Colon, stomach, and small intestine NEC lesions were present in 28 (68%), 16 (39%), and 14 (34%) out of the total 41 NEC cases in the study, respectively, thus all gut regions were involved in NEC development, with colon lesions occurring most frequently. NEC lesions in the stomach region were reduced in rhIGF-1 pigs versus control pigs (p < 0.05), whereas no significant differences were observed in NEC scores in small intestine and colon between groups (Figure 4D).
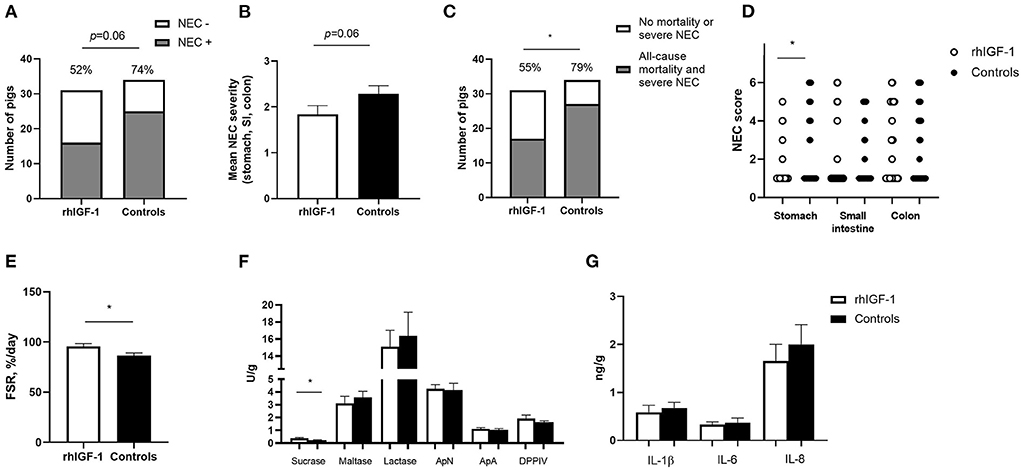
Figure 4. Intestinal parameters in Experiment 1. (A) Incidence of NEC (score ≥ 3 in at least one gut region) day 2–9 in rhIGF-1 and control preterm pigs (n = 31–34). (B) Average NEC severity score across gut regions in rhIGF-1 and control preterm pigs day 2–9 (n = 31–34). (C) Incidence of all-cause mortality and severe NEC day 2–9 (n = 31–34). (D) Individual NEC score distribution in stomach, small intestine (highest score of proximal, middle and distal section) and colon in rhIGF-1 and control preterm pigs day 2–9. (E) Fractional protein synthesis rate (FSR) in the distal small intestine of rhIGF-1 (n = 19) and control (n = 14) preterm pigs. (F) Brush border enzyme activities in proximal small intestine (n = 23–24). (G) Pro-inflammatory cytokines in colon (n = 22–24). rhIGF-1, recombinant human insulin-like growth factor-1; NEC, necrotizing enterocolitis; ApN, aminopeptidase N; ApA, aminopeptidase A; DPPIV, dipeptidylpeptidase; IL, interleukin. Values are mean ± SEM. *p < 0.05.
Gut protein synthesis, morphology, function and inflammation
Protein synthesis in the distal small intestine was higher in rhIGF-1 than control pigs (+11%, 95.8 ± 2.7 vs. 86.6 ± 2.5, n = 14–19, p < 0.05, Figure 4E). No differences were observed between rhIGF-1 pigs and control pigs for villus heights (388 ± 27 vs. 396 ± 31 μm), crypt depths (84 ± 2 vs. 83 ± 2 μm), villus:crypt ratio (4.6 ± 0.3 vs. 4.8 ± 0.4), caspase-3 density (0.85 ± 0.42 vs. 0.79 ± 0.32%) or ki-67 density (32.8 ± 4.7 vs. 30.0 ± 3.3%) in the proximal small intestine (n = 19–20). This conclusion did not change after removing pigs with NEC lesions specifically in the proximal small intestine.
rhIGF-1 treated pigs had significantly increased levels of sucrase activity in the proximal region of the small intestine, compared with control pigs (p < 0.05, Figure 4F), whereas no differences were observed between groups in brush border enzymes in the distal region of the small intestine (data not shown). The levels of pro-inflammatory cytokines in colon were similar between rhIGF-1 and control pigs (Figure 4G) and no differences were observed for intestinal permeability (urinary lactulose/mannitol ratio, rhIGF-1: 0.13 ± 0.06, n = 15 vs. controls: 0.08 ± 0.03, n = 14). Likewise, the groups did not differ in density of bacteria detected in the bone marrow (logarithm of number colony-forming units: 3.98 ± 0.37, n = 21 vs. 3.82 ± 0.40, n = 19, respectively).
Experiment 2: Short-term continuous systemic rhIGF-1 administration
Clinical values and circulating IGF-1 levels
No differences were found between rhIGF-1 pigs and control pigs in baseline conditions such as birth weight (876 ± 52 vs. 890 ± 54 g) or rectal temperature at 1 h (36.8 ± 0.1 vs. 36.5 ± 0.2) and 24 h after birth (37.5 ± 0.1 vs. 37.7 ± 0.1) (Supplemental Table 7). No consistent differences in clinical and fecal score were noted between groups and no differences in overall mortality were found. One control pig was euthanized on day 2 because of clinical NEC symptoms and one rhIGF-1 pig was euthanized on day 3 because of catheter-related problems. The remaining pigs (18 pigs/group) were euthanized as planned on day 5 with body and organ weights shown in Table 1. Weight gain and organ weights were similar between groups, except for the spleen, which showed increased weight in rhIGF-1 pigs (+28%, p = 0.001). Further, plasma levels of creatine kinase, creatinine, aspartate aminotransferase and potassium were reduced in rhIGF-1 pigs (all p ≤ 0.05, Table 2). There was no difference in blood glucose on day 3 (3.5 ± 0.3 vs. 4.2 ± 0.3 mmol/L). Basal insulin levels could not be detected by our assay, neither on day 3 or 5, in neither of the groups (data not shown). At euthanasia day 5, plasma IGF-1 levels were higher in rhIGF-1 than control pigs (70.9 ± 4.1, n = 17 vs. 27.7 ± 4.2 ng/mL, n = 18, Figure 5A).
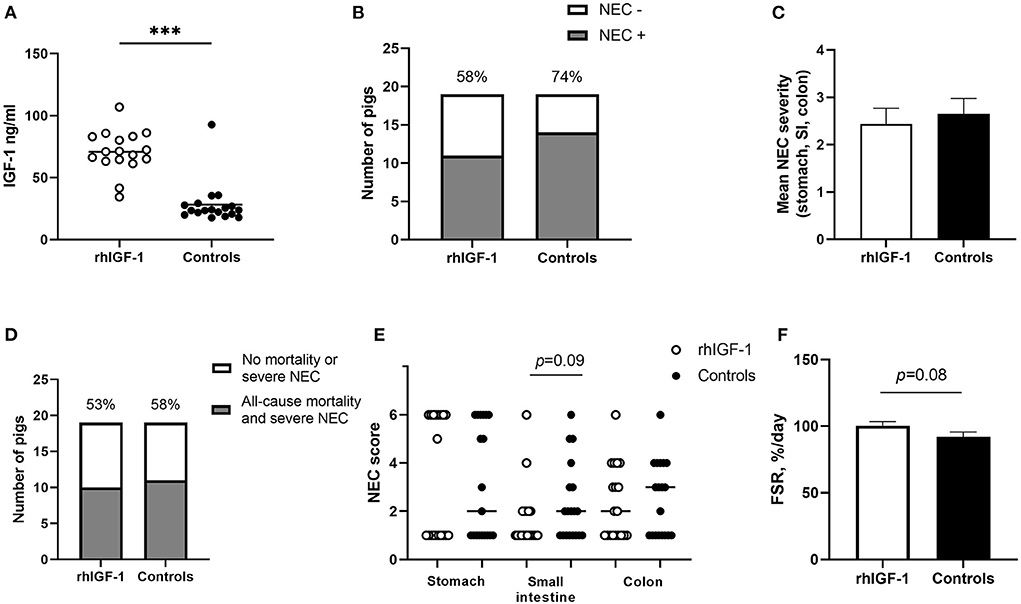
Figure 5. IGF-levels and intestinal parameters in Experiment 2. (A) Circulating IGF-1 levels at day 5 among rhIGF-1 pigs and control pigs (n = 17–18). (B) Incidence of NEC (score ≥ 3 in at least one gut region) day 2–5 in rhIGF-1 and control preterm pigs (n = 19). (C) Average NEC severity score across gut regions in rhIGF-1 pigs and control pigs day 2–5 (n = 19). (D) Incidence of all-cause mortality and severe NEC, day 2–5 (n = 19). (E) Individual NEC score distribution in stomach, small intestine (highest score of proximal, middle and distal section) and colon in rhIGF-1 and control preterm pigs day 2–5. F) Fractional protein synthesis rate (FSR) in the distal small intestine of rhIGF-1 (n = 17) and control (n = 14) preterm pigs. rhIGF-1, recombinant human insulin-like growth factor-1; NEC, necrotizing enterocolitis; SI, small intestine. Values in (A), (D) and (F) are means ± SEM. ***p < 0.001.
Hematology and immunological parameters
No differences in hematological values were observed between rhIGF-1 pigs and control pigs on day 5 (Table 4). Also, FACS analysis showed no significant difference in phagocytic activity and phagocytic capacity of neutrophils (rhIGF-1: 71.5 ± 6% and 4.3 ± 0.0 log (MFI), n = 18 vs. controls: 65.8 ± 6% and 4.3 ± 0.0 log (MFI), n = 17). For TLR4 stimulation of whole blood, no difference in TNF-α levels was observed between the groups.
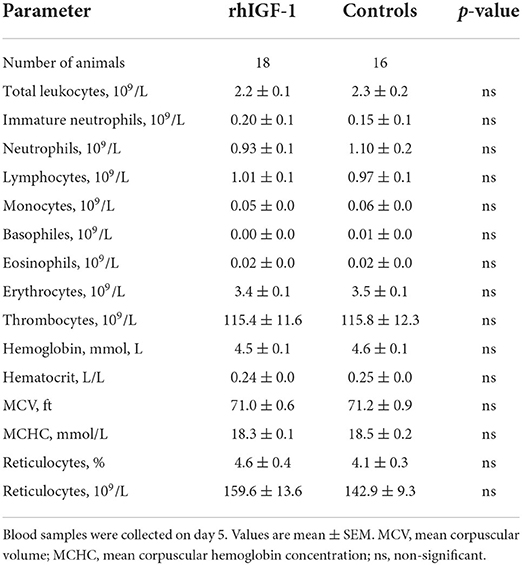
Table 4. Hematology of preterm pigs treated with rhIGF-1 or vehicle formulation, continuous intra-arterially for 4 days in Experiment 2.
Gut NEC lesions, morphology, function and inflammation markers
Across the 2–5 day period, NEC incidence (rhIGF-1: 58%, 11/19 vs. controls: 74%, 14/19, Figure 5B) and the average NEC score across the gut (2.4 ± 0.3 vs. 2.7 ± 0.3, Figure 5C) did not differ between groups. Likewise, the combined incidence of all-cause mortality (day 2–4) and severe NEC on day 5 (53%, 10/19 vs. 58%, 11/19, Figure 5D) did not differ between groups. Colon, stomach, and small intestine NEC lesions were present in 18 (72%), 18 (72%) and 8 (32%) of the total 25 NEC cases. rhIGF-1 treated pigs tended to have reduced NEC scores in the small intestine (p = 0.09, Figure 5E).
The mean fractional protein synthesis rate in the distal small intestine was 9% higher in rhIGF-1 pigs compared with controls (100.4 ± 3.0 vs. 92.1 ± 3.6, n = 14–17/group, p = 0.08, Figure 5F). There were no differences in villus height (525 ± 30 vs. 524 ± 35 μm), crypt depth (118 ± 6 vs.111 ± 5 μm) or villus to crypt ratio (4.7 ± 0.4 vs. 4.5 ± 0.3) in the proximal small intestine of rhIGF-1 and control pigs, respectively. Removing pigs with NEC in the proximal intestine did not change this conclusion. No differences were observed in lactulose/mannitol ratio (0.12 ± 0.03 vs. 0.09 ± 0.02, n = 11–17) and activities of gut enzymes (sucrase, maltase, lactase, ApN, ApA, and DPPIV) in proximal and distal small intestine. Likewise, no differences in IL-1β, IL-6 and IL-8 levels in the colon were observed.
Discussion
The association between circulating IGF-1 levels and a range of morbidities in preterm infants related to gut, lungs, bones and brain is well-documented (5–8, 12, 14, 15, 42). Yet, there is limited evidence of direct effects on such morbidities by supplementing IGF-1 during the first weeks after preterm birth. Thus, it is important to investigate the preclinical safety and efficacy of IGF-1 supplementation using clinically relevant administration methods and appropriate animal models of preterm infants. Preterm pigs delivered at 90% gestation show morbidities similar to those in many preterm infants, including reduced growth (41), impaired postnatal gut, brain and immune development (32–34, 41, 43, 44, 60, 64), neonatal hypoglycemia (41, 63) and reduced IGF-1 levels (41, 42), relative to their term-born counterparts. In this study, preterm pigs treated with rhIGF-1 s.c. for 8 days achieved circulating IGF-1 levels similar to those in term suckling pigs (42), reduced incidence of severe complications (reflected by the combined incidence of all-cause mortality day 2-8 and severe NEC on day 9), and increased intestinal weight and protein anabolic response. There were limited effects on a large series of measured gut structural, functional and immunological indices as well as on blood chemistry, hematology, glucose homeostasis and systemic immunity development. Collectively, the findings support our previous 5-day study (42) and other in vivo animal studies demonstrating increased intestinal mucosal growth after parenteral (65) and especially oral IGF-1 administration (35, 36, 66, 67), as well as the reduced NEC incidence in a rodent study (68).
In the present study, only the longer-term rhIGF-1 s.c. supplementation for 8 days improved gut growth in preterm neonates and tended to reduce NEC. Continuous systemic administration of rhIGF-1 for just 4 days had limited effects on NEC and gut parameters, contrasting the more clear effects in our earlier study, using twice daily s.c. administrations (42). Yet, the plasma IGF-1 levels appeared to be within the desired physiological range in both studies. Treatment effects may depend on the mode, dose and length of IGF-1 administration, and studies are required to know if different methods of IGF-1 supplementation during the first week(s) after preterm birth exert differential short- and long-term effects on the maturation of the gut as well as other organs. The reason why our continuous, intra-arterial infusion failed to demonstrate efficacy on stimulating gut parameters is currently unknown. Possibly, intermittent rhIGF-1 dosing is most efficacious for physiological responses at receptor levels in the gut of immature newborns. Also, we cannot exclude the possibility that the active rhIGF-1 drug interacted with components of the PN solution, negatively affecting its physiological effects.
Considering the trophic effect on the intestine after 8 days of s.c. rhIGF-1 treatment, it was surprising that IGF-1 did not affect mucosal structure, function or permeability. Also, we were unable to demonstrate proliferative or anti-apoptotic effects on the small intestinal epithelium, as tested by immunohistochemical staining. Possibly, longer-term, supra-physiological, IGF-1 administration is required to induce more robust effects on mucosal growth and function, acting via IGF-1 receptors on the intestinal epithelial cells (69). Evidence suggests that there may be only early and transient changes in intestinal crypt and villi morphology in response to trophic pharmacological therapies and that IGF-1 preferentially may target intestinal epithelial stem cells (IESC), located at the crypt base (70). Thus, IESC expansion and subsequent crypt fission (i.e., synthesis of new crypts) may reflect the adaptive growth and response to IGF-1 therapy more accurately (70). Beyond the gut, IGF-1 treatment did not appear to change the clinical status of the pigs, except for few indications of mild effects on biochemical markers of muscle, liver and kidney function. None of the findings were consistent across the current two experiments or our previous intervention study (42) and the levels were in the normal reference range of preterm pigs (e.g., aspartate aminotransferase, creatinine, sodium, potassium) (71, 72) or weaning farm piglets (e.g., gamma-glutamyltransferase) (73). Because local and systemic IGF-1 exposure potentially plays a role for liver, kidney, lung, bone and brain development and function (5–8, 12, 14, 15, 74, 75), we have recently conducted a longer-term study in preterm pigs to investigate if supplemental rhIGF-1 for 18 days affects these organs. By postnatal day 19, overall mortality was again reduced and spleen weight increased in rhIGF-1 treated pigs, whereas the biochemical profile was similar between groups (unpublished observations). Detailed organ-related and immune analyses from this study will be reported as a separate publication. In both infants and pigs, treatment effects may be highly organ-specific and local IGF-1 levels and receptor-mediated actions in the tissues may not necessarily reflect circulating IGF-1 levels. In this regard, preterm pigs are a valuable tool to test drug delivery procedures (enteral, parenteral, bolus, continuous, s.c., intravenous, intraperitoneal) for preterm infants and to investigate the pharmacodynamics of rhIGF-1, together with cell- and tissue-specific effects.
Disturbed glucose homeostasis is a common complication in preterm infants (54) and a clinical trial indicated improved glucose metabolism and tolerance after rhIGF-1 treatment (18). On the other hand, it is critical that rhIGF-1 treatment does not lead to hypoglycemia. In our study, basal glucose levels and plasma glucose and insulin responses to IAGTT, were similar between rhIGF-1 and control pigs. Some insulin resistance was evident in preterm pigs during the first 4–5 days of life as indicated by the higher insulin secretory response after IAGTT. This finding is consistent with other recent reports showing that preterm pigs are insulin resistant, compared with term pigs (76, 77). Later, insulin sensitivity and glucose tolerance were improved, probably due to maturation of peripheral insulin signaling, glucose transport and pancreatic β-cell function. Previous studies showed improved insulin sensitivity in neonatal pigs fed intermittent enteral formula compared with continuous PN (78, 79). Thus, improved insulin sensitivity in preterm pigs may also be associated with a reduction in continuous PN supply and increase in intermittent EN feeding throughout the study. In this context, the high mortality observed in Experiment 1 may partly result from hyperglycemia in response to the relatively high loads of glucose supplied via the PN. As previously described for critically ill adults and preterm infants, excessive supply of glucose via PN may lead to increased mortality and sepsis (55–57). The mechanisms behind such glucose-induced toxicity is not clear, but can probably be explained by glucose overload, mitochondrial dysfunction and oxidative phosphorylation in cellular systems with passive, insulin-independent glucose uptake, such as neurons, hepatocytes, endothelial and immune cells (80). Several studies have also shown an association between hyperglycemia and impaired innate immunity (80). We cannot exclude that the serious tissue perfusion problems observed in four control pigs (euthanized ahead of time) were also related to high glucose load but this, and the possible effects of rhIGF-1 supplementation to prevent glucose-related adverse symptoms, remain to be further investigated.
Like infants, pigs born preterm have impaired bacterial phagocytosis and pro-inflammatory responses related to innate immune defects (43, 44, 81, 82) and both species have high susceptibility to infection and bacteremia (17, 83–85). The role of IGF-1 in systemic immune development and function is complex and results are partly contradicting. The IGF-1 receptor (IGF-1R) is expressed on various leukocyte populations and IGF-1 supports differentiation, proliferation, survival and metabolism of T cell, B cell, granulocytic, and monocytic lineages and immunoglobulin production and class-switching (86–88). In neonates, IGF-1 promotes phytohemagglutinin (PHA)-induced proliferation, maturation, survival and IL-6 expression in cord blood mononuclear cells (CBMC) (86, 87). However, IGF-1 also displays anti-inflammatory properties suppressing IFN-γ expression and DNA binding activity of transcription factor nuclear factor-κB (NF-κB) and activator protein-1 (AP-1) in stimulated CBMC (89) and the TLR4/NF-κB pathway in ileum of a neonatal NEC rat model (68). This supports that rhIGF-1 treatment reduced mean blood TNF-α production after in vitro stimulation by ~50% in Experiment 1 (although the difference did not reach statistical significance) and also reduced basal blood IFN-γ and TNF-α expressions in our recent 19 day study (unpublished observations). A reduced immune response may potentially increase sepsis sensitivity. Effects of IGF-1 treatment on infant sepsis in the study of Ley et al. (18) were not significant (38 vs. 25% in controls), but possible immune effects of IGF-1 treatment warrant further study.
In preterm pigs, the leukocyte and neutrophil numbers, as well as phagocytic capacity, increase sharply in the second week of life, as part of a natural physiological maturation of the immune system (43). Interestingly, rhIGF-1 treatment increased the spleen weight both on day 5 and day 9, but we were unable to demonstrate any systemic immune effects by hematology, FACS or in vitro analysis of the blood. Accordingly, Clark et al. (88) showed that adult mice treated with continuous s.c. IGF-1 for 7 and 14 days had markedly increased spleen, thymus and kidney weights. The increased spleen weight was shown to be due to a pronounced increase in T cells and B cell numbers. However, among peripheral blood leukocytes only neutrophil counts were significantly increased. Clearly, there is a need to better understand the effects of supplemental rhIGF-1 on the primary and secondary lymphoid organs in preterm neonates following different stages of immaturity at birth and postnatal ages.
The highly sensitive preterm pig model enables preclinical studies of many clinically relevant topics and interventions that cannot be examined in preterm infants because of low incidence of selected diseases or ethical reasons. On the other hand, direct translation between infants and piglets, and across organs, is difficult due to species- and organ-specific maturational patterns (90). Thus, the nature and timing of spontaneous organ lesions, including NEC, may differ between 90% gestation preterm pigs and 60–80% gestation preterm infants. Yet, the high sensitivity to sepsis and NEC lesions in formula-fed preterm pigs, immature blood and tissue immune systems, and moderately immature brain structure/functions, make preterm pigs a useful model for very preterm infants within many areas of neonatology, with opportunities to control several external factors and access to all organs using clinically-relevant procedures.
In conclusion, neither longer-term intermittent nor short-term continuous systemic IGF-1 supplementation showed any marked effects on clinical or paraclinical outcomes related to growth, metabolism and immunity. However, the findings did suggest a positive effect of IGF-1 supplementation on potential overall viability and gut maturation and growth, particularly after a longer period of s.c treatment. The effects on spleen weight and immune responses deserve further investigation. It is important to investigate longer-term effects of various forms of IGF-1 supplementation across a range of organs and the preterm pig may be an excellent model for this. Collectively, the results add further preclinical evidence for the safety and efficacy of supplemental IGF-1 to hospitalized extremely preterm infants.
Data availability statement
The original contributions presented in the study are included in the article/Supplementary material, further inquiries can be directed to the corresponding author.
Ethics statement
The animal study was reviewed and approved by the Danish Animal Experiments Inspectorate, Ministry of Environment and Food of Denmark.
Author contributions
KH conceived and designed the experiments, acquired, analyzed and interpreted data, and wrote the paper. MR analyzed and interpreted data and wrote the paper. PTS conceived and designed the experiments, analyzed and interpreted data, helped to draft the paper, and took final responsibility for its contents. DB analyzed and interpreted data. TT and GC conceived and designed the experiments. All authors revised the manuscript critically for important intellectual content and approved the final version. All authors contributed to the article and approved the submitted version.
Funding
The authors declare that this study received funding from Takeda, MA, USA. The funder was not involved in the study design, collection, analysis, interpretation of data, the writing of this article or the decision to submit it for publication.
Acknowledgments
The authors like to thank Elin Skytte, Kristina Møller, and Shuqiang Ren for laboratory technical assistance and Jane Connie Povlsen, Britta Karlsson, Karoline Aasmul-Olsen, Nicole Lind Henriksen, and Anders Brunse for assistance with pig care.
Conflict of interest
Author GC was employed by the company Takeda, MA, USA. Author PTS is currently involved in a patent application directed to use of rhIGF-1 for preterm infants.
The remaining authors declare that the research was conducted in the absence of any commercial or financial relationships that could be construed as a potential conflict of interest.
Publisher's note
All claims expressed in this article are solely those of the authors and do not necessarily represent those of their affiliated organizations, or those of the publisher, the editors and the reviewers. Any product that may be evaluated in this article, or claim that may be made by its manufacturer, is not guaranteed or endorsed by the publisher.
Supplementary material
The Supplementary Material for this article can be found online at: https://www.frontiersin.org/articles/10.3389/fped.2022.868911/full#supplementary-material
References
1. Blencowe H, Cousens S, Oestergaard MZ, Chou D, Moller A-B, Narwal R, et al. National, regional, and worldwide estimates of preterm birth rates in the year 2010 with time trends since 1990 for selected countries: a systematic analysis and implications. Lancet (London, England). (2012) 379:2162–72. doi: 10.1016/S0140-6736(12)60820-4
2. Lineham JD, Smith RM, Dahlenburg GW, King RA, Haslam RR, Stuart MC, et al. Circulating insulin-like growth factor I levels in newborn premature and full-term infants followed longitudinally. Early Hum Dev. (1986) 13:37–46. doi: 10.1016/0378-3782(86)90096-4
3. Diaz-Gomez NM, Domenech E, Barroso F. Breast-feeding and growth factors in preterm newborn infants. J Pediatr Gastroenterol Nutr. (1997) 24:322–7. doi: 10.1097/00005176-199703000-00016
4. Hellstrom A, Ley D, Hansen-Pupp I, Hallberg B, Lofqvist C, van Marter L, et al. Insulin-like growth factor 1 has multisystem effects on foetal and preterm infant development. Acta Paediatr. (2016) 105:576–86. doi: 10.1111/apa.13350
5. Hellstrom A, Engstrom E, Hard A-L, Albertsson-Wikland K, Carlsson B, Niklasson A, et al. Postnatal serum insulin-like growth factor I deficiency is associated with retinopathy of prematurity and other complications of premature birth. Pediatrics. (2003) 112:1016–20. doi: 10.1542/peds.112.5.1016
6. Kajantie E, Dunkel L, Rutanen E-M, Seppala M, Koistinen R, Sarnesto A, et al. IGF-I, IGF binding protein (IGFBP)-3, phosphoisoforms of IGFBP-1, and postnatal growth in very low birth weight infants. J Clin Endocrinol Metab. (2002) 87:2171–9. doi: 10.1210/jcem.87.5.8457
7. Lo HC, Tsao LY, Hsu WY, Chen HN Yu WK, Chi CY. Relation of cord serum levels of growth hormone, insulin-like growth factors, insulin-like growth factor binding proteins, leptin, and interleukin-6 with birth weight, birth length, and head circumference in term and preterm neonates. Nutrition. (2002) 18:604–8. doi: 10.1016/S0899-9007(01)00811-5
8. de Jong M, Cranendonk A, Twisk JWR, van Weissenbruch MM, IGF-I. and relation to growth in infancy and early childhood in very-low-birth-weight infants and term born infants. PLoS ONE. (2017) 12:e0171650. doi: 10.1371/journal.pone.0171650
9. Beardsall K, Vanhaesebrouck S, Frystyk J, Ogilvy-Stuart AL, Vanhole C, van Weissenbruch M, et al. Relationship between insulin-like growth factor I levels, early insulin treatment, and clinical outcomes of very low birth weight infants. J Pediatr. (2014) 164:1038–44.e1. doi: 10.1016/j.jpeds.2013.12.046
10. Hansen-Pupp I, Hellstrom-Westas L, Cilio CM, Andersson S, Fellman V, Ley D. Inflammation at birth and the insulin-like growth factor system in very preterm infants. Acta Paediatr. (2007) 96:830–6. doi: 10.1111/j.1651-2227.2007.00276.x
11. Klevebro S, Hellgren G, Hansen-Pupp I, Wackernagel D, Hallberg B, Borg J, et al. Elevated levels of IL-6 and IGFBP-1 predict low serum IGF-1 levels during continuous infusion of rhIGF-1/rhIGFBP-3 in extremely preterm infants. Growth Horm IGF Res. (2020) 50:1–8. doi: 10.1016/j.ghir.2019.11.001
12. Löfqvist C, Hellgren G, Niklasson A, Engström E, Ley D, Hansen-Pupp I. Low postnatal serum IGF-I levels are associated with bronchopulmonary dysplasia (BPD). Acta Paediatr. (2012) 101:1211–6. doi: 10.1111/j.1651-2227.2012.02826.x
13. Liegl R, Löfqvist C, Hellström A, Smith LEH. IGF-1 in retinopathy of prematurity, a CNS neurovascular disease. Early Hum Dev. (2016) 102:13–9. doi: 10.1016/j.earlhumdev.2016.09.008
14. Hansen-Pupp I, Hovel H, Lofqvist C, Hellstrom-Westas L, Fellman V, Huppi PS, et al. Circulatory insulin-like growth factor-I and brain volumes in relation to neurodevelopmental outcome in very preterm infants. Pediatr Res. (2013) 74:564–9. doi: 10.1038/pr.2013.135
15. Hansen-Pupp I, Hovel H, Hellstrom A, Hellstrom-Westas L, Lofqvist C, Larsson E-M, et al. Postnatal decrease in circulating insulin-like growth factor-I and low brain volumes in very preterm infants. J Clin Endocrinol Metab. (2011) 96:1129–35. doi: 10.1210/jc.2010-2440
16. Norman M, Hallberg B, Abrahamsson T, Bjorklund LJ, Domellof M, Farooqi A, et al. Association Between Year of Birth and 1-Year Survival Among Extremely Preterm Infants in Sweden During 2004-2007 and 2014-2016. JAMA. (2019) 321:1188–99. doi: 10.1001/jama.2019.2021
17. Stoll BJ, Hansen NI, Bell EF, Shankaran S, Laptook AR, Walsh MC, et al. Neonatal outcomes of extremely preterm infants from the NICHD Neonatal Research Network. Pediatrics. (2010) 126:443–56. doi: 10.1542/peds.2009-2959
18. Ley D, Hallberg B, Hansen-Pupp I, Dani C, Ramenghi LA, Marlow N, et al. rhIGF-1/rhIGFBP-3 in Preterm Infants: a Phase 2 Randomized Controlled Trial. J Pediatr. (2019) 206:56–65.e8. doi: 10.1016/j.jpeds.2018.10.033
19. Baker J, Liu JP, Robertson EJ, Efstratiadis A. Role of insulin-like growth factors in embryonic and postnatal growth. Cell. (1993) 75:73–82. doi: 10.1016/S0092-8674(05)80085-6
20. Chiesa C, Osborn JF, Haass C, Natale F, Spinelli M, Scapillati E, et al. Ghrelin, leptin, IGF-1, IGFBP-3, and insulin concentrations at birth: is there a relationship with fetal growth and neonatal anthropometry? Clin Chem. (2008) 54:550–8. doi: 10.1373/clinchem.2007.095299
21. Akcakus M, Koklu E, Kurtoglu S, Kula M, Koklu SS. The relationship among intrauterine growth, insulinlike growth factor I (IGF-I), IGF-binding protein-3, and bone mineral status in newborn infants. Am J Perinatol. (2006) 23:473–80. doi: 10.1055/s-2006-954822
22. Langford K, Nicolaides K, Miell JP. Maternal and fetal insulin-like growth factors and their binding proteins in the second and third trimesters of human pregnancy. Hum Reprod. (1998) 13:1389–93. doi: 10.1093/humrep/13.5.1389
23. Lassarre C, Hardouin S, Daffos F, Forestier F, Frankenne F, Binoux M. Serum insulin-like growth factors and insulin-like growth factor binding proteins in the human fetus. Relationships with growth in normal subjects and in subjects with intrauterine growth retardation. Pediatr Res. (1991) 29:219–25. doi: 10.1203/00006450-199103000-00001
24. Iniguez G, Ong K, Bazaes R, Avila A, Salazar T, Dunger D, et al. Longitudinal changes in insulin-like growth factor-I, insulin sensitivity, and secretion from birth to age three years in small-for-gestational-age children. J Clin Endocrinol Metab. (2006) 91:4645–9. doi: 10.1210/jc.2006-0844
25. Spencer GS, Hill DJ, Garssen GJ, Macdonald AA, Colenbrander B. Somatomedin activity and growth hormone levels in body fluids of the fetal pig: effect of chronic hyperinsulinaemia. J Endocrinol. (1983) 96:107–14. doi: 10.1677/joe.0.0960107
26. Rajaram S, Baylink DJ, Mohan S. Insulin-like growth factor-binding proteins in serum and other biological fluids: regulation and functions. Endocr Rev. (1997) 18:801–31. doi: 10.1210/edrv.18.6.0321
27. D'Ercole AJ, Hill DJ, Strain AJ, Underwood LE. Tissue and plasma somatomedin-C/insulin-like growth factor I concentrations in the human fetus during the first half of gestation. Pediatr Res. (1986) 20:253–5. doi: 10.1203/00006450-198603000-00011
28. Han VK, D'Ercole AJ, Lund PK. Cellular localization of somatomedin (insulin-like growth factor) messenger RNA in the human fetus. Science. (1987) 236:193–7. doi: 10.1126/science.3563497
29. Suikkari AM. Insulin-like growth factor (IGF-I) and its low molecular weight binding protein in human milk. Eur J Obstet Gynecol Reprod Biol. (1989) 30:19–25. doi: 10.1016/0028-2243(89)90089-0
30. Baxter RC, Zaltsman Z, Turtle JR. Immunoreactive somatomedin-C/insulin-like growth factor I and its binding protein in human milk. J Clin Endocrinol Metab. (1984) 58:955–9. doi: 10.1210/jcem-58-6-955
31. Read LC, Upton FM, Francis GL, Wallace JC, Dahlenberg GW, Ballard FJ. Changes in the growth-promoting activity of human milk during lactation. Pediatr Res. (1984) 18:133–9. doi: 10.1203/00006450-198402000-00004
32. Bjornvad CR, Thymann T, Deutz NE, Burrin DG, Jensen SK, Jensen BB, et al. Enteral feeding induces diet-dependent mucosal dysfunction, bacterial proliferation, and necrotizing enterocolitis in preterm pigs on parenteral nutrition. Am J Physiol Gastrointest Liver Physiol. (2008) 295:G1092–103. doi: 10.1152/ajpgi.00414.2007
33. Stoy ACF, Heegaard PMH, Thymann T, Bjerre M, Skovgaard K, Boye M, et al. Bovine colostrum improves intestinal function following formula-induced gut inflammation in preterm pigs. Clin Nutr. (2014) 33:322–9. doi: 10.1016/j.clnu.2013.05.013
34. Sangild PT, Siggers RH, Schmidt M, Elnif J, Bjornvad CR, Thymann T, et al. Diet- and colonization-dependent intestinal dysfunction predisposes to necrotizing enterocolitis in preterm pigs. Gastroenterology. (2006) 130:1776–92. doi: 10.1053/j.gastro.2006.02.026
35. Burrin DG, Wester TJ, Davis TA, Amick S, Heath JP. Orally administered IGF-I increases intestinal mucosal growth in formula-fed neonatal pigs. Am J Physiol. (1996) 270:R1085–91. doi: 10.1152/ajpregu.1996.270.5.R1085
36. Houle VM, Schroeder EA, Odle J, Donovan SM. Small intestinal disaccharidase activity and ileal villus height are increased in piglets consuming formula containing recombinant human insulin-like growth factor-I. Pediatr Res. (1997) 42:78–86. doi: 10.1203/00006450-199707000-00013
37. Alexander AN, Carey H V. Oral IGF-I enhances nutrient and electrolyte absorption in neonatal piglet intestine. Am J Physiol. (1999) 277:G619–25. doi: 10.1152/ajpgi.1999.277.3.G619
38. Jeschke MG, Bolder U, Chung DH, Przkora R, Mueller U, Thompson JC, et al. Gut mucosal homeostasis and cellular mediators after severe thermal trauma and the effect of insulin-like growth factor-I in combination with insulin-like growth factor binding protein-3. Endocrinology. (2007) 148:354–62. doi: 10.1210/en.2006-0883
39. Ozen S, Akisu M, Baka M, Yalaz M, Sozmen EY, Berdeli A, et al. Insulin-like growth factor attenuates apoptosis and mucosal damage in hypoxia/reoxygenation-induced intestinal injury. Biol Neonate. (2005) 87:91–6. doi: 10.1159/000081897
40. Hunninghake GW, Doerschug KC, Nymon AB, Schmidt GA, Meyerholz DK, Ashare A. Insulin-like growth factor-1 levels contribute to the development of bacterial translocation in sepsis. Am J Respir Crit Care Med. (2010) 182:517–25. doi: 10.1164/rccm.200911-1757OC
41. Andersen AD, Sangild PT, Munch SL, van der Beek EM, Renes IB, van Ginneken C, et al. Delayed growth, motor function and learning in preterm pigs during early postnatal life. Am J Physiol - Regul Integr Comp Physiol. (2016) 310:R481–92. doi: 10.1152/ajpregu.00349.2015
42. Holgersen K, Gao X, Narayanan R, Gaur T, Carey G, Barton N, et al. Supplemental Insulin-like growth factor-1 and necrotizing enterocolitis in preterm pigs. Front Pediatr. (2021) 8:602047. doi: 10.3389/fped.2020.602047
43. Nguyen DN, Jiang P, Frokiaer H, Heegaard PMH, Thymann T, Sangild PT. Delayed development of systemic immunity in preterm pigs as a model for preterm infants. Sci Rep. (2016) 6:36816. doi: 10.1038/srep36816
44. Ren S, Hui Y, Obelitz-Ryom K, Brandt AB, Kot W, Nielsen DS, et al. Neonatal gut and immune maturation is determined more by postnatal age than by postconceptional age in moderately preterm pigs. Am J Physiol Gastrointest Liver Physiol. (2018) 315:G855–67. doi: 10.1152/ajpgi.00169.2018
45. Cheng CM, Reinhardt RR, Lee WH, Joncas G, Patel SC, Bondy CA. Insulin-like growth factor 1 regulates developing brain glucose metabolism. Proc Natl Acad Sci U S A. (2000) 97:10236–41. doi: 10.1073/pnas.170008497
46. Liu JL, Fernandez AM. Wu Y, Schally A V, Frystyk J, et al. Liver-specific igf-1 gene deletion leads to muscle insulin insensitivity. Diabetes. (2001) 50:1110–8. doi: 10.2337/diabetes.50.5.1110
47. Sjogren K, Wallenius K, Liu JL. Bohlooly-Y M, Pacini G, Svensson L, et al. Liver-derived IGF-I is of importance for normal carbohydrate and lipid metabolism. Diabetes. (2001) 50:1539–45. doi: 10.2337/diabetes.50.7.1539
48. Sandhu MS, Heald AH, Gibson JM, Cruickshank JK, Dunger DB, Wareham NJ. Circulating concentrations of insulin-like growth factor-I and development of glucose intolerance: a prospective observational study. Lancet (London, England). (2002) 359:1740–5. doi: 10.1016/S0140-6736(02)08655-5
49. Carroll P V, Christ ER, Umpleby AM, Gowrie I, Jackson N, Bowes SB, et al. treatment in adults with type 1 diabetes: effects on glucose and protein metabolism in the fasting state and during a hyperinsulinemic-euglycemic amino acid clamp. Diabetes. (2000) 49:789–96. doi: 10.2337/diabetes.49.5.789
50. Crowne EC, Samra JS, Cheetham T, Acerini CL, Watts A, Holly JM, et al. The role of IGF-binding proteins in mediating the effects of recombinant human IGF-I on insulin requirements in type 1 diabetes mellitus. J Clin Endocrinol Metab. (2001) 86:3686–91,. doi: 10.1210/jcem.86.8.7722
51. Quattrin T, Thrailkill K, Baker L, Litton J, Dwigun K, Rearson M, et al. Dual hormonal replacement with insulin and recombinant human insulin-like growth factor I in IDDM. Effects on glycemic control, IGF-I levels, and safety profile. Diabetes Care. (1997) 20:374–80. doi: 10.2337/diacare.20.3.374
52. Duvanel CB, Fawer CL, Cotting J, Hohlfeld P, Matthieu JM. Long-term effects of neonatal hypoglycemia on brain growth and psychomotor development in small-for-gestational-age preterm infants. J Pediatr. (1999) 134:492–8. doi: 10.1016/S0022-3476(99)70209-X
53. Lucas A, Morley R, Cole TJ. Adverse neurodevelopmental outcome of moderate neonatal hypoglycaemia. BMJ. (1988) 297:1304–8. doi: 10.1136/bmj.297.6659.1304
54. Mitanchez D. Glucose regulation in preterm newborn infants. Horm Res. (2007) 68:265–71. doi: 10.1159/000104174
55. Zamir I, Tornevi A, Abrahamsson T, Ahlsson F, Engstrom E, Hallberg B, et al. Hyperglycemia in extremely preterm infants-insulin treatment, mortality and nutrient intakes. J Pediatr. (2018) 200:104–10.e1. doi: 10.1016/j.jpeds.2018.03.049
56. van der Lugt NM, Smits-Wintjens VEHJ, van Zwieten PHT, Walther FJ. Short and long term outcome of neonatal hyperglycemia in very preterm infants: a retrospective follow-up study. BMC Pediatr. (2010) 10:52. doi: 10.1186/1471-2431-10-52
57. van den Berghe G, Wouters P, Weekers F, Verwaest C, Bruyninckx F, Schetz M, et al. Intensive insulin therapy in critically ill patients. N Engl J Med. (2001) 345:1359–67. doi: 10.1056/NEJMoa011300
58. Beardsall K, Vanhaesebrouck S, Ogilvy-Stuart AL, Vanhole C, Palmer CR, van Weissenbruch M, et al. Early insulin therapy in very-low-birth-weight infants. N Engl J Med. (2008) 359:1873–84. doi: 10.1056/NEJMoa0803725
59. Percie du Sert N, Hurst V, Ahluwalia A, Alam S, Avey MT, Baker M, et al. The ARRIVE guidelines 2.0: updated guidelines for reporting animal research. PLoS BIOL. (2020) 18:e3000410. doi: 10.1371/journal.pbio.3000410
60. Cilieborg MS, Boye M, Thymann T, Jensen BB, Sangild PT. Diet-dependent effects of minimal enteral nutrition on intestinal function and necrotizing enterocolitis in preterm pigs. JPEN J Parenter Enteral Nutr. (2011) 35:32–42. doi: 10.1177/0148607110377206
61. Rudar M, Naberhuis JK, Suryawan A, Nguyen H V, Stoll B, Style CC, et al. Intermittent bolus feeding does not enhance protein synthesis, myonuclear accretion, or lean growth more than continuous feeding in a premature piglet model. Am J Physiol Endocrinol Metab. (2021) 321:E737–52. doi: 10.1152/ajpendo.00236.2021
62. Jensen AR, Elnif J, Burrin DG, Sangild PT. Development of intestinal immunoglobulin absorption and enzyme activities in neonatal pigs is diet dependent. J Nutr. (2001) 131:3259–65. doi: 10.1093/jn/131.12.3259
63. Holme Nielsen C, Bladt Brandt A, Thymann T, Obelitz-Ryom K, Jiang P, Vanden Hole C, et al. Rapid postnatal adaptation of neurodevelopment in pigs born late preterm. Dev Neurosci. (2018) 40:586–600. doi: 10.1159/000499127
64. Bjornvad CR, Schmidt M, Petersen YM, Jensen SK, Offenberg H, Elnif J, et al. Preterm birth makes the immature intestine sensitive to feeding-induced intestinal atrophy. Am J Physiol Regul Integr Comp Physiol. (2005) 289:R1212–22. doi: 10.1152/ajpregu.00776.2004
65. Fholenhag K, Arrhenius-Nyberg V, Sjogren I, Malmlof K. Effects of insulin-like growth factor I (IGF-I) on the small intestine: a comparison between oral and subcutaneous administration in the weaned rat. Growth Factors. (1997) 14:81–8. doi: 10.3109/08977199709021512
66. Kim WK Ryu YH, Seo DS, Lee CY, Ko Y. Effects of oral administration of insulin-like growth factor-I on circulating concentration of insulin-like growth factor-I and growth of internal organs in weanling mice. Biol Neonate. (2006) 89:199–204. doi: 10.1159/000089796
67. Houle VM, Park YK, Laswell SC, Freund GG, Dudley MA, Donovan SM. Investigation of three doses of oral insulin-like growth factor-I on jejunal lactase phlorizin hydrolase activity and gene expression and enterocyte proliferation and migration in piglets. Pediatr Res. (2000) 48:497–503. doi: 10.1203/00006450-200010000-00013
68. Tian F, Liu G-R, Li N, Yuan G. Insulin-like growth factor I reduces the occurrence of necrotizing enterocolitis by reducing inflammatory response and protecting intestinal mucosal barrier in neonatal rats model. Eur Rev Med Pharmacol Sci. (2017) 21:4711–9.
69. Ney DM, Huss DJ, Gillingham MB, Kritsch KR, Dahly EM, Talamantez JL, et al. Investigation of insulin-like growth factor (IGF)-I and insulin receptor binding and expression in jejunum of parenterally fed rats treated with IGF-I or growth hormone. Endocrinology. (1999) 140:4850–60. doi: 10.1210/endo.140.10.7029
70. Bortvedt SF, Lund PK. Insulin-like growth factor 1: common mediator of multiple enterotrophic hormones and growth factors. Curr Opin Gastroenterol. (2012) 28:89–98. doi: 10.1097/MOG.0b013e32835004c6
71. Sun J, Li Y, Nguyen DN, Mortensen MS, van den Akker CHP, Skeath T, et al. Nutrient fortification of human donor milk affects intestinal function and protein metabolism in preterm pigs. J Nutr. (2018) 148:336–47. doi: 10.1093/jn/nxx033
72. Li Y, Pan X, Nguyen DN, Ren S, Moodley A, Sangild PT. Bovine colostrum before or after formula feeding improves systemic immune protection and gut function in newborn preterm pigs. Front Immunol. (2019) 10:3062. doi: 10.3389/fimmu.2019.03062
73. Yu K, Canalias F, Solà-Oriol D, Arroyo L, Pato R, Saco Y, et al. Age-related serum biochemical reference intervals established for unweaned calves and piglets in the post-weaning period. Front Vet Sci. (2019) 6:123. doi: 10.3389/fvets.2019.00123
74. Desbois-Mouthon C, Wendum D, Cadoret A, Rey C, Leneuve P, Blaise A, et al. Hepatocyte proliferation during liver regeneration is impaired in mice with liver-specific IGF-1R knockout. FASEB J. (2006) 20:773–5. doi: 10.1096/fj.05-4704fje
75. Kamenický P, Mazziotti G, Lombès M, Giustina A, Chanson P. Growth hormone, insulin-like growth factor-1, and the kidney: pathophysiological and clinical implications. Endocr Rev. (2014) 35:234–81. doi: 10.1210/er.2013-1071
76. Naberhuis JK, Suryawan A, Nguyen HV, Hernandez-Garcia A, Cruz SM, Lau PE, et al. Prematurity blunts the feeding-induced stimulation of translation initiation signaling and protein synthesis in muscle of neonatal piglets. Am J Physiol Endocrinol Metab. (2019) 317:E839–51. doi: 10.1152/ajpendo.00151.2019
77. Rudar M, Naberhuis JK, Suryawan A, Nguyen HV, Stoll B, Style CC, et al. Prematurity blunts the insulin- and amino acid-induced stimulation of translation initiation and protein synthesis in skeletal muscle of neonatal pigs. Am J Physiol Endocrinol Metab. (2021) 320:E551–65. doi: 10.1152/ajpendo.00203.2020
78. Stoll B, Puiman PJ, Cui L, Chang X, Benight NM, Bauchart-Thevret C, et al. Continuous parenteral and enteral nutrition induces metabolic dysfunction in neonatal pigs. JPEN J Parenter Enteral Nutr. (2012) 36:538–50. doi: 10.1177/0148607112444756
79. Stoll B, Horst DA, Cui L, Chang X, Ellis KJ, Hadsell DL, et al. Chronic parenteral nutrition induces hepatic inflammation, steatosis, and insulin resistance in neonatal pigs. J Nutr. (2010) 140:2193–200. doi: 10.3945/jn.110.125799
80. Van den Berghe G. How does blood glucose control with insulin save lives in intensive care? J Clin Invest. (2004) 114:1187–95. doi: 10.1172/JCI23506
81. Tissieres P, Ochoda A, Dunn-Siegrist I, Drifte G, Morales M, Pfister R, et al. Innate immune deficiency of extremely premature neonates can be reversed by interferon-gamma. PLoS ONE. (2012) 7:e32863. doi: 10.1371/journal.pone.0032863
82. Shen C-M, Lin S-C, Niu D-M, Kou YR. Development of monocyte Toll-like receptor 2 and Toll-like receptor 4 in preterm newborns during the first few months of life. Pediatr Res. (2013) 73:685–91. doi: 10.1038/pr.2013.36
83. Stoll BJ, Hansen NI, Bell EF, Walsh MC, Carlo WA, Shankaran S, et al. Trends in care practices, morbidity, and mortality of extremely preterm neonates, 1993-2012. JAMA. (2015) 314:1039–51. doi: 10.1001/jama.2015.10244
84. Stoll BJ, Hansen N, Fanaroff AA, Wright LL, Carlo WA, Ehrenkranz RA, et al. Late-onset sepsis in very low birth weight neonates: the experience of the NICHD neonatal research network. Pediatrics. (2002) 110:285–91. doi: 10.1542/peds.110.2.285
85. Bæk O, Brunse A, Nguyen DN, Moodley A, Thymann T, Sangild PT. Diet modulates the high sensitivity to systemic infection in newborn preterm pigs. Front Immunol. (2020) 11:1–14. doi: 10.3389/fimmu.2020.01019
86. Law HKW, Tu W, Liu E, Lau YL. Insulin-like growth factor I promotes cord blood T cell maturation through monocytes and inhibits their apoptosis in part through interleukin-6. BMC Immunol. (2008) 9:74. doi: 10.1186/1471-2172-9-74
87. Tu W, Zhang DK, Cheung PT, Tsao SW, Lau YL. Effect of insulin-like growth factor 1 on PHA-stimulated cord blood mononuclear cell telomerase activity. Br J Haematol. (1999) 104:785–94. doi: 10.1046/j.1365-2141.1999.01272.x
88. Clark R, Strasser J, McCabe S, Robbins K, Jardieu P. Insulin-like growth factor-1 stimulation of lymphopoiesis. J Clin Invest. (1993) 92:540–8. doi: 10.1172/JCI116621
89. Puzik A, Rupp J, Troger B, Gopel W, Herting E, Hartel C. Insulin-like growth factor-I regulates the neonatal immune response in infection and maturation by suppression of IFN-gamma. Cytokine. (2012) 60:369–76. doi: 10.1016/j.cyto.2012.07.025
Keywords: insulin-like growth factor-1, preterm, pig, gut, development
Citation: Holgersen K, Rasmussen MB, Carey G, Burrin DG, Thymann T and Sangild PT (2022) Clinical outcome and gut development after insulin-like growth factor-1 supplementation to preterm pigs. Front. Pediatr. 10:868911. doi: 10.3389/fped.2022.868911
Received: 03 February 2022; Accepted: 08 July 2022;
Published: 05 August 2022.
Edited by:
Maria Elisabetta Baldassarre, University of Bari Aldo Moro, ItalyReviewed by:
Terri Marin, Augusta University, United StatesBrian Scottoline, Oregon Health and Science University, United States
Elizabeth Maga, University of California, Davis, United States
Copyright © 2022 Holgersen, Rasmussen, Carey, Burrin, Thymann and Sangild. This is an open-access article distributed under the terms of the Creative Commons Attribution License (CC BY). The use, distribution or reproduction in other forums is permitted, provided the original author(s) and the copyright owner(s) are credited and that the original publication in this journal is cited, in accordance with accepted academic practice. No use, distribution or reproduction is permitted which does not comply with these terms.
*Correspondence: Per Torp Sangild, pts@sund.ku.dk