- 1Biosensing Laboratory, Department of Electrical Engineering, University of Hawaii at Manoa, Honolulu, HI, United States
- 2Department of Electrical and Electronic Engineering, University of Dhaka, Dhaka, Bangladesh
During this COVID-19 pandemic time, an unprecedented number of patients with severe respiratory illness require intensive care units (ICUs) under mechanical ventilation (MV) for sustaining life. Patient–ventilator asynchrony (PVA) is very common, and it occurs due to the mismatch between the normal variability of the patients’ breathing patterns and ventilator parameters. Asynchronies during invasive ventilation are causing the patients discomfort, fatigue, anxiety, neurovascular nerve damage, and mortality. However, currently, the only way to detect the asynchrony is through visual inspections by the healthcare professionals and adjust manually. In this article, we propose an opinion on the conceptual framework of a system composed of radio frequency (RF)-based noncontact life-sensing technology that can extract different respiratory features unobtrusively and continuously and can reduce the patient–ventilator asynchrony. After extracting respiratory features of patients from the radar data, it can provide optimally and continuously supplemental oxygen by adjusting the function of the existing mechanical ventilator. This will reduce the sufferings and mortalities, as well as less stress for emergency nurses and doctors to handle patients more effectively.
Introduction
Coronavirus disease is now a global pandemic disease, caused by a virus called SARS-CoV-2 (CDC, 2020). This new deadly contagious respiratory disease was first detected in Wuhan, China, and reported to the world health organization (WHO) on December 31st, 2019 (WHO, 2019). Since then, at the time of writing, 60 million people have been infected all over the world, and 12 million people have been infected in the United States (Johns Hopkins University, 2020). The world is experiencing unprecedented challenges due to COVID-19. This deadly virus is affecting people in different ways (CDC, 2020). Infected people have a wide range of symptoms like fever, cough, and shortness of breathing (CDC, 2020). From the various clinical studies, it has been proven that most of the people who are infected by COVID-19 experience mild to moderate respiratory illness and, in some cases, severe respiratory illness (Singhal, 2020). From previously reported results and recent pandemic experiences, it is evident that severe acute respiratory syndrome (SARS)-related viruses generally affect the lung at a level of critical condition (Singhal, 2020). In critical conditions, patients require hospitalization and, in some cases, visit an intensive care unit (ICU) with invasive mechanical ventilation (IMV) for receiving supplemental oxygen (Perez-Nieto et al., 2020). Asynchronies in between critically ill patients’ respiratory parameters and IMV are very common and reported in prior research for acute respiratory distress syndrome (ARDS) related diseases (Blanch et al., 2015). Additionally, a study reported that there were 5,700 patients in NY, United States with COVID-19 who needed intubation and IMV which have presented a challenge for the physicians and have been associated with a mortality rate of 24.5% (Richardson et al., 2020). The current mechanical ventilator (MV) lacks the synchronization and timing of the breathing pattern and parameters of patients for not having an integrated continuous respiratory monitoring system shown in Figure 1A. Although, to date, there has been no discussion about the prevalence of asynchronies in patients with ARDS during IMV and no potential technological solutions have been presented (Perez-Nieto et al., 2020).
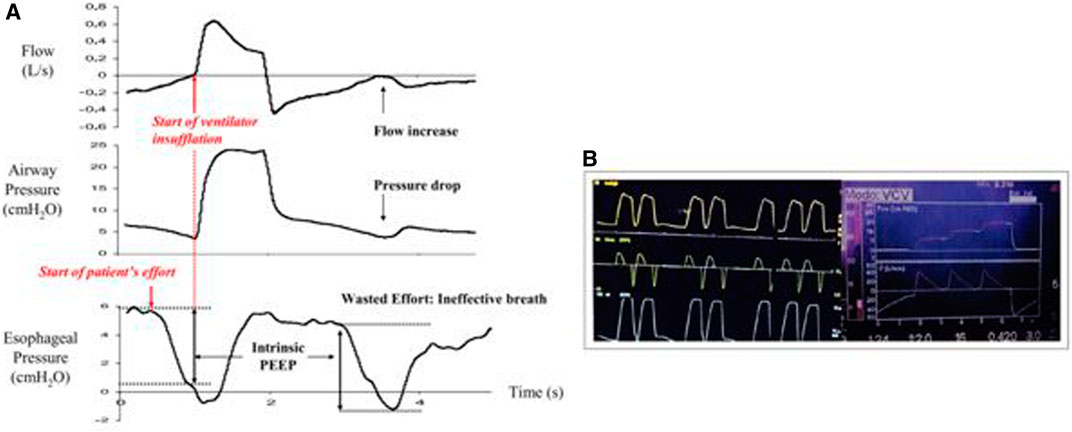
FIGURE 1. (A) Flow, airway pressure, and esophageal pressure signal which demonstrates the intrinsic positive end expiratory pressure (PEEP), which is defined as the pressure drop required before triggering the ventilator. Ineffective triggering is occurring due to the mismatch between the airflow profile of the patients and ventilator (from Gilstrap and MacIntyre, 2013). (B) Asynchronies shown for critically ill COVID-19 patients. Left picture illustrates the double triggering, and the right picture shows triple triggering in the mechanical ventilator display. Here, VCV = volume cycled ventilation, Paw = pressure airway, V.C = volume control, VCV = volume cycled ventilation (taken From (Perez-Nieto et al., 2020).
In critically COVID-19 ill patients, proper interaction with the mechanical ventilator is very important throughout ventilatory support (Blanch et al., 2015). Poor patient–ventilator interaction generally causes discomfort, extended ICU length of stay, prolonged mechanical ventilation, respiratory muscle injury, and mortality (Gilstrap and MacIntyre, 2013). At present, for assessing PVA, generally, healthcare professionals (e.g., nurses) apply their knowledge of respiratory physiology to interpret patients’ physical signs based on airflow and airway pressure waveforms (Blanch et al., 2015). The extreme situation in patients who have complications with the ventilator due to respiratory pattern mismatch is easily detectable and adjustable (Blanch et al., 2015). However, the less evident situation is hard to detect and adjust, which may cause different complications (Blanch et al., 2015). Due to the contagiousness of this disease, healthcare professionals need to take extra protection for these visual inspections to adjust ventilator parameters. Moreover, in this outbreak, the overwhelming condition of fatigue and burnout of the hospital healthcare professionals makes it hard to adjust the parameters of the mechanical ventilator continuously. Human respiratory pattern (inhale, exhale, and tidal volume) and airflow profile are controlled by a central neural mechanism which is a collection of neurons located in the brainstem and also changes with physiological activities, emotional stresses, and disease-related trauma (Benchetrit, 2000). The ability of mechanical ventilatory support to provide adequate gas exchange is essential, and otherwise, it can create pronounced dyspnea (Benchetrit, 2000). Thus, the proposed noncontact and continuous respiratory monitoring system will extract breathing features and feed them to the existing ventilator for optimal interaction between patient and MV, which will have a significant medical impact as listed below:
Length of stay at the hospital would be minimized
Mortality and morbidity rate would be less
Stresses of the healthcare professionals would be reduced
Efficient utilization of timing of the medical professionals
At present, there are two ways of monitoring respiratory patterns continuously and in a noncontact manner: vision-based (Tan et al., 2010) and a radio frequency (RF)-based technology (Islam et al., 2020a). Radar remote respiratory sensing technology is gaining attention and much more popular than camera-based technology because it is less invasive of one’s privacy, less physically obtrusive, and requires less amount of data for processing (Massaroni et al., 2020; Taylor et al., 2020). This article provides an in-depth discussion of how radar-based noncontact life sensing technology can help to reduce the patient–ventilator asynchronies to optimally supply supplemental oxygen to COVID-19 patients. The core contribution of this work is to demonstrate the feasibility of noncontact radar-based remote life sensing technology to minimize the mismatch of the respiratory parameters between patients and MV, and also disseminate the idea of this potential technological solution to wide audiences. Prior reported research focused on discussing the state of the art of noncontact sensing technique without elaborating this potential scope to fight against COVID-19 (Massaroni et al., 2020; Taylor et al., 2020). To the best of the author’s knowledge, this is the first reported opinion article on utilizing radar remote respiration sensing technology to reduce the life-threatening asynchronies of the mechanical ventilator.
The rest of the article is organized as follows: Impact of PVA due to COVID-19 section discusses the impact of PVA due to COVID-19, whereas section-III is focused on the discussion of the proposed technological solution. Discussion section finally concludes this article.
Impact of PVA due to COVID-19
PVA is a mismatch regarding inspiratory time, expiratory time, airflow profile, tidal volume, or pressure demands between the patient and the mechanical ventilator, which supplies oxygen during ventilation (Holanda et al., 2018). PVA is related to triggering, cycling, and airflow amount. Triggering problems occur due to a mismatch of the initiation of the respiratory cycle by the ventilator in response to patients’ respiratory efforts (Holanda et al., 2018). Triggering asynchronies of MV consist of ineffective triggering, auto triggering, and double effort (Holanda et al., 2018). Additionally, airflow asynchrony can be divided into two types: insufficient inspiratory flow and excessive inspiratory flow which occurs due to the mismatch of the tidal volume of the patient’s demand.
Recently, a reported study was conducted on nine patients who experienced severe breathing problems and were diagnosed with ARDS for COVID-19 and mechanical ventilation in Mexico (Perez-Nieto et al., 2020). Among them, six patients were men, with an average age of 52 years (47–55 years), and all of them were ventilated by assisted volume-controlled mode, with a tidal volume (VT) of 6 ml/kg of predicted weight. Four patients died, in which double and triple triggering were predominant during their ventilation, as shown in Figure 1B. Moreover, the average 1-min asynchrony rate was around 17% for patients who died in contrast to 2.4% for patients who survived (Perez-Nieto et al., 2020). In a previous investigation (Blanch et al., 2015), they reported that if the 1-min average asynchrony rate is greater than 10% (number of asynchronies among the total respiratory cycles multiplied by 100), then the chance of mortality is higher for ARDS patients. Additionally, the study reported by P. Nieto et al. also demonstrated that double and triple triggering caused diffuse alveolar damage, scaling of pneumocytes, and hyaline membrane formations in dying patients’ lungs. Therefore, the air volume (6 ml/kg) injected by the MV could not satisfy their “air hunger,” generating an excessive inspiratory effort which creates volutrauma, barotrauma, and neuromuscular damage that may be a potential cause of death (Perez-Nieto et al., 2020). Moreover, due to the overwhelming condition at the hospital during this pandemic, most of the physicians are fatigued and burned out. However, these types of critical care patients with COVID-19 under IMV need continuous respiratory monitoring and proper adjustment of the ventilatory parameters.
Proposed Technological Solutions to Reduce PVA
Remote respiration sensing using microwave Doppler radar has shown great promises in healthcare applications, with the proof of concepts demonstrated for various applications (Li et al., 2013). In a typical Doppler radar system, a transmitter emits an RF signal, and its receiver receives a motion-modulated reflection of the target (Massagram et al., 2013). Due to the radial surface velocity of the target, the reflected signal frequency is shifted, which is proportional to the velocity of the target. More specifically, periodically moving targets such as the torso wall of a breathing person are characterized as a phase change proportional to the tiny movement of the chest surface due to cardio-respiratory activities. If the target motion is small (∼chest displacement 12 mm) compared to the transmitted radio wavelength, then a radio receiver circuit that couples both the transmitted and reflected waves to a mixer can produce a baseband signal with a low-frequency component that carries respiratory signatures (breathing rate and heart rate). The efficacy of the RF-based noncontact breathing monitoring system has been proved in the literature and is also available as a product in the market, which shows medical-grade accuracy (error <1 breath/min) when the patient is stationary (Massaroni et al., 2020). In one of the most recent attempts, Massaroni argued that remote breathing monitoring using radar technology would be an important contribution to facing the current COVID-19 crisis (Massaroni et al., 2020). Apart from breathing monitoring, radar can also track different important respiratory features such as inhale starting time, exhale starting time, tidal volume, inhale/exhale rate, and inhale/exhale area ratio which can help to synchronize the ventilator parameters (Islam et al., 2020b). From these remote respiratory feature extractions, we can store those respiratory features into an FPGA/microcontroller-based feedback system which will be connected with the ventilators to supply optimal oxygen synchronously to patients need—by continuously adjusting the ventilator variables. Figure 2 illustrates the proposed idea in a block diagram.
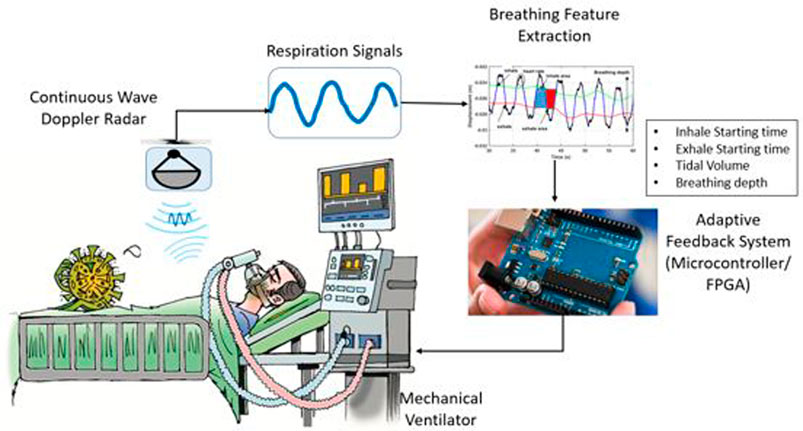
FIGURE 2. Proposed technological solution that can be used for reducing the patient ventilator asynchrony. Radar can capture the respiratory signatures of a person remotely without attaching any sensor to the body, and different important features can be extracted and fed as an adaptive feedback system for optimal interaction of patients with ventilators.
Medical studies have demonstrated that there is a linear relationship between lung volume and chest wall displacement (Massagram et al., 2013). Thus, based on the linear relationship between chest wall displacement and air volume changes, the Doppler radar system is capable of tracking the tiny movement of the chest surface so it can also extract the lung volume and airflow parameters (Masaagram et al., 2013). Generally, taking the fast Fourier transform (FFT) of the radar captured signal provides information on the breathing rate and heart rate (Islam et al., 2020b). Additionally, from the peak search process, breathing depth can be calculated by considering the total displacement from a minimum peak to a maximum peak (Islam et al., 2020b). Inhale/exhale speed, along with starting and ending time, can also be calculated from the information of the peak positions and their time indexes of the radar-captured respiration signal. Moreover, the tidal volume can also be measured from the peak search process of the radar-captured signal, and prior research also demonstrates the good correlation of the radar VT measurement with the VT from the spirometer (Massagram et al., 2013). Prior research also demonstrated the dynamic segmentation technique which segments a 1-min breathing cycle episode with 30–70% amplitude and calculates the average area ratio of the inhale and exhale segments (Islam et al., 2020b). An initial study on variability of sedentary radar measured respiratory pattern can be found in our prior attempt as a foundation of this proposed research (Supplementary Material (Islam et al 2020b)). This breath ratio feature illustrates how one initiates the next cycle of breathing pattern and helps to extract the airflow profile of a human. Previous research also showed the efficacy of identification of people based on radar-captured breathing ratios and airflow profile (Islam et al., 2020c). Prior research also demonstrated the successful isolation of noise and interferences to extract reliable vital sign information utilizing signal processing techniques such as independent component analysis (Islam et al., 2018) and adaptive filtering (Islam et al., 2021). There are several advantages to the conceptual framework of the proposed system. Continuous monitoring will be possible without attaching any sensor to the human chest surfaces. Optimal interaction between the patient and ventilator can be maintained by adjusting the respiratory parameters from radar measurement. The novelty of the proposed noncontact technique is the extraction of different respiratory features (inhale/exhale starting time, rate, and tidal volume) continuously and automatically adjusting different respiratory patterns and parameters for synchronization and optimized delivery of supplemental oxygen to patients by converting the existing MV to patient-centric smart MV.
The proposed system described above is just a conceptual framework where the efficacy of the radar remote life sensing technology has been demonstrated in previously reported research (Massagram et al., 2013; Islam et al., 2020b). After extracting features from radar, a feedback system will need to be integrated with the existing MV, and comparative analysis of the performances remains our future work to explore. As the coronavirus pandemic (COVID-19) is increasing rapidly, a huge number of critically ill patients require mechanical ventilation and are facing the challenges of asynchronies of the ventilator. Technological applications and initiatives should be multiplied to bring this proposed system into the real world for reducing the distress of humanity.
Discussion
Noncontact radar-based life sensing technology is expected to play an important role in the context of COVID-19 (Massaroni et al., 2020). It has the potential to facilitate vital sign sensing assistance for self-isolated COVID-19 patients as well as critically ill patients who are under mechanical ventilation. The improvement and development of the proposed system have the potential to reduce the suffering of the patients under mechanical ventilation for sustaining their valuable lives. Automatic detection of PVA remains a critical challenge in medical technology. Adjustment of the ventilator parameters by visual inspection is difficult, stressful, and time consuming during this pandemic situation; it is also hard to manage an unprecedentedly large number of critically ill patients. The proposed conceptual framework should be brought into the real world quickly to reduce the mortality and morbidity rate of patients, mitigate the burden on hospitals, and decrease the risk of infection for healthcare professionals. Noncontact, automatic, continuous, and remote respiratory parameters monitoring and adjusting the ventilator appropriately would be an important contribution to mitigating the current COVID-19 crisis.
Data Availability Statement
The original contributions presented in the study are included in the article/Supplementary Material, and further inquiries can be directed to the corresponding author.
Author Contributions
MK and SI contributed to the conception and design of the work. SI and MK contributed to the manuscript writing, critical revision of the article, and approval for the final version of the article.
Conflict of Interest
The authors declare that the research was conducted in the absence of any commercial or financial relationships that could be construed as a potential conflict of interest.
Supplementary Material
The Supplementary Material for this article can be found online at: https://www.frontiersin.org/articles/10.3389/frcmn.2020.636006/full#supplementary-material.
References
Benchetrit, G. (2000). Breathing pattern in humans: diversity and individuality. Respir. Physiol. 122 (3), 123–129. doi:10.1016/S0034-5687(00)00154-7
Blanch, L., Villagra, A., Sales, B., Montanya, J., Lucangelo, U., Luján, M., et al. (2015). Asynchronies during mechanical ventilation are associated with mortality. Intensive Care Med. 41 (4), 633–641. doi:10.1007/s00134-015-3692-6
CDC (2020). Symptoms at coronavirus. Available at: https://www.cdc.gov/coronavirus/2019-ncov/symptoms-testing/symptoms.html (Accessed Nov 20, 2020).
Gilstrap, D., and MacIntyre, N. (2013). Patient-ventilator interactions. Implications for clinical management. Am. J. Respir. Crit. Care Med. 188 (9), 1058–1068. doi:10.1164/rccm.201212-2214CI
Holanda, M. A., Vasconcelos, R. D. S., Ferreira, J. C., and Pinheiro, B. V. 2018). Patient-ventilator asynchrony. J. Bras. Pneumol. 44 (4), 321–333. doi:10.1590/S1806-37562017000000185
Islam, S. M. M., Boric-Lubecke, O., and Lubekce, V. M. (2020a). Concurrent respiration monitoring of multiple subjects by phase-comparison monopulse radar using independent component analysis (ICA) with JADE algorithm and direction of arrival (DOA). IEEE Access 8, 73558–73569. doi:10.1109/ACCESS.2020.2988038
Islam, S. M., Sylvester, A., Orpilla, G., and Lubecke, V. M. (2020b). “Respiratory feature extraction for radar-based continuous identity authentication,” in IEEE Radio and Wireless Symposium (RWS), 26-29 January, 2020, San Antonio, TX, USA, 119–122. doi:10.1109/RWS45077.2020.9050013
Islam, S. M. M., Borić-Lubecke, O., Zheng, Y., and Lubecke, V. M. (2020c). Radar-based non-contact continuous identity authentication. Rem. Sens. 12 (14), 2279–2299. doi:10.3390/rs12142279
Islam, S. M. M., Lubecke, L. C., Grado, C., and Lubecke, V. M. (2021). “An adaptive filter technique for platform motion compensation in unmanned aerial vehicle-based remote life sensing radar,” in 50th European Microwave Week (EuMW’20), Utrecht, Netherland, 10–15 January, 2021.
Islam, S. M. M., Yavari, E., Rahman, A., Lubecke, V. M., and Boric-Lubecke, O. (2018). “Separation of respiratory signatures for multiple subjects using independent component analysis with the JADE algorithm,” in 40th Annual International Conference of the IEEE Engineering in Medicine and Biology Society, 17–21 July 2018, (Honolulu, HI, USA: EMBC), 1234–1237. doi:10.1109/EMBC.2018.8512583
Johns Hopkins University (2020). COVID-19 dashboard by the center for systems science and engineering at johns Hopkins university. Available at: https://coronavirus.jhu.edu/map.html (Accessed Nov 20, 2020).
Li, C., Lubecke, V. M., Boric-Lubecke, O., and Lin, J. (2013). A review on recent advances in Doppler radar sensors for noncontact healthcare monitoring. IEEE Trans. Microw. Theor. Tech. 61 (5), 2046–2060. doi:10.1109/TMTT.2013.2256924
Massagram, W., Hafner, N., Lubecke, V., and Boric-Lubecke, O. (2013). Tidal volume measurement through non-contact Doppler radar with DC reconstruction. IEEE Sensor. J. 13 (9), 3397–3404. doi:10.1109/JSEN.2013.2257733
Massaroni, C., Nicolò, A., Schena, E., and Sacchetti, M. (2020). Remote respiratory monitoring in the time of COVID-19. Front. Physiol. 11, 635–636. doi:10.3389/fphys.2020.00635
Perez-Nieto, O. R., Guerrero-Guiterrez, M. A., Zamarron-Lopez, E. I., Deloya-Tomas, E., Aldama, J. C. G., Namendys-Silva, S. A., et al. (2020). Impact of asynchornies in acute respiratory distress syndrome due to CoronaVirus disease 2019. Crit. Care Explor. 2 (8), e0200. doi:10.1097/CCE.0000000000000200
Richardson, S., Hirsch, J. S., Narasimhan, M., Crawford, J. M., McGinn, T., Davidson, K. W., et al. (2020). Presenting characteristics, comorbidities, and outcomes among 5700 patients hospitalized with COVID-19 in the New York city area. JAMA 323 (20), 2052–2059. doi:10.1001/jama.2020.6775
Singhal, T. (2020). A review of coronavirus disease-2019 (COVID-19). Indian J. Pediatr. 87 (4), 281–286. doi:10.1007/s12098-020-03263-6
Tan, K. S., Saatchi, R., Elphick, H., and Burke, D. (2010). Real-time vision based respiration monitoring system. 7th International Symposium on Communication Systems, Networks & Digital Signal Processing (CSNDSP 2010), 17-21 July 2018, (Newcastle, UK: Northumbria University) 770–774. doi:10.1109/CSNDSP16145.2010.5580316
Taylor, W., Abbasi, Q. H., Dashtipour, K., Ansari, S., Shah, S. A., Khalid, A., et al. (2020). A review of the state of the art in non-contact sensing for COVID-19. Sensors 20 (19), 5665–5685. doi:10.3390/s20195665
WHO (2019). Coronavirus disease (COVID-19) pandemic. Available at: https://www.who.int/emergencies/diseases/novel-coronavirus-2019 (Accessed Nov 20, 2020).
Keywords: patient–ventilator asynchrony, remote respiration sensing, respiratory features, vital signs, tidal volume
Citation: Islam SMM and Kiber MA (2021) Optimizing Patient–Ventilator Synchrony Utilizing Radar-Based Respiratory Features for Monitoring COVID-19 Patients. Front. Comms. Net 1:636006. doi: 10.3389/frcmn.2020.636006
Received: 30 November 2020; Accepted: 31 December 2020;
Published: 22 February 2021.
Edited by:
Hina Tabassum, York University, CanadaReviewed by:
Kia Dashtipour, University of Glasgow, United KingdomDaniyal Haider, De Montfort University, United Kingdom
Copyright © 2021 Islam and Kiber. This is an open-access article distributed under the terms of the Creative Commons Attribution License (CC BY). The use, distribution or reproduction in other forums is permitted, provided the original author(s) and the copyright owner(s) are credited and that the original publication in this journal is cited, in accordance with accepted academic practice. No use, distribution or reproduction is permitted which does not comply with these terms.
*Correspondence: Shekh M. M. Islam, c2hla2hAaGF3YWlpLmVkdQ==