Change in urban forest age structure affects the value of ecosystem services provided
- 1Faculty of Agriculture and Forestry, University of Helsinki, Helsinki, Finland
- 2Horticulture Technologies, Natural Resources Institute Finland, Piikkiö, Finland
To achieve resilience goals, urban planners and decision-makers need accurate information on the benefits provided by urban trees and on the effects that management may have on them. This study investigates the impacts of management and disturbances on urban forest structure and function in Turku, Finland. Using a comprehensive urban tree database and the i-Tree software suite, we assessed the current structure and estimated the value of ecosystem services provided by Turku's urban forest. Additionally, we simulated changes in the urban forest over a 50-year period, considering different tree planting scenarios and the potential outbreak of the Asian longhorned beetle (ALB). Turku's urban forest comprised 38,438 public trees, dominated by Acer platanoides, Pinus sylvestris, Tilia × europaea, and Betula pendula. The estimated carbon storage was 12,336 t, valued at 1.98 million €, with an annual sequestration rate of 284 t (45,549 €/year). The trees also removed 8.97 t of pollutants annually, with an estimated value of 153,273 €. At the current rate of tree planting, the number of trees would decline over the course of 50 years resulting in a gradual decrease in the provision of ecosystem services. Although doubling the tree planting rate could slowly increase carbon storage and sequestration even under moderate ALB attack, it was insufficient to offset the damage caused by ALB if tree mortality rate reaches 50%. Compared to carbon storage and sequestration, changes in urban forest age structure had a more immediate impact on the removal of air pollution. These findings emphasize the importance of prioritizing investments in urban forests on grounds of their capacity to provide diverse ecosystem services. Incorporating these findings into decision-making processes would promote sustainable and resilient urban environments.
Introduction
The growth of cities is driving the shift toward urban living at an increasing rate (UN-Habitat, 2022). The growing population density and impact of climate change in urban areas have made cities increasingly vulnerable (FAO, 2016). Air pollution, flooding, and urban heat islands are among the environmental threats that urban planners face while seeking to promote resilient cities. High population density increases reliance on urban forests and their associated benefits (ecosystem services). To reduce the vulnerability of urban forests, it is important to understand the mechanisms that may lead to changes in their structure and function.
Healthy urban forests are crucial for providing benefits to citizens (Turner-Skoff and Cavender, 2019) and should be given priority in urban planning (FAO, 2016). The urban forest constitutes the cornerstone of green infrastructure that delivers diverse ecosystem services. Urban forest consists of all individual trees, tree lines, clusters of trees (<0.5 ha), and woodlands (>0.5 ha) located in an urban area (Davies et al., 2017). Trees can be found in forests, streets, public and private yards, parks, and gardens. The structure of an urban forest, including attributes such as the number, age, and size of trees, species composition, health, density, and spatial distribution of the trees, forms the basis for various ecosystem functions, such as gas exchange and growth (Nowak et al., 2008). Ecosystem functions, in turn, supply ecosystem services—the benefits derived by humans from ecosystems. The benefits provided by urban forests include, for example, reducing air pollution, mitigating stormwater runoff, reducing the risk of flooding, and sequestering and storing carbon (Elmqvist et al., 2013). The size and quantity of trees play a key role in shaping the provision of ecosystem services, as most of the benefits delivered by trees are linked to their healthy leaf area, like reducing air pollution, cooling, and carbon binding (Nowak, 2021).
Urban forest management is usually aimed at establishing sustainable urban forests that maximize the benefits provided by trees, align with established safety standards, and meet landscape and visual expectations and economic frames. Management goals can be achieved through targeted actions, including tree planting or removal, protecting existing forests, and selecting appropriate tree species and locations, which collectively influence the forest structure and further the benefits (Pretzsch et al., 2023). The structure of an urban forest, however, is not determined solely by management practices. Natural processes like regeneration, growth, mortality, and events such as storms and pest outbreaks, as well as human actions like the introduction of exotic species or climate change, also play significant roles in shaping the forest structure (Nowak et al., 2022). Invasive pests and diseases represent a substantial threat to healthy urban forests (Tubby and Webber, 2010, Bajeux et al., 2020). Non-native pests and diseases, which are spreading to new areas through natural and human-mediated processes, can result in substantial damage, as native trees may lack defenses against unfamiliar pathogens. The ecological and economic impacts of these outbreaks can be extensive, leading to changes in tree growth, increased tree mortality, and shifts in the composition, structure, and ecosystem services provided by urban forests.
The Asian longhorned beetle (Anoplophora glabripennis; ALB) poses a risk to many broadleaf tree species, as it is a wood-boring pest with a wide host range, capable of infesting multiple tree genera (Sjöman et al., 2014; van der Gaag and Loomans, 2014). Its preferred host genera are Acer, Populus, Salix, and Ulmus (Hérard et al., 2009), but other known hosts include for instance Aesculus, Alnus, Betula, Carpinus, Fagus, Fraxinus, Morus, Platanus, Prunus, Pyrus, Rosa, and Sorbus. Many of these tree genera are widely distributed in green areas, making ALB a significant threat to urban forests.
ALB has caused considerable tree mortality outside of its native range and has been designated as a high-risk quarantine pest (MacLeod et al., 2002). ALB outbreaks are more probable in the Northern Hemisphere than in the Southern Hemisphere, with Europe having the highest percentage of areas highly suitable for it (Zhou et al., 2021). In Finland ALB was detected for the first time in 2015. After 5 years of monitoring, the infestation was considered eradicated in 2020 (Ruokavirasto, 2022). In the future, the threat of ALB will increase due to climate change. In Finland the areas suitable for ALB will expand as parts of the country that are currently marginal regions for ALB, become more favorable habitats (Zhou et al., 2021).
A prominent level of species diversity is considered a key factor in reducing vulnerability of urban forests and maintaining ecosystem stability (McBride and Jacobs, 1976). An excessive number of trees belonging to the same species or genus can increase the susceptibility of the urban forest to pests and diseases. The “10-20-30 rule” proposed by Santamour (1990) suggests that no more than 10% of any species, 20% of any genus, and 30% of any family should be planted in the urban forest. This guideline has been used as a reference point and implemented in many cities all over the world, including in Finland (e.g., City of Turku, n.d.).
Age diversity is also a critical factor for ensuring the long-term stability of urban forests. Understanding current age structure is key for predicting future benefits of urban forests and determining management needs (McPherson and Rowntree, 1989). The optimal age structure of a city's tree population depends on, e.g., the intended ecological and societal objectives (Gundersen et al., 2005). A diverse age distribution, with both young and mature trees for all species, is commonly considered ideal (Peper et al., 2007). Until now, there has been limited research carried out on the age diversity of urban forests (Morgenroth et al., 2020), as previous studies have concentrated mostly on street trees (Richards, 1983; McPherson and Rowntree, 1989; Wang et al., 2018). Recent studies have included age class distribution as a key element in optimizing urban forest ecosystem services and adapting to urbanization and environmental changes (Rötzer et al., 2019; Pretzsch et al., 2023).
Decision-makers and stakeholders have shown a growing interest in quantifying and managing the various ecosystem services provided by urban forests (Lovell and Taylor, 2013; Niemelä, 2014; Kremer et al., 2016), as cities take on more responsibility to respond to the challenges posed by climate change (Castán Broto, 2017). However, urban forests are still often given a low priority when allocating city budgets, as they are primarily valued for their aesthetic appeal rather than the ecosystem services they provide (FAO, 2016; Treglia et al., 2021). The management costs of urban trees are often widely reported while the ecosystem services and benefits provided by urban trees are ignored because their monetary value is not well known (Konijnendijk, 2008).
According to Keith et al. (2023) recent global events, such as the COVID-19 pandemic, have made it even more challenging to prioritize and fund urban planning and development projects. Governments are facing competing demands and limited financial resources, which are constraining their ability to invest in urban forests. As a result, there is less political and financial support for expanding or even maintaining existing urban initiatives, although they are essential for promoting sustainable and resilient urban environment. A shortage of funding and inadequate management presents a risk to urban forests, hindering the full potential for benefits to be realized (Treglia et al., 2021). To address this challenge, assessing the benefits of urban forests in monetary terms can be a valuable approach. It allows for easier comparison of alternative plans and facilitates decision-making processes (Daily et al., 2009).
Our study aims to investigate the effects of management and disturbance on urban forest structure and function. More specifically, we first assessed the current structure of the urban forest in Turku, Finland, and estimated the amount and value of ecosystem services provided by it. Secondly, we simulated changes in the age structure and function of an urban forest caused by different tree planting schemes and by ALB invasion. Our hypothesis was that the rate of tree planting has a greater impact on the structure of urban forest and the provision of ecosystem services, such as carbon sequestration, and air pollution reduction, than the potential outbreak of the ALB. Ultimately, our study aims to make urban decision-makers and stakeholders aware of the value and benefits of urban trees.
Materials and methods
The study was carried out in the city of Turku, Finland (latitude 60.4518° N, longitude 22.2666° E) (Figure 1). The climate type in Turku is cold with warm summers and no dry season (Peel et al., 2007). The average annual temperature is 6.2°C and the average annual precipitation is 675 mm (Ilmatieteen laitos, 2022). Turku is the sixth largest city in Finland, with a population of 192,962 (Turun väestökatsaus, 2020) and a land area of 247 km2 (Maanmittauslaitoksen vuositilastoja 2019, 2019), resulting in a population density of 781 persons per km2.
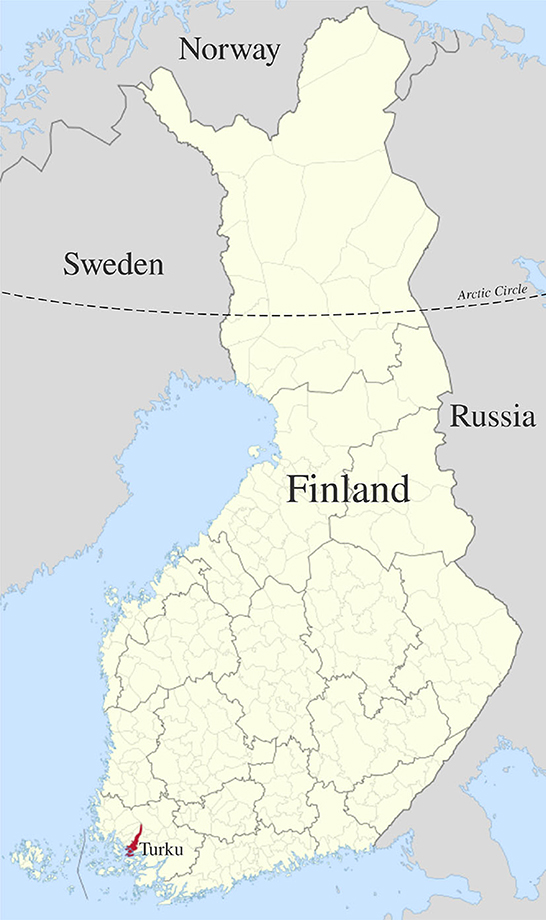
Figure 1. The city of Turku is located on the shore of the Baltic Sea in southwestern Finland in Northern Europe. The background map was originally sourced from Wikipedia under the CC BY-SA 3.0 license and has been modified to suit the specific needs of this illustration. Credit for the original map creation goes to Fenn-O-maniC, as required by the license terms.
Turku was the first city in Finland to establish an urban tree strategy, outlining goals for urban tree management and ensuring a thriving urban tree population in the future (City of Turku, n.d.). The Supplementary material S1 contains a map illustrating the distribution of urban trees in tree database in Turku. The main goal of the strategy is proactive risk management, aiming to preserve trees and their benefits for both human wellbeing and urban biodiversity. The strategy acknowledges that the impacts of climate change and the increased risks of diseases and pests present new challenges for the city's future tree population. We aimed to assess the susceptibility of Turku's urban tree population to pest infestations, seeking to understand how the goals outlined in the strategy would be achieved.
Tree data
In Turku, all street and park trees maintained by the Municipal Property Corporation have been listed in the urban tree database since 2015 (City of Turku, n.d.). To assess the structure of the urban forest in Turku, we utilized the comprehensive database consisting of over 33,000 urban trees located in public green spaces and streets. In addition, about 5,000 trees on city-owned plots, such as school yards and sporting areas, were included in the analysis. The precise locations of these trees can be viewed on a map provided in Supplementary material S1. Trees growing in forests or on unplanned grounds were excluded, as were trees managed by non-city parties.
Several studies have proposed ideal structural distributions for an urban forest based on the diameter at breast height (DBH at 1.3 m) as an indicator of age (Richards, 1983; Millward and Sabir, 2010). We used the distribution classes proposed by Wang et al. (2018). In the ideal structural distribution, the target proportions are 40% for young trees (DBH 0–15 cm), 25% for maturing trees (DBH 15–30 cm), 25% for mature trees (DBH 30–60 cm), and 10% for old trees (DBH > 60 cm).
The Importance Value (IV) is a more comprehensive indicator of a species' functional importance than the relative abundance or size alone. The higher IV a species has, the larger is its contribution to the ecosystem services provided by the urban forest. The IV was calculated for each tree species as the sum of its relative abundance (expressed as the percentage of total trees) and leaf area (expressed as the percentage of total leaf area) (Nowak, 2021).
The structure and benefits of the current urban forest
We used the i-Tree software suite (i-Tree version 6.1.46, Eco version 6.0.31), developed by the USDA Forest Service and its partners, to evaluate and quantify the value of the urban forest in Turku. I-Tree Eco employs a framework that follows the sequence of structure → function → services → benefits → values to evaluate forest structure and transform these structural assessments into estimations of services, benefits, and values (Nowak, 2021). Nowak (2021) explains that structure encompasses fundamental data on the physical forest resource (e.g., the number and size of trees, species composition, and location). These attributes are directly measured on-site or estimated (e.g., leaf area) by i-Tree based on structural measurements. Utilizing data on urban forest structure and local environmental conditions (e.g., weather), diverse tree functions (e.g., gas exchange and growth) are estimated. These functions are then translated into ecosystem services (e.g., pollution removal) using relevant local data (e.g., pollution concentrations). The services are further converted into benefits (e.g., enhanced air quality and human health impacts), considering additional data (e.g., local atmospheric conditions and demographic statistics). Benefits are then translated into economic values through varied economic methodologies.
In i-Tree Eco, the core tree variables used are species name, DBH at 1.3 m, total tree height, crown size, crown dieback, and crown light exposure (Nowak, 2021). The minimum requirements for analysis are species name and DBH. When species specific information is unavailable, i-Tree uses values from higher taxonomic categories like genus, family, order, or superorder (Nowak, 2021). Based on these measured characteristics, secondary structural variables are derived, such as leaf area, leaf biomass, leaf area index, and total tree biomass. The measured and derived variables are then used to estimate the provision of ecosystem services.
The tree data was downloaded from the municipal tree database in April 2019. Turku updates tree information in their database at intervals of 1–7 years, depending on the tree's condition. The aim is to update information for every tree at least once every 7 years (Salo, 2021).
We used species name, DBH at 1.3 m, and total tree height for analyzing the urban tree population of Turku. Tree trunk measurements, recorded in the database as circumferences, were converted to diameters by dividing them by 3.14. In the database, tree height was categorized into classes (0–5 m, 5–10 m, 10–15 m, 15–20 m, 20–25 m, and over 25 m). These were transformed into corresponding values (4, 7.5, 12.5, 17.5, 22.5, and 27.5 m) for the i-Tree analysis. The tree database did not contain information regarding crown size or light exposure, and information on tree condition was not applicable as the estimates were mostly based on the condition of the trunk rather than the canopy.
The i-Tree Eco software uses a combination of tree data and local hourly air pollution and weather data to determine the urban forest structure, the ecosystem services it provides, and their monetary value (Nowak, 2021). We used data from a weather station (Artukainen) located 5 km from the city center and modeled the monetary value of the following services: carbon sequestration and storage, air pollution removal, and overall replacement value. i-Tree default values were used for all ecosystem services analyzed. For carbon storage and carbon sequestration the default value was €160.67 t−1. Pollution removal was estimated for carbon monoxide (CO: €1,105.04 t−1), ozone (O3: €11,287.80 t−1), nitrogen dioxide (NO2: €1,685.74 t−1), sulfur dioxide (SO2: €614.12 t−1), particulate matter with diameter under 2.5 microns (PM2.5: €391,857.54 t−1), and over 2.5 but under 10 microns (PM10: €1,913.97 t−1).
Forecasting future urban forest changes
The forecast component of i-Tree Eco predicts future changes in the structure, function, and value of an urban forest. It uses i-Tree Eco methods (Nowak et al., 2008; Nowak, 2021) to simulate changes in the number, size, and distribution of trees by combining initial tree growth with user-specified or predetermined rates of tree mortality and tree establishment. The model runs simulations at 1-year intervals for a user-defined period, providing a comprehensive prediction of changes in the structure and function of the urban forest. The Forecast model can also be used to simulate the effects of extreme events such as pest outbreaks or severe weather phenomena. For a more detailed explanation of the model, see Nowak (2021).
We used the Forecast model to predict the changes in the urban forest of Turku over a 50-year period, considering different tree planting rate scenarios and ALB outbreaks (Table 1). Default values were used for simulation unless specified otherwise.
In the Forecast model, the base mortality rates are determined on grounds of tree dieback level: healthy (0–49% dieback), sick (50–74% dieback), and dying (75–99% dieback). Since we did not have any data about canopy health, i-Tree automatically classified all trees as being in a fair condition, indicating that the trees have at most 25% dieback. This means that Forecasting model used the base mortality rate set for healthy trees. For base annual mortality of healthy trees, we used a rate of 1.5% instead of the default mortality rate 3.0% that was considered too high by the municipal tree officer.1 The same mortality rate (1.5%) has been used for example by McPherson et al. (1999).
The annual number of trees planted was based on the tree planting data collected over the last 10 years in Turku. The average number of new park and street trees planted each year is 432, which is ~1.3% of the total tree number of park and street trees (see text footnote 1). Since the study data included trees growing on public plots in addition to park and street trees, the 1.3% planting rate would mean 500 trees annually for all public trees. Consequently, the annual tree planting rate was set to 500 for the current planting rate scenarios (scenarios 1, 3, 5) and to 1,000 for scenarios in which the planting rate was doubled (scenarios 2, 4, 6).
The DBH for the new trees was set at 5.0 cm, aligning with standard planting practices in Turku. Known host trees for ALB were included in the tree planting scenarios, acknowledging their role in species composition despite the risk of an ALB outbreak. For species composition of new trees, the i-Tree forecast model assumes proportions based on current species composition.
Initially, i-Tree Forecast had the annual mortality rate due to the ALB infestation set at 2.3% by default. However, Nowak et al. (2001) estimated losses between 12 and 61% of the tree population following an ALB infestation in several cities in the U.S. Consequently, the mortality rate was conservatively set to 10% in low infestation scenarios (scenarios 3 and 4) and to 50% in high infestation scenarios (scenarios 5 and 6).
Results
The current structure of the urban forest
There were 38,438 public trees in Turku with a total canopy cover of 152.8 ha. We identified 133 tree species, the most dominant being Acer platanoides (12.3%), Pinus sylvestris (12.2%), Tilia × europaea (12.0%), and Betula pendula (11.4%) (Table 2). A complete list of species can be found in the Supplementary material S2. The five most common species covered 53.3% of the total tree population. The most dominant tree genera were Betula (13.7%), Pinus (13.5%), Tilia (13.4%), Acer (13.1%), and Sorbus (7%).
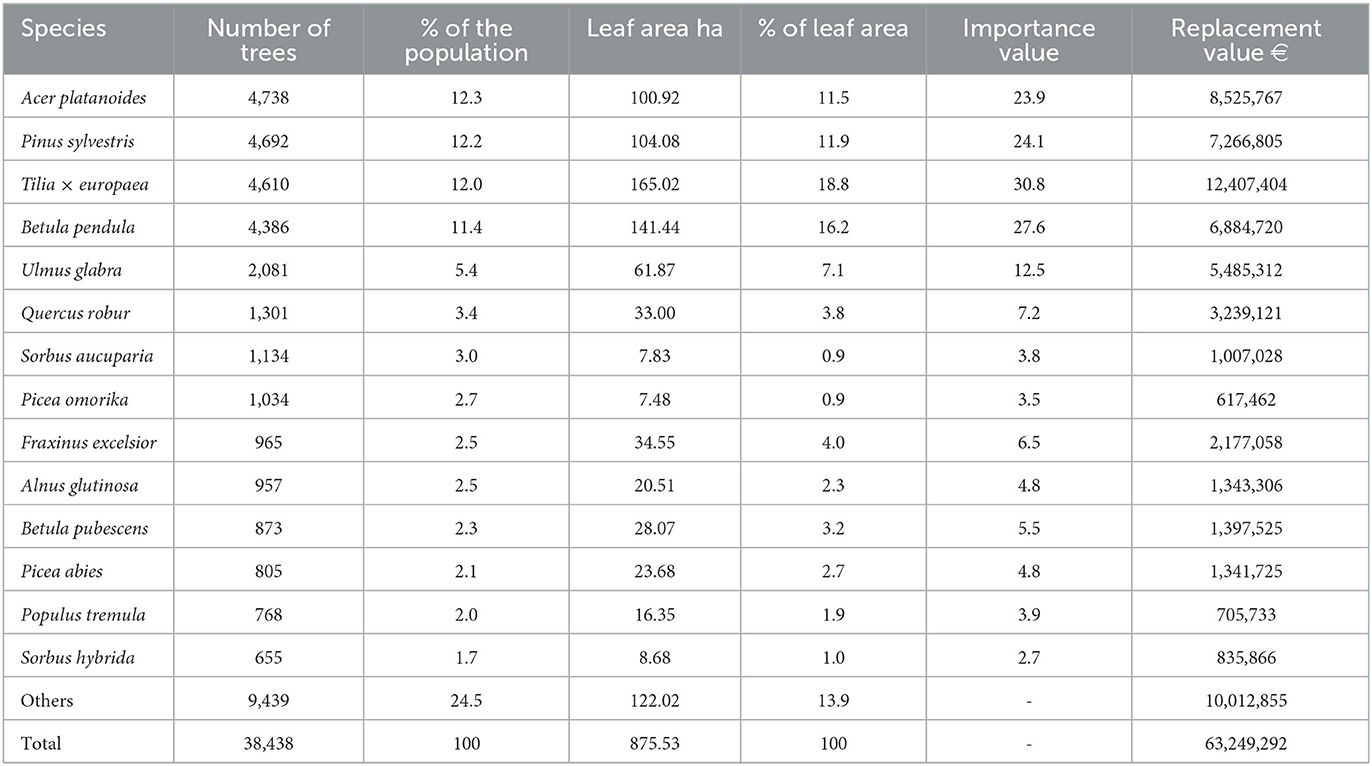
Table 2. The number, leaf area, importance value, and replacement value of the most common tree species in Turku.
The age distribution of the urban forest in Turku was quite even with 29% of the trees classified as young, 33% as maturing, and 33% as mature (Figure 2). Only the share of old trees was quite low, comprising just 5% of the total. The number of species decreased along with the age: young trees comprised 120 species while the maturing, mature, and old trees represented 86, 84, and 50 species, respectively. Only 43 species had at least one individual tree in each age class.
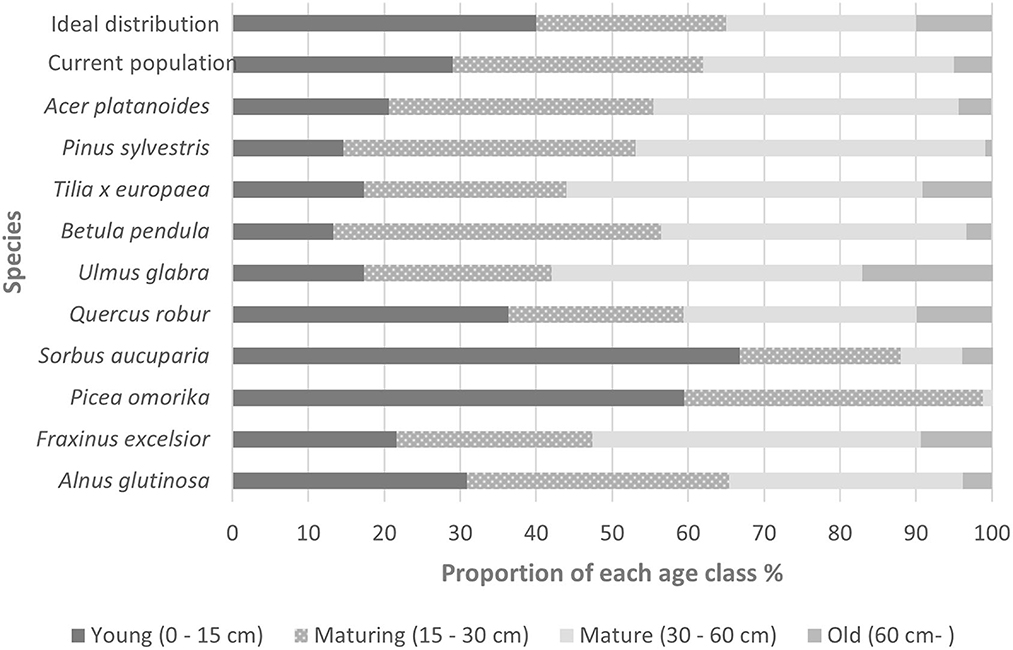
Figure 2. The age distribution of the entire tree population and the ten most abundant species in Turku, highlighting their deviation from the ideal age distribution.
The total leaf area of the urban trees in Turku was 876 ha (Table 2). Canopies of the five most abundant species constituted 65.5% of the total leaf area. The species with the largest leaf area was Tilia × europaea, with a value of 165.02 hectares. Other significant contributors to the leaf area were Betula pendula, Pinus sylvestris, and Acer platanoides.
The city of Turku relied the most on the functional capacity of Tilia × europaea, which had the highest importance value of 30.8 (Table 2). This was due to its large leaf area, which exceeded that of the two more abundant species, Pinus sylvestris and Acer platanoides. Similarly, the substantial leaf area of Betula pendula also resulted in a higher importance value than more prevalent species.
Ecosystem services provided by the current urban forest
The urban trees in Turku stored an estimated 12,336 t of carbon, valued at 1.98 million euros (Table 3). The top five tree species accounted for 61% of the total carbon stored in the city trees. Acer platanoides held the greatest carbon stock, estimated as 1,828 t (14.8% of the total) worth of 293,766 €.
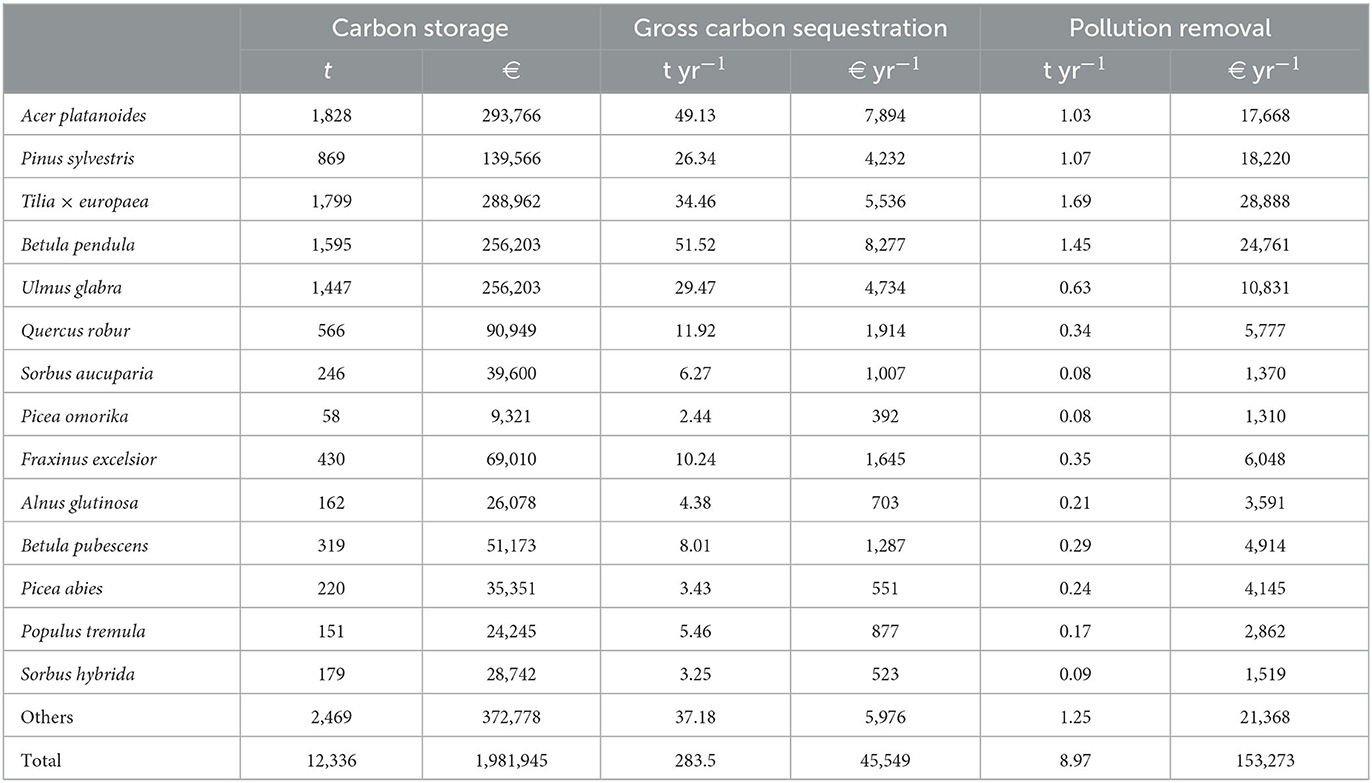
Table 3. Ecosystem service values of carbon storage, gross carbon sequestration, and pollution removal for most abundant tree species.
Turku's trees were estimated to sequester yearly a total of 284 t carbon, worth 45,549 € (Table 3). The top five tree species accounted for 67% of the total carbon sequestered. Betula pendula sequestered the greatest amount of carbon, estimated as 5.52 t yr−1(18.2% of the total) and valued at 8,277 €.
The trees in Turku were able to remove 897 t of pollutants from the atmosphere each year (Table 3). The highest quantities of pollutants removed were O3 and NO2 (Table 4). The removal of pollutants had an annual total value of 153,273 €. Tilia × europaea contributed the most to pollution removal: 1.69 t yr−1 (18.8% of total), valued at 28,888 €.
The total replacement value of urban trees in Turku was 63.2 million €. The tree species with the greatest replacement value were Tilia × europaea, Acer platanoides, and Pinus sylvestris.
The effect of planting rate on forecasting future changes in urban forest
In Scenario 1, the projections showed that with current planting rates, the number of trees would decrease by 7.8% (Figure 3) and the canopy cover would decrease by 1.1% over the next 50 years. The age distribution of trees would shift toward older trees, with a decrease in the proportion of the young and maturing trees and an increase in the old tree classes (Figure 4). Despite the decrease in the number of trees, the total leaf area would increase by 7.8%. The maturing tree population and growing leaf area would also lead to an increase in carbon storage and carbon sequestration. Carbon storage would rise by 25.6% (Figure 5), and the gross carbon sequestration rate would increase by 11.7% (Figure 6). However, due to the declining tree population and diminishing canopy cover, the amount of pollution removed would decrease by 8.4% (Figure 7). By the 50th year, the combined value of carbon storage, carbon sequestration, and reduction in air pollution would amount to 2,680,439 €, which is 22.9% higher than the current value with the existing tree population (Table 5).
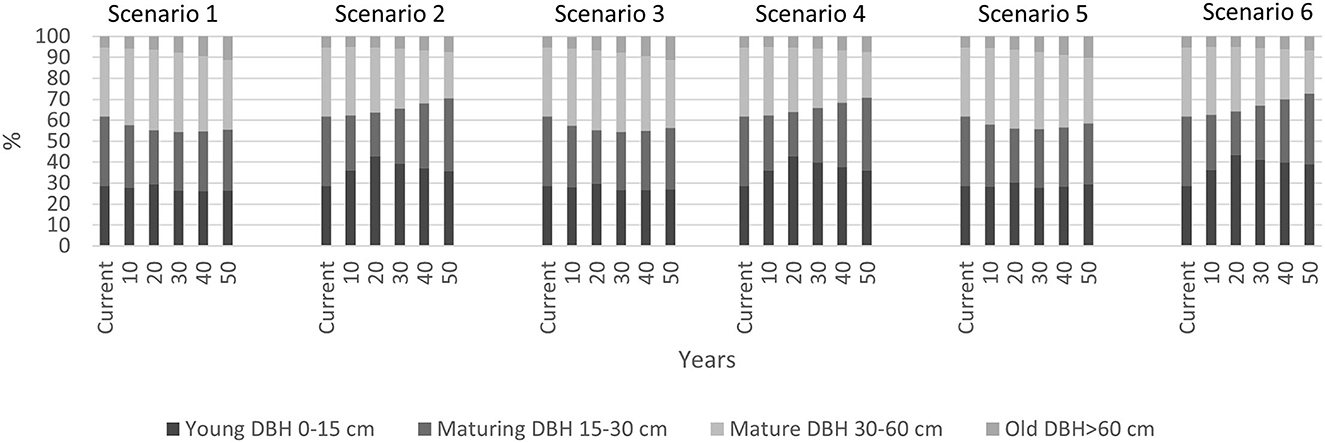
Figure 4. The relative proportions of trees in different age classes over time. The total number of trees (100%) varies between scenarios.
Under Scenario 2, where the current tree planting rate was doubled, the number of trees would experience a 38% increase over 50 years (Figure 3), signifying a considerable improvement compared to Scenario 1. The age distribution of the trees would shift toward younger classes, with a higher proportion of trees in the young and maturing categories and a lower proportion in the mature and old (Figure 4). The total leaf area would increase by 21.9%, carbon storage would rise by 31.7% (Figure 5), and the gross carbon sequestration rate would increase by 27.5% (Figure 6). Additionally, the total amount of pollution removed was projected to increase by 6.8% (Figure 7). By the 50th year, the combined value of carbon storage, carbon sequestration, and air pollution reduction was estimated to reach 2,833,193 €, indicating a 29.9% increase compared to the current value with the existing tree population (Table 5).
The effect of pest outbreak on forecasting future changes in urban forest
The i-Tree Eco analysis suggested that the ALB had the potential to harm 41.2% of the trees in the study area, resulting in a potential economic loss of 27.4 million euros in terms of replacement cost.
In Scenario 3, tree planting would continue at the current rate, with a 10% annual mortality caused by the ALB. Over the next 50 years, the number of trees would decrease by 10.5% (Figure 3), shifting the age distribution toward older trees, like in Scenario 1 (Figure 4). The total leaf area would increase by 3.5%, carbon storage by 19.7% (Figure 5), and the annual gross carbon sequestration rate by 6.0% (Figure 6). Despite a predicted 11.7% decrease in pollution removal (Figure 7), the combined value of these benefits was estimated to increase by 17.3% to 2,557,079 € by year 50 (Table 5).
In Scenario 4, with a doubled tree planting rate and a 10% annual mortality due to the ALB, the number of trees would increase by 35.6% (Figure 3). The age distribution would shift toward younger and maturing trees, as in Scenario 2 (Figure 4). Total leaf area would increase by 18.0%, carbon storage by 27.3% (Figure 5), gross carbon sequestration rate by 22.9% (Figure 6) and pollutant removal by 4.5% (Figure 7). By year 50, the combined economic value of these benefits would reach 2,739,521€ annually, a 25.6% increase from the current value (Table 5).
In Scenario 5, with the current tree planting rate and a 50% mortality rate due to the ALB, the number of trees would decline by 22% (Figure 3). The age distribution would shift, with more young and old trees and fewer mature trees (Figure 4). The model predicted a 14.5% decrease in total leaf area, a 7.3% decrease in carbon storage (Figure 5), an 18% decrease in gross carbon sequestration rate (Figure 6), and a 26.8% decrease in air pollutant removal (Figure 7). The total value of these benefits was estimated to be 1,986,504€ by year 50, representing an 8.9% decrease compared to the current value (Table 5).
In Scenario 6, with double tree planting and 50% ALB mortality rate, the number of trees would increase by 18.5% (Figure 3). The age distribution would shift toward more young and maturing trees, with a slight decrease in mature trees and a slight increase in old trees (Figure 4). Total leaf area, carbon storage, gross carbon sequestration rate, and the amount of air pollutants removed would decrease by 3.2, 2.1, 4.2, and 13.9%, respectively (Figures 5–7). The total value of these benefits would amount to 2,115,988 € by year 50, representing a 3.0% decrease compared to the current value with the existing tree population (Table 5).
Discussion
Urban decision-makers and stakeholders play a crucial role in shaping the future of our cities. They are responsible for allocating resources, planning, and designing urban environments, and making decisions that affect resident's quality of life. It is essential that they are informed about the value of urban trees and the benefits they provide in terms of ecosystem services.
The current and forecasted age structure of the urban forest
The urban forest's structure is crucial for its appearance and vegetation quantity. Achieving a healthy and sustainable urban tree population requires an elevated level of species and genus diversity (McBride and Jacobs, 1976; Raupp et al., 2006). In Turku, there were 38,438 trees comprising 133 species. Given Finland's limited number of around 30 native tree species (Luonnonvarakeskus, n.d.), it is not surprising that the urban forests in Turku exhibit greater tree species richness compared to the surrounding natural forested areas. Urban areas often have higher species richness than rural areas due to factors such as the presence of introduced species, socio-economic factors, land use and cover heterogeneity, and diverse environmental factors such as soil and climate (Richards, 1983; Hope et al., 2003; Kendal et al., 2014).
Urban forests are often characterized by high species richness (Pauleit et al., 2002), although a few dominant species tend to comprise a considerable proportion of the tree population (Bassuk et al., 2009). In Turku, four species (Acer platanoides, Pinus sylvestris, Tilia × europaea, and Betula pendula) accounted for roughly half (47.9%) of the total number of trees, exceeding the recommended guideline of 10% for species diversity in urban forests (Smiley et al., 1986; Santamour, 1990; Miller and Miller, 1991). At the genus and family level, the benchmarks proposed by Santamour (1990) were not surpassed. In Turku, the species distribution was more diverse than the global average. A study of 108 cities worldwide revealed that 20% of trees in urban forests belonged to the same species, 26% to the same genus, and 32% to the same family (Kendal et al., 2014). Nevertheless, Santamour (1990) acknowledged that his formula may not ensure the stability of urban forests when faced with polyphagous pests like ALB, which have a wide range of host preferences.
The dominance of certain species could indicate a lack of resilience and increased vulnerability to pest outbreaks. The ALB has the potential to damage 41.2% of urban trees in Turku. Acer and Betula, which were among the most frequent tree genera, are highly susceptible to ALB infestation (Haack et al., 2006). While Tilia species are not listed as ALB hosts in the i-Tree database, some publications suggest that ALB may feed on them (Nowak et al., 2001; Haack et al., 2006), while others indicate that Tilia species are rarely affected (Raupp et al., 2006). Other common tree genera in Turku's urban forests, such as Ulmus and Sorbus, are also susceptible to ALB infestation (Haack et al., 2010).
Age is also a critical factor affecting both the increase in biomass and the structural development of an urban forest (Rötzer et al., 2019), which impacts the provision of ecosystem services. When compared to the ideal distribution suggested by Wang et al. (2018), we found that the percentages of young and old trees were below the optimum, while both mature and maturing trees exceeded the ideal proportions. None of the five most dominant species reached the ideal percentage for young trees (40%) (Figure 2). For maturing trees, only Tilia × europaea (27%) was close to ideal (25%), while the other four most common species exceeded the optimum of maturing trees by 11% or more. All top-five species had a larger than ideal share of mature trees (40–47%).
McPherson and Rowntree (1989) identified three prominent types of stem diameter distributions for urban tree population. The type 1 pattern aligns with Richards' (1983) recommendation for a sustainable age distribution in urban tree populations. This distribution is characterized by a high proportion of trees in small diameter class (young trees), which is necessary to offset mortality rates during the establishment phase.
The urban trees in Turku showed the type 2 diameter distribution pattern, with more trees in the maturing and mature diameter classes and fewer in the young tree class compared to type 1 populations (McPherson and Rowntree, 1989). It is also worth noting that none of the five most dominant tree species achieved the ideal age distribution. This kind of distribution indicates that the urban tree population in Turku primarily consists of trees that were planted several decades ago and are now mature, providing maximum benefits with minimal maintenance requirements. However, as these trees continue to age, maintenance costs may increase.
In 50 years, the current urban tree population of Turku is likely to transfer to type 3 age structure, which has an almost equal distribution of trees in all diameter classes, implying a higher proportion of old trees compared to type 1 and type 2 populations. This suggests that many of the trees in type 3 populations were planted more than 50 years ago and are now mature or senescent. While these trees still provide significant benefits due to their large aboveground biomass, increased hazard liability and removal costs may partially offset these benefits. Removing large trees can have a significant impact on canopy cover and benefits provided (Sousa-Silva et al., 2023). This suggests that there may be a need for more emphasis on planting young trees to achieve a more balanced age distribution among the urban tree population. However, newly planted trees are particularly vulnerable to environmental and physiological stresses in the years immediately following planting, making this period critical for their survival and growth (Wattenhofer and Johnson, 2021) and it should be noted that not all planted trees will reach maturity. Therefore, to increase the canopy cover, the rate of tree planting should exceed the rate of removal (Sousa-Silva et al., 2023).
A further benefit of an uneven-aged tree population is that it enables managers to distribute the maintenance costs evenly over several years (Peper et al., 2007). By doing so, they can ensure that the expenses associated with maintaining the trees do not accumulate in a single year, but rather are spread out over a more extended period. Additionally, an uneven-aged population can help to maintain a stable urban tree population, since it allows for a continuous supply of younger trees that can grow and replace older ones as they die or are removed (Richards, 1983).
The current and forecasted ecosystem services
Urban forests are frequently integrated into climate change mitigation strategies at the municipal level due to their provisioning of ecosystem services. The ecosystem services provided are intricately linked to the amount of live tree biomass, its growth rate, and the canopy cover and volume, as highlighted by Nowak et al. (2008) and Ziter et al. (2019). Healthy and structurally diverse forests are likely to be resilient and provide the most ecosystem services.
We investigated the impact of changes in urban forest structure on ecosystem service provision, considering variations in tree planting rates and pest outbreaks. We found that if the current tree planting rate is maintained, the number of trees will decline over a 50-year period. Initially, maturing trees can compensate for the decrease in tree cover and contribute to increased carbon storage and sequestration. However, as older trees die, the insufficient number of maturing trees leads to a gradual decline in both carbon storage and sequestration. This decline is particularly pronounced in scenarios where the urban forest is affected by the ALB. For instance, with a 10% mortality rate, the carbon sequestration rate can be sustained until the fourth decade, followed by a slow decline. In the case of a 50% mortality rate due to the ALB, the decline in carbon sequestration begins within the first decade, resulting in significantly lower carbon sequestration compared to the current situation. Consequently, the decline in carbon sequestration also affects the accumulation of carbon storage over time.
Doubling the current tree planting rate led to a steady increase in carbon sequestration with no disturbances. In the case of a pest outbreak causing 10% mortality, the planting of new trees could still offset the loss and maintain an increasing carbon sequestration rate over the 50-year period, albeit at a slower pace. However, if the pest outbreak resulted in a 50% mortality rate, doubling the tree planting rate would be insufficient to compensate for the losses of mature trees. As a result, carbon sequestration began to decline after 30 years, resulting in a slightly lower rate compared to the present situation after 50 years.
A 50% mortality rate significantly affected carbon storage in each tree planting scenario. In scenario 5, where the current tree planting rate was maintained, the decline in carbon storage accelerated after 40 years. In scenario 6, with a two-fold tree planting rate, the decline was more gradual. In both scenarios, carbon storage after 50 years was smaller than the current level. These findings align with the results of Steenberg et al. (2017), indicating that areas with high vulnerability to ALB experience a pronounced reduction in carbon storage, while less vulnerable locations may encounter a limited carbon stock increase. The actual losses could be even more severe than our conservative model indicates. Sjöman and Östberg (2019) came to conclusion that in the worst-case scenario, ALB could lead to potential tree losses ranging from 41 to 96% in urban forests.
Changes in urban forest structure had a more immediate and pronounced impact on air pollution removal than on carbon sequestration. The decline in air pollution removal began with a decrease in the number of trees, even if the overall leaf area continued to increase. The decrease in air pollution removal was more noticeable than the decrease in tree numbers, which may be attributed to the reduction in tree cover directly used in the i-Tree model for calculating air pollution removal. As noted by Nowak (2000), large and healthy trees can remove ~70 times more pollution per year than small trees. This emphasizes the crucial role of larger trees in effectively reducing air pollution in urban environments.
The planting rate and the occurrence of pest outbreaks significantly influenced the value of the ecosystem services that urban forests provide. By doubling the planting rate (scenario 2), more ecosystem services were provided, and the overall value increased. The difference in value between the current planting rate (scenario 1) and a double planting rate (scenario 2) amounted to 122,174€ for carbon storage after 50 years. For carbon sequestration and pollution removal, the difference was 7,327 and 23,072€ per year, respectively. In the scenarios with ALB outbreaks, the differences were more pronounced. The lowest provision of ecosystem services occurred when the planting rate was low, and the infestation rate was high (scenario 5). Comparing the value of ecosystem services with the least detrimental scenario, where the planting rate was twice the current level and the infestation rate was low (scenario 4), the differences become apparent. In terms of carbon storage, the difference amounted to 686,672€ in year 50, while carbon sequestration showed a difference of 18,622€ per year, and air pollution removal accounted for a difference of 47,723€ per year in year 50. It is important to note that the figures for carbon sequestration and pollution removal represent annual values, and so the distinction between the scenarios will become increasingly pronounced in the long term.
Insights and limitations
This study was the first implementation of the i-Tree methodology in Finland, providing valuable insights into the assessment of urban forests in our country. The findings contribute to improved urban forest planning practices and form the basis of future i-Tree studies in northern cities. Moreover, the study's findings can be easily extrapolated to urban areas in general. While there may be variations in specific environmental and socio-economic factors, the fundamental principles and relationships between management decisions, disturbances, and their effects on urban forests hold true. As a result, this study offers valuable insights into urban forest management strategies across diverse urban areas.
The strengths of this study stem from the full inventory data of urban trees in Turku that allowed robust analyses and sound conclusions about the structure and function of the city-owned urban forest. Nevertheless, it is good to acknowledge that the inventory data did not encompass forested areas within the city or privately owned lands, leading to an underestimation of the ecosystem services offered by the entire urban forest in Turku. Our study also focuses on a narrow range of urban forest benefits. Additional services, like avoided runoff, cooling, noise mitigation, and a diverse array of intangible benefits and cultural values (Elmqvist et al., 2013), were ignored. It is worth noting that we did not consider the disservices associated with urban trees, such as leaf litter, pollen, or tree damage.
Conclusions
Our research in Turku examined the impact of management and disturbances on the urban forest. We assessed the current urban forest, quantified its ecosystem services, and used simulations to explore tree planting strategies and the effects of an ALB invasion. The study shows that tree planting rates affect the urban forest, ecosystem services provided, and the value of the benefits more than an ALB outbreak. The results of this study reveal that management choices substantially impact the economic value of ecosystem services, with important implications for urban forest management, city budgeting, and resource allocation.
Maintaining a healthy and diverse urban forest structure is crucial for maximizing ecosystem services like carbon storage, sequestration, and air pollution removal. We identified several key steps that can guide urban planners and managers toward achieving the goals for sustainable urban forests. Firstly, prioritizing a diversified species distribution can enhance the resilience of the urban forest and reduce susceptibility to pests like the Asian longhorn beetle (ALB). Secondly, achieving a balanced age structure by strategically planting young trees will contribute to consistent canopy cover and stabilizes maintenance costs. Thirdly, addressing the dominance of certain vulnerable tree species by introducing less susceptible alternatives can help mitigate risks. Lastly, the promotion of a stable urban tree population by emphasizing planting rates that exceed removal rates contributes to the overall resilience of the urban forest.
Prioritizing urban forests is essential for optimizing their benefits. Addressing potential threats like pests through proactive management is also important. Allocating sufficient resources to urban forests supports climate change mitigation and enhances the wellbeing and quality of life for city residents. To tackle these challenges, using a monetary assessment of urban forest benefits can be a powerful approach. This method helps decision-making by allowing easy comparisons of different plans, aiding resource allocation, and promoting sustainable urban initiatives. As cities navigate the interplay of environmental concerns, urbanization, and limited resources, understanding the concrete benefits of urban forests becomes crucial. By thorough assessment and resource allocation, urban forests can rightfully claim their place in urban planning and significantly contribute to resilient, sustainable, and thriving urban environments.
Data availability statement
The raw data supporting the conclusions of this article will be made available by the authors, without undue reservation.
Author contributions
MM: Writing—original draft, Writing—review & editing. LL: Supervision, Writing—review & editing. E-MT: Supervision, Writing—review & editing.
Funding
The author(s) declare that financial support was received for the research, authorship, and/or publication of this article. The research was funded by Maiju ja Yrjö Rikalan Puutarhasäätiö sr - Foundation, Societas pro Fauna et Flora Fennica, and Jenny and Antti Wihuri Foundation (00190250, 00200235, 00210227, and 00220235).
Acknowledgments
We want to thank Aki Männistö, a tree specialist from the City of Turku, for their valuable contributions to our study.
Conflict of interest
The authors declare that the research was conducted in the absence of any commercial or financial relationships that could be construed as a potential conflict of interest.
Publisher's note
All claims expressed in this article are solely those of the authors and do not necessarily represent those of their affiliated organizations, or those of the publisher, the editors and the reviewers. Any product that may be evaluated in this article, or claim that may be made by its manufacturer, is not guaranteed or endorsed by the publisher.
Supplementary material
The Supplementary Material for this article can be found online at: https://www.frontiersin.org/articles/10.3389/frsc.2023.1265610/full#supplementary-material
Footnotes
1. ^Männistö, A. Personal communication by email. (2022).
References
Bajeux, N., Arino, J., Portet, S., and Westwood, R. (2020). Spread of Dutch elm disease in an urban forest. Ecol. Model. 438, 109293. doi: 10.1016/j.ecolmodel.2020.109293
Bassuk, N., Deanna, F. C., Marranca, B. Z., and Barb, N. (2009). Recommended Urban Trees: Site Assessment and Tree Selection for Stress Tolerance. Ithaca, NY: Urban Horticulture Institute, Cornell University.
Castán Broto, V. (2017). Urban governance and the politics of climate change. World Dev. 93, 1–15. doi: 10.1016/j.worlddev.2016.12.031
City of Turku (n.d.). The Urban Tree Policy of Turku. City of the Trees. Turku - the Arboretum of the Future. Available oline at: https://www.turku.fi/sites/default/files/atoms/files//turku_puulajilinjaus_esite_160x210_eng_lr.pdf (accessed October 4 2022).
Daily, G. C., Polasky, S., Goldstein, J., Kareiva, P. M., Mooney, H. A., Pejchar, L., et al. (2009). Ecosystem services in decision making: time to deliver. Front. Ecol. Env. 7, e080025. doi: 10.1890/080025
Davies, H., Doick, K., Handley, P., O'Brien, L., and Wilson, J. (2017). Delivery of Ecosystem Services by Urban Forests. Forestry Commission Research Report. Edinburgh: Forestry Commission.
Elmqvist, T., Marcotullio, P. J., Goodness, J., Schewenius, M., Parnell, S., McDonald, R. I., et al. (2013). Urbanization, Biodiversity and Ecosystem Services: Challenges and Opportunities. A Global Assessment. Dordrecht: Springer. doi: 10.1007/978-94-007-7088-1
FAO (2016). “Guidelines on Urban and Peri-Urban Forestry,” eds. F. Salbitano, S. Borelli, M. Conigliaro, and Y. Chen. FAO Forestry Paper No. 178. Rome: Food and Agriculture Organization of the United Nations.
Gundersen, V., Frivold, L. H., Löfström, I., Jorgensen, B. B., Falck, J., and Oyen, B.-H. (2005). Urban woodland management – The case of 13 major Nordic cities. Urban Forest Urban Green 3, 189–202. doi: 10.1016/j.ufug.2005.03.001
Haack, R. A., Bauer, L. S., Gao, R. T., McCarthy, J. J., and Miller, D. L. (2006). Anoplophora glabripennis within-tree distribution, seasonal development, and host suitability in China and Chicago. Gt. Lakes Entomol. 102, 1075–1084.
Haack, R. A., Hérard, F., Sun, J., and Turgeon, J. J. (2010). Managing invasive populations of Asian longhorned beetle and citrus longhorned beetle: a worldwide perspective. Annu. Rev. Entomol. 55, 521–546. doi: 10.1146/annurev-ento-112408-085427
Hérard, F., Maspero, M., Ramualde, N., Jucker, C., Colombo, M., Ciampitti, M., et al. (2009). Anoplophora glabripennis infestation (Col.: Cerambycidae) in Italy. Bulletin OEPP 39, 146–152.
Hope, D., Gries, C., Zhu, W., Fagan, W. F., Redman, C. L., Grimm, N. B., et al. (2003). Socioeconomics drive urban plant diversity. Proc. Natl. Acad. Sci. 22, 8788–8792. doi: 10.1073/pnas.1537557100
Ilmatieteen laitos (2022). Lämpötila-ja sadetilastoja vuodesta 1961. Available online at: https://www.ilmatieteenlaitos.fi/tilastoja-vuodesta-1961 (accessed October 4, 2022).
Keith, M., Birch, E., Buchoud, N. J., Cardama, M., Cobbett, W., Cohen, M., et al. (2023). A new urban narrative for sustainable development. Nat. Sustainab. 6, 115–117. doi: 10.1038/s41893-022-00979-5
Kendal, D., Dobbs, C., and Lohr, V. I. (2014). Global patterns of diversity in the urban forest: is there evidence to support the 10/20/30 rule? Urban Forest. Urban Green. 13, 411–417. doi: 10.1016/j.ufug.2014.04.004
Konijnendijk, C. C. (2008). The Forest and the City: The Cultural Landscape of Urban Woodland. Berlin: Springer.
Kremer, P., Hamstead, Z. A., and McPherson, T. (2016). The value of urban ecosystem services in New York City: a spatially explicit multicriteria analysis of landscape scale valuation scenarios. Env. Sci. Policy 62, 57–68. doi: 10.1016/j.envsci.2016.04.012
Lovell, S. T., and Taylor, J. R. (2013). Supplying urban ecosystem services through multifunctional green infrastructure in the United States. Landsc. Ecol. 28, 1447–1463. doi: 10.1007/s10980-013-9912-y
Luonnonvarakeskus (n.d.). Tietoa puulajeista ja niiden menestymisestä. Available online at: https://metsainfo.luke.fi/fi/cms/metsavarat/puulajit/tietoa-puulajeista (accessed March 12, 2023).
Maanmittauslaitoksen vuositilastoja 2019 (2019). https://www.maanmittauslaitos.fi/sites/maanmittauslaitos.fi/files/attachments/2020/02/vuositilasto_2019_0.pdf (accessed October 4, 2022).
MacLeod, A., Evans, H. F., and Baker, R. H. A. (2002). An analysis of pest risk from an Asian longhorn beetle (Anoplophora glabripennis) to hardwood trees in the European community. Crop. Prot. 21, 635–645. doi: 10.1016/S0261-2194(02)00016-9
McBride, J., and Jacobs, D. (1976). Urban forest development: a case study, Menlo Park, California. Urban Ecol. 2, 1–14. doi: 10.1016/0304-4009(76)90002-4
McPherson, E. G., and Rowntree, R. A. (1989). Using structural measures to compare twenty-two U.S. street tree populations. Landsc. J. 8, 13–23. doi: 10.3368/lj.8.1.13
McPherson, E. G., Simpson, J. R., Peper, P. J., and Xiao, Q. (1999). Benefits-cost analysis of Modesto's municipal urban forest. J. Arboric. 25, 235–248. doi: 10.48044/jauf.1999.033
Miller, R. H., and Miller, R. W. (1991). Planting survival of selected street tree taxa. J. Arboric. 17, 185–191. doi: 10.48044/jauf.1991.046
Millward, A. A., and Sabir, S. (2010). Structure of a forested urban park: implications for strategic management. J. Environ. Manage. 91, 2215–2224. doi: 10.1016/j.jenvman.2010.06.006
Morgenroth, J., Nowak, D. J., and Koeser, A. K. (2020). DBH distributions in America's urban forests—An overview of structural diversity. Forests 11, 135. doi: 10.3390/f11020135
Niemelä, J. (2014). Ecology of urban green spaces: the way forward in answering major research questions. Landscape Urban Plan. 125, 298–303. doi: 10.1016/j.landurbplan.2013.07.014
Nowak, D. (2021). Understanding i-Tree: 2021 Summary of Programs and Methods. NRS-200-2021. Madison, WI: USDA Forest Service One Gifford Pinchot Drive.
Nowak, D. J. (2000). The Effects of Urban Trees on Air Quality. Syracuse, NY: USDA Forest Service. p. 1–4.
Nowak, D. J., Crane, D. E., Stevens, J. C., Hoehn, R. E., Walton, J. T., and Bond, J. (2008). A ground-based method of assessing urban forest structure and ecosystem services. Arboric. Urban For. 34, 347–358. doi: 10.48044/jauf.2008.048
Nowak, D. J., Greenfield, E. J., and Ellis, A. (2022). Assessing urban forest threats across the conterminous United States. J. For. 120, 676–692. doi: 10.1093/jofore/fvac019
Nowak, D. J., Pasek, J. E., Sequeira, R. A., Crane, D. E., and Mastro, V. C. (2001). Potential effect of Anoplophora glabripennis (Coleoptera: Cerambycidae) on urban trees in the United States. J. Econ. Entomol. 94, 116–122. doi: 10.1603/0022-0493-94.1.116
Pauleit, S., Jones, N., Garcia-Martin, G., Garcia-Valdecantos, J. L., Riviere, L. M., Vidal-Beaudet, L., et al. (2002). Tree establishment practise in towns and cities – Results from a European survey. Urban Forest. Urban Green. 1, 83–96. doi: 10.1078/1618-8667-00009
Peel, M. C., Finlayson, B. L., and McMahon, T. A. (2007). Updated world map of the Köppen-Geiger climate classification. Hydrol. Earth Syst. Sci. 11, 1633–1644. doi: 10.5194/hess-11-1633-2007
Peper, P. J., McPherson, E. G., Simpson, J. R., Gardner, S. L., Vargas, K. E., and Xiao, Q. (2007). New York City, New York Municipal Forest Resource Analysis. Davis, CA: Center for Urban Forest Research, Pacific Southwest Research Station, USDA Forest Service.
Pretzsch, H., Moser-Reischl, A., Rahman, M. A., Pauleit, S., and Rötzer, T. (2023). Towards sustainable management of the stock and ecosystem services of urban trees. From theory to model and application. Trees 37, 177–196. doi: 10.1007/s00468-021-02100-3
Raupp, M. J., Buckelew-Cumming, A., and Raupp, E. C. (2006). Street tree diversity in eastern North America and its potential for tree loss to exotic borers. Arboric. Urban For. 32, 297–304. doi: 10.48044/jauf.2006.038
Richards, N. A. (1983). Diversity and stability in a street tree population. Urban Ecol. 7, 159–171. doi: 10.1016/0304-4009(83)90034-7
Rötzer, T., Rahman, M. A., Moser-Reischl, A., Pauleit, S., and Pretzsch, H. (2019). Process based simulation of tree growth and ecosystem services of urban trees under present and future climate conditions. Sci. Total Environ. 676, 651–664. doi: 10.1016/j.scitotenv.2019.04.235
Ruokavirasto (2022). Vantaan aasianrunkojääräesiintymä 2015–2020. Available online at: https://www.ruokavirasto.fi/kasvit/kasvitaudit-ja-tuholaiset/kasvintuhoojahaku/karanteenituhoojat/aasianrunkojaara/vantaan-esiintyma/ (accessed May 20, 2023).
Salo, I. (2021). “Evaluation of ecosystem services of trees of Kupittaanpuisto using i-Tree Eco,” (master's thesis). University of Helsinki, Helsinki, Finland.
Santamour, F. S. (1990). “Trees for urban planting: diversity, uniformity, and common sense,” in Trees for the Nineties: Landscape Tree Selection, Testing, Evaluation, and Introduction (Lisle, IL: U.S. The Morton Arboretum), p. 57–65.
Sjöman, H., and Östberg, J. (2019). Vulnerability of ten major Nordic cities to potential tree losses caused by longhorned beetles. Urban Ecosyst. 22, 385–395. doi: 10.1007/s11252-019-0824-8
Sjöman, H., Östberg, J., and Nilsson, J. (2014). Review of host trees for the wood-boring pests Anoplophora glabripennis and Anoplophora chinensis: an urban forest perspective. Arboric. Urban For. 40, 143–164. doi: 10.48044/jauf.2014.016
Smiley, E. T., Kielbaso, J. J., and Proffer, T. J. (1986). Maple disease epidemic in southeastern Michigan. J. Arboric. 12, 126–128. doi: 10.48044/jauf.1986.027
Sousa-Silva, R., Duflos, M., Ordonez Barona, C., and Paquette, A. (2023). Keys to better planning and integrating urban tree planting initiatives. Landscape Urban Plan. 231, 104649. doi: 10.1016/j.landurbplan.2022.104649
Steenberg, J. W. N., Millward, A. A., Nowak, D. J., Robinson, P. J., and Ellis, A. (2017). Forecasting urban forest ecosystem structure, function, and vulnerability. Environ. Manage. 59, 373–392. doi: 10.1007/s00267-016-0782-3
Treglia, M. L., Acosta-Morel, M., Crabtree, D., Galbo, K., Lin-Moges, T., Van Slooten, A., et al. (2021). The State of the Urban Forest in New York City. The Nature Conservancy. doi: 10.5281/zenodo.5532876
Tubby, K. V., and Webber, J. F. (2010). Pests and diseases threatening urban trees under a changing climate. Forestry 83, 451–459. doi: 10.1093/forestry/cpq027
Turner-Skoff, J. B., and Cavender, N. (2019). The benefits of trees for livable and sustainable communities. Plants People Planet 1, 323–335. doi: 10.1002/ppp3.39
Turun väestökatsaus (2020). Available online at: https://www.turku.fi/sites/default/files/atoms/files//vaestokatsaus_2_2020.pdf (accessed October 4, 2022).
UN-Habitat (2022). World Cities Report 2022: Envisaging the Future of Cities. Nairobi: United Nations Human Settlements Programme.
van der Gaag, D. J., and Loomans, A. J. M. (2014). Host plants of Anoplophora glabripennis: a review. EPPO Bull. 44, 518–528. doi: 10.1111/epp.12151
Wang, X., Yao, J., Yu, S., Miao, C., Chen, W., and He, X. (2018). Street trees in a Chinese forest city: structure, benefits and costs. Sustainability 10, 674. doi: 10.3390/su10030674
Wattenhofer, D. J., and Johnson, G. R. (2021). Understanding why young urban trees die can improve future success. Urban Forest. Urban Green. 64, 127247. doi: 10.1016/j.ufug.2021.127247
Zhou, Y., Ge, X., Zou, Y., Guo, S., Wang, T., and Zong, S. (2021). Prediction of the potential global distribution of the Asian longhorned beetle Anoplophora glabripennis (Coleoptera: Cerambycidae) under climate change. Agr. Forest Entomol. 23, 557–568. doi: 10.1111/afe.12461
Keywords: air pollutant, Asian longhorned beetle, carbon sequestration, carbon storage, green infrastructure, i-Tree, planting rate, urban planning
Citation: Mänttäri MM, Lindén L and Tuhkanen E-M (2023) Change in urban forest age structure affects the value of ecosystem services provided. Front. Sustain. Cities 5:1265610. doi: 10.3389/frsc.2023.1265610
Received: 23 July 2023; Accepted: 09 October 2023;
Published: 07 November 2023.
Edited by:
Wenjie Wang, Northeast Forestry University, ChinaReviewed by:
Rongfang Lyu, Lanzhou University, ChinaYaser Abunnasr, American University of Beirut, Lebanon
Copyright © 2023 Mänttäri, Lindén and Tuhkanen. This is an open-access article distributed under the terms of the Creative Commons Attribution License (CC BY). The use, distribution or reproduction in other forums is permitted, provided the original author(s) and the copyright owner(s) are credited and that the original publication in this journal is cited, in accordance with accepted academic practice. No use, distribution or reproduction is permitted which does not comply with these terms.
*Correspondence: Miia M. Mänttäri, miia.manttari@helsinki.fi