- 1College of Horticulture and Landscape Architecture, Zhongkai University of Agriculture and Engineering, Guangzhou, China
- 2Key Laboratory of Aquatic Botany and Watershed Ecology, Wuhan Botanical Garden, Chinese Academy of Sciences, Wuhan, China
The input of dissolved organic carbon (DOC) into soil affects soil organic carbon mineralization and microbial community composition by changing carbon availability. However, up to now, there is little knowledge about the microbial groups that utilize the added DOC and how the incorporation process may vary over time. In this study, we added 13C-labeled litter-derived DOC (treatment) or pure water (control) to a forest soil from different layers to investigate the effects of DOC addition on soil microbial biomass and community composition in a 180-d laboratory incubation experiment. Soil microbial phospholipid fatty acid (PLFA) were measured to assess changes in the microbial community composition. The 13C incorporation into microbial biomass and PLFAs was analyzed to trace the microbial utilization of litter-derived DOC. Our results indicated that DOC addition increased the biomass of gram-negative bacteria, gram-positive bacteria, fungi, and actinomycetes, but the microbial community composition manifested a similar trend for both treatment and control soils at the end of incubation. Proportions of added DOC in different depths of soil microbial PLFAs had no significant difference. Moreover, 17:0 cy and 15:0 PLFAs which are described as the bacterial biomarkers had a greater amount of 13C incorporation than other PLFAs for the topsoil, which indicated that 13C-labeled litter-derived DOC was more easily assimilated by some specific bacterial community. Soil microbial biomass and the incorporation of 13C into PLFA reached its maximum around 30 days after DOC addition and then rapidly reduced to the level comparable to control. Overall, this study demonstrated that the incorporation of 13C-labeled litter-derived DOC into PLFA in different depth soil had no significant difference, and the incorporation of 13C by bacteria was higher than other microbial groups.
Introduction
The soil organic carbon (SOC) pool is the biggest carbon pool in terrestrial ecosystems (1), which is three times larger than the atmospheric carbon pool (2). Plant materials, such as litter, dead roots, or root exudates, are major sources of soil organic matter in terrestrial ecosystems (3–5). The input of fresh organic matter could modify SOC dynamics (6–8), causing long-time variations in soil C pool and ecosystem carbon balances (9). Moreover, SOC turnover and dynamics was controlled by soil microbial community. Many studies have found that plant-derived DOC can stimulate the soil carbon mineralization (10–12), but we know little about the changes in the microbial community.
The addition of C substrates of varying quantity and quality could have different influences on soil microbial community. However, most researches focused on the effect of simple organic substances (e.g., glucose, formate, fructose, oxalic acid, and catechol) inputs on soil microbial community (13–16). As a key natural component of forest carbon cycle, plant-derived DOC is more complex than simple organic substances and includes a rapidly decomposable fraction and a slowly decomposable fraction (17, 18), and the addition of litter-derived DOC might affect the microbial biomass and extracellular enzyme activity as well as causing a possible shift in the microbial community composition (19). Previous studies proved that glucose addition caused an increase in microbial biomass in 1–2 days (20, 21), while litter addition caused the maximum increase of microbial biomass in a few months (22, 23). Recent research showed that the proportion of added litter-derived C entering soil microbial groups nearly disappeared after 18 months (1–2 years), indicating little long-term utilization of litter-derived C by soil microorganisms (16). Thus, compared to the effects of simple organic matter input into the soil, the addition of plant-derived DOC into soils may have different effects. Therefore, it is necessary to investigate the effect of plant-derived DOC on soil microbial communities.
Different microbial groups [e.g., fungi, gram-positive (GP), and gram-negative (GN) bacteria] have different effects on SOC decomposition (24–26). Previous studies considered that bacteria prefer to use rapidly decomposable substances, while fungi prefer to degrade recalcitrant compounds present in SOM by producing a wide range of enzymes and can connect labile C with stable SOM via transport through their hyphae (27). But more recent studies have proven a high fungal use of labile C (28). It is widely accepted that microbial growth is stimulated only by the addition of easily available organic substances, which may consequently affect the mineralization of SOC (29, 30) and change the C flow within the soil microbial community (31–33). Many studies have proved that 13C-phospholipid fatty acid analysis can trace C flow from 13C-labeled substrates into soil microbial community. For example, some experiments found that 16:0 and 18:1ω9c PLFAs which are described as the bacterial biomarkers, had greater amounts of 13C incorporation than other PLFAs with the addition of leaf-litter in subtropical forest soils (34, 35), and other studies showed that 13C-glucose was incorporated into bacterial PLFAs first, especially in GP bacteria (36). However, our knowledge on which groups of soil microbes utilize the natural complex plant-derived DOC is very limited.
Carbon stored in deep soils (below 20–300 cm) is three times more than the C stored in the top 20 cm (37). Meanwhile, the deep SOC is more stable than topsoil SOC (38–40). Microbial community structure and activity in deep soils are different from that in surface soils (41, 42). Thus, the effects of fresh organic matter input into deep soil could have different effects on carbon mineralization on that in surface soil. A few studies have shown that the input of fresh organic matter into deep soil could have stronger effects on carbon dynamics than in surface soil (21, 22, 43). But few studies have investigated the effects of plant-derived DOC addition on deep soil microbial communities.
In this study, we added 13C-labeled plant-derived DOC (treatment) or pure water (control) to soil samples of different depths to determine the 13C incorporation into the PLFA of different microbial groups. We hypothesized that (1) the biomass of bacteria increased with the addition of DOC and the proportion of 13C in bacteria was higher than other soil microbial communities owing to their preference for rapidly decomposable substances; (2) the effect of DOC addition on the incorporation of 13C into PLFA of different soil microbial groups was different with the simple organic matter addition.
Materials and Methods
Soil Collection and Laboratory Incubation
Soils were collected from a deciduous, humid, mountainous forest dominated by Fagus lucida in the Badagongshan National Nature Reserve, Hunan Province, in the mid-subtropical zone of China (29°46.04′N, 110°5.24′E). The climate is subtropical mountain humid monsoon with annual average precipitation of 2,105 mm and an annual mean temperature of 11.5°C. The soil was classified as a Hapludalf with a silt loam texture. We dug three trenches and used a shovel to collect soil samples at three fixed depths: 0–10 cm (topsoil), 10–30 cm (midsoil), and 30–60 cm (subsoil). Sieved soils were refilled into 10 cm diameter PVC tubes as soil columns with thicknesses of 10, 30, and 60 cm (Supplementary Figure 1). The soils were separately refilled as three layers of 0–10, 10–30, and 30–60 cm. The bottoms of the PVC tubes were covered with a nylon net to avoid soil loss while allowing liquid to pass through. Each soil layer was also separated with this nylon net. There were 36 replicate PVC tubes for each type of soil column. After pre-incubation, 13C-labeled litter-derived DOC was added into half of the PVC tubes by an intravenous tubing as the treatment, and the other half of the PVC tubes received distilled water as the control. Litter-derived DOC was produced by the 13C-labeled Fagus lucida leaves and distilled water. The concentration and the δ13C value of litter-derived DOC were 71.7 mg L−1 and 318.4‰, respectively. And the proportion of the labile carbon and calcitrant carbon of litter-derived DOC was 46.18 and 53.82%. The specific process of soil sampling, incubation, the preparation and properties of 13C-labeled litter-derived DOC were described in Wang et al. (44). The primary properties of the soil are given in Supplementary Table 1.
Soil Sampling and Lab Analysis
Three replicate PVC tubes of each treatment and each depth were destructively sampled at 10, 20, 30, 60, 90, and 180 days. Each soil sample collected from PVC tubes was divided into two subsamples. One subsample of fresh soil was used to extract soil microbial biomass carbon. Another subsample of fresh soil was freeze-dried for the determination of soil phospholipid fatty acids (PLFAs) and δ13C value of PLFAs. Soil microbial biomass carbon was extracted by the fumigation-extraction method (45). Soil microbial biomass carbon in extracts (the extraction coefficient was 0.45) were measured using a TOC Analyzer (vario TOC, Elemental, Germany).
PLFA Extraction and Analysis
PLFA analysis procedures (soil lipids extraction, phospholipids separation, and methylation) was conducted according to the literature (46). Briefly, 4 g of freeze-dried soil were extracted with chloroform: methanol: citric acid buffer (1: 2: 0.8), and the phospholipids in the extracts were separated after being eluted using methanol on a silica column (Thermo scientific, USA). The phospholipids were subjected to the methanolysis with a 0.2 mol/L KOH, after which the fatty acid methyl esters (FAMEs) were separated on a capillary as chromatograph.Take methyl non-adeconoate 19:0 as internal standards, the FAMEs were analyzed on a capillary gas chromatography-mass spectrometry (GC-MS, Agilent 6850 Series, Agilent Technologies Inc., USA) equipped with a FID detector and using a Agilent 19091B-102 column (25 m × 200 μm × 0.33 μm). The temperature program of the GC-MS system was the initial temperature was 50°C for 1 min, then 12°C min−1 until reaching 180°C where it was held for 2 min, 6°C min−1 until 220°C where it was held for 2 min, 15°C min−1 until 240°C where it was held for 1 min, and further 15°C min−1 until 260°C where it was held for 15 min. The identification and quantification of FAMEs were performed on MIDI system (MIDI Inc., USA). The amounts of total or individual PLFA were calculated based on those of FAMEs (nmol g/1). Each group in the microbial community was described by the sum of corresponding PLFAs. The represent gram-negative bacteria PLFA was by 17:0cy, 19:0cy, 18:1ω7c, 18:1ω9c; the represent gram-positive bacteria PLFA was by i14:0, i15;0, i16:0, a15:0, i17;0, a17:0; the represent bacteria PLFA was by 17:0 cy, 19:0 cy, 18:1ω7c, 18:1ω9c, i14:0, i15;0, i16:0, a15:0, i17;0, a17:0, 14:0,15:0,16:0, 17:0,18:0; the represent fungi PLFA was by 16:1ω5c and 18:2ω6c; and the represent actinomycetes PLFA was by 10Me16:0, 10Me17:0, 10Me18:0 (47–49).
The δ13C values of individual PLFA were determined using gas chromatography-combustion-isotopic ratio mass spectrometry (GC-C-IRMS, Thermo Fisher Flash 2000, USA). Samples were run in splitless mode, injector temperature was 250°C, and helium flow rate 1.5 ml min−1 (Column DB-5, 30 m × 0.25 mm × 0.25 mm, Agilent Technologies, Inc.). The oven temperature was 120°C for 1 min, then increasing 8°C min−1 until reaching 285°C and further 10°C min−1 until 310°C where it was held for 1 min. Peaks were identified by comparing the relative retention times and chromatograms with those from the GC-MS analyses. Due to the different measurement methods, more PLFAs could be detected with GC-MS than GC-C-IRMS (10–20% more), and only those PLFAs which were detected with both methods are presented here. PLFAs were omitted from the analyses if their δ13C values could not be determined due to too small peak area. The peaks were manually integrated three times and the average of the three integrations for δ13C value was used.
Calculation and Statistical Analysis
The relative change in microbial community biomass was calculated using the following equation:
Where PLFAstreatment is the concentration of PLFAs in the soils with litter-derived DOC addition, PLFAscontrol is the concentration of PLFAs in the control soils.
An additional C atom is added to the fatty acid molecule during the methylation step, thus the δ13C values of PLFA were calculated with the following equation (31, 50).
Where NPLFA is the number of C atoms of the PLFA molecule, 13CFAME is the measured δ13C value of the methylated PLFA, and 13CMeOH is the known δ13C value of the methanol used for methylation.
The proportion of litter-derived DOC in each PLFA was determined using a mass balance approach (51).
Where δ13Ctreatment is the δ13C value (‰) of individual PLFA in the soils with litter-derived DOC addition, δ13Ccontrol is the δ13C value (‰) of individual PLFA in the control soils, and δ13Clitter−derived is the δ13C value of litter-derived DOC (‰).
Repeated measures ANOVA was used to assess the relative change of PLFAs in different depth soil during the incubation. Post-hoc multiple comparisons were determined using LSD when ANOVAs were significant. The significance level in all analyses was P < 0.05. Principle Component Analysis (PCA) was conducted in the vegan package in R v 3.3.1 [(52); R Core Team, 2014] to explore the relationship of microbial community in different depth soil and different incubation time. Permutational multivariate analysis of variance (PerMANOVA) was employed in the Past 3 software to explore the influence of temporal trends on the microbial community between treatment and control. Other statistical analyses were performed by IBM SPSS statistics v 21 (1989-2012 International Business Machines Corp., USA). Figures were drawn with OriginPro 2016 (©1991-2015 OriginLab Corporation, USA).
Results
Soil Microbial Biomass Increased Significantly
At the end of incubation, the proportion of the labile carbon of litter-derived DOC decreased from 46.18 to 37.87%, the specific data was showed in our previous study (44). With the decrease of the labile carbon DOC, microbial biomass carbon significant increased during the incubation (Figure 1, F = 141.78, P < 0.01). The microbial biomass carbon in all soil layers increased over time from the beginning of incubation, to a peak at 1,270 μg g−1 soil on day 30, then decreased at the later stage of incubation. The significant difference of microbial biomass carbon between control and treatment was only found on day 30 (topsoil: F = 7.03, P = 0.03; midsoil: F = 1.68, P = 0.04; subsoil: F = 1.71, P = 0.01).
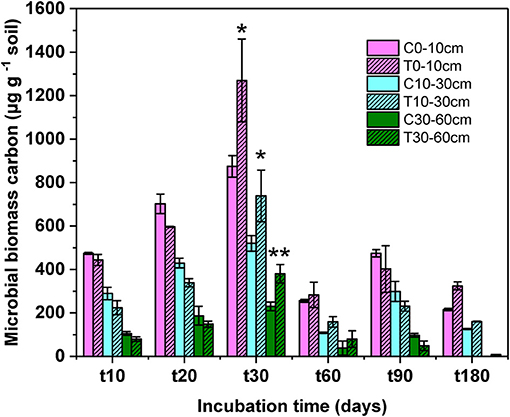
Figure 1. Microbial biomass carbon at different soil depth during the incubation. C, control; T, treatment; t0, t10, t20, t30, t60, and t180 are the destructive sampling time. Values are means ± SD (n = 3). *P < 0.05, **P < 0.01.
PLFAs Had Significant Temporal Change
After DOC addition, total soil microbial carbon, gram-positive bacteria, gram-negative bacteria, other bacteria, fungi, and actinomycetes PLFAs significantly increased from the beginning of incubation to day 30, and then declined to values comparable with the control (Figure 2, Table 1). The biomass of all microbial groups reached its maximum on day 30. The value of increasing rate of soil gram-positive bacteria was larger than other groups of the soil microbial community, but there was no significant difference among them during incubation (Figure 2, Table 2). Although the biomass of fungi and bacteria increased, the ratio of fungi to bacteria biomass was stable during the incubation (Figure 3A, F = 1.56, P > 0.05). The ratio of gram-positive bacteria to gram-negative bacteria biomass in midsoil on day 10 and subsoil on day 20 had an obvious increase, then declined to a control level (Figure 3B).
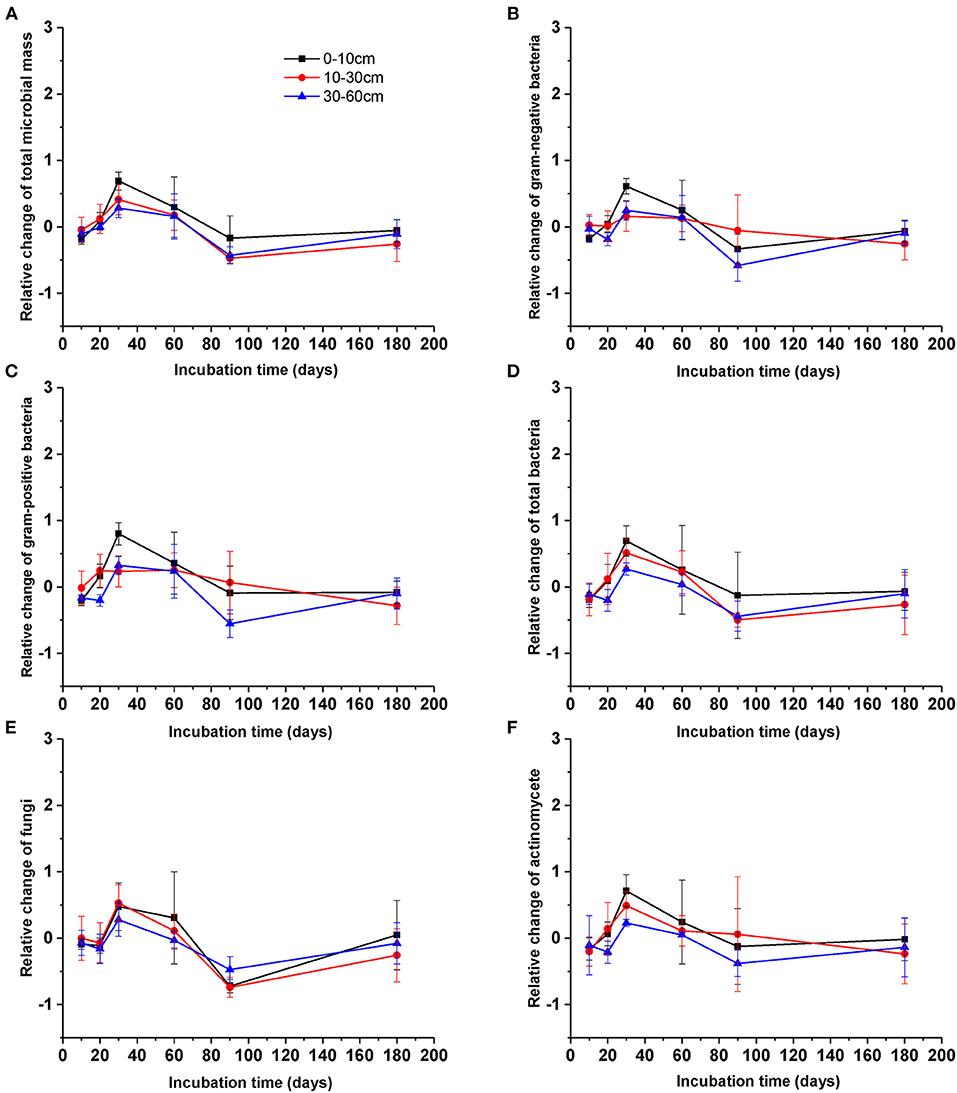
Figure 2. Relative change of different microbial groups [(A) total microbial community, (B) gram-negative bacteria, (C) gram-positive bacteria, (D) bacteria including (A,B), (E) fungi, (F) actinomycetes] during the incubation. Values are means ± SD (n = 3).
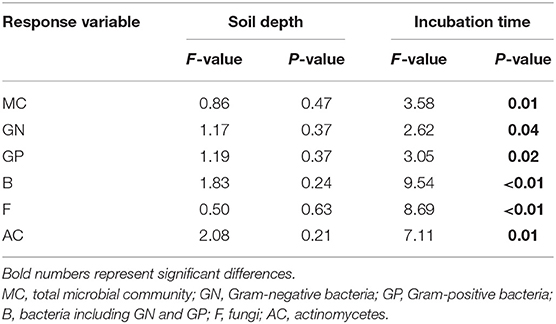
Table 1. Results of ANOVA with repeated measures to test the effects of soil depth and incubation time on the relative change of PLFAs.
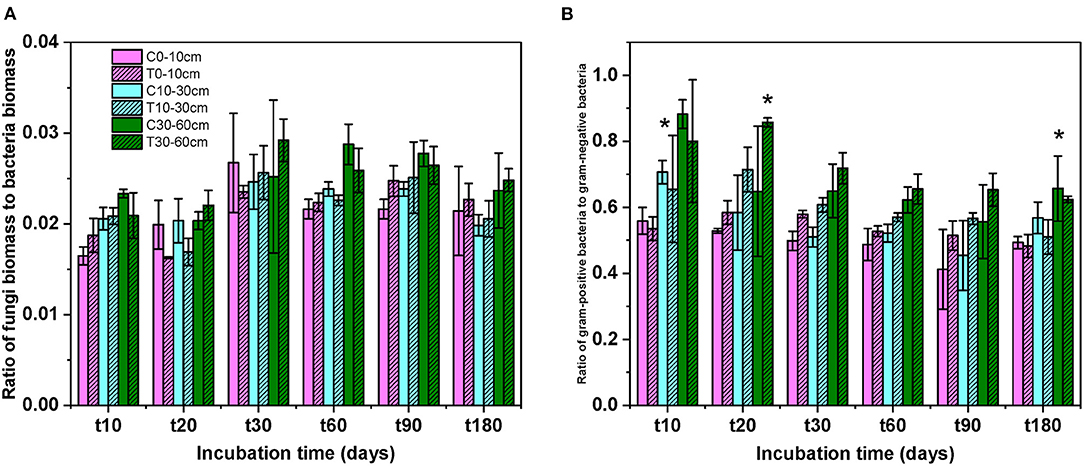
Figure 3. The ratios of fungi to bacteria (A) and gram-positive to gram-negative bacteria (B) at different soil depths during the incubation. C, control; T, treatment; t10, t20, t30, t60, and t180 are the destructive sampling time. Values are mean ± SD (n = 3). *P < 0.05.
Additionally, the principal component analysis (PCA) of the PLFAs data (the normalized responses were used, accounting for 59.15% of the variation) clearly separated three layers of soils (i.e., topsoil, midsoil, and subsoil) (Figure 4). The microbial community of topsoil was dominated by gram-negative bacteria including 19:0 cy, 18:1ω7c, 18:1ω9c whereas the subsoil was dominated by microbial groups including i17;0, cy17:0, a17:0, and 10Me16:0. With the addition of DOC, soil microbial communities in topsoil (Figure 5, Table 3, F = 10.74, P < 0.01) and midsoil (Figure 5, Table 3, F = 3.66, P < 0.01) had a significant change at different incubation time, but the soil microbial communities in treatment and control were similar at the end of incubation (Figure 5, Table 3). This result demonstrated DOC addition only diminished but not changed the direction of the changes in microbial community composition. In the earlier stage of incubation, microbial community was dominated by gram-positive bacteria including i14:0, i15;0, and a15:0, while in the middle and later stage of incubation, 16:1ω5c and 18:2ω6c which are described as the fungi biomarkers and 10Me16:0, 10Me17:0, 10Me18:0 which are described as the actinomycetes biomarkers began to occur (Figure 5). For all horizons of soils, actinomycetes dominance increased at the end of incubation.
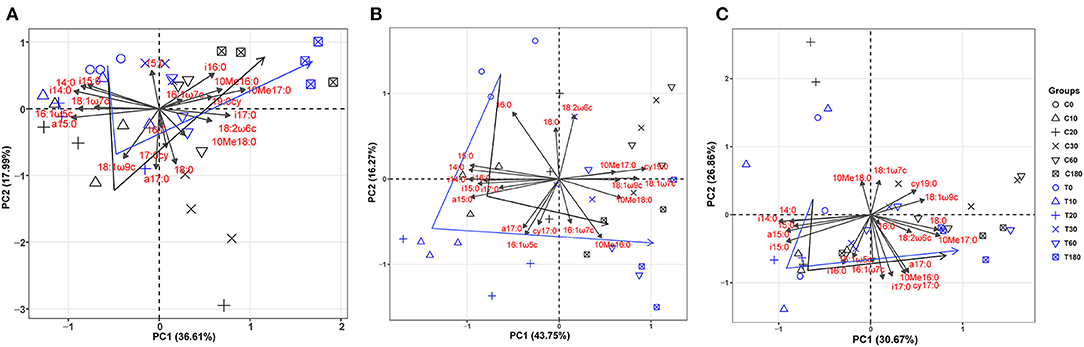
Figure 5. Principal component analysis (PCA) of microbial PLFAs as affected by treatment and incubation time in topsoil (A), midsoil (B), and subsoil (C). The arrows indicate the direction of change in the microbial community. C, control; T, treatment; 0, 10, 20, 30, 60, and 180 are the destructive sampling time. Black line indicates the change of microbial community in control, and blue line indicated the change of microbial community in treatment.
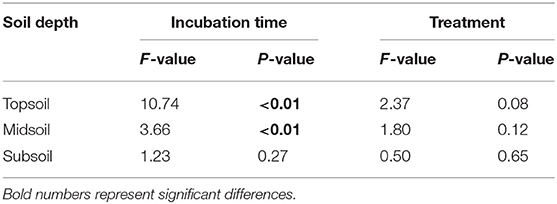
Table 3. Permutational multivariate analysis of variance (PERMANOVA) in soil microbial community composition in different incubation time and treatment.
DOC-C in Microbial PLFAs Had Significant Difference
After the addition of litter-derived DOC, the proportion of litter-derived DOC in soil microbial groups significantly increased in the early stage of incubation and the values reached their maximum on day 30 (Figure 6, F = 17.47, P < 0.01). And the proportion of litter-derived DOC in topsoil bacteria on day 20 an 30 was significantly higher than the other microbial groups (Table 4, day 20: F = 17.58, P < 0.01; day 30: F = 47.75, P < 0.01). After reaching the maximum, the proportions of DOC-C in microbial PLFAs declined, but the values at the end of incubation were also higher than the initial values. However, the proportions of DOC-C in microbial PLFAs were not significantly different among soils from different depths (Figure 6, F = 0.85, P = 0.48).
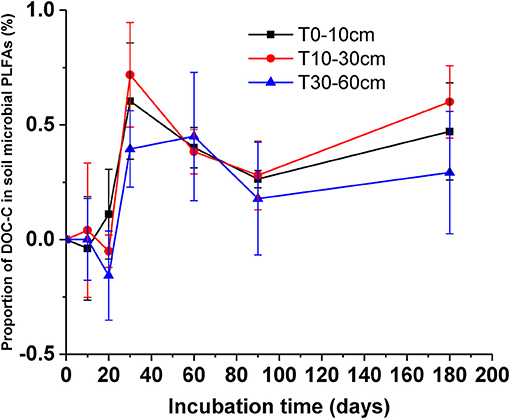
Figure 6. Proportions of added DOC in soil microbial PLFAs for different soils during the incubation. Values are means ± SD (n = 3).

Table 4. Results of ANOVA for the proportion of 13C in different microbial groups during incubation.
Proportions of added DOC in topsoil, midsoil, and subsoil microbial PLFAs indicated that the assimilation of litter-derived DOC varied by different soil microbial groups (Figure 7, Supplementary Table 2). Overall, the assimilated DOC in PLFAs reached its maximum at day 30, especially for topsoil. After reaching the maximum, the assimilated DOC in PLFAs declined over time. For topsoil, litter-derived DOC was mainly incorporated into 17:0cy, i15:0, 15:0, 16:0, and 16:1ω5c, followed by 16:1ω7c, i16:0, i17:0, and a17:0. For midsoil, litter-derived DOC was mainly incorporated into 17:0cy and 15:0, followed by 10Me17:1ω7c and 10Me18:0. For subsoil, litter-derived DOC was mainly incorporated into 10Me18:0, followed by 16:1ω7c, 17:0cy, i17:0, a17:0, 16:0, and 10Me16:0.
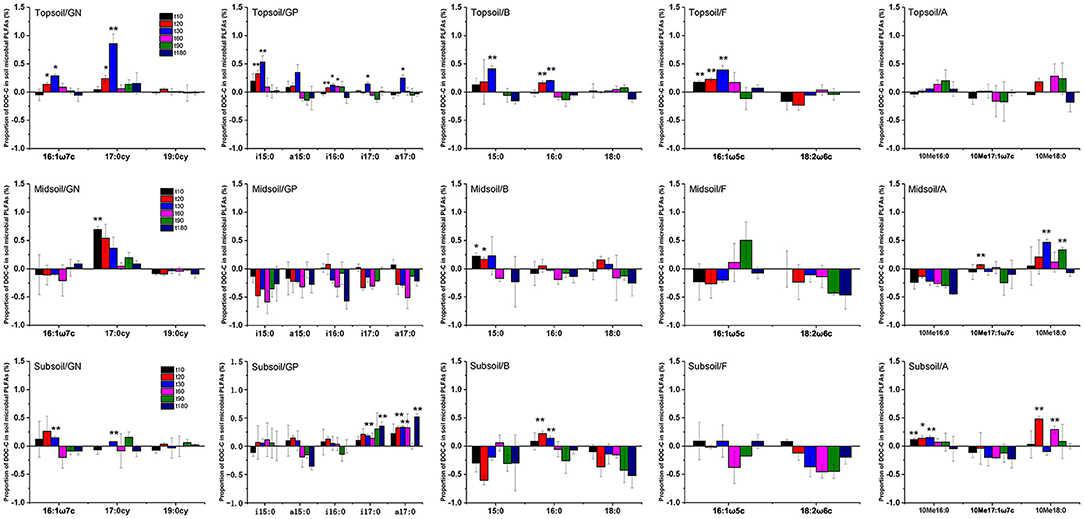
Figure 7. Proportion of DOC-C in soil microbial PLFAs (GN, Gram-negative bacteria; GP, Gram-positive bacteria; B, bacteria other than GN and GP; F, fungi; A, actinomycetes) for topsoil, midsoil, and subsoil during the incubation. t10, t20, t30, t60, t90, and t180 are the destructive sampling time. Values are means ± SE (n = 3). *P < 0.05, **P < 0.01, which indicated there were significant differences between initial and other times.
Discussion
The addition of labile carbon into soil increase microbial biomass and activity, then affect microbial community structure (15, 32, 53, 54). Our incubation experiment confirmed that soil microbial biomass of different depths significantly increased after DOC addition, and reached the maximum around day 30 (Figure 2). These results suggest that the addition of DOC provided carbon sources for microbes and promoted their growth, thus increased their biomass (11, 35). However, the duration of the effect of DOC addition on microbial biomass was different from other carbon sources. For example, the addition of glucose may cause an increase in microbial biomass in 1–2 days (20, 21), while with the addition of litter, microbial biomass usually reaches its maximum after a few months (22, 23). The observed duration and magnitude of the increase of microbial biomass probably depend on the quality and quantity of added C. Previous studies found that if the quantity of addition active carbon was less than native soil microbial biomass C, it only increased microbial activity but did not cause the increase of microbial biomass (55). But in our study, the quantity of added DOC is about 10% of soil microbial biomass C, soil microbial biomass nearly increased 1/3 on day 30. The possible reason is that the composition of DOC is more complex and could meet the needs of more diverse microbial groups, thus it had a bigger effect on microbial growth (10, 56, 57).
Microbial community composition changed significantly during the incubation with the addition of DOC. Previous studies had shown that bacteria and fungi had different responses to exogenous carbon addition, bacteria prefer to use simple compounds while fungi and actinobacteria prefer to use complex compounds (58–61). Thus, at the early stage of incubation, the 13C incorporation into bacteria was higher than that of fungi and actinobacteria (Table 4, Figure 7). But at the later incubation stages, the 13C incorporation into fungi and actinobacteria was might come from the necromass of microorganisms that took up the label before. Previous studies demonstrated that both fungi and actinomycetes are well-known to “recycle” carbon from microbial necromass (28). Therefore, litter-derived DOC may be utilized by bacteria, fungi, or actinomycetes, then cause a shift of microbial community structure.
Among the microbial groups, the gram-positive bacteria showed the biggest relative change rate (Figure 2). This indicated that added DOC had a stronger effect on gram-positive bacteria, which was probably due to the different cell wall composition between gram-positive bacteria and gram-negative bacteria (42, 50). Although DOC addition caused transient changes in microbial community composition, the microbial community compositions of the treatment and control showed a similar temporal trend during the incubation (Figure 5). This revealed that DOC addition only temporally changed the relative composition of different soil microbial groups, but did not alter the general trend of the shifting of microbial community composition. In the early stages of incubation, DOC addition provided C energy for microbes and then caused the transformation of microbial community. But with the depletion of DOC during the incubation, this effect faded away, the structure of microbial community in treatment at the end of incubation was similar to that of the control. These results indicated that the addition of DOC had a short-time effect to transform soil microbial community composition. Microbial community composition in different soil depths had similar responses to DOC addition (Figure 5).
According to the proportion of DOC-C in soil microbial PLFAs, we found that the order of the utilization DOC-C by microorganisms was different among soil layers. In topsoil, the DOC-C was used by both bacteria and fungi, and the δ13C of bacteria had a significant increase on day 20 and up to the maximum on day 30. Actinomycetes did not use DOC-C during the whole incubation experiment. In midsoil and subsoil, the δ13C of fungi had no significant change during incubation, but actinomycetes had a 13C enrichment (Figure 7). Therefore, the added DOC in surface soil was mainly used by fungi and bacteria, while in the middle and deep layer, DOC used by actinomycetes was likely re-used 13C that was taken up by bacteria before. Although both bacteria and fungi had assimilated DOC-C, the amount of DOC assimilated into bacteria is higher than fungi (Table 4, Figure 7). And our results showed that 13C in DOC was mainly assimilated by 17:0cy (gram-negative bacteria) and 15:0 (common bacteria) (Figure 7). These results confirmed hypothesis 1 that the proportion of 13C in bacteria was higher than other soil microbial communities. This indicated that DOC is more easily assimilated into bacteria (34–36). However, previous studies indicated that 13C-labeled amino acid mixture was assimilated into the biomarker PLFA of all microbial groups (62). This discrepancy may be due to that plant-derived DOC used in our study was harder to decompose than amino acid mixture. Therefore, compare to previous studies, 13C in DOC was assimilated by different PLFAs, which is consistent with our hypothesis 2 that the effect of DOC addition on the incorporation of 13C into PLFA of different soil microbial groups was different with the simple organic matter addition. At the same time, the proportion of litter-derived DOC in gram-negative bacteria was higher than gram-positive bacteria, which indicated that plant-derived carbon prefers to be assimilated by gram-negative bacteria other than gram-positive bacteria. This may be due to the higher growth rate of gram-negative bacteria than gram-positive bacteria (6, 33), thus gram-negative bacteria have higher efficiency in using DOC than gram-positive bacteria.But some studies showed that the δ13C of gram-negative bacteria, gram-positive bacteria, and fungi were similar in assimilating carbon sources (63). In addition, the assimilation effect of microorganisms on DOC was underestimated to a certain extent owing to some PLFAs omitted from the analyses if their δ13C values could not be determined due to too small peak area. With regard to these inconsistent findings, how soil microbial community respond to DOC addition in forest soils still needs further study.
Conclusion
In conclusion, soil microbial biomass increased with DOC addition and the peak of the biomass was occurred on day 30. Although DOC addition increased soil microbial biomass and temporally shifted microbial community structure, the overall microbial community structure at the end of incubation was similar for both treatment and control soils. These indicated that a small amount of DOC input only had a transient effect on soil microbial community composition. Litter-derived DOC could be assimilated by different groups of soil microbes for soils of different depths. But the overall proportions of litter-derived DOC-C in soil microbial community composition were not significantly different among depths. The proportions of litter-derived DOC-C in soil microbes reached their maximum on day 30 and then declined over time during the incubation. A higher amount of DOC was incorporated into the 17:0cy and 15:0 PLFAs suggests that bacteria played a greater role in DOC cycling. These results improved our understanding of how DOC may affect different depth soil microbial community composition structure. However, these findings were obtained from a laboratory incubation experiment, in which soil columns and environmental conditions are quite different from the field. So, more studies should be conducted in situ to gain a more accurate and complete understanding of the issue.
Data Availability Statement
The original contributions presented in the study are included in the article/Supplementary Material, further inquiries can be directed to the corresponding author/s.
Author Contributions
MW: conceptualization, methodology, and writing—original draft. QT: methodology and validation. CL and RZ: investigation. FL: supervision and writing—review and editing. All authors contributed to the article and approved the submitted version.
Funding
This study was supported by the National Natural Sciences Foundation of China (31400463, 31470526).
Conflict of Interest
The authors declare that the research was conducted in the absence of any commercial or financial relationships that could be construed as a potential conflict of interest.
Publisher's Note
All claims expressed in this article are solely those of the authors and do not necessarily represent those of their affiliated organizations, or those of the publisher, the editors and the reviewers. Any product that may be evaluated in this article, or claim that may be made by its manufacturer, is not guaranteed or endorsed by the publisher.
Acknowledgments
We thank the Badagongshan Nature Reserve for their logistic supports during the field trips.
Supplementary Material
The Supplementary Material for this article can be found online at: https://www.frontiersin.org/articles/10.3389/fsoil.2021.733431/full#supplementary-material
References
1. Batjes NH, Sombroek WG. Possibilities for carbon sequestration in tropical and subtropical soils. Glob Chang Biol. (1997) 3:161–73. doi: 10.1046/j.1365-2486.1997.00062.x
2. Schlesinger WH, Andrews JA. Soil respiration and the global carbon cycle. Biogeochemistry. (2000) 48:7–20. doi: 10.1023/A:1006247623877
3. Kramer C, Trumbore S, Froeberg M, Dozal LMC, Zhang D, Xu X, et al. Recent (<4 year old) leaf litter is not a major source of microbial carbon in a temperate forest mineral soil. Soil Biol Biochem. (2010) 42:1028–37. doi: 10.1016/j.soilbio.2010.02.021
4. Zhu B, Cheng W. Rhizosphere priming effect increases the temperature sensitivity of soil organic matter decomposition. Glob Chang Biol. (2011) 17:2172–83. doi: 10.1111/j.1365-2486.2010.02354.x
5. Yuan Q, Hernandez M, Dumont MG, Rui J, Scavino AF, Conrad R. Soil bacterial community mediates the effect of plant material on methanogenic decomposition of soil organic matter. Soil Biol Biochem. (2018) 116:99–109. doi: 10.1016/j.soilbio.2017.10.004
6. Kuzyakov Y, Friedel J, Stahr K. Review of mechanisms and quantification of priming effects. Soil Biol Biochem. (2000) 32:1485–98. doi: 10.1016/S0038-0717(00)00084-5
7. Guenet B, Neill C, Bardoux G, Abbadie L. Is there a linear relationship between priming effect intensity and the amount of organic matter input? Appl Soil Ecol. (2010) 46:436–42. doi: 10.1016/j.apsoil.2010.09.006
8. Paterson E, Sim A. Soil-specific response functions of organic matter mineralization to the availability of labile carbon. Glob Chang Biol. (2013) 19:1562–71. doi: 10.1111/gcb.12140
9. Wieder WR, Bonan GB, Allison SD. Global soil carbon projections are improved by modelling microbial processes. Nat Clim Chang. (2013) 3:909–12. doi: 10.1038/nclimate1951
10. Di Lonardo DP, De Boer W, Gunnewiek PJAK, Hannula SE, Van der Wal A. Priming of soil organic matter: chemical structure of added compounds is more important than the energy content. Soil Biol Biochem. (2017) 108:41–54. doi: 10.1016/j.soilbio.2017.01.017
11. Liang X, Yuan J, Yang E, Meng J. Responses of soil organic carbon decomposition and microbial community to the addition of plant residues with different C:N ratio. Eur J Soil Biol. (2017) 82:50–5. doi: 10.1016/j.ejsobi.2017.08.005
12. Miao S, Ye R, Qiao Y, Zhu-Barker X, Doane TA, Horwath WR. The solubility of carbon inputs affects the priming of soil organic matter. Plant Soil. (2017) 410:129–38. doi: 10.1007/s11104-016-2991-1
13. Hamer U, Marschner B. Priming effects of sugars, amino acids, organic acids and catechol on the mineralization of lignin and peat. J Plant Nutr Soil Sci. (2002) 165:261–8. doi: 10.1002/1522-2624(200206)165:3<261::AID-JPLN261>3.0.CO;2-I
14. Hamer U, Marschner B. Priming effects in different soil types induced by fructose, alanine, oxalic acid and catechol additions. Soil Biol Biochem. (2005) 37:445–54. doi: 10.1016/j.soilbio.2004.07.037
15. Nottingham AT, Griffiths H, Chamberlain PM, Stott AW, Tanner EVJ. Soil priming by sugar and leaf-litter substrates: a link to microbial groups. Appl Soil Ecol. (2009) 42:183–90. doi: 10.1016/j.apsoil.2009.03.003
16. Preusser S, Liebmann P, Stucke A, Wirsching J, Müller K, Mikutta R, et al. Microbial utilisation of aboveground litter-derived organic carbon within a sandy dystric cambisol profile. Front Soil Sci. (2021) 1:666950. doi: 10.3389/fsoil.2021.666950
17. Kalbitz K, Schmerwitz J, Schwesig D, Matzner E. Biodegradation of soil-derived dissolved organic matter as related to its properties. Geoderma. (2003) 113:273–91. doi: 10.1016/S0016-7061(02)00365-8
18. Scott EE, Rothstein DE. The dynamic exchange of dissolved organic matter percolating through six diverse soils. Soil Biol Biochem. (2014) 69:83–92. doi: 10.1016/j.soilbio.2013.10.052
19. Qiu Q, Wu L, Ouyang Z, Li B, Xu Y. Different effects of plant-derived dissolved organic matter (DOM) and urea on the priming of soil organic carbon. Environ Sci Process Impact. (2016) 18:330–41. doi: 10.1039/C5EM00446B
20. Dungait JAJ, Kemmitt SJ, Michallon L, Guo S, Wen Q, Brookes PC, et al. Variable responses of the soil microbial biomass to trace concentrations of 13C-labelled glucose, using 13C-PLFA analysis. Eur J Soil Sci. (2011) 62:117–26. doi: 10.1111/j.1365-2389.2010.01321.x
21. Tian Q, Yang X, Wang X, Liao C, Li Q, Wang M, et al. Microbial community mediated response of organic carbon mineralization to labile carbon and nitrogen addition in topsoil and subsoil. Biogeochemistry. (2016) 128:125–39. doi: 10.1007/s10533-016-0198-4
22. Wang Q, Wang Y, Wang S, He T, Liu L. Fresh carbon and nitrogen inputs alter organic carbon mineralization and microbial community in forest deep soil layers. Soil Biol Biochem. (2014) 72:145–51. doi: 10.1016/j.soilbio.2014.01.020
23. Xu Y, Sun L, Lal R, Bol R, Wang Y, Gao X, et al. Microbial assimilation dynamics differs but total mineralization from added root and shoot residues is similar in agricultural Alfisols. Soil Biol Biochem. (2020) 148:107901. doi: 10.1016/j.soilbio.2020.107901
24. Blagodatskaya E, Khomyakov N, Myachina O, Bogomolova I, Blagodatsky S, Kuzyakov Y. Microbial interactions affect sources of priming induced by cellulose. Soil Biol Biochem. (2014) 74:39–49. doi: 10.1016/j.soilbio.2014.02.017
25. Creamer CA, de Menezes AB, Krull ES, Sanderman J, Newton-Walters R, Farrell M. Microbial community structure mediates response of soil C decomposition to litter addition and warming. Soil Biol Biochem. (2015) 80:175–88. doi: 10.1016/j.soilbio.2014.10.008
26. Xiao C, Guenet B, Zhou Y, Su J, Janssens IA. Priming of soil organic matter decomposition scales linearly with microbial biomass response to litter input in steppe vegetation. Oikos. (2015) 124:649–57. doi: 10.1111/oik.01728
27. Boberg JB, Nasholm T, Finlay RD, Stenlid J, Lindahl BD. Nitrogen availability affects saprotrophic basidiomycetes decomposing pine needles in a long term laboratory study. Fungal Ecol. (2011) 4:408–16. doi: 10.1016/j.funeco.2011.03.004
28. de Vries FT, Caruso T. Eating from the same plate? Revisiting the role of labile carbon inputs in the soil food web. Soil Biol Biochem. (2016) 102:4–9. doi: 10.1016/j.soilbio.2016.06.023
29. Fontaine S, Mariotti A, Abbadie L. The priming effect of organic matter: a question of microbial competition? Soil Biol Biochem. (2003) 35:837–43. doi: 10.1016/S0038-0717(03)00123-8
30. Fontaine S, Barot S. Size and functional diversity of microbe populations control plant persistence and long-term soil carbon accumulation. Ecol Lett. (2005) 8:1075–87. doi: 10.1111/j.1461-0248.2005.00813.x
31. Williams MA, Myrold DD, Bottomley PJ. Carbon flow from C-13-labeled straw and root residues into the phospholipid fatty acids of a soil microbial community under field conditions. Soil Biol Biochem. (2006) 38:759–68. doi: 10.1016/j.soilbio.2005.07.001
32. Moore-Kucera J, Dick RP. Application of C-13-labeled litter and root materials for in situ decomposition studies using phospholipid fatty acids. Soil Biol Biochem. (2008) 40:2485–93. doi: 10.1016/j.soilbio.2008.06.002
33. Garcia-Pausas J, Paterson E. Microbial community abundance and structure are determinants of soil organic matter mineralisation in the presence of labile carbon. Soil Biol Biochem. (2011) 43:1705–13. doi: 10.1016/j.soilbio.2011.04.016
34. Yao H, Thornton B, Paterson E. Incorporation of C-13-labelled rice rhizodeposition carbon into soil microbial communities under different water status. Soil Biol Biochem. (2012) 53:72–7. doi: 10.1016/j.soilbio.2012.05.006
35. Wang Q, Wang S, He T, Liu L, Wu J. Response of organic carbon mineralization and microbial community to leaf litter and nutrient additions in subtropical forest soils. Soil Biol Biochem. (2014) 71:13–20. doi: 10.1016/j.soilbio.2014.01.004
36. Zhang H, Ding W, Yu H, He X. Carbon uptake by a microbial community during 30-day treatment with C-13-glucose of a sandy loam soil fertilized for 20 years with NPK or compost as determined by a GC-C-IRMS analysis of phospholipid fatty acids. Soil Biol Biochem. (2013) 57:228–36. doi: 10.1016/j.soilbio.2012.08.024
37. Fontaine S, Barot S, Barre P, Bdioui N, Mary B, Rumpel C. Stability of organic carbon in deep soil layers controlled by fresh carbon supply. Nature. (2007) 450:277–80. doi: 10.1038/nature06275
38. Trumbore S. Age of soil organic matter and soil respiration: radiocarbon constraints on belowground C dynamics. Ecol Appl. (2000) 10:399–411. doi: 10.1890/1051-0761(2000)010[0399:AOSOMA]2.0.CO;2
39. Ewing SA, Sanderman J, Baisden WT, Wang Y, Amundson R. Role of large-scale soil structure in organic carbon turnover: evidence from California grassland soils. J Geophys Res Biogeosci. (2006) 111:G3. doi: 10.1029/2006JG000174
40. Schimel JP, Wetterstedt JAM, Holden PA, Trumbore SE. Drying/rewetting cycles mobilize old C from deep soils from a California annual grassland. Soil Biol Biochem. (2011) 43:1101–3. doi: 10.1016/j.soilbio.2011.01.008
41. Fierer N, Allen AS, Schimel JP, Holden PA. Controls on microbial CO2 production: a comparison of surface and subsurface soil horizons. Glob Chang Biol. (2003) 9:1322–32. doi: 10.1046/j.1365-2486.2003.00663.x
42. Kramer C, Gleixner G. Soil organic matter in soil depth profiles: distinct carbon preferences of microbial groups during carbon transformation. Soil Biol Biochem. (2008) 40:425–33. doi: 10.1016/j.soilbio.2007.09.016
43. Karhu K, Hilasvuori E, Fritze H, Biasi C, Nykänen H, Liski J, et al. Priming effect increases with depth in a boreal forest soil. Soil Biol Biochem. (2016) 99:104–7. doi: 10.1016/j.soilbio.2016.05.001
44. Wang M, Tian Q, Liao C, Zhao R, Wang D, Wu Y, et al. The fate of litter-derived dissolved organic carbon in forest soils: results from an incubation experiment. Biogeochemistry. (2019) 144:133–47. doi: 10.1007/s10533-019-00576-3
45. Vance ED, Brookes PC, Jenkinson DS. An extraction method for measuring soil microbial biomass C. Soil Biol Biochem. (1987) 19:703–7. doi: 10.1016/0038-0717(87)90052-6
46. Luo X, Fu X, Yang Y, Cai P, Peng S, Chen W, et al. Microbial communities play important roles in modulating paddy soil fertility. Sci Rep. (2016) 6:srep20326. doi: 10.1038/srep20326
47. Bossio DA, Scow KM. Impacts of carbon and flooding on soil microbial communities: phospholipid fatty acid profiles and substrate utilization patterns. Microb Ecol. (1998) 35:265–78. doi: 10.1007/s002489900082
48. Frostegard A, Tunlid A, Baath E. Use and misuse of PLFA measurements in soils. Soil Biol Biochem. (2011) 43:1621–5. doi: 10.1016/j.soilbio.2010.11.021
49. Bååth E. The use of neutral lipid fatty acids to indicate the physiological conditions of soil fungi. Microb Ecol. (2003) 45:373–83. doi: 10.1007/s00248-003-2002-y
50. Tavi NM, Martikainen PJ, Lokko K, Kontro M, Wild B, Richter A, et al. Linking microbial community structure and allocation of plant-derived carbon in an organic agricultural soil using (CO2)-C-13 pulse-chase labelling combined with C-13-PLFA profiling. Soil Biol Biochem. (2013) 58:207–15. doi: 10.1016/j.soilbio.2012.11.013
51. Phillips DL, Gregg JW. Source partitioning using stable isotopes: coping with too many sources. Oecologia. (2003) 136:261–9. doi: 10.1007/s00442-003-1218-3
52. Oksanen J, Blanchet GF, Friendly M, Kindt R, Legendre P, McGlinn D, et al. vegan: Community Ecology Package. R Package Version 2.4-2. (2017). Available online at: https://github.com/vegandevs/vegan
53. Waldrop MP, Zak DR, Sinsabaugh RL. Microbial community response to nitrogen deposition in northern forest ecosystems. Soil Biol Biochem. (2004) 36:1443–51. doi: 10.1016/j.soilbio.2004.04.023
54. Wang Q, Liu S, Wang S. Debris manipulation alters soil CO2 efflux in a subtropical plantation forest. Geoderma. (2013) 192:316–22. doi: 10.1016/j.geoderma.2012.06.027
55. Blagodatskaya E, Kuzyakov Y. Mechanisms of real and apparent priming effects and their dependence on soil microbial biomass and community structure: critical review. Biol Fertil Soils. (2008) 45:115–31. doi: 10.1007/s00374-008-0334-y
56. Kalbitz K, Solinger S, Park JH, Michalzik B, Matzner E. Controls on the dynamics of dissolved organic matter in soils: a review. Soil Sci. (2000) 165:277–304. doi: 10.1097/00010694-200004000-00001
57. Kindler R, Siemens J, Kaiser K, Walmsley DC, Bernhofer C, Buchmann N, et al. Dissolved carbon leaching from soil is a crucial component of the net ecosystem carbon balance. Glob Chang Biol. (2011) 17:1167–85. doi: 10.1111/j.1365-2486.2010.02282.x
58. Rousk J, Baath E. Fungal and bacterial growth in soil with plant materials of different C/N ratios. FEMS Microbiol Ecol. (2007) 62:258–67. doi: 10.1111/j.1574-6941.2007.00398.x
59. Meidute S, Demoling F, Baath E. Antagonistic and synergistic effects of fungal and bacterial growth in soil after adding different carbon and nitrogen sources. Soil Biol Biochem. (2008) 40:2334–43. doi: 10.1016/j.soilbio.2008.05.011
60. McCarthy AJ, Williams ST. Actinomycetes as agents of biodegradation in the environment - a review. Gene. (1992) 115:189–92. doi: 10.1016/0378-1119(92)90558-7
61. Jiang X, Denef K, Stewart CE, Cotrufo MF. Controls and dynamics of biochar decomposition and soil microbial abundance, composition, and carbon use efficiency during long-term biochar-amended soil incubations. Biol Fertil Soils. (2016) 52:1–14. doi: 10.1007/s00374-015-1047-7
62. Dungait JAJ, Kemmitt SJ, Michallon L, Guo S, Wen Q, Brookes PC, et al. The variable response of soil microorganisms to trace concentrations of low molecular weight organic substrates of increasing complexity. Soil Biol Biochem. (2013) 64:57–64. doi: 10.1016/j.soilbio.2013.03.036
Keywords: carbon isotopes, dissolved organic carbon, microbial community, phospholipid fatty acids, soil layer
Citation: Wang M, Tian Q, Liao C, Zhao R and Liu F (2021) Effect of Litter-Derived Dissolved Organic Carbon Addition on Forest Soil Microbial Community Composition. Front. Soil Sci. 1:733431. doi: 10.3389/fsoil.2021.733431
Received: 30 June 2021; Accepted: 24 August 2021;
Published: 20 September 2021.
Edited by:
Haijian Bing, Institute of Mountain Hazards and Environment, Chinese Academy of Sciences (CAS), ChinaReviewed by:
Jipeng Wang, Chengdu University of Technology, ChinaJiabao Li, Chengdu Institute of Biology, Chinese Academy of Sciences, China
Copyright © 2021 Wang, Tian, Liao, Zhao and Liu. This is an open-access article distributed under the terms of the Creative Commons Attribution License (CC BY). The use, distribution or reproduction in other forums is permitted, provided the original author(s) and the copyright owner(s) are credited and that the original publication in this journal is cited, in accordance with accepted academic practice. No use, distribution or reproduction is permitted which does not comply with these terms.
*Correspondence: Feng Liu, bGl1ZmVuZ0B3YmdjYXMuY24=