- 1Department of Forestry and Wildlife Management, Faculty of Applied Ecology, Agricultural Sciences and Biotechnology, Inland Norway University of Applied Sciences, Koppang, Norway
- 2Center for Zoo and Wild Animal Health, Copenhagen Zoo, Frederiksberg, Denmark
- 3ALS Scandinavia AB, Luleå University of Technology, Luleå, Sweden
- 4Department of Wildlife, Fish and Environmental Studies, Faculty of Forest Sciences, Swedish University of Agricultural Sciences, Umeå, Sweden
Lead (Pb) exposure is associated with adverse health effects in both humans and wildlife. Blood lead levels (BLL) of sentinel wildlife species can be used to monitor environmental lead exposure and ecosystem health. BLL analyzers, such as the LeadCare®, are validated for use in humans, assessed for use in some avian species and cattle, and are increasingly being used on wildlife to monitor lead exposure. The LeadCare® analyzers use a technique called anodic stripping voltammetry (ASV). Species-specific conversion equations have been proposed to approximate the levels found with gold standard measuring methods such as inductively coupled plasma mass spectrometry (ICP-MS) because the ASV method has been shown to underestimate BLL in some species. In this study we assessed the LeadCare® Plus (LCP) for use on Scandinavian brown bears (Ursus arctos). LCP measurements were correlated with ICP-MS with a Bland-Altman analyzed bias of 16.3–22.5%, showing a consistent overestimation of BLL analyzed with LCP. Based on this analysis we provide conversion equations for calculating ICP-MS BLL based on the LCP results in Scandinavian brown bears. Our study shows that the LeadCare® Plus can be used for monitoring of lead exposure by approximating gold standard levels using conversion equations. This enables comparison with other gold standard measured BLL within the observed range of this study (38.20–174.00 μg/L). Our study also found that Scandinavian brown bears are highly exposed to environmental lead.
Introduction
Environmental contamination with and subsequent exposure to lead (Pb) impacts both humans and animals living in a polluted ecosystem. In humans lead has been associated with a wide range of harmful health effects, including reduced IQ (1) and cardiovascular disease (2, 3). The World Health Organization states that there are no safe levels of lead in the body (4) and even low level exposure poses a health risk, especially during developing stages (5). Although the harmful effects of lead exposure in humans are well-documented, these results have yet to be applied in legislation and regulation on all areas of lead usage, e.g., lead-based ammunition for hunting. The European Food Safety Authority (EFSA) has set the blood lead level (BLL) of concern for developmental neurotoxicity in humans to 12 μg/L (6). The level of concern of the American Centers for Disease Control and Prevention (CDC), now termed the “reference level” under the current US administration, is 50 μg/L (7, 8).
Lead is absorbed through ingestion and inhalation (9), with higher absorption from the gastrointestinal tract in children than in adults (10). Lead can be measured in the blood (11). Lead compounds are inorganic, organic or ionic, where organic lead can be metabolized to inorganic and ionic lead (12). Ionic lead exerts similar toxicities as inorganic lead. As a bio accumulative toxicant, 94% of the lead body burden is stored in bone (13). The half-life for inorganic lead is 30 days in blood and 10–30 years in bone (6, 10). The half-life seems to be positively correlated to the length of exposure (14) and varies with age and sex (15).
Lead follows a three-compartment distribution model between blood, soft tissue and bone (11). Lead is excreted via urine and feces as well as in hair, sweat and nails (11). BLL can be used to measure recent exposure, but bone-stored lead can be reabsorbed and become an endogenous source of lead, keeping the blood level elevated. Increased calcium mobilization through bone resorption and concurrent reabsorption of lead to the blood is seen during pregnancy and lactation in humans (16), with highest mobilization of bone stored lead in the postpartum period (17). This causes prenatal and neonatal exposure through the placenta (18) and nursing in humans (19) and mice (20). The lactose content of the milk promotes calcium absorption in the gut, and thereby also increases lead absorption (21).
The gold standard of lead analysis in blood or tissue is generally accepted to be graphite-furnace atomic absorption spectrometry (GFAAS) or inductively coupled plasma mass spectrometry (ICP-MS) (22). ICP-MS and GFAAS has been determined to be statistically equivalent when measuring BLLs for clinical use and biomonitoring (23, 24). Both methods are expensive and time-consuming and require laboratory involvement. An alternative analytical method that both simplifies the process and lowers the cost of determining BLL has been introduced: Anodic stripping voltammetry (ASV) is a relatively new method that uses electrochemistry to measure lead in blood (25).
The LeadCare® analyzers from Magellan Diagnostics Inc. (North Billerica, MA, USA) apply ASV technology. These analyzers are validated for humans (26), some avian species (27, 28) and in cattle (29) by comparison to the GFAAS and/or ICP-MS. Furthermore, ASV has been used in studies investigating lead exposure in grizzly bears (Ursus arctos), black bears (U. americanus), gray wolves (Canis lupus) and cougars (Puma concolor) (30), and multiple avian species (31, 32). The analyzers are portable and require little training to operate. This is beneficial in wildlife research and biomonitoring of lead exposure in the field.
In studies comparing the LeadCare® systems with GFAAS or ICP-MS, the ASV analyzers had a negative bias (i.e., underestimation) when measuring the BLL of avian wildlife (27, 33) and cattle (29). Validation for each species has been proposed to verify the conversion rate when using the LeadCare® to monitor exposure (23). No significant difference was found between the different LeadCare® systems in birds (23). In the present study, the LeadCare® Plus (LCP) was used as it has the lowest detection range of the currently available LeadCare® analyzers.
The objective of this study was to compare LCP and ICP-MS for measurement of BLL in Scandinavian brown bears. Based on previous studies on hunting with lead-based ammunition in Scandinavia (34), the scavenging behavior of Scandinavian brown bears (35) and assessment of the LeadCare® system in other species, we predicted (1) to find detectable levels of lead in blood of some bears and (2) to find that LCP was negatively biased when compared to ICP-MS.
Methods and Materials
The present study was conducted in Dalarna and Gävleborg counties in south-central Sweden (61°N 15°E) and Hedmark County in south-eastern Norway (61°N 18°E) as part of the Scandinavian Brown Bear Research Project (36). Captures, handling and sampling of bears were approved by the Swedish Ethical Committee on Animal Research (Uppsala, Sweden; #C18/15), the Swedish Environmental Protection Agency (Stockholm, Sweden; NV-0758-14), and the Swedish Board of Agriculture (#31-11102/12) and were carried out according to an established protocol (37).
Blood was collected from the jugular vein in 4 ml EDTA tubes using the Vacuette® system (Greiner Bio-One, Kremsmünster, Austria) from 54 bears captured during April-May 2018. The blood samples were analyzed on the LCP according to the protocol for the analyzer, either within 6 h of sampling, or after refrigeration at 4°C for up to 72 h. In addition, 70 frozen samples stored at −20°C for 1 to 8 years (2010: N = 11, 2013: N = 23, 2017: N = 36) were analyzed. The frozen samples were thawed at room temperature and inverted to homogenize the content before being tested on the LCP with the same method as the fresh blood samples.
Fifty microliter whole blood was transferred using a calibrated autopipette (Eppendorf Research® 10–100 μL, pipette tips 10–100 μL, Eppendorf, Hamburg, Germany) into a test vial provided in the test kit for the analyzer. Test vials contain hydrochloric acid solution that breaks down the red blood cells. The vial was inverted 8–10 times, then stored out of sunlight at room temperature for 24 h. After 24 h, the test vial was inverted 8–10 times and 30 μL was transferred with the autopipette to the test strip of the analyzer. The analysis took 3 min and the result was provided in μg/dL. The analyzer does not have memory, so the result was written down before the used sample strip was removed.
For each day of analysis with the LCP a quality control set from the test kit was analyzed. All quality controls analyzed during this study were within the control range given for the respective test kits used.
After analysis on the LCP, the samples were stored at −20°C in the collection tubes. The samples were then sent for analysis at ALS Scandinavia AB (Luleå, Sweden) to determine the BLL with ICP-MS. The freezing chain was not further interrupted. The samples were thawed, inverted for several minutes, and an aliquot of whole blood was digested with concentrated nitric acid (closed vessel, MW-assisted digestion using Mars5 laboratory digestion system from CEM) followed by dilution with distilled, de-ionized water. Diluted digests were analyzed by ICP-SFMS (ELEMENT2, ThermoScientific, Bremen, Germany), using combination of internal standardization and external calibration. Details on preparation method, measuring parameters and operation conditions can be found elsewhere (38, 39). Method blanks prepared and analyzed alongside with blood samples contain <0.5 μg/L Pb. Accuracy of the method in Pb concentration range 10–460 μg/L was controlled using reference materials from Sero AS (Oslo, Norway, Seronorm Trace elements in Human Whole Blood, Lot 1702826A, L1, L2, and L3) reconstituted according to the manufacturer's instructions.
Statistical Method
All statistical analyses were carried out with R (40). Linear regression modeling was used to assess the agreement between the LCP and the ICP-MS concentrations. BLLICP−MS was modeled as response with BLLLCP as explanatory variable. Regression coefficients of the model outputs were used to develop conversion equations. The LCP and the ICP-MS BLLs were log transformed to improve normality of error and distribution.
The BLLs were analyzed both pooled and separated into fresh and frozen. One sample was excluded as an extreme outlier with BLLLCP > 650 μg/L (over detection limit of the analyzer) and BLLICP−MS = 174 μg/L. The final sample size was n = 124 with 54 fresh and 70 frozen samples. The LCP concentration in μg/dL was converted to μg/L. As quantity of frozen samples from each sample year was not enough to analyse each year statistically, all frozen samples were analyzed as one category.
Previous wildlife studies have identified a bias of the ASV method, so Bland-Altman method comparison analysis (41) using R package blandr (42) was used to determine a potential bias on BLL of Scandinavian brown bears between the analysis methods of ASV and ICP-MS.
Models tested on log-transformed data:
Significance for analysis was set at α = 0.05. Diagnostics plots for the regression models were checked.
Results
BLL analyzed with the LCP had a median of 99 μg/L (mean 106.3 μg/L) in a range of 40–231 μg/L. BLL analyzed with ICP-MS had a mean of 88.1 μg/L (median 83 μg/L) in a range of 32.5–173.0 μg/L.
The LCP was significantly correlated to the ICP-MS BLL both for overall as well as fresh and frozen samples separately (Table 1, Figure 1) and conversion equations were based on the models 1, 2 and 3. An overall positive bias of the LCP was found with Bland-Altman analysis. For pooled samples, 19.01% (CI: 16.62–21.40%), for fresh samples at 22.50% (CI: 19.53–25.47%), and for frozen samples 16.32% (CI: 12.83–19.82%) (Figure 2) showing an overestimation of the BLL compared to ICP-MS results.

Table 1. Model parameters with P-values, r2 and Bland-Altman analyzed bias showing a positive bias of the LeadCare® Plus (LCP) for pooled (N = 124), fresh (N = 54), and frozen (N = 70) samples of blood lead levels in Scandinavian brown bear (Ursus arctos) and conversion equation based on the regression coefficients for the models 1, 2, and 3 to estimate blood lead levels when analyzed with Inductively coupled plasma mass spectrometry (ICP-MS).
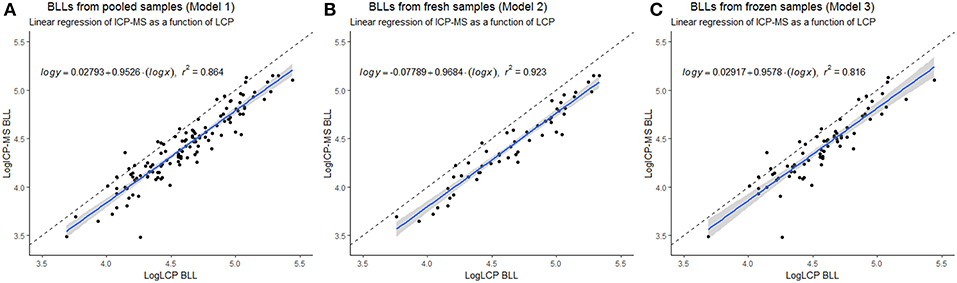
Figure 1. Linear regression of pooled, Model 1 (A), fresh, Model 2 (B), and frozen, Model 3 (C) blood lead levels (BLL) of Scandinavian Brown bear (Ursus arctos) (sampled 2010–2018) measured with inductively coupled plasma mass spectrometry (ICP-MS) as a function of BLL measured with LeadCare® Plus (LCP). Linear regression model (blue) with 95% confidence interval (gray) and added dashed line of optimal 1 to 1 comparison, and equation of the model with r2. Plotted with log transformed data.
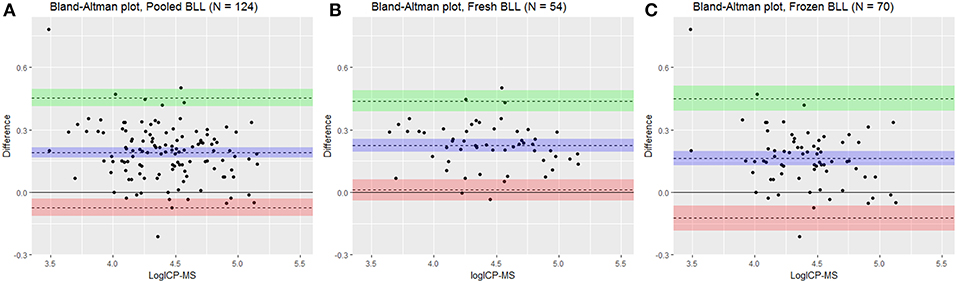
Figure 2. Bland-Altman plot for log transformed pooled (A), fresh (B), and frozen (C) blood lead levels (BLL) in Scandinavian brown bear (Ursus arctos) comparing analysis methods Inductively coupled plasma mass spectrometry (ICP-MS) and LeadCare® Plus (LCP). X axis shows the log ICP-MS as gold standard reference and Y axis shows differences (logBLLLCP-logBLLICP−MS). Represented is mean difference (bias) in blue line with 95% confidence interval in shaded blue with upper limit of agreement of Bland-Altman analysis in green line with 95% confidence interval in shaded green (+1.96*standard deviation) and lower limit of agreement (−1-96*standard deviation) in red line with 95% confidence interval in shaded red.
When applying the conversion equation on the BLLLCP, the new, calculated BLL was not significantly different from the BLLICP−MS (pooled, P = 0.933; fresh, P = 0.978; frozen, P = 0.988) with similar range.
Discussion
The results showed a high correlation between LCP and ICP-MS, although with a linear positive bias (overestimation) of 16.3–22.5% of the LCP. Conversion equations for BLLLCP were established to estimate BLLICP−MS in brown bears for the observed range of this study. Furthermore, the results indicate that Scandinavian brown bears are exposed to elevated BLL.
The positive bias of the LCP contrasts with all other comparison studies conducted on wildlife and cattle, as these studies found a negative bias on the analyzers using the same method (ASV). The frozen samples of our study had a smaller bias when compared to the gold standard which is in alignment with findings by Herring et al. (23) in avian species. Conversion equations for the LCP results to estimate ICP-MS BLL both for pooled, fresh and frozen samples were made, which can be used for future studies in brown bears to approximate the gold standard levels. The pooled conversion formula was provided for the use with a small sample size comprising of both fresh and frozen samples. The higher r2-value of the pooled conversion formula could be influenced by the larger sample size in this group.
The BLLs of this study were higher than the levels Rogers et al. (30) found in black bears and grizzly bears in Yellowstone, however these levels were only analyzed on a LeadCare® system, and were not validated.
BLLs cannot be directly compared with levels measured using other methods because of the identified bias. Using LCP BLL without correcting for the bias could lead to an overestimation of the actual lead exposure within the observed range of this study.
Factors Affecting BLL or Measurements
The LeadCare® manufacturer recommends to use fresh blood samples, and the FDA permits the use of the machine when used for capillary samples for humans (43). Freezing has been shown to impact the results of BLLs in wildlife (23) and humans (44), and the length of freezing may have had an effect on the measured BLL in this study as the freezing causes evaporation. We saw a smaller bias between the LCP and the ICP-MS in our samples that had been stored frozen prior to LCP analysis, with cause yet to be identified. To examine possible evaporation due to freezing, the samples could in future analysis be weighed before freezing and after thawing for reanalysis after long term storage.
Packed cell volume (PCV) could affect the measured BLLs, as stress throughout the capture, and especially during the chasing period, can cause dehydration and contraction of the spleen during exertion (45). A higher PCV likely gives a higher BLL reading using any analysis method, because of a relatively higher concentration of red blood cells, to which a majority of the lead is bound. Reference PCV for free-ranging brown bears is 0.41–0.54% (46) following the same capture methods as used in this study, with a seasonal variation of higher PCV in winter compared to summer (47, 48).
BLLs show recent lead exposure or endogenous lead remobilised due to bone resorption. During hibernation, the bear maintains bone mass through balanced bone resorption and formation activity (49, 50). Continuous endogenous exposure could keep the BLLs elevated during hibernation and possibly result in the high concentrations observed in the spring.
A trend of higher bone resorption is seen in bears that give birth (51). As lead is transferred through the placenta and through the milk during the lactational period (18), there could be a higher endogenous exposure in females but overall lower body lead burden because of higher excretion compared to males. The accumulative body lead burden can be measured in bone to investigate life history exposure and sex differences of the bears. However, accumulation site depends on bone formation activity at time of exposure (52). This is in alignment with findings by Herring et al. (53) that measured bone lead in the femoral bone of brown bears in Croatia. They found differing concentrations within the same individual, dependent on location of sampling site for both trabecular and compact bone.
The LeadCare® analyzers were recalled in May 2017 (54) due to underestimations of BLLs when using venous blood samples in humans. Caldwell et al. (55) reported that the CDC quality assurance program in the last five quality challenges found that participating laboratories were able to accurately analyse BLLs with a LeadCare® Ultra or Plus in 70% of the cases with a set evaluation criteria limit of ±20 μg/L on bovine venous samples that had been stored frozen until distribution. In the same study, overall accuracy of all participating laboratories with no distinction between methods was 89% with the same evaluation criteria limit of ±20 μg/L. This inaccuracy is to be kept in mind when analyzing LCP results.
Perspectives
Lead exposure from spent hunting ammunition is considered a major ecological and One Health concern (56). Ingestion of lead ammunition has not definitively been identified in bears (30). However, novel ways to identify source of exposure from other wildlife studies can be implemented, like measuring antimony (Sb) (57), another component of lead alloy in lead ammunition (58, 59). As lead is present in the environment, other food sources such as berries and ants should be investigated as potential sources, as well as exposure from active lead mines and secondary lead smelters.
The BLLs found in this study show a significant exposure to lead with unknown behavioral or physiological impact on the bears. Accurate monitoring of lead exposure in wildlife species is essential for identifying risks associated with toxicants that are proven harmful. The bear could be used as a sentinel species, as it is an opportunistic omnivore (60) and scavenger (35, 61). Using bear blood as a biomarker for recent exposure, or to compare temporal studies before and after legislative changes, is a relatively easy and readily available tool in biomonitoring.
Monitoring of acute or endogenous lead exposure in individuals, for health assessment, as well as on population level can be carried out using portable lead analyzers such as the LeadCare® Plus with provided conversion equations to approximate gold standard levels within the observed range of this study.
This is the initiating study on lead in Scandinavian brown bears and further research is necessary to investigate potential harmful effects and possible sources of exposure.
Data Availability
The dataset for this study is available upon request.
Author Contributions
JA: study design. AB, AT, BF, AE, and JA: capture and sampling. AB, AT, and AE: LeadCare® analysis. IR: inductively coupled plasma mass spectrometry analysis. AB, AT, and BF: data analysis. AB: drafting of manuscript. AB, AT, BF, AE, MB, IR, and JA: revision of manuscript and approval of submitted version.
Funding
The present study was mainly funded by the Inland Norway University of Applied Sciences, with contributions from the ALS Scandinavia AB. The Scandinavian Brown Bear Research Project is primarily funded by the Swedish Environmental Protection Agency, the Norwegian Environment Agency, the Austrian Science Fund, the Swedish Association for Hunting and Wildlife Management and the research council of Norway.
Conflict of Interest Statement
The authors declare that the research was conducted in the absence of any commercial or financial relationships that could be construed as a potential conflict of interest.
Acknowledgments
We thank the veterinarians, biologists, technicians, students and helicopter pilots who participated in the field work. This is scientific paper no. 280 from the Scandinavian Brown Bear Research Project.
References
1. Lanphear BP, Hornung R, Khoury J, Yolton K, Baghurst P, Bellinger DC, et al. Low-level environmental lead exposure and children's intellectual function: an international pooled analysis. Environ Health Perspect. (2005) 113:894–9. doi: 10.1289/ehp.7688
2. Lanphear BP, Rauch S, Auinger P, Allen RW, Hornung RW. Low-level lead exposure and mortality in US adults: a population-based cohort study. Lancet Public Health. (2018) 3:e177–84. doi: 10.1016/S2468-2667(18)30025-2
3. Nawrot TS, Staessen JA. Low-level environmental exposure to lead unmasked as silent killer. Circulation. (2006) 114:1347–9. doi: 10.1161/CIRCULATIONAHA.106.650440
4. WHO. Lead Poisoning and Health. (2018). Available online at: http://www.who.int/news-room/fact-sheets/detail/lead-poisoning-and-health (accessed September 11, 2018).
5. Liu J, Gao D, Chen Y, Jing J, Hu Q, Chen Y. Lead exposure at each stage of pregnancy and neurobehavioral development of neonates. Neurotoxicology. (2014) 44:1–7. doi: 10.1016/j.neuro.2014.03.003
6. EFSA and Panel on Contaminants in the Food Chain (CONTAM). Scientific opinion on lead in food. EFSA J. (2013) 2013:1–321. doi: 10.2903/j.efsa.2010.1570
7. CDC. Response to Advisory Committee on Childhood Lead Poisoning Prevention Recommendations in “Low Level Lead Exposure Harms Children: A Renewed Call of Primary Prevention”. (2012). Available online at: http://www.cdc.gov/nceh/lead/ACCLPP/CDC_Response_Lead_Exposure_Recs.pdf (accessed September 11, 2018).
8. CDC. New Blood Lead Level Information—What Do Parents Need to Know to Protect Their Children? (2017). Available online at: https://www.cdc.gov/nceh/lead/acclpp/blood_lead_levels.htm (accessed November 20, 2018).
9. Chamberlain AC. Effect of airborne lead on blood lead. Atmos Environ. (1982) 17:677–92. doi: 10.1016/0004-6981(83)90415-8
10. Agency for Toxic Substances and Disease Registry. Toxicological Profile for Lead. ATSDR (2007). Available online at: https://europepmc.org/books/NBK158766 (accessed December 5, 2018).
11. Rabinowitz MB, Wetherill GW, Kopple JD. Kinetic analysis of lead metabolism in healthy humans. J Clin Invest. (1976) 58:260–70. doi: 10.1172/JCI108467
12. International Agency for Research on Cancer. (2006). Inorganic and Organic Lead Compounds (IARC). Available online at: www.inchem.org/documents/iarc/vol87/volume87.pdf (accessed May 22, 2019).
13. Barry PSI, Mossman DB. Lead concentrations in human tissues. Br J Ind Med. (1970) 27:339–51. doi: 10.1136/oem.27.4.339
14. O'flaherty EJ, Hammond PB, Lerner SI. Dependence of apparent blood lead half-life on the length of previous lead exposure in humans. Toxicol Sci. (1982) 2:49–54. doi: 10.1093/toxsci/2.1.49
15. Gulson B. Stable lead isotopes in environmental health with emphasis on human investigations. Sci Total Environ. (2008) 400:75–92. doi: 10.1016/j.scitotenv.2008.06.059
16. Silbergeld EK. Lead in bone: implications for toxicology during pregnancy and lactation. Environ Health Perspect. (1991) 91:63–70. doi: 10.1289/ehp.919163
17. Gulson BL, Mahaffey KR, Jameson CW, Mizon KJ, Korsch MJ, Cameron MA, et al. Mobilization of lead from the skeleton during the postnatal period is larger than during pregnancy. J Lab Clin Med. (1998) 133:324–9. doi: 10.1016/S0022-2143(98)90182-2
18. Xie X, Ding G, Cui C, Chen L, Gao Y, Zhou Y, et al. The effects of low-level prenatal lead exposure on birth outcomes. Environ Pollut. (2013) 175:30–4. doi: 10.1016/j.envpol.2012.12.013
19. Ettinger AS, Roy A, Amarasiriwardena CJ, Smith D, Lupoli N. Maternal blood, plasma, and breast milk lead: lactational transfer and contribution to infant exposure. Environ Health Perspect. (2014) 122:87–92. doi: 10.1289/ehp.1307187
20. Keller CA, Doherty RA. Bone lead mobilization in lactating mice and lead transfer to suckling offspring. Top Catal. (1980) 55:220–8. doi: 10.1016/0041-008X(80)90083-6
21. Bushnell PJ, DeLuca HF. Lactose facilitates the intestinal absorption of lead in weanling rats. Science. (1981) 211:61–3. doi: 10.1126/science.7444448
22. Bonnefoy C, Menudier A, Moesch C, Lachâtre G, Mermet JM. Validation of the determination of lead in whole blood by ICP-MS. J Anal Atom Spectrom. (2002) 17:1161–5. doi: 10.1039/b201889f
23. Herring G, Eagles-Smith CA, Bedrosian B, Craighead D, Domenech R, Langner HW, et al. Critically assessing the utility of portable lead analyzers for wildlife conservation. Wildl Soc Bull. (2018) 42:284–94. doi: 10.1002/wsb.892
24. Palmer CD, Lewis ME, Geraghty CM, Barbosa F, Parsons PJ. Determination of lead, cadmium and mercury in blood for assessment of environmental exposure: a comparison between inductively coupled plasma-mass spectrometry and atomic absorption spectrometry. Spectrochim Acta Part B Atom Spectrosc. (2006) 61:980–90. doi: 10.1016/j.sab.2006.09.001
25. Barón-Jaimez J, Joya MR, Barba-Ortega J. Anodic stripping voltammetry—ASV for determination of heavy metals. J Phys Conf Ser. (2013) 466:2023. doi: 10.1088/1742-6596/466/1/012023
26. Pineau A, Fauconneau B, Rafael M, Viallefont A, Guillard O. Determination of lead in whole blood: comparison of the leadCare blood lead testing system with Zeeman longitudinal electrothermal atomic absorption spectrometry. J Trace Elem Med Biol. (2002) 16:113–7. doi: 10.1016/S0946-672X(02)80037-2
27. Craighead D, Bedrosian B. Blood lead levels of common ravens with access to big-game offal. J Wildl Manag. (2008) 72:240–5. doi: 10.2193/2007-120
28. Langner HW, Domenech R, Slabe VA, Sullivan SP. Lead and mercury in fall migrant golden eagles from western North America. Arch Environ Contam Toxicol. (2015) 69:54–61. doi: 10.1007/s00244-015-0139-6
29. Bischoff K, Gaskill C, Erb HN, Ebel JG, Hillebrandt J. Comparison of two methods for blood lead analysis in cattle: graphite-furnace atomic absorption spectrometry and leadcare(R) II system. J Vet Diagn Invest. (2010) 22:729–33. doi: 10.1177/104063871002200510
30. Rogers TA, Bedrosian B, Graham J, Foresman KR. Lead exposure in large carnivores in the greater Yellowstone ecosystem. J Wildl Manag. (2012) 76:575–82. doi: 10.1002/jwmg.277
31. Brown CS, Luebbert J, Mulcahy D, Schamber J, Rosenberg DH. Blood lead levels of wild Steller's Eiders (Polysticta stelleri) and black scoters (Melanitta nigra) in Alaska using a portable blood lead analyzer. J Zoo Wildl Med. (2006) 37:361–5. doi: 10.1638/05-092.1
32. González F, López I, Suarez L, Moraleda V, Rodríguez C. Levels of blood lead in Griffon vultures from a Wildlife Rehabilitation Center in Spain. Ecotoxicol Environ Saf. (2017) 143:143–50. doi: 10.1016/j.ecoenv.2017.05.010
33. Bedrosian B, Parish CN, Craighead D. Differences between blood lead level detection techniques: Analysis within and among three techniques and four avian species. In: Watson RT, Fuller M, Pokras M, Hunt WG editors. Ingestion of Lead from Spent Ammunition: Implications for Wildlife and Humans. Boise, ID: The Peregrine Fund (2009). doi: 10.4080/ilsa.2009.0122
34. Stokke S, Brainerd S, Arnemo JM. Metal deposition of copper and lead bullets in moose harvested in Fennoscandia. Wildl Soc Bull. (2017) 41:98–106. doi: 10.1002/wsb.731
35. Rauset GR, Kindberg J, Swenson JE. Modeling female brown bear kill rates on moose calves using global positioning satellite data. J Wildl Manag. (2012) 76:1597–606. doi: 10.1002/jwmg.452
36. SBBRP. Scandinavian Brown Bear Research Project. (2019). Available online at: http://bearproject.info/about-the-project/ (accessed March 7, 2019).
37. Arnemo JM, Evans AL. Biomedical protocols for free-ranging brown bears, wolves, wolverines and lynx. Inl Norw Univ Appl Sci. (2017) 2017:1–16. doi: 10.13140/RG.2.1.2717.7120
38. Rodushkin I, Ödman F, Branth S. Multi-element analysis of environmental samples by high resolution inductively coupled plasma mass spectrometry. Fresenius J Anal Chem. (1999) 364:338–46. doi: 10.1007/s002160051346
39. Rodushkin I, Ödman F, Olofsson R, Axelsson MD. Determination of 60 elements in whole blood by sector field inductively coupled plasma mass spectrometry. J Anal At Spectrom. (2000) 15:937–44. doi: 10.1039/B201889F
40. R Core Team. R: A Language and Environment for Statistical Computing. (2018). Available online at: https://www.r-project.org/ (accessed September 7, 2018).
41. Bland JM, Altman DG. Measuring agreement in method comparison studies. Stat Methods Med Res. (1999) 8:135–60. doi: 10.1177/096228029900800204
43. FDA UFDA. Recalls LeadCare Plus and Ultra Testing Systems due to Inaccurate Test Results. Magellan Diagnostics Inc. fda.gov (2018). Available online at: https://www.fda.gov/MedicalDevices/Safety/ListofRecalls/ucm560534.htm (accessed March 7, 2019).
45. Vatner SF, Higgins CB, Millard RW, Franklin D. Role of the spleen in the peripheral vascular response to severe exercise in untethered dogs. Cardiovasc Res. (1974) 8:276–82. doi: 10.1093/cvr/8.2.276
46. Græsli AR, Fahlman Å, Evans AL, Bertelsen MF, Arnemo JM, Nielsen SS. Haematological and biochemical reference intervals for free-ranging brown bears (Ursus arctos) in Sweden. BMC Vet Res. (2014) 10:1–9. doi: 10.1186/s12917-014-0183-x
47. Evans AL, Sahlén V, Støen OG, Fahlman Å, Brunberg S, Madslien K, et al. Capture, anesthesia, and disturbance of free-ranging brown bears (Ursus arctos) during hibernation. PLoS ONE. (2012) 7:1–8. doi: 10.1371/journal.pone.0040520
48. Græsli AR, Evans AL, Fahlman Å, Bertelsen MF, Blanc S, Arnemo JM. Seasonal variation in haematological and biochemical variables in free-ranging subadult brown bears (Ursus arctos) in Sweden. BMC Vet Res. (2015) 11:1–9. doi: 10.1186/s12917-015-0615-2
49. Donahue SW, Galley SA, Vaughan MR, Patterson-Buckendahl P, Demers LM, Vance JL, et al. Parathyroid hormone may maintain bone formation in hibernating black bears (Ursus americanus) to prevent disuse osteoporosis. J Exp Biol. (2006) 209:1630–8. doi: 10.1242/jeb.02185
51. Donahue SW, Vaughan MR, Demers LM, Donahue HJ. Serum markers of bone metabolism show bone loss in hibernating bears. Clin Orthop Relat Res. (2003) 295–301. doi: 10.1097/00003086-200303000-00040
52. Tarragó O, Brown MJ. Case studies in environmental medicine (CSEM). Lead Toxicity. (2017) P. 66. Available online at: https://www.atsdr.cdc.gov/csem/csem.asp?csem=34&po=0
53. Lazarus M, Orct T, Reljić S, Sedak M, Bilandžić N, Jurasović J, et al. Trace and macro elements in the femoral bone as indicators of long-term environmental exposure to toxic metals in European brown bear (Ursus arctos) from Croatia. Environ Sci Pollut Res. (2018) 25:21656–70. doi: 10.1007/s11356-018-2296-4
54. FDA UFDA. (2017). FDA Warns Against Using Magellan Diagnostics LeadCare Testing Systems with Blood Obtained from a Vein: FDA Safety Communication. Available online at: https://www.fda.gov/MedicalDevices/Safety/AlertsandNotices/ucm558733.htm (accessed February, 9, 2019).
55. Caldwell KL, Cheng P, Vance KA, Makhmudov A, Jarrett JM, Caudill SP, et al. LAMP : a CDC program to ensure the quality of blood-lead laboratory Measurements. (2019) 25:23–30. doi: 10.1097/PHH.0000000000000886
56. Hampton JO, Laidlaw M, Buenz E, Arnemo JM. Heads in the sand : public health and ecological risks of lead-based bullets for wildlife shooting in Australia. Wildl Res. (2018) 45:287–306. doi: 10.1071/WR17180
57. French AD. Toxicokinetics and Sources of Lead in American Woodcock (Scolopax minor). (2017) Available online at: http://hdl.handle.net/2346/73544 (accessed December 3, 2018).
58. Anderson CG. The metallurgy of antimony. Chemie Erde. (2012) 72:3–8. doi: 10.1016/j.chemer.2012.04.001
59. Storgaard S, Willems M, Storgaard BS. The fate of lead in soils: the transformation of lead pellets in shooting-range soils. R Swedish Acad Sci. (1987) 16:11–5.
60. Stenset NE, Lutnæs PN, Bjarnadóttir V, Dahle B, Fossum KH, Jigsved P, et al. Seasonal and annual variation in the diet of brown bears Ursus arctos in the boreal forest of southcentral Sweden. Wildl Biol. (2016) 22:107–16. doi: 10.2981/wlb.00194
Keywords: blood lead, lead exposure, Ursus, anodic stripping voltammetry, Pb
Citation: Boesen AH, Thiel A, Fuchs B, Evans AL, Bertelsen MF, Rodushkin I and Arnemo JM (2019) Assessment of the LeadCare® Plus for Use on Scandinavian Brown Bears (Ursus arctos). Front. Vet. Sci. 6:285. doi: 10.3389/fvets.2019.00285
Received: 06 June 2019; Accepted: 12 August 2019;
Published: 28 August 2019.
Edited by:
Nicole Indra Stacy, University of Florida, United StatesReviewed by:
Nadine Lamberski, Zoological Society of San Diego, United StatesEnrique Yarto, Centro Veterinario Mexico, Mexico
Copyright © 2019 Boesen, Thiel, Fuchs, Evans, Bertelsen, Rodushkin and Arnemo. This is an open-access article distributed under the terms of the Creative Commons Attribution License (CC BY). The use, distribution or reproduction in other forums is permitted, provided the original author(s) and the copyright owner(s) are credited and that the original publication in this journal is cited, in accordance with accepted academic practice. No use, distribution or reproduction is permitted which does not comply with these terms.
*Correspondence: Amanda H. Boesen, YWhiLmdvcm1AZ21haWwuY29t