- 1College of Veterinary Medicine, University of Missouri, Columbia, MO, United States
- 2Department of Veterinary Medicine and Surgery, College of Veterinary Medicine, University of Missouri, Columbia, MO, United States
- 3Comparative Internal Medicine Laboratory, University of Missouri, Columbia, MO, United States
- 4University of Missouri Metagenomics Center, University of Missouri, Columbia, MO, United States
- 5Department of Veterinary Pathobiology, College of Veterinary Medicine, University of Missouri, Columbia, MO, United States
It is unknown how the respiratory microbiome influences and is influenced by bacterial pneumonia in dogs, as culture of lung samples and not microbial sequencing guides clinical practice. While accurate identification of pathogens are essential for treatment, not all bacteria are cultivable and the impact of respiratory dysbiosis on development of pneumonia is unclear. The study purposes were to (1) characterize the lung microbiome in canine bacterial pneumonia and compare deviations in dominant microbial populations with historical healthy controls, (2) compare bacteria identified by culture vs. 16S rDNA sequencing from bronchoalveolar lavage fluid (BALF) culture-, and (3) evaluate similarities in lung and oropharyngeal (OP) microbial communities in community-acquired and secondary bacterial pneumonia. Twenty BALF samples from 15 client-owned dogs diagnosed with bacterial pneumonia were enrolled. From a subset of dogs, OP swabs were collected. Extracted DNA underwent PCR of the 16S rRNA gene. Relative abundance of operational taxonomic units (OTUs) were determined. The relative abundance of bacterial community members found in health was decreased in dogs with pneumonia. Taxa identified via culture were not always the dominant phylotype identified with sequencing. Dogs with community-acquired pneumonia were more likely to have overgrowth of a single organism suggesting loss of dominant species associated with health. Dogs with secondary bacterial pneumonia had a greater regional continuity between the upper and lower airways. Collectively, these data suggest that dysbiosis occurs in canine bacterial pneumonia, and culture-independent techniques may provide greater depth of understanding of the changes in bacterial community composition that occur in disease.
Introduction
Canine bacterial pneumonia is a common respiratory disorder, occurring as primary disease process, or secondary to aspiration, viral infections (1, 2), immunodeficiency, or a nosocomial event (3). In both humans and companion animals, bacterial pneumonia can be life-threatening making prompt diagnosis and targeted treatment essential. Diagnostic approaches to identify the causative agents have traditionally relied on ex vivo culture from carefully collected airway lavage [e.g., bronchoalveolar lavage fluid (BALF)]. This method is predicated on the belief that the deep airways are largely free of bacteria and any growth on selective media represents aberrant colonization. The recent development of culture-independent molecular techniques has revealed that in humans (4), cats (5), dogs (6), sheep (7), and likely other host species, the healthy lungs harbor low biomass microbial populations seeded via direct extension from upper airway communities, repeated microaspiration, and inhalation of bacteria in air (8). Moreover, these culture-independent methods have reinforced that a lack of cultivable organisms does not necessarily indicate a sterile environment (9). Collectively, such findings suggest that sequencing methods might have clinical utility in the identification of microbes associated with bacterial pneumonia. Toward that end, the current study compared the results of traditional culture-based methods and a targeted sequencing approach applied to 20 BALF samples collected from 15 dogs affected with bacterial pneumonia in a referral veterinary hospital setting.
Canine bacterial pneumonia is categorized as either community-acquired pneumonia (CAP) or secondary bacterial pneumonia (SBP) based on the etiology, clinical presentation, and patient history. As the name implies, CAP is typified by known contagious pathogens, such as Bordetella bronchiseptica and Streptococcus equi subspecies zooepidemicus and is often seen in dogs with a history of acute onset clinical signs following exposure to reservoirs of infectious agents, such as shelters, boarding facilities, and dog parks (10, 11). Secondary bacterial pneumonia, on the other hand, occurs as a sequela to a predisposing anatomic or physiological condition, such as megaesophagus, laryngeal paralysis, or ciliary dyskinesia (12), and the microbes recovered in a diagnostic sample are often not primary contagious pathogens, per se. Rather, dysfunction of the upper respiratory tract or gastrointestinal tract allows or facilitates increased translocation of material to the lower airways and/or prevents effective microbial clearance, leading to the hypothesis that the lower and upper airway microbiota would be more similar in cases of SBP relative to cases of CAP. To address this question, oropharyngeal (OP) swabs were collected from a subset of dogs alongside BALF samples and the compositional similarity of OP and BALF microbiota was evaluated in the context of clinical diagnoses and predisposing anatomic factors.
Materials and Methods
Experimental Design
The current study was performed prospectively at the University of Missouri Veterinary Health Center (VHC), a referral and primary care veterinary hospital located in Columbia, MO, USA. All dogs contributing samples to the current study presented to the VHC with clinical signs related to bacterial pneumonia between August 2016 and December 2017. Bronchoscopic examination and diagnostic collection of BALF were performed as part of their standard care. Peripheral blood was also collected at presentation for hematologic and serum chemistry analyses. Dogs were then diagnosed with bacterial pneumonia based on clinical signs associated with septic suppurative inflammation or a positive aerobic or anaerobic culture result of BALF, and categorized by type of pneumonia based on the history, clinical signs and other diagnostic findings. Dogs were of various breeds and ages; a table showing the range of patient demographics is provided in Table 2.
Sample Collection
Anesthetic protocols were performed at the discretion of a board certified veterinary anesthesiologist. Samples were collected as previously described (5). Briefly, after induction for anesthesia, while avoiding the rest of the oral cavity, a sterile swab was used to vigorously rub the caudodorsal aspect of the oropharynx, from a subset of patients (see Table 1). The swab was added to 800 μL lysis buffer adapted from Yu and Morrison (13). Dogs were initially intubated using sterile endotracheal tubes. Control samples were obtained by running a 10 ml aliquot of sterile saline through the endoscope channel before its use. Immediately prior to the bronchoscopy, the endotracheal tube was replaced with a sterile red rubber catheter to provide oxygen and the endoscope was passed directly through the larynx into the tracheobronchial tree. BALF collection was performed by instilling one or two 20 mL aliquots of sterile saline through the channel of a sterile bronchoscope when wedged in an airway. All dogs provided one BALF sample with the following exceptions: dog I provided one sample on 1/12/2017 (I1) and two samples from the left and right lung lobes in on 11/2016 (I2 and I3, respectively), dog M provided samples on 11/4 and 12/20 of 2016 (M1 and M2), and dog G provided samples on 11/11, 12/1, and 12/21 of 2016 (G1, G2, and G3, respectively). Following collection of BALF, samples were split to provide a minimum of 1 mL of material to the University of Missouri Veterinary Medical Diagnostic Laboratory for culture on Blood agar and MacConkey agar plates for aerobic cultures, and chocolate agar plates for anaerobic cultures. All aerobic samples were incubated at 35°C, and anaerobic cultures were incubated at 35°C with 95% air and 5% CO2 for 24–36 h. The laboratory does not culture Mycoplasma spp. in large part due to challenging growth requirements. Bacterial isolates were Gram-stained and identified with conventional biochemical reactions (14), the Automated Sensititre AP-80 or AP 90 for aerobic bacteria or the MALDI-TOF identification system (Matrix Assisted Laser Desorption/Ionization-Time of Flight: Bruker Daltonics, Inc. 40 Manning Road, Manning Park, Billerica, MA 01821). Aerobic susceptibility testing was performed with the Sensititre Micro-Broth (Thermofisher Scientific 12076 Santa Fe Drive, Lenexa, KS 66215) dilution minimal inhibitory concentration system. Up to 30 mL of the remaining BALF material was promptly centrifuged, and the resulting pellet was frozen and maintained at −80°C until DNA extraction was performed.
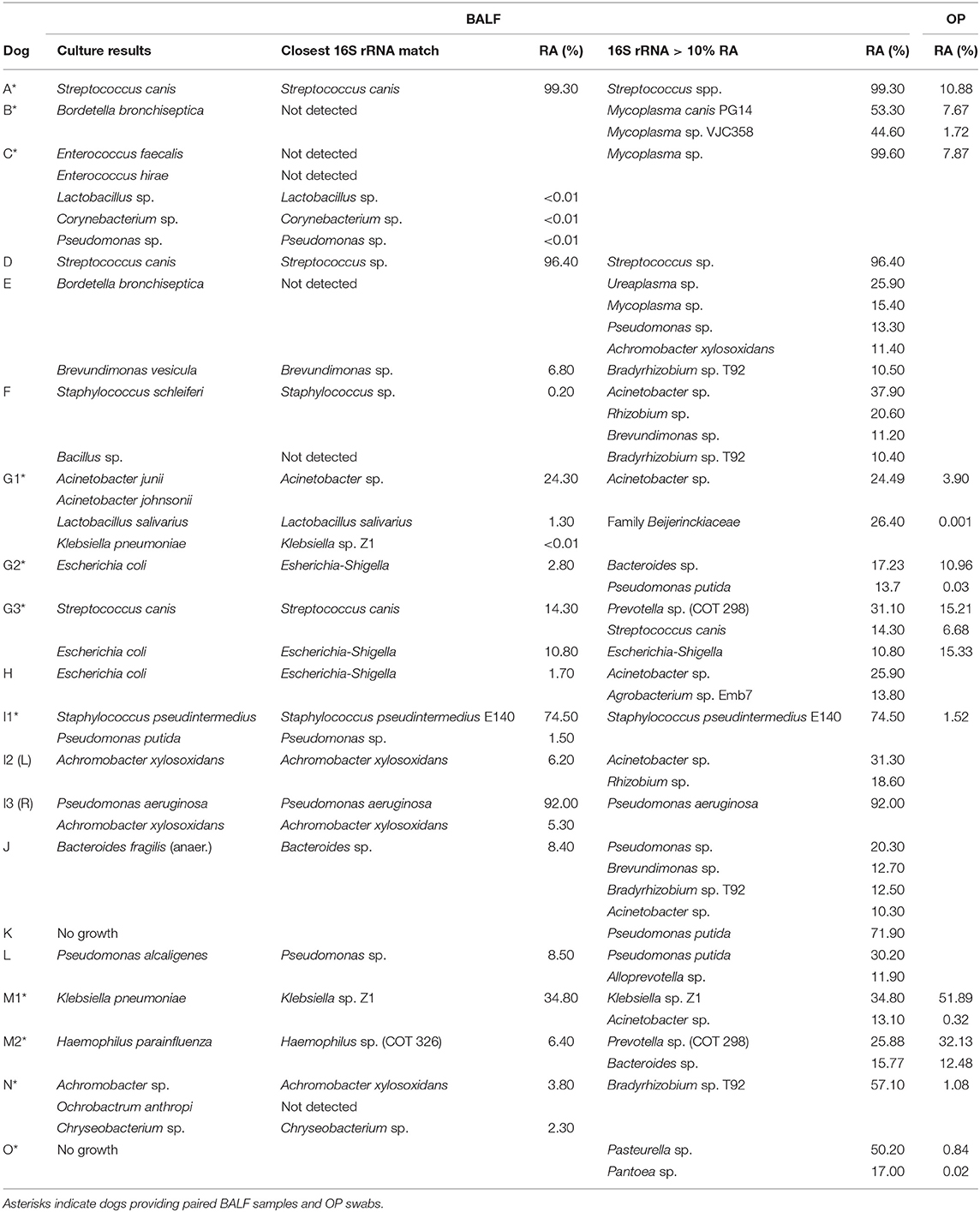
Table 1. Comparison of culture and targeted sequencing results for lower airways (BALF) and relative abundance of predominant OTU in BALF found in upper airways.
DNA Extraction
To maximize yields, DNA was first extracted using a manual nucleic acid precipitation, followed by resuspension of DNA in buffer and purification using DNeasy kits (Qiagen) according to manufacturer's instructions with minor modifications. Briefly, BALF was first centrifuged at 5,000 × g for 10 min at room temperature, followed by removal of the supernatant and resuspension in 800 μL lysis buffer as adapted from Yu and Morrison (13). Samples were then incubated at 70°C for 20 min with periodic mixing and centrifuged as before. Next, 10 mM ammonium acetate (200 μL) was added to the supernatant and samples were incubated on ice for 5 min, before centrifugation at 5,000 × g for 10 min at room temperature. Up to 750 μL of the supernatant was then mixed with an equal volume of chilled isopropanol and incubated on ice for 30 min. Samples were then centrifuged at 16,000 × g for 15 min at 4°C. Precipitated nucleic acids were then washed with 70% ethanol, resuspended in 150 μL Tris-EDTA (10 mM Tris and 1 mM EDTA), and processed according to the DNeasy kit's manufacturer's instructions, with the following modification. Instead of eluting in the AE buffer provided with the kits, DNA was eluted in the comparable, but EDTA-free, EB buffer (Qiagen). Yields were determined via fluorometry (Qubit 2.0) using Qubit dsDNA BR assays (Life Technologies, Carlsbad, CA). Samples were stored at −20°C until library preparation was performed.
16S rRNA Library Preparation and Sequencing
Library construction and sequencing were performed at the University of Missouri DNA Core facility, as previously described (6). Briefly, 16S rRNA amplicons were generated via amplification of the V4 region of the 16S rRNA gene using single-indexed universal primers (U515F/806R) (15, 16) flanked by Illumina standard adapter sequences. Following amplification, products were pooled for sequencing using the Illumina MiSeq platform and V2 chemistry with 2 × 250 bp paired-end reads.
Informatics
Assembly, annotation, and binning of DNA sequences were performed at the University of Missouri Informatics Research Core facility. Contiguous DNA sequences were assembled using FLASH software (17) and removed if found to be short after trimming for a base quality <31. Qiime v1.9.1 software (18) was used to perform de novo and reference-based chimera detection and removal, and remaining contiguous sequences were assigned to operational taxonomic units (OTUs) via de novo OTU clustering and a criterion of 97% nucleotide identity. Taxonomy was determined for selected OTUs using BLAST against the SILVA database (19, 20). Principal coordinate analyses (PCoA) were performed using ¼ root-transformed OTU relative abundance data in PAST 3.17 (21). Metrics of richness and α-diversity were determined based on a rarefied dataset subsampled to a uniform read count of 2,289 reads per sample using beta_diversity_through_plots.py, available at http://qiime.org/scripts/beta_diversity_through_plots.html.
Statistical Analysis
Distribution of read counts in experimental and control samples was first tested for normality using the Shapiro-Wilk method, and differences in read count were then determined using a Mann-Whitney rank sum test due to non-normality, implemented in SigmaPlot 13.0. Differences in β-diversity between BALF and OP swab communities were determined using one-way permutational multivariate analysis of variance (PERMANOVA), implemented in Past 3.18 (21). To evaluate the similarity between OP and BALF bacterial communities in the context of SBP vs. CAP, time-matched OP and BALF samples were collected from a subset of patients (designated with a * in Table 2) and the intra-subject similarities between the OP and BALF communities in patients with SBP and CAP were visualized and tested for significance (between disease type) via principal coordinate analysis (PCoA) and one-way PERMANOVA, respectively. In both analyses (i.e., PCoA and PERMANOVA), comparisons were performed using both unweighted (i.e., Jaccard) and weighted (i.e., Bray-Curtis) metrics. Briefly, the Jaccard similarity is based on the agreement between two samples with regard to the proportion of shared taxa while the Bray-Curtis similarity also accounts for agreement between two samples with regard to the relative abundance of shared taxa. In all cases, significance was established as p < 0.05.
Results
Twenty BALF samples were collected from 15 different dogs that met the enrollment criteria, i.e., a diagnosis of bacterial pneumonia based on clinical signs and associated with BALF septic suppurative inflammation or a positive culture result. Eighteen of those 20 samples had positive bacterial cultures, from which ten of 18 (56%) yielded one bacterial isolate, and eight of 18 (44%) yielded between two and five isolates.
Sequencing of 16S rRNA amplicon libraries generated from BALF and control fluid flushed through the bronchoscopes resulted in significantly different (p = 0.004) mean (± SEM) read counts of 31,524 (± 7,663) and 4,728 (±1,576) reads per sample, respectively. Additionally, seven BALF samples returning read counts within two standard deviations of the mean read counts generated from control samples showed generally good overall agreement with culture results indicating that the data were still meaningful. In contrast to the number of taxa identified based by culture, the DNA detected in patient BALF samples represented between 22 and 185 distinct operational taxonomic units (OTUs). Thus, while traditional culture methods provide evidence of live and cultivable bacteria in a sample and allow antimicrobial susceptibility testing, 16S rRNA sequencing provides a more comprehensive profile of taxa present in a sample, whether or not they are viable or cultivable. Additionally, mean ± SD numbers of unique OTUs in BALF from dogs with CAP were significantly lower than those with SBP (26 ± 16 and 82 ± 30 OTUs, respectively; p = 0.0002).
In many cases, there was remarkable agreement between the two methods, particularly in instances of a marked overgrowth of a dominant taxon. Specifically, samples from dogs A and D were both culture positive for Streptococcus canis alone, and 16S rRNA sequencing detected S. canis or Streptococcus sp. at 99.3 and 96.4% relative abundance (RA), respectively (Table 1). Similarly, samples I1, I3, and M1 were culture positive for Staphylococcus pseudintermedius and Pseudomonas putida (I1), Pseudomonas aeruginosa and Achromobacter xylosoxidans (I3), and Klebsiella pneumoniae (M1), with 16S sequencing detecting S. pseudintermedius (74.5% RA) and P. putida (1.5% RA), P. aeruginosa (92.0% RA) and A. xylosoxidans (5.3% RA), and Klebsiella sp. Z1 (34.8% RA), respectively in the same samples.
In the majority of the remaining samples, taxa detected via culture were also detected via sequencing but not as the dominant phylotype. In samples I2, M2, G1, G2, G3, H, J, L, F, and N, the dominant taxon determined by sequencing was not cultured despite many of the detected species being readily cultivable. In the aforementioned group of samples, cultured bacteria represented from <0.01 to 24.3% of the DNA detected via 16S rRNA sequencing (median 2.55% RA) or, in four of the eighteen culture-positive cases (22.2%), were not detected at all in the sequencing data (i.e., Bordetella bronchiseptica in samples F and H, Enterococcus spp. in sample G, and Ochrobacterium anthropi in sample N).
In cases of SBP, we hypothesized that the same factors predisposing the dog to pneumonia would facilitate greater translocation of upper airway microbes to the lung, relative to what occurs in CAP. PCoA analysis revealed complete separation of BALF and OP samples in dogs with CAP, and substantial overlap between BALF and OP samples in dogs with SBP (Figure 1). Accordingly, PERMANOVA detected a significant difference between samples sites when based on the Jaccard similarity (p = 0.0003; F = 3.21), but not Bray-Curtis (p = 0.15; F = 1.32), indicating that the BALF and OP communities differ based on the presence or absence of certain taxa, but not with regard to the relative abundance of shared taxa. Factoring in individual variability, the intra-subject similarity between BALF and OP communities was low in the three cases of CAP from whom OP samples were collected (i.e., dogs A–C), regardless of the similarity index used (Figure 2). In contrast, samples from severely dysphagic dogs with confirmed aspiration pneumonia (e.g., dogs G and M) evinced a high degree of compositional similarity between BALF and OP microbiota (Figure 3). It is worth noting that, despite the apparent dissimilarity between BALF and OP swabs in samples from dogs diagnosed with CAP, the dominant taxa detected in the lower airways were consistently detected in the matched upper airway samples, albeit at a much lower relative abundance. Relative abundance (RA) of the dominant taxon is another metric used to describe resident bacterial communities in CAP and SBP. In 4/6 dogs with CAP, and in 1/9 dogs with SBP, there was near eradication of the microbial diversity in the lower airways, with predominant OTUs found in RAs between 92.4 and 99.9%.
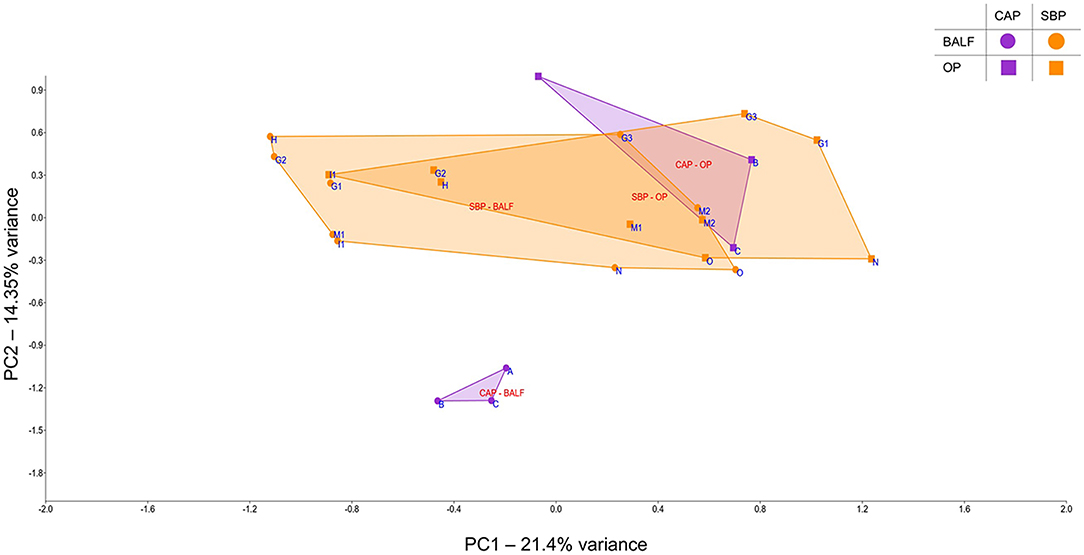
Figure 1. Principal component analysis of samples from bronchoalveolar lavage fluid (BALF) and oropharyngeal swabs (OP), for a select number of cases of community-acquired pneumonia (CAP) and secondary bacterial pneumonia (SBP); circles represent BALF, squares represent OP, SBP samples are in orange and CAP samples are in purple.
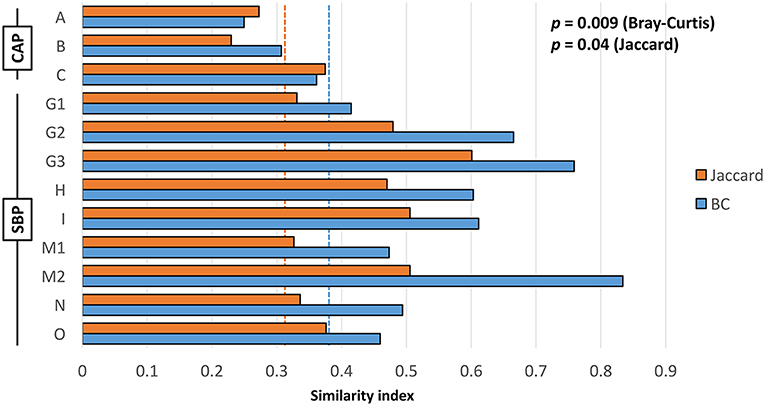
Figure 2. Intra-subject Jaccard (orange) and Bray-Curtis (blue) similarity between BALF and OP microbiota in cases of community-acquired pneumonia (CAP) and secondary bacterial pneumonia (SBP); dotted lines indicate mean inter-subject similarity between all samples. Results of Student's t-test shown on chart.
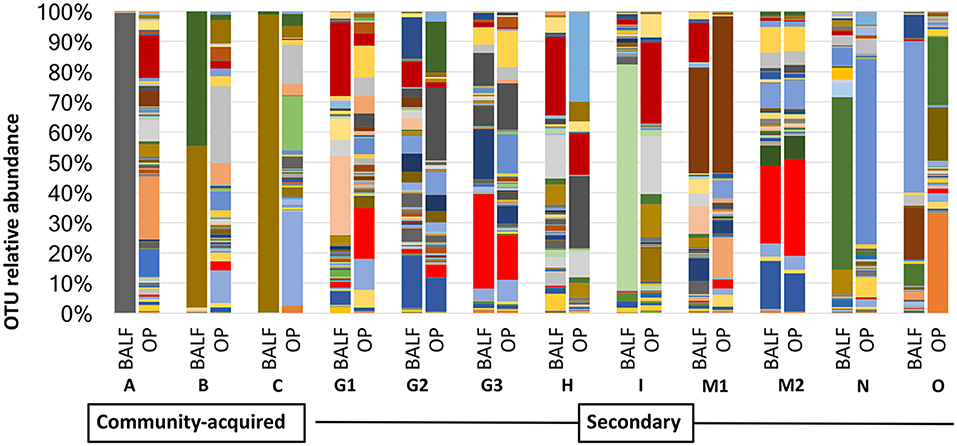
Figure 3. Comparison of relative abundance in BALF and OP between community acquired and secondary bacterial pneumonia.
Discussion
Methods complementary to traditional laboratory-based culture of BALF in dogs with bacterial pneumonia, such as 16S rRNA sequencing, are useful to understand the complex relationship between pathogen and resident microbes. In this study, cultures of BALF, used by clinicians to provide insight into underlying pathogens, identified between zero and five bacterial species in dogs with bacterial pneumonia. This was in stark contrast to DNA sequencing that revealed rich microbial communities ranging from 22 to 185 distinct OTUs. Dysbiosis was appreciated by increases in the relative abundance of specific taxa, and interlinked with this change, a decrease in the predominating taxa found in healthy dog lungs (6). In the majority of BALF samples, the cultured bacteria were present in the sequencing data, despite variation between dogs as to whether the dominant taxa determined by culture was identical to the dominant taxa based on sequencing. This phenomenon has also been described in mechanically ventilated patients in which 75% of organisms identified via culture were the most abundant organisms identified via sequencing (22). An advantage of molecular techniques is their enhanced ability to provide insight as to how microbial communities change in disease and allow comparisons in regional differences in populations (i.e., upper vs. lower airways). Bacterial populations present in the lungs during pneumonia depend on the clinical scenario with differences between dogs with CAP and SBP. In the former, there was generally a stark loss of microbial diversity and replacement with a predominant taxon. In the latter, with secondary risk factors for pneumonia, such as laryngeal or esophageal dysfunction, lower airway communities are likely heavily derived from those present in the upper airways.
In a previous analysis of the lung microbiota of 16 healthy dogs at the same institution (6), the most abundant taxa were Acinetobacter johnsonii, Brevundimonas diminuta and members for the family Pseudomonadaceae [mean (range) % RA 19.81 (13.89–26.72), 22.48 (17.36–26.67), and 29.6 (18.57–34.94), respectively]. The remainder of the taxa identified in healthy dogs were present at a RA of 2.73% or less. This is in stark contrast to the findings in this study, where Acinetobacter, Brevundimonas, and members of the family Pseudomonadaceae were present between 10 and 50% RA in 9/20 samples, suggesting respiratory dysbiosis is a common component of canine bacterial pneumonia.
In dogs with bacterial pneumonia, cultures of BALF would seemingly support a single dominant or very small number (<5) of lung pathogens; however, results of DNA sequencing underscore the complexity of microbial communities in the lung, even in the presence of infection. Mean numbers of distinct OTUs identified in BALF of dogs with CAP (23) and SBP (82) were similar to a prior study of the healthy canine lower respiratory tract microbiome (6). Thus, richness (overall numbers of distinct OTUs), while variable in pneumonia, was discordant with the paucity of cultivable bacteria. Similarly, in a study evaluating respiratory microbial communities of mechanically ventilated patients with pneumonia, 12 out of 56 patients (21%) had positive cultures. These patients had a significant decrease in microbial richness compared to culture negative subjects there was a wide range in terms of richness (22). In patients that developed pneumonia after receiving lung transplantation also demonstrated a decrease in microbial richness (24). Most of the microbes found via sequencing are not cultivable using standard techniques, making it challenging to discern the larger picture of microbial interactions within the lung. It is unclear how many uncultured or as yet unidentified lung pathogens are responsible for bacterial pneumonia, but study of the respiratory microbiome will be critical moving forward.
The fact that, in 4 of 6 dogs with CAP, the predominant OTUs represented between 92.4 and 99.9% of recovered DNA suggests overgrowth of these organisms and reflect dramatic changes from that reported in healthy dogs (6). It has been proposed that disease is associated not just with pathogens gained, but with resident species that are lost and that a greater risk of repopulation with virulent organisms may be more likely with disappearance of dominant species associated with health (25). In dogs diagnosed with CAP, microbial diversity was abolished relative to dogs with SBP.
With regard to those bacteria identified via standard culture but not found in the sequencing data, Bordetella bronchiseptica is a common and important canine respiratory pathogen causing CAP that is also as a commensal organism in healthy, asymptomatic dogs (23, 26). It is unclear as to why B. bronchiseptica or closely related taxa were not detected in the 16S rRNA dataset. While the SILVA database used to annotate the current data does contain multiple sequences specific to other Bordetella spp., it is worth noting that the 16S rRNA sequence of Achromobacter and Bordetella spp. share a high degree of homology, and A. xylosoxidans was sequenced in both samples. It is also possible that the Enterococcus and Bacillus species identified via culture were a result of contamination, as these organisms are considered to be ubiquitous. There are clinical implications to identification of organisms on culture that are not present in the sample according to targeted sequencing. In large part, results of standard culture techniques are relied upon for information to guide treatment. This could potentially lead to incorrect antibiotic selection.
Conversely, there are also limitations to the utility of molecular approaches, the most apparent of which is the inability to distinguish viable and dead bacteria using standard 16S rRNA sequencing methods. The fact that many of the bacterial species found in the sequencing data were also cultured indicates that at least some of the bacteria present in the lungs are viable, and even if DNA detected in BALF represents bacteria that have been killed by the immune defenses of the lung (e.g., pulmonary alveolar macrophages), it does not obviate an influence of those bacteria on airway health. Without a viable isolate, it is also not possible to perform antimicrobial susceptibility testing on taxa identified via sequencing. Moreover, whether applying traditional culture-based or culture-independent molecular techniques, there is an inherent risk of contamination and identification of false positives. However, while a single CFU can theoretically be detected on culture, the competitive nature of 16S rRNA sequencing (i.e., an overabundance of DNA amidst a limited number of binding substrates on the sequencer flow cell) renders single CFUs as background noise and inadvertent contaminants will not be detected as dominant taxa in the data. Lastly, clinicians are limited by our still nascent appreciation of these microbial communities. Until there is a better understanding of the lung microbiota in the context of both health and disease, it will be challenging to incorporate sequencing data into clinical decisions.
The development of targeted sequencing techniques highlights limitations associated with standard culture. The belief that the lower airways are sterile is still perpetuated in textbooks; however, the notion of lung sterility has been refuted by a number of studies following the first culture-independent report of the healthy human lung microbiome (27). Healthy dogs and other species have diverse and dynamically changing microbial communities inhabiting specific ecologic niches in the lower airways in the absence of clinical evidence of infection (6, 28–31). The composition of the lung microbiome depends on microbial immigration into the airways, microbial elimination and the relative reproduction rates of host microbial communities determined by regional growth conditions (32). Immigration is influenced by local mucosal extension from the upper airways that lack a physical barrier separating them from the lower airways, microaspiration, and inhalation of bacteria from ambient air (8, 33). Protective reflexes, such as cough, mucociliary function, and innate and adaptive mucosal immune responses affect elimination (8). Although bacterial pneumonia has been regarded as resulting from invasion and growth of a pathogen in the lungs, recent work suggests a primary driver of disease is disruption of homeostasis of the complex microbial ecosystem (34). The upper respiratory tract has been called the gatekeeper to respiratory health, wherein “colonization resistance” is provided by local bacterial communities preventing establishment of mucosal infections capable of spreading to the lower respiratory tract. Thus, while the airway microbiome has the capacity to blunt growth of pathogenic species during states of equilibrium, dysbiosis of upper airways in humans has been linked to CAP (35), SBP (36), and ventilator associated pneumonia (22). In the subset of dogs with paired OP and BALF samples, 3 dogs had CAP, and 8 had SBP. The dogs with CAP were more likely to have a single OTU predominate. In contrast, dogs with SBP were more likely to have ≥2 OTUs. It is interesting to note that in several cases (G3, M1, M2), one of the OTUs found in BALF at a relative abundance of >10% was also present in the OP at an even greater relative abundance. Taking into consideration an underlying process disrupting homeostasis in SBP, it was not surprising that dogs with SBP had more similar microbial compositions of the upper and lower airways (i.e., more evident regional continuity) and greater diversity than dogs with CAP.
While traditional culture methods provide evidence of live and cultivable bacteria in a sample and allow antimicrobial susceptibility testing, 16S rRNA sequencing provides a more comprehensive profile of taxa present in a sample, whether or not they are viable or cultivable. Additionally, these data highlight that just as lack of growth does not imply a sterile environment, identification of a particular organism with either approach does not imply that this organism is the causative agent of disease (37). Lack of growth could also be related to the presence of fastidious organisms, such as Mycobacterium that require specific media or growth conditions using standard methods as it has been documented in people with pneumonia (38) Bacterial culture with sensitivity testing guides treatment of clinical infections and thus will still play a key role in therapy of bacterial pneumonia. However, culture-independent techniques may provide greater depth of understanding of the changes in microbial composition that occur in bacterial pneumonia. These methods could allow for identification of pathogens that may not be readily cultivable, help discriminate true pathogens from colonizing bacteria (37) and provide insight into potential treatment strategies that restore balance toward a microbial population associated with health.
Conclusions
In comparing standard culture and targeted sequencing techniques to identify organisms found in BALF of dogs with bacterial pneumonia, we demonstrated discrepancies between these techniques in terms of presence or absence of predominating taxa and numbers of unique bacteria. Dysbiosis of the respiratory microbiome is a key feature of canine pneumonia, with decreased relative abundance of bacterial community members found in health. Additionally, there appears to be greater regional continuity between the upper and lower airways in dogs with SBP. While much more commonly observed in dogs with CAP than SBP, obliteration of microbial diversity with evidence of overgrowth of one organism was noted in one-third of the dogs in this study. This may suggest that loss of dominant species associated with health could underlie disease pathology. Clinical application of DNA sequencing may be employed if culture is negative in dogs with compatible clinical signs and septic suppurative BALF cytology, or if targeted antimicrobial therapy against the cultivable bacteria fails to produce disease resolution. Future studies aimed at restoring a dysbiotic airway microbiome in canine bacterial pneumonia are warranted.
Data Availability Statement
All sequence data have been deposited in the NCBIA Sequence Read Archive (SRA) under the BioProject accession number: PRJNA510415.
Ethics Statement
All studies were performed in accordance with the Guide for the Use and Care of Laboratory Animals, and were approved by the University of Missouri Institutional Animal Care and Use Committee (MU IACUC protocol # 8240).
Author Contributions
AV-P participated in the conception and design of the study, sample collection, DNA extraction, data interpretation, and co-authored the manuscript. AE participated in the conception and design of the study, contributed resources, interpreted sequence data, and co-authored the manuscript. CR participated in conception and design of the study, sample collection, data interpretation, and co-authored the manuscript. HR assisted with DNA extraction, sample collection, and study coordination. All authors read and approved the final manuscript.
Conflict of Interest
The authors declare that the research was conducted in the absence of any commercial or financial relationships that could be construed as a potential conflict of interest.
Acknowledgments
The authors wish to acknowledge Matt Haight and Savannah Smith for their assistance in sample collection and Karen Clifford for assistance formatting figures. AE is supported by K01 (OD019924) from the NIH, Office of Research Infrastructure Programs.
Abbreviations
BALF, bronchoalveolar lavage fluid; CAP, community-acquired pneumonia; OUT, operational taxonomic unit; PCoA, principal coordinate analysis; RA, relative abundance; SBP, secondary bacterial pneumonia.
References
1. McCullers JA, English BK. Improving therapeutic strategies for secondary bacterial pneumonia following influenza. Fut Microbiol. (2008) 3:397–404. doi: 10.2217/17460913.3.4.397
2. Polsky B, Gold JW, Whimbey E, Dryjanski J, Brown AE, Schiffman G, et al. Bacterial pneumonia in patients with the acquired immunodeficiency syndrome. Ann Intern Med. (1986) 104:38–41. doi: 10.7326/0003-4819-104-1-38
3. Jones RN. Microbial etiologies of hospital-acquired bacterial pneumonia and ventilator-associated bacterial pneumonia. Clin Infect Dis. (2010) 51:S81–87. doi: 10.1086/653053
4. Erb-Downward JR, Thompson DL, Han MK, Freeman CM, McCloskey L, Schmidt LA, et al. Analysis of the lung microbiome in the “healthy” smoker and in COPD. PLoS ONE. (2011) 6:e16384. doi: 10.1371/journal.pone.0016384
5. Vientos-Plotts AI, Ericsson AC, Rindt H, Reinero CR. Oral probiotics alter healthy feline respiratory microbiota. Front Microbiol. (2017) 8:1287. doi: 10.3389/fmicb.2017.01287
6. Ericsson AC, Personett AR, Grobman ME, Rindt H, Reinero CR. Composition and predicted metabolic capacity of upper and lower airway microbiota of healthy dogs in relation to the fecal microbiota. PLoS ONE. (2016) 11:e0154646. doi: 10.1371/journal.pone.0154646
7. Glendinning L, Collie D, Wright S, Rutherford KMD, McLachlan G. Comparing microbiotas in the upper aerodigestive and lower respiratory tracts of lambs. Microbiome. (2017) 5:145. doi: 10.1186/s40168-017-0364-5
8. Dickson RP, Erb-Downward JR, Martinez FJ, Huffnagle GB. The microbiome and the respiratory tract. Annu Rev Physiol. (2016) 78:481–504. doi: 10.1146/annurev-physiol-021115-105238
9. Patel JB. 16S rRNA gene sequencing for bacterial pathogen identification in the clinical laboratory. Mol Diagn. (2001) 6:313–21. doi: 10.2165/00066982-200106040-00012
10. Lamm CG, Ferguson AC, Lehenbauer TW, Love BC. Streptococcal infection in dogs: a retrospective study of 393 cases. Vet Pathol. (2010) 47:387–95. doi: 10.1177/0300985809359601
11. Priestnall SL, Erles K, Brooks HW, Cardwell JM, Waller AS, Paillot R, et al. Characterization of pneumonia due to Streptococcus equi subsp. zooepidemicus in dogs. Clin Vaccine Immunol. (2010) 17:1790–6. doi: 10.1128/CVI.00188-10
12. Dhein CR, Prieur DJ, Riggs MW, Potter KA, Widders PR. Suspected ciliary dysfunction in Chinese Shar Pei pups with pneumonia. Am J Vet Res. (1990) 51:439–46.
13. Yu Z, Morrison M. Improved extraction of PCR-quality community DNA from digesta and fecal samples. BioTechniques. (2004) 36:808–12. doi: 10.2144/04365ST04
14. Wormser GP, Stratton C. Manual of Clinical Microbiology, 9th Edition Edited by Patrick R. Murray, Ellen Jo Baron, James H. Jorgensen, Marie Louise Landry, and Michael A. Pfaller Washington, DC: ASM Press, 2007, 2488 pp., illustrated. $209.95 (hardcover). Clin Infect Dis. (2008) 46:153. doi: 10.1086/524076
15. Caporaso JG, Lauber CL, Walters WA, Berg-Lyons D, Lozupone CA, Turnbaugh PJ, et al. Global patterns of 16S rRNA diversity at a depth of millions of sequences per sample. Proc Natl Acad Sci USA. (2011) 108:4516–22. doi: 10.1073/pnas.1000080107
16. Walters WA, Caporaso JG, Lauber CL, Berg-Lyons D, Fierer N, Knight R. PrimerProspector: de novo design and taxonomic analysis of barcoded polymerase chain reaction primers. Bioinformatics. (2011) 27:1159–61. doi: 10.1093/bioinformatics/btr087
17. Magoc T, Salzberg SL. FLASH: fast length adjustment of short reads to improve genome assemblies. Bioinformatics. (2011) 27:2957–63. doi: 10.1093/bioinformatics/btr507
18. Kuczynski J, Stombaugh J, Walters WA, Gonzalez A, Caporaso JG, Knight R. Using QIIME to analyze 16S rRNA gene sequences from microbial communities. Curr Protoc Bioinformatics. (2011) 36:10.7.1–10.7.20. doi: 10.1002/0471250953.bi1007s36
19. Pruesse E, Quast C, Knittel K, Fuchs BM, Ludwig W, Peplies J, et al. SILVA: a comprehensive online resource for quality checked and aligned ribosomal RNA sequence data compatible with ARB. Nucleic Acids Res. (2007) 35:7188–96. doi: 10.1093/nar/gkm864
20. Quast C, Pruesse E, Yilmaz P, Gerken J, Schweer T, Yarza P, et al. The SILVA ribosomal RNA gene database project: improved data processing and web-based tools. Nucleic Acids Res. (2013) 41:D590–6. doi: 10.1093/nar/gks1219
21. Hammer O, Harper DAT. PAST: paleontological statistics software package for education and data analysis. Palaeontol Electron. (2011) 4:1–9.
22. Kitsios GD, Fitch A, Manatakis DV, Rapport SF, Li K, Qin S, et al. Respiratory microbiome profiling for etiologic diagnosis of pneumonia in mechanically ventilated patients. Front Microbiol. (2018) 9:1413. doi: 10.3389/fmicb.2018.01413
23. Canonne AM, Billen F, Tual C, Ramery E, Roels E, Peters I, et al. Quantitative PCR and cytology of bronchoalveolar lavage fluid in dogs with Bordetella bronchiseptica infection J Vet Intern Med. (2016) 30:1204–9. doi: 10.1111/jvim.14366
24. Shankar J, Nguyen MH, Crespo MM, Kwak EJ, Lucas SK, McHugh KJ, et al. Looking beyond respiratory cultures: microbiome-cytokine signatures of bacterial pneumonia and tracheobronchitis in lung transplant recipients. Am J Transplant. (2016) 16:1766–78. doi: 10.1111/ajt.13676
25. Blaser MJ, Falkow S. What are the consequences of the disappearing human microbiota? Nat Rev Microbiol. (2009) 7:887–94. doi: 10.1038/nrmicro2245
26. Chalker VJ, Toomey C, Opperman S, Brooks HW, Ibuoye MA, Brownlie J, et al. Respiratory disease in kennelled dogs: serological responses to Bordetella bronchiseptica lipopolysaccharide do not correlate with bacterial isolation or clinical respiratory symptoms. Clin Diagn Lab Immunol. (2003) 10:352–6. doi: 10.1128/CDLI.10.3.352-356.2003
27. Hilty M, Burke C, Pedro H, Cardenas P, Bush A, Bossley C, et al. Disordered microbial communities in asthmatic airways. PLoS ONE. (2010) 5:e8578. doi: 10.1371/journal.pone.0008578
28. Bassis CM, Erb-Downward JR, Dickson RP, Freeman CM, Schmidt TM, Young VB, et al. Analysis of the upper respiratory tract microbiotas as the source of the lung and gastric microbiotas in healthy individuals. mBio. (2015) 6:e00037. doi: 10.1128/mBio.00037-15
29. Glendinning L, Wright S, Pollock J, Tennant P, Collie D, McLachlan G. Variability of the sheep lung microbiota. Appl Environ Microbiol. (2016) 82:3225–38. doi: 10.1128/AEM.00540-16
30. Man WH, de Steenhuijsen Piters WA, Bogaert D. The microbiota of the respiratory tract: gatekeeper to respiratory health. Nat Rev Microbiol. (2017) 15:259–70. doi: 10.1038/nrmicro.2017.14
31. Vientos-Plotts AI, Ericsson AC, Rindt H, Grobman ME, Graham A, Bishop K, et al. Dynamic changes of the respiratory microbiota and its relationship to fecal and blood microbiota in healthy young cats. PLoS ONE. (2017) 12:e0173818. doi: 10.1371/journal.pone.0173818
32. Dickson RP, Huffnagle GB. The lung microbiome: new principles for respiratory bacteriology in health and disease. PLoS Pathog. (2015) 11:e1004923. doi: 10.1371/journal.ppat.1004923
33. Segal LN, Clemente JC, Tsay JC, Koralov SB, Keller BC, Wu BG, et al. Enrichment of the lung microbiome with oral taxa is associated with lung inflammation of a Th17 phenotype. Nat Microbiol. (2016) 1:16031. doi: 10.1038/nmicrobiol.2016.31
34. Dickson RP, Erb-Downward JR, Huffnagle GB. Towards an ecology of the lung: new conceptual models of pulmonary microbiology and pneumonia pathogenesis. Lancet Respir Med. (2014) 2:238–46. doi: 10.1016/S2213-2600(14)70028-1
35. de Steenhuijsen Piters WA, Huijskens EG, Wyllie AL, Biesbroek G, van den Bergh MR, Veenhoven RH, et al. Dysbiosis of upper respiratory tract microbiota in elderly pneumonia patients. Isme J. (2016) 10:97–108. doi: 10.1038/ismej.2015.99
36. Lu HF, Li A, Zhang T, Ren ZG, He KX, Zhang H, et al. Disordered oropharyngeal microbial communities in H7N9 patients with or without secondary bacterial lung infection. Emerg Microbes Infect. (2017) 6:e112. doi: 10.1038/emi.2017.101
37. Kawanami T, Yatera K, Yamasaki K, Noguchi S, Fukuda K, Akata K, et al. Clinical impact of methicillin-resistant staphylococcus aureus on bacterial pneumonia: cultivation and 16S ribosomal RNA gene analysis of bronchoalveolar lavage fluid. BMC Infect Dis. (2016) 16:155. doi: 10.1186/s12879-016-1493-3
Keywords: microbiota, pneumonia, respiratory, dog, bronchoalveolar lavage, culture, sequencing, dysbiosis
Citation: Vientós-Plotts AI, Ericsson AC, Rindt H and Reinero CR (2019) Respiratory Dysbiosis in Canine Bacterial Pneumonia: Standard Culture vs. Microbiome Sequencing. Front. Vet. Sci. 6:354. doi: 10.3389/fvets.2019.00354
Received: 04 February 2019; Accepted: 26 September 2019;
Published: 11 October 2019.
Edited by:
Timothy J. Johnson, University of Minnesota Twin Cities, United StatesReviewed by:
Dilip Kumar Garikipati, Cleveland Clinic, United StatesHyeun Bum Kim, Dankook University, South Korea
Copyright © 2019 Vientós-Plotts, Ericsson, Rindt and Reinero. This is an open-access article distributed under the terms of the Creative Commons Attribution License (CC BY). The use, distribution or reproduction in other forums is permitted, provided the original author(s) and the copyright owner(s) are credited and that the original publication in this journal is cited, in accordance with accepted academic practice. No use, distribution or reproduction is permitted which does not comply with these terms.
*Correspondence: Aaron C. Ericsson, ZXJpY3Nzb25hQG1pc3NvdXJpLmVkdQ==