Insulin-Producing Cell Transplantation Platform for Veterinary Practice
- 1Veterinary Stem Cell and Bioengineering Innovation Center (VSCBIC), Veterinary Pharmacology and Stem Cell Research Laboratory, Faculty of Veterinary Science, Chulalongkorn University, Bangkok, Thailand
- 2Department of Veterinary Anatomy, Faculty of Veterinary Medicine, Universitas Airlangga, Surabaya, Indonesia
- 3Department of Anatomy, Faculty of Veterinary Science, Chulalongkorn University, Bangkok, Thailand
- 4Department of Surgery, Faculty of Veterinary Science, Chulalongkorn University, Bangkok, Thailand
- 5Veterinary Clinical Stem Cell and Bioengineering Research Unit, Faculty of Veterinary Science, Chulalongkorn University, Bangkok, Thailand
- 6Department of Pharmacology, Faculty of Veterinary Science, Chulalongkorn University, Bangkok, Thailand
Diabetes mellitus (DM) remains a global concern in both human and veterinary medicine. Type I DM requires prolonged and consistent exogenous insulin administration to address hyperglycemia, which can increase the risk of diabetes complications such as retinopathy, nephropathy, neuropathy, and heart disorders. Cell-based therapies have been successful in human medicine using the Edmonton protocol. These therapies help maintain the production of endogenous insulin and stabilize blood glucose levels and may possibly be adapted to veterinary clinical practice. The limited number of cadaveric pancreas donors and the long-term use of immunosuppressive agents are the main obstacles for this protocol. Over the past decade, the development of potential therapies for DM has mainly focused on the generation of effective insulin-producing cells (IPCs) from various sources of stem cells that can be transplanted into the body. Another successful application of stem cells in type I DM therapies is transplanting generated IPCs. Encapsulation can be an alternative strategy to protect IPCs from rejection by the body due to their immunoisolation properties. This review summarizes current concepts of IPCs and encapsulation technology for veterinary clinical application and proposes a potential stem-cell-based platform for veterinary diabetic regenerative therapy.
Introduction
Diabetes mellitus (DM), a chronic metabolic disease, is caused by the pancreas' inability to produce/secrete insulin or the inability of the body to use insulin effectively. There are two main types of DM in the veterinary field. Type I DM, formerly classified as insulin-dependent diabetes, is associated with immunological disorders. Defects in pancreatic islet cells are mediated by autoimmune disorders, resulting in the absence of insulin. Type II DM, formerly classified as non-insulin-dependent diabetes, is caused by ineffective pancreatic insulin secretion and/or insulin usage by the body (1). Type I DM requires prolonged and consistent exogenous insulin administration to address hyperglycemia, which can increase the risk of diabetes complications, such as retinopathy, nephropathy, neuropathy, and heart disorders. Hyperglycemia is a common clinical sign in diabetic patients and can lead to diabetes-related complications (2, 3). In human medicine, the first successful pancreatic islet transplantation using the Edmonton protocol occurred in 2000 (4–6). This transplantation can maintain the production of endogenous insulin and stabilize the blood glucose of patients with type I DM (4). Obstacles to applying the Edmonton protocol have also been reported, including the limited number of cadaveric pancreas donors and the long-term use of immunosuppressive agents. Many investigators have raised concerns about the side effects of long-term use of steroids or immunosuppressants (5, 6). Currently, there is no effective strategy to treat DM in veterinary practice. The current strategy is to use a combination of exogenous insulin, non-insulin therapeutic agents, and diet management (7). However, successful cell-based therapies in human medicine using the Edmonton protocol can likely be adapted to veterinary clinical practice. Therefore, an effective protocol is needed to overcome the limitations of the Edmonton protocol. This protocol is focused on an alternative source of beta cells by generating IPCs from stem cells and a delivery device that can protect the generated IPCs from immune attacks by encapsulation.
DM in Veterinary Medicine
An epidemiological study on DM in the veterinary field reported that the prevalence of DM in dogs in the UK from August 2009 to June 2012 was 0.34%. These data were obtained from 128,210 dogs that visited veterinary clinics in the UK. A higher risk of DM was reported for neutered male dogs (8). For 193,435 cats in the UK (2009–2014), the prevalence of DM was 0.58%. The risk factors of this disease are obesity (above 4 kg of cat body weight) and age (above 6 years old) (9). Type 1 DM, which has the specific feature of the destruction of beta cells due to autoimmune disorder, commonly occurs in geriatric dogs. Unlike in dogs, human type I DM usually occurs in youths or children. Type II DM, which is characterized by the presence of amyloid, very commonly occurs in cats (10). Type 1 DM in dog is characterized by persistent hyperglycemia due to insulin deficiency. The clinical manifestation of canine type I DM is similar to that in humans. Some key differences between human and canine type I DM are that, in the pancreatic histological finding, insulitis is not found in canine type I DM and that the most common complications of canine type I DM are cataract and retinopathy compared with microvascular disease and atherosclerotic cardiovascular disease (ASCVD) in humans (11, 12). Many researchers have established the genetic factor in dogs with type I DM. A study of dogs showed that groups of dog leukocyte antigen (DLA) haplotypes likely play a role in type I DM, such as DLA-DRB1*009-DQA1*001-DQB1*008 (DRB1*009), DLA-DRB1*015-DQA1*006-DQB1*0.23 (DRB1*015), and DLA-DRB1*002-DQA1*009-DQB1*001 (DRB1*002) (13, 14). Another finding also showed that the haplotype gene of DLA-DQA1*004-DQB1*013, which is responsible for DM protection, is also reduced. In Samoyed, the breed of dog with the highest risk of type I DM, the DLA-DRB1*009 and DLA-DRB1*015 haplotype genes are frequently expressed, while DLA-DRB1*015 is commonly expressed in Cairn and Tibetan terriers, which causes the same condition. In contrast, in Golden retrievers, Boxers, and German shepherds, which have a lower risk of type I DM, the DLA-DRB1*009 haplotype gene is not expressed (15). Due to the similarity between human and canine type I DM, many researchers use dogs as a translational animal model with some consideration. Naturally occurring DM in dogs is more preferable compared with induced DM (pancreatectomy and/or chemical induction) (11, 12). Table 1 summarizes the advantages and disadvantages of a diabetes model in dogs.
Although DM is caused by complex factors, this disease can still be treated. Currently, in the veterinary field, therapy management of DM aims to control blood glucose under the renal threshold for at least a 24-h period and prevent the occurrence of hypoglycemia. The American Animal Hospital Association (AAHA) recommends a combination of insulin product, non-insulin therapeutic agents (sulfonylureas, α-glycosidase inhibitors, or incretins), and dietary management to treat DM. As in human medicine, exogenous insulin preparations can be obtained from porcine insulin zinc suspension (intermediate acting) and recombinant DNA-originated human insulin (long-acting). However, the successful therapy of DM in the veterinary field requires commitment and effort by the veterinarian, patient, and owner, which is the main challenge. Moreover, an effective monitoring system to maintain stable blood glucose is also needed, which poses another challenge (7). Table 2 summarizes the conventional approaches for DM therapy in dogs.
Stem-cell-based therapies are growing rapidly in the veterinary field. However, stem-cell-based therapies in the veterinary field are mainly used for curing musculoskeletal injuries in sport and companion animals (23, 24). The first clinical application of stem-cell-based therapy in the veterinary field was in 2003. Smith et al. isolated bone-marrow-derived mesenchymal stem cells (BM-MSCs) and performed an autotransplantation in the injured tendon of an 11-year-old polo pony horse (25). Until present, no study has reported the clinical application of stem cell therapy to treat DM in the veterinary field. In 2011, genetically modified BM-MSCs carrying insulin genes were studied for treating type 1 DM in humans using Beagle dogs as an animal model (26).
The ethical issues are still hampering the application of regenerative medicine in the veterinary field. Legalization of manufacturing standard operational procedure (SOP), marketing requirements for cell-based veterinary pharmaceuticals, and veterinary medical prescription requirements for cell-based veterinary pharmaceutical products are not well-developed. A genetic engineering approach that might be used for the production of cell-based veterinary pharmaceutical product is one of the additional obstacles for its translation in veterinary clinics. Pharmaceutical products containing genetically modified organisms (GMOs) have not been authorized under pharmaceutical law (27). According to the study of the economic aspects of beta-cell replacement therapy in humans, the high cost of therapy using islet transplantation was notable (28). This financial issue also makes another obstacle for clinical application in the veterinary field. To cope with this issue, the optimized mass production of autogenic stem-cell-derived beta cells will eliminate this problem by reducing the manufacturing cost (28).
Transplantation Platform for Insulin-Producing Cells (IPCs)
Adult Stem Cells vs. Induced Pluripotent Stem Cells (iPSCs)
Adult stem cells, known as resident stem cells or tissue-restricted stem cells, are undifferentiated cells that can be obtained from differentiated tissues in postnatal animals. This type of cell is needed to keep the tissue or organ in a physiological state (tissue regeneration/renewal), but many researchers have shown that adult stem cells have the ability to differentiate toward various types of cells (29–31). This proved that adult stem cells can be classified as multipotent stem cells. They have the ability to self-renew and to differentiate toward mature cells of the neighboring tissues (32). These stem cells can be obtained from various tissues, such as the bone marrow, skin, retina, brain, pancreas, intestinal crypts, skeletal muscle, and liver (33). Among the various types of adult stem cells, hematopoietic and mesenchymal stem cells are the most promising for regenerative therapy (34). Kim and Park (35) reported the advantages of adult stem cells, including that they can be obtained from various types of body tissues, require less complicated isolation and manipulation protocols, have multipotency properties and immunomodulation abilities, and can be used in autogenic or allogeneic transplantation. Adult stem cells also avoid ethical issues since they do not involve the embryonic stage in contrast to embryonic stem cells (ESCs) from the inner cell mass of the blastocyst. Moreover, tumorigenicity can be avoided by using adult stem cells, while this advantage cannot be obtained from ESCs or iPSCs (35). Despite these advantages, there are still obstacles to the utilization of adult stem cells for regenerative therapy. Cellular senescence is one of the main issues affecting the potency of proliferation and differentiation (36). There are several approaches to combat senescence in adult stem cells. Some researchers have reported that using lentivirus-carrying telomerase reverse transcriptase (TERT) prolonged the life span of stem cells (37). However, genetic modification for clinical applications should be done with caution due to the potential of tumorigenicity. Rapamycin has been reported as an agent that can reduce senescence through the inhibition of the mTOR signaling pathway (38). In addition, tuning the oxidative stress level can be done in a hypoxic environment (39) and by adding antioxidant agents such as ascorbic acid and N-acetyl cysteine to reduce senescence in adult stem cells (40).
iPSCs are reprogrammed adult (somatic) cells that have similar characteristics as ESCs. iPSCs were developed in response to the controversial issues of ESCs (35). Currently, iPSCs can be generated from various somatic cells from tissues such as the mucosal layer of the gastrointestinal tract, liver, skin fibroblast, nerve cells, and blood cells (41, 42). Yamanaka et al. were the first to investigate iPSCs in 2006. They successfully converted adult mouse fibroblasts to iPSCs using retroviral transfection to transduce selected genes; however, the generated iPSCs could not produce viable chimera (43). In 2007, Yamanaka improved the next generation of iPSCs, as he could produce viable chimeras from generated iPSCs (44). Further, in late 2007, Yamanaka et al. generated human iPSCs using a retroviral system (45). This invention earned him the Nobel Prize in 2012. Serial sets of genes that have been used to reprogram adult cells toward iPSCs, currently known as Yamanaka factors, consist of Oct4, Sox2, c-Myc, and KLF4, while Thomson factors consist of Oct4, Sox2, NANOG, and LIN28 (41). Even though the iPSCs have good potential for clinical applications, there are still three main obstacles. First, the efficiency of reprogramming using both Yamanaka and Thomson factors remains very low. Second, the involvement of retrovirus as a transduction system of selected genes leads to concerns about mutations that can cause tumors. Last, a feeder cell system was involved in culturing human iPSCs, which can introduce immunogenic antigens into human iPSCs (41). A study on tumorigenesis in iPSCs reported that utilizing reprogramming factors could attenuate the tumor suppressor gene p53 and that the failure of cell reprogramming through the p53-dependent apoptosis pathway occurred when the expression of the p53 gene was increased (42).
Generating IPCs
Stem-cell-based therapy for tissue regeneration is mainly aimed to replace damaged cells that cause many various diseases such as congenital disorders (46–48), tissue defects (49–52), autoimmune diseases (53–55), degenerative diseases (56–59), and hematological disorders (60). Adult stem cells were chosen as a promising strategy because they have many advantages, such as a low risk of teratoma formation and no ethical issues, since an embryo is not required to develop this type of cell. MSCs are the most commonly used source for stem-cell-based therapies (61). The special characteristics of MSCs, such as the high ability of cell proliferation, paracrine effect ability, multipotent plasticity, and immunomodulation ability, make MSCs a good candidate for clinical application (62, 63). Despite these advantages of MSCs, some obstacles to clinical application should be considered to maintain the viability, property, and function of the cells (61).
Overcoming the limited number of cadaveric pancreas requires an alternative source of pancreatic islets for type I DM therapies. The endogenous reprogramming of non-beta cells into beta cells is one strategy (64). The conversion of pancreatic acinar cells toward beta cells involves combining three developmental regulators of beta cells, such as NGN3, PDX1, and MafA (65). Another earlier study showed the success of the endogenous reprogramming of alpha cells toward beta cells using adeno-associated virus-carrying PDX1 and MafA (66). In 2006, a new concept was established regarding the induction of somatic cells toward iPSCs, triggering the development of various strategies to reprogram somatic cells (64). In the last decade, there have been several studies regarding the in vitro differentiation of MSCs. A comparative study of chemical induction between BM-MSCs and adipose tissue-derived mesenchymal stem cell (AT-MSC) differentiation toward IPCs showed no difference in terms of gene expression level, C-peptide, and insulin production (67). Another study showed that the combination of induction medium and adenovirus-mediated expression of pancreatic endocrine transcription factors (PDX1, MafA, NGN3, and PAX1) could induce gallbladder and cystic duct primary cells (GBCs) toward pancreatic beta-cell-like structures (68). A study of the differentiation of IPCs obtained from human dental pulp stem cells (hDPSCs) and human periodontal ligament stem cells (hPDLSCs) showed that the hDPSCs had better differentiation ability than hPDLSCs (69). A similar study on human natal dental pulp stem cells (hNDPSCs) also showed their differentiation ability toward IPCs (70). For generating IPCs, Lu et al. (71) reported that IPCs could be generated from various types of cells, such as ESCs, mesenchymal stem cells, iPSCs, and somatic cells (71). Table 3 summarizes the details of the various strategies for generating IPCs from various cell types.
In pancreatic endocrine lineage development, many transcription factors are involved in islet differentiation. The differentiation is initiated from pluripotent or multipotent stem cells. A previous study reported that the transcription factors Oct4, Sox2, NANOG, and Rex1 are expressed as pluripotent stem cell markers (121). This stage is continued by definitive endoderm induction through a bipotential mesendoderm progenitor, which expresses Mixl1 and Brachyury transcription factors. The specific transcription factors Cxcr4, Goosecoid, FoxA2, Sox17, and BMP2 are associated with definitive endoderm induction (122). PDX1 and Hnf6 have been reported to play important roles as pancreatic endoderm markers (123). Another study showed that PDX1 and Ptf1a were representative of the multipotent progenitor markers that are gradually restricted by the expression of NGN3 (all cell types of the endocrine progenitor) (124). Schiesser and Wells reported that transcription factors NGN3, NeuroD1, MafB, Nkx2.2, and Pax4 have important roles in pancreatic endocrine differentiation and that the final stage of beta-cell differentiation is associated with insulin, Nkx6.1, MafA, and Glut2 (122). Another study reported that Nkx2.2 might be a critical regulator. Nkx2.2 is not only involved in the pancreatic progenitor phase but also has an additional function during the endocrine progenitor phase for developing a beta-cell population. The absence of Nkx2.2 in both pancreatic progenitor and endocrine progenitor leads to the absence of beta-cell differentiation (124). Figure 1 summarizes the stages of IPC differentiation. Several signaling pathways are involved in the complexities of lineage differentiation of pancreas. According to the studies, a variety of signaling pathways have already been reported such as Wnt/β-catenin (125), transforming growth factor/TGF-β (126), and notch signaling (69). The absence or reduction of pancreatic endocrine and exocrine cells occurred when Wnt/β-catenin in early pancreatic progenitor was depleted. The endocrine genes during endocrinogenesis, Nkx2.2 and Pax4, were induced by the deletion of Wnt9a. Beta-cell maturation occurred after the differentiation stage; wnt4 and wnt5a were reported to be involved in this step (125). Cell and organ development is also regulated by the TGF-β superfamily protein. The signaling is initiated through the phosphorylation of smad proteins in the cytoplasmic and the translocation of this protein into the nucleus to alter gene transcription. Short-term inhibition of smad2/3 in the cell culture induced Nkx2.2-positive cells and promoted the maturation of endocrine cells (126). In pancreas development, notch signaling also regulates the cell fate. The pool of PDX-1-positive cells in the early stage will be maintained by suppressing NGN3 expression to avoid premature endocrine differentiation. The inhibition of notch signaling by DAPT (γ-secretase inhibitor) promoted the differentiation and maturation of IPCs (69).
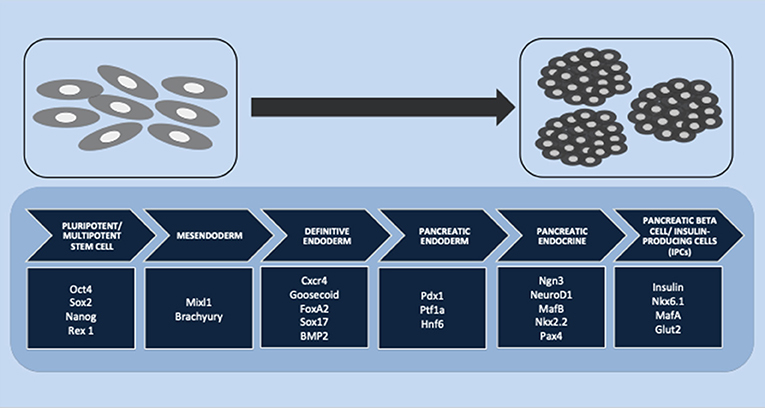
Figure 1. Stages of insulin-producing cell (IPC) differentiation. Multiple stages of IPC differentiation from pluripotent/multipotent stem cells and the role of transcription factors involved in each stage, starting from mesendoderm and definitive endoderm induction, followed by pancreatic endoderm and pancreatic endocrine differentiation, and finally, the final stage of pancreatic beta cells or IPC induction.
Promising Regenerative Therapy for DM
History of Cell Encapsulation
Cell encapsulation is a promising method for immobilizing cells within semipermeable materials that have the immunoisolating ability to avoid rejection by the host immune system. This platform might be suitable for allogeneic or xenogeneic cell transplantation (127–129). Bisceglie performed the first study on cell encapsulation in 1933, which involved encapsulated tumor cells implanted into a pig abdomen (130). This study proved that encapsulation can provide immunoisolation to the encapsulated cells, thereby allowing them to survive for long periods (129). In 1964, Chang used encapsulation to avoid immune attacks from the host. This concept is now called artificial cells. The establishment of this concept led to the first xenograft pancreatic islet transplantation to maintain blood glucose in a diabetic animal model in 1980. In 1994, an adult diabetic man (38 years old) received an allogeneic pancreatic islet transplantation, which was the first clinical trial of encapsulated pancreatic islet in humans (131). Since then, many clinical trials have been performed using various encapsulated cells to cure many diseases, such as the encapsulation of modified xenogeneic cells for delivering ciliary neurotrophic factor (CNTF) in amyotrophic lateral sclerosis (ALS) patients in 1996 (132), delivering CNTF by specific encapsulated cells to treat retinitis pigmentosa in 2002 (133), intravitreal implantation using encapsulated cells in 2017 (134), and intraparenchymal transplantation of encapsulated cells into the brain to deliver nerve growth factor (NGF) to treat patients with Alzheimer's disease (128, 129).
Strategy and Materials for Cell Encapsulation
As a promising strategy, encapsulation technology offers a solution to many obstacles for cell transplantation (128). The main objective of this technology is to provide a protected environment that increases the cells' survival rate and maintains their functions. To achieve this objective, encapsulation should allow the transportation of nutrients and oxygen, which diffuse into the capsule, and the cells' waste and secretory products, which diffuse out from the capsule (127). The cells should be covered by semipermeable materials with specific structures of polymerization and pore size that provide an immunoisolation barrier to avoid rejection (128).
Encapsulation technology is classified based on the geometric structure: microencapsulation encloses the cells with a micron scale, while macroencapsulation encloses the cells at a larger scale, which can also be used to collect and keep the microencapsulated cells in a specific device (127). Microencapsulation provides a large surface area for mass transportation, so the diffusion of both nutrients and secretory or waste products can occur easily. Excessive capsule transplantation might become a concern since it can increase the risk of transplantation failure or complications. While macroencapsulation can act as a diffusion chamber, it has limited mass transportation of nutrients, oxygen, and waste products, which can lead to necrosis in the middle of the capsule. For clinical applications, a combination of microencapsulation and macroencapsulation may be considered to overcome the limitations of each type of encapsulation (135).
Over the past two decades, various kinds of materials have been investigated as sources of material for cell encapsulation. The various encapsulation materials that have been reported for cell encapsulation are alginate (136), agarose (137), chitosan (138), cellulose (139), poly-L-lysine (PLL) (140), collagen, polyethylene glycol, polyurethane, polyethersulfone, sodium polystyrene, polypropylene, and polyacrylate (141). The specific requirements for cell encapsulation materials are that they should be able to keep and maintain the survival rate of encapsulated cells, have a flexible form, have a soft character, be stable, and should allow the transportation of specific molecules (127). Therefore, the most important factors for encapsulation material properties are stability, biocompatibility, and permeability (142). Figure 2 summarizes these important factors for cell encapsulation.
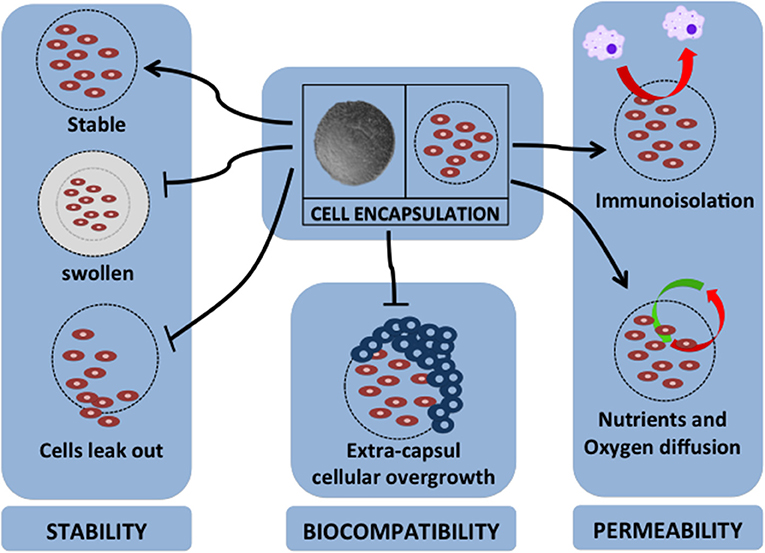
Figure 2. Major factors for cell encapsulation materials. The successful application of cell encapsulation depends on the important factors of encapsulation properties. These properties are as follows: (1) for stability of long-term grafting, the encapsulation material should maintain its own structure in a stable geometrical form, not swell due to the host environment, and should immobilize the cells to prevent leaking; (2) it should have biocompatibility to protect extra-capsular cellular overgrowth that is associated with fibrotic response; (3) it should have permeability for nutrient and oxygen diffusion, provide immunoisolation by avoiding cell-to-cell attachment, and act as barrier for pro-inflammatory cytokines.
A challenge for mammalian cell encapsulation is choosing the appropriate materials to form a suitable environment for the cells to promote the survival rate and maintain the phenotype of the cells (137). Alginate is an encapsulation material that is commonly used in therapeutic applications, so many studies have investigated alginate (143, 144). Many studies have also reported the major property factors of alginate for cell encapsulation. The most common issue is the sensitivity of alginate to destabilization due to the physiological environment of the host. Adding barium ions in gelling solutions can provide stable beads to prevent the cells from leaking out and capsule swelling (145). However, the use of high concentrations of barium ions should be avoided to prevent toxic effects in the host, since an earlier study reported in mice that the presence of barium ions in blood and femur was due to barium concentrations used as a gelling agent in implanted alginate, which caused divalent cations to leak from the alginate beads (146). Regarding the biocompatibility of alginate encapsulation, extra-capsular cellular overgrowth as an impact of fibrosis should be avoided, as it can inhibit the diffusion of nutrients and oxygen (142). Using alginate with a lower G (guluronate) content has been reported to lead to an absence of fibrotic occurrence, compared with using a higher G content (147). However, alginate with a lower G content produces low stability of the encapsulated beads. Combining with an enzyme (mannuronan epimerase) to produce alginate beads with lower G content can generate very stable beads (148). Another strategy to improve the biocompatibility of alginate encapsulation is generating larger beads. Beads of 1.5-mm diameter showed lower fibrosis response compared to 0.5-mm-diameter beads (149). Permeability also plays an important role in alginate encapsulation, so it is important to achieve an appropriate pore size that allows the diffusion of nutrients and oxygen but restricts the entrance of immunoglobulin and other pro-inflammatory cytokines. Using a suitable polycation for the gelling agent and tuning the exposure time can be done for this purpose (150).
Cell Encapsulation for Regenerative Medicine
The rapid development of successful encapsulated cell transplantation in animal models can provide a basic concept for translational studies for clinical applications in human medicine. This was pioneered in a translational study of cell encapsulation therapy for DM (151). Cell encapsulation is mainly aimed at providing an immunoisolation barrier to avoid graft rejection due to non-autologous cell transplantation. Previously, to avoid rejection, long-term immunosuppressive drugs were necessary, but these might cause negative effects such as infections due to the weakened immune system, bone and heart problems, and cancer (151, 152).
Initially, cell encapsulation was studied to provide immunoisolation to cells that produce specific proteins with therapeutic potential to cure diseases. At present, mammalian cell encapsulation has been used to regenerate tissues or organs (152). There have been many studies of various encapsulation materials and various types of cells that can produce therapeutic proteins to treat diseases such as DM, hyperlipidemia, osteoporosis, heart disease, brain tumors, hemophilia, and dwarfism (153–155). Also, other studies have investigated cell encapsulation to repair defects in tissues or organs, such as encapsulated MSCs for bone tissue engineering (156, 157), encapsulated fibroblasts for cardiac disease (158, 159), encapsulated hepatocytes for liver tissue engineering (160–162), and choroid plexus encapsulation for Huntington's disease (127, 128, 152).
Encapsulation Platform for IPCs
In the past decade, there have been many studies on stem-cell-derived IPCs as an alternative to the use of cadaveric pancreatic islet transplantation (163). Today, there are still some obstacles to applying this research into clinical practice. The establishment of a suitable delivery device platform for IPCs is one issue that might concern many investigators. Immunoisolation can be achieved by preventing cell-to-cell contact to avoid the activation of cytotoxic CD8+ cells, thereby avoiding transplant rejection (164, 165) and eliminating the negative effects of the prolonged administration of immunosuppressants (166). For a successful encapsulation strategy, the hydrogel polymer should be biocompatible and permeable to allow oxygen and nutrients to diffuse in and the metabolic waste and secretory product to diffuse out across the membrane (167, 168). An earlier study reported that 0.5–1% alginate could generate a pore diameter range from 7.2 to 8.0 nm. This pore size restricts 21–25 kDa of dextran and 78–103 kDa of protein. Based on this finding, the generated pore will be sufficient to protect the cells inside the capsule from immunoglobulin G protein (169). Moreover, the protective ability can be increased by increasing the concentration of alginate (170).
Alginate has been widely used in biomedical science and in regenerative medicine. The first study of alginate microencapsulation was done in 1980, but the results were not satisfactory due to poor biocompatibility (171). In 1997, another investigator reported that using purified alginate can improve the biocompatibility of the materials (172). With dogs as an animal model and transplantation with the pancreatic islet, the result showed that the alginate encapsulation of this encapsulated pancreatic islet could maintain its function for the long term (174 days) without immunosuppressant administration (165). A similar result was reported by another study involving alginate-encapsulated IPCs in mice (173). An in vitro study of alginate encapsulation in terms of immunological reaction has also been done. In that study, alginate-encapsulated rat MSCs, co-cultured with lymphocytes, induced less secretion of IL-2 (174). A study on MSC encapsulation in alginate hydrogel reported that this encapsulation platform could maintain cell viability in hAD-MSCs (175), enhance osteogenic differentiation (176), and maintain the viability and function of BM-MSC-derived IPCs (177). Another aspect of cell encapsulation is avoiding the protrusion of cells from capsules, which can lead to rejection and fibrotic responses, followed by the necrosis of encapsulated cells. The multilayer immunoisolation encapsulation of alginate and PLL can prevent cell protrusion and improve the surface of capsules (155). Double-layer alginate encapsulation using PLL, chitosan, or PEG can provide a second barrier against the host immune system by decreasing the permeability of larger molecules (174, 178).
Although many studies have been conducted on manipulating the rejection of IPCs, some studies have aimed to cure DM in humans. The utilization of animal models can have a beneficial effect in the veterinary field, since the findings of these studies can be adapted to treat animal DM. The latest study of IPC encapsulation that involved an animal model reported that macroencapsulation using a specific device called “TheraCyte” successfully provided immunoisolation for hBM-MSC-derived IPC transplantation in mongrel dogs; blood glucose level was maintained in the physiological state for the long term (179). Based on the findings of this study, it seems that IPC encapsulation can be applied to clinical practice in the veterinary field.
Conclusion
Until present, there is no effective strategy to treat DM in the veterinary field. Researchers have studied the strategy of generating IPCs and encapsulation technology in both humans and various animal species. The results can be adapted into veterinary clinical applications. MSCs as adult stem cells have been widely used in clinical application in humans, and therefore, they seem to be a good candidate for generating IPCs. Generated IPCs from MSCs can be an alternative to pancreatic islet donors. The ability of MSCs to modulate the immune system allows successful non-autogenic transplantation. Combining this with the promising strategy of encapsulation technology can enhance the viability of IPCs by providing an immunoisolation barrier to avoid graft rejection. Furthermore, the negative effects of prolonged administration of immunosuppressive agents can be eliminated.
Consequently, this strategy can overcome many obstacles to cell transplantation, and this platform might be suitable for allogeneic or xenogeneic cell transplantation in the future. The IPC encapsulation platform can be a promising strategy for DM stem-cell-based therapy in veterinary practice. To fulfill this concept, further studies should be conducted to find an effective strategy for generating the IPCs, select a suitable material, improve encapsulation, and investigate the cell behavior after encapsulation. These studies should be followed by clinical trials in animals to evaluate the functional effect and safety of encapsulation.
Author Contributions
SK reviewed the literature and wrote the manuscript. SS and KK wrote and edited the manuscript. CS conceived the presented idea, wrote and edited the manuscript. All authors read and approved the final version of the manuscript.
Funding
SK was supported by a combined scholarship between the 100th Anniversary Chulalongkorn University Fund for Doctoral Scholarship and Scholarship for International Graduate Students of Chulalongkorn University. CS was supported by the Veterinary Clinical Stem Cell and Bioengineering Research Unit, Ratchadaphiseksomphot Endowment Fund, Chulalongkorn University; the Research Grant, Faculty of Veterinary Science, Chulalongkorn University; and the Government Research Fund.
Conflict of Interest
The authors declare that the research was conducted in the absence of any commercial or financial relationships that could be construed as a potential conflict of interest.
References
1. Gilor C, Niessen SJM, Furrow E, DiBartola SP. What's in a name? Classification of diabetes mellitus in veterinary medicine and why it matters. J Vet Intern Med. (2016) 30:927–40. doi: 10.1111/jvim.14357
2. Rattananinsruang P, Dechsukhum C, Leeanansaksiri W. Establishment of insulin-producing cells from human embryonic stem cells underhypoxic condition for cell based therapy. Front Cell Dev Biol. (2018) 6:49. doi: 10.3389/fcell.2018.00049
3. Iqbal A, Novodvorsky P, Heller SR. Recent updates on type 1 diabetes mellitus management for clinicians. Diabetes Metab J. (2018) 42:3–18. doi: 10.4093/dmj.2018.42.1.3
4. Shapiro AM, Ricordi C, Hering BJ, Auchincloss H, Lindblad R, Robertson RP, et al. International trial of the Edmonton protocol for islet transplantation. N Engl J Med. (2006) 355:1318–30. doi: 10.1056/NEJMoa061267
5. Medical Advisory Secretariat. Islet transplantation: an evidence-based analysis. Ont Health Technol Assess Ser. (2003) 3:1–45.
6. Brennan DC, Kopetskie HA, Sayre PH, Alejandro R, Cagliero E, Shapiro AM, et al. Long-term follow-up of the edmonton protocol of islet transplantation in the United States. Am J Transplant. (2016) 16:509–17. doi: 10.1111/ajt.13458
7. Behrend E, Holford A, Lathan P, Rucinsky R, Schulman R. 2018 AAHA diabetes management guidelines for dogs and cats. J Am Anim Hosp Assoc. (2018) 54:1–21. doi: 10.5326/JAAHA-MS-6822
8. Mattin M, O'Neill D, Church D, McGreevy PD, Thomson PC, Brodbelt D. An epidemiological study of diabetes mellitus in dogs attending first opinion practice in the UK. Vet Rec. (2014) 174:349. doi: 10.1136/vr.101950
9. O'Neill DG, Gostelow R, Orme C, Church DB, Niessen SJM, Verheyen K, et al. Epidemiology of diabetes mellitus among 193,435 cats attending primary-care veterinary practices in England. J Vet Intern Med. (2016) 30:964–72. doi: 10.1111/jvim.14365
10. Hoenig M. Chapter twelve - carbohydrate metabolism and pathogenesis of diabetes mellitus in dogs and cats. In: Tao YX, editor. Progress in Molecular Biology and Translational Science. Vol. 121. Academic Press (2014). p. 377–412. doi: 10.1016/B978-0-12-800101-1.00012-0
11. Moshref M, Tangey B, Gilor C, Papas KK, Williamson P, Loomba-Albrecht L, et al. Concise review: canine diabetes mellitus as a translational model for innovative regenerative medicine approaches. Stem Cells Transl Med. (2019) 8:450–5. doi: 10.1002/sctm.18-0163
12. Adin CA, Gilor C. The diabetic dog as a translational model for human islet transplantation. Yale J Biol Med. (2017) 90:509–15.
13. Niaz K, Maqbool F, Khan F, Hassan FI, Momtaz S, Abdollahi M. Comparative occurrence of diabetes in canine, feline, and few wild animals and their association with pancreatic diseases and ketoacidosis with therapeutic approach. Vet World. (2018) 11:410–22. doi: 10.14202/vetworld.2018.410-422
14. Catchpole B, Adams JP, Holder AL, Short AD, Ollier WE, Kennedy LJ. Genetics of canine diabetes mellitus: are the diabetes susceptibility genes identified in humans involved in breed susceptibility to diabetes mellitus in dogs? Vet J. (2013) 195:139–47. doi: 10.1016/j.tvjl.2012.11.013
15. Catchpole B, Kennedy LJ, Davison LJ, Ollier WE. Canine diabetes mellitus: from phenotype to genotype. J Small Anim Pract. (2008) 49:4–10. doi: 10.1111/j.1748-5827.2007.00398.x
16. Oda H, Mori A, Ishii S, Shono S, Onozawa E, Sako T. Time-action profiles of insulin degludec in healthy dogs and its effects on glycemic control in diabetic dogs. J Vet Med Sci. (2018) 80:1720–3. doi: 10.1292/jvms.17-0714
17. Fracassi F, Linari G, Del Baldo F, Di Cunzolo A, D'Angelo S, Malerba E, et al. Comparison of lente insulin and NPH insulin therapy for the treatment of newly diagnosed diabetic dogs: a randomised study. Vet Rec. (2018) 183:262. doi: 10.1136/vr.104818
18. Fracassi F, Corradini S, Hafner M, Boretti FS, Sieber-Ruckstuhl NS, Reusch CE. Detemir insulin for the treatment of diabetes mellitus in dogs. J Am Vet Med Assoc. (2015) 247:73–8. doi: 10.2460/javma.247.1.73
19. Hess RS, Drobatz KJ. Glargine insulin for treatment of naturally occurring diabetes mellitus in dogs. J Am Vet Med Assoc. (2013) 243:1154–61. doi: 10.2460/javma.243.8.1154
20. Oda H, Mori A, Lee P, Saeki K, Ishioka K, Arai T, et al. Characterization of the use of liraglutide for glycemic control in healthy and Type 1 diabetes mellitus suffering dogs. Res Vet Sci. (2013) 95:381–8. doi: 10.1016/j.rvsc.2013.04.003
21. Sears KW, Drobatz KJ, Hess RS. Use of lispro insulin for treatment of diabetic ketoacidosis in dogs. J Vet Emerg Crit Care. (2012) 22:211–8. doi: 10.1111/j.1476-4431.2012.00719.x
22. Maggiore AD, Nelson RW, Dennis J, Johnson E, Kass PH. Efficacy of protamine zinc recombinant human insulin for controlling hyperglycemia in dogs with diabetes mellitus. J Vet Intern Med. (2012) 26:109–15. doi: 10.1111/j.1939-1676.2011.00861.x
23. Markoski MM. Advances in the use of stem cells in veterinary medicine: from basic research to clinical practice. Scientifica. (2016) 2016:4516920. doi: 10.1155/2016/4516920
24. Fortier LA, Travis AJ. Stem cells in veterinary medicine. Stem Cell Res Ther. (2011) 2:9. doi: 10.1186/scrt50
25. Smith RK, Korda M, Blunn GW, Goodship AE. Isolation and implantation of autologous equine mesenchymal stem cells from bone marrow into the superficial digital flexor tendon as a potential novel treatment. Equine Vet J. (2003) 35:99–102. doi: 10.2746/042516403775467388
26. Zhu S, Lu Y, Zhu J, Xu J, Huang H, Zhu M, et al. Effects of intrahepatic bone-derived mesenchymal stem cells autotransplantation on the diabetic Beagle dogs. J Surg Res. (2011) 168:213–23. doi: 10.1016/j.jss.2009.10.008
27. Faltus T, Brehm W. Cell-based veterinary pharmaceuticals - basic legal parameters set by the veterinary pharmaceutical law and the genetic engineering law of the European union. Front Vet Sci. (2016) 3:101. doi: 10.3389/fvets.2016.00101
28. Bandeiras C, Hwa AJ, Cabral JMS, Ferreira FC, Finkelstein SN, Gabbay RA. Economics of beta-cell replacement therapy. Curr Diab Rep. (2019) 19:75. doi: 10.1007/s11892-019-1203-9
29. Williams DP. Application of hepatocyte-like cells to enhance hepatic safety risk assessment in drug discovery. Philos Trans R Soc Lond B Biol Sci. (2018) 373:20170228. doi: 10.1098/rstb.2017.0228
30. Chuye LB, Dimitri A, Desai A, Handelmann C, Bae Y, Johari P, et al. Brain organoids: expanding our understanding of human development and disease. Results Probl Cell Differ. (2018) 66:183–206. doi: 10.1007/978-3-319-93485-3_8
31. Zheng W, Li Q, Zhao C, Da Y, Zhang HL, Chen Z. Differentiation of glial cells from hiPSCs: potential applications in neurological diseases and cell replacement therapy. Front Cell Neurosci. (2018) 12:239. doi: 10.3389/fncel.2018.00239
32. Fortier LA. Stem cells: classifications, controversies, and clinical applications. Vet Surg. (2005) 34:415–23. doi: 10.1111/j.1532-950X.2005.00063.x
33. Titushkin I, Sun S, Shin J, Cho M. Physicochemical control of adult stem cell differentiation: shedding light on potential molecular mechanisms. J Biomed Biotechnol. (2010) 2010:743476. doi: 10.1155/2010/743476
34. Dulak J, Szade K, Szade A, Nowak W, Jozkowicz A. Adult stem cells: hopes and hypes of regenerative medicine. Acta Biochim Pol. (2015) 62:329–37. doi: 10.18388/abp.2015_1023
35. Kim HJ, Park JS. Usage of human mesenchymal stem cells in cell-based therapy: advantages and disadvantages. Dev Reprod. (2017) 21:1–10. doi: 10.12717/DR.2017.21.1.001
36. Turinetto V, Vitale E, Giachino C. Senescence in human mesenchymal stem cells: functional changes and implications in stem cell-based therapy. Int J Mol Sci. (2016) 17:E1164. doi: 10.3390/ijms17071164
37. Tang H, Xiang Y, Jiang X, Ke Y, Xiao Z, Guo Y, et al. Dual expression of hTERT and VEGF prolongs life span and enhances angiogenic ability of aged BMSCs. Biochem Biophys Res Commun. (2013) 440:502–8. doi: 10.1016/j.bbrc.2013.09.053
38. Gu Z, Tan W, Ji J, Feng G, Meng Y, Da Z, et al. Rapamycin reverses the senescent phenotype and improves immunoregulation of mesenchymal stem cells from MRL/lpr mice and systemic lupus erythematosus patients through inhibition of the mTOR signaling pathway. Aging. (2016) 8:1102–14. doi: 10.18632/aging.100925
39. Jin Y, Kato T, Furu M, Nasu A, Kajita Y, Mitsui H, et al. Mesenchymal stem cells cultured under hypoxia escape from senescence via down-regulation of p16 and extracellular signal regulated kinase. Biochem Biophys Res Commun. (2010) 391:1471–6. doi: 10.1016/j.bbrc.2009.12.096
40. Lin TM, Tsai JL, Lin SD, Lai CS, Chang CC. Accelerated growth and prolonged lifespan of adipose tissue-derived human mesenchymal stem cells in a medium using reduced calcium and antioxidants. Stem Cells Dev. (2005) 14:92–102. doi: 10.1089/scd.2005.14.92
41. Yu J, Thomson JA. Chapter 30: Induced pluripotent stem cells. In: Lanza R, Langer R, Vacanti J, editors. Principles of Tissue Engineering. Academic Press (2014). p. 581–94. doi: 10.1016/B978-0-12-398358-9.00030-6
42. Xiao B, Ng HH, Takahashi R, Tan EK. Induced pluripotent stem cells in Parkinson's disease: scientific and clinical challenges. J Neurol Neurosurg Psychiatry. (2016) 87:697–702. doi: 10.1136/jnnp-2015-312036
43. Takahashi K, Yamanaka S. Induction of pluripotent stem cells from mouse embryonic and adult fibroblast cultures by defined factors. Cell. (2006) 126:663–76. doi: 10.1016/j.cell.2006.07.024
44. Okita K, Ichisaka T, Yamanaka S. Generation of germline-competent induced pluripotent stem cells. Nature. (2007) 448:313–7. doi: 10.1038/nature05934
45. Yamanaka S, Takahashi K, Okita K, Nakagawa M. Induction of pluripotent stem cells from fibroblast cultures. Nat Protoc. (2007) 2:3081–9. doi: 10.1038/nprot.2007.418
46. Wang A, Brown EG, Lankford L, Keller BA, Pivetti CD, Sitkin NA, et al. Placental mesenchymal stromal cells rescue ambulation in ovine myelomeningocele. Stem Cells Transl Med. (2015) 4:659–69. doi: 10.5966/sctm.2014-0296
47. Gao Y, Jacot JG. Stem cells and progenitor cells for tissue-engineered solutions to congenital heart defects. Biomark Insights. (2015) 10(Suppl. 1):139–46. doi: 10.4137/BMI.S20058
48. Fauza DO. Tissue engineering in congenital diaphragmatic hernia. Semin Pediatr Surg. (2014) 23:135–40. doi: 10.1053/j.sempedsurg.2014.04.004
49. Abd El Aziz MT, Abd El Nabi EA, Abd El Hamid M, Sabry D, Atta HM, Rahed LA, et al. Endothelial progenitor cells regenerate infracted myocardium with neovascularisation development. J Adv Res. (2015) 6:133–44. doi: 10.1016/j.jare.2013.12.006
50. Guijarro D, Lebrin M, Lairez O, Bourin P, Piriou N, Pozzo J, et al. Intramyocardial transplantation of mesenchymal stromal cells for chronic myocardial ischemia and impaired left ventricular function: Results of the MESAMI 1 pilot trial. Int J Cardiol. (2016) 209:258–65. doi: 10.1016/j.ijcard.2016.02.016
51. Jiang MH, Li G, Liu J, Liu L, Wu B, Huang W, et al. Nestin(+) kidney resident mesenchymal stem cells for the treatment of acute kidney ischemia injury. Biomaterials. (2015) 50:56–66. doi: 10.1016/j.biomaterials.2015.01.029
52. Fouad H, Sabry D, Elsetohy K, Fathy N. Therapeutic efficacy of amniotic membrane stem cells and adipose tissue stem cells in rats with chemically induced ovarian failure. J Adv Res. (2016) 7:233–41. doi: 10.1016/j.jare.2015.05.002
53. De Bari C. Are mesenchymal stem cells in rheumatoid arthritis the good or bad guys? Arthritis Res Ther. (2015) 17:113. doi: 10.1186/s13075-015-0634-1
54. Gharibi T, Ahmadi M, Seyfizadeh N, Jadidi-Niaragh F, Yousefi M. Immunomodulatory characteristics of mesenchymal stem cells and their role in the treatment of multiple sclerosis. Cell Immunol. (2015) 293:113–21. doi: 10.1016/j.cellimm.2015.01.002
55. Thakkar UG, Trivedi HL, Vanikar AV, Dave SD. Insulin-secreting adipose-derived mesenchymal stromal cells with bone marrow-derived hematopoietic stem cells from autologous and allogenic sources for type 1 diabetes mellitus. Cytotherapy. (2015) 17:940–7. doi: 10.1016/j.jcyt.2015.03.608
56. Vishwakarma SK, Bardia A, Tiwari SK, Paspala SAB, Khan AA. Current concept in neural regeneration research: NSCs isolation, characterization and transplantation in various neurodegenerative diseases and stroke: a review. J Adv Res. (2014) 5:277–94. doi: 10.1016/j.jare.2013.04.005
57. Choi MY, Yeo SW, Park KH. Hearing restoration in a deaf animal model with intravenous transplantation of mesenchymal stem cells derived from human umbilical cord blood. Biochem Biophys Res Commun. (2012) 427:629–36. doi: 10.1016/j.bbrc.2012.09.111
58. Parmar N, Ahmadi R, Day RM. A novel method for differentiation of human mesenchymal stem cells into smooth muscle-like cells on clinically deliverable thermally induced phase separation microspheres. Tissue Eng Part C Methods. (2015) 21:404–12. doi: 10.1089/ten.tec.2014.0431
59. Marfia G, Campanella R, Navone SE, Zucca I, Scotti A, Figini M, et al. Potential use of human adipose mesenchymal stromal cells for intervertebral disc regeneration: a preliminary study on biglycan-deficient murine model of chronic disc degeneration. Arthritis Res Ther. (2014) 16:457. doi: 10.1186/s13075-014-0457-5
60. Mahmoud HK, Elhaddad AM, Fahmy OA, Samra MA, Abdelfattah RM, El-Nahass YH, et al. Allogeneic hematopoietic stem cell transplantation for non-malignant hematological disorders. J Adv Res. (2015) 6:449–58. doi: 10.1016/j.jare.2014.11.001
61. Liu S, Zhou J, Zhang X, Liu Y, Chen J, Hu B, et al. Strategies to optimize adult stem cell therapy for tissue regeneration. Int J Mol Sci. (2016) 17:982. doi: 10.3390/ijms17060982
62. Trounson A, Thakar RG, Lomax G, Gibbons D. Clinical trials for stem cell therapies. BMC Med. (2011) 9:52. doi: 10.1186/1741-7015-9-52
63. Nauta AJ, Fibbe WE. Immunomodulatory properties of mesenchymal stromal cells. Blood. (2007) 110:3499–506. doi: 10.1182/blood-2007-02-069716
64. Zhou Q, Melton DA. Pancreas regeneration. Nature. (2018) 557:351–8. doi: 10.1038/s41586-018-0088-0
65. Zhou Q, Brown J, Kanarek A, Rajagopal J, Melton DA. In vivo reprogramming of adult pancreatic exocrine cells to beta-cells. Nature. (2008) 455:627–32. doi: 10.1038/nature07314
66. Xiao X, Guo P, Shiota C, Zhang T, Coudriet GM, Fischbach S, et al. Endogenous reprogramming of alpha cells into beta cells, induced by viral gene therapy, reverses autoimmune diabetes. Cell Stem Cell. (2018) 22:78–90.e4. doi: 10.1016/j.stem.2017.11.020
67. Gabr MM, Zakaria MM, Refaie AF, Abdel-Rahman EA, Reda AM, Ali SS, et al. From human mesenchymal stem cells to insulin-producing cells: comparison between bone marrow- and adipose tissue-derived cells. BioMed Res Int. (2017) 2017:3854232. doi: 10.1155/2017/3854232
68. Galivo F, Benedetti E, Wang Y, Pelz C, Schug J, Kaestner KH, et al. Reprogramming human gallbladder cells into insulin-producing β-like cells. PLoS ONE. (2017) 12:e0181812. doi: 10.1371/journal.pone.0181812
69. Sawangmake C, Nowwarote N, Pavasant P, Chansiripornchai P, Osathanon T. A feasibility study of an in vitro differentiation potential toward insulin-producing cells by dental tissue-derived mesenchymal stem cells. Biochem Biophys Res Commun. (2014) 452:581–7. doi: 10.1016/j.bbrc.2014.08.121
70. Suchánek J, Nasry SA, Soukup T. The differentiation potential of human natal dental pulp stem cells into insulin-producing cells. Folia Biol. (2017) 63:132–8.
71. Lu J, Xia Q, Zhou Q. How to make insulin-producing pancreatic beta cells for diabetes treatment. Sci China Life Sci. (2017) 60:239–48. doi: 10.1007/s11427-016-0211-3
72. Bruin JE, Erener S, Vela J, Hu X, Johnson JD, Kurata HT, et al. Characterization of polyhormonal insulin-producing cells derived in vitro from human embryonic stem cells. Stem Cell Res. (2014) 12:194–208. doi: 10.1016/j.scr.2013.10.003
73. Agulnick AD, Ambruzs DM, Moorman MA, Bhoumik A, Cesario RM, Payne JK, et al. Insulin-producing endocrine cells differentiated in vitro from human embryonic stem cells function in macroencapsulation devices in vivo. Stem Cells Transl Med. (2015) 4:1214–22. doi: 10.5966/sctm.2015-0079
74. Russ HA, Parent AV, Ringler JJ, Hennings TG, Nair GG, Shveygert M, et al. Controlled induction of human pancreatic progenitors produces functional beta-like cells in vitro. EMBO J. (2015) 34:1759–72. doi: 10.15252/embj.201591058
75. Aguayo-Mazzucato C, DiIenno A, Hollister-Lock J, Cahill C, Sharma A, Weir G, et al. MAFA and T3 drive maturation of both fetal human islets and insulin-producing cells differentiated from hESC. J Clin Endocrinol Metab. (2015) 100:3651–9. doi: 10.1210/jc.2015-2632
76. Kim Y, Kim H, Ko UH, Oh Y, Lim A, Sohn JW, et al. Islet-like organoids derived from human pluripotent stem cells efficiently function in the glucose responsiveness in vitro and in vivo. Sci Rep. (2016) 6:35145. doi: 10.1038/srep35145
77. Kondo Y, Toyoda T, Ito R, Funato M, Hosokawa Y, Matsui S, et al. Identification of a small molecule that facilitates the differentiation of human iPSCs/ESCs and mouse embryonic pancreatic explants into pancreatic endocrine cells. Diabetologia. (2017) 60:1454–66. doi: 10.1007/s00125-017-4302-7
78. Petersen MBK, Azad A, Ingvorsen C, Hess K, Hansson M, Grapin-Botton A, et al. Single-cell gene expression analysis of a human ESC model of pancreatic endocrine development reveals different paths to beta-cell differentiation. Stem Cell Rep. (2017) 9:1246–61. doi: 10.1016/j.stemcr.2017.08.009
79. Lopez-Beas J, Capilla-Gonzalez V, Aguilera Y, Mellado N, Lachaud CC, Martin F, et al. miR-7 modulates hESC differentiation into insulin-producing beta-like cells and contributes to cell maturation. Mol Ther Nucleic Acids. (2018) 12:463–77. doi: 10.1016/j.omtn.2018.06.002
80. Zhao Q, Yang Y, Hu J, Shan Z, Wu Y, Lei L. Exendin-4 enhances expression of Neurod1 and Glut2 in insulin-producing cells derived from mouse embryonic stem cells. Arch Med Sci. (2016) 12:199–207. doi: 10.5114/aoms.2016.57596
81. Elham H, Mahmoud H. The effect of pancreas islet-releasing factors on the direction of embryonic stem cells towards Pdx1 expressing cells. Appl Biochem Biotechnol. (2018) 186:371–83. doi: 10.1007/s12010-018-2733-3
82. Jafarian A, Taghikhani M, Abroun S, Pourpak Z, Allahverdi A, Soleimani M. Generation of high-yield insulin producing cells from human bone marrow mesenchymal stem cells. Mol Biol Rep. (2014) 41:4783–94. doi: 10.1007/s11033-014-3349-5
83. Czubak P, Bojarska-Junak A, Tabarkiewicz J, Putowski L. A modified method of insulin producing cells' generation from bone marrow-derived mesenchymal stem cells. J Diabetes Res. (2014) 2014:628591. doi: 10.1155/2014/628591
84. Ouyang J, Huang W, Yu W, Xiong W, Mula RV, Zou H, et al. Generation of insulin-producing cells from rat mesenchymal stem cells using an aminopyrrole derivative XW4.4. Chem Biol Interact. (2014) 208:1–7. doi: 10.1016/j.cbi.2013.11.007
85. Kim SY, Kim YR, Park WJ, Kim HS, Jung SC, Woo SY, et al. Characterisation of insulin-producing cells differentiated from tonsil derived mesenchymal stem cells. Differentiation. (2015) 90:27–39. doi: 10.1016/j.diff.2015.08.001
86. Jafarian A, Taghikani M, Abroun S, Allahverdi A, Lamei M, Lakpour N, et al. The generation of insulin producing cells from human mesenchymal stem cells by MiR-375 and anti-MiR-9. PLoS ONE. (2015) 10:e0128650. doi: 10.1371/journal.pone.0128650
87. Seyedi F, Farsinejad A, Moshrefi M, Nematollahi-Mahani SN. In vitro evaluation of different protocols for the induction of mesenchymal stem cells to insulin-producing cells. In Vitro Cell Dev Biol Anim. (2015) 51:866–78. doi: 10.1007/s11626-015-9890-2
88. Nekoei SM, Azarpira N, Sadeghi L, Kamalifar S. In vitro differentiation of human umbilical cord Wharton's jelly mesenchymal stromal cells to insulin producing clusters. World J Clin Cases. (2015) 3:640–9. doi: 10.12998/wjcc.v3.i7.640
89. Khorsandi L, Khodadadi A, Nejad-Dehbashi F, Saremy S. Three-dimensional differentiation of adipose-derived mesenchymal stem cells into insulin-producing cells. Cell Tissue Res. (2015) 361:745–53. doi: 10.1007/s00441-015-2140-9
90. Khorsandi L, Saremy S, Khodadadi A, Dehbashi F. Effects of exendine-4 on the differentiation of insulin producing cells from rat adipose-derived mesenchymal stem cells. Cell J. (2016) 17:720–9. doi: 10.22074/cellj.2016.3844
91. Pokrywczynska M, Lewandowska MA, Krzyzanowska S, Jundzill A, Rasmus M, Warda K, et al. Transdifferentiation of bone marrow mesenchymal stem cells into the islet-like cells: the role of extracellular matrix proteins. Arch Immunol Ther Exp. (2015) 63:377–84. doi: 10.1007/s00005-015-0340-3
92. Nadri S, Barati G, Mostafavi H, Esmaeilzadeh A, Enderami SE. Differentiation of conjunctiva mesenchymal stem cells into secreting islet beta cells on plasma treated electrospun nanofibrous scaffold. Artif Cells Nanomed Biotechnol. (2018) 46(Suppl. 1):178–87. doi: 10.1080/21691401.2017.1416391
93. Mu XP, Ren LQ, Yan HW, Zhang XM, Xu TM, Wei AH, et al. Enhanced differentiation of human amniotic fluid-derived stem cells into insulin-producing cells in vitro. J Diabetes Investig. (2017) 8:34–43. doi: 10.1111/jdi.12544
94. Piran M, Enderami SE, Piran M, Sedeh HS, Seyedjafari E, Ardeshirylajimi A. Insulin producing cells generation by overexpression of miR-375 in adipose-derived mesenchymal stem cells from diabetic patients. Biologicals. (2017) 46:23–8. doi: 10.1016/j.biologicals.2016.12.004
95. Enderami SE, Soleimani M, Mortazavi Y, Nadri S, Salimi A. Generation of insulin-producing cells from human adipose-derived mesenchymal stem cells on PVA scaffold by optimized differentiation protocol. J Cell Physiol. (2018) 233:4327–37. doi: 10.1002/jcp.26266
96. Sun LL, Liu TJ, Li L, Tang W, Zou JJ, Chen XF, et al. Transplantation of betatrophin-expressing adipose-derived mesenchymal stem cells induces beta-cell proliferation in diabetic mice. Int J Mol Med. (2017) 39:936–48. doi: 10.3892/ijmm.2017.2914
97. Xu L, Xu C, Zhou S, Liu X, Wang J, Liu X, et al. PAX4 promotes PDX1-induced differentiation of mesenchymal stem cells into insulin-secreting cells. Am J Transl Res. (2017) 9:874–86.
98. Shaer A, Azarpira N, Karimi MH. Differentiation of human induced pluripotent stem cells into insulin-like cell clusters with miR-186 and miR-375 by using chemical transfection. Appl Biochem Biotechnol. (2014) 174:242–58. doi: 10.1007/s12010-014-1045-5
99. Yabe SG, Fukuda S, Takeda F, Nashiro K, Shimoda M, Okochi H. Efficient generation of functional pancreatic beta-cells from human induced pluripotent stem cells. J Diabetes. (2017) 9:168–79. doi: 10.1111/1753-0407.12400
100. Enderami SE, Mortazavi Y, Soleimani M, Nadri S, Biglari A, Mansour RN. Generation of insulin-producing cells from human-induced pluripotent stem cells using a stepwise differentiation protocol optimized with platelet-rich plasma. J Cell Physiol. (2017) 232:2878–86. doi: 10.1002/jcp.25721
101. Rajaei B, Shamsara M, Sanati MH. In vitro generation of glucose-responsive insulin-secreting cells from pancreatic and duodenal homeobox 1-overexpressing human-induced pluripotent stem cell derived from diabetic patient. ASAIO J. (2017) 64:819–26. doi: 10.1097/MAT.0000000000000728
102. Rajaei B, Shamsara M, Amirabad LM, Massumi M, Sanati MH. Pancreatic endoderm-derived from diabetic patient-specific induced pluripotent stem cell generates glucose-responsive insulin-secreting cells. J Cell Physiol. (2017) 232:2616–25. doi: 10.1002/jcp.25459
103. Gupta SK, Wesolowska-Andersen A, Ringgaard AK, Jaiswal H, Song L, Hastoy B, et al. NKX6.1 induced pluripotent stem cell reporter lines for isolation and analysis of functionally relevant neuronal and pancreas populations. Stem Cell Res. (2018) 29:220–31. doi: 10.1016/j.scr.2018.04.010
104. Abazari MF, Soleimanifar F, Nouri Aleagha M, Torabinejad S, Nasiri N, Khamisipour G, et al. PCL/PVA nanofibrous scaffold improve insulin-producing cells generation from human induced pluripotent stem cells. Gene. (2018) 671:50–7. doi: 10.1016/j.gene.2018.05.115
105. Mobarra N, Soleimani M, Pakzad R, Enderami SE, Pasalar P. Three-dimensional nanofiberous PLLA/PCL scaffold improved biochemical and molecular markers hiPS cell-derived insulin-producing islet-like cells. Artif Cells Nanomed Biotechnol. (2018) 46(Suppl. 3):S685–92. doi: 10.1080/21691401.2018.1505747
106. Chakravarthy H, Gu X, Enge M, Dai X, Wang Y, Damond N, et al. Converting adult pancreatic islet alpha cells into beta cells by targeting both dnmt1 and arx. Cell Metab. (2017) 25:622–34. doi: 10.1016/j.cmet.2017.01.009
107. Hill CM, Banga A, Abrahante JE, Yuan C, Mutch LA, Janecek J, et al. Establishing a large-animal model for in vivo reprogramming of bile duct cells into insulin-secreting cells to treat diabetes. Hum Gene Ther Clin Dev. (2017) 28:87–95. doi: 10.1089/humc.2017.011
108. Sasaki S, Miyatsuka T, Matsuoka TA, Takahara M, Yamamoto Y, Yasuda T, et al. Activation of GLP-1 and gastrin signalling induces in vivo reprogramming of pancreatic exocrine cells into beta cells in mice. Diabetologia. (2015) 58:2582–91. doi: 10.1007/s00125-015-3728-z
109. Klein D, Alvarez-Cubela S, Lanzoni G, Vargas N, Prabakar KR, Boulina M, et al. BMP-7 induces adult human pancreatic exocrine-to-endocrine conversion. Diabetes. (2015) 64:4123–34. doi: 10.2337/db15-0688
110. Lemper M, Leuckx G, Heremans Y, German MS, Heimberg H, Bouwens L, et al. Reprogramming of human pancreatic exocrine cells to beta-like cells. Cell Death Differ. (2015) 22:1117–30. doi: 10.1038/cdd.2014.193
111. Cavelti-Weder C, Li W, Zumsteg A, Stemann-Andersen M, Zhang Y, Yamada T, et al. Hyperglycaemia attenuates in vivo reprogramming of pancreatic exocrine cells to beta cells in mice. Diabetologia. (2016) 59:522–32. doi: 10.1007/s00125-015-3838-7
112. Lu J, Dong H, Lin L, Wang Q, Huang L, Tan J. miRNA-302 facilitates reprogramming of human adult hepatocytes into pancreatic islets-like cells in combination with a chemical defined media. Biochem Biophys Res Commun. (2014) 453:405–10. doi: 10.1016/j.bbrc.2014.09.095
113. Luo H, Chen R, Yang R, Liu Y, Chen Y, Shu Y, et al. Reprogramming of mice primary hepatocytes into insulin-producing cells by transfection with multicistronic vectors. J Diabetes Res. (2014) 2014:716163. doi: 10.1155/2014/716163
114. Donelan W, Li S, Wang H, Lu S, Xie C, Tang D, et al. Pancreatic and duodenal homeobox gene 1 (Pdx1) down-regulates hepatic transcription factor 1 alpha (HNF1alpha) expression during reprogramming of human hepatic cells into insulin-producing cells. Am J Transl Res. (2015) 7:995–1008.
115. Yang XF, Ren LW, Yang L, Deng CY, Li FR. In vivo direct reprogramming of liver cells to insulin producing cells by virus-free overexpression of defined factors. Endocr J. (2017) 64:291–302. doi: 10.1507/endocrj.EJ16-0463
116. Pan G, Hao H, Liu J. Induction of hepatocytes-derived insulin-producing cells using small molecules and identification of microRNA profiles during this procedure. Biochem Biophys Res Commun. (2018) 498:646–53. doi: 10.1016/j.bbrc.2018.03.036
117. Chen YJ, Finkbeiner SR, Weinblatt D, Emmett MJ, Tameire F, Yousefi M, et al. De novo formation of insulin-producing “neo-beta cell islets” from intestinal crypts. Cell Rep. (2014) 6:1046–58. doi: 10.1016/j.celrep.2014.02.013
118. Duan FF, Liu JH, March JC. Engineered commensal bacteria reprogram intestinal cells into glucose-responsive insulin-secreting cells for the treatment of diabetes. Diabetes. (2015) 64:1794–803. doi: 10.2337/db14-0635
119. Zhang M, Lin Q, Qi T, Wang T, Chen CC, Riggs AD, et al. Growth factors and medium hyperglycemia induce Sox9+ ductal cell differentiation into beta cells in mice with reversal of diabetes. Proc Natl Acad Sci USA. (2016) 113:650–5. doi: 10.1073/pnas.1524200113
120. Rhee M, Lee SH, Kim JW, Ham DS, Park HS, Yang HK, et al. Preadipocyte factor 1 induces pancreatic ductal cell differentiation into insulin-producing cells. Sci Rep. (2016) 6:23960. doi: 10.1038/srep23960
121. Trivanovic D, Jaukovic A, Popovic B, Krstic J, Mojsilovic S, Okic-Djordjevic I, et al. Mesenchymal stem cells of different origin: comparative evaluation of proliferative capacity, telomere length and pluripotency marker expression. Life Sci. (2015) 141:61–73. doi: 10.1016/j.lfs.2015.09.019
122. Schiesser JV, Wells JM. Generation of β cells from human pluripotent stem cells: are we there yet? Ann NY Acad Sci. (2014) 1311:124–37. doi: 10.1111/nyas.12369
123. Wilding L, Gannon M. The role of pdx1 and HNF6 in proliferation and differentiation of endocrine precursors. Diabetes Metab Res Rev. (2004) 20:114–23. doi: 10.1002/dmrr.429
124. Churchill AJ, Gutierrez GD, Singer RA, Lorberbaum DS, Fischer KA, Sussel L. Genetic evidence that Nkx2.2 acts primarily downstream of Neurog3 in pancreatic endocrine lineage development. Elife. (2017) 6:e20010. doi: 10.7554/eLife.20010
125. Scheibner K, Bakhti M, Bastidas-Ponce A, Lickert H. Wnt signaling: implications in endoderm development and pancreas organogenesis. Curr Opin Cell Biol. (2019) 61:48–55. doi: 10.1016/j.ceb.2019.07.002
126. Prasadan K, Shiota C, Xiangwei X, Ricks D, Fusco J, Gittes G. A synopsis of factors regulating beta cell development and beta cell mass. Cell Mol Life Sci. (2016) 73:3623–37. doi: 10.1007/s00018-016-2231-0
127. Farina M, Alexander JF, Thekkedath U, Ferrari M, Grattoni A. Cell encapsulation: Overcoming barriers in cell transplantation in diabetes and beyond. Adv Drug Deliv Rev. (2018) 139:92–115. doi: 10.1016/j.addr.2018.04.018
128. Orive G, Santos E, Poncelet D, Hernandez RM, Pedraz JL, Wahlberg LU, et al. Cell encapsulation: technical and clinical advances. Trends Pharmacol Sci. (2015) 36:537–46. doi: 10.1016/j.tips.2015.05.003
129. Orive G, Hernandez RM, Rodriguez Gascon A, Calafiore R, Chang TM, de Vos P, et al. History, challenges and perspectives of cell microencapsulation. Trends Biotechnol. (2004) 22:87–92. doi: 10.1016/j.tibtech.2003.11.004
130. Bisceglie V. Uber die antineoplastische immunitat; heterologe Einpflnzung von Tumoren in Huhner-embryonen. Ztschr Krebsforsch. (1933) 40:122–40.
131. de Vos P, Hamel AF, Tatarkiewicz K. Considerations for successful transplantation of encapsulated pancreatic islets. Diabetologia. (2002) 45:159–73. doi: 10.1007/s00125-001-0729-x
132. Aebischer P, Schluep M, Deglon N, Joseph JM, Hirt L, Heyd B, et al. Intrathecal delivery of CNTF using encapsulated genetically modified xenogeneic cells in amyotrophic lateral sclerosis patients. Nat Med. (1996) 2:696–9. doi: 10.1038/nm0696-696
133. Tao W, Wen R, Goddard MB, Sherman SD, O'Rourke PJ, Stabila PF, et al. Encapsulated cell-based delivery of CNTF reduces photoreceptor degeneration in animal models of retinitis pigmentosa. Invest Ophthalmol Vis Sci. (2002) 43:3292–8.
134. Wong FSY, Tsang KK, Lo ACY. Delivery of therapeutics to posterior eye segment: cell-encapsulating systems. Neural Regener Res. (2017) 12:576–7. doi: 10.4103/1673-5374.205093
135. An D, Chiu A, Flanders JA, Song W, Shou D, Lu YC, et al. Designing a retrievable and scalable cell encapsulation device for potential treatment of type 1 diabetes. Proc Natl Acad Sci USA. (2018) 115:E263–72. doi: 10.1073/pnas.1708806115
136. Paredes Juárez GA, Spasojevic M, Faas MM, de Vos P. Immunological and technical considerations in application of alginate-based microencapsulation systems. Front Bioeng Biotechnol. (2014) 2:26. doi: 10.3389/fbioe.2014.00026
137. Gasperini L, Mano JF, Reis RL. Natural polymers for the microencapsulation of cells. J R Soc Interface. (2014) 11:20140817. doi: 10.1098/rsif.2014.0817
138. Sobol M, Bartkowiak A, de Haan B, de Vos P. Cytotoxicity study of novel water-soluble chitosan derivatives applied as membrane material of alginate microcapsules. J Biomed Mater Res Part A. (2012) 101A:1907–14. doi: 10.1002/jbm.a.34500
139. Löhr M, Hoffmeyer A, Kröger JC. Safety, feasibility and clinical benefit of localized chemotherapy using microencapsulated cells for inoperable pancreatic carcinoma in a phase I/II trial. Cancer Ther. (2003) 1:121–31.
140. Bhatia SR, Khattak SF, Roberts SC. Polyelectrolytes for cell encapsulation. Curr Opin Coll Interface Sci. (2005) 10:45–51. doi: 10.1016/j.cocis.2005.05.004
141. de Vos P, Lazarjani HA, Poncelet D, Faas MM. Polymers in cell encapsulation from an enveloped cell perspective. Adv Drug Del Rev. (2014) 67–68:15–34. doi: 10.1016/j.addr.2013.11.005
142. Strand BL, Coron AE, Skjak-Braek G. Current and future perspectives on alginate encapsulated pancreatic islet. Stem Cells Transl Med. (2017) 6:1053–8. doi: 10.1002/sctm.16-0116
143. Goh CH, Heng PWS, Chan LW. Alginates as a useful natural polymer for microencapsulation and therapeutic applications. Carbohydr Polym. (2012) 88:1–12. doi: 10.1016/j.carbpol.2011.11.012
144. Murua A, Portero A, Orive G, Hernández RM, de Castro M, Pedraz JL. Cell microencapsulation technology: towards clinical application. J Control Release. (2008) 132:76–83. doi: 10.1016/j.jconrel.2008.08.010
145. Morch YA, Donati I, Strand BL, Skjak-Braek G. Effect of Ca2+, Ba2+, and Sr2+ on alginate microbeads. Biomacromolecules. (2006) 7:1471–80. doi: 10.1021/bm060010d
146. Morch YA, Qi M, Gundersen PO, Formo K, Lacik I, Skjak-Braek G, et al. Binding and leakage of barium in alginate microbeads. J Biomed Mater Res A. (2012) 100:2939–47. doi: 10.1002/jbm.a.34237
147. Tam SK, Dusseault J, Bilodeau S, Langlois G, Halle JP, Yahia L. Factors influencing alginate gel biocompatibility. J Biomed Mater Res A. (2011) 98:40–52. doi: 10.1002/jbm.a.33047
148. Morch YA, Donati I, Strand BL, Skjak-Braek G. Molecular engineering as an approach to design new functional properties of alginate. Biomacromolecules. (2007) 8:2809–14. doi: 10.1021/bm700502b
149. Veiseh O, Doloff JC, Ma M, Vegas AJ, Tam HH, Bader AR, et al. Size- and shape-dependent foreign body immune response to materials implanted in rodents and non-human primates. Nature materials. (2015) 14:643–51. doi: 10.1038/nmat4290
150. Kulseng B, Thu B, Espevik T, Skjak-Braek G. Alginate polylysine microcapsules as immune barrier: permeability of cytokines and immunoglobulins over the capsule membrane. Cell Transplant. (1997) 6:387–94. doi: 10.1177/096368979700600405
151. Du J, Yarema KJ. Chapter 10: Cell microencapsulation for tissue engineering and regenerative medicine. In: Karp JM, Zhao W, editors. Micro- and Nanoengineering of the Cell Surface. Oxford: William Andrew Publishing (2014). p. 215–39. doi: 10.1016/B978-1-4557-3146-6.00010-6
152. Hunt NC, Grover LM. Cell encapsulation using biopolymer gels for regenerative medicine. Biotechnol Lett. (2010) 32:733–42. doi: 10.1007/s10529-010-0221-0
153. Pérez-Luna V, González-Reynoso O. Encapsulation of biological agents in hydrogels for therapeutic applications. Gels. (2018) 4:61. doi: 10.3390/gels4030061
154. Krishnan R, Alexander M, Robles L, Foster CE III, Lakey JR. Islet and stem cell encapsulation for clinical transplantation. Rev Diabet Stud. (2014) 11:84–101. doi: 10.1900/RDS.2014.11.84
155. Bhujbal SV, de Haan B, Niclou SP, de Vos P. A novel multilayer immunoisolating encapsulation system overcoming protrusion of cells. Sci Rep. (2014) 4:6856. doi: 10.1038/srep06856
156. Shi X, Wang Y, Ren L, Huang W, Wang DA. A protein/antibiotic releasing poly(lactic-co-glycolic acid)/lecithin scaffold for bone repair applications. Int J Pharm. (2009) 373:85–92. doi: 10.1016/j.ijpharm.2009.02.013
157. Kim SH, Kim JE, Kim SH, Jung Y. Substance P/dexamethasone-encapsulated PLGA scaffold fabricated using supercritical fluid process for calvarial bone regeneration. J Tissue Eng Regen Med. (2017) 11:3469–80. doi: 10.1002/term.2260
158. Saini H, Navaei A, Van Putten A, Nikkhah M. 3D cardiac microtissues encapsulated with the co-culture of cardiomyocytes and cardiac fibroblasts. Adv Healthcare Mater. (2015) 4:1961–71. doi: 10.1002/adhm.201500331
159. Castellano D, Blanes M, Marco B, Cerrada I, Ruiz-Sauri A, Pelacho B, et al. A comparison of electrospun polymers reveals poly(3-hydroxybutyrate) fiber as a superior scaffold for cardiac repair. Stem Cells Dev. (2014) 23:1479–90. doi: 10.1089/scd.2013.0578
160. Jitraruch S, Dhawan A, Hughes RD, Filippi C, Lehec SC, Glover L, et al. Cryopreservation of hepatocyte microbeads for clinical transplantation. Cell Transplant. (2017) 26:1341–54. doi: 10.1177/0963689717720050
161. Meier RP, Montanari E, Morel P, Pimenta J, Schuurman HJ, Wandrey C, et al. Microencapsulation of hepatocytes and mesenchymal stem cells for therapeutic applications. Methods Mol Biol. (2017) 1506:259–71. doi: 10.1007/978-1-4939-6506-9_18
162. Ham DS, Song MS, Park HS, Rhee M, Yang HK, Lee SH, et al. Successful xenotransplantation with re-aggregated and encapsulated neonatal pig liver cells for treatment of mice with acute liver failure. Xenotransplantation. (2015) 22:249–59. doi: 10.1111/xen.12177
163. Halberstadt C, Williams D, Gores P. Isolation of human cadaveric pancreatic islets for clinical transplantation. Methods Mol Biol. (2013) 1001:227–59. doi: 10.1007/978-1-62703-363-3_20
164. Saenz Del Burgo L, Ciriza J, Espona-Noguera A, Illa X, Cabruja E, Orive G, et al. 3D printed porous polyamide macrocapsule combined with alginate microcapsules for safer cell-based therapies. Sci Rep. (2018) 8:8512. doi: 10.1038/s41598-018-26869-5
165. Hwang PT, Shah DK, Garcia JA, Bae CY, Lim DJ, Huiszoon RC, et al. Progress and challenges of the bioartificial pancreas. Nano Converg. (2016) 3:28. doi: 10.1186/s40580-016-0088-4
166. de Vos P, Faas MM, Strand B, Calafiore R. Alginate-based microcapsules for immunoisolation of pancreatic islets. Biomaterials. (2006) 27:5603–17. doi: 10.1016/j.biomaterials.2006.07.010
167. O'Sullivan ES, Vegas A, Anderson DG, Weir GC. Islets transplanted in immunoisolation devices: a review of the progress and the challenges that remain. Endocr Rev. (2011) 32:827–44. doi: 10.1210/er.2010-0026
168. Ellis C, Ramzy A, Kieffer TJ. Regenerative medicine and cell-based approaches to restore pancreatic function. Nat Rev Gastroenterol Hepatol. (2017) 14:612–28. doi: 10.1038/nrgastro.2017.93
169. Dembczynski R, Jankowski T. Determination of pore diameter and molecular weight cut-off of hydrogel-membrane liquid-core capsules for immunoisolation. J Biomater Sci Polym Ed. (2001) 12:1051–8. doi: 10.1163/156856201753252552
170. Wang N, Adams G, Buttery L, Falcone FH, Stolnik S. Alginate encapsulation technology supports embryonic stem cells differentiation into insulin-producing cells. J Biotechnol. (2009) 144:304–12. doi: 10.1016/j.jbiotec.2009.08.008
171. Lim F, Sun AM. Microencapsulated islets as bioartificial endocrine pancreas. Science. (1980) 210:908–10. doi: 10.1126/science.6776628
172. De Vos P, De Haan BJ, Wolters GH, Strubbe JH, Van Schilfgaarde R. Improved biocompatibility but limited graft survival after purification of alginate for microencapsulation of pancreatic islets. Diabetologia. (1997) 40:262–70. doi: 10.1007/s001250050673
173. Vegas AJ, Veiseh O, Gurtler M, Millman JR, Pagliuca FW, Bader AR, et al. Long-term glycemic control using polymer-encapsulated human stem cell-derived beta cells in immune-competent mice. Nat Med. (2016) 22:306–11. doi: 10.1038/nm.4030
174. Ramezanzadeh Andevari R, Hashemi-Najafabadi S, Bagheri F. Immunoisolation of stem cells by simultaneous encapsulation and PEGylation. Progr Biomater. (2018) 7:55–60. doi: 10.1007/s40204-018-0084-3
175. Swioklo S, Ding P, Pacek AW, Connon CJ. Process parameters for the high-scale production of alginate-encapsulated stem cells for storage and distribution throughout the cell therapy supply chain. Process Biochem. (2017) 59:289–96. doi: 10.1016/j.procbio.2016.06.005
176. Chan HF, Zhang Y, Ho YP, Chiu YL, Jung Y, Leong KW. Rapid formation of multicellular spheroids in double-emulsion droplets with controllable microenvironment. Sci Rep. (2013) 3:3462. doi: 10.1038/srep03462
177. Sabek OM, Farina M, Fraga DW, Afshar S, Ballerini A, Filgueira CS, et al. Three-dimensional printed polymeric system to encapsulate human mesenchymal stem cells differentiated into islet-like insulin-producing aggregates for diabetes treatment. J Tissue Eng. (2016) 7:2041731416638198. doi: 10.1177/2041731416638198
178. Tam SK, Bilodeau S, Dusseault J, Langlois G, Hallé JP, Yahia LH. Biocompatibility and physicochemical characteristics of alginate–polycation microcapsules. Acta Biomater. (2011) 7:1683–92. doi: 10.1016/j.actbio.2010.12.006
Keywords: diabetes mellitus, veterinary stem cell-based therapy, regenerative medicine, encapsulation, tissue engineering
Citation: Kuncorojakti S, Srisuwatanasagul S, Kradangnga K and Sawangmake C (2020) Insulin-Producing Cell Transplantation Platform for Veterinary Practice. Front. Vet. Sci. 7:4. doi: 10.3389/fvets.2020.00004
Received: 10 October 2019; Accepted: 06 January 2020;
Published: 12 February 2020.
Edited by:
Fausto Cremonesi, University of Milan, ItalyReviewed by:
Jeongik Lee, Konkuk University, South KoreaDavid John Argyle, University of Edinburgh, United Kingdom
Copyright © 2020 Kuncorojakti, Srisuwatanasagul, Kradangnga and Sawangmake. This is an open-access article distributed under the terms of the Creative Commons Attribution License (CC BY). The use, distribution or reproduction in other forums is permitted, provided the original author(s) and the copyright owner(s) are credited and that the original publication in this journal is cited, in accordance with accepted academic practice. No use, distribution or reproduction is permitted which does not comply with these terms.
*Correspondence: Chenphop Sawangmake, chenphop.s@chula.ac.th; chenphop@gmail.com