- 1Animal Bioscience and Biotechnology Laboratory, United States Department of Agriculture, Beltsville Agricultural Research Center, Agricultural Research Service, Beltsville, MD, United States
- 2Arm & Hammer Animal and Food Production, Waukesha, WI, United States
- 3Department of Pediatrics, University of Maryland School of Medicine, Baltimore, MD, United States
Direct-fed microbials (DFMs) are dietary supplements containing live microorganisms which confer a performance and health benefit to the host, but the mechanisms are unclear. Here, a metabolomics approach was used to identify changes in intestinal metabolite levels in chickens fed an unsupplemented diet or a diet supplemented with B. subtilis strain 1781 or strain 747. Body weight gains of chickens fed the B. subtilis-supplemented diets were increased up to 5.6% in the B. subtilis 1781 group and 7.6% in the B. subtilis 747 group compared with chickens fed the unsupplemented diet. Compared with unsupplemented controls, the levels of 83 metabolites were altered (p < 0.05) (25 increased, 58 decreased) in chickens given the B. subtilis 1781-supplemented diet, while 50 were altered (p < 0.05) (12 increased, 38 decreased) with the B. subtilis 747-supplemented diet. Twenty-two metabolites were altered (p < 0.05) (18 increased, 4 decreased) in the B. subtilis 1781 vs. B. subtilis 747 groups. A random forest analysis of the B. subtilis 1781 vs. control groups gave a predictive accuracy of 87.5%, while that of the B. subtilis 747 vs. control groups was 62.5%. A random forest analysis of the B. subtilis 1781 vs. B. subtilis 747 groups gave a predictive accuracy of 75.0%. Changes in the levels of these intestinal biochemicals provided a distinctive biochemical signature unique to each B. subtilis-supplemented group, and were characterized by alterations in the levels of dipeptides (alanylleucine, glutaminylleucine, phenylalanylalanine, valylglutamine), nucleosides (N1-methyladenosine, N6-methyladenosine, guanine, 2-deoxyguanosine), fatty acids (sebacate, valerylglycine, linoleoylcholine), and carbohydrates (fructose). These results provide the foundation for future studies to identify biochemicals that might be used to improve poultry growth performance in the absence of antibiotic growth promoters.
Introduction
Microbial resistance to antibiotics is a serious and growing public health problem worldwide with multiple causes (1, 2). One likely contributory factor is the widespread use of antibiotics as growth promoters in commercial livestock and poultry production. Dietary antibiotic growth promoters (AGPs) have been used in the food animal industry for more than 60 years to increase feed efficiency and improve growth performance (3, 4). Increasing evidence, however, suggests that AGP use in food animal production leads to the development of antibiotic resistance among the endogenous gut commensal microbiota with the potential for transfer to the human population (5–9). Consequently, there is an unmet need to elucidate the molecular and cellular interactions between the intestinal microbiota and host that might be modulated by other means to promote food animal growth in the absence of AGPs. Among the substances that have been tested as alternatives to AGPs are probiotics and direct-fed microbials (DFMs) (10–12).
DFMs are viable, naturally occurring microbial cultures, including bacteria belonging to the genera Lactobacillus, Enterococcus, Streptococcus, Bifidobacterium, and Propionibacterium, which when fed to the host animal, generate a beneficial health response through their ability to modulate the diversity and composition of the gut microbiota. Various species of Bacillus also have been tested as DFMs on the basis of their ability to inhibit pathogens by producing antimicrobials (13–15). Bacillus has the added advantage as a DFM of producing spores that are resistant to low pH, bile salts, and other harsh conditions of the gastric environment (16–19). In poultry, multiple strains of B. subtilis have been used as DFMs to promote growth, immunity, and overall gut health (11, 12). In humans, both observational studies and randomized controlled trials have documented the health-promoting effects of probiotic Bacillus spp., particularly when used to treat intestinal disorders (20).
Our prior report demonstrated that dietary supplementation of healthy broiler chickens with B. subtilis strain 1781 as a DFM increased body weight gains, compared with chickens fed an unsupplemented diet (21). Further, following infection with Eimeria maxima, oral supplementation of chickens with B. subtilis reduced the clinical signs of experimental avian coccidiosis, compared with unsupplemented and infected controls (22). Independently, we also reported that the AGPs, virginiamycin and bacitracin methylene disalicylate, altered the chicken intestinal metabolome and speculated that their growth enhancing effects were due to the selection of gut microbial species capable of producing metabolites that promoted more efficient energy utilization (23). Therefore, the current study was undertaken to characterize the metabolic alterations in the chicken gut following dietary supplementation with B. subtilis DFMs with the goal of identifying potential chemical compounds that might be directly used to improve poultry growth performance without the use of AGPs.
Methods
Animals
Eighty-four, day-old, male Ross 708 broiler chickens (Longenecker's Hatchery, Elizabethtown, PA) were randomly housed in starter brooder cages (Petersime, Zulte, Belgium) and provided with starter feed.
Experimental Design, Growth Performance, and Intestinal Metabolomics Analysis
At 14 days of age, chickens were allocated to 1 of 3 dietary treatments in a randomized complete block design. The dietary treatments included a basal diet (Table 1), or a basal diet supplemented with 1.5 × 105 CFU/g feed of B. subtilis 1781 or B. subtilis 747 (Arm & Hammer Co., Inc., Waukesha, WI). The dose of B. subtilis was chosen based on the previous study of Lee et al. (24). Each treatment group contained 4 cages with 7 chickens/cage. Cage dimensions were 0.65 × 0.75 m for a total of 0.4875 m2, resulting in 14.4 chickens/m2. All chickens were housed in the same room and provided ad-libitum access to water and feed throughout the study. Feed additions were weighed and recorded daily, and feeders were shaken once per day. The chickens and feed were weighed at 14 and 21 days of age for computation of growth performance. Dead chickens were removed and weighed to calculate mortality and adjust growth performance data. At 21 days of age, 8 chickens/group were euthanized by cervical dislocation and the intestinal ileum harvested. Intestinal ileal contents were collected aseptically by gently finger-stripping the ileal segment, immediately placed on dry ice, and stored at −80°C. Global metabolomic profiling of the intestinal contents was performed by mass spectrometry (MS) (Metabolon, Durham, NC) as described (23). Raw data was extracted and processed using the DiscoveryHD4 global metabolomics platform. Compounds were identified by comparison to library entries of purified standards or recurrent unknown entities based on retention index, accurate mass match to the library ±10 ppm, and MS/MS forward and reverse scores between experimental data and authentic standards. MS/MS scores were based on comparison of the ions present in the experimental spectrum to the ions present in the library spectrum.
Statistical Analysis
Each cage was considered the experimental unit. The type of experimental diet was considered the treatment factor, and each cage was considered as a blocking factor. Data were analyzed using a mixed model methodology (PROC MIXED, SAS Institute, Cary NC). For growth performance, mean ± SEM values were calculated for initial body weight (IBW) at 14 days, final body weight (FBW) at 21 days, body weight gain (BWG) between 14 and 21 days, feed intake (FI) between 14 and 21 days, and feed efficiency (FE = BWG/FI) between 14 and 21 days. Differences between means were compared using the 2-tailed Student's t-test with p ≤ 0.05 considered significantly different. All data analysis were conducted with the same way of Gadde et al. (23). Briefly, ANOVA was used to identify the biochemical changes among the 3 dietary groups following median scaling, log transformation, and imputation of missing values. Array Studio software (OmicSoft, Cary, NC) was used for Standard statistical analyses of log-transformed data. For analyses that were not standard in Array Studio, the programs R (R Foundation for Statistical Computing, Vienna, Austria) or JMP (SAS Institute) were used. Changes in biochemical levels with p ≤ 0.05 were considered statistically significant. To measure biochemical importance, Random Forest Analysis (RFA) was performed by computing the Mean Decrease Accuracy (MDA).
Results
B. subtilis DFMs Increase Chicken Growth Performance
Chickens were fed from 14 to 21 days post-hatch with a basal diet, or a basal diet supplemented with 1.5 × 105 colony forming units (CFU)/g feed of B. subtilis strain 1781 or B. subtilis strain 787. Body weights at 14 and 21 days of age, as well as feed intake and feed efficiency between 14 and 21 days, were identical between the 3 groups (Table 2). However, body weight gains between 14 and 21 days were greater for chickens fed with either of the Bacillus-supplemented diets, compared with unsupplemented controls.
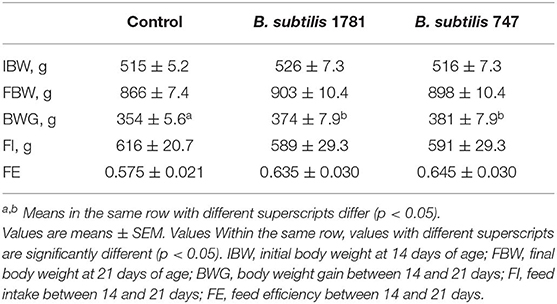
Table 2. Growth performance of chickens fed an unsupplemented control diet or a diet containing B. subtilis strain 1781 or B. subtilis strain 747.
B. subtilis DFMs Alter Global Intestinal Metabolite Levels
A total of 674 biochemicals were identified in the intestinal contents of chickens fed an unsupplemented, control diet, or a diet supplemented with B. subtilis 1781 or B. subtilis 747. In the B. subtilis 1781 vs. control groups, the levels of 209 metabolites were increased and 461 were decreased. Of these, 25 of the increased and 58 of the decreased compounds were statistically significant (p < 0.05) In the B. subtilis 747 vs. control groups, 265 metabolites were increased and 402 were decreased. Of these, 12 of the increased and 38 of the decreased compounds were statistically significant (p < 0.05). In the B. subtilis 747 vs. B. subtilis 747 groups, 383 metabolites were increased and 279 were decreased. Of these, 18 of the increased and 4 of the decreased compounds were statistically significant (p < 0.05).
Intestinal Metabolite Signatures and Biochemical Importance Analyses
A random forest analysis (RFA) was performed to identify metabolite signatures and the biochemical importance of the 30 most significantly altered metabolites for distinguishing the B. subtilis 1781 vs. control, B. subtilis 747 vs. control, and B. subtilis 1781 vs. B. subtilis 747 groups (Table 3). RFA of the B. subtilis 1781 vs. control groups gave a predictive accuracy of 87.5%, while that of the B. subtilis 747 vs. control groups was 62.5%, suggesting that these metabolites are candidate biomarkers for distinguishing between each of the 2 treatment groups and the control group. RFA of the B. subtilis 1781 vs. B. subtilis 747 groups gave a predictive accuracy of 75.0%. Compared with the control group, all 8 intestinal samples analyzed from the B. subtilis 1781 group, and 5 samples from the B. subtilis 747 group, were predicted to belong to their respective group. Three samples from B. subtilis 747 group were predicted to belong to the control group. Of 8 control group samples, 2 were predicted to belong to the B. subtilis 1781 group and 3 were predicted to belong to the B. subtilis 747 group. When compared between the 2 B. subtilis DFM groups, 8 of the B. subtilis 1781 samples, and 4 of the B. subtilis 747 samples, were predicted to belong to their respective group.
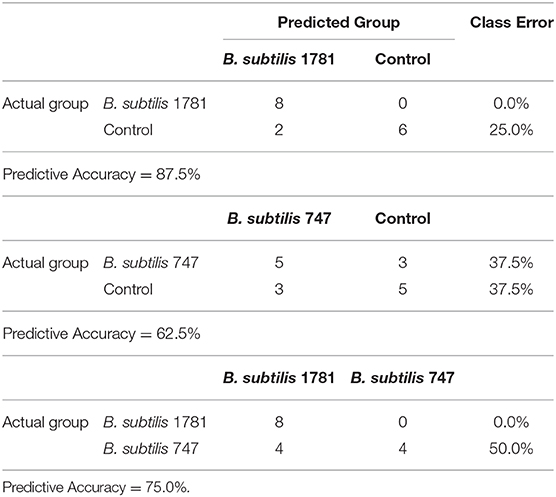
Table 3. Random forest analysis of the altered biochemicals distinguishing between the B. subtilis 1781 vs. control, B. subtilis 747 vs. control, and B. subtilis 1781 vs. B. subtilis 747 groups based on 8 independent samples.
Specific Intestinal Metabolites Altered Following Dietary B. subtilis Supplementation
Metabolites of amino acids (26.7%), lipids (26.7%), vitamins and cofactors (16.7%), and nucleosides (10.3%) accounted for the majority of biochemicals classified as the most important for distinguishing between the B. subtilis 1781 vs. control groups (Figure 1A). Metabolites of lipids (33.0%), amino acids (20.0%), and peptides (20.0%) accounted for the majority of biochemicals for distinguishing between the B. subtilis 747 vs. control groups (Figure 1B). Metabolites of carbohydrates (30.0%), amino acids (20.0%), and lipid (20.0%) accounted for the majority of biochemicals for distinguishing between the B. subtilis 1781 vs. B. subtilis 747 groups (Figure 1C). Among the amino acid metabolites most highly elevated in the B. subtilis 1781 vs. control and B. subtilis 747 vs. control groups were leucine-containing dipeptides. The levels of alanylleucine (Ala-Leu), glutaminylleucine (Gln-Leu), valylleucine (Val-Leu), and glycylisoleucine (Gly-Ile) were increased 3.28-, 3.01-, 3.98-, and 1.98-fold, respectively, in the intestinal contents of chicken fed the B. subtilis 1781 diet, compared with unsupplemented controls (Figure 2A). These same dipeptides were increased 2.49-, 3.34-, 1.53- and 2.82-fold in B. subtilis 747-treated chickens, compared with controls. The alanine-associated dipeptide phenylalanylalanine (Phe-Ala), and the glutamine-associated dipeptide valylglutamine (Val-Gln) were increased 2.12- and 2.31-fold in the B. subtilis 1781 vs. control groups, and 1.87- and 2.14-fold in the B. subtilis 747 vs. control groups. Biochemicals associated with purine metabolism that were increased in the B. subtilis 1781- or B. subtilis 747-supplemented diets vs. controls included N1-methyladenosine (3.45-, and 1.89-fold, respectively), N6-methyladenosine (4.43-, 2.51-fold), guanine (1.10-, 4.12-fold), and 2-deoxyguanosine (2.00-, 4.42-fold) (Figure 2B). Biochemicals associated with pyrimidine metabolism that were decreased in the B. subtilis 1781 or B. subtilis 747-supplemented diets vs. controls included uridine-5′-monophosphate (0.26-, and 0.17-fold) and cytidine (0.37-, and 0.52-fold) (Figure 2B). Fatty acids and their metabolites also contributed to the biochemical signatures that distinguished chickens given the B. subtilis-supplemented diets from unsupplemented controls. For example, sebacate (C10-DC), valerylglycine, and linoleoylcholine were increased 1.34-, 1.74, and 1.62-fold in the B. subtilis 747 diet vs. controls (Figure 2C). By contrast, sterols and bile acids were decreased in both Bacillus subtilis vs. control groups. Cholesterol, chenodeoxycholate, and 3-dehydrodeoxycholate were decreased 0.54-, 0.50-, and 0.38-fold in the B. subtilis 1781-supplemented diet vs. controls (Figure 2C). Related to benzoate metabolism, the levels of 2-(4-hydroxyphenyl)propionate were decreased in both B. subtilis groups vs. control group (~0.53-fold), whereas the levels of salicylate-glucoside were increased in the B. subtilis 747 vs. control groups (1.92-fold) (Figure 2D). Related to nicotinamide metabolism, the levels of nicotinamide ribonucleotide (NMN) and nicotinamide adenine dinucleotide (NAD+) were reduced <80% in both B. subtilis-supplemented chickens, compared with controls. Finally, related to carbohydrate metabolism, fructose levels were elevated in the B. subtilis 1781 vs. control (2.01-fold) and B. subtilis 747 vs. control (2.64-fold) groups, while lactate levels were decreased in both B. subtilis groups vs. control group (0.27-fold) (Figure 2D).
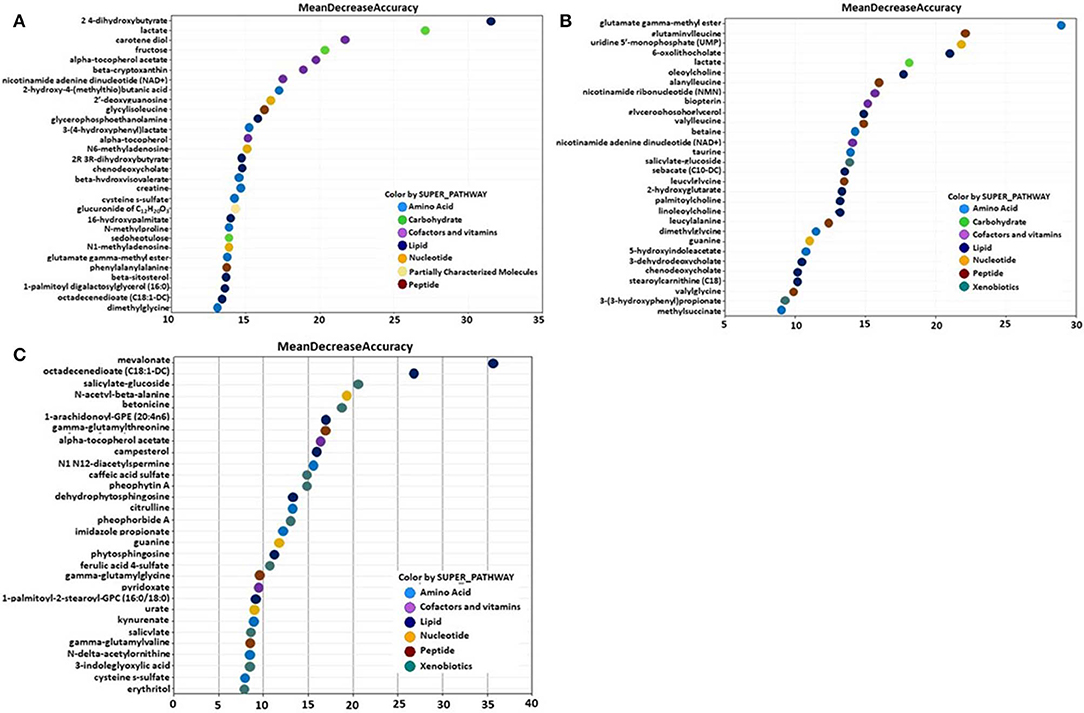
Figure 1. Random forest plots of the top 30 biochemicals whose levels were altered in the (A) B. subtilis 1781 vs. control, (B) B. subtilis 747 vs. control, and (C) B. subtilis 1781 vs. B. subtilis 747 groups. Biochemicals are listed from bottom to top in increasing order of importance for contributing to the biochemical signatures separating the respective treatment groups, and are plotted in color-coded symbols according to chemical classification.
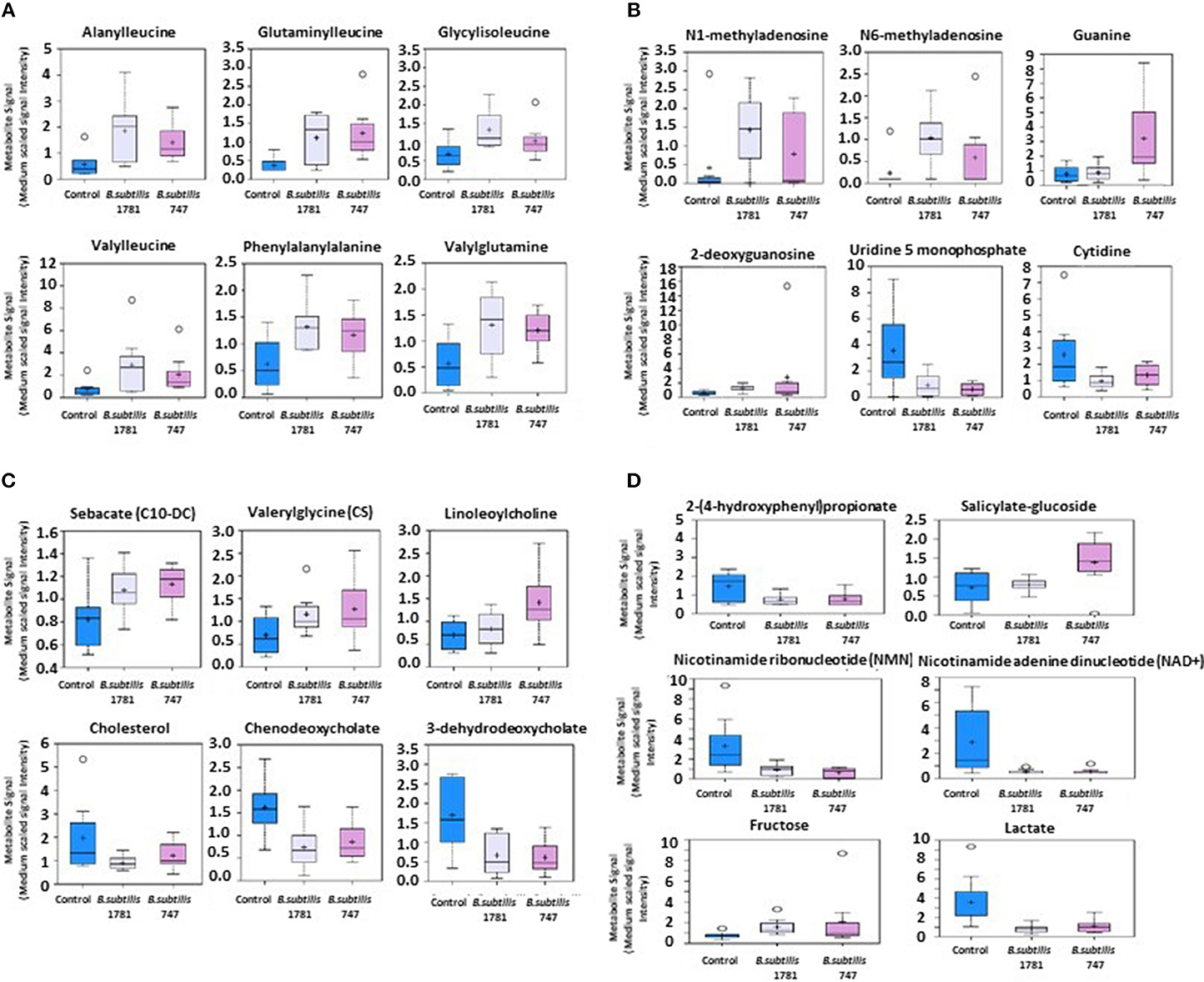
Figure 2. Box-and-whisker plots of the levels of (A) amino acids, (B) nucleotides, (C) fatty acids, and (D) others, including xenobiotics, vitamins, cofactors, and carbohydrates in the intestine of chickens fed an unsupplemented control diet (blue), or a diet supplemented with B. subtilis 1781 (gray) or B. subtilis 747 (pink). The boxes represent the interquartile range (IQR) defined by the 25th and 75th percentiles. The horizontal line represents the median value. The cross represents the mean value. The upper whisker represents Q3 + (1.5 × IQR), while the lower whisker represents Q1 – (1.5 × IQR). Circles represent outliers.
Discussion
We previously reported that dietary supplementation of broiler chickens with B. subtilis-based DFMs increased body weight gains, compared with unsupplemented controls (21). Further, chickens fed a B. subtilis-containing diet had increased intestinal villus height and crypt depth, augmented mitogen- and antigen-induced spleen cell proliferation, greater macrophage phagocytosis of Salmonella bacteria, decreased serum levels of α-1-acid glycoprotein and nitric oxide, altered expression of cytokine genes (interferon-γ, interleukin-1β, CXCL2) in intestinal lymphocytes, and a modified CD4+/CD8+ ratio of peripheral blood lymphocytes (24–28). The current study extends these prior results to now demonstrate that dietary supplementation with B. subtilis 1781 or B. subtilis 787 alters the chicken intestinal metabolome.
Supplementing these studies, other investigators have documented the growth promoting effects of Bacillus-based DFMs in poultry. Opalinski et al. (29) reported reduced feed conversion ratio (FCR), a measure of feed input divided by body weight gain, in chickens fed a diet supplemented with B. subtilis, compared with unsupplemented controls, although final weight gains were unaffected. Aliakbarpour et al. (30) confirmed that dietary B. subtilis decreased FCR, and increased weight gains and MUC2 mucin gene expression in intestinal goblet cells, compared with controls. Jeong et al. (31) replicated the effects of B. subtilis DFMs on chicken FCR and weight gains, and also demonstrated increased Lactobacillus spp. and reduced Escherichia coli levels in the intestinal contents of DFM-supplemented birds, compared with controls. Increased growth performance, nutrient utilization, and intestinal villus/crypt morphometry, as well as altered composition of gut microbiota, also were observed in chickens orally administered with Bacillus DFMs, compared with controls (32–35).
In addition to their effects on healthy animals, Bacillus DFMs also have been shown to ameliorate the deleterious outcomes associated with enteric bacterial infections of poultry. B. subtilis dietary supplementation reversed the effects of Clostridium perfringens infection on decreased growth performance and alterations in the intestinal microbiota, compared with unsupplemented controls (24). In a C. perfringens/Eimeria maxima coinfection model of avian necrotic enteritis, feeding of B. subtilis reduced gut pathology and animal mortality, and restored alterations in the intestinal microbiome, compared with controls (22). Other studies have confirmed the ability of Bacillus DFMs to reduce C. perfringens colonization of the intestinal mucosa and improve animal growth performance in avian necrotic enteritis (36–41). In general, while the beneficial effect of Bacillus DFMs on body weight gains in healthy, uninfected chickens is generally moderate (<10%), a more pronounced outcome of the dietary supplements has been observed when weight loss is aggravated as a consequence of pathogenic infection (42).
Given the reported effects of DFMs on modulating the chicken gut microbiome (10–12), and the ability of intestinal bacteria to synthesize vitamins and nutrients beneficial to the host (43), it is somewhat surprising that few reports have examined the ability of DFMs to alter the avian metabolome (35, 44). Cao et al. (35) reported that dietary supplementation of chickens with B. amyloliquefaciens altered the levels of gut metabolites related to amino acid and glyceride metabolism. More specifically, increased levels of 4-aminobutyric acid, gentiobiose, quinic acid, 3,7,12-trihydroxycoprostane, N-ethylglycine, glycine, N-acetyl-D-galactosamine, and 5-hydroxyindole-3-acetic acid, and reduced the levels of diglycerol and N-acetyl-β-D-mannosamine, were seen in the DFM-supplemented chickens, compared with unsupplemented controls. In another study, Wang et al. (44) observed that chickens fed a diet containing the yeast Kluyveromyces marxianus had altered serum levels of 60 biochemical metabolites (39 increased, 21 decreased), compared with controls. Biochemicals involved in carbohydrate and amino acid, primarily glutamate and glutamine, metabolism were identified as the most relevant.
In humans, probiotics have been used to treat a variety of diseases and disorders, and in some cases, metabolic profiling has begun to unravel their mechanisms of action. For example, in a double-blind, randomized, placebo-controlled clinical trial of a fermented milk probiotic provided to patients with irritable bowel syndrome, decreased selected clinical symptoms was correlated with increased serum glucose and tyrosine levels (45). In women with mastitis, consumption of a Lactobacillus probiotic decreased staphylococcal/streptococcal bacterial load in breast milk and breast pain, while increasing urinary levels of creatinine, hippuric acid (a glycine conjugate of benzoic acid), and trimethylamine-N-oxide (a choline metabolite) (46). Oral administration of a Bifidobacterium animalis probiotic to patients with atopic dermatitis improved dermatology-specific quality-of-life scores, while increasing fecal kynurenic acid and decreasing 3-hydroxyproprionic acid levels, compared with placebo controls (47). In a study of chronic kidney disease patients orally administered with a probiotic containing Streptococcus thermophilus, Lactobacillus acidophilus, and Bifidobacterium longum, serum levels of metabolites related to carbohydrate, choline, and energy metabolism differentiated individuals with increased vs. decreased blood urea nitrogen levels (48). Per os administration of a probiotic mixture containing 4 strains of lactobacilli, 3 strains of bifidobacteria, and 1 strain of Streptococcus thermophilus to infants with colic reduced clinical symptoms, while increasing fecal levels of alanine, leucine, isoleucine, acetate, and pyruvate and decreasing the levels of uracil and propylene glycol, compared with placebo controls (49). Finally, a similar relationship between reduction of disease scores and alterations in metabolic profiles induced by administration of bacterial probiotics has been reported in animal models of human diseases, including fecal metabolites in mice with chemical-induced colits (50) and serum metabolites in rats with depression-related behavioral changes (51).
The alteration of metabolites observed in the current study suggest that these small molecules (gut metabolites) might play a role in the recognition of pathogen-associated molecular patterns (PAMPs) through influencing the host immune response to maintain homeostasis against intestinal diseases and inflammation. In this way, these responses may ultimately lead to improved growth performance (52–55). Short-chain fatty acids (SCFAs), such as acetate, n-propionate, and n-butyrate, are known to play a role in the recognition of PAMPs (52), however, alternations in SCFA levels in the gut were not found in the current study. SCFAs can be sensed by G-protein coupled receptors (GPRs) which are expressed on many cells, such as epithelial cells, neutrophils, and macrophages (52). GPR pathways are associated with cytokines and tight junction protein expression (56, 57). Lipid metabolites in the current study may also be sensed by GPRs, which implies that lipid metabolites can affect the regulation of immunity or inflammation in the gut.
In summary, to the best of our knowledge, this is the first report to demonstrate that dietary supplementation with B. subtilis has profound effects on the levels of a wide variety of chemical metabolites in the chicken gut, particularly those related to amino acids, nucleosides, fatty acids, and carbohydrates. Compared with unsupplemented controls, these altered metabolite levels provide a biochemical signature unique to each B. subtilis supplementation group. Our result suggest that altered metabolites can be used to maintain gut homeostasis within epithelial or immune cells, which might account for their affect on overall gut health as well as chicken growth. Through future in vitro and/or in vivo studies, identification of the altered metabolites that confer properties of AGPs would suggest their potential use as antibiotic alternatives.
Data Availability Statement
All datasets generated for this study are included in the article/supplementary material.
Ethics Statement
The animal study was reviewed and approved by the Beltsville Agricultural Research Center Institutional Animal Care and Use Committee and performed in accordance with the guidelines and regulations.
Author Contributions
IP and HL designed the research and conducted research. IP, EL, and HL analyzed data. IP, NZ, AS, TR, EL, and HL had responsibility for content. All authors read and approved the final manuscript.
Funding
This work was supported by ARS CRIS Project 8042-32000-107-00D.
Conflict of Interest
NZ, AS, and TR were employed by the Arm & Hammer Animal and Food Production.
The remaining authors declare that the research was conducted in the absence of any commercial or financial relationships that could be construed as a potential conflict of interest.
References
1. Ferri M, Ranucci E, Romagnoli P, Giaccone V. Antimicrobial resistance: a global emerging threat to public health systems. Crit Rev Food Sci Nutr. (2017) 57:2857–76. doi: 10.1080/10408398.2015.1077192
2. Aslam B, Wang W, Arshad MI, Khurshid M, Muzammil S, Rasool MH, et al. Antibiotic resistance: a rundown of a global crisis. Infect Drug Resist. (2018) 11:1645–58. doi: 10.2147/IDR.S173867
3. Lillehoj H, Liu Y, Calsamiglia S, Fernandez-Miyakawa ME, Chi F, Cravens RL, et al. Phytochemicals as antibiotic alternatives to promote growth and enhance host health. Vet Res. (2018) 49:76. doi: 10.1186/s13567-018-0562-6
4. Oh S, Lillehoj HS, Lee Y, Bravo D, Lillehoj EP. Dietary antibiotic growth promoters down-regulate intestinal inflammatory cytokine expression in chickens challenged with LPS or co-infected with Eimeria maxima and Clostridium perfringens. Front Vet Sci. (2019) 6:420. doi: 10.3389/fvets.2019.00420
5. Ahmed MO, Baptiste KE. Vancomycin-resistant enterococci: a review of antimicrobial resistance mechanisms and perspectives of human and animal health. Microb Drug Resist. (2018) 24:5. doi: 10.1089/mdr.2017.0147
6. van den Bogaard AE, Stobberingh EE. Epidemiology of resistance to antibiotics: links between animals and humans. Int J Antimicrob Agents. (2000) 14:327–35. doi: 10.1016/S0924-8579(00)00145-X
7. Bacanli M, Başaran N. Importance of antibiotic residues in animal food. Food Chem Toxicol. (2019) 125:462–6. doi: 10.1016/j.fct.2019.01.033
8. Lekshmi M, Ammini P, Kumar S, Varela MF. The food production environment and the development of antimicrobial resistance in human pathogens of animal origin. Microorganisms. (2017) 5:11. doi: 10.3390/microorganisms5010011
9. Nhung NT, Chansiripornchai N, Carrique-Mas JJ. Antimicrobial resistance in bacterial poultry pathogens: a review. Front Vet Sci. (2017) 4:126. doi: 10.3389/fvets.2017.00126
10. Zoumpopoulou G, Tzouvanou A, Mavrogonatou E, Alexandraki V, Georgalaki M, Anastasiou R, et al. Probiotic features of lactic acid bacteria isolated from a diverse pool of traditional greek dairy products regarding specific strain-host interactions. Probiotics Antimicrob Proteins. (2018) 10:313–22. doi: 10.1007/s12602-017-9311-9
11. Gadde U, Kim WH, Oh ST, Lillehoj HS. Alternatives to antibiotics for maximizing growth performance and feed efficiency in poultry: a review. Anim Health Res Rev. (2017) 18:26–45. doi: 10.1017/S1466252316000207
12. Grant A, Gay CG, Lillehoj HS. Bacillus spp. as direct-fed microbial antibiotic alternatives to enhance growth, immunity, and gut health in poultry. Avian Pathol. (2018) 47:339–51. doi: 10.1080/03079457.2018.1464117
13. Ramlucken U, Ramchuran SO, Moonsamy G, Lalloo R, Thantsha MS, van Rensburg CJ. A novel Bacillus based multi-strain probiotic improves growth performance and intestinal properties of Clostridium perfringens challenged broilers. Poult Sci. (2020) 1:331–42. doi: 10.3382/ps/pez496
14. Adhikari B, Hernandez-Patlan D, Solis-Cruz B, Kwon YM, Arreguin MA, Latorre JD, et al. Evaluation of the antimicrobial and anti-inflammatory properties of Bacillus-DFM (NorumTM) in broiler chickens infected with Salmonella Enteritidis. Front Vet Sci. (2019) 27:282. doi: 10.3389/fvets.2019.00282
15. Sumi C, Yang B, Yeo IC, Hahm Y. Antimicrobial peptides of the genus Bacillus: a new era for antibiotics. Can J Microbiol. (2015) 61: 93–103. doi: 10.1139/cjm-2014-0613
16. Lee S, Lee J, Jin Y, Jeong J, Chang YH, Lee Y, et al. Probiotic characteristics of Bacillus strains isolated from Korean traditional soy sauce. LWT Food Sci Technol. (2017) 79:518–24. doi: 10.1016/j.lwt.2016.08.040
17. Penaloza-Vazquez A, Ma LM, Rayas-Duarte P. Isolation and characterization of Bacillus spp. strains as potential probiotics for poultry. Can J Microbiol. (2017) 65:762–74. doi: 10.1139/cjm-2019-0019
18. Shivaramaiah S, Pumford NR, Morgan MJ, Wolfenden RE, Wolfenden AD, Torres-Rodríguez A, et al. Evaluation of Bacillus species as potential candidates for direct-fed microbials in commercial poultry. Poult Sci. (2011) 90:1574–80. doi: 10.3382/ps.2010-00745
19. Gupta TB, Brightwell G. Farm level survey of spore-forming bacteria on four dairy farms in the Waikato region of New Zealand. Microbiologyopen. (2017) 6:e00457. doi: 10.1002/mbo3.457
20. Elshaghabee FMF, Rokana N, Gulhane RD, Sharma C, Panwar H. Bacillus as potential probiotics: status, concerns, and future perspectives. Front Microbiol. (2017) 8:1490. doi: 10.3389/fmicb.2017.01490
21. Gadde U, Oh ST, Lee YS, Davis E, Zimmerman N, Rehberger T, et al. The effects of direct-fed microbial supplementation, as an alternative to antibiotics, on growth performance, intestinal immune status, and epithelial barrier gene expression in broiler chickens. Probiotics Antimicrob Proteins. (2017) 9:1–9. doi: 10.1007/s12602-017-9275-9
22. Lee KW, Lillehoj HS, Jang SI, Li G, Lee SH, Lillehoj EP, et al. Effect of Bacillus-based direct-fed microbials on Eimeria maxima infection in broiler chickens. Comp Immunol Microbiol Infect Dis. (2010) 33:e105–10. doi: 10.1016/j.cimid.2010.06.001
23. Gadde UD, Oh S, Lillehoj HS, Lillehoj EP. Antibiotic growth promoters virginiamycin and bacitracin methylene disalicylate alter the chicken intestinal metabolome. Sci Rep. (2018) 8:3592. doi: 10.1038/s41598-018-22004-6
24. Lee KW, Lillehoj HS, Jang SI, Lee SH, Bautista DA, Siragusa GR. Effect of Bacillus subtilis-based direct-fed microbials on immune status in broiler chickens raised on fresh or used litter. Asian Austral J Anim Sci. (2013) 26:1592–7. doi: 10.5713/ajas.2013.13178
25. Lee KW, Lee SH, Lillehoj HS, Li GX, Jang SI, Babu US, et al. Effects of direct-fed microbials on growth performance, gut morphometry, and immune characteristics in broiler chickens. Poult Sci. (2010) 89:203–16. doi: 10.3382/ps.2009-00418
26. Lee KW, Li G, Lillehoj HS, Lee SH, Jang SI, Babu US, et al. Bacillus subtilis-based direct-fed microbials augment macrophage function in broiler chickens. Res Vet Sci. (2011) 91:e87–91. doi: 10.1016/j.rvsc.2011.01.018
27. Lee KW, Lillehoj HS, Jang SI, Lee SH. Effects of salinomycin and Bacillus subtilis on growth performance and immune responses in broiler chickens. Res Vet Sci. (2014) 97:304–8. doi: 10.1016/j.rvsc.2014.07.021
28. Gadde UD, Oh S, Lee Y, Davis E, Zimmerman N, Rehberger T, et al. Dietary Bacillus subtilis-based direct-fed microbials alleviate LPS-induced intestinal immunological stress and improve intestinal barrier gene expression in commercial broiler chickens. Res Vet Sci. (2017) 114:236–43. doi: 10.1016/j.rvsc.2017.05.004
29. Opalinski M, Maiorka A, Dahlke F, Cunha F, Vargas FSC, Cardozo E. On the use of a probiotic (B. subtilis-strain DSM 17299) as growth promoter in broiler diets. Braz J Poultry Sci. (2007) 9:99–103. doi: 10.1590/S1516-635X2007000200004
30. Aliakbarpour HR, Chamani M, Rahimi G, Sadeghi AA, Qujeq D. The B. subtilis and lactic acid bacteria probiotics influences intestinal mucin gene expression, histomorphology and growth performance in broilers. Asian Austral J Anim Sci. (2012) 25:1285–93. doi: 10.5713/ajas.2012.12110
31. Jeong JS, Kim IH. Effect of B. subtilis C-3102 spores as a probiotic feed supplement on growth performance, noxious gas emission, and intestinal microflora in broilers. Poult Sci. (2014) 93:3097–103. doi: 10.3382/ps.2014-04086
32. Lei X, Piao X, Ru Y, Zhang H, Péron A, Zhang H. Effect of Bacillus amyloliquefaciens-based direct-fed microbial on performance, nutrient utilization, intestinal morphology and cecal microflora in broiler chickens. Asian Austral J Anim Sci. (2015) 28:239–46. doi: 10.5713/ajas.14.0330
33. Latorre JD, Hernandez-Velasco X, Vicente JL, Wolfenden R, Hargis BM, Tellez G. Effects of the inclusion of a Bacillus direct-fed microbial on performance parameters, bone quality, recovered gut microflora, and intestinal morphology in broilers consuming a grower diet containing corn distillers dried grains with solubles. Poult Sci. (2017) 96:2728–35. doi: 10.3382/ps/pex082
34. Ma Y, Wang W, Zhang H, Wang J, Zhang W, Gao J, et al. Supplemental Bacillus subtilis DSM 32315 manipulates intestinal structure and microbial composition in broiler chickens. Sci Rep. (2018) 8:15358. doi: 10.1038/s41598-018-33762-8
35. Cao GT, Zhan XA, Zhang LL, Zeng XF, Chen AG, Yang CM. Modulation of broilers' caecal microflora and metabolites in response to a potential probiotic Bacillus amyloliquefaciens. J Anim Physiol Anim Nutr. (2018) 102:e909–17. doi: 10.1111/jpn.12856
36. Jayaraman S, Thangavel G, Kurian H, Mani R, Mukkalil R, Chirakkal H. Bacillus subtilis PB6 improves intestinal health of broiler chickens challenged with Clostridium perfringens-induced necrotic enteritis. Poult Sci. (2013) 92:370–4. doi: 10.3382/ps.2012-02528
37. Tactacan GB, Schmidt JK, Miille MJ, Jimenez DR. A Bacillus subtilis (QST 713) spore-based probiotic for necrotic enteritis control in broiler chickens. J Appl Poult Res. (2013) 22:825–31. doi: 10.3382/japr.2013-00730
38. Whelan RA, Doranalli K, Rinttilä T, Vienola K, Jurgens G, Apajalahti J. The impact of B. subtilis DSM 32315 on the pathology, performance, and intestinal microbiome of broiler chickens in a necrotic enteritis challenge. Poult Sci. (2018) 98:3450–63. doi: 10.3382/ps/pey500
39. Hernandez-Patlan D, Solis-Cruz B, Pontin KP, Hernandez-Velasco X, Merino-Guzman R, Adhikari B, et al. Impact of a Bacillus direct-fed microbial on growth performance, intestinal barrier integrity, necrotic enteritis lesions, and ileal microbiota in broiler chickens using a laboratory challenge model. Front Vet Sci. (2019) 6:108. doi: 10.3389/fvets.2019.00108
40. Sokale AO, Menconi A, Mathis GF, Lumpkins B, Sims MD, Whelan RA, et al. Effect of Bacillus subtilis DSM 32315 on the intestinal structural integrity and growth performance of broiler chickens under necrotic enteritis challenge. Poult Sci. (2019) 98:5392–400. doi: 10.3382/ps/pez368
41. Bortoluzzi C, Serpa Vieira B, de Paula Dorigam JC, Menconi A, Sokale A, Doranalli K, et al. B. subtilis DSM 32315 supplementation attenuates the effects of Clostridium perfringens challenge on the growth performance and intestinal microbiota of broiler chickens. Microorganisms. (2019) 7:71. doi: 10.3390/microorganisms7030071
42. Bernardeau M, Vernoux JP. Overview of differences between microbial feed additives and probiotics for food regarding regulation, growth promotion effects and health properties and consequences for extrapolation of farm animal results to humans. Clin Microbiol Infect. (2013) 19:321–30. doi: 10.1111/1469-0691.12130
43. Pan D, Yu Z. Intestinal microbiome of poultry and its interaction with host and diet. Gut Microbes. (2014) 5:108–19. doi: 10.4161/gmic.26945
44. Wang W, Li Z, Gan L, Fan H, Guo Y. Dietary supplemental Kluyveromyces marxianus alters the serum metabolite profile in broiler chickens. Food Funct. (2018) 9:3776–87. doi: 10.1039/C8FO00268A
45. Hong YS, Hong KS, Park MH, Ahn YT, Lee JH, Huh CS, et al. Metabonomic understanding of probiotic effects in humans with irritable bowel syndrome. J Clin Gastroenterol. (2011) 45:415–25. doi: 10.1097/MCG.0b013e318207f76c
46. Vázquez-Fresno R, Llorach R, Marinic J, Tulipani S, Garcia-Aloy M, Espinosa-Martos I, et al. Urinary metabolomic fingerprinting after consumption of a probiotic strain in women with mastitis. Pharmacol Res. (2014) 87:160–5. doi: 10.1016/j.phrs.2014.05.010
47. Matsumoto M, Ebata T, Hirooka J, Hosoya R, Inoue N, Itami S, et al. Antipruritic effects of the probiotic strain LKM512 in adults with atopic dermatitis. Ann Allergy Asthma Immunol. (2014) 113:209–16. doi: 10.1016/j.anai.2014.05.002
48. Saggi SJ, Mercier K, Gooding JR, Friedman E, Vyas U, Ranganathan N, et al. Metabolic profiling of a chronic kidney disease cohort reveals metabolic phenotype more likely to benefit from a probiotic. Int J Probiotics Prebiotics. (2017) 12:43–54.
49. Baldassarre ME, Baldassarre ME, Di Mauro A, Tafuri S, Rizzo V, Gallone MS, Mastromarino P, et al. Effectiveness and safety of a probiotic-mixture for the treatment of infantile colic: a double-blind, randomized, placebo-controlled clinical trial with fecal real-time PCR and NMR-based metabolomics analysis. Nutrients. (2018) 10:e195. doi: 10.3390/nu10020195
50. Hong YS, Ahn YT, Park JC, Lee JH, Lee H, Huh CS, et al. 1H NMR-based metabonomic assessment of probiotic effects in a colitis mouse model. Arch Pharm Res. (2010) 33:1091–101. doi: 10.1007/s12272-010-0716-1
51. Abildgaard A, Elfving B, Hokland M, Wegener G, Lund S. Probiotic treatment reduces depressive-like behaviour in rats independently of diet. Psychoneuroendocrinology. (2017) 79:40–8. doi: 10.1016/j.psyneuen.2017.02.014
52. Sun M, Wu w, Liu Z, Cong Y. Microbiota metabolite short chain fatty acids, GCPR, and inflammatory bowel diseases. J gastroenterol. (2017) 52:1–8. doi: 10.1007/s00535-016-1242-9
53. Haghikia A, Jorg S, Duscha A, Berg J, Manzel A, Waschbisch A, et al. Dietary fatty acids directly impact central nervous system autoimmunity via the small intestine. Immunity. (2015) 43:817–29. doi: 10.1016/j.immuni.2015.09.007
54. Macia L, Thorburn AN, Binge LC, Marino E, Rogers KE, Maslowski KM, et al. Microbial influences on epithelial integrity and immune function as a basis for inflammatory diseases. Immunol Rev. (2012) 245:164–76. doi: 10.1111/j.1600-065X.2011.01080.x
55. Smith PM, Howitt MR, Panikov N, Michaud M, Gallini CA, Bohlooly YM, et al. The microbial metabolites, short-chain fatty acids, regulate colonic Treg cell homeostasis. Science. (2013) 341:569–73. doi: 10.1126/science.1241165
56. Wlodarska M, Thaiss CA, Nowarski R, Henao-Mejia J, Zhang JP, Brown EM, et al. NLRP6 inflammasome orchestrates the colonic host-microbial interface by regulating goblet cell mucus secretion. Cell. (2014) 156:1045–59. doi: 10.1016/j.cell.2014.01.026
Keywords: amino acid, fatty acid, gut, metabolomics, nucleoside, probiotics
Citation: Park I, Zimmerman NP, Smith AH, Rehberger TG, Lillehoj EP and Lillehoj HS (2020) Dietary Supplementation With Bacillus subtilis Direct-Fed Microbials Alters Chicken Intestinal Metabolite Levels. Front. Vet. Sci. 7:123. doi: 10.3389/fvets.2020.00123
Received: 06 December 2019; Accepted: 19 February 2020;
Published: 04 March 2020.
Edited by:
Vincenzo Tufarelli, University of Bari Aldo Moro, ItalyReviewed by:
Mohamed E. Abd El-Hack, Zagazig University, EgyptCristiano Bortoluzzi, University of Georgia, Georgia
Copyright © 2020 Park, Zimmerman, Smith, Rehberger, Lillehoj and Lillehoj. This is an open-access article distributed under the terms of the Creative Commons Attribution License (CC BY). The use, distribution or reproduction in other forums is permitted, provided the original author(s) and the copyright owner(s) are credited and that the original publication in this journal is cited, in accordance with accepted academic practice. No use, distribution or reproduction is permitted which does not comply with these terms.
*Correspondence: Hyun S. Lillehoj, aHl1bi5saWxsZWhvakBhcnMudXNkYS5nb3Y=