- 1Department of Veterinary Clinical Science, Small Animal Clinic, Justus-Liebig-University Giessen, Giessen, Germany
- 2Unit for Biomathematics and Data Processing, Faculty of Veterinary Medicine, Justus Liebig-University Giessen, Giessen, Germany
- 3Department of Internal Diseases With a Clinic for Horses, Dogs and Cats, Faculty of Veterinary Medicine, Wrocław University of Environmental and Life Sciences, Wrocław, Poland
- 4Center of Experimental Diagnostics and Innovative Biomedical Technologies, Faculty of Veterinary Medicine, Wrocław University of Environmental and Life Sciences, Wrocław, Poland
- 5Department of General Radiology and Interventional Radiology and Neuroradiology, Wrocław Medical University, Wrocław, Poland
Proton magnetic resonance spectroscopy (H1-MRS) could provide insight into the metabolic pathophysiology of the temporal lobe of canine brain after seizure. Currently, there is no evidence-based data available on MRS of temporal lobe in dogs with idiopathic epilepsy (IE). The aim of this prospective, cross-sectional study was to evaluate the interictal metabolic activity of the temporal lobe in IE dogs compared to a control group with the use of H1-MRS. Ten healthy dogs and 27 client-owned dogs with IE underwent 1.5-Tesla magnetic resonance imaging (MRI) and single-voxel H1-MRS. The MRS studies were acquired as spin echoes with a repetition time (TR) of 2,000 ms and an echo time (TE) of 144 ms. A cubic voxel (10 ×10 ×10 mm) was positioned bilaterally into the region of the left and right temporal lobe, including a middle part of the hippocampus and the amygdala. The N-acetylaspartate (NAA)-to-creatine (NAA/Cr), NAA-to-choline (NAA/Cho), choline-to-creatine (Cho/Cr), and choline-to-NAA (Cho/NAA) ratios were determined in both hemispheres and compared to controls. No significant differences in all metabolite ratios between epileptic dogs and the control group could be found. A time-dependent decrease in the NAA/Cho ratio as well as an increase in the Cho/NAA ratio was found with proximity in time to the last seizure. We found no correlation between metabolite ratios and age or sex in this animal group. Time span from the last seizure to the acquisition of MRS significantly correlated with NAA/Cho and Cho/NAA ratio. We conclude that without a time relation, metabolite ratios in dogs with IE do not differ from those of the control group.
Introduction
Epilepsy is the most common neurological disease in dogs (1–6). An increasing number of gene defects have been discovered in canine seizure disorders (6, 7), in which abnormal electrical activity arises from structurally intact neural tissue or a microstructurally deviant group of cells, which cannot be visualized in conventional MRI (8). Generally, the diagnosis of canine idiopathic epilepsy (IE) is made without evidence of MRI structural or morphological lesions or insults that secondarily influence the neuronal network (9). This concept might change in the face of growing evidence of documented structural changes in dogs with IE, like changes of apparent diffusion coefficient (ADC) parameters (10) or volumetric changes of white-to-gray matter ratio (11) or hippocampus (12, 13). The main knowledge about generalized tonic–clonic seizures (GTCSs) is based on electrophysiological experiments that indicated involvement of bilateral cortical, subcortical, and brain stem networks in the seizure activity (14–16). Seizures can arise from temporal lobe structures, including the hippocampus and amygdala, representing the most common form of epilepsy in humans and possibly in animals (1, 17–21). In human patients, the associated pathological substrate is usually a disorganization in the temporal lobes, amygdala, and hippocampal cytoarchitecture and gliotic atrophy of the pyramidal cell band of the cornu ammonis fields (hippocampal sclerosis) (22, 23). Alterations of hippocampal neurons and limbic structures have been found in histopathological examinations of the brain in epileptic dogs but were rather the consequence of seizure activity (24–26). Although the role of the hippocampus as a seizure generator in dogs is controversial (27), a growing body of evidence suggests at least some role of the temporal lobe structures in canine epilepsy (2, 11, 28, 29). T2-weighted (T2-w) hyperintense signals and atrophy of the hippocampus could be demonstrated in MRI investigations of epileptic dogs (2, 11, 28, 29), which is an indicator of structural changes of the hippocampus in humans (30, 31). An increased ADC has been measured in the temporal lobe of epileptic dogs (10). Finally, abnormal activity on electroencephalography (EEG), localized within the temporal lobe in dogs with epileptic seizures, has been documented in association with hippocampal atrophy (12, 32). These results warrant further investigation of the temporal region in canines with suspected idiopathic generalized epilepsy, helping to understand the changes of this brain area under seizure exposition.
Proton magnetic resonance spectroscopy (H1-MRS) provides biochemical information about specific brain regions of interest and visualizes alterations in metabolite concentrations before structural changes may be observed. In humans with temporal lobe epilepsy (TLE), it is sensitive in detecting metabolic alterations in dysfunctional epileptogenic regions such as the hippocampal formation before increased T2-signal intensity, loss of volume, and contrast enhancement have set in (33, 34). H1-MRS is widely used in human medicine for the detection of metabolic disturbances indicating neuronal injury/loss in the hippocampus in patients with generalized epilepsy (35–38). H1-MRS has now emerged as a technology with possible benefit for the study of canine brain function (39, 40) and various disease states (41–44) and can potentially provide insight into the cellular and metabolic pathophysiology of the temporal lobe.
The aim of our study was to evaluate the interictal metabolic activity of the temporal lobe region in dogs with IE using H1-MRS and compare the data to those of healthy controls. We hypothesized that dogs with IE would have relevant changes in examined metabolite ratios [N-acetylaspartate (NAA)-to-creatine (NAA/Cr), choline-to-creatine (Cho/Cr), NAA-to-choline (NAA/Cho), choline-to-NAA (Cho/NAA)] in comparison to the healthy group.
Materials and Methods
Ethics Statement
The study was conducted according to the University in Giessen (Germany) and in Wroclaw (Poland) institutional guidelines. This study was approved by the institutional animal care and use committee and adheres to the principles for the humane treatment of animals as stated by the Polish Institutes of Health guidelines (45). The owners of the dogs in the study and control group gave their written consent to the enrollment in this study.
Study Population
The prospective study was performed in the Department of Internal Medicine with a Clinic for Horses, Dogs and Cats, Faculty of Veterinary Medicine, Wroclaw University of Environmental and Life Sciences, Wroclaw, Poland. All dogs underwent clinical and neurological examinations by a veterinary neurologist. Pre-anesthetic laboratory investigations comprised complete blood cell count and serum biochemistry panel, electrolytes as well as fasted ammonia, bile acids, and urinalysis. MRI of the brain was performed in all dogs. The cerebrospinal fluid (CSF) was obtained by puncture of the cerebellomedullary cistern under general anesthesia immediately after the MRI examination. Cytologic and biochemical analyses of the CSF including specific gravity, electrolytes, leukocyte count, complete blood cell count, protein concentration, Pandy's reaction, and glucose concentration were performed. Except bile acids, ammonia testing, all investigations were also performed in the control group.
Only dogs diagnosed with IE that manifested generalized tonic–clonic seizures were included in the study group. The diagnosis of IE was based on: (1) the clinical history of repeated seizures based on the anamnesis with the owner and the presence of a seizure diary for evaluation in dogs, with a special focus on the last observed generalized seizure occurrence; (2) the age between 6 months and 6 years at the time of the first seizure onset; (3) unremarkable interictal physical and neurological examinations; (4) normal complete blood cell count, biochemistry panel, and electrolytes, as well as fasted serum ammonia and bile acids, CSF examination, and urinalysis; (5) normal MRI of the brain, performed according to the International Veterinary Epilepsy Task Force recommendations (46). All patients included in the study group did not receive any epileptic treatment at the time of the examination.
Cases suspected of paroxysmal events other than epileptic origin (e.g., cardiogenic syncope, vestibular disease, narcolepsy) were excluded. Dogs included in the control group underwent the examination of the cervical spine for various clinical reasons and were included in the study if having no history of epileptic seizures and fulfilled the abovementioned inclusion criteria.
Anesthetic Protocol
Each patient underwent premedication using medetomidine [0.02–0.03 mg/kg intramuscularly (i.m.)] with butorphanol (0.1–0.4 mg/kg). General anesthesia was induced with propofol [4–8 mg/kg intravenously (i.v.)] and maintained by inhaled anesthetics (1.8% isoflurane in oxygen).
Magnetic Resonance Imaging
All MRI and MRS data were acquired in ventral recumbency with a 1.5-Tesla MR scanner (Ingenia; Philips Medical Systems, 2013) using Stream HeadSpine 15-channel coil (Philips Medical Systems, 2013). All animals enrolled in the study underwent a standardized MRI protocol (International Veterinary Epilepsy Task Force-IVETF recommended protocol) that included 2-mm T2-w spin echo (SE) images, 2-mm fluid-attenuated inversion recovery (FLAIR) sequence, T2* sequence, 0.8-mm T1-weighted three-dimensional (3D), pre- and post-contrast images, following intravenous administration of gadolinium (Gadobutrol 604.72 mg/mmol, solution for injection). All MR sequences were performed as follows: conventional transverse and sagittal T2-w images [repetition time (TR) 4,423.5 ms/echo time (TE) 100 ms], dorsal FLAIR (TR 9,000 ms/TE 140 ms/inversion time (TI) 2,450 ms), 3D T1-w pre- and post-contrast images (TR 25 ms/TE 5.1 ms).
Magnetic Resonance Spectroscopy
In all dogs, an additional single-voxel proton MRS was introduced to the standard epileptic MRI protocol performed before intravenous administration of the contrast medium. The MRS studies were targeted to regions of interest (temporal lobe, hippocampal area; Figures 1A–C). A cubic voxel (10 ×10 ×10 mm) was identical for all studies and manually positioned bilaterally, symmetrically at a thin, 0.8-mm T1-w 3D pre-contrast dorsal, sagittal, and transverse into the region of left and right temporal lobe, including a middle part of the hippocampus and the amygdala.
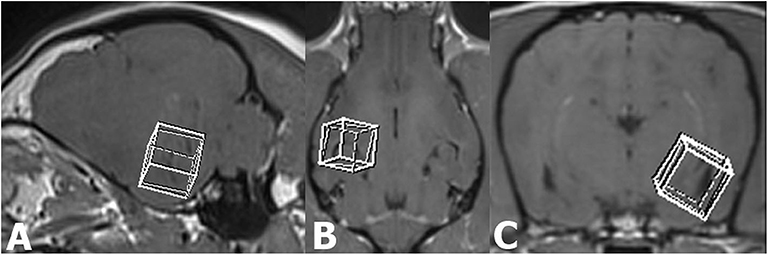
Figure 1. Sagittal (A), dorsal (B), and transverse (C) T1-weighted MR images illustrating the voxel placement in the temporal lobe including the hippocampus and part of the amygdala.
Voxel positioning was carefully done, with avoidance of the temporal bone, and lateral ventricle inclusion to prevent unwanted signal contributions or chemical shift contamination from non-nervous tissues. Volume selective excitation [point resolved spectroscopy (PRESS)] with chemical shift-selective (CHESS) pulse for water suppression (bandwidth 50 Hz) was used. The MRS studies were acquired as spin echoes with a TR of 2,000 ms, a TE of 144 ms, and a number of phase-encoding steps (NP) of 1.024. A total of 256 images were averaged for an acquisition time of 10 min for each investigated side of the mesial temporal/middle hippocampal/amygdala area.
Spectrum Analysis
MRI studies were anonymized and presented in a randomized order. Metabolite peaks were identified according to their resonance frequency position along the horizontal axis. The analyzed metabolites included NAA, Cho, and Cr (Figures 2A,B), as they were the only repeatable and readable metabolites detected via 1.5-Tesla scanner.
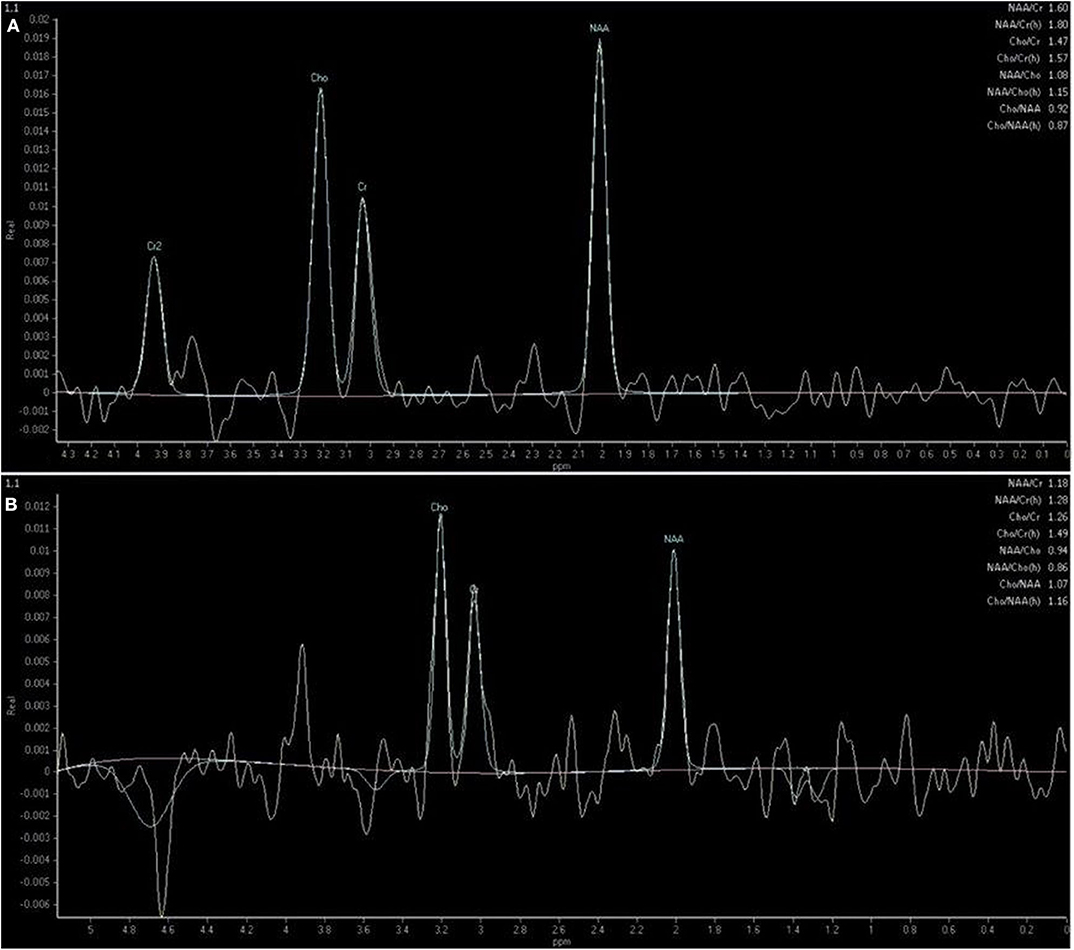
Figure 2. Single-voxel spectrum acquired at the level of the temporal lobe showing metabolite peaks of N-acetylaspartate (NAA) at 2.0 ppm, creatine (Cr) at 3.0 ppm, choline (Cho) at 3.2 ppm and the calculated metabolite ratios in a mixed-breed dog with degenerative disc disease included in the control group (A) and in a 5-year-old mixed-breed male dog with idiopathic epilepsy and last seizure 7 days ago (B).
An automatic and operator-non-dependent data processing system was used to obtain the spectra recorded for our study (SpectroView Analysis software, Philips Healthcare). Spectral post-processing included baseline adjustment, noise level filtering, metabolite peak calibration, and the application of spectral plotting, followed by Fourier transformation. The desired signals of metabolite peaks were detected as follows: NAA at 2.02 ppm, Cho at 3.22 ppm, and Cr at 3.02 ppm. The relative ratios of NAA/Cr, Cho/Cr, NAA/Cho, and Cho/NAA were automatically calculated for both groups using resonance areas.
Statistical Analysis
The collected data were analyzed using statistical software (BMDP/Dynamic, Statistical Software, Inc., Release 8.1). Descriptive statistics were calculated for peak area ratios at MRS. The statistical evaluation included the four metabolite ratios (NAA/Cho, NAA/Cr, Cho/Cr, and Cho/NAA ratios), evaluated equally for both groups. Normal distribution of the data was assessed using a Kolmogorov–Smirnov test. The mean and standard deviation (SD) for the metabolite ratios (NAA/Cho, NAA/Cr, Cho/Cr, and Cho/NAA ratios) for the left and right side were calculated. A two-factor repeated-measures ANOVA test with respect to a side was used to compare the ratio values of the control to those of the epileptic dogs group. A three-factor analysis of covariance (ANCOVA) with repeated measure with respect to a side was used to examine the correlation between the metabolite ratios and the covariates age and sex in the groups. A single-factor ANCOVA with repeated measures with respect to a side of the voxel was applied to examine a possible correlation between the values of the metabolite ratios, seizure duration before examination, and time from the last seizure. The level of significance (α) was P <0.05 for all applied tests.
Results
The IE group comprised in total 27 dogs of different breeds with diagnosed IE and structurally normal MRI of the brain. A list of breeds, age, and gender in the group is given in Table 1. Fifteen dogs in the IE group were male, and 12 were female. Ten dogs without brain disease were included in the control group (Table 2). The mean age of the IE dogs was 3.5 years (with a range from 1 to 7 years), whereas the mean age of the control group was 2.7 years (with a range from 1 to 4 years). All dogs in the IE group showed GTCS with symmetrical bilateral involvement, salivation, and urination.
No significant differences were observed when comparing the metabolite ratios between the control and IE group (Table 3). Moreover, the ratios for the left and right regions of interest similarly did not vary significantly between the examined groups. There was no significant influence of age for all examined metabolite ratios (NAA/Cr: P = 0.598; Cho/Cr: P = 0.862; NAA/Cho: P = 0.898; Cho/NAA: P = 0.788). Similarly, no significant correlation between sex and metabolite ratios was found (NAA/Cr: P = 0.0633; Cho/Cr: P = 0.517; NAA/Cho: P = 0.065; Cho/NAA: P = 0.063).
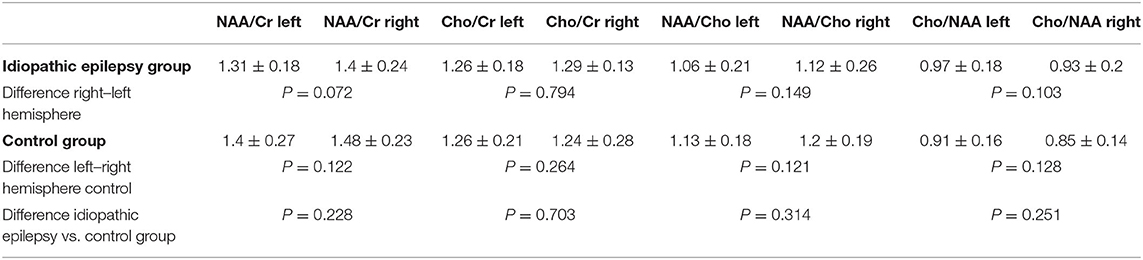
Table 3. Mean, standard deviation, and P-values for calculated brain metabolite ratios [N-acetylaspartate (NAA)/creatine (Cr), choline (Cho)/Cr, NAA/Cho, Cho/NAA] of dogs included in the idiopathic epilepsy and control groups.
A significant positive correlation was found between NAA/Cho ratio (P = 0.03) and Cho/NAA ratio (P = 0.01) and time from last seizure. The regression coefficient was −0.005 in NAA/Cho, indicating higher values of this ratio with closer distance from the last seizure (Table 4). For the Cho/NAA ratio, the regression coefficient was positive (0.008), indicating lower values in dogs with recent seizures. The ratios NAA/Cr (P = 0.21) and Cho/Cr (P = 0.169) showed no significant correlation with time from the last seizure event.
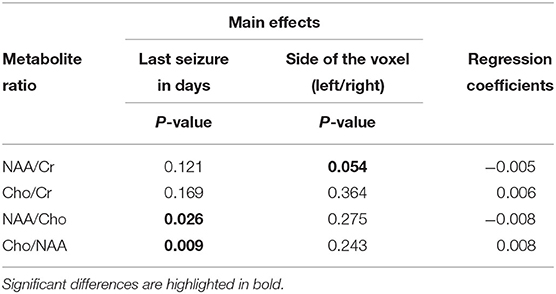
Table 4. Significant positive correlation found between N-acetylaspartate (NAA)/choline (Cho) ratio (P = 0.03) and Cho/NAA ratio (P = 0.01) and time span from last seizure and the acquisition of magnetic resonance spectroscopy (MRS) in comparison to the control group with relation to the side of the voxel. Calculated regression coefficients show how the metabolite ratio increases [NAA/creatine (Cr), NAA/Cho] and decreases (Cho/Cr, Cho/NAA) over time.
Discussion
In this study, we present data of interictal single-voxel H1-MRS of the temporal lobe with hippocampus and amygdala region in dogs with IE and a structurally normal brain. No significant changes in the investigated metabolite ratios were observed in the IE group compared to the controls if a time span from the last seizure was not taken under consideration. Several studies in human medicine on the degree of metabolite ratio changes in patients during ictal, postictal, and interictal phases were performed (47–49). The common findings include a significant decrease in NAA/Cr and NAA/Cho ratios in the affected brain region, usually during ictal and postictal phases. Metabolite disturbances tend to normalize in the affected area in the postictal and interictal period, strongly suggesting rather the functional changes of neurons (48, 49). However, in one study, no significant differences in metabolite ratios in the postictal and interictal phases were observed (47). It is possible that examined metabolite ratios normalized in the interictal phase or there were primarily no metabolite ratio abnormalities in the examined region of interest.
A relation between metabolite ratios and the time from the last seizure was found, i.e., the closer the MRS of the temporal lobe was obtained from the time of the last seizure, the lower was the Cho/NAA ratio. NAA/Cho ratio was analogously higher in dogs with recent seizures and decreased over time. The significantly higher NAA/Cho ratio in our study in dogs with recent seizures is potentially caused by higher/stable NAA concentration or lower choline concentration in the examined brain region.
The major brain metabolites analyzed with long echo time H1-MRS of brain tissue using 1.5-Tesla MRI include NAA, Cho, and Cr, as these metabolites have the most prominent and readily identifiable peaks. NAA, Cho, and Cr compounds could represent the neuronal, astrocytic, and mitochondrial associated dysfunction in epilepsy and therefore were selected for further investigation in our patients (50–52). NAA is synthetized from L-aspartate and acetyl coenzyme A mostly in neurons (53, 54). Various NAA-fixation staining techniques allowed the visualization of NAA at different levels with different neuronal populations including higher levels in pyramidal neurons with longer axons and more extensive myelination than in smaller interneurons (55, 56). NAA is one of the most abundant amino acids in the central nervous system and is involved in energy metabolism (57). It has also been suggested to play a role in the synthesis of myelin and lipids in the central nervous system (58). Loss of NAA signals is considered to be consistent with loss of neuronal integrity but can also be used as a marker of impaired neuronal function and reduced oxidative metabolism (59). Increase in NAA concentration as a result of toxic buildup of NAA and secondary cell death or impaired brain metabolism associated with the inability to catabolize NAA can be related to osmotic stress (60, 61). Significantly higher NAA/Cho ratio in the presented study could potentially be caused by higher/stable NAA in canines with recent seizures. High concentrations of NAA delivered to the lateral ventricle of rats were found to induce seizures (62, 63). Further MRS studies during the ictal period are needed to evaluate these changes. Cho is an essential metabolite responsible for the synthesis of cell membranes and its degeneration (membrane turnover). Moreover, Cho is a precursor of phosphocholine, and acetylcholine and is involved in lipid transport and methyl-group metabolism (64). The concentration of Cho represents cellular density and cell water turnover, and it differs depending on the localization. Excessive concentrations of acetylcholine and its precursor Cho were also found in the cortex and hippocampus in rat brain during epileptic seizures and were responsive to pharmacological manipulation (65). The decreased level of Cho results in lower acetylcholine synthesis and decreased concentration in the hippocampus leading to impaired sleep and memory (66). A significant change in NAA/Cho ratio in our study on dogs with recent seizures could also suggest a secondary lower level of Cho and acetylcholine in our dogs. Cr plays an essential role in energy synthesis being converted by creatine kinase (CK) to phosphocreatine (PCr), which is directly used in a production of adenosine triphosphate (ATP) (61, 67). Cr is synthesized in axonal mitochondria, oligodendrocytes, dendrites, synapses, and neuronal cell bodies. Total Cr is often applied as an internal reference for MRS due to its relative stability in both normal and pathological brain tissue (68–71). Cr spectrum signal was also found significantly reduced in MRS in animal models of epilepsy (72) and in a canine model of pentylentetrazole-induced generalized seizures (73).
In human patients with IE, spectroscopic studies of the posterior hippocampus, amygdala, and mesial temporal lobe area are mainly used to determine the lateralization of the epileptogenic zone. The unilateral decrease in NAA/Cr, NAA/Cho, or Cho/Cr ratios showed good concordance with the localization of the epileptogenic zone in the respective hippocampus (35, 36) and predicts a positive surgical outcome (74). Our study showed no significant changes in metabolite ratios between the left and right regions of interest. Furthermore, resection of the hippocampus is also performed in patients with structural or functional extra-temporal seizure foci if changes in the hippocampus can be detected (75–77). The presence of hippocampal damage and/or dysfunction, in association with a lesion outside the temporal lobe, is assumed to be due to the propagation of epileptogenic activity from the remote focus into the hippocampus formation, inducing secondary epileptogenic effects (78). Interictal spectroscopic examination revealed reduced hippocampal NAA/Cr and NAA/Cho in humans with extra-temporal epilepsy (37, 78).
Our understanding of epilepsy in dogs is still limited and somewhat focused on the existence of primary TLE (8). However, the proof of any negative effect of the hippocampus on seizure generation or enhancement in epileptic dogs might take us a step forward and encourage veterinary neurosurgeons to consider resection of the temporal lobe in dogs with refractory epilepsy (13). The finding of this study, namely, that GTCS did not create long-term changes in metabolic spectra measured in the mesial temporal region including middle hippocampus, may suggest that repeated seizures do not cause secondary damage in this area in dogs. Alternatively, changes were observed only in the short time period after the seizure (79). Another possibility is that dogs that did not show changes in the MRS of this area were suffering from an epileptogenic zone in a different brain region. Extensive studies on GTCS in human medicine presented thalamo-cortical dysfunction with the GTCS-associated necrosis in thalamic relay neurons (80) as well as abnormal functional connectivity between various cortical and subcortical regions including cingulate cortex and temporal and olfactory lobes (16). The MRS studies of thalamus in patients with absence epilepsy showed decreased NAA/Cr ratio, suggesting neuronal injury in this area (81). The focus of this research did not involve the investigation of adjacent areas or other regions of the brain. Placement of the voxel in the temporal lobe area in dogs with GTCS in the study group was based on recent studies in veterinary medicine, which suggested the active involvement of those structures in the development of GTCS. Metabolic alterations in the thalamus, cingulate cortex, as well as other cortical areas should be further studied in canines with IE to examine the origin of GTCS and compare those results.
However, the fact that other studies documented functional or structural changes of the hippocampus (10, 12, 13, 28) warrants further investigations limited to hippocampus formation using H1-MRS. Further detailed classification of dogs with focal seizures and focal seizures evolving to generalized seizures, as well as use of continuous long-term electroencephalography, should be considered in order to improve anatomical localization of epileptic activity.
The results of our study might have been limited due to a number of reasons. Although we could not document a correlation between the duration of seizure activity before imaging, the influence of seizure frequency and duration cannot be ruled out. Humans with TLE, studied within 24 h postictal period, showed no changes in metabolite ratios, suggesting that these ratios are insensitive to immediate seizure history (47, 82). Another study found no relationship between NAA/Cho, NAA/Cr, and seizure duration, frequency, or lifetime estimated seizures (39). We found a correlation between time distance to the last seizure and decrease in NAA/Cho. If all dogs would have been examined at a comparable time distance to the last seizure, the differences in the metabolite ratios might have become significant. The difference between ratios between left and right hippocampus formation almost reached significance, which might also increase with standardized imaging modalities. These possible connections should be further investigated. Metabolite ratios can also differ along the long axis of the hippocampus with lower ratios of NAA/Cho and NAA/Cr in the anterior as compared with the posterior part of the hippocampus (83).
We decided for single-voxel measurement as it provides volume selectivity of the acquired signal and therefore high signal-to-noise ratio, resulting in high-quality spectra suitable for quantitative analysis. However, using single-voxel acquisition, it was technically not possible to reduce the voxel <1 cm in size. Therefore, the spectra were not measured exclusively in the temporal lobe but contained information from adjacent brain tissue, especially from the thalamus. Overlapping the thalamus however, in our opinion, produces much less signal contamination in comparison to overlapping the calvaria and producing poor-quality spectra due to fat contamination (84). This can be seen as a systematic error that occurred in every examined dog, but still with the use of 1.5-Tesla magnet and 1-cm voxel placing, it was technically unavoidable and still could be used as a reference for further studies.
Multi-voxel acquisition techniques offer the advantage of examining smaller regions of interest, which is important when studying small or irregularly shaped anatomic structures such as the hippocampus formation. However, spectral contamination from adjacent voxels can have a high influence on the values, which makes quantification of the spectra difficult (54). Ratios determined by Warrington obtained in the temporal lobe of 10 healthy beagles using multi-voxel acquisition were higher than those in our control group (39). Both absolute values and NAA/Cho, NAA/Cr, and Cho/Cr ratios have a larger range and median values, most likely reflecting the differences of single- vs. multi-voxel acquisition. Nevertheless, these MRS results should be compared with caution, given that ratios obtained by Warrington were evaluated using 3-Tesla MRI. Metabolite ratios may vary depending on the type of the software, field strength, and protocol type for MRS (85). On the other hand, in the study by Kim et al. (86), no significant differences were observed between the two field strengths.
Systematic comparison between spectroscopic data obtained by single- and multi-voxel acquisition has been performed in dog cadavers (84). According to the recent preliminary findings (87), single- and multi-voxel spectroscopy techniques yield comparable results for similar sized regions of interest in the normal canine brain.
The absolute metabolite quantification can be obtained in two different ways: as ratios or relative metabolite concentrations (relative to water content of the brain) (54). The results of our spectra are expressed in terms of ratios, which is considered to be a stable indicator to describe parenchymal changes in humans (34, 88). Metabolite ratios such as NAA/Cr and Cho/Cr have been used commonly for quantification, instead of relative metabolite levels (71, 83). However, the use of metabolite ratios potentially increases the variability of the result compared with the individual components over half the time (88).
In contrary, relative metabolite concentrations can be measured using the so-called linear combination model (LCModel). LCModel analysis method is particularly attractive for the evaluation of the uncertainty (e.g., Cramer-Rao lower bounds), which estimates the quality of the spectra used for further analysis. The dedicated LCModel software is widely available in human medicine and also has been already used in some veterinary medicine studies (40–44). The main advantage of the LCModel is that the software is highly automated, has built-in corrections, and requires little user input. It can estimate concentrations of even minor metabolites to high internal precision, which enables to detect a broader spectrum of metabolites of interest. The LCModel is used to analyze the complete spectra, rather than individual peaks, which is an advantage that enables to distinguish two metabolites in one frequency region with nearly identical spectra but different signals in other parts of the spectrum (89). To our knowledge. There is no study in veterinary medicine evaluating the use of LCModel in epileptic canines. Future MRS studies on epilepsy in dogs should focus on comparison of both quantification techniques.
Among the single-voxel techniques, PRESS and stimulated echo acquisition mode (STEAM) are generally used for MRS. Molecules selected for our study (NAA, Cho, Cr) can only be detected on 1.5-Tesla field strength at long echo times. Thus, long echo time PRESS sequence was used in our study because of the improved signal intensity-to-noise ratio (SNR) and a more readable spectrum which contains less signal from the lipid and metabolites with short T2 values (54). In contrary, STEAM technique is favorable for exposure of small metabolites with shorter T2 times (glutamine, glutamate, myoinositol) (90, 91). Nevertheless, a higher field MR scanner is required for the examination at short echo T2 times. Metabolites with shorter T2 relaxation times are not resolved from each other at 1.5 Tesla, thus cannot be observed on the spectrum. Glutamate, glutamine, and myoinositol as well as lipids could have not been measured in studied dogs. Further investigation is necessary to present if these compounds would be observed in the interictal phase in dogs with seizure activity. Several studies in human medicine presented increased lactate and myoinositol and decreased glutamate and glutamine in patients experiencing active seizures (48, 51). Further studies on epileptic dogs examining metabolites at short and long echo times using PRESS and STEAM techniques simultaneously need to be performed.
It is also unclear as to whether the measured spectra and ratios might be breed-specific or breed specifically biased due to different skull conformations and brain volume. We included two brachycephalic dogs in our IE group. Curved shape of the analyzed region might have caused slight changes in tissue components in the examined voxels, as the hippocampal volume differs significantly in size in allometric relationships to brain and body weight (92), but there are no data on this issue. Comparisons between larger cohorts of homogeneous groups of dogs of the same breed will be necessary to prove such breed influence on MRS spectra.
It was shown that magnetic field strength has a dependent influence on the measured spectra (93). With the increase of magnetic field strength, an increase in absolute chemical shift is also induced so that the metabolite peaks can be better delineated. When the magnetic field strength is doubled from 1.5 to 3 Tesla, the signal of the metabolite peaks also increases, since the SNR depends on the magnetic strength (85). Future veterinary studies comparing diagnostic accuracy of the obtained spectra at 3 Tesla with 1.5 Tesla should be considered; however, 1.5-Tesla magnets are currently the most available for veterinary patients.
Interictal single-voxel proton MRS of the mesial temporal lobe, middle hippocampus, and amygdala area in dogs with IE and structurally normal MRI revealed no statistically relevant changes in examined metabolite ratios (NAA/Cr, Cho/Cr, NAA/Cho, Cho/NAA) in comparison to the healthy group. However, the NAA/Cho and Cho/NAA ratios were correlated with time duration to seizure. Our findings support the concept that MRS provides further information about the status of brain function and proves reversible metabolic dysfunction.
Data Availability Statement
The datasets used and analyzed during the current study are available from the corresponding author on reasonable request.
Ethics Statement
The animal study was reviewed and approved by Local Ethical Committee of the Justus Liebig University Gießen, Germany and National Ethics Committee on Animal Experimentation, Poland. Written informed consent was obtained from the owners for the participation of their animals in this study.
Author Contributions
AO participated in the design of the study, acquisition of data, and drafting of the manuscript. MW, PP, and JN made a substantial contribution to the acquisition of data. KF performed the statistical analysis. MW and MS: made a substantial contribution to the design of the study and helped to draft the manuscript. All authors read and approved the final manuscript.
Conflict of Interest
The authors declare that the research was conducted in the absence of any commercial or financial relationships that could be construed as a potential conflict of interest.
The reviewer IC declared a past co-authorship with one of the authors MS to the handling editor.
Acknowledgments
The content of the presented manuscript has previously appeared online as part of a doctoral thesis (94).
Abbreviations
ADC, apparent diffusion coefficient; ANCOVA, analysis of covariance; ANOVA, analysis of variance; ATP, adenosine triphosphate; CHESS, chemical shift-selective; Cho, choline; Cho/Cr, choline-to-creatine ratio; Cho/NAA, choline-to-N-acetylaspartate ratio; CK, creatine kinase; Cr, creatine; CSF, cerebrospinal fluid; EEG, electroencephalography; FLAIR, fluid-attenuated inversion recovery; GTCS, generalized tonic–clonic seizure; H1-MRS, proton magnetic resonance spectroscopy; IE, idiopathic epilepsy; IVETF, International Veterinary Epilepsy Task Force; LCModel, linear combination model; MRI, magnetic resonance imaging; MRS, magnetic resonance spectroscopy; NAA, N-acetylaspartate; NAA/Cho, N-acetylaspartate-to-choline ratio; NAA/Cr, N-acetylaspartate-to-creatine ratio; NP, number of phase-encoding steps; PCr, phosphocreatine; PRESS, point resolved spectroscopy sequence; SD, standard deviation; SE, spin echo; SNR, signal intensity-to-noise ratio; STEAM, stimulated echo acquisition mode; T1-w, T1-weighted; T2-w, T2-weighted; TE, echo time; TI, inversion time; TLE, temporal lobe epilepsy; TR, repetition time.
References
1. Löscher W. Animal models of intractable epilepsy. Prog Neurobiol. (1997) 53:239–58. doi: 10.1016/S0301-0082(97)00035-X
2. Thomas WB. Idiopathic epilepsy in dogs and cats. Vet Clin N Am Small Anim Pract. (2010) 40:161–79. doi: 10.1016/j.cvsm.2009.09.004
3. Bellumori TP, Famula TR, Bannasch DL. Prevalence of inherited disorders among mixed-breed and purebred dogs: 27.254 cases (1995–2010). J Am Vet Med Assoc. (2013) 242:1549–55. doi: 10.2460/javma.242.11.1549
4. Kearsley-Fleet L, O'Neill DG, Volk HA, Church DB, Brodbelt DC. Prevalence and risk factors for canine epilepsy of unknown origin in the UK. Vet Rec. (2013) 172:338–8. doi: 10.1136/vr.101133
5. Heske L, Nødtvedt A, Jäderlund KH. A cohort study of epilepsy among 665,000 insured dogs: incidence, mortality and survival after diagnosis. Vet J. (2014) 202:471–6. doi: 10.1016/j.tvjl.2014.09.023
6. Hülsmeyer VI, Fischer A, Mandigers PJ. International veterinary epilepsy task force's current understanding of idiopathic epilepsy of genetic or suspected genetic origin in purebred dogs. BMC Vet Res. (2015) 11:175. doi: 10.1186/s12917-015-0463-0
7. Wielaender F, Sarviaho R, James F, Hytönen MK, Cortez MA, Kluger G. Generalized myoclonic epilepsy with photosensitivity in juvenile dogs caused by a defective DIRAS family. Proc Natl Acad Sci USA. (2017) 10:2669–74. doi: 10.1073/pnas.1614478114
8. Hasegawa D. Diagnostic techniques to detect the epileptogenic zone: pathophysiological and presurgical analysis of epilepsy in dogs and cats. Vet J. (2016) 215:64–75. doi: 10.1016/j.tvjl.2016.03.005
9. Berendt M, Farquhar RG, Mandigers PJ, Pakozdy A, Bhatti SF, De Risio L, et al. International veterinary epilepsy task force consensus report on epilepsy definition, classification and terminology in companion animals. BMC Vet Res. (2015) 11:182. doi: 10.1186/s12917-015-0461-2
10. Hartmann A, Sager S, Failing K, Sparenberg M, Schmidt MJ. Diffusion-weighted imaging of the brains of dogs with idiopathic epilepsy. BMC Vet Res. (2017) 13:338. doi: 10.1186/s12917-017-1268-0
11. Wrzosek M, Podgórski P, Drobot P, Bodys W, Nicpoń J. The MR volumetric brain assessment in canine epileptic patient—a pilot study. In: Oral Presentation at the 29th Symposium of the ESVN-ECVN. Edinburgh (2016).
12. Czerwik A, Płonek M, Podgórski P, Wrzosek M. Comparison of electroencephalographic findings with hippocampal magnetic resonance imaging volumetry in dogs with idiopathic epilepsy. J Vet Int Med. (2018) 32:2037–44. doi: 10.1111/jvim.15323
13. Estey CM, Dewey CW, Rishniw M. A subset of dogs with presumptive idiopathic epilepsy show hippocampal asymmetry: a volumetric comparison with non-epileptic dogs using MRI. Front Vet Sci. (2017) 4:183. doi: 10.3389/fvets.2017.00183
14. Gloor P, Metrakos J, Metrakos K, Andermann E, van Gelder N. Neurophysiological, genetic and biochemical nature of the epileptic diathesis. Electroencephalogr. Clin. Neurophysiol. (1982) 35:45–56.
15. Fisher RS, Cross JH, French JA, Higurashi N, Hirsch E, Jansen FE, et al. Operational classification of seizure types by the International League Against Epilepsy: position paper of the ILAE Commission for Classification and Terminology. Epilepsia. (2017) 58:522–30. doi: 10.1111/epi.13670
16. Ji GJ, Zhang Z, Xu Q, Zang YF, Liao W, Lu G. Generalized tonic–clonic seizures: aberrant interhemispheric functional and anatomical connectivity. Radiology. (2014) 271:839–47. doi: 10.1148/radiol.13131638
17. Hauser WA, Annegers JF, Kurland LT. Prevalence of epilepsy in Rochester, Minnesota: 1940–1980. Epilepsia. (1991) 32:429–45. doi: 10.1111/j.1528-1157.1991.tb04675.x
18. Spencer SS, Marks D, Katz A, Kim J, Spencer DD. Anatomic correlates of interhippocampal seizure propagation time. Epilepsia. (1992) 33:862–73. doi: 10.1111/j.1528-1157.1992.tb02194.x
19. Calcagnotto ME, Barbarosie M, Avoli M. Hippocampus-entorhinal cortex loop and seizure generation in the young rodent limbic system. J Neurophysiol. (2000) 83:3183–7. doi: 10.1152/jn.2000.83.5.3183
20. Hasegawa D, Nakamura S, Fujita M, Takahashi K, Orima H. A dog showing Klüver-Bucy syndrome-like behavior and bilateral limbic necrosis after status epilepticus. Vet Neurol Neurosurg. (2005) 7:1–14. doi: 10.4159/9780674038400
21. Kitz S, Thalhammer JG, Glantschnigg U, Wrzosek M, Klang A, Halasz P, et al. Feline temporal lobe epilepsy: review of the experimental literature. J Vet Int Med. (2017) 31:633–40. doi: 10.1111/jvim.14699
22. Mathern GW, Babb L, Pretoriuq K. Reactive synaptogenesis and neuron densities for neuropeptide and glutamate decarboxylase immunoreactivity in the epileptogenic human fascia dentata. J Neurosci. (1995) 15:3990–4004. doi: 10.1523/JNEUROSCI.15-05-03990.1995
23. Blümcke I, Thom M, Wiestler OD. Ammon's horn sclerosis: a maldevelopmental disorder associated with temporal lobe epilepsy. Brain Path. (2002) 12:199–211. doi: 10.1111/j.1750-3639.2002.tb00436.x
24. Montgomery DL, Lee AC. Brain damage in the epileptic beagle dog. Vet Pathol. (1983) 169:160–9. doi: 10.1177/030098588302000203
26. Yamasaki H, Uruoka HF, Kechi MT, Itakura C. Neuronal loss and gliosis in limbic system in an epileptic dog. Vet Pathol. (1991) 28:540–2. doi: 10.1177/030098589102800614
27. Buckmaster PS, Smith MO, Buckmaster CL, Lecouteur RA, Dudek FE. Absence of temporal lobe epilepsy pathology in dogs with medically intractable epilepsy. J Vet Int Med. (2002) 16:95–9. doi: 10.1111/j.1939-1676.2002.tb01612.x
28. Kuwabara T, Hasegawa D, Kobayashi M, Fujita M, Orima H. Clinical magnetic resonance volumetry of the hippocampus in 58 epileptic dogs. Vet Radiol Ultrasound. (2010) 51:485–90. doi: 10.1111/j.1740-8261.2010.01700.x
29. Milne ME, Anderson GA, Chow KE, O'Brien MD, Moffat BA Long SN. Description of technique and lower reference limit for magnetic resonance imaging of hippocampal volumetry in dogs. Am J Vet Res. (2013) 74:224–31. doi: 10.2460/ajvr.74.2.224
30. Jack CR, Sharbrough FW, Cascino GD, Hirschorn KA, O'Brien PC, Marsh WR. Magnetic resonance image-based hippocampal volumetry: correlation with outcome after temporal lobectomy. Ann Neurol. (1992) 31:138–46. doi: 10.1002/ana.410310204
31. Cendes F, Andermann F, Gloor P, Evans A, Jones-Gotman M, Watson C, et al. MRI volumetric measurement of amygdala and hippocampus in temporal lobe epilepsy. Neurology. (1993) 43:719–25. doi: 10.1212/WNL.43.4.719
32. Berendt M, Høgenhaven H, Flagstad A, Dam M. Electroencephalography in dogs with epilepsy: similarities between human and canine findings. Acta Neurol Scand. (1999) 99:276–83. doi: 10.1111/j.1600-0404.1999.tb00676.x
33. Cendes F, Andermann F, Preul MC, Arnold DL. Lateralization of temporal lobe epilepsy based on regional metabolic abnormalities in proton magnetic resonance spectroscopic images. Ann Neurol. (1994) 35:211–6. doi: 10.1002/ana.410350213
34. Kuzniecky RI, Jackson GD. Magnetic Resonance in Epilepsy : Neuroimaging Techniques. 2nd ed. Burlington, MA: Elsevier Academic Press (2015). p. 333–43.
35. Hetherington HP, Kuzniecky RI, Pan JW. Application of high field spectroscopic imaging in the evaluation of temporal lobe epilepsy. Magn Reson Imaging. (1995) 13:1175–80. doi: 10.1016/0730-725X(95)02029-S
36. Li LM, Cendes F, Antel SB. Prognostic value of proton magnetic resonance spectroscopic imaging for surgical outcome in patients with intractable temporal lobe epilepsy and bilateral hippocampal atrophy. Ann Neurol. (2000) 47:195–200. doi: 10.1002/1531-8249(200002)47:2<195::AID-ANA9>3.0.CO;2-2
37. Mueller SG, Laxer KD, Cashdollar N. Spectroscopic evidence of hippocampal abnormalities in neocortical epilepsy. Eur J Neurol. (2006) 13:256–60. doi: 10.1111/j.1468-1331.2006.01188.x
38. Hetherington HP, Kuzniecky RI, Vives K. A subcortical network of dysfunction in TLE measured by magnetic resonance spectroscopy. Neurology. (2007) 69:2256–65. doi: 10.1212/01.wnl.0000286945.21270.6d
39. Warrington CD, Feeney DA, Ober CP. Relative metabolite concentrations and ratios determined by use of 3-T region-specific proton magnetic resonance spectroscopy of the brain of healthy beagles. Am J Vet Res. (2013) 74:1291–303. doi: 10.2460/ajvr.74.10.1291
40. Carrera I, Richter H, Meier D, Kircher PR, Dennler M. Regional metabolite concentrations in the brain of healthy dogs measured by use of short echo time, single voxel proton magnetic resonance spectroscopy at 3.0 Tesla. Am J Vet Res. (2015) 76:129–41. doi: 10.2460/ajvr.76.2.129
41. Carrera I, Kircher PR, Meier D, Richter H, Beckman K, Dennler M. In vivo proton magnetic resonance spectroscopy for the evaluation of hepatic encephalopathy in dogs. Am J Vet Res. (2014) 75:818–27. doi: 10.2460/ajvr.75.9.818
42. Carrera I, Richter H, Beckmann K, Meier D, Dennler M, Kircher P. Evaluation of intracranial neoplasia and noninfectious meningoencephalitis in dogs by use of short echo time, single voxel proton magnetic resonance spectroscopy at 3.0 Tesla. Am J Vet Res. (2016) 77:452–62. doi: 10.2460/ajvr.77.5.452
43. Beckmann K, Carrera I, Steffen F, Golini L, Kircher PR, Schneider U, et al. A newly designed radiation therapy protocol in combination with prednisolone as treatment for meningoencephalitis of unknown origin in dogs: a prospective pilot study introducing magnetic resonance spectroscopy as monitor tool. Acta Vet Scand. (2015) 571–4. doi: 10.1186/s13028-015-0093-3
44. Sievert C, Richter H, Beckmann K, Kircher PR, Carrera I. Comparison between proton magnetic resonance spectroscopy findings in dogs with tick-borne encephalitis and clinically normal dogs. Vet Rad Ultrasound. (2016) 58:53–61. doi: 10.1111/vru.12427
45. Rakoczy B. Act on the protection of animals used for scientific or educational purposes—legal regulation review. Dz U. (2015) 266:79–88. doi: 10.12775/PYEL.2015.004
46. Rusbridge C, Long S, Jovanovik J, Milne M, Berendt M, Bhatti SF, et al. International veterinary epilepsy task force recommendations for a veterinary epilepsy-specific MRI protocol. BMC Vet Res. (2015) 11:194. doi: 10.1186/s12917-015-0466-x
47. Maton B, Londono A, Sawrie S. Postictal stability of proton magnetic resonance spectroscopy imaging (1H-MRSI) ratios in temporal lobe epilepsy. Neurology. (2001) 56:251–3. doi: 10.1212/WNL.56.2.251
48. Simister RJ, McLean MA, Salmenpera TM, Barker GJ, Duncan JS. The effect of epileptic seizures on proton MRS visible neurochemical concentrations. Epilepsy Res. (2008) 81:36–43. doi: 10.1016/j.eplepsyres.2008.04.009
49. Fadaie F, Mobarakeh NM, Fesharaki SS, Harirchian MH, Kharazi HH, Rad HS, et al. 1H-MRS metabolite's ratios show temporal alteration in temporal lobe seizure: comparison between interictal and postictal phases. Epilepsy Res. (2016) 12;128:158–62. doi: 10.1016/j.eplepsyres.2016.08.015
50. De Lanerolle NC, Lee TS, Spencer DD. Astrocytes and epilepsy. Neurotherapeutics. (2010) 7:424–38. doi: 10.1016/j.nurt.2010.08.002
51. Filibian M, Frasca A, Maggioni D. In vivo imaging of glia activation using 1H-magnetic resonance spectroscopy to detect putative biomarkers of tissue epileptogenicity. Epilepsia. (2012) 53:1907–16. doi: 10.1111/j.1528-1167.2012.03685.x
52. Folbergrová J, Kunz WS. Mitochondrial dysfunction in epilepsy. Mitochondrion. (2012) 12:35–40. doi: 10.1016/j.mito.2011.04.004
53. Urenjak J, Williams SR, Gadian DG. Specific expression of N-acetylaspartate in neurons, oligodendrocyte-type-2 astrocyte progenitors, and immature oligodendrocytes in vitro. J Neurochem. (1992) 59:55–61. doi: 10.1111/j.1471-4159.1992.tb08875.x
54. Barker P, Bizzi A, De Stefano N, Gullapalli R, Lin D. Clinical MR Spectroscopy: Techniques and Applications. Cambridge: Cambridge University Press (2009). p. 9–21. doi: 10.1017/CBO9780511770647
55. Moffett JR, Namboodiri MA, Neale JH. Enhanced carbodiimide fixation for immunohistochemistry: application to the comparative distributions of N-acetylaspartylglutamate and N-acetylaspartate immunoreactivities in rat brain. J Histochem Cytochem. (1993) 41:559–70. doi: 10.1177/41.4.8450195
56. Moffett JR, Namboodiri MA. Differential distribution of N-acetylaspartylglutamate and N-acetylaspartate immunoreactivities in rat forebrain. J Neurocytol. (1995) 24:409–33. doi: 10.1007/BF01181604
57. Miyake M, Kakimoto Y, Sorimachi M. A gas chromatographic method for the determination of N-acetyl-L-aspartic acid, N-acetyl-alpha-aspartylglutamic acid and beta-citryl-L-glutamic acid and their distributions in the brain and other organs of various species of animals. J Neurochem. (1981) 36:804–10. doi: 10.1111/j.1471-4159.1981.tb01665.x
58. Moffett JR, Ross B, Arun P, Madhavarao CN, Namboodiri AMA. N-Acetylaspartate in the CNS: from neurodiagnostics to neurobiology. Prog Neurobiol. (2007) 81:89–131. doi: 10.1016/j.pneurobio.2006.12.003
59. Woermann FG, McLean MA, Bartlett PA. Short echo time single-voxel 1H magnetic resonance spectroscopy in magnetic resonance imaging-negative temporal lobe epilepsy: different biochemical profile compared with hippocampal sclerosis. Ann Neurol. (1999) 45:369–76. doi: 10.1002/1531-8249(199903)45:3<369::AID-ANA13>3.0.CO;2-Q
60. Pederzolli CD, Rockenbach FJ, Zanin FR, Henn NT, Romagna EC, Sgaravatti AM. Intracerebroventricular administration of N-acetylaspartic acid impairs antioxidant defenses and promotes protein oxidation in cerebral cortex of rats. Metab Brain Dis. (2009) 24:283–98. doi: 10.1007/s11011-009-9137-6
61. Stagg CJ, Rothman DL. Magnetic Resonance Spectroscopy: Tools for Neuroscience Research and Emerging Clinical Applications. Waltham, MA: Elsevier Academic Press (2013). p. 71–104.
62. Akimitsu T, Kurisu K, Hanaya R, Iida K, Kiura Y, Arita K. Epileptic seizures induced by N-acetyl-L-aspartate in rats: in vivo and in vitro studies. Brain Res. (2000) 861:143–50. doi: 10.1016/S0006-8993(00)02028-X
63. Kitada K, Akimitsu T, Shigematsu Y, Kondo A, Maihara T, Yokoi N. Accumulation of N-acetyl-L-aspartate in the brain of the tremor rat, a mutant exhibiting absence-like seizure and spongiform degeneration in the central nervous system. J Neurochem. (2000) 74:2512–9. doi: 10.1046/j.1471-4159.2000.0742512.x
64. Loffelholz K, Klein J, Koppen A. Choline, a precursor of acetylcholine and phospholipids in the brain. Prog Brain Res. (1993) 98:197–200. doi: 10.1016/S0079-6123(08)62399-7
65. Jope RS, Gu X. Seizures increase acetylcholine and choline concentrations in rat brain regions. Neurochem Res. (1991) 16:1219–26. doi: 10.1007/BF00966699
66. Gais S, Scharf B, Wagner U, Born J. Low acetylcholine during slow-wave sleep is critical for memory consolidation. J Psychophysiol. (2003) 17:148–8. doi: 10.1073/pnas.0305404101
67. Steen C, Wilczak N, Hoogduin JM. Reduced creatine kinase B activity in multiple sclerosis normal appearing white matter. PLoS ONE. (2010) 5:10811. doi: 10.1371/journal.pone.0010811
68. Condon B. Magnetic resonance imaging and spectroscopy: how useful is it for prediction and prognosis? EPMA J. (2011) 2:403–10. doi: 10.1007/s13167-011-0086-x
69. Connett RJ. Analysis of metabolic control: new insights using scaled creatine kinase model. Am J Physiol. (1988) 254:949–59. doi: 10.1152/ajpregu.1988.254.6.R949
70. De Stefano N, Narayanan S, Francis SJ. Diffuse axonal and tissue injury in patients with multiple sclerosis with low cerebral lesion load and no disability. Arch Neurol. (2002) 59:1565–71. doi: 10.1001/archneur.59.10.1565
71. Tartaglia MC, Narayanan S, De Stefano N. Choline is increased in pre-lesional normal appearing white matter in multiple sclerosis. J Neurol. (2002) 249:1382–90. doi: 10.1007/s00415-002-0846-6
72. Hiremath GK, Najm IM. Magnetic resonance spectroscopy in animal models of epilepsy. Epilepsia. (2007) 48:47–55. doi: 10.1111/j.1528-1167.2007.01241.x
73. Neppl R, Nguyen CM, Bowen W. In vivo detection of postictal perturbations of cerebral metabolism by use of proton MR spectroscopy: preliminary results in a canine model of prolonged generalized seizures. AJNR Am J Neuroradiol. (2001) 22:1933–43. Available online at: http://www.ajnr.org/content/22/10/1933
74. Suhy J, Laxer KD, Capizzano AA. H MRSI predicts surgical outcome in MRI-negative temporal lobe epilepsy. Neurology. (2002) 58:821–3. doi: 10.1212/WNL.58.5.821
75. Cascino GD, Jack CR, Parisi JE. Operative strategy in patients with MRI-identified dual pathology and temporal lobe epilepsy. Epilepsy Res. (1993) 14:175–82. doi: 10.1016/0920-1211(93)90022-Y
76. Li LM, Cendes F, Watson C, Andermann F. Surgical treatment of patients with single and dual pathology: relevance of lesion and of hippocampal atrophy to seizure outcome. Neurology. (1997) 48:437–44. doi: 10.1212/WNL.48.2.437
77. Li LM, Andermann F. Surgical outcome in patients with epilepsy and dual pathology. Brain. (1999) 122:799–805. doi: 10.1093/brain/122.5.799
78. Miller SP, Li LM, Cendes F, Tasch E. Medial temporal lobe neuronal damage in temporal and extratemporal lesional epilepsy. Neurology. (2000) 54:1465–70. doi: 10.1212/WNL.54.7.1465
79. Mellema LM, Koblik PD, Kortz GD, LeCouteur RA, Chechowitz MA, Dickinson PJ. Reversible magnetic resonance imaging abnormalities in dogs following seizures. Vet Radiol Ultrasound. (1999) 40:588–95. doi: 10.1111/j.1740-8261.1999.tb00884.x
80. Nevander G, Ingvar M, Auer R, Siesjö BK. Status epilepticus in well-oxygenated rats causes neuronal necrosis. Ann Neurol. (1985) 18:281–90. doi: 10.1002/ana.410180303
81. Fojtiková D, Brázdil M, Skoch A, Jíru F, Horký J, Marecek R, et al. Magnetic resonance spectroscopy of the thalamus in patients with mesial temporal lobe epilepsy and hippocampal sclerosis. Epileptic Disord. (2007) 1:S59–67. doi: 10.1684/epd.2007.0148
82. Cendes F, Andermann F, Dubeau F. Normalization of neuronal metabolic dysfunction after surgery for temporal lobe epilepsy. Evidence from proton MR spectroscopic imaging. Neurology. (1997) 49:1525–33. doi: 10.1212/WNL.49.6.1525
83. Vermathen P, Laxer KD, Matson GB. Hippocampal structures: anteroposterior N-acetylaspartate differences in patients with epilepsy and control subjects as shown with proton MR spectroscopic imaging. Radiology. (2000) 214:403–10. doi: 10.1148/radiology.214.2.r00fe43403
84. Ober CP, Warrington CD, Feeney DA. Optimizing a protocol for (1) H-magnetic resonance spectroscopy of the canine brain at 3T. Vet Rad Ultrasound. (2013) 54:149–58. doi: 10.1111/vru.12010
85. Rahman A, Choudhary MI. Applications of NMR Spectroscopy. Vol. 2. Bentham Science Publishers (2015). p. 99–102.
86. Kim JH, Chang KH, Na DG. Comparison of 1.5T and 3T 1H MR spectroscopy for human brain tumors. Korean J Radiol. (2006) 7:156–61. doi: 10.3348/kjr.2006.7.3.156
87. Lee AJ, Beasley MD, Barrett ER, James J, Gambino J. Single-voxel and multi-voxel spectroscopy yield comparable results in the normal juvenile canine brain when using 3 Tesla magnetic resonance imaging. Vet Radiol Ultrasound. (2018) 59:577–86. doi: 10.1111/vru.12634
88. Li BSY, Wang H, Gonen O. Metabolite ratios to assumed stable creatine level may confound the quantification of proton brain MR spectroscopy. Magn Reson Imaging. (2003) 8:923–8. doi: 10.1016/S0730-725X(03)00181-4
89. Provencher SW. Automatic quantitation of localized in vivo 1H spectra with LCModel. NMR Biomed. (2001) 14:260–4. doi: 10.1002/nbm.698
90. Castillo M, Kwock L, Mukherji SK. Clinical applications of proton MR spectroscopy. AJNR Am J Neuroradiol. (1996) 17:1–15.
92. Frahm HD, Zilles K. Volumetric comparison of hippocampal regions in 44 primate species. J Hirnforsch. (1994) 35:343–54.
93. Inglese M, Spindler M, Babb JS, Sunenshine P, Law M, Gonen O. Field, coil, and echo-time influence on sensitivity and reproducibility of brain proton MR spectroscopy. AJNR Am J Neuroradiol. (2006) 27:6848. Available online at: https://pubmed.ncbi.nlm.nih.gov/8770242/
Keywords: dog, idiopathic epilepsy, MRI, MRS, seizures
Citation: Olszewska A, Schmidt MJ, Failing K, Nicpoń J, Podgórski P and Wrzosek MA (2020) Interictal Single-Voxel Proton Magnetic Resonance Spectroscopy of the Temporal Lobe in Dogs With Idiopathic Epilepsy. Front. Vet. Sci. 7:644. doi: 10.3389/fvets.2020.00644
Received: 20 May 2020; Accepted: 10 August 2020;
Published: 24 September 2020.
Edited by:
John Henry Rossmeisl, Virginia Tech, United StatesReviewed by:
Daisuke Hasegawa, Nippon Veterinary and Life Science University, JapanInes Carrera, Willows Veterinary Centre, United Kingdom
Copyright © 2020 Olszewska, Schmidt, Failing, Nicpoń, Podgórski and Wrzosek. This is an open-access article distributed under the terms of the Creative Commons Attribution License (CC BY). The use, distribution or reproduction in other forums is permitted, provided the original author(s) and the copyright owner(s) are credited and that the original publication in this journal is cited, in accordance with accepted academic practice. No use, distribution or reproduction is permitted which does not comply with these terms.
*Correspondence: Agnieszka Olszewska, YWduaWVzemthLm9sc3pld3NrYUB2ZXRtZWQudW5pLWdpZXNzZW4uZGU=