- 1Department of Animal and Dairy Sciences, Mississippi State University, Starkville, MS, United States
- 2Department of Animal Genetics, Kastamonu University, Kastamonu, Turkey
- 3Department of Reproduction and Artificial Insemination, Selcuk University, Konya, Turkey
- 4URUS Group LP, Madison, WI, United States
- 5Department of Basic Sciences, College of Veterinary Medicine, Mississippi State University, Starkville, MS, United States
The objective of this study was to ascertain the cellular and functional parameters as well as ROS related changes in sperm from bulls with varied sperm freezability phenotypes. Using principal component analysis (PCA), the variables were reduced to two principal components, of which PC1 explained 48% of the variance, and PC2 explained 24% of the variance, and clustered animals into two distinct groups of good freezability (GF) and poor freezability (PF). In ROS associated pathophysiology, there were more dead superoxide anion positive (Dead SO+) sperm in GF bulls than those in PF (15.72 and 12.00%; P = 0.024), and that Dead SO+ and live hydrogen positive cells (live H2O2+) were positively correlated with freezability, respectively (R2 = 0.55, P < 0.0130) and (rs = 0.63, P = 0.0498). Related to sperm functional integrity, sperm from PF bulls had greater dead intact acrosome (DIAC) than those from GF bulls (26.29 and 16.10%; P = 0.028) whereas sperm from GF bulls tended to have greater live intact acrosome (LIAC) than those from PF bulls (64.47 and 50.05%; P = 0.084). Sperm with dead reacted acrosome (DRAC) in PF bulls were greater compared to those in GF (19.27 and 11.48%; P = 0.007). While DIAC (R2 = 0.56, P = 0.0124) and DRAC (R2 = 0.57, P < 0.0111) were negatively correlated with freezability phenotype, LIAC (R2 = 0.36, P = 0.0628) was positively correlated. Protamine deficiency (PRM) was similar between sperm from GF and PF bulls (7.20 and 0.64%; P = 0.206) and (rs = 0.70, P = 0.0251) was correlated with freezability. Sperm characteristics associated with cryotolerance are important for advancing both fundamental andrology and assisted reproductive technologies across mammals.
Introduction
Bull fertility, regarded as the ability of viable sperm to fertilize the egg and then support early embryonic development, is essential for the propagation of species, and it is an important economic trait for animal breeding programs. There is a global need for modern and sustainable food farming of animals such as cattle for the production of quality milk and meat to feed the world. The use of fresh or frozen sperm through artificial insemination (AI) combined with genomic selection has accelerated the genetic improvement of livestock (1, 2). Cryopreservation, defined as the successive stabilization of temperature with the process of dehydration, freezing, thawing, is a useful and profitable procedure for long-term storage of sperm in domestic animals and humans (3). Cryopreservability or freezability of sperm is crucial for the success of AI, which has become the preferred method of breeding in the cattle industry, especially in dairy cattle agriculture. The bovine livestock industry has also been evolved by more advanced protocols and cryoprotectants that has resulted in the improvements of the fertility of frozen-thawed sperm (4–6).
Harsh procedures of cooling, freezing, and thawing cause dramatic changes in the cell which induce injuries to the sperm membrane, thereby reducing sperm quality (7). More specifically, cooling, freezing, and thawing sperm result in high levels of structural and physiological damage from oxidative stress, osmotic injury, and formation of intracellular ice crystals (8–10). All of these contribute to the decrease in fertility, largely due to the impairment of the cell membrane with roughly 50% reduction in viability and motility as well as acrosome integrity (11–13). Optimal freezing temperatures and rates rather than extreme fast and slow seem to be required to avoid the formation of intercellular ice crystals, and to achieve post-thaw survival because more than half of the cryopreserved sperm die post-thaw (14–16). These freezing-induced changes disrupt cell membranes (17) and oxidation of lipids caused by cryopreservation techniques that stimulate the production of reactive oxygen species (ROS) by generating free radicals and osmotic stress resulting in DNA fragmentation in sperm (18–20).
Oxidative stress is a potential factor for poor fertility status with abnormal sperm parameters and indicates the imbalance between the overproduction of ROS and total antioxidant capacity in the cell. Oxidative stress gives rise to impaired sperm function by causing DNA damage, thus remains a challenging factor for male infertility and potential pregnancy losses (21–23). At the physiological concentrations, ROS is necessary for aerobic metabolism, membrane fluidity, and sperm fertilizing ability, while also critical for acrosome reaction and capacitation by modulating cAMP synthesis and tyrosine phosphorylation, and by signaling to support fertilization (24–26). Overproduction of ROS that exceeds the antioxidant capacity of the seminal plasma deteriorates sperm function (27, 28). The procedures of sperm cryopreservation induce production of free radicals caused by the removal of the great majority of seminal plasma, cold shock, and osmotic stress as well as the ubiquitous presence of dead and damaged spermatozoa (29–31).
Despite the significance of sperm freezability affecting bull fertility and its economic impact on cattle farming, there exists no optimal extenders and no conventional methods to accurately evaluate sperm freezability. These gaps in the knowledge and technology bases are important because they are preventing advances both in cryobiology of mammalian male gamete and in the ART. Causes underlying differing freezability remain to be elusive, although physical damage to the membranes and biochemical changes have been associated with cryo-induced oxidative stress (32–34). The overall goal of this study was to test the central hypothesis that sperm cellular and functional phenomes are associated with sperm integrity and freezability.
The specific objective was to ascertain the cellular and functional parameters as well as ROS related changes in sperm from bulls with varied sperm freezability phenotypes. The results of this study advance our understanding of sperm cryopreservation and the development of more integrative methods for precision reproduction through applied biology.
Results
Principal Component Analysis for Functional Parameters of Freezability
Using PCA, we reduced the variables to two principal components, PC1 explaining 48% of the variances and PC2 explaining 24% of the variances, and clustered animals into two distinct groups of GF and PF, with an outlier for the GF (Figure 1). Based on the patterns of these variables, PC1 was primarily correlated with FRAP (r = 0.91; P = 0.0002), live H2O2− (r = 0.97; P < 0.0001), dead H2O2− (r = 0.96; P < 0.0001), dead H2O2+ (r = 0.63; P = 0.052), live SO+ (r = 0.98; P < 0.0001), dead SO– (r = 0.95; P < 0.0001), DIAC (r = 0.68; P = 0.031), and DRAC (r = 0.70; P = 0.025), LIAC (r = −0.84; P = 0.003), LRAC (r = 0.63; P = 0.049), and MitoSOX– (r = 0.96; P < 0.0001). From the PCA patterns, PC2 was the component that separated the GF and PF groups. It was correlated with LPO (r = 0.68; P = 0.030), live H2O2+ (r = 0.70; P = 0.025), dead SO+ (r = 0.90; P = 0.0003), and PRM (r = 0.72; P = 0.0176).
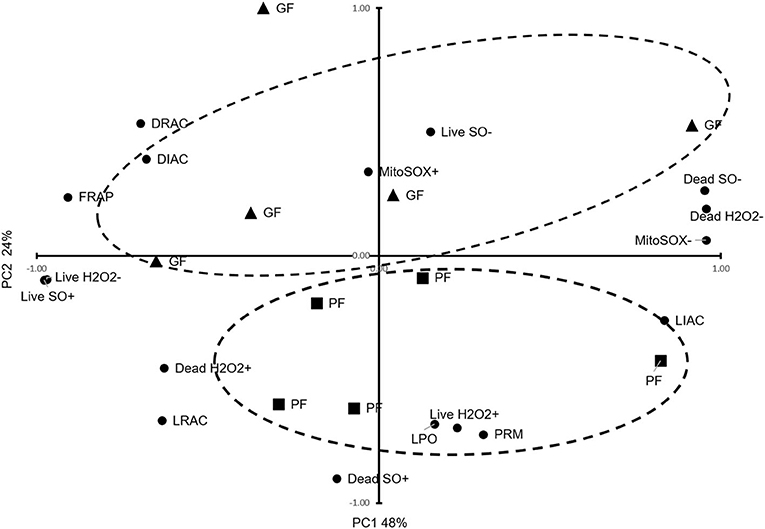
Figure 1. Principal Component Analysis for functional parameters of freezability. The dispersion of bulls regarding important sperm functional parameters, TAC, oxidative parameters, PRM deficiency, and freezability status are provided. The dashed ellipses represent good freezability (GF) and poor freezability (PF).
Sperm Total Antioxidant Capacity (TAC) and Oxidative Parameters in GF and PF Bulls
By assessing the total antioxidant capacity (TAC) in the GF and PF bulls, the current study revealed that the FRAP values of GF and PF were similar (55.26 and 63.35 μM, respectively; P = 0.462). Lipid peroxidation (LPO), as a second messenger of oxidative stress, in bull sperm was measured by the reaction of thiobarbituric acid (TBA). The difference between the GF and PF bulls was similar for lipid peroxidation (4.57 and 3.98 μM, respectively; P = 0.272). The percentages of live hydrogen peroxide negative (Live H2O2−) and live hydrogen peroxide positive (Live H2O2+) sperm cells did not differ between the GF and PF bulls, respectively (47.65 and 47.96%, P = 0.974) and (1.10 and 0.012%, P = 0.364). Likewise, percentages of dead H2O2 negative (Dead H2O2−) and dead H2O2 positive (Dead H2O2+) sperm were similar GF and PF bulls, respectively (51.02 and 51.98%, P = 0.92) and (0.19 and 0.03%, P = 0.16). As for the assessment of superoxide anion (SO) in semen from GF and PF bulls, the percentages of live superoxide anion (Live SO–) and live superoxide anion positive (Live SO+) were similar between the freezability groups, respectively (0.03 and 0.28%, P = 0.284), (48.44 and 49.84%, P = 0.878). The percentage of dead SO– (Dead SO–) were similar between the GF and PF groups (35.79 and 37.93%, respectively; P = 0.823), while the dead superoxide anion positive (Dead SO+) sperm in the GF bulls were greater than in the PF (15.72 and 12.00%, respectively; P = 0.024). The levels of mitochondria-specific reactive oxygen species were measured in sperm from bulls with different sperm freezability phenotypes. The percentages of mitochondrial negative ROS (MitoSOX–) and positive ROS (MitoSOX+) were similar between the GF and PF, respectively (47.33 and 43.13%, P = 0.686) and (1.79 and 2.59%, P = 0.489) as depicted in Table 1.
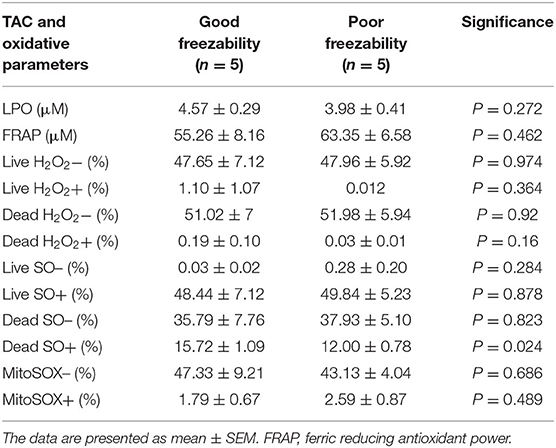
Table 1. Sperm total antioxidant capacity (TAC), lipid peroxidation (LPO)-oxidative parameters in good and poor freezability bulls.
Sperm Functional Parameters in GF and PF Bulls
Analyses of data on sperm functional parameters revealed that GF had greater PTV than PF (63.83 and 52.10%, respectively; P = 0.0001). The results generated by the pattern of forward and side scatter (FSC-SSC) light revealed the acrosome status of sperm from different freezability bulls. Sperm samples from PF bulls had greater dead intact acrosome (DIAC) than those from the GF bulls (26.29 and 16.10%, respectively; P = 0.028) whereas GF bulls had almost greater live intact acrosome (LIAC) than the PF bulls (64.47 and 50.05, respectively; P = 0.084). There was also greater dead reacted acrosome (DRAC) in PF bulls compared to those from the GF (19.27 and 11.48%, respectively; P = 0.007). However, live reacted acrosome (LRAC) percentages were similar between the GF and PF bulls (7.54 and 4.10%, respectively; P = 0.17). We also demonstrated through protamine deficiency assay that the levels of PRM were similar between the GF and PF bulls (7.20 and 0.64%, respectively; P = 0.206) as depicted in Table 2.
Associations Between Post-thaw Viability and Sperm Characteristics
Pearson correlation was performed for the sperm parameters between the GF and PF groups. Using scatter plots, we demonstrated the distributions of bulls for sperm functional parameters in relation to freezability status. The number of cells with dead intact acrosome (DIAC) (R2 = 0.56, P = 0.0124) and dead reacted acrosome (DRAC) (R2 = 0.57, P < 0.0111) were negatively correlated with freezability phenotype. The number of cells with live intact acrosome (LIAC) (R2 = 0.36, P = 0.0628) and Dead SO+ (R2 = 0.55, P < 0.0130) were positively correlated with freezability (Figure 2). The nonparametric measure of spearman rank correlation of PTV with live H2O2+, dead H2O2+, live SO–, and PRM in GF (n = 5) and PF (n = 5) were performed for freezability bulls. The PTV was significantly correlated with live H2O2+ (rs = 0.63, P = 0.0498), and PRM (rs = 0.70, P = 0.0251) whereas dead H2O2+ (rs = 0.26061, P = 0.4671) and live SO– (rs = −0.28049, P = 0.4325) have no significant association with PTV.
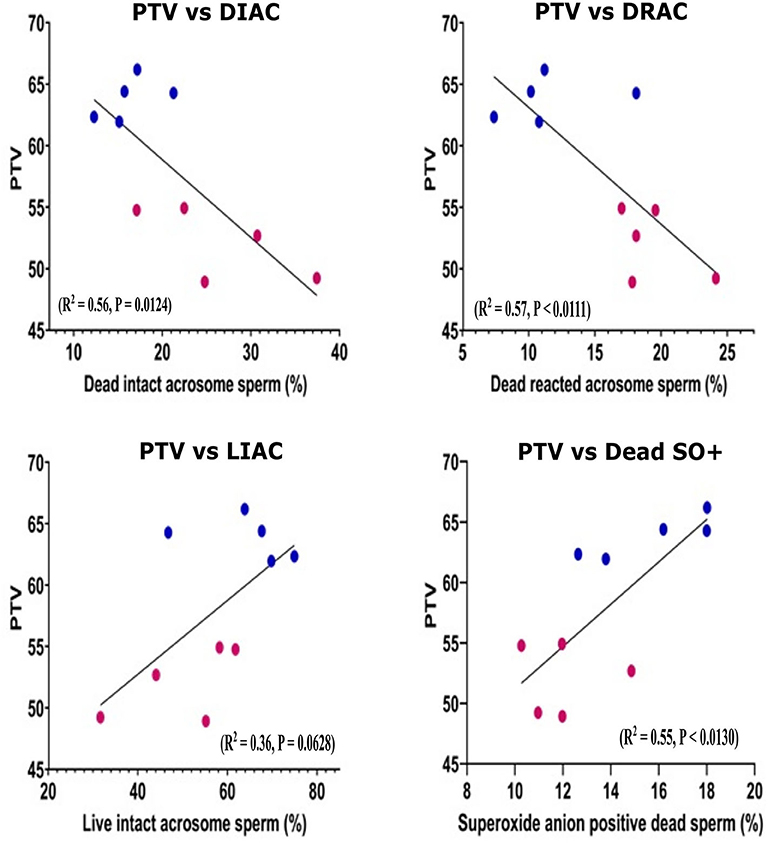
Figure 2. Scatter plot distribution of different sperm parameters. Scatter plot distribution of different sperm parameter in good (n = 5) and poor (n = 5) freezability bulls. Lower (claret) dots: PF bulls, upper (blue) dots: GF bulls. The Pearson correlation is indicated. PTV, Post thaw viability; DIAC, dead intact acrosome; DRAC, dead reacted acrosome; LIAC, live intact acrosome; LRAC, live reacted acrosome; Dead SO+, dead superoxide anion.
Discussion
The cellular and functional health of sperm in cryopreservation, as well as dynamics of ROS and antioxidant capacity, are poorly defined. Lack of such knowledge is an important problem because it hinders the understanding process in cryobiology of mammalian male gametes and the advancement of ART. The overarching goal of this study was to ascertain cellular and functional phenomes associated with sperm integrity and freezability using methods in contemporary biology and sperm from phenotypically distinct bulls.
In this study, the two principal components explained 72% of the variance of PTV which clustered animals into two distinct groups of GF and PF, with an outlier for the GF. PC1 explaining 48% of variance correlated with FRAP, live H2O2−, dead H2O2−, dead H2O2+, live SO+, dead SO–, MitoSOX–, DIAC, DRAC, and LIAC. This showed that total antioxidant capacity and acrosome status as well as some oxidative parameters define PC1. However, PC2 explained 24% of the variance, which was correlated with LPO, live H2O2+, dead SO+, and LRAC, and PRM. These findings showed that lipid peroxidation, oxidative parameters, and protamine deficiency status accounted for the variance in PC2 (Figure 1). The PCA suggests that a combination of analyses from different variables of cellular and functional parameters as well as oxidative parameters can help distinguish the freezability status of bulls.
Sperm are susceptible to oxidative stress due to the presence of large amounts of polyunsaturated fatty acids in sperm membranes as potential targets of oxidative stress (35). In addition, the sperm cytoplasm is small and contains low levels of protective enzymes and antioxidants. As such, sperm membranes are prone to LPO during freezing and thawing cycles (27). In our study, we did not find a correlation between LPO and freezability phenotypes, and the concentration was similar between GF and PF bulls (Table 1). In support of these findings, LPO was not related to freezing-thawing of fresh and frozen human sperm (36). In rams, LPO did not change during cooling and thawing procedures of cryopreservation (37). Moreover, young and old buffalo bulls had similar levels of lipid peroxidation in fresh vs. frozen-thawed sperm (38). It was shown that lipid peroxidation of bull sperm has no relationship with the concentration of some of the enzymatic antioxidants (39). However, Gürler et al. (40) reported that SOD and LPO were positively correlated, which was not anticipated because SOD causes dismutation of O2 which then renders to H2O2. They also, in similar lines with literature, found a negative relationship between LPO and total antioxidant capacity 3 h after thawing and stimulation of LPO by t-butyl hydroperoxide. We inferred that sperm cells still maintain the balance between TAC and oxidative stress induced LPO for a while and without any induction. Therefore, our findings in LPO experiments may have resulted from the stability of the sperm membrane counteracting the lipid compositions during freezing-thawing.
The antioxidant systems in sperm are of cytoplasmic origin and counterbalance the detrimental effects of cryopreservation which results from lipid peroxidation and ROS (41, 42). In our study, there was no correlation between TAC and freezability phenotypes, and the concentrations were similar between GF and PF bulls (Table 1). Although the parameters of enzymatic antioxidant defense mechanisms were not evaluated in our study, the enzymatic antioxidant defenses play important roles against lipid peroxidation and that the seminal plasma is the main source of antioxidants (43, 44). However, it was reported that GPX and SOD enzymes are not sufficient to alleviate cryodamage because intercellular antioxidants may support only physiological changes in sperm cells (32).
Production of ROS is one of the primary underlying reasons for sperm damage in cryopreservation which in turn impairs post-thaw motility, viability, and other sperm parameters (45, 46). However, the exact involvement of H2O2 and superoxide anion (SO) in male fertility remains elusive. In bovine sperm cells, Gürler et al. (33) showed that H2O2 causes DNA damage without any changes in other ROS and sperm viability. Unexpectedly, while the levels of live H2O2+ were similar between the freezability groups (Table 1), PTV was correlated with live H2O2+, implying that a higher percentage of viable sperm cells in the GF bulls might still be producing ROS without compromising sperm freezability.
The ATP is produced by oxidative phosphorylation catalyzed by the respiratory chain in the mitochondria which generate ROS, adequate levels of which are required for sperm hyperactivation, capacitation, acrosome reaction, and for the fusion of spermatozoon and oocyte (47, 48). On the other hand, excessive production of ROS can be detrimental for proteins and membrane lipids (49). In our study, we found that mitochondrial ROS generation was not associated with PTV status in sperm from the bulls with different sperm freezability phenotypes. Because there was no significant difference among both groups in mitochondrial oxidative stress (Table 1), we inferred the machinery was able to maintain a balance between generation and removal of ROS by mitochondrial enzymatic antioxidant in the mitochondrial matrix. This may lead to a small amount of H2O2 released into the extracellular (50) which was consistent with our result. This was supported by Armstrong et al. (51) and De Lamirande and Gagnon (52) that action of H2O2 in human sperm is not dependent on mitochondrial oxidative phosphorylation. In our study, the probe used reacts with superoxide in viable cells of mitochondria which actively respires. Our study may explain that optimal intercellular ROS production by mitochondria could be maintained while sperm confront cryopreservation. Due to the fact mitochondrial ROS is produced through metabolic substrates and the extracellular environment including mitochondrial membrane potential (53, 54), rather than through cryodamage. Disruption of mitochondria is likely to lead to an increased production of cellular ROS in sperm causing an increase in superoxide anions in frozen-thawed sperm as compared to fresh sperm (50, 55). In our study, live negative and positive, and dead superoxide anion negative sperm cells were not correlated with PTV, while dead superoxide anion positive (SO+) cells correlated with PTV (Figure 2) as well as with higher levels in GF than PF bulls (Table 1). Interestingly, it was demonstrated that increased concentrations of sperm contribute to higher superoxide anion generation (56). Likewise, in fresh sperm, a reduction in sperm concentration was found to alleviate oxidative stress in bulls (57). This is likely because freezability may also be affected by some other factors of cryopreservation, such as seasonal variations, cooling–thawing rates, sperm source, and type of extender or cryoprotectants.
While cryopreservation induces production of ROS by increasing superoxide anion, acrosome reaction is linked to superoxide anion production. The acrosome reaction is correlated with the generation of extracellular O2− and H2O2 and that low levels of ROS function as signaling transducers and are critical for the initiation of acrosome reaction (58, 59). On the other hand, excessive ROS harms the acrosome membrane, as an example, sperm acrosome integrity injuries occurred after cryopreservation that led to cryocapacitation thus premature acrosome reaction due to abnormalities in membrane fluidity (60, 61). Our findings that dead intact (DIAC) and dead reacted acrosome (DRAC) were negatively correlated with the freezabilitiy phenotype of PTV (Figure 2). Additionally, PF bulls had higher DIAC and DRAC than GF bulls (Table 2). Our study showed that proportions of live intact acrosome were closely related to freezability, however, levels of LRAC were similar between the GF and PF bulls (Table 2). This is consistent with reports that intact acrosome is an important factor for the quality of frozen sperm cells in bulls (62) as well as for the success of fertilizing capacity of cryopreserved bovine sperm (63). However, live reacted acrosome was not associated with PTV and was similar between the GF and PF bulls (Table 2). Acrosome reaction was claimed to be better suitable for evaluating frozen-thawed sperm before keeping males as breeding bulls (64) and in ram, acrosomal integrity was linked with PTV, therefore, results of our study elucidate acrosomal integrity as an integral part of freezability, and support findings that the physiological ROS are essential for acrosome reaction.
Impaired and deficient protamination is an indication of chromatin immaturity that can influence sperm function (65–67). Therefore, we employed CMA3 that competes with protamines for binding to the minor groove of DNA which indirectly shows the levels of protamine deficiency in the sperm chromatin. Sperm protamine content measured in human and bull indicates that sperm protamine deficiency can be linked to DNA damage (68–70). In addition, freeze-thaw may impair the bonding of disulfide bridges in protamine, thus lead to increased DNA damage (71), protamine deficiency did not always result in DNA damage though (72). On the other hand, it is also intriguing that protamine deficiency was found to be low in bull compared to human (73). This seems to be the likely reason that bull sperm are more resilient against the detriments of freezing-thawing protocol (74). In contrast, results of our study revealed that the percentage of protamine deficiency did not change in GF and PF sperm, but correlated with freezability status (Table 2) which is supported by the previous reports that protamine deficiency did not differ between fresh and freeze-thawed spermatozoa (75). Variations in protamine contents are detectable in sperm from different bulls sperm (76) and that sperm from different aged bulls had distinct protamine deficiency levels (74).
A comprehensive evaluation of the sperm cellular and functional physiopathology is fundamental to the male reproduction research to advance knowledgebase in cryobiology through elucidating and improving the freezability status. In addition to the physiological role of ROS, the structural integrity of sperm organelles such as the acrosome is more important for assessing proper sperm functions. The limitations of this study were that because of the lack of feasibility factors, we were unable to analyze the fresh samples before freezing, and that the sample size could be increased. However, through integrative approaches and analyses of cells with phenotypes, we demonstrated the cellular and functional dynamics of sperm stemming from cryodamage that can affect sperm physiology and reproductive health.
Materials and Methods
Semen Collection and Determination of Bull Semen Freezability
The sperm samples analyzed in this study were obtained from Alta Genetics Inc. (Watertown, WI); therefore, the experiments conducted in our laboratories did not involve live animals. Five bulls were assigned to a good freezability group (GF); whereas the other five were assigned to poor freezability group (PF) according to the post-thaw viability (PTV) data provided by Alta Genetics Inc. Post-thaw viability of sperm was assessed using a fluorescent stain combination of SYBR-14 with propidium iodide (SYBR-14/PI, Live/Dead Sperm Viability Kit L-7011, Thermo Fisher Scientific) as described previously (Figure 3) (16, 77). Collectively, a unique freezability phenotype was generated to characterize variations among bulls for their lifetime PTV of sperm. For this research, we used post-thaw viability data generated over 8 years between 2008 and 2016. The database included 100,448 ejaculates from 860 Holstein bulls that were collected at least 20 different times in ~3 months. The average and standard deviations of PTV for the individual bull were calculated, and bulls were ranked based on average PTV, which represents the freezability score. The average post-thaw viabilities of all bulls of 10 bulls ranged from 48.93 (n = 5) to 66.19% (n = 5) (population average 57.9 ± 6.5%) (P = 0.0001) (Figure 4). The bulls were then arbitrarily classified as GF and PF based on average post-thaw viability score and the difference from the population average (Table 3). The bulls were housed in the same nutrition and management environments to prevent sample variations. Semen from 10 bulls (GF, n = 5; PF, n = 5) was collected using an artificial vagina and processed immediately. Bull semen was preserved in a commercial Egg-Yolk-Tris based extender and frozen at Alta Genetics' laboratories using standard protocols (78). Cryopreserved sperm samples were shipped to Mississippi State University (MSU) in a liquid nitrogen containing tank.
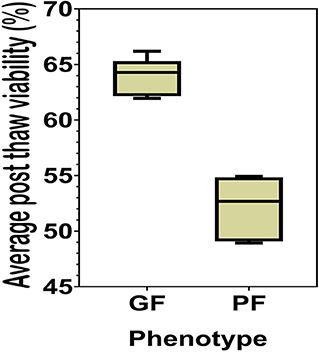
Figure 3. Staining of sperm cells with SYBR-14 and PI indicating viability. Representative image of sperm stained with SYBR-14 and PI indicating viability viewed with fluorescence microscope (20×) is provided. Arrows indicate the viable cells stained green with SYBR-14; asterisks indicate dead cells stained red with PI; and the moribund sperm stained with both colors.
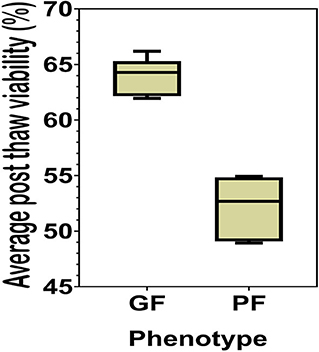
Figure 4. Percent averages of GF and PF phenotype bulls. The average post-thaw viability in bulls from the categories of the Good freezability phenotype and the Poor freezability phenotype. Bulls were arbitrarily selected and placed into one of the two categories based on the percent average of post-thaw viability from the population average (P < 0.0001).
Thiobarbituric Acid Reactive Substances (TBARS) Assay
For thiobarbituric acid reactive substances (TBARS) assay, sperm were thawed at 37°C for 30 s and then the extender was removed by centrifugation at 800 × g for 10 min. Sperm were washed twice with PBS and diluted to a concentration of 5 × 106 cells in 100 μL of PBS solution. Thiobarbituric acid reactive substances assay was employed to evaluate lipid peroxidation by measuring malondialdehyde (MDA) produced from the oxidation of polyunsaturated fatty acids in the sperm according to the previously described method (79). Briefly, 100 μL of sperm were mixed with 300 μL stock solution (TCA-TBA-HCl, 15% trichloroacetic acid, 0.375% thiobarbituric acid, and 0.25 N HCl) and the mixture was heated in 90°C water bath for 30 min, with gentle rocking once every 10 min, followed by quick cooling on ice-water for 5 min. The mixture was then centrifuged at 10,000 × g in 4°C for 10 min to pellet the precipitated proteins. The clear supernatant (200 μL) was collected and transferred into a 96-well plate (Santacruz, CA, USA, #sc-204463), together with an MDA calibration curve. The absorbance was measured at 532 nm in a microplate reader (FlexStation® 3 Benchtop Multi-Mode Microplate Reader, Orleans Drive, Sunnyvale, CA, USA). The TBARS value was expressed as μM of MDA.
Ferric-Reducing Antioxidant Power Assay (FRAP)
Cryopreserved sperm in straws were thawed the same way as above and centrifuged at 500 × g for 6 min to remove the extender. The pellet was washed twice with PBS and suspended in modified Tyrode Hepes-buffered medium (sp-TALPH) at a concentration of 5 × 106 cells in 200 μL. Antioxidants in sperm were extracted by sonication (Fisher Scientific, CPX962217R, PA, USA). The total antioxidant capacity (TAC) was assessed by the method of the ferric reducing antioxidant power (FRAP) (80). The FRAP solution [300 mM acetate buffer (pH 3.6), 10 mM 2,4,6-tripyridyl-S-triazine (TPTZ) in 40 mM HCl, and 20 mM FeCl3.6H2O in the ratio of 10:1:1] was mixed with 200 μL sperm cell extract and vortexed. The absorbance was measured at 593 nm against a reagent blank and absorbance of a standard solution (FeSO4.7H2O). The FRAP value was expressed as μM of FeSO4.7H2O.
Assessment of Reactive Oxygen Species (ROS)
Sperm pellet was washed twice in 1 mL of PBS at 800 × g for 10 min. Also, 2 × 106 sperm cells were suspended in 300 μL of PBS for the flow cytometry assays, including mitochondrial ROS, acrosome reaction, and protamine deficiency assay. Sperm ROS were measured by following a previously reported protocol (81), with some modifications. Briefly, 10 μL of 2 mM 2′,7′-dichlorodihydrofluorescein diacetate (H2DCFDA, Invitrogen, D399), 10 μL of 40 μM dihydroethidium (DHE; Invitrogen, D11347), and 10 μL of 40 μM Hoechst 33258 (Invitrogen, H3569) were added to the resuspended sperm cells (2 × 106 sperm/mL) and incubated at 37°C in the water bath in the dark for 25 min. Following the incubation, the excitation of Hoechst 33258 was performed with a laser-excited fluorescence channel (VL1) at 405 nm and detected using a 445/45 nm pass filter. The excitation of DHE was performed with laser-excited fluorescence channel (YL2) at 561 nm and detected using a 615 nm pass filter whereas excitation of H2DCFDA was performed with laser-excited fluorescence channel (BL1) at 488 nm and detected using a 530 nm filter. A total of 10,000 events was evaluated for each bull sample and cell populations were gated as percentages of live-dead H2O2+, and live-dead superoxide anion positive and negative (SO+, SO–). Sperm stained with H2DCFDA and Hoechst indicating ROS and live cells are depicted in Figure 5. Flow cytometry assays were performed using ACEA NovoCyte® 3000 flow cytometer and NovoExpress software (ACEA) was used for data acquisition and analyses.
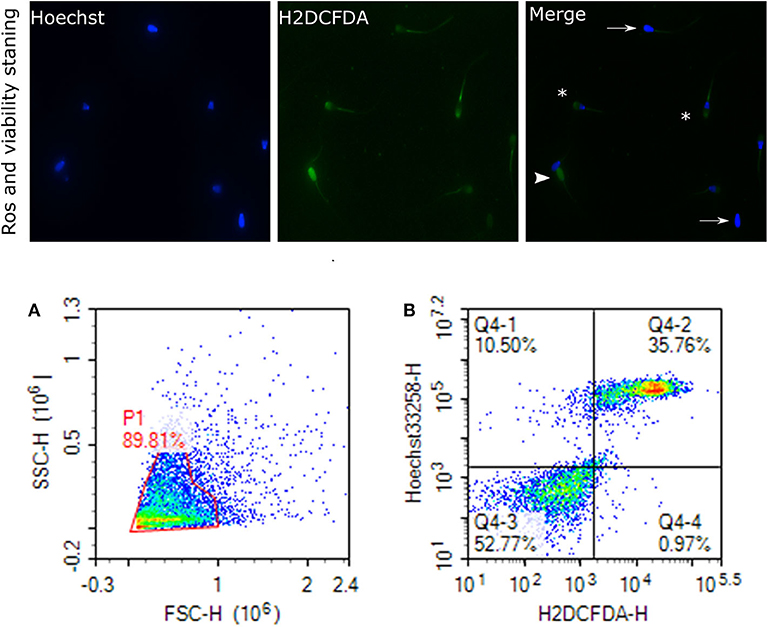
Figure 5. Reactive oxygen species (H2O2) and viability. Representative images of sperm stained with H2DCFDA and Hoechst indicating ROS and live cells, viewed with fluorescence microscope (60×) are depicted. Arrows indicate the live cells stained with uniform blue Hoechst; asterisks indicate the ROS stained and live cells; arrowhead show the dead and ROS stained cells. This figure is presented as two categories because two ROS probes were used. (A) gated sperm cell population. (B) Q1 viable cell H2O2 negative cells, Q2 viable H2O2 positive, Q3 nonviable H2O2 negative, and Q4 nonviable H2O2 positive.
Assessment of Mitochondrial ROS
Mitochondrial ROS (mROS) levels in sperm cells were determined using a modified method of the fluorescent probe of Mito-SOX™ Red (Molecular Probes, Invitrogen, cat. no. M36008) (82). Briefly, cryopreserved sperm in straws were thawed at 37°C for 30 s and, a total of 2 ×106 cells was used for each analysis. Sperm were incubated in 3 mM of MitoSOX™ Red and 0.05 μM of SYTOX® Green (Molecular Probes, Invitrogen, cat. no. S34860) at 37°C in the dark for 15 min. Following the incubation, samples were then washed using PBS and analyzed using flow cytometer; the excitation of Mito-SOX™ Red was performed with laser-excited fluorescence channel (YL2) at 561 nm and detected using a 620 nm pass filter whereas excitation of SYTOX was performed with laser-excited fluorescence channel (BL1) at 488 nm and detected using a 530 nm filter. A total of 10,000 events was counted after gating as percentages of live MitoSOX– and MitoSOX+. Sperm stained with MitoSOX red for mitochondrial superoxide generation, and SYTOX (green) for dead cells are depicted in Figure 6.
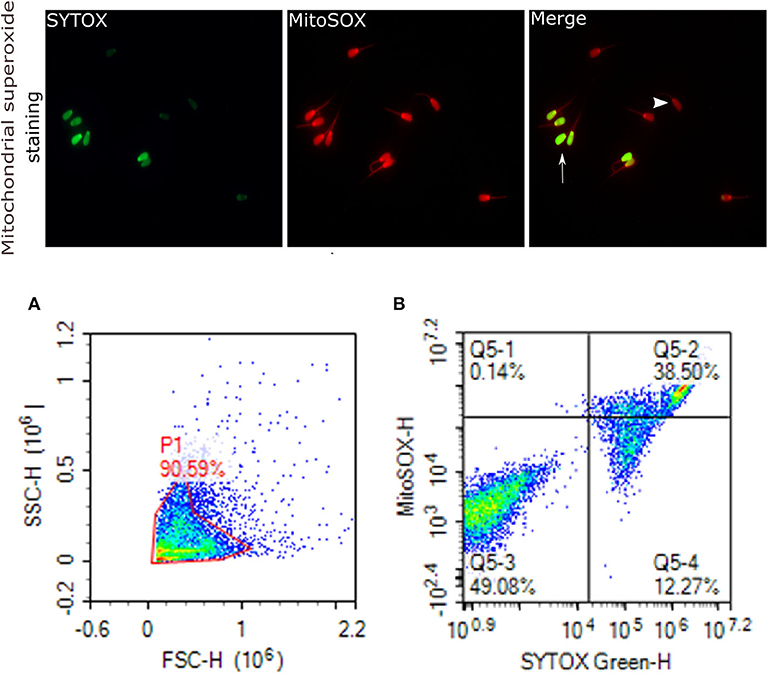
Figure 6. Mitochondrial superoxide generation. Representative images that were viewed with fluorescence microscope (60×) are provided for sperm stained with MitoSOX red for sperm positive mitochondrial superoxide generation, and SYTOX (green) for dead cells. Arrows indicate dead superoxide positive sperm; arrowhead shows live superoxide positive. (A) gated sperm cell population. (B) Q1 viable MitoSOX+, Q2 dead MitoSOX+, Q3 viable MitoSOX–, Q4 dead MitoSOX–.
Acrosome Integrity Assay
Status of the acrosome reaction was evaluated using fluorescein isothiocyanate-peanut agglutinin (FITC-PNA; Sigma- Aldrich, St. Louis, MO) according to the methods described by (83), with modifications. Briefly, 10 μL of FITC-PNA and 1.2 μL of PI (2.4 mM) were mixed with 300 μL of 2 ×106 cells and then incubated at 37°C in a water bath in the dark for 20 min. The excitation of FITC-PNA was performed with laser-excited fluorescence channel (BL1) at 488 nm and detected using a 530 nm filter, and PI was excited at (BL3) at 488 nm and detected using a 620 nm filter. A total of 10,000 events using a flow cytometer was evaluated for each sample. The cell populations were distinguished as live reacted acrosome (LRAC), live intact acrosome (LIAC), dead reacted acrosome (DRAC), and dead intact acrosome (DIAC). Sperm stained with FITC-PNA and PI indicating acrosome and dead cells were viewed using fluorescence microscopy (Figure 7).
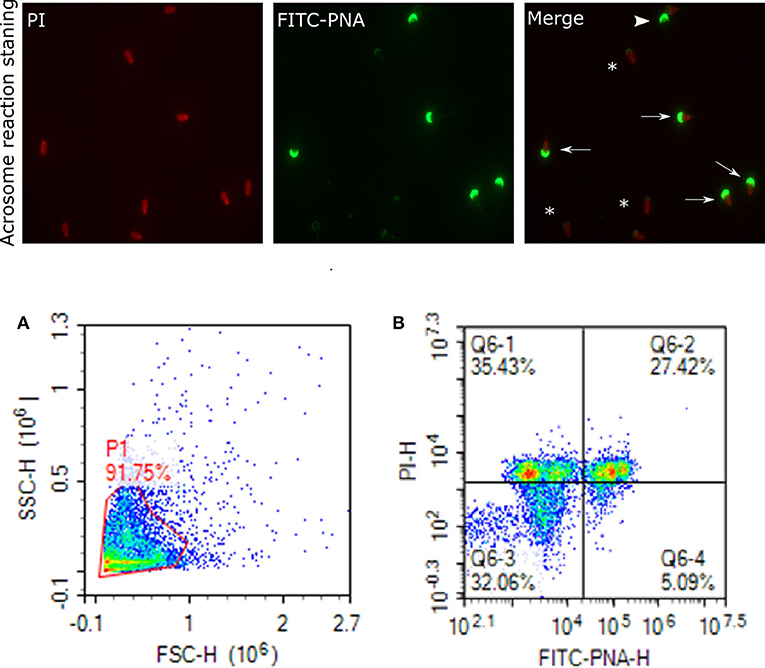
Figure 7. Acrosome staining of sperm cells. Representative images of sperm stained with FITC-PNA and PI indicating acrosome and dead cells, viewed with fluorescence microscope (60×). Arrows indicate dead and intact acrosome cells with uniform green FITC-PNA fluorescence of acrosome site; asteriks indicate acrosome-reacted cells with fluorescence along its outline; arrowhead shows viable cells stained with uniform green FITC-PNA fluorescence of acrosome. (A) gated sperm cell population. (B) Q1 dead intact acrosome (DIAC), Q2 dead reacted acrosome (DRAC), Q3 live intact acrosome (LIAC), live reacted acrosome (LRAC).
Protamine Deficiency (PRM) Assay
Protamine deficiency assay was performed to assess the extent of replacement of histones by protamines using chromomycin A3 (CMA3) according to a previously reported assay (70). Sperm pellets containing 2 ×106 sperm cells were resuspended in TNE buffer (10 mM Tris, 1 mM EDTA, 100 mM NaCl) after thawing and centrifugation twice in PBS at 5,000 × g at room temperature for 15 min. The CMA3 solution (0.25 mg/mL) was prepared by dissolving CMA3 (Sigma-Aldrich) in McIlvaine's buffer (LabChem, Inc. LC163004); 200 μL of which were added to spermatozoa. The resuspended sperm was incubated at 37°C in a water bath in the dark for 20 min and washed twice in PBS by centrifugation (500 × g at room temperature for 10 min). Sperm cells were gated using FSC and SSC and identified by PI-positive signal excited using the fluorescence channel (BL3) at 488 nm. The signals were detected using a 620 nm filter whereas CMA3 was excited using a laser-excited fluorescence channel (VL2) at 405 nm and detected with a 530 nm pass filter. For each bull sample, a minimum 10,000 of events was assessed. The percentage of CMA3 positivity was expressed as median fluorescence intensity (MFI) read from the histogram, and measured by the ratio between the MFI of each sample and MFI of negative cells.
Statistical Methods
Principal component analysis (PCA) of standardized data (mean-centered and divided by the standard deviation of each variable) was used to reduce sperm functional parameters and oxidative stress parameters to two principal components (PC) while maintaining total variance in data. The loadings, representing correlation coefficients of variables with principal component 1 (PC1; horizontal coordinate) and principal component 2 (PC2; vertical coordinate), were used to map these variables in a biplot. The PC scores or rankings were used to map bulls having different freezability phenotype on the same biplot. The CORR procedure of SAS for Windows v9.4 (SAS Institute, Inc., Cary, NC) was used to determine Pearson and Spearman's correlation coefficients (in case of non-parametric data) between post-thaw viability with sperm functional and oxidative stress parameters. The t-test procedure of SAS 9.4 was used to conduct a two-sample t-test. For non-normally distributed outcomes, Wilcoxon rank-sum tests were performed using Proc NPAR1WAY. The actual probability values of statistical significance were reported.
Data Availability Statement
The raw data supporting the conclusions of this article will be made available by the authors, without undue reservation.
Author Contributions
MH, TD, EM, and AK: conception and design of the study. AK, ET, MH, DS, and TD: sample acquisition and processing. MH, MU, TD, DS, EM, AK, and WT: acquisition, analysis, and interpretation of data. MH, MU, TD, AK, and EM: drafting the article or revising it critically for important intellectual content. All authors contributed to the article and approved the submitted version.
Funding
This project was supported by Agriculture and Food Research Initiative Competitive Grant no. 2017-67016-26507 from the USDA National Institute of Food and Agriculture. Partial funding was provided by Mississippi Agricultural Forestry Experiment Station, and by URUS Group LP, Madison, Wisconsin, USA. MU was funded through a competitive graduate fellowship from the Turkish Ministry of National Education. MH was funded through a competitive international postdoctoral fellowship by the Scientific and Technological Research Council of Turkey (TUBITAK-1059B191800016). The Flow Facility (Core C) was supported by the NIH COBRE (#P20GM103646). The fluorescent microscopy facility was funded through the USDA-ARS Biophotonics Initiative #58-6402-3-018.
Conflict of Interest
ET employed by the company URUS Group LP.
The remaining authors declare that the research was conducted in the absence of any commercial or financial relationships that could be construed as a potential conflict of interest.
References
1. Vishwanath R. Artificial insemination: the state of the art. Theriogenology. (2003) 59:571–84. doi: 10.1016/S0093-691X(02)01241-4
2. Cazaux Velho AL, Menezes E, Dinh T, Kaya A, Topper E, Moura AA, et al. Metabolomic markers of fertility in bull seminal plasma. PLoS ONE. (2018) 13:e0195279. doi: 10.1371/journal.pone.0195279
3. Sharma V. Sperm storage for cancer patients in the UK: a review of current practice. Hum Reprod. (2011) 26:2935–43. doi: 10.1093/humrep/der281
4. Fuller BJ. Cryoprotectants: the essential antifreezes to protect life in the frozen state. Cryo Lett. (2004) 25:375–88. doi: 10.1201/9780203647073
5. Petrunkina AM, Waberski D, Günzel-Apel AR, Töpfer-Petersen E. Determinants of sperm quality and fertility in domestic species. Reproduction. (2007) 134:3–17. doi: 10.1530/REP-07-0046
6. Ugur MR, Saber Abdelrahman A, Evans HC, Gilmore AA, Hitit M, Arifiantini RI, et al. Advances in cryopreservation of bull sperm. Front Vet Sci. (2019) 6:268. doi: 10.3389/fvets.2019.00268
7. Nijs M, Creemers E, Cox A, Janssen M, Vanheusden E, Castro-Sanchez Y, et al. Influence of freeze-thawing on hyaluronic acid binding of human spermatozoa. Reprod Biomed Online. (2009) 19:202–6. doi: 10.1016/S1472-6483(10)60073-9
8. Vishwanath R, Shannon P. Storage of bovine semen in liquid and frozen state. Anim Reprod Sci. (2000) 62:23–53. doi: 10.1016/S0378-4320(00)00153-6
9. Isachenko E, Isachenko V, Katkov II, Dessole S, Nawroth F. Vitrification of mammalian spermatozoa in the absence of cryoprotectants: from past practical difficulties to present success. Reprod Biomed Online. (2003) 6:191–200. doi: 10.1016/S1472-6483(10)61710-5
10. Grötter LG, Cattaneo L, Marini PE, Kjelland ME, Ferré LB. Recent advances in bovine sperm cryopreservation techniques with a focus on sperm post-thaw quality optimization. Reprod Domest Anim. (2019) 54:655–65. doi: 10.1111/rda.13409
11. Salamon S, Maxwell WMC. Storage of ram semen. Anim Reprod Sci. (2000) 62:77–111. doi: 10.1016/S0378-4320(00)00155-X
12. Yoshida M. Conservation of sperms: current status and new trends. Anim Reprod Sci. (2000) 60:349–55. doi: 10.1016/S0378-4320(00)00125-1
13. Ezzati M, Shanehbandi D, Hamdi K, Rahbar S, Pashaiasl M. Influence of cryopreservation on structure and function of mammalian spermatozoa: an overview. Cell Tissue Bank. (2020) 21:1–5. doi: 10.1007/s10561-019-09797-0
14. Mazur P. Cryobiology: the freezing of biological systems. Science. (1970) 168:939–49. doi: 10.1126/science.168.3934.939
15. Chen Y, Foote RH, Brockett CC. Effect of sucrose, trehalose, hypotaurine, taurine, and blood serum on survival of frozen bull sperm. Cryobiology. (1993) 30:423–31. doi: 10.1006/cryo.1993.1042
16. Nagy S, Jansen J, Topper EK, Gadella BM. A triple-stain flow cytometric method to assess plasma- and acrosome-membrane integrity of cryopreserved bovine sperm immediately after thawing in presence of egg-yolk particles. Biol Reprod. (2004) 68:1828–35. doi: 10.1095/biolreprod.102.011445
17. Watson PF. Recent Advances in Sperm Freezing. In vitro Fertilization and Donor Insemination. London: Royal College of Obstetricians and Gynaecologists (1985). p. 261–7.
18. Watson PF. Cooling of spermatozoa and fertilizing capacity. Reprod Domest Anim. (1995) 31:135–40. doi: 10.1111/j.1439-0531.1995.tb00016.x
19. Aitken RJ. Free radicals, lipid peroxidation and sperm function. Reprod Fertil Dev. (1995) 7:659–68. doi: 10.1071/RD9950659
20. Lusignan MF, Li X, Herrero B, Delbes G, Chan PTK. Effects of different cryopreservation methods on DNA integrity and sperm chromatin quality in men. Andrology. (2018) 6:829–35. doi: 10.1111/andr.12529
21. Tremellen K. Oxidative stress and male infertility—a clinical perspective. Hum Reprod Update. (2008) 14:243–58. doi: 10.1093/humupd/dmn004
22. Agarwal A, Selvam MKP, Baskaran S, Cho CL. Sperm DNA damage and its impact on male reproductive health: a critical review for clinicians, reproductive professionals and researchers. Expert Rev Mol Diagn. (2019) 19:443–57. doi: 10.1080/14737159.2019.1614916
23. Jayasena CN, Alkaabi FM, Liebers CS, Handley T, Franks S, Dhillo WS. A systematic review of randomized controlled trials investigating the efficacy and safety of testosterone therapy for female sexual dysfunction in postmenopausal women. Clin Endocrinol. (2019) 90:391–414. doi: 10.1111/cen.13906
24. De Lamirande E, Gagnon C. A positive role for the superoxide anion in triggering hyperactivation and capacitation of human spermatozoa. Int J Androl. (1993) 16:21–5. doi: 10.1111/j.1365-2605.1993.tb01148.x
25. Aitken RJ. Reactive oxygen species as mediators of sperm capacitation and pathological damage. Mol Reprod Dev. (2017) 84:1039–52. doi: 10.1002/mrd.22871
26. Sabeti P, Pourmasumi S, Rahiminia T, Akyash F, Talebi AR. Etiologies of sperm oxidative stress. Int J Reprod Biomed. (2016) 14:231–40. doi: 10.29252/ijrm.14.4.231
27. Bansal AK, Bilaspuri GS. Impacts of oxidative stress and antioxidants on semen functions. Vet Med Int. (2011) 2011:7. doi: 10.4061/2011/686137
28. Hammerstedt RH. Maintenance of bioenergetic balance in sperm and prevention of lipid peroxidation: a review of the effect on design of storage preservation systems. Reprod Fertil Dev. (1993) 5:675–90. doi: 10.1071/RD9930675
29. Bilodeau JF, Chatterjee S, Sirard MA, Gagnon C. Levels of antioxidant defenses are decreased in bovine spermatozoa after a cycle of freezing and thawing. Mol Reprod Dev. (2000) 55:282–8. doi: 10.1002/(SICI)1098-2795(200003)55:3<282::AID-MRD6>3.0.CO;2-7
30. Ball BA. Oxidative stress, osmotic stress and apoptosis: impacts on sperm function and preservation in the horse. Anim Reprod Sci. (2008) 107:257–67. doi: 10.1016/j.anireprosci.2008.04.014
31. Sariözkan S, Bucak MN, Tuncer PB, Ulutaş PA, Bilgen A. The influence of cysteine and taurine on microscopic-oxidative stress parameters and fertilizing ability of bull semen following cryopreservation. Cryobiology. (2009) 58:134–8. doi: 10.1016/j.cryobiol.2008.11.006
32. Castro LS, Hamilton TRS, Mendes CM, Nichi M, Barnabe VH, Visintin JA, et al. Sperm cryodamage occurs after rapid freezing phase: flow cytometry approach and antioxidant enzymes activity at different stages of cryopreservation. J Anim Sci Biotechnol. (2016) 7:17. doi: 10.1186/s40104-016-0076-x
33. Gürler H, Malama E, Heppelmann M, Calisici O, Leiding C, Kastelic JP, et al. Effects of cryopreservation on sperm viability, synthesis of reactive oxygen species, and DNA damage of bovine sperm. Theriogenology. (2016) 86:562–71. doi: 10.1016/j.theriogenology.2016.02.007
34. Peris-Frau P, Soler AJ, Iniesta-Cuerda M, Martín-Maestro A, Sánchez-Ajofrín I, Medina-Chávez DA, et al. Sperm cryodamage in ruminants: understanding the molecular changes induced by the cryopreservation process to optimize sperm quality. Int J Mol Sci. (2020) 21:2781. doi: 10.3390/ijms21082781
35. Alvarez JG, Storey BT. Differential incorporation of fatty acids into and peroxidative loss of fatty acids from phospholipids of human spermatozoa. Mol Reprod Dev. (1995) 42:334–46. doi: 10.1002/mrd.1080420311
36. Wang Y, Sharma RK, Agarwal A. Effect of cryopreservation and sperm concentration on lipid peroxidation in human semen. Urology. (1997) 50:409–13. doi: 10.1016/S0090-4295(97)00219-7
37. Evangelista-Vargas S, Santiani A. Detection of intracellular reactive oxygen species (superoxide anion and hydrogen peroxide) and lipid peroxidation during cryopreservation of alpaca spermatozoa. Reprod Domest Anim. (2017) 52:819–24. doi: 10.1111/rda.12984
38. Ahmed S, Khan MI-ur-R, Ahmad M, Iqbal S. Effect of age on lipid peroxidation of fresh and frozen-thawed semen of Nili-Ravi buffalo bulls. Ital J Anim Sci. (2018) 17:730–5. doi: 10.1080/1828051X.2018.1424569
39. Kasimanickam R, Kasimanickam V, Thatcher CD, Nebel RL, Cassell BG. Relationships among lipid peroxidation, glutathione peroxidase, superoxide dismutase, sperm parameters, and competitive index in dairy bulls. Theriogenology. (2007) 67:1004–12. doi: 10.1016/j.theriogenology.2006.11.013
40. Gürler H, Calisici O, Bollwein H. Inter- and intra-individual variability of total antioxidant capacity of bovine seminal plasma and relationships with sperm quality before and after cryopreservation. Anim Reprod Sci. (2015) 155:99–105. doi: 10.1016/j.anireprosci.2015.02.006
41. Sariözkan S, Tuncer PB, Bucak MN, Ulutaş PA. Influence of various antioxidants on microscopic-oxidative stress indicators and fertilizing ability of frozen-thawed bull semen. Acta Vet Brno. (2009) 78:463–9. doi: 10.2754/avb200978030463
42. Mehdipour M, Daghigh Kia H, Najafi A, Vaseghi Dodaran H, García-Álvarez O. Effect of green tea (Camellia sinensis) extract and pre-freezing equilibration time on the post-thawing quality of ram semen cryopreserved in a soybean lecithin-based extender. Cryobiology. (2016) 73:297–303. doi: 10.1016/j.cryobiol.2016.10.008
43. Mann T. The biochemistry of semen and of the male reproductive tract. Biochem Semen Male Reprod Tract. (1964) 1:652–3. doi: 10.5694/j.1326-5377.1965.tb72030.x
44. Storey B. Biochemistry of the induction and prevention of lipoperoxidative damage in human spermatozoa. Mol Hum Reprod. (1997) 3:203–13. doi: 10.1093/molehr/3.3.203
45. Baumber J, Ball BA, Gravance CG, Medina V, Davies-Morel MCG. The effect of reactive oxygen species on equine sperm motility, viability, acrosomal integrity, mitochondrial membrane potential, and membrane lipid peroxidation. J Androl. (2000) 21:895–902. doi: 10.1002/j.1939-4640.2000.tb03420.x
46. McCarthy MJ, Baumber J, Kass PH, Meyers SA. Osmotic stress induces oxidative cell damage to rhesus macaque spermatozoa. Biol Reprod. (2009) 82:644–51. doi: 10.1095/biolreprod.109.080507
47. Griveau JE, Renard P, Le Lannou D. An in vitro promoting role for hydrogen peroxide in human sperm capacitation. Int J Androl. (1994) 17:300–7. doi: 10.1111/j.1365-2605.1994.tb01260.x
48. Agarwal A, Varghese AC, Sharma RK. Markers of oxidative stress and sperm chromatin integrity. Mol Endocrinol. (2009) 590:377–402. doi: 10.1007/978-1-60327-378-7_24
49. Mostek A, Westfalewicz B, Słowińska M, Dietrich MA, Judycka S, Ciereszko A. Differences in sperm protein abundance and carbonylation level in bull ejaculates of low and high quality. PLoS ONE. (2018) 13:e0206150. doi: 10.1371/journal.pone.0206150
50. Koppers AJ, De Iuliis GN, Finnie JM, McLaughlin EA, Aitken RJ. Significance of mitochondrial reactive oxygen species in the generation of oxidative stress in spermatozoa. J Clin Endocrinol Metab. (2008) 93:3199–207. doi: 10.1210/jc.2007-2616
51. Armstrong JS, Rajasekaran M, Chamulitrat W, Gatti P, Hellstrom WJ, Sikka SC. Characterization of reactive oxygen species induced effects on human spermatozoa movement and energy metabolism. Free Radic Biol Med. (1999) 26:869–80. doi: 10.1016/S0891-5849(98)00275-5
52. De Lamirande E, Gagnon C. Reactive oxygen species and human spermatozoa. II. Depletion of adenosine triphosphate plays an important role in the inhibition of sperm motility. J Androl. (1992) 13:379–86.
53. Starkov AA. The role of mitochondria in reactive oxygen species metabolism and signaling. Ann N Y Acad Sci. (2008) 1147:37–52. doi: 10.1196/annals.1427.015
54. Mailloux RJ, Harper ME. Mitochondrial proticity and ROS signaling: lessons from the uncoupling proteins. Trends Endocrinol Metab. (2012) 23:451–8. doi: 10.1016/j.tem.2012.04.004
55. Słowińska M, Liszewska E, Judycka S, Konopka M, Ciereszko A. Mitochondrial membrane potential and reactive oxygen species in liquid stored and cryopreserved Turkey (Meleagris gallopavo) spermatozoa. Poult Sci. (2018) 97:3709–17. doi: 10.3382/ps/pey209
56. de Lamirande E, O'Flaherty C. Sperm activation: role of reactive oxygen species and kinases. Biochim Biophys Acta. (2008) 1784:106–15. doi: 10.1016/j.bbapap.2007.08.024
57. Murphy C, Fahey AG, Shafat A, Fair S. Reducing sperm concentration is critical to limiting the oxidative stress challenge in liquid bull semen. J Dairy Sci. (2013) 96:4447–54. doi: 10.3168/jds.2012-6484
58. De Lamirande E, Tsai C, Harakat A, Gagnon C. Involvement of reactive oxygen species in human sperm arcosome reaction induced by A23187, lysophosphatidylcholine, and biological fluid ultrafiltrates. J Androl. (1998) 19:585–94.
59. O'Flaherty C, Beorlegui N, Beconi M. Role of superoxide anion and hydrogen peroxide in acrosome reaction of bovine spermatozoa. Andrology. (2001) 103–8.
60. Duru NK, Morshedi M, Schuffner A, Oehninger S. Cryopreservation-thawing of fractionated human spermatozoa and plasma membrane translocation of phosphatidylserine. Fertil Steril. (2001) 75:263–8. doi: 10.1016/S0015-0282(00)01694-0
61. Benchaib M, Lornage J, Mazoyer C, Lejeune H, Salle B, François Guerin J. Sperm deoxyribonucleic acid fragmentation as a prognostic indicator of assisted reproductive technology outcome. Fertil Steril. (2007) 87:93–100. doi: 10.1016/j.fertnstert.2006.05.057
62. Morrell JM, Valeanu AS, Lundeheim N, Johannisson A. Sperm quality in frozen beef and dairy bull semen. Acta Vet Scand. (2018) 60:41. doi: 10.1186/s13028-018-0396-2
63. O'Flaherty C, Beorlegui N, Beconi MT. Participation of superoxide anion in the capacitation of cryopreserved bovine sperm. Int J Androl. (2003) 26:109–14. doi: 10.1046/j.1365-2605.2003.00404.x
64. Zodinsanga V, Mavi PS, Cheema RS, Kumar A, Gandotra VK. Relationship between routine analysis/sperm function and fertility tests of cattle bull semen. Asian J Anim. (2015) 9:37–44. doi: 10.3923/ajas.2015.37.44
65. Steven Ward W, Coffey DS. DNA packaging and organization in mammalian spermatozoa: comparison with somatic cell. Biol Reprod. (1991) 44:569–74. doi: 10.1095/biolreprod44.4.569
66. Bianchi PG, Manicardi GC, Bizzaro D, Bianchi U, Sakkas D. Effect of deoxyribonucleic acid protamination on fluorochrome staining and in situ nick-translation of murine and human mature spermatozoa. Biol Reprod. (1993) 49:1083–8. doi: 10.1095/biolreprod49.5.1083
67. Manicardi GC, Bianchi PG, Pantano S, Azzoni P, Bizzaro D, Bianchi U, et al. Presence of endogenous nicks in DNA of ejaculated human spermatozoa and its relationship to chromomycin A3 accessibility. Biol Reprod. (1995) 52:864–7. doi: 10.1095/biolreprod52.4.864
68. Tavalaee M, Kiani A, Arbabian M, Deemeh MR, Nasr Esfahani MH. Flow cytometry: a new approach for indirect assessment of sperm protamine deficiency. Int J Fertil Steril. (2010) 3:177–184.
69. Fathi Z, Tavalaee M, Kiani A, Deemeh MR, Modaresi M, Nasr-Esfahani MH. Flow cytometry: a novel approach for indirect assessment of protamine deficiency by CMA3 staining, taking into account the presence of M540 or apoptotic bodies. Int J Fertil Steril. (2011) 5:128–33.
70. Fortes MRS, Satake N, Corbet DH, Corbet NJ, Burns BM, Moore SS, et al. Sperm protamine deficiency correlates with sperm DNA damage in Bos indicus bulls. Andrology. (2014) 2:370–8. doi: 10.1111/j.2047-2927.2014.00196.x
71. Flores E, Ramió-Lluch L, Bucci D, Fernández-Novell JM, Peña A, Rodríguez-Gil JE. Freezing-thawing induces alterations in histone H1-DNA binding and the breaking of protein-DNA disulfide bonds in boar sperm. Theriogenology. (2011) 76:1450–64. doi: 10.1016/j.theriogenology.2011.05.039
72. Banaszewska D, Andraszek K, Biesiada-Drzazga B. Evaluation of sperm chromatin structure in boar semen. Bull Vet Inst Pulawy. (2015) 59:271–7. doi: 10.1515/bvip-2015-0040
73. Carreira JT, Trevizan JT, Kipper BH, Perri SHV, Carvalho IR, Rodrigues LH, et al. Impaired protamination and sperm DNA damage in a Nellore bull with high percentages of morphological sperm defects in comparison to normospermic bulls. Arq Bras Med Vet e Zootec. (2015) 67:417–23. doi: 10.1590/1678-7046
74. Carreira JT, Trevizan JT, Carvalho IR, Kipper B, Rodrigues LH, Silva C, et al. Does sperm quality and DNA integrity differ in cryopreserved semen samples from young, adult, and aged Nellore bulls? Basic Clin Androl. (2017) 27:1–8. doi: 10.1186/s12610-017-0056-9
75. Nakata K, Yoshida K, Yamashita N. Preclinical evaluation of a new cryopreservation container for a limited number of human spermatozoa. J Reprod Dev. (2019) 65:297–304. doi: 10.1262/jrd.2017-086
76. Simões R, Feitosa WB, Mendes CM, Marques MG, Nicacio AC, De Barros FRO, et al. Use of chromomycin A3 staining in bovine sperm cells for detection of protamine deficiency. Biotech Histochem. (2009) 84:79–83. doi: 10.1080/10520290902843595
77. Garner DL, Johnson LA, Yue ST, Roth BL, Haugland RP. Dual DNA staining assessment of bovine sperm viability using SYBR-14 and propidium Iodide. J Androl. (1994) 15:620–9.
78. Pace MM, Sullivan JJ, Elliott FI, Graham EF, Coulter GH. Effects of thawing temperature, number of spermatozoa and spermatozoal quality on fertility of bovine spermatozoa packaged in. 5-ml French straws. J Anim Sci. (1981) 53:693–701. doi: 10.2527/jas1981.533693x
79. Nichi M, Goovaerts IGF, Cortada CNM, Barnabe VH, De Clercq JBP, Bols PEJ. Roles of lipid peroxidation and cytoplasmic droplets on in vitro fertilization capacity of sperm collected from bovine epididymides stored at 4 and 34°C. Theriogenology. (2007) 67:334–40. doi: 10.1016/j.theriogenology.2006.08.002
80. Benzie IFF, Strain JJ. Ferric reducing/antioxidant power assay: direct measure of total antioxidant activity of biological fluids and modified version for simultaneous measurement of total antioxidant power and ascorbic acid concentration. Methods Enzymol. (1999) 299:15–27. doi: 10.1016/S0076-6879(99)99005-5
81. Guthrie HD, Welch GR. Determination of intracellular reactive oxygen species and high mitochondrial membrane potential in Percoll-treated viable boar sperm using fluorescence-activated flow cytometry. J Anim Sci. (2006) 84:2089–100. doi: 10.2527/jas.2005-766
82. Kumaresan A, Johannisson A, Al-Essawe EM, Morrell JM. Sperm viability, reactive oxygen species, and DNA fragmentation index combined can discriminate between above- and below-average fertility bulls. J Dairy Sci. (2017) 100:5824–36. doi: 10.3168/jds.2016-12484
Keywords: sperm biology, bull, freezability, cellular, functional parameters
Citation: Hitit M, Ugur MR, Dinh TTN, Sajeev D, Kaya A, Topper E, Tan W and Memili E (2020) Cellular and Functional Physiopathology of Bull Sperm With Altered Sperm Freezability. Front. Vet. Sci. 7:581137. doi: 10.3389/fvets.2020.581137
Received: 07 July 2020; Accepted: 17 September 2020;
Published: 23 October 2020.
Edited by:
Arumugam Kumaresan, National Dairy Research Institute (ICAR), IndiaReviewed by:
N. Srivastava, National University of Singapore, SingaporeSellappan Selvaraju, National Institute of Animal Nutrition and Physiology (ICAR), India
Copyright © 2020 Hitit, Ugur, Dinh, Sajeev, Kaya, Topper, Tan and Memili. This is an open-access article distributed under the terms of the Creative Commons Attribution License (CC BY). The use, distribution or reproduction in other forums is permitted, provided the original author(s) and the copyright owner(s) are credited and that the original publication in this journal is cited, in accordance with accepted academic practice. No use, distribution or reproduction is permitted which does not comply with these terms.
*Correspondence: Erdogan Memili, ZW0xNDlAYWRzLm1zc3RhdGUuZWR1