- 1Department of Food Science and Human Nutrition, Iowa State University, Ames, IA, United States
- 2Interdepartmental Microbiology Graduate Program, Iowa State University, Ames, IA, United States
Commercial poultry farms frequently use live bacterial prophylactics like vaccines and probiotics to prevent bacterial infections. Due to the emergence of antibiotic-resistant bacteria in poultry animals, a closer examination into the health benefits and limitations of commercial, live prophylactics as an alternative to antibiotics is urgently needed. In this review, we summarize the peer-reviewed literature of several commercial live bacterial vaccines and probiotics. Per our estimation, there is a paucity of peer-reviewed published research regarding these products, making repeatability, product-comparison, and understanding biological mechanisms difficult. Furthermore, we briefly-outline significant issues such as probiotic-label accuracy, lack of commercially available live bacterial vaccines for major poultry-related bacteria such as Campylobacter and Clostridium perfringens, as well research gaps (i.e., probiotic-mediated vaccine adjuvancy, gut-brain-microbiota axis). Increased emphasis on these areas would open several avenues for research, ranging from improving protection against bacterial pathogens to using these prophylactics to modulate animal behavior.
Introduction
Poultry animals like layers and broilers are some of the most critical food animals, with 90 billion tons of chickens meat being produced globally per year (1) and 290 eggs consumed per capita in the United States (2). Over the years, poultry have been domesticated to maximize particular functions like meat and egg production. Although selecting for greater weight gain and egg-laying rates has improved poultry productivity, specific selection for bacterial diseases resistance has not been pursued as diligently. This is problematic, as poultry animals are becoming increasingly at-risk for bacterial infections given the push for cage-free rearing [reviewed by (3)] and serve as major reservoirs for foodborne pathogens like Salmonella and Campylobacter, contaminating their products [(4); reviewed in (5)]. Furthermore, the emergence of antimicrobial-resistant (AMR) bacterial pathogens threaten poultry animals and humans health alike (6). Specifically, avian pathogenic Escherichia coli (APEC), Pasteurella multocida, and Mycoplasma gallisepticum are causal agents of disease and mortality in poultry animals, which have the potential to harbor AMR genes [(7–9); reviewed in (10)]. Additionally, chickens are common carriers of bacteria like Salmonella and Campylobacter, which reside as commensals in their gastrointestinal tract [reviewed in (11, 12)]. However, these bacteria are frequent contaminators of poultry products and cause gastrointestinal disease in human consumers [reviewed in (13–15)]. Even worse, these microbes can horizontally-exchange AMR genes with commensals or other pathogens (16–18). This has created a dangerous situation in which bacterial pathogens (chicken and human alike) may become highly-difficult to treat with conventional antibiotics. Thus, cost-effective additives that can boost resistance to pathogenic and AMR bacteria are needed to further optimize both poultry health and productivity.
Among the strategies currently used to promote productivity in animal agriculture includes use of live microorganisms. This includes live bacterial vaccines, which are attenuated bacteria typically used to immunize animals against particular pathogens [reviewed in (19)], and probiotics, which are live, non-attenuated microbes that confer health benefits to the animal host (20). Probiotics are typically delivered via feed, although spray and intraocular administration are commonly-used to deliver live bacterial vaccines. Additionally, both live bacterial vaccines and probiotics can be cultured in vitro, which drastically lowers production costs [reviewed in (21); reviewed in (22)]. In this review, we will outline live bacterial vaccines and probiotics commercially-available in poultry, describing the peer-reviewed studies using these commercial products in poultry animals. Additionally, we discuss probiotic labels and reliability-concerns. Lastly, this review will discuss the potential for novel live vaccines, synergism between live prophylactics, and a possible role for live prophylactics in less-studied biological mechanisms such as behavior.
Live Bacterial Vaccines
General
The earliest recorded live bacterial vaccination was in 1884, where Spanish clinicians utilized a weak Vibrio cholerae isolate to combat cholera outbreaks (23). Techniques for purposefully-attenuating bacterial strains while maintaining immunogenicity have been improved, using targeted modifications at the genetic level (24). Concerns over virulence-reversion by live bacterial vaccines have driven researchers to develop antigen-based vaccines incapable of sustaining disease. However, the successful development of antigen vaccines with long-term efficacy is relatively rare, mainly due to evolutionary adaptations by pathogens (i.e., antigenic loss/drift, serotype diversity) and design, as antigens have much-fewer epitopes compared to live bacteria vaccines, limiting protection against multiple, antigenically-diverse strains of a certain pathogen (25). Thus, live bacterial vaccines provide a lucrative alternative that can circumvent many issues with vaccination in poultry.
Like their wild-type counterparts, live bacterial vaccines can be easily cultured in vitro with low input costs, providing an inexpensive means of manufacturing large quantities of vaccine vs. the protein extraction steps required for antigen-based vaccines (21). Therefore, these vaccines can simultaneously prevent disease caused by their wild-type parent bacterium as well as additional pathogens (bacterial, viral, etc.) because cross-reactivity or via genetic insertions of genes encoding foreign antigen (24), creating an avenue for broad protection unachievable by many prophylactics currently available. In this review, we summarize characteristics and peer-reviewed findings for commercial live Salmonella enterica, Escherichia coli, Mycoplasma gallisepticum, and Pasteurella multocida vaccines available for poultry in Table 1.
Commercial Live Bacterial Vaccines
Salmonella
Although Salmonella enterica induces inflammatory in the chicken gut at an early age (46–48), this bacterium can persist by restructuring the intestinal environment to promote immunological tolerance, allowing for asymptomatically-shedding via feces from poultry animals [reviewed in (11)], resulting in potential contamination of meat and egg products. Human consumption of these contaminated poultry products is one of the major routes of salmonellosis incidence in the United States (49, 50). Live Salmonella vaccines are typically delivered orally via spray or drinking water to reduce Salmonella load in poultry. To improve food safety, live Salmonella vaccines are augmented with genetic deficiencies to limit intestinal replication while maintaining high levels of immunogenicity (24), although serotype and genetic attenuations are important drivers of vaccine efficacy (51, 52). Furthermore, these vaccines can be readily-modified to carry exogenous antigens (53, 54), enabling protection against additional pathogens.
Megan® Vac-1 is a ΔcyaΔcrp S. Typhimurium vaccine [parent strain Δ3761 or UK-1 (55)], genetically-attenuated to knockout adenylate cyclase (Δcya) and cAMP receptor protein (Δcrp). These mutations reduce pathogenicity and persistence of this live vaccine in the intestine while maintaining high immunogenicity, as demonstrated by the decrease of a challenge Salmonella invasion and intestinal colonization in vaccinated layer pullets (26). However, the protection of this vaccine against Salmonella appears to be inconsistent. A previous study testing protection against a wild-type S. Typhimurium strain in broiler chicks found the Megan® Vac-1 only reduced challenge Salmonella load in one of the two challenge experiments, although the failure in the first experiment may have been related to in ovo antibiotic administration (27). Furthermore, the vaccine strain was frequently-recovered from internal organs and ceca of vaccinated birds (27), although sampled animals were only 1-week-old and thus are not representative of broilers at final slaughter. In support of this, Dórea and colleagues determined that Megan® Vac-1 significantly-reduced detection of Salmonella in commercial broiler carcasses, minimizing carcass condemnation (28).
Poulvac® ST (Zoetis) is another metabolically-attenuated S. Typhimurium strain with ΔserC (phosphoserine aminotransferase) and ΔaroA (3-phosphoshikimate 1-carboxyvinyltransferase) deletions. Despite these deletions, Poulvac® ST is still immunogenic, inducing anti-lipopolysaccharide IgA and IgY responses in intestinal washes at day 13 (29) despite a reduction in ileal macrophages and CD4+ T cells (30). Furthermore, vaccinated broilers had reduced S. Heidelberg loads in the ceca when challenged at 21 days old (31). This response may have been facilitated by recruitment of intestinal CD8+ T cells (30), which have been previously demonstrated to improve Salmonella clearance in chickens (56). However, this vaccine was unable to reduce Salmonella Heidelberg load in the ceca when challenged at 3 days (31). This may be due to serovar-specific, as Bailey and colleagues found that Poulvac® ST alone did reduce challenge Salmonella Typhimurium and Enteritidis invasion of internal organs and ceca colonization in 3 and 13-day-old chicks (29).
Unlike the previously-described Salmonella vaccines, Gallivac® SE (Merial Select) is a S. Enteritidis strain (ΔadeΔhis) developed via chemical mutagenesis. Similar to the other vaccines, Gallivac® SE can provide protection against non-Enteritidis serovars, as orally-delivered Gallivac® SE reduced S. Typhimurium burden in the liver and ceca up to week 71 in layer hens vs. unvaccinated hens (32). Although live Salmonella vaccines are normally given orally, intraocular administration of Gallivac® SE increased IFNΔ, IL-8, and iNOs production by splenic cells (33), suggesting that this vaccine is capable of inducing robust immune responses, which extend from mucosal barriers. Unfortunately, to the authors' knowledge, these are the only two peer-reviewed studies which investigated the immunological potential of Gallivac® SE in vivo.
Escherichia coli
One of the major drivers of mortality and carcass condemnation in poultry, APEC are a major problem in commercial production (57). In addition, APEC are characterized by the possession of large virulence plasmids that often carry numerous resistances to antibiotics and heavy metals [reviewed in (58–60)]. These plasmids can be horizontally-transferred to other gut commensals as well bacteria like Salmonella (61), making the reduction of APEC in poultry a major priority. Given their antigenic variability (62), vaccines with broad protection have proved problematic. Notably, APEC colonize the gastrointestinal tract as commensals (63, 64) and only cause colibacillosis when they translocate the lung epithelium upon fecal aerosolization (65, 66). Thus, orally-delivered live vaccines are a feasible strategy to reduce abundances of these microbes in the gut while also inducing systemic immunity for extraintestinal resistance.
As of this review, Poulvac® E. coli (Zoetis) is the only live E. coli vaccine for poultry on the market. Poulvac® E. coli has a O78 serotype and, similar to Poulvac® ST, is a ΔaroA mutant. When implemented in broilers, this vaccine increased the number of healthy carcasses and reduced collibacillosis of a O78 APEC field isolate compared to non-vaccinated controls (67). Similarly, Poulvac® E. coli decreased bacterial load of an O78 APEC in internal organs compared to non-vaccinated controls, possibly related to improvements in E.coli O78 antigen-specific IgY serum levels and splenocyte proliferation (34). However, this protection appears to be serotype-specific, as Poulvac® E. coli did not confer any protection against challenge with an O1 APEC serotype (35). Furthermore, route appears to be a major determinant of efficacy, as Poulvac® E. coli administered to broilers via drinking water did not confer any protection to an O78 APEC, whereas coarse spray-administration did (35).
Mycoplasma gallisepticum
The etiological agent of chronic respiratory disease and infectious sinusitis in poultry animals [reviewed in (68); reviewed in (69)], M. gallisepticum is a major cause of carcass condemnation, reductions in egg-laying efficiency and weight gain, and mortality in commercial poultry (70–73). Given its antigen variability, details regarding its entire pathogenesis are unclear (74). Initially, M. gallisepticum binds to sialic acid residues on lung epithelial cells (75) and can cause damage high inflammatory damage locally (76) or in deeper lymphoid tissues like the bursa of Fabricius (77). Although birds at all ages are susceptible to this bacterium, immunocompromised birds are especially at-risk for infection (78). Currently, live vaccines are typically used to prevent M. gallisepticum infection in poultry.
MG TS-11 (Merial Select) is a live attenuated strain of M. gallisepticum that is delivered via intraocular route (i.e., eyedrop). Its complete genome sequence is available to the public (79). This vaccine strain can prevent development of clinical airsacculitis, peribronchitis, and interstitial pneumonia via R-strain M. gallisepticum challenge without reducing egg-laying productivity (36, 37). More recently, research groups have sought to improve the efficacy of the TS-11 vaccine. Muneta and colleagues found that a recombinant TS-11 expressing IFNΔ increased cellular immunity via increased splenocyte-IFNΔ production and a non-edematous infiltration of heterophils into the trachea mucosa (36). Furthermore, TS-304, a TS-11 derivative that expresses the cytadherence molecule GapA, was shown to be more efficacious than TS-11 at a lower dose (80) likely related to its ability to more-effectively improve tracheal barrier function (76).
MYCOVAC-L® (Merck) is an attenuated 6/85 strain of M. gallisepticum. Similar to TS-11, its complete genome sequence is readily-available (38). Typically delivered via spray, rehydration of the vaccine via distilled water (standard practice for many bacterial vaccines) results in much lower MYCOVAC-L® viability vs. resuspension in PBS (38). In addition, although vaccination dose at the manufacturer's recommendation confers protective immunity against virulent M. gallisepticum, egg production may be negatively-impacted. However, hens previously vaccinated with fifteen times the recommended dose did not exhibit any deficiencies in egg-laying efficiency and produced more antibodies (39), suggesting a greater inoculum concentration is needed to negate certain side effects of MYCOVAC-L®.
Poulvac® MycoF (Zoetis) is an F strain of M. gallisepticum typically administered via spray. Using spray, Evans and colleagues showed MycoF-vaccinated animals did not exhibit M. gallisepticum-induced airsacculitis compared to control animals (40). Similar to MYCOVAC-L®, resuspension medium prior to MycoF immunization had a major impact on viability and antibody production immediately post-vaccination (81). When given via intraocular route, MycoF demonstrated protection against spread of M. gallisepticum in a co-mingled poultry system (82), suggesting that different vaccination routes may deliver similar success. However, when delivered in the same study via eyedrop, nares, or orally, intraocular MycoF vaccination induced the greatest antibody response (41), although this study did not investigate differences in M. gallisepticum resistance in vivo.
Another F strain vaccine, AviPro® MG-F (Elanco) was similarly able prevent airsacculitis via M. gallisepticum challenge (40). Although recommended delivery is in drinking water, Evans and colleagues found that when delivered via spray, MG-F delivered similar protection against M. gallisepticum infection as MycoF. Additionally, MG-F induced less antibody production vs. MycoF at one and ten-times recommended dose (42). However, MG-F induced superior immune responses compared to TS-11 and MYCOVAC-L® live vaccines (43, 44), suggesting that these M. gallisepticum vaccines induce immune responses in a vaccine strain-specific manner.
Pasteurella multocida
In the 1880s, Louis Pasteur developed one of the earliest live bacterial vaccines by isolating avian P. multocida, the etiological agent of fowl cholera, and using old cultures for immunization (83). Although a commensal member of the oropharyngeal microbiota, P. multocida can become an opportunistic pathogen in the respiratory tract (84). If able to bypass the lung epithelium, it can induce a highly-lethal septicemia (i.e., fowl cholera), causing major economic losses in poultry production (85, 86), though turkeys are more-affected (85). Thus, wing-web immunization of live P. multocida vaccines, superior to bacterin-based vaccines for this pathogen (87), is the most common method of prophylaxes against this pathogen. Although the exact mechanisms for protection are somewhat unclear, these live vaccines can induce broad protection independent of serotype and lipopolysaccharide composition (88). However, to the author's knowledge, very little peer-reviewed research has been performed using these Pasteurella live vaccines.
M-NINEVAX®-C (Merck) is an M-9 vaccine strain used in vaccinating commercial turkey flock against P. multocida and in combination with other live vaccines (89). Of the few studies using this vaccine, Sharaf and colleagues found this vaccine induced a potent antibody response against P. multocida (45). Similarly, PM-ONEVAX®-C, a PM-1 strain, induces protection against P. multocida challenge in vivo, accompanied with a high antibody titer (90). Unfortunately, no peer-reviewed studies on these live vaccines have been performed in the last two decades.
Probiotics
General
Probiotics are live microorganisms including bacteria (i.e., Lactobacillus acidophilus) and yeast (i.e., Saccharomyces cerevisiae) that are commonly supplemented in poultry feed to improve animal well-being through a variety of mechanisms. Probiotics have a variety of functions in host, which are mainly triggered by their outer membrane composition and metabolic outputs. In this section, we will discuss major classes of probiotics used in poultry and their general functions. Furthermore, we will summarize the findings of peer-reviewed studies using commercial probiotic products, organized by probiotics composed of a single class or mixture of classes (Table 2). This review will be limited to the effects of these commercial products on host immune function, productivity measures, and bacterial resistance (specifically, intestinal colonizers like Salmonella, Campylobacter, E. coli, and Clostridium perfringens). Given the limited focus on mechanisms with these commercial probiotics in poultry, this review will outline observed outcomes in-general in peer-reviewed studies.
Lactic Acid Bacteria
Lactobacillus, Enterococcus, and Pediococcus are gut commensals and examples of lactic acid (i.e., lactate in the ionized form)-producing bacteria (LAB), which protect against pathogens by several mechanisms. LAB are frequently used by poultry producers in part due their ability to produce several digestive enzymes (amylases, chitinases, lipases, phytases, and proteases), which greatly enhance the digestive process and improve feed conversion [reviewed in (120)]. Lactate is the major product of sugar metabolism across all LAB (121). Lactate can inhibit pathogenic bacterial growth by lowering the pH of the intestinal environment (122) or directly by disturbing normal bacterial metabolism (123). Select LAB also produce inhibitory compounds like bacteriocins, which are bactericidal compounds that target specific microorganisms (124). LAB can also directly stimulate immune cells via secretory factors (125) and toll-like receptor stimulation (125, 126). Given this wide array of functions, LAB are common components in several commercial probiotics used in poultry agriculture. Some examples of commercial LAB products for poultry animals are discussed below.
FloraMax®-B11 is a probiotic supplement composed of Lactobacillus salivarius and Pediococcus parvulus. Upon oral challenge with Salmonella Enteritidis, broilers fed FloraMax®-B11 showed reduced colonization of Salmonella Enteritidis, improved gut barrier function, and reduced percentages of heterophils, lymphocytes, eosinophils, and basophils of peripheral blood compared to control broilers (92). Given the role of immune inflammation in clearing intestinal Salmonella (127) and observed-reduction of circulatory immune cells, this suggests this product may have directly-reduced Salmonella load in the intestine. This mechanism of direct competition is supported in which each probiotic bacterium in FloraMax®-B11 directly-reduced Salmonella Enteritidis, E. coli, and Campylobacter jejuni growth in vitro (93). Although Prado-Rebolledo and colleagues did not investigate phenotypic changes in these immune cells, FloraMax®-B11 reduces intestinal gene expression associated with the NFκB complex and aldose reductase (91), suggesting this probiotic also reduces expression of inflammatory genes. In combination with the perinatal supplement EarlyBird (Pacific Vet Group USA Inc.), FloraMax®-B11 was shown to improve gut morphology and significantly decrease Salmonella recovery, incidence, and horizontal transmission to broiler chicks (94). Lastly, broilers supplemented with FloraMax®-B11 showed significant body weight gain, lower total Clostridium perfringens (the causal agent of necrotic enteritis), and lower necrotic enteritis-induced mortality when compared to control broilers after C. perfringens challenge (95).
Cylactin® is composed of a single LAB, Enterococcus faecium NCIMB 1045 (128). Implementation of Cylactin® to the diets of broilers has shown to have positive effects on average body weight, greatly-decreased counts of Clostridium spp. and E. coli in intestinal tract and excreta compared to controls, and had improved lactate production as well as short-chain and branched-chain fatty acids (96). However, Cylactin® alone did not reduce Salmonella Enteritidis load in the layer intestine (97). Although tested in a non-avian model, administration of Cylactin® in the diet of piglets showed significantly reduced mucus-adherent extraintestinal pathogenic strains of E. coli (129), suggesting that this probiotic could have direct effects on APEC found in the chicken intestine.
Bacillus
Similarly to LAB, Bacillus species secrete digestive enzymes that improve feed conversion and competitive exclusion, which limit the ability of pathogens to invade the host (130–132). However, B. subtilis specifically limits pathogen colonization by production and secretion of lipopeptides and other antimicrobial compounds, as 4–5% of a B. subtilis genome is devoted to the production of antimicrobials [reviewed in (133)]. In contrast to LAB, B. subtilis can form endospores (134), improving their survival in the harsh conditions of the intestinal tract and food preparation processes better than other probiotics (135, 136). B. subtilis has also been shown to alter the morphology of the intestinal tract via elevated villi height and increased villi height-to-crypt depths (137), increasing the surface area for nutrient absorption. Notably, the host immune response toward B. subtilis is driven based on whether it is in its metabolically-inactive (i.e., endospore) or active (i.e., vegetative) state, as T cell differentiation was driven toward inflammatory, intracellular TH1 responses and extracellular TH2 responses via sporous and vegetative B. subtilis, respectively (138). Thus, de-sporulation in the intestine is a critical factor that could have major consequences on the host immune response.
GalliPro® consists of a single strain, B. subtilis DSM 17229 which improved performance, and reduced ammonia emission from the excreta in broilers (98). GalliPro® has been shown to reverse loss of splenic mass in Salmonella-infected birds, although no immune parameters were changed when non-infected birds were fed this probiotic (67). Furthermore, GalliPro® increased the liberation of crude protein from the diet, consequently decreasing broiler feeding costs and increasing body weight and feed conversion ratios (100). However, this study did not show whether GalliPro® was directly involved in this liberation or indirectly through a shift in the microbiota. Addition of GalliPro® to feed reduced Salmonella in cecum samples and greatly reduced Salmonella-positive drag swabs when compared to control broilers (99). Lastly, GalliPro® facilitates complete elimination of C. perfringens colonization in the ileum of challenged birds (101).
CloSTAT® contains a single strain of B. subtilis, PB6. When included to the diet of C. perfringens-challenged broilers at 1 × 109 CFU CloSTAT®/g feed, these broilers had statistically increased body weight and feed intake counts compared to challenged broilers without probiotics (139). However, CloSTAT® supplementation did not significantly change bacterial load of lactobacilli nor C. perfringens in the ileal digesta (139).When investigating the mortality rates from E. coli challenge comparing broilers fed CloSTAT®, control, and antibiotic growth promoters, CloSTAT® showed reduction comparable to the antibiotic growth promoter (both significantly compared to control) (103). Similarly to GalliPro®, CloSTAT® also reduced C. perfringens colonization of the ileum upon challenge (102).
Norum™ is a direct-fed microbial culture that consists of two B. amyloliquefaciens strains (AM0938 and JD17) Addition of Norum™ has shown an increase in productivity parameters like body weight, body weight gain and feed conversion (105, 140). Norum™ greatly reduced the gut permeability and leakage of mucosal, immunological effectors like IgA into serum (105). In a necrotic enteritis model in which birds were challenged by Salmonella Typhimurium, Eimeria maxima, and Clostridium perfringens at days 1, 13, and 18–19 post-hatch, respectively, Norum™ significantly improved lesion scores (105). Lastly, in ovo administration of Norum™ to the feed greatly decreased the horizontal transmission of virulent E. coli and infection of broiler chickens during hatch, possibly through alterations of microbiota composition and community structure (108).
Bifidobacterium, Saccharomyces, and Multi-Species Probiotics
To the authors' knowledge, there are no commercial poultry probiotics solely-constituted of Bifidobacterium spp. However, Bifidobacterium spp. are widely used in combination with Lactobacillus probiotics (ex: PrimaLac®) and other combination products (ex: MicroGuard®). Bifidobacterium directly affects IgA secretion in the gut (141) as well as stimulates professional phagocytes and pancreatic elastase production via secretion of the serine protease inhibitor Serpin (142). This pro-inflammatory mechanism action suggests that Bifidobacterium Serpin-production is involved in the homeostasis of the gut microbiota. Additionally, Bifidobacterium spp. produces acetate and lactate, which are subsequently-used by microbial gut fermenters to produce butyrate and propionate (143). These two short-chain fatty acids (SCFAs) promote colonic regulatory T cell differentiation (144, 145) as well as increase bactericidal functions of intestinal macrophages (146). Furthermore, the high GC content of the Bifidobacterium genome interacts with TLR9 that is present on the surface of mammalian immune cells (141, 147), although it is not clear whether Bifidobacterium DNA has a similar effect on the avian analog TLR21 (148).
Although the scope of this review is live bacterial prophylactics, the eukaryotic Sacchormyces species S. cerevisiae and S. boulardii [although S. boulardii is arguably a sub-species of S. cerevisiae (149)] are widely-implemented in poultry probiotic mixtures (i.e., Gro-2-Max® and MicroGuard®, respectively) and thus will be briefly-mentioned. Despite these two species being highly-similar, S. boulardii has greater heat and acid tolerance vs. S. cerevisiae, making it more competitive in the gut microenvironment [reviewed in (150)]. Additionally, both Saccharomyces species increased SCFA production via shifts in the microbiome (151, 152). Furthermore, S. cerevisiae and S. boulardii can directly-eliminate pathogens via secretory antimicrobials (153, 154). However, only S. boulardii appears to possess membrane-associated inulin, which can agglutinate pathogens (155, 156).
Lavipan® consists of several LAB (Lactobacillus casei LOCK 0915, Lactobacillus lactis IBB 500, Carnobacterium divergens S-1, and Lactobacillus plantarum LOCK 0862, all at 1 × 109 CFU/g product) and Saccharomyces cerevisiae LOCK 0141 (1 × 107 CFU/g) and was shown to competitively exclude pathogenic bacteria such as Campylobacter spp. and Salmonella Enteritidis (109). This probiotic also improved villi morphometric parameters (i.e., villus width and surface area) of the duodenum, jejunum, and ileum compared to control group (110). Lavipan® supplementation also caused reduced Clostridium spp. and Escherichia coli when compared to the control broilers, which was increased with the addition of prebiotics (i.e., raffinose family oligosaccharides) (96), which are non-viable food components like that improve host health via direct modification of the commensal microbiota (157). Thus, adding prebiotics to commercial probiotic products may improve health outcomes in poultry animals.
PrimaLac® is composed of Lactobacillus acidophilus, Lactobacillus casei, Enterococcus faecium, and Bifidobacterium bifidium, all at 1 × 106 CFU/g (158). The use of PrimaLac has been shown to limit the colonization of Salmonella and E. coli (111) as well as C. jejuni (115). However, this probiotic does not induce any changes in ceca lactobacilli (113, 115). Supplementation of this probiotic to broilers in ovo produced an upregulation of iNOS, crucial for improving macrophage-killing of bacteria, in the ileum at day-of-hatch. However, later time points observed PrimaLac®-mediated downregulation of immune genes encoding toll-like receptors, cytokines, and iNOS in the ileum and ceca tonsil (116). The addition of PrimaLac® to the feed of turkey poults reduced Salmonella colonization upon challenge when compared to the control birds (111). When compared to an antibiotic growth promoter and control groups, addition of PrimaLac® increased reduction of C. perfringens, as well as improved broiler performance (114).
MicroGuard® contains 11 microorganisms (Bacillus licheniformis, B. megaterium, B. mesentricus, B. polymyxa, B. subtilis, Saccharomyces boulardii, Bifidobacterium bifidum, Lactobacillus acidophilus, L. bulgaricus, L. plantarum, and Streptococcus faecium) (159). The addition of MicroGuard® to the commercial broilers increased final bodyweight, weight gain, high density lipoprotein, triglyceride, and antibody titers against Newcastle disease and avian influenza levels (117). The addition of MicroGuard® also limited colonization of both Salmonella Enteritids and E. coli due to the above mentioned mechanisms, competitive exclusion, and possibly the production of bacteriocins (117).
Lastly, Gro-2-Max® is a multi-species probiotic product containing LAB (Lactobacillus acidophilus, Pediococcus pentosaceus, P. acidilactici), Bacillus subtilis, and Saccharomyces cerevisiae. When comparing route and length of treatment, Gro-2-Max® supplementation via food or water had general physiological impacts like reduced total triglycerides, low-density lipoprotein cholesterol, circulating lymphocytes, and viral vaccine-specific antibody titers. Additionally, ceca Enterobacteriaceae levels were inconsistently increased or decreased by Gro-2-Max®, regardless the route of inoculation (119). Our team has recently demonstrated changes in chicken intestinal Enterobacteriaceae levels via Gro-2-Max®, with layers only fed Gro-2-Max® exhibiting increased Enterobacteriaceae fecal shedding compared to the control birds (160). Furthermore, layers showed increased resistance to both APEC and Salmonella Kentucky when fed with both live Salmonella vaccine and Gro-2-Max® (118), suggesting this probiotic has adjuvant activities.
Future Directions for Live Prophylactics
Although much progress has been made in protecting poultry against bacterial disease, the movement of poultry animals to cage-free facilities has driven an increase in bacterial infections [reviewed by (3)], which pose risks to both animal and human health. Although the previously-described commercial vaccines and probiotics are used in practice, there are emerging technologies and strategies to improve food safety that warrant discussion. For the duration of this review, we will highlight issues in probiotic label-accuracy, novel yet non-commercial live vaccine strategies, and research gaps where the effects of probiotics and live vaccines are largely-understudied.
Probiotic Product Label Reliability
Although probiotics are widely-implemented in animal agriculture, label accuracy is a major concern that can drastically-influence product efficacy and health outcomes in poultry animals. More than 28% of the commercial cultures intended for human or animal use were misidentified at the genus or species level through rapid detection methods (161). Looking specifically at poultry probiotics, Redweik and colleagues use PCR to confirm the identification of all probiotic bacteria in Gro-2-Max® but detected Saccharomyces pastorianus (not S. cerevisiae as advertised) (160). Using four different methods to taxonomically-identify LAB present in FloraMax®-B11, Menconi and colleagues found that each method produced mixed results (93). Although 16S sequencing was the most accurate method used in this study, it is nearly impossible to speciate bacteria via 16S sequencing unless they are highly-characterized [reviewed in (162)], demonstrating its limited application. Using whole-genome shotgun sequencing is a far more accurate tool in addressing current labeling issues and false positive results for species not listed as components (163). Finally, another major concern is the accuracy of bacterial concentrations in these commercial products, as total viable cell counts often do not correspond with the concentrations given on the label (164). Altogether, it is imperative that researchers studying commercial probiotic activities in poultry verify label accuracy to improve repeatability.
Novel Live Vaccine Strategies
Although live vaccine technologies for Salmonella, APEC, Mycoplasma gallisepticum, and Pasteurella multocida are commercially-available for poultry animals, there is no commercial live vaccines for Campylobacter nor Clostridium available. Campylobacter, a major foodborne pathogen responsible for intestinal and extraintestinal disease in humans (165, 166), typically colonizes the chicken gut as a commensal (167). Despite several studies evaluating the use of whole-cell Campylobacter vaccines (168–171) and antigen-based vaccines (172–177), there is no vaccine commercially-available for Campylobacter reduction in the intestine. A major issue with orally-delivered, live Campylobacter vaccines may arise in distinguishing between vaccine and pathogenic strains during meat processing. To avoid this issue, one solution could be to use another vaccine strain that is genetically-modified to express conserved Campylobacter antigens. Several studies have explored the use of Lactococcus lactis (178), Salmonella (179), and E. coli (180) to carry these antigens for anti-Campylobacter immune development. However, a major limitation to using antigen-based strategies against Campylobacter is that they are highly, antigenically-variable between strains (181), making the identification of a conserved target difficult.
Although necrotic enteritis is a major cause of mortality and reduced productivity in young birds (182, 183), no vaccine is available against its causative agent Clostridium perfringens. Non-virulent C. perfringens can be used to promote intestinal immunity against pathogenic strains (184). Furthermore, Salmonella vaccines carrying recombinant C. perfringens antigens have been successful in potent protection against necrotic enteritis (185, 186). Thus, there is much potential for a live, oral vaccine that can protect against C. perfringens-induced necrotic enteritis, which might be further-improved through support with probiotics like CylactinΔ, GalliPro®, and CloSTAT® which, on their own, offer protection (96, 101, 102).
Research Gaps
Most studies evaluate probiotic and live vaccine-efficacy by comparing mono-treated animals vs. non-treated controls. While this experimental design is a crucial first-step in identifying the usefulness of a live prophylactic, this format is not representative of natural commercial conditions and it ignores the impact other vaccines, feed, etc. may have on the animal's response to that live prophylactic of-interest. This is of extreme-importance, as commercial farms routinely use a wide repertoire of prophylactics (live, inactivated, and subunit alike) on their poultry animals without knowing how they might improve or nullify each other's effects. Probiotics are widely-reported to serve as biological, vaccine adjuvants [reviewed in (187)]. However, the role of probiotics in vaccine-responsiveness is largely-understudied in poultry. As mentioned briefly, efficacy and weight gain of a live, recombinant Campylobacter vaccine was drastically-improved in broilers which were also given Anaerosporobacter mobilis as a probiotic (180). This improvement in vaccine response is even found for live vaccines outside the scope of this review. The protection against the eukaryotic pathogen Eimeria was highest when a live coccidiosis vaccine was combined with probiotics (188). Use of Gro-2-MaxΔ in combination with a live Salmonella vaccine improved resistance to both intestinal Salmonella Kentucky colonization and extraintestinal infection by an O78 APEC (118). This latter study suggests that probiotics can even exert their benefits outside of the intestine, potentially through activation of immune phagocytes via TLR-dependent pathways (126). Another commercial probiotic (Cylactin®) also may be a useful vaccine adjuvant, as combining this product with the live Salmonella vaccine Gallivac® SE increased Salmonella-specific IgA in layers (97). Thus, it is imperative that future studies look at the synergistic-effect other prophylactics may have on one another. Given the expensive nature of trying to fully-model the spread of prophylactics used in poultry agriculture, one could feasibly use commercially-available birds already given their respective prophylactics prior to experimental treatment.
Although parameters such as weight gain, food-conversion, egg laying efficiency, and bacterial resistance are commonly-used to study prophylactic-efficacy, there are many other mechanisms in which these live microbes could affect the host. Gut bacteria play a major role in the maturation of the enteric nervous system (189) and mediate animal behavior via the gut-brain-microbiota axis (190–192). These interactions are largely-driven by the ability of probiotic bacteria, Salmonella, and E. coli to directly synthesize and respond-to neurochemicals through a bidirectional communication network called microbial endocrinology (193–195). Animal models have demonstrated the ability for probiotics like Lactobacillus and Bifidobacterium (196, 197) as well as C. perfringens (198) to modulate behavior, although only the latter has been shown in chickens. Recently, a Δ3761-derived Salmonella vaccine and Gro-2-MaxΔ were shown to modulate gut catecholamine (but not serotonin) metabolism in layer pullets, depending if the live prophylactics were given individually or in combination (160). Altogether, these findings suggest that the prophylactics used may have a direct impact on animal behavior. Thus, a novel target for live prophylactics could be to manipulate poultry animals into exhibiting positive behaviors (feeding, dust-bathing) while mitigating negative social behaviors like pecking. However, a major consideration is whether effects of these live prophylactics on the gut-brain-microbiota axis are maintained by chickens with different gut microbiotas. Given the variability of the chicken gut microbiome due to factors like geographical location, litter, breed, and feed [reviewed by (199)], it is very possible that other commensal bacteria might nullify, reduce, or amplify the effects live prophylactics might have on animal behavior and neurochemical metabolism.
Conclusions
Commercial live bacterial vaccines and probiotics offer several advantages in improving poultry health against bacterial disease and colonization (summarized in Figure 1) However, a paucity of peer-reviewed research studies, inconsistencies with product labels, limited cross-protection against certain pathogens, and a vague understanding of synergistic effects when using multiple prophylactics have encumbered our ability to optimize poultry health. Additionally, it is crucial that future studies must investigate whether these live prophylactics may facilitate animal behavior changes via the gut-brain axis (Figure 1), providing a convenient means of improving social behaviors among poultry flock.
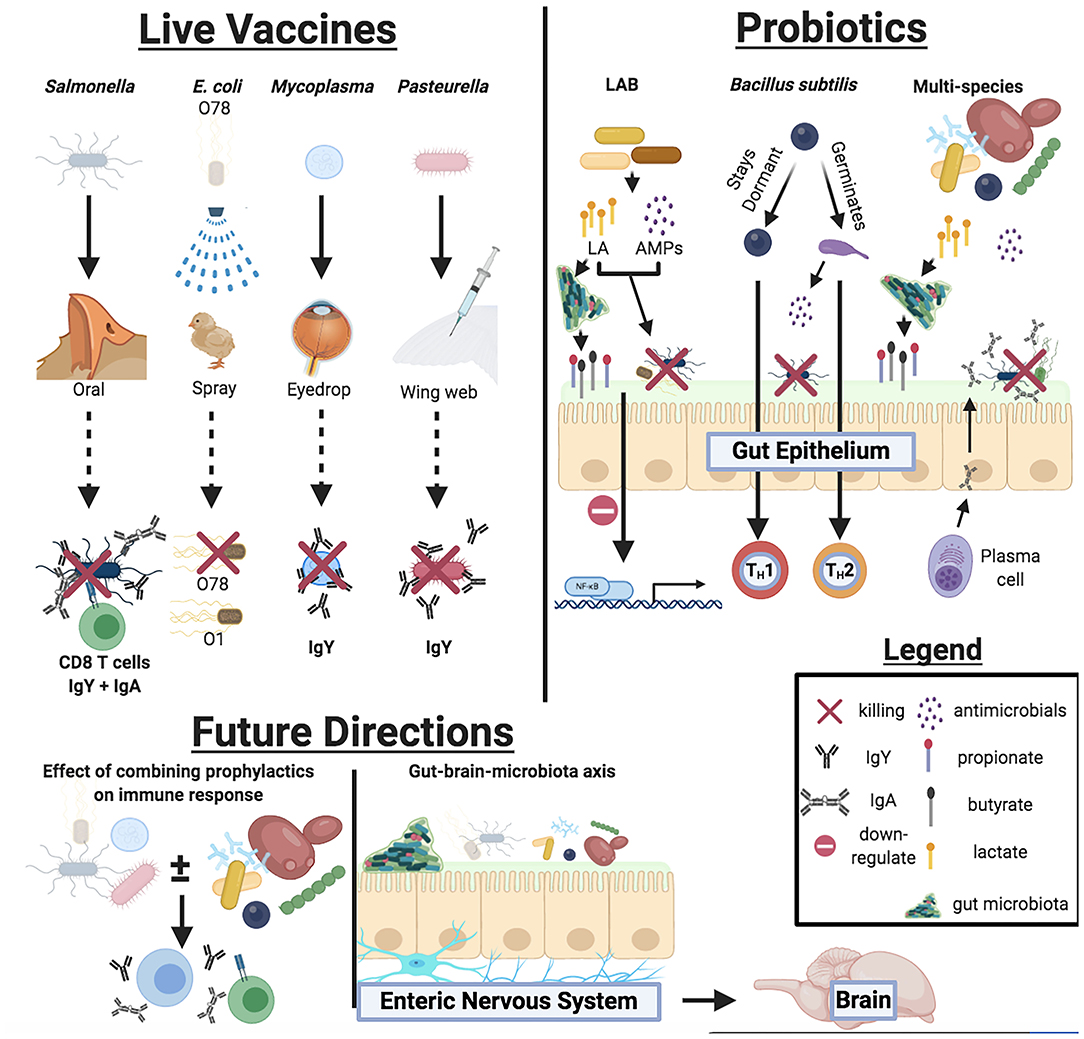
Figure 1. Overview of mechanisms live bacterial vaccines and probiotics participate in to improve host health, responses against bacterial pathogens, and future directions for live prophylactic research.
Author Contributions
GR and JJ wrote manuscript and developed figures and tables. MM revised manuscript. GR and MM provided funding and conceptualized review topic. All authors contributed to the article and approved the submitted version.
Funding
This review was supported by Iowa State University Start-up funding to MM and USDA-National Institute of Food and Agriculture (NIFA) project #021069-00001 to GR. The funders had no role in study design, data collection, and interpretation, or the decision to submit the work for publication.
Disclaimer
Mention of commercial products is for the sole purpose of providing specific information, not a recommendation or endorsement by the authors nor USDA.
Conflict of Interest
The authors declare that the research was conducted in the absence of any commercial or financial relationships that could be construed as a potential conflict of interest.
References
1. Food and Agriculture Organization of the United Nations. FAOSTAT: Live Animals Data. (2017). Available online from: http://www.fao.org/faostat/en
3. Lay DC, Fulton RM, Hester PY, Karcher DM, Kjaer JB, Mench JA, et al. Hen welfare in different housing systems. Poult Sci. (2011) 90:278–94. doi: 10.3382/ps.2010-00962
4. Uddin J, Hossain K, Hossain S, Saha K, Jubyda FT, Haque R, et al. Bacteriological assessments of foodborne pathogens in poultry meat at different super shops in Dhaka, Bangladesh. Ital J Food Saf. (2019) 8:6720. doi: 10.4081/ijfs.2019.6720
5. Heredia N, García S. Animals as sources of food-borne pathogens: a review. Anim Nutr. (2018) 4:250–5. doi: 10.1016/j.aninu.2018.04.006
6. Wegener HC. Antibiotic resistance- linking human and animal health. In: Institute of Medicine (US). Improving Food Safety Through a One Health Approach: Workshop Summary. Washington, DC: National Academies Press (2012). p. A15. Available online at: https://www.ncbi.nlm.nih.gov/books/NBK114485/
7. Tadesse DA, Zhao S, Tong E, Ayers S, Singh A, Bartholomew MJ, et al. Antimicrobial drug resistance in Escherichia coli from humans and food animals, United States, 1950-2002. Emerg Infect Dis. (2012) 18:741–9. doi: 10.3201/eid1805.111153
8. Simoneit C, Burow E, Tenhagen BA, Käsbohrer A. Oral administration of antimicrobials increase antimicrobial resistance in E. coli from chicken- a systematic review. Prev Vet Med. (2015) 118:1–7. doi: 10.1016/j.prevetmed.2014.11.010
9. Kheiri R, Akhtari L. Antimicrobial resistance and integron gene cassette arrays in commensal Escherichia coli from human and animal sources in IRI. Gut Pathog. (2016) 8:40. doi: 10.1186/s13099-016-0123-3
10. Nhung NT, Chansiripornchai N, Carrique-Mas JJ. Antimicrobial resistance in bacterial poultry pathogens: a review. Front Vet Sci. (2017) 4:126. doi: 10.3389/fvets.2017.00126
11. Wigley P. Salmonella enterica in the chicken: how it has helped our understanding of immunology in a non-biomedical model species. Front Immunol. (2014) 5:482. doi: 10.3389/fimmu.2014.00482
12. Wilson DJ, Gabriel E, Leatherbarrow AJ, Cheesbrough J, Gee S, Bolton E, et al. Tracing the source of campylobacteriosis. PLoS Genet. (2008) 4:e1000203. doi: 10.1371/journal.pgen.1000203
13. Antunes P, Mourão J, Campos J, Peixe L. Salmonellosis: the role of poultry meat. Clin Microbiol Infect. (2016) 22:110–21. doi: 10.1016/j.cmi.2015.12.004
14. Friedman CR, Neimann J, Wegener HC, Tauxe RV. Epidemiology of Campylobacter jejuni infections in the United States and other industrialized nations. In: Nachamkin I, Blaser MJ, editors. Campylobacter. 2nd ed. Washington, DC: American Society for Microbiology (2000). p. 121–138.
15. European food safety authority and european centre for disease prevention and control. The European union summary report on trends and sources of zoonoses, zoonotic agents and food-borne outbreaks in 2014. EFSA J. (2015) 13:4329–8. doi: 10.2903/j.efsa.2015.4329
16. Fricke WF, McDermott PF, Mammel MK, Zhao S, Johnson TJ, Rasko DA, et al. Antimicrobial resistance-conferring plasmids with similarity to virulence plasmids from avian pathogenic Escherichia coli strains in Salmonella enterica serovar kentucky isolates from poultry. Appl Environ Microbiol. (2009) 75:5963–71. doi: 10.1128/AEM.00786-09
17. Card RM, Cawthraw SA, Nunez-Garcia J, Ellis RJ, Kay G, Pallen MJ, et al. An in vitro chicken gut model demonstrates transfer of a multidrug resistance plasmid from Salmonella to commensal Escherichia coli. mBio. (2017) 8:00777–17. doi: 10.1128/mBio.00777-17
18. Avrain L, Vernozy-Rozand C, Kempf I. Evidence for natural horizontal transfer of tetO gene between Campylobacter jejuni strains in chickens. J Appl Microbiol. (2004) 97:134–40. doi: 10.1111/j.1365-2672.2004.02306.x
19. Rabie NS, Amin Girh ZMS. Bacterial vaccines in poultry. Bull Natl Res Cent. (2020) 44:15. doi: 10.1186/s42269-019-0260-1
20. Hill C, Guarner F, Reid G, Gibson GR, Merenstein DJ, Pot B, et al. Expert consensus document. The international scientific association for probiotics and prebiotics consensus statement on the scope and appropriate use of the term probiotic. Nat Rev Gastroenterol Hepatol. (2014) 11:506–14. doi: 10.1038/nrgastro.2014.66
21. Lin IY, Van TT, Smooker PM. Live-attenuated bacterial vectors: tools for vaccine and therapeutic agent delivery. Vaccines. (2015) 3:940–72. doi: 10.3390/vaccines3040940
22. Fenster K, Freeburg B, Hollard C, Wong C, Rønhave Laursen R, Ouwehand AC. The production and delivery of probiotics: a review of a practical approach. Microorganisms. (2019) 7:83. doi: 10.3390/microorganisms7030083
23. Detmer A, Glenting J. Live bacterial vaccines- a review and identification of potential hazards. Microb Cell Fact. (2006) 5:23. doi: 10.1186/1475-2859-5-23
24. Clark-Curtiss JE, Curtiss R. Vaccines: conduits for protective antigens. J Immunol. (2018) 200:39–48. doi: 10.4049/jimmunol.1600608
25. Kennedy DA, Read AF. Why does drug resistance readily evolve but vaccine resistance does not? Proc Biol Sci. (2017) 284:20162562. doi: 10.1098/rspb.2016.2562
26. Hassan JO, Curtiss R. Virulent Salmonella typhimurium-induced lymphocyte depletion and immunosuppression in chickens. Infect Immun. (1994) 62:2027–36. doi: 10.1128/IAI.62.5.2027-2036.1994
27. McReynolds JL, Moore RW, McElroy AP, Hargis BM, Caldwell DJ. Evaluation of a competitive exclusion culture and megan Vac 1 on Salmonella Typhimurium colonization in neonatal broiler chickens. J Appl Poult Res. (2007) 16:456–63. doi: 10.1093/japr/16.3.456
28. Dórea FC, Cole DJ, Hofacre C, Zamperini K, Mathis D, Doyle MP, et al. Effect of Salmonella vaccination of breeder chickens on contamination of broiler chicken carcasses in integrated poultry operations. Appl Environ Microbiol. (2010) 76:7820–5. doi: 10.1128/AEM.01320-10
29. Bailey JS, Rolon A, Hofacre CL, Holt PS, Wilson JL, Cosby DE, et al. Intestinal humoral immune response and resistance to Salmonella challenge of progeny from breeders vaccinated with killed antigen. Int J Poult Sci. (2007) 6:417–23. doi: 10.3923/ijps.2007.417.423
30. Hayashi RM, Tujimoto-Silva A, Muniz EC, Verdi R, Santin E. Salmonella Typhimurium vaccine to control a brazilian Salmonella heidelberg strain in broiler chickens. ARS Vet. (2018) 34:105–14. doi: 10.15361/2175-0106.2018v34n3p105-114
31. Muniz EC, Verdi R, Leão JA, Back A, Nascimento VPD. Evaluation of the effectiveness and safety of a genetically modified live vaccine in broilers challenged with Salmonella Heidelberg. Avian Pathol. (2017) 46:676–82. doi: 10.1080/03079457.2017.1348598
32. Springer S, Lindner T, Ahrens M, Woitow G, Prandini F, Selbitz HJ. Duration of immunity induced in chickens by an attenuated live Salmonella enteritidis vaccine and an inactivated Salmonella enteritidis/typhimurium vaccine. Berl Munch Tierarztl Wochenschr. (2011) 124:89–93.
33. Campagnari E, Rossi G, Franciosi C, Girelli D, Giovanardi D, et al. In vitro evaluation of live attenuated vaccines against Salmonella enteritidis: cell-mediated immune response. Italian J Anim Sci. (2007) 6:301–4. doi: 10.4081/ijas.2007.301
34. Sadeyen J, Wu Z, Davies H. Immune responses associated with homologous protection conferred by commercial vaccines for control of avian pathogenic Escherichia coli in turkeys. Vet Res. (2015) 46:5. doi: 10.1186/s13567-014-0132-5
35. Mohamed MA, Bakhit BM, Ibrahim AA, Saleh M. Evaluation of the living Escherichia coli-O78 deleted aroA vaccine against homologous and heterologous E. coli challenge in broiler chickens. J Adv Vet Res. (2016) 6:89–92.
36. Bíró J, Povazsán J, Korösi L, Glávits R, Hufnagel L, Stipkovits L. Safety and efficacy of Mycoplasma gallisepticum TS-11 vaccine for the protection of layer pullets against challenge with virulent M. gallisepticum R-strain. Avian Pathol. (2005) 34:341–7. doi: 10.1080/03079450500179913
37. Branton SL, Lott BD, May JD, Maslin WR, Pharr GT, Bearson SD, et al. The effects of ts-11 strain Mycoplasma gallisepticum vaccination in commercial layers on egg production and selected egg quality parameters. Avian Dis. (2000) 44:618–23.
38. Leigh SA, Evans JD, Branton SL, Collier SD. The effects of increasing sodium chloride concentration on Mycoplasma gallisepticum vaccine survival in solution. Avian Dis. (2008) 52:136–8. doi: 10.1637/7979-040507-ResNote
39. Evans JD, Leigh SA, Branton SL, Collier SD. Effects of increased dosages of the Mycoplasma gallisepticum vaccine MYCOVAC-L in layer chickens subsequently challenged with virulent M. gallisepticum: egg production and serologic response. Avian Dis. (2007) 51:912–7. doi: 10.1637/7931-020807-REGR2.1
40. Evans JD, Leigh SA, Purswell JL, Jacob R, Peebles ED, Collier SD, et al. A comparative study of live attenuated F strain-derived Mycoplasma gallisepticum vaccines. Avian Dis. (2012) 56:396–401. doi: 10.1637/9951-092711-Reg.1
41. Leigh SA, Evans ED, Collier SD, Branton SL. The impact of vaccination route on Mycoplasma gallisepticum vaccine efficacy. Poult Sci. (2018) 97:3072–5. doi: 10.3382/ps/pey188
42. Evans JD, Jacob R, Leigh SA, Collier SD, Peebles ED, Branton SL. Spray application of live attenuated F strain-derived Mycoplasma gallisepticum vaccines. J Appl Poult Res. (2013) 22:842–8. doi: 10.3382/japr.2013-00749
43. Peebles ED, Jacob R, Branton SL, Evans JD, Leigh SA, Gerard PD. Effects of different vaccine combinations against Mycoplasma gallisepticum on blood characteristics in commercial layer chickens. Poult Sci. (2015) 94:2108–13. doi: 10.3382/ps/pev225
44. Umar S, Munir MT, Rehman Z, Subhan S, Azam T, Shah MAA. Mycoplasmosis in poultry: update on diagnosis and preventive measures. Poult Sci J. (2017) 73:1–12. doi: 10.1017/S0043933916000830
45. Sharaf MM, Nestor KE, Saif YM, Sacco RE, Havenstein GB. Antibody response to Newcastle disease virus and Pasteurella multocida of two strains of turkeys. Poult Sci. (1988) 67:1372–7. doi: 10.3382/ps.0671372
46. Berndt A, Wilhelm A, Jugert C, Pieper J, Sachse K, et al. Chicken cecum immune response to Salmonella enterica serovars of different levels of invasiveness. Infect Immun. (2007) 75:5993–6007. doi: 10.1128/IAI.00695-07
47. Setta AM, Barrow PA, Kaiser P, Jones MA. Early immune dynamics following infection with Salmonella enterica serovars enteritidis, infantis, pullorum and gallinarum: cytokine and chemokine gene expression profile and cellular changes of chicken cecal tonsils. Comp Immunol Microbiol Infect Dis. (2012) 35:397–410. doi: 10.1016/j.cimid.2012.03.004
48. Varmuzova K, Matulova ME, Sebkova A, Sekelova Z, Havlickova H, et al. The early innate response of chickens to Salmonella enterica is dependent on the presence of o-antigen but not on serovar classification. PLoS ONE. (2014) 9:e96116. doi: 10.1371/journal.pone.0096116
49. Batz MB, Hoffmann S, Morris JG. Ranking the disease burden of 14 pathogens in food sources in the United States using attribution data from outbreak investigations and expert elicitation. J Food Prot. (2012) 75:1278–91. doi: 10.4315/0362-028X.JFP-11-418
50. Center for Disease Control and Prevention (2020). Salmonella Homepage. Avaialble online at: https://www.cdc.gov/salmonella/inde.html (accessed June 16, 2020).
51. Pei Y, Parreira V, Roland K, Curtiss R, Prescott J. Assessment of attenuated Salmonella vaccine strains in controlling experimental Salmonella Typhimurium infection in chickens. Can J Vet Res. (2014) 78:23–30.
52. Pei Y, Parreira V, Roland K, Curtiss R, Prescott J. Assessment of attenuated Salmonella vaccine strains in controlling experimental Salmonella Typhimurium infection in chickens. Can J Vet Res. (2014) 78:23–30.
53. Juárez-Rodríguez MD, Arteaga-Cortés LT, Kader R, Curtiss R 3rd, Clark-Curtiss JE. Live attenuated Salmonella vaccines against Mycobacterium tuberculosis with antigen delivery via the type III secretion system. Infect Immun. (2012) 80:798–814. doi: 10.1128/IAI.05525-11
54. Chaudhari AA, Matsuda K, Lee JH. Construction of an attenuated Salmonella delivery system harboring genes encoding various virulence factors of avian pathogenic Escherichia coli and its potential as a candidate vaccine for chicken colibacillosis. Avian Dis. (2013) 57:88–96. doi: 10.1637/10277-061312-Reg.1
55. Luo Y, Kong Q, Yang J, Mitra A, Golden G, Wanda SY, et al. Comparative genome analysis of the high pathogenicity Salmonella Typhimurium strain UK-1. PLoS ONE. (2012) 7:e40645. doi: 10.1371/journal.pone.0040645
56. Withanage GS, Wigley P, Kaiser P, Mastroeni P, Brooks H, Powers C, et al. Cytokine and chemokine responses associated with clearance of a primary Salmonella enterica serovar Typhimurium infection in the chicken and in protective immunity to rechallenge. Infect Immun. (2005) 73:5173–82. doi: 10.1128/IAI.73.8.5173-5182.2005
57. Mellata M. Human and avian extraintestinal pathogenic Escherichia coli: infections, zoonotic risks, and antibiotic resistance trends. Foodborne Pathog Dis. (2013) 10:916–32. doi: 10.1089/fpd.2013.1533
58. Rolain JM. Food and human gut as reservoirs of transferable antibiotic resistance encoding genes. Front Microbiol. (2013) 4:173. doi: 10.3389/fmicb.2013.00173
59. Johnson TJ, Siek KE, Johnson SJ, Nolan LK. DNA sequence and comparative genomics of pAPEC-O2-R, an avian pathogenic Escherichia coli transmissible R plasmid. Antimicrob Agents Chemother. (2005) 49:4681–8. doi: 10.1128/AAC.49.11.4681-4688.2005
60. Yang QE, Sun J, Li L, Deng H, Liu BT, Fang LX, et al. IncF plasmid diversity in multi-drug resistant Escherichia coli strains from animals in China. Front Microbiol. (2015) 6:964. doi: 10.3389/fmicb.2015.00964
61. Johnson TJ, Thorsness JL, Anderson CP, Lynne AM, Foley SL, Han J, et al. Horizontal gene transfer of a ColV plasmid has resulted in a dominant avian clonal type of Salmonella enterica serovar kentucky. PLoS ONE. (2010) 5:e15524. doi: 10.1371/journal.pone.0015524
62. Solà-Ginés M, Cameron-Veas K, Badiola I, Dolz R, Majó N, Dahbi G, et al. Diversity of multi-drug resistant avian pathogenic Escherichia coli (APEC) causing outbreaks of colibacillosis in broilers during 2012 in Spain. PLoS ONE. (2015) 10:e0143191. doi: 10.1371/journal.pone.0143191
63. Ewers C, Antão EM, Diehl I, Philipp HC, Wieler LH. Intestine and environment of the chicken as reservoirs for extraintestinal pathogenic Escherichia coli strains with zoonotic potential. Appl Environ Microbiol. (2009) 75:184–92. doi: 10.1128/AEM.01324-08
64. Stromberg ZR, Johnson JR, Fairbrother JM, Kilbourne J, Van Goor A, Curtiss R, et al. Evaluation of Escherichia coli isolates from healthy chickens to determine their potential risk to poultry and human health. PLoS ONE. (2017) 12:e0180599. doi: 10.1371/journal.pone.0180599
65. Duan H, Chai T, Cai Y, Zhong Z, Yao M, Zhang X. Transmission identification of Escherichia coli aerosol in chicken houses to their environments using ERIC-PCR. Sci China C Life Sci. (2008) 51:164–73. doi: 10.1007/s11427-008-0021-0
66. Chien YC, Chen CJ, Lin TH, Chen SH. Characteristics of microbial aerosols released from chicken and swine feces. J Air Waste Manag Assoc. (2011) 61:882–9. doi: 10.3155/1047-3289.61.8.882
67. Sadeghi AA, Shawrang P, Shakorzadeh S. Immune response of Salmonella challenged broiler chickens fed diets containing gallipro®, a Bacillus subtilis probiotic. Probiotics Antimicrob Proteins. (2015) 7:24–30. doi: 10.1007/s12602-014-9175-1
68. Evans JD, Leigh SA, Branton SL, Collier SD, Pharr GT, Bearson SMD. Mycoplasma gallisepticum: current and developing means to control the avian pathogen. J Appl Poult Res. (2005) 14:757–63. doi: 10.1093/japr/14.4.757
69. Shoaib M. Mycoplasmosis in poultry, a perpetual problem. J Microbiol Biotechnol Food Sci. (2019) 8:1271–5. doi: 10.15414/jmbfs.2019.8.6.1271-1275
70. Stipkovits L, Kempf I. Mycoplasmoses in poultry. Rev Sci Tech. (1996) 15:1495–525. doi: 10.20506/rst.15.4.986
71. Charleston B, Gate JJ, Aitken IA, Reeve-Johnson L. Assessment of the efficacy of tilmicosin as a treatment for Mycoplasma gallisepticum infections in chickens. Avian Pathol. (1998) 27:190–5. doi: 10.1080/03079459808419322
73. Mohammed HO, Carpenter TE, Yamamoto R. Economic impact of Mycoplasma gallisepticum and M. synoviae in commercial layer flocks. Avian Dis. (1987) 31:477–82. doi: 10.2307/1590727
74. Levisohn S, Dykstra MJ. A quantitative study of single and mixed infection of the chicken trachea by Mycoplasma gallisepticum. Avian Dis. (1987) 31:1–12. doi: 10.2307/1590765
75. Minion FC. Molecular pathogenesis of mycoplasma animal respiratory pathogens. Front Biosci. (2002) 7:d1410–22. doi: 10.2741/A849
76. Kulappu Arachchige SN, Young ND, Shil PK, Legione AR, Kanci Condello A, Browning GF, et al. Differential response of the chicken trachea to chronic infection with virulent Mycoplasma gallisepticum strain Ap3AS and vaxsafe MG (Strain ts-304): a transcriptional profile. Infect Immun. (2020) 88:e00053–20. doi: 10.1128/IAI.00053-20
77. Zhang W, Liu Y, Zhang Q, Waqas Ali Shah S, Wu Z, Wang J, et al. Infection impaired the structural integrity and immune function of bursa of fabricius in chicken: implication of oxidative stress and apoptosis. Front Vet Sci. (2020) 7:225. doi: 10.3389/fvets.2020.00225
78. Nunoya T, Yagihashi T, Tajima M, Nagasawa Y. Occurrence of keratoconjunctivitis apparently caused by Mycoplasma gallisepticum in layer chickens. Vet Pathol. (1995) 32:11–8. doi: 10.1177/030098589503200102
79. Leigh SA, Evans JD, Branton SL. Complete genome sequences of two vaccine strains and one field isolate of Mycoplasma gallisepticum. Microbiol Resour Announc. (2019) 8:e01237–19. doi: 10.1128/MRA.01237-19
80. Shil PK, Kanci A, Browning GF, Marenda MS, Noormohammadi AH, Markham PF. GapA+ Mycoplasma gallisepticum ts-11 has improved vaccine characteristics. Microbiology. (2011) 157:1740–9. doi: 10.1099/mic.0.046789-0
81. Leigh SA, Branton SL, Collier SD, Evans JD. Analysis of the effect of diluent for rehydration of poulvac® MycoF on vaccination seroconversion results. Int J Poult Sci. (2011) 10:397–400. doi: 10.3923/ijps.2011.397.400
82. Purswell JL, Evans JD, Leigh SA, Collier SD, Olanrewaju HA, et al. Mycoplasma gallisepticum transmission: comparison of commercial F-strain vaccine versus layer complex-derived field strains in a tunnel ventilated house. Poult Sci. (2012) 91:3072–9. doi: 10.3382/ps.2012-02619
83. Glisson JR, Hofacre CL, Christensen JP. Fowl cholera. In: Saif YM, Fadly AM, editors. Diseases of Poultry. 12th ed. Ames, IA: Blackwell Publishing (2008). p. 739–58.
84. Boyce JD, Harper M, Wilkie IW, Adler B. Pasteurella. In: Gyles CL, Prescott JF, Songer JG, Thoen CO, editors. Pathogenesis of Bacterial Infections in Animals. 4th ed. Ames, IA: Wiley-Blackwell (2010). p. 325–46.
85. Petersen KD, Christensen JP, Permin A, Bisgaard M. Virulence of Pasteurella multocida subsp. multocida isolated from outbreaks of fowl cholera in wild birds for domestic poultry and game birds. Avian Pathol. (2001) 30:27–31. doi: 10.1080/03079450020023168
86. Singh R, Remington B, Blackall P, Turni C. Epidemiology of fowl cholera in free range broilers. Avian Dis. (2014) 58:124–8. doi: 10.1637/10656-090313-Reg.1
87. OBS Commission Editor. Fowl Cholera, in Manual of Diagnostic Tests and Vaccines for Terrestrial Animals. Paris: World Organisation for Animal Health (OIE) (2012). p. 500–506.
88. Harper M, John M, Edmunds M, Wright A, Ford M, Turni C, et al. Protective efficacy afforded by live Pasteurella multocida vaccines in chickens is independent of lipopolysaccharide outer core structure. Vaccine. (2016) 34:1696–703. doi: 10.1016/j.vaccine.2016.02.017
89. Taylor KJM, Ngunjiri JM, Abundo MC, Jang H, Elaish M, Ghorbani A, et al. Respiratory and gut microbiota in commercial turkey flocks with disparate weight gain trajectories display differential compositional dynamics. Appl Environ Microbiol. (2020) 86:e00431–20. doi: 10.1128/AEM.00431-20
90. Sander JE, Resurreccion RS, Waltman WD, McMurray BL. Pasteurella challenge and ELISA serology evaluation of broiler breeders vaccinated with live cholera vaccine. Avian Dis. (1998) 42:190–3. doi: 10.2307/1592595
91. Higgins SE, Wolfenden AD, Tellez G, Hargis BM, Porter TE. Transcriptional profiling of cecal gene expression in probiotic- and Salmonella-challenged neonatal chicks. Poult Sci. (2011) 90:901–13. doi: 10.3382/ps.2010-00907
92. Prado-Rebolledo OF Delgado-Machuca JDJ Macedo-Barragan RJ Garcia-Márquez LJ Morales-Barrera JE Latorre JD . Evaluation of a selected lactic acid bacteria-based probiotic on Salmonella enterica serovar enteritidis colonization and intestinal permeability in broiler chickens. Avian Pathol. (2017) 46:90–4. doi: 10.1080/03079457.2016.1222808
93. Menconi A, Kallapura G, Latorre JD, Morgan MJ, Pumford NR, Hargis BM, et al. Identification and characterization of lactic acid bacteria in a commercial probiotic culture. Biosci Microbiota Food Health. (2014) 33:25–30. doi: 10.12938/bmfh.33.25
94. Biloni A, Quintana CF, Menconi A, Kallapura G, Latorre J, Pixley C, et al. Evaluation of effects of earlybird associated with floramax-B11 on Salmonella enteritidis, intestinal morphology, and performance of broiler chickens. Poult Sci. (2013) 92:2337–46. doi: 10.3382/ps.2013-03279
95. Layton SL, Hernandez-Velasco X, Chaitanya S, Xavier J, Menconi A, Latorre JD, et al. The effect of a Lactobacillus-based probiotic for the control of necrotic enteritis in broilers. Food Nutr Sci. (2013) 4:1–7. doi: 10.4236/fns.2013.411A001
96. Slizewska K, Markowiak-Kopeć P, Zbikowski A, Szeleszczuk P. The effect of synbiotic preparations on the intestinal microbiota and her metabolism in broiler chickens. Sci Rep. (2020) 10:4281. doi: 10.1038/s41598-020-61256-z
97. Beirão BCB, Ingberman M, Favaro C Jr, Mesa D, Bittencourt LC, Fascina VB, et al. Effect of an Enterococcus faecium probiotic on specific IgA following live Salmonella enteritidis vaccination of layer chickens. Avian Pathol. (2018) 47:325–33. doi: 10.1080/03079457.2018.1450487
98. Upadhaya SD, Rudeaux F, Kim IH. Effects of inclusion of Bacillus subtilis (Gallipro) to energy- and protein-reduced diet on growth performance, nutrient digestibility, and meat quality and gas emission in broilers. Poult Sci. (2019) 98:2169–78. doi: 10.3382/ps/pey573
99. Knap I, Kehlet AB, Bennedsen M, Mathis GF, Hofacre CL, Lumpkins BS, et al. Bacillus subtilis (DSM17299) significantly reduces Salmonella in broilers. Poult Sci. (2011) 90:1690–4. doi: 10.3382/ps.2010-01056
100. Zaghari M, Zahroojian N, Riahi M, Parhizkar S. Effect of Bacillus Subtilis spore (GalliPro®) nutrients equivalency value on broiler chicken performance. Italian J Anim Sci. (2015) 14:3555. doi: 10.4081/ijas.2015.3555
101. Abudabos AM, Al-Batshan HA, Murshed MA. Effects of prebiotics and probiotics on the performance and bacterial colonization of broiler chickens. South Afr J Anim Sci. (2015) 45:419. doi: 10.4314/sajas.v45i4.8
102. Abudabos AM, Alyemni AH. Bacillus subtilis PB6 based-probiotic (CloSTATTM) improves intestinal morphological and microbiological status of broiler chickens under Clostridium perfringens challenge. Int J Agric Biol. (2013) 15:973–82.
103. Teo AYL, Tan HM. Effect of Bacillus subtilis PB6 (CloSTAT) on broilers infected with a pathogenic strain of Escherichia coli. J Appl Poult Res. (2006) 15:229–35. doi: 10.1093/japr/15.2.229
104. Latorre JD, Hernandez-Velasco X, Wolfenden RE, Vicente JL, Wolfenden AD, Menconi A, et al. Evaluation and selection of bacillus species based on enzyme production, antimicrobial activity, and biofilm synthesis as direct-fed microbial candidates for poultry. Front Vet Sci. (2016) 3:95. doi: 10.3389/fvets.2016.00095
105. Hernandez-Patlan D, Solis-Cruz B, Pontin KP, Hernandez-Velasco X, Merino-Guzman R, Adhikari B, et al. Impact of a Bacillus direct-fed microbial on growth performance, intestinal barrier integrity, necrotic enteritis lesions, and ileal microbiota in broiler chickens using a laboratory challenge model. Front Vet Sci. (2019) 6:108. doi: 10.3389/fvets.2019.00108
106. Adhikari B, Hernandez-Patlan D, Solis-Cruz B, Kwon YM, Arreguin MA, Latorre JD, et al. Evaluation of the antimicrobial and anti-inflammatory properties of bacillus-DFM (NorumTM) in broiler chickens infected with Salmonella enteritidis. Front Vet Sci. (2019) 6:282. doi: 10.3389/fvets.2019.00282
107. Tellez G, Arreguin-Nava MA, Maguey JA, Michel MA, Latorre JD, Merino-Guzman R, et al. Effect of Bacillus-direct-fed microbial on leaky gut, serum peptide YY concentration, bone mineralization, and ammonia excretion in neonatal female turkey poults fed with a rye-based diet. Poult Sci. (2020) 99:4514–20. doi: 10.1016/j.psj.2020.06.018
108. Arreguin-Nava MA, Graham BD, Adhikari B, Agnello M, Selby CM, Hernandez-Velasco X, et al. Evaluation of in ovo Bacillus spp. based probiotic administration on horizontal transmission of virulent Escherichia coli in neonatal broiler chickens. Poult Sci. (2019) 98:6483–91. doi: 10.3382/ps/pez544
109. Smialek M, Burchardt S, Koncicki A. The influence of probiotic supplementation in broiler chickens on population and carcass contamination with Campylobacter spp. Res Vet Sci. (2018) 118:312–6. doi: 10.1016/j.rvsc.2018.03.009
110. Bogucka J, Ribeiro DM, Bogusławska-Tryk M, Dankowiakowska A, Costa RPR, da Bednarczyk M. Microstructure of the small intestine in broiler chickens fed a diet with probiotic or synbiotic supplementation. J Anim Physiol Anim Nutr. (2019) 103:1785–91. doi: 10.1111/jpn.13182
111. Grimes JL, Rahimi S, Oviedo E, Sheldon BW, Santos FBO. Effects of a direct-fed microbial (Primalac) on turkey poult performance and susceptibility to oral Salmonella challenge. Poult Sci. (2008) 87:1464–70. doi: 10.3382/ps.2008-00498
112. Naseri K, Rahimi S, Khaki P. Comparison of the effects of probiotic, organic acid, and medicinal plant on Campylobacter jejuni challenged broiler chickens. J Agricult Sci Tech. (2012) 14:1485–96.
113. Embrahimi H, Rahimi S, Khaki P. The effect of organic acid, probiotic and Echinacea purpurea usage on gastrointestinal microflora and immune system in broiler chickens. J Vet Res. (2015) 70:293–9.
114. Abudabos AM. Effect of primalac® or enramycin supplementation on performance, intestinal morphology and microbiology of broilers under Clostridium perfringens challenge. J Food Agric Environ. (2012) 10:595-9.
115. Embrahimi H, Rahimi S, Khaki P, Grimes JL, Kathariou S. The effects of probiotics, organic acid, and a medicinal plant on the immune system and gastrointestinal microflora in broilers challenged with Campylobacter jejuni. Turk J Vet Anim Sci. (2016) 40:329–36. doi: 10.3906/vet-1502-68
116. Pender CM, Kim S, Potter TD, Ritzi MM, Young M, Dalloul RA. In ovo supplementation of probiotics and its effects on performance and immune-related gene expression in broiler chicks. Poult Sci. (2017) 96:1052–62. doi: 10.3382/ps/pew381
117. Manafi M, Hedayati M, Mirzaie S. Probiotic Bacillus species and Saccharomyces boulardii improve performance, gut histology and immunity in broiler chickens. South Afr J Anim Sci. (2018) 48:379–89. doi: 10.4314/sajas.v48i2.19
118. Redweik GAJ, Daniels K, Severin AJ, Lyte M, Mellata M. Oral treatments with probiotics and live Salmonella vaccine induce unique changes in gut neurochemicals and microbiome in chickens. Front Microbiol. (2020) 10:3064. doi: 10.3389/fmicb.2019.03064
119. Abd El-Baky AA, Shalaby NA, Abdelgayed SS. Assessment of growth performance, hemato-biochemical parameters, immunological and histopathological alterations associated with new bacterial multistrain probiotic (Gro-2-Max) supplementation on broiler chicken. Int J ChemTech Res. (2016) 9:996–1016.
120. Liao SF, Nyachoti M. Using probiotics to improve swine gut health and nutrient utilization. Anim Nutr. (2017) 3:331–43. doi: 10.1016/j.aninu.2017.06.007
121. Russo P, Arena MP, Fiocco D, Capozzi V, Drider D, Spano G. Lactobacillus plantarum with broad antifungal activity: a promising approach to increase safety and shelf-life of cereal-based products. Int J Food Microb. (2017) 247:48–54. doi: 10.1016/j.ijfoodmicro.2016.04.027
122. Dittoe DK, Ricke SC, Kiess AS. Organic acids and potential for modifying the avian gastrointestinal tract and reducing pathogens and disease. Front Vet Sci. (2018) 5:216. doi: 10.3389/fvets.2018.00216
123. Zhitnitsky D, Rose J, Lewinson O. The highly synergistic, broad spectrum, antibacterial activity of organic acids and transition metals. Sci Rep. (2017) 7:44554. doi: 10.1038/srep44554
124. Vieco-Saiz N, Belguesmia Y, Raspoet R, Auclair E, Gancel F, Isabelle K, et al. Benefits and inputs from lactic acid bacteria and their bacteriocins as alternatives to antibiotic growth promoters during food-animal production. Front Microbiol. (2019) 10:57. doi: 10.3389/fmicb.2019.00057
125. Ren C, Cheng L, Sun Y, Zhang Q, De Haan BJ, Zhang H, et al. Lactic acid bacteria secrete toll like receptor 2 stimulating and macrophage immunomodulating bioactive factors. J Funct Foods. (2020) 66:103783. doi: 10.1016/j.jff.2020.103783
126. DvoroŽnáková E, Bucková B, Hurníková Z, Revajová V, Lauková A. Effect of probiotic bacteria on phagocytosis and respiratory burst activity of blood polymorphonuclear leukocytes (PMNL) in mice infected with Trichinella spiralis. Vet Parasitol. (2016) 231:69–76. doi: 10.1016/j.vetpar.2016.07.004
127. Kogut MH, Tellez GI, McGruder ED, Hargis BM, Williams JD, Corrier DE, et al. Heterophils are decisive components in the early responses of chickens to Salmonella enteritidis infections. Microb Pathog. (1994) 16:141–51. doi: 10.1006/mpat.1994.1015
128. Mirza RA. Probiotics and prebiotics for the health of poultry. in Probiotics and Prebiotics in Animal Health and Food Safety. Erbil: Springer International Publishing (2018). p. 127–54.
129. Bednorz C, Guenther S, Oelgeschläger K, Kinnemann B, Pieper R, Hartmann S, et al. Feeding the probiotic Enterococcus faecium strain NCIMB 10415 to piglets specifically reduces the number of Escherichia coli pathotypes that adhere to the gut mucosa. Appl Environ Microbiol. (2013) 79:7896–904. doi: 10.1128/AEM.03138-13
130. Penaloza-Vazquez A, Ma LM, Rayas-Duarte P. Isolation and characterization of Bacillus spp. Can J Microbiol. (2019) 65:762–74. doi: 10.1139/cjm-2019-0019
131. La Ragione RM, Woodward MJ. Competitive exclusion by Bacillus subtilis spores of Salmonella enterica serotype enteritidis and Clostridium perfringens in young chickens. Vet Microbiol. (2003) 94:245–56. doi: 10.1016/S0378-1135(03)00077-4
132. Whelan RA, Doranalli K, Rinttilä T, Vienola K, Jurgens G, Apajalahti J. The impact of Bacillus subtilis DSM 32315 on the pathology, performance, and intestinal microbiome of broiler chickens in a necrotic enteritis challenge. Poult Sci. (2019) 98:3450–63. doi: 10.3382/ps/pey500
133. Stein T. Bacillus subtilis antibiotics: structures, syntheses and specific functions. Mol Microbiol. (2005) 56:845–57. doi: 10.1111/j.1365-2958.2005.04587.x
134. Piggot PJ, Hilbert DW. Sporulation of Bacillus subtilis. Curr Opin Microbiol. (2004) 7:579–86. doi: 10.1016/j.mib.2004.10.001
135. Schallmey M, Singh A, Ward OP. Developments in the use of Bacillus species for industrial production. Can J Microbiol. (2004) 50:1–17. doi: 10.1139/w03-076
136. Bernardeau M, Lehtinen MJ, Forssten SD, Nurminen P. Importance of the gastrointestinal life cycle of Bacillus for probiotic functionality. J Food Sci Tech. (2017) 54:2570–84. doi: 10.1007/s13197-017-2688-3
137. Ma M. Evaluation of a commercially available probiotic and organic acid blend product on production parameters and economics in broiler breeders. Nutr Food Tech Open Access. (2017) 3:1–5. doi: 10.16966/2470-6086.139
138. Uyen NQ, Hong HA, Cutting SM. Enhanced immunization and expression strategies using bacterial spores as heat-stable vaccine delivery vehicles. Vaccine. (2007) 25:356–65. doi: 10.1016/j.vaccine.2006.07.025
139. Khalique A, Naseem T, Haque N, Rasool Z. Effect of Bacillus subtilis Pb6 on growth and gut microflora in Clostridium perfringens challenged broilers. Int J Anim Vet Sci. (2017) 10:763–6. doi: 10.5281/zenodo.1340216
140. Solis-Cruz B, Hernandez-Patlan D, Petrone VM, Pontin KP, Latorre JD, Beyssac E, et al. Evaluation of a Bacillus-based direct-fed microbial on aflatoxin B1 toxic effects, performance, immunologic status, and serum biochemical parameters in broiler chickens. Avian Dis. (2019) 63:659–69. doi: 10.1637/aviandiseases-D-19-00100
141. Ruiz L, Delgado S, Ruas-Madiedo P, Sánchez B, Margolles A. Bifidobacteria and their molecular communication with the immune system. Front Microbiol. (2017) 8:2345. doi: 10.3389/fmicb.2017.02345
142. Ivanov D, Emonet C, Foata F, Affolter M, Delley M, Fisseha M, et al. A serpin from the gut bacterium bifidobacterium longum inhibits eukaryotic elastase-like serine proteases. J Bio Chem. (2006) 281:17246–52. doi: 10.1074/jbc.M601678200
143. Flint HJ, Duncan SH, Scott KP, Louis P. Links between diet, gut microbiota composition and gut metabolism. Proc Nutr Soc. (2015) 74:13–22. doi: 10.1017/S0029665114001463
144. Arpaia N, Campbell C, Fan X, Dikiy S, van der Veeken J, deRoos P, et al. Metabolites produced by commensal bacteria promote peripheral regulatory T-cell generation. Nature. (2013) 504:451–5. doi: 10.1038/nature12726
145. Smith PM, Howitt MR, Panikov N, Michaud M, Gallini CA, Bohlooly Y, et al. The microbial metabolites, short-chain fatty acids, regulate colonic Treg cell homeostasis. Science. (2013) 341:569–73. doi: 10.1126/science.1241165
146. Schulthess J, Pandey S, Capitani M, Rue-Albrecht KC, Arnold I, Franchini F, et al. The short chain fatty acid butyrate imprints an antimicrobial program in macrophages. Immunity. (2019) 50:432–45.e7. doi: 10.1016/j.immuni.2018.12.018
147. MéNard O, Gafa V, Kapel N, Rodriguez B, Butel MJ, Waligora-Dupriet AJ. Characterization of immunostimulatory CpG-rich sequences from different Bifidobacterium species. Appl Environ Microbiol. (2010) 76:2846–55. doi: 10.1128/AEM.01714-09
148. Keestra AM, de Zoete MR, Bouwman LI, van Putten JPM. Chicken TLR21 is an innate CpG DNA receptor distinct from mammalian TLR9. J Immunol. (2010) 185:460–7. doi: 10.4049/jimmunol.0901921
149. Rajkowska K, Kunicka-Styczynska A. Phenotypic and genotypic characterization of probiotic yeasts. Biotechnol Biotechnol Equip. (2009) 23:662–5. doi: 10.1080/13102818.2009.10818511
150. Sen S, Mansell TJ. Yeasts as probiotics: mechanisms, outcomes, and future potential. Fungal Gen Bio. (2020) 137:103333. doi: 10.1016/j.fgb.2020.103333
151. Schneider SM, Girard-Pipau F, Filippi J, Hebuterne X, Moyse D, Hinojosa GC, et al. Effects of Saccharomyces boulardii on fecal short-chain fatty acids and microflora in patients on long-term total enteral nutrition. World J Gastroenterol. (2005) 11:6165–9. doi: 10.3748/wjg.v11.i39.6165
152. Kiros TG, Luise D, Derakhshani H, Petri R, Trevisi P, D'Inca R, et al. Effect of live yeast Saccharomyces cerevisiae supplementation on the performance and cecum microbial profile of suckling piglets. PLoS ONE. (2019) 14:e0219557. doi: 10.1371/journal.pone.0219557
153. Naimah AK, Al-Manhel AJA, Al-Shawi MJ. Isolation, purification and characterization of antimicrobial peptides produced from Saccharomyces boulardii. Int J Peptide Res Therap. (2018) 24:455–61. doi: 10.1007/s10989-017-9632-2
154. Branco P, Francisco D, Monteiro M, Almeida MG, Caldeira J, Arneborg N, et al. Antimicrobial properties and death-inducing mechanisms of saccharomycin, a biocide secreted by Saccharomyces cerevisiae. Appl Microbiol Biotechnol. (2017) 101:159–71. doi: 10.1007/s00253-016-7755-6
155. Reya G, Ritun C, Kasturi S. Inulin induced co-aggregation of Saccharomyces boulardii with potential pathogenic bacteria. Int J Probiotics Prebiotics. (2019) 14:18–23. doi: 10.37290/ijpp2641-7197.14:18-23
156. Negro MJ, Ballesteros I, Manzanares P, Oliva JM, Sáez F, Ballesteros M. Inulin-containing biomass for ethanol production: carbohydrate extraction and ethanol fermentation. Appl Biochem Biotechnol. (2006) 129–132:922–32. doi: 10.1385/ABAB:132:1:922
157. Food and Agriculture Organization (FAO) Technical Meeting on Prebiotics: Food Quality and Standards Service (AGNS). FAO Technical Meeting Report. Rome, FAO (2007). p. 15–16.
158. Alagawany M, Abd El-Hack ME, Farag MR, Sachan S, Karthik K, Dhama K. The use of probiotics as eco-friendly alternatives for antibiotics in poultry nutrition. Environ Sci Pollution Res. (2018) 25:10611–8. doi: 10.1007/s11356-018-1687-x
159. Markowiak P, Slizewska K. The role of probiotics, prebiotics and synbiotics in animal nutrition. Gut Pathog. (2018) 10:21. doi: 10.1186/s13099-018-0250-0
160. Redweik GAJ, Stromberg ZR, Van Goor A, Mellata M. Protection against avian pathogenic Escherichia coli and Salmonella Kentucky exhibited in chickens given both probiotics and live Salmonella vaccine. Poult Sci. (2019) 99:752–62. doi: 10.1016/j.psj.2019.10.038
161. Huys G, Vancanneyt M, D'Haene K, Vankerckhoven V, Goossens H, Swings J. Accuracy of species identity of commercial bacterial cultures intended for probiotic or nutritional use. Res Microbiol. (2006) 157:803–10. doi: 10.1016/j.resmic.2006.06.006
162. Tellez G, Pixley C, Wolfenden RE, Layton SL, Hargis BM. Probiotics/direct fed microbials for Salmonella control in poultry. Food Res Int. (2012) 45:628–33. doi: 10.1016/j.foodres.2011.03.047
163. Seol D, Jhang SY, Kim H, Kim SY, Kwak HS, Kim SH, et al. Accurate and strict identification of probiotic species based on coverage of whole-metagenome shotgun sequencing data. Front Microbiol. (2019) 10:1683. doi: 10.3389/fmicb.2019.01683
164. Chen T, Wu Q, Zhou H, Deng K, Wang X, Meng F, et al. Assessment of commercial probiotic products in China for labelling accuracy and probiotic characterisation of selected isolates. Int J Dairy Tech. (2017) 70:119–26. doi: 10.1111/1471-0307.12331
165. Dasti JI, Tareen AM, Lugert R, Zautner AE, Gross U. Campylobacter jejuni: a brief overview on pathogenicity-associated factors and disease-mediating mechanisms. Int J Med Microbiol. (2010) 300:205–11. doi: 10.1016/j.ijmm.2009.07.002
166. European Food Safety Authority (EFSA). Scientific Opinion on Campylobacter in Broiler Meat Production: Control Options and Performance Objectives and/or Targets at Different Stages of the Food Chain. Parma. (2011).
167. Beery JT, Hugdahl MB, Doyle MP. Colonization of gastrointestinal tracts of chicks by campylobacter jejuni. Appl Environ Microbiol. (1988) 54:2365–70. doi: 10.1128/AEM.54.10.2365-2370.1988
168. Glunder G, Spiering N, Hinz K, (eds.). Investigations on parenteral immunization of chickens with a campylobacter mineral oil vaccine. In: COST Action 97 Pathogenic Micro-Organisms in Poultry and Eggs. Budapest (1997).
169. Ziprin RL, Hume ME, Young CR, Harvey RB. Inoculation of chicks with viable non-colonizing strains of Campylobacter jejuni: evaluation of protection against a colonizing strain. Curr Microbiol. (2002) 44:221–3. doi: 10.1007/s00284-001-0088-3
170. Noor SM, Husband AJ, Widders PR. In ovo oral vaccination with Campylobacter jejuni establishes early development of intestinal immunity in chickens. Br Poult Sci. (1995) 36:563–73. doi: 10.1080/00071669508417802
171. Widders PR, Thomas LM, Long KA, Tokhi MA, Panaccio M, Apos E. The specificity of antibody in chickens immunized to reduce intestinal colonization with Campylobacter jejuni. Vet Microbiol. (1998) 64:39–50. doi: 10.1016/S0378-1135(98)00251-X
172. Khoury CA, Meinersmann RJ. A genetic hybrid of the Campylobacter jejuni flaA gene with LT-B of Escherichia coli and assessment of the efficacy of the hybrid protein as an oral chicken vaccine. Avian Dis. (1995) 39:812–20. doi: 10.2307/1592418
173. Huang JL, Yin YX, Pan ZM, Zhang G, Zhu AP, Liu XF, et al. Intranasal immunization with chitosan/ pCAGGS-flaA nanoparticles inhibits campylobacter jejuni in a white leghorn model. J Biomed Biotechn. (2010) 2010:589476. doi: 10.1155/2010/589476
174. Neal-McKinney JM, Samuelson DR, Eucker TP, Nissen MS, Crespo R, Konkel ME. Reducing Campylobacter jejuni colonization of poultry via vaccination. PLoS ONE. (2014) 9:e114254. doi: 10.1371/journal.pone.0114254
175. Annamalai T, Pina-Mimbela R, Kumar A, Binjawadagi B, Liu Z, Renukaradhya GJ, et al. Evaluation of nanoparticle-encapsulated outer membrane proteins for the control of Campylobacter jejuni colonization in chickens. Poult Sci. (2013) 92:2201–11. doi: 10.3382/ps.2012-03004
176. Theoret JR, Cooper KK, Zekarias B, Roland KL, Law BF, Curtiss R, et al. The Campylobacter jejuni Dps homologue is important for in vitro biofilm formation and cecal colonization of poultry and may serve as a protective antigen for vaccination. Clin Vaccine Immunol. (2012) 19:1426–31. doi: 10.1128/CVI.00151-12
177. Nothaft H, Davis B, Lock YY, Perez-Munoz ME, Vinogradov E, Walter J, et al. Engineering the Campylobacter jejuni N-glycan to create an effective chicken vaccine. Sci Rep. (2016) 6:26511. doi: 10.1038/srep26511
178. Wang C, Zhou H, Guo F, Yang B, Su X, Lin J, et al. Oral immunization of chickens with Lactococcus lactis expressing cjaA temporarily reduces Campylobacter jejuni colonization. Foodborne Pathog Dis. (2020) 17:366–72. doi: 10.1089/fpd.2019.2727
179. Layton SL, Morgan MJ, Cole K, Kwon YM, Donoghue DJ, Hargis BM, et al. Evaluation of Salmonella-vectored Campylobacter peptide epitopes for reduction of Campylobacter jejuni in broiler chickens. Clin Vaccine Immunol. (2011) 18:449–54. doi: 10.1128/CVI.00379-10
180. Nothaft H, Perez-Muñoz ME, Gouveia GJ, Duar RM, Wanford JJ, Lango-Scholey L, et al. Coadministration of the Campylobacter jejuni N-glycan-based vaccine with probiotics improves vaccine performance in broiler chickens. Appl Environ Microbiol. (2017) 83:e01523–17. doi: 10.1128/AEM.01523-17
181. Sahin O, Zhang Q, Meitzler JC, Harr BS, Morishita TY, Mohan R. Prevalence, antigenic specificity, and bactericidal activity of poultry anti-Campylobacter maternal antibodies. Appl Environ Microbiol. (2001) 67:3951–7. doi: 10.1128/AEM.67.9.3951-3957.2001
182. Fernandes da Costa SP, Mot D, Geeraerts S, Bokori-Brown M, Van Immerseel F, Titball RW. Variable protection against experimental broiler necrotic enteritis after immunization with the C-terminal fragment of Clostridium perfringens alpha-toxin and a non-toxic NetB variant. Avian Pathol. (2016) 45:381–8. doi: 10.1080/03079457.2015.1129663
183. Timbermont L, Haesebrouck F, Ducatelle R, Van Immerseel F. Necrotic enteritis in broilers: an updated review on the pathogenesis. Avian Pathol. (2011) 40:341–7. doi: 10.1080/03079457.2011.590967
184. Mishra N, Smyth JA. Oral vaccination of broiler chickens against necrotic enteritis using a non-virulent NetB positive strain of Clostridium perfringens type A. Vaccine. (2017) 35(49 Pt B):6858–65. doi: 10.1016/j.vaccine.2017.10.030
185. Jiang Y, Mo H, Willingham C, Wang S, Park J-Y, Kong W, et al. Protection against necrotic enteritis in broiler chickens by regulated delayed lysis Salmonella vaccines. Avian Dis. (2015) 59:475–85. doi: 10.1637/11094-041715-Reg
186. Wilde S, Jiang Y, Tafoya AM, Horsman J, Yousif M, Vazquez LA, et al. Salmonella-vectored vaccine delivering three Clostridium perfringens antigens protects poultry against necrotic enteritis. PLoS ONE. (2019) 14:e0197721. doi: 10.1371/journal.pone.0197721
187. Licciardi PV, Tang ML. Vaccine adjuvant properties of probiotic bacteria. Discov Med. (2011) 12:525–33.
188. Ritzi MM, Abdelrahman W, van-Heerden K, Mohnl M, Barrett NW, Dalloul RA. Combination of probiotics and coccidiosis vaccine enhances protection against an Eimeria challenge. Vet Res. (2016) 47:111. doi: 10.1186/s13567-016-0397-y
189. De Vadder F, Grasset E, Mannerås Holm L, Karsenty G, Macpherson AJ, Olofsson LE, et al. Gut microbiota regulates maturation of the adult enteric nervous system via enteric serotonin networks. Proc Natl Acad Sci USA. (2018) 115:6458–63. doi: 10.1073/pnas.1720017115
190. Dinan TG, Stilling RM, Stanton C, Cryan JF. Collective unconscious: how gut microbes shape human behavior. J Psychiatr Res. (2015) 63:1–9. doi: 10.1016/j.jpsychires.2015.02.021
191. Martin CR, Osadchiy V, Kalani A, Mayer EA. The brain-gut-microbiome axis. Cell Mol Gastroenterol Hepatol. (2018) 6:133–48. doi: 10.1016/j.jcmgh.2018.04.003
192. Ball AG, Bell EA, Sienerth K, Uno JK. Using a zebrafish model to study the gut microbiota's effect on the brain's neurochemistry and behavior. FASEB. (2018) 32(S1):765.8. doi: 10.1096/fasebj.2018.32.1_supplement.765.8
193. Lyte M. Probiotics function mechanistically as delivery vehicles for neuroactive compounds: microbial endocrinology in the design and use of probiotics. Bioessays. (2011) 33:574–81. doi: 10.1002/bies.201100024
194. Lyte M. Microbial endocrinology: an ongoing personal journey. Adv Exp Med Biol. (2016) 874:1–24. doi: 10.1007/978-3-319-20215-0_1
195. Villageliú DN, Rasmussen S, Lyte M. A microbial endocrinology-based simulated small intestinal medium for the evaluation of neurochemical production by gut microbiota. FEMS Microbiol Ecol. (2018) 94:1–9. doi: 10.1093/femsec/fiy096
196. Valcarce DG, Martínez-Vázquez JM, Riesco MF, Robles V. Probiotics reduce anxiety-related behavior in zebrafish. Heliyon. (2020) 6:e03973. doi: 10.1016/j.heliyon.2020.e03973
197. Borrelli L, Aceto S, Agnisola C, De Paolo S, Dipineto L, Stilling RM, et al. Probiotic modulation of the microbiota-gut-brain axis and behaviour in zebrafish. Sci Rep. (2016) 6:30046. doi: 10.1038/srep30046
198. Calefi AS, Fonesca JGS, Cohn DWH, Honda BTB, Costola-de-Souza C, Tsugiyama LE, et al. The gut-brain axis interactions during heat stress and avian necrotic enteritis. Poult Sci. (2016) 95:1005–14. doi: 10.3382/ps/pew021
Keywords: probiotics, live vaccines, food safety, immunology, chickens
Citation: Redweik GAJ, Jochum J and Mellata M (2020) Live Bacterial Prophylactics in Modern Poultry. Front. Vet. Sci. 7:592312. doi: 10.3389/fvets.2020.592312
Received: 06 August 2020; Accepted: 21 September 2020;
Published: 28 October 2020.
Edited by:
Guillermo Tellez, University of Arkansas, United StatesReviewed by:
Lisa Bielke, The Ohio State University, United StatesArantxa Morales Mena, National Autonomous University of Mexico, Mexico
Copyright © 2020 Redweik, Jochum and Mellata. This is an open-access article distributed under the terms of the Creative Commons Attribution License (CC BY). The use, distribution or reproduction in other forums is permitted, provided the original author(s) and the copyright owner(s) are credited and that the original publication in this journal is cited, in accordance with accepted academic practice. No use, distribution or reproduction is permitted which does not comply with these terms.
*Correspondence: Melha Mellata, bW1lbGxhdGFAaWFzdGF0ZS5lZHU=