Trichuris trichiura (Linnaeus, 1771) From Human and Non-human Primates: Morphology, Biometry, Host Specificity, Molecular Characterization, and Phylogeny
- Department of Microbiology and Parasitology, Faculty of Pharmacy, University of Seville, Seville, Spain
Human trichuriasis is a Neglected Tropical Disease, which affects hundreds of millions of persons worldwide. Several studies have reported that non-human primates (NHP) represent important reservoirs for several known zoonotic infectious diseases. In this context, Trichuris infections have been found in a range of NHP species living in natural habitats, including colobus monkeys, macaques, baboons, and chimpanzees. To date, the systematics of the genus Trichuris parasitizing humans and NHP is unclear. During many years, Trichuris trichiura was considered as the whipworm present in humans and primates. Subsequently, molecular studies suggested that Trichuris spp. in humans and NHP represent several species that differ in host specificity. This work examines the current knowledge of T. trichiura and its relationship to whipworm parasites in other primate host species. A phylogenetic hypothesis, based on three mitochondrial genes (cytochrome c oxidase subunit 1, cytochrome b, and large subunit rRNA-encoding gene) and two fragments of ribosomal DNA (Internal Transcribed Spacer 1 and 2), allowed us to define a complex of populations of T. trichiura hosting in a large variety of NHP species, in addition to humans. These populations were divided into four phylogenetic groups with a different degree of host specificity. From these data, we carry out a new morphological and biometrical description of the populations of Trichuris based on data cited by other authors as well as those provided in this study. The presence of T. trichiura is analyzed in several NHP species in captivity from different garden zoos as possible reservoir of trichuriasis for humans. This study contributes to clarify questions that lead to identification of new taxa and will determine parasite transmission routes between these primates, allowing the implementation of appropriate control and prevention measures.
Introduction
Worldwide, ~1.5 billion people, nearly 24% of the world's population, are infected with soil-borne helminths. Soil-borne helminthiasis is widely distributed in tropical and subtropical areas, especially in sub-Saharan Africa, The Americas, China, and East Asia. More than 267 million preschoolers and more than 568 million school-age children live in areas with severe transmission of these parasites and need treatment and preventive interventions. The main species of soil-borne helminths that infect humans are Ascaris lumbricoides (roundworm), Trichuris trichiura (whipworm), and Necator americanus and Ancylostoma duodenale (hookworms) (1).
T. trichiura, is the etiological agent of the parasitic disease known as “trichuriasis,” which is considered as a Neglected Tropical Disease. T. trichiura is the second most common helminth in humans, and Trichuriasis has a worldwide geographical distribution. The prevalence is higher in places with warm and humid weather, where there is a lack of basic sanitation services. Between 30 and 80% of cases are recorded in children, who suffer the greatest parasitic burden and those with the most significant symptoms (2). Transmission of this parasite occurs after ingestion of embryonated eggs. These eggs can enter new hosts through contaminated hands, food, soil, and water. Then these hatch in the intestine, where L1 larvae are released. Larvae penetrate the epithelial layer of the large intestine and grow to adult stage. After mating, the non-embryonated eggs are released from the females and again reach the environment through the host's feces.
Up to date, whipworms isolated from humans and other primates have traditionally been regarded as T. trichiura (3–5), while those recovered from pigs and wild boars are known as Trichuris suis (6, 7). It is well-known that differentiation between closely related species of Trichuris is very difficult due to the phenotypic plasticity of the organisms themselves; host-induced variation, paucity of morphological features, and overlapping morphological characteristics that occur among species (8–11). Thus, many studies on Trichuris have focused on the morphological and molecular differentiation of T. trichiura and T. suis, which are molecularly different but morphologically similar (7, 12–14).
On the other hand, the relationship between Trichuris from humans and non-human primates (NHP) in terms of genetic and evolutionary aspects is poorly understood. In recent years, some publications addressed the question of whether Trichuris species are shared between humans and NHP or whether there are different species. The genus Trichuris is likely a candidate to harbor cryptic species as it has a wide geographical distribution and infects several host species (15). As revealed by recent studies, there is more than one taxon capable of infecting humans and other primates, including individuals in captivity, suggesting that T. trichiura should be considered a complex species that includes different cryptic units (16, 17). In addition, based on morpho-biometric and molecular parameters, new species of Trichuris have been described in primates, such as Trichuris rhinopiptheroxella (18) that was found in the golden snub-nosed monkey (Rhinopithecus roxellana), Trichuris colobae from Colobus guereza kikuyuensis (19), and Trichuris ursinus from Papio ursinus (20). Therefore, these studies confirmed that T. trichiura is not the only whipworm found in primates.
Currently, the systematics of the genus Trichuris shows significant gaps. This is due to two main reasons: (i) the lack of comparative morpho-biometric data through the use of multiple parameters and statistical tests applied to the taxonomic study of these species and (ii) the paucity of published research on the genetics of the different Trichuris species in humans, NHP, and pigs. Nowadays, researchers have not yet managed to finally establish the degree of divergence between the different genetic lineages that appear to exist in Trichuris species parasitizing these hosts.
In this paper, we carried out an update of the morphological and biometric characteristics of T. trichiura isolated from human and NHP. Besides, the molecular phylogenetic relationships between these populations are analyzed based on molecular data (mitochondrial and nuclear markers). Furthermore, some phylogenetic hypotheses were inferred for Trichuris spp. to shed light on the degree of divergence between different genetic lineages. In addition, the presence of T. trichiura was analyzed in several NHP species in captivity from different garden zoos as possible reservoir of trichuriasis for humans.
Materials and Methods
Ethics Statement
This study does not require approval by an ethics committee. Macaca sylvanus and C. g. kikuyensis, from which Trichuris specimens were collected from their caeca postmortem, died of natural death. The specimens were handled and housed in a zoo in strict accordance with good animal practices. The other specimens and eggs of Trichuris sp. were recovered from the feces after routine anthelmintic treatment.
Isolation of Material
In this study, we sampled Trichuris's adults and eggs of a total of five NHP host species, including the Barbary macaque (M. sylvanus), vervet monkey (Chlorocebus aethiops), patas monkey (Erythrocebus patas), Guinea baboon (Papio papio), and black and white colobus (C. g. kikuyuensis) from the Zoo Castellar (Cádiz, Spain), Selwo Aventura (Málaga, Spain), Zoo Barcelona (Barcelona, Spain), Parque de la Naturaleza de Cabárceno (Cantabria, Spain), and Bioparc Fuengirola (Málaga, Spain), respectively (Table 1).
Only three adult whipworm specimens (two females and one male) were collected from Guinea baboon, sixty-five adults (32 females and 33 males) from a male Barbary macaque (17, 21) and five adults from a C. g. kikuyuensis. Adult's worms were washed separately in saline solution (0.9% w/v), then frozen at −20°C until posterior studies. Whipworm's eggs were isolated from feces of all NHP species. The sequences successfully obtained of different molecular markers are summarized in the Table 1.
The fecal eggs were concentrated using a Sheather's sugar solution (22) and then embryonated at 32°C for 3–4 weeks added with potassium dichromate 0.2% w/v solution to give humidity to the medium and prevent fungal and bacterial growth (23). Subsequently, the worms were measured and genomic DNA, from both worms and eggs, was extracted.
Morphological Study
Three adult whipworms from Guinea baboon were identified according to previous studies (7, 19, 20). We carried out morphological studies as described Oliveros et al. (24) and Skrjabin et al. (25). Trichuris specimens were measured according to parameters reported by Spakulová and Lýsek (26), Suriano and Navone (27), and Robles et al. (28), and a comparative study was carried out with T. trichiura's specimens previously analyzed biometrically and molecularly (7, 14, 17, 21).
Molecular Study
PCR and Sequencing of Specimens
Genomic DNA from adult worms and a pack of 45–200 isolated eggs were extracted using DNeasy Blood and Tissue Kit (Qiagen) according to the manufacturer's protocol. Quality of extractions was assessed using 0.8% agarose gel electrophoresis infused with SYBR® Safe DNA gel stain.
All molecular markers sequenced in the present study [cox1, cob and rrnL mitochondrial DNA (mtDNA) and ITS1 and ITS2 ribosomal DNA (rDNA)] were amplified using the polymerase chain reaction (PCR) by a thermal cycler (Eppendorf AG; Hamburg, Germany). PCR mix, PCR conditions, and PCR primers are summarized in the Supplementary Table 1.
The PCR products were checked on SYBR® Safe stained with 2% w/v Tris–borate–EDTA (TBE) agarose gels. Bands were eluted and purified from the agarose gel using the QWizard SV Gel and PCR Clean-Up System Kit (Promega, Madison, WI, U.S.A.). Once purified and concentrated, the products were sequenced by Stab Vida (Lisbon, Portugal).
Phylogenetic Analysis
To assess the similarity among all marker sequences of Trichuris sp. obtained in the present study and other Trichuris species, the number of nucleotide differences per sequence was analyzed using Compute Pairwise Distances based on the number of differences method of MEGA v7.0 (29).
To obtain a nucleotide sequence alignment file, the MUSCLE alignment method was used in MEGA v7.0 (29). Additional sequences from the National Center for Biotechnology Information (NCBI) GenBank® database were incorporated into the alignments (Supplementary Table 2).
Assessment of nucleotide substitution saturation, an indicator of whether the genetic marker is useful, was performed using DAMBE v5.3.32 (30, 31). Saturation was based on the values of Iss (index of substitution saturation) and Iss.c (critical Iss value), where Iss < Iss.c indicated that the genetic marker was not saturated and vice versa. Haplotype diversity (h) and nucleotide diversity (π) were calculated using DnaSP v6.12.03 (32).
All phylogenetic trees were inferred based on nucleotide data and obtained by two methods: Maximum Likelihood (ML) and Bayesian Inference (BI). PHYML package was used to generate ML trees (33), and MrBayes v3.2.6 to generate BI (34). jModelTest was employed to resolve the best-fit substitution model for the parasite data (35). Models of evolution were selected for subsequent analysis according to the Akaike Information Criterion (36). To examine the dataset containing the concatenation of four markers used (ITS1, ITS2, cox1, and cob), analyses based on BI were partitioned by gene and models for individual genes within partitions were those selected by jModelTest. For ML inference, best-fit nucleotide substitution models included the general time-reversible (GTR) model with gamma-distributed rate variation (G) and a proportion of invariable sites (I), GTR + G (ITS1 and ITS2), GTR + I + G (cox1), GTR + I + G (cob), and GTR + G (rrnL). Support for the topology was examined using bootstrapping (heuristic option) (37) over 1,000 replications to assess the relative reliability of clades. The commands used in MrBayes for BI were nst = 6 with gamma rates (ITS1, ITS2, and rrnL), nst = 6 with invgamma rates (cox1 and cob), and nst = mixed (concatenated phylogenetic trees). The standard deviation of split frequencies was used to determine whether the number of generations completed was sufficient; the chain was sampled every 500 generations, and each dataset was run for 10 million generations. Trees from the first million generations were discarded based on an assessment of convergence. Burn-in was determined empirically by examination of the log likelihood values of the chains. The Bayesian Posterior Probabilities (BPP) comprise the percentage converted.
Results
Molecular Analysis
Annotation and Features of Ribosomal and Mitochondrial Genomes
The successfully sequenced specimens, the length of the different sequences, the content of G + C%, and haplotypes of ribosomal and mitochondrial markers analyzed at the present study are shown in Table 1. Different repetitive nucleotide sequences, termed ≪ microsatellites ≫, were found in the ITS2 sequences of Trichuris sp. from human and different NHP. Thus, Poly (GCA), Poly (CGA), and Poly (GCG) were observed in positions 280, 302, and 344, respectively. Furthermore, Poly (GCA) and Poly (GGC) were found in the ITS1 sequences in positions 77 and 203, respectively. Also, a common area to all species of Trichuris was observed in positions 280 (GATCTGGGTGT) and 286 (GCCGCCGGTT) in this ITS1 sequence.
Nucleotide sequence data reported in this study were deposited at the GenBank™, EMBL, and DDBJ databases, and the accession numbers are available in Supplementary Table 2.
Phylogenetic Analysis
All phylogenetic trees based on ribosomal and mitochondrial markers (partitioned and concatenated) confirmed the existence of two main clades: clade 1: “Trichuris suis” and clade 2: “Trichuris trichiura” (Figures 1–3 and Supplementary Figures 1–3).
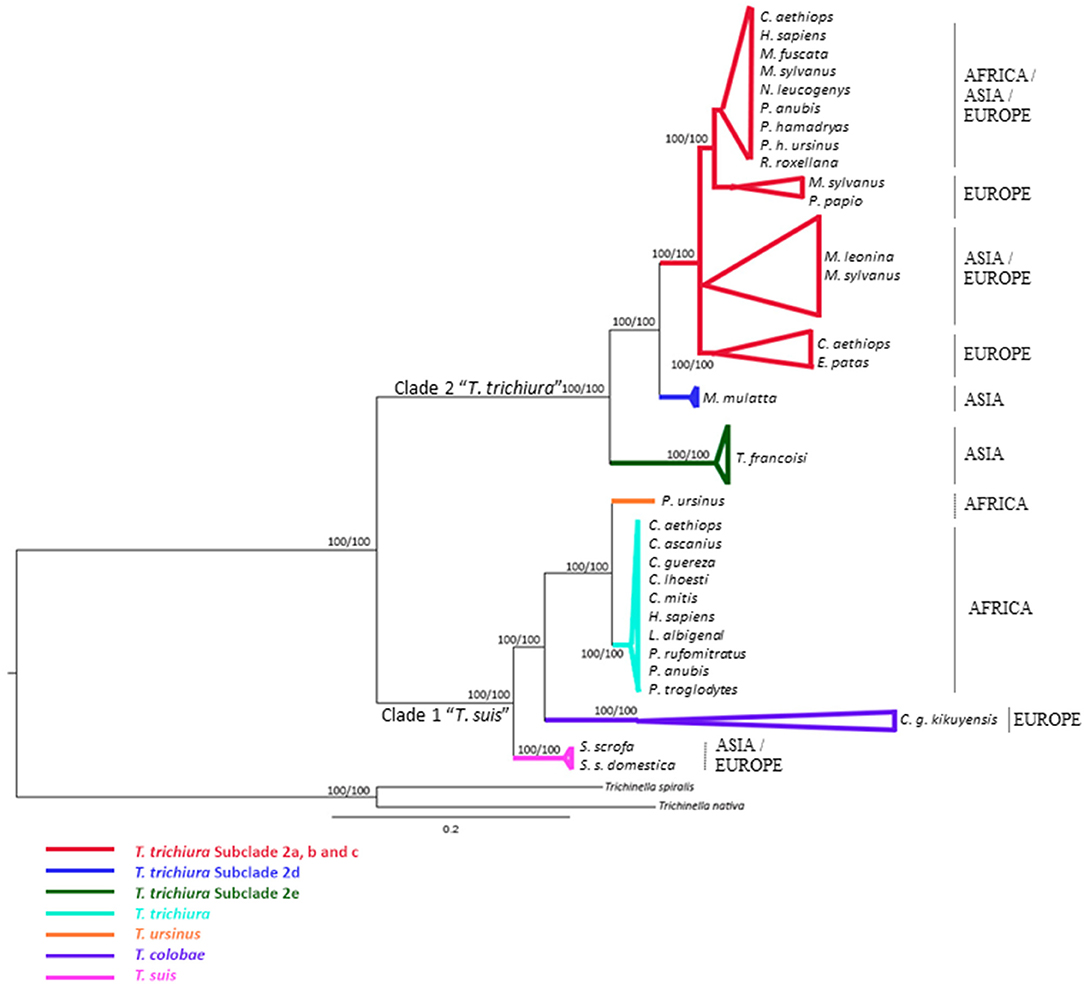
Figure 1. Phylogenetic tree of Trichuris species based on combined analysis of ribosomal DNA (ITS1 and ITS2) inferred using Bayesian Inference. Maximum Likelihood bootstrap values of clades are listed first, followed by Bayesian Posterior Probabilities, respectively, for clade frequencies exceeding 60%.
The alignment of 46 ITS1 and 79 ITS2 rDNA sequences of Trichuris species yielded a dataset of 876 and 863 characters, respectively. Based on ITS1 and ITS2 sequences, the concatenated phylogenetic tree revealed the existence of two highly supported phylogenetic groups within clade 2 “T. trichiura”: One group corresponded to T. trichiura lineage (100% ML and 100% BPP) (Supplementary Table 3) clustering Trichuris sp. from different hosts and geographical regions in six subclades (100% ML and 100% BPP) that included Trichuris sp. from Trachypithecus francoisi (Figure 1). The other 5 subclades (except Trichuris sp. from T. francoisi) showed a high homology each other, ranging 94.65–99.65% (Supplementary Table 4).
The phylogeny inferred on mitochondrial datasets (partitioned and concatenated) revealed similar topologies (Figure 2 and Supplementary Figures 1–3). Thus, four main clades were observed in “T. trichiura lineage” where Trichuris sp. from P. papio, C. aethiops, and E. patas clustered together in the subclade named 2c with T. trichiura from Homo sapiens from Uganda and Trichuris sp. from other hosts from Africa and Europe (Figure 2 and Supplementary Figures 1, 2).
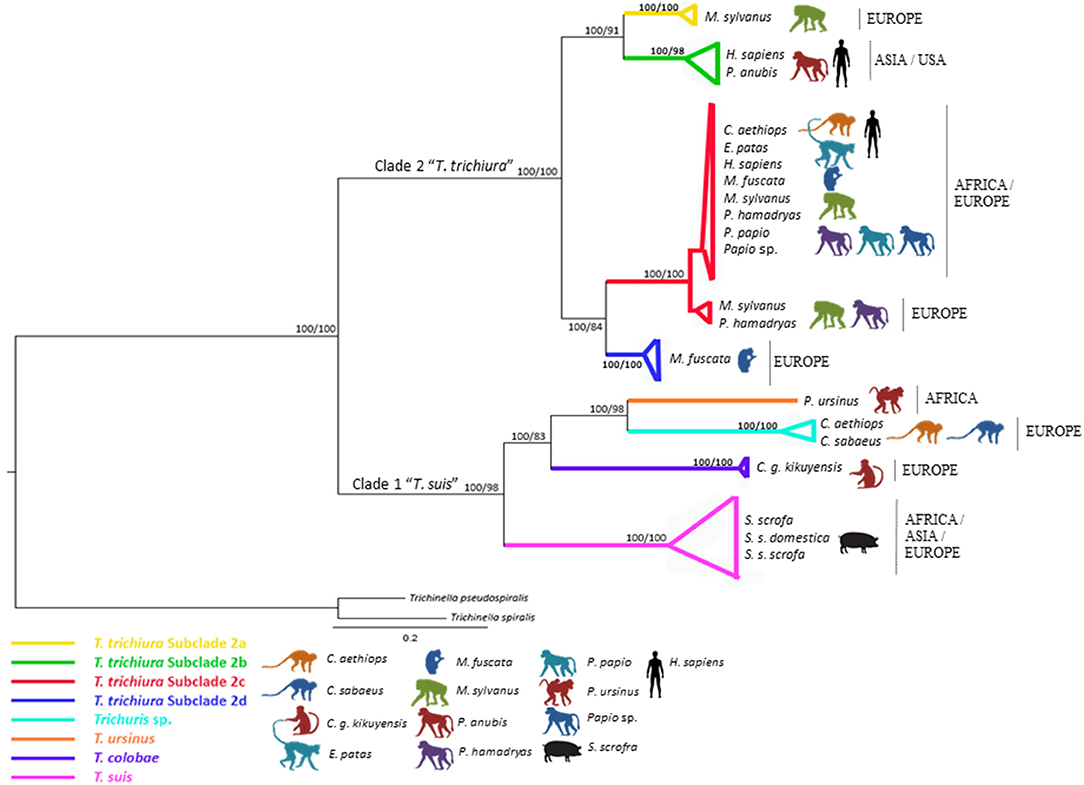
Figure 2. Phylogenetic tree of Trichuris species based on combined analysis of mitochondrial DNA (cox1 and cob) inferred using Bayesian Inference. Maximum Likelihood bootstrap values of clades are listed first, followed by Bayesian Posterior Probabilities, respectively, for clade frequencies exceeding 60%.
The multiple alignments of 48 cox1 nucleotide sequences (including outgroups) yielded a dataset of 296 characters. The phylogenetic tree based on cox1 showed Trichuris from E. patas, C. aethiops, and P. papio clustering in subclade 2c (European and African origin) and related with Macaca fuscata (subclade 2d). This marker did not resolve subclade 2a (Asian and USA origin) appearing in polytomy (Supplementary Figure 1 and Supplementary Table 3).
The multiple alignments of 47 cob nucleotide sequences (including outgroups) yielded a dataset of 444 characters. The phylogenetic tree based on cob was in congruence with rrnL phylogenetic inferences of T. trichiura lineage. Nevertheless, the sister relationship between subclades 2a, 2b, and 2d was not supported (Supplementary Figure 2 and Supplementary Table 3).
The rrnL dataset included 358 aligned positions and 76 taxa, including outgroups. ML and BI methods showed congruence between each other revealing two main clades (“T. suis lineage” and “T. trichiura lineage”) and respect to the sister-group relationships between Trichuris spp. from NHP, humans and pigs (Supplementary Figure 3 and Supplementary Table 3). Within clade 2 “T. trichiura lineage,” phylogenetic trees confirmed the existence of four different subclades highly supported clustering subclade 2b: T. trichiura from H. sapiens from China and Papio anubis from the USA and subclade 2a including the minority haplotype of T. trichiura from M. sylvanus from Spain; subclade 2c: T. trichiura from P. papio, Chlorocebus sabaeus and M. sylvanus from Spain, H. sapiens from Uganda, Papio hamadryas from Europe, and two haplotypes of Trichuris sp. from M. fuscata from Europe; and subclade 2d: T. trichiura from M. fuscata from Europe (Supplementary Figure 3). Curiously, the minority haplotype of M. sylvanus (subclade 2a) and M. fuscata (subclade 2d) (European origin) appeared related with those from Asia and USA (subclade 2b) (Supplementary Figure 3 and Supplementary Table 3). In addition, a sister relationship between T. trichiura and Trichuris sp. from T. francoisi was observed, both species within “T. trichiura lineage” (clade 2).
The concatenated dataset of mitochondrial gene (cox1 and cob) sequences (Figure 2) revealed the subclade 2c as the main one clustering the majority of T. trichiura parasitizing African humans and different NHP from Africa and Europe, showing a sister relationship between 2c and 2d and besides between 2a and 2b (Asian and USA origins) (Figure 2).
The concatenated dataset of ribosomal (ITS1 and ITS2) and mitochondrial (cox1 and cob) gene sequences included 2,479 aligned sites and only 13 taxa, since only we could concatenate sequences of the same individual. Phylogenetic analyses of this dataset yielded a tree with branches strongly supported. Thus, the T. trichiura population was separated in only three different subclades, the subclade 2d not being represented due to the absence of sequences in all the markers. Subclade 2c was the most representative subclade including populations from a high variety of host species (Figure 3 and Supplementary Table 3).
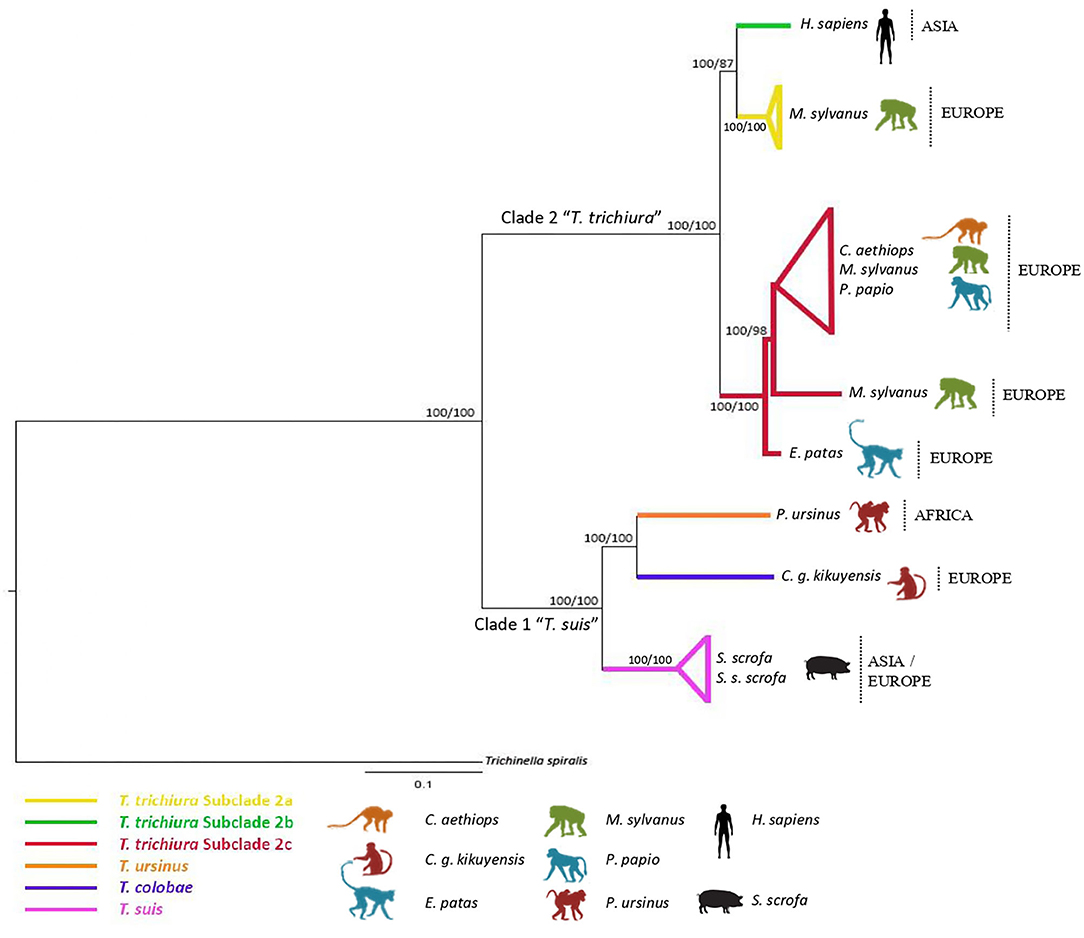
Figure 3. Phylogenetic tree of Trichuris species based on combined analysis of mitochondrial DNA (cox1, cob) and nuclear ribosomal DNA (ITS1 and ITS2) inferred using Bayesian Inference. Maximum Likelihood bootstrap values of clades are listed first, followed by Bayesian Posterior Probabilities, respectively, for clade frequencies exceeding 60%.
The phylogenetic inferences revealed that the populations of Trichuris from P. papio, C. aethiops, and E. patas analyzed in the present study clustered mainly in the subclade of T. trichiura (subclade 2c).
Comparative Sequence Analysis
In order to analyze the intraspecific and interspecific similarity in T. trichiura and between T. trichiura and Trichuris spp. parasitizing NHP as well as T. suis, we carried out a comparative study considering the different clades and subclades previously described for Trichuris spp. hosting humans, NHP, and swine (Supplementary Tables 4–7).
Thus, by examining ITS1 and ITS2 sequences, specimens obtained from P. papio, C. aethiops, and E. patas from Spain revealed a high similarity with populations of T. trichiura corresponding to subclades 2a, 2b, and 2c and subclade Macaca mulatta from different geographical origins (94.65 to 100%) (Supplementary Table 4). Further, the similarity was lower when clade 2 (T. trichiura lineage) and clade 1 (T. suis) were compared (90.62–92.23%).
Mitochondrial sequences (cox1 and cob) from T. trichiura from P. papio, C. aethiops, and E. patas from Spain showed the highest intraspecific similarity within subclade 2c (97.3 to 100% and 92.12 to 100%, respectively) (Supplementary Tables 5, 6). For rrnL sequences, similar results were observed for T. trichiura from M. sylvanus and P. papio (97.77–100%). However, based on three mitochondrial markers, T. trichiura from M. sylvanus showed two different lineages corresponding to subclades 2a and 2c (Supplementary Table 7). Based on three mitochondrial markers, the similarity between clade 2 and T. suis ranged from 68.47 to 82.68%, values lower than those shown within clade 2.
Analysis of genetic diversity for clade 2 based on ITS and mitochondrial sequences revealed a haplotype diversity of 1.0 and 0.95–0.93, respectively (Table 2). In addition, nucleotide diversity based on ITS and rrnL sequences was 0.05; nevertheless, mitochondrial genes (cox1 and cob genes) revealed a higher nucleotide diversity (0.09–0.11) with the maximum values for cox1 (0.11) (Table 2). Within clade 2 (subclades 2a, 2b, 2c, and 2d), haplotype diversity of mitochondrial genes ranged 0.71–1.0 for cox1, 0.67–1.0 for cob, and 0.88–1.0 for rrnL with the maximum values for subclade 2a. Nucleotide diversity of mitochondrial genes ranged 0.01–0.04 for cox1 and cob with the maximum values for subclade 2b, and the value for rrnL was 0.01 (Table 2).
The analysis of nucleotide substitution saturation based on nuclear markers showed Iss > Iss.c (P < 0.005), indicating that ITS regions were saturated. Nevertheless, mitochondrial genes (cox1, cob, and rrnL) were not saturated (Iss < Iss.c, P < 0.005). Genetic diversity measures for all markers are summarized in Table 2.
Update Morphological Description of T. trichiura (Linnaeus, 1771)
Taxonomic summary
- Class Enoplea (Order Trichocephalida) (12, 38).
- Host: H. sapiens (14), Pan troglodytes (7), M. sylvanus (17, 21), P. papio (present study).
General: This is a parasite with a filiform anterior part and a broad and handle-like posterior part. The thin anterior portion of the parasite displays 2 types of cuticle patterns. One side is distinctly striated with transverse grooves and the other side in a finely tuberculate band under higher magnification reveals small circular elevated bodies, which are evenly distributed (Figure 4A). The ventral side of the anterior part of the body is slightly tapered toward the cephalic end which presents a broad longitudinal elongated “bacillary band” showing typical cuticle inflations (Figure 4B). The mouth is surrounded by a group of cephalic papillae arranged in two circles (an inner circle and a lateral circle) with a conspicuous organ, the stylet (Figure 4C), protruding from the middle portion of the mouth cavity. Adults present a moniliform esophagus constituted with a short muscular zone and a long stichosome with 1 row of stichocytes (Figure 4D), and 1 pair of conspicuous cells at esophagus-intestinal junction level (Figures 4D, 5A,B). The site where the esophagus transforms into the intestine corresponds to the place of transition of the thin anterior part into the thick posterior and shows typical glandular cells at this point (Figures 4D, 5A,B).
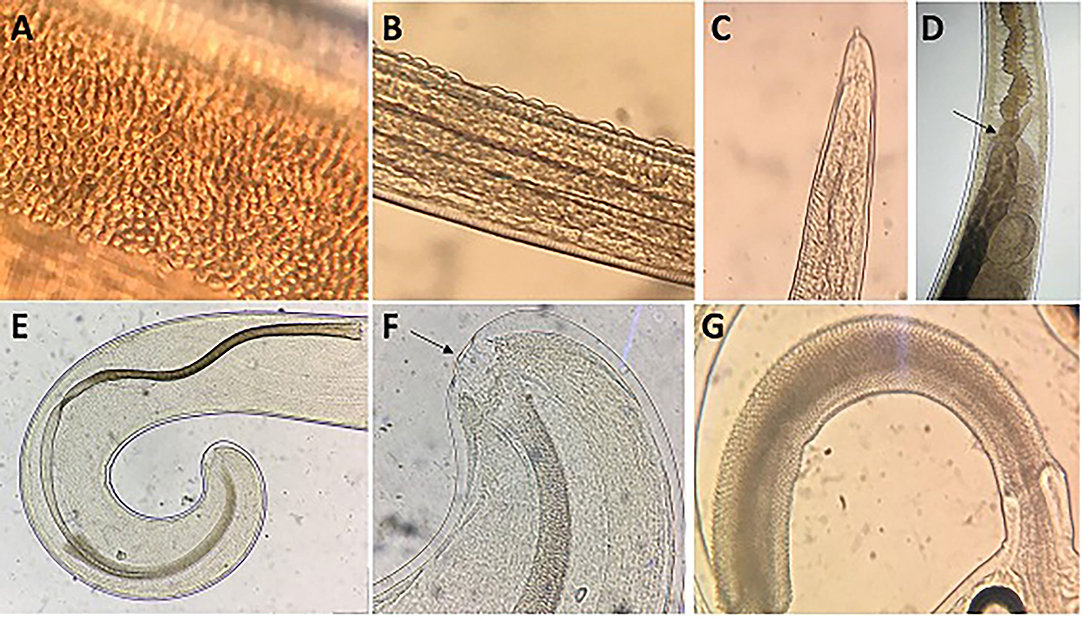
Figure 4. Morphology of males of T. trichiura. (A) Tuberculate band. (B) Bacillary band showing typical cuticular inflations. (C) The mouth with the stylet. (D) Stichosome with 1 row of stichocytes and 1 pair of conspicuous cells at esophagus–intestinal junction level (arrowed). (E) Spicule. (F) Papillae pericloacal. (G) Spicule sheath.
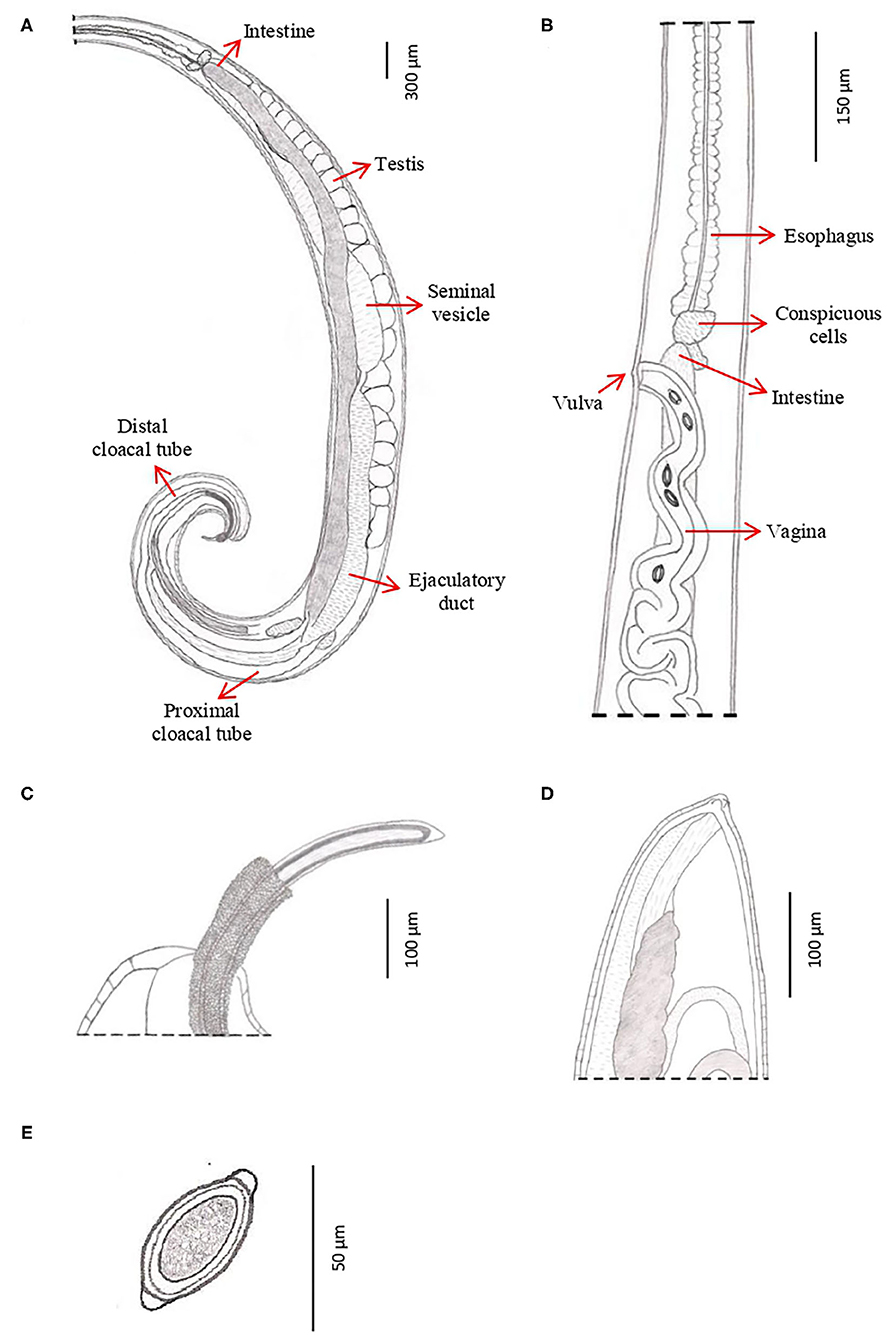
Figure 5. Drawings of T. trichiura from P. papio. (A) Male, posterior end, spiny spicule sheath, spicule, spicule tube and proximal and distal cloacal tube, lateral view. (B) Female, esophagus–intestine junction, vulva and vagina, lateral view. (C) Male, detail of the posterior end, lateral view. (D) Female, posterior end, lateral view. (E) Eggs.
Male: The body is 25–49.3 mm long. The ratio between anterior and posterior body length is 2.6–4:1. The thin anterior part is 1:1.4–1.7 of the entire length of the body. The length of the esophagus is 18–28.9 mm. The width of the body in the esophageal end is 0.15–0.49 mm, and the maximum width (in the posterior part of the body) is 0.39–0.74 mm (Table 3). Posterior end of the body ventrally incurved (Figures 4E–G).
The genital apparatus of the male is a long tube whose sections differ from each other in structure bearing different functions (Figure 5A). The first section of the genital apparatus is the testis, which is very long and strongly convoluted, beginning in the posterior part of the male body, directed anteriorly, and lying along the long axis of the body terminating at a short distance from the transition of the esophagus into the intestine (Figure 5A). The testis ends near the union of the ejaculator conduct and intestine. The testis is followed by the vas deferens which at first runs somewhat anteriorly along the intestine, and then, at the level of the esophageal end and somewhat short of it, describes a convolution, turns backward, and terminates in a small constriction, connecting it with the following section of the genital apparatus, the seminal vesicle. The seminal vesicle runs parallel to the intestine but does not describe sharp convolutions and via a narrow tube with thick muscular walls joins the ejaculatory duct, which is 1.07–2.34 mm long (Figure 5A). The ejaculatory duct joins the intestine to form the cloaca, which opens at the posterior end of the male body. The cloaca with anus subterminal and one pair of paracloacal papilla not ornamented (Figure 4F) was observed when the spicule sheath was invaginated. No cluster of papillae was observed. The proximal cloacal tube is wide and continued with the distal cloacal tube (1.10–2.75 mm) that contains the spicule which projects into the anterior portion of the body in a spicule tube (Figure 5A). There is only one spicule, which is elongated with a pointed tip. This spicule presents two chitinized extreme zones and a light central part and is 1.61–3.81 mm long (Figures 4E, 5A). The spicule is surrounded by a peculiar spicule sheath (0.22–1.23 mm long), which may protrude externally together with the spicule (Figure 4G). The maximum width of the spicule sheath is 0.06–0.11 mm and is covered throughout its length by densely located chitinous spines from the proximal to distal portion and is cylindrical without a distal bulb (Figures 4G, 5C and Table 3).
Female: The body is 20–48.6 mm long. The ratio between anterior and posterior body length is 2.1–2.2:1. The thin anterior part is 1: 0.65–0.67 of the entire length of the body. The length of the esophagus is 13–33 mm. The width of the body around the esophageal end is 0.13–0.48 mm, and the maximum width (in the posterior part of the body) is 0.38–0.90 mm (Table 4). The uterus is unpaired. The vulva is located at esophagus–intestine junction level (Figures 5B, 6A,B). This part of body thereafter changes into a transversely striated body cuticle. The vulva is non-protrusive and has no ornamentation (Figures 6A,B). The vagina has strong walls and, when everted, shows small papillae (Figure 6B). This vagina is long and presents one zone straight near the vulva while presenting circumvolutions nearly the uterus (Figures 5B, 6A). The ovary is long and continues with the oviduct in the back of the body (Figure 6C). The anus lies, subterminal, at the tip of the tail (Figures 5D, 6D). The eggs are barrel-shaped with clear, mucoid-appeared polar plugs, in addition to a vitelline membrane, and have a triple shell, the outermost layer of which is brown (Figure 5E).
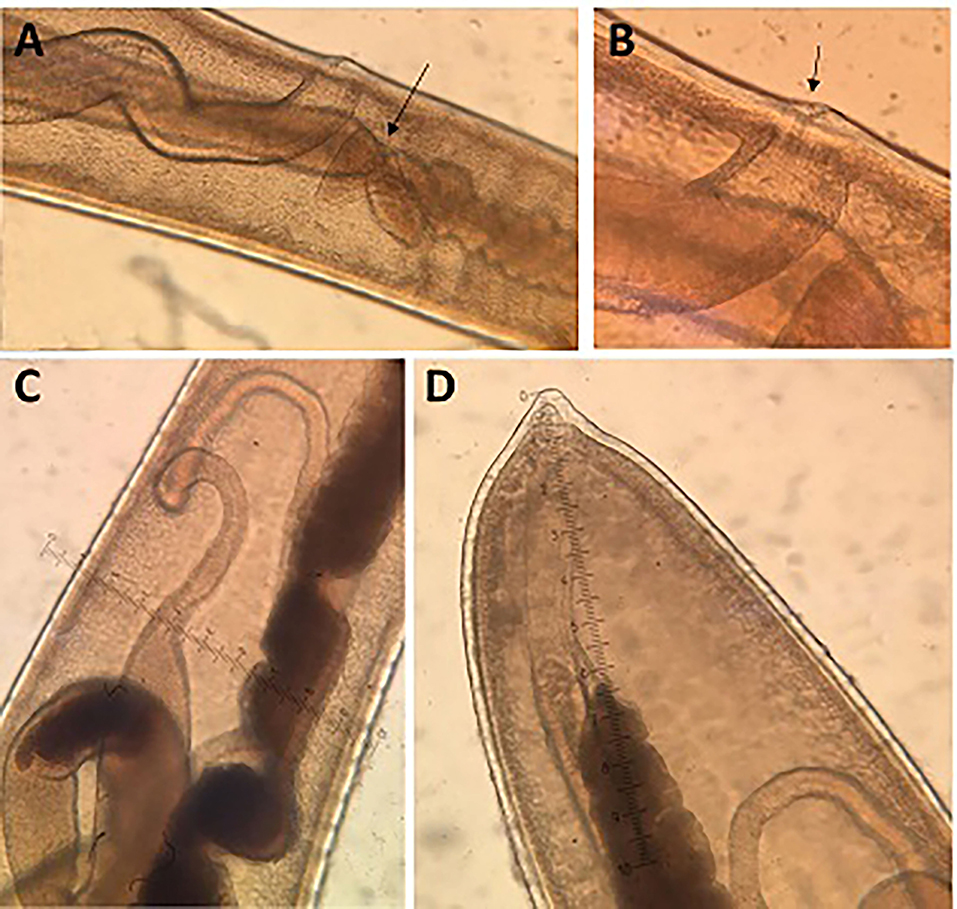
Figure 6. Morphology of females of T. trichiura. (A) Esophagus–intestinal junction level (arrowed), vulva and vagina. (B) Vulva (arrowed). (C) Oviduct. (D) Posterior end.
Discussion
In the present paper, we address an updated morphological and biometric description of T. trichiura, based on the results provided in the present study as well as on previous studies by different authors who characterized this species combining the analysis of morphological, biometric, and molecular characteristics. Thus, for the emendation of the description of T. trichiura, we have considered the populations of P. troglodytes (7), H. sapiens (14), M. sylvanus (17, 21), and P. papio (present study). For many years, different authors have based on the description of T. trichiura isolated from humans and NHP, on morphological and biometric characteristics exclusively (6, 25, 39–43), which was not molecularly confirmed to have corresponded to T. trichiura.
Thus, we found that females of T. trichiura are characterized by a non-protrusive vulva without ornamentation. However, some authors have described the vulva with a surface covered with spines like those of the male's spicule sheath (6, 25). Likewise, based on the morphological characteristics of males, T. trichiura is characterized by the presence of a lanceolate spicule that tapers at the end (41). However, Tenora et al. (42) observed a spicule with a cylindrical end in isolated samples of H. sapiens. Furthermore, males of T. trichiura isolated from P. papio and M. sylvanus presented a spicule with two chitinized extreme zones and a lighter central part (17). This feature was not observed in males of T. trichiura described by several authors who exclusively carried out morphological and biometric studies (6, 7, 25, 39–42).
On the other hand, the spicule sheath is cylindrical without a distal bulb with triangular spines; however, other authors described these spines with different shapes and sizes, some of them with blunt points in T. trichiura from humans and NHP (25, 42). Besides, we found that the males of T. trichiura are characterized by the presence of a pair of paracloacal papillae which are observed when the sheath is invaginated (17); however, a proximal group of small papillae is not observed, as described by Ooi et al. (6) in a morpho-biometric study carried out on samples of monkeys, baboons, and humans. These papillae cluster was described in T. colobae by Cutillas et al. (19) who characterized a new species of Trichuris present in C. g. kikuyuensis based on morphological, biometric, and molecular data. This fact could suggest that the descriptions carried out by Ooi et al. (6) of Trichuris populations from humans and NHP based exclusively on morphological and biometric characters could correspond to different species closed to T. trichiura.
Biometrically, there is a concordance between the measures of T. trichiura obtained by different authors and those provided in the present paper, since all the values overlapped within the range of defined measures. However, regarding males, Dinnik et al. (40) reported that the maximum total body length and the maximum length of the anterior part [total body length of adult worm (M1) and length of esophageal region of body (M2)] were slightly larger (52 and 34 mm, respectively). Furthermore, the maximum width of the posterior region of body (thickness) (M4), length of spicule (M8), and length of ejaculatory duct (M14) has also been reported as slightly higher by Skrjabin et al. (25) (M4 = 0.76 mm, M8 = 3.9 mm, and M14 = 3.9 mm). Regarding females, the maximum total body length of adult worm (M1) showed slightly higher values in the studies of Dinnik et al. (40), Skrjabin et al. (25), and Vigot-Fréres (39) (50, 52.7, and 50 mm, respectively). In addition, the maximum length of the esophageal region of body (M2) and the maximum body width in the place of junction of esophagus and the intestine (F5) were slightly higher (35.6 and 0.50 mm, respectively) in the data provided by Skrjabin et al. (25).
In addition, there are differences in the population of Trichuris sp. from chimpanzees showing a shorter size of the males and females collected from chimpanzees with respect to T. trichiura from H. sapiens, M. sylvanus, and P. papio. García-Sánchez et al. (21) who reported the differentiation of Trichuris species using a morphometric approach cited these results previously. The occurrence of different biometrical measurements in the same species may be explained by the phenotypic plasticity of these organisms themselves (8–11).
Morphological and biometric data for T. trichiura provided in the present study allow the differentiation of this species with respect to other species of Trichuris parasitizing NHP such as T. colobae (19) and T. ursinus (20). The typical T. trichiura spicule has a clear central part, which is not present in that of T. colobae and T. ursinus. Furthermore, the typical papilla group is only present in T. colobae (19). Females of T. trichiura and T. ursinus appear to have a non-protrusive vulva (20); nevertheless, females of T. colobae present a vulva like a crater with papillae (19). The vagina is very long and straight in T. ursinus (20) but appear with circumvolutions in T. trichiura.
With respect to the biometric characteristics, most of the measurements do not allow the specific differentiation between T. trichiura and other Trichuris spp. of NHP since these values overlapped for most of the measurements. However, males of T. trichiura have a smaller range of values in terms of distance from the end of the head to beginning of bacillary stripes and length of bacillary stripes (M6 and M7) compared to T. colobae, and values of length of minor bacillary stripes regarding T. ursinus. Regarding the specific differentiation of females based on biometric measurements, T. trichiura presents a lower range of values of length of bacillary stripes and distance of the tail end of the body and posterior fold of the seminal receptacle (F7 and F12) respect to T. colobae. In addition, the maximum and minimum values of distance of the vulva from the place of junction of the esophagus and the intestine were below than those observed in T. ursinus (19, 20).
From a molecular point of view, molecular markers which have been used by different authors to resolve species-level questions in Trichuris include the ITS1 and ITS2 nuclear regions (7, 44–53), 18S nuclear rRNA gene (38, 53, 54), mtDNA 16S rRNA gene (rrnL) (17, 50, 55), and protein-coding mitochondrial genes, including the 13 common genes obtained from the mitochondrial complete genome (12, 16, 56), cox1 mtDNA partial gene (17, 20, 38, 53, 55, 57), and cob mtDNA partial gene (17, 20, 55, 57, 58).
Different ITS rDNA types have been cited by different authors in some species of Trichuris (45–47). These sequence differences among ITS repeats in the rDNA array appeared to be a consequence of (intrachromosomal) mutational exchange during DNA replication (59). Thus, different reports suggest that different sequence types are most likely to be a result of base changes at certain positions in the sequence of a proportion of rDNA repeats because of mutational exchange during DNA replication, the extent of which appears to differ depending on the taxonomic group (60–63).
The results observed in ITS sequences revealed a percentage of similarity between 2a, 2b, 2c, and 2d subclades higher than those previously observed by other authors for species of the genus Trichuris (50, 57). Genetic analysis revealed that ITS regions were saturated and showed poor nucleotide diversity for clade 2 (T. trichiura). Thus, ITS sequences were not useful to infer the phylogenetic relationships between the different populations of T. trichiura (clade 2). On the other hand, some sequences from T. trichiura from Uganda and Cameroon (4) appeared within clade 1, suggesting that this population could be included on T. ursinus due to the high interpopulation similarity observed between both populations (Supplementary Table 4 and Figure 1). Therefore, the different genetic lineages within T. trichiura were delimited exclusively based on analysis of the mitochondrial genes in agreement with Chan et al. (64), who evaluated the utility of mitochondrial and ribosomal genes for molecular systematics of parasitic nematodes. ITS regions accumulated substitutions substantially more slowly than mtDNA and showed nucleotide saturation.
Studies of interpopulation similarity between the different 2a, 2b, 2c, and 2d subclades, based on the mitochondrial markers (cox1 and cob), revealed a range of similarity lower than those observed by ITS sequences, and similar to those observed among other clearly defined species such as for example T. suis and T. colobae, T. colobae, and T. ursinus [cited by present authors, Supplementary Tables 5, 6 and previously by Callejón et al. (20) and Rivero et al. (17)]. However, this similarity range showed higher values between rrnL sequences presenting a strong resolution of the different T. trichiura lineages (Supplementary Table 7). These results agree with those of Chan et al. (64), who evaluated the utility of mitochondrial ribosomal genes for molecular systematics of parasitic nematodes. These authors cited that 18S and 28S rRNA genes as well as 12S (rrnS) and 16S (rrnL) rRNA and cox1 genes showed a higher resolution for phylogenetic studies indicating that these five genes have potentially to be used as markers. Furthermore, they demonstrated that mitochondrial 12S and 16S genes present a resolution power at both lower and higher taxonomic levels for species and clade discrimination (64). For this reason and considering the similarity values observed in the different markers, we suggest that the populations of T. trichiura corresponding to the 2a, 2b, 2c, and 2d subclades correspond to different genetic lineages.
On the other hand, within clade 2 “T. trichiura lineage,” results based on rrnL revealed an interpopulation similarity of 83.80–86.31% between T. trichiura populations and Trichuris sp. from T. francoisi. Hence, we suggest that this species has a close relationship with T. trichiura. Our results agree with Liu et al. (12) who considered that Trichuris populations from T. francoisi are a new species of Trichuris.
The phylogeny inferred from mitochondrial datasets revealed the same topology of those based on rDNA with respect to the two main clades (clade 1 and clade 2). We were able to identify several genetically distinct subgroups (subclades) of whipworms, which were present in the sampled primates. The subclades 2b and 2c showed a broad host range and were not restricted to NHP species. However, the subclades 2a and 2d showed a higher host specificity corresponding with the T. trichiura population from M. sylvanus and H. sapiens (subclade 2a) and M. fuscata (subclade 2d) exclusively. In agreement to this study, similar results were observed on Trichuris sp. from M. fuscata (44, 55). This population also showed two potentially distinct entities of Trichuris present in two different subclades: subclade 2d [analogous to subclade MF reported by Cavallero et al. (44, 55)] and subclade 2c. These authors suggested the possibility of two different sources of infection for Japanese macaques corresponding with two Trichuris taxa, one potentially able to also infect humans.
In our study, all phylogenetic trees (partitioned and concatenated) reported the existence of two main clades, which has been previously reported (3, 7, 12, 17, 20, 33, 44, 55, 58). A clear differentiation between T. suis (clade 1) and T. trichiura (clade 2) can be confirmed according to our results. Within clade 2 “T. trichiura lineage,” T. trichiura can be also divided into 4 subclades, suggesting a complex of different genetic lineages. The analysis of the intraspecific similarity between the populations of T. trichiura from P. papio, C. aethiops, and E. patas from Spain revealed their highest value when compared with the populations of T. trichiura belonging to subclade 2c [previously described by Rivero et al. (17)] in all analyzed markers.
In addition to T. trichiura, a complex of Trichuris species showed infecting primates (Trichuris sp. from T. francoisi within clade 2 “T. trichiura lineage” and from C. aethiops from Italy and C. sabaeus from Czech Republic within clade 1 “T. suis lineage”). Different authors (17, 65) have previously reported the presence of several groups of T. trichiura. These results emphasize that the taxonomy and genetic variations of Trichuris are more complicated than previously acknowledged (65). Ravasi et al. (3) identified two distinct Trichuris genotypes that infect both humans and NHP. These authors identified “heterozygotes” confirming the identification of two distinct Trichuris genotypes in primates. On the other hand, Nissen et al. (14) identified “heterozygote” worms isolated from humans suggesting that T. trichiura might consist of several subspecies, some being found mainly in NHP. Ghai et al. (66) suggested that the Trichuris taxon should be considered a multi-host pathogen that is capable of infecting wild primates and humans. Finally, Betson et al. (5) reported that Trichuris infecting primates represents a complex of cryptic species with some species being able to infect both humans and NHP.
On the other hand, the existence of populations of Trichuris sp. associated with clade 1 “T. suis lineage” (based on all partitioned and concatenated markers) could indicate the possible origin of the change of host from NHP to pigs and, therefore, the origin of a new species, T. suis. This fact has been suggested by Hawash et al. (16) who found evidence of an African origin of T. trichiura that was later transmitted with human ancestors to Asia and then to South America. These authors suggested the possibility of a change of host to pigs in Asia where T. suis appears to have been transmitted globally by a combination of natural host dispersion and anthropogenic factors.
The present work examines the taxonomy, genetics, and phylogeny of T. trichiura parasitizing human and NHP and its relationship to Trichuris spp. from other NHP host species. A similar analysis was carried out on whipworms from humans (5). These authors suggested the existence of zoonotic transmission, especially regarding T. trichiura infections in NHP and, possibly, also for T. suis from pigs and Trichuris vulpis from dogs. In consequence, Trichuris may represent different species with the potential differences in endemicity, which may have important implications for implementing effective control strategies (5).
Several studies have reported that NHP represent an important reservoir for several known zoonotic infectious diseases (67–69). In this context, Trichuris infections have been found in a range of NHP species living in natural habitats including colobus monkeys, macaques, baboons, and chimpanzees (70–78). Based on molecular studies described above, some Trichuris species seem to be specific to a particular NHP, while others likely have the potential to circulate between humans and NHP, as they are genetically identical. This is particularly important when humans and NHP live in close proximity, as it is becoming increasingly common with human encroachment on pristine habitats and NHP accessing gardens and farms in search of food, and it has significant implications for human health and wildlife conservation (5).
The taxonomic, genetic, and phylogenetic results obtained in the present study confirm that T. trichiura exists as a complex (four subclades) with different host affinities and cross-infection capabilities corresponding with four different genetic lineages. Specifically, two of the four subclades show little host specificity and can develop trichuriasis in a wider variety of NHP species (C. sabaeus, C. aethiops, M. fuscata, M. sylvanus, E. patas, P. papio, P. hamadryas, Papio sp., and P. anubis) shared with humans. For this reason, we suggest the existence of a possible reservoir in the previously mentioned NHP species for human trichuriasis, which constitutes a serious public health risk (17). However, previous studies showed that the majority of the population of M. fuscata was included in a specific subclade [2d, subclade MF cited by Cavallero et al. (44, 55)] including only specimens from macaques.
Therefore, considering the following arguments for the taxonomic and phylogenetic study of populations of T. trichiura: (i) ITS regions were saturated and accumulated substitutions substantially slowly than mtDNA; therefore, they are not good genetic markers to delimit different genetic lineages. This is in agreement with previous studies where intraspecific variation was not observed when using nuclear DNA (64) suggesting that is not a useful genetic marker for intraspecies discrimination. (ii) The mitochondrial genes cox1, cob, and rrnL were not saturated, indicating that these three genes could have potential to be used as markers. Nevertheless, although cox1 presents the advantage for the extensive availability of database sequences, allowing for thorough comparisons of unknown sequences, cox1 sequences showed a high intraspecific variability which hinders resolution between closely related species. (iii) Nucleotide diversity showed that the rrnL gene present a lower intraspecific variability than cox1 and cob genes. However, rrnL interspecific genetic distance values allowed the phylogenetic resolution of the different T. trichiura subclades. Whence, rrnL was used successfully for inter-lineage discrimination of closely related populations within the T. trichiura lineage.
We suggest the utility of rrnL rRNA gene as a useful genetic marker for Trichuris species discrimination. Different authors who evaluated the utility of different mitochondrial genes for phylogenetic analyses (64, 79–81) have cited similar results. Future studies should focus on developing sequence databases for rRNA genes, which can serve as alternative genetic.
In conclusion, the present work provides an extensive study of biometric, morphological and molecular data for the unification of criteria that allows an update description of T. trichiura as well as a complex taxonomic, genetic, and phylogenetic study of the cited species applying multiple genetic markers to whipworm populations collected from humans and NHP from sympatric areas and worldwide locations. This study provides useful results for future studies aimed at the identification of new subspecies and hybridization events between existing species and enables a much clearer and more detailed understanding of dispersal patterns. This will reveal parasite transmission routes between these primates and will allow the implementation of appropriate control and prevention measures.
Data Availability Statement
The datasets presented in this study can be found in online repositories. The names of the repository/repositories and accession number(s) can be found in the article/Supplementary Material.
Author Contributions
JR, RC, and CC contribute conception, design of the study, and wrote the manuscript. All the authors contributed to the manuscript revision and read and approved the submitted version.
Funding
This work was financially supported by FEDER/Ministry of Science and Innovation, State Research Agency (CGL2017-83057). The Junta de Andalucía (BIO-338), and a grant from the V and VI Plan Propio de Investigación of the University of Seville, Spain.
Conflict of Interest
The authors declare that the research was conducted in the absence of any commercial or financial relationships that could be construed as a potential conflict of interest.
The handling Editor declared a past co-authorship with several of the authors CC and RC.
Acknowledgments
The authors thank Zoo Castellar (Cádiz, Spain) for providing samples of Trichuris sp. from M. sylvanus which naturally died. Further, we thank to Zoo Barcelona, Selwo Aventura (Málaga), Parque de la Naturaleza de Cabárceno (Cantabria) and Bioparc Fuengirola (Málaga) from Spain.
Supplementary Material
The Supplementary Material for this article can be found online at: https://www.frontiersin.org/articles/10.3389/fvets.2020.626120/full#supplementary-material
References
1. World Health Organization. Soil-transmitted helminth infections (2020). Available online at: https://www.who.int/es/news-room/fact-sheets/detail/soil-transmitted-helminth-infections (accessed March 2, 2020).
2. Mcdowell MA, Rafati S, editors. Neglected Tropical Diseases – Middle East and North Africa. Wien: Springer-Verlag Wien (2014). p. 281.
3. Ravasi DF, O'Riain MJ, Davids F, Illing N. Phylogenetic evidence that two distinct Trichuris genotypes infect both humans and non-human primates. PLoS ONE. (2012) 7:e44187. doi: 10.1371/journal.pone.0044187
4. Ghai RR, Chapman CA, Omeja PA, Davies TJ, Goldberg TL. Nodule worm infection in humans and wild primates in Uganda: cryptic species in a newly identified region of human transmission. PLoS Negl Trop Dis. (2014) 8:e2641. doi: 10.1371/journal.pntd.0002641
5. Betson M, Søe M, Nejsum P. Human trichuriasis: whipworm genetics, phylogeny, transmission and future research directions. Curr Trop Med Rep. (2015) 2:209–17. doi: 10.1007/s40475-015-0062-y
6. Ooi HK, Tenora F, Itoh K, Kamiya M. Comparative study of Trichuris trichiura from nonhuman primates and from man, and their differences with Trichuris suis. J Vet Med Sci. (1993) 55:363–6. doi: 10.1292/jvms.55.363
7. Cutillas C, Callejón R, De Rojas M, Tewes B, Úbeda JM, Ariza C, et al. Trichuris suis and Trichuris trichiura are different nematode species. Acta Trop. (2009) 111:299–307. doi: 10.1016/j.actatropica.2009.05.011
8. Knight RA. Morphological differences in Trichuris ovis associated with different host species. J Parasitol. (1984) 70:842–3.
9. Spakulová M. Discriminant analysis as a method for the numerical evaluation of taxonomic characters in male trichurid nematodes. Syst Parasitol. (1994) 29:113–9. doi: 10.1007/BF00009807
10. Robles MdR, Navone GT. Redescription of Trichuris laevitestis (Nematoda: trichuridae) from Akodon azarae and Scapteromys aquaticus (Sigmodontinae: Cricetidae) in Buenos Aires province, Argentina. J Parasitol. (2006) 92:1053–7. doi: 10.1645/GE-827R.1
11. Robles MdR. New species of Trichuris (Nematoda: Trichuridae) from Akodon montensis Thomas, 1913, of the Paranaense Forest in Argentina. J Parasitol. (2011) 97:319–27. doi: 10.1645/GE-2434.1
12. Liu GH, Gasser RB, Su A, Nejsum P, Peng L, Lin RQ, et al. Clear genetic distinctiveness between human-and pig-derived Trichuris based on analysis of mitochondrial datasets. PLoS Negl Trop Dis. (2012) 6:e1539. doi: 10.1371/journal.pntd.0001539
13. Liu GH, Zhou W, Nisbet AJ, Xu MJ, Zhou DH, Zhao GH, et al. Characterization of Trichuris trichiura from humans and T. suis from pigs in China using internal transcribed spacers of nuclear ribosomal DNA. J Helminthol. (2014) 88:64–8. doi: 10.1017/S0022149X12000740
14. Nissen S, Aljubury A, Hansen TV, Olsen A, Christensen H, Thamsborg SM, et al. Genetic analysis of Trichuris suis and Trichuris trichiura recovered from humans and pigs in a sympatric setting in Uganda. Vet Parasitol. (2012) 188:68–77. doi: 10.1016/j.vetpar.2012.03.004
15. Nadler S, De Leon G. Integrating molecular and morphological approaches for characterizing parasite cryptic species: implications for parasitology. Parasitology. (2011) 138:1688–709. doi: 10.1017/S003118201000168X
16. Hawash MB, Andersen LO, Gasser RB, Stensvold C, Nejsum P. Mitochondrial genome analyses suggest multiple Trichuris species in humans, baboons, and pigs from different geographical regions. PLoS Negl Trop Dis. (2015) 9:e0004059. doi: 10.1371/journal.pntd.0004059
17. Rivero J, García-Sánchez A, Zurita A, Cutillas C, Callejón R. Trichuris trichiura isolated from Macaca sylvanus: morphological, biometrical, and molecular study. BMC Vet Res. (2020) 16:445. doi: 10.1186/s12917-020-02661-4
18. Wang H, Zhang H, Song L, Zhu L, Chen M, Ren G, et al. Morphological and molecular confirmation of the validity of Trichuris rhinopiptheroxella in the endangered golden snub-nosed monkey (Rhinopithecus roxellana). J Helminthol. (2019) 93:601–7. doi: 10.1017/S0022149X18000500
19. Cutillas C, De Rojas M, Zurita A, Oliveros R, Callejón R. Trichuris colobae n. sp. (Nematoda: Trichuridae), a new species of Trichuris from Colobus guereza kikuyensis. Parasitol Res. (2014) 113:2725–32. doi: 10.1007/s00436-014-3933-6
20. Callejón R, Halajian A, Cutillas C. Description of a new species, Trichuris ursinus n. sp. (Nematoda: Trichuridae) from Papio ursinus Keer, 1792 from South Africa. Infect Genet Evol. (2017) 51:182–93. doi: 10.1016/j.meegid.2017.04.002
21. García-Sánchez ÁM, Rivero J, Zurita A, Callejón R, Cutillas C. Differentiation of Trichuris species using a morphometric approach. Int J Parasitol Parasites Wildl. (2019) 9:218–23. doi: 10.1016/j.ijppaw.2019.05.012
22. Gupta SK, Singla LD. Veterinary diagnostics. In: Gupta RP, Garg SR, Nehra V, Lather D, editors. Chapter 6: Diagnostic Trends in Parasitic Diseases of Animals. Delhi: Statish Serial Publishing House (2013). p. 81–112.
23. Horii Y, Usui M. Experimental transmission of Trichuris ova from monkeys to man. Trans R Soc Trop Med Hyg. (1985) 79:423. doi: 10.1016/0035-9203(85)90403-1
24. Oliveros R, Cutillas C, Aris P, Guevara D. Morphologic, biometric and isoenzyme characterization of Trichuris suis. Parasitol Res. (1998) 84:513–5. doi: 10.1007/s004360050438
25. Skrjabin KI, Shikhobalova NP, Orlow IV. Essentials of Nematodology, vol. VI. In: Greenberg D, editors. Translated by Birron A. Trichocephalidae and Capillariidae of animals and the man and the diseases caused by them. Jerusalem: Keter Press Wiener Binder Ltd. (1957).
26. Spakulová M, Lýsek H. A biometric study of two populations of Trichocephalus suis Schrank, 1788 from swine and wild boars. Helminthologia. (1981) 18:91–8.
27. Suriano DM, Navone GT. Three new species of the genus Trichuris Roederer, 1761 (Nematoda: Trichuridae) from Cricetidae and Octodontidae rodents in Argentina. Res Re Parasitol. (1994) 54:39–46.
28. Robles MdR, Navone GT, Notarnicola J. A new species of Trichuris (Nematoda: Trichuridae) from Phyllotini rodents in Argentina. J Parasitol. (2006) 92:100–4. doi: 10.1645/GE-GE-552R.1
29. Kumar S, Stecher G, Tamura K. MEGA7: molecular evolutionary genetics analysis version 7.0 for bigger datasets. Mol Biol Evol. (2016) 33:1870–4. doi: 10.1093/molbev/msw054
30. Xia X, Xie Z, Salemi M, Chen L, Wang Y. An index of substitution saturation and its application. Mol Phylogenetics Evol. (2003) 26:1–7. doi: 10.1016/s1055-7903(02)00326-3
31. Xia X, Lemey P. Assessing substitution saturation with DAMBE. 2nd ed. In: Lemey P, Salemi M, Vandamme AM, editors. The Phylogenetic Handbook: A Practical Approach to DNA and Protein Phylogeny. Oxford: Cambridge University Press. (2009). p. 615–30.
32. Rozas J, Ferrer-Mata A, Sánchez-DelBarrio JC, Guirao-Rico S, Librado P, Ramos-Onsins SE, et al. DnaSP v6: DNA sequence polymorphism analysis of large datasets. Mol Biol Evol. (2017) 34:3299–302. doi: 10.1093/molbev/msx248
33. Guindon S, Gascuel O. A simple, fast, and accurate algorithm to estimate large phylogenies by maximum likelihood. Syst Biol. (2003) 52:696–704. doi: 10.1080/10635150390235520
34. Ronquist F, Huelsenbeck JP. MrBAYES 3: Bayesian phylogenetic inference under mixed models. Bioinformatics. (2003) 19:1572–4. doi: 10.1093/bioinformatics/btg180
35. Posada D. jModelTest: phylogenetic model averaging. Mol Biol Evol. (2008) 25:1253–6. doi: 10.1093/molbev/msn083
36. Posada D, Buckley TR. Model selection and model averaging in phylogenetics: advantages of akaike information criterion and bayesian approaches over likelihood ratio tests. Syst Biol. (2004) 53:793–808. doi: 10.1080/10635150490522304
37. Felsenstein J. Confidence limits on phylogenies: an approach using the bootstrap. Evolution. (1985) 39:783–91. doi: 10.1111/j.1558-5646.1985.tb00420.x
38. Callejón R, Nadler S, De Rojas M, Zurita A, Petrášová J, Cutillas C. Molecular characterization and phylogeny of whipworm nematodes inferred from DNA sequences of cox1 mtDNA and 18S rDNA. Parasitol Res. (2013) 112:3933–49. doi: 10.1007/s00436-013-3584-z
39. Frères V, Neveu-Lemaire M. Traité d'helminthologie médicale et vétérinaire. (1936) S1–16:641–2. doi: 10.4269/ajtmh.1936.s1-16.641
41. Zaman V. Scanning electron microscopy of Trichuris trichiura (Nematoda). Acta Trop. (1984) 41:287–92.
42. Tenora F, Hovorka I, Hejlkova D. A supplement to the scanning electron microscopy of some Trichocephalus spp. (Nematoda). Helminthologia. (1988) 25:227–34.
43. Tenora F, Stanek M, Spakulová M. On the possibility of morphological differentiation of Trichocephalus trichiurus (L., 1771) and T. suis (Schrank, 1788) species. In: Conference Aktuálne otázky humánnej parazitológie. (1992).
44. Cavallero S, De Liberato C, Friedrich KG, Di Cave D, Masella V, D'Amelio S, et al. Genetic heterogeneity and phylogeny of Trichuris spp. from captive non-human primates based on ribosomal DNA sequence data. Infect Genet Evol. (2015) 34:450–6. doi: 10.1016/j.meegid.2015.06.009
45. Oliveros R, Cutillas C, de Rojas M, Arias P. Characterazition of four species of Trichuris (Nematoda: Enoplida) by their second internal transcribed spacer ribosomal DNA sequence. Parasitol Res. (2000) 86:1008–13. doi: 10.1007/pl00008519
46. Cutillas C, Oliveros R, De Rojas M, Guevara DC. Determination of Trichuris muris from murid hosts and Trichuris arvicolae (Nematoda) from arvicolid rodents by amplification and sequentiation of the ITS1-5.8S-ITS2 segment of the ribosomal DNA. Parasitol Res. (2002) 88:574–82. doi: 10.1007/s00436-002-0596-5
47. Cutillas C, Oliveros R, De Rojas M, Guevara DC. Determination of Trichuris skrjabini by sequencing of the ITS1-5.8S-ITS2 segment of the ribosomal DNA: comparative molecular study of different species of trichurids. J Parasitol. (2004) 90:648–52. doi: 10.1645/GE-3295RN
48. Cutillas C, De Rojas M, Ariza C, Úbeda JM, Guevara DC. Molecular identification of Trichuris vulpis and Trichuris suis isolated from different hosts. Parasitol Res. (2007) 100:383–9. doi: 10.1007/s00436-006-0275-z
49. Callejón R, Gutiérrez-Avilés L, Halajian A, Zurita A, de Rojas M, Cutillas C. Taxonomy and phylogeny of Trichuris globulosa Von Linstow, 1901 from camels. A review of Trichuris species parasitizing herbivorous. Infect Genet Evol. (2015) 34:61–74. doi: 10.1016/j.meegid.2015.06.011
50. Callejón R, Halajian A, De Rojas M, Marrugal A, Guevara DC, Cutillas C. 16S partial gene DNA and internal transcribed spacers ribosomal DNA as differential markers of Trichuris discolor populations. Vet Parasitol. (2012) 186:350–63. doi: 10.1016/j.vetpar.2011.11.033
51. Salaba O, Rylková K, Vadlejch J, Petrtýl M, Schánková Š, Brožová A, et al. The first determination of Trichuris sp. from roe deer by amplification and sequentiation of the ITS1-5.8S-ITS2 segment of ribosomal DNA. Parasitol Res. (2013) 112:955–60. doi: 10.1007/s00436-012-3215-0
52. Robles MdR, Cutillas C, Panei CJ, Callejón R. Morphological and molecular characterization of a new Trichuris species (Nematoda-Trichuridae), and phylogenetic relationships of Trichuris species of cricetid rodents from Argentina. PLoS ONE. (2014) 9:e112069. doi: 10.1371/journal.pone.0112069
53. DoleŽalová J, Oborník M, Hajdušková E, Jirku M, PetrŽelková KJ, Bolechová P, et al. How many species of whipworms do we share? Whipworms from man and other primates form two phylogenetic lineages. Folia Parasitol (Praha). (2015) 62:1–12. doi: 10.14411/fp.2015.063
54. Guardone L, Deplazes P, Macchioni F, Magi M, Mathis A. Ribosomal and mitochondrial DNA analysis of Trichuridae nematodes of carnivores and small mammals. Vet Parasitol. (2013) 197:364–9. doi: 10.1016/j.vetpar.2013.06.022
55. Cavallero S, Nejsum P, Cutillas C, Callejón R, DoleŽalová J, Modrý D, et al. Insights into the molecular systematics of Trichuris infecting captive primates based on mitochondrial DNA analysis. Vet Parasitol. (2019) 272:23–30. doi: 10.1016/j.vetpar.2019.06.019
56. Liu GH, Gasser RB, Nejsum P, Wang Y, Chen Q, Song HQ, et al. Mitochondrial and nuclear ribosomal DNA evidence supports the existence of a new Trichuris species in the endangered françois' leaf-monkey. PLoS ONE. (2013) 8:e66249. doi: 10.1371/journal.pone.0066249
57. Callejón R, Robles MdR, Panei CJ, Cutillas C. Molecular diversification of Trichuris spp. from Sigmodontinae (Cricetidae) rodents from Argentina based on mitochondrial DNA sequences. Parasitol Res. (2016) 115:2933–45. doi: 10.1007/s00436-016-5045-y
58. Callejón R, Cutillas C, Nadler SA. Nuclear and mitochondrial genes for inferring Trichuris phylogeny. Parasitol Res. (2015) 114:4591–9. doi: 10.1007/s00436-015-4705-7
59. Schlötterer C, Tautz D. Chromosomal homogeneity of Drosophila ribosomal DNA arrays suggests intrachromosomal exchanges drive concerted evolution. Curr Biol. (1994) 4:777–83. doi: 10.1016/s0960-9822(00)00175-5
60. Wesson DM, Porter CH, Collins FH. Sequence and secondary structure comparisons of ITS rDNA in mosquitoes (Diptera: Culicidae). Mol Phylogenet Evol. (1992) 1:253–69. doi: 10.1016/1055-7903(92)90001-w
61. Campbell AJ, Gasser RB, Chilton NB. Differences in a ribosomal DNA sequence of Strongylus species allows identification of single eggs. Int J Parasitol. (1995) 25:359–65. doi: 10.1016/0020-7519(94)00116-6
62. Hoste H, Chilton NB, Gasser RB, Beveridge I. Differences in the second internal transcribed spacer (ribosomal DNA) between five species of Trichostrongylus (Nematoda: Trichostrongylidae). Int J Parasitol. (1995) 25:75–80. doi: 10.1016/0020-7519(94)00085-3
63. Gasser RB, Stewart LE, Speare R. Genetic markers in ribosomal DNA for hookworm identification. Acta Trop. (1996) 62:15–21. doi: 10.1016/s0001-706x(96)00015-0
64. Chan AHE, Chaisiri K, Morand S, Saralamba N, Thaenkham U. Evaluation and utility of mitochondrial ribosomal genes for molecular systematics of parasitic nematodes. Parasit Vectors. (2020) 13:364. doi: 10.1186/s13071-020-04242-8
65. Xie Y, Zhao B, Hoberg EP, Li M, Zhou X, Gu X, et al. Genetic characterisation and phylogenetic status of whipworms (Trichuris spp.) from captive non-human primates in China, determined by nuclear and mitochondrial sequencing. Parasit Vectors. (2018) 11:516. doi: 10.1186/s13071-018-3100-5
66. Ghai RR, Simons ND, Chapman CA, Omeja PA, Davies TJ, Ting N, et al. Hidden population structure and cross-species transmission of whipworms (Trichuris sp.) in humans and non-human primates in Uganda. PLoS Negl Trop Dis. (2014) 8:e3256. doi: 10.1371/journal.pntd.0003256
67. Geerts S, Gryseels B. Anthelmintic resistance in human helminths: a review. Tropical Med Int Health. (2001) 6:915–21. doi: 10.1046/j.1365-3156.2001.00774.x
68. World Health Organization. Helminth control in school-age children. A guide for managers of control programmes. Available online at: https://apps.who.int/iris/bitstream/handle/10665/44671/9789241548267_eng.pdf?sequence=1 (2011) (accessed September, 2011).
69. Global Burden of Disease Study 2013 Collaborators. Global, regional, and national incidence, prevalence, and years lived with disability for 301 acute and chronic diseases and injuries in 188 countries, 1990–2013: a systematic analysis for the Global Burden of Disease Study. Lancet. (2015) 386:743–800. doi: 10.1016/S0140-6736(15)60692-4
70. Arizono N, Yamada M, Tegoshi T, Onishi K. Molecular identification of Oesophagostomum and Trichuris eggs isolated from wild Japanese macaques. Korean J Parasitol. (2012) 50:253–7. doi: 10.3347/kjp.2012.50.3.253
71. Gillespie TR, Lonsdorf EV, Canfield EP, Meyer DJ, Nadler Y, Raphael J, et al. Demographic and ecological effects on patterns of parasitism in eastern chimpanzees (Pan troglodytes schweinfurthii) in Gombe National Park, Tanzania. Am J Phys Anthropol. (2010) 143:534–44. doi: 10.1002/ajpa.21348
72. Kooriyama T, Hasegawa H, Shimozuru M, Tsubota T, Nishida T, Iwaki T. Parasitology of five primates in Mahale Mountains National Park Tanzania. Primates. (2012) 53:365–75. doi: 10.1007/s10329-012-0311-9
73. Levecke B, Dorny P, Vercammen F, Visser LG, Van EM, Vercruysse J, et al. Transmission of Entamoeba nuttalli and Trichuris trichiura from non-human primates to humans. Emerg Infect Dis. (2015) 21:1871–2. doi: 10.3201/eid2110.141456
74. Li M, Zhao B, Li B, Wang Q, Niu L, Deng J, et al. Prevalence of gastrointestinal parasites in captive non-human primates of twenty-four zoological gardens in China. J Med Primatol. (2015) 44:168–73. doi: 10.1111/jmp.12170
75. Muriuki SMK, Murugu RK, Munene E, Karere GM, Chai DC. Some gastro-intestinal parasites of zoonotic (public health) importance commonly observed in old world non-human primates in Kenya. Acta Trop. (1998) 71:73–82. doi: 10.1016/s0001-706x(98)00040-0
76. Pourrut X, Diffo JLD, Somo RM, Bilong Bilong CF, Delaporte E, LeBreton M, et al. Prevalence of gastrointestinal parasites in primate bushmeat and pets in Cameroon. Vet Parasitol. (2011) 175:187–91. doi: 10.1016/j.vetpar.2010.09.023
77. Yao C, Walkush J, Shim D, Cruz K, Ketzis J. Molecular species identification of Trichuris trichiura in African green monkey on St. Kitts, West Indies. Vet Parasitol Reg Stud Reports. (2018) 11:22–6. doi: 10.1016/j.vprsr.2017.11.004
78. Zanzani SA, Gazzonis AL, Epis S, Manfredi MT. Study of the gastrointestinal parasitic fauna of captive non-human primates (Macaca fascicularis). Parasitol Res. (2016) 115:307–12. doi: 10.1007/s00436-015-4748-9
79. Medina M, Walsh PJ. Molecular systematics of the order Anaspidea based on mitochondrial DNA sequence (12S, 16S, and COI). Mol Phylogenet Evol. (2000) 15:41–58. doi: 10.1006/mpev.1999.0736
80. Paternina LE, Verbel Vergara D, Bejarano EE. Comparación y utilidad de las regiones mitocondriales de los genes 16S y COX1 para los análisis genéticos en garrapatas (Acari: Ixodidae). Biomedica. (2016) 36:295–302. doi: 10.7705/biomedica.v36i2.3116
81. Chan AHE, Chaisiri K, Dusitsittipon S, Jakkul W, Charoennitiwat V, Komalamisra C, et al. Mitochondrial ribosomal genes as novel genetic markers for discrimination of closely related species in the Angiostrongylus cantonensis lineage. Acta Trop. (2020) 211:105645. doi: 10.1016/j.actatropica.2020.105645
Keywords: Trichuris trichiura, non-human primates, ribosomal DNA, mitochondrial DNA, zoonoses
Citation: Rivero J, Cutillas C and Callejón R (2021) Trichuris trichiura (Linnaeus, 1771) From Human and Non-human Primates: Morphology, Biometry, Host Specificity, Molecular Characterization, and Phylogeny. Front. Vet. Sci. 7:626120. doi: 10.3389/fvets.2020.626120
Received: 04 November 2020; Accepted: 31 December 2020;
Published: 09 February 2021.
Edited by:
Serena Cavallero, Sapienza University of Rome, ItalyReviewed by:
Viliam Šnábel, Institute of Parasitology (SAS), SlovakiaChristina Strube, University of Veterinary Medicine Hannover, Germany
Copyright © 2021 Rivero, Cutillas and Callejón. This is an open-access article distributed under the terms of the Creative Commons Attribution License (CC BY). The use, distribution or reproduction in other forums is permitted, provided the original author(s) and the copyright owner(s) are credited and that the original publication in this journal is cited, in accordance with accepted academic practice. No use, distribution or reproduction is permitted which does not comply with these terms.
*Correspondence: Cristina Cutillas, cutillas@us.es