Interleukin-1β in tendon injury enhances reparative gene and protein expression in mesenchymal stem cells
- 1Department of Clinical Sciences, College of Veterinary Medicine, North Carolina State University, Raleigh, NC, United States
- 2Comparative Medicine Institute, North Carolina State University, Raleigh, NC, United States
- 3Department of Molecular Biomedical Sciences, College of Veterinary Medicine, North Carolina State University, Raleigh, NC, United States
Tendon injury in the horse carries a high morbidity and monetary burden. Despite appropriate therapy, reinjury is estimated to occur in 50–65% of cases. Although intralesional mesenchymal stem cell (MSC) therapy has improved tissue architecture and reinjury rates, the mechanisms by which they promote repair are still being investigated. Additionally, reevaluating our application of MSCs in tendon injury is necessary given recent evidence that suggests MSCs exposed to inflammation (deemed MSC licensing) have an enhanced reparative effect. However, applying MSC therapy in this context is limited by the inadequate quantification of the temporal cytokine profile in tendon injury, which hinders our ability to administer MSCs into an environment that could potentiate their effect. Therefore, the objectives of this study were to define the temporal cytokine microenvironment in a surgically induced model of equine tendon injury using ultrafiltration probes and subsequently evaluate changes in MSC gene and protein expression following in vitro inflammatory licensing with cytokines of similar concentration as identified in vivo. In our in vivo surgically induced tendon injury model, IL-1β and IL-6 were the predominant pro-inflammatory cytokines present in tendon ultrafiltrate where a discrete peak in cytokine concentration occurred within 48 h following injury. Thereafter, MSCs were licensed in vitro with IL-1β and IL-6 at a concentration identified from the in vivo study; however, only IL-1β induced upregulation of multiple genes beneficial to tendon healing as identified by RNA-sequencing. Specifically, vascular development, ECM synthesis and remodeling, chemokine and growth factor function alteration, and immunomodulation and tissue reparative genes were significantly upregulated. A significant increase in the protein expression of IL-6, VEGF, and PGE2 was confirmed in IL-1β-licensed MSCs compared to naïve MSCs. This study improves our knowledge of the temporal tendon cytokine microenvironment following injury, which could be beneficial for the development and determining optimal timing of administration of regenerative therapies. Furthermore, these data support the need to further study the benefit of MSCs administered within the inflamed tendon microenvironment or exogenously licensed with IL-1β in vitro prior to treatment as licensed MSCs could enhance their therapeutic benefit in the healing tendon.
Introduction
Tendon injury in the horse, most commonly affecting the superficial digital flexor tendon (SDFT), has a high morbidity and monetary burden associated with treatment, rehabilitation, and lost athletic performance (1, 2). Despite appropriate therapy, high reinjury rates are estimated to occur in 50–65% of cases (1–3). In the last 20 years, implementation of mesenchymal stem cell (MSC) therapy has secured a prominent position in treating a variety of musculoskeletal injuries. Specifically, intralesional administration of MSCs in horses has improved SDFT architecture in experimental settings and reduced reinjury rate in clinical cases (3, 4). Despite these improved outcomes following MSC therapy, new insights into MSC biology have created a paradigm shift. It is now believed that the effect of MSCs is not due to local engraftment and differentiation, but instead from secretion of bioactive paracrine factors commonly referred as the secretome (5, 6). Particularly, data have shown that inflammatory molecules stimulate, or license, MSCs to enhance their secretion of reparative and immunomodulatory factors to a greater degree than in their naïve, or unlicensed, state (7–10). While this has translated to increased research in licensed MSCs for other inflammatory diseases, minimal work has examined inflammatory licensed MSCs for the treatment of tendon injuries (11).
Understanding the tissue microenvironment during injury guides our ability to develop new therapies to combat detrimental molecular mechanisms and encourage local reparative factors to improve healing. However, as recently reviewed (12), our knowledge of the temporal cytokine profile following tendon injury is limited due, in part, to the difficulty in obtaining adequate antemortem samples without compromising tendon structure and function. Our current understanding of the tendon microenvironment following injury has relied upon simplified models of injury in cell culture, intermittent post-mortem analyses in terminal animal disease models, or evaluation of human clinical patients with naturally occurring, but non-standardized tendon injuries (13, 14). Therefore, more thoroughly determining the temporal change in the cytokine profile in a standardized tendon injury model would advance our knowledge of the molecular mechanisms that drive healing and disease, which could subsequently advance our ability to develop more effective therapies for tendon injury. Furthermore, examining how this inflammatory microenvironment affects MSCs and their secreted factors in the context of MSC licensing has the potential to advance MSC therapy for tendon injuries.
The objectives of this study were 2-fold: (1) define the in vivo temporal cytokine profile of the tendon microenvironment using a novel ultrafiltration probe implanted into a surgically induced tendon lesion in the horse; and (2) determine how the predominant inflammatory cytokines identified in (author?) (1) affect the MSC gene and protein expression in vitro. We hypothesized that the use of a novel ultrafiltration probe would allow us to define the temporal cytokine profile in a surgically induced model of tendon injury. Further, we hypothesized that licensing of MSCs separately with IL-1β or IL-6 at the concentration identified in the in vivo tendon microenvironment would enhance tendon-beneficial gene and protein expression.
Materials and methods
Animal use and welfare
Seven Thoroughbred horses (age median 5 years, range 4–13 years; median weight 490 kg, 405–513 kg), free of clinical lameness and palpable or ultrasonographically evident tendon injury were enrolled, and underwent surgical induction of a core lesion of both forelimb SDFTs in an experimental protocol approved by the Institutional Animal Care and Use Committee of North Carolina State University (Protocol #20-389). For the entire study period, horses were housed in an individual 12 × 12 foot concrete stall with rubber floor mats with bedding and provided water and grass hay ad libitum in a temperature-controlled facility. Preoperative complete blood count and serum chemistry were performed prior to surgery and weekly thereafter.
Ultrafiltration probe development and surgical technique
The ultrafiltration probes used were a modified version of a commercially available probe (BASi Research Products, West Layfayette, IN, USA) used for pharmacokinetic and pharmacodynamic (PK/PD) modeling studies. These probes consist of three loops of membrane with a 30 kDa molecular weight cutoff, joined to a single, non-permeable conducting tube (15, 16). Our group, with assistance from BASi Research Products, performed both ex vivo and in vivo pilot studies to custom design a modified ultrafiltration probe with a slimmer profile consisting of a single loop of membrane with a 100 kDa molecular weight cutoff to ensure collection of larger cytokines like TNF-α (17).
Horses were anesthetized, moved to an operating theater, and maintained with general inhalant anesthesia. Core lesions were created in the extrasynovial portion of both forelimb SDFTs of seven horses using a previously published method shown to induce consistent injury with a similar ultrasonographic and histologic progression encountered in naturally occurring equine SDFT injury (18). Briefly, following aseptic preparation, local anesthesia with bupivacaine liposome injectable suspension (Nocita®, Elanco Animal Health, Inc., Greenfield, IN) of the medial and lateral palmar metacarpal nerves and medial and lateral metacarpal nerves was performed immediately distal to the head of the second and fourth metacarpal bones and tendon lesions induced under ultrasonographic guidance as previously described. Thereafter, a custom-designed 100 kDa ultrafiltration probe (BASi Research Products, West Lafayette, IN, USA) was threaded retrograde through a 12-gauge venous catheter (Zoetis, Inc., Kalamazoo, MI, USA) to seat the probe entirely within the lumen (Figures 1A,B). The probe-venous catheter was then inserted within the lumen of an ultrafiltration probe introducer needle (BASi Research Products, West Lafayette, IN, USA, Figure 1C). Using this method, the function of the introducer needle was placement of the ultrafiltration probe within the lesion while the venous catheter provided support at the probe base to ensure it was maintained within the tendon when the introducer needle was removed. Following placement of ultrafiltration probes in six horses, the catheter and introducer needle were removed, and probe location confirmed with ultrasonography (Figures 1D–G). A single horse underwent creation of bilateral SDFT lesions without placement of ultrafiltration probes to serve as control. Skin incisions of all horses were closed with 2-0 polypropylene in a simple interrupted pattern, the limbs bandaged standardly, and horses recovered from general inhalant anesthesia.
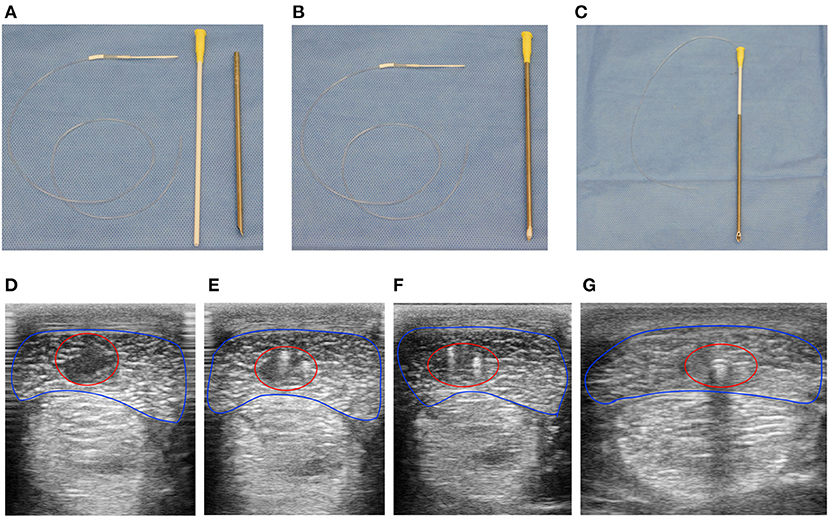
Figure 1. Ultrafiltration probes were easily placed under ultrasound guidance within the center of surgically induced equine SDFT lesions. Instruments required to place ultrafiltration probes within surgically induced lesions of the SDFT. (A) Ultrafiltration probe (left), venous catheter (middle), and Basi® introducer needle (right). The venous catheter was threaded normograde into the introducer needle (B), followed by retrograde threading of the ultrafiltration probe into the venous catheter-introducer needle (C). Using ultrasound guidance (D–G), the device facilitated placement of the ultrafiltration probe within tendon lesions (red circle) of the SDFT (blue outline) following surgical induction. At the most proximal extent (D) a large hypoechoic lesion with loss of tendon fiber is visualized. Moving distally, the two fibers of the ultrafiltration probe loop can be visualized as hyperechoic foci within the hypoechoic lesion (E,F). Finally, acoustic shadowing due to gas within the lumen of the conducting tubing (G) was observed at the most distal extent of the tendon lesion proximal to tube exit from the tendon and skin.
Horses received prophylactic gentamicin sulfate (6.6 mg/kg, q 24 h, IV) and penicillin G potassium (22,000 IU/kg, q 6 h, IV) prior to surgery and for 3 days after. Postoperative analgesia was provided by morphine sulfate (0.05–0.1 mg/kg, q 12–24 h, IM) for 2–3 days depending on comfort. Non-steroidal anti-inflammatory drugs (NSAIDs) were purposefully avoided to prevent alteration of the tendon cytokine profile. Following recovery from general anesthesia, each limb was unbandaged, a sterile evacuated tube containing protease inhibitors (BD, Franklin Lakes, NJ) attached to the needle and conducting tube system of the ultrafiltration probe, and the limb rebandaged. Tendon ultrafiltrate was collected from evacuated tubes within the first 3–5 h following surgery and a new tube replaced and changed every 12 h thereafter. Samples were aliquoted into cryovials immediately following collection and stored at −80°C until analysis within 4 months following collection. Ultrasound-guided percutaneous aspiration of lesions of the single control horse was attempted on day one, three, seven, and ten postoperatively and samples aliquoted and stored as with tendon ultrafiltrate. Horses were hand walked for five (week one), ten (week two), and fifteen (week three) minutes twice daily until the conclusion of the study. At 21 days following surgery, horses were sedated and euthanized with an intravenous overdose of sodium pentobarbital. Tendon from a representative limb was collected for histopathology in two horses.
Cytokine characterization
Cytokine concentration for equine-specific interleukin-1β (IL-1β),−6,−8,−10, fibroblast growth factor-2 (FGF-2), tumor necrosis factor-α (TNF-α), interferon-γ (IFN-γ), interferon inducible protein-10 (IP-10), transforming growth factor-β1 (TGF-β1),−2, and−3 were measured in tendon ultrafiltrate with a fluorescent bead-based multiplex assay (MILLIPLEX, Equine Multiplex Assay, MilleporeSigma, MA, USA) and performed according to manufacturer's instructions. For the multiplex assay, concentrations measured below the lower limit of quantification (LLoQ) were assigned a value of 0.1 of the LLoQ. Prostaglandin E2 (PGE2) was determined using a colorimetric competitive ELISA according to manufacturer's instructions (Enzo Life Sciences, Inc., Farmingdale, NY, USA). All samples were analyzed in duplicate. Tendon ultrafiltrate from later timepoints was pooled for TGF-β and PGE2 analysis due to small sample volume.
RNA sequencing of licensed MSCs
Banked equine bone marrow-derived MSCs (n = 6, donor horse age median 9 years, range 6–12 years) were utilized for this study and were isolated as previously described (19). MSCs were thawed and seeded in complete MSC media (19) containing 10% fetal bovine serum (FBS) and when cultures reached 80% confluency, MSCs were passaged with Accutase cell-dissociation solution (Innovative Cell Technologies) and 0.35 × 106 cells plated on 100 mm culture plates for RNA sequencing experiments. MSCs were passaged 2–3 times prior to use. Twenty-four hours following passage and adherence, cells were licensed by adding complete MSC media containing 2 ng/ml recombinant human IL-1β (R&D Systems) or 2 ng/ml recombinant human IL-6 (BioLegend) for 72 h with media exchanged at 48 h. After 72 h, cells were washed and lifted in preparation for RNA-sequencing.
Total RNA was extracted from paired P3 or P4 naïve, IL-1β licensed, and IL-6 licensed MSCs from six donor horses using the RNeasy Mini kit (Qiagen). Libraries were generated and poly(A) enriched using 1 μg of RNA as input. Indexed samples were sequenced using a 150 base pair paired-end protocol on a HiSeq 2500 (Illumina) according to the manufacturer's protocol. Sequence reads were trimmed to remove possible adaptor sequences and nucleotides with poor quality using Trimmomatic v.0.36. The trimmed reads were mapped to the EquCab 3.0 reference genome available on ENSEMBL using the STAR aligner v2.5.2b. Unique gene hit counts were calculated using featureCounts from the Subread package v1.5.2. Using DESeq2, a comparison of gene expression between the naive and IL-1β or IL-6 licensed MSCs was performed. The Wald test was used to generate p-values and log2 fold changes. Genes with an adjusted p-value of <0.05 and log2 fold change >1 were determined to be differentially expressed for each comparison. The quantification and poly(A) selection of mRNA, library preparation, sequencing, and bioinformatics were outsourced to GENEWIZ, Inc.
MSC protein expression
Supernatants from paired naïve and licensed MSCs (n = 4, donor horse age median 17 years, range 12–23 years) were collected, spun at 500xg for 10 min to remove cellular debris, and stored at −80°C until analysis. Protein expression of IL-1β licensed MSCs were evaluated with ELISA assays for equine IL-6 (R&D Systems, Minneapolis, MN, USA), human PGE2 (Enzo Biochem, Inc., Farmingdale, NY, USA), and equine VEGF (Kingfisher Biotech, Saint Paul, MN, USA) and performed per manufacturer's instructions. For PGE2 analysis, supernatant from IL-1β licensed MSCs was diluted 1:100 in reagent diluent.
Statistical analysis
Tendon ultrafiltrate volumes were assessed for normal distribution by Shapiro-Wilk test. Descriptive analysis was performed for both tendon ultrafiltrate volume and cytokine concentration. Normally distributed data was reported as mean ± standard deviation while non-parametric data reported with median and interquartile range. A one-tailed paired t-test was used to examine difference in protein expression for ELISA assays. All analysis and figure preparation were performed using GraphPad Prism (GraphPad Software Inc; La Jolla, CA, USA). The functional enrichment analysis of RNA-sequencing data was performed using g:Profiler (version e104_eg51_p15_3922dba) with g:SCS multiple testing correction method applying significance threshold of 0.05.
Results
Animals and surgical implantation
An ultrafiltration probe was implanted in each core lesion of six horses. A single horse did not have ultrafiltration probes implanted to serve as control. All horses recovered from general anesthesia without incident and remained comfortable through the entirety of the 21-day study. Complete blood count and serum chemistry for all horses were unremarkable for the duration of the study. Ultrafiltration probes were easy to place within surgically induced lesions; however, maintenance of ultrafiltrate flow following surgery was more difficult as early ultrafiltrate had the tendency to slow or fully obstruct flow at the lumen of the needle connecting the conducting tubing to the evacuated tubes. However, once a new needle was exchanged, collection of tendon ultrafiltrate into the evacuated tubes resumed.
Cytokines in tendon microenvironment
Tendon ultrafiltrate was collected from the majority of ultrafiltration probes throughout the 21-day period; however, volume and frequency of collection varied between horses and between limbs of the same horse throughout (Supplementary Table 1). No significant difference was found in total ultrafiltrate volume collected over the 21-day period between individual horses and was normally distributed (4.411 ± 2.325 ml; p = 0.944).
Overall, pro-inflammatory cytokines peaked within the first 24–48 h (Figure 2A). IL-1β at 48 h (median 2,097 pg/ml, 668–5,505 pg/ml), IL-6 at 24 h (median 1,971 pg/ml, 1,114–2,475 pg/ml), and IL-8 at 24 h (median 110 pg/ml, 96–120 pg/ml). Quantifiable levels of IFN-γ, TNF-α, IP-10, IL-10 were not detected. More acutely, the highest levels of FGF-2 were identified within the first sampling period before 12 h (median 1,315 pg/ml, 605–1,423 pg/ml, Figure 2B). TGF-β isoforms were detected at varying times throughout the 21-day period (Figure 2B). TGF-β1 was measured in tendon ultrafiltrate throughout with a biphasic peak at 24 h (median 285 pg/ml, 221–314 pg/ml) and days 11–14 (median 344 pg/ml, 23.0–885 pg/ml). TGF-β2 levels peaked at day 3 (194 pg/ml, 5.4–399 pg/ml) and remained similar through the first week before declining over the final 10 days of the study. Finally, TGF-β3 was below the lower limit of detection except for on days 8–10 and at its peak at days 11–14 (median 85.4 pg/ml, 3.60–249 pg/ml). The concentration of PGE2 was initially measured in two horses and was below quantifiable limits in samples prior to day 15–18. Due to concerns about volume remaining for other assays, further characterization of PGE2 was abandoned. Only small volumes of hemorrhagic sample were obtained during attempts at ultrasound-guided percutaneous aspiration of the control horse lesions. Most cytokines measured from samples were below quantifiable level. This along with concern regarding peripheral blood contamination prevented analysis of samples from the single control horse.
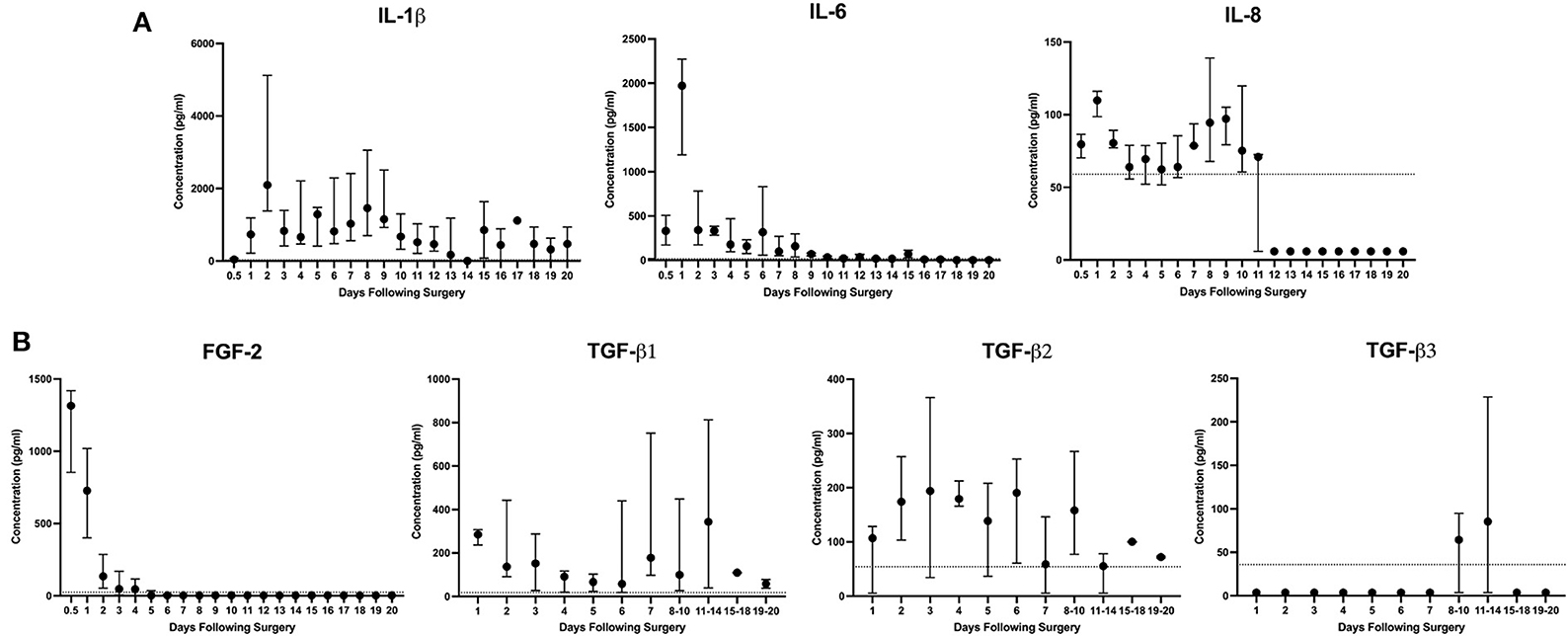
Figure 2. IL-1β and IL-6 are the predominant inflammatory cytokines present following surgically induced equine SDFT injury. Descriptive report of (A) inflammatory cytokines and (B) growth factors FGF-2 and TGF-β1,−2, and−3 in tendon ultrafiltrate were measured by ELISA following bilateral surgically induced SDFT injury of the forelimb over 21 days in 6 horses. Due to insufficient volume, samples from days 8 onward were grouped for TGF-β isoform analysis. When present, the dashed line at the y-axis represents the lower limit of quantification (LLoQ) for that cytokine or growth factor as determined by the assay manufacturer (MilliporeSigma, MA, USA). Concentrations measured below the LLoQ were assigned a value of 0.1 of the LLoQ.
Postmortem histopathology (hematoxylin and eosin) of representative tendons revealed presence of mildly reactive fibroblasts with extracellular matrix deposition within the tendon and surrounding ultrafiltration probe fibers (Supplementary Figure 1). Due to lack of indicators of chronic inflammation such as adjacent leukocyte infiltration, ultrafiltration probe fibers were determined to be inert as consistent with previous literature on the use of ultrafiltration probes in other locations within the body (15, 20, 21).
IL-1β licensed MSCs, but not IL-6 licensed, have enhanced reparative gene expression
Following IL-1β licensing of MSCs, a total of 788 significant differentially expressed genes (DEGs) were identified with 575 genes upregulated and 213 genes downregulated (Figure 3A). Those genes with the largest increase in expression included: NOS2, a key regulator of nitric oxide production, a reactive free radical involved in a variety of biologic processes (22); CCL20, an inflammatory chemokine that binds exclusively to CCR6 receptors commonly found in cells of the immune system (23); IL6, a pleotropic cytokine associated with inflammation, tissue healing, metabolism, and lymphocyte development (24); and FOXN4, a member of the forkhead box/winged-helix transcription superfamily that determines cell fate in neural and non-neural tissues (25). Genes with the largest downregulation included LOC106780963, an uncharacterized gene from chromosome 9; LMCD1, a regulator of MSC osteogenic differentiation (26); PGFS, an enzyme responsible for prostaglandin F production which causes bronchial and arterial smooth muscle contraction (27); and MGP, a vitamin K-dependent protein associated with vascular calcification (28). Gene ontology (GO) enrichment analysis identified the top overrepresented terms in the entire DEG set. The top ten molecular functions (MF), biological processes (BP), and cellular components (CC) for these data are listed (Figure 3B). Overrepresented terms identified by GO analysis included growth factor activity, cytokine activity, cell communication, extracellular matrix, and collagen-containing extracellular matrix among others (Figure 3B). For the top 100 upregulated genes, all significant GO terms for MF, BP, CC, KEGG pathways, and human phenotype ontology (HP) are shown in Supplementary Figure 2.
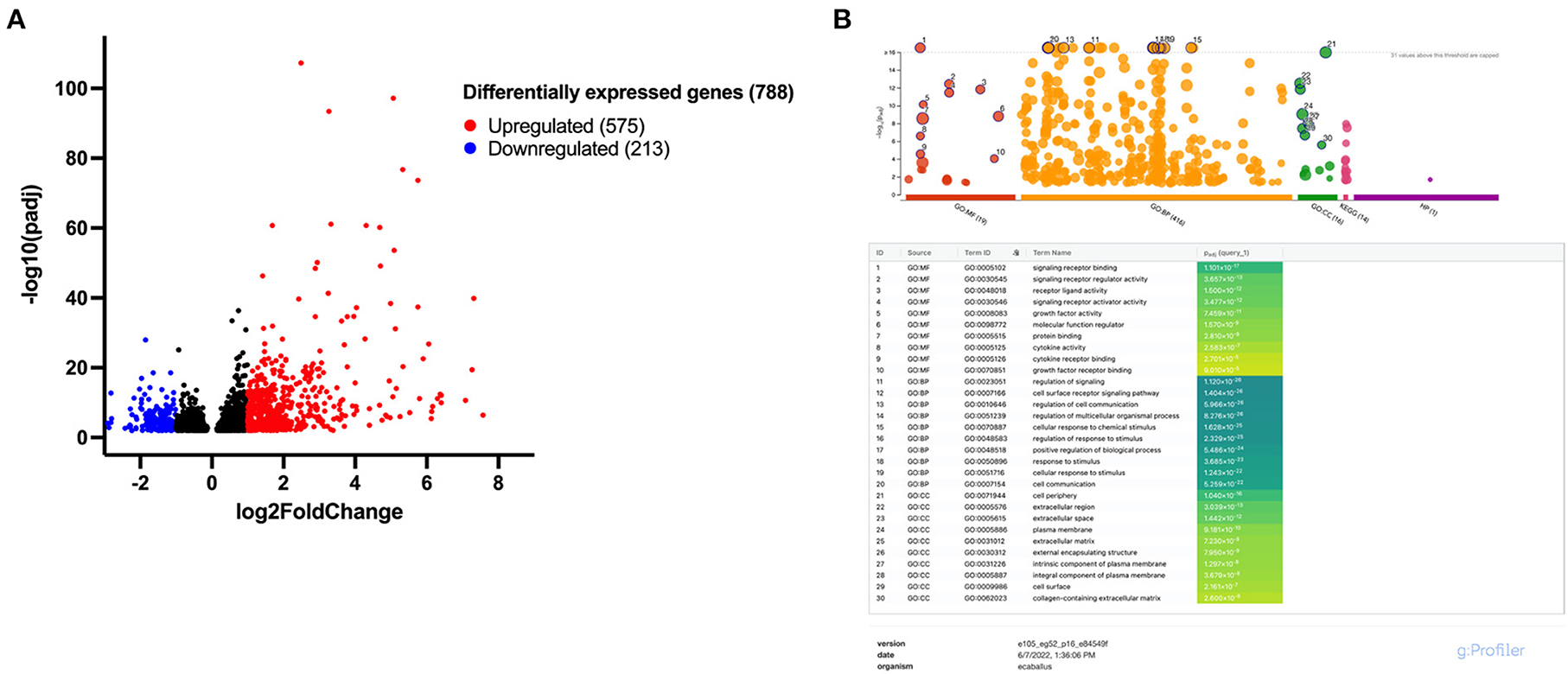
Figure 3. Approximately 788 differentially expressed genes (DEGs) were identified following IL-1β licensing of equine MSCs with overrepresented terms including growth factor and cytokine activity and extracellular matrix-associated functions. (A) Volcano plot for differential expression analysis depicting the log2 fold change and -log10 (adjusted p value) for gene transcripts in IL-1β licensed MSCs detected by RNA-sequencing. Significantly upregulated DEGs are presented in red and significantly downregulated DEGs in blue with absolute fold change >1.0 and a p-value < 0.01. (B) Manhattan plots from g:Profiler illustrating GO term enrichment analysis of DEGs of MSCs following IL-1β licensing. The top 10 enriched pathways for each GO term (MF, molecular function; BP, biological process; CC, cellular component) are presented.
Of the 788 DEGs, multiple genes were identified that could be relevant—whether beneficial or detrimental—to the healing tendon (Figure 4; Table 1). Multiple differentially regulated genes were identified, that if translated to their downstream proteins, could have tendon-relevant effects (Table 1) through tendon or MSC-specific function including those associated with vascular development (ANGPT1/2, ANGTPL4, CXCL6, VEGF-A, VEGF-C), synthesis and remodeling of the tendon ECM (ADAMTS4/5, COL5A1/A2, IL6, MMP1/3/17, NOS2, TIMP1/3), chemokine and growth factor function alteration (MMP1/3), and immunomodulation and tissue reparative effects (IL6, NOS2, PTGES, PTGS2). Following MSC licensing with IL-6, EGR3 and FOSB were the lone DEGs identified. Neither was considered relevant to MSC function in the context of tendon development or healing.
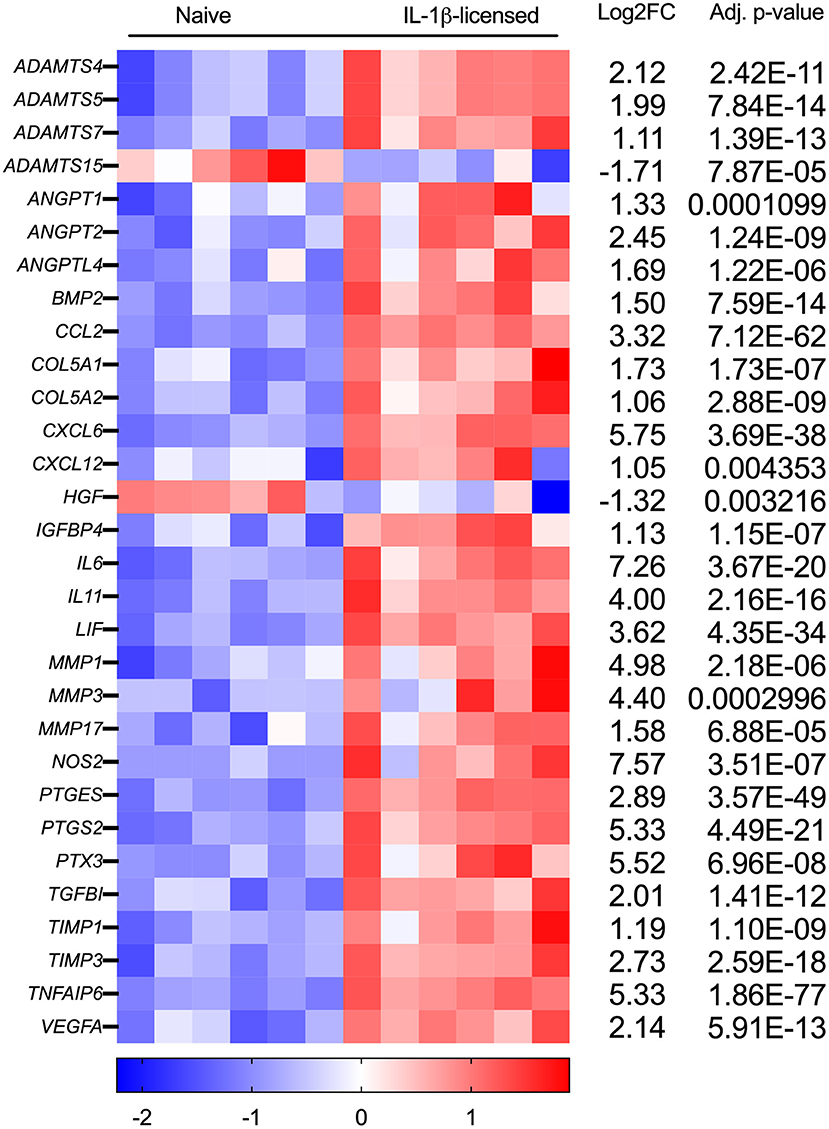
Figure 4. Tendon-relevant genes are significantly differentially regulated in equine MSCs following IL-1β licensing. Heat map representation using log2fold change of select significantly differentially expressed genes (DEGs) from RNA sequencing analysis following IL-1β licensing (n = 6 horses). Compared to naïve MSCs, gene downregulation is noted in blue and gene upregulation in red.
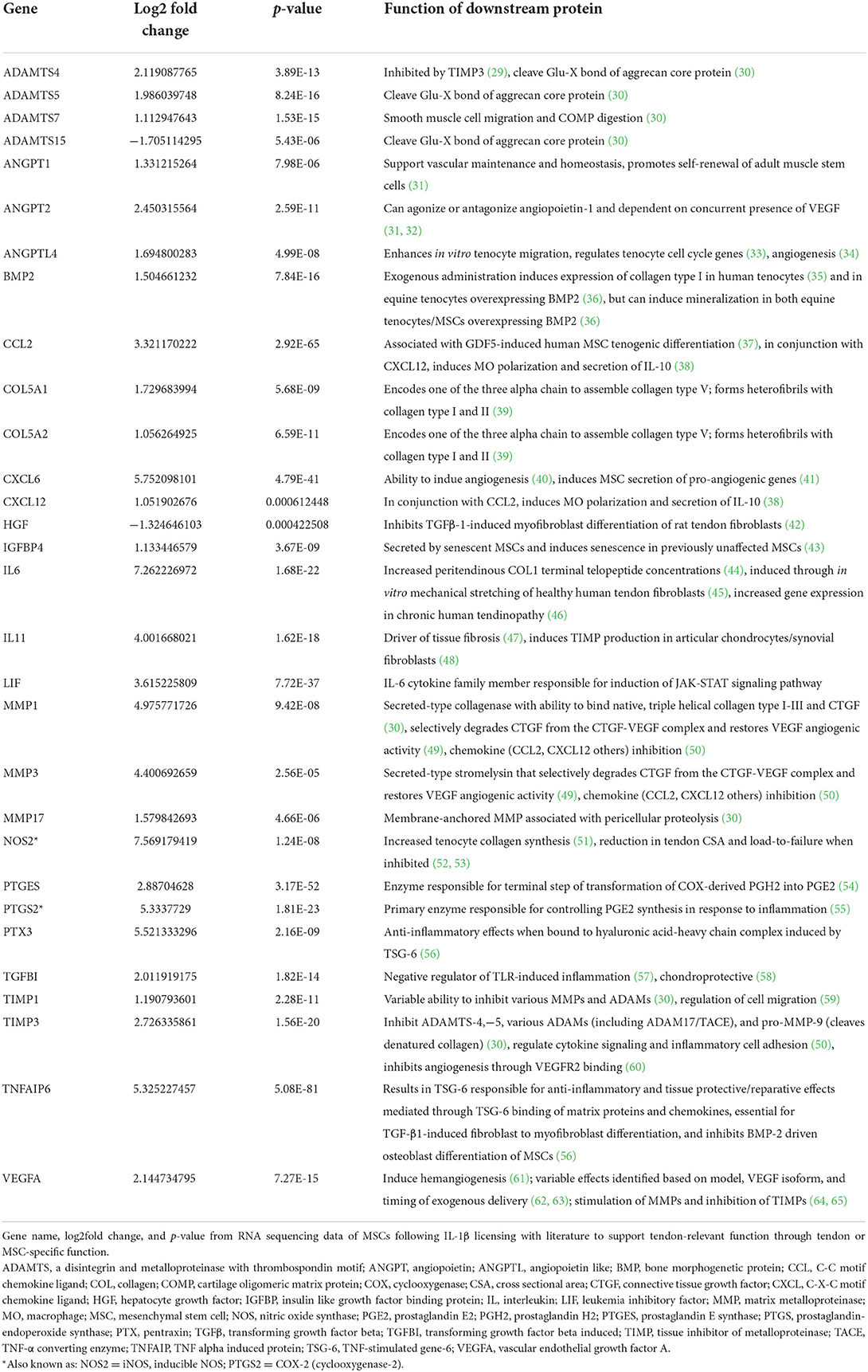
Table 1. Function of downstream proteins produced from DEGs identified from IL-1β licensed MSCs that could impart tendon-relevant effects.
IL-1β licensed MSCs secrete significantly more IL-6, PGE2, and VEGF than naïve MSCs
Three DEGs in IL-1β-licensed MSCs identified in RNA-seq data were chosen due to their known relevance to tendon healing, to confirm downstream protein expression. Cell culture supernatant obtained from IL-1β licensed MSCs produced significantly more IL-6 (p = 0.0126), PGE2 (p = 0.0193), and VEGF (p = 0.0162) compared to their paired naïve controls (Figure 5). IL-6 is a pleotropic cytokine present following tendon injury and regulates leukocyte infiltration and controls duration of pro-inflammatory mediator release (12, 66). Prostaglandin E2 is a primary mediator of equine MSC immunomodulation, is present following tendon injury, and likely plays a critical, concentration-dependent role in both the reparative response as well as the development of chronic injury (67, 68). VEGF, present following tendon loading and tenocyte mechanical stimulation, appears to improve strength of healing tendon when administered exogenously (69). These data support that IL-1β licensing is inducing changes in gene and protein expression in MSCs and this phenotype could provide tendon-relevant effects.
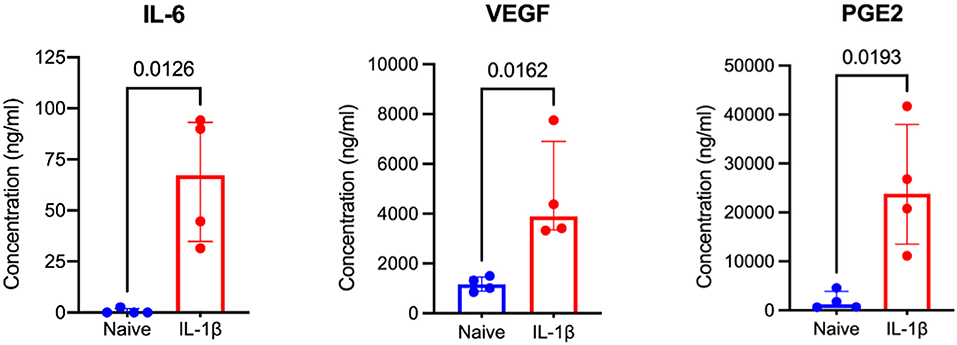
Figure 5. IL-1β licensed equine MSCs produce significantly more IL-6, VEGF, and PGE2 than naïve MSCs. From RNA sequencing data, three proteins known to have tendon-relevant effects were selected to confirm downstream protein expression. Analysis of MSC cell culture supernatants by ELISA assay confirmed increased protein expression of IL-6, VEGF, and PGE2 following IL-1β licensing compared to naïve MSC controls. A one-tailed paired t-test was used to examine difference in protein expression between groups.
Discussion
This is the first study of its kind to define the temporal cytokine profile following surgically induced tendon injury. A novel method of implantation of an ultrafiltration probe within the center of the equine SDFT was implemented where it was determined that inflammation was predominated by IL-1β and IL-6. Additionally, concentrations of both cytokines peaked at ~2,000 pg/ml (2 ng/ml) within 48 h following injury. When MSCs were subsequently licensed with a similar concentration of exogenous recombinant human IL-1β, licensed MSCs upregulated expression of multiple genes that could impart benefit to the healing tendon. Further, downstream expression for three select genes were supported by significantly higher protein concentrations measured in culture supernatant compared to naïve MSCs. These data further improve our understanding of the tendon microenvironment following injury and provide evidence that IL-1β licensed MSCs could provide enhanced therapeutic benefit compared to their naïve counterparts.
Elucidating the temporal cytokine profile in tendon following injury is critical to guide development of novel therapies that aim to return the biochemical composition and biomechanical strength of healing tendon to its native state. Attempts to characterize the interplay of cytokines in the tendon microenvironment in both health and disease have employed cell culture, tissue biopsy, and subcutaneous microdialysis as recently review by Ellis et al. (12). However, the inability to repeatedly sample the injured tendon microenvironment in conjunction with inconsistent standardization of samples from naturally occurring disease limit our understanding of the temporal change that occurs following injury (13, 14). To rectify this, our group was able to sample the tendon microenvironment twice daily for 21 days in a surgically induced model using a novel method that employed ultrafiltration probes. The distinct advantages of this technique include standardization of tendon lesions, implementation of a relevant large animal model of tendon injury, and daily temporal characterization of the tendon microenvironment that would otherwise require large numbers of terminal animal studies (18, 70–72). We demonstrate that ultrafiltration probes were effective in facilitating characterization of the cytokine milieu in tendon injury. Additionally, lack of histopathologic evidence of foreign body reaction (73) within examined tendons indicate the cytokines at their measured concentration were an accurate reflection of the tendon microenvironment following injury. While we did not attempt to elucidate the cell-specific source of these cytokines, in vitro studies support this milieu is likely secreted by a combination of local and infiltrating cells including tendon fibroblasts, tendon stem/progenitor cells, and immune cells (14, 74, 75). The authors recognize that although the cytokine quantification herein is limited to the analytes present in the commercial kits and does not represent all analytes involved in the molecular cascade, the novel method employed to characterize the tendon microenvironment is an essential first step and establishes a new technique to collect further data on the microenvironment following injury and in response to therapy. Despite the associated bias when selecting cytokines to analyze tendon ultrafiltrate, published literature of the injured tendon microenvironment (12, 13, 76) was used to support selection of the most relevant cytokines in our custom multiplex within species-specific assay constraints.
While the therapeutic effect of MSCs was previously attributed to local engraftment and cell differentiation, it is now understood that MSC functions include their ability to stimulate angiogenesis, apoptosis, immunomodulation, and recruitment and stimulation of systemic and endogenous progenitor cells through secretion of paracrine mediators (77, 78). Interestingly, these functions can be dramatically altered when naive MSCs are administered within an injured tissue microenvironment or following exogenous exposure to pro-inflammatory cytokines, deemed MSC licensing (5, 77). In experimental settings, administration of MCSs licensed with inflammatory cytokines or molecules such as bacterial lipopolysaccharide (LPS) can ameliorate pathologic change in the colon (79), improve corneal allograft survival (8), and improve cardiac fibrosis following cardiac ischemia (80). Despite these benefits in various body systems, only two studies have been published that implement MSC licensing and their effect on tendon tissue. Positive outcomes were noted, respectively, in tenocyte gene expression and tendon healing in projects that examined the in vitro effect of licensing MSCs with pioglitazone, a hypoglycemic agent, on tenocytes (81) and TNF-α licensed MSCs in a PLG scaffold in a rat Achilles tendon defect (11). From the in vivo tendon microenvironment cytokine data, IL-1β and IL-6 were chosen to license MSCs to evaluate effects on gene and protein expression while IL-8 was avoided where it was present at low levels in the tendon microenvironment. Further, from RNA-seq data, naïve equine MSCs were not determined to express IL-8 receptors CXCR1 and−2. Although we understand the effect of IL-1β on MCS gene and protein expression, the influence of IL-6 is less understood (82, 83). Additionally, even less is understood of equine MSCs response following exposure to inflammation with what we do know based on proteomic analysis after IL-1β treatment during chondrogenic differentiation (84). As this is the first report of transcriptome-wide changes in equine MSCs following exposure to inflammatory cytokines, these data presented advance our understanding of MSC biology and will serve as a foundation for future work studying the therapeutic benefit of licensed MSCs.
While increased expression of many genes following IL-1β licensing of MSCs could be beneficial to the healing tendon, it would be remiss to ignore others that conversely could be detrimental. It plausible that IL-1β licensed MCS secretion of IL-11 (tissue fibrosis), reduction in HGF (inhibitor of myofibroblast differentiation), or excessive PGE2 production could be unwanted side effects of treatment. What is poorly understood at this time is how this IL-1β licensed MSC secretome would affect the tendon microenvironment and whether certain molecules impart benefit and trump detrimental effects of others. Assessing the effect of the IL-1β licensed MSC secretome on tenocytes is a critical next step and a current focus of our laboratory. Finally, while genes expanded upon herein were discussed due to their known association with tenocyte and/or MSC function or their role in tendon healing, the authors recognize that many of the other differentially regulated genes could greatly impact the tendon microenvironment if IL-1β licensed MSCs were administered in vivo. However, discussion of these genes was omitted as they lack experimental evidence for their role in tendon development and healing.
Our interest is in improving therapy for equine tendon injuries, however, the utility of the horse as a comparative and preclinical model for developing translational therapies for human tendon injury is promising. Human tendon injuries account for more than 32 million musculoskeletal injuries a year in the United States with an increasing incidence and health care-associated cost due to an aging population pursuing more athletic activity (85–87). Despite appropriate therapy, continued high postoperative reinjury rates have shifted focus toward regenerative medicine approaches to improve outcomes (88, 89). However, the lack of randomized, blinded clinical trials combined with tepid results in case series have prevented support for their continued use (90, 91). While no animal model perfectly recapitulates human tendon injury, equine SDFT injury is an accepted comparative model for exercise-induced Achilles injury in humans (72). Additionally, a recent report suggests equine tenocytes have a tendon marker, collagen expression, and ECM gene repertoire most similar to humans (92). With this in mind, data presented herein could be the first step in enhancing MSC therapy for equine tendon injuries while providing the foundation for future translational studies.
Limitations of this study include inconsistent ultrafiltrate collection between limbs of the same horse and between individual horses along with wide individual horse variation in tendon ultrafiltrate cytokine concentration. It is also likely that the cytokine profile described in the context of this acute injury model does not represent the cytokine profile of chronic tendon injury. Characterization of the later and chronic stages of tendon injury are still warranted and may shed light on the potential therapeutic benefit of licensed MSCs for such injury stages. Despite significant upregulation of multiple MSC genes and downstream proteins that could be beneficial to the healing tendon, it is unknown whether greater expression or these bioactive factors all in combination would truly enhance repair and requires further examination. Finally, the authors recognize that while licensing of cells with a single cytokine does appear to improve the reparative gene and protein expression of MSCs, this does not represent all the cytokines, chemokines, and growth factors they would experience in an in vivo system and could therefore drastically alter their transcriptome compared to in vitro assays.
In conclusion, this is the first study to quantify the temporal cytokine profile over 21 days in an equine model of surgically induced tendon injury. Our results indicate that the tendon microenvironment within the first 48 h following injury contains a concentration of IL-1β that beneficially modulates MSC gene and protein expression and then rapidly resolves. Because current culture expansion techniques require weeks to prepare both newly isolated and banked MSCs, a situation would rarely arise allowing a veterinarian to administer cells into an actively inflamed tendon as a method to improve MSC therapeutic potential. Therefore, an alternative approach could include in vitro licensing of MSCs prior to treatment to mimic exposure to this environment. However, before it can be recommended to implement IL-1β licensed MSCs in clinical cases of equine tendon injury, further studies are required examining both their effect on tenocytes in culture as well as in preclinical in vivo equine studies if in vitro experiments support their use. Overall, data generated in this study greatly expand our knowledge of the cytokine microenvironment during tendon injury and support the continued investigation of IL-1β licensed MSCs as a potential therapeutic.
Data availability statement
The data presented in the study are deposited in the NCBI GEO repository, accession number GSE208569, and can be found at https://www.ncbi.nlm.nih.gov/geo/query/acc.cgi?acc=GSE208569.
Ethics statement
The animal study was reviewed and approved by North Carolina State University Institutional Animal Care and Use Committee.
Author contributions
DK, AB, KM, JG, and LS contributed to study conception and design. DK and AB performed statistical analyses. DK drafted the manuscript with all authors contributing edits. All authors contributed to acquisition of data and interpretation of data. All authors read and approved the final manuscript.
Funding
This work was supported by the Grayson-Jockey Club Research Foundation (LS) and the Fund for Orthopedic Research in honor of Gus and Equine athletes (F.O.R.G.E; LS) with stipend support from the National Institutes of Health grants T32OD011130 (DK), K01OD027037 (AB), and T35OD011070 (IE).
Acknowledgments
The authors would like to thank Ms. Julie Long for laboratory technical assistance and NCSU Laboratory Animal Resources staff for their help with animal care and handling. The authors would also like to thank the team at Basi Research Products, and especially Dr. Lauren Elolf, for the extensive assistance provided in the development of the custom 100 kDa ultrafiltration probes used in this study.
Conflict of interest
The authors declare that the research was conducted in the absence of any commercial or financial relationships that could be construed as a potential conflict of interest.
Publisher's note
All claims expressed in this article are solely those of the authors and do not necessarily represent those of their affiliated organizations, or those of the publisher, the editors and the reviewers. Any product that may be evaluated in this article, or claim that may be made by its manufacturer, is not guaranteed or endorsed by the publisher.
Supplementary material
The Supplementary Material for this article can be found online at: https://www.frontiersin.org/articles/10.3389/fvets.2022.963759/full#supplementary-material
References
1. Patterson-Kane JC, Firth EC. The pathobiology of exercise-induced superficial digital flexor tendon injury in Thoroughbred racehorses. Vet J. (2009) 181:79–89. doi: 10.1016/j.tvjl.2008.02.009
2. O'meara B, Bladon B, Parkin TDH, Fraser B, Lischer CJ. An investigation of the relationship between race performance and superficial digital flexor tendonitis in the Thoroughbred racehorse. Eq Vet J. (2010) 42:322–26. doi: 10.1111/j.2042-3306.2009.00021.x
3. Godwin EE, Young NJ, Dudhia J, Beamish IC, Smith RKW. Implantation of bone marrow-derived mesenchymal stem cells demonstrates improved outcome in horses with overstrain injury of the superficial digital flexor tendon. Equine Vet J. (2012) 44:25–32. doi: 10.1111/j.2042-3306.2011.00363.x
4. Schnabel LV, Lynch ME, van der Meulen MCH, Yeager AE, Kornatowski MA, Nixon AJ. Mesenchymal stem cells and insulin-like growth factor-I gene-enhanced mesenchymal stem cells improve structural aspects of healing in equine flexor digitorum superficialis tendons. J Orthopaed Res. (2009) 27:1392–98. doi: 10.1002/jor.20887
5. Shi Y, Wang Y, Li Q, Liu K, Hou J, Shao C, et al. Immunoregulatory mechanisms of mesenchymal stem and stromal cells in inflammatory diseases. Nat Rev Nephrol. (2018) 14:493–507. doi: 10.1038/s41581-018-0023-5
6. Krampera M, Galipeau J, Shi Y, Tarte K, Sensebe L. Immunological characterization of multipotent mesenchymal stromal cells-The international society for cellular therapy (ISCT) working proposal. Cytotherapy. (2013) 15:1054–61. doi: 10.1016/j.jcyt.2013.02.010
7. Broekman W, Amatngalim GD, de Mooij-Eijk Y, Oostendorp J, Roelofs H, Taube C, et al. TNF-aα and IL-1β-activated human mesenchymal stromal cells increase airway epithelial wound healing in vitro via activation of the epidermal growth factor receptor. Respirat. Res. (2016) 17:1–12. doi: 10.1186/s12931-015-0316-1
8. Murphy N, Treacy O, Lynch K, Morcos M, Lohan P, Howard L, et al. TNF-α/IL-1β–licensed mesenchymal stromal cells promote corneal allograft survival via myeloid cell-mediated induction of Foxp3+ regulatory T cells in the lung. FASEB J. (2019) 33:9404–21. doi: 10.1096/fj.201900047R
9. Cassano JM, Schnabel LV, Goodale MB, Fortier LA. Inflammatory licensed equine MSCs are chondroprotective and exhibit enhanced immunomodulation in an inflammatory environment. Stem Cell Res Therapy. (2018) 9:1–13. doi: 10.1186/s13287-018-0840-2
10. Planat-Benard V, Varin A, Casteilla L. MSCs and inflammatory cells crosstalk in regenerative medicine: concerted actions for optimized resolution driven by energy metabolism. Front Immunol. (2021) 12:626755. doi: 10.3389/fimmu.2021.626755
11. Aktas E, Chamberlain CS, Saether EE, Duenwald-Kuehl SE, Kondratko-Mittnacht J, Stitgen M, et al. Immune modulation with primed mesenchymal stem cells delivered via biodegradable scaffold to repair an Achilles tendon segmental defect. J Orthopaed Res. (2017) 35:269–80. doi: 10.1002/jor.23258
12. Ellis IM, Schnabel LV, Berglund AK. Defining the profile: characterizing cytokines in tendon injury to improve clinical therapy. J Immunol Regener Med. (2022) 16:100059. doi: 10.1016/j.regen.2022.100059
13. Morita W, Dakin SG, Snelling SJB, Carr AJ. Cytokines in tendon disease: a systematic review. Bone Joint Res. (2017) 6:656–64. doi: 10.1302/2046-3758.612.BJR-2017-0112.R1
14. Schulze-Tanzil G, Al-Sadi O, Wiegand E, Ertel W, Busch C, Kohl B, et al. The role of pro-inflammatory and immunoregulatory cytokines in tendon healing and rupture: new insights. Scand J Med Sci Sports. (2011) 21:337–51. doi: 10.1111/j.1600-0838.2010.01265.x
15. Messenger KM, Wofford JA, Papich MG. Carprofen pharmacokinetics in plasma and in control and inflamed canine tissue fluid using in vivo ultrafiltration. J Vet Pharmacol Ther. (2016) 39:32–9. doi: 10.1111/jvp.12233
16. Messenger KM, Papich MG, Blikslager AT. Distribution of enrofloxacin and its active metabolite, using an in vivo ultrafiltration sampling technique after the injection of enrofloxacin to pigs. J Vet Pharmacol Ther. (2012) 35:452–59. doi: 10.1111/j.1365-2885.2011.01338.x
18. Schramme M, Hunter S, Campbell N, Blikslager A, Smith R. A surgical tendonitis model in horses: technique, clinical, ultrasonographic and histological characterisation. Vet Comp Orthopaed Traumatol. (2010) 23:231–9. doi: 10.3415/VCOT-09-10-0106
19. Schnabel LV, Pezzanite LM, Antczak DF, Felippe MJB, Fortier LA. Equine bone marrow-derived mesenchymal stromal cells are heterogeneous in MHC class II expression and capable of inciting an immune response in vitro. Stem Cell Res Therapy. (2014) 5:13. doi: 10.1186/scrt402
20. Mzyk DA, Bublitz CM, Sylvester H, Mullen KAE, Hobgood GD, Baynes RE, et al. Short communication: use of an ultrafiltration device in gland cistern for continuous sampling of healthy and mastitic quarters of lactating cattle for pharmacokinetic modeling. J Dairy Sci. (2018) 101:10414–20. doi: 10.3168/jds.2018-14849
21. Underwood C, Collins SN, van Eps AW, Allavena RE, Medina-Torres CE, Pollitt CC. Ultrafiltration of equine digital lamellar tissue. Vet J. (2014) 202:314–22. doi: 10.1016/j.tvjl.2014.05.007
22. Förstermann U, Sessa WC. Nitric oxide synthases: regulation and function. Eur Heart J. (2012) 33:829-37. doi: 10.1093/eurheartj/ehr304
23. Lee AYS, Eri R, Lyons AB, Grimm MC, Korner H. CC chemokine ligand 20 and its cognate receptor CCR6 in mucosal T cell immunology and inflammatory bowel disease: odd couple or axis of evil? Front Immunol. (2013) 4:194. doi: 10.3389/fimmu.2013.00194
24. Tanaka T, Narazaki M, Kishimoto T. Il-6 in inflammation, immunity, and disease. Cold Spring Harbor Perspect Biol. (2014) 6:1–16. doi: 10.1101/cshperspect.a016295
25. Xiang MQ Li SG. Foxn4: a multi-faceted transcriptional regulator of cell fates in vertebrate development. Sci China Life Sci. (2013) 56:985–93. doi: 10.1007/s11427-013-4543-8
26. Zhu B, Xue F, Zhang C, Li G. LMCD1 promotes osteogenic differentiation of human bone marrow stem cells by regulating BMP signaling. Cell Death Dis. (2019) 10:1–11. doi: 10.1038/s41419-019-1876-7
27. Watanabe K. Prostaglandin F synthase. Prostagland Other Lipid Mediat. (2002) 68–9:401–7. doi: 10.1016/S0090-6980(02)00044-8
28. Bjørklund G, Svanberg E, Dadar M, Card DJ, Chirumbolo S, Harrington DJ, et al. The role of matrix Gla protein (MGP) in vascular calcification. Curr Med Chem. (2019) 27:1647–60. doi: 10.2174/0929867325666180716104159
29. Porter S, Clark IM, Kevorkian L, Edwards DR. The ADMTS metalloproteinases. Biochem J. (2005) 386:15–27. doi: 10.1042/BJ20040424
30. Shiomi T, Lemaître V, D'Armiento J, Okada Y. Matrix metalloproteinases, a disintegrin and metalloproteinases, and a disintegrin and metalloproteinases with thrombospondin motifs in non-neoplastic diseases: review article. Pathol Int. (2010) 60:477–96. doi: 10.1111/j.1440-1827.2010.02547.x
31. Koh GY. Orchestral actions of angiopoietin-1 in vascular regeneration. Trends Mol Med. (2013) 19:31–9. doi: 10.1016/j.molmed.2012.10.010
32. Nowicki M, Wierzbowska A, Małachowski R, Robak T, Grzybowska-Izydorczyk O, Pluta A, et al. ANGPT1, ANGPT2, and MMP-9 expression in the autologous hematopoietic stem cell transplantation and its impact on the time to engraftment. Ann Hematol. (2017) 96:2103–12. doi: 10.1007/s00277-017-3133-4
33. Jamil S, Mousavizadeh R, Roshan-Moniri M, Tebbutt SJ, McCormack RG, Duronio V, et al. Angiopoietin-like 4 enhances the proliferation and migration of tendon fibroblasts. Med Sci Sports Exerc. (2017) 49:1769–77. doi: 10.1249/MSS.0000000000001294
34. la Paglia L, Listì A, Caruso S, Amodeo V, Passiglia F, Bazan V, et al. Potential role of ANGPTL4 in the cross talk between metabolism and cancer through PPAR signaling pathway. PPAR Res. (2017) 2017:1–15. doi: 10.1155/2017/8187235
35. Pauly S, Klatte F, Strobel C, Schmidmaier G, Greiner S, Scheibel M, et al. BMP-2 and BMP-7 affect human rotator cuff tendon cells in vitro. J Shoulder Elbow Surg. (2012) 21:464–73. doi: 10.1016/j.jse.2011.01.015
36. Murray SJ, Santangelo KS, Bertone AL. Evaluation of early cellular influences of bone morphogenetic proteins 12 and 2 on equine superficial digital flexor tenocytes and bone marrow-derived mesenchymal stem cells in vitro. Am J Vet Res. (2010) 71:103–14. doi: 10.2460/ajvr.71.1.103
37. Tan SL, Ahmad TS, Ng WM, Azlina AA, Azhar MM, Selvaratnam L, et al. Identification of pathways mediating growth differentiation factor5-induced tenogenic differentiation in human bone marrow stromal cells. PLoS ONE. (2015) 10:e0140869. doi: 10.1371/journal.pone.0140869
38. Galipeau J. Macrophages at the nexus of mesenchymal stromal cell potency: the emerging role of chemokine cooperativity. Stem Cells. (2021) 39:1145–54. doi: 10.1002/stem.3380
39. Gelse K, Pöschl E, Aigner T. Collagens - structure, function, and biosynthesis. Adv Drug Deliv Rev. (2003) 55:1531–46. doi: 10.1016/j.addr.2003.08.002
40. Strieter RM, Polverini PJ, Kunkel SL, Arenberg DA, Burdick MD, Kasper J, et al. The functional role of the ELR motif in CXC chemokine-mediated angiogenesis. J Biol Chem. (1995) 270:27348–57. doi: 10.1074/jbc.270.45.27348
41. Kim SW, Lee DW Yu LH, Zhang HZ, Kim CE, Kim JM, Park TH, et al. Mesenchymal stem cells overexpressing GCP-2 improve heart function through enhanced angiogenic properties in a myocardial infarction model. Cardiovasc Res. (2012) 95:495–506. doi: 10.1093/cvr/cvs224
42. Cui Q, Fu S, Li Z. Hepatocyte growth factor inhibits TGF-β1-induced myofibroblast differentiation in tendon fibroblasts: Role of AMPK signaling pathway. J Physiol Sci. (2013) 63:163–70. doi: 10.1007/s12576-013-0251-1
43. Severino V, Alessio N, Farina A, Sandomenico A, Cipollaro M, Peluso G, et al. Insulin-like growth factor binding proteins 4 and 7 released by senescent cells promote premature senescence in mesenchymal stem cells. Cell Death Dis. (2013) 4:e911. doi: 10.1038/cddis.2013.445
44. Andersen MB, Pingel J, Kjær M, Langberg H. Interleukin-6: A growth factor stimulating collagen synthesis in human tendon. J Appl Physiol. (2011) 110:1549–54. doi: 10.1152/japplphysiol.00037.2010
45. Skutek M, van Griensven M, Zeichen J, Brauer N, Bosch U. Cyclic mechanical stretching enhances secretion of Interleukin 6 in human tendon fibroblasts. Knee Surg Sports Traumatol Arthrosc. (2001) 9:322–26. doi: 10.1007/s001670100217
46. Legerlotz K, Jones ER, Screen HRC, Riley GP. Increased expression of IL-6 family members in tendon pathology. Rheumatology. (2012) 51:1161–65. doi: 10.1093/rheumatology/kes002
47. Cook SA, Schafer S. Hiding in plain sight: interleukin-11 emerges as a master regulator of fibrosis, tissue integrity, and stromal inflammation. Annu Rev Med. (2020) 71:263–76. doi: 10.1146/annurev-med-041818-011649
48. Maier R, Ganu V, Lotz M. Interleukin-11, an inducible cytokine in human articular chondrocytes and synoviocytes, stimulates the production of the tissue inhibitor of metalloproteinases. J Biol Chem. (1993) 268:21527–32. doi: 10.1016/S0021-9258(20)80573-0
49. Hashimoto G, Inoki I, Fujii Y, Aoki T, Ikeda E, Okada Y. Matrix metalloproteinases cleave connective tissue growth factor and reactivate angiogenic activity of vascular endothelial growth factor 165. J Biol Chem. (2002) 277:36288–95. doi: 10.1074/jbc.M201674200
50. Gill SE, Parks WC. Metalloproteinases and their inhibitors: regulators of wound healing. Int J Biochem Cell Biol. (2008) 40:1334–47. doi: 10.1016/j.biocel.2007.10.024
51. Xia W, Szomor Z, Wang Y, Murrell GAC. Nitric oxide enhances collagen synthesis in cultured human tendon cells. J Orthopaed Res. (2006) 24:159–72. doi: 10.1002/jor.20060
52. Xia W, Wang Y, Appleyard RC, Smythe GA, Murrell GAC. Spontaneous recovery of injured Achilles tendon in inducible nitric oxide synthase gene knockout mice. Inflamm Res. (2006) 55:40–5. doi: 10.1007/s00011-005-0006-4
53. Murrell GAC, Szabo C, Hannafin JA, Jang D, Dolan MM, Deng XH, et al. Modulation of tendon healing by nitric oxide. Inflamm Res. (1997) 46:19–27. doi: 10.1007/s000110050027
54. Murakami M, Nakatani Y, Tanioka T, Kudo I. Prostaglandin E synthase. Prostagland Other Lipid Mediat. (2002) 68–9:383–99. doi: 10.1016/S0090-6980(02)00043-6
55. Park JY, Pillinger MH, Abramson SB. Prostaglandin E2 synthesis and secretion: the role of PGE2 synthases. Clin Immunol. (2006) 119:229–40. doi: 10.1016/j.clim.2006.01.016
56. Day AJ, Milner CM. TSG-6: a multifunctional protein with anti-inflammatory and tissue-protective properties. Matrix Biol. (2019) 78–9:60–83. doi: 10.1016/j.matbio.2018.01.011
57. Yang Y, Sun H, Li X, Ding Q, Wei P, Zhou J. Transforming growth factor beta-induced is essential for endotoxin tolerance induced by a low dose of lipopolysaccharide in human peripheral blood mononuclear cells. Iran J Allergy Asthma Immunol. (2015) 14:321–30.
58. Ruiz M, Toupet K, Maumus M, Rozier P, Jorgensen C, Noël D, et al. secreted by mesenchymal stromal cells ameliorates osteoarthritis and is detected in extracellular vesicles. Biomaterials. (2020) 226:119544. doi: 10.1016/j.biomaterials.2019.119544
59. Chen P, Farivar AS, Mulligan MS, Madtes DK. Tissue inhibitor of metalloproteinase-1 deficiency abrogates obliterative airway disease after heterotopic tracheal transplantation. Am J Respir Cell Mol Biol. (2006) 34:464–72. doi: 10.1165/rcmb.2005-0344OC
60. Qi JH, Ebrahem Q, Moore N, Murphy G, Claesson-Welsh L, Bond M, et al. novel function for tissue inhibitor of metalloproteinases-3 (TIMP3): Inhibition of angiogenesis by blockage of VEGF binding to VEGF receptor-2. Nat Med. (2003) 9:407–15. doi: 10.1038/nm846
61. Rauniyar K, Jha SK, Jeltsch M. Biology of vascular endothelial growth factor C in the morphogenesis of lymphatic vessels. Front Bioeng Biotechnol. (2018) 6:7. doi: 10.3389/fbioe.2018.00007
62. Zhang F, Liu H, Stile F, Lei MP, Pang Y, Oswald TM, et al. Effect of vascular endothelial growth factor on rat achilles tendon healing. Plast Reconstr Surg. (2003) 112:1613–19. doi: 10.1097/01.PRS.0000086772.72535.A4
63. Liu X, Zhu B, Li Y, Liu X, Guo S, Wang C, et al. The role of vascular endothelial growth factor in tendon healing. Front Physiol. (2021) 12:766080. doi: 10.3389/fphys.2021.766080
64. Pufe T, Petersen WJ, Mentlein R, Tillmann BN. The role of vasculature and angiogenesis for the pathogenesis of degenerative tendons disease. Scand J Med Sci Sports. (2005) 15:211–22. doi: 10.1111/j.1600-0838.2005.00465.x
65. Halper J. Advances in the use of growth factors for treatment of disorders of soft tissues. In: Halper J, editor. Progress in Heritable Soft Connective Tissue Disorders. Dordrecht: Springer (2013). p. 69–76.
66. Millar NL, Murrell GAC, Mcinnes IB. Inflammatory mechanisms in tendinopathy - towards translation. Nat Rev Rheumatol. (2017) 13:110–22. doi: 10.1038/nrrheum.2016.213
67. Carrade Holt DD, Wood JA, Granick JL, Walker NJ, Clark KC, Borjesson DL. Equine mesenchymal stem cells inhibit T cell proliferation through different mechanisms depending on tissue source. Stem Cells Dev. (2014) 23:1258–65. doi: 10.1089/scd.2013.0537
68. Zhang J, Wang JHC. Prostaglandin E2 (PGE2) exerts biphasic effects on human tendon stem cells. PLoS ONE. (2014) 9:e87706. doi: 10.1371/journal.pone.0087706
69. Vasta S, di Martino A, Zampogna B, Torre G, Papalia R, Denaro V. Role of VEGF, nitric oxide, and sympathetic neurotransmitters in the pathogenesis of tendinopathy: a review of the current evidences. Front Aging Neurosci. (2016) 8:186. doi: 10.3389/fnagi.2016.00186
70. Cadby JA, David F, van de Lest C, Bosch G, van Weeren PR, Snedeker JG, et al. Further characterisation of an experimental model of tendinopathy in the horse. Equine Vet J. (2013) 45:642–8. doi: 10.1111/evj.12035
71. Patterson-Kane JC, Rich T. Achilles tendon injuries in elite athletes: Lessons in pathophysiology from their equine Counterparts. ILAR J. (2014) 55:86–99. doi: 10.1093/ilar/ilu004
72. Patterson-Kane JC, Becker DL, Rich T. The pathogenesis of tendon microdamage in athletes: the horse as a natural model for basic cellular research. J Comp Pathol. (2012) 147:227–47. doi: 10.1016/j.jcpa.2012.05.010
73. Kumar V, Abbas AK, Aster JC. Inflammation and repair. In: Kumar V, Abbas AK, Aster JC, editors. Robbins and Cotran Pathologic Basis of Disease. Philadelphia, PA: Elsevier Saunders (2015). p. 69–111.
74. Tarafder S, Chen E, Jun Y, Kao K, Sim KH, Back J, et al. Tendon stem/progenitor cells regulate inflammation in tendon healing via JNK and STAT3 signaling. FASEB J. (2017) 31:3991–8. doi: 10.1096/fj.201700071R
75. Stolk M, Klatte-Schulz F, Schmock A, Minkwitz S, Wildemann B, Seifert M. New insights into tenocyte-immune cell interplay in an in vitro model of inflammation. Sci Rep. (2017) 7:9801. doi: 10.1038/s41598-017-09875-x
76. Müller SA, Todorov A, Heisterbach PE, Martin I, Majewski M. Tendon healing: an overview of physiology, biology, and pathology of tendon healing and systematic review of state of the art in tendon bioengineering. Knee Surg Sports Traumatol Arthrosc. (2015) 23:2097–105. doi: 10.1007/s00167-013-2680-z
77. Ma S, Xie N, Li W, Yuan B, Shi Y, Wang Y. Immunobiology of mesenchymal stem cells. Cell Death Differ. (2014) 21:216–25. doi: 10.1038/cdd.2013.158
78. da Silva Meirelles L, Fontes AM, Covas DT, Caplan AI. Mechanisms involved in the therapeutic properties of mesenchymal stem cells. Cytok Growth Factor Rev. (2009) 20:419–27. doi: 10.1016/j.cytogfr.2009.10.002
79. Fan H, Zhao G, Liu L, Liu F, Gong W, Liu X, et al. Pre-treatment with IL-1β enhances the efficacy of MSC transplantation in DSS-induced colitis. Cell Mol Immunol. (2012) 9:473–81. doi: 10.1038/cmi.2012.40
80. Yao Y, Zhang F, Wang L, Zhang G, Wang Z, Chen J, et al. Lipopolysaccharide preconditioning enhances the efficacy of mesenchymal stem cells transplantation in a rat model of acute myocardial infarction. J Biomed Sci. (2009) 16:74. doi: 10.1186/1423-0127-16-74
81. Kim W, Lee SK, Kwon YW, Chung SG, Kim S. Pioglitazone-primed mesenchymal stem cells stimulate cell proliferation, collagen synthesis and matrix gene expression in tenocytes. Int J Mol Sci. (2019) 20:472. doi: 10.3390/ijms20030472
82. Ren G, Zhao X, Zhang L, Zhang J, L'Huillier A, Ling W, et al. Inflammatory cytokine-induced intercellular adhesion molecule-1 and vascular cell adhesion molecule-1 in mesenchymal stem cells are critical for immunosuppression. J Immunol. (2010) 184:2321–28. doi: 10.4049/jimmunol.0902023
83. Ren G, Zhang L, Zhao X, Xu G, Zhang Y, Roberts AI, et al. Mesenchymal stem cell-mediated immunosuppression occurs via concerted action of chemokines and nitric oxide. Cell Stem Cell. (2008) 2:141–50. doi: 10.1016/j.stem.2007.11.014
84. Bundgaard L, Stensballe A, Elbæk KJ, Berg LC. Mass spectrometric analysis of the in vitro secretome from equine bone marrow-derived mesenchymal stromal cells to assess the effect of chondrogenic differentiation on response to interleukin-1β treatment. Stem Cell Res Therapy. (2020) 11:187. doi: 10.1186/s13287-020-01706-7
85. Raikin SM, Garras DN, Krapchev PV. Achilles tendon injuries in a United States population. Foot Ankle Int. (2013) 34:475–80. doi: 10.1177/1071100713477621
86. Rajpar I, Barrett JG. Multi-differentiation potential is necessary for optimal tenogenesis of tendon stem cells. Stem Cell Res Therapy. (2020) 11:152. doi: 10.1186/s13287-020-01640-8
87. Lipman K, Wang C, Ting K, Soo C, Zheng Z. Tendinopathy: injury, repair, and current exploration. Drug Des Devel Ther. (2018) 12:591–603. doi: 10.2147/DDDT.S154660
88. Park SH, Lee HS, Young KW, Seo SG. Treatment of acute achilles tendon rupture. Clin Orthoped Surg. (2020) 12:1–8. doi: 10.4055/cios.2020.12.1.1
89. Tsekes D, Konstantopoulos G, Khan WS, Rossouw D, Elvey M, Singh J. Use of stem cells and growth factors in rotator cuff tendon repair. Eur J Orthopaed Surg Traumatol. (2019) 29:747–57. doi: 10.1007/s00590-019-02366-x
90. Cho WS, Chung SG, Kim W, Jo CH, Lee SU, Lee SY. Mesenchymal stem cells use in the treatment of tendon disorders: a systematic review and meta-analysis of prospective clinical studies. Ann Rehabil Med. (2021) 45:274–83. doi: 10.5535/arm.21078
91. van den Boom NAC, Winters M, Haisma HJ, Moen MH. Efficacy of stem cell therapy for tendon disorders: a systematic review. Orthopaed J Sports Med. (2020) 8:1–10. doi: 10.1177/2325967120915857
Keywords: tendon, cytokine, ultrafiltration probe, mesenchymal stem cell, licensing
Citation: Koch DW, Berglund AK, Messenger KM, Gilbertie JM, Ellis IM and Schnabel LV (2022) Interleukin-1β in tendon injury enhances reparative gene and protein expression in mesenchymal stem cells. Front. Vet. Sci. 9:963759. doi: 10.3389/fvets.2022.963759
Received: 07 June 2022; Accepted: 18 July 2022;
Published: 11 August 2022.
Edited by:
Chavaunne T. Thorpe, Royal Veterinary College (RVC), United KingdomReviewed by:
Sushmitha S. Durgam, The Ohio State University, United StatesDanae Zamboulis, Royal Veterinary College (RVC), United Kingdom
Copyright © 2022 Koch, Berglund, Messenger, Gilbertie, Ellis and Schnabel. This is an open-access article distributed under the terms of the Creative Commons Attribution License (CC BY). The use, distribution or reproduction in other forums is permitted, provided the original author(s) and the copyright owner(s) are credited and that the original publication in this journal is cited, in accordance with accepted academic practice. No use, distribution or reproduction is permitted which does not comply with these terms.
*Correspondence: Lauren V. Schnabel, lvschnab@ncsu.edu