- 1Dipartimento di Scienze Mediche Veterinarie, Università di Bologna, Bologna, Italy
- 2Vetagro S.p.A., Reggio Emilia, Italy
- 3Vetagro Inc., Chicago, IL, United States
Pharmacological doses of zinc oxide (ZnO) have been widely used in pig industry to control post-weaning diarrhea (PWD) symptoms exacerbated by enterotoxigenic Escherichia coli F4 infections. Because of environmental issues and regulatory restrictions, ZnO is no longer sustainable, and novel nutritional alternatives to manage PWD are urgently required. Botanicals represent a wide class of compounds employed in animal nutrition because of their diverse beneficial functions. The aim of this study was to investigate the in vitro protective action of a panel of essential oils and natural extracts on intestinal Caco-2 cells against an E. coli F4 infection. Moreover, we explored the potential mechanisms of action of all the botanicals compared to ZnO. Amongst the others, thyme essential oil, grape seed extract, and Capsicum oleoresin were the most effective in maintaining epithelial integrity and reducing bacterial translocation. Their mechanism of action was related to the modulation of cellular inflammatory response, the protection of tight junctions' expression and function, and the control of bacterial virulence, thus resembling the positive functions of ZnO. Moreover, despite their mild effects on the host side, ginger and tea tree essential oils provided promising results in the control of pathogen adhesion when employed during the challenge. These outcomes support the advantages of employing selected botanicals to manage E. coli F4 infections in vitro, therefore offering novel environmentally-friendly alternatives to pharmacological doses of ZnO capable to modulate host-pathogen interaction at different levels during PWD in pigs.
Introduction
Zinc oxide (ZnO) is a molecule widely employed at pharmacological doses in animal husbandry (1,000–3,000 ppm in complete feed) to treat post-weaning diarrhea (PWD). This condition mainly affects piglets, causing significant economic losses because of reduced animal growth performance, costs for treatments, and mortality (1). The onset of PWD is primarily linked to Enterotoxigenic Escherichia coli (ETEC) strain F4, which exploits piglets' weaning stress to target the gastrointestinal tract (2). ETEC overgrowth in the small intestine triggers the expression of several virulence genes, like bacterial adhesins or heat-labile and heat-stable toxins. Thus, pathogen easily adhere to enterocytes, where they secrete toxins to finally elicit the production of diarrhea (3). Studies demonstrated that E. coli F4 infection further impairs weaning stress by stimulating inflammation and reducing gut integrity in one of the most delicate phases of the pig production cycle (4–7).
It has been suggested that the reason why ZnO is particularly effective in managing PWD symptoms is related to its antimicrobial effect (8–10). However, whether it is true that ZnO possesses the capacity to reduce bacterial growth in vitro, several in vivo studies showed that antimicrobial effects against E. coli F4 are mild and limited to medicinal doses (11–13). Rather, the mechanism of action of ZnO seems broadly due to its antioxidant, anti-inflammatory, and anti-adhesive properties, together with its extensive supportive action on the intestinal mucosa (1, 14).
However, the large and prolonged utilization of pharmacological doses of ZnO in pig nutrition led to the onset of environmental issues and the acceleration of antimicrobial and heavy metal resistance spread amongst bacteria (15–17). For these reasons, European authorities decided to impose a ban on medicinal doses of ZnO (18), so novel alternatives to manage PWD and weaning stress are urgently required (19). The new substitutes should not only represent safe and sustainable ZnO replacements, but also embody the same multi-factorial properties that pharmacological ZnO exerts at the gut level.
A wide reservoir of potential novel molecules comes from nature, where a large number of bioactive compounds are innately synthesized by plants to protect themselves against pathogens and face stressful situations (20–22). Essential oils (EO) and powder extracts are widely studied in animal nutrition to support growth performance and control microbial growth (23, 24). Other than antibacterial activity, such compounds also exert anti-inflammatory and antioxidant actions, together with their capacity to enhance epithelial integrity and function at the intestinal level (25). All the effects of natural products are largely ascribed to the active principles they contain, such as polyphenols and terpenes (25, 26). The bioactive functions of these molecules generally follow the ones exerted by ZnO, so EO and extracts represent promising candidates to help piglets face weaning inflammation and stress, especially when triggered by E. coli F4 infections (1).
The aim of this study was to explore the protective effects of several EO and powder extracts during an in vitro infection by E. coli F4 on Caco-2 cells, a recognized model for intestinal studies (27). In particular, we tried to elucidate the potential mechanism of action of all the tested compounds, to identify the most effective to maintain enterocyte's monolayer integrity by modulating the inflammatory response, reducing cellular susceptibility to the pathogen, and affecting ETEC adhesive properties.
Materials and methods
Chemicals and reagents
Cell culture reagents and chemicals were obtained from Sigma-Aldrich (Milan, Italy), unless otherwise specified. Thyme essential oil (ThyEO, 48% thymol), Capsicum oleoresin (CapOR, 6% total capsaicinoids), ginger essential oil (GEO, 29–40% zingiberene, 10–14% sesquiphellandrene), and tea tree oil (TTO, >30% terpinene-4-ol, 5–13% α-terpinene, 10–28% γ-terpinene) were provided by Frey+Lau (Henstedt-Ulzburg, Germany). Grape seed extract (GSE, >80% total polyphenols as catechin equivalents at 280 nm—UV/VIS) and olive leaf extract (OE, 10% oleuropein) were obtained from Layn Natural Ingredients (Shangai, China). Zinc oxide (ZnO) was obtained from Alfa Aesar (Karlsruhe, Germany).
Stock solutions of all the bioactive compounds were prepared in ethanol 100% (v/v) and supplemented in culture medium at a final working concentration of ethanol ≤ 1% (v/v). Zinc oxide stock solution was prepared in 5% acetic acid (v/v) and supplemented in culture medium at a final working concentration of acetic acid ≤ 0.1% (v/v).
Final tested concentrations were 20 ppm for ThyEO, 100 ppm for GSE, CapOR, GEO, TTO, OE, and 0.2 mM for ZnO. For essential oils and extracts, the 100 ppm concentration was chosen basing on their effect on viability and their antioxidant potential previously assessed on unchallenged Caco-2 cells by our research group (28). ThyEO and ZnO concentrations differ from the others because higher doses were not tolerated by Caco-2 cells on 3.0 μm diameter Transwell® inserts, as shown by our preliminary experiments (see Supplementary Figures 1, 2), in which high ThyEO and ZnO alone (without bacterial challenge) produced a considerable drop in TER.
Cell line and culture conditions
The human colon carcinoma cell line (Caco-2) was obtained from DSMZ (Braunschweig, Germany). Cells were used at a passage between 10 and 20, and were routinary maintained at 37°C, 5% CO2 atmosphere, and 95% relative humidity. Basal maintenance medium was composed of Dulbecco's modified Eagle's medium (DMEM) high-glucose supplemented with 10% fetal bovine serum, 1% L-glutamine, 1% penicillin/streptomycin, and 1% non-essential amino acids.
Bacteria and culture conditions
The bacterium employed in the study was a field strain of Escherichia coli K88/F4, expressing heat-labile and heat-stable toxins (LT+, STa+, STb+), and originally isolated from the intestine of a piglet with post-weaning diarrhea. Bacteria were routinely cultured in brain-heart infusion broth (BHI, WVR International, Milan, Italy) at +37°C for 24 h. Daily 1:100 passage was performed to maintain active cultures (29).
On challenge day, bacterial inoculum was prepared by making a 1:80 passage of the overnight culture in a fresh BHI tube. After 4 h, when bacteria reached the late-midlog phase of growth, bacterial turbidity was measured by assessing absorbance at 630 nm. The obtained turbidity was interpolated in an absorbance—CFU/mL growth curve previously prepared to obtain a precise bacterial count for the inoculum of the following experiments, as previously described by Roselli et al. (14).
Infection of Caco-2 cells on porous filters
To measure Transepithelial Electrical Resistance (TER) and Bacterial Translocation (BT) during a bacterial challenge, Caco-2 cells were grown and differentiated for 30 days in 12 well plates on porous Transwell® inserts (3.0 μm diameter pores) (Corning, Massachusetts, USA) (30).
Infection method was adapted from previously published studies (14, 31, 32). Briefly, on the challenge day, TER was measured using an epithelial tissue voltohmmeter (Millicell ERS-2, Merck, Darmstadt, Germany) to obtain basal TER values for each filter. Then, cells were washed twice with DPBS and apically infected with 5 × 107 CFU/mL bacteria (multiplicity of infection of 100) in basal medium without P/S and supplemented with the various tested substances. Treatments were also included in the basolateral media, which consisted of basal media without P/S. Two controls were prepared: a negative control (CTR), without bacteria, and a positive control (CTR+), with only the bacteria.
At 2 and 4 h after the beginning of the infection, cellular integrity was monitored by measuring TER. At both timepoints, 100 μL of basolateral medium were collected from each filter and serially diluted in sterile saline. Then, aliquots of the most appropriate dilutions were seeded on BHI plates. After 24 h of incubation at +37°C, viable translocated bacteria were counted.
At the end of all the TER measurements, infection medium was removed, cells were washed twice with DPBS, harvested, and stored at −80°C until further processing for gene expression analysis.
Gene expression of infected Caco-2 cells
Gene expression was performed according to previously published studies on similar in vitro models of Caco-2 cells (28, 30). Briefly, after thawing of the harvested samples, Caco-2 RNA was extracted using NucleoSpin RNA Kit (Macherey-Nagel, Düren, Germany) with DNase digestion according to manufacturer's instructions. RNA yield and purity was assessed by A230, A260, and A280 nm measurements at the spectrophotometer (μDrop Plate and Varioskan LUX, Thermo Fisher Scientific, Waltham, MA, USA).
RNA was then retrotranscribed with iScript cDNA Synthesis Kit (Bio-Rad Laboratories, Hercules, CA, USA) according to manufacturer's instruction. cDNA was subsequently diluted to 5 ng/μL and analyzed via qPCR analysis. Reactions were prepared using iTaq Universal SYBR Green Supermix (Bio-Rad Laboratories, Hercules, CA, USA) and analyzed through CFX96 Real-Time PCR Detection System (Bio-Rad Laboratories, Hercules, CA, USA) under the following conditions: 3 min at 95°C, followed by 40 cycles of 95°C for 10 s and 60°C for 30 s. The specificity of each reaction was evaluated by melting-curve analysis.
Gene expression was normalized using two reference genes, i.e., ribosomal protein lateral stalk subunit P0 (RPLP0) and glyceraldehyde-3-phosphate dehydrogenase (GAPDH). The relative changes in gene expression were calculated using the 2−ΔΔCt method (33).
Table 1 displays forward and reverse primers of selected target genes, which were obtained from Merck (Darmstadt, Germany).
Adhesion assay
As a marker of bacterial virulence, an adhesion assay was performed to assess bacterial ability to interact with target cells (14). Caco-2 cells were differentiated in 24 well plates; on the infection day, after two washes with DPBS, Caco-2 were infected with 5 × 107 CFU/mL bacteria in basal medium without P/S and supplemented with the various tested substances. Two controls were prepared: a negative control (CTR), without bacteria, and a positive control (CTR+), with only the bacteria.
After 1 h of incubation, bacteria in suspension were eliminated by washing cells four times with DPBS. Then, Caco-2 were lysed with 0.5% Triton X-100 in DPBS for 10 min and, after serially diluting lysed cells in sterile saline, appropriate dilutions were seeded on BHI agar. After 24 h of incubation at +37°C, viable adhered bacteria were counted.
Immunofluorescence assay
To assess the localization of zonula occludens 1 (ZO-1), a tight junction protein, during the bacterial challenge, Caco-2 cells were stained via an immunofluorescence assay. Cells were cultivated on glass coverslips maintained inside 6 well plates, with each well corresponding to a single group of treatment.
After differentiation, Caco-2 cells were washed twice with DPBS, and then infected with 5 × 107 CFU/mL bacteria in basal medium without P/S and supplemented with the various tested substances. Two controls were prepared: a negative control (CTR), without bacteria, and a positive control (CTR+).
After 2 h of incubation, bacteria in suspension were eliminated and cells washed twice with DPBS. Then, Caco-2 were fixed with 4% paraformaldehyde in DPBS for 20 min. Subsequently, cells were permeabilized with 0.5% Triton X-100 for 15 min, and then blocked in 10% goat serum for 1 h. ZO-1 primary monoclonal antibody (ThermoFisher Scientific, Walthan, MA, USA) was diluted in DPBS containing 2% bovine serum albumin (BSA) and 0.05% saponins, and incubated on Caco-2 cells for 3 h at +4°C in a humidified chamber. After three washes with 0.2% BSA + 0.05% saponins in DPBS, secondary antibody conjugated to fluorescein isothiocyanate (FITC) (ThermoFisher Scientific, Walthan, MA, USA) was used to probe for 1 h the bounded primary antibody. Two washes were finally performed with DPBS supplemented with 0.2% BSA and 0.05% saponins to remove unbounded antibodies. Slides were mounted with Fluoroshield containing 4′,6-diamidino-2-phenylindole (DAPI), images acquired using a fluorescence microscope (Nikon Corporation, Tokyo, Japan), and pictures processed using NIS-Elements software (Nikon Corporation, Tokyo, Japan).
Statistical analysis
Results are obtained from at least two independent experiments. For each experiment, the experimental unit was the well, with n = 6. Data are displayed on graphs as means ± SEM. For TER, BT, gene expression, and adhesion assays, data were analyzed using GraphPad Prism v.9.4.0 (GraphPad Software, Inc., San Diego, CA, USA) performing One-Way ANOVA analysis with Dunnett multiple comparisons test, comparing all the experimental groups with the mean of CTR+ group. Differences were considered significant when p ≤ 0.05, trends were identified when p ≤ 0.1.
Results
Epithelial integrity
Figure 1 reports the TER measurements of Caco-2 cells treated with selected compounds during an E. coli F4 challenge. Results are presented as percentage of TER referred to the negative control group, considered as the normal TER value in a steady condition of culture.
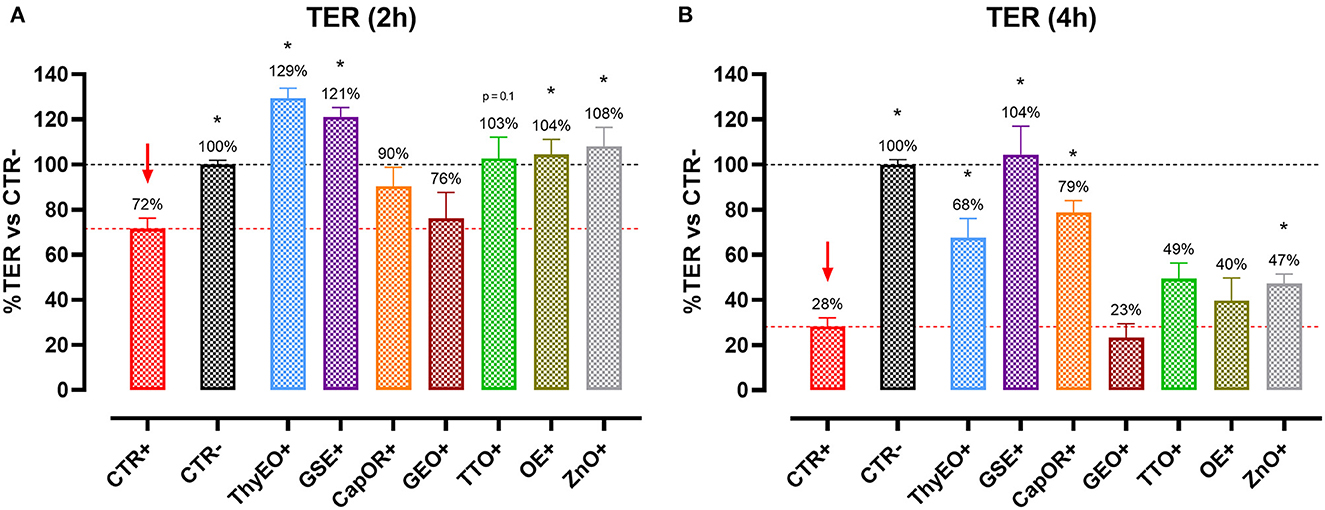
Figure 1. TER of Caco-2 cells treated with essential oils or powder extracts and challenged with E. coli F4 at 2 h (A) and 4 h (B) after the beginning of the infection. Groups with bacterial infection are represented with a “+” in the name. Data in the graphs are represented as means ± SEM; percentage values are referred to negative control (CTR–) for both the investigated timepoints. One-Way ANOVA analysis is performed against positive control (CTR+), identified with a red arrow; asterisks “*” denote significant differences with p < 0.05, while tendencies are highlighted by their p-value.
Data show that the ETEC challenge could significantly reduce the integrity of the cellular monolayer at both 2 h and 4 h after the beginning of the infection. At 4 h, considerable damages on infected Caco-2 led TER to a value equal to 28% of the negative control.
Despite the presence of the challenge, several treatments were effective in protecting Caco-2 cells from the drop in TER exerted by E. coli F4. In particular, ThyEO and GSE kept TER at a level significantly higher than the positive control, with values even greater than the negative control at the first timepoint (129% for ThyEO+ and 121% for GSE+ groups). Moreover, CapOR limited the drop in TER that Caco-2 cells would have experienced at 4 h (79% of CapOR+ instead of 28% of CTR+). Also, ZnO significantly protected cells from the effects of the challenge, even if its action was considerable at 2 h (108%), but mild at 4 h (47%). Similar behaviors were registered also for TTO and OE, while GEO did not show significant improvements in TER at both timepoints.
Bacterial translocation
To understand the capacity of the tested bioactive compounds to limit E. coli F4 passage across the cellular monolayer, a bacterial translocation assay was performed. Results are presented in Figure 2 and expressed as a percentage of bacterial translocation referred to the value obtained at each timepoint by CTR+, considered the highest possible passage in our system.
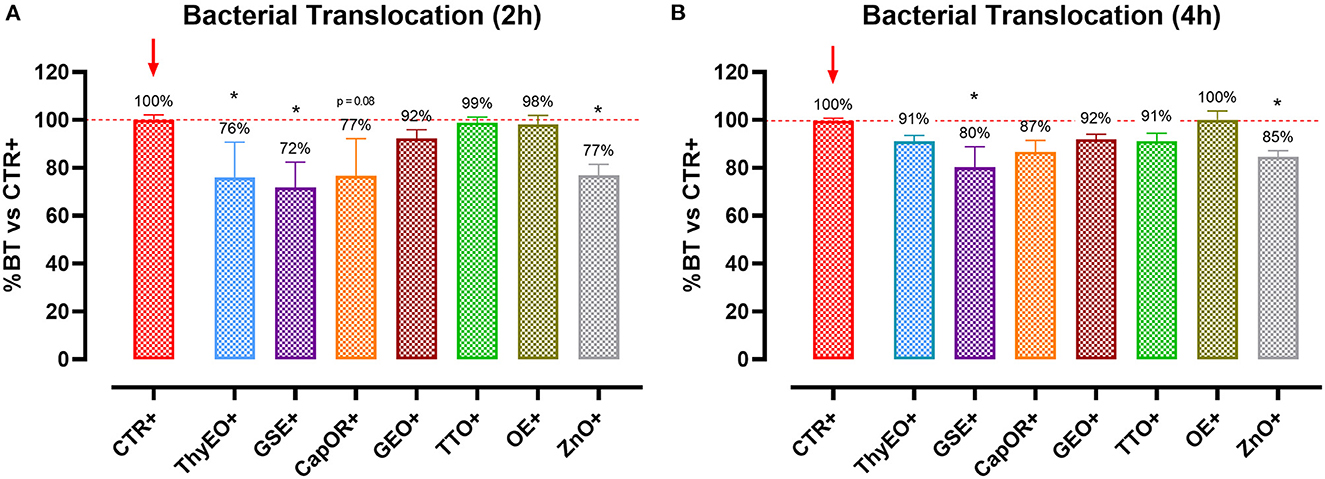
Figure 2. Bacterial translocation of E. coli F4 across Caco-2 cells treated with essential oils or powder extracts at 2 h (A) and 4 h (B) after the beginning of the bacterial infection. Groups with bacterial infection are represented with a “+” in the name. Data in the graphs are represented as means ± SEM; percentage values are referred to positive control (CTR+) for both the investigated timepoints. One-Way ANOVA analysis is performed against positive control (CTR+), identified with a red arrow; asterisks “*” denote significant differences with p < 0.05, while tendencies are highlighted by their p-value.
Results show that ThyEO and GSE significantly reduced bacterial passage across Caco-2 cells at 2 h (−24 and −28%, respectively), with mean values numerically close to the ones of ZnO (77% of CTR+). A reduction trend was measured for CapOR, while other treatments, despite improving TER, did not significantly reduce bacterial translocation.
At 4 h differences are less evident, even if GSE significantly reduced E. coli F4 passage at the same extent of ZnO (−20 and −15%, respectively). Other treatments, like ThyEO, CapOR, TTO, and GEO, could only numerically reduce bacterial translocation, while OE did not help cells to avoid pathogen passage across the enterocyte monolayer.
Gene expression of tight junctions and inflammatory markers
Infected cells were harvested and mRNA levels investigated to monitor the ability of the tested bioactive principles to modulate the expression of tight junctions (Figure 3) and innate immune response markers (Figure 4). Tight junction integrity was assessed by investigating the expression of zonula occludens 1 (ZO-1), zonula occludens 2 (ZO-2), and claudin 1 (CLD-1) genes, while immune response was evaluated by analyzing the expression of cytokines like TNFα, IL-1β, IL-8, and beta defensin 1 (BD-1).
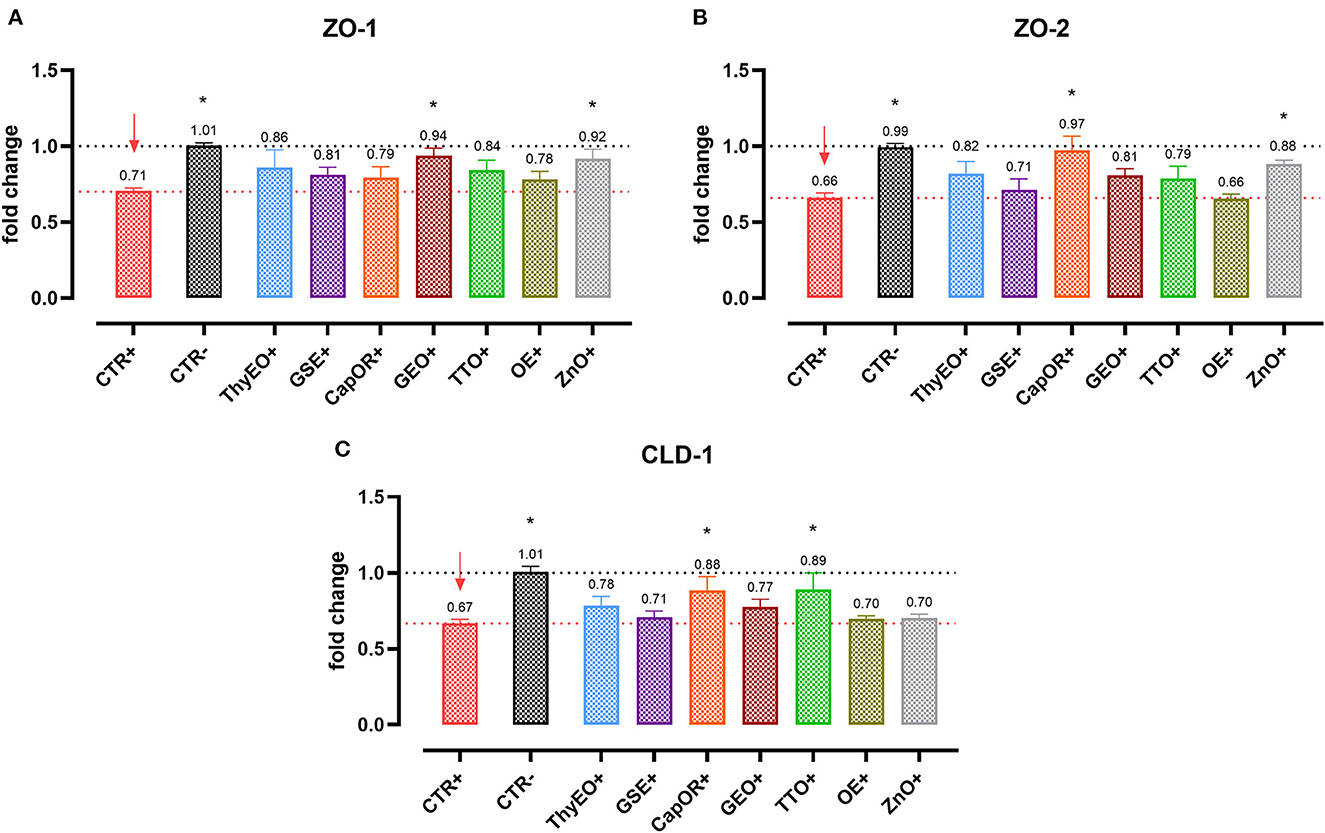
Figure 3. Gene expression analysis of Caco-2 cells treated with essential oils or powder extracts and challenged with E. coli F4 at 4 h after the beginning of the infection. The analyzed markers of cellular monolayer integrity are ZO-1 (A), ZO-2 (B), and CLD-1 (C). Groups with bacterial infection are represented with a “+” in the name. Data in the graphs are represented as means ± SEM. One-Way ANOVA analysis is performed against positive control (CTR+), identified with a red arrow; asterisks “*” denote significant differences with p < 0.05, while tendencies are highlighted by their p-value.
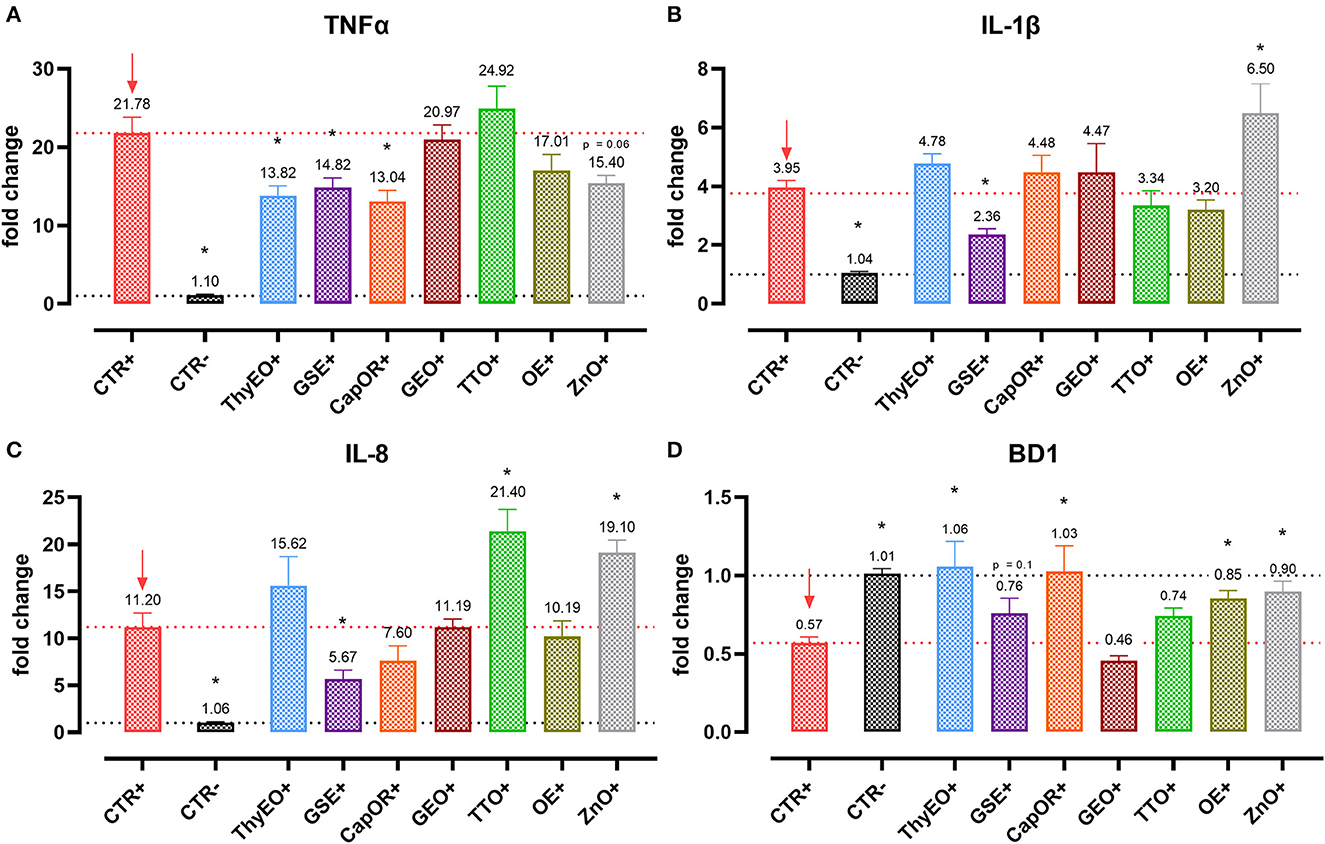
Figure 4. Gene expression analysis of Caco-2 cells treated with essential oils or powder extracts and challenged with E. coli F4 at 4 h after the beginning of the infection. The analyzed markers of innate immune response are TNFα (A), IL-1β (B), IL-8 (C), and BD1 (D). Groups with bacterial infection are represented with a “+” in the name. Data in the graphs are represented as means ± SEM. One-Way ANOVA analysis is performed against positive control (CTR+), identified with a red arrow; asterisks “*” denote significant differences with p < 0.05, while tendencies are highlighted by their p-value.
Escherichia coli F4 challenge significantly reduced the expression of the three intestinal integrity markers involved in the organization of tight junctions. On the contrary, many of the treatments improved the expression of the three considered genes, even if at different extents. ZO-1 expression was ameliorated by GEO (+32%), while ZO-2 levels were enhanced by CapOR (+47%); ZnO increased mRNA levels of the two markers. Conversely, while ZnO did not improve CLD-1 expression, both CapOR and TTO significantly increased CLD-1 (+32%) compared to CTR+. ThyEO could numerically improve the levels of the three tight junctional markers, even if differences were not significant.
Despite not substantially ameliorating the expression of ZO-1, ZO-2, and CLD-1, GSE significantly decreased TNFα (−32%), IL-1β (−40%), and IL-8 (−50%), counteracting their dramatic activation when an E. coli F4 challenge was applied to Caco-2 cells. TNFα levels were also reduced by ThyEO (−37%) and CapOR (−40%), while ZnO tended to decrease its expression as well. On the other hand, TTO, ZnO and, to a lesser extent, ThyEO, increased the mRNA amount of IL-8 compared to CTR+, while ZnO also significantly augmented IL-1β levels.
Even though the bacterial challenge reduced the mRNA levels of BD1, ThyEO, CapOR, OE, ZnO, and GSE improved or restored its expression at the same value of the negative control.
Adhesion assay
To investigate the ability of the employed essential oils and powder extracts to influence bacterial virulence by modulating their interaction with target cells, an adhesion assay was performed. Results are displayed by Figure 5. All the compounds significantly reduced the capacity of E. coli F4 to adhere to Caco-2 cells, except for OE. The most effective ones were ThyEO and GEO, followed by GSE. CapOR, ZnO, and TTO significantly reduced the adhesion of the pathogen as well, even if to a lesser extent.
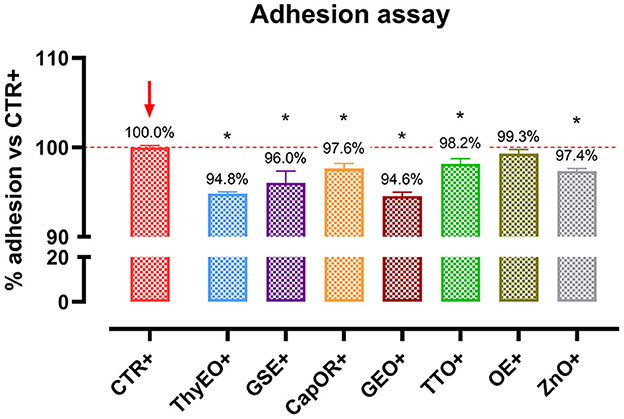
Figure 5. Escherichia coli F4 adhesion assay performed on Caco-2 cells treated with essential oils or powder extracts for 1 h. Groups with bacterial infection are represented with a “+” in the name. Data in the graphs are represented as means ± SEM. One-Way ANOVA analysis is performed against positive control (CTR+), identified with a red arrow; asterisks “*” denote significant differences with p < 0.05.
Immunofluorescence assay for ZO-1
The immunofluorescence staining for ZO-1 is shown in Figures 6, 7. The infection of Caco-2 with E. coli F4 produced significant damages on the cellular monolayer. In particular, intestinal epithelial cells suffered from ZO-1 loss of sealing capacity, producing open holes between adjacent cells. Moreover, bacterial challenge caused areas of significant mortality, with cells detaching from the surface of glass slides, generating acellular zones. Finally, in some areas, ZO-1 lost its correct localization, to form irregular agglomerates near cell borders, differing from the homogeneous and uniform pattern usually highlighted in unchallenged Caco-2 enterocytes.
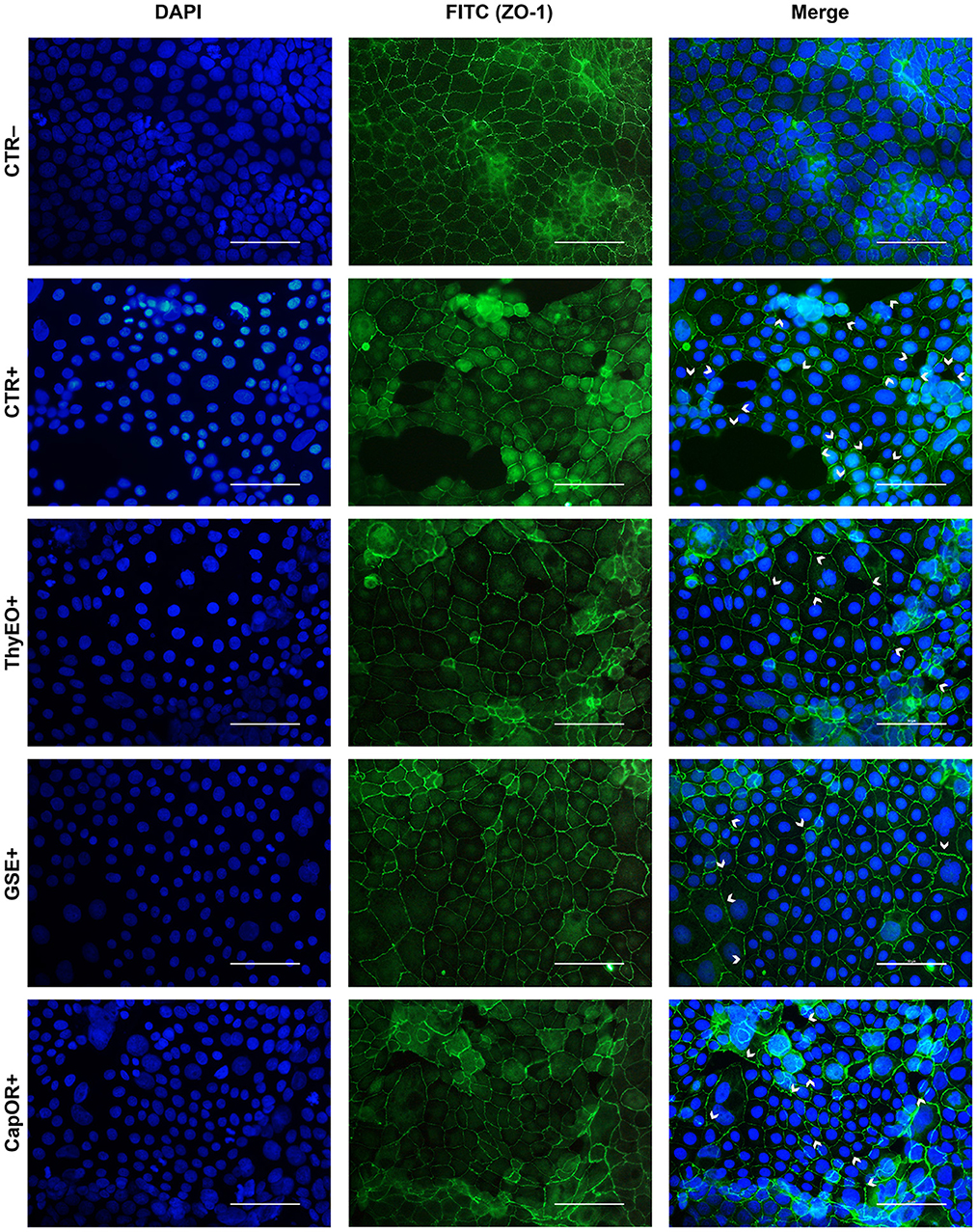
Figure 6. Immunofluorescence staining of Caco-2 cells treated with essential oils or powder extracts and simultaneously challenged with E. coli F4 for 2 h. Groups with bacterial infection are represented with a “+” in the name. In the image, the left column represents DAPI staining, the central column ZO-1 staining with FITC, while right column depicts the merge of the first two images, where white arrows identify areas of tight-junction detachment, loss of cells, holes in the monolayer or anomalies in ZO-1 disposition. Each row displays a different treatment.
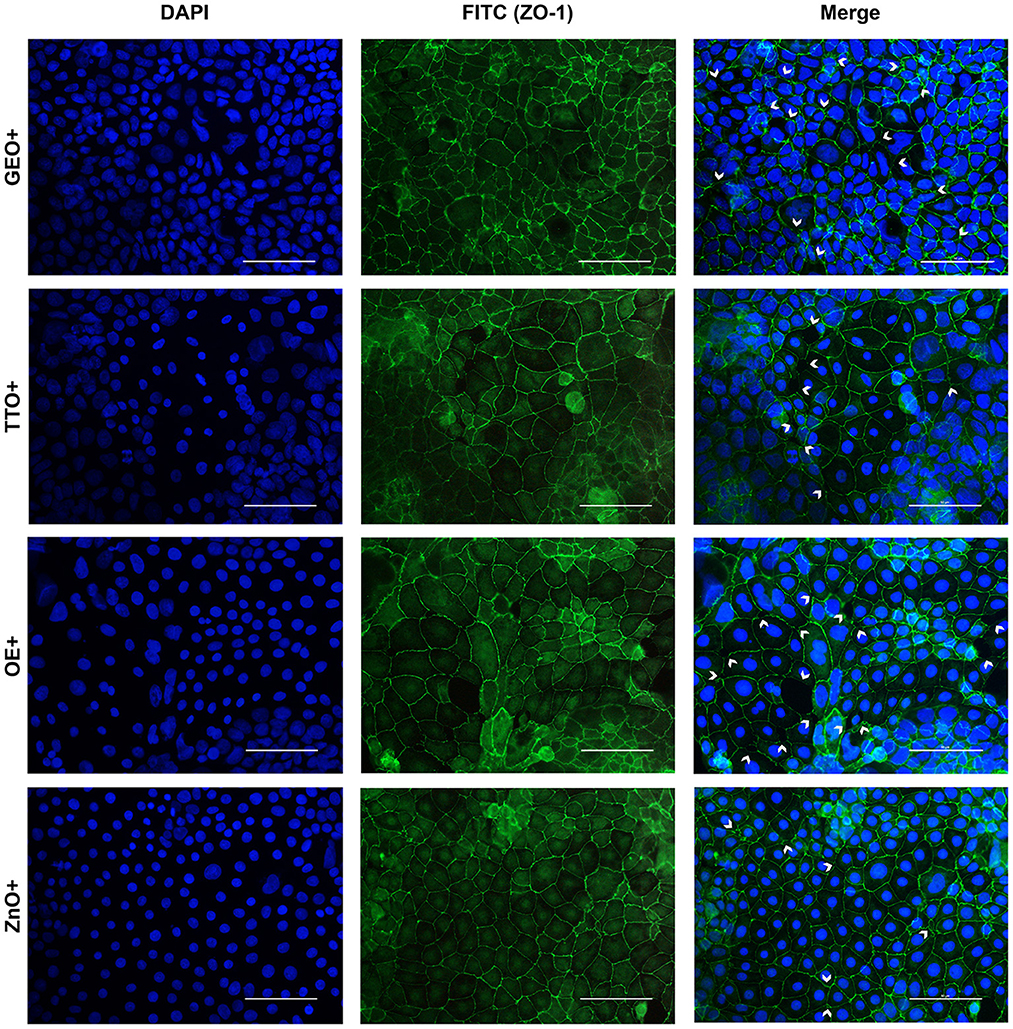
Figure 7. Immunofluorescence staining of Caco-2 cells treated with essential oils or powder extracts and simultaneously challenged with E. coli F4 for 2 h. Groups with bacterial infection are represented with a “+” in the name. In the image, the left column represents DAPI staining, the central column ZO-1 staining with FITC, while right column depicts the merge of the first two images, where white arrows identify areas of tight-junction detachment, loss of cells, holes in the monolayer or anomalies in ZO-1 disposition. Each row displays a different treatment.
Amongst treatments, ZnO significantly reduced the damages exerted by E. coli F4 infection: the integrity of Caco-2 monolayer was maintained, with only minor sites of ZO-1 misplacement or loss of tightness. Similar results were obtained with GSE, while ThyEO and CapOR, despite showing some areas of tight junction disruption, were generally effective in preventing profound damages or extensive losses of cell-to-cell contacts.
On the contrary, broader damages were observed for TTO and OE: a higher number of areas left opened between adjacent cells were found with TTO and OE treatments, together with zones of partial impairment in ZO-1 localization, even if not at the same extent of CTR+.
Despite showing a high degree of cellularity, without evident holes in the Caco-2 monolayer, treatment with GEO during the bacterial challenge did not protect cells from alterations to ZO-1 disposition along the cellular margins. In fact, sites of cell-to-cell contact loss were evident, together with frequent zones of ZO-1 abnormal localization, far from the common regular arrangement with sharp cellular borders.
Discussions
Historically, pharmacological doses of ZnO were widely employed during the weaning phase of piglets to prevent the onset of PWD symptoms (34, 35). For a long time, it was believed that ZnO had a strong antimicrobial activity, but several studies demonstrated a remarkable action only at very high doses, principally against Gram-positive species (36, 37). Indeed, many in vivo trials reported how ZnO exerts mild effects on the fecal excretion of E. coli F4, the Gram-negative pathogen mainly responsible of PWD in piglets (38).
The ZnO mechanism of action seems rather related to a multifactorial effect on small intestinal enterocytes, the main target of ETEC infections (1). As confirmed by our results, ZnO protects cultured intestinal cells against E. coli F4 by maintaining a better epithelial integrity, thus reducing bacterial translocation across the cellular monolayer. The increased expression of selected tight-junctions, the modulation of inflammatory cytokines expression, and the enhanced levels of beta defensins, all contribute to explain the higher resilience that Caco-2 cells have when treated with ZnO during a bacterial challenge. Moreover, consistently with the outcomes reported by Roselli et al. (14), ZnO significantly reduced the count of viable E. coli F4 adhered to enterocytes, since it interferes with bacterial adhesins expression and function (39).
Despite their powerful activity, pharmacological doses of ZnO are no longer sustainable because of the risks related to their extensive use in animal husbandry (17). To overcome these issues, novel environmentally friendly alternatives to support piglets' weaning transition at the nutritional level are urgently needed. Natural extracts represent ideal candidates to substitute ZnO because the bioactive molecules they convey possess diverse mechanisms of action which modulate pathogens growth, while simultaneously supporting intestinal morphology and function (25, 32).
Maintaining intestinal integrity is a key factor to ensure piglets health and a smoother weaning transition: the loss of the gastrointestinal barrier elicits the establishment of a mild inflammatory status which results in the onset of diarrhea (40–43). Our infection model proved that, when E. coli F4 was incubated on Caco-2 cells with ThyEO, GSE, and CapOR, the damages of the challenge were significantly reduced, with TER levels in line with negative control. Thanks to the enhancement of the barrier integrity, a reduced bacterial translocation was also reported for the three extracts. Data are supported by the immunofluorescence staining of infected cells: a significant reduction of damages and an improved tight junction function was registered when ThyEO, GSE, and CapOR were added to the system, in agreement with ZnO. The positive effects of the three botanicals can be ascribed to their mechanisms of action.
Intestinal integrity is ensured by a complex system of sealing proteins that form tight junctions between adjacent cells and strictly regulate paracellular pathways (44). Two of the main actors are zonula occludens and claudins (45, 46). Escherichia coli F4 deeply affects the structure and functionality of these proteins: studies showed that ETEC toxins disrupt junctions by targeting ZO-1, CLD-1, and many cytoskeletal components (4, 7, 47). This is confirmed by our results: the pathogen impaired the expression of all the analyzed markers. However, when CapOR was added to the system, it increased the expression of CLD-1 and ZO-2, showing the ability to modulate two markers of intestinal integrity as occurs with ZnO.
Capsaicin, the main component of CapOR, transiently increases tight junction permeability without affecting their structure (48). This phenomenon is also reported by our data: at 2 h after the beginning of the infection, CapOR reported a lower TER, if compared to several other treatments. However, at 4 h, TER was maintained at a high level, demonstrating how the opening of the paracellular way is only temporary, and that it is reversed by an increase in tight junction expression (49, 50), as also displayed by the qPCR results. The CapOR transient loosening in epithelial integrity is not due to a disruption of cellular monolayer but, instead, it is tightly regulated (51–53): despite the slight drop in TER at 2 h, bacterial translocation still showed a reduction trend compared to CTR+.
The protective action of CapOR on tight junctions during E. coli F4 challenge is likely related to its main bioactive principle, capsaicin, the ligand of TRPV1 channels (54). Activation of TRPV1 by capsaicin reduced inflammation in LPS-challenged mice (55). Moreover, after binding TRPV1, capsaicin modulated the inflammatory activation in human endothelial cells treated with LPS, resulting in a decreased cytokine release (56). Consistently, our results show that CapOR controlled the upregulation of TNFα and IL-8 expression during an E. coli F4 challenge. This is in agreement with the findings of Alhamoruni et al., that confirmed how TRPV1 agonists protect Caco-2 intestinal cells from the increased permeability generated by high pro-inflammatory cytokines levels (57).
Slight numerical increases in tight junctions' expression and a modulation of TNFα was registered also for ThyEO during the E. coli F4 infection on Caco-2 cells. ThyEO is particularly rich in thymol, a bioactive compound recognized as a candidate agonist of TRPV1 (58) and a direct ligand of TRPV3 (59). Thymol's ability to interact with the endocannabinoid system and stimulate its anti-inflammatory potential could explain its ability to counteract ETEC negative effects. Thymol has also a direct action on the cellular inflammatory response, because its phenolic hydroxyl group interacts with NF-kB, a transcription factor involved in the response to stressful stimuli (60, 61) and associated with the MAPK pro-inflammatory pathway (62–64).
Other than modulating cellular inflammatory response, thymol deeply influences bacterial virulence genes expression (65, 66). Several studies demonstrated how thymol not only limits bacterial growth, but also controls E. coli F4 quorum sensing effectors, reducing ETEC ability to target intestinal cells (29, 67). This secondary mechanism of action against pathogens could explain why ThyEO did not exert extensive effects on Caco-2 cells, though keeping high TER values. Instead of exclusively acting on the intestinal mucosa side, thymol effect could be also oriented toward bacterial virulence, as previously demonstrated with Salmonella typhimurium (68). As a confirmation, thymol reduced the adhesion of the pathogen to Caco-2 cells at an extent higher than ZnO.
A similar effect is registered also for GSE, an extract rich in polyphenols. Traditionally, polyphenols were considered antinutritional factors because of their ability to bind proteins (69, 70). However, since many bacterial toxins are proteins, researchers have explored the impact of polyphenols against bacteria (71–73). Polyphenols are effective in inhibiting Vibrio cholerae CT toxin by preventing its cellular internalization (74–76). These results are of particular interest since CT toxin shares a high sequence homology to ETEC LT toxins (74, 77), and contribute to explain why GSE protected Caco-2 cells from the E. coli F4 infection at levels close to ZnO. Moreover, since polyphenol-rich extracts are able to control the expression of E. coli virulence as well (78), it is possible that the bacterial adhesion reduction in the GSE-treated Caco-2 cells is due to polyphenols' ability to interfere with ETEC quorum sensing systems.
Phenolic compounds from grape by-products embody a wide number of antioxidant molecules (79). Bacterial components such as LPS, after binding TLR4 receptors, induce an inflammatory response: the stimulation of transcription factors like NF-kB triggers oxidative enzymes and the uncontrolled accumulation of ROS (80, 81). In turn, ROS promote the inflammasome formation, creating a self-amplifying cycle that dramatically impairs cellular health (82). Grape by-products such as GSE directly detoxify ROS species and break this pro-inflammatory loop (83), improving cellular integrity, as highlighted by our results. The increased variability registered for this result might be due to the indirect effect that this extract exerts against the bacterial infection: the degree of activation of anti-virulence and anti-inflammatory responses relies on complex pathways that might produce a higher variability in the final response if compared to compounds that also possess more direct mechanisms of action such as the antimicrobial one, as happens for ThyEO.
Our study showed that GSE significantly downregulated TNFα, IL-1β, and IL-8 pro-inflammatory cytokines during E. coli F4 infection. This effect is due to the capacity of polyphenols to inhibit NF-kB by repressing the phosphorylation and elimination of IkB (84–86), the peptide that prevents NF-kB translocation into the nucleus. This is probably due to the scavenging ability of GSE polyphenols: by limiting ROS production, IkB phosphorylation is reduced, and NF-kB translocation is repressed (81). Furthermore, polyphenols activate Nrf2, a transcription factor that triggers several antioxidant enzymes (87). That is, polyphenols promote the degradation of the Nrf2 inhibitor Keap1 and increase Nrf2 nuclear translocation, revealing their dualistic effect in controlling both ROS production and the inflammatory cellular response (88, 89).
The supplementation of GEO, TTO, or OE did not significantly help cells against the E. coli F4 challenge. While some TER improvements were registered for TTO and OE at 2 h, the same were quickly lost at 4 h: the extracts were not able to maintain the positive effects during time. The results are confirmed by ETEC translocation, proving that structural damages to intestinal cells were not prevented. As a matter of fact, more irregular zones of ZO-1 distribution were observed in GEO, TTO, and OE groups, showing structural impairments to tight junctions. Their disposition, with ruffles and spikes, is in fact correlated with a higher epithelial permeability (90).
The reason why OE did not exert positive effects during the E. coli F4 infection is probably related to its main active principle, oleuropein. As an anticancer agent (91), oleuropein might dramatically exacerbate cellular apoptosis and autophagy, two natural tightly-regulated defense mechanisms of intestinal cells against ETEC (92), vanishing the mild antioxidant effect that the extract has (93). This hypothesis is corroborated by the high bacterial translocation and the loss of cells evidenced by the immunofluorescence staining of OE-infected enterocytes.
TTO and GEO are well known for their antioxidant and anti-inflammatory activity (94–97). However, our data demonstrate that, during an E. coli F4 infection, the two extracts could neither reduce cytokine expression, nor extensively restore tight-junction or defensin levels, except for TTO with CLD-1. It is possible that the experimental timeframe did not allow TTO and GEO to exert their beneficial effect on Caco-2 cells. Moreover, as previously demonstrated, their antioxidant potential is not as high as the one of ThyEO, GSE, or CapOR: during an oxidative challenge, TTO and GEO reduced ROS levels, but not at the same extent of the other extracts (28). This also depends on the exact composition of the essential oils employed, because different extracts harbor diverse bioactive principles at unique ratios.
Nevertheless, both GEO and TTO show interesting properties related to the control of E. coli F4 virulence (98–100). This is confirmed by our outcomes: even if they were not able to strongly help cells against the challenge, they still reduced bacterial adhesion to Caco-2 enterocytes, suggesting a potential modulation of ETEC adhesins. Further studies might be directed toward the confirmation of this mechanism of action and could also assess if GEO and TTO might be more effective as preventive treatments to prime intestinal cells before an infection.
Conclusions
In conclusion, despite individual differences, ThyEO, GSE, and CapOR, were all effective in controlling E. coli F4 infection on intestinal enterocytes, showing peculiar mechanisms of action that mimic the ones of ZnO. While ThyEO had stronger effects on pathogen control itself and the production of defensins, GSE effectively mitigated the inflammatory response, and CapOR markedly re-established the expression of tight junctions, thus acting at many levels in the host-pathogen interaction. These outcomes support the utilization of botanicals to manage PWD in piglets, and future studies should now explore their effectiveness also in vivo. Furthermore, other researches could also evaluate the benefits of employing technologies like microencapsulation to ensure the delivery of unaltered botanicals' bioactive principles, preventing unwanted gastric modifications and enabling a more precise control of their inclusion in the feed. Other investigations might include the evaluation of their combination, to analyse if synergistic effects would enhance and complement the action of each single extract. Moreover, it would be interesting to explore how such botanicals could prepare cells to better react to E. coli F4, or to recover after a bacterial challenge.
Data availability statement
The raw data supporting the conclusions of this article will be made available by the authors, without undue reservation.
Author contributions
AB participated to the conceptualization of the research, performed the experiments, and wrote the original draft of the manuscript. EG conceptualized the research, participated during the investigation, reviewed the manuscript, and supervised the research. AP supervised the research. All authors approved the final version of the manuscript.
Funding
This research was supported by a Grant from Vetagro S.p.A.
Conflict of interest
AP serves as a professor at the University of Bologna and is a member of the board of directors of Vetagro S.p.A. EG serves as an assistant professor at the University of Bologna and is a member of the board of directors of Vetagro Inc.
The remaining author declares that the research was conducted in the absence of any commercial or financial relationships that could be construed as a potential conflict of interest.
Publisher's note
All claims expressed in this article are solely those of the authors and do not necessarily represent those of their affiliated organizations, or those of the publisher, the editors and the reviewers. Any product that may be evaluated in this article, or claim that may be made by its manufacturer, is not guaranteed or endorsed by the publisher.
Supplementary material
The Supplementary Material for this article can be found online at: https://www.frontiersin.org/articles/10.3389/fvets.2023.1141561/full#supplementary-material
References
1. Bonetti A, Tugnoli B, Piva A, Grilli E. Towards zero zinc oxide: feeding strategies to manage post-weaning diarrhea in piglets. Animals. (2021) 11:1–24. doi: 10.3390/ani11030642
2. Luppi A. Swine enteric colibacillosis: diagnosis, therapy and antimicrobial resistance. Porcine Health Manag. (2017) 3:1–18. doi: 10.1186/s40813-017-0063-4
3. Dubreuil JD, Isaacson RE, Schifferli DM. Animal enterotoxigenic Escherichia coli. EcoSal Plus. (2016) 7:1–47. doi: 10.1128/ecosalplus.ESP-0006-2016
4. Johnson AM, Kaushik RS, Hardwidge PR. Disruption of transepithelial resistance by enterotoxigenic Escherichia coli. Vet Microbiol. (2010) 141:115–9. doi: 10.1016/j.vetmic.2009.08.020
5. Kern M, Günzel D, Aschenbach JR, Tedin K, Bondzio A, Lodemann U. Altered cytokine expression and barrier properties after in vitro infection of porcine epithelial cells with enterotoxigenic Escherichia coli and probiotic Enterococcus faecium. Mediators Inflamm. (2017) 2017:8192. doi: 10.1155/2017/2748192
6. Bahrami B, Macfarlane S, Macfarlane GT. Induction of cytokine formation by human intestinal bacteria in gut epithelial cell lines. J Appl Microbiol. (2011) 110:353–63. doi: 10.1111/j.1365-2672.2010.04889.x
7. Dubreuil JD. Enterotoxigenic Escherichia coli targeting intestinal epithelial tight junctions: an effective way to alter the barrier integrity. Microb Pathog. (2017) 113:129–34. doi: 10.1016/j.micpath.2017.10.037
8. Pasquet J, Chevalier Y, Pelletier J, Couval E, Bouvier D, Bolzinger MA. The contribution of zinc ions to the antimicrobial activity of zinc oxide. Colloids Surf A Physicochem Eng Asp. (2014) 457:263–74. doi: 10.1016/j.colsurfa.2014.05.057
9. Söderberg TA, Sunzel B, Holm S, Elmros T, Hallmans G, Sjöberg S. Antibacterial effect of zinc oxide in vitro. Scand J Plast Reconstr Surg Hand Surg. (1990) 24:193–7. doi: 10.3109/02844319009041278
10. Yousef MJ, Danial EN. In vitro antibacterial activity and minimum inhibitory concentration of zinc oxide and nano-particle zinc oxide against pathogenic strains. Int J Health Sci. (2012) 2:38–42. doi: 10.5923/j.health.20120204.04
11. Mores N, Cristani J, Piffer IA, Barioni W Jr, Lima GMM. Effects of zinc oxide on postweaning diarrhea control in pigs experimentally infected with E. coli. Arq Bras Med Vet Zootec. (1998) 50:513–23.
12. Katouli M, Melin L, Jensen-Waern M, Wallgren P, Möllby R. The effect of zinc oxide supplementation on the stability of the intestinal flora with special reference to composition of coliforms in weaned pigs. J Appl Microbiol. (1999) 87:564–73. doi: 10.1046/j.1365-2672.1999.00853.x
13. Højberg O, Canibe N, Poulsen HD, Hedemann MS, Jensen BB. Influence of dietary zinc oxide and copper sulfate on the gastrointestinal ecosystem in newly weaned piglets. Appl Environ Microbiol. (2005) 71:2267–77. doi: 10.1128/AEM.71.5.2267-2277.2005
14. Roselli M, Finamore A, Garaguso I, Britti MS, Mengheri E. Zinc oxide protects cultured enterocytes from the damage induced by Escherichia coli. J Nutr. (2003) 133:4077–82. doi: 10.1093/jn/133.12.4077
15. Ciesinski L, Guenther S, Pieper R, Kalisch M, Bednorz C, Wieler LH. High dietary zinc feeding promotes persistence of multi-resistant E. coli in the swine gut PLoS ONE. (2018) 13:e0191660. doi: 10.1371/journal.pone.0191660
16. Gräber I, Hansen JF, Olesen SE, Petersen J, Øtergaard HS, Krogh L. Accumulation of copper and zinc in Danish agricultural soils in intensive pig production areas. Geografisk Tidsskrift. (2005) 105:15–22. doi: 10.1080/00167223.2005.10649536
17. EMA CVMP. EMA - Zinc Oxide - Annex II - Scientific Conclusions Grounds for the Refusal of the Marketing Authorisation for Withdrawal of the Existing Marketing Authorisations. Amsterdam (2017). Available online at: https://www.ema.europa.eu/en/medicines/veterinary/referrals/zinc-oxide (accessed October 12, 2019).
18. European Commission. Commission implementing decision of 26.6.2017 concerning. In: The Framework of Article 35 of Directive 2001/82/EC of the European Parliament and of the Council, the Marketing Authorisations for Veterinary Medicinal Products Containing “Zinc Oxide” to be Ad. Brussels: European Commission (2017).
19. Rhouma M, Fairbrother JM, Beaudry F, Letellier A. Post weaning diarrhea in pigs: risk factors and non-colistin-based control strategies. Acta Vet Scand. (2017) 59:1–19. doi: 10.1186/s13028-017-0299-7
20. Bakkali F, Averbeck S, Averbeck D, Idaomar M. Biological effects of essential oils - a review. Food Chem Toxicol. (2008) 46:446–75. doi: 10.1016/j.fct.2007.09.106
21. Zhai H, Liu H, Wang S, Wu J, Kluenter A-M. Potential of essential oils for poultry and pigs. Anim Nutr. (2018) 4:179–86. doi: 10.1016/j.aninu.2018.01.005
22. Tiku AR. Antimicrobial compounds and their role in plant defense. In: Molecular Aspects of Plant-Pathogen Interaction. (2018). p. 283–307. doi: 10.1007/978-981-10-7371-7_13
23. Franz C, Baser K, Windisch W. Essential oils and aromatic plants in animal feeding - a European perspective. A review Flavour Fragr J. (2010) 25:327–40. doi: 10.1002/ffj.1967
24. Zeng Z, Zhang S, Wang H, Piao X. Essential oil and aromatic plants as feed additives in non-ruminant nutrition: a review. J Anim Sci Biotechnol. (2015) 6:7. doi: 10.1186/s40104-015-0004-5
25. Rossi B, Toschi A, Piva A, Grilli E. Single components of botanicals and nature-identical compounds as a non-antibiotic strategy to ameliorate health status and improve performance in poultry and pigs. Nutr Res Rev. (2020) 33:218–34. doi: 10.1017/S0954422420000013
26. Gessner DK, Ringseis R, Eder K. Potential of plant polyphenols to combat oxidative stress and inflammatory processes in farm animals. J Anim Physiol Anim Nutr. (2017) 101:605–28. doi: 10.1111/jpn.12579
27. Ghiselli F, Rossi B, Piva A, Grilli E. Assessing intestinal health. In vitro and ex vivo gut barrier models of farm animals: benefits and limitations. Front Vet Sci. (2021) 8:723387. doi: 10.3389/fvets.2021.723387
28. Toschi A, Piva A, Grilli E. Phenol-rich botanicals modulate oxidative stress and epithelial integrity in intestinal epithelial cells. Animals. (2022) 12:188. doi: 10.3390/ani12172188
29. Bonetti A, Tugnoli B, Rossi B, Giovagnoni G, Piva A, Grilli E. Nature-identical compounds and organic acids reduce E. coli K88 growth and virulence gene expression in vitro. Toxins. (2020) 12:468. doi: 10.3390/toxins12080468
30. Toschi A, Rossi B, Tugnoli B, Piva A, Grilli E. Nature-identical compounds and organic acids ameliorate and prevent the damages induced by an inflammatory challenge in caco-2 cell culture. Molecules. (2020) 25:296. doi: 10.3390/molecules25184296
31. Ling K-H, Wan MLY, El-Nezami H, Wang M. Protective capacity of resveratrol, a natural polyphenolic compound, against deoxynivalenol-induced intestinal barrier dysfunction and bacterial translocation. Chem Res Toxicol. (2016) 29:823–33. doi: 10.1021/acs.chemrestox.6b00001
32. Roselli M, Britti MS, le Huërou-Luron I, Marfaing H, Zhu WY, Mengheri E. Effect of different plant extracts and natural substances (PENS) against membrane damage induced by enterotoxigenic Escherichia coli K88 in pig intestinal cells. Toxicol Vitro. (2007) 21:224–9. doi: 10.1016/j.tiv.2006.09.012
33. Livak KJ, Schmittgen TD. Analysis of relative gene expression data using real-time quantitative PCR and the 2-ΔΔCT method. Methods. (2001) 25:402–8. doi: 10.1006/meth.2001.1262
34. Carlson MS, Hill GM, Link JE. Early- and traditionally weaned nursery pigs benefit from phase-feeding pharmacological concentrations of zinc oxide: effect on metallothionein and mineral concentrations. J Anim Sci. (1999) 77:1199–207. doi: 10.2527/1999.7751199x
35. Grilli E, Tugnoli B, Vitari F, Domeneghini C, Morlacchini M, Piva A, et al. Low doses of microencapsulated zinc oxide improve performance and modulate the ileum architecture, inflammatory cytokines and tight junctions expression of weaned pigs. Animal. (2015) 9:1760–8. doi: 10.1017/S1751731115001329
36. Starke IC, Pieper R, Neumann K, Zentek J, Vahjen W. The impact of high dietary zinc oxide on the development of the intestinal microbiota in weaned piglets. FEMS Microbiol Ecol. (2014) 87:416–27. doi: 10.1111/1574-6941.12233
37. Vahjen W, Pieper R, Zentek J. Bar-coded pyrosequencing of 16S rRNA gene amplicons reveals changes in ileal porcine bacterial communities due to high dietary zinc intake. Appl Environ Microbiol. (2010) 76:6689–91. doi: 10.1128/AEM.03075-09
38. Pieper R, Vahjen W, Neumann K, van Kessel AG, Zentek J. Dose-dependent effects of dietary zinc oxide on bacterial communities and metabolic profiles in the ileum of weaned pigs. J Anim Physiol Anim Nutr. (2012) 96:825–33. doi: 10.1111/j.1439-0396.2011.01231.x
39. Kjærgaard K, Sørensen JK, Schembri MA, Klemm P. Sequestration of zinc oxide by fimbrial designer chelators. Appl Environ Microbiol. (2000) 66:10–4. doi: 10.1128/AEM.66.1.10-14.2000
40. Smith F, Clark JE, Overman BL, Tozel CC, Huang JH, Rivier JEF, et al. Early weaning stress impairs development of mucosal barrier function in the porcine intestine. Am J Physiol Gastrointest Liver Physiol. (2010) 298:352–63. doi: 10.1152/ajpgi.00081.2009
41. Pié S, Lallès JP, Blazy F, Laffitte J, Sève B, Oswald IP. Weaning is associated with an upregulation of expression of inflammatory cytokines in the intestine of piglets. J Nutr. (2004) 134:641–7. doi: 10.1093/jn/134.3.641
42. Moeser AJ, Pohl CS, Rajput M. Weaning stress and gastrointestinal barrier development: implications for lifelong gut health in pigs. Anim Nutr. (2017) 3:313–21. doi: 10.1016/j.aninu.2017.06.003
43. McLamb BL, Gibson AJ, Overman EL, Stahl C, Moeser AJ. Early weaning stress in pigs impairs innate mucosal immune responses to enterotoxigenic E. coli challenge and exacerbates intestinal injury and clinical disease. PLoS ONE. (2013) 8:e0059838. doi: 10.1371/journal.pone.0059838
44. Schneeberger EE, Lynch RD. The tight junction: a multifunctional complex. Am J Physiol Cell Physiol. (2004) 286:1213–28. doi: 10.1152/ajpcell.00558.2003
45. Günzel D, Yu ASL. Claudins and the modulation of tight junction permeability. Physiol Rev. (2013) 93:525–69. doi: 10.1152/physrev.00019.2012
46. Otani T, Furuse M. Tight junction structure and function revisited. Trends Cell Biol. (2020) 30:805–17. doi: 10.1016/j.tcb.2020.08.004
47. Mukiza CN, Dubreuil DJ. Escherichia coli heat-stable toxin b impairs intestinal epithelial barrier function by altering tight junction proteins. Infect Immun. (2013) 81:2819–27. doi: 10.1128/IAI.00455-13
48. Kaiser M, Burek M, Britz S, Lankamp F, Ketelhut S, Kemper B, Förster C, Gorzelanny C, Goycoolea FM. The influence of capsaicin on the integrity of microvascular endothelial cell monolayers. Int J Mol Sci. (2019) 20:122. doi: 10.3390/ijms20010122
49. Han J, Isoda H, Maekawa T. Analysis of the Mechanism of the Tight-Junctional Permeability Increase by Capsaicin Treatment on the Intestinal Caco-2 Cells. (2002). p. 93–98. Available online at: https://www.ncbi.nlm.nih.gov/pmc/articles/PMC3449523/pdf/10616_2004_Article_5117248.pdf (accessed June 30, 2019).
50. Han JK, Akutsu M, Talorete TPN, Maekawa T, Tanaka T, Isoda H. Capsaicin-enhanced ribosomal protein P2 expression in human intestinal Caco-2 cells. Cytotechnology. (2005) 47:89–96 doi: 10.1007/s10616-005-3756-5
51. Shiobara T, Usui T, Han J, Isoda H, Nagumo Y. The reversible increase in tight junction permeability induced by capsaicin is mediated via cofilin-actin cytoskeletal dynamics and decreased level of occludin. PLoS ONE. (2013) 8:e0079954. doi: 10.1371/journal.pone.0079954
52. Nagumo Y, Han J, Bellila A, Isoda H, Tanaka T. Cofilin mediates tight-junction opening by redistributing actin and tight-junction proteins. Biochem Biophys Res Commun. (2008) 377:921–5. doi: 10.1016/j.bbrc.2008.10.071
53. Nagumo Y, Han J, Arimoto M, Isoda H, Tanaka T. Capsaicin induces cofilin dephosphorylation in human intestinal cells: the triggering role of cofilin in tight-junction signaling. Biochem Biophys Res Commun. (2007) 355:520–5. doi: 10.1016/j.bbrc.2007.02.002
54. Bujak JK, Kosmala D, Szopa IM, Majchrzak K, Bednarczyk P. Inflammation, cancer and immunity—implication of TRPV1 channel. Front Oncol. (2019) 9:e01087. doi: 10.3389/fonc.2019.01087
55. Kobayashi M, Watanabe K, Yokoyama S, Matsumoto C, Hirata M, Tominari T, et al. Capsaicin, a TRPV1 ligand, suppresses bone resorption by inhibiting the prostaglandin E production of osteoblasts, and attenuates the inflammatory bone loss induced by lipopolysaccharide. ISRN Pharmacol. (2012) 2012:1–6. doi: 10.5402/2012/439860
56. Wang Y, Cui L, Xu H, Liu S, Zhu F, Yan F, et al. TRPV1 agonism inhibits endothelial cell inflammation via activation of eNOS/NO pathway. Atherosclerosis. (2017) 260:13–9. doi: 10.1016/j.atherosclerosis.2017.03.016
57. Alhamoruni A, Wright K, Larvin M, O'Sullivan S. Cannabinoids mediate opposing effects on inflammation-induced intestinal permeability. Br J Pharmacol. (2012) 165:2598–610. doi: 10.1111/j.1476-5381.2011.01589.x
58. Toschi A, Tugnoli B, Rossi B, Piva A, Grilli E. Thymol modulates the endocannabinoid system and gut chemosensing of weaning pigs. BMC Vet Res. (2020) 16:289. doi: 10.1186/s12917-020-02516-y
59. Xu H, Delling M, Jun JC, Clapham DE. Oregano, thyme and clove-derived flavors and skin sensitizers activate specific TRP channels. Nat Neurosci. (2006) 9:628–35. doi: 10.1038/nn1692
60. Liang D, Li F, Fu Y, Cao Y, Song X, Wang T, et al. Thymol inhibits LPS-stimulated inflammatory response via down-regulation of NF-κB and MAPK signaling pathways in mouse mammary epithelial cells. Inflammation. (2014) 37:214–22. doi: 10.1007/s10753-013-9732-x
61. Zheng W, Wang SY. Antioxidant activity and phenolic compounds in selected herbs. J Agric Food Chem. (2001) 49:5165–70. doi: 10.1021/jf010697n
62. Nagoor Meeran MF, Javed H, Taee H al, Azimullah S, Ojha SK. Pharmacological properties and molecular mechanisms of thymol: prospects for its therapeutic potential and pharmaceutical development. Front Pharmacol. (2017) 8:e00380. doi: 10.3389/fphar.2017.00380
63. Wang Q, Cheng F, Xu Y, Zhang J, Qi J, Liu X, et al. Thymol alleviates lipopolysaccharide-stimulated inflammatory response via downregulation of RhoA-mediated NF-κB signalling pathway in human peritoneal mesothelial cells. Eur J Pharmacol. (2018) 833:210–20. doi: 10.1016/j.ejphar.2018.06.003
64. Shi JH, Sun SC. Tumor necrosis factor receptor-associated factor regulation of nuclear factor κB and mitogen-activated protein kinase pathways. Front Immunol. (2018) 9:e01849. doi: 10.3389/fimmu.2018.01849
65. Lee JH, Kim YG, Lee J. Carvacrol-rich oregano oil and thymol-rich thyme red oil inhibit biofilm formation and the virulence of uropathogenic Escherichia coli. J Appl Microbiol. (2017) 123:1420–8. doi: 10.1111/jam.13602
66. Yuan W, Yuk H-G. Effects of sublethal thymol, carvacrol, and trans-cinnamaldehyde adaptation on virulence properties of Escherichia coli O157:H7. Appl Environ Microbiol. (2019) 85:1–11. doi: 10.1128/AEM.00271-19
67. Bonetti A, Tugnoli B, Piva A, Grilli E. Thymol as an adjuvant to restore antibiotic efficacy and reduce antimicrobial resistance and virulence gene expression in enterotoxigenic Escherichia coli strains. Antibiotics. (2022) 11:1073. doi: 10.3390/antibiotics11081073
68. Giovagnoni G, Rossi B, Tugnoli B, Ghiselli F, Bonetti A, Piva A, et al. Thymol and carvacrol downregulate the expression of Salmonella typhimurium virulence genes during an in vitro infection on caco-2 cells. Microorganisms. (2020) 8:1–11. doi: 10.3390/microorganisms8060862
69. Jakobek L. Interactions of polyphenols with carbohydrates, lipids and proteins. Food Chem. (2015) 175:556–67. doi: 10.1016/j.foodchem.2014.12.013
70. Bandyopadhyay P, Ghosh AK, Ghosh C. Recent developments on polyphenol-protein interactions: effects on tea and coffee taste, antioxidant properties and the digestive system. Food Funct. (2012) 3:592–605. doi: 10.1039/c2fo00006g
71. Dubreuil JD. Antibacterial and antidiarrheal activities of plant products against enterotoxinogenic Escherichia coli. Toxins. (2013) 5:2009–41. doi: 10.3390/toxins5112009
72. Brenes A, Viveros A, Chamorro S, Arija I. Use of polyphenol-rich grape by-products in monogastric nutrition. A review Anim Feed Sci Technol. (2016) 211:1–17. doi: 10.1016/j.anifeedsci.2015.09.016
73. Olszewska MA, Gedas A, Simões M. Antimicrobial polyphenol-rich extracts: applications and limitations in the food industry. Food Res Int. (2020) 134:109214. doi: 10.1016/j.foodres.2020.109214
74. Cherubin P, Garcia MC, Curtis D, Britt CBT, Craft JW, Burress H, et al. Inhibition of cholera toxin and other AB toxins by polyphenolic compounds. PLoS ONE. (2016) 11:e0166477. doi: 10.1371/journal.pone.0166477
75. Morinaga N, Iwamarul Y, Yahiro K, Tagashira M, Moss J, Noda M. Differential activities of plant polyphenols on the binding and internalization of cholera toxin in vero cells. J Biol Chem. (2005) 280:23303–9. doi: 10.1074/jbc.M502093200
76. Saito T, Miyake M, Toba M, Okamatsu H, Shimizu S, Noda M. Inhibition by apple polyphenols of ADP-ribosyltransferase activity of cholera toxin and toxin-induced fluid accumulation in mice. Microbiol Immunol. (2002) 46:249–55. doi: 10.1111/j.1348-0421.2002.tb02693.x
77. Dubreuil JD. Fruit extracts to control pathogenic Escherichia coli: a sweet solution. Heliyon. (2020) 6:e03410. doi: 10.1016/j.heliyon.2020.e03410
78. Sheng L, Olsen SA, Hu J, Yue W, Means WJ, Zhu MJ. Inhibitory effects of grape seed extract on growth, quorum sensing, and virulence factors of CDC “top-six” non-O157 Shiga toxin producing E. coli. Int J Food Microbiol. (2016) 229:24–32. doi: 10.1016/j.ijfoodmicro.2016.04.001
79. Leopoldini M, Russo N, Toscano M. The molecular basis of working mechanism of natural polyphenolic antioxidants. Food Chem. (2011) 125:288–306. doi: 10.1016/j.foodchem.2010.08.012
80. Morgan MJ, Liu ZG. Crosstalk of reactive oxygen species and NF-κB signaling. Cell Res. (2011) 21:103–15. doi: 10.1038/cr.2010.178
81. Lingappan K. NF-κB in oxidative stress. Curr Opin Toxicol. (2018) 7:81–6. doi: 10.1016/j.cotox.2017.11.002
82. Chatterjee S. Oxidative Stress, Inflammation, and Disease. Oxidative Stress and Biomaterials. Elsevier (2016). p. 35–58.
83. Hussain T, Tan B, Yin Y, Blachier F, Tossou MCB, Rahu N. Oxidative stress and inflammation: what polyphenols can do for us? Oxid Med Cell Longev. (2016) 2016:797. doi: 10.1155/2016/7432797
84. Erlejman AG, Jaggers G, Fraga CG, Oteiza PI. TNFα-induced NF-κB activation and cell oxidant production are modulated by hexameric procyanidins in Caco-2 cells. Arch Biochem Biophys. (2008) 476:186–95. doi: 10.1016/j.abb.2008.01.024
85. Romier B, van de Walle J, During A, Larondelle Y, Schneider YJ. Modulation of signalling nuclear factor-κB activation pathway by polyphenols in human intestinal Caco-2 cells. Br J Nutr. (2008) 100:542–51. doi: 10.1017/S0007114508966666
86. Gessner DK, Fiesel A, Most E, Dinges J, Wen G, Ringseis R, et al. Supplementation of a grape seed and grape marc meal extract decreases activities of the oxidative stress-responsive transcription factors NF-κB and Nrf2 in the duodenal mucosa of pigs. Acta Vet Scand. (2013) 55:18. doi: 10.1186/1751-0147-55-18
87. Martínez-Huélamo M, Rodríguez-Morató J, Boronat A, de la Torre R. Modulation of Nrf2 by olive oil and wine polyphenols and neuroprotection. Antioxidants. (2017) 6:73. doi: 10.3390/antiox6040073
88. Zhou Y, Jiang Z, Lu H, Xu Z, Tong R, Shi J, et al. Recent advances of natural polyphenols activators for Keap1-Nrf2 signaling pathway. Chem Biodivers. (2019) 16:400. doi: 10.1002/cbdv.201900400
89. Gessner DK, Ringseis R, Siebers M, Keller J, Kloster J, Wen G, et al. Inhibition of the pro-inflammatory NF-κB pathway by a grape seed and grape marc meal extract in intestinal epithelial cells. J Anim Physiol Anim Nutr. (2012) 96:1074–83. doi: 10.1111/j.1439-0396.2011.01222.x
90. Lynn KS, Peterson RJ, Koval M. Ruffles and spikes: control of tight junction morphology and permeability by claudins. Biochim Biophys Acta Biomembr. (2020) 1862:3339. doi: 10.1016/j.bbamem.2020.183339
91. Shamshoum H, Vlavcheski F, Tsiani E. Anticancer effects of oleuropein. BioFactors. (2017) 43:517–28. doi: 10.1002/biof.1366
92. Tang Y, Li F, Tan B, Liu G, Kong X, Hardwidge PR, et al. Enterotoxigenic Escherichia coli infection induces intestinal epithelial cell autophagy. Vet Microbiol. (2014) 171:160–4. doi: 10.1016/j.vetmic.2014.03.025
93. Xu F, Li Y, Zheng M, Xi X, Zhang X, Han C. Structure properties, acquisition protocols, and biological activities of oleuropein aglycone. Front Chem. (2018) 6:e00239. doi: 10.3389/fchem.2018.00239
94. Yong Y, Fang B, Huang Y, Li J, Yu T, Wu L, et al. Tea tree oil terpinen-4-ol protects gut barrier integrity by upregulation of tight junction proteins via the ERK1/2-signaling pathway. Front Nutr. (2022) 8:612. doi: 10.3389/fnut.2021.805612
95. Zhang C, Huang Y, Li P, Chen X, Liu F, Hou Q. Ginger relieves intestinal hypersensitivity of diarrhea predominant irritable bowel syndrome by inhibiting proinflammatory reaction. BMC Complement Med Ther. (2020) 20:279. doi: 10.1186/s12906-020-03059-3
96. Yan Z, Zhong Y, Duan Y, Chen Q, Li F. Antioxidant mechanism of tea polyphenols and its impact on health benefits. Anim Nutr. (2020) 6:115–23. doi: 10.1016/j.aninu.2020.01.001
97. Mao QQ, Xu XY, Cao SY, Gan RY, Corke H, Beta T, et al. Bioactive compounds and bioactivities of ginger (zingiber officinale roscoe). Foods. (2019) 8:185. doi: 10.3390/foods8060185
98. Shi C, Zhao X, Yan H, Meng R, Zhang Y, Li W, et al. Effect of tea tree oil on Staphylococcus aureus growth and enterotoxin production. Food Control. (2016) 62:257–63. doi: 10.1016/j.foodcont.2015.10.049
99. Liu Z, Meng R, Zhao X, Shi C, Zhang X, Zhang Y, et al. Inhibition effect of tea tree oil on Listeria monocytogenes growth and exotoxin proteins listeriolysin O and p60 secretion. Lett Appl Microbiol. (2016) 63:450–7. doi: 10.1111/lam.12666
100. Vijendra Kumar N, Murthy PS, Manjunatha JR, Bettadaiah BK. Synthesis and quorum sensing inhibitory activity of key phenolic compounds of ginger and their derivatives. Food Chem. (2014) 159:451–7. doi: 10.1016/j.foodchem.2014.03.039
101. Park H-Y, Kunitake Y, Hirasaki N, Tanaka M, Matsui T. Theaflavins enhance intestinal barrier of Caco-2 Cell monolayers through the expression of AMP-activated protein kinase-mediated Occludin, Claudin-1, and ZO-1. Biosci Biotechnol Biochem. (2015) 79:130–7. doi: 10.1080/09168451.2014.951027
102. Furrie E, Macfarlane S, Kennedy A, Cummings JH, Walsh S V, O'Neil DA, et al. Synbiotic therapy (Bifidobacterium longum/Synergy 1) initiates resolution of inflammation in patients with active ulcerative colitis: a randomised controlled pilot trial. Gut. (2005) 54:242–9. doi: 10.1136/gut.2004.044834
103. Chang KW, Kuo CY. 6-Gingerol modulates proinflammatory responses in dextran sodium sulfate (DSS)-treated Caco-2 cells and experimental colitis in mice through adenosine monophosphate-activated protein kinase (AMPK) activation. Food Funct. (2015) 6:3334–41. doi: 10.1039/C5FO00513B
104. Parlesak A, Haller D, Brinz S, Baeuerlein A, Bode C. Modulation of cytokine release by differentiated CACO-2 cells in a compartmentalized coculture model with mononuclear leucocytes and nonpathogenic bacteria. Scand J Immunol. (2004) 60:477–85. doi: 10.1111/j.0300-9475.2004.01495.x
105. Wehkamp J, Fellermann K, Herrlinger KR, Baxmann S, Schmidt K, Schwind B, et al. Human β-defensin 2 but not β-defensin 1 is expressed preferentially in colonic mucosa of inflammatory bowel disease. Eur J Gastroenterol Hepatol. (2002) 14:745–52. doi: 10.1097/00042737-200207000-00006
106. Bondo Dydensborg A, Herring E, Auclair J, Tremblay E, Beaulieu J-F, Bondo A. Innovative methodology normalizing genes for quantitative RT-PCR in differentiating human intestinal epithelial cells and adenocarcinomas of the colon. Am J Physiol Gastrointest Liver Physiol. (2006) 290:1067–74. doi: 10.1152/ajpgi.00234.2005
Keywords: piglets, weaning, post-weaning diarrhea, Escherichia coli F4, zinc oxide, essential oils, natural extracts, botanicals
Citation: Bonetti A, Piva A and Grilli E (2023) Botanicals as a zinc oxide alternative to protect intestinal cells from an Escherichia coli F4 infection in vitro by modulation of enterocyte inflammatory response and bacterial virulence. Front. Vet. Sci. 10:1141561. doi: 10.3389/fvets.2023.1141561
Received: 10 January 2023; Accepted: 22 February 2023;
Published: 09 March 2023.
Edited by:
Deguang Song, Yale University, United StatesReviewed by:
Sara Damiano, University of Naples Federico II, ItalyCarlo Corino, University of Milan, Italy
Raffaella Rossi, University of Milan, Italy
Copyright © 2023 Bonetti, Piva and Grilli. This is an open-access article distributed under the terms of the Creative Commons Attribution License (CC BY). The use, distribution or reproduction in other forums is permitted, provided the original author(s) and the copyright owner(s) are credited and that the original publication in this journal is cited, in accordance with accepted academic practice. No use, distribution or reproduction is permitted which does not comply with these terms.
*Correspondence: Ester Grilli, ZXN0ZXIuZ3JpbGxpQHVuaWJvLml0