- 1Department of Biomedical Sciences, Ontario Veterinary College, University of Guelph, Guelph, ON, Canada
- 2Department of Clinical Studies, Ontario Veterinary College, University of Guelph, Guelph, ON, Canada
- 3Department of Animal Biosciences, Ontario Agricultural College, University of Guelph, Guelph, ON, Canada
- 4Department of Human Health and Nutritional Sciences, College of Biological Sciences, University of Guelph, Guelph, ON, Canada
Introduction: Due to the involvement in one-carbon metabolism and lipid mobilization, choline and L-carnitine supplementation have been recommended to minimize hepatic lipid accumulation and support fat oxidation, respectively. This study investigated the lipotropic benefits of choline or L-carnitine supplementation in lean and obese cats maintaining body weight (BW).
Methods: Lean [n = 9; body condition score (BCS): 4–5/9] and obese (n = 9; BCS: 8–9/9) adult male neutered colony cats were used in a replicated 3 x 3 complete Latin square design. Treatments included choline (378 mg/kg BW0.67), L-carnitine (200 mg/kg BW) and control (no supplement). Treatments were supplemented to the food for 6 weeks each, with a 2-week washout between treatments. Cats were fed once daily to maintenance energy requirements, and BW and BCS were assessed weekly. Fasted blood collection, indirect calorimetry, and dual-energy X-ray absorptiometry occurred at the end of each treatment period. Serum was analyzed for cholesterol (CHOL), high-density lipoprotein CHOL (HDL-C), triglycerides (TAG), non-esterified fatty acids (NEFA), glucose, creatinine (CREAT), urea, alkaline phosphatase (ALP) and alanine aminotransferase (ALT). Very low-density lipoprotein CHOL (VLDL) and low-density lipoprotein CHOL (LDL-C) were calculated. Data were analyzed using proc GLIMMIX, with group and period as random effects, and treatment, body condition, and their interaction as fixed effects, followed by a Tukey's post-hoc test when significance occurred.
Results: Cats supplemented choline had lower food intake (P = 0.025). Treatment did not change BW, BCS and body composition (P > 0.05). Obese cats had greater ALP, TAG, and VLDL, and lower HDL-C compared to lean cats (P < 0.05). Choline resulted in greater CHOL, HDL-C, LDL-C and ALT (P < 0.05). L-carnitine resulted in lower CREAT (P = 0.010). Following the post-hoc test, differences between treatment means were not present for ALP (P = 0.042). No differences were found for glucose, urea or NEFA (P > 0.05). Obese cats had a lower fed respiratory quotient (RQ), regardless of treatment (P = 0.045). Treatment did not affect fed or fasted RQ and energy expenditure (P > 0.05).
Discussion: Choline appeared to increase circulating lipid and lipoprotein concentrations regardless of body condition, likely through enhanced lipid mobilization and hepatic elimination. Neither dietary choline or L-carnitine altered body composition or energy metabolism in the lean or obese cats, as compared to control.
1. Introduction
Feline hepatic lipidosis (FHL) is defined by the excess storage of hepatic lipids and is considered the most common liver disease affecting cats in North America (1). Although the exact pathophysiology of FHL has not been identified, there is evidence that the mobilization of free fatty acids from the adipose tissue leads to the accumulation of lipids within the livers of affected cats (2). Food deprivation, whether due to secondary anorexia or due to an imposed high degree of energy restriction, is considered a key factor in the development of FHL (1, 3–6). Left untreated, the prognosis for affected cats is considered poor and may result in liver failure and death (7). Obese cats are at an increased risk of developing FHL due to their increased levels of adipose tissue and subsequent stores of fatty acids available for mobilization (2, 8). Also, higher concentrations of hepatic triglycerides (TAG) and an increased risk of concurrent insulin resistance are believed to leave obese cats more susceptible to FHL (9, 10). Additionally, energy restriction is often recommended in obese cats in order to lose weight. However, a low degree of dietary energy restriction often does not result in weight loss in an obese cat, and therefore may result in a higher degree of energy restriction may be implemented (11, 12).
Under normal conditions, fatty acids within the hepatocytes can undergo β-oxidation within the mitochondria for the production of acetyl-CoA, or they can be re-esterified to TAG (13, 14). These TAG can be secreted into the circulation or they can continue to accumulate within the hepatocytes. It is unclear what leads to the accumulation of hepatic TAG during FHL. However, the metabolic idiosyncrasies that result from their obligate carnivorous nature put cats at risk for deficiencies in certain dietary essential nutrients. Insufficient intakes of dietary choline and carnitine during energy restriction have been proposed as potential risk factors in the pathogenesis of FHL (15).
Choline is a precursor for the biosynthesis of phosphatidylcholine (PC). Specifically, PC is required for packaging TAG and cholesterol into very low-density lipoproteins (VLDL) for export out of the liver (16). Betaine, a derivative of choline, also serves as a methyl group donor for one of the pathways through which homocysteine is remethylated to methionine. The production of methionine subsequently results in the methylation and synthesis of numerous metabolites, including carnitine (17). Previous research in mammals, including cats, found that choline deficiency leads to the development of fatty liver, which can be reversed and/or prevented when dietary choline is added back to the diet in sufficient quantities (18–20). In cats, increased dietary choline supplementation leads to increased concentrations of serum lipids and lipoproteins in both obese and overweight cats (21, 22), suggesting increased lipid hepatic mobilization through the synthesis and secretion of VLDL from the liver. Additionally, choline and its derivative betaine may reduce fat mass gain, as suggested in livestock species and growing kittens fed ad libitum post-gonadectomy (23–25).
In comparison, carnitine is required to facilitate the entry of fatty acids into the mitochondria for β-oxidation. This is considered the main pathway for the disposal of fatty acids under normal physiological conditions and is important for the production of ATP by the mitochondria (13). L-carnitine increases lipid oxidation in overweight cats, as suggested by increased palmitate oxidation (26), energy expenditure (EE) and lower post-prandial respiratory quotient (RQ) (27). Additionally, L-carnitine supplementation may decrease food intake and weight gain in ad libitum feeding scenarios (28). For these reasons, L-carnitine is commonly used by the pet food industry in the formulation of weight control and weight loss diets for cats. In human medicine, reduced β-oxidation has been suggested as a potential cause of lipid accumulation in the liver of patients with non-alcoholic fatty liver disease (NAFLD) (29). Thus, it has been proposed that an insufficient supply of carnitine may similarly be an important factor for the development of FHL, although the evidence to support this has been inconsistent (30–34).
Nutrients and nutraceuticals that can reduce the risk of hepatic lipid accumulation in obese cats and assist in mitigating weight gain would benefit the pet food industry and the veterinary community. Currently, there is evidence that dietary choline may provide such benefits to overweight and obese cats (21, 22). However, there have been no studies comparing the lipotropic benefits of dietary choline to L-carnitine, a dietary supplement commonly used by the pet food industry. Therefore, the objective of this research was to investigate the lipotropic effects of dietary choline compared to L-carnitine (a positive control) and no supplement (a negative control), on serum lipid and lipoprotein profiles, body composition, EE and RQ in obese and lean adult cats. We hypothesized that supplemental choline would result in greater serum lipid and lipoprotein concentrations, as compared to L-carnitine or control, whereas L-carnitine would increase EE and lower RQ only in the obese cats, suggesting favored fatty acid oxidation. Additionally, we hypothesized that both choline and L-carnitine would improve body composition by decreasing fat mass and increasing lean body mass only in the obese cats, as compared to control.
2. Materials and methods
All procedures were approved by the University of Guelph Animal Care Committee (AUP#4496), in compliance with provincial and national guidelines regarding the care and use of animals in research.
2.1. Animals and housing
Eighteen domestic shorthair (DSH) male neutered cats (Marshall's Bio Resources, Waverly, NY, United States of America) were enrolled in this trial. The cats were between 1 to 2 years of age (mean ± SEM: 2.00 ± 0.11 years; range: 1.28–2.29 years) and were considered healthy prior to the start of the trial, based on physical examination, medical history, and the results of complete blood count (CBC) and serum biochemistry completed within 6 months of the trial. The cats in the present study were fed using a modified ad libitum protocol during growth (25). At the start of the trial, the 18 cats were classified into two groups, “obese” or “lean”, based on assigned body condition score (BCS) (35). Nine of the 18 cats were classified as obese, with a BCS of ≥ 8/9 (36), and a mean body weight (BW) of 6.46 ± 0.15 kg. The remaining nine were classified as lean, with an assigned BCS of 4–5/9 and BW of 4.62 ± 0.15 kg.
All 18 cats were housed together in a free-living environment (23 ft x 19 ft) at the Animal Biosciences Cattery at the Ontario Agricultural College of the University of Guelph (Guelph, ON, Canada). The room had a controlled 12 h light 12 h dark cycle, with the lights turning on at 0700 h and off at 1,900 h. Humidity and temperature were maintained at 40% and 24°C respectively throughout the trial.
Water was available to the cats ad libitum in bowls and through an open tap. The room was cleaned daily and enrichment was provided through regular interaction with familiar people and within the environment, as previously described in Frayne et al. (37).
2.2. Diet
Four weeks leading up to the trial (adaptation period) and throughout the entire length of the trial, cats remained on a commercial extruded diet (Nutram Total Grain-Free® Chicken and Turkey Recipe, Elmira Pet Products, Elmira, ON, Canada) formulated for feline adult maintenance, per the Association of American Feed Control Officials (AAFCO) (35). To be fed individually, cats were separated and placed in individual cages for 1 h daily (08:00 h). Food was provided in quantities to maintain current BW based on historic dietary intake data. Individual orts were measured and recorded daily. Fasted BW and BCS were recorded weekly by the same assessor (A.R.).
As previously detailed in Rankovic et al. (22), nutrient analysis of the diet (Table 1) was performed using methods described by the Association of Official Analytical Chemists (AOAC) and the American Oil Chemist Society (AOCS) (Bureau Veritas, Mississauga, ON, Canada) (38, 39). This included the measurement of moisture (AOAC 935.29), crude protein (AOAC 990.03), crude fat (AOAC 920.39), crude fiber (AOCS Ba6a-05), ash (AOAC 942.05), total dietary fiber (AOAC 991.43, 985.29), pyridoxine [B6, AOAC 985.32 (modified)], folate (B9; AOAC 2004.5), cobalamin (B12; AOAC 986.23), choline (AOAC 999.14). Dietary carnitine concentrations were measured by liquid chromatography-mass spectrometry, according to AOAC 2012.17 (38). In addition, concentrations of dietary amino acids were measured using ultra-performance liquid chromatography (UPLC), as previously described by Cargo-Froom et al. (40). Nitrogen free extract (NFE) was calculated by difference (NFE % = 100-moisture-protein-fat-crude fiber-ash), and metabolizable energy (ME) was estimated using the Modified Atwater equation [ME = 10 x (3.5 x %crude protein) + (8.5 x %fat) + (3.5 x %NFE)] (41).
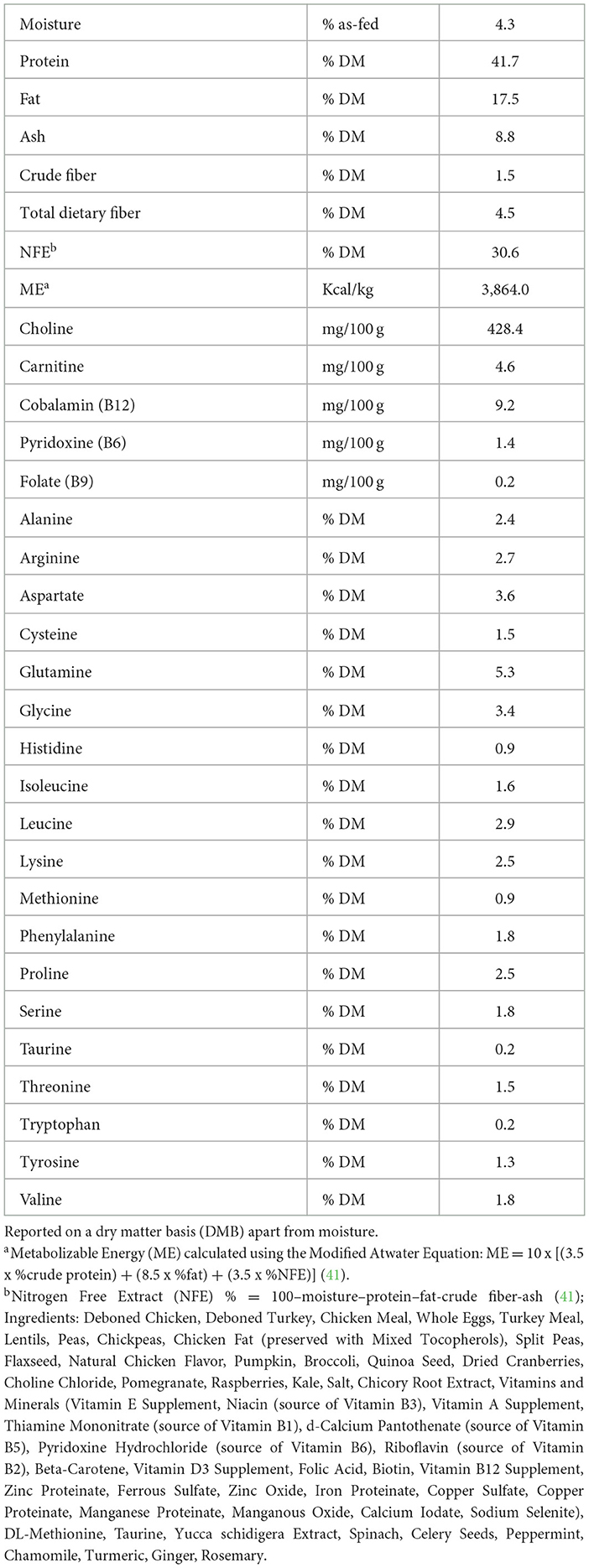
Table 1. The proximate analysis, energy content, fiber, choline, carnitine, selected B-vitamin and amino acid concentrations of a commercial extruded adult cat food fed at maintenance energy requirements to obese (n = 9) and lean adult cats (n = 9).
2.3. Study design and dietary choline and L-carnitine supplementation
All cats received each of the three treatments (choline, carnitine and control) in a 3 x 3 Latin square design. Prior to the trial, the 18 cats were split into three groups of six. Each group was balanced for BW and consisted of three obese and three lean cats. Each treatment was provided for a 6-week period, and cats were placed on a 2-week washout between periods where they received only the commercial cat food with no supplementation. The same food without supplementation continued to be provided in the same quantities to maintain BW during washout, as during the treatment periods.
As per previous research (22), choline (PuraChol, 70% aqueous choline chloride, 52.2% choline ion; Balchem Corporation, New Hampton, NY, United States of America) was supplemented to provide a daily choline intake of six times the recommended allowance (RA) for dietary choline published by the National Research Council (NRC) [6 x 63 mg/kg BW0.67 (378 mg/kg BW0.67)] (22, 41). The choline was measured daily, before feeding, with an adjustable volume pipette (Research plus™ Variable Adjustable Volume Pipette: 100–1,000 μL Single-Channel, Eppendorf Canada Ltd., Mississauga, ON, Canada). The estimated choline intake from the diet (4.3 mg choline/g diet as-fed) was accounted for when calculating the choline supplement dose for each individual cat. L-carnitine (48.5% L-carnitine tartrate, Bill Barr & Company, Overland Park, KS, United States of America) was supplemented at 200 mg/kg BW daily (26, 28). The L-carnitine was pre-measured daily before feeding and was mixed with a small amount of water. Each cat received their food once daily. Prior to feeding, each cat's daily food amount was split into two rations. The first ration provided of the daily food intake. The dietary treatments (choline or L-carnitine) were top-dressed onto this first ration and left to soak for 20 min prior to feeding. Once consumed, each cat was provided their second ration (3/4 daily food intake). Cats on the control treatment and during washout similarly received two rations but did not have anything top-dressed onto their first ration.
2.4. Indirect calorimetry
Indirect calorimetry was performed on the last day of each 6-week period (day 42), to determine individual EE and RQ. Each 24-h session comprised of a gas equilibrium period of 30 min, pre-prandial (fasted) measurements (1.5 h), and fed and extended postprandial measurements (22 h). Cats were acclimated to the chambers, using the methods previously outlined by Gooding et al. (42). Calibration of gases and protocol followed the methods previously described (22, 25).
Briefly, an open circuit, ventilated system (Qubit C950 Multi Channel Gas Exchange, Qubit Systems Inc., Kingston, ON, Canada) was used, with plexiglass chambers measuring 53 x 53 x 79 cm (length x width x height). The volume of space within the chamber measured 221.91 L. To maintain levels of CO2 within the chambers between 0.4% and 0.7%, room air was pulled through the chambers at a flow rate between 4.1–6.2 L/min.
The cats had access to a litter box, a water bowl and a hammock within the chamber. Water was filled before placing the cats in the calorimetry chambers and starting measurements. During each calorimetry session, cats continued to receive their food and treatment in the same 2-ration method as outlined previously.
Respiratory quotient and EE were calculated by the C950-Multi Channel Gas Exchange system (Qubit Systems Inc, Kingston, ON, Canada), using the following equations (43):
2.5. Blood collection and laboratory analyses
Following the completion of each 24 h indirect calorimetry session, cats were anesthetized for blood and tissue sample collection. Tissue samples are subject to further analysis and are not part of this study. Cats were pre-medicated with hydromorphone (0.05 mg/kg BW IM) and acepromazine (0.04 mg/kg BW IM), and induced with alfaxalone (1–3 mg/kg BW IV, to effect) and midazolam (0.3 mg/kg BW IV) (44). Fasted blood samples were collected from the jugular vein (5 mL). Following collection, the whole blood was transferred to serum-separating tubes and stored at 5°C until centrifugation. Centrifugation of collected whole blood occurred within 10 h of collection. Samples were centrifuged at 2,500 g x 15 min at 4°C (LegendRT, Kendro Laboratory Products 2002, Germany). Serum was separated, aliquoted and submitted (0.5 mL) to the Animal Health Laboratory at the University of Guelph (Guelph, ON, Canada) for analysis of serum CHOL, TAG, non-esterified fatty acids (NEFA), high-density lipoprotein CHOL (HDL-C), alkaline phosphatase (ALP), alanine aminotransferase (ALT), blood urea nitrogen (BUN), creatinine (CREAT) and glucose (GLUC), via photometry using a Roche Cobas 6000 c501 analyzer (Roche Diagnostics, Basel, Switzerland). The Friedewald equation was used to calculate very low-density lipoprotein CHOL (VLDL) and low-density lipoprotein CHOL (LDL-C) (45):
2.6. Dual energy x-ray absorptiometry
Following blood collection, on the same day, body composition was assessed by dual energy x-ray absorptiometry (DXA). If needed, cats were administered additional sedation using dexmedetomidine (0.3 mg/kg BW IM). Atipamezole (0.2 mg/kg BW IM) was used to reverse sedation following DXA scans (44). Scans were performed in duplicate for each individual cat using a fan-beam DXA device (Prodigy® Advance GE Healthcare, Madison, WI, United States of America) to estimate whole body fat % (BF %), total tissue mass (TTM), fat mass (FM), and lean soft tissue mass (LSTM) (enCORE Version 16; GE Healthcare, Madison, WI, United States of America). The Small Animal Mode with Thin Setting was used. Each cat was individually positioned onto the DXA scanner in dorsal recumbency with forelimbs extended cranially (46). The cats were repositioned as necessary between scans. Each scan lasted ~10 min.
2.7. Statistical analyses
Residuals of serum biochemistry and lipoprotein profile, body composition, indirect calorimetry, BW, BCS, and intake data were all assessed for normality with the Shapiro-Wilk test. Food intake, energy intake, BCS, fed EE, fed RQ, choline and carnitine intake (mg/day, mg/kg BW, and mg/kg BW0.67) were not normally distributed and underwent a log transformation as a result. The data was back-transformed to obtain least square means (LSM) for each response variable.
The trapezoidal method was used to calculate area under the curve for RQ (AUCRQ), both in the fasted (pre-prandial) and fed states (0–120 min, 120–480 min, 480–820 min, 820–1,300 min) (9.3.1, GraphPad Software, San Diego, CA, United States of America). Statistical analyses of serum ALP, ALT, BUN, CREAT, GLUC, lipid and lipoprotein concentrations (CHOL, NEFA, HDL-C, LDL-C, VLDL, and TAG), body composition data (BF %, TTM, FM, and LSTM), EE, RQ and AUCRQ, were performed using SAS (SAS Studio 3.8, SAS Institute, Cary, NC, United States) as a generalized linear mixed model. The proc GLIMMIX procedure was used, with treatment, “body condition” (obese or lean), and treatment x body condition interaction set as the fixed effects, period and group as the random effects, and cat as the subject. Food intake and BW were included as covariates when assessing differences in RQ and EE. The covariance matrix resulting in the smallest Akaike information criterion value was used.
Differences in BW and BCS over time were similarly assessed through Proc GLIMMIX with treatment, body condition and their subsequent interaction as fixed effects, period and group as the random effects, week as the repeated term, and cat as the subject. Baseline BCS and BW were used as covariates when assessing change in BW and BCS.
Tukey's post hoc test was performed for all multiple comparisons where a significant effect of treatment, body condition, or treatment and body condition interaction was present. Results are expressed as LSM ± standard error of mean (SEM). A P-value < 0.05 was considered significant, and a P-value of < 0.10 was considered a trend.
3. Results
One obese cat was removed during period three due to a medical condition not related to the study. As a result, data from this cat were not included for period three (choline treatment). Supplements were accepted by the cats with no observed adverse health effects throughout the trial.
3.1. Food, energy, choline, and L-carnitine intake
Obese cats had greater food intake and subsequent energy intake compared to lean cats (PCondition < 0.001; Table 2). Choline, but not L-carnitine, resulted in lower food and energy intake in both lean and obese cats (PTreatment = 0.025), with no effect of treatment x body condition interaction (PTreatmentxCondition= 0.669).
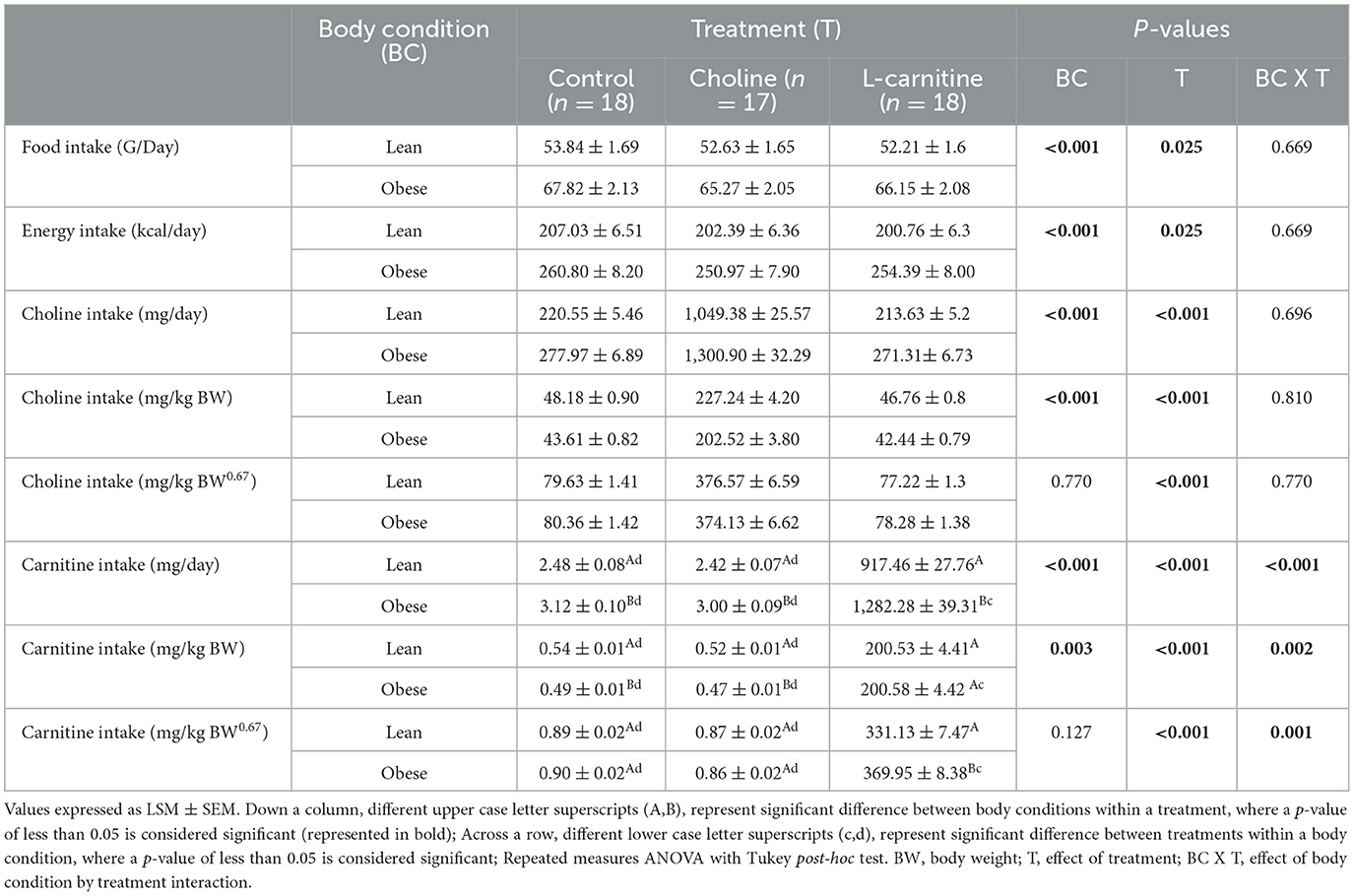
Table 2. Food, energy, choline and L-carnitine intake of lean (n = 9) and obese (n = 9 for control and L-carnitine; n = 8 for choline) adult cats receiving supplemental dietary choline (378 mg/kg BW0.67), supplemental L-carnitine (200 mg/kg BW), or control (no additional supplement) top-dressed onto a commercial extruded adult feline diet and fed to maintenance energy requirements in a 3 x 3 Latin square design for 6-week periods.
As expected, treatment affected choline and L-carnitine intake (PTreatment < 0.001), where supplementation resulted in greater intake. Additionally, there was an effect of body condition when choline and L-carnitine intake were expressed on a mg/day and mg/kg BW basis (Mg/day: PCondition < 0.001, and < 0.001, respectively; mg/kg BW: PCondition < 0.001, and 0.003, respectively). When expressed as mg/day, choline and L-carnitine intake were greater in obese cats. However, obese cats had a lower intake of both choline and L-carnitine when expressed on a mg/kg BW basis. Treatment x body condition interaction also affected L-carnitine intake (mg/day: PTreatmentxCondition < 0.001; mg/kg BW: PTreatmentxCondition = 0.002; mg/kg BW0.67: PTreatmentxCondition = 0.001), where obese cats receiving the L-carnitine treatment had the greatest intakes of L-carnitine. There was no effect of treatment x body condition interaction on choline intake (mg/day: PTreatmentxCondition 0.696; mg/kg BW: PTreatmentxCondition = 0.810; mg/kg BW0.67: PTreatmentxCondition = 0.770).
3.2. Body weight, body condition score and body composition
Body weight, BCS and body composition data are presented in Table 3. Both BW and BCS were higher in the obese cats than in the lean cats (PCondition < 0.001) and neither changed treatment or treatment x body condition interaction (PTreatment = 0.419, and 0.667, respectively; PTreatmentxCondition= 0.885, and 0.667 respectively). There were no changes in BW or BCS over time, in both lean and obese cats, or with any of the three treatments (P > 0.05; data not shown).
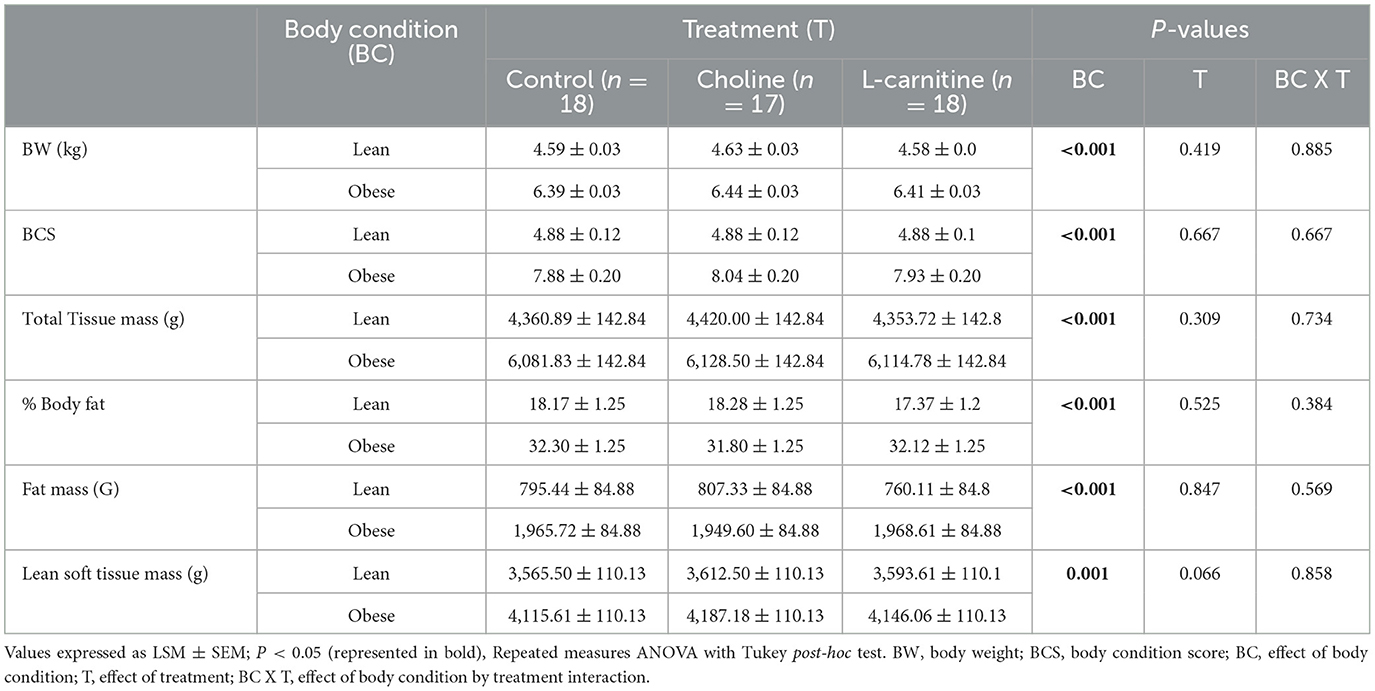
Table 3. Body weight, BCS, and body composition of lean (n = 9) and obese (n = 9 for control and L-carnitine; n = 8 for choline) adult cats receiving supplemental dietary choline (378 mg/kg BW0.67), supplemental L-carnitine (200 mg/kg BW), or control (no additional supplement top-dressed onto a commercial extruded adult feline diet) and fed to maintenance energy requirements in a 3 x 3 Latin square design for 6-week periods.
As expected, TTM, FM, and BF % were higher in the obese cats than in the lean cats (PCondition < 0.001). However, treatment and treatment x body condition interaction did not change any of these parameters (PTreatment = 0.309, 0.847, and 0.525, respectively; PTreatmentxCondition= 0.734, 0.569, and 0.384, respectively). Obese cats had greater LSTM (PCondition= 0.001). There was a trend for LSTM to change with treatment (PTreatment = 0.066), but there was no effect of treatment x body condition interaction on LSTM (PTreatmentxCondition= 0.858).
3.3. Serum biochemistry and lipoprotein profile
Mean serum biochemistry and lipoprotein values fell within reference intervals published by the Animal Health Laboratory (Guelph, ON, Canada), and are presented in Table 4. Serum TAG, HDL-C and VLDL concentrations differed between lean and obese cats (PCondition= 0.001, 0.041, and 0.001, respectively). Concentrations of serum TAG and VLDL were greater in obese cats across all three treatments. Conversely, serum HDL-C was lower in obese cats, as compared to lean cats. Serum HDL-C concentrations were also affected by treatment (PTreatment = 0.005); concentrations were higher with choline supplementation, as compared to control and L-carnitine. Serum CHOL and LDL-C concentrations were also greater with choline supplementation in all cats (PTreatment = 0.005, and 0.042, respectively). However, neither CHOL or LDL-C were affected by body condition (PCondition = 0.104, and 0.453, respectively). Concentrations of serum TAG and VLDL were not affected by treatment alone (PTreatment = 0.340, and 0.340, respectively), but tended to increase with L-carnitine supplementation in obese cats (PTreatmentxCondition = 0.064, and 0.064, respectively). There was also a trend for treatment to increase serum NEFA (PTreatment = 0.099). However, neither body condition or treatment x body condition interaction affected NEFA (PCondition= 0.241, and PTreatmentxCondition = 0.884). No significant treatment x body condition interactions were noted for CHOL, HDL-C and LDL-C (PTreatmentxCondition = 0.619, 0.242 and 0.586, respectively).
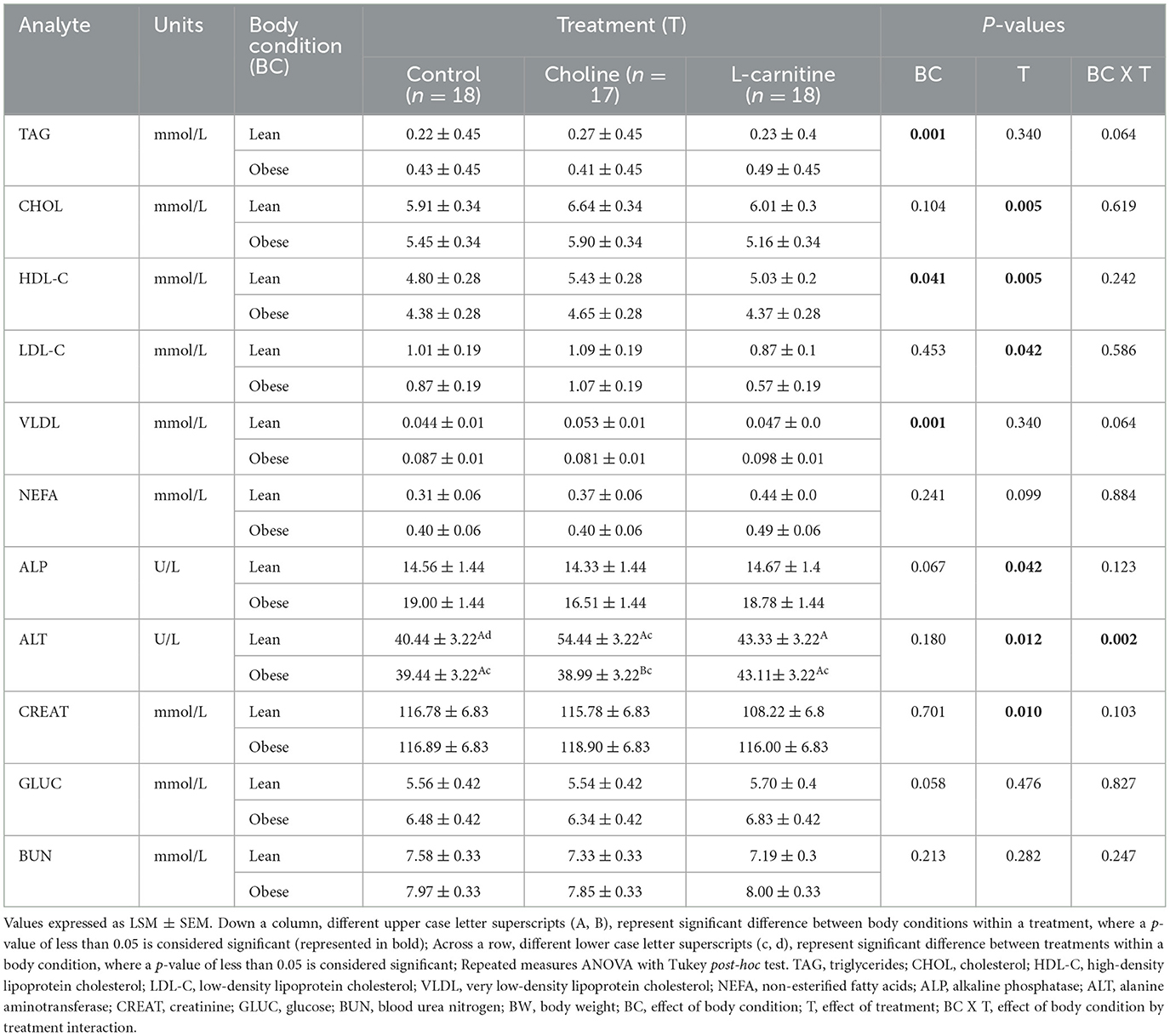
Table 4. Fasted serum biochemistry, lipid and lipoprotein profile values of lean (n = 9) and obese (n = 9 for control and L-carnitine; n = 8 for choline) adult cats receiving supplemental dietary choline (378 mg/kg BW0.67), supplemental L-carnitine (200 mg/kg BW), or control (no additional supplement top-dressed onto a commercial extruded adult feline diet) and fed to maintenance energy requirements in a 3 x 3 Latin square design for 6-week periods.
Treatment did affect serum ALP, ALT, and CREAT (PTreatment = 0.042, 0.012, and 0.010, respectively). There were no differences between ALP means following a Tukey's post-hoc. Serum ALT concentrations were lower with control, as compared to the choline treatment. Conversely, L-carnitine supplementation reduced serum CREAT, as compared to both control and choline, in both lean and obese cats. Lean cats consuming choline had greater concentrations of serum ALT as compared to lean cats consuming control and L-carnitine, and obese cats consuming L-carnitine (PTreatmentxCondition = 0.002). There was a trend for body condition to change serum GLUC (PCondition= 0.058). However, GLUC did not change with treatment or treatment x body condition interaction (PTreatment = 0.476, and PTreatmentxCondition = 0.827). Serum BUN was not affected by treatment, body condition, or treatment x body condition interaction (PTreatment = 0.282, PCondition= 0.213, PTreatmentxCondition = 0.247).
3.4. Indirect calorimetry
There was a trend for fasted EE to change with treatment (PTreatment = 0.087; Table 5), but there was no change with body condition or treatment x body condition interaction (PCondition= 0.685 and PTreatmentxCondition = 0.189). These outcomes did not change when fasted EE was adjusted for BW (PTreatment = 0.088, PCondition= 0.756, and PTreatmentxCondition = 0.191). Fed EE was not affected by treatment or treatment x body condition interaction (PTreatment = 0.915, and PTreatmentxCondition = 0.874), but tended to be lower in the obese cats (PCondition= 0.090). Treatment, condition and treatment x body condition interaction did not change fed EE when adjusted for BW or food intake (PTreatment = 0.905, and 0.761, respectively; PCondition= 0.264, and 0.090, respectively; PTreatmentxCondition = 0.881, and 0.767, respectively).
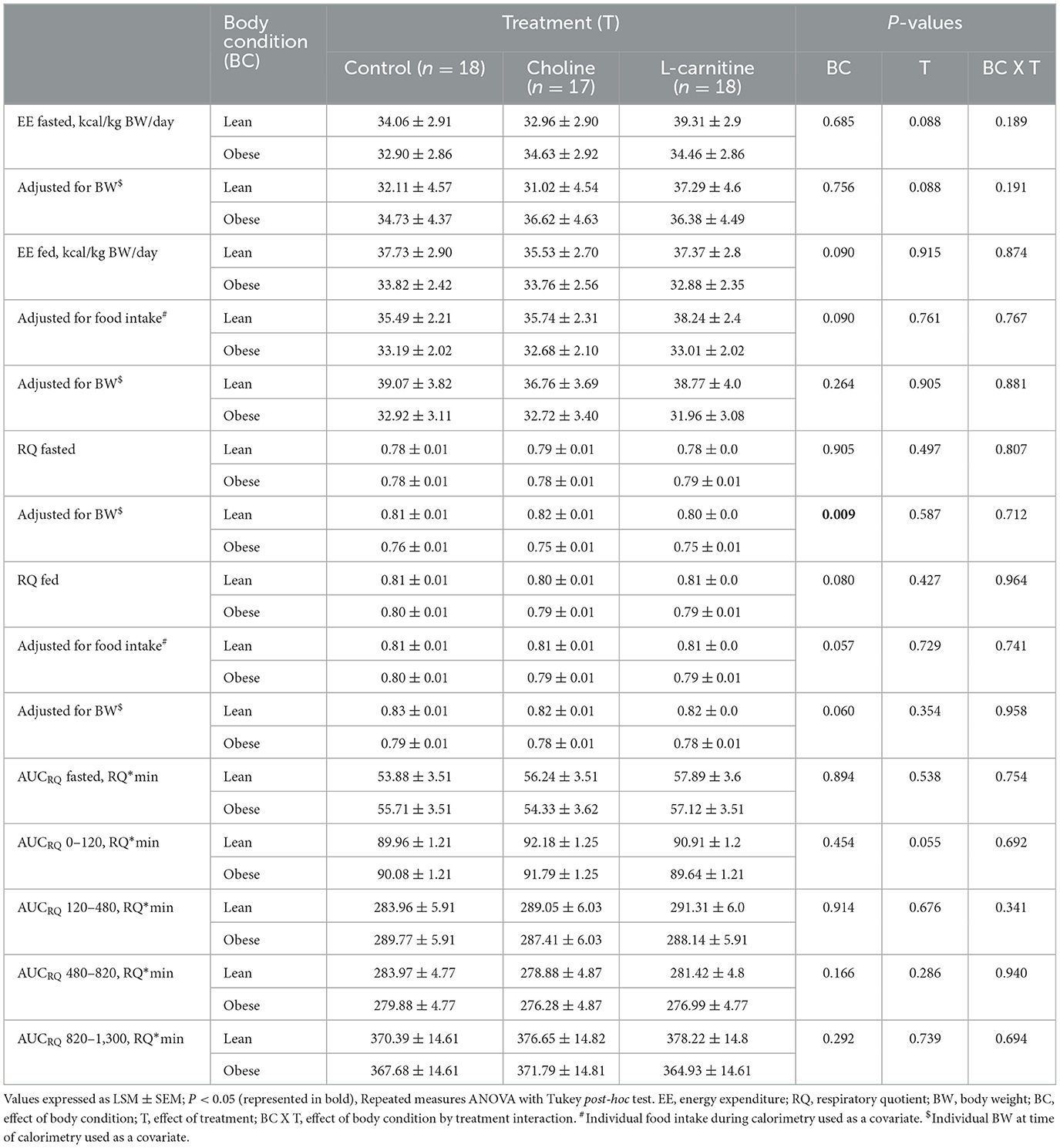
Table 5. EE and RQ of lean (n = 9) and obese (n = 9 for control and L-carnitine; n = 8 for choline) adult cats receiving supplemental dietary choline (378 mg/kg BW0.67), supplemental L-carnitine (200 mg/kg BW), or control (no additional supplement top-dressed onto a commercial extruded adult feline diet) and fed to maintenance energy requirements in a 3 x 3 Latin square design for 6-week periods.
Fasted RQ did not change with treatment, condition or treatment x body condition interaction (PTreatment = 0.497, PCondition= 0.905, PTreatmentxCondition = 0.807). When adjusted for BW, fasted RQ was lower in the obese cats (PCondition= 0.009). The effects of treatment and treatment x body condition interaction remained insignificant (PTreatment = 0.587, and PTreatmentxCondition = 0.712). Fed RQ was similarly not affected by treatment or treatment x body condition interaction (PTreatment = 0.427, and PTreatmentxCondition = 0.964), but there was a trend for fed RQ to change with body condition (PCondition= 0.080). These outcomes remained the same when fed RQ was adjusted for both BW (PTreatment = 0.354, PCondition= 0.060, and PTreatmentxCondition = 0.958), and food intake (PTreatment = 0.729, PCondition= 0.057, and PTreatmentxCondition = 0.741).
There was a tendency for AUCRQ 0–120 min postprandial to change with treatment in both lean and obese cats (PTreatment = 0.055). However, no effect of body condition or treatment x body condition interaction was observed (PCondition= 0.454, and PTreatmentxCondition = 0.692). Fasted AUC, and the remaining postprandial AUCRQ intervals (120–480, 480–820, and 1,300 min) were not affected by body condition (PCondition = 0.894, 0.914, 0.166, and 0.292, respectively), treatment (PTreatment = 0.538, 0.676, 0.286, and 0.739, respectively), or treatment x body condition interaction (PTreatmentxCondition = 0.754, 0.341, 0.940, and 0.694, respectively).
4. Discussion
To the authors' knowledge, this is the first study to investigate the effects of dietary choline supplementation in comparison to L-carnitine supplementation in obese and lean cats fed to maintain BW. Specifically, we sought to investigate lipotropic changes, as determined by serum lipid and lipoprotein profile, EE, RQ, and body composition data, between the two supplements. Previously, we reported that dietary choline at 5 and 6 x NRC RA increased serum lipid and lipoprotein profiles in overweight and obese cats, likely representing increased mobilization of hepatic lipids into circulation (21, 22). However, to allow the pet food industry and veterinary clinicians to make informed decisions regarding dietary supplementation and feline weight loss, it is important to assess the lipotropic effects of choline at the previously determined dose of 6 x NRC RA against L-carnitine (22), a supplement that has been frequently researched and used by the pet food industry (26–28). The dose of L-carnitine supplemented to the cats in the present trial was similarly based on previous research in overweight and obese cats (26, 28, 47, 48).
As expected, obese cats had greater food intake, energy intake, BW, BCS, and body composition values (total tissue mass, BF %, fat mass and LSTM), when compared to lean cats. These obese cats were specifically enrolled in this study due to their increased adiposity and were fed at energy intakes to support their current BW and BCS. A limitation of the present study was the approach to defining adiposity at enrollment, as the cats were classified as obese based solely on BCS. Body composition was not assessed by DXA at enrollment to evaluate BF % of the cats before group allocation and treatment. Although BF % differed between the lean and obese groups, the assigned mean BCS of the obese cats was higher than the respective mean BF % analyzed by DXA. The mean BF % of the obese cats was 32%, which corresponds with BCS 7/9 (36). Overall, all cats in the obese group could still be considered overweight or obese based on BF % (36), and BF % was still greater than in the lean cats. Because BCS is a subjective method of assessment, the BCS of the cats may have been overestimated due to localized fat deposits and/or abdominal fat pads which may have consisted of skin only. Similar limitations in investigator bias have previously been published in feline research (49, 50). Furthermore, differences in DXA machine, protocol, and computer software used by researchers can lead to differences in body composition and fat mass measurements among feline obesity studies (51).
Obesity results in alterations in the secretion of adipokines, leading to the development of other secondary health concerns such as insulin resistance and dyslipidemia (52–54). The obese cats in the present study had differences in their serum lipid and lipoprotein profiles, independent of treatment, when compared to the lean cats. Specifically, obese cats had overall concentrations of TAG and VLDL that were 59% greater than the lean cats. Additionally, overall concentrations of serum HDL-C were 13% lower in the obese cats compared to lean cats. To the authors' knowledge, there are no published reference ranges in cats for serum TAG, VLDL or HDL-C. However, the concentrations of serum TAG, VLDL and HDL-C reported herein were similar to those previously reported in overweight and obese adult cats receiving supplemental choline (21, 22). Serum TAG concentrations were also similar to those previously reported in healthy adult cats (55, 56). The present results align with previous reports of similarly higher circulating TAG, VLDL and lower HDL-C in obese cats, as compared to a lean control group (57–60). However, said authors also reported greater circulating NEFA, CHOL, and LDL-C concentrations in obese cats, which was not observed in the present study. As the mean age of the cats in the present study was lower than the mean age reported in these previous studies (57, 60), it is unclear if age may have also had an impact. To our knowledge, the effect of age on serum lipid, biochemistry and/or metabolomic profiles in overweight or obese cats has not specifically been investigated. However, cats under the age of two were reported to have lower serum CHOL and LDL-C concentrations as compared to cats over the age of two (BCS unknown) (61). Additionally, as these cats gained weight during a 12-week modified ad libitum feeding protocol during growth (25), there is a question of whether acute vs. chronic obesity may also impact lipoprotein profile and circulating metabolites. It is also worth considering that the increase in serum LDL and CHOL with choline treatment in both the lean and obese cats in the present study may have masked an effect of body condition that may have otherwise been observed. The same is possible for serum NEFA, where L-carnitine had a tendency to increase concentrations across both lean and obese cats.
The choline treatment in the present study provided a daily dietary choline intake of 6 x NRC RA, whereas cats on the L-carnitine and control treatments consumed a daily average choline intake of 1.2 and 1.3 x NRC RA, respectively. Both lean and obese cats had greater concentrations of CHOL, HDL-C-and LDL-C with the choline treatment, as compared to L-carnitine treatment (11, 7 and 33 % greater, respectively) and control (9, 9 and 13% greater, respectively). Serum CHOL concentrations remained within reference range for all treatments (2–12 mmol/L), and serum HDL-C and LDL-C concentrations were similar to those previously reported in obese and overweight adult cats consuming supplemental choline (21, 22). The greater concentrations of CHOL, HDL-C and LDL-C with choline in the present study are not surprising as similar changes were previously observed in obese and overweight cats consuming choline at 5 and 6 x NRC RA daily, respectively (21, 22). Choline is a precursor for PC, which is considered essential in the formation of VLDL. The VLDL are responsible for packaging TAG and CHOL, and exporting them out of the liver and into circulation (16). Similarly, both HDL-C and LCL-C have important roles in CHOL transport and circulation throughout the body, and require PC for their assembly (62, 63). Nascent HDL-C also requires PC for its formation within the liver (64). Contrary to what was observed, it was expected that serum TAG and VLDL would increase with choline supplementation in the present study. The present findings are contrary to our previous findings in obese and overweight cats consuming choline at 5 and 6 x NRC RA, where both serum TAG and VLDL concentrations were greater as compared to control (21, 22). Instead, obese cats receiving the L-carnitine treatment tended to have the greatest concentrations of serum TAG and VLDL. These results contradict the current research that exists on L-carnitine supplementation in hyperlipidemic animals and obese human patients with non-alcoholic steatohepatitis, in which TAG concentrations were reduced or unchanged (65–69). Although Rahbar et al. (70) observed increases in fasting plasma TAG concentrations when L-carnitine was supplemented to human patients with type II diabetes mellitus, the number of cats enrolled in the current study and the differences in BF % between the lean and obese group may not have been sufficient to detect these differences.
Although fasted serum glucose concentrations remained within reference range (4.4–7.7 mmol/L) for all the cats, glucose tended to be 16% greater in the obese cats than in the lean cats in the present study. Impaired glucose tolerance and insulin resistance are common findings in obese cats (9, 71–73), and a 30% decrease in insulin sensitivity has been reported with each additional kg of BW in cats (73). Over time, the decrease in insulin sensitivity may result in the development of type II diabetes mellitus (74), which is considered four times more likely in obese cats, as compared to lean cats (75). It is unclear if the increased TAG concentrations in the obese cats receiving L-carnitine may have been reflective of decreased glucose tolerance. Similar results have not been published in cats, and the use of L-carnitine in the treatment of obesity and diabetes mellitus in cats requires further investigation.
Serum ALP concentrations in the present study were within reference range for all cats throughout all three treatment periods (12–60 U/L), but tended to be, on average, 22% higher in the obese cats when compared to the lean cats. To the author's knowledge, there have been no published reports of increased ALP concentrations in healthy obese cats. However, in humans, a significant positive linear relationship between ALP and body mass index has been reported (76); where obese subjects had ALP concentrations that were on average 15 and 16% greater when compared to the non-obese and lean subjects, respectively (76, 77). This is because adipose tissue can express and release ALP into circulation (77). In the present study, it is likely that the greater serum ALP concentrations in the obese cats were representative of their increased adiposity, instead of their liver health. None of the cats showed any clinical signs of hepatic malfunction at any point throughout the study. However, serum liver enzyme values alone cannot adequately assess feline hepatic health and/or function. In the future, ultrasonography and histopathology of liver biopsies should be considered, along with pre- and postprandial bile acid profiles.
Conversely, lean cats consuming choline had the greatest concentrations of serum ALT. However, the concentrations similarly remained within reference range (31–105 U/L), suggesting that hepatobiliary diseases were absent in the present cats (78). Serum ALT concentrations were 26% greater in lean cats receiving the choline treatment as compared to control, and 20% greater as compared to the L-carnitine supplement. The present finding is unexpected, as ALT has been reported to increase in cases of non-alcoholic steatohepatitis resulting from choline deficiency in humans and in rodent models (79, 80). Additionally, these results contradict previous work by Verbrugghe et al. (81), where obese cats had higher ALT concentrations as compared to lean cats. Previously, choline supplementation did not affect serum ALT levels in overweight or obese cats (21, 22). The cats in the present study did not show signs of hepatic health concerns, regardless of treatment or body condition. However, as mentioned, hepatic health was not thoroughly investigated in the present study.
L-carnitine supplementation resulted in lower serum CREAT concentrations, as compared to the other two dietary treatments. This finding aligns with previous research in rodent models, where L-carnitine levels were inversely correlated with serum CREAT and renal function was improved with supplementation (82). The protective effects of L-carnitine on renal function are believed to be a result of its ability to reduce lipid peroxidation and free radical generation (83). Similarly, adult cats with chronic kidney disease (International Renal Interest Society Stages 1–2) consuming an average of 211 mg L-carnitine/kg BW from a test diet had serum CREAT levels that increased at a slower rate, as compared to the control group consuming an average of 2 mg L-carnitine/kg BW. However, the test diet and control diet differed in their overall nutrient profiles, and therefore the change in serum CREAT cannot be attributed to solely dietary L-carnitine intake (84). All cats in the present study were fed the same base diet, deemed in good health prior to enrollment with a complete blood count and serum biochemistry profile, and showed no signs of renal health concerns throughout the trial. Urinalysis and symmetric dimethyl arginine concentrations were not evaluated but should be considered for future L-carnitine studies.
Food and energy intakes were 0.3 % lower with choline treatment when compared to L-carnitine treatment, and 3% lower when compared to control. It is unclear whether the differences in food intake between treatments may have affected circulating metabolite concentrations, and to what effect. However, this reduction was not enough to influence BW, BCS, BF % and/or fat mass over the treatment periods. Similarly, it is also unlikely that this level of energy reduction was enough to put the cats in a negative energy balance and cause the observed lipid mobilization with choline treatment. It is unclear whether the change in food intake was due to palatability or due to a potential effect of satiety. A similar reduction in food intake was previously reported in growing kittens consuming choline at 3 x NRC RA. However, there were no changes in the analyzed fasted serum satiety hormone concentrations (ghrelin, leptin, glucagon-like peptide-1, peptide-YY or gastric inhibitory polypeptide) of said kittens with choline treatment (25). The mechanism through which choline may influence food intake in cats requires further elucidation.
Although there were no differences between dietary treatments on FM or BF %, there was a trend for greater LSTM in both obese and lean cats with treatment. On average, LSTM was 1.5% greater with choline treatment as compared to control, and 0.8% greater as compared to L-carnitine treatment. Supplementing betaine (a derivative of choline) in livestock species such as pigs has resulted in increased LSTM (23, 24). Although the exact mechanism has not been identified, it is presumably due to the role that betaine has as a possible methyl donor for the re-methylation of methionine; thus sparing methionine for protein synthesis and leading to increased s-adenosylmethionine production (85). There were no differences in LSTM with choline supplementation at 3 x NRC RA in growing kittens being fed ad libitum (25). However, supplemented kittens had lower food and subsequent protein intakes which may have impacted these results. In the present study, LSTM was 0.8% greater with L-carnitine compared to control. This observation aligns with previous research by Center et al. (26) in which overweight cats consumed a weight loss diet supplemented with 0 (control), 50, 100, or 150 mg carnitine/kg diet. Cats supplemented with carnitine achieved an increase in LSTM and a decrease in FM sooner (by day 42) compared to the cats receiving control (by day 84). These differences were attributed to lower RQ with treatment, indicating increased fat oxidation. Similarly, underweight and lean cats (BCS: 2.5–4/5) fed at 120% MER had lower fat deposition when supplemented 188 mg L-carnitine/kg diet compared to 121 mg L-carnitine/kg diet (28). However, the mechanism of action was unclear as there were no changes in EE, RQ or voluntary physical activity with dose throughout said 16-week trial.
Although EE is dependent on LSTM (86), the differences in LSTM between choline and the remaining treatments in the present study were minor and were likely not enough to elicit changes in EE. Instead, choline tended to increase RQ in the 120-min postprandial period, as compared to the other two treatments. The increase in RQ immediately post-feeding (0–120 min) may suggest a greater reliance on carbohydrate oxidation and less on gluconeogenesis with choline supplementation. This finding was not previously observed in overweight and obese cats or in guinea pigs supplemented with choline (21, 22, 87). It remains unclear why this may have occurred in the present study. There was a trend for greater fasted EE across all cats with the L-carnitine treatment. These findings align with previous research investigating L-carnitine supplementation in overweight cats. Overweight cats receiving 100 mg L-carnitine/kg BW similarly had greater fasted EE after 21 and 42 days of supplementation, as compared to overweight cats fed control (27). Although fed EE did not change with treatment in the present study, there was a trend for lower fed EE and fed RQ in the obese cats, as compared to the lean cats. Lower EE in overweight and obese cats as compared to lean cats is a common finding as an animal's surface area will influence EE (27, 86, 88). Perseghin et al. (89) similarly observed that overweight human participants had lower fed RQ when compared to lean participants, although not significant. The authors suggested that the increased leptin in the overweight participants may have played a role in promoting fatty acids toward oxidation instead of storage (90). However, said overweight study participants had normal insulin and leptin sensitivity. Although there are reports of decreased insulin and leptin sensitivity with weight gain in cats (71, 91), neither leptin nor insulin were measured in the obese cats in the present study and we therefore cannot conclude if the same association was present.
In agreement with previous publications, the present study found clear differences in circulating lipid and lipoprotein concentrations of obese and lean cats. Specifically, obese cats had greater concentrations of circulating TAG and VLDL, and lower concentrations of circulating HDL-C. Despite the differences associated with obesity, choline supplementation at six times the published RA resulted in greater HDL-C, CHOL and LDL-C in all cats. These findings are in alignment with our previous research in overweight and obese cats, which similarly found greater circulating lipid and lipoprotein concentrations with choline supplementation. These results suggest that choline supplementation may be beneficial in mobilizing lipids out of the liver and into circulation. Based on the findings of the present research, choline shows more promise than L-carnitine as a supplement for combating the risk of FHL in cats. While choline decreased food intake and had a tendency to improve LSTM, it did not increase EE in the same manner that L-carnitine did. Thus, future research should focus on evaluating the synergistic benefits of supplementing dietary choline and L-carnitine together to obese cats on a weight loss program. Together, L-carnitine and choline may prove useful in promoting weight loss, while maintaining LSTM and minimizing hepatic lipid stores in these cats.
Data availability statement
The raw data supporting the conclusions of this article will be made available by the authors, without undue reservation.
Ethics statement
The animal study was reviewed and approved by University of Guelph Animal Care Committee (AUP#4496).
Author contributions
AV, MB, and GK secured funding for this research. AR, AS, MB, GK, and AV were responsible for designing this study. AR, SV-S, and AV were responsible for data collection. AR was responsible for statistical analysis and writing of the manuscript. All authors contributed to editing and reviewing this manuscript.
Funding
Funding for this research was provided by a Natural Sciences and Engineering Research Council (NSERC) Collaborative Research and Development Grant (CRDPJ #472710-16), in partnership with Elmira Pet Products (Elmira, ON, Canada). The choline chloride and L-carnitine supplements were kindly provided by Balchem (New Hampton, NY, United States of America).
Acknowledgments
We would like to thank all the graduate students, summer students, and student volunteers within the Verbrugghe and Shoveller labs for their assistance with this trial and Drs. Sarah Dodd, Melissa Sinclair, Tainor Tisotti, and Flavio Freitag for helping with the anesthesia and blood collection. Additionally, a special thank you to Susan Kinsella for her assistance with material procurement.
Conflict of interest
AR declares that they have participated in paid engagements with and received scholarships from pet food companies within Canada. At the time of the study, SV-S held the position of Registered Veterinary Technician in Clinical Nutrition funded by Nestlé Purina Proplan Veterinary Diets, they were previously employed by Hill's Pet Nutrition, and received honoraria from pet food manufacturers. AS is the Champion Petfoods Chair in Canine and Feline Nutrition, Physiology and Metabolism, consults for Champion Petfood, was previously employed by P&G and Mars Pet Care, serves on the Scientific Advisory Board for Trouw Nutrition, and has received honoraria and research funding from various commodity groups, pet food manufacturers, and ingredient suppliers. AV is the Royal Canin Veterinary Diets Endowed Chair in Canine and Feline Clinical Nutrition, serves on the Health and Nutrition Advisory Board for Vetdiet and has received honoraria and research funding from various pet food manufacturers and ingredient suppliers.
The remaining authors declare that the research was conducted in the absence of any commercial or financial relationships that could be construed as a potential conflict of interest.
Publisher's note
All claims expressed in this article are solely those of the authors and do not necessarily represent those of their affiliated organizations, or those of the publisher, the editors and the reviewers. Any product that may be evaluated in this article, or claim that may be made by its manufacturer, is not guaranteed or endorsed by the publisher.
References
1. Armstrong PJ, Blanchard G. Hepatic lipidosis in cats. Vet Clin North Am Small Anim Pract. (2009) 39:599–616. doi: 10.1016/j.cvsm.2009.03.003
2. Hall JA, Barstad LA, Connor WE. Lipid composition of hepatic and adipose tissues from normal cats and from cats with idiopathic hepatic lipidosis. J Vet Intern Med Am Coll Vet Intern Med. (1997) 11:238–42. doi: 10.1111/j.1939-1676.1997.tb00097.x
3. Biourge VC, Groff JM, Munn RJ, Kirk CA, Nyland TG, Madeiros VA, et al. Experimental induction of hepatic lipidosis in cats. Am J Vet Res. (1994) 55:1291–302.
4. Biourge V, Bee D, Rogers QR, Fisher C, Groff JM, Morris JG. Nitrogen balance, plasma free amino acid concentrations and urinary orotic acid excretion during long-term fasting in cats. J Nutr. (1994) 124:1094–103. doi: 10.1093/jn/124.7.1094
5. Blanchard G, Paragon BM, Serougne C, Lutton C, Ferezou J, Milliat F, et al. Plasma lipids, lipoprotein composition and profile during induction and treatment of hepatic lipidosis in cats and the metabolic effect of one daily meal in healthy cats. J Anim Physiol Anim Nutr. (2004) 88:73–87. doi: 10.1111/j.1439-0396.2003.00462.x
6. Center SA. Feline hepatic lipidosis. Vet Clin North Am Small Anim Pract. (2005) 35:225–69. doi: 10.1016/j.cvsm.2004.10.002
7. Kuzi S, Segev G, Kedar S, Yas E, Aroch I. Prognostic markers in feline hepatic lipidosis: A retrospective study of 71 cats. Vet Rec. (2017) 181:512–512. doi: 10.1136/vr.104252
8. Brown B, Mauldin GE, Armstrong J, Moroff SD, Mauldin GN. Metabolic and hormonal alterations in cats with hepatic lipidosis. J Vet Intern Med. (2010) 14:20–6. doi: 10.1111/j.1939-1676.2000.tb01494.x
9. Biourge V, Nelson RW, Feldman EC, Willits NH, Morris JG, Rogers QR. Effect of weight gain and subsequent weight loss on glucose tolerance and insulin response in healthy cats. J Vet Intern Med Am Coll Vet Intern Med. (1997) 11:86–91. doi: 10.1111/j.1939-1676.1997.tb00078.x
10. Clark MH, Larsen R, Lu W, Hoenig M. Investigation of 1H MRS for quantification of hepatic triglyceride in lean and obese cats. Res Vet Sci. (2013) 95:678–80. doi: 10.1016/j.rvsc.2013.04.004
11. German AJ, Holden S, Bissot T, Morris PJ, Biourge V. Changes in body composition during weight loss in obese client-owned cats: loss of lean tissue mass correlates with overall percentage of weight lost. J Feline Med Surg. (2008) 10:452–9. doi: 10.1016/j.jfms.2008.02.004
12. Bissot T, Servet E, Vidal S, Deboise M, Sergheraert R, Egron G, et al. Novel dietary strategies can improve the outcome of weight loss programmes in obese client-owned cats. J Feline Med Surg. (2010) 12:104–12. doi: 10.1016/j.jfms.2009.07.003
13. Fritz IB. Carnitine and its role in fatty acid metabolism. Adv Lipid Res. (1963) 1:285–334. doi: 10.1016/B978-1-4831-9937-5.50014-4
14. Nguyen P, Leray V, Diez M, Serisier S, Le Bloc'h J, Siliart B, et al. Liver lipid metabolism. J Anim Physiol Anim Nutr. (2008) 92:272–83. doi: 10.1111/j.1439-0396.2007.00752.x
15. Verbrugghe A, Bakovic M. Peculiarities of one-carbon metabolism in the strict carnivorous cat and the role in feline hepatic lipidosis. Nutrients. (2013) 5:2811–35. doi: 10.3390/nu5072811
16. Yao Z, Vance DE. The active synthesis of phosphatidylcholine Is required for very low density lipoprotein secretion from rat hepatocytes. J Biol Chem. (1988) 263:2998–3004. doi: 10.1016/S0021-9258(18)69166-5
17. Stead LM, Brosnan JT, Brosnan ME, Vance DE, Jacobs RL. Is it time to reevaluate methyl balance in humans? Am J Clin Nutr. (2006) 83:5–10. doi: 10.1093/ajcn/83.1.5
18. da Silva AC, Mansur Guerios MF, Monsao SR. The domestic cat as a laboratory animal for experimental nutrition studies: VI. Choline deficiency. J Nutr. (1959) 67:537–47. doi: 10.1093/jn/67.4.537
19. Anderson PA, Baker DH, Sherry PA, Corbin JE. Choline-methionine interrelationship in feline nutrition. J Anim Sci. (1979) 49:522–7. doi: 10.2527/jas1979.492522x
20. Schaeffer MC, Rogers QR, Morris JG. The choline requirement of the growing kitten in the presence of just adequate dietary methionine. Nutr Res. (1982) 2:289–99. doi: 10.1016/S0271-5317(82)80010-9
21. Verbrugghe A, Rankovic A, Armstrong S, Santarossa A, Kirby GM, Bakovic M. Serum lipid, amino acid and acylcarnitine profiles of obese cats supplemented with dietary choline and fed to maintenance energy requirements. Animals. (2021) 11:2196. doi: 10.3390/ani11082196
22. Rankovic A, Godfrey H, Grant CE, Shoveller AK, Bakovic M, Kirby G, et al. Dose-response relationship between dietary choline and serum lipid profile, energy expenditure and respiratory quotient in overweight adult cats fed at maintenance energy requirements. J Anim Sci. (2022) 100:skac202. doi: 10.1093/jas/skac202
23. Matthews JO, Southern LL, Higbie AD, Persica MA, Bidner TD. Effects of betaine on growth, carcass characteristics, pork quality, and plasma metabolites of finishing pigs. J Anim Sci. (2001) 79:722–8. doi: 10.2527/2001.793722x
24. Zhan XA Li JX, Xu ZR, Zhao RQ. Effects of methionine and betaine supplementation on growth performance, carcase composition and metabolism of lipids in male broilers. Br Poult Sci. (2006) 47:576–80. doi: 10.1080/00071660600963438
25. Godfrey H, Rankovic A, Grant CE, Shoveller AK, Bakovic M, Abood SK, et al. Dietary choline in gonadectomized kittens improved food intake and body composition but not satiety, serum lipids, or energy expenditure. PLoS ONE. (2022) 17:e0264321. doi: 10.1371/journal.pone.0264321
26. Center SA, Warner KL, Randolph JF, Sunvold GD, Vickers JR. Influence of dietary supplementation with l-carnitine on metabolic rate, fatty acid oxidation, body condition, and weight loss in overweight cats. Am J Vet Res. (2012) 73:1002–15. doi: 10.2460/ajvr.73.7.1002
27. Shoveller AK, Minikhiem DL, Carnagey K, Brewer J, Westendorf R, DiGennaro J, et al. Low level of supplemental dietary L-carnitine increases energy expenditure in overweight, but not lean, cats fed a moderate energy density diet to maintain body weight. Int J Appl Res Vet Med. (2014) 12:33–43.
28. Gooding MA, Minikhiem DL, Shoveller AK. Cats in positive energy balance have lower rates of adipose gain when fed diets containing 188 versus 121 ppm L-carnitine. Sci World J. (2016) 2016:1–7. doi: 10.1155/2016/2649093
29. Wei Y, Rector R, Thyfault J, Ibdath J. Non-alcoholic fatty liver disease and mitochondrial dysfunction. World J Gastroenterol. (2008) 14:193–9. doi: 10.3748/wjg.14.193
30. Armstrong P. Feline hepatic lipidosis. In: Proceedings of the 7th Annual ACVIM Forum. San Diego, CA: ACVIM (1989). p. 335–7.
31. Jacobs G, Cornelius L, Keene B, Rakich P, Shug A. Comparison of plasma, liver, and skeletal muscle carnitine concentrations in cats with idiopathic hepatic lipidosis and in healthy cats. Am J Vet Res. (1990) 51:1349–51.
32. Blanchard G, Paragon BM, Milliat F, Lutton C. Dietary l-carnitine supplementation in obese cats alters carnitine metabolism and decreases ketosis during fasting and induced hepatic lipidosis. J Nutr. (2002) 132:204–10. doi: 10.1093/jn/132.2.204
33. Aroch I, Shechter-Polak M, Segev G. A retrospective study of serum β-hydroxybutyric acid in 215 ill cats: clinical signs, laboratory findings and diagnoses. Vet J. (2012) 191:240–5. doi: 10.1016/j.tvjl.2011.01.010
34. Gorman L, Sharkey LC, Armstrong PJ, Little K, Rendahl A. Serum beta hydroxybutyrate concentrations in cats with chronic kidney disease, hyperthyroidism, or hepatic lipidosis. J Vet Intern Med. (2016) 30:611–6. doi: 10.1111/jvim.13826
35. The Association of American Feed Control Officials. Official Publication. Champaign, IL: The Association of American Feed Control Officials (2021).
36. Laflamme D. Development and validation of a body condition score system for cats: a clinical tool. Feline Pract St Barbara Calif. (1997) 25:13–8.
37. Frayne J, Murray SM, Croney C, Flickinger E, Edwards M, Shoveller AK. The behavioural effects of innovative litter developed to attract cats. Animals. (2019) 9:683. doi: 10.3390/ani9090683
38. Horwitz W, Chichilo P, Reynolds H. Official Methods of Analysis of the Association of Official Analytical Chemists. Washington, DC: Association of Official Analytical Chemists (1970).
39. American Oil Chemists' Society, Firestone D. Official Methods and Recommended Practices of the American Oil Chemists' Society. Urbana, IL: AOCS press (1994).
40. Cargo-Froom CL, Fan MZ, Pfeuti G, Pendlebury C, Shoveller AK. Apparent and true digestibility of macro and micro nutrients in adult maintenance dog foods containing either a majority of animal or vegetable proteins1. J Anim Sci. (2019) 97:1010–9. doi: 10.1093/jas/skz001
41. National Research Council. Nutrient Requirements of Dogs and Cats. Washington, DC: The National Academies Press (2006).
42. Gooding MA, Duncan IJH, Atkinson JL, Shoveller AK. Development and validation of a behavioral acclimation protocol for cats to respiration chambers used for indirect calorimetry studies. J Appl Anim Welf Sci. (2012) 15:144–62. doi: 10.1080/10888705.2012.658332
43. Weir J. New methods for calculating metabolic rate with special reference to protein metabolism. J Physiol. (1949) 109:1–9. doi: 10.1113/jphysiol.1949.sp004363
45. Friedewald WT, Levy RI, Fredrickson DS. Estimation of the concentration of low-density lipoprotein cholesterol in plasma, without use of the preparative ultracentrifuge. Clin Chem. (1972) 18:499–502. doi: 10.1093/clinchem/18.6.499
46. Bjørnvad CR, Nielsen ME, Hansen SEM, Nielsen DH. The effect of position on the precision of dual-energy X-ray absorptiometry and correlation with body condition score in dogs and cats. J Nutr Sci. (2017) 6:e20. doi: 10.1017/jns.2017.16
47. Sunvold GD, Tetrick MA, Davenport GM. Process and Product for Promoting Weight Loss in Overweight Dogs. (2000). Available online at: https://patents.google.com/patent/WO2000000039A1/en?oq=cats+and+carnitine (accessed March 1, 2023).
48. Shug AL, Keene BW. L-carnitine Enriched Pet Food. (1995). Available online at: https://patents.google.com/patent/CA1336505C/en?oq=cats+and+carnitine (accessed March, 1 2023).
49. Keller C, Liesegang A, Frey D, Wichert B. Metabolic response to three different diets in lean cats and cats predisposed to overweight. BMC Vet Res. (2017) 13:1–10. doi: 10.1186/s12917-017-1107-3
50. Shoveller AK, DiGennaro J, Lanman C, Spangler D. Trained vs untrained evaluator assessment of body condition score as a predictor of percent body fat in adult cats. J Feline Med Surg. (2014) 16:957–65. doi: 10.1177/1098612X14527472
51. Bjørnvad CR, Nielsen DH, Armstrong PJ, McEvoy F, Hoelmkjaer KM, Jensen KS, et al. Evaluation of a nine-point body condition scoring system in physically inactive pet cats. Am J Vet Res. (2011) 72:433–7. doi: 10.2460/ajvr.72.4.433
52. Coppack SW. Pro-inflammatory cytokines and adipose tissue. Proc Nutr Soc. (2001) 60:349–56. doi: 10.1079/PNS2001110
53. Sowers M, Jannausch M, Stein E, Jamadar D, Hochberg M, Lachance L. C-reactive protein as a biomarker of emergent osteoarthritis. Osteoarthritis Cartilage. (2002) 10:595–601. doi: 10.1053/joca.2002.0800
54. Trayhurn P. Adipose tissue in obesity—an inflammatory issue. Endocrinology. (2005) 146:1003–5. doi: 10.1210/en.2004-1597
55. Pazak HE, Bartges JW, Cornelius LC, Scott MA, Gross K, Huber TL. Characterization of serum lipoprotein profiles of healthy, adult cats and idiopathic feline hepatic lipidosis patients. J Nutr. (1998) 128:2747S–50S. doi: 10.1093/jn/128.12.2747S
56. Templeman JR, Hogan K, Blanchard A, Marinangeli CP, Camara A, Verbrugghe A, et al. Effect of raw and encapsulated policosanol on lipid profiles, blood biochemistry, activity, energy expenditure and macronutrient metabolism of adult cats. J Feline Med Surg. (2021) 24:177–84. doi: 10.1177/1098612X211013738
57. Jordan E, Kley S, Le NA, Waldron M, Hoenig M. Dyslipidemia in obese cats. Domest Anim Endocrinol. (2008) 35:290–9. doi: 10.1016/j.domaniend.2008.05.008
58. Hoenig M, Wilkins C, Holson JC, Ferguson DC. Effects of obesity on lipid profiles in neutered male and female cats. (2003) 64:299–303. doi: 10.2460/ajvr.2003.64.299
59. Muranaka S, Mori N, Hatano Y, Saito TR, Lee P, Kojima M, et al. Obesity induced changes to plasma adiponectin concentration and cholesterol lipoprotein composition profile in cats. Res Vet Sci. (2011) 91:358–61. doi: 10.1016/j.rvsc.2010.09.012
60. Chala IV, Feshchenk DV, Dubova OA, Bakhur TI, Zghozinska OA, Rusak VS. Changes in the lipid profile of neutered cats' blood in cases of obesity and diabetes. Vet Arh. (2021) 91:635–45. doi: 10.24099/vet.arhiv.1087
61. Osorio JH, Cañas EZ, Pérez JE. Comparison of lipid profile in domestic cat by gender and age. Bol Cient Mus Hist Nat. (2012) 16:175–82.
62. Fielding PE, Fielding CJ. Dynamics of lipoprotein transport in the human circulatory system. In: New Comprehensive Biochemistry. Amsterdam: Elsevier (2002). p. 527–52.
63. Cole LK, Vance JE, Vance DE. Phosphatidylcholine biosynthesis and lipoprotein metabolism. Triglyceride Metab Dis. (2012) 1821:754–61. doi: 10.1016/j.bbalip.2011.09.009
64. Forte TM, Bielicki JK, Goth-Goldstein R, Selmek J, McCall MR. Recruitment of cell phospholipids and cholesterol by apolipoproteins A-II and AI: formation of nascent apolipoprotein-specific HDL that differ in size, phospholipid composition, and reactivity with LCAT. J Lipid Res. (1995) 36:148–57. doi: 10.1016/S0022-2275(20)39763-7
65. Maccari F, Arseni A, Chiodi P, Ramacci MT, Angelucci L, Hulsmann WC. L-carnitine effect on plasma lipoproteins of hyperlipidemic fat-loaded rats. Lipids. (1987) 22:1005–8. doi: 10.1007/BF02536440
66. Seccombe DW, James L, Hahn P, Jones E. L-carnitine treatment in the hyperlipidemic rabbit. Metabolism. (1987) 36:1192–6. doi: 10.1016/0026-0495(87)90247-2
67. James L, Jalaluddin Bhuiyan AKM, Foster D, Seccombe D. Effect of L-carnitine treatment on very low density lipoprotein kinetics in the hyperlipidemic rabbit. Clin Biochem. (1995) 28:451–8. doi: 10.1016/0009-9120(95)00026-6
68. Malaguarnera M, Gargante MP, Russo C, Antic T, Vacante M, Malaguarnera M, et al. L-carnitine supplementation to diet: a new tool in treatment of nonalcoholic steatohepatitis—a randomized and controlled clinical trial. Off J Am Coll Gastroenterol ACG. (2010) 105:1338–45. doi: 10.1038/ajg.2009.719
69. Malek Mahdavi A, Mahdavi R, Kolahi S, Zemestani M, Vatankhah AM. L-carnitine supplementation improved clinical status without changing oxidative stress and lipid profile in women with knee osteoarthritis. Nutr Res. (2015) 35:707–15. doi: 10.1016/j.nutres.2015.06.003
70. Rahbar AR, Shakerhosseini R, Saadat N, Taleban F, Pordal A, Gollestan B. Effect of L-carnitine on plasma glycemic and lipidemic profile in patients with type II diabetes mellitus. Eur J Clin Nutr. (2005) 59:592–6. doi: 10.1038/sj.ejcn.1602109
71. Appleton DJ, Rand JS, Sunvold GD. Insulin sensitivity decreases with obesity, and lean cats with low insulin sensitivity are at greatest risk of glucose intolerance with weight gain. J Feline Med Surg. (2001) 3:211–28. doi: 10.1053/jfms.2001.0138
72. Hoenig M, Alexander S, Holson J, Ferguson DC. Influence of glucose dosage on interpretation of intravenous glucose tolerance tests in lean and obese cats. J Vet Intern Med. (2002) 16:529–32. doi: 10.1111/j.1939-1676.2002.tb02382.x
73. Hoenig M, Thomaseth K, Waldron M, Ferguson DC. Insulin sensitivity, fat distribution, and adipocytokine response to different diets in lean and obese cats before and after weight loss. Am J Physiol-Regul Integr Comp Physiol. (2007) 292:R227–34. doi: 10.1152/ajpregu.00313.2006
74. Reaven G. Banting lecture 1988. Role of insulin resistance in human disease. Diabetes. (1988) 37:1595–607. doi: 10.2337/diabetes.37.12.1595
75. Scarlett JM, Donoghue S. Associations between body condition and disease in cats. J Am Vet Med Assoc. (1998) 212:1725–31.
76. Khan AR, Awan FR, Najam S, Islam M, Siddique T, Zain M. Elevated serum level of human alkaline phosphatase in obesity. Age Years. (2015) 48:42–5.
77. Ali AT, Paiker JE, Crowther NJ. The relationship between anthropometry and serum concentrations of alkaline phosphatase isoenzymes, liver enzymes, albumin, and bilirubin. Am J Clin Pathol. (2006) 126:437–42. doi: 10.1309/9N346GXX67B6PX5W
78. Wagner KA, Hartmann FA, Trepanier LA. Bacterial culture results from liver, gallbladder, or bile in 248 dogs and cats evaluated for hepatobiliary disease: 1998–2003. J Vet Intern Med. (2007) 21:417–24. doi: 10.1111/j.1939-1676.2007.tb02984.x
79. Zeisel SH, Da Costa KA, Franklin PD, Alexander EA, Lamont TA, Sheard NF, et al. Choline, an essential nutrient for humans. FASEB J. (1991) 5:2093–8. doi: 10.1096/fasebj.5.7.2010061
80. Rinella ME, Green RM. The methionine-choline deficient dietary model of steatohepatitis does not exhibit insulin resistance. J Hepatol. (2004) 40:47–51. doi: 10.1016/j.jhep.2003.09.020
81. Verbrugghe A, Hesta M, Gommeren K, Daminet S, Wuyts B, Buyse J, et al. Oligofructose and inulin modulate glucose and amino acid metabolism through propionate production in normal-weight and obese cats. Br J Nutr. (2009) 102:694–702. doi: 10.1017/S0007114509288982
82. Abu Ahmad N, Armaly Z, Berman S, Jabour A, Aga-Mizrachi S, Mosenego-Ornan E, et al. l-Carnitine improves cognitive and renal functions in a rat model of chronic kidney disease. Physiol Behav. (2016) 164:182–8. doi: 10.1016/j.physbeh.2016.05.036
83. Mister M, Noris M, Szymczuk J, Azzollini N, Aiello S, Abbate M, et al. Propionyl-l-carnitine prevents renal function deterioration due to ischemia/reperfusion. Kidney Int. (2002) 61:1064–78. doi: 10.1046/j.1523-1755.2002.00212.x
84. Hall JA, Fritsch DA, Jewell DE, Burris PA, Gross KL. Cats with IRIS stage 1 and 2 chronic kidney disease maintain body weight and lean muscle mass when fed food having increased caloric density, and enhanced concentrations of carnitine and essential amino acids. Vet Rec. (2019) 184:190–190. doi: 10.1136/vr.104865
85. Robinson JL, Harding SV, Brunton JA, Bertolo RF. Dietary methyl donors contribute to whole-body protein turnover and protein synthesis in skeletal muscle and the jejunum in neonatal piglets. J Nutr. (2016) 146:2007–12. doi: 10.3945/jn.115.226035
86. Center SA, Warner KL, Randolph JF, Wakshlag JJ, Sunvold GD. Resting energy expenditure per lean body mass determined by indirect calorimetry and bioelectrical impedance analysis in cats. J Vet Intern Med. (2011) 25:1341–50. doi: 10.1111/j.1939-1676.2011.00824.x
87. Daily JW, Hongu N, Mynatt RL, Sachan DS. Choline supplementation increases tissue concentrations of carnitine and lowers body fat in guinea pigs. J Nutr Biochem. (1998) 9:464–70. doi: 10.1016/S0955-2863(98)00044-8
88. Heymsfield SB, Thomas D, Bosy-Westphal A, Shen W, Peterson CM, Müller MJ. Evolving concepts on adjusting human resting energy expenditure measurements for body size. Obes Rev. (2012) 13:1001–14. doi: 10.1111/j.1467-789X.2012.01019.x
89. Perseghin G, Scifo P, Danna M, Battezzati A, Benedini S, Meneghini E, et al. Normal insulin sensitivity and IMCL content in overweight humans are associated with higher fasting lipid oxidation. Am J Physiol-Endocrinol Metab. (2002) 283:E556–64. doi: 10.1152/ajpendo.00127.2002
90. Unger RH, Zhou YT, Orci L. Regulation of fatty acid homeostasis in cells: novel role of leptin. Proc Natl Acad Sci. (1999) 96:2327–32. doi: 10.1073/pnas.96.5.2327
Keywords: dual-energy x-ray absorptiometry, feline nutrition, indirect calorimetry, methionine, methyl donor, one carbon metabolism, pet obesity
Citation: Rankovic A, Verton-Shaw S, Shoveller AK, Bakovic M, Kirby G and Verbrugghe A (2023) Dietary choline, but not L-carnitine, increases circulating lipid and lipoprotein concentrations, without affecting body composition, energy expenditure or respiratory quotient in lean and obese male cats during weight maintenance. Front. Vet. Sci. 10:1198175. doi: 10.3389/fvets.2023.1198175
Received: 31 March 2023; Accepted: 11 July 2023;
Published: 26 July 2023.
Edited by:
Bangyuan Wu, China West Normal University, ChinaReviewed by:
Lynn Weber, University of Saskatchewan, CanadaChristian Visscher, University of Veterinary Medicine Hannover, Germany
Copyright © 2023 Rankovic, Verton-Shaw, Shoveller, Bakovic, Kirby and Verbrugghe. This is an open-access article distributed under the terms of the Creative Commons Attribution License (CC BY). The use, distribution or reproduction in other forums is permitted, provided the original author(s) and the copyright owner(s) are credited and that the original publication in this journal is cited, in accordance with accepted academic practice. No use, distribution or reproduction is permitted which does not comply with these terms.
*Correspondence: Adronie Verbrugghe, YXZlcmJydWdAdW9ndWVscGguY2E=