- 1Section of Neurology, Department of Small Animals, Vetsuisse Faculty Zurich, University of Zurich, Zurich, Switzerland
- 2Graduate School for Cellular and Biomedical Sciences, University of Bern, Bern, Switzerland
- 3Clinic for Diagnostic Imaging, Department of Diagnostics and Clinical Services, Vetsuisse-Faculty Zurich, University of Zurich, Zurich, Switzerland
- 4Department of Small Animal Medicine and Surgery, University of Veterinary Medicine Hannover, Hannover, Germany
- 5Section of Anaesthesiology, Department of Clinical Diagnostics and Services, Vetsuisse-Faculty Zurich, University of Zurich, Zurich, Switzerland
- 6Vet Oracle Teleradiology, Norfolk, United Kingdom
- 7Department of Surgical Sciences, Radiology, Uppsala University, Uppsala, Sweden
- 8Faculty of Medicine, University of Geneva, Geneva, Switzerland
Introduction: The understanding of epileptic seizure pathogenesis has evolved over time, and it is now generally accepted that not only are cortical and subcortical areas involved but also the connection of these regions in the white matter (WM). Recent human neuroimaging studies confirmed the involvement of the WM in several epilepsy syndromes. Neuroimaging studies investigating WM integrity with diffusion tensor imaging (DTI) in canine idiopathic epilepsy are lacking. This study aimed to test the hypothesis that WM diffusion changes can be found in dogs affected by idiopathic epilepsy.
Method: Twenty-six dogs with idiopathic epilepsy (15 Border Collies and 11 Greater Swiss Mountain dogs) and 24 healthy controls (11 Beagle dogs, 5 Border Collies, and 8 Greater Swiss Mountain dogs) were prospectively enrolled. Most dogs with idiopathic epilepsy (17/26) were enrolled within 3 months after seizure onset. Diffusion tensor imaging of the brain with 32 diffusion directions (low b value = 0 s/mm2; maximal b value = 800 s/mm2) was performed in a 3 Tesla scanner. Tract-based spatial statistics (TBSS), a voxel-based approach, was used to investigate changes in fractional anisotropy (FA) and mean diffusivity (MD) in the idiopathic epilepsy group compared to the healthy control group. Additionally, FA and MD were investigated in the region of corpus callosum and cingulate white matter in both groups.
Results: We observed subtle changes in WM DTI between the idiopathic epilepsy group and the healthy control group limited to cingulate WM, with a significantly lower FA in the idiopathic epilepsy group compared to the healthy control group in the region of interest (ROI) approach (p = 0.027). No significant changes were found between the idiopathic epilepsy group and the healthy control group in the TBSS analysis and in the corpus callosum in the ROI approach.
Conclusion: This study supports the cingulate area as a target structure in canine epilepsy. The subtle changes only might be explained by the short duration of epilepsy, small sample sizes, and the higher variability in canine brain anatomy. Furthermore, all included dogs showed generalized tonic-clonic seizures, possibly affected by generalized epilepsy syndrome, which are also associated with less pronounced DTI changes in humans than focal epilepsy syndromes.
1 Introduction
Canine idiopathic epilepsy is diagnosed based on the age of the dog at the onset of the epileptic seizure, unremarkable inter-ictal physical and neurological examination, and exclusion of metabolic, toxic, and structural cerebral disorders by means of diagnostic investigations (1). Magnetic resonance imaging (MRI) of the brain is routinely used as an important diagnostic step for idiopathic epilepsy, and the diagnosis is based on a normal structural brain MRI (2). However, epilepsy is increasingly recognized as a disease of the brain network, involving both gray matter and white matter, which cannot be assessed from conventional MRI sequences, but only with advanced MRI techniques (3–5). Diffusion tensor imaging (DTI) offers a unique opportunity to investigate the white matter structures non-invasively in vivo (6).
In order to detect diffusion within tissues, magnetic field gradients are used to create an image that is sensitized to diffusion in a particular direction, and thus, diffusion can be measured by estimating a three-dimensional diffusion model or tensor (7). This tensor is characterized by three orthogonal vectors. The average diffusivity of all three vectors in DTI represents the mean diffusivity (MD) value (8). The MD can be used to measure the microstructural properties of the gray and the white matter and is dependent on the amount of extracellular water (7).
A preferential diffusion in a particular direction is called anisotropic diffusion. White matter is organized in tracts that consist of axonal bundles. The cellular membranes of these axons with some contributions from the myelination and the packing of the axons give a preferential direction of diffusion along the orientation of the axons leading to an anisotropic diffusion within the white matter (7). The most widely used metric for assessing anisotropy is fractional anisotropy (FA), and it is often considered a measure of white matter integrity (7). In cases of compromised white matter integrity, such as demyelination or axonal loss, reduced FA and increased MD values are expected (Figure 1).
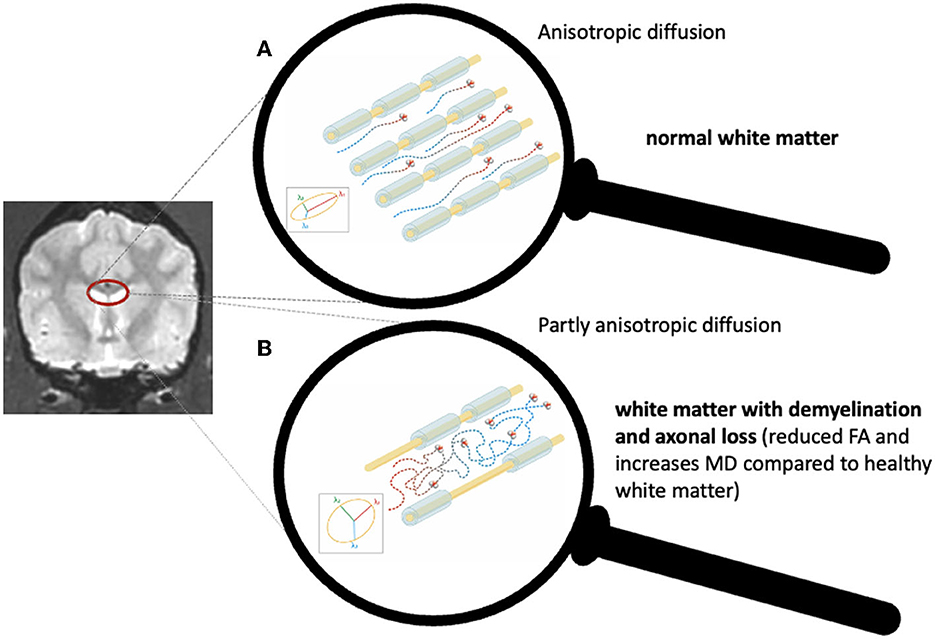
Figure 1. Schematic representation of normal water molecule diffusion along the direction of intact axonal bundles (A) and reduced anisotropy caused by axonal loss and demyelination (B).
In 2020, the ENIGMA Epilepsy study investigated DTI data of 1,249 human patients affected by a variety of epilepsy syndromes, including temporal lobe epilepsy, genetic generalized epilepsy, and non-lesional extratemporal epilepsy (5). Across all these epilepsy syndromes, the FA was lower in most fiber tracts. This effect was most prominent in the corpus callosum, cingulum, and external capsule. The reduction in FA was accompanied by a less robust increase in MD (5).
So far, a single study has been performed in veterinary medicine to investigate diffusion changes in dogs with idiopathic epilepsy. This study focused on apparent diffusion coefficient (ADC) metrics, a technique that describes the overall diffusion within a voxel but lacks a tensor imaging technique (9). Although DTI of the canine brain has been used to detect age-related changes (10), to detect differences between humans and dogs in Krabbe's disease (11), and to investigate the white matter in a compulsive behavioral disorder in dogs (12), the involvement of microstructural white matter changes in canine idiopathic epilepsy remains an unexplored field. Characterizing microstructural white matter changes, which are otherwise undetected in conventional MRI, could release the potential of establishing prognostic non-invasive biomarkers or objective quantitative monitoring parameters for the brain tissue in patients treated with novel treatment strategies such as epilepsy surgery or deep brain stimulation (13, 14).
The study aimed to investigate white matter diffusion changes in dogs affected by idiopathic epilepsy with generalized tonic-clonic seizures. We hypothesized that dogs with idiopathic epilepsy would have lower FA and higher MD in several white matter tracts compared to healthy controls and that this effect would be most pronounced in the corpus callosum and cingulate white matter.
2 Methods
2.1 Animals
Border Collies and Greater Swiss Mountain dogs diagnosed with idiopathic epilepsy according to the veterinary epilepsy task force criteria (15) and healthy controls of the same breeds were prospectively enrolled in this study during a period of 5 years (2017–2022). Additionally, 11 research Beagles were included in the healthy control group. These Beagle dogs have been part of a preliminary study investigating the feasibility of resting state network detection under general anesthesia (16). Functional MRI and magnetic resonance spectroscopy data from the same scan of most of the Border Collies and Greater Swiss Mountain dogs with idiopathic epilepsy and healthy controls have also been analyzed in previous studies (17–19). This study was approved by the Swiss Federal Veterinary Office Zurich (animal license numbers ZH272/16 and ZH046/20). The authors complied with the Animal Research: Reporting of In Vivo (ARRIVE Experiments) guidelines.
A physical and neurological examination was conducted by a board-certified veterinary neurologist in all dogs. Minimum database information was collected as recommended by the international veterinary epilepsy task force (IVETF) for investigations of idiopathic epilepsy, including a seizure history and family seizure history for idiopathic epilepsy, complete blood cell count, and serum biochemistry panel, electrolytes as well as fasted ammonia, bile acids, and urinalysis (1). Information on seizure semiology was collected from all owners in a personal interview based on the questionnaire recommended by the IVETF (1, 15).
Border Collies and Greater Swiss Mountain dogs with idiopathic epilepsy were included only, if they fulfilled the IVETF Tier II criteria and suffered from generalized tonic-clonic seizures (1). Healthy controls were included if they had no history of seizures.
Exclusion criteria were age younger than 1 year [to exclude the influence of incomplete maturation (20)] and older than 10 years [to exclude changes from aging (10)], DTI of insufficient quality, abnormal clinical or neurological examination, abnormal cerebrospinal fluid analyses, and identification of an underlying cause for the epilepsy or structural brain lesion on MRI.
Dogs were divided into two groups: the healthy control group and the idiopathic epilepsy group.
For the region of interest (ROI) analysis, the healthy control group was further subdivided into a healthy control group with a first-degree relative with idiopathic epilepsy and a healthy control group with no family history of idiopathic epilepsy.
2.2 Imaging acquisition
All dogs underwent an MRI scan of the brain with a 3 Tesla MRI (Philips Ingenia scanner, Philips AG, Zurich, Switzerland) and a 16-channel receive–transmit head coil (dStream HeadSpine coil solution, Philips AG, Zurich, Switzerland) under general anesthesia with a standardized anesthetic protocol (16).
Conventional morphological MR images included T2-weighted (T2W) turbo spin-echo sequences in transverse, dorsal, and sagittal planes, a transverse fluid-attenuated inversion recovery (FLAIR), a transverse T2* or a susceptibility-weighted sequence, and a 3D T1W gradient echo sequence.
In the dogs with idiopathic epilepsy, the 3D T1W sequence was repeated after intravenous injection of contrast media [Gadodiamide (Omniscan) GE HealthcareAG, Glattbrugg, Switzerland, or Gadoteric-acid (Dotarem), Guerbet AG, Zürich, Switzerland].
The echo-planar DTI sequence was performed in a transverse plane (TR 8,191 ms; TE 81 ms) with 32 diffusion directions (single low b value = 0 s/mm2; maximal b value = 800 s/mm2; isometric voxel size of 1.5 mm, in-plan field of view of 160 × 160 mm, and acquisition matrix of 108 × 105).
2.3 Postprocessing
DICOM images were converted to 4D NIFTI format using dcm2niix (University of South Carolina, South Carolina, USA). Further processing was performed using FSL (FMRIB Software Library v6.0.5.1, Oxford, UK).
Using FSL commands, data were corrected for eddy current and motion distortion, and an automated mask was used to remove extraneural tissues. Then, a diffusion tensor model was fitted to the processed images using the FSL “dtifit” command, which provides a matrix-valued tensor for each voxel. Diffusion tensor maps for each diffusivity parameter were generated for each subject and visually inspected to ensure the quality of the preprocessing, volume registration, and orientation.
2.4 Tract-based spatial statistics
A modified tract-based spatial statistical (TBSS) analysis (21, 22) adapted to dogs (10) was conducted. As previously described by Barry et al. (10), the subjects' FA images were processed according to the human TBSS pipeline until step three (tbss_3_postreg).
Each subject's FA image was registered using a non-linear transformation to the target FA image (the most representative subject identified in step three of the human TBSS pipeline) with FNIRT (23). A mean FA image was created by concatenating the target space FA images into a single 4D file that was then averaged using fslmaths. A mean FA skeleton was created by thresholding the mean FA at a lower threshold of 0.2 and an upper threshold of 0.8. This thresholded FA skeleton was then binarized to create an FA skeleton mask. The skeleton mask was then applied to a 4D FA file to create a 4D FA skeleton image. MD was processed according to the same steps outlined above and extracted using the FA skeleton. FA and MD values at the location of the FA skeleton mask were then exported for statistical analysis.
2.5 Region of interest analysis
ROI analysis was performed for two regions compromised across all epilepsy syndromes in humans, the corpus callosum and the cingulate white matter (5). The ROIs were selected in the mean FA skeleton mask overlaid with a T1W image of the target dog allowing visualization of the corpus callosum and cingulate white matter. The created mask included the cingulate region bilaterally (Supplementary Figure 1). The corpus callosum mask was created following Barry et al. (10), but with all regions of the corpus callosum in a single mask (Supplementary Figure 2). Using fslstats, these ROIs were then applied to the 4D FA skeleton and to the 4D MD skeleton image (24).
2.6 Statistics
For a voxel-based TBSS analysis, permutation testing using FSL's randomize tool was used to conduct an independent t-test to evaluate differences in diffusion metrics between the idiopathic epilepsy group and the healthy control group using both threshold-free cluster enhancement and family-wise error correction to control for multiple comparisons (25–27).
For the ROI analysis, statistics were performed using R (2023.06.0 in RStudio) (28). In the first step, a Kruskal–Wallis test was performed to investigate differences in FA and MD values in the corpus callosum ROI and the cingulate ROI for two distinct groups: the idiopathic epilepsy group and the healthy control group. In a subsequent step, a Kruskal–Wallis test was conducted to examine the differences in FA and MD values in the corpus callosum ROI and the cingulate ROI across three distinct groups: the idiopathic epilepsy group, the healthy control group with a first-degree relative with idiopathic epilepsy, and the healthy control group with no family history of idiopathic epilepsy. Post-hoc pairwise comparisons among the groups were carried out using the Dunn test. To mitigate the issue of multiple comparisons, the Bonferroni correction was applied to adjust the p-values. In the event of statistical significance, an effect size (r) was computed to quantify the magnitude of differences observed between the group means. Overall, p < 0.05 was considered statistically significant.
3 Results
3.1 Study population
A total of 59 dogs were prospectively enrolled. Nine dogs were excluded because of insufficient imaging quality. A total of 50 dogs were included in the data analysis. Population characteristics are given in Table 1. In the healthy control group, all Greater Swiss Mountain dogs, and all but one Border Collie had a first-degree relative with idiopathic epilepsy. Information regarding the seizure semiology is reported in Table 2. Unfortunately, in cases with focal onset, the owners could not reliably report the side of the focal onset in most cases.
3.2 Voxel-based analysis with TBSS
No significant differences in FA and MD were identified between the idiopathic epilepsy group and the healthy control group using TBSS. The results from TBSS are displayed in Figure 2.
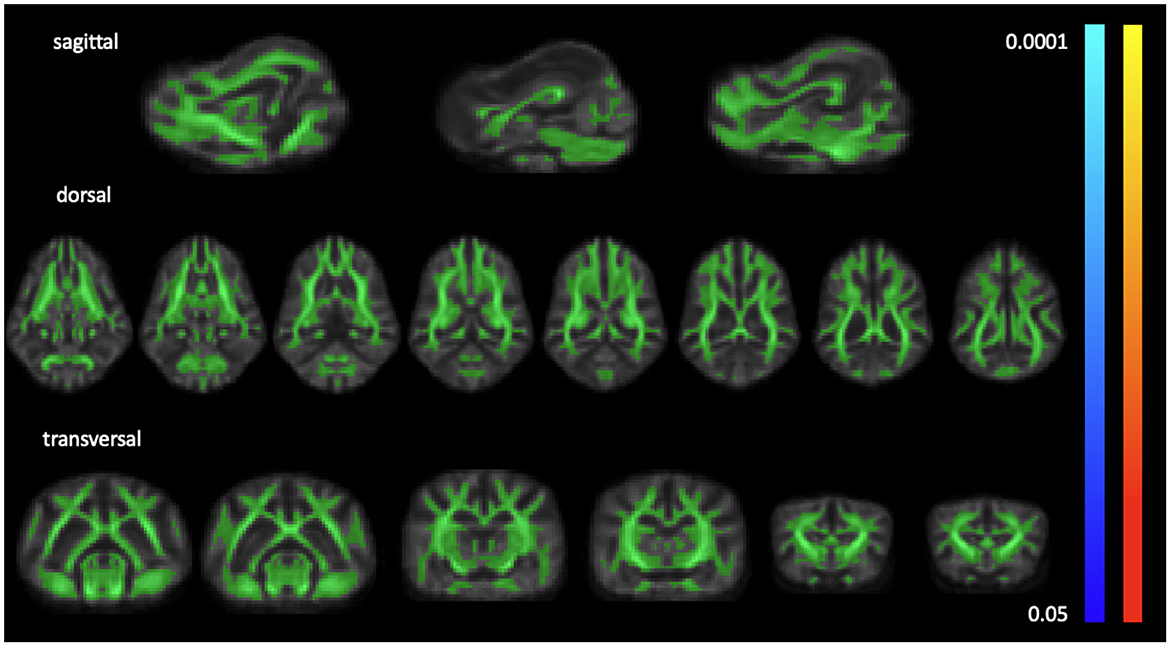
Figure 2. Light green overlay of the average white matter mask over sample FA template slices in sagittal, dorsal, and transverse planes. TBSS detected no significant differences from p < 0.05 to p < 0.0001 for FA (coolmap) and MD (heatmap).
3.3 ROI analysis
The ROI analysis showed a significant difference in the FA of the cingulate white matter in the idiopathic epilepsy group compared to the healthy control group (p = 0.027) with lower FA in the idiopathic epilepsy group. The effect size was moderate (r = −0.313). No significant differences were found in the FA of the corpus callosum and MD of the corpus callosum and the cingulate white matter between the idiopathic epilepsy group and the healthy control group. The results are displayed in Figure 3.
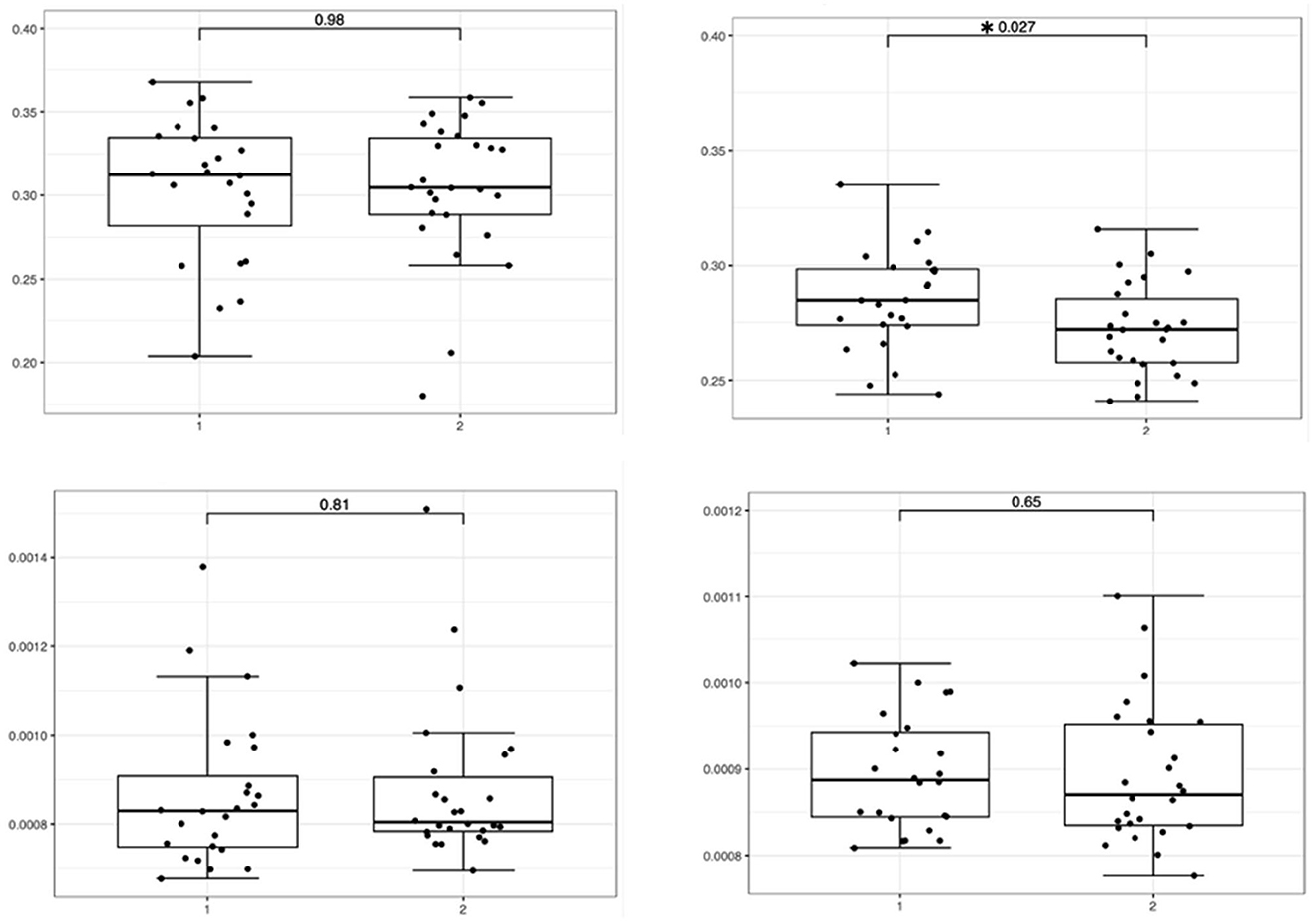
Figure 3. Boxplots featuring bilateral FA and MD differences in the corpus callosum and cingulate white matter with significance levels highlighted with an asterisk featuring the relevant p-value. The boxes on the left side represent the healthy controls (1), and the boxes on the right side (2) represent dogs with idiopathic epilepsy.
The Kruskal–Wallis test of FA and MD values of the corpus callosum ROI and the cingulate ROI across the three distinct groups revealed a significant difference in the cingulate FA and MD values (p = 0.012 and p = 0.001) but not in the callosal FA and MD values (p = 0.644 and p = 0.122).
For FA of the cingulate white matter, a pairwise comparison of the healthy control group with no familiar history of idiopathic epilepsy and the idiopathic epilepsy group showed a significant difference (p = 0.009) with a lower FA in the idiopathic epilepsy group (effect sizes r = −0.48).
For MD of the cingulate white matter, a pairwise comparison of the healthy control group with no familiar history of idiopathic epilepsy and the healthy control group with a first-degree relative with idiopathic epilepsy showed significant difference (p = 0.0007) with a large effect size (r = −0.748) as well as a significance between the healthy control group with a first-degree relative with idiopathic epilepsy and idiopathic epilepsy group (p = 0.036) with a moderate effect size (r = −0.408). The results are displayed in Figure 4.
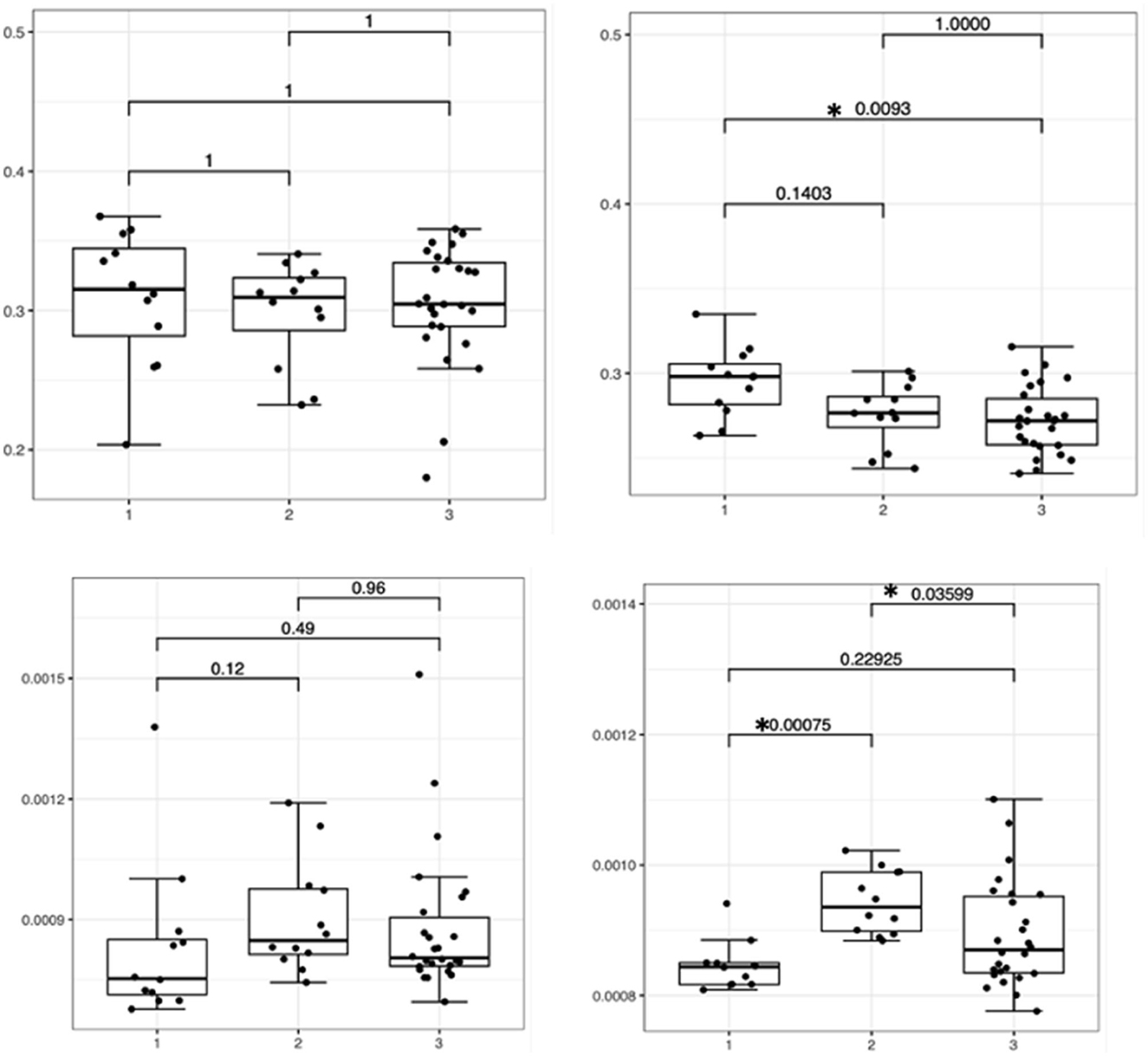
Figure 4. Boxplots featuring bilateral FA and MD differences in the corpus callosum and cingulate white matter with significance levels highlighted with an asterisk featuring the relevant p-value. Boxes on the left side indicate healthy controls with no familiar history of epilepsy (1), boxes in the middle indicate healthy controls with a first-degree relative with idiopathic epilepsy (2), and boxes on the right side indicate dogs with idiopathic epilepsy (3).
4 Discussion
In this single-center prospective study, we investigated white matter integrity in Border Collies and Greater Swiss Mountain dogs with idiopathic epilepsy. We hypothesized that we would find impaired white matter integrity in dogs with idiopathic epilepsy compared to healthy controls manifesting as a decrease in FA and an increase in MD. While a voxel-based analysis (TBSS) did not detect any significant differences between the idiopathic epilepsy group and the healthy control group, the ROI analysis of the corpus callosum and cingulate white matter showed significant differences in the FA in the cingulate white matter between the idiopathic epilepsy group and the healthy control group only, with lower FA values in the idiopathic epilepsy group.
Note that in general voxel-based analysis and ROI analysis in DTI do correlate well, but they do not always provide the same results (29). Automated voxel-based analysis offers the advantage that no prior assumption needs to be performed and the whole brain can be analyzed. However, in our study, the whole brain TBSS includes all 73,713 voxels in the analysis. The analysis is performed with a multiple comparison correction to prevent false-positive results. If the effect is very subtle, these multiple comparison corrections may mask small changes. In contrast, the ROI analysis first combines the signal across multiple voxels within the ROI (645 voxels for the cingulate gyrus ROI and 316 voxels for the corpus callosum ROI) leading to an increased signal-to-noise ratio. Second, as only two analyses are performed in the ROI analysis, the effect of multiple comparisons correction is minimal. Furthermore, voxel-based analysis heavily depends on the exact anatomical registration of each voxel to the study template, and the more diverse shape of the canine brain and a not as well validated processing pipeline might have had an influence on the voxel registration and therefore on the voxel-based analysis (29).
In contrast to canine epilepsy, plenty of DTI studies have been performed in human epilepsy and microstructural compromises of the white matter have been identified in a wide variety of epilepsy syndromes using DTI (5). Decreased FA and increased MD were seen across all epilepsy syndromes in a variety of white matter structures including the corpus callosum and cingulum (5).
While this effect has been very clearly identified in the large-scale ENIGMA human epilepsy study, including more than 1,000 individuals, the results from smaller studies with fewer patients have been less conclusive (5, 30, 31). In smaller studies like ours, with smaller sample sizes, it is more difficult to account for a heterogenic study population and it is more difficult to compensate for additional contributing factors, such as lateralization of the diseases, age, or gender. For example, we did not have information regarding lateralization of the seizure signs in most dogs included in the study, and gender and age were not matched between the idiopathic epilepsy group and the healthy control groups. Furthermore, a stringent classification of epilepsy into a subcategory was lacking in our canine patients. All these factors might have led to the detection of reduced FA in the cingulate white matter only.
In humans, the DTI changes were most pronounced in ipsilateral to mesial temporal lesions and the changes were less pronounced in non-lesional epilepsy (5). We included dogs with generalized epileptic seizures and no visible lesions on MRI, where the effect might be less pronounced compared to focal lesional epilepsy syndromes in humans.
Furthermore, most dogs included in our study were newly diagnosed. In humans, it has been speculated that the white matter changes represent secondary effects, rather than being causal, but large longitudinal studies are lacking (5). Therefore, enrolling dogs with idiopathic epilepsy at the first stages of clinical manifestation of the disease might have had an impact on the ability to detect white matter microstructural abnormalities, and eventually, long-term follow-up examinations could lead to different results.
Previously, inter-ictal brain diffusion in canine idiopathic epilepsy has been investigated with simple diffusion-weighted imaging and a ROI-based approach only. Gray and white matter ROIs have been investigated, but neither the corpus callosum nor the cingulate white matter were included (9). Increased ADC values in dogs with idiopathic epilepsy have been identified in the semioval center and the piriform lobe in this study (9). In contrast to this former study, with our ROI-based approach, we have focused on the white matter structures most commonly involved in human epilepsy (corpus callosum and cingulate white matter). Additionally, we have performed a voxel-based whole-brain approach, which did not depict increased MD in the semioval center or in the piriform lobe.
A more recent retrospective multicentric study focused on peri-ictal imaging findings and found mixed ADC results, including normal, increased, and decreased diffusivity in affected areas (32). The cingulate gyrus was affected in 6/19 cases and had either decreased ADC values in the cortex and increased ADC values in the white matter, or increased ADC values in the white matter only (32). The cingulate gyrus was commonly affected by peri-ictal imaging changes, which supports the cingulate gyrus as a target area in dogs with idiopathic epilepsy. However, in our study, we investigated MD as a measure for overall diffusion and could not detect any significant difference in the MD, but significantly reduced FA in dogs with idiopathic epilepsy compared to healthy controls in the cingulate white matter. While both studies suggest the cingulate white matter as a target structure in canine idiopathic epilepsy, the microstructural changes might differ inter-ictally and peri-ictally and longitudinal studies including peri-ictal and inter-ictal time points could give insights into the time course of such changes.
The pathophysiology of white matter changes and its correlation with DTI changes in epilepsy is not well understood. It is still unknown whether the identified white matter abnormalities predate the development of epilepsy or whether they are a secondary effect of ongoing seizures (5). No histological examination was performed to look for a correlation between the reduced FA and possible histological changes in the brains of the dogs with idiopathic epilepsy included in our study. However, various mechanisms have been suggested, including changes related to the underlying epileptogenic process, axonal degeneration, and compensatory white matter reorganization (31, 33). Correlation between histopathological changes and DTI changes in epilepsy is sparse and is derived from rat models and surgically removed brain tissue in humans (34, 35). In the rat model of status epilepticus, myelin staining was reduced in the fimbria of the fornix in correlation with reduced FA 8 weeks after induced status epilepticus (34). In humans with temporal lobe epilepsy, increased extra-axonal fraction, and reduced cumulative axonal membrane circumference and myelin area were found in the surgically extracted fimbria of the fornix (35). Studies investigating histopathological changes in canine epilepsy are sparse (36). In contrast to humans, there is less evidence of temporal lobe involvement in dogs (36). Similar to humans with epilepsy originating from the limbic system, neuronal loss and gliosis were found in the limbic system, including the cingulate gyrus, amygdaloid nucleus, dorsal and ventral parts of the hippocampus, and dorsomedial nucleus of the thalamus in an epileptic Shetland Sheepdog family (37, 38). Involvement of the cingulate cortex would make an effect on the cingulate white matter a reasonable possibility. In contrast, Buckmaster et al. failed to identify any neuropathological changes in the temporal lobe of dogs with medically intractable epilepsy (39). In addition, guidelines for pathological examination of epileptic canine brains have been redefined (40); large studies reporting pathological abnormalities are still lacking.
We investigated idiopathic epilepsy in Border Collies and Greater Swiss Mountain dogs, two dog breeds with a suspected genetic background for epilepsy (41–43). Over the last decade, idiopathic epilepsy with a proven or suspected genetic background has been reported for a number of dog breeds with most studies focusing on clinical characteristics and genetic aspects. Nevertheless, most studies have not yet identified causative gene mutations, suggesting that inheritance may be complex (44). We have chosen Border Collies and Greater Swiss Mountain dogs because of their severe form of epilepsy and because these are commonly affected breeds in the author's institution. Choosing dog breeds with well-known epilepsy offered the advantage of more narrowly defined, more similar seizure phenotypes. Furthermore, the true prevalence of epilepsy in these breeds is currently unknown, but based on the literature, it is assumed that it is rather high (42–44). In pursuit of generating comparable data from groups of animals as homogeneous as possible, healthy first-degree relatives from the affected dogs with idiopathic epilepsy were recruited. What seemed to be an advantage when we designed the study might have had a negative impact on our results. Of the healthy Border Collies and Greater Swiss Mountain dogs included in our study, all but one had a first-degree relative with idiopathic epilepsy. In humans, heritability of the white matter microstructure has been demonstrated (45, 46), and in patients with MRI-negative temporal lobe epilepsy, an increase in MD has been identified even in asymptomatic siblings (47). Increased MD was also found in the healthy control dogs with a first-degree relative with idiopathic epilepsy compared to healthy controls without a first-degree relative with idiopathic epilepsy (Figure 4). It is therefore possible that in close relatives of dogs with suspected genetic idiopathic epilepsy, white matter could be compromised even in unaffected animals. Unfortunately, all but one healthy control dog without a first-degree relative with idiopathic epilepsy were from a different breed (Beagle dogs), and to test the concept of hereditary white matter changes, we would have needed more dogs of the same breed without a family history of epilepsy. Furthermore, the high number of close relatives included in our study may have hidden differences in MD between dogs with idiopathic epilepsy and healthy controls because of heritable white matter changes. Such heritable white matter changes could even turn out to be diffusion-based endophenotypes for epilepsy in dogs. Such diffusion-based endophenotypes might support an imaging-based diagnosis for genetic epilepsy in the future (47).
4.1 Limitations
One of the strongest limitations of our study is the heterogenicity of the study population. We were not able to recruit a control group with the same age and gender profile as the idiopathic epilepsy group. The median age in the control group was almost twice as high as in the idiopathic epilepsy group. Barry et al. have shown significant differences in FA and MD between dogs of <7 years and dogs of >10 years of age (10). We did not include dogs older than 10 years of age, but we cannot rule out that the different ages in both groups had an influence on the results. The gender ratio was reversed between the two groups. The idiopathic epilepsy group included mainly male dogs, while the control group included more females than males. The influence of sex on the microstructure has been proven in humans; for example, one study has shown increased FA in male participants compared to female participants in all subregions of the corpus callosum (48). Such gender-related differences could mask differences due to illness as there was a higher percentage of male dogs in the idiopathic epilepsy group compared to the control group.
We also have different breed distributions in both groups, but to date, it is not known whether there is any regional difference between FA and MD in different dog breeds.
In humans, countless DTI studies have been conducted over the last decade, and post-processing has evolved massively. Well-tested and adapted pipelines exist. In contrast, in veterinary medicine, an adapted version of the TBSS pipeline has just recently been published (10), and although we followed this pipeline in the main, we had to do without the distortion correction due to our imaging acquisition. This might be of even more compromise in dogs than in humans. Dogs have larger frontal sinuses compared to humans, which can cause problems in susceptibility-sensitive sequences (49). In DTI post-processing, it is possible to account for these susceptibility-induced field distortions (50, 51). Unfortunately, the data in our study were acquired with one B0 image only, and at least two B0 images with opposite phase encoding directions are needed for field estimation (50). Therefore, no correction for susceptibility-induced distortions was performed, and diffusion images were displayed with geometric mismatches compared to the structural images. We cannot rule out that the different degrees of distortion in the individual images potentially influenced our results.
5 Conclusion
We aimed to investigate white matter diffusion changes in dogs affected by idiopathic epilepsy with generalized tonic-clonic seizures. We observed subtle changes in DTI between dogs with idiopathic epilepsy and healthy controls limited to cingulate white matter, with a significantly lower FA in dogs with idiopathic epilepsy compared to healthy controls using a ROI approach. No significant changes were found between dogs with idiopathic epilepsy and healthy controls in the TBSS analysis and in the corpus callosum in the ROI approach between both groups. This study supports the cingulate area as a target structure in canine epilepsy. The subtle changes only might be explained by the small sample size and the higher variability in canine brain anatomy. Furthermore, all included dogs showed generalized tonic-clonic seizures, possibly suffering from generalized epilepsy syndrome, which is also associated with less pronounced DTI changes in humans than focal epilepsy syndromes.
Data availability statement
The raw data supporting the conclusions of this article will be made available by the authors, without undue reservation.
Ethics statement
The animal studies were approved by Swiss Federal Veterinary Office Zurich. The studies were conducted in accordance with the local legislation and institutional requirements. Written informed consent was obtained from the owners for the participation of their animals in this study.
Author contributions
KB: Conceptualization, Data curation, Formal analysis, Funding acquisition, Investigation, Methodology, Project administration, Resources, Validation, Visualization, Writing—original draft, Writing—review & editing. AW-L: Methodology, Writing—review & editing, Writing—original draft. FS: Writing—review & editing. HR: Formal analysis, Project administration, Writing—review & editing. MD: Writing—review & editing. RB: Writing—review & editing. IC: Conceptualization, Methodology, Writing—review & editing. SH: Conceptualization, Investigation, Methodology, Supervision, Writing—review & editing.
Funding
The author(s) declare financial support was received for the research, authorship, and/or publication of this article. This research was partially financially supported by the Albert-Heim-Stiftung and the Stiftung für Kleintiere der Vetsuisse-Fakultät Universität Zürich.
Acknowledgments
Preliminary data of these studies were presented at the 35th ECVN Symposium 2023 in Venice by KB. We are grateful for the support of our colleagues from the Department of Neurology and the Clinic for Diagnostic Imaging from the Vetsuisse Faculty Zurich. We also must thank Manuela Wieser (Section of Anesthesiology, Vetsuisse Faculty Zurich) for her assistance in the conduction of the study. We would also like to thank Jeanne Peter (Vetcom team, Vetsuisse Faculty, University of Zurich) for creating Figure 1.
Conflict of interest
IC was employed at Vet Oracle Teleradiology.
The remaining authors declare that the research was conducted in the absence of any commercial or financial relationships that could be construed as a potential conflict of interest.
The author(s) declared that they were an editorial board member of Frontiers, at the time of submission. This had no impact on the peer review process and the final decision.
Publisher's note
All claims expressed in this article are solely those of the authors and do not necessarily represent those of their affiliated organizations, or those of the publisher, the editors and the reviewers. Any product that may be evaluated in this article, or claim that may be made by its manufacturer, is not guaranteed or endorsed by the publisher.
Supplementary material
The Supplementary Material for this article can be found online at: https://www.frontiersin.org/articles/10.3389/fvets.2023.1325521/full#supplementary-material
Supplementary Figure 1. Sagittal, dorsal, and transverse views of the delineation of the cingulate ROI (blue) overlayed on the average white matter mask (green) over sample FA template.
Supplementary Figure 2. Sagittal, dorsal, and transverse views of the delineation of the corpus callosum ROI (purple) overlayed on the average white matter mask (green) over sample FA template.
Abbreviations
ADC, apparent diffusion coefficient; DTI, diffusion tensor imaging; FA, fractional anisotropy; IVETF, international veterinary epilepsy task force; MD, mean diffusivity; MRI, magnetic resonance imaging; ROI, region of interest; TBSS, tract-based special statistics.
References
1. De Risio L, Bhatti S, Munana K, Penderis J, Stein V, Tipold A, et al. International veterinary epilepsy task force consensus proposal: diagnostic approach to epilepsy in dogs. BMC Vet Res. (2015) 11:148. doi: 10.1186/s12917-015-0462-1
2. Rusbridge C, Long S, Jovanovik J, Milne M, Berendt M, Bhatti SF, et al. International Veterinary Epilepsy Task Force recommendations for a veterinary epilepsy-specific MRI protocol. BMC Vet Res. (2015) 11:194. doi: 10.1186/s12917-015-0466-x
3. Kramer MA, Cash SS. Epilepsy as a disorder of cortical network organization. Neuroscientist. (2012) 18:360–72. doi: 10.1177/1073858411422754
4. Berg AT, Berkovic SF, Brodie MJ, Buchhalter J, Cross JH, van Emde Boas W, et al. Revised terminology and concepts for organization of seizures and epilepsies: report of the ILAE Commission on Classification and Terminology, 2005-2009. Epilepsia. (2010) 51:676–85. doi: 10.1111/j.1528-1167.2010.02522.x
5. Hatton SN, Huynh KH, Bonilha L, Abela E, Alhusaini S, Altmann A, et al. White matter abnormalities across different epilepsy syndromes in adults: an ENIGMA-Epilepsy study. Brain. (2020) 143:2454–73. doi: 10.1093/brain/awaa200
6. Beaulieu C. The basis of anisotropic water diffusion in the nervous system - a technical review. NMR Biomed. (2002) 15:435–55. doi: 10.1002/nbm.782
7. O'Donnell LJ, Westin C-F. An introduction to diffusion tensor image analysis. Neurosurg Clin N Am. (2011) 22:185–96. doi: 10.1016/j.nec.2010.12.004
8. Pierpaoli C, Jezzard P, Basser PJ, Barnett A, Di Chiro G. Diffusion tensor MR imaging of the human brain. Radiology. (1996) 201:637–48. doi: 10.1148/radiology.201.3.8939209
9. Hartmann A, Sager S, Failing K, Sparenberg M, Schmidt MJ. Diffusion-weighted imaging of the brains of dogs with idiopathic epilepsy. BMC Vet Res. (2017) 13:338. doi: 10.1186/s12917-017-1268-0
10. Barry EF, Loftus JP, Luh WM, de Leon MJ, Niogi SN, Johnson PJ. Diffusion tensor-based analysis of white matter in the healthy aging canine brain. Neurobiol Aging. (2021) 105:129–36. doi: 10.1016/j.neurobiolaging.2021.04.021
11. Li JY, Middleton DM, Chen S, White L, Corado CR, Vite C, et al. Quantitative DTI metrics in a canine model of Krabbe disease: comparisons versus age-matched controls across multiple ages. Neuroradiol J. (2018) 31:168–76. doi: 10.1177/1971400917733431
12. Ogata N, Gillis TE, Liu X, Cunningham SM, Lowen SB, Adams BL, et al. Brain structural abnormalities in Doberman pinschers with canine compulsive disorder. Prog Neuropsychopharmacol Biol Psychiatry. (2013) 45:1–6. doi: 10.1016/j.pnpbp.2013.04.002
13. Hasegawa D, Saito M, Kitagawa M. Neurosurgery in canine epilepsy. Vet J. (2022) 285:105852. doi: 10.1016/j.tvjl.2022.105852
14. Nowakowska M, Üçal M, Charalambous M, Bhatti SFM, Denison T, Meller S, et al. Neurostimulation as a method of treatment and a preventive measure in canine drug-resistant Epilepsy: current state and future prospects. Front Veter Sci. (2022) 9:889561. doi: 10.3389/fvets.2022.889561
15. Berendt M, Farquhar RG, Mandigers PJ, Pakozdy A, Bhatti SF, De Risio L, et al. International veterinary epilepsy task force consensus report on epilepsy definition, classification and terminology in companion animals. BMC Vet Res. (2015) 11:182. doi: 10.1186/s12917-015-0461-2
16. Beckmann KM, Wang-Leandro A, Dennler M, Carrera I, Richter H, Bektas RN, et al. Resting state networks of the canine brain under sevoflurane anaesthesia. PLoS ONE. (2020) 15:e0231955. doi: 10.1371/journal.pone.0231955
17. Beckmann KM, Wang-Leandro A, Richter H, Bektas RN, Steffen F, Dennler M, et al. Increased resting state connectivity in the anterior default mode network of idiopathic epileptic dogs. Sci Rep. (2021) 11:23854. doi: 10.1038/s41598-021-03349-x
18. Mauri N, Richter H, Steffen F, Zölch N, Beckmann KM. Single-voxel proton magnetic resonance spectroscopy of the thalamus in idiopathic epileptic dogs and in healthy control dogs. Front Vet Sci. (2022) 9:885044. doi: 10.3389/fvets.2022.885044
19. Wieser M, Beckmann KM, Kutter APN, Mauri N, Richter H, Zölch N, et al. Ketamine administration in idiopathic epileptic and healthy control dogs: can we detect differences in brain metabolite response with spectroscopy? Front Veter Sci. (2023) 9:1093267. doi: 10.3389/fvets.2022.1093267
20. Saksena S, Husain N, Malik GK, Trivedi R, Sarma M, Rathore RS, et al. Comparative evaluation of the cerebral and cerebellar white matter development in pediatric age group using quantitative diffusion tensor imaging. Cerebellum. (2008) 7:392–400. doi: 10.1007/s12311-008-0041-0
21. Jbabdi S, Behrens TEJ, Smith SM. Crossing fibres in tract-based spatial statistics. Neuroimage. (2010) 49:249–56. doi: 10.1016/j.neuroimage.2009.08.039
22. Smith SM, Jenkinson M, Johansen-Berg H, Rueckert D, Nichols TE, Mackay CE, et al. Tract-based spatial statistics: voxelwise analysis of multi-subject diffusion data. Neuroimage. (2006) 31:1487–505. doi: 10.1016/j.neuroimage.2006.02.024
23. Andersson JLR, Jenkinson M, Smith S. Non-linear registration, aka Spatial normalisation. In: FMRIB technical report TR07JA2 (2007).
24. Jenkinson M, Beckmann CF, Behrens TE, Woolrich MW, Smith SM. Fsl. Neuroimage. (2012) 62:782–90. doi: 10.1016/j.neuroimage.2011.09.015
25. Salimi-Khorshidi G, Smith SM, Nichols TE. Adjusting the effect of nonstationarity in cluster-based and TFCE inference. Neuroimage. (2011) 54:2006–19. doi: 10.1016/j.neuroimage.2010.09.088
26. Smith SM, Nichols TE. Threshold-free cluster enhancement: addressing problems of smoothing, threshold dependence and localisation in cluster inference. Neuroimage. (2009) 44:83–98. doi: 10.1016/j.neuroimage.2008.03.061
27. Winkler AM, Ridgway GR, Webster MA, Smith SM, Nichols TE. Permutation inference for the general linear model. Neuroimage. (2014) 92:381–97. doi: 10.1016/j.neuroimage.2014.01.060
28. A Language and Environment for Statistical Computing. R Foundation for Statistical Computing Available online at: https://www.R-project.org/ (accessed July 20, 2023).
29. Snook L, Plewes C, Beaulieu C. Voxel based versus region of interest analysis in diffusion tensor imaging of neurodevelopment. Neuroimage. (2007) 34:243–52. doi: 10.1016/j.neuroimage.2006.07.021
30. Slinger G, Sinke MR, Braun KP, Otte WM. White matter abnormalities at a regional and voxel level in focal and generalized epilepsy: a systematic review and meta-analysis. Neuroimage Clin. (2016) 12:902–9. doi: 10.1016/j.nicl.2016.10.025
31. Otte WM, van Eijsden P, Sander JW, Duncan JS, Dijkhuizen RM, Braun KP, et al. A meta-analysis of white matter changes in temporal lobe epilepsy as studied with diffusion tensor imaging. Epilepsia. (2012) 53:659–67. doi: 10.1111/j.1528-1167.2012.03426.x
32. Nagendran A, McConnell JF, De Risio L, José-López R, Quintana RG, Robinson K, et al. Peri-ictal magnetic resonance imaging characteristics in dogs with suspected idiopathic epilepsy. J Veter Internal Med. (2021) 35:1008–17. doi: 10.1111/jvim.16058
33. Gross DW, Concha L, Beaulieu C. Extratemporal white matter abnormalities in mesial temporal lobe epilepsy demonstrated with diffusion tensor imaging. Epilepsia. (2006) 47:1360–3. doi: 10.1111/j.1528-1167.2006.00603.x
34. van Eijsden P, Otte WM, van der Hel WS, van Nieuwenhuizen O, Dijkhuizen RM, de Graaf RA, et al. In vivo diffusion tensor imaging and ex vivo histologic characterization of white matter pathology in a post-status epilepticus model of temporal lobe epilepsy. Epilepsia. (2011) 52:841–5. doi: 10.1111/j.1528-1167.2011.02991.x
35. Concha L, Livy DJ, Beaulieu C, Wheatley BM, Gross DW. Diffusion tensor imaging and histopathology of the fimbria-fornix in temporal lobe Epilepsy. J Neurosci. (2010) 30:996–1002. doi: 10.1523/JNEUROSCI.1619-09.2010
36. Löscher W. Dogs as a natural animal model of Epilepsy. Front Veter Sci. (2022) 9:928009. doi: 10.3389/fvets.2022.928009
37. Yamasaki H, Furuoka H, Takechi M, Itakura C. Neuronal loss and gliosis in limbic system in an epileptic dog. Vet Pathol. (1991) 28:540–2. doi: 10.1177/030098589102800614
38. Morita T, Shimada A, Takeuchi T, Hikasa Y, Sawada M, Ohiwa S, et al. Cliniconeuropathologic findings of familial frontal lobe epilepsy in Shetland sheepdogs. Can J Vet Res. (2002) 66:35–41.
39. Buckmaster PS, Smith MO, Buckmaster CL, LeCouteur RA, Dudek FE. Absence of temporal lobe epilepsy pathology in dogs with medically intractable Epilepsy. J Veter Internal Med. (2002) 16:95–9. doi: 10.1111/j.1939-1676.2002.tb01612.x
40. Matiasek K, Pumarola IBM, Rosati M, Fernandez-Flores F, Fischer A, Wagner E, et al. International veterinary epilepsy task force recommendations for systematic sampling and processing of brains from epileptic dogs and cats. BMC Vet Res. (2015) 11:216. doi: 10.1186/s12917-015-0467-9
41. Hulsmeyer V, Zimmermann R, Brauer C, Sauter-Louis C, Fischer A. Epilepsy in Border Collies: clinical manifestation, outcome, and mode of inheritance. J Veter Internal Med. (2010) 24:171–8. doi: 10.1111/j.1939-1676.2009.0438.x
42. Sauer-Delhées S, Steffen F, Reichler I, Beckmann K. Clinical characteristics of Idiopathic Epilepsy in greater Swiss mountain dogs in Switzerland. Schweiz Arch Tierheilkd. (2020) 162:697–706. doi: 10.17236/sat00279
43. Ostermann TE, Nessler JN, Urankar H, Bachmann N, Fechler C, Bathen-Nöthen A, et al. Phenotype of idiopathic epilepsy in great swiss mountain dogs in germany—a retrospective study. Front Veter Sci. (2022) 9:921134. doi: 10.3389/fvets.2022.921134
44. Hulsmeyer VI, Fischer A, Mandigers PJ, DeRisio L, Berendt M, Rusbridge C, et al. International veterinary Epilepsy task force's current understanding of idiopathic epilepsy of genetic or suspected genetic origin in purebred dogs. BMC Vet Res. (2015) 11:175. doi: 10.1186/s12917-015-0463-0
45. Chiang MC, Barysheva M, Shattuck DW, Lee AD, Madsen SK, Avedissian C, et al. Genetics of brain fiber architecture and intellectual performance. J Neurosci. (2009) 29:2212–24. doi: 10.1523/JNEUROSCI.4184-08.2009
46. Kochunov P, Glahn DC, Lancaster JL, Winkler AM, Smith S, Thompson PM, et al. Genetics of microstructure of cerebral white matter using diffusion tensor imaging. Neuroimage. (2010) 53:1109–16. doi: 10.1016/j.neuroimage.2010.01.078
47. Whelan CD, Alhusaini S, O'Hanlon E, Cheung M, Iyer PM, Meaney JF, et al. White matter alterations in patients with MRI-negative temporal lobe epilepsy and their asymptomatic siblings. Epilepsia. (2015) 56:1551–61. doi: 10.1111/epi.13103
48. Menzler K, Belke M, Wehrmann E, Krakow K, Lengler U, Jansen A, et al. Men and women are different: diffusion tensor imaging reveals sexual dimorphism in the microstructure of the thalamus, corpus callosum and cingulum. Neuroimage. (2011) 54:2557–62. doi: 10.1016/j.neuroimage.2010.11.029
49. Wolfer N, Wang-Leandro A, Beckmann KM, Richter H, Dennler M. Intracranial lesion detection and artifact characterization: comparative study of susceptibility and T2(*)-weighted imaging in dogs and cats. Front Veter Sci. (2021) 8:779515. doi: 10.3389/fvets.2021.779515
50. Andersson JLR, Skare S, Ashburner J. How to correct susceptibility distortions in spin-echo echo-planar images: application to diffusion tensor imaging. Neuroimage. (2003) 20:870–88. doi: 10.1016/S1053-8119(03)00336-7
Keywords: TBSS, white matter integrity, seizures, Border Collie, Greater Swiss Mountain dog, MRI, DTI, canine
Citation: Beckmann KM, Wang-Leandro A, Steffen F, Richter H, Dennler M, Bektas R, Carrera I and Haller S (2023) Diffusion tensor-based analysis of white matter in dogs with idiopathic epilepsy. Front. Vet. Sci. 10:1325521. doi: 10.3389/fvets.2023.1325521
Received: 21 October 2023; Accepted: 23 November 2023;
Published: 18 December 2023.
Edited by:
Daisuke Hasegawa, Nippon Veterinary and Life Science University, JapanReviewed by:
Philippa Johnson, Cornell University, United StatesYuji Hamamoto, Rakuno Gakuen University, Japan
Copyright © 2023 Beckmann, Wang-Leandro, Steffen, Richter, Dennler, Bektas, Carrera and Haller. This is an open-access article distributed under the terms of the Creative Commons Attribution License (CC BY). The use, distribution or reproduction in other forums is permitted, provided the original author(s) and the copyright owner(s) are credited and that the original publication in this journal is cited, in accordance with accepted academic practice. No use, distribution or reproduction is permitted which does not comply with these terms.
*Correspondence: Katrin M. Beckmann, a2JlY2ttYW5uQHZldGNsaW5pY3MudXpoLmNo
†These authors have contributed equally to this work and share last authorship