- 1US Army Medical Research Institute of Infectious Diseases, Fort Detrick, MD, United States
- 2Uniformed Services University School of Medicine, Bethesda, MD, United States
- 3Joint Program Executive Office for Chemical, Biological, Radiological and Nuclear Defense, Frederick, MD, United States
- 4Davis Defense Group, Stafford, VA, United States
- 5San Antonio Military Medical Center, San Antonio, TX, United States
- 6University of Florida Research and Academic Center, Orlando, FL, United States
- 7US Army Medical Materiel Development Activity, Fort Detrick, MD, United States
- 8Johns Hopkins University School of Medicine, Baltimore, MD, United States
Background: Tularemia is caused by the intracellular bacterium Francisella tularensis (Ft). It was weaponized historically due to low infectious aerosol dose, high morbidity, and mortality rates for pneumonic disease. The US Army developed the attenuated Live Vaccine Strain (LVS) from stocks provided by the former Soviet Union in the 1950s. The vaccine has been safe and immunogenic over the ensuing decades in multiple clinical trials including human challenge studies.
Methods: Two sequential FDA-regulated, non-randomized, single-arm LVS trials enrolled at-risk laboratory personnel working on tularemia in bio-containment laboratories under IND#157. Volunteers received a single dose of LVS manufactured in 1962 by scarification. Positive immunization was based on local scarification site “take reaction,” and either a >1:20 tularemia antigen microagglutination (MA) titer (protocol FY03-24; 2004-8) or >4-fold rise in MA titer (protocol FY07-15; 2009-2017). Those still negative by week 4 were offered a second dose.
Results: The LVS vaccine was safe, well tolerated, and highly immunogenic. Between the two studies, all recipients (100%) had positive “take reactions,” with 95.5% of those in study FY03-24 having a positive response following initial vaccination. All but three subjects (98%) in protocol FY03-24 had positive MA titer results defined as >1:20, most within 28–35 days. In protocol FY07-15, 95% of subjects had a 4-fold or greater rise in MA titer, the primary immunogenicity endpoint for that study.
Discussion: LVS vaccine administered to laboratory workers at risk for tularemia exposure over 12 years was safe and highly immunogenic. Response rates remained robust despite the vaccine lots employed having been manufactured 42–55 years prior to vaccination. The results and historical comparator data presented here serve as a benchmark for future studies. LVS remains unlicensed due to instability in culture and the potential for reversion to the wild-type pathogen. Despite the threat, there are no FDA-approved vaccines. In the absence of a clinical-stage commercial development effort, an ongoing LVS vaccine protocol under investigational new drug (IND) application for at-risk laboratory workers to prevent occupationally acquired disease should be considered based on extensive favorable data for this vaccine.
Clinical trial registration: ClinicalTrials.gov, identifiers NCT00584844 (trial FY03-24) and NCT00787826 (trial FY07-15).
Introduction
Francisella tularensis infects vertebrate and invertebrate animal hosts causing the disease tularemia (Francis, 1919). Transmission to mammals in nature occurs largely through multiple vectors including ticks, mosquitos, and biting flies, and contact with remains of infected animals. Clinical presentations vary with skin, eye, lymphatic, gastrointestinal, and pharyngeal involvement common following a 3–5-day incubation period (Sanders and Haun, 1968; Bartelloni, 1969). While less commonly fatal, even naturally transmitted disease can last several weeks followed, in some cases, by months of chronic fatigue (Jacobs, 1998). An extremely low infectious dose (10–50 organisms via aerosol), ease of aerosolization, and greater virulence when inhaled make it an ideal bioweapon (Dennis et al., 2001) capable of incapacitating and killing large enemy formations. Aerosolized Francisella may be fatal in 60% of untreated individuals (Hornick and Fort Detrick, 1958). Francisella has been classified on the US Federal Select Agents and Toxins List as a Tier 1, CDC Category A biological agent, and Risk Group 3 pathogen capable of causing serious disease (US Centers for Disease Control and Prevention, 2020).
F. tularensis can cause infection by multiple routes, causing various cutaneous, glandular, and ocular syndromes. The more serious pneumonic form of the disease occurs commonly in laboratory workers exposed by the aerosol or oral routes. Laboratory-acquired infection with F. tularensis (Ft) is a well-recognized hazard of Ft biomedical research, with >200 cases described in the American medical literature (Van Metre and Kadull, 1959; Overholt et al., 1961). Pneumonic disease is the syndrome most likely to be encountered in a deliberate attack. It is characterized by fever, malaise, pneumonic infiltrates on X-ray, severe prostration, and diarrhea lasting 3–6 weeks. Survivors may suffer chronic fatigue for months. Individuals suffering pneumonia or pleuritic infection face a poor prognosis. Death is most common with the pneumonic form. Tularemia contracted from zoonotic sources can be treated with antibiotics including streptomycin, gentamicin, doxycycline, and ciprofloxacin. Recommended treatment duration is 10–21 days depending on the stage of illness and medication used, although it is unclear how well conventional antibiotics would perform in a deliberate aerosol attack. Substantial insights on its potential for weaponization were gained in part by 40 challenge experiments on healthy volunteers, participants in “Operation White Coat,” exposed to various doses over 10 years starting in 1958 (Williams et al., 2019). There is a clear infectious dose–response relationship with reduced time of onset and more severe disease occurring at higher exposures. Aerosolized F. tularensis resulting in infection may relapse if not treated with 10–14 days of sufficient antibiotic therapy equivalent to >2 g/day tetracycline (Williams et al., 2019). Although tetracycline itself is no longer recommended, doxycycline continues to be a treatment option as well as fluoroquinolones and aminoglycosides (Yeni et al., 2021).
While there are no approved vaccines in the US, the attenuated “live vaccine strain” (LVS) for protection against tularemia has been used experimentally in the US since the 1950s (Eigelsbach et al., 1962). By further attenuating original F. tularensis vaccine stocks provided by the N. F. Gamaleya Federal Research Center for Epidemiology & Microbiology (Falbich, 1946; Eigelsbach and Downs, 1961), the US Army Medical Research Institute of Infectious Disease (USAMRIID) subsequently derived the LVS vaccine. The vaccine is derived from a milder subspecies of F. tularensis known as Ft. holarctica or type B, found in the Northern hemisphere and the only naturally occurring European subspecies. The vaccine has been used in nearly 4,000 volunteers since the late 1950s. Both cellular and humoral immunity play a role in the vaccine’s effectiveness. It is administered by scarification, similar to the smallpox vaccine. The most reliable sign of successful immunization remains a “take” reaction at the scarification site. This has been attributed to a delayed hypersensitivity T-cell response to the tularin protein (Koskela and Herva, 1982). While the extent of clinical protective efficacy has been explored perhaps more than any other biodefense vaccine produced to date including human challenge trials, specific immune correlates of protection in humans remain elusive.
Although studied under IND for decades, LVS has never received marketing approval by a stringent regulatory authority. Pursuit of full licensure has been hampered by concerns regarding potential vaccine strain reversion to wild type (though this has not been documented in clinical studies), purported challenges for vaccine manufacture at commercial scale, and lack of a commercial partner. In the absence of an alternative candidate in clinical development, USAMRIID has continued to vaccinate laboratory workers at risk for occupational Francisella exposure under the Special Immunizations Program (SIP) (Pittman and Plotkin, 2018). The SIP was maintained for several decades at USAMRIID and vaccinated workers from numerous bio-containment laboratories in the US working on F. tularensis and other select agents. The work has continued under IND in the hopes of adding to the clinical database, providing critical comparative data for emerging alternatives. The vaccine is also thought to impart volunteer laboratory workers a potential added layer of protection against Ft infection beyond standard biosafety laboratory practices. We report here data from two longitudinal protocols of LVS administered open label to at-risk personnel, namely, study FY03-24 and study FY07-15. At present, no further studies of LVS are planned, and these may represent the last clinical trial data of the LVS vaccine.
Methods
Study design
Two consecutive longitudinal uncontrolled open-label, phase 2 safety and immunogenicity studies of the LVS vaccine were conducted under separate but similar protocols between 2004 and 2017 under IND 157 (US FDA). Individuals who entered containment laboratory suites where Francisella might be aerosolized, those with possible contact with contaminated equipment or materials, and, in some cases, those travelling to endemic countries were vaccinated. Placebo and/or other controls were not used, as the volunteers were all at risk of occupational laboratory-acquired tularemia infection.
Vaccine
The vaccine used for both studies was F. tularensis Vaccine, Live, NDBR 101, Lot 4 manufactured by the National Drug Company in 1962 under Investigational New Drug Application 157. The vaccine consists of live, attenuated F. tularensis, in a modified casein partial hydrolysate medium manufactured on 28 May 1962. The vaccine was stabilized with a solution of glucose cysteine hemin agar and sucrose gelatin agar stabilizer solution without preservative and stored between −10°C and −30°C. Potency testing in guinea pigs under Good Laboratory Practices (GLP) was performed on an annual basis by Southern Research Institute and/or USAMRIID and reported to FDA. Vaccine use was continued annually based on demonstrated protection of guinea pigs against challenge with virulent F. tularensis under FDA Good Laboratory Practices.
Vaccine vial vacuum seal integrity was confirmed using a high frequency generator (the SPARK test). Vials with an intact seal were reconstituted in 2.0 mL sterile water for injection, USP at a concentration of approximately 1.0 × 109 organisms/mL. Vaccine could be stored once reconstituted for up to 8 h at 2–8°C. Roughly 0.06 mL of vaccine was administered to the volar forearm with 15 superficial punctures in protocol FY07-15, compared to 0.0025 mL in protocol FY03-24. The difference in final dosage estimates between the two protocols was due to differences in scarification techniques employed. Exact administered dose with the scarification technique is impossible to precisely quantitate. In the earlier FY03-24 protocol following alcohol disinfection, the needle was dipped in the vaccine vial, then lightly dabbed into the skin. In the FY07-15 protocol, following acetone disinfection, the droplet was placed directly on the skin first, and the needle was jabbed with greater force to produce a slight return of blood. Excess vaccine was gently dabbed with sterile gauze, and the injection site was allowed to air dry for 30 min.
Study subjects
Volunteers at risk for infection with Francisella from various BSL-3 laboratories in the US were enrolled at USAMRIID (Fort Detrick, MD) under IND protocols FY07-15 and FY03-24. Volunteers had to be between 18 and 65 years old with known risk for F. tularensis exposure and willing to participate in the protocol for the duration of the study, approximately 6 months. They had to be medically cleared to participate by an investigator based on history, physical exam, and laboratory testing. Volunteers for enrollment in FY07-15 could not have had a documented tularemia infection or have been vaccinated against tularemia in the preceding 10 years. For study FY03-24, volunteers could not have ever received a tularemia vaccination. Subjects could not have received antibiotics within the prior 7 days or vaccination with another vaccine within 4 weeks, be immunodeficient, have confirmed HIV, or use immunosuppressing medications. Volunteers were also excluded if pregnant or lactating, allergic to vaccine components, or had clinically significant abnormal laboratory results within 60 days prior to vaccination.
Experimental methods
Following informed consent, volunteers were screened for inclusion in the study by medical history, physical examination, chest X-ray, electrocardiogram (EKG), CBC, chemistry, urinalysis, liver function tests, hepatitis, and HIV antibodies. A baseline microagglutination titer was obtained, and volunteers were excluded if positive. Female volunteers had a urine or serum pregnancy test. Those eligible were administered vaccine on study day 0, with follow-up for take reaction on days 1, 2, and between day 5 and 9. Volunteers returned between days 12–16 and 28–35 for adverse event assessment, with microagglutination titers (MA) obtained between days 28 and 35. A close-out interview and final scarification site assessment were completed at 6 months for protocol 07-15 and at 1 year for 03-24. Second doses of vaccine were not routinely administered unless a subject did not have an initial take reaction. Subjects with insufficient initial responses to vaccine at 28–35 days had MA titers recorded. In protocol 07-15, subjects returned for follow-up examination on days 1 and 2, between days 5–9, 12–16, and 28–35, and at 6 months (± 14 days) after vaccination or revaccination for clinical evaluation of adverse events (AEs) and for documentation of responses to vaccine. Additionally, subjects were, in some cases, asked to return on days 56–84 for laboratory tests.
Immunogenicity and serology
Vaccination effectiveness was assessed based on formation of an erythematous papule, vesicle, and/or eschar with or without underlying induration, known as a “take reaction” between 5 and 9 days after vaccination. MAs were performed by the USAMRIID Research Serology lab using previously described methods (Massey and Mangiafico, 1974; Sato et al., 1990). Briefly, patient serum collected at the indicated time points below was incubated with a standardized 1:5 dilution Ft antigen for 24+/−4 h at serial dilutions from 1:10 to 1:20,480. The highest serum dilution observed with an even lattice of agglutination and only a small transparent button of stained cells at the bottom of the microtube well was considered the endpoint titer. Rabbit antiserum was used as a positive control and pre-tested human serum as a negative control. An MA titer >1:20 was considered a positive response in protocol FY03-24, while a 4-fold rise in titer from baseline were considered a positive antibody response for protocol FY07-15. The change in protocol FY07-15 was made at least partly in response to a participant who had a high background titer of 1:40. The participant was not vaccinated and subsequently developed tularemia due to a laboratory exposure (Lam et al., 2012). MA titer was repeated at day 56–84 if the day 28–35 MA titer increased <4-fold. If there was no positive take reaction following the initial vaccination, a second dose was recommended and offered at least 28 days after the previous vaccination. If there was no positive take reaction 28–35 days after the second dose, or MA titer increased <4-fold, a third dose was considered.
Safety
Participants were monitored continuously for adverse events throughout the study. Adverse events were described using contemporary versions of the National Cancer Institute Common Terminology Criteria for Adverse Events (CTCAE). Adverse events and subject safety concerns were reviewed by the Investigator and the Sponsor’s clinical research and medical monitors. Subjects were monitored for 30 min following vaccination. Subjects were seen in clinic for follow-up on days 1, 2, 7, 14, and 28. The study staff contacted subjects at week 3 by phone or email to ensure attendance at the week 4–5 safety and immunogenicity assessment.
Statistical analysis
At the conclusion of the study, data were transformed to SAS® data format using DBMS/COPY™ or system-specific data transfer programs. Descriptive tables of demographics, compliance, frequency and rate of each reaction, responder and non-responder rates, study deviations, concomitant medications, any local injection measurements or vitals taken, and out-of-reference range laboratory values were compiled.
Endpoint measurements were evaluated for all intent-to-treat subjects regardless of compliance with titer schedule. However, titers obtained on samples collected outside the protocol prescribed window were excluded from further analysis. Data from the final statistical analysis report from each study were reviewed; no missing data were identified in pre-analysis review. Key immunogenicity outcome measures included “take reaction” and MA titers. MA titer criteria differed by study with a >1:20 considered positive in the FY03-24 study compared to a 4-fold rise for the FY07-15 study. Safety endpoints included vaccine relatedness of adverse events and frequency and severity.
Titer values less than the lower limit of detection (LLD) were set at LLD/√2. Titer values were log transformed, and these log-transformed titer values were determined to be normally distributed using the Shapiro–Francia test at a significance level of α = 0.05. The studies were designed as open enrollment to offer tularemia vaccination to all laboratory workers who desired it; as such, no pre-hoc sample size estimations or power calculations for pre-specified hypothesis tests were used. Post-hoc analysis showed that the sample size was sufficient for each protocol at the day 28–35 time point to estimate the mean log titer with less than a width of 0.4 at a 95% confidence level.
Descriptive statistics of geometric mean titer response and take reactions are presented for all time points. For the purposes of statistical analysis, comparisons of titer response and take reaction at the day 28–35 time point following initial vaccination were compared between protocols. Additionally, differences in titer response by sex and age were evaluated within each protocol and overall, at the day 28–35 time point, and at the month 12 time point for the FY 03-24 study. Comparisons of geometric mean of titer values and take reactions by categorical variables (i.e., sex) were conducted using t-test and Pearson’s chi-squared analysis, respectively. Comparisons of titer response and take reaction by continuous variables (i.e., age) were conducted using simple linear regression and simple logistic regression analysis, respectively. A multivariate regression model of titer response by age and sex was also performed at day 28–35 for FY03-24, FY07-15 and overall, and month 12 for the FY03-24 study. Statistical significance was defined at the two-tailed 95% confidence level. All analysis was performed using GraphPad Prism version 8.0.0 for Windows (GraphPad Software, San Diego, CA, USA, www.graphpad.com).
Study approval
All human subject research studies described were approved by the Institutional Review Board of the US Army Medical Research and Development Command (formerly Medical Research and Materiel Command) prior to commencement. Protocol FY03-24 was conducted from 1 October 2004 to 6 October 2009, while protocol FY07-15 ran from 28 August 2009 to 4 December 2017. Both studies were registered at ClinicalTrials.gov as NCT00584844 (trial FY03-24) and NCT00787826 (trial FY07-15). An additional protocol FY15-14 was registered as NCT03867162 but never enrolled subjects. Informed consent was obtained from all subjects prior to enrollment. The investigators have adhered to the policies for the protection of human subjects as prescribed in 45 CFR 46.
Results
There were 484 subjects screened for study FY03-24, which ran between October 2004 and September 2008. Of the 462 vaccinated, 405 completed the final study visit at 6 months. To enroll in the study, subjects had to have a titer <1:20, no previous vaccination, and no documented tularemia infection. A small number (Sawyer et al., 1962) were revaccinated with the same initial dose of vaccine, based on inadequate response to the initial vaccination (titer <1:20) and two required a second revaccination (third dose) (Figure 1A).
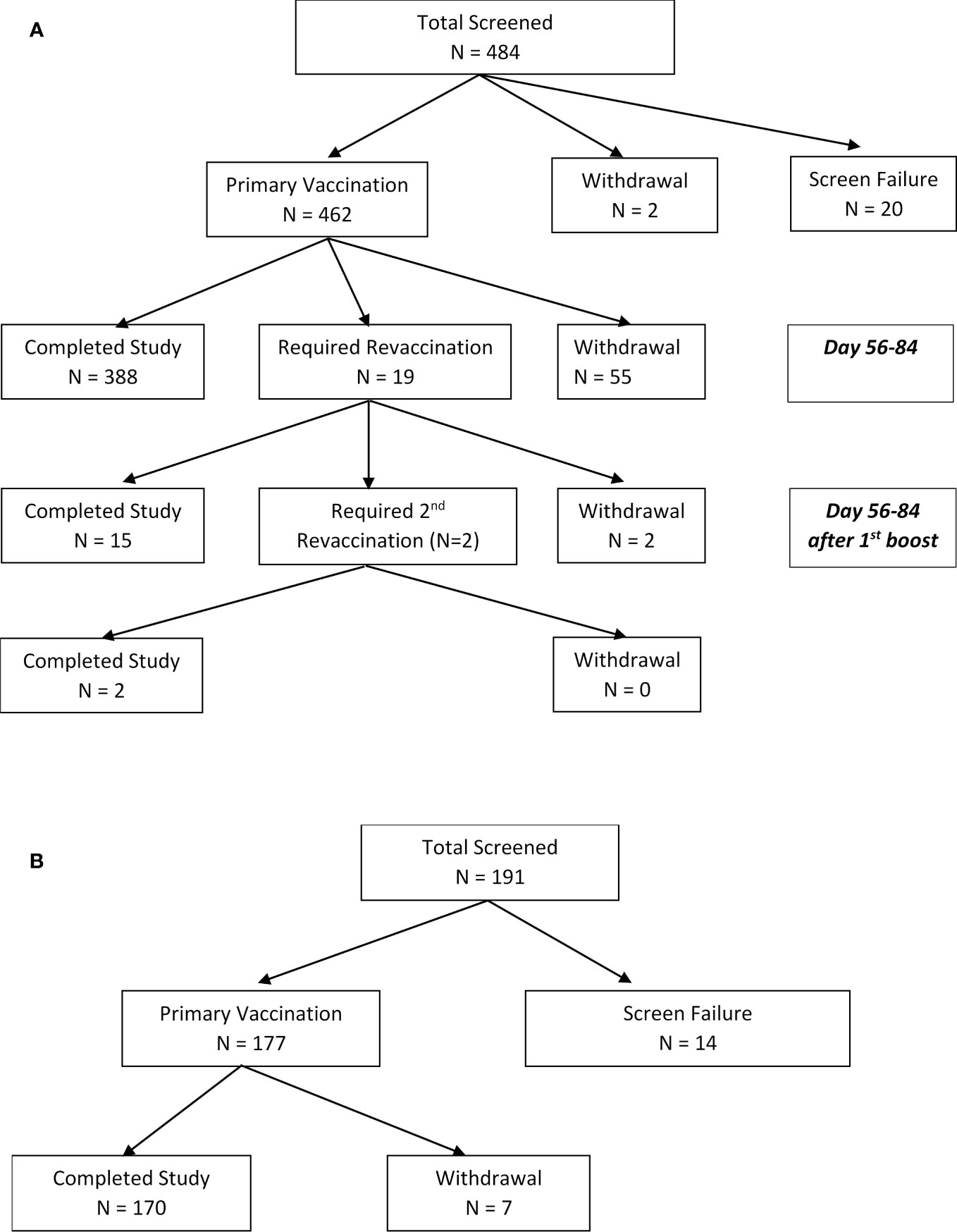
Figure 1 (A) CONSORT diagram. Disposition of subjects enrolled to LVS tularemia vaccine study FY03-24 (enrollment from 01/10/2004 to 15/09/2008). Reasons for screening failures included having previously received the vaccine or having a positive titer (n=10), previous exposure (Francis, 1919), medical reason (Francis, 1919), not able to travel to USAMRIID to receive the vaccine (Francis, 1919), or no longer at risk (US Centers for Disease Control and Prevention, 2020). (B) CONSORT diagram. Disposition of subjects enrolled to LVS tularemia vaccine FY 07-15 (enrollment from 28/08/2009 to 05/05/2017). To enroll in the study, subjects could not have had a prior tularemia vaccination within the past 10 years. Reasons for screening failure included medical (Dennis et al., 2001), relocation prior to vaccination (Bartelloni, 1969), and no longer at occupational risk (Hornick and Fort Detrick, 1958). Subjects were considered to be fully vaccinated if they had a 4-fold rise from baseline and a positive “take reaction”.
There were 191 subjects screened and 177 vaccinated for study FY07-15 which ran between August 2009 and May 2017 (Figure 1B). In contrast to FY03-24, subjects in FY071-15 could not have had a prior tularemia vaccination within the past 10 years. In FY07-15, subjects with inadequate titers were not revaccinated. Adequate titers were also defined differently, as greater than 4-fold rise over baseline. There were 170 completing FY07-15 with seven withdrawals before the final study visit at 6 months. There were seven withdrawals during the course of the study, all due to relocation or change in employment no longer exposing them to tularemia risk. More than 60% of subjects in both studies were 20–40 years old, >60% male, and >85% Caucasian. Demographic characteristics of participants in both studies are shown in Table 1. More than 10% of subjects entering FY03-24 and 25% of those in FY07-15 had baseline microagglutination titers against tularemia >1:20. It should be noted that having a titer >1:20 was not exclusionary in FY07-15. All participants in FY07-15 study were coded as the participant’s initial vaccination.
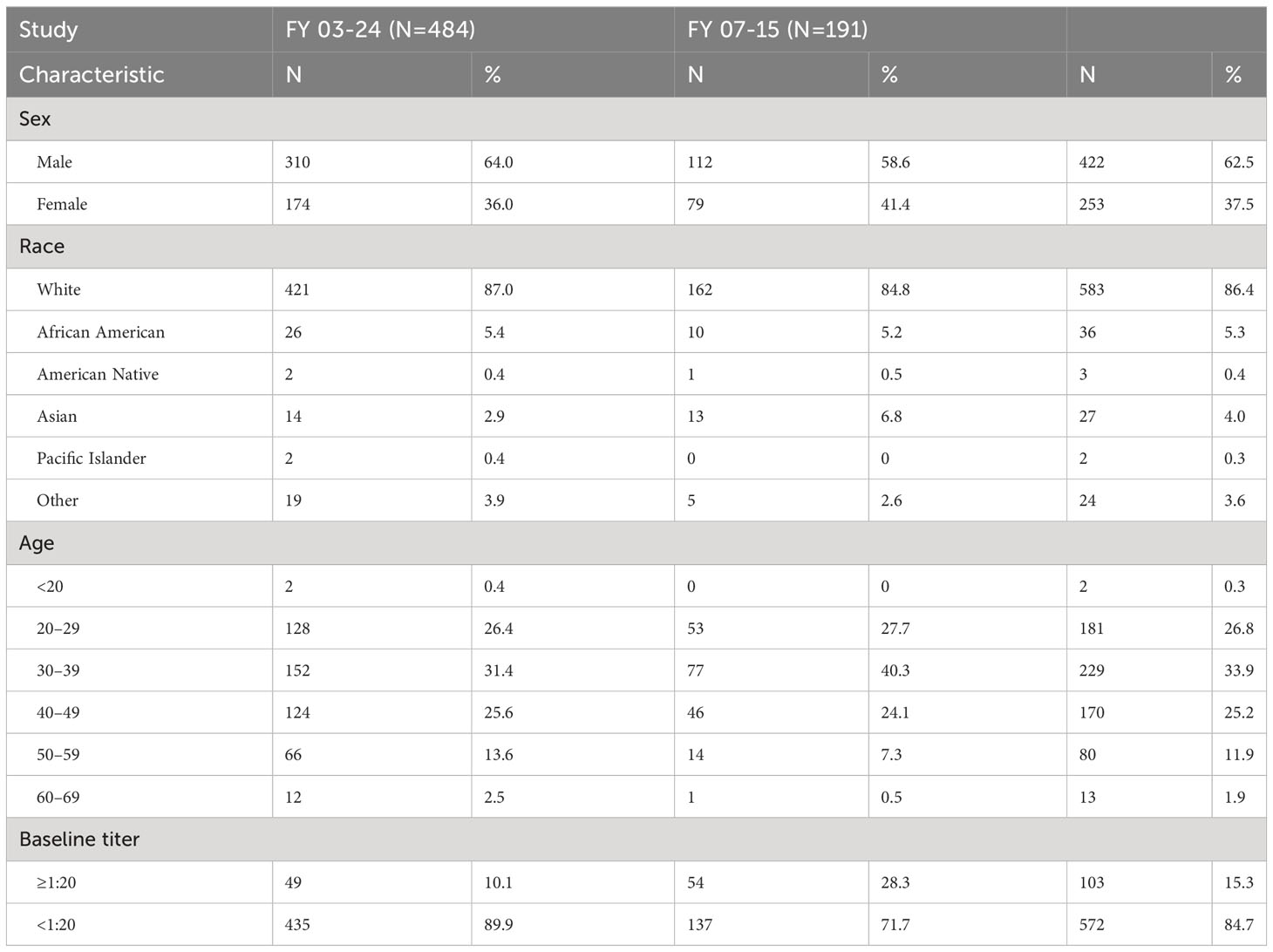
Table 1 Participant demographics. Characteristics of participants screened for the two studies—FY 03-24 (2004–2008) and FY 07-15 (2009–2017).
Immunogenicity
Overall, the live F. tularensis vaccine (NDBR-101, Lot 4) had comparable rates of immunogenicity to those seen in prior studies based on the two principle measures—microagglutination antibody titers and take reaction (Table 2). Figure 2 demonstrates live “take reactions” in three individuals after a single estimated dose of 0.06 mL of LVS vaccine using the scarification method. Immunogenicity response rates were uniformly high. In both studies, all protocol-compliant subjects had a measurable immune response (titer >1:20 and “take reaction”). A positive “take reaction” was defined as development of one of the following: erythematous papule, vesicle, and/or eschar formation. All 177 subjects vaccinated (100%) in FY07-15 had a positive “take reaction” within 9 days of vaccination. In FY03-24, 95.5% of subjects (n=441; SEP=0.010) had a positive take reaction at first vaccination. Subjects in FY03-24 without adequate initial responses were revaccinated. Positive take reactions were seen in 89.5% (n=17; SEP=0.007) of those receiving a first revaccination, and 100% receiving a second revaccination. No statistically significant differences in initial “take reaction” rates in the FY 03-24 study for any vaccination event were observed when analyzed by sex (via Pearson’s chi-square) or age (simple logistic regression).
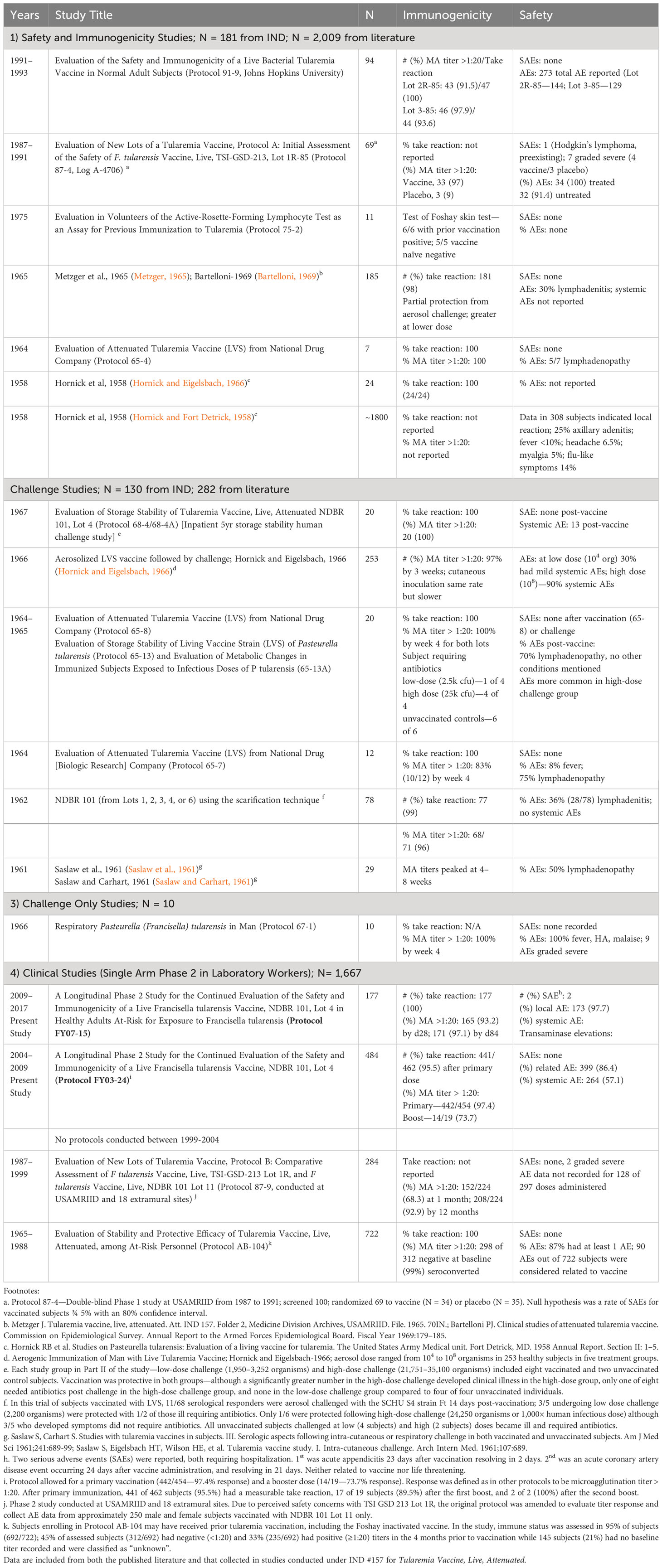
Table 2 Summary of LVS vaccine studies conducted at USAMRIID and partnered facilities. Between the mid-1950s and 2017, the LVS tularemia vaccine was tested in numerous clinical trials to include 1) healthy volunteer studies for safety and immunogenicity, 2) human challenge studies with tuleramia via the cuteneous and aerosol routes with and 3) without vaccination, in the latter case to better understand the natural history of human challenge with the Schu-S4 tularemia strain, and 4) studies in otherwise healthy laboratory workers potentially exposed to tularemia.
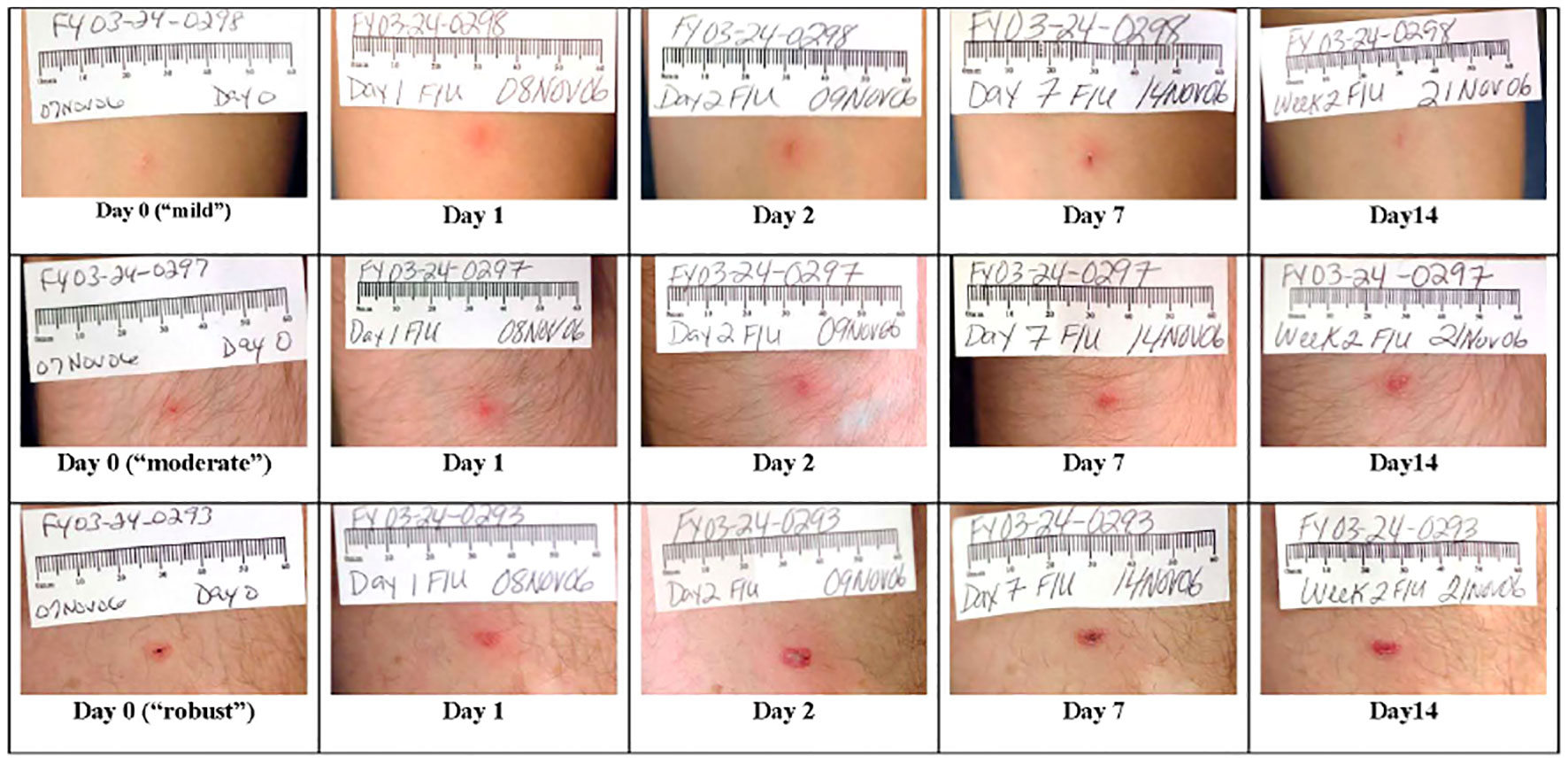
Figure 2 LVS live, attenuated tularemia vaccine “take reactions” in three representative individuals. A single dose of 0.06 mL of LVS vaccine at a concentration of 1.0 × 109 organisms/mL was inoculated into the volar forearm of each volunteer using the scarification method. Although the three individuals depicted were immunized using the same technique, these pictures show a range of possible reactions. The three individuals had day 28–35 MA titers of 160, 80, and 640, respectively. As shown in the photographs, in all three cases, there is appreciable healing of the site by day 14 following vaccination. By day 28, only a small hyper-pigmented macule remained at each vaccination site (not shown).
In protocol FY03-24, of the 454 protocol-compliant post-vaccination titers collected at day 28–35, 442 (98.6%) were >1:20. The geometric mean of month 12 titers was 117 (95%CI=105–130) in the FY03-24 group. LVS was also highly immunogenic in the FY07-15 study where immunogenicity was defined as a ≥4-fold rise in MA antibody titer following vaccination. For FY07-15, a limited sample of 14 participants completed a 6-month titer for which the geometric mean was 101.7 (95%CI=24.6–421).
This immunogenicity endpoint definition differed from prior studies, including FY03-24 where a >1:20 titer value was considered immunogenic. All but nine subjects vaccinated in FY07-15 had a ≥4-fold rise in titer by day 28–35 and were not evaluated again. There were nine subjects (5%) who had titers repeated at day 56–84, and of these, six of nine (67%) had a ≥4-fold rise in titer, while three remained non-responders (33.3%). However, all three of the latter individuals had “take reactions.” One of the three non-responders developed an upper respiratory infection and was treated with antibiotics within the first week after vaccination, providing a likely cause for non-response. Geometric mean microagglutination titers are shown in Figure 3 with baseline values for FY03-24 of 7.98 (95% confidence interval (95%CI) =7.69–8.28) and 9.56 (95%CI=8.67–10.54) for FY07-15. Peak geometric mean titers at vaccination day 28–35 were 181 for FY03-24 (95%CI=161–204) but significantly higher at 269 for FY07-15 (95%CI=223–323; p=0.0006 for t-test of log-transformed data).
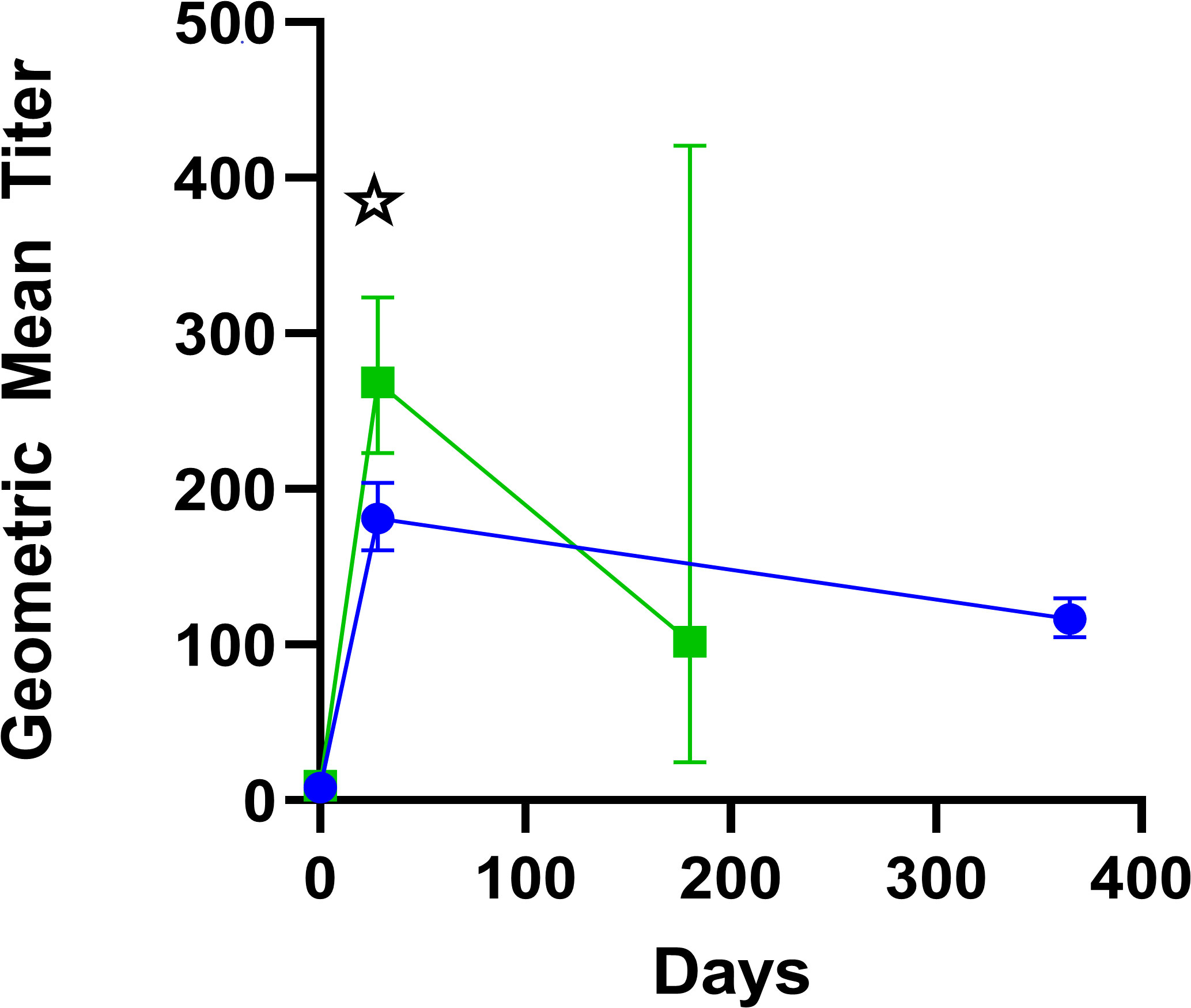
Figure 3 Geometric mean titers with 95% confidence intervals of microagglutination titers. Green squares represent participants in the FY 07-15 study, in which titers were drawn at baseline, day 28–35, and 6 months. Blue circles represent participants in the FY 03-24 study in which titers were drawn at baseline, day 28–35, and 12 months. Significant differences in geometric mean titers between the protocols at common time points are marked with a star.
There were very few follow-up titers at the 6-month time point for FY 07-15 (n=14 of the original 191 vaccinated), as these were only performed if the day 28–35 response was inadequate. Substantially more had 12-month follow-up titers in FY 03-24 (n=407 of the 459 vaccinated). Where available, titer levels were substantially less at month 12 for the small number revaccinated with geometric mean values of 19.54 (95% CI 18.38–52.03); n=18) for first revaccination and 28.28 (95% CI, 0.346–2312) for the two undergoing a second revaccination. It is noteworthy that the same lot of vaccine was used for both studies, although geometric mean of titer responses were higher following initial vaccination in study FY07-15 (268.5 vs. 181.0; p= 0.00064).
There were no significant differences in geometric mean of day 28–35 titer values for either study, or overall, by sex (Figure 4A). Additionally, there was no statistically significant difference in geometric mean titer values between male and female individuals for the month 12 titer in FY03-24 (111.4 vs 126.0; p = 0.278). Simple linear regression of titer values by age revealed modest statistically significant declines with increasing age for the day 28–35 time point for study FY03-24 and overall, but not for FY07-15 (Figure 4B). For the month 12 titer of FY 03-24, there was also a statistically significant difference in log titer by age(y=−0.007x +2.324x+273.2; p = 0.003). Multivariate models of log titer regressed by age and sex revealed the same findings for all time points, with age being significantly associated with titer at day 28–35 for FY03-24 and overall (βage = −0.029, p < 0.001; βage = −0.026, p < 0.001, respectively) and at month 12 in the FY03-24 protocol (βage = −0.019, p < 0.001), but not at the day 28–35 time point for FY07-15 (βage = −0.011, p = 0.015). Sex was not found to be significantly associated with log titer at any of the time points assessed in multivariate models (day 28–35: FY03-24 βsex = 0.239 p = 0.056, FY07-15 βsex = 0.102 p = 0.540, overall βsex = 0.182 p = 0.085; Month 12 FY03-24 βsex = -0.039 p = 0.732).
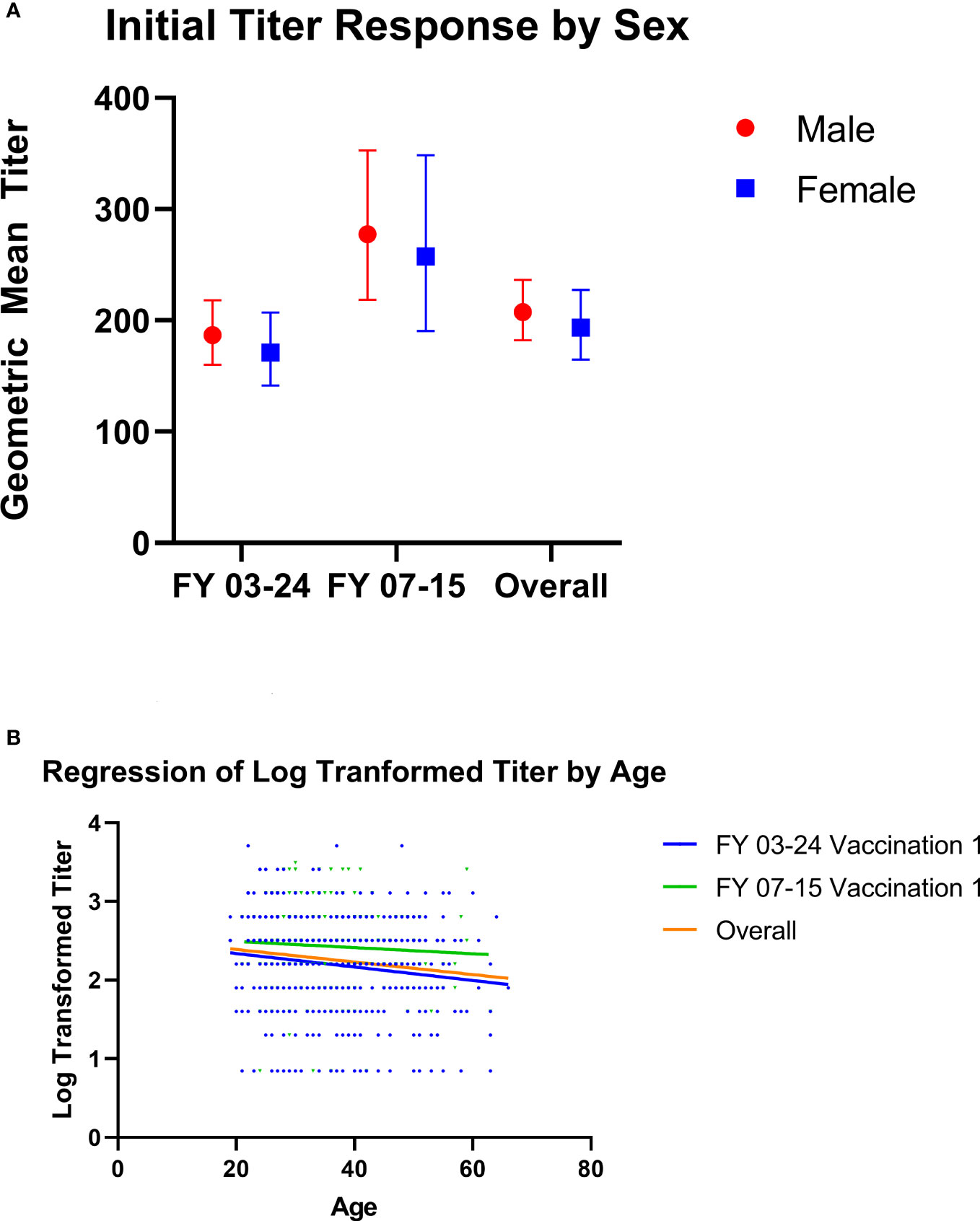
Figure 4 (A, B) Microagglutination titer values by age and sex at day 28–35. (A) Geometric mean microagglutination titer values are shown for male (red circle) and female (blue squares) subjects from Study FY07-15 and FY03-24 and for the two studies combined following first vaccination. There were no significant differences in either study by sex. Geometric mean titers (with 95% confidence intervals) in FY03-24 were 186.9 (160.2–218.1) for male and 171.2 (141.6–207.1) for female subjects (unpaired t-test, p = 0.488). Geometric mean FY07-15 titers were 277.6 (218.4–352.7) for male subjects and 257.6 (190.5–348.5) for female subjects (unpaired t-test p = 0.699). Overall geometric mean titers were 207.6 (182.2–236.5) for male and 193.6 (164.7–227.5) for female subjects (t-test, p=0.5110). (B) Simple linear regression of log-transformed titer values by age is shown for FY03-24 (blue lines/dots), FY07-15 (green lines/dots), and the two studies combined (orange lines). While there was a statistically significant decline by age in FY 03-24 (y=−0.00857x+2.510; p = 0.0016) and overall (Y = −0.00799 + 2.550; p = 0.0008), decline was not significant in FY07-15 alone (Y = −0.00392 + 2.571; p = 0.4088).
Adverse events are shown in Table 3. In study FY03-24, 401 of 462 vaccinated subjects (86.6%) had at least one adverse event during the course of the study. In FY07-15, 170 of 177 (96.1%) reported at least one AE. Local AEs were most common, reported in 84.1% in FY03-24, and 81.4% in FY07-15 with only one local event graded as severe in the former study. Scarification site lesions and pain were the most prominent overall, accounting for nearly two-thirds of local reactions. Systemic adverse events occurred in fewer than 17% of those vaccinated with leading causes including fatigue (4.8%), headache (3.9%), myalgia (2.9%), or fever/chills (1.5%). Pharyngitis, often unilateral and with a tonsillar exudate, was seen in <1% of subjects. Most AE rates were consistent across the two studies, with the exception of lymphadenopathy, which was noticeably higher in FY03-24 (18.2% vs. 2.8%). Anatomic sites of observed lymphadenopathy in study FY03-24 included 65 axillary, 15 epitrochlear, 2 cervical, and 143 unspecified with 17 reported as epitrochlear in FY07-15 (and the remainder unspecified). Scarification site pain was more commonly reported in FY07-15 (43.5% vs. 16.6%).
All adverse events, including serious adverse events, had onset dates up to 37 days post-vaccination. The duration of adverse events, including serious adverse events, ranged from 1 days to 367 days. Two serious adverse events (SAEs) were reported. Both required hospitalization. One—a case of acute appendicitis 23 days after vaccination—resolved within 2 days. The second was a coronary artery disease event occurring 24 days after vaccine administration, which resolved in 21 days. Neither was determined to be related to the vaccine nor deemed to be life threatening.
Discussion
The results seen from the most recent LVS protocols at USAMRIID parallel those from a large body of clinical trials conducted from the mid-1950s to the present in late 2017. The data presented here from 2004 to 2017 may represent the final LVS doses provided to volunteers under an IND clinical study. The clinical experience with the F. tularensis Vaccine, Live, NDBR 101 and related LVS vaccine lots is summarized in Table 2 below. Lot 4, NDBR 101 manufactured in 1962 has been in at least 1,998 volunteers under IND 157. Combined with literature reports using other vaccine lots, LVS has been administered to roughly 4,279 volunteers. Overall, the vaccine has been shown to be safe. Common side effects include local lymphadenitis and scarification site pain in up to 80% within a few days of inoculation. Short-lived mild to moderate systemic symptoms (fever, malaise, and myalgia) are also common in 15%–20% within a few days to 1 week of inoculation. There have been only a handful of serious adverse events reported over this long period of use, none of which have so far been attributed to the vaccine. Particularly remarkable is that vaccine lots used in the present were up to 55 years old, retaining remarkable potency under controlled storage conditions. Immunogenicity as measured by the “take reaction” has been consistently high with 95%–100% of volunteers reacting after the first vaccination. Nearly all respond after a booster, even many years after lot manufacture. Likewise, immune reactions as measured by micro-agglutination titers >1:20 are typically seen in 90% of recipients by 4 weeks and approaching 100% by 8 weeks. These simple, well-established findings should serve as benchmarks for future tularemia vaccine candidates.
As a result of years of diligent effort, a tremendous amount is known about the clinical characteristics of live, attenuated self-replicating tularemia vaccines. While there has not been an opportunity to demonstrate vaccine efficacy against a deliberate biological attack, carefully conducted human challenge studies under Operation White Coat using the SCHU-S4 strain reveal important insights into vaccine performance parameters (Williams et al., 2019). Antibody levels >1:20 by microagglutination assay, an amount that has been historically thought to confer protection, were reached in the vast majority of volunteers following a single LVS dose (Table 2). Table 4 summarizes published challenge study reports. LVS was protective in most cases against low-concentration SCHU-S4 challenge (2,500 organisms), although vaccine efficacy could be overcome at higher challenge concentrations (25,000 organisms)—100-1,000-fold times greater than the minimum human infectious doses (Sawyer et al., 1962; Metzger, 1965; Bartelloni, 1969). Protection was afforded against both aerosol and intradermal challenge, although greater against the latter; see Table 4. While it is unlikely that such studies could be approved today on ethical grounds, it should be noted that it was rare even for unvaccinated subjects challenged with low concentrations of F. tularensis to require antibiotic therapy. Many vaccinated subjects did not require antibiotic therapy at higher challenge concentrations, although unvaccinated subjects did so universally (Hornick and Eigelsbach, 1966). In addition to direct evidence from challenge, indirect evidence comes from demonstrated reductions in occupational exposure infections among at-risk laboratory workers after introduction of LVS (Burke, 1977). The role of vaccination in an individual with an active infection or after virulent Francisella exposure is unclear and should be evaluated in the future.
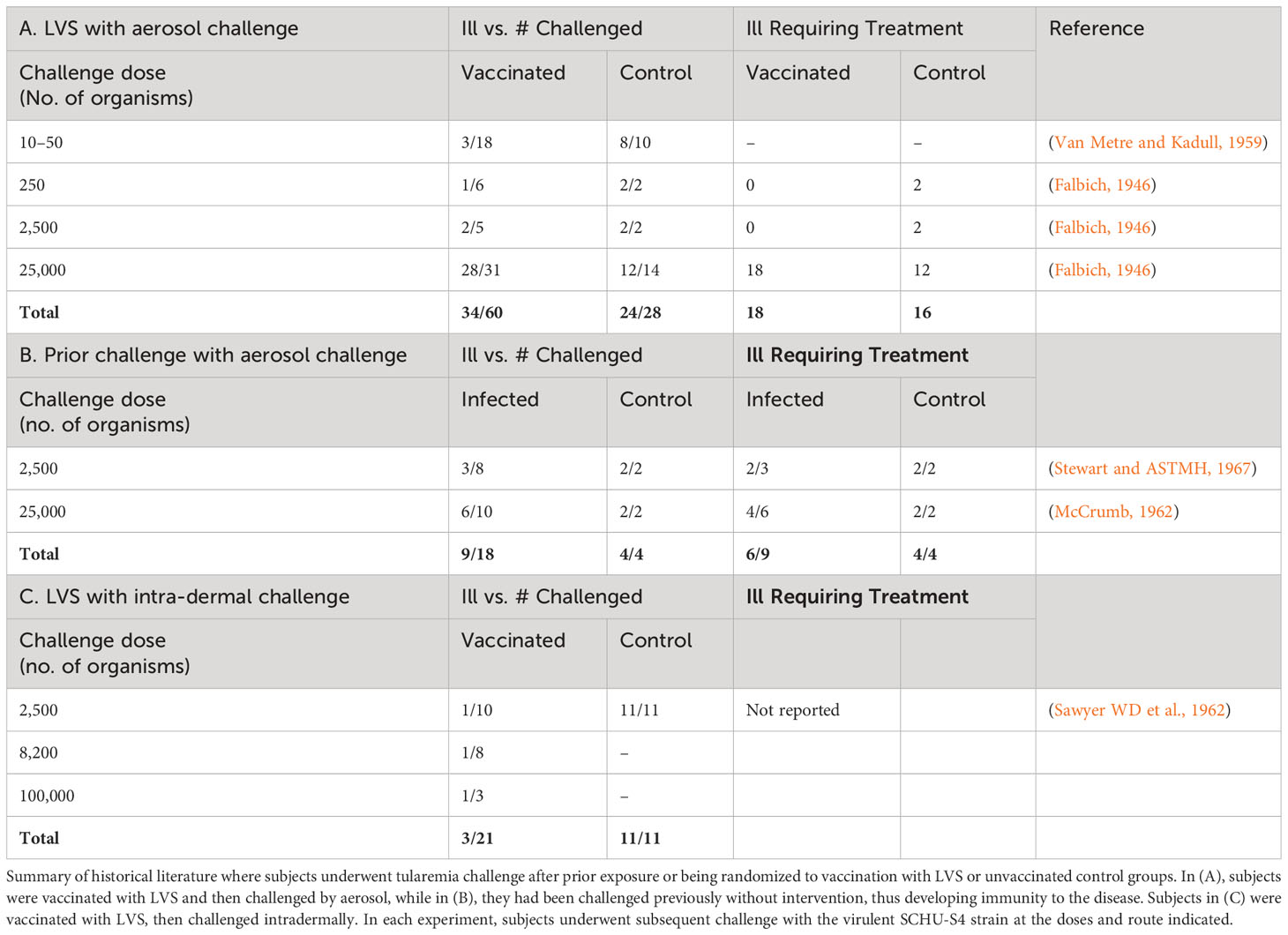
Table 4 Protective effects of live attenuated tularemia vaccine (LVS) or prior tularemia exposure against subsequent tularemia challenge.
Microagglutination titers remained remarkably stable in the present study spanning 13 years of vaccination with the same product lot manufactured in 1962. The resiliency of present vaccine lot immunogenicity observed is consistent with a recent active comparator study (Mulligan et al., 2017a). No differences were found in take reaction or seroconversion by microagglutination between the USAMRIID LVS vaccine and a newer vaccine lot manufactured for which NDBR 101, lot 4 served as seed material (Mulligan et al., 2017b). Surprisingly, mean titers were modestly higher among subjects in the later study (FY07-15) despite the continued aging of lot 4. While there is no immediate explanation for this, titers were only modestly elevated (535) compared to averages in FY03-24 (mean=380). Despite this long-term stability and the aforementioned protective efficacy, specific immune correlates of protection remain elusive. The role of humoral immunity in clinical protection from F. tularensis remains controversial and poorly understood. Conventional wisdom would indicate that an intracellular bacterium might require a cellular immune response (Kirimanjeswara et al., 2008), although an extracellular form of the organism has been identified (Forestal et al., 2007). In humans, circulating antibodies to F. tularensis were initially measured with agglutination assays and enzyme-linked immunosorbent assays (ELISA) (Carlsson et al., 1979; Viljanen et al., 1983; Rusnak et al., 2004). In clinical research, a >1:20 microagglutination titer has been used to indicate an adequate vaccine response. LVS administered to subjects by scarification has reliably exceeded this benchmark over more than 6 decades (see Table 2). Bacterial agglutinins have been shown to increase and persist for at least 3 years (Eigelsbach et al., 1962). Limited evidence in mice indicates that antibody response is targeted to the lipopolysaccharide (Ft LPS) in both mice and humans. At least partial immunity is provided in passive antibody transfer studies from both murine and human donors with prior LVS vaccination (Drabick et al., 1994; Rhinehart-Jones et al., 1994) and levofloxacin-treated mice who survived SCHU-S4 challenge (Klimpel et al., 2008). This appears to be mediated by the Fc-γ receptor (FcγR) (Kirimanjeswara et al., 2007). Currently available serologic markers of infection are not helpful for real-time diagnosis given a typical delayed rise in IgM, concurrent with IgG 2–3 weeks after symptom onset, nor do they indicate successful treatment as IgG can remain elevated for several years (Stanek and Saunders, 2020). Recently, an outbred rabbit (Rb) model, coupled with a series of live attenuated S4-based vaccines (S4ΔaroD, S4ΔguaBA, and S4ΔclpB) and wild-type Schu S4 aerosol challenge (~2,000 CFU) were used to identify clinically measurable correlates of protection (COP). Analysis of individual rabbits (Rb) enabled retrospective identification of Rb COP that predicted S4 challenge outcome. In pilot studies, the group found several “antigenic” COP in plasma of 80% of LVS-vaccinated humans (Shoudy et al., 2021).
It is thought that cellular immunity may play a dominant role in the protection from F. tularensis given intracellular replication, predominantly in macrophages (Steiner et al., 2014). The “take reaction” following LVS vaccination is the most reliable indicator of cellular immunity, occurring in 90% or more of vaccinations (Table 2). However, take reaction assessment has been implicated in divergence between take and MA titer results, highlighting the importance of experienced readers. Immunogenicity in the present study differed from a recent study comparing USAMRIID LVS to a more recently manufactured batch by Dynport Vaccine Company (DVC-LVS) where there was greater discordance between MA and take reaction results (Mulligan et al., 2017b). Following immunization or exposure to disease, a rarely used skin test (the Foshay test) has been shown to remain positive for up to 10 years (Foshay, 1932; Koskela and Herva, 1982). Cellular immunity in humans is also measurable by a lymphocyte transformation test (Tärnvik et al., 1985). While CD4 and CD8+ T cells have been thought to be predominantly responsible, roles of dendritic cells, macrophages, neutrophils, and natural killer cells have also been described (Steiner et al., 2014). Cellular responses appear 2 weeks after vaccination and tularemia disease (Syrjälä et al., 1984; Tärnvik et al., 1985), in comparison to serology, which typically becomes positive in 2–4 weeks (Chernecky, 2013). However, evidence for the precise immune components involved in cell-mediated immunity remains incomplete. It appears likely that both humoral and cellular immunity play a role. Passive transfer of spleen cells from vaccinated mice were protective against challenge with doses of LVS normally lethal to mice (but not humans) (Fortier et al., 1991). Transfer of thoracic duct lymphocytes from immunized rats reduced the burden of infection following a low-dose challenge with Ft LVS organisms in naive rats, while rats given serum from immunized rats were not protected against Ft challenge (Kostiala et al., 1975). Rats vaccinated with aerosolized LVS survived subsequent lethal aerosol challenge with SCHU S4 without systemic infection. F. tularensis replication in the lungs and characteristic pulmonary granulomatous lesions were reduced substantially although not eliminated (Jemski, 1981), suggesting cellular protection. However, the translation of animal model findings to humans has remained elusive. At least some recent progress has been made at elucidating correlates in a rat co-culture model (Lindgren et al., 2020). In the model, T cells from vaccinated rats are incubated ex vivo with F. tularensis-infected rat macrophages. It is thought that the same approach might be applied to human vaccine studies, which better elucidate cellular correlates of protection. A human model has been developed but has yet to be validated or submitted to a stringent regulatory authority for approval as a surrogate endpoint (Eneslätt et al., 2018).
There may also be a role for mucosal immunity, given that both aerosolized LVS and aerosolized F. tularensis themselves lead to the development of immunity, the former more rapidly though of the same peak magnitude by 4 weeks as the intradermal route (Hornick and Eigelsbach, 1966). When comparing cutaneous to aerosol vaccination in non-human primates, vaccine organisms were recoverable from the site of inoculation initially. Within 28 days, they could be isolated from the lymph nodes, liver, and spleen but were cleared from the body by 90 days (Eigelsbach et al., 1962). IgA-deficient mice were not protected against aerosol challenge following vaccination (Baron et al., 2007; Rawool et al., 2008). Immunity following aerosol challenge has been demonstrated in non-human primates (Macaca mulatta) with a clear dose–response enhancing survival in animals given higher LVS doses (107 organisms) (Hornick and Eigelsbach, 1966). In addition to dermal inoculation, LVS has proven to be protective as an aerosolized vaccine in humans, although side effects following higher doses required for protection may limit the practicality of this approach. In comparison, one study indicates that side effects at higher doses of inhaled SCHU S4 strain organisms (106–108) included fever and flu-like symptoms lasting 2–3 days (Hornick and Fort Detrick, 1958). Syrjala et al. demonstrated that all of the 6 of 16 individuals protected against challenge with the virulent SCHU S4 Ft. strain by previous aerosol exposure to LVS had measurable circulating antibody, while only 1 of the 10 individuals not protected did (Syrjälä et al., 1986). Of note, the individuals in this study had active tularemia infections, confirmed with serology, rather than vaccinated individuals. Whether LVS would be effective and marketable via trans-mucosal delivery route remains to be seen but may be worth investigating among vaccine candidates.
There is no identified replacement or competitor to LVS in clinical stage development at the time of writing. Key characteristics of an optimal tularemia vaccine could include lack of potential for reversion to wild-type F. tularensis in the case of live vaccines, a safety profile in humans equivalent or better than LVS, ease and low cost of manufacture, and efficacy against Ft-type A challenge in well-controlled large animal models. Comparable human immune correlates would need to be demonstrated in healthy volunteers, promising approaches demonstrated in murine (De Pascalis et al., 2012) and human ex vivo co-culture model (Eneslätt et al., 2018). A large slate of candidates have been produced over the years, and there is an excellent review on the subject published elsewhere (Sunagar et al., 2016). Approaches have focused on live, attenuated, and inactivated tularemia vaccines employing various Francisella strains, particularly F. holoarctica and F. novacida (Golovliov et al., 2013a). At least one live, attenuated candidate vaccine featuring a Schu-S4 strain with a clpB gene deletion mutation has been advanced to pre-clinical studies and demonstrated safety and efficacy in murine models (Golovliov et al., 2013b). Inactivated vaccines may provide a safety advantage over live attenuated vaccines but have yet to demonstrate comparable protection in animal models (Baron et al., 2007). Protein subunit vaccines would have a decided safety advantage and also eliminate concerns for pathogenic strain reversion. However, this approach has yet to yield an effective candidate in animal models. DNA and vectored vaccine approaches using attenuated virus or bacteria for the purpose of introducing F. tularensis antigens have similarly resulted in suboptimal levels of animal protection (Rotem et al., 2014; Sunagar et al., 2016). Vaccines targeting the Fc-gamma receptor (FcγR) have been developed, given FcγR’s role in cellular phagocytosis and antigen presentation with mixed success (Franz et al., 2015).
In summary, the LVS vaccine was well tolerated and proved once again to deliver high levels of positive take reactions and microagglutination titers in at-risk laboratory workers over a 13-year period. Of particular interest is the resilience of the vaccine in long-term storage at USAMRIID. Despite manufacturing 42–55 years prior to the studies, sustained high response levels continued to be observed. An incompletely understood mechanism of attenuation, hypothetical concerns for potential reversion to wild type, and instability in culture remain challenges for licensure and safe fielding of a live, attenuated vaccine (Hartley et al., 2006). A successful effort to remanufacture and test the vaccine in 2006 demonstrated that LVS could be manufactured using modern methods (Mulligan et al., 2017b). LVS attenuation mechanisms have also been partially elucidated with mutations in a Type IV pilin gene (pilA) and an outer membrane protein (FTT0918) implicated in murine model virulence (Salomonsson et al., 2009). It is notable that despite largely identical sequences between LVS-vaccine-attenuated organism strains, variability in vaccine immunogenicity has been observed with relatively small changes in sequence (Kurtz et al., 2018). Despite use for more than 70 years and a large safety database, there is currently no plan to pursue full licensure of LVS. One reason for this may be due to testing of other products prior to LVS lot 4 with variable human and animal immunological responses (Hartley et al., 2006; Salomonsson et al., 2009). As potentially the last laboratory worker was vaccinated under a clinical trial in 2017, the present work serves as a benchmark for safety, immunogenicity, and long-term potency against which to compare future alternative vaccine candidates. The long-term potency of LVS would lend itself to a new vaccine protocol under IND for at risk personnel. These would reasonably include laboratory workers, military personnel, and/or limited disaster preparedness stockpiles intended to deter deliberate biowarfare use. Given that the US has no licensed measure for immunoprophylaxis against tularemia disease, a critical biodefense gap against a category A agent remains. Significant ongoing investment is needed.
Data availability statement
The original contributions presented in the study are included in the article/Supplementary Material. Further inquiries can be directed to the corresponding author.
Ethics statement
The studies involving humans were approved by Institutional Review Board of the U.S. Army Medical Research and Development Command. The studies were conducted in accordance with the local legislation and institutional requirements. The participants provided their written informed consent to participate in this study.
Author contributions
DS: Conceptualization, Data curation, Formal analysis, Investigation, Project administration, Supervision, Writing – original draft, Writing – review & editing. BP: Conceptualization, Data curation, Formal analysis, Investigation, Software, Writing – original draft, Writing – review & editing. JH: Conceptualization, Data curation, Formal analysis, Investigation, Methodology, Project administration, Writing – original draft, Writing – review & editing. SN: Conceptualization, Data curation, Formal analysis, Investigation, Methodology, Software, Validation, Visualization, Writing – original draft, Writing – review & editing. AC: Investigation, Writing – review & editing. RR: Investigation, Writing – review & editing. AO: Investigation, Project administration, Writing – review & editing. EB: Conceptualization, Investigation, Methodology, Writing – review & editing. JR: Conceptualization, Investigation, Methodology, Project administration, Writing – review & editing. DD: Data curation, Methodology, Writing – review & editing. BP: Investigation, Writing – review & editing. JB: Data curation, Investigation, Methodology, Writing – review & editing. ET: Writing – review & editing. ID: Writing – review & editing. DL: Data curation, Investigation, Writing – review & editing. PP: Data curation, Investigation, Methodology, Writing – review & editing. TP: Funding acquisition, Resources, Writing – review & editing. MG: Data curation, Investigation, Project administration, Writing – review & editing. MK: Conceptualization, Investigation, Project administration, Resources, Supervision, Writing – review & editing. FG: Investigation, Project administration, Supervision, Writing – review & editing. JA: Data curation, Investigation, Project administration, Writing – review & editing. MK-J: Data curation, Formal Analysis, Investigation, Project administration, Writing – original draft, Writing – review & editing. PP: Conceptualization, Data curation, Formal Analysis, Funding acquisition, Investigation, Methodology, Project administration, Resources, Supervision, Validation, Writing – original draft, Writing – review & editing.
Funding
The author(s) declare financial support was received for the research, authorship, and/or publication of this article. Funding for the studies was provided by the US Department of Defense through the US Army Medical Research and Development Command (USAMRDC).
Acknowledgments
The authors acknowledge the hard work and dedication of all of the members of the investigative and regulatory teams who supported tularemia protocols over the years at USAMRIID, particularly Robert Rivard, Craig Koca, Jason Regules, Mark Paxton, Dixion Rwakasyaguri, Sandi Parriott, Lisa Borek, Paileen Mongeli, Latricia Dye, Cynthia Donovan, Denise Clizbe, Kimberly Pozzouli, Arthur Freedlander, Lawrence Korman, Vincent Fulton, Joel Bozue, and Beverly Foghtman. Most importantly, we would like to acknowledge the volunteers who did the hard work to advance knowledge on countering tularemia and other biothreats by virtue of their professions and their selfless service.
Conflict of interest
The authors declare that the research was conducted in the absence of any commercial or financial relationships that could be construed as a potential conflict of interest.
The Reviewer LMR declared a shared affiliation with the author MK-J at the time of the review.
Publisher’s note
All claims expressed in this article are solely those of the authors and do not necessarily represent those of their affiliated organizations, or those of the publisher, the editors and the reviewers. Any product that may be evaluated in this article, or claim that may be made by its manufacturer, is not guaranteed or endorsed by the publisher.
Author disclaimer
The contents of this abstract are the sole responsibility of the authors and do not necessarily reflect the views, opinions, or policies of the Department of Defense (DoD) or the United States.
Supplementary material
The Supplementary Material for this article can be found online at: https://www.frontiersin.org/articles/10.3389/fbrio.2023.1289461/full#supplementary-material
References
Baron S. D., Singh R., Metzger D. W. (2007). Inactivated Francisella tularensis live vaccine strain protects against respiratory tularemia by intranasal vaccination in an immunoglobulin A-dependent fashion. Infect. Immun. 75 (5), 2152–2162. doi: 10.1128/IAI.01606-06
Burke D. S. (1977). Immunization against tularemia: analysis of the effectiveness of live Francisella tularensis vaccine in prevention of laboratory-acquired tularemia. J. Infect. Diseases. 135 (1), 55–60. doi: 10.1093/infdis/135.1.55
Carlsson H. E., Lindberg A. A., Lindberg G., Hederstedt B., Karlsson K.-A., Agell B. O. (1979). Enzyme-linked immunosorbent assay for immunological diagnosis of human tularemia. J. Clin. Microbiol. 10 (5), 615–621. doi: 10.1128/jcm.10.5.615-621.1979
Chernecky C. C. B. B. (2013). Laboratory Tests and Diagnostic Procedures. Ed. Chernecky C. C. B. B. (Saint Louis, MO: Elsevier Saunders), 1052–1135.
De Pascalis R., Chou A. Y., Bosio C. M., Huang C.-Y., Follmann D. A., Elkins K. L. (2012). Development of functional and molecular correlates of vaccine-induced protection for a model intracellular pathogen, F. tularensis LVS. PLoS pathogens 8, 1. doi: 10.1371/journal.ppat.1002494
Dennis D. T., Inglesby T. V., Henderson D. A., Bartlett J. G., Ascher M. S., Eitzen E., et al. (2001). Tularemia as a biological weapon: medical and public health management. JAMA. 285 (21), 2763–2773. doi: 10.1001/jama.285.21.2763
Drabick J. J., Narayanan R. B., Williams J. C., Leduc J. W., Nacy C. A. (1994). Passive protection of mice against lethal Francisella tularensis (live tularemia vaccine strain) infection by the sera of human recipients of the live tularemia vaccine. Am. J. Med. Sci. 308 (2), 83–87. doi: 10.1097/00000441-199408000-00003
Eigelsbach H. T., Downs C. M. (1961). Prophylactic effectiveness of live and killed tularemia vaccines. I. Production of vaccine and evaluation in the white mouse and Guinea pig. J. Immunol. 87, 415–425. doi: 10.4049/jimmunol.87.4.415
Eigelsbach H. T., Tulis J. J., McGavran M. H., White J. D. (1962). LIVE TULAREMIA VACCINE I. : host-parasite relationship in monkeys vaccinated intracutaneously or aerogenically. J. Bacteriol. 84 (5), 1020–1027. doi: 10.1128/jb.84.5.1020-1027.1962
Eneslätt K., Golovliov I., Rydén P., Sjöstedt A. (2018). Vaccine-mediated mechanisms controlling replication of Francisella tularensis in human peripheral blood mononuclear cells using a co-culture system. Front. Cell. infection Microbiol. 8, 27. doi: 10.3389/fcimb.2018.00027
Forestal C. A., Malik M., Catlett S. V., Savitt A. G., Benach J. L., Sellati T. J., et al. (2007). Francisella tularensis has a significant extracellular phase in infected mice. J. Infect. Dis. 196 (1), 134–137. doi: 10.1086/518611
Fortier A. H., Slayter M., Ziemba R., Meltzer M., Nacy C. (1991). Live vaccine strain of Francisella tularensis: infection and immunity in mice. Infection immunity. 59 (9), 2922–2928. doi: 10.1128/iai.59.9.2922-2928.1991
Foshay L. (1932). Tularemia: Accurate and earlier diagnosis by means of the intradermal reaction. J. Infect. Diseases. , 286–291. doi: 10.1093/infdis/51.2.286
Francis E. (1919). Deer-fly fever, or Pahvant Valley Plague. A disease of man of hitherto unknown etiology. Public Health Rep. 34, 2061–2062. doi: 10.2307/4575306
Franz B. J., Li Y., Bitsaktsis C., Iglesias B. V., Pham G., Sunagar R., et al. (2015). Downmodulation of vaccine-induced immunity and protection against the intracellular bacterium Francisella tularensis by the inhibitory receptor FcgammaRIIB. J. Immunol. Res. 2015, 840842. doi: 10.1155/2015/840842
Golovliov I., Twine S. M., Shen H., Sjostedt A., Conlan W. (2013a). A ΔclpB mutant of Francisella tularensis subspecies holarctica strain, FSC200, is a more effective live vaccine than F. tularensis LVS in a mouse respiratory challenge model of tularemia. PloS One 8 (11), e78671. doi: 10.1371/journal.pone.0078671
Golovliov I., Twine S. M., Shen H., Sjostedt A., Conlan W. (2013b). A DeltaclpB mutant of Francisella tularensis subspecies holarctica strain, FSC200, is a more effective live vaccine than F. tularensis LVS in a mouse respiratory challenge model of tularemia. PloS One 8 (11), e78671. doi: 10.1371/journal.pone.0078671
Hartley G., Taylor R., Prior J., Newstead S., Hitchen P. G., Morris H. R., et al. (2006). Grey variants of the live vaccine strain of Francisella tularensis lack lipopolysaccharide O-antigen, show reduced ability to survive in macrophages and do not induce protective immunity in mice. Vaccine. 24 (7), 989–996. doi: 10.1016/j.vaccine.2005.08.075
Hornick R. B., Eigelsbach H. T. (1966). Aerogenic immunization of man with live tularemia vaccine. Bacteriological Rev. 30 (3), 532–538. doi: 10.1128/br.30.3.532-538.1966
Jacobs R. F. (1998). Harrison’s Principles of Internal Medicine. Eds. Fauci A. S. B. E., Isselbacher K. J., et al. (New York, NY: McGraw Hill Professional), 971.
Jemski J. V. (1981). Respiratory tularemia: comparison of selected routes of vaccination in Fischer 344 rats. Infection Immunity. 34 (3), 766–772. doi: 10.1128/iai.34.3.766-772.1981
Kirimanjeswara G. S., Golden J. M., Bakshi C. S., Metzger D. W. (2007). Prophylactic and therapeutic use of antibodies for protection against respiratory infection with Francisella tularensis. J. Immunol. 179 (1), 532–539. doi: 10.4049/jimmunol.179.1.532
Kirimanjeswara G. S., Olmos S., Bakshi C. S., Metzger D. W. (2008). Humoral and cell-mediated immunity to the intracellular pathogen Francisella tularensis. Immunol. Rev. 225, 244–255. doi: 10.1111/j.1600-065X.2008.00689.x
Klimpel G. R., Eaves-Pyles T., Moen S. T., Taormina J., Peterson J. W., Chopra A. K., et al. (2008). Levofloxacin rescues mice from lethal intra-nasal infections with virulent Francisella tularensis and induces immunity and production of protective antibody. Vaccine. 26 (52), 6874–6882. doi: 10.1016/j.vaccine.2008.09.077
Koskela P., Herva E. (1982). Cell-mediated and humoral immunity induced by a live Francisella tularensis vaccine. Infect. Immun. 36 (3), 983–989. doi: 10.1128/iai.36.3.983-989.1982
Kostiala A., McGregor D., Logie P. S. (1975). Tularaemia in the rat. I. The cellular basis on host resistance to infection. Immunology. 28 (5), 855.
Kurtz S. L., Voskanian-Kordi A., Simonyan V., Elkins K. L. (2018). Sequence comparison of Francisella tularensis LVS, LVS-G and LVS-R. Pathog. Dis. 76 (7). doi: 10.1093/femspd/fty067
Lam S. T., Sammons-Jackson W., Sherwood J., Ressner R. (2012). Laboratory-acquired tularemia successfully treated with ciprofloxacin: a case report. Infect. Dis. Clin. Practice. 20 (3), 204–207. doi: 10.1097/IPC.0b013e318234c383
Lindgren H., Eneslätt K., Golovliov I., Gelhaus C., Rydén P., Wu T., et al. (2020). Vaccine-mediated mechanisms controlling Francisella tularensis SCHU S4 growth in a rat co-culture system. Pathogens. 9 (5), 338. doi: 10.3390/pathogens9050338
Massey E. D., Mangiafico J. A. (1974). Microagglutination test for detecting and measuring serum agglutinins of Francisella tularensis. Appl. Microbiol. 27 (1), 25–27. doi: 10.1128/am.27.1.25-27.1974
McCrumb J. (1962). Commission on Epidemiological Survey. Review of tularemia: Studies on tularemia vaccine 1960–62. Annu. Rep., 81–86.
Metzger J. (1965). Tularemia vaccine, live, attenuated. Att. IND 157. Folder 2, Med Div Archives (USAMRIID). File 70IN.
Mulligan M. J., Stapleton J. T., Keitel W. A., Frey S. E., Chen W. H., Rouphael N., et al. (2017a). Tularemia vaccine: Safety, reactogenicity, "Take" skin reactions, and antibody responses following vaccination with a new lot of the Francisella tularensis live vaccine strain - A phase 2 randomized clinical Trial. Vaccine. 35 (36), 4730–4737. doi: 10.1016/j.vaccine.2017.07.024
Mulligan M. J., Stapleton J. T., Keitel W. A., Frey S. E., Chen W. H., Rouphael N., et al. (2017b). Tularemia vaccine: Safety, reactogenicity,”Take” skin reactions, and antibody responses following vaccination with a new lot of the Francisella tularensis live vaccine strain–A phase 2 randomized clinical Trial. Vaccine. 35 (36), 4730–4737. doi: 10.1016/j.vaccine.2017.07.024
Overholt E. L., Tigertt W. D., Kadull P. J., Ward M. K., Charkes N. D., Rene R. M., et al. (1961). An analysis of forty-two cases of laboratory-acquired tularemia. Treatment with broad spectrum antibiotics. Am. J. Med. 30, 785–806. doi: 10.1016/0002-9343(61)90214-5
Rawool D. B., Bitsaktsis C., Li Y., Gosselin D. R., Lin Y., Kurkure N. V., et al. (2008). Utilization of Fc receptors as a mucosal vaccine strategy against an intracellular bacterium, Francisella tularensis. J. Immunol. 180 (8), 5548–5557. doi: 10.4049/jimmunol.180.8.5548
Rhinehart-Jones T. R., Fortier A. H., Elkins K. L. (1994). Transfer of immunity against lethal murine Francisella infection by specific antibody depends on host gamma interferon and T cells. Infect. Immun. 62 (8), 3129–3137. doi: 10.1128/iai.62.8.3129-3137.1994
Rotem S., Cohen O., Bar-Haim E., Bar-On L., Ehrlich S., Shafferman A. (2014). Protective immunity against lethal F. tularensis holarctica LVS provided by vaccination with selected novel CD8+ T cell epitopes. PloS One 9 (1), e85215.
Rusnak J. M., Kortepeter M. G., Hawley R. J., Anderson A. O., Boudreau E., Eitzen E. (2004). Risk of occupationally acquired illnesses from biological threat agents in unvaccinated laboratory workers. Biosecur. bioterrorism: biodefense strategy practice science. 2 (4), 281–293. doi: 10.1089/bsp.2004.2.281
Salomonsson E., Kuoppa K., Forslund A.-L., Zingmark C., Golovliov I., Sjöstedt A., et al. (2009). Reintroduction of two deleted virulence loci restores full virulence to the live vaccine strain of Francisella tularensis. Infection immunity. 77 (8), 3424–3431. doi: 10.1128/IAI.00196-09
Sanders C., Haun R. (1968). Analysis of 106 cases of tularemia. J. Louisiana State Med. Soc 120, 391–393.
Saslaw S., Carhart S. (1961). Studies with tularemia vaccines in volunteers. III. Serologic aspects following intracutaneous or respiratory challenge in both vaccinated and nonvaccinated volunteers. Am. J. Med. Sci. 241, 689–699. doi: 10.1097/00000441-196106000-00001
Saslaw S., Eigelsbach H. T., Prior J. A., Wilson H. E., Carhart S. (1961). Tularemia vaccine study: II. Respiratory challenge. Arch. Internal Med. 107 (5), 702–714.
Sato T., Fujita H., Ohara Y., Homma M. (1990). Microagglutination test for early and specific serodiagnosis of tularemia. J. Clin. Microbiol. 28 (10), 2372–2374. doi: 10.1128/jcm.28.10.2372-2374.1990
Sawyer W., Tigertt W., Crozier D. (1962). Annual Progress Report – United States Army Medical Unit FY1962, Vol. 153. Fort Detrick, MD; US Army Office of the Surgeon General
Shoudy L. E., Namjoshi P., Giordano G., Kumar S., Bowling J. D., Gelhaus C., et al. (2021). The O-ag antibody response to francisella is distinct in rodents and higher animals and can serve as a correlate of protection. Pathogens. 10 (12), 1646. doi: 10.3390/pathogens10121646
Stanek S. M., Saunders D. L. (Eds.) (2020). USAMRIID's medical management of biological casualties handbook, 9th Edition (Washington, DC: US Government Printing Office).
Steiner D. J., Furuya Y., Metzger D. W. (2014). Host–pathogen interactions and immune evasion strategies in Francisella tularensis pathogenicity. Infection Drug resistance. 7, 239.
Stewart and G., ASTMH (1967). Natural Nidality of Transmissible Diseases: by Evegeny N. Pavlovsky, edited by Norman D. Levine, translated by Frederick K. Plous. University of Illinois Press, Urbana and London, (1966)
Sunagar R., Kumar S., Franz B. J., Gosselin E. J. (2016). Tularemia vaccine development: paralysis or progress? Vaccine: Dev. Ther. 6, 9.
Syrjälä H., Herva E., Ilonen J., Saukkonen K., Salminen A. (1984). A whole-blood lymphocyte stimulation test for the diagnosis of human tularemia. J. Infect. Diseases. 150 (6), 912–915. doi: 10.1093/infdis/150.6.912
Syrjälä H., Koskela P., Ripatti T., Salminen A., Herva E. (1986). Agglutination and ELISA methods in the diagnosis of tularemia in different clinical forms and severities of the disease. J. Infect. diseases. 153 (1), 142–145. doi: 10.1093/infdis/153.1.142
Tärnvik A., Löfgren M., Löfgren S., Sandström G., Wolf-Watz H. (1985). Long-lasting cell-mediated immunity induced by a live Francisella tularensis vaccine. J. Clin. Microbiol. 22 (4), 527–530. doi: 10.1128/jcm.22.4.527-530.1985
US Centers for Disease Control and Prevention (2020) Key facts about tularemia. can tularemia be used as a weapon? Available at: https://emergency.cdc.gov/agent/tularemia/facts.asp.
Van Metre T. E. Jr., Kadull P. J. (1959). Laboratory-acquired tularemia in vaccinated individuals: a report of 62 cases. Ann. Intern. Med. 50 (3), 621–632.
Viljanen M. K., Nurmi T., Salminen A. (1983). Enzyme-linked immunosorbent assay (ELISA) with bacterial sonicate antigen for IgM, IgA, and IgG antibodies to Francisella tularensis: comparison with bacterial agglutination test and ELISA with lipopolysaccharide antigen. J. Infect. diseases. 148 (4), 715–720. doi: 10.1093/infdis/148.4.715
Williams M. S., Baker M. R., Guina T., Hewitt J. A., Lanning L., Hill H., et al. (2019). Retrospective analysis of pneumonic tularemia in operation whitecoat human subjects: disease progression and tetracycline efficacy. Front. Med. (Lausanne). 6, 229. doi: 10.3389/fmed.2019.00229
Keywords: tularemia, vaccine, immunogenicity, safety, clinical trial
Citation: Saunders DL, Pierson BC, Haller J, Norris S, Cardile AP, Reisler RB, Okwesili AC, Boudreau E, Rusnak J, Danner DK, Purcell BK, Barth JF, Tompkins EL, Downs IL, Liggett D, Pettit P, Pratt T, Goldberg M, Kortepeter MG, Guerena FB, Aldis JW, Keshtkar-Jahromi M and Pittman PR (2024) Longitudinal phase 2 clinical trials of live, attenuated tularemia vaccine in healthy research laboratory workers. Front. Bacteriol. 2:1289461. doi: 10.3389/fbrio.2023.1289461
Received: 06 September 2023; Accepted: 29 November 2023;
Published: 03 January 2024.
Edited by:
Myron Christodoulides, University of Southampton, United KingdomReviewed by:
Kurt Henry Piepenbrink, University of Nebraska-Lincoln, United StatesLydia Marie Roberts, National Institute of Allergy and Infectious Diseases (NIH), United States
Copyright © 2024 Saunders, Pierson, Haller, Norris, Cardile, Reisler, Okwesili, Boudreau, Rusnak, Danner, Purcell, Barth, Tompkins, Downs, Liggett, Pettit, Pratt, Goldberg, Kortepeter, Guerena, Aldis, Keshtkar-Jahromi and Pittman. This is an open-access article distributed under the terms of the Creative Commons Attribution License (CC BY). The use, distribution or reproduction in other forums is permitted, provided the original author(s) and the copyright owner(s) are credited and that the original publication in this journal is cited, in accordance with accepted academic practice. No use, distribution or reproduction is permitted which does not comply with these terms.
*Correspondence: David L. Saunders, ZGF2aWQuc2F1bmRlcnNAdXN1aHMuZWR1
†Deceased