- 1Division of Fostering Required Medical Human Resources, Center for Infectious Disease Education and Research (CiDER), Osaka University, Osaka, Japan
- 2Department of Transformative Protection to Infectious Disease, Graduate School of Medicine, Osaka University, Osaka, Japan
- 3Department of Infection Control and Prevention, Graduate School of Medicine, Osaka University, Osaka, Japan
- 4Department of Transformative Analysis for Human Specimen, Graduate School of Medicine, Osaka University, Osaka, Japan
- 5Division of Infection Control and Prevention, Osaka University Hospital, Osaka University, Osaka, Japan
- 6Osaka Institute of Public Health, Osaka, Japan
Streptococcus pneumoniae (S. pneumoniae) causes otitis media, pneumonia, and invasive pneumococcal diseases (IPDs) such as meningitis and septicemia in humans. IPDs are fatal in children and can cause irreversible sequelae such as brain damage and impaired hearing. The introduction of 7- and 13-valent pneumococcal, conjugate, polysaccharide-based vaccines (PCV7, Prevnar 7®; and PCV13, Prevnar 13®) has decreased pediatric IPD infections. However, PCV13 provides limited protection against S. pneumoniae serotype 3. A new candidate pneumococcal vaccine antigen, pneumococcal surface protein A (PspA), which is found in almost all S. pneumoniae serotypes, addresses the drawbacks of CPS-based vaccines. In a previous study, the PspA3 + 2 protein was developed as a broad-spectrum vaccine candidate that combines PspA clades 2 and 3. We assessed whether vaccinating pregnant mice with PspA3 + 2 would transfer anti-PspA3 + 2 antibodies to pups, and if so, whether the transferred antibodies would protect against bacteremia caused by S. pneumoniae serotype 3. A PspA3 + 2 vaccine containing aluminum hydroxide gel (alum) and cytosine-phosphate-guanosine oligodeoxynucleotide K3 adjuvants induced anti-PspA antibodies in adult female mice, and an enzyme-linked immunosorbent assay revealed anti-PspA antibodies in serum samples from their offspring. Survival rates after lethal infection with S. pneumoniae serotype 3 were significantly higher among these neonates than in negative controls. These findings suggest that anti-PspA3 + 2 antibodies transferred from maternal mice vaccinated with PspA3 + 2 protect against bacteremia caused by S. pneumoniae serotype 3 in newborn pups.
1 Introduction
Streptococcus pneumoniae (S. pneumoniae) causes otitis media, pneumonia, and invasive pneumococcal diseases (IPDs) such as meningitis and septicemia in humans. Pneumococcal meningitis in children is a threatening infectious disease that can be fatal and has sequelae such as brain damage and impaired hearing (Baraff et al., 1993; Jit, 2010; Shinjoh et al., 2014; Wahl et al., 2018; El Kareh et al., 2020).
The 7- and 13-valent pneumococcal conjugate vaccines (PCV7, Prevnar7®; and PCV13, Prevnar 13®), which use capsular polysaccharide (CPS) as a vaccine antigen, were introduced into Japan’s routine vaccination program for children in 2010 and 2013, respectively. A comparison of the number of pediatric pneumococcal meningitis cases in Japan before (2008-2012) and after (2014-2016) the introduction of routine PCV7 vaccination revealed a 70.3% decrease (Iwata et al., 2021). However, in recent years, among 34 reported cases of pediatric pneumococcal meningitis post-PCV13 introduction, the majority (30/34) were caused by non-PCV13 serotypes. Notably, all cases with sequelae (6 cases) or fatal outcomes (4 cases) were attributable to non-PCV13 serotypes (Kurihara et al., 2022). Furthermore, 9 of the 34 S. pneumoniae isolates that caused pediatric pneumococcal meningitis were penicillin-resistant and belonged to non-PCV13 serotypes (Kurihara et al., 2022). These findings highlight the increasing incidence of infections caused by non-PCV13 pneumococcal serotypes and the emergence of antibiotic-resistant S. pneumoniae as critical clinical concerns (Iwata et al., 2021; Kurihara et al., 2022).
Thus, new CPS-based vaccines have been developed, such as the 15-valent pneumococcal conjugate vaccine (PCV15, VAXNEUVANCE®) and 20-valent pneumococcal conjugate vaccine (PCV20, Prevnar 20®) (Peterson et al., 2019; Hurley et al., 2021). The trend of pediatric IPDs caused by serotypes not covered by PCV13 has continued. Therefore, routine pneumococcal vaccination with PCV13 transitioned to PCV15 and PCV20 in Japan during 2024 (Suga et al., 2015; Kurihara et al., 2022). However, CPS is the target of the vaccine, which is associated with the risk that serotype substitution could result in the emergence of non-target serotypes. In addition to serotype replacement, the effectiveness of PCV13 against S. pneumoniae serotype 3 might be limited (Groves et al., 2019; Domínguez et al., 2017; Azarian et al., 2018; Deceuninck et al., 2023; Calvo-Silveria et al., 2024; Silva-Costa et al., 2022). S. pneumoniae serotype 3 has thicker capsules than do other serotypes (except serotype 37), and despite being covered by PCV13, it remains a major cause of pneumococcal infectious diseases (Luck et al., 2020).
The proportion of diseases caused by S. pneumoniae serotype 3 has notably not decreased, despite serotype 3 CPS being included in PCV13 (Azarian et al., 2018; Katoh et al., 2017; Silva-Costa et al., 2022; Wijayasri et al., 2019; Lansbury et al., 2024; Calvo-Silveria et al., 2024). Pneumococcal pneumonia with pleural effusion or pulmonary edema caused by S. pneumoniae serotype 3 remains a significant concern in Germany, where routine PCV13 vaccination for children has been implemented (Goettler et al., 2020). S. pneumoniae serotype 3 has caused more septic shock than have other serotypes in Sweden (Ahl et al., 2013). Major cardiovascular adverse events are more frequent among Colombian patients with IPDs caused by S. pneumoniae serotype 3 (Africano et al., 2021). The limited effects of PCV13 against S. pneumoniae serotype 3 have been attributed to serotype 3 CPS not being covalently attached to the bacteria surface, which allows it to shed (Cartee et al., 2005; Choi et al., 2016). Consequently, more anti-CPS antibodies are required to protect against IPDs caused by S. pneumoniae serotype 3 than by other S. pneumoniae serotypes (Choi et al., 2016; Andrews et al., 2014).
Pneumococcal surface protein A (PspA) is a candidate antigen for a CPS-independent pneumococcal vaccine that can overcome the deficiencies of CPS-based vaccines (McDaniel et al., 1991; Tart et al., 1996; Xin et al., 2009; Piao et al., 2014; Fukuyama et al., 2015). PspA functions in evading host immune defenses by preventing killing via host-secreted lactoferrin, escaping destruction via neutrophil extracellular traps, inhibiting C3 complement deposition, and facilitating adhesion to glyceraldehyde-3-phosphate dehydrogenase (Hammerschmidt et al., 1999; Tu et al., 1999; Ren et al., 2003; Daniels et al., 2006; Hollingshead et al., 2006; Martinez et al., 2019; Park et al., 2021). PspA comprises the following five domains: a signal peptide, an α-helical highly charged domain (α-HD), a proline-rich domain (PRD), a choline-binding domain, and a short hydrophobic tail. The C-terminal 100 amino acids of the α-HD form a clade-defining region (CDR). PspA is classified into three families and six clades based on the amino acid sequence of its CDR (family 1, clades 1 and 2; family 2, clades 3–5; and family 3, clade 6) (Hollingshead et al., 2000). The PRD of PspA is classified into three groups, PRD1–3, according to its amino acid sequence (Mukerji et al., 2018). Approximately 98% of pneumococcal strains from adult patients with IPDs in Japan express PspA clades 1–4 (Chang et al., 2021). The PspA3 + 2 vaccine antigen was developed considering cross-reactivity, as a single PspA does not provide sufficient protection against lethal infection by S. pneumoniae of another PspA clade family (Darrieux et al., 2007; Piao et al., 2014; Genschmer et al., 2019). This vaccine combines the α-HDs and PRDs from TIGR4 (PspA clade 3/PDR 1) and WU2 (PspA clade 2/PDR 3) and has been shown to protect mice from lethal infection by S. pneumoniae expressing PspA clades 1–5 (Piao et al., 2014).
Preventing IPDs in children with undeveloped immune systems is challenging. We therefore hypothesized that vaccinating prenatal mice with PspA3 + 2 would generate anti-PspA3 + 2 immunoglobulin G (IgG) antibodies that could be transferred to neonates, thus providing protection against infection. Maternal antibodies induced by PspA-based vaccines have protected infant mice against pneumococcal infection (Katsurahara et al., 2008; Hotomi et al., 2011; Kono et al., 2011, Kono et al., 2023). In these studies, cholera toxin was used as an adjuvant for nasal immunization. While cholera toxin is a potent mucosal adjuvant, adverse effects have been reported (Lewis et al., 2009). In this study, unlike previous studies, we evaluated the protective efficacy of a PspA-based vaccine using an alternative adjuvant suitable for intramuscular (i.m.) administration. Specifically, we investigated the protective effect of a PspA3 + 2 vaccine formulated with aluminum salts, which are used in PCV13 and other vaccines, and cytosine-phosphate-guanine oligodeoxynucleotide (CpG-ODN) K3, a toll-like receptor ligand with confirmed tolerability (Verthelyi et al., 2001; Pfizer Inc., 2010; Ezoe et al., 2020; Otsuka et al., 2022) and ability to induce anti-PspA antibodies as an adjuvant (Piao et al., 2014; Yokota et al., 2023). The present study provides insights into the clinical applications of the PspA-based vaccine, including the use of CpG-ODN K3 adjuvants.
2 Material and methods
2.1 Bacterial strains
Streptococcus pneumoniae WU2 (serotype 3/PspA clade 2/PRD 3) was incubated at 37°C under a 5% CO2 atmosphere in trypticase soy agar with 5% sheep blood (TSA II; BD, Franklin Lakes, NJ, USA) (Hollingshead et al., 2000; Piao et al., 2014) and in Todd-Hewitt broth supplemented with 0.5% yeast extract (THYB; BD).
2.2 Recombinant PspA protein purification
The construction of the pET28a (+) vector encoding the PspA3 + 2 protein and the purification of recombinant PspA3 + 2 were performed as described previously (Piao et al., 2014; Yokota et al., 2023). Briefly, Escherichia coli BL21 was transformed with the pET28a (+) vector encoding PspA3 + 2 and cultured in LB medium (Merck, Darmstadt, Germany) supplemented with 50 μg/mL kanamycin and Overnight Express™ Autoinduction System 1 (Merck) according to the manufacturer’s instructions. The bacterial culture was centrifuged, and the cell pellet was washed with phosphate buffered saline (PBS) before being resuspended in PBS (pH 8.0) containing 10 mM imidazole (Nacalai Tesque, Inc., Kyoto, Japan) and a protease inhibitor cocktail (Merck). The cells were lysed via sonication, and the lysate was centrifuged to collect the supernatant, which was subsequently filtered through a 0.45-μm membrane filter (Merck). The filtrate was purified using a His-Trap HP column (Cytiva, Marlborough, MA, USA) installed on an NGC Quest 10 Chromatography System (Bio-Rad, Hercules, CA, USA). The PspA3 + 2 protein was collected, concentrated using an Amicon Ultra-15 10 K device (Merck), and further purified with a HiLoad 26/600 Superdex 200 pg column (Cytiva). PspA3 + 2 concentration was determined using a bicinchoninic acid protein assay kit (Takara Bio, Inc., Kusatsu, Japan). PspA3 + 2 was used for mouse immunization and enzyme-linked immunosorbent assay (ELISA).
2.3 ELISA for anti-PspA antibody
We performed ELISA based on previously described protocols (Morino et al., 2020; Kuroda et al., 2023). Ninety-six-well plates (Greiner Bio-One, Frickenhausen, Germany) with a carbonate–bicarbonate buffer (Fujifilm Wako Pure Chemical Corp., Osaka, Japan) were coated with 1 μg/mL recombinant PspA3 + 2. Mouse serum samples (mother mouse serum 1:400 initial dilution, pooled infant mouse serum 1: 200 initial dilution) and goat anti-mouse IgG horseradish peroxidase secondary antibody (1:10,000; Abcam, Cambridge, UK) were used in this assay. The optical density (OD) was measured at 450 nm using an ELISA plate reader (Thermo Fisher Scientific Inc., Waltham, MA, USA). Reciprocal log2 titers were determined based on the highest antibody dilution that registered an OD of 0.1.
2.4 Animal studies
Nine-to-twelve-week-old male adult BALB/c and five-week-old female adult BALB/c were obtained from Japan SLC, Inc. (Shizuoka, Japan). Male mice were only used for mating. After one week of housing, the female mice were immunized via i.m. injections of 5 μg alum (Fujifilm Wako Pure Chemical Corp.) and 2.5 μg CpG-ODN K3 (Ajinomoto Bio-Pharma Services Platform Technologies, Osaka, Japan) with or without (negative controls) recombinant PspA3 + 2 protein (0.1 μg) into their thigh muscles three times at 7-day intervals. The vaccinated and negative control female mice were mated, and newborn pups were housed under the same rearing conditions. Serum samples were collected from the postnatal mice (but not immediately before or after delivery), and from the pups on days 0 (birth day), 4, 9, and 14 starting from 1 week after the last vaccination to determine anti-PspA antibody titers. Serum samples from littermates were pooled according to their mother and date of birth (Table 1).We intraperitoneally (i.p.) injected 8-day-old (infant) mice born to seven immunized mice and five negative control mice with five colony-forming units of S. pneumoniae serotype 3. We then assessed survival rates 6 days later to determine the protective performance of the vaccine.
The Institutional Animal Care and Use Committee at the Graduate School of Medicine, Osaka University, Japan approved all the animal experiments (Approval ID: 04-021-005).
2.5 Statistical analysis
Quantitative data are presented as means and standard errors. Data were statistically analyzed using GraphPad Prism 8 (GraphPad Software Inc., Boston, MA, USA).
3 Results
3.1 Antibody titer of the PspA3 + 2 vaccine in immunized maternal mice
Maternal mice received three i.m. injections at 1-week intervals with alum and CpG-ODN K3 without (negative controls) or with (vaccinated) PspA3 + 2 (Figure 1A). Serum samples were obtained from the vaccinated and negative control mice at intervals of 21–100 days after the first immunization (Figure 1A). IgG antibody titers against the PspA3 + 2 protein in the sera of vaccinated and negative control mice were measured using ELISA and then compared using software (Figure 1B). The results revealed higher titers in the vaccinated mice than in the control mice.
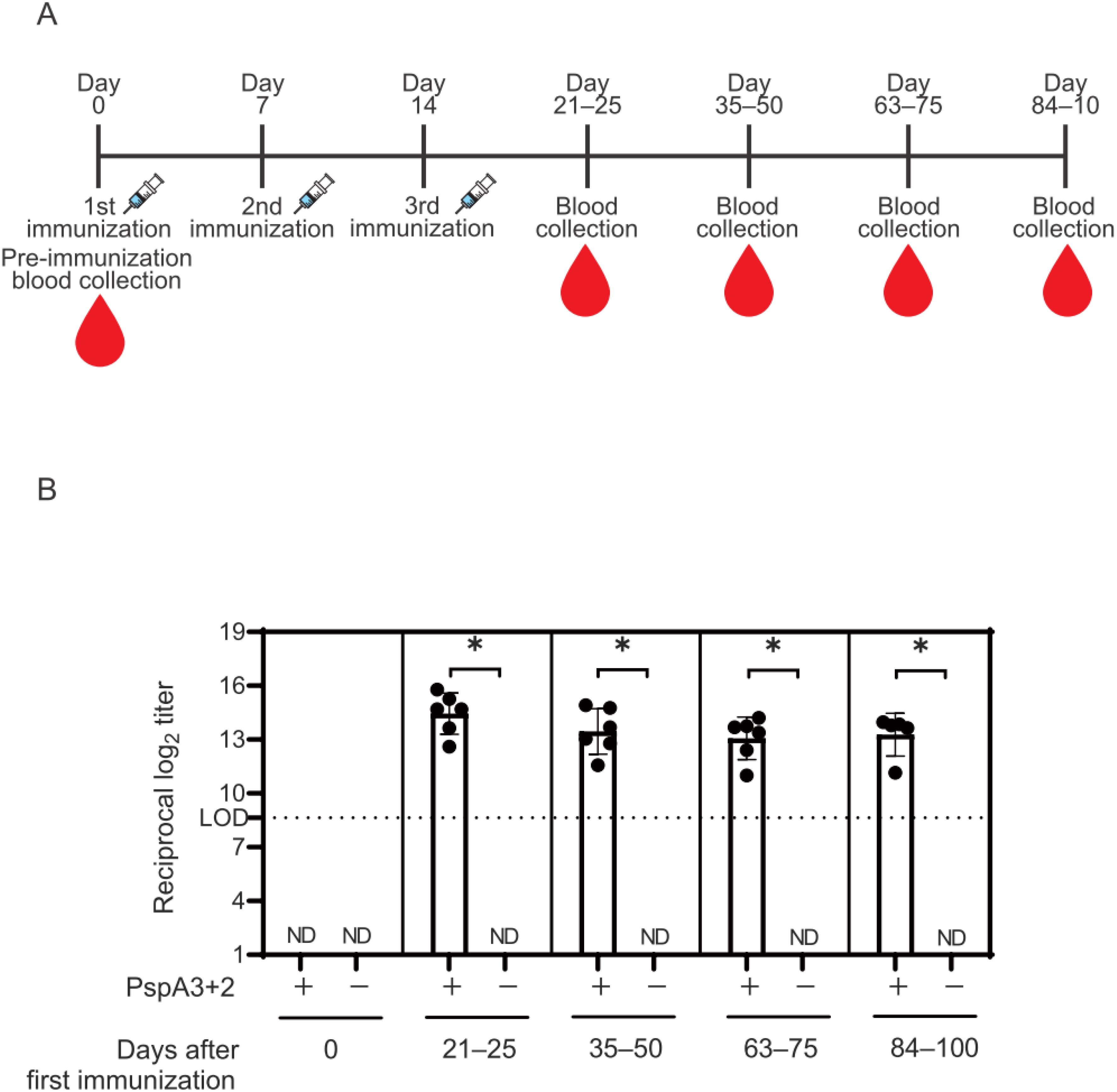
Figure 1. Titers of IgG antibodies against the PspA3 + 2 protein in the sera of prenatal mice. (A) Immunization schedule and blood collected from prenatal mice. Day 0: time point immediately before first immunization. (B) Titers of IgG antibodies against PspA3 + 2 in the sera of prenatal vaccinated (n = 6) and negative controls (n = 3) determined using ELISA. One prenatal mouse was missing between days 84–100 due to sampling error. The initial dilution of mouse serum used in the ELISA was 1:400. The limit of detection (LOD) was determined as log2(400) = 8.64 for 1:400. Error bars indicate standard error of the mean. ND, not detected. The IgG antibody titer of the mouse serum was measured in a single independent experiment. Statistically significant: *p < 0.05 (Mann-Whitney U-test). In the Mann-Whitney U-test, the negative controls were below the LOD; therefore, statistical analysis was performed using a twofold dilution of the initial serum dilution, log2(400/2)=7.64.
3.2 Antibody titers of offspring born to PspA3 + 2-vaccinated mice
We assessed anti-PspA antibody transfer from vaccinated mice to 0-, 4-, 9-, and 14-day-old offspring (Figure 2A). IgG antibody titers against the PspA3 + 2 protein in serum samples were analyzed using ELISA (Figure 2B). The results revealed significantly increased antibody titers in infants delivered by vaccinated mice compared with those in negative controls, excluding 0-day-old offspring (Figure 2B).
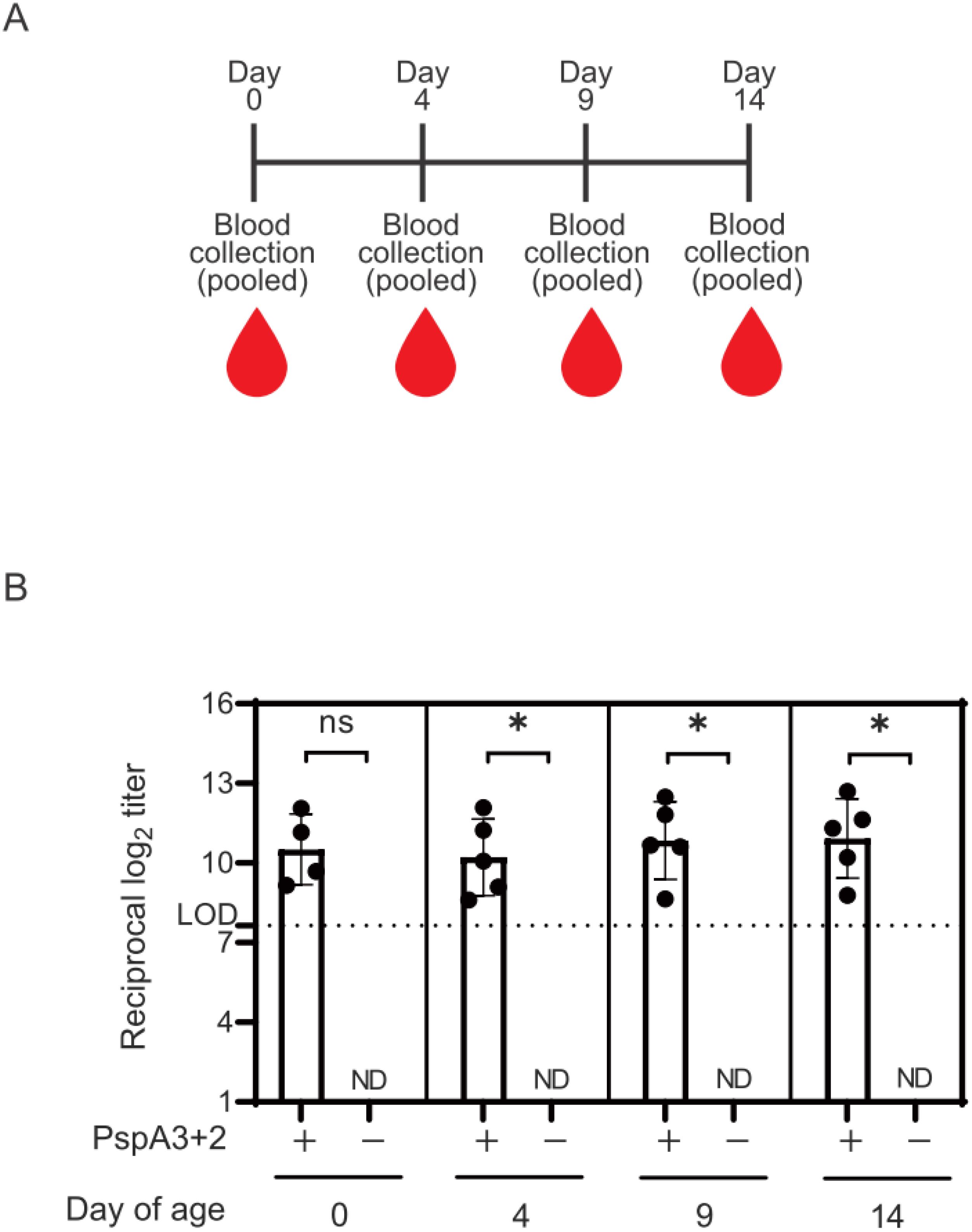
Figure 2. Titers of IgG antibodies against the PspA3 + 2 protein in the sera of infant mice. (A) Schedule of blood collection from infant mice. (B) Date of birth was taken as day 0. Titers of IgG antibodies against PspA3 + 2 in the sera of neonatal mice at birth and 4, 9, and 14 days thereafter determined using ELISA. Serum samples from littermates were pooled according to their mother and date of birth. The initial dilution of mouse serum used in the ELISA was 1:200. The limit of detection (LOD) was determined as log2(200) = 7.64 for an initial serum dilution of 1:200. Error bars indicate standard errors of means. ND, not detected. The IgG antibody titer of the mouse serum was measured in a single independent experiment. Statistically significant: *p < 0.05, non-significant (ns): p ≥ 0.05 (Mann-Whitney U-test). In the Mann-Whitney U-test, the negative controls were below the LOD; therefore, statistical analysis was performed using a twofold dilution of the initial serum dilution, log2(200/2)=6.64.
3.3 Ability of transferred IgG antibodies to protect infant mice against lethal S. pneumoniae serotype 3 infection
We investigated whether anti-PspA antibodies could protect infant mice against bacteremia caused by S. pneumoniae serotype 3 WU2 expressing PspA clade 2. We compared survival rates between infants born to vaccinated and unvaccinated (negative controls) mice administered lethal doses of S. pneumoniae WU2 i.p. Survival rates were significantly higher among infants born to the vaccinated mice than among the negative controls (54.3% vs. 18.2%; Figure 3).
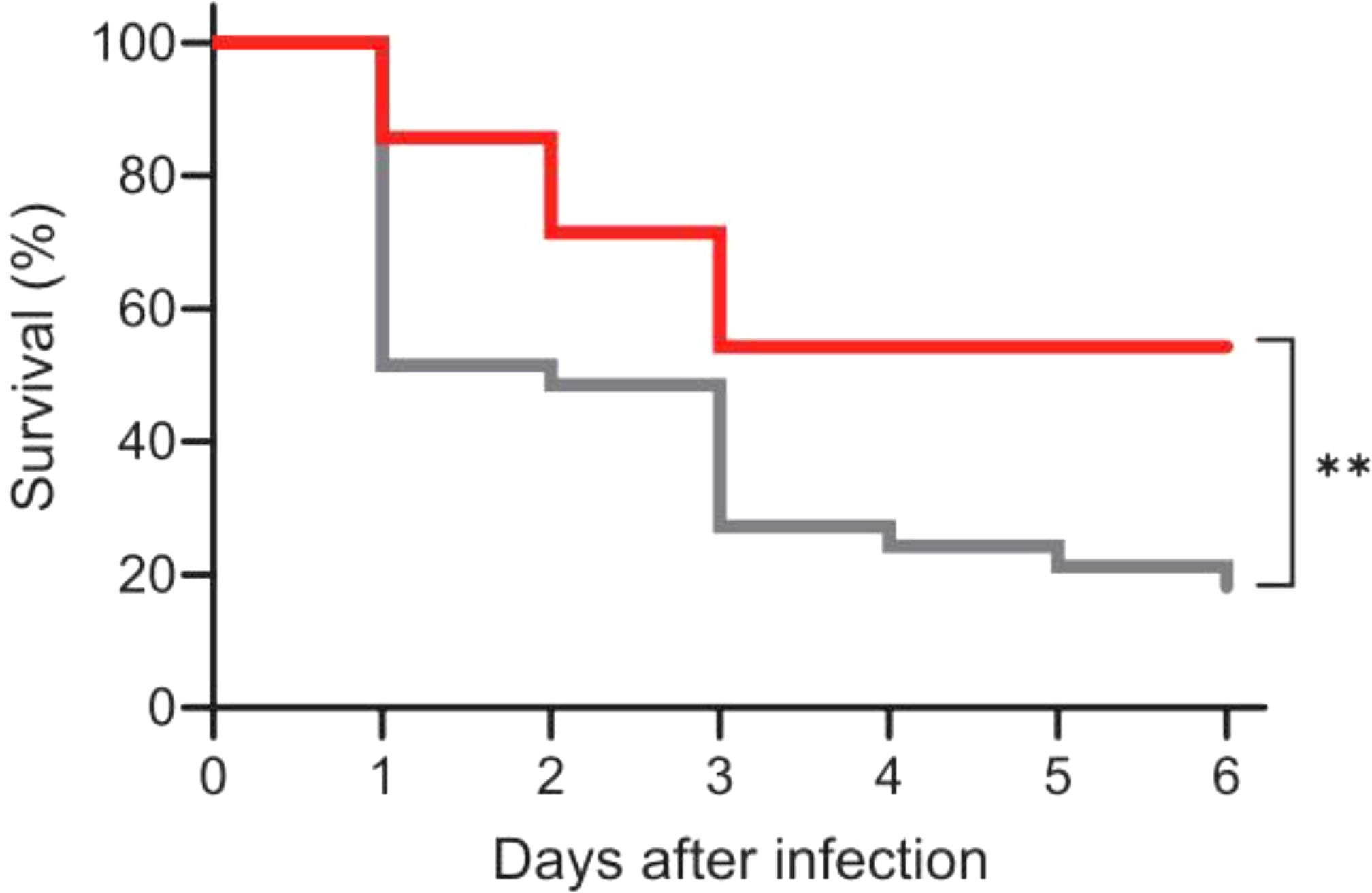
Figure 3. Protection against lethal infection by Streptococcus pneumoniae WU2 serotype 3 in mice with maternal anti-PspA3 + 2 antibodies. Eight-day-old mice were intraperitoneally injected with S. pneumoniae serotype 3 WU2, and the survival rates were monitored for 6 days. Red and gray lines indicate infant mice delivered by pregnant mice vaccinated with (n = 35) or without (negative controls; n = 33) PspA3 + 2, respectively. Comparison of survival rates of lethal S. pneumoniae serotype 3 WU2 infections between immunized and negative control mice. Statistically significant **p < 0.01 (log-rank test).
4 Discussion
We vaccinated pregnant mice with a recombinant PspA3 + 2 protein with alum and CpG-ODN K3 as adjuvants. Maternal anti-PspA3 + 2 antibodies were transferred to offspring, providing partial protection against fatal pneumococcal bacteremia caused by S. pneumoniae serotype 3.
In this study, the PspA3 + 2 vaccine consisted of 0.1 μg of PspA3 + 2, 2.5 μg of CpG-ODN K3, and 5 μg of alum administered via i.m. injection. This dosage and route of administration were selected based on previous studies in adult mice, in which they induced a robust antibody response and provided protection against lethal S. pneumoniae infection (Piao et al., 2014). Following a similar approach, we investigated the protective effect of maternal i.m. immunization with the PspA3 + 2 vaccine on offspring against S. pneumoniae infection. This study also aimed to evaluate an immunization strategy with potential applicability to humans. CpG-ODN K3 was chosen as an adjuvant in part due to its demonstrated tolerability in humans (Ezoe et al., 2020; Otsuka et al., 2022).
Previous studies on PspA-based vaccines have utilized antigen doses ranging from 1–20 μg and employed subcutaneous (s.c.) or intranasal (i.n.) administration (Vadesilho et al., 2014; Kuipers et al., 2015; Tamborrini et al., 2015; Converso et al., 2017; Goulart et al., 2017; Kono et al., 2023; Yokota et al., 2023). In this study, the antigen dose (0.1 μg) was considerably lower; therefore, increasing the antigen and adjuvant doses may enhance maternal antibody production, improving protection in offspring. S.c. and i.n. administration routes may also have potential for inducing immunity. Future studies comparing different doses, adjuvants, and administration routes will be necessary to further optimize the immunization strategy.
A potential limitation of this study is that the level of lipopolysaccharide (LPS) contamination in the recombinant protein preparations was not assessed. Although our purification steps, including affinity and size-exclusion chromatography, likely reduced LPS contamination, we cannot exclude the possibility that residual LPS influenced the experimental outcomes. In future studies, LPS removal strategies and quantitative assays, such as the Limulus amebocyte lysate assay, will be implemented to ensure that LPS contamination does not confound the results.
S. pneumoniae colonizes the nasopharynx of infants and young children, with rates of approximately 50% in Japan (Hashida et al., 2011; Chang et al., 2020; Otsuka et al., 2013) and 90% in Finland (Holmlund et al., 2006). Children naturally become sensitized to S. pneumoniae through routine PCV13 vaccination and nasopharyngeal carriage and gradually acquire antibodies against pneumococcus as they age. For example, the level of anti-PspA IgG is the lowest in infants aged 6–11 months and highest in preschool and school-aged children (Morino et al., 2020). However, children lack protective immunity during a vulnerable period before receiving PCV13 or natural sensitization. Since approximately 60% of mothers in developed countries breastfeed their offspring for 6 months, anti-PspA antibodies in breast milk and transferred via the placenta can protect against S. pneumoniae during this period (Hotomi et al., 2011; Victora et al., 2016).
In Japan, an investigation of 39 pediatric pneumococcal meningitis cases from 2014 to 2016 revealed that 34 were caused by non-PCV13 S. pneumoniae serotypes (34/39) (Iwata et al., 2021). Among these, 13 were attributable to PCV20-covered serotypes, 13 to non-PCV20 serotypes, and 8 to serotype 15 (15A, 15B, or 15C) (Iwata et al., 2021). These findings suggest that even with the introduction of PCV20, non-PCV20 serotypes of S. pneumoniae remain a threat. PCV20 is expected to be somewhat effective against serotype replacement; however, future vaccines should either include a broader range of serotypes or target antigens common to all S. pneumoniae strains. The development of PspA-based candidates for a universal pneumococcal vaccine is promising for controlling pneumococcal infections. Predicting the occurrence of bacteremia in children is challenging, but if maternally transferred antibodies can prevent severe pneumococcal bacteremia, then administering a PspA-based vaccine to prenatal women might prevent bacteremia in children.
Data availability statement
The datasets presented in this article are not readily available because they were obtained from third-party sources and are subject to ownership and distribution rights held by the original providers. The sources of these materials are cited in the manuscript. Requests to access the datasets should be directed to the corresponding author, and access will be facilitated by coordinating with the original providers as appropriate.
Ethics statement
The animal study was approved by The Institutional Animal Care and Use Committee at the Graduate School of Medicine, Osaka University. The study was conducted in accordance with the local legislation and institutional requirements.
Author contributions
EK: Conceptualization, Investigation, Writing – original draft, Writing – review & editing. YT: Data curation, Methodology, Writing – review & editing. SH: Conceptualization, Funding acquisition, Investigation, Project administration, Supervision, Writing – review & editing.
Funding
The author(s) declare that financial support was received for the research and/or publication of this article. This study was supported in part by the Japan Society for the Promotion of Science, KAKENHI (grant number 24K11651).
Acknowledgments
We are grateful to Dr. David E. Briles (University of Alabama; Birmingham, AL, USA) for providing S. pneumoniae WU2. This study was conducted as a part of The Nippon Foundation - Osaka University Project for Infectious Disease Prevention. We thank Editage (www.editage.com) for English language editing.
Conflict of interest
The authors declare that the research was conducted in the absence of any commercial or financial relationships that could be construed as a potential conflict of interest.
Generative AI statement
The author(s) declare that no Generative AI was used in the creation of this manuscript.
Publisher’s note
All claims expressed in this article are solely those of the authors and do not necessarily represent those of their affiliated organizations, or those of the publisher, the editors and the reviewers. Any product that may be evaluated in this article, or claim that may be made by its manufacturer, is not guaranteed or endorsed by the publisher.
References
Africano H. F., Serrano-Mayorga C. C., Ramirez-Valbuena P. C., Bustos I. G., Bastidas A., Vargas H. A., et al. (2021). Major adverse cardiovascular events during invasive pneumococcal disease are serotype dependent. Clin. Infect. Dis. 72, e711–e719. doi: 10.1093/cid/ciaa1427
Ahl J., Littorin N., Forsgren A., Odenholt I., Resman F., and Riesbeck K. (2013). High incidence of septic shock caused by Streptococcus pneumoniae serotype 3-a retrospective epidemiological study. BMC Infect. Dis. 13, 492. doi: 10.1186/1471-2334-13-492
Andrews N. J., Waight P. A., Burbidge P., Pearce E., Roalfe L., Zancolli M., et al. (2014). Serotype-specific effectiveness and correlates of protection for the 13-valent Pneumococcal Conjugate Vaccine: a postlicensure indirect cohort study. Lancet Infect. Dis. 14, 839–846. doi: 10.1016/S1473-3099(14)70822-9
Azarian T., Mitchell P. K., Georgieva M., Thompson C. M., Ghouila A., Pollard A. J., et al. (2018). Global emergence and population dynamics of divergent serotype 3 CC180 pneumococci. PloS Pathog. 14, e1007438. doi: 10.1371/journal.ppat.1007438
Baraff L. J., Lee S. I., and Schriger D. L. (1993). Outcomes of bacterial meningitis in children: a meta-analysis. Pediatr Infect Dis J 12, 389–94. doi: 10.1097/00006454-199305000-00008
Calvo-Silveria S., González-Díaz A., Grau I., Marimón J. M., Cercenado E., Quesada M. D., et al. (2024). Evolution of invasive pneumococcal disease by serotype 3 in adults: a Spanish three-decade retrospective study. Lancet Reg. Health Eur. 41, 100913. doi: 10.1016/j.lanepe.2024.100913
Cartee R. T., Forsee W. T., and Yother J. (2005). Initiation and synthesis of the Streptococcus pneumoniae type 3 capsule on a phosphatidylglycerol membrane anchor. J. Bacteriol. 187, 4470–4479. doi: 10.1128/JB.187.13.4470-4479.2005
Chang B., Akeda H., Nakamura Y., Hamabata H., Ameku K., Toma T., et al. (2020). Impact of thirteen-valent Pneumococcal Conjugate Vaccine on nasopharyngeal carriage in healthy children under 24 months in Okinawa, Japan. J. Infect. Chemother. 26, 465–470. doi: 10.1016/j.jiac.2019.12.009
Chang B., Kinjo Y., Morita M., Tamura K., Watanabe H., Tanabe Y., et al. (2021). Distribution and variation of serotypes and pneumococcal surface protein A clades of Streptococcus pneumoniae strains isolated from adult patients with invasive pneumococcal disease in Japan. Front. Cell. Infect. Microbiol. 11. doi: 10.3389/fcimb.2021.617573
Choi E. H., Zhang F., Lu Y. J., and Malley R. (2016). Capsular polysaccharide (CPS) release by serotype 3 pneumococcal strains reduces the protective effect of anti-type 3 CPS antibodies. Clin. Vaccine Immunol. 23, 162–167. doi: 10.1128/CVI.00591-15
Converso T. R., Goulart C., Darrieux M., and Leite L. C. C. (2017). A protein chimera including PspA in fusion with PotD is protective against invasive pneumococcal infection and reduces nasopharyngeal colonization in mice. Vaccine. 35, 5140–5147. doi: 10.1016/j.vaccine.2017.08.010
Daniels C. C., Briles T. C., Mirza S., Håkansson A. P., and Briles D. E. (2006). Capsule does not block antibody binding to PspA, a surface virulence protein of Streptococcus pneumoniae. Microb. Pathog. 40, 228–233. doi: 10.1016/j.micpath.2006.01.007
Darrieux M., Miyaji E. N., Ferreira D. M., Lopes L. M., Lopes A. P. Y., Ren B., et al. (2007). Fusion proteins containing family 1 and family 2 PspA fragments elicit protection against Streptococcus pneumoniae that correlates with antibody-mediated enhancement of complement deposition. Infect. Immun. 75, 5930–5938. doi: 10.1128/IAI.00940-07
Deceuninck G., Brousseau N., Lefebvre B., Quach C., Tapiero B., Bui Y. G., et al. (2023). Effectiveness of thirteen-valent Pneumococcal Conjugate Vaccine to prevent serotype 3 invasive pneumococcal disease in Quebec in children, Canada. Vaccine. 41, 5486–5489. doi: 10.1016/j.vaccine.2023.07.049
Domínguez Á., Ciruela P., Hernández S., García-García: J. J., Soldevila N., Izquierdo C., et al. (2017). Effectiveness of the 13-valent pneumococcal conjugate vaccine in preventing invasive pneumococcal disease in children aged 7-59 months. A matched case-control study. PloS One 12, e0183191. doi: 10.1371/journal.pone.0183191
El Kareh A., El Hage S., Safi S., Assouad E., Mokled E., and Salameh P. (2020). Epidemiology of bacterial meningitis in Lebanon from 2011 to 2019. J. Clin. Neurosci. 81, 32–36. doi: 10.1016/j.jocn.2020.09.011
Ezoe S., Palacpac N. M. Q., Tetsutani K., Yamamoto K., Okada K., Taira M., et al. (2020). First-in-human randomised trial and follow-up study of Plasmodium falciparum blood-stage malaria vaccine BK-SE36 with CpG-ODN(K3). Vaccine. 38, 7246–7257. doi: 10.1016/j.vaccine.2020.09.056
Fukuyama Y., Yuki Y., Katakai Y., Harada N., Takahashi H., Takeda S., et al. (2015). Nanogel-based pneumococcal surface protein A nasal vaccine induces microRNA-associated Th17 cell responses with neutralizing antibodies against Streptococcus pneumoniae in macaques. Mucosal Immunol. 8, 1144–1153. doi: 10.1038/mi.2015.5
Genschmer K. R., Vadesilho C. F. M., McDaniel L. S., Park S. S., Hale Y., Miyaji E. N., et al. (2019). The modified surface killing assay distinguishes between protective and nonprotective antibodies to PspA. mSphere. 4, e00589–e00519. doi: 10.1128/mSphere.00589-19
Goettler D., Streng A., Kemmling D., Schoen C., von Kries R., Rose M. A., et al. (2020). Increase in Streptococcus pneumoniae serotype 3 associated parapneumonic pleural effusion/empyema after the introduction of PCV13 in Germany. Vaccine. 38, 570–577. doi: 10.1016/j.vaccine.2019.10.056
Goulart C., Rodriguez D., Kanno A. I., Lu Y. J., Malley R., and Leite L. C. C. (2017). Recombinant BCG expressing a PspA-PdT fusion protein protects mice against pneumococcal lethal challenge in a prime-boost strategy. Vaccine. 35, 1683–1691. doi: 10.1016/j.vaccine.2017.02.029
Groves N., Sheppard C. L., Litt D., Rose S., Silva A., Njoku N., et al. (2019). Evolution of Streptococcus pneumoniae serotype 3 in England and Wales: a major vaccine evader. Genes. 10, 845. doi: 10.3390/genes10110845
Hammerschmidt S., Bethe G., Remane P. H., and Chhatwal G. S. (1999). Identification of pneumococcal surface protein A as a lactoferrin-binding protein of Streptococcus pneumoniae. Infect. Immun. 67, 1683–1687. doi: 10.1128/IAI.67.4.1683-1687.1999
Hashida K., Shiomori T., Hohchi N., Ohkubo J. I., Ohbuchi T., Mori T., et al. (2011). Nasopharyngeal Streptococcus pneumoniae carriage in Japanese children attending day-care centers. Int. J. Pediatr. Orl. 75, 664–669. doi: 10.1016/j.ijporl.2011.02.005
Hollingshead S. K., Baril L., Ferro S., King J., Coan P., Briles D. E., et al. (2006). Pneumococcal surface protein A (PspA) family distribution among clinical isolates from adults over 50 years of age collected in seven countries. J. Med. Microbiol. 55, 215–221. doi: 10.1099/jmm.0.46268-0
Hollingshead S. K., Becker R., and Briles D. E. (2000). Diversity of PspA: mosaic genes and evidence for past recombination in Streptococcus pneumoniae. Infect. Immun. 68, 5889–5900. doi: 10.1128/IAI.68.10.5889-5900.2000
Holmlund E., Quiambao B., Ollgren J., Nohynek H., and Käyhty H. (2006). Development of natural antibodies to pneumococcal surface protein A, pneumococcal surface adhesin A and pneumolysin in Filipino pregnant women and their infants in relation to pneumococcal carriage. Vaccine. 24, 57–65. doi: 10.1016/j.vaccine.2005.07.055
Hotomi M., Kono M., Hollingshead S. K., Briles D. E., and Yamanaka N. (2011). “Protection of pneumococcal infection by maternal intranasal immunization with pneumococcal surface protein A,” in Advances in oto-rhino-laryngology (Karger Publishers, Basel). doi: 10.1159/000324656
Hurley D., Griffin C., Young M. Jr., Scott D. A., Pride M. W., Scully I. L., et al. (2021). Safety, tolerability, and immunogenicity of a 20-valent Pneumococcal Conjugate Vaccine (PCV20) in adults 60 to 64 years of age. Clin. Infect. Dis. 73, e1489–e1497. doi: 10.1093/cid/ciaa1045
Iwata S., Takata M., Morozumi M., Miyairi I., Matsubara K., Ubukata K., et al. (2021). Drastic reduction in pneumococcal meningitis in children owing to the introduction of pneumococcal conjugate vaccines: Longitudinal analysis from 2002 to 2016 in Japan. J. Infect. Chemother. 27, 604–612. doi: 10.1016/j.jiac.2020.11.019
Jit M. (2010). The risk of sequelae due to pneumococcal meningitis in high-income countries: a systematic review and meta-analysis. J. Infect. 61, 114–124. doi: 10.1016/j.jinf.2010.04.008
Katoh S., Suzuki M., Ariyoshi K., and Morimoto K. (2017). Serotype replacement in adult pneumococcal pneumonia after the introduction of seven-valent Pneumococcal Conjugate Vaccines for children in Japan: a systematic literature review and pooled data analysis. Jpn. J. Infect. Dis. 70, 495–501. doi: 10.7883/yoken.JJID.2016.311
Katsurahara T., Hotomi M., Yamauchi K., Billal D. S., and Yamanaka N. (2008). Protection against systemic fatal pneumococcal infection by maternal intranasal immunization with pneumococcal surface protein A (PspA). J. Infect. Chemother. 14, 393–398. doi: 10.1007/s10156-008-0647-7
Kono M., Hotomi M., Hollingshead S. K., Briles D. E., and Yamanaka N. (2011). Maternal immunization with pneumococcal surface protein A protects against pneumococcal infections among derived offspring. PloS One 6, e27102. doi: 10.1371/journal.pone.0027102
Kono M., Iyo T., Murakami D., Sakatani H., Nanushaj D., and Hotomi M. (2023). Maternal immunization with pneumococcal surface protein A provides the immune memories of offspring against pneumococcal infection. Front. Cell. Infect. Microbiol. 13. doi: 10.3389/fcimb.2023.1059603
Kuipers K., Daleke-Schermerhorn M. H., Jong W. S. P., ten Hagen-Jongman C. M., van Opzeeland F., Simonetti E., et al. (2015). Salmonella outer membrane vesicles displaying high densities of pneumococcal antigen at the surface offer protection against colonization. Vaccine. 33, 2022–2029. doi: 10.1016/j.vaccine.2015.03.010
Kurihara E., Takeshita K., Tanaka S., Takeuchi N., Ohkusu M., Hishiki H., et al. (2022). Clinical and bacteriological analysis of pediatric pneumococcal meningitis after 13-valent Pneumococcal Conjugate Vaccine introduction in Japan. Microbiol. Spec. 10, e0182221. doi: 10.1128/spectrum.01822-21
Kuroda E., Koizumi Y., Piao Z., Nakayama H., Tomono K., Oishi K., et al. (2023). Establishment of a modified opsonophagocytic killing assay for anti-pneumococcal surface protein A antibody. J. Microbiol. Methods 212, 106804. doi: 10.1016/j.mimet.2023.106804
Lansbury L., Lawrence H., McKeever T. M., French N., Aston S., Hill A. T., et al. (2024). Pneumococcal serotypes and risk factors in adult community-acquired pneumonia 2018–20; a multicentre UK cohort study. Lancet Reg. Health Eur. 37, 100812. doi: 10.1016/j.lanepe.2023.100812
Lewis D. J. M., Huo Z., Barnett S., Kromann I., Giemza R., Galiza E., et al. (2009). Transient facial nerve paralysis (Bell’s palsy) following intranasal delivery of a genetically detoxified mutant of Escherichia coli heat labile toxin. PloS One 4, , e6999. doi: 10.1371/journal.pone.0006999
Luck J. N., Tettelin H., and Orihuela C. J. (2020). Sugar-coated killer: serotype 3 pneumococcal disease. Front. Cell. Infect. Microbiol. 10. doi: 10.3389/fcimb.2020.613287
Martinez P. J., Farhan A., Mustafa M., Javaid N., Darkoh C., Garrido-Sanabria E., et al. (2019). PspA facilitates evasion of pneumococci from bactericidal activity of neutrophil extracellular traps (NETs). Microb. Pathog. 136, 103653. doi: 10.1016/j.micpath.2019.103653
McDaniel L. S., Sheffield J. S., Delucchi P., and Briles D. E. (1991). PspA, a surface protein of Streptococcus pneumoniae, is capable of eliciting protection against pneumococci of more than one capsular type. Infect. Immun. 59, 222–228. doi: 10.1128/iai.59.1.222-228.1991
Morino S., Kitagami E., Nakayama H., Koizumi Y., Tanaka-Taya K., Kinjo Y., et al. (2020). Seroepidemiological analysis of anti-pneumococcal surface protein A (PspA) immunoglobulin G by clades in Japanese population. Vaccine. 38, 7479–7484. doi: 10.1016/j.vaccine.2020.09.068
Mukerji R., Hendrickson C., Genschmer K. R., Park S. S., Bouchet V., Goldstein R., et al. (2018). The diversity of the proline-rich domain of pneumococcal surface protein A (PspA): Potential relevance to a broad-spectrum vaccine. Vaccine. 36, 6834–6843. doi: 10.1016/j.vaccine.2018.08.045
Otsuka T., Chang B., Shirai T., Iwaya A., Wada A., Yamanaka N., et al (2013). Individual risk factors associated with nasopharyngeal colonization with Streptococcus pneumoniae and Haemophilus influenzae: a Japanese birth cohort study. Pediatr. Infect. Dis. J. 32, 709–714. doi: 10.1097/INF.0b013e31828701ea
Otsuka T., Nishida S., Shibahara T., Temizoz B., Hamaguchi M., Shiroyama T., et al. (2022). CpG ODN (K3)-Toll-like receptor 9 agonist-induces Th1-type immune response and enhances cytotoxic activity in advanced lung cancer patients: a phase I study. BMC Cancer. 22, 744. doi: 10.1186/s12885-022-09818-4
Park S. S., Gonzalez-Juarbe N., Riegler A. N., Im H., Hale Y., Platt M. P., et al. (2021). Streptococcus pneumoniae binds to host GAPDH on dying lung epithelial cells worsening secondary infection following influenza. Cell Rep. 35, 109267. doi: 10.1016/j.celrep.2021.109267
Peterson J. T., Stacey H. L., MacNair J. E., Li J., Hartzel J. S., Sterling T. M., et al. (2019). Safety and immunogenicity of 15-valent Pneumococcal Conjugate Vaccine compared to 13-valent Pneumococcal Conjugate Vaccine in adults ≥ 65 years of age previously vaccinated with 23-valent pneumococcal polysaccharide vaccine. Hum. Vaccin. Immunother. 15, 540–548. doi: 10.1080/21645515.2018.1532250
Piao Z., Akeda Y., Takeuchi D., Ishii K. J., Ubukata K., Briles D. E., et al. (2014). Protective properties of a fusion pneumococcal surface protein A (PspA) vaccine against pneumococcal challenge by five different PspA clades in mice. Vaccine. 32, 5607–5613. doi: 10.1016/j.vaccine.2014.07.108
Ren B., Szalai A. J., Thomas O., Hollingshead S. K., and Briles D. E. (2003). Both family 1 and family 2 PspA proteins can inhibit complement deposition and confer virulence to a capsular serotype 3 strain of Streptococcus pneumoniae. Infect. Immun. 71, 75–85. doi: 10.1128/IAI.71.1.75-85.2003
Shinjoh M., Iwata S., Yagihashi T., Sato Y., Akita H., Takahashi T., et al. (2014). Recent trends in pediatric bacterial meningitis in Japan–a country where Haemophilus influenzae type b and Streptococcus pneumoniae conjugated vaccines have just been introduced. J. Infect. Chemother. 20, 477–483. doi: 10.1016/j.jiac.2014.04.007
Silva-Costa C., Gomes-Silva J., Pinho M. D., Friães A., Ramirez M., and Melo-Cristino J. (2022). Continued vaccine breakthrough cases of serotype 3 complicated pneumonia in vaccinated children, Portugal 2016–2019). Microbiol. Spectr. 10, e0107722. doi: 10.1128/spectrum.01077-22
Suga S., Chang B., Asada K., Akeda H., Nishi J., Okada K., et al. (2015). Nationwide population-based surveillance of invasive pneumococcal disease in Japanese children: effects of the seven-valent pneumococcal conjugate vaccine. Vaccine 33, 6054–6060. doi: 10.1016/j.vaccine.2015.07.069
Tamborrini M., Geib N., Marrero-Nodarse A., Jud M., Hauser J., Aho C., et al. (2015). A synthetic virus-like particle streptococcal vaccine candidate using B-cell epitopes from the proline-rich region of pneumococcal surface protein A. Vaccines. 3, 850–874. doi: 10.3390/vaccines3040850
Tart R. C., McDaniel L. S., Ralph B. A., and Briles D. E. (1996). Truncated Streptococcus pneumoniae PspA molecules elicit cross-protective immunity against pneumococcal challenge in mice. J. Infect. Dis. 173, 380–386. doi: 10.1093/infdis/173.2.380
Tu A. H. T., Fulgham R. L., McCrory M. A., Briles D. E., and Szalai A. J. (1999). Pneumococcal surface protein A inhibits complement activation by Streptococcus pneumoniae. Infect. Immun. 67, 4720–4724. doi: 10.1128/IAI.67.9.4720-4724.1999
Vadesilho C. F., Ferreira D. M., Gordon S. B., Briles D. E., Moreno A. T., Oliveira M. L. S., et al. (2014). Mapping of epitopes recognized by antibodies induced by immunization of mice with PspA and PspC. Clin. Vaccine Immunol. 21, 940–948. doi: 10.1128/CVI.00239-14
Verthelyi D., Ishii K. J., Gursel M., Takeshita F., and Klinman D. M. (2001). Human peripheral blood cells differentially recognize and respond to two distinct CPG motifs. J. Immunol. 166, 2372–2377. doi: 10.4049/jimmunol.166.4.2372
Victora C. G., Bahl R., Barros A. J. D., França G. V. A., Horton S., Krasevec J., et al. (2016). Breastfeeding in the 21st century: epidemiology, mechanisms, and lifelong effect. Lancet. 387, 475–490. doi: 10.1016/S0140-6736(15)01024-7
Wahl B., O’Brien K. L., Greenbaum A., Majumder A., Liu L., Chu Y., et al. (2018). Burden of Streptococcus pneumoniae and Haemophilus influenzae type b disease in children in the era of conjugate vaccines: global, regional, and national estimates for 2000–15. Lancet Glob. Health 6, e744–e757. doi: 10.1016/S2214-109X(18)30247-X
Wijayasri S., Hillier K., Lim G. H., Harris T. M., Wilson S. E., and Deeks S. L. (2019). The shifting epidemiology and serotype distribution of invasive pneumococcal disease in Ontario, Canada 2007–2017. PloS One 14, e0226353. doi: 10.1371/journal.pone.0226353
Xin W., Li Y., Mo H., Roland K. L., and Curtiss R. III (2009). PspA family fusion proteins delivered by attenuated Salmonella enterica serovar Typhimurium extend and enhance protection against Streptococcus pneumoniae. Infect. Immun. 77, 4518–4528. doi: 10.1128/IAI.00486-09
Keywords: Streptococcus pneumoniae, vaccine, lethal bacteremia, prenatal antibody transfer, PspA
Citation: Kuroda E, Tanaka Y and Hamaguchi S (2025) Anti-pneumococcal surface protein A antibodies transferred from maternal mice protect offspring against lethal infection with Streptococcus pneumoniae serotype 3. Front. Bacteriol. 4:1578341. doi: 10.3389/fbrio.2025.1578341
Received: 17 February 2025; Accepted: 03 April 2025;
Published: 19 May 2025.
Edited by:
Zhuo Ma, Albany College of Pharmacy and Health Sciences, United StatesReviewed by:
Masaya Yamaguchi, National Institutes of Biomedical Innovation, Health and Nutrition, JapanItziar Chapartegui-González, Karolinska Institutet (KI), Sweden
Copyright © 2025 Kuroda, Tanaka and Hamaguchi. This is an open-access article distributed under the terms of the Creative Commons Attribution License (CC BY). The use, distribution or reproduction in other forums is permitted, provided the original author(s) and the copyright owner(s) are credited and that the original publication in this journal is cited, in accordance with accepted academic practice. No use, distribution or reproduction is permitted which does not comply with these terms.
*Correspondence: Shigeto Hamaguchi, aGFtYWd1Y2hpQGhwLWluZmVjdC5tZWQub3Nha2EtdS5hYy5qcA==
†Present address: Shigeto Hamaguchi, Osaka Institute of Public Health, Osaka, Japan