- 1Department of Radiology, Shanghai Songjiang District Central Hospital, Shanghai, China
- 2Department of Nuclear Medicine, Shanghai General Hospital, Shanghai Jiao Tong University School of Medicine, Shanghai, China
We report the preparation and characterization of gadolinium (Gd)- or manganese (Mn)-loaded dendrimers and Gd-loaded dendrimer-entrapped gold nanoparticles (Gd-Au DENPs) to examine the relationship between the number of metal ion chelators and r1 relaxivity. In this study, amine-terminated fifth-generation poly(amidoamine) dendrimers (G5.NH2) modified with different numbers of DOTA-NHS chelators were used to chelate Gd and Mn ions. The remaining amine groups were then acetylated completely, followed by the use of materials with better r1 relaxivities and T1-weighted imaging performances as templates to synthesize Gd-Au DENPs. The Gd and Mn chelators as well as Gd-Au DENPs were characterized via different techniques. We show that the r1 relaxivity and T1 imaging performance increase with loading of greater numbers of Gd and Mn ions on the G5.NH2 and that the acetylation process affects the relaxivity and imaging properties to a certain extent. After entrapment with Au NPs, the r1 relaxivity and T1-weighted imaging performance of Gd-Au DENPs decrease with greater loading of Au NPs. This systematic study of the relaxivities and T1-weighted imaging performances of Gd, Mn, and Gd-Au DENP chelators are expected to be a theoretical basis for developing multifunctional dual-mode contrast agents.
Introduction
Magnetic resonance (MR) imaging is one of the most powerful noninvasive medical imaging techniques with good spatial resolution and high sensitivity, offering superior 3D details and topographic information on soft-tissue contrast (Chan and Wong, 2007; Taboada et al., 2007; Xiao et al., 2023). To increase the signal-to-noise ratio of information acquired from normal tissues and tumors, it is necessary to use contrast agents (Aime et al., 2009). To date, various contrast agents have been used for clinical diagnosis, such as gadolinium (Gd)-based small molecular (DTPA, DOTA, NOTA) contrast agents in T1-weighted MR imaging and iron oxide nanoparticles (NPs)-based contrast agents in T2-weighted MR imaging (Miao et al., 2021; Meng et al., 2022). Unfortunately, these small-molecule-based contrast agents cannot be used for long-circulation imaging owing to their short circulation times in the blood and quick elimination from the body through urine (Prencipe et al., 2009). Although iron oxide NPs display excellent MR imaging properties and are widely used as negative MR contrast agents (Le et al., 2022; Luo et al., 2022), their clinical use has several disadvantages; in particular, negative contrast effects and magnetic susceptibility artifacts are observed, which could mislead the clinical diagnosis. Therefore, it is crucial to develop novel carriers for MR imaging contrast agents to overcome these drawbacks.
Among the macromolecular family, poly(amidoamine) (PAMAM) dendrimers are a class of highly branched, monodispersed, and synthetic macromolecules with well-defined three-dimensional architectures, composition, and abundant terminal functional groups (Shi et al., 2008). The unique structural properties of PAMAM allow their use as a platform for constructing various kinds of contrast agents, such as dendrimer-based Mn- or Gd-loaded agents for MR imaging, Gd-loaded dendrimer-entrapped gold nanoparticles (Gd-Au DENPs) for CT/MR applications (Chen et al., 2013), and 99mTc labeled Mn-loaded dendrimer-based contrast agents for SPECT/MR imaging applications (Liu et al., 2012; Fan et al., 2020). The PAMAM dendrimer has been widely researched for single- and multi-mode contrast agents, but there are still some questions that have not been well resolved. First, the relationship between the r1 relaxivity of the chelate numbers modified onto the dendrimer and loading amount of the metal ions is not clearly understood. Second, given the same modified condition (same number of chelates and same mole amounts of metal ions), which among Mn- or Gd-based contrast agents have better r1 relaxivity and T1-weighted MR imaging performance? Third, after entrapping gold NPs, the effects of r1 relaxivity and T1-weighted MR imaging performance of Gd-loaded Au DENPs as CT/MR dual-mode contrast agents are not investigated in detail. Based on these questions, in the present study, the amine-terminated fifth-generation poly(amidoamine) (G5.NH2) dendrimers were first modified with 2, 2′, 2′′-(10-(2-(2, 5-dioxopyrrolidin1-aryloxy)-2-oxoethyl)-1, 4, 7,1 0-tetraazacyclododecane-1, 4, 7-triyl) triacetin acid (DOTA-NHS) in mole ratios of 1:5, 1:10, 1:20, and 1:30; then, the modified G5.NH2 dendrimers were used as templates to chelate Gd(III) and Mn(II). After acetylation of the remaining dendrimer terminal amines, G5.NHAc-DOTA-Gd or G5.NHAc-DOTA-Mn were formed. The formed Gd and Mn chelators were characterized thoroughly via different techniques, and the material with the highest r1 relaxivity was chosen as the template to entrap Au NPs to prepare the Gd-Au DENPs.
Experimental methods
Materials
Ethylenediamine core G5.NH2 PAMAM dendrimers (molecular weight = 26,010 g/mol) with polydispersity index values less than 1.08 were purchased from Dendritech (Midland, MI, USA). Polyethylene glycol (PEG) monomethyl ether with a carboxyl group at one end (mPEG-COOH) was obtained from Shanghai Yanyi Biotechnology Corporation (Shanghai, China). Gd(NO3)3.6H2O, MnSO4.H2O, HAuCl4.4H2O, acetic anhydride, triethylamine, and all other chemicals as well as solvents were purchased from Sinopharm Chemical Reagent Co., Ltd. (Shanghai, China). Sodium borohydride was purchased from J&K Chemical Ltd. (Shanghai, China). DOTA-NHS was purchased from CheMatech (Dijon, France). The water used in all experiments was purified using a Milli-Q Plus185 water purification system (Millipore, Bedford, MA) with a resistivity greater than 18.2 MΩ cm.
Fabrication of G5.NH2 with DOTA
About 13.00 mg of G5.NH2 dissolved in DMSO (4 mL) was reacted with 5 molar equivalents of DOTA-NHS (1.87 mg, 2 mL in DMSO) under vigorous magnetic stirring, and purification was performed similar to that noted in our previous report (Wen et al., 2013). The reaction was stopped after 24 h to obtain the raw product G5.NH2-DOTA5. Then, G5.NH2-DOTA10, G5.NH2-DOTA20, and G5.NH2-DOTA30 were fabricated in a similar manner.
Synthesis of G5.NH2-DOTA-Mn and G5.NH2-DOTA-Gd
The formed G5.NH2-DOTA5, G5.NH2-DOTA10, G5.NH2-DOTA20, and G5.NH2-DOTA30 were used as templates to chelate Mn(II) (Figure 1A) or Gd(III) (Figure 1B) ions. Aqueous MnSO4 (1.27 mg/mL, 1 mL in water) and Gd(NO3)3 (3.39 mg/mL, 1 mL in water) solutions with MnSO4/DOTA and Gd(NO3)3/DOTA in a molar ratio of 1.5:1 were added to G5.NH2-DOTA5 under vigorous stirring to chelate Mn(II) or Gd(III) ions, respectively. The reaction was performed for 24 h to obtain G5.NH2-DOTA5(Mn) or G5.NH2-DOTA5(Gd) as the products. Then, half the volumes of G5.NH2-DOTA5(Mn) and G5.NH2-DOTA5(Gd) solutions were removed for dialysis for 3 days (three times per day, 2 L of water each time) to remove the excess Mn(II) or Gd(III) ions. Six molar equivalents of the dendrimer terminal amine triethylamine (53 μL) were added to the remaining G5.NH2-DOTA5(Mn)5 and G5.NH2-DOTA5(Gd)5 solutions. After 30 min, acetic anhydride (33 μL, equal to 5 molar equivalents of the dendrimer terminal amine) was added dropwise into the solutions under vigorous magnetic stirring, and the solutions were allowed to react at room temperature with stirring for 24 h. Then, the DMSO, excess reactants, and byproducts were removed from the mixture by extensive dialysis with water (9 times, 2 L) for 3 days, followed by lyophilization to obtain the G5.NHAc-DOTA5(Mn) and G5.NHAc-DOTA5(Gd). The G5.NH2-DOTA10(Mn), G5.NH2-DOTA20(Mn), G5.NH2-DOTA30(Mn), G5.NH2-DOTA10(Gd), G5.NH2-DOTA20(Gd), G5.NH2-DOTA30(Gd), and their acetylated materials were also formed similarly.
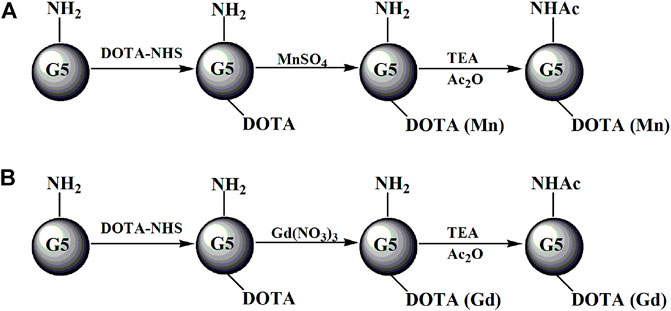
FIGURE 1. Schematic illustration of the preparation of G5.NHAc-DOTAn(Gd) (A) or G5.NHAc-DOTAn(Mn) (B). TEA and Ac2O represent triethylamine and acetic anhydride, respectively.
Synthesis of (Au0)n-G5.NHAc-mPEG15-DOTA30(Gd)
About 13.00 mg of G5.NH2 dissolved in DMSO (4 mL) was reacted with 30 molar equivalents of DOTA-NHS (11.22 mg, 5 mL in DMSO) under vigorous magnetic stirring (Figure 2). The reaction was stopped after 24 h to obtain G5.NH2-DOTA30 as the raw product. Then, 15.00 mg of mPEG-COOH dissolved in 5 mL DMSO and 15 molar equivalents of G5.NH2 was reacted with EDC (14.38 mg in 2 mL DMSO) for 15 min at room temperature. Next, NHS (8.63 mg, in 1 mL DMSO) was added to the above solution under vigorous magnetic stirring for 3 h. The EDC/NHS-activated mPEG-COOH was then added dropwise into the DMSO solution of the raw product of G5.NH2-DOTA30 under vigorous magnetic stirring. The reaction was continued for 3 days to obtain G5.NH2-DOTA30-mPEG15 conjugates as the raw products. Then, HAuCl4 solution (5 mg/mL, 2.06 mL in water) was added under vigorous stirring. After 1 h, an icy cold NaBH4 solution (4.73 mg/mL, 1 mL in water/methanol, v/v = 2:1) with 5 molar equivalents of the gold salt was added to the gold salt/dendrimer mixture under vigorous stirring. The solution turned a deep-red color after the addition of NaBH4, and the stirring was continued for 3 h to complete the reaction. Then, acetylation of the excess terminal amines was performed similar to the process in our previous work (Liu et al., 2012). The mixture was purified by dialysis as described previously to remove the excess DMSO and other reagents. The Au DENPs were then purified and dried by lyophilization.

FIGURE 2. Schematic illustration of the preparation of (Au0)n-G5.NHAc-mPEG-DOTA30(Gd). TEA and Ac2O represent triethylamine and acetic anhydride, respectively.
Characterization techniques
1H NMR spectra were recorded with a Bruker DRX 400 nuclear magnetic resonance spectrometer. Samples were dissolved in D2O before the measurements. The size and morphology of the Gd-Au DENPs were characterized by using a JEOL 2010F analytical electron microscope (JEOL, Japan) operating at 200 kV. Transmission electron microscopy (TEM) samples were prepared by the deposition of a dilute particle suspension (1 mg/mL, 5 μL) onto a carbon-coated copper grid and air dried before measurements. For each sample, at least 100 NPs from different TEM images were randomly selected and measured using ImageJ software (http://www.rsb.info.nih.gov/ij/download.html) to assess the average size and size distribution. The compositions of Mn, Gd, and Au of the formed materials were determined by inductively coupled plasma optical emission spectroscopy (ICP-OES, Leeman Prodigy, USA). The surface potentials of each material before and after acetylation were measured using a Zetasizer Nano ZS system (Worcestershire, UK) equipped with a standard 633 nm laser. The r1 relaxivity and T1-weighted images of the Gd and Mn chelates were obtained using a 0.5 T NMI20 equipment (Newman, China) with a wrist receiver coil.
Results and discussion
Synthesis and characterization of G5.NH2-DOTA
The 1H NMR technique was used to investigate the actual number of DOTA units conjugated onto each G5.NH2 molecule, as in our previous work (Supplementary Figure S1;Wen et al., 2013). After conjugation of different ratios of DOTA-NHS, the numbers of remaining dendrimer terminal amine groups in each of the G5.NH2 molecules were estimated to be 105.8, 102.5, 99.6, and 90.1, and the numbers of DOTA units per G5.NH2 molecule were calculated as 4.2, 7.5, 10.4, and 19.9 (Supplementary Table S1); these numbers are slightly lower than the theoretical values of 5, 10, 20, and 30 based on the initial molar feed ratios.
Fabrication and characterization of G5.NHAc–DOTA(Mn) and G5.NHAc-DOTA(Gd)
The numbers of Gd(III) and Mn(II) ions complexed with each dendrimer were measured as shown in Supplementary Table S2. It is clear that the number of ions per G5.NH2 molecule of the Gd-based chelate is slightly higher than the DOTA number on the surface of the dendrimer, which is consistent with the observations in our previous work (Wen et al., 2013). The reason for this is likely the fact that besides the Gd(III) ions chelated within the DOTA ligands on the surfaces of the G5 dendrimers, small portions of the Gd(III) ions are complexed with the interior tertiary amine groups of the dendrimer. In contrast, the number of Mn(II) ions complexed with each G5.NH2 molecule was estimated to be 23.3 when the initial molar feed ratio of the DOTA/dendrimer was 30:1; this is less than the theoretical value of 30 attached DOTA moieties on each dendrimer. Compared with the Mn-based chelates, the Gd-based chelates thus have better chelating abilities.
To utilize the G5.NH2 modifications to Gd- and Mn-based chelates in biological applications, it is necessary to explore their cytotoxicities before and after acetylation. Our previous report shows that the G5.NH2 displays significant cytotoxicity because of more than 100 amino groups on the surface of the G5.NH2 dendrimer. To investigate the changes in the zeta potentials of each of the materials before and after acetylation, the zeta potential of each material was measured. As shown in Supplementary Table S3, the zeta potential of each material decreased sharply after acetylation. This may be attributed to the redundant amino groups of G5.NH2 being changed into acetamide groups with acetic anhydride through acetylation.
Synthesis and characterization of {(Au0)n-G5.NHAc-mPEG15-DOTA3(Gd)} DENPs
The size and morphology of Au DENPs of different compositions were characterized by TEM (Figure 3). The diameters of the (Au0)50-G5.NHAc-mPEG15-DOTA30-Gd, (Au0)75-G5.NHAc-mPEG15-DOTA30-Gd, and (Au0)100-G5.NHAc-mPEG15-DOTA30-Gd DENPs were 4.1, 3.7, and 3.0 nm, respectively. The diameter of the Au DENPs increased slightly with the high dendrimer/Au salt molar ratio, which is very close to that noted in our previous study (Wen et al., 2013).
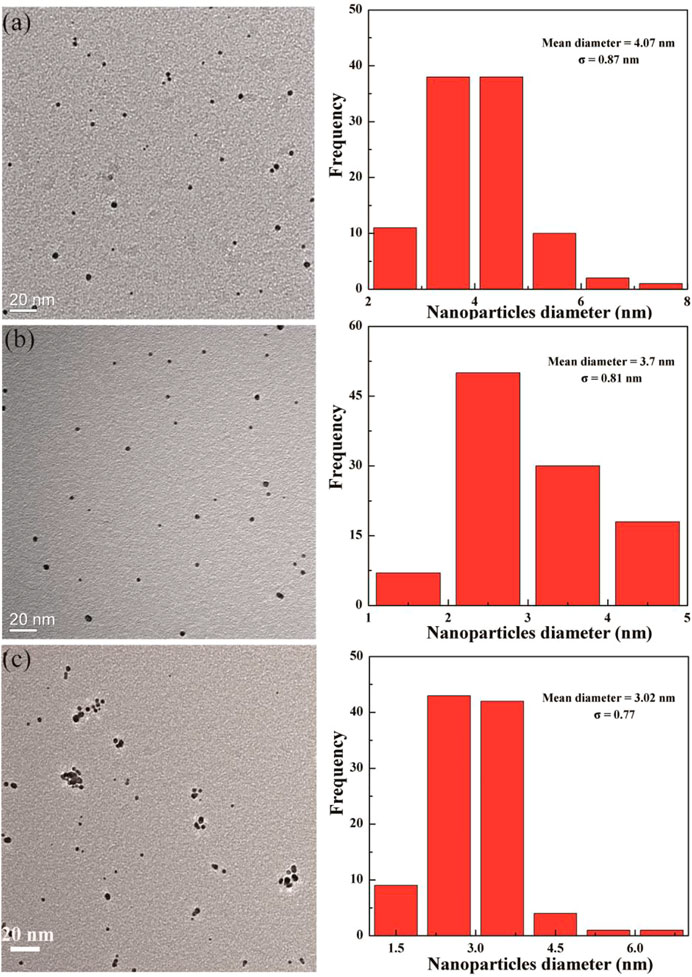
FIGURE 3. Transmission electron microscopy images of (Au0)50G5.NHAc-mPEG-DOTA30(Gd) (A), (Au0)75 G5.NHAc-mPEG-DOTA30(Gd) (B), and (Au0)100G5.NHAc-mPEG-DOTA30(Gd) (C) DENPs.
T1-weighted imaging and r1 relaxivity of Mn-based and Gd-based chelates
T1-weighted imaging was conducted to verify the potential of the formed Mn-based (Figure 4) as well as Gd-based (Figure 5) materials as MR contrast agents. The proton longitudinal relaxation times (T1) of these sixteen samples in water were measured with a 0.5 T NMI20 MRI system (Newman, China) with Mn2+ and Gd3+ concentrations of 0.1, 0.2, 0.4, 0.8, and 1.6 mM. As shown in Figure 3 and Figure 4, signal enhancements are observed in the T1-weighted images in a number-dependent manner with increases in the numbers of DOTA-Mn and DOTA-Gd ions per G5.NH2. For the Mn-chelates, the signal enhancements in the T1-weighted images were inconspicuous even though the number of DOTA-Mn increased to 20. We found that the signals of the Mn chelates weakened after acetylation. On the contrary, the signals of the Gd chelates increased slightly after acetylation. As shown in Table 1, the r1 relaxivity of the DOTA-Mn increased from 1.16 to 2.54 mM−1s−1 with increase in number from 5 to 30 per G5.NH2. After acetylation, the r1 relaxivity of the DOTA-Mn decreased obviously from 1.90 to 0.55 mM−1s−1. The r1 relaxivity results follow the same trends as the T1-weighted images. The r1 relaxivity of the Gd-based chelates increased from 5.77 to 7.69 mM−1s−1 with increase in the numbers of DOTA-Gd from 5 to 30 per G5.NH2. However, the r1 relaxivities of the Gd-based chelates increased from 7.06 to 9.77 after acetylation, which is different from those of the Mn-based chelates. We speculate that the increased r1 relaxivities of the Gd- and Mn-based chelates may be due to the additional DOTA conjugates on the G5.NH2 to form nanoclusters as it has been noted in literature that the cluster structure could promote water exchange rate to increase the r1 relaxivity (Matsumoto and Jasanoff, 2008).
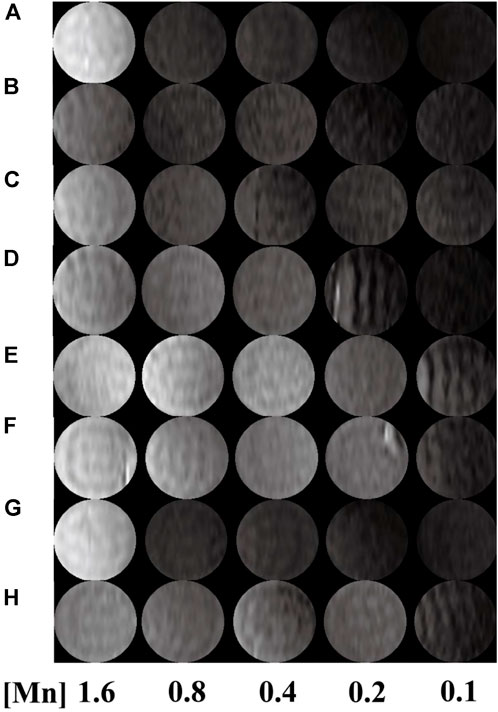
FIGURE 4. T1-weighted images of G5.NH2-DOTA5(Mn), G5.NH2-DOTA10(Mn), G5.NH2-DOTA20(Mn), and G5.NH2-DOTA30(Mn) (A, C, E, G) before and (B, D, F, H) after acetylation as functions of Mn2+ concentrations.
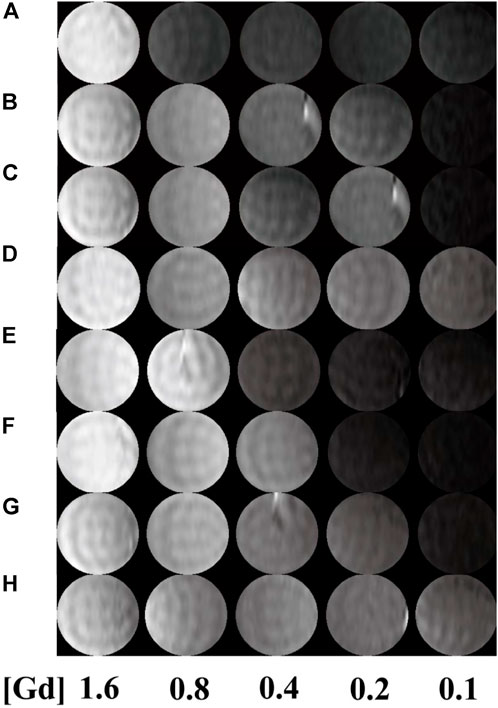
FIGURE 5. T1-weighted images G5.NH2-DOTA5(Gd), G5.NH2-DOTA10(Gd), G5.NH2-DOTA20(Gd), and G5.NH2-DOTA30(Gd) (A, C, E, G) before and (B, D, F, H) after acetylation as functions of Gd3+ concentrations.
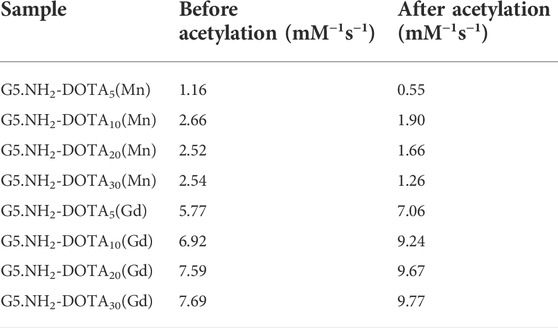
TABLE 1. Linear fitting of the r1 relaxivities of G5.NH2-DOTA(Mn) and G5.NH2-DOTA(Gd) before and after acetylation as functions of Mn and Gd concentrations, respectively.
T1-weighted imaging and r1 relaxivity of Gd-Au DENPs
It has been reported that Au NPs could be used as CT contrast agents because they have good biocompatibility. To investigate the relationship between the number of gold NPs and r1 relaxivity of the Gd-based chelates, we fabricated different G5:Au mole ratios of 1:50, 1:75, and 1:100 NPs and measured the r1 relaxivities (Figure 6) of Gd-Au DENPs as well as obtained their T1-weighted images (Figure 7). Figure 4 shows that with the increase in the amount of gold atoms per G5.NH2, the r1 relaxivity decreased from 13.11 to 7.50 mM−1s−1. This is attributed to the fact that the gold NPs being entrapped in the interior of the G5.NH2 primary cavity structure changed and that the G5.NH2 molecule became tight, leading to reduced water exchange rate between its interior and exterior.
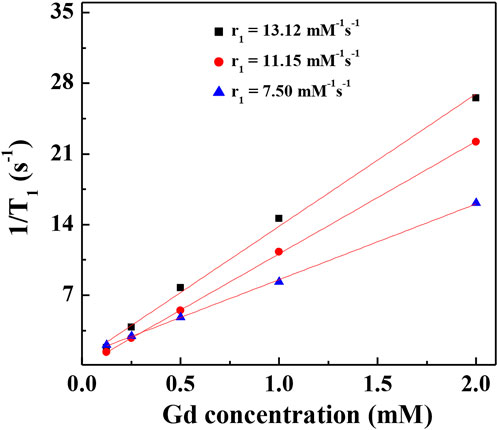
FIGURE 6. Linear fitting 1/T1 of (Au0)50G5.NHAc-mPEG-DOTA30(Gd) (black square), (Au0)75 G5.NHAc-mPEG-DOTA30(Gd) (red circle), and (Au0)100G5.NHAc-mPEG-DOTA30(Gd) (blue triangle) DENPs at Gd concentrations of 2, 1, 0.5, 0.25 and 0.125 mM.
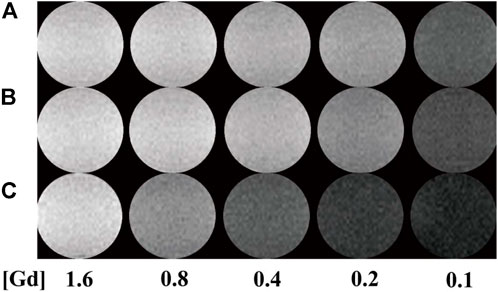
FIGURE 7. T1-weighted images of (A–C) (Au0)50G5.NHAc-mPEG-DOTA30(Gd), (Au0)75 G5.NHAc-mPEG-DOTA30(Gd), and (Au0)100G5.NHAc-mPEG-DOTA30(Gd) DENPs at Gd concentrations of 1.6, 0.8, 0.4, 0.2, and 0.1 mM.
Conclusion
We systematically investigated the relationship between r1 relaxivity and number of metal ions per dendrimer in this study. T1 relaxometry measurements show that the formed G5.NHAc-DOTA(Gd) NPs have an r1 relaxivity of 9.77 mM−1 s−1 when the number of DOTA is 30. Compared with dendrimer-based Gd chelators, the Mn-based materials show lower r1 relaxivity and poor T1 imaging properties for the same number of DOTA units. When the mole ratio of gold to G5 is as high as 100:1, the r1 relaxivity of G5.NHAc-DOTA30-Gd decreased to 7.50 mM−1s−1 and T1 imaging property was weakened. With appropriate tuning of the number of ions per G5 and the Gd/Au composition, the formed Gd-based or Gd/Au NPs may be applied in dual-mode MR/CT imaging and diagnosis of particular diseases (e.g., cancer) with high accuracies.
Data availability statement
The original contributions presented in the study are included in the article/Supplementary Material, and further inquiries can be directed to the corresponding author.
Author contributions
KL and CL contributed equally to this work. Experiment design: JX; experiments: KL and CL; data analysis: KL, CL, and JX; manuscript writing: KL, CL, and JX. The final version has been approved by all authors.
Funding
This work was funded by the Songjiang District Science and Technology Commission (Mechanism of BmK CT-mediated radioiodine combined with temozolomide in the treatment of glioblastoma: No. 21SJKJGG155).
Conflict of interest
The authors declare that the research was conducted in the absence of any commercial or financial relationships that could be construed as a potential conflict of interest.
Publisher’s note
All claims expressed in this article are solely those of the authors and do not necessarily represent those of their affiliated organizations, or those of the publisher, the editors, and the reviewers. Any product that may be evaluated in this article or claim that may be made by its manufacturer is not guaranteed or endorsed by the publisher.
Supplementary material
The Supplementary Material for this article can be found online at https://www.frontiersin.org/articles/10.3389/fbioe.2022.1004414/full#supplementary-material
References
Aime, S., Castelli, D. D., Crich, S. G., Gianolio, E., and Terreno, E. (2009). Pushing the sensitivity envelope of lanthanide-based magnetic resonance imaging (MRI) contrast agents for molecular imaging applications. Acc. Chem. Res. 42 (7), 822–831. doi:10.1021/ar800192p
Chan, K. W.-Y., and Wong, W.-T. (2007). Small molecular gadolinium (III) complexes as MRI contrast agents for diagnostic imaging. Coord. Chem. Rev. 251 (17), 2428–2451. doi:10.1016/j.ccr.2007.04.018
Chen, Q., Li, K., Wen, S., Liu, H., Peng, C., Cai, H., et al. (2013). Targeted CT/MR dual mode imaging of tumors using multifunctional dendrimer-entrapped gold nanoparticles. Biomaterials 34 (21), 5200–5209. doi:10.1016/j.biomaterials.2013.03.009
Fan, Y., Tu, W., Shen, M., Chen, X., Ning, Y., Li, J., et al. (2020). Dendrimer‐based nanosensitizers: Targeted tumor hypoxia dual‐mode CT/MR imaging and enhanced radiation therapy using dendrimer‐based nanosensitizers (adv. Funct. Mater. 13/2020). Adv. Funct. Mat. 30 (13), 2070082. doi:10.1002/adfm.202070082
Le, T. T., Nguyen, T. N. L., Nguyen, H. D., Phan, T. H. T., Pham, H. N., Le, D. G., et al. (2022). Multimodal imaging contrast property of nano hybrid Fe3O4@Ag fabricated by seed-growth for medicinal diagnosis. Chemistryselect 7 (25). doi:10.1002/slct.202201374
Liu, H., Shen, M., Zhao, J., Guo, R., Cao, X., Zhang, G., et al. (2012). Tunable synthesis and acetylation of dendrimer-entrapped or dendrimer-stabilized gold–silver alloy nanoparticles. Colloids Surfaces B Biointerfaces 94, 58–67. doi:10.1016/j.colsurfb.2012.01.019
Luo, M., Yukawa, H., and Baba, Y. (2022). Fluorescent/magnetic nano-aggregation via electrostatic force between modified quantum dot and iron oxide nanoparticles for bimodal imaging of U87MG tumor cells. Anal. Sci. 38, 1141–1147. doi:10.1007/s44211-022-00153-z
Matsumoto, Y., and Jasanoff, A. (2008). T2 relaxation induced by clusters of superparamagnetic nanoparticles: Monte Carlo simulations. Magn. Reson. Imaging 26 (7), 994–998. doi:10.1016/j.mri.2008.01.039
Meng, Q., Wu, M., Shang, Z., Zhang, Z., and Zhang, R. (2022). Responsive gadolinium(III) complex-based small molecule magnetic resonance imaging probes: Design, mechanism and application. Coord. Chem. Rev. 2022, 214398. doi:10.1016/j.ccr.2021.214398
Miao, Y., Chen, P., Yan, M., Xiao, J., Hong, B., Zhou, K., et al. (2021). Highly sensitive T-1-T-2 dual-mode MRI probe based on ultra-small gadolinium oxide-decorated iron oxide nanocrystals. Biomed. Mat. 16 (4), 044104. doi:10.1088/1748-605x/abef54
Prencipe, G., Tabakman, S. M., Welsher, K., Liu, Z., Goodwin, A. P., Zhang, L., et al. (2009). PEG branched polymer for functionalization of nanomaterials with ultralong blood circulation. J. Am. Chem. Soc. 131 (13), 4783–4787. doi:10.1021/ja809086q
Shi, X., Wang, S. H., Swanson, S. D., Ge, S., Cao, Z., Van Antwerp, M. E., et al. (2008). Dendrimer‐functionalized shell‐crosslinked iron oxide nanoparticles for in-vivo magnetic resonance imaging of tumors. Adv. Mat. 20 (9), 1671–1678. doi:10.1002/adma.200702770
Taboada, E., Rodríguez, E., Roig, A., Oró, J., Roch, A., and Muller, R. N. (2007). Relaxometric and magnetic characterization of ultrasmall iron oxide nanoparticles with high magnetization evaluation as potential T1 magnetic resonance imaging contrast agents for molecular imaging. Langmuir 23 (8), 4583–4588. doi:10.1021/la063415s
Wen, S., Li, K., Cai, H., Chen, Q., Shen, M., Huang, Y., et al. (2013). Multifunctional dendrimer-entrapped gold nanoparticles for dual mode CT/MR imaging applications. Biomaterials 34 (5), 1570–1580. doi:10.1016/j.biomaterials.2012.11.010
Xiao, X., Cai, H., Huang, Q., Wang, B., Wang, X., Luo, Q., et al. (2023). Polymeric dual-modal imaging nanoprobe with two-photon aggregation-induced emission for fluorescence imaging and gadolinium-chelation for magnetic resonance imaging. Bioact. Mat. 19, 538–549. doi:10.1016/j.bioactmat.2022.04.026
Keywords: dendrimer, manganese, gadolinium, r1 relaxivity, T1-weighted MR imaging
Citation: Liu K, Liu C and Xia J (2022) The r1 relaxivity and T1 imaging properties of dendrimer-based manganese and gadolinium chelators in magnetic resonance imaging. Front. Bioeng. Biotechnol. 10:1004414. doi: 10.3389/fbioe.2022.1004414
Received: 27 July 2022; Accepted: 30 August 2022;
Published: 10 October 2022.
Edited by:
Yu Luo, Shanghai University of Engineering Sciences, ChinaReviewed by:
Xilin Bai, Northeast Normal University, ChinaXijian Liu, Shanghai University of Engineering Sciences, China
Yu Chong, Soochow University, China
Copyright © 2022 Liu, Liu and Xia. This is an open-access article distributed under the terms of the Creative Commons Attribution License (CC BY). The use, distribution or reproduction in other forums is permitted, provided the original author(s) and the copyright owner(s) are credited and that the original publication in this journal is cited, in accordance with accepted academic practice. No use, distribution or reproduction is permitted which does not comply with these terms.
*Correspondence: Jindong Xia, c2p6eHl5eGlhamRfMjFAMTI2LmNvbQ==