- Guangxi Medical University Cancer Hospital, Nanning, China
Introduction: Reactive oxygen species (ROS)-mediated therapies have typically been considered as noninvasive tumor treatments owing to their high selectivity and efficiency. However, the harsh tumor microenvironment severely impairs their efficiency.
Methods: Herein, the biodegradable Cu-doped zeolitic imidazolate framework-8 (ZIF-8) was synthesized for loading photosensitizer Chlorin e6 (Ce6) and CaO2 nanoparticles, followed by surface decoration by hyaluronic acid (HA), obtaining HA/CaO2-Ce6@Cu-ZIF nano platform.
Results and Discussion: Once HA/CaO2-Ce6@Cu-ZIF targets tumor sites, the degradation of Ce6 and CaO2 release from the HA/CaO2-Ce6@Cu-ZIF in response to the acid environment, while the Cu2+ active sites on Cu-ZIF are exposed. The released CaO2 decompose to generate hydrogen peroxide (H2O2) and oxygen (O2), which alleviate the insufficiency of intracellular H2O2 and hypoxia in tumor microenvironment (TME), effectively enhancing the production of hydroxyl radical (•OH) and singlet oxygen (1O2) in Cu2+-mediated chemodynamic therapy (CDT) and Ce6-induced photodynamic therapy (PDT), respectively. Importantly, Ca2+ originating from CaO2 could further enhance oxidative stress and result in mitochondrial dysfunction induced by Ca2+ overloading.
Conclusion: Thus, the H2O2/O2 self-supplying and Ca2+ overloading ZIF-based nanoplatform for cascade-amplified CDT/PDT synergistic strategy is promising for highly efficient anticancer therapy.
1 Introduction
Cancer is one of the most lethal diseases and causes millions of deaths annually with increasing mortality worldwide. Considering the high risk and death rate of cancer, scientists around the world have dedicated themselves to achieving effective and precise diagnoses as well as safe and hazard-free therapy to fight against it. With the rapid development in nanotechnology over the past 2 decades, nanomaterials have provided an advanced approach from anti-cancer experts and are expected to be used in cancer imaging and treatment therapy. (Wang et al., 2021a; Yang et al., 2021a; Zhang et al., 2021; Zhou et al., 2021; Shan et al., 2022; Zhang et al., 2022; Li et al., 2023; Lu et al., 2023). Metal-organic frameworks (MOFs) with potential biological performance, such as biocompatibility, cytotoxicity, and biodistribution, have been extensively studied in nanotherapeutics. (Wang et al., 2019a; Xie et al., 2019a; Yang et al., 2019a; Zhang et al., 2019a; Zhao et al., 2019; Pandey et al., 2020). MOFs are a series of crystallized porous materials coordinated by metal-containing cores (e.g., metal ions and clusters) and organic linkers (e.g., carboxylate ligands, phosphonates, sulfonates, and other negatively charged ligands). MOFs are not only good carriers of nanocargo (drugs and contrast agents) because of their porous and oriented structure but also contrast agents themselves due to their multifunctional building blocks. (Wang et al., 2019b; Cai et al., 2019; Ren et al., 2019; Rojas et al., 2019). Importantly, with good biodegradability and biocompatibility, MOF composites could be constructed as physiological environment-accommodative synergist therapy platforms. (Wang et al., 2021b; Liang et al., 2021; Bian et al., 2022). Because of this, incorporating functionalized compositions and comprehensive structures within MOFs to obtain nanoplatforms with collective properties and advanced performance has attracted much attention.
As a major molecule produced during oxidative stress, reactive oxygen species (ROS) contains singlet oxygen (1O2), superoxide anions (O2−), and hydroxyl radicals (•OH), which are considered to be essential factors in the occurrence, development, and recurrence of cancer. (Yang et al., 2019b; Li et al., 2021a; Tao et al., 2022; Truong Hoang et al., 2022; Yu et al., 2022; Cao et al., 2023). Furthermore, depending on their high selectivity and unrecognized drug resistance, ROS-mediated therapies such as chemodynamic therapy (CDT) (Zhao et al., 2021a; Yang et al., 2021b; Zhou et al., 2021) and photodynamic therapy (PDT) (Zhang et al., 2019b; Zhao et al., 2021b; Rui et al., 2021) have been considered as noninvasive anti-cancer treatments. CDT utilizes the Fenton/Fenton-like reaction between catalysts and hydrogen peroxide (H2O2) to generate cytotoxic •OH, (Chen et al., 2020; Wang et al., 2020; Cao et al., 2021), while PDT relies on nontoxic photosensitizers that are activated by visible or/and near-infrared (NIR) light to convert oxygen (O2) to 1O2. (Deng et al., 2017; Xie et al., 2019a; Yang et al., 2019c; Monro et al., 2019; Sivasubramanian et al., 2019). However, the harsh tumor microenvironment (TME) is an obstacle against achieving highly efficient therapeutic efficacy. Compared to normal cells, TME exhibits unique characteristics, such as mildly acidic conditions (pH = 5.5–6.5), internal hypoxic environment, high levels of intracellular glutathione (GSH, ∼10 × 10−3 M), excessive H2O2, (50−100 × 10−6 M), and hypoxia conditions. (Wang et al., 2018; Peng et al., 2021; Chang et al., 2022). The low intracellular H2O2 concentration and inherent hypoxia at tumor sites result in the low ROS production efficiency of CDT and PDT, respectively. In addition, the strong antioxidant GSH in TME also would downregulate the ROS level, aggravating the attenuation of antitumor efficiency. Li et al. loaded the chemotherapy prodrug disulfiram (DSF) and coated glucose oxidase (GOD) on the surface of Cu/ZIF-8 nanospheres and finally encapsulated manganese dioxide (MnO2) nanoshells to achieve efficient DSF-based cancer chemotherapy and dual-enhanced CDT. The MnO2 layer could achieve GSH depletion and relieve tumor hypoxia in the TME, the released Mn2+ could initiate T1-MRI for the tracking of the nanocatalyst in vivo, and the O2 produced in the reaction could oxidize glucose to H2O2 and gluconic acid in the presence of GOD. (Li et al., 2021b). Thus, engineering H2O2/O2 self-supplying therapeutic nanoplatforms to increase in situ the H2O2 and O2 concentration at tumor sites and constructing a CDT/PDT strategy to achieve a more synergistic effect than that of single-mode might be possible solutions.
More attractively, most of the latest research has provided approaches to improve the propagation of H2O2 and relieve hypoxia at tumor sites. (Wang et al., 2019c; Liu et al., 2019). Among them, a highly biocompatible metal peroxide, calcium peroxide (CaO2), has received widespread attention because of its excellent advantages, such as the simultaneous generation of O2 and H2O2 immediately following a reaction with water, serving as a donor of H2O2, and eliminating GSH in response to TME. (Sun et al., 2021a; Sun et al., 2021b; Liu et al., 2022a; Liu et al., 2022b). Additionally, overloaded exogenous Ca2+ could induce mitochondrial damage and further disorder the oxidative stress, resulting in the imbalance of calcium transport channel and accelerating tumor calcification-mediated apoptosis. (Zhang et al., 2019c; He et al., 2021; Wan et al., 2021; Docampo and Vercesi, 2022; Zheng et al., 2022). Hence, CaO2 could be appreciated as an advanced candidate for the rational design of multifunctional nanoplatforms for promoting CDT and PDT efficiency while achieving mitochondrial-localized Ca2+ overloading, ultimately allowing amplification of intracellular ROS-mediated therapeutic effect. (Hu et al., 2020; Shen et al., 2021; Chen et al., 2022).
Zeolitic imidazolate framework-8 (ZIF-8), composed of the coordination of Zn ions with 2-methylimidazole (2-MeIM), is a promising MOF for the construction of therapeutic nanoplatforms. (Xie et al., 2019b; Qin et al., 2019; Yang et al., 2020; Wang et al., 2021c; Jiang et al., 2022; Li et al., 2022). In this study, the biodegradable Cu-doped ZIF-8 was synthesized for loading photosensitizer Chlorin e6 (Ce6) and CaO2 nanoparticles (NPs), followed by surface modification by hyaluronic acid (HA), finally obtaining HA/CaO2-Ce6@Cu-ZIF nano platform. Once HA/CaO2-Ce6@Cu-ZIF targets tumor sites through HA-mediated active endocytosis and degrading by hyaluronidase (HAase), the degradation of Ce6 and CaO2 is released from the HA/CaO2-Ce6@Cu-ZIF in response to the acid environment, while the Cu2+ active sites on Cu-ZIF are exposed. The released CaO2 decompose to generate H2O2 and O2, which alleviates the insufficiency of intracellular H2O2 and hypoxia in TME, effectively amplifying the production of •OH and 1O2 in Cu2+-mediated CDT and Ce6-induced PDT, respectively. Importantly, Ca2+ originating from CaO2 could further amplify the oxidative stress and lead to mitochondrial dysfunction induced by Ca2+ overloading. Thus, the H2O2/O2 self-supplying and Ca2+ overloading MOF-based nanoplatform for cascade-amplified CDT/PDT synergistic strategy is promising for highly efficient anticancer therapy.
2 Experimental section
2.1 Chemicals
Zn(NO3)2•6H2O (0.1 M), 2-MeIM (C4H6N2, 99%), Cu(NO3)2•3H2O (AR), CaCl2 (97%), and HA (10 k) were purchased from Shanghai Aladdin Technology Co., Ltd. Ce6, DAPI, MTT, calcein-AM, and PI were supplied by Sigma-Aldrich. The annexin V-FITC/PI apoptosis kit was obtained from MultiScience Biotech Co., Ltd. All liquid chemical reagents were used without further purification.
2.2 Synthesis of CaO2 NPs
CaO2 NPs were obtained by a hydrolysis–precipitation process. A specific amount of CaCl2 (1 g) was sent into the HA (50 mL, 0.1 M) solution at room temperature under continuous stirring for 30 min. After that, NH3•H2O (5 mL, 1 M) and H2O2 (1.5 mL, 30%) were sequentially injected and synthesized for 3 h. Afterward, NaOH (1.0 mL, 1 M) was added under ultrasound. Finally, the CaO2 NPs were purified by centrifugation (13,000 rpm, 10 min) and sequentially washed with NaOH solution, pure water, and anhydrous ethanol three times.
2.3 Synthesis of HA/CaO2-Ce6@Cu-ZIF
The HA/CaO2-Ce6@Cu-ZIF was prepared via an unfussy one-step method. A specific Zn(NO3)2•6H2O (300 mg) and Cu(NO3)2•3H2O (50 mg) were dissolved in methanol (100 mL) and formed an uniform solution. Then, 2-MeIM (190 mg), HA-stabilized CaO2 NPs (50 mg), and Ce6 (20 mg) dissolved in the methanol solution (100 mL) were added drip by drip and reacted for at least 30 min under N2 atmosphere. Finally, the HA/CaO2-Ce6@Cu-ZIF was collected by centrifugation (13,000 rpm, 10 min) and washed with methanol three times.
2.4 Characterizations
TME images and corresponding elemental mapping were collected from Tecnai T20 at an accelerating voltage of 200 kV. The size of nanoparticles was calculated using Image J for 100 counting number. XRD patterns were obtained from Bruker D8 ADVANCE (Cu Kα radiation (λ = 0.154 nm) at 40 kV and 40 mA. Zeta potential and DLS measurements were gained by Zetasizer Ultra with He-Ne laser (633 nm). UV-vis absorption spectra were acquired from Shimadzu UV-1601. XPS spectra were analyzed from Rigaku DMAX-2400. FT-IR spectrum was accumulated from Nicolet Avatar 360 with the KBr wafer technique. ICP-OES measurements were surveyed from iCAP 6000 series. CLSM images were captured from Leica SP8. Flow cytometry was measured using BD accuri C6.
2.5 ROS generation estimation
The generation of •OH was analyzed by TMB chromogenic reaction in pH, concentration, and time-dependent manners. The generation of 1O2 was determined by the DPBF chemical probe.
2.6 In vitro experiments
Cellular uptake of as-synthesized materials was operated on Panc02 cells. Cells were seeded in 6-well plates with a density of 1 × 105 cells per well. The MTT cell assay was employed to evaluate the biocompatibility and toxicity of as-synthesized materials on L929 and Panc02 cells, respectively. Moreover, the live/dead cell assay was conducted to verify the cytotoxicity of the material on Panc02 cells. For intracellular ROS detection, a DCFH-DA chemical fluorescence probe was used. For the mitochondrial integrity assay, JC-1 staining kits were used to determine the J-monomer and J-aggregates separately. The intracellular fluorescence was observed by CLSM.
2.7 In vivo experiments
To investigate the biodistribution, the Panc02 tumor-bearing C57BL/6 mice were intravenously administered as-synthesized materials. For biodistribution investigation, the mice were sacrificed after 0, 2, 6, 12, 24, and 48 h. The heart, liver, lungs, spleen, kidneys, and tumors were collected for Cu contraction measurement. To estimate the anti-tumor efficacy of as-synthesized materials, the Panc02 tumor-bearing C57BL/6 mice were randomly placed into five groups (n = 5): control, CaO2, CaO2@Cu-ZIF, HA/CaO2-Ce6@Cu-ZIF, and HA/CaO2-Ce6@Cu-ZIF + Laser. During the treatment process, the tumor sizes and weights of mice were recorded once every 2 days: tumor volume = (tumor length) × (tumor width)2/2 (mm3).
2.8 Histology examination
After treatment process, the tumor and main organs (heart, liver, spleen, lung, and kidney) were collected for (H&E) staining according to the standard protocol for confirming caused injury.
2.9 Statistical analysis
All results were presented as mean ± S.D. Means were indicated using the student’s t-test. Statistical significance was determined at a value of *p < 0.05, **p < 0.01, ***p < 0.001.
3 Results and discussion
3.1 Characterization of HA/CaO2-Ce6@Cu-ZIF nanoplatform
The synthesis of HA/CaO2-Ce6@Cu-ZIF was done through a two-step process. At first, CaO2 NPs were synthesized through a hydrolysis-precipitation process. Then, the HA/CaO2-Ce6@Cu-ZIF was synthesized through a simple one-step method. In detail, a specific Zn(NO3)2•6H2O and Cu(NO3)2•3H2O were dissolved in methanol and formed a uniform solution. Following this, 2-MeIM, HA-stabilized CaO2 NPs, and Ce6 dissolved in the methanol solution were added drop by drop and reacted for 30 min to obtain HA/CaO2-Ce6@Cu-ZIF. As revealed by transmission electron microscopy (TEM), the CaO2 NPs are about 90 ± 2.3 nm, demonstrating the uniform size distribution. (Figures 1A, B). X-ray diffraction (XRD) pattern reveals that the synthesized CaO2 NPs show obvious peaks at 30.1°, 35.6°, and 47.3° (Figure 1F), which is consistent with the JCPDS, No. 03-0865 according to previous literature for CaO2. (Sun et al., 2021a). After this, Cu-ZIF was utilized to encapsulate the CaO2 NPs and Ce6 via a self-assembly method to obtain the HA/CaO2-Ce6@Cu-ZIF nanoplatform. The TEM image shows that the HA/CaO2-Ce6@Cu-ZIF presents a regular octahedral shape with a particle size of around 110 ± 3.8 nm (Figures 1C, D). The homogeneous distributions of Zn, Cu, Ca, N, and O elements in HA/CaO2-Ce6@Cu-ZIF are revealed by the elemental mapping, which demonstrates the successful loading of CaO2 NPs (Figure 1E). Moreover, the XRD pattern of HA/CaO2-Ce6@Cu-ZIF is consistent with that of ZIF-8, indicating that the as-synthesized materials are well held in the crystal structure of ZIF-8 (Figure 1F). (Li et al., 2021b) To endow the CaO2-Ce6@Cu-ZIF with higher hydrophilicity for further biological application, HA with superior biocompatibility and targeted ability was employed for surface modification. As displayed in Figure 1G, the zeta potentials of CaO2, CaO2@Cu-ZIF, CaO2-Ce6@Cu-ZIF, and HA/CaO2-Ce6@Cu-ZIF are −20.03, +10.12, +23.9, and −25.6 mV, respectively, indicating that the CaO2 NPs and Ce6 are successfully introduced into the Cu-ZIF and HA are effectively modified on the surface of as-synthesized materials. Meanwhile, the size distribution of CaO2, CaO2-Ce6@Cu-ZIF, and HA/CaO2-Ce6@Cu-ZIF was obtained from the dynamic light scattering (DLS) measurements, the polydispersity index of which was 0.18, 0.19, and 0.17, respectively, demonstrating the good stable ability of HA modification. Figure 1I shows the hydrodynamic diameter is 142, 164, and 220 nm, respectively. The Fourier transform infrared (FT-IR) spectrum was recorded in the wavelength range of 500–4,000 cm–1 (Figure 1H), also suggesting the sequential addition of CaO2, Ce6, and HA, finally forming HA/CaO2-Ce6@Cu-ZIF. (Li et al., 2021a). As shown in X-ray photoelectron spectroscopy (XPS), HA/CaO2-Ce6@Cu-ZIF was also performed to evaluate the valence electron distribution, and the spectra are presented in which the coexistence of Zn, Cu, Ca, N, and O signals appear (Figure 1J). The high-resolution XPS of Zn, Cu, and Ca are shown in Figures 1K–M. In the high-resolution XPS of Cu spectrum, 933.3 and 953.6 eV peaks are assigned to Cu 2P3/2 and Cu 2p1/2, respectively. In addition, the satellite peaks at around 943.1 eV demonstrate the presence of Cu2+. (Li et al., 2021b). All the above materials’ characterizations imply the rational design and synthesis of H2O2/O2 self-supply and Ca2+ overloading MOF-based nanoplatform.
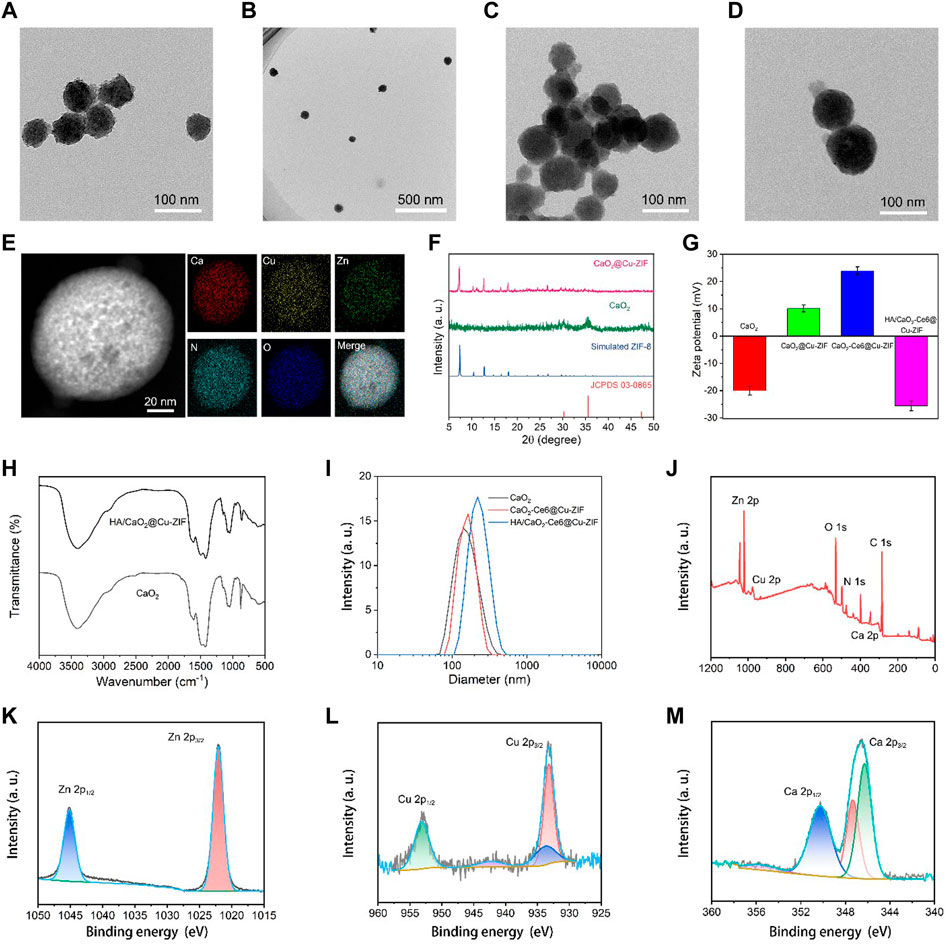
FIGURE 1. TEM images of (A,B) CaO2 NPs and (C,D) CaO2@Cu-ZIF. (E) Corresponding area-elemental mapping of CaO2@Cu-ZIF. (F) XRD patterns of stimulated ZIF-8, CaO2, and CaO2@Cu-ZIF. (G) Zeta potentials of CaO2, CaO2@Cu-ZIF, CaO2-Ce6@Cu-ZIF, and HA/CaO2-Ce6@Cu-ZIF. (H) FT-IR spectrum of CaO2 and HA/CaO2-Ce6@Cu-ZIF. (I) DLS of CaO2, CaO2-Ce6@Cu-ZIF, and HA/CaO2-Ce6@Cu-ZIF. (J) XPS spectrum of HA/CaO2-Ce6@Cu-ZIF. (K–M) High-resolution XPS spectrum of Zn, Cu, and Ca, respectively.
3.2 CDT/PDT synergistic effect of HA/CaO2-Ce6@Cu-ZIF nano platform
The stability experiments of HA/CaO2-Ce6@Cu-ZIF show that the as-synthesized materials maintain good dispersion within 7 days in cell medium (Figure 2A).The ultraviolet-visible (UV-vis) absorption spectra of Ce6, CaO2, CaO2@Cu-ZIF, and HA/CaO2-Ce6@Cu-ZIF was shown in Figure 2B. Compared with the broad peak of CaO2 and CaO2@Cu-ZIF ranging from 450 to 800 nm, the absorption band of HA/CaO2-Ce6@Cu-ZIF not only has the broad peak of CaO2@Cu-ZIF but also exhibits the typical characteristic peak of Ce6 around 650 nm. Encouraged by the results from the photo-properties of HA/CaO2-Ce6@Cu-ZIF, the 1O2 generation of PDT effect was explored by the UV-vis spectrum, where the 1,3-diphenylisobenzofuran (DPBF) was used as a real-time probe. The HA/CaO2-Ce6@Cu-ZIF and PBS solutions were irradiated by 650 nm laser (0.5 W/cm2), respectively. At first, the HA/CaO2-Ce6@Cu-ZIF could release Ce6 under acidic conditions. Then, DPBF could be oxidized by 1O2 which was generated from the combination of the released Ce6, light, and self-supplying O2, so that the absorption peak of the DPBF (the specific absorption wavelength was at 410 nm) gradually decreased along with time increase (Figure 2C). However, the absorption peak of the DPBF solution that was treated with PBS was almost unchanged (Figure 2D). The relative intensity value of the UV-vis absorption peak at 410 nm for DPBF mixed with HA/CaO2-Ce6@Cu-ZIF and PBS, respectively, further demonstrates the apparent decrease of DPBF absorption intensity (Figure 2E). To further confirm the production of 1O2, the 2′,7′-dichlorodihydrofluorescein diacetate (DCFH-DA) was also used (Figure 2F). And the results are consistent with the above. For •OH detection, a typical colorimetric analysis based on 3,3′,5,5′-tetramethyl-benzidine (TMB) was utilized to investigate the CDT effect of HA/CaO2-Ce6@Cu-ZIF. HA/CaO2-Ce6@Cu-ZIF can catalyze the oxidation of TMB to yield blue-colored oxTMB with typical absorbances at 370 and 652 nm. Considering the biodegradable properties related to the pH value of HA/CaO2-Ce6@Cu-ZIF, the influence of the pH on •OH generation was first analyzed (pH = 4.5, 5.5, 6.5, and 7.4). The result shows that the pH has a significant influence on the •OH generation (Figure 2G). There is no evident •OH generation at pH 7.4, while the ability of •OH generation remarkably increases with the downregulation of pH. Then the concentration effect of HA/CaO2-Ce6@Cu-ZIF for •OH generation was also investigated (Figure 2H). It shows an advanced ability of •OH generation along with the increased concentration (5, 15, and 20 μg/mL under pH 6.5). The •OH generation ability of HA/CaO2-Ce6@Cu-ZIF related to time was also investigated (Figure 2I).
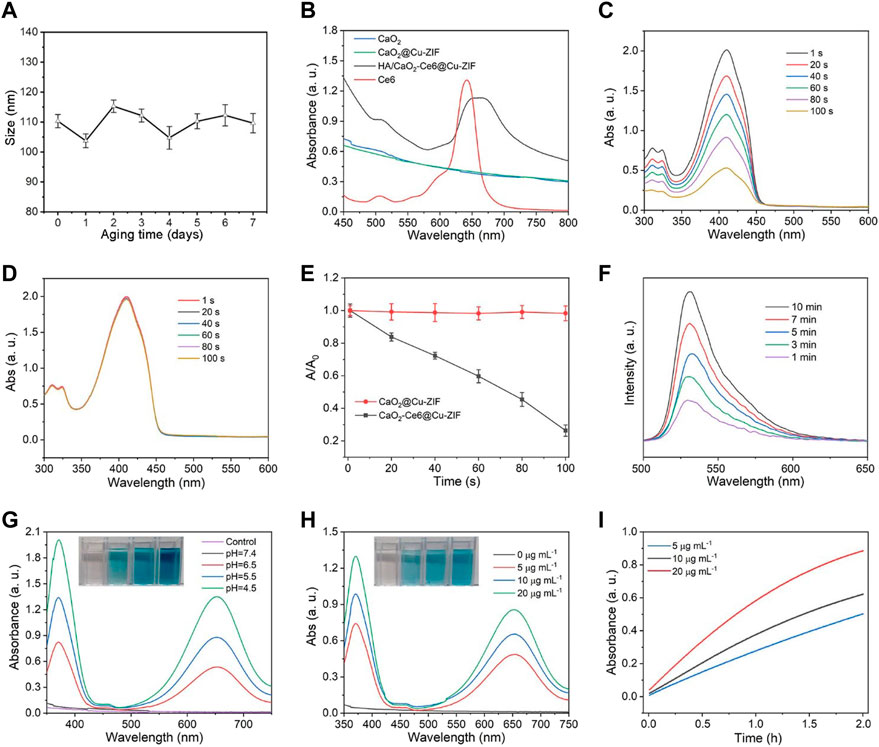
FIGURE 2. (A) Particle sizes of HA/CaO2-Ce6@Cu-ZIF within 7 days in cell medium. (B) UV-vis absorption spectra of Ce6, CaO2, CaO2@Cu-ZIF, and HA/CaO2-Ce6@Cu-ZIF. (C) UV-vis absorption of DPBF mixed with HA/CaO2-Ce6@Cu-ZIF as a function of reaction time under 650 nm laser irradiation. (D) UV-vis absorption of DPBF mixed with PBS at same condition. (E) Relative intensity value of UV-vis absorption peak at 410 nm for DPBF mixed with HA/CaO2-Ce6@Cu-ZIF and PBS, respectively. (F) Fluorescence spectra of DCFH-DA mixed with HA/CaO2-Ce6@Cu-ZIF under 650 nm laser irradiation for different time. (G) UV-vis spectra of TMB + HA/CaO2-Ce6@Cu-ZIF under pH at 4.5, 5.5, 6.5, and 7.4. (H) UV-vis spectra of TMB + HA/CaO2-Ce6@Cu-ZIF at the concentration of HA/CaO2-Ce6@Cu-ZIF as 0, 5, 15, and 20 μg/mL under pH 6.5. (I) UV-vis absorption peak at 650 nm for TMB + HA/CaO2-Ce6@Cu-ZIF at the concentration of HA/CaO2-Ce6@Cu-ZIF as 5, 15, and 20 μg/mL with different times. All laser pump powers are 0.5 W/cm2.
3.3 Invitro experiments of HA/CaO2-Ce6@Cu-ZIF nanoplatform
Given the successful construction of HA/CaO2-Ce6@Cu-ZIF and advanced ROS generation capacity, the therapeutic effect of HA/CaO2-Ce6@Cu-ZIF against Panc02 cells in vitro was further investigated. The therapeutic performance was first examined through the calcein-AM and propidium iodide (PI) double-staining assay (Figure 3A). The confocal laser scanning microscopy (CLSM) images show that the HA/CaO2-Ce6@Cu-ZIF + Laser group exhibits the highest red-green ratio, where the red represents dead cells and green represents living cells, indicating the excellent anti-cancer effect of HA/CaO2-Ce6@Cu-ZIF. Meanwhile, the flow cytometric apoptosis assay with Annexin V-FITC and PI staining was used to calculate the apoptotic cell death mediated by HA/CaO2-Ce6@Cu-ZIF. The apoptotic ratio induced by HA/CaO2-Ce6@Cu-ZIF under irradiation was 51.83% (the sum of Q2 and Q3), which was markedly higher than other groups under the same condition. This is mainly attributed to synergistic H2O2/O2 self-supplying CDT/PDT synergistic effect. The intracellular ROS triggered by HA/CaO2-Ce6@Cu-ZIF under laser irradiation was further investigated using a 2,7-dichlorofluorescein diacetate (DCFH-DA) probe, which can be hydrolyzed to DCFH. This can be rapidly oxidized by the generated ROS and form DCF with green-fluorescent (excited by 488 nm). The CLSM images exhibit that there is almost no green fluorescence in the control and CaO2 groups. On the contrary, weak green fluorescence is exhibited in CaO2@Cu-ZIF and HA/CaO2-Ce6@Cu-ZIF groups. The strongest green fluorescence in the HA/CaO2-Ce6@Cu-ZIF + Lase group indicates that HA/CaO2-Ce6@Cu-ZIF under laser irradiation could generate more toxic ROS to induce tumor cell death (Figure 3B). The cytocompatibility of HA/CaO2-Ce6@Cu-ZIF on L929 normal cells was evaluated by the 3-(4,5-dimethylthiazol-2-yl)-2,5-diphenyltetrazolium bromide (MTT) method (tetramethylazole salt microenzyme reaction colorimetric assay). As shown in Supplementary Figure S1, HA/CaO2-Ce6@Cu-ZIF does not exhibit significant cytotoxicity to L929 cells, and the viability of cells treated with as-synthesized material for 24 h was 92.5% even at a concentration of 500 μg/mL, demonstrating the “silent” HA in the normal cellular microenvironment. Afterward, MTT assay was also used to estimate the cytotoxicity on Panc02 cells. Compared with others, the inhibition rate of HA/CaO2-Ce6@Cu-ZIF under laser irradiation is as high as 53.5%, where the concentration of HA/CaO2-Ce6@Cu-ZIF is 200 μg/mL (Figure 3C). Given that the Ca2+ overloading originating from CaO2 could further enhance the oxidative stress and result in mitochondrial dysfunction, the mitochondrial integrities of different treatment groups were examined through JC-1 staining flow cytometry (Figure 3D). The qualitative comparison of J-monomer (green) and J-aggregates (red) following various treatments shows that the group treated with HA/CaO2-Ce6@Cu-ZIF under laser irradiation exhibits abundant mitochondria damage. The endocytosis process of HA/CaO2-Ce6@Cu-ZIF in Panc02 cells was evaluated using specific fluorescence properties of Ce6. As is known, when excited with 488 nm light, the loaded Ce6 can radiate green fluorescence. As shown in Figure 3E, the results suggest that HA/CaO2-Ce6@Cu-ZIF could be effectively endocytosed by Panc02 cells and the internalization amount increased with prolonged time.
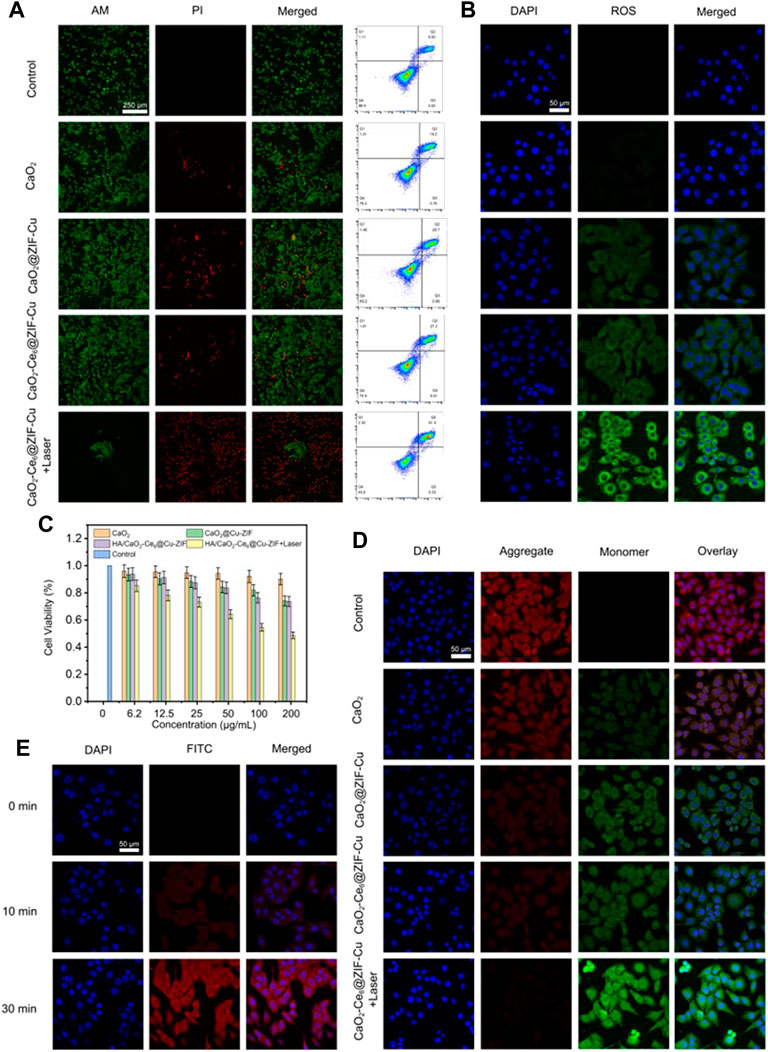
FIGURE 3. (A) Calcein-AM/PI double staining of Panc02 cells with different treatments and corresponding flow cytometry analysis by annexin V-FITC apoptosis detection kit. (B) Intracellular ROS level of Panc02 cells with different treatments. (C) Relative cell viabilities of Panc02 cells after treatment with different samples. (D) JC-1 staining of Panc02 cells after different treatments. (E) CLSM images of Panc02 cells incubated with HA/CaO2-Ce6@Cu-ZIF for different times.
3.4 In vivo experiments of HA/CaO2-Ce6@Cu-ZIF nanoplatform
Inspired by the promising in vitro CDT/PDT synergistic effect of HA/CaO2-Ce6@Cu-ZIF nanoplatform, the in vivo therapeutic assay in Panc02 tumor-bearing C57BL/6 mice model was conducted. When the tumor sizes reached about 100 mm3, twenty-five Panc02 tumor-bearing mice were randomly divided into five groups, followed by treatments: control, CaO2, CaO2@Cu-ZIF, HA/CaO2-Ce6@Cu-ZIF, and v) HA/CaO2-Ce6@Cu-ZIF + Laser. As depicted in Figure 4A, Panc02 tumor-bearing mice were treated by intravenous administration on 1 and 7 days with injection doses of 15 mg/kg of mouse body weight. The body weight (Figure 4B) and tumor volume (Figure 4C) of mice were measured every 2 days during the treatment process. Moreover, the time-dependent Cu biodistribution of HA/CaO2-Ce6@Cu-ZIF at the tumor and major organs were evaluated (Figure 4D). The results indicate an effective accumulation of HA/CaO2-Ce6@Cu-ZIF at the tumor site, ensuring the following synergistic CDT/PDT therapeutics. In Figure 4B, during the treatment period, all the mice feature slight weight increases, demonstrating the negligible negative impacts of these treatments on the health of mice. As exhibited in Figure 4C, the relative tumor volume was notably suppressed in HA/CaO2-Ce6@Cu-ZIF + Laser group in comparison with the other groups. Specifically, the suppression rate of the HA/CaO2-Ce6@Cu-ZIF + Laser group was determined to be 60.8%, calculated from the variation in the relative tumor volume. This high suppression is attributed to the HA/CaO2-Ce6@Cu-ZIF induced cascade-amplified CDT/PDT therapy as follows: 1) CaO2 decomposed to generate H2O2 and O2, which alleviated the insufficiency of intracellular H2O2 and relieved hypoxia conditions in TME; 2) H2O2/O2 self-supplying effectively enhanced the production of •OH and 1O2 in Cu2+-mediated CDT and Ce6-induced PDT, respectively and 3) Ca2+ originated from CaO2 could further enhance the oxidative stress and result in mitochondrial dysfunction induced by Ca2+ overloading. Intensive therapeutic efficacy was also confirmed by hematoxylin and eosin (H&E) staining of tumor sections from each group (Figure 4E). The results were consistent with the above tumor growth data. Additionally, the histological observations of major organs (heart, liver, spleen, lung, and kidney) present negligible acute pathological toxicities and adverse effects during the treatment duration for the control or treated groups (Figure 5). These results demonstrate that HA/CaO2-Ce6@Cu-ZIF is of high biocompatibility.
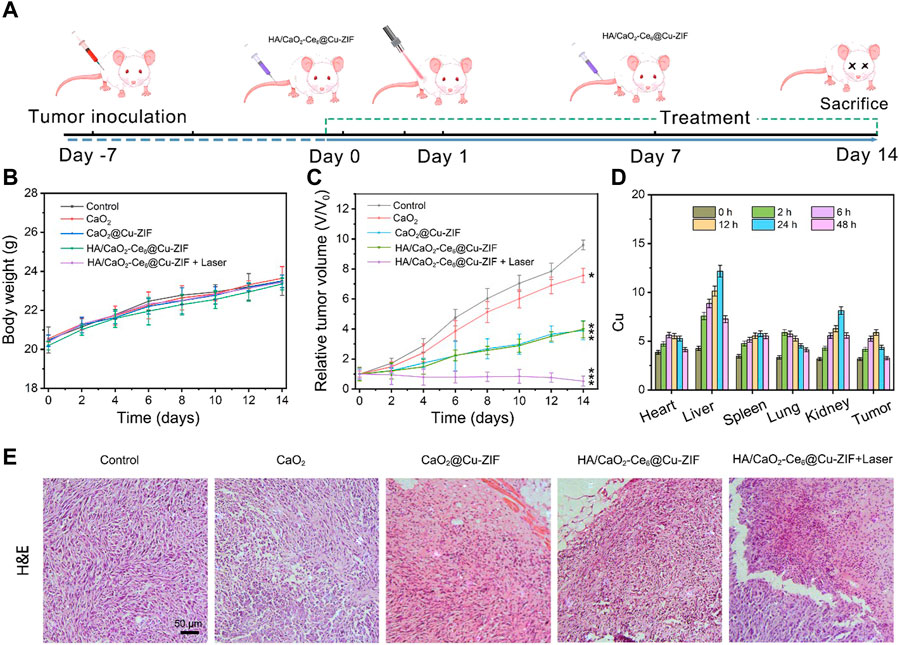
FIGURE 4. (A) Schematic illustration of Panc02 tumor-bearing mice model and treatment process. (B) Body weights and (C) relative tumor volume change curves of Panc02 tumor-bearing mice after various treatments. (D) Biodistribution of HA/CaO2-Ce6@Cu-ZIF in main organs and tumors at different time points (n = 3). (E) H&E-stained photographs of tumor slices obtained from Panc02 tumor-bearing mice in different groups after treatment.
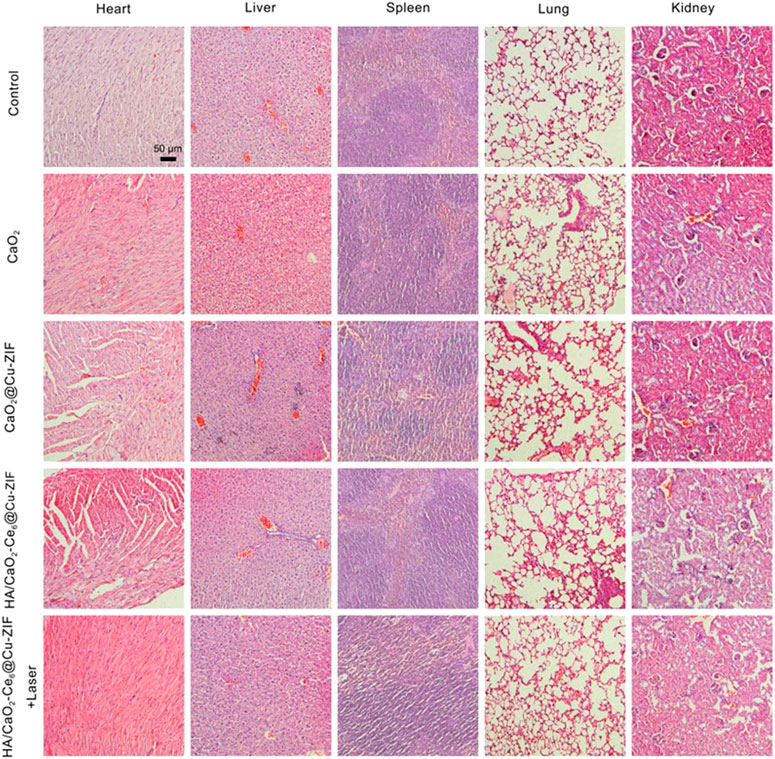
FIGURE 5. Representative H&E tissue sections from mice to monitor the histological change in heart, liver, spleen, lung, and kidney excised from different groups after treatment.
4 Conclusion
In summary, a biodegradable HA/CaO2-Ce6@Cu-ZIF nanoplatform was rationally constructed for a H2O2/O2 self-supplying and Ca2+ overloading CDT/PDT synergistic strategy. After arriving at tumor sites via the specific HA targeted effect, HA/CaO2-Ce6@Cu-ZIF responded to acidic conditions in TME and released CaO2 NPs and Ce6, as well as exposed Cu2+ active sites within Cu-ZIF. The released CaO2 NPs further decomposed to efficiently generate H2O2 and O2 simultaneously for enhancing •OH and 1O2 production in Cu2+-mediated CDT and Ce6-participated PDT, respectively. In addition, the accompanying Ca2+ overloading generated by the decomposition of CaO2 NPs could induce mitochondrial dysfunction in tumor cells, further contributing to the combined CDT/PDT. Thus, this work provides an alternative strategy for smart reprogramming TME to improve the efficacy of synergistic CDT/PDT treatment.
Data availability statement
The original contributions presented in the study are included in the article/Supplementary Material, further inquiries can be directed to the corresponding authors.
Ethics statement
The animal study was reviewed and approved by Guangxi Medical University Cancer Hospital.
Author contributions
Experimental design: YuL; experiments: YaL, ZC, CS, and LX; data analysis: CS, YT, and LX; and manuscript writing: YuL and XL. All authors listed have made a substantial, direct, and intellectual contribution to the work and approved it for publication.
Funding
This study was partially supported by the National Natural Science Foundation (No. 81260083).
Conflict of interest
The authors declare that the research was conducted in the absence of any commercial or financial relationships that could be construed as a potential conflict of interest.
Publisher’s note
All claims expressed in this article are solely those of the authors and do not necessarily represent those of their affiliated organizations, or those of the publisher, the editors and the reviewers. Any product that may be evaluated in this article, or claim that may be made by its manufacturer, is not guaranteed or endorsed by the publisher.
Supplementary material
The Supplementary Material for this article can be found online at: https://www.frontiersin.org/articles/10.3389/fbioe.2023.1196839/full#supplementary-material
SUPPLEMENTARY FIGURE S1 | Biocompatibility of CaO2 @Cu-ZIF and HA/CaO2-Ce6@Cu-ZIF.
References
Bian, Y., Liu, B., Liang, S., Ding, B., Zhao, Y., Jiang, F., et al. (2022). Cu-based MOFs decorated dendritic mesoporous silica as tumor microenvironment responsive nanoreactor for enhanced tumor multimodal therapy. Chem. Eng. J. 435, 135046. doi:10.1016/j.cej.2022.135046
Cai, W., Wang, J., Chu, C., Chen, W., Wu, C., and Liu, G. (2019). Metal-organic framework-based stimuli-responsive systems for drug delivery. Adv. Sci. 6 (1), 1801526. doi:10.1002/advs.201801526
Cao, C., Wang, X., Yang, N., Song, X., and Dong, X. (2021). Recent advances of cancer chemodynamic therapy based on Fenton/Fenton-like chemistry. Chem. Sci. 13, 863–889. doi:10.1039/d1sc05482a
Cao, X., Luo, Q., Song, F., Liu, G., Chen, S., Li, Y., et al. (2023). Effects of oxidative torrefaction on the physicochemical properties and pyrolysis products of hemicellulose in bamboo processing residues. Industrial Crops Prod. 191, 115986. doi:10.1016/j.indcrop.2022.115986
Chang, M., Hou, Z., Wang, M., Li, C., Al Kheraif, A. A., and Lin, J. (2022). Tumor microenvironment responsive single-atom nanozymes for enhanced antitumor therapy. Chemistry 28 (15), e202104081. doi:10.1002/chem.202104081
Chen, Q., Yang, D., Yu, L., Jing, X., and Chen, Y. (2020). Catalytic chemistry of iron-free Fenton nanocatalysts for versatile radical nanotherapeutics. Mater. Horizons 7 (2), 317–337. doi:10.1039/c9mh01565e
Chen, Y. C., Liu, Y. J., Lee, C. L., Pham, K. Y., Manoharan, D., Thangudu, S., et al. (2022). Engineering H2 O2 and O2 self-supplying nanoreactor to conduct synergistic chemiexcited photodynamic and calcium-overloaded therapy in orthotopic hepatic tumors. Adv. Healthc. Mater 11, e2201613. doi:10.1002/adhm.202201613
Deng, K., Li, C., Huang, S., Xing, B., Jin, D., Zeng, Q., et al. (2017). Recent progress in near infrared light triggered photodynamic therapy. Small 13 (44), 1702299. doi:10.1002/smll.201702299
Docampo, R., and Vercesi, A. E. (2022). Mitochondrial Ca(2+) and reactive oxygen species in trypanosomatids. Antioxid. Redox Signal 36 (13-15), 969–983. doi:10.1089/ars.2021.0058
He, J., Fu, L. H., Qi, C., Lin, J., and Huang, P. (2021). Metal peroxides for cancer treatment. Bioact. Mater 6 (9), 2698–2710. doi:10.1016/j.bioactmat.2021.01.026
Hu, Y., Wang, X., Zhao, P., Wang, H., Gu, W., and Ye, L. (2020). Nanozyme-catalyzed oxygen release from calcium peroxide nanoparticles for accelerated hypoxia relief and image-guided super-efficient photodynamic therapy. Biomater. Sci. 8 (10), 2931–2938. doi:10.1039/d0bm00187b
Jiang, F., Zhao, Y., Yang, C., Cheng, Z., Liu, M., Xing, B., et al. (2022). A tumor microenvironment-responsive Co/ZIF-8/ICG/Pt nanoplatform for chemodynamic and enhanced photodynamic antitumor therapy. Dalton Trans. 51 (7), 2798–2804. doi:10.1039/d1dt04120g
Li, X., Sun, H., Li, H., Hu, C., Luo, Y., Shi, X., et al. (2021). Multi-Responsive biodegradable cationic nanogels for highly efficient treatment of tumors. Adv. Funct. Mater. 31 (26), 2100227. doi:10.1002/adfm.202100227
Li, W., Zhou, X., Liu, S., Zhou, J., Ding, H., Gai, S., et al. (2021). Biodegradable nanocatalyst with self-supplying fenton-like ions and H2O2 for catalytic cascade-amplified tumor therapy. Acs Appl. Mater. Interfaces 13 (43), 50760–50773. doi:10.1021/acsami.1c14598
Li, C., Ye, J., Yang, X., Liu, S., Zhang, Z., Wang, J., et al. (2022). Fe/Mn bimetal-doped ZIF-8-coated luminescent nanoparticles with up/downconversion dual-mode emission for tumor self-enhanced NIR-II imaging and catalytic therapy. ACS Nano 16, 18143–18156. doi:10.1021/acsnano.2c05152
Li, X., Hetjens, L., Wolter, N., Li, H., Shi, X., and Pich, A. (2023). Charge-reversible and biodegradable chitosan-based microgels for lysozyme-triggered release of vancomycin. J. Adv. Res. 43, 87–96. doi:10.1016/j.jare.2022.02.014
Liang, S., Xiao, X., Bai, L., Liu, B., Yuan, M., Ma, P., et al. (2021). Conferring Ti-based MOFs with defects for enhanced sonodynamic cancer therapy. Adv. Mater 33, e2100333. doi:10.1002/adma.202100333
Liu, J., Liu, T., Du, P., Zhang, L., and Lei, J. (2019). Metal-organic framework (MOF) hybrid as a tandem catalyst for enhanced therapy against hypoxic tumor cells. Angew. Chem. Int. Ed. 58 (23), 7890–7894. doi:10.1002/ange.201903475
Liu, B., Bian, Y., Yuan, M., Zhu, Y., Liu, S., Ding, H., et al. (2022). L-buthionine sulfoximine encapsulated hollow calcium peroxide as a chloroperoxidase nanocarrier for enhanced enzyme dynamic therapy. Biomaterials 289, 121746. doi:10.1016/j.biomaterials.2022.121746
Liu, B., Bian, Y., Liang, S., Yuan, M., Dong, S., He, F., et al. (2022). One-step integration of tumor microenvironment-responsive calcium and copper peroxides nanocomposite for enhanced chemodynamic/ion-interference therapy. Acs Nano 16 (1), 617–630. doi:10.1021/acsnano.1c07893
Lu, Y., Luo, Q., Jia, X., Tam, J. P., Yang, H., Shen, Y., et al. (2023). Multidisciplinary strategies to enhance therapeutic effects of flavonoids from Epimedii Folium: Integration of herbal medicine, enzyme engineering, and nanotechnology. J. Pharm. Analysis 13 (3), 239–254. doi:10.1016/j.jpha.2022.12.001
Monro, S., Colon, K. L., Yin, H., Roque, J., Konda, P., Gujar, S., et al. (2019). Transition metal complexes and photodynamic therapy from a tumor-centered approach: Challenges, opportunities, and highlights from the development of TLD1433. Chem. Rev. 119 (2), 797–828. doi:10.1021/acs.chemrev.8b00211
Pandey, A., Dhas, N., Deshmukh, P., Caro, C., Patil, P., Luisa García-Martín, M., et al. (2020). Heterogeneous surface architectured metal-organic frameworks for cancer therapy, imaging, and biosensing: A state-of-the-art review. Coord. Chem. Rev. 409, 213212. doi:10.1016/j.ccr.2020.213212
Peng, S., Xiao, F., Chen, M., and Gao, H. (2021). Tumor-microenvironment-Responsive nanomedicine for enhanced cancer immunotherapy. Adv. Sci. (Weinh) 9, e2103836. doi:10.1002/advs.202103836
Qin, Y. T., Peng, H., He, X. W., Li, W. Y., and Zhang, Y. K. (2019). pH-Responsive polymer-stabilized ZIF-8 nanocomposites for fluorescence and magnetic resonance dual-modal imaging-guided chemo-/photodynamic combinational cancer therapy. ACS Appl. Mater Interfaces 11 (37), 34268–34281. doi:10.1021/acsami.9b12641
Ren, S.-Z., Zhu, D., Zhu, X.-H., Wang, B., Yang, Y.-S., Sun, W.-X., et al. (2019). Nanoscale metal–organic-frameworks coated by biodegradable organosilica for pH and redox dual responsive drug release and high-performance anticancer therapy. ACS Appl. Mater. Interfaces 11 (23), 20678–20688. doi:10.1021/acsami.9b04236
Rojas, S., Arenas-Vivo, A., and Horcajada, P. (2019). Metal-organic frameworks: A novel platform for combined advanced therapies. Coord. Chem. Rev. 388, 202–226. doi:10.1016/j.ccr.2019.02.032
Rui, W., Xingshu, L., and Juyoung, Y. (2021). Organelle-targeted photosensitizers for precision photodynamic therapy. ACS Appl. Mater. Interfaces 13, 19543. doi:10.1021/acsami.1c02019
Shan, X., Zhao, Z., Wang, C., Sun, J., He, Z., Luo, C., et al. (2022). Emerging prodrug-engineered nanomedicines for synergistic chemo-phototherapy. Chem. Eng. J. 442, 136383. doi:10.1016/j.cej.2022.136383
Shen, J., Yu, H., Shu, Y., Ma, M., and Chen, H. (2021). A robust ROS generation strategy for enhanced chemodynamic/photodynamic therapy via H2O2/O-2 self-supply and Ca2+ overloading. Adv. Funct. Mater. 31 (50), 2106106. doi:10.1002/adfm.202106106
Sivasubramanian, M., Chuang, Y. C., and Lo, L. W. (2019). Evolution of nanoparticle-mediated photodynamic therapy: From superficial to deep-seated cancers. Molecules 24 (3), 520. doi:10.3390/molecules24030520
Sun, Q., Liu, B., Zhao, R., Feng, L., Wang, Z., Dong, S., et al. (2021). Calcium peroxide-based nanosystem with cancer microenvironment-activated capabilities for imaging guided combination therapy via mitochondrial Ca2+ overload and chemotherapy. Acs Appl. Mater. Interfaces 13 (37), 44096–44107. doi:10.1021/acsami.1c13304
Sun, Q., Liu, B., Wang, Z., Feng, L., Zhao, R., Dong, S., et al. (2021). H2O2/O2 self-supplementing and GSH-depleting Ca2+ nanogenerator with hyperthermia-triggered, TME-responsive capacities for combination cancer therapy. Chem. Eng. J. 425, 131485. doi:10.1016/j.cej.2021.131485
Tao, W., Wang, N., Ruan, J., Cheng, X., Fan, L., Zhang, P., et al. (2022). Enhanced ROS-boosted phototherapy against pancreatic cancer via nrf2-mediated stress-defense pathway suppression and ferroptosis induction. ACS Appl. Mater Interfaces 14 (5), 6404–6416. doi:10.1021/acsami.1c22861
Truong Hoang, Q., Ravichandran, V., Nguyen Cao, T. G., Kang, J. H., Ko, Y. T., Lee, T. I., et al. (2022). Piezoelectric Au-decorated ZnO nanorods: Ultrasound-triggered generation of ROS for piezocatalytic cancer therapy. Chem. Eng. J. 435, 135039. doi:10.1016/j.cej.2022.135039
Wan, Y., Fu, L. H., Li, C., Lin, J., and Huang, P. (2021). Conquering the hypoxia limitation for photodynamic therapy. Adv. Mater 33 (48), e2103978. doi:10.1002/adma.202103978
Wang, L., Huo, M., Chen, Y., and Shi, J. (2018). Tumor microenvironment-enabled nanotherapy. Adv. Healthc. Mater 7 (8), e1701156. doi:10.1002/adhm.201701156
Wang, H., Yu, D., Fang, J., Cao, C., Liu, Z., Ren, J., et al. (2019). Renal-clearable porphyrinic metal–organic framework nanodots for enhanced photodynamic therapy. ACS Nano 13 (8), 9206–9217. doi:10.1021/acsnano.9b03531
Wang, C., Jia, X., Zhen, W., Zhang, M., and Jiang, X. (2019). Small-sized MOF-constructed multifunctional diagnosis and therapy platform for tumor. ACS Biomaterials Sci. Eng. 5 (9), 4435–4441. doi:10.1021/acsbiomaterials.9b00813
Wang, D., Wu, H., Lim, W. Q., Phua, S. Z. F., Xu, P., Chen, Q., et al. (2019). A mesoporous nanoenzyme derived from metal-organic frameworks with endogenous oxygen generation to alleviate tumor hypoxia for significantly enhanced photodynamic therapy. Adv. Mater 31 (27), e1901893. doi:10.1002/adma.201901893
Wang, W., Jin, Y., Xu, Z., Liu, X., Bajwa, S. Z., Khan, W. S., et al. (2020). Stimuli-activatable nanomedicines for chemodynamic therapy of cancer. Wiley Interdiscip. Rev. Nanomed Nanobiotechnol 12, e1614. doi:10.1002/wnan.1614
Wang, H., Gao, L., Fan, T., Zhang, C., Zhang, B., Al-Hartomy, O. A., et al. (2021). Strategic design of intelligent-responsive nanogel carriers for cancer therapy. ACS Appl. Mater Interfaces 13 (46), 54621–54647. doi:10.1021/acsami.1c13634
Wang, Z., Sun, Q., Liu, B., Kuang, Y., Gulzar, A., He, F., et al. (2021). Recent advances in porphyrin-based MOFs for cancer therapy and diagnosis therapy. Coord. Chem. Rev. 439, 213945. doi:10.1016/j.ccr.2021.213945
Wang, W., Pan, X., Yang, H., Wang, H., Wu, Q., Zheng, L., et al. (2021). Bioactive metal-organic frameworks with specific metal-nitrogen (M-N) active sites for efficient sonodynamic tumor therapy. ACS Nano 15, 20003–20012. doi:10.1021/acsnano.1c07547
Xie, Z., Cai, X., Sun, C., Liang, S., Shao, S., Huang, S., et al. (2019). O2-Loaded pH-responsive multifunctional nanodrug carrier for overcoming hypoxia and highly efficient chemo-photodynamic cancer therapy. Chem. Mater. 31 (2), 483–490. doi:10.1021/acs.chemmater.8b04321
Xie, Z., Liang, S., Cai, X., Ding, B., Huang, S., Hou, Z., et al. (2019). O2-Cu/ZIF-8@Ce6/ZIF-8@F127 composite as a tumor microenvironment-responsive nanoplatform with enhanced photo-/chemodynamic antitumor efficacy. ACS Appl. Mater. Interfaces 11, 31671. doi:10.1021/acsami.9b10685
Yang, P., Men, Y., Tian, Y., Cao, Y., Zhang, L., Yao, X., et al. (2019). Metal-organic framework nanoparticles with near-infrared dye for multimodal imaging and guided phototherapy. ACS Appl. Mater Interfaces 11 (12), 11209–11219. doi:10.1021/acsami.9b01286
Yang, B., Chen, Y., and Shi, J. (2019). Reactive oxygen species (ROS)-Based nanomedicine. Chem. Rev. 119 (8), 4881–4985. doi:10.1021/acs.chemrev.8b00626
Yang, G., Phua, S. Z. F., Lim, W. Q., Zhang, R., Feng, L., Liu, G., et al. (2019). A hypoxia-responsive albumin-based nanosystem for deep tumor penetration and excellent therapeutic efficacy. Adv. Mater 31 (25), e1901513. doi:10.1002/adma.201901513
Yang, X. X., Feng, P., Cao, J., Liu, W., and Tang, Y. (2020). Composition-engineered metal-organic framework-based microneedles for glucose-mediated transdermal insulin delivery. ACS Appl. Mater Interfaces 12 (12), 13613–13621. doi:10.1021/acsami.9b20774
Yang, Y., Wu, H., Liu, B., and Liu, Z. (2021). Tumor microenvironment-responsive dynamic inorganic nanoassemblies for cancer imaging and treatment. Adv. Drug Deliv. Rev. 179, 114004. doi:10.1016/j.addr.2021.114004
Yang, L., Shaojie, Z., Xingwu, J., Yanyan, L., Kun, W., Chaochao, W., et al. (2021). Intracellular mutual promotion of redox homeostasis regulation and iron metabolism disruption for enduring chemodynamic therapy. Adv. Funct. Mater. 31, 2010390. doi:10.1002/adfm.202010390
Yu, Y., Wu, S., Zhang, L., Xu, S., Dai, C., Gan, S., et al. (2022). Cationization to boost both type I and type II ROS generation for photodynamic therapy. Biomaterials 280, 121255. doi:10.1016/j.biomaterials.2021.121255
Zhang, Z., Sang, W., Xie, L., and Dai, Y. (2019). Metal-organic frameworks for multimodal bioimaging and synergistic cancer chemotherapy. Coord. Chem. Rev. 399, 213022. doi:10.1016/j.ccr.2019.213022
Zhang, Y., Bo, S., Feng, T., Qin, X., Wan, Y., Jiang, S., et al. (2019). A versatile theranostic nanoemulsion for architecture-dependent multimodal imaging and dually augmented photodynamic therapy. Adv. Mater 31 (21), e1806444. doi:10.1002/adma.201806444
Zhang, M., Song, R., Liu, Y., Yi, Z., Meng, X., Zhang, J., et al. (2019). Calcium-overload-mediated tumor therapy by calcium peroxide nanoparticles. Chem 5 (8), 2171–2182. doi:10.1016/j.chempr.2019.06.003
Zhang, Y., Zhang, X., Yang, H., Yu, L., Xu, Y., Sharma, A., et al. (2021). Advanced biotechnology-assisted precise sonodynamic therapy. Chem. Soc. Rev. 50, 11227. doi:10.1039/d1cs00403d
Zhang, S., Liu, Y., Cao, Y., Zhang, S., Sun, J., Wang, Y., et al. (2022). Targeting the microenvironment of vulnerable atherosclerotic plaques: An emerging diagnosis and therapy strategy for atherosclerosis. Adv. Mater 34 (29), e2110660. doi:10.1002/adma.202110660
Zhao, X., Zhang, Z., Cai, X., Ding, B., Sun, C., Liu, G., et al. (2019). Postsynthetic ligand exchange of metal–organic framework for photodynamic therapy. ACS Appl. Mater. Interfaces 11 (8), 7884–7892. doi:10.1021/acsami.9b00740
Zhao, P., Jiang, Y., Tang, Z., Li, Y., Sun, B., Wu, Y., et al. (2021). Constructing electron levers in perovskite nanocrystals to regulate the local electron density for intensive chemodynamic therapy. Angew. Chem. Int. Ed. Engl. 60 (16), 8905–8912. doi:10.1002/anie.202100864
Zhao, L. P., Chen, S. Y., Zheng, R. R., Kong, R. J., Rao, X. N., Chen, A. L., et al. (2021). Self-delivery nanomedicine for glutamine-starvation enhanced photodynamic tumor therapy. Adv. Healthc. Mater 11, e2102038. doi:10.1002/adhm.202102038
Zheng, P., Ding, B., Zhu, G., Li, C., and Lin, J. (2022). Biodegradable Ca2+ nanomodulators activate pyroptosis through mitochondrial Ca2+ overload for cancer immunotherapy. Angew. Chem. Int. Ed. Engl. 134. doi:10.1002/ange.202204904
Keywords: CaO2, MOFs, H2O2/O2 self-supply, chemodynamic therapy, photodynamic therapy
Citation: Liang Y, Cai Z, Tang Y, Su C, Xie L, Li Y and Liang X (2023) H2O2/O2 self-supply and Ca2+ overloading MOF-based nanoplatform for cascade-amplified chemodynamic and photodynamic therapy. Front. Bioeng. Biotechnol. 11:1196839. doi: 10.3389/fbioe.2023.1196839
Received: 30 March 2023; Accepted: 05 May 2023;
Published: 24 May 2023.
Edited by:
Xin Li, Leibniz Institute for Interactive Materials (DWI), GermanyReviewed by:
Muhammad Nafees, University of Engineering and Technology, PakistanSe-Hyeong Jung, ETH Zürich, Switzerland
Yi Lu, RWTH Aachen University, Germany
Copyright © 2023 Liang, Cai, Tang, Su, Xie, Li and Liang. This is an open-access article distributed under the terms of the Creative Commons Attribution License (CC BY). The use, distribution or reproduction in other forums is permitted, provided the original author(s) and the copyright owner(s) are credited and that the original publication in this journal is cited, in accordance with accepted academic practice. No use, distribution or reproduction is permitted which does not comply with these terms.
*Correspondence: Yan Li, bGl5YW4yNkBneG11LmVkdS5jbg==; Xinqiang Liang, eHgwMzcxNkAxNjMuY29t