- 1Program of Molecular and Cellular Biology, Institute of Biomedical Sciences, Faculty of Medicine, Universidad de Chile, Santiago, Chile
- 2Millennium Nucleus of Ion Channel-Associated Diseases (MiNICAD), Santiago, Valdivia, Chile
- 3Institute of Health Sciences, Universidad de O’Higgins, Rancagua, Chile
- 4Institute of Physiology, Faculty of Medicine, Universidad Austral de Chile, Valdivia, Chile
Ion channels are integral membrane proteins mediating ion flow in response to changes in their environment. Among the different types of ion channels reported to date, the super-family of TRP channels stands out since its members have been linked to many pathophysiological processes. The family comprises 6 subfamilies and 28 members in mammals, which are widely distributed throughout most tissues and organs and have an important role in several aspects of cellular physiology. It has been evidenced that abnormal expression, post-translational modifications, and channel trafficking are associated with several pathologies, such as cancer, cardiovascular disease, diabetes, and brain disorders, among others. In this review, we present an updated summary of the mechanisms involved in the subcellular trafficking of TRP channels, with a special emphasis on whether different post-translational modifications and naturally occurring mutagenesis affect both expression and trafficking. Additionally, we describe how such changes have been associated with the development and progress of diverse pathologies associated with the gain or loss of functional phenotypes. The study of these processes will not only contribute to a better understanding the role of TRP channels in the different tissues but will also present novel possible therapeutic targets in diseases where their activity is dysregulated.
Introduction
The super-family of TRP ion channels
The TRP superfamily of ion channels is composed of 28 members that are subdivided into six subfamilies in mammals. TRP channels are characterized by their low sequence homology and family clustering based on overall structural similarities (Clapham, 2003; Clapham et al., 2003; Venkatachalam and Montell, 2007; Cabezas-Bratesco et al., 2022).
The different TRP subfamilies are TRPC (canonical), TRPV (vanilloid), TRPM (melastatin), TRPA (ankyrin), TRPP (polycystin), and TRPML (mucolipin) (Ramsey et al., 2006). These families can be divided into two large groups, where TRPC, V, M, and A form the Class I group while polycystins and mucolipins form Class II group of TRP channels (Venkatachalam and Montell, 2007). Here, we will focus on the Class I type expressed in humans.
The first highlights in the discovery of TRP channels originated from observations of a phenotypic characteristic in a mutant strain of the fruit fly Drosophila (Cosens and Manning, 1969), where this mutant strain exhibited abnormal responses to light stimuli, indicating potential involvement of specific genes in sensory transduction processes. It was not until 1989 that Montell and Rubin assigned an identity using cDNA libraries obtained from mRNA extracted from the heads of adult Drosophila. They managed to identify the mRNA, which they named TRP (Montell and Rubin, 1989). Later, in 1992, they provided the first evidence that TRP proteins function as ion channels (Hardie and Minke, 1992).
TRP channels are tetrameric, non-selective, cation permeating channels structurally similar to voltage-gated potassium channels (Kalia and Swartz, 2013). Each TRP channel protomer comprises six transmembrane segments (S1–6), a pore region between S5 and S6, and intracellularly located N- and C-terminal domains, reviewed in (Cao, 2020; Zhang et al., 2023).
These channels can form homotetramers and/or heterotetramers depending on whether the subunits are identical or present one or more subunits from other TRP channels (Nilius, 2007; Gaudet, 2008; Cabezas-Bratesco et al., 2022). Furthermore, these channels interact with several accessory proteins that can regulate their expression and sub-cellular localization (Moiseenkova-Bell and Wensel, 2011; Cabezas-Bratesco et al., 2022; Cai and Chen, 2023).
Different mechanisms can regulate TRP channels, but in this review, we will focus on regulating the amount of TRP ion channels in the cell surface or trafficking to/from the plasma membrane (Royle and Murrell-Lagnado, 2003). In this context, the localization of an ion channel at the cell surface is determined partly by the balance between its synthesis and degradation, which occurs on a relatively slow time scale, as well as by its constitutive and regulated trafficking mechanisms (Bezzerides et al., 2004; Ambudkar, 2007; Dong et al., 2010; Toro et al., 2011). One of the main regulatory mechanisms of ion channels involves modulating the number of channel molecules expressed at the cell surface, either through the synthesis of new channels or the degradation of existing ones. However, if rapid regulation is needed, it can be achieved by either inserting or removing already synthesized channels stored in intracellular vesicles, which makes trafficking an important mechanism for regulating the activity of ion channels in a rapid fashion (Royle and Murrell-Lagnado, 2003). It is known that the expression of surface proteins can be regulated in various ways, one of which might be due to an increase in protein retention through its interaction with scaffold proteins during or after their insertion into the plasma membrane (Altschuler et al., 2003; Yu et al., 2011; Zhao et al., 2015; Van den Eynde et al., 2021). While the surface expression of ion channels requires the fusion of transporting vesicles mediated by the exocytic pathway, their internalization mechanisms require the activation of endocytic pathways (Royle and Murrell-Lagnado, 2003; Estadella et al., 2020).
Concerning the endocytosis of ion channels, the mechanism relies on various proteins and lipids that are present in specific domains of the plasma membrane, such as clathrin and caveolin, the soluble N-ethylmaleimide-sensitive factor attachment receptor protein (SNARE), or phosphoinositides such as PIP2, (Jahn et al., 2003; Laude and Prior, 2004). Different studies have shown that TRP channels traffic to specific cell regions to perform their function in polarized cells such as in the kidney, gastrointestinal tissue, or neurons. However, the mechanisms involved in this specific destination are still unclear for the most part (Altschuler et al., 2003; Yu et al., 2011; Zhao et al., 2015; Van den Eynde et al., 2021). Thus, a better understanding of the differential trafficking of these proteins could explain, for example, how TRPC6 reaches the basolateral and apical membranes of polarized epithelial cells. Also, TRPC3 is present on the apical side and TRPC1 is found mainly on the basolateral membrane (Singh et al., 2001; Bandyopadhyay et al., 2005; Goel et al., 2007).
This review will focus on the mechanisms reported for the trafficking and surface localization of the different class I TRP channels in mammalian cells, providing insights into the proteins involved in their subcellular targeting and trafficking. Moreover, as defects in the localization of ion channels have often been linked to the development of several pathologies, we will also provide an overview of diseases that have been related to the changes in the localization of these channels.
The TRPC subfamily of ion channels, insights into their trafficking and physiological significance
The TRPC channel subfamily comprises seven members in mammals (TRPC1-7). TRPC2 is a human pseudogene and, therefore, was not considered in this review. Like other members of the TRP channel family (TRPA and TRPV), TRPC channels feature ankyrin repeat sequences within their N-terminal domain. Additionally, like the TRPM subfamily, these channels possess a proline-rich TRP structural domain located in the C-terminal region, close to the channel’s sixth transmembrane segment. On the other hand, in the N-terminal portion are three or four ankyrin repeats depending on the channel and a coiled-coil domain (Philipp et al., 2000; Lepage et al., 2006).
Like other ion channels, TRPC channels can form heterotetramers. For example, the TRPC1 channel can co-assemble with TRPC3-TRPC7 (Wu et al., 2004). TRPC channels can be activated through different mechanisms, such as Gq/11 receptors or receptor tyrosine kinase (RTK) downstream of the phospholipase C pathway (Trebak et al., 2007). Once phospholipase C (PLC) is activated, TRPC3/6/7 channels are activated by diacylglycerol (DAG) independently of protein kinase C (PKC), indicating that DAG mediates their physiological activation (Hofmann et al., 1999; Ma et al., 2000; Venkatachalam et al., 2002). On the other hand, TRPC1/4 and 5 channels are activated by receptor-induced PLC activation and are not responsive to DAG (Hofmann et al., 1999; Venkatachalam et al., 2003).
The function of TRPC channels is determined by their interactions with several proteins that affect their regulation, trafficking, and scaffolding, in addition to their effects on other downstream cellular processes (Ong and Ambudkar, 2011). It has been proposed that these interactions determine their localization and function in specialized plasma membrane microdomains (Ong and Ambudkar, 2011). TRPC channels have various conserved protein-protein interaction motifs in the N- and C-terminal regions, together with those that interact with WW-repeat domains, Cav1-scaffolding domains, PDZ-domains, and lipids such as PIP2. Moreover, they have ankyrin repeats and numerous coiled-coiled regions that appear particularly important in the assembly of the channels and their plasma membrane localization. Numerous proteins such as Homer, STIM1, Caveolin, NHERF, and PI3K, interact with specific TRPC channels and regulate their surface expression and channel activity (Table 1). Any alterations in these domains and interactions can result in dysregulation of the surface expression of the channels, contributing to the development of pathologies, reviewed in (Englisch et al., 2022).
TRPC1
TRPC1 is expressed in a large range of cell types and tissues. TRPC1 was originally found in the fetal brain, liver, and kidney tissues, as well as the adult heart, testes, ovaries, and brain (Wes et al., 1995). After these first descriptions, different groups have found the channel to be broadly expressed in mammalian tissues (Vazquez et al., 2004a; Beech, 2005; Ramsey et al., 2006; Ambudkar, 2013; Dietrich et al., 2014). Up to this point, TRPC1 performs a role in intracellular Na+ and Ca2+ homeostasis and although its function is not entirely clear (Hoth and Penner, 1992; Feske et al., 2006; Vig et al., 2006; Dietrich et al., 2014).
The trafficking of TRPC1 channels is controlled by several mechanisms involving interactions with lipid modifications, scaffolding proteins, and post-translational modifications (Ferrandiz-Huertas et al., 2014a). For instance, the interaction of TRPC1 with the scaffolding protein Homer has been shown to support its transfer to the plasma membrane, whereas palmitoylation of TRPC1 channels is involved in regulating their permanence and localization in the plasma membrane (Kiselyov et al., 1998; Torihashi et al., 2002; Yuan et al., 2003; Ambudkar et al., 2004). Moreover, TRPC1 localization is regulated by remodeling of the cytoskeleton through interaction with several proteins, including β-tubulin, IP3 receptor, calmodulin (CaM), PLC, and several other proteins (Clark et al., 2008). TRPC1 interacts with caveolin-1 through the C- and N-terminal domains. This interaction seems relevant in assembling a signaling complex localized in lipid rafts, important for regulating Ca2+ signaling (Sundivakkam et al., 2009).
The expression of an N terminal-truncated TRPC1 or a dominant-negative caveolin mutant prevents the TRPC1 trafficking to the plasma membrane affecting Ca2+ influx associated with direct activation of TRPC1 by agonists or passive store depletion. Additionally, in endothelial cells, trafficking of TRPC1 to the membrane occurs following thrombin stimulation (Mehta et al., 2003). Following activation, TRPC1 assembles in a complex with the IP3R and Ras homolog family member A (RhoA), a protein essential for the remodeling of the cytoskeleton (Liu et al., 2000; Lockwich et al., 2000; Brazer et al., 2003; Isshiki and Anderson, 2003; Mehta et al., 2003; Ambudkar et al., 2004; Brownlow et al., 2004; Bollimuntha et al., 2005). Impairments in TRPC1 channel trafficking have been related to various diseases, including cardiac hypertrophy. In ventricular myocytes, TRPC1 colocalized with sarcoplasmic/endoplasmic reticulum Ca2+-ATPase 2 (SERCA2), an established marker of sarcoplasmic reticulum (SR), and sarcomeric α-actinin, which is a marker of the Z-line of the cardiomyocytes (Hu et al., 2020).
In this context, mutations in the TRPC1 gene have been identified in patients with familial essential hypertension (Jiang et al., 2014). Moreover, the abdominal aortic-banded model (AAB) in animals generates an upregulation of TRPC1 expression and an increased store-operated calcium entry (SOCE) in hypertrophied cardiomyocytes. In contrast, the knockdown of TRPC1 decreased SOCE. In this study, cardiomyocytes were treated with d-amino acid peptide HYD1 (MTI-101), which induces trafficking of TRPC1 to the membrane. Co-immunoprecipitation studies indicate that MTI-101 treatment induces the formation of a TRPC1- stromal interaction molecule 1 (STIM1) complex (Gebhard et al., 2013). This influx of Ca2+ activates the SOCE pathway and allows TRPC1 trafficking and insertion into the plasma membrane. Similar observations were made in NCI-H929 and U266 myeloma cell lines where aberrant trafficking of TRPC1 channels has been linked to the growth and metastasis of tumor cells (Gebhard et al., 2013; Myeong et al., 2018; Elzamzamy et al., 2020; Elzamzamy et al., 2021). Overall, the proper trafficking of TRPC1 channels to the membrane is crucial for its function in SOCE, and defects in trafficking can have profound effects on cellular physiology and human health.
TRPC3
TRPC3 is a non-selective cation permeable channel that performs a critical role in several physiological activities, including cardiac hypertrophy, vascular smooth muscle contraction, and insulin secretion among others (Vazquez et al., 2004a). Various factors, including post-translational modifications and the activity of molecular chaperones through protein-protein interactions control this process (Li et al., 1999; Gonzalez-Cobos and Trebak, 2010). The proper localization of TRPC3 channels to the plasma membrane is vital for their function (Xia et al., 2015; Oda et al., 2017; Fan et al., 2018).
TRPC3 can be found assembled in a multimeric protein complex with key Ca2+ signaling proteins such as PLCβ, G alpha-q (Gαq) subunit of heterotrimeric G protein (Gαq/11), Inositol trisphosphate receptor (IP3Rs), CaM and PLCγ (Kiselyov et al., 1998; Lockwich et al., 2001; Zhang et al., 2001; Patterson et al., 2002; Wedel et al., 2003). However, the precise mechanisms governing TRPC3 trafficking to the plasma membrane remain unresolved.
Singh and colleagues proposed that agonist-induced activation of TRPCs also triggers exocytotic trafficking of the channel. They observed that carbachol (CCh) induces a vesicle-associated membrane protein 2 (VAMP-2)-dependent increase in the level of TRPC3 in the plasma membrane, independently of intracellular calcium concentration. TRPC3-containing vesicles were found to be positioned immediately beneath the plasma membrane, likely pre-docked to specific sites through the action of docking and scaffolding proteins. Moreover, they suggested that the surface expression of TRPC3 is regulated by both a recycling-type trafficking mechanism and a regulated event stimulated by CCh (Singh et al., 2004). Furthermore, in 2008, Bandyopadhyay and colleagues reported that the receptor for activated C-kinase-1 (RACK1) serves as a scaffold for localizing TRPC3 in the plasma membrane of cells. This interaction plays a crucial role in recruiting the channel into an IP3R-associated signaling complex and facilitating its insertion into the cell surface membrane upon stimulation by agonists (Bandyopadhyay et al., 2008).
In this context, CCh-induced exocytic trafficking of TRPC3 has been probed by surface biotinylation labeling showing that channel externalization occurs in the presence of the calcium chelator 1,2-bis (2- aminophenoxy) ethane-N,N,N,N-tetraacetic acid (BAPTA), reducing the possibility of a secondary effect by the Ca2+ mobilizing activity of the agonists (Cayouette et al., 2004; Smyth et al., 2006). On the other hand, several studies propose that TRPC3 trafficking is not mandatory for channel activation. They hypothesize that channel activation by second messengers, including DAG or conformational coupling of TRPC channels with the IP3R, could lead to secretion-like trafficking of channels to the plasma membrane (Vazquez et al., 2004b; Putney et al., 2004). Moreover, Smyth and colleagues demonstrated that Gd3+ binds to TRPC3 channels at an extracellular site, causing impaired internalization. Gd3+ effectively “locks” TRPC3 in the plasma membrane, favoring constitutive exocytosis over endocytosis and resulting in a swift accumulation of TRPC3 in the membrane. Furthermore, they observed an elevation in peripheral TRPC3 localization induced by epidermal growth factor (EGF), as assessed by total internal reflection fluorescence (TIRF) microscopy. This suggests that EGF may similarly stabilize TRPC3 in the plasma membrane by impeding its endocytosis, although the precise mechanism remains unclear (Smyth et al., 2006). Nonetheless, it is increasingly evident that the constitutive cycling of plasma membrane proteins is a fundamental mechanism for regulating surface membrane proteins (Royle and Murrell-Lagnado, 2003). TRPC3 also interacts with caveolin-1, a marker for caveolae, and disruption of the actin cytoskeleton deregulates the localization of caveolin-1 and TRPC3 at the plasma membrane (Ma et al., 2000; Lockwich et al., 2001).
Additionally, it has been reported that the N-terminal of TRPC3, specifically in amino acids 123-221, interacts directly with VAMP-2. VAMP-2 is located in the membrane of intracellular trafficking vesicles and mediates the fusion of vesicles to the plasma membrane, promoting the insertion of TRPC3 in the plasma membrane (van Rossum et al., 2000; Singh et al., 2004). Up to this point, the involvement of SNARE proteins in regulating TRPC3 localization at the plasma membrane is still not completely clear.
Importantly, atypical trafficking of TRPC3 channels can lead to diseases, including cardiac hypertrophy, hypertension, and Alzheimer’s disease (Tano et al., 2010; Smedlund et al., 2012; Tiapko and Groschner, 2018). It has been described that in cardiac hypertrophy, excessive trafficking of TRPC3 channels can lead to enhanced Ca2+ influx and stimulation of downstream signaling pathways like nuclear factor of activated T-cells (NFAT) and mammalian target of rapamycin (mTOR), leading to the development of hypertrophy (Brenner and Dolmetsch, 2007). These channels have been associated with regulating blood pressure, and changes in TRPC3 trafficking have been linked to pulmonary hypertension (Tano et al., 2010). In the brain, it is involved in the pathogenesis of Alzheimer’s disease, where Aβ peptides, which are linked to the development of Alzheimer’s disease, correlate with TRPC3-dependent calcium influx, leading to neurotoxicity (Montecinos-Oliva et al., 2014; Wang L. et al., 2017).
TRPC4/5
TRPC4/5 channels are ubiquitously expressed in various tissues. They play a role in calcium signaling and ion homeostasis in various relevant physiological processes, including taste and vision, microvascular permeability, vasorelaxation, gastrointestinal motility, and neurotransmitter release (Fujita et al., 2017; Kim et al., 2019). Like many other membrane proteins, TRPC4 is synthesized in the ER, undergoing post-translational modifications, including glycosylation and phosphorylation. Then it is shipped to the Golgi apparatus, where it is further modified and sorted for delivery to the plasma membrane (Duan et al., 2018). Moreover, TRPC channels are particularly sensitive to mutations and deletions. These modifications may lead to reduced trafficking to the plasma membrane and their retention in the ER or Golgi (Xu et al., 2001; van de Graaf et al., 2003; Wedel et al., 2003; Andrade et al., 2005).
Additionally, two different studies showed that TRPC4 and TRPC5 interact with PDZ-domain-containing proteins such as the Na+/H+ exchanger regulatory factor (NHERF) and the gap junction protein zona occludens 1 (ZO-1) via their C-terminal PDZ-interacting domains (Weinman et al., 1995; Tang et al., 2000). The interface with NHERF facilitates the association of TRPC4 and TRPC5 with PLCβ, which, in turn, positively regulates their surface expression (Mery et al., 2002). Moreover, cellular stimulation by EGF activates protein tyrosine kinases, leading to the specific phosphorylation of two tyrosine residues located at the C-terminal end of TRPC4 (Mery et al., 2002). Such post-translational modification boosts the interaction of TRPC4 and NHERF and the plasma membrane expression of the channel (Mery et al., 2002).
TRPC4 is regulated by the growth factor receptor signaling proteins, such as ezrin, in addition to NHERF binding proteins, which regulate the vesicular trafficking of TRPC4 (Mery et al., 2002). In this context, it has been observed that TRPC5 is trafficked to certain sites in hippocampal neurons and that TRPC5 homomers are found in growth cones (Greka et al., 2003). Trafficking of TRPC5 to the growth cone is facilitated via attaching to the exocytic protein stathmin 2 (a protein associated with ALS), where Ca2+ influx through the channel prevents the extension of growth cones. On the other hand, SNARE proteins interact with the TRPC5 trafficking complex (Greka et al., 2003). TRPC5 channels are localized in vesicles, and fast trafficking of these vesicles to the plasma membrane is triggered in response to stimulation with EGF and nerve growth factor (NGF) and a faint response to brain-derived neurotrophic factor (BDNF) and insulin-like growth factor-1 (IGF-1). Integration of the channel into the plasma membrane also involves Phosphoinositide 3-kinase (PI3K) and the GTPase Rac Family Small GTPase 1 (Rac1), as well as PI4P-5 kinase (Bezzerides et al., 2004). Additionally, TRPC5-including vesicles seem to be retained in a subplasma membrane region from where they are rapidly recruited to the membrane (Ambudkar, 2013).
Mery et al. (2002) showed that the proximal N-terminal region of TRPC4 (amino acids between 23 and 29 in the mice ortholog) is vital for membrane insertion of TRPC4 channels. Altering this domain might cause a decrease in trafficking to the plasma membrane by preventing either the appropriate folding or heterotetrameric assembly with wild-type TRPC4 (Mery et al., 2002). Myeong et al. (2014) demonstrated that the N- and C-terminal regions interact with each other to make a tetrameric structure and that PI(4,5)P2 is a strong candidate involved in the interaction with the membrane-targeting domain at the N terminus of TRPC4/5 heteromers. They reported that the N terminal (amino acids 98–124) and C-terminal (amino acids 700–728) were necessary for the tetrameric assembly of TRPC4 (Myeong et al., 2014; Hong et al., 2015). The interaction between the scaffold ERM-binding phosphoprotein 50 (EBP50) (the NHERF human ortholog) and the membrane-cytoskeleton adaptors can attach TRPC4 to the cytoskeleton and prevent its internalization. NHE3 kinase A regulatory protein (E3KARP), a member of the ezrin/radixin/moesin (ERM) family, plays an active role in the cell surface delivery of TRPC4 by enabling the translocation of TRPC4-bearing vesicles from the cortical actin layer to the plasma membrane. Removal of the last three C-terminal amino acids in TRPC4 blocks the interaction with EBP50 and alters the channel’s localization and surface expression (Mery et al., 2002). In the same study, the authors suggested that TRPC4 can reach the submembranous compartment but is not delivered to the cell surface (Mery et al., 2002).
Dysregulation of TRPC4/5 trafficking and/or function can lead to various diseases (Myeong et al., 2014) in the brain (Mori et al., 1998; Fowler et al., 2007), peripheral sensory neurons (Wu et al., 2008), and gastrointestinal organs. Moreover, a few articles have implicated TRPC4 in ocular diseases, including retinitis pigmentosa and glaucoma (Yang et al., 2005; Reinach et al., 2015; Yang et al., 2022). In retinitis pigmentosa, mutations in TRPC4 result in abnormal protein trafficking to the plasma membrane, leading to photoreceptor cell death and vision loss. In glaucoma, the dysregulation of TRPC4 trafficking is thought to contribute to the death of retinal ganglion cells, which leads to optic nerve damage and vision loss (Yang et al., 2005; Reinach et al., 2015; Yang et al., 2022).
TRPC4 is also associated with several cardiovascular diseases, including cardiac hypertrophy, heart failure, and hypertension. TRPC4 plays a crucial role in regulating the heart’s electrical activity, and abnormal trafficking of TRPC4 can lead to dysregulation of these processes. Mutations in the TRPC4 gene have been associated with atrial fibrillation, Brugada syndrome, and long QT syndrome, whereas TRPC5 has been linked to the development of cardiac hypertrophy and heart failure, as well as pulmonary hypertension (Watanabe et al., 2008; Camacho et al., 2015; Lüscher, 2015; Du et al., 2021). TRPC5 regulates Ca2+ signaling and vascular smooth muscle tone, crucial cardiovascular physiology processes. Impaired TRPC4 trafficking can lead to calcium overload in cardiomyocytes, which dysregulates their function and can lead to heart dysfunction. TRPC4 is also involved in regulating vascular smooth muscle tone, and alterations in its activity can lead to increased blood pressure and hypertension (Watanabe et al., 2008; Camacho et al., 2015; Lüscher, 2015; Du et al., 2021).
TRPC4 has been implicated in several neurological disorders, including multiple sclerosis and Parkinson’s disease, due to its involvement in the regulation of calcium signaling and neuronal excitability (Balbuena et al., 2012; Wang L.-K. et al., 2017; Enders et al., 2020). On the other hand, TRPC5 has been linked to depression, anxiety, and drug addiction (Yang et al., 2005; Nazıroglu and Demirdas, 2015; Yang et al., 2015; Sharma and Hopkins, 2019). Studies have shown that TRPC5 regulates the release of neurotransmitters and modulates synaptic plasticity, which are important processes in the pathogenesis of these disorders (Puram et al., 2011; He et al., 2012). Recent studies have shown that TRPC4 regulates the volume of airway epithelial cells, and its dysfunction may contribute to the pathogenesis of cystic fibrosis (Müller et al., 2022). TRPC5 is predominantly expressed in the renal system and has been linked to the pathogenesis of several renal diseases, including focal segmental glomerulosclerosis (FSGS), diabetic nephropathy, and polycystic kidney disease (Zhou et al., 2017; Yu et al., 2019; Walsh et al., 2021).
TRPC6
Several factors can affect the TRPC6 expression on the plasma membrane of channels in non-excitable cells. For instance, the stimulation of endogenous muscarinic receptors with CCh and the depletion of intracellular calcium stores generate the translocation of TRPC6 toward the plasma membrane (Cayouette et al., 2004). There is still no clear evidence of the participation of SNARE proteins in the trafficking of TRPC6. It has been reported that the Human myxovirus resistance protein 1 (MxA), closely related to the membrane-remodeling GTPase dynamin, interacts with TRPC6 (Lussier et al., 2005). Among the proteins involved in TRPC6 trafficking induced by DAG stimulation, the mammalian uncoordinated-13 (Munc13) family of proteins participates in the fusion of TRPC6-containing vesicles with the plasma membrane, providing insights into the DAG-regulated vesicle fusion mechanism (Xie et al., 2020).
Regarding the glycosylation of TRPC6, it has been described that it is glycosylated in residues N474 and N561. The removal of glycosylation in residue N561 is sufficient to convert it into a constitutively active channel, while trafficking and surface expression remain unaffected in glycosylation-deficient mutants (Dietrich et al., 2003; Talbot et al., 2019).
As mentioned above, TRPC6 can form heterotetramers with other members of the TRPC channel family. Three independent studies observed that once activated, TRPC6 is translocated to the plasma membrane along with TRPC3 (Cayouette et al., 2004; Singh et al., 2004; Kim et al., 2006). In another study, HEK293 cells were stimulated with CCh, resulting in the translocation of TRPC6 in the caveolae, which are closely linked to vesicular traffic (Cayouette et al., 2004; Cayouette and Boulay, 2007). Interestingly, this translocation mechanism is independent of intracellular calcium increases (Stojilkovic et al., 2005).
Moreover, another mechanism involved in TRPC6 trafficking was reported by Kanda et al. (2011), who demonstrated that phosphorylation of a single residue (Y284 in the mice ortholog) is required for channel surface expression. Moreover, a tyrosine to phenylalanine mutation (Y284F) showed decreased expression on the cell surface of both HEK293T cells podocyte cultures, suggesting that the residue is necessary for PLC-γ1 binding (Kanda et al., 2011). In addition, the authors observed that in podocytes, the nephrin protein binds directly to TRPC6 after phosphorylation of Y284, suggesting that a naturally occurring mutation in this position might be associated with focal segmental glomerulosclerosis disease (FSGS) (Kanda et al., 2011). Coincidently, Hagmann and colleagues (2018) have proposed that phosphorylation at S14 in mice TRPC6 enhances channel conductance by increasing the membrane expression of TRPC6 (Hagmann et al., 2018).
TRPC7
The TRPC7 channel is the most recently identified member of the TRPC family, and little is known about the mechanisms governing its trafficking to the plasma membrane. Additionally, there is not much information about naturally occurring mutations that can affect the channel’s expression and/or trafficking. Lussier et al. (2005) demonstrated through different approaches, including GST-pull-down and co-immunoprecipitation assays, that MxA interacts with the second ankyrin-like repeat domain of TRPC7 and other TRP channels. It has been proposed that MxA enhances channel activity by regulating trafficking to the plasma membrane (Lussier et al., 2005). However, this functional role seen in other TRP channels has not been tested on TRPC7 yet.
Figure 1 shows a summary of the different proteins/molecules involved in the regulation of the trafficking of TRPC channels to the plasma membrane as were described in this section of the review.
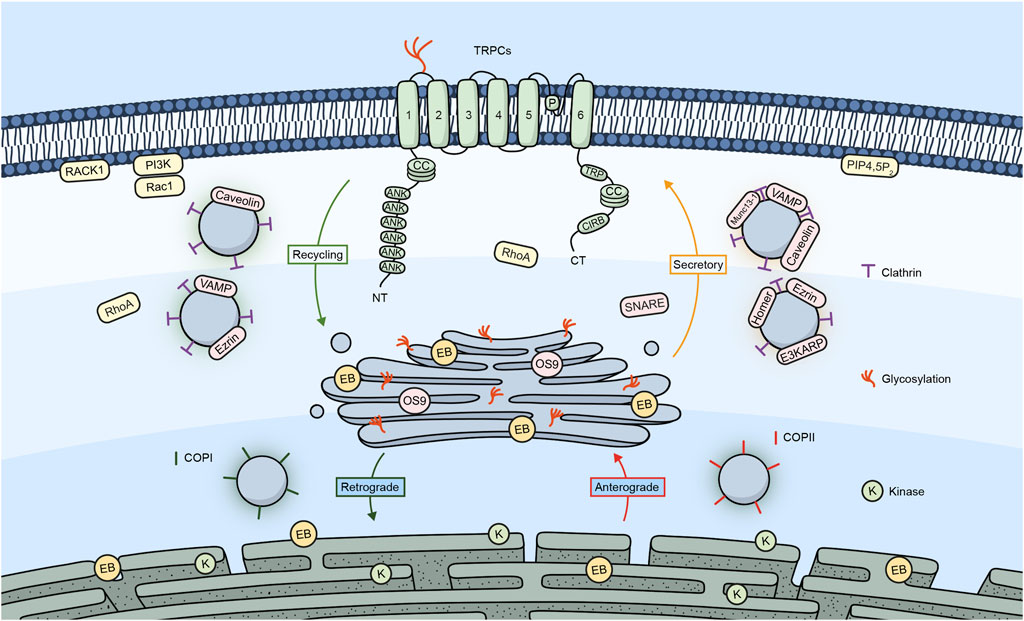
Figure 1. TRPC channels and their trafficking regulators. Embedded in the plasma membrane is a monomeric subunit representing the various domains and regions in TRPC channels. These include ANK (ankyrin repeats), CC (coiled-coil), TRP, which refers to the TRP box common to all TRP channels and a calmodulin, and IP3R binding site (CIRB). The diagram shows a summary of the different regulators involved in the trafficking of TRPC channels.
The TRPM subfamily of ion channels, insights into their trafficking and physiological significance
The human TRPM subfamily is the largest among those belonging to the superfamily of TRP channels and was named after the founding member Melastatin (TRPM1) (Fleig and Penner, 2004). Since the first description and cloning of TRPM1 in 1998 (Duncan et al., 1998), seven more members have been added to this subfamily, which can be grouped into four pairs based on their structural similarity (TRPM1/TRPM3; TRPM2/TRPM8; TRPM4/TRPM5; and TRPM6/TRPM7) (Cohen and Moiseenkova-Bell, 2014). As the rest of the TRP channels, TRPMs are intrinsic membrane proteins with six transmembrane domains that form functional tetramers. TRPMs are widely expressed in the human body. Most TRPMs are Ca2+-permeable cation channels, except for TRPM4 and TRPM5, which are Ca2+-activated, non-selective, monovalent permeable cation channels (Chen et al., 2019; Yamaguchi et al., 2019). The members of the TRPM subfamily of ion channels are characterized by their polymodal nature of activation as they are regulated by different stimuli such as voltage, temperature, and the binding of ions, lipids, or other ligands (Ramon et al., 2007; Diaz-Franulic et al., 2021).
The TRPM subfamily has garnered greater attention due to its reported involvement in several physiological processes, such as taste transduction (Liu and Liman, 2003), temperature sensing (McKemy et al., 2002; Brauchi et al., 2004), synaptogenesis and neurite outgrowth (Abumaria et al., 2019), cell death (McNulty and Fonfria, 2005), and regulation of vasculature (McNulty and Fonfria, 2005). Moreover, the abnormal expression and/or function of TRPM channels has also been involved in a plethora of pathological processes such as cancer (Hantute-Ghesquier et al., 2018; Saldías et al., 2021), neurological disorders (Sita et al., 2018; Lavanderos et al., 2020), kidney disorders (Hsu et al., 2007), and others (Jimenez et al., 2020). All this poses TRPM channels and their regulation as important therapeutic targets.
In the following section, we will discuss the main mechanisms involved in the trafficking of TRPM channels and how the dysregulation of this process might trigger different pathologies. Like TRPC channels, the trafficking of TRPM channels is regulated by specific protein interactions summarized in Table 2.
TRPM1
TRPM1 received the name Melastatin due to its initial discovery in the B16 mouse melanoma cell line (Duncan et al., 1998). TRPM1 expression is not as ubiquitous as with other TRPM channels. Its expression has been reported in the heart, brain, skin, and retina (Fonfria et al., 2006; Oancea and Wicks, 2011). As mentioned before, TRPM1 is involved in Melanoma progression, where expression of TRPM1 inversely correlates with tumor aggressiveness and tumor thickness (Deeds et al., 2000; Guo et al., 2012). It has also been linked to disease-free survival in patients with melanoma (Brozyna et al., 2017), suggesting that TRPM1 may function as a metastatic suppressor. Additionally, it has been reported that TRPM1 is the ion channel that initiates the ON visual pathway in vertebrate vision in the eye (Koike et al., 2010; Nakamura et al., 2010; Iosifidis et al., 2022). TRPM1 trafficking mechanisms have not been fully described yet, but a few reports have suggested possible mechanisms. Nakamura and colleagues showed in ON bipolar cells that mutations R624C and F1075S lead to lower expression in the dendritic tips and mislocalization of TRPM1, which translates to stationary night blindness in patients. These results suggest that these residues are important for the protein trafficking of TRPM1 (Nakamura et al., 2010). Interestingly, the metabotropic glutamate receptor 6 (mGluR6), specifically its C-terminal region, appears to have an important role in the correct localization of TRPM1 to the dendritic tips of depolarizing bipolar cells, as the mGluR6 knockout and other mutants show reduced to no expression of TRPM1 in this region (Xu et al., 2012; Agosto et al., 2021). Moreover, it has been demonstrated that nyctalopin also forms complexes with TRPM1 and is necessary for the correct channel localization (Cao et al., 2011). Additionally, another molecule that has been involved in the trafficking of TRPM1 and the formation of its complex with nyctalopin and mGluR6 is Leucine Rich Repeat, Ig-Like And Transmembrane Domains 3 (LRIT3), which might serve as a scaffold for the formation of this complex since the knockout mouse model for this protein showed no localization of TRPM1 at the dendritic spines of ON bipolar cells (Neuillé et al., 2015; Hasan et al., 2019). These reports suggest that mutations in the interacting residues of TRPM1 with proteins might result in channel trafficking defects.
TRPM2
TRPM2 is a ligand-gated Ca2+-permeable non-selective cationic channel. Despite having a structure that is very similar to the rest of the TRPM subfamily, TRPM2 possesses a C-terminal Nudix hydrolase 9 homologue (NUDT9-H) domain that serves as a binding site for cytosolic adenosine diphosphate ribose (ADPR) (Huang et al., 2018). The gating mechanism of TRPM2 channels seems to require simultaneous co-activation by Calcium (Csanády and Törocsik, 2009), PIP2 (Tóth and Csanády, 2012), and ADPR (Perraud et al., 2001; Hara et al., 2002). Although TRPM2 contains this NUDT9-H domain, it has little to no ADPR-degrading ability and would only act as a regulatory ligand binding site (Iordanov et al., 2016). In terms of expression, TRPM2 is nearly ubiquitous in all human tissues and, thus, has been implicated in regulating several physiological and pathological processes (Belrose and Jackson, 2018). TRPM2 plays an important role in migration and chemokine release (Yamamoto et al., 2008). TRPM2 modulates cell migration and invasion in neuroblastoma, and its expression has been associated with poor patient prognosis (Bao et al., 2022), whereas it regulates autophagy during replication of the Hepatitis B virus (Chen et al., 2022). Moreover, it has also been involved in regulating cell death in several cell models (Malko et al., 2019; Zielinska et al., 2021) and as a potential therapeutic target for treating neurological diseases (Sita et al., 2018; Malko et al., 2019).
About trafficking, the most studied regulatory mechanism of TRPM2 is the effect that reactive oxygen species (ROS) have over its localization. Reports have shown that ROS increase TRPM2 activity (Ali et al., 2021) and treatments with either H2O2 or the non-opioid analgesic acetaminophen (both leading to the production of ROS) increase TRPM2 in the plasma membrane (Kheradpezhouh et al., 2018). Moreover, Mei and Jiang reported that although they saw no effect for the N-terminal coiled-coil domain of TRPM2 in the trafficking of the channel, they could not discard a minor contribution of this domain to the transport of the channel (Mei and Jiang, 2009). Additionally, the expression of a short splicing variant of TRPM2 decreased the channel’s activity, mostly by decreasing the trafficking of the full-length channel to the plasma membrane (Yamamoto et al., 2019).
TRPM3
TRPM3 is a Ca2+ permeable, non-selective cation channel activated by heat and chemical activators which, interestingly, shares certain homology with the heat sensitive TRPV channels (Vriens et al., 2011). As with other TRPM channels, TRPM3 is expressed in a wide variety of tissues in the body (Held et al., 2015), with a relatively higher expression in the brain, spinal cord, sensory neurons, pituitary, kidney and eye (Fonfria et al., 2006; Shaham et al., 2013; Oberwinkler and Philipp, 2014). TRPM3 plays an important role in pancreatic β-cells, where it regulates zinc uptake (Wagner et al., 2010), and in the eye, where its mutations lead to cataracts and glaucoma (Bennett et al., 2014; Zhou et al., 2021). However, its most reported role is the one it plays as a nociceptor in detecting noxious heat and developing inflammatory heat hypersensitivity (Held et al., 2015; Mickle et al., 2015; Held and Tóth, 2021).
The mechanisms involved in TRPM3 trafficking are not well understood. However, we have some information from the few works that have addressed the topic. It has been reported that an 18 amino acid sequence encoded by exon 13 of the trpm3 gene regulates channel insertion in the plasma membrane and is key for sustaining TRPM3 activity (Frühwald et al., 2012). Moreover, it has been recently proposed that mutations in the N-terminal part of the protein, specifically the L769V mutation, affect trafficking to the plasma membrane and result in a non-functional channel (Burglen et al., 2023). Additionally, it has been reported that the activating transcription factor 4 (ATF4), a member of the CREB family of proteins, regulates kinesin family member 17 (KIF17) -mediated TRPM3 trafficking by interacting with KIF17-TRPM3 complex and modulating dorsal root ganglia (DRG) neuron response to heat stimuli (Xie et al., 2021).
TRPM4
TRPM4 differs from most other TRP channels by not being permeable to Ca2+ but only to monovalent cations (showing a high selectivity for Na+). However, one of its main features is that intracellular Ca2+ can directly activate it (Launay et al., 2002; Ullrich et al., 2005). TRPM4 is expressed widely throughout the different body tissues, although several reports have shown a higher expression level in the colon, heart, and prostate (Launay et al., 2002; Fonfria et al., 2006; Syam et al., 2016; Borgstrom et al., 2021). Moreover, TRPM4 expression has been reported in several other cell types, such as immune cells, neuronal tissue, and pancreatic β-cells (Cheng et al., 2007; Barbet et al., 2008; Schattling et al., 2012). This wide expression pattern underlines the important role this channel might play in several physiological and pathological processes. TRPM4 has been reported to play a role in pathologies such as different types of cancer (Rivas et al., 2020; Borgstrom et al., 2021; Wang et al., 2022), and cardiovascular (Stallmeyer et al., 2012; Syam et al., 2016; Wang et al., 2018) and immune system-associated diseases (Barbet et al., 2008; Schattling et al., 2012; Serafini et al., 2012).
Trafficking mechanisms of TRPM4, unlike other TRPM channels, have been more widely studied in recent years. Cho et al. (2014) demonstrated that membrane targeting of the channel is regulated by interaction with the 14-3-3γ protein through the N-terminal region of TRPM4 and that S88 in the human ortholog, which might be a target of phosphorylation, is key for 14-3-3γ binding and anterograde trafficking of TRPM4 (Cho et al., 2014). On the other hand, in patients with human progressive familial heart block type I, the E7K mutation at the N-terminal domain caused attenuated deSUMOylation of the channel, which impaired channel endocytosis and led to an increased activity of TRPM4 due to an elevated concentration of the channel in the plasma membrane (Kruse et al., 2009). Interestingly, several other mutations showed a similar effect in patients with isolated cardiac conduction. Mutations R164W, A432T, and G844D enhance TRPM4 current density due to increased channel abundance in the membrane caused by SUMOylation defects and impaired endocytosis (Liu et al., 2010). Moreover, in patients with congenital or childhood atrioventricular block, mutations A432T and A432T/G582S showed decreased protein expression at the cell membrane, whereas G582S alone showed increased membrane expression of the channel (Syam et al., 2016). Although N-glycosylation also plays an important role in the surface expression and trafficking of ion channels, its role in TRPM4 trafficking is somehow controversial. Several reports showed that the N988 residue in rats and N992/N932 residues in humans are glycosylation sites for TRPM4, but these mutations did not affect the surface expression of the channel but rather modulated its stability (Woo et al., 2013; Syam et al., 2014). Interestingly, phosphorylation also appears to have a role in TRPM4 trafficking. Phosphorylation of S839 is necessary for basolateral localization of TRPM4 in epithelial cells (Cerda et al., 2015). Other mutations have been reported to impact TRPM4 trafficking without an apparent change in the electrophysiological properties of the channel, such as those described in Brugada syndrome, where P779R and K914X showed a decreased membrane expression whereas T873I and L1075P showed an increase in membrane localization of TRPM4 (Liu et al., 2013). Another mechanism reported for TRPM4 anterograde trafficking is its interaction with end-binding (EB) proteins. Blanco et al. (2019) showed that mutations in the EB binding motif in TRPM4 prevented its interaction with EB proteins, which led to a reduced expression of the channel in the plasma membrane and retention in the ER (Blanco et al., 2019). Interestingly, TRPM4 localization in the plasma membrane is also modulated by ROS, given that hydrogen peroxide treatment lowers the channel density in mouse cortical collecting duct cells (Wu M. et al., 2016).
TRPM5
TRPM5, like TRPM4, is a nonselective monovalent cation channel impermeable to divalent cations activated by Ca2+ and has a high permeability for Na+ (Prawitt et al., 2003). However, unlike TRPM4, which is widely expressed in several tissues and organs, TRPM5 expression is more limited to highly specialized cells. For example, high expression of the channel has been reported in β cells from pancreatic islets of Langerhans, where it regulates the frequency of Ca2+ oscillations that lead to glucose-stimulated insulin secretion by β-cells (Brixel et al., 2010; Ketterer et al., 2011). Moreover, its most iconic role is played in taste-sensing receptor cells, where it is co-expressed with other receptors and signaling molecules involved in the process of bitter, sweet, and umami stimuli detection (Perez et al., 2002; Zhang et al., 2003; Dutta et al., 2018). TRPM5 expression has also been reported in other chemosensory cells in the olfactory, respiratory, and digestive systems, suggesting a broader role in chemosensory processes (Kaske et al., 2007; Bezencon et al., 2008; Lin et al., 2008). The available information regarding TRPM5 trafficking is insufficient to relate this process to specific cellular or tissue failure. However, unlike what has been reported for surface proteins (Khanna et al., 2001), the N-glycosylation would not be involved in the trafficking of TRPM5 to the plasma membrane but rather regulates channel function (Syam et al., 2014).
TRPM6
TRPM6 is a Ca2+ permeable ion channel, which is also highly permeable to Mg2+ (Schlingmann et al., 2007). Interestingly, it exhibits an unusual feature, as it contains a transmembrane segment fused to a cytosolic α-type serine/threonine protein kinase domain, which allows it to act as a chanzyme (Chubanov et al., 2018). TRPM6 expression is more restricted than most TRPM channels, with the highest levels of expression reported in the kidney and the gastrointestinal tract, where it is, interestingly, regulated by dietary magnesium (Groenestege et al., 2006; Lameris et al., 2015). The most important role of TRPM6 is related to the homeostasis of systemic Mg2+ homeostasis, where different mutations and dysregulation of its activity have been widely reported in patients with different forms of hypomagnesemia (Schlingmann et al., 2002; Chubanov et al., 2007; Lainez et al., 2014) while also being important for the correct functioning of colonic epithelial cells (Luongo et al., 2018).
It has been reported that patients with hypomagnesemia with secondary hypocalcemia present the S141L mutation, which causes a decrease in the expression of TRPM6 at the plasma membrane (Chubanov et al., 2004). Moreover, Bouras et al. (2020) showed that short-term exposure to type 2 diabetes mellitus drug metformin (1,1-dimethylbiguanide) increased TRPM6 expression in the plasma membrane while, interestingly, long-term exposure significantly decreased the plasma membrane expression of the channel (Bouras et al., 2020). Another interesting mechanism reported for TRPM6 trafficking suggests activating the EGF receptor increased TRPM6 trafficking to the plasma membrane through the src kinase-Rac1 pathway (Thebault et al., 2009). Moreover, Furukawa et al. suggested that Tumor necrosis factor α (TNFα) could regulate TRPM6 trafficking to the plasma membrane (Furukawa et al., 2017). Additionally, although phosphorylation by the close relative TRPM7 and TRPM6/TRPM7 multimers does not appear to alter TRPM6 trafficking (Brandao et al., 2014), it appears TRPM7 is still necessary for the correct trafficking of TRPM6 to the plasma membrane (Schmitz et al., 2005; Shari et al., 2010).
TRPM7
TRPM7, although being discovered independently, is very similar in structure and function to its homolog TRPM6, being also a Mg2+/Ca2+ permeable ion channel (Nadler et al., 2001; Runnels et al., 2001; Schmitz et al., 2003). As with TRPM6, it contains the unique C-terminal serine/threonine protein kinase domain, which shares a low homology with other kinases and is important for the autoregulation of channel activity (Schlingmann et al., 2007; Cabezas-Bratesco et al., 2015; Chubanov et al., 2018). Unlike TRPM6, TRPM7 is widely expressed in many tissues and organs (Nadler et al., 2001; Runnels et al., 2001) but a higher expression has been reported in the heart and kidney (Montell, 2005).
Given its ubiquitous expression, TRPM7 has been involved in several physiological and pathological processes (Visser et al., 2014). For example, in the brain, it regulates synaptic transmission and plasticity, while on the other hand, it also regulates neuronal death following ischemia or neuron injury (Aarts et al., 2003; Krapivinsky et al., 2006; Abumaria et al., 2018). In this context, the fusion of synaptic-like vesicles in PC12 cells is somewhat modulated by vesicular TRPM7 activity (Brauchi et al., 2008). Moreover, it has been reported in several types of cancer, such as breast (Guilbert et al., 2013) and ovarian cancer (Wang et al., 2014), as a metastasis promoter. In contrast, in the heart, it has been involved in forming cardiac fibrosis (Yu et al., 2014).
In the context of TRPM7 trafficking, its most studied regulator is its close homolog, TRPM6. It has been reported that the TRPM6 kinase domain can phosphorylate TRPM7 in serine residues, resulting in altered TRPM7 trafficking. However, TRPM7 phosphorylation of TRPM6 showed no effect on its localization (Schmitz et al., 2005; Brandao et al., 2014).
Interestingly, another regulator of TRPM7 trafficking is TRPM7 itself. It has been shown that S1360 of TRPM7 is a key residue for autophosphorylation, mediating both TRPM7 stability and intracellular trafficking. Other important autophosphorylation residues in the channel were S1403 and S1567, whose autophosphorylation by TRPM7’s kinase activity regulates its interaction with the 14-3-3θ chaperon protein, which regulates channel trafficking (Cai et al., 2018).
TRPM7 channels experience two types of trafficking. The complete gene product traffics to the plasma membrane, where a proteolytic cleavage product containing the kinase domain (M7CKs) is generated and translocated to the nucleus. These fragments establish interactions with proteins composing chromatin remodeling complexes (Krapivinsky et al., 2014). This mechanism is relevant during development (Desai et al., 2012), and the transient overexpression of mouse TRPM7 in HEK293 cells was found to significantly alter the cellular transcription of hundreds of genes (Lee et al., 2011). Finally, it has been shown that sheer stress on blood vessels can increase TRPM7 in the plasma membrane (Oancea et al., 2006).
TRPM8
TRPM8 is a non-selective Ca2+-permeable channel activated by cold, membrane depolarization, and different cooling compounds and is considered the most important thermoreceptor in cold perception (McKemy et al., 2002; Brauchi et al., 2004; Bautista et al., 2007). TRPM8 was initially identified in prostate tissue and reported as upregulated in prostate cancer (Tsavaler et al., 2001). The cold current was first identified in DRG sensory neurons (Reid and Flonta, 2001), and then, the channel was identified, cloned, and described (McKemy et al., 2002).
Although mainly expressed in sensory neurons, channel expression has also been reported in several other tissues and organs, such as the cardiovascular system, lungs, bladder, and urogenital tract (Dhaka et al., 2008; Iftinca and Altier, 2020; Liu et al., 2020). Growing evidence has linked TRPM8 expression and function to several types of cancer, including prostate, lung, and breast cancer (Fuessel et al., 2003; Dhennin-Duthille et al., 2011; Hantute-Ghesquier et al., 2018). Additionally, it has also been involved in the development of neuropathic pain (Proudfoot et al., 2006; De Caro et al., 2019) and irritable bowel syndrome (de Jong et al., 2015).
As one of the most studied TRP channels, there’s a wide variety of articles regarding TRPM8 trafficking and membrane context. It was initially reported that TRPM8 channels are located in lipid rafts (Morenilla-Palao et al., 2009). These overexpressed TRPM8 channels reside in a near membrane compartment, actively recycling in and out of the plasma membrane where its motility is restricted (Veliz et al., 2010; Ghosh et al., 2014). This recycling is associated with the SNARE protein VAMP7 (Ghosh et al., 2016). These recycling modes have been shown to vary in response to channel activation, modulating the number of available channels at the plasma membrane in vitro and in vivo (Toro and Brauchi, 2015). It is worth mentioning that in vitro experiments challenged the later observation (Ghosh et al., 2014). Recently, Cornejo et al. (2020) demonstrated that trafficking of TRPM8 along the cold-sensitive peripheral axons depended on the axonal ARF-GEF Golgi-specific brefeldin A-resistance factor 1 (GBF1) (Cornejo et al., 2020).
Moreover, the same group demonstrated that phosphorylation of the channel is also a regulator of TRPM8 trafficking, demonstrating the versatility of mechanisms involved in this process (Rivera et al., 2021). Interestingly, two other factors named TRP channel–associated factors (TCAFs) have been described as interactors of TRPM8 that regulate its trafficking but with opposite effects on TRPM8 gating properties, with TCAF1 decreasing its activity and tumoral properties of prostate cancer cells and TCAF2 increasing it along with also promoting migration of prostate cancer cells (Gkika et al., 2015).
Additionally, Tsuruda et al. (2006) demonstrated that the C-terminal of the channel contains a coiled-coil domain that is necessary for channel assembly and trafficking (Tsuruda et al., 2006). On the other hand, it has been suggested that the coiled-coil domain was not necessary for trafficking but rather for channel stabilization and added that the mutation N934Q lowered the glycosylation of the channel and its plasma membrane trafficking. However, it did not prevent it completely, suggesting that glycosylation is not mandatory for channel trafficking (Erler et al., 2006). Moreover, the L1089P mutant prevented channel oligomerization and reduced the surface expression of the channel (Erler et al., 2006). Figure 2 shows a summary of the different proteins/molecules involved in the regulation of the trafficking of TRPM channels to the plasma membrane as were described in this section of the review.
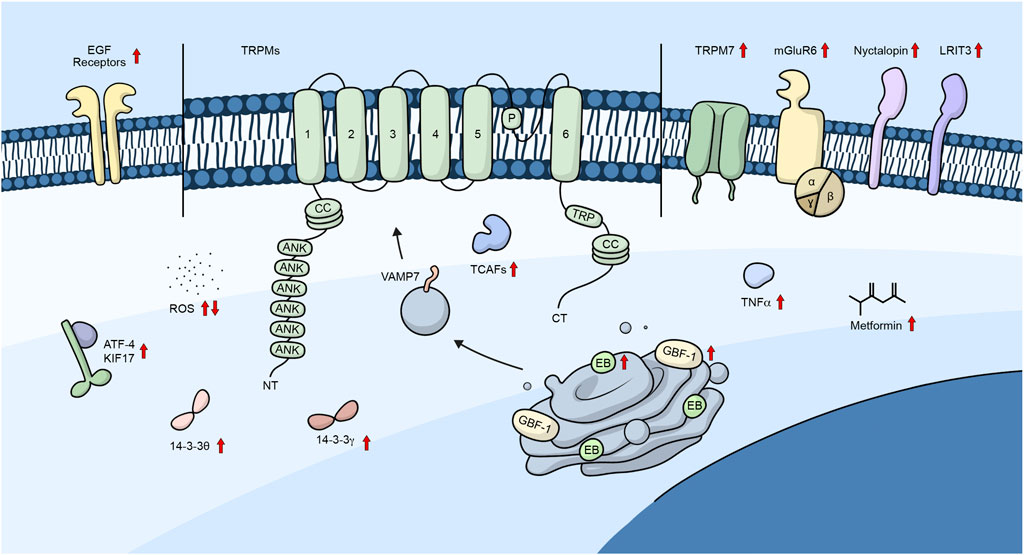
Figure 2. TRPM channels and their trafficking regulators. Diagram showing the different regulators involved in the trafficking of TRPM channels. Embedded in the plasma membrane is a monomeric subunit representing the various domains and regions in TRPM channels. These include ANK (ankyrin repeats), CC (coiled-coil), and TRP, which refers to the TRP box common to all TRP channels. Upward arrows represent regulators that promote channel trafficking to the plasma membrane, whereas downward arrows represent regulators that lower the number of channels in the plasma membrane.
The TRPV subfamily of ion channels, insights into their trafficking and physiological significance
TRPV channels and their trafficking
Six subfamilies of TRP channels have been reported in mammals, categorized according to the homology of their sequences. The subfamily of TRPV1-6 (Vanilloid) plays a significant role in all tissues. TRPV1- 4 are thermosensitive and non-selective cation channels, while TRPV5 and TRPV6 are selective to the calcium cation but not sensitive to temperature (Pumroy et al., 2020).
TRPV family has six ankyrin (Ank) repeat domains in the N-terminal and TRP-box in the C-terminal region. The trafficking of TRPV channels is a complex mechanism involving several proteins that vary in the different types of TRPV channels. Studies based on the membrane yeast two-hybrid approach have demonstrated that different trafficking-related proteins interact with TRPV channels, including SNAP-associated protein and synaptotagmin 9, which interact with TRPV1 and TRPV2 (Doñate-Macián et al., 2019). Furthermore, TRPV2 interacts with other proteins, such as lipid phosphatase SAC1, phosphatidylinositide phosphatase, syndecan 3, and SHISHA family member 6, while TRPV4 interacts with Annexin A2 and cyclin-dependent kinase 16 (CDK16) (Doñate-Macián et al., 2019).
Although the trafficking mechanisms of TRPV channels are not yet fully understood, several proteins that interact with these channels play important roles in their trafficking. For example, CDK16 has been found to interact with the coat protein complex II (COPII) complex, allowing the trafficking of TRPV channels (Palmer et al., 2005). Table 3 summarizes some of the known TRPV trafficking-related interactions.
Pathologies associated with trafficking of TRPV channels
TRPV1
Transient receptor potential vanilloid 1 (TRPV1), the first identified member of the TRPV subfamily, is a tetramer that contains an ATP-binding site and a CaM-binding site in an ankyrin repeat (Sasase et al., 2022). The TRPV1 plays a key role in the perception of peripheral thermal and inflammatory pain.
Yeast two-hybrid screening for identifying proteins that associate with the N terminus of TRPV1 revealed two vesicular proteins, Snapin and synaptotagmin IX (Syt IX). These proteins were found to strongly interact with the TRPV1 N-terminal domain both in vitro and in vivo, where TRPV1 co-localizes with Syt IX and the vesicular protein synaptobrevin in vesicles. PKC activation leads to a swift delivery of functional TRPV1 channels to the plasma membrane, which suggests that PKC signaling promotes the SNARE-dependent exocytosis of TRPV1 to the cell surface (Morenilla-Palao et al., 2004).
The SNARE family of proteins plays a crucial role in TRPV1 channel trafficking, whose main function is to mediate fusion between membranes (Sudhof and Rothman, 2009). It has been shown that TNFα induced surface trafficking of TRPV1 where Munc18-1, SNAP-25, and VAMP1 are important for transporting TRPV1 to the plasma membrane. During this process, TRPV1 channels are packaged into calcitonin gene-related peptide (CGRP)- and VAMP1-containing vesicles and delivered to the plasma membrane involving the formation of SNARE complexes composed of SNAP-25, syntaxin 1, and VAMP1, as well as Munc18–1 (Meng et al., 2016).
Proinflammatory factors have also been studied for potentiating TRPV1 membrane expression, and it was shown that the SNARE complex mediates TRPV1 exocytosis. These findings suggest that the SNARE complex could be a potential therapeutic target for TRPV1-related disorders (Camprubi-Robles et al., 2009).
Studies have reported that TRPV1 interacts with γ-amino butyric acid A-type (GABAA) receptor-associated protein (GABARAP) in HEK293 cells and neurons. The presence of GABARAP selectively enhances the interaction between tubulin and the C-terminal domain of TRPV1. Moreover, nocodazole treatment reduces the capsaicin-evoked current in cells expressing TRPV1 and GABARAP. This indicates that GABARAP plays an important role in the TRPV1 trafficking and clustering on the plasma membrane (Laínez et al., 2010). Another protein recently described to regulate TRPV1 transport is the cyclin-dependent kinase 5 (CDK5). CDK5 positively controls TRPV1 membrane transport mediating kinesin family member KIF13B-TRPV1 association without altering the total amount of TRPV1. Co-immunoprecipitation experiments confirmed the interaction between TRPV1 and potassium channel auxiliary subunit Kvβ2, exerting a chaperone-like effect that increases the cell surface expression of TRPV1. This increase in TRPV1 cell surface expression is associated with an enhancement in capsaicin sensitivity assay in a heterologous recombinant system in HEK293 cells (Ferrandiz-Huertas et al., 2014b).
TRPV channels have been associated with various pathologies, where many of them are related to the functionality and expression of TRPV channels, including neuropathic pain, migraine, dry eye disease (DED). It is worth mentioning that while TRPV1 functionality may be compromised in these conditions, while its trafficking remains unaffected (Sasase et al., 2022).
TRPV2
Studies on TRPV channels have revealed the significance of their trafficking to the plasma membrane in various pathologies. Deletion of the distal N-terminus of TRPV2 depletes the channel’s trafficking to the plasma membrane (Donate-Macian et al., 2015).
The recombinase gene activator protein (RGA) has been associated with TRPV2 trafficking. It acts as a chaperone-like protein that controls the translocation of TRPV2 to the plasma membrane in mast cells. RGA is localized to a vesicular sub compartment of the ER/Golgi apparatus and functions as a chaperone-like protein, promoting the membrane trafficking of TRPV2 (Barnhill et al., 2004).
An elevation of cAMP leads to the translocation of TRPV2 to the plasma membrane, indicating that any stimulus that increases this secondary messenger could regulate TRPV2 trafficking (Kanzaki et al., 1999). The rapid exocytotic response induced by insulin is accompanied by membrane insertion of TRPV2 in a PI3K-dependent manner (Aoyagi et al., 2010). Studies on TRPV2 knockout mice have shown decreased systolic function and impaired cardiac functional response to forced treadmill exercise, but the trafficking of these channels in these pathologies remains unclear (Sasase et al., 2022).
TRPV3
The TRPV3 ion channel is widely expressed in skin keratinocytes, and its exact trafficking mechanism to/from the plasma membrane is unknown. It has been reported that the vesicular trafficking protein sorting nexin 11 (SNX11) downregulates the TRPV3 plasma membrane protein level. SNX11 interacts with TRPV3 and promotes the trafficking of TRPV3 from the plasma membrane to lysosomes for degradation via protein-protein interactions (Li et al., 2016).
TRPV3 plays an important regulatory role in temperature perception, pain transduction, skin physiology, inflammation, cancer, and other diseases, such as pruritic and atopic dermatitis, psoriasis, rosacea, myocardial hypertrophy where the overexpression of this channel is involved (Su et al., 2023). However, there is currently no evidence suggesting that TRPV3 trafficking is altered in these conditions.
TRPV4
The interaction between the N-terminus of TRPV4 and the ubiquitously expressed ER-associated protein OS-9 prevents the channel from trafficking to the plasma membrane. This interaction impedes the release of TRPV4 from the ER, resulting in decreased protein levels at the plasma membrane. Furthermore, OS-9 appears to bind preferentially to immature variants and monomers of TRPV4 are located in the ER and attenuate their polyubiquitination (Wang et al., 2007). Moreover, it has also been reported that deleting the C-terminal region causes retention in the ER (Becker et al., 2008). Taken together, these findings suggest that the process of TRPV channel trafficking is a complicated process that involves several proteins, which may vary depending on the specific type of TRPV channel involved. In the context of TRPV channel mutations, TRPV4 serves as an example of a channel associated with various channelopathies resulting from mutations in different channel motifs, including both the C and N terminal regions and the pore. Some of these mutations have been shown to affect channel conductivity, while others may interfere with proper channel trafficking due to their location in the N- and C-terminal regions, which are essential for this process (Verma et al., 2010). For instance, a mutation of Asn-651 into Gln has been found to increase the constitutive membrane trafficking of TRPV4 without affecting overall channel expression. This mutation is situated in the consensus N-linked glycosylation motif between S5 and S6, suggesting that glycosylation of this residue could regulate channel trafficking (Xu et al., 2006). Additionally, phosphorylation of two other residues, S824 (Shin et al., 2012) and Y110 (Wegierski et al., 2009), is important for their abundance in the plasma membrane and proper trafficking of TRPV4. The atrophin-interacting protein 4 (AIP-4) facilitates ubiquitination of TRPV4 and renders the channel available for endocytosis (Wegierski et al., 2006). The interaction of TRPV4 with PACSIN 3 isoform regulates endocytosis enhancing the ratio of plasma membrane associated to cytosolic TRPV4 (Cuajungco et al., 2006). In HEK293 and vascular endothelial cells, depletion of intracellular Ca2+ stores leads to the vesicular trafficking of heteromeric TRPV4-C1 channels to the plasma membrane (Ma et al., 2010).
In contrast, it has been observed that splicing variants of TRPV4, which lack the ankyrin domain, do not oligomerize and are retained in the ER (Arniges et al., 2006). Some of these variants have been detected in two unrelated human respiratory tracts (CFT1-LCFSN and HBE epithelial cell lines), highlighting the significance of these channels in the respiratory tract and, therefore, potentially associated pathologies.
TRPV5
Deleting the distal TRPV5 N-terminus is sufficient to deplete the channel trafficking to the plasma membrane (Donate-Macian et al., 2015). Moreover, the complexly glycosylated TRPV5 that appears at the plasma membrane is enhanced by WNK3, a member of the With No Lysine (K) family of protein serine/threonine kinases. The microtubule inhibitor colchicine blocks the effect of WNK3 on TRPV5, indicating that WNK3 positively regulates the transcellular Ca2+ transport pathway by increasing the exocytosis of TRPV5 (Zhang et al., 2008). Conversely, TRPV5 internalization occurs through the clathrin protein (van de Graaf et al., 2008).
Familial hypomagnesemia with hypercalciuria and nephrocalcinosis (FHHNC) is a paracellular channelopathy caused by mutations in the claudin-16 and claudin-19 genes. The missense FHHNC mutation c.908C>G (p.T303R) in the claudin-16 gene disrupts the phosphorylation of the claudin-16 protein. The phosphomimetic claudin-16 protein carrying the T303E mutation, but not the wildtype claudin-16 or the T303R mutant protein, increases the TRPV5 channel conductance and membrane abundance in human kidney cells (Hou et al., 2019).
TRPV6
Annexin 2 also interacts with TRPV6 (Borthwick et al., 2008). Its known functions include intracellular trafficking of vesicles, maintenance and organization of cell membranes. Annexin A2 was also identified on early endosomes participating in the endocytic pathway of these channels (Gerke and Moss, 2002).
Pharmacological modulation of TRPV channels can treat certain pathologies by affecting their functionality (Seebohm and Schreiber, 2021). Nevertheless, mutations in these channels can also affect their trafficking, leading to various diseases, which will be described below.
Mutations in the TRPV6 channel have been studied and found to cause insufficient Ca2+ transport, resulting in transient neonatal hyperparathyroidism (TNHP) characterized by respiratory distress and under-mineralization of the skeleton. Two mutations, I223T and G428R, were identified in TRPV6 that affect its trafficking to the plasma membrane. The I223T mutation is located in the fourth ankyrin repeat domain (ANK4) and decreases the amount of TRPV6 channel in the plasma membrane (Yamashita et al., 2019).
Similarly, the G428R mutation located in the S2 and S3 transmembrane domains also affects trafficking to the plasma membrane and interferes with TRPV6’s normal function. We thus conclude that interference with placental maternal-fetal calcium transport caused by TRPV6 loss-of-function mutations results in fetal calcium deficiency, hyperparathyroidism, and metabolic bone disease (Suzuki et al., 2018).
Figure 3 shows a summary of the different proteins/molecules involved in the regulation of the trafficking of TRPV channels to the plasma membrane.
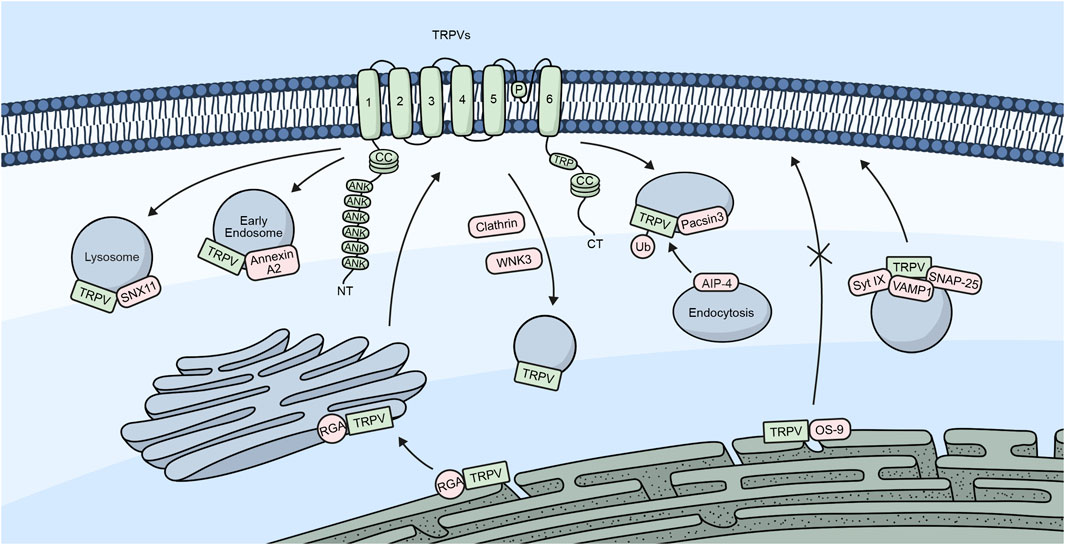
Figure 3. TRPV channels and their trafficking regulators. Embedded in the plasma membrane is a monomeric subunit representing the different domains and regions in TRPV channels. These include ANK (ankyrin repeats), CC (coiled-coil), and TRP, which refers to the TRP box common to all TRP channels. The diagram shows a summary of the different regulators involved in the trafficking of TRPV channels.
TRPA1 in nociception: function, membrane expression, and sensitization mechanisms
TRPA1 was initially identified as a nociceptive channel that is expressed in subpopulations of primary sensory neurons in the DRG, vagal ganglia (VG), and trigeminal ganglia (TG), which is activated by noxious compounds and low temperatures (Story et al., 2003; Bautista et al., 2005). TRPA1 is predominantly expressed in unmyelinated C-fibers and thinly myelinated Aδ-fibers, which transmit pain signals from the periphery to the central nervous system. TRPA1 expression in large, myelinated fibers is less frequent, and its function in these fibers is poorly understood (Story et al., 2003). This selective expression pattern suggests that TRPA1 plays a specific role in nociception, which is the perception of pain and helps to ensure that noxious stimuli are transmitted effectively to the central nervous system.
The expression of TRPA1 can vary between species, with some species having a higher expression of TRPA1 in certain populations of primary sensory neurons. At least 25% of TRPA1-expressing neurons are peptidergic, which means they can release substance P (SP) and CGRP. However, TRPA1 has also been found to colocalize with non-peptidergic neuron markers, such as isolectin B4 (IB4), the purinergic P2X3 receptor, and the NaV1.8 channel, which suggests that TRPA1 plays a complex role in nociception and other physiological processes (Kim et al., 2010; Kim et al., 2011; Ryskamp et al., 2011).
TRPA1 is present in 30%–50% of TRPV1-expressing neurons (Kobayashi et al., 2005). Evidence for a TRPV1-TRPA1 interaction suggests that the two proteins might form a heteromeric channel, which could play a role in nociception and other physiological processes (Fischer et al., 2014). These two channels are thought to play a crucial role in transmitting sensory signals during inflammation, as they were found on vesicles containing CGRP and co-localized on nerve fibers and sensory neuron cell bodies. Furthermore, TNFα was observed to increase the surface presence of TRPV1 and TRPA1, indicating upregulation or enhanced transport to the cell membrane (Meng et al., 2016; Nugent et al., 2018). The proximity of TRPV1 and TRPA1 suggests potential co-trafficking in response to TNFα. Notably, Munc18–1 and VAMP1 were identified as essential for TNFα-induced channel trafficking and CGRP release. Additionally, Botulinum neurotoxins, particularly BoNT/C1 or/A, effectively inhibited TNFα-induced channel delivery, indicating their therapeutic potential in mitigating sensitization during inflammation (Wu C. et al., 2016; Meng et al., 2016). The trafficking of TRPA1 is regulated by interactions with several proteins, as summarized in Table 4.
Inflammation can sensitize peripheral nociceptors and cause symptoms such as allodynia and hyperalgesia. Bradykinin is one of the key inflammatory mediators known to sensitize TRPA1 ion channels. The sensitization of TRPA1 is dependent on PLC and PKA activation, but not PKC activation. It diminishes the nociceptor activation thresholds, which can result in symptoms of allodynia and hyperalgesia (Wang et al., 2008).
The effect of PLC on sensitization may involve hydrolysis of PIP2 (Dai et al., 2007; Karashima et al., 2008; Kim et al., 2008). Both PKA and PLC activation increase the membrane expression of TRPA1 in trigeminal neurons by increasing the trafficking of the channel (Schmidt et al., 2009). PKA-mediated sensitization of TRPA1 has been confirmed in some studies, with three residues in the NH2-terminal domain (S86, S317, and S428) and one residue in the COOH-terminal domain (S972) identified as the main phosphorylation sites for PKA (Meents et al., 2017). Other studies have also investigated the phosphorylation of TRPA1 by cyclin-dependent kinase 5 (Cdk5), with sites S242, T416, S449, T485, and T673 identified as susceptible phosphorylation sites (Hynkova et al., 2016; Hall et al., 2018; Sulak et al., 2018). During TRPA1 phosphorylation by both PKA and PKC, the anchoring protein AKAP (A-Kinase Anchoring Protein 79/150) is essential in anchoring these kinases to TRPA1 (Brackley et al., 2017). TRPA1 contains an N-terminal ankyrin repeat domain (ARD) with a total of 17 ankyrin repeats. This domain is believed to be essential for plasma membrane localization (Nilius et al., 2011; Hall et al., 2018). TRPA1 phosphorylation may contribute to painful pathologies associated with the channel, including chemotherapy-induced peripheral neuropathy (CIPN).
Sigma-1 receptor (Sigma-1R), an endoplasmic reticulum chaperone, is known to modulate the function of various ion channels and receptors (Hanner et al., 1996; Alonso et al., 2000; Delprat et al., 2020). Sigma-1 receptor antagonist (S1RA) has been shown to interact with TRPA1. Antagonizing Sigma-1R disrupted the formation of the molecular complex between Sigma-1R and TRPA1, affecting the trafficking of TRPA1 to the plasma membrane. Moreover, S1RA significantly inhibited the plasma membrane expression and function of human TRPA1 channels, reducing the excitability of nociceptor neurons sensitized by oxaliplatin (Marcotti et al., 2023).
TRPA1 channels were also shown to sense moderate hypoxia in astrocytes associated with the anterior inferior cerebellar artery (AICA) in the parafacial respiratory group/retrotrapezoid nucleus (pFRG/RTN) region of the ventral medullary surface. TRPA1 prevents its internalization through prolyl hydroxylases (PHD) and neural precursor cell-expressed developmentally downregulated protein 4 (NEDD4-1)-mediated mechanisms, resulting in plasma membrane retention. Hypoxia-induced TRPA1 channel activity leads to Ca2+ influx. It accelerates ATP release from pFRG/RTN astrocytes, impacting neuronal circuits in the respiratory center and increasing the amplitude of the inspiratory discharge rhythm. This study suggests a connection between TRPA1 trafficking regulation and the cellular response to hypoxia, highlighting the role of TRPA1 in astrocytic sensing of acute hypoxia (Uchiyama et al., 2020).
Recently, IQ motif containing GTPase activating protein 1 (IQGAP1) was shown to interact with TRPA1, mainly in sensory dorsal root ganglia neurons in mice. The expression of IQGAP1 increases in chronic pain conditions, and TRPA1 undergoes increased trafficking to the neuronal membrane, catalyzed by the small GTPase Cdc42, which is associated with IQGAP1. Activation of PKA can also induce TRPA1 trafficking and sensitization. Notably, the absence of IQGAP1 prevents the responses, implying a mechanism entirely dependent on the presence of IQGAP1. These findings suggest the possibility of IQGAP1 in promoting chronic pain by facilitating the trafficking and signaling mechanisms of TRPA1 channels (Khan et al., 2023). Altogether, TRPA1 channels are crucial in pain perception and potentially affect chronic pain conditions.
Concluding remarks
The TRP family of ion channels plays an essential role in many physiological processes by regulating intracellular levels of various ions, such as sodium, calcium, and magnesium. Indeed, the dysregulation of the activity of these channels can contribute to and/or lead to the development of several pathological disorders, such as cancer, brain diseases, and heart and kidney malfunction. Interestingly, one of the primary means that cells must regulate the activity of TRP channels is the modulation of the number of channels in the plasma membrane. In this review, we have presented an in-depth exploration of the mechanisms that have been described for the trafficking of the different members of the TRP family of ion channels and the pathologies associated with the dysregulation of these processes. In this context, although these channels share a high homology in their amino acid sequence, we have found that their trafficking mechanisms are widely different regarding interacting proteins and signaling pathways involved, even amongst channels of the same sub-family, which provides the intracellular targeting characteristic of these channels.
Nevertheless, we can point to specific general mechanisms shared amongst TRP channels necessary for correct trafficking and localization. For example, post-translational modifications such as glycosylation, phosphorylation, and SUMOylation are involved in the trafficking of several TRP channels. On the other hand, protein-protein interactions seem to be of great importance for the trafficking of TRP channels, where proteins such as PKA, PKC, and PLC are common regulators of the localization of some members of this family. Additionally, the subcellular microenvironment also appears to be an important feature for the trafficking of several TRP channels.
As a final remark, we have also briefly described the wide variety of processes and pathologies in which TRP channels are highly involved. In recent years, several reports have appeared showing the therapeutical potential that targeting these ion channels through modulators and other mechanisms might have (Moran et al., 2011; Zsombok and Derbenev, 2016; Hong et al., 2020; Fallah et al., 2022; Koivisto et al., 2022). In general, most of these studies have been dedicated to the identification of novel pharmacological modulators addressed to the conductive activity of these channels. However, ion channels can also have non-conductive activities, serving as scaffolding proteins and signaling hubs in different processes. Then, their specific trafficking and localization might regulate these functions. Therefore, further studies dedicated to targeting the trafficking and targeting of TRP channels might serve as potential therapeutic strategies for different diseases.
In conclusion, the trafficking of TRP channels is characterized by its intricate nature, the different mechanisms involved, such as vesicular transport, anterograde, and retrograde pathways, and the many diseases associated with this process’s dysregulation. The present overview underscores the importance of expanding our understanding of the mechanisms involved in the trafficking of TRP channels to provide novel therapeutic targets and treatments for the increasing number of pathologies associated with the TRP family of ion channels.
Author contributions
BR: Conceptualization, Writing–review and editing, Data curation, Investigation, Methodology, Writing–original draft. OO: Conceptualization, Data curation, Investigation, Methodology, Writing–original draft, Writing–review and editing. ES: Investigation, Methodology, Writing–original draft. RS: Investigation, Methodology, Writing–original draft. SB: Conceptualization, Data curation, Funding acquisition, Project administration, Supervision, Validation, Writing–review and editing. OC: Conceptualization, Data curation, Funding acquisition, Project administration, Supervision, Validation, Writing–review and editing.
Funding
The author(s) declare that financial support was received for the research, authorship, and/or publication of this article. FONDECYT Grants 1200917 and 1240633 (to OC), and 1241753 (to SB) and the Millennium Nucleus of Ion Channel-Associated Diseases (MiNICAD) fund this research. MiNICAD is a Millennium Nucleus supported by the Iniciativa Científica Milenio of the National Agency of Research and Development (ANID). ANID Postdoctoral Fellowship 3210419 (to BR), 3210387 (to OO) and 3240187 (to ES).
Acknowledgments
We thank Mr. Diego Morales for preparing the figures.
Conflict of interest
The authors declare that the research was conducted in the absence of any commercial or financial relationships that could be construed as a potential conflict of interest.
Publisher’s note
All claims expressed in this article are solely those of the authors and do not necessarily represent those of their affiliated organizations, or those of the publisher, the editors and the reviewers. Any product that may be evaluated in this article, or claim that may be made by its manufacturer, is not guaranteed or endorsed by the publisher.
References
Aarts, M., Iihara, K., Wei, W. L., Xiong, Z. G., Arundine, M., Cerwinski, W., et al. (2003). A key role for TRPM7 channels in anoxic neuronal death. Cell 115, 863–877. doi:10.1016/s0092-8674(03)01017-1
Abumaria, N., Li, W., and Clarkson, A. N. (2019). Role of the chanzyme TRPM7 in the nervous system in health and disease. Cell Mol. Life Sci. 76, 3301–3310. doi:10.1007/s00018-019-03124-2
Abumaria, N., Li, W., and Liu, Y. (2018). TRPM7 functions in non-neuronal and neuronal systems: perspectives on its role in the adult brain. Behav. Brain Res. 340, 81–86. doi:10.1016/j.bbr.2016.08.038
Agosto, M. A., Adeosun, A. A. R., Kumar, N., and Wensel, T. G. (2021). The mGluR6 ligand-binding domain, but not the C-terminal domain, is required for synaptic localization in retinal ON-bipolar cells. J. Biol. Chem. 297, 101418. doi:10.1016/j.jbc.2021.101418
Ali, E. S., Rychkov, G. Y., and Barritt, G. J. (2021). TRPM2 non-selective cation channels in liver injury mediated by reactive oxygen species. Antioxidants (Basel) 10, 1243. doi:10.3390/antiox10081243
Alonso, G., Phan, V., Guillemain, I., Saunier, M., Legrand, A., Anoal, M., et al. (2000). Immunocytochemical localization of the sigma(1) receptor in the adult rat central nervous system. Neuroscience 97, 155–170. doi:10.1016/s0306-4522(00)00014-2
Altschuler, Y., Hodson, C., and Milgram, S. L. (2003). The apical compartment: trafficking pathways, regulators and scaffolding proteins. Curr. Opin. Cell Biol. 15, 423–429. doi:10.1016/s0955-0674(03)00084-x
Ambudkar, I. S. (2007). Trafficking of TRP channels: determinants of channel function. Handb. Exp. Pharmacol. 179, 541–557. doi:10.1007/978-3-540-34891-7_32
Ambudkar, I. S. (2013). TRPC1: getting physical in space. Cell Cycle 12, 3355–3356. doi:10.4161/cc.26637
Ambudkar, I. S., Brazer, S. C., Liu, X., Lockwich, T., and Singh, B. (2004). Plasma membrane localization of TRPC channels: role of caveolar lipid rafts. Novartis Found. Symp. 258, 263–266.
Andrade, Y. N., Fernandes, J., Vazquez, E., Fernandez-Fernandez, J. M., Arniges, M., Sanchez, T. M., et al. (2005). TRPV4 channel is involved in the coupling of fluid viscosity changes to epithelial ciliary activity. J. Cell Biol. 168, 869–874. doi:10.1083/jcb.200409070
Aoyagi, K., Ohara-Imaizumi, M., Nishiwaki, C., Nakamichi, Y., and Nagamatsu, S. (2010). Insulin/phosphoinositide 3-kinase pathway accelerates the glucose-induced first-phase insulin secretion through TrpV2 recruitment in pancreatic β-cells. Biochem. J. 432, 375–386. doi:10.1042/BJ20100864
Arniges, M., Fernandez-Fernandez, J. M., Albrecht, N., Schaefer, M., and Valverde, M. A. (2006). Human TRPV4 channel splice variants revealed a key role of ankyrin domains in multimerization and trafficking. J. Biol. Chem. 281, 1580–1586. doi:10.1074/jbc.M511456200
Balbuena, P., Li, W., Rzigalinski, B. A., and Ehrich, M. (2012). Malathion/oxon and lead acetate increase gene expression and protein levels of transient receptor potential canonical channel subunits TRPC1 and TRPC4 in rat endothelial cells of the blood–brain barrier. Int. J. Toxicol. 31, 238–249. doi:10.1177/1091581812442688
Bandyopadhyay, B. C., Ong, H. L., Lockwich, T. P., Liu, X., Paria, B. C., Singh, B. B., et al. (2008). TRPC3 controls agonist-stimulated intracellular Ca2+ release by mediating the interaction between inositol 1,4,5-trisphosphate receptor and RACK1. J. Biol. Chem. 283, 32821–32830. doi:10.1074/jbc.M805382200
Bandyopadhyay, B. C., Swaim, W. D., Liu, X., Redman, R. S., Patterson, R. L., and Ambudkar, I. S. (2005). Apical localization of a functional TRPC3/TRPC6-Ca2+-signaling complex in polarized epithelial cells. Role in apical Ca2+ influx. J. Biol. Chem. 280, 12908–12916. doi:10.1074/jbc.M410013200
Bao, L., Festa, F., Hirschler-Laszkiewicz, I., Keefer, K., Wang, H. G., Cheung, J. Y., et al. (2022). The human ion channel TRPM2 modulates migration and invasion in neuroblastoma through regulation of integrin expression. Sci. Rep-Uk 12, 20544. doi:10.1038/s41598-022-25138-w
Barbet, G., Demion, M., Moura, I. C., Serafini, N., Leger, T., Vrtovsnik, F., et al. (2008). The calcium-activated nonselective cation channel TRPM4 is essential for the migration but not the maturation of dendritic cells. Nat. Immunol. 9, 1148–1156. doi:10.1038/ni.1648
Barnhill, J. C., Stokes, A. J., Koblan-Huberson, M., Shimoda, L. M., Muraguchi, A., Adra, C. N., et al. (2004). RGA protein associates with a TRPV ion channel during biosynthesis and trafficking. J. Cell Biochem. 91, 808–820. doi:10.1002/jcb.10775
Bautista, D. M., Movahed, P., Hinman, A., Axelsson, H. E., Sterner, O., Hogestatt, E. D., et al. (2005). Pungent products from garlic activate the sensory ion channel TRPA1. Proc. Natl. Acad. Sci. U. S. A. 102, 12248–12252. doi:10.1073/pnas.0505356102
Bautista, D. M., Siemens, J., Glazer, J. M., Tsuruda, P. R., Basbaum, A. I., Stucky, C. L., et al. (2007). The menthol receptor TRPM8 is the principal detector of environmental cold. Nature 448, 204–208. doi:10.1038/nature05910
Becker, D., Müller, M., Leuner, K., and Jendrach, M. (2008). The C-terminal domain of TRPV4 is essential for plasma membrane localization. Mol. Membr. Biol. 25, 139–151. doi:10.1080/09687680701635237
Beech, D. J. (2005). TRPC1: store-operated channel and more. Pflugers Arch. 451, 53–60. doi:10.1007/s00424-005-1441-3
Belrose, J. C., and Jackson, M. F. (2018). TRPM2: a candidate therapeutic target for treating neurological diseases. Acta Pharmacol. Sin. 39, 722–732. doi:10.1038/aps.2018.31
Bennett, T. M., Mackay, D. S., Siegfried, C. J., and Shiels, A. (2014). Mutation of the melastatin-related cation channel, TRPM3, underlies inherited cataract and glaucoma. Plos One 9, e104000. doi:10.1371/journal.pone.0104000
Bezencon, C., Furholz, A., Raymond, F., Mansourian, R., Metairon, S., Le Coutre, J., et al. (2008). Murine intestinal cells expressing Trpm5 are mostly brush cells and express markers of neuronal and inflammatory cells. J. Comp. Neurol. 509, 514–525. doi:10.1002/cne.21768
Bezzerides, V. J., Ramsey, I. S., Kotecha, S., Greka, A., and Clapham, D. E. (2004). Rapid vesicular translocation and insertion of TRP channels. Nat. Cell Biol. 6, 709–720. doi:10.1038/ncb1150
Blanco, C., Morales, D., Mogollones, I., Vergara-Jaque, A., Vargas, C., Alvarez, A., et al. (2019). EB1- and EB2-dependent anterograde trafficking of TRPM4 regulates focal adhesion turnover and cell invasion. FASEB J. 33, 9434–9452. doi:10.1096/fj.201900136R
Bollimuntha, S., Cornatzer, E., and Singh, B. B. (2005). Plasma membrane localization and function of TRPC1 is dependent on its interaction with beta-tubulin in retinal epithelium cells. Vis. Neurosci. 22, 163–170. doi:10.1017/S0952523805222058
Borgstrom, A., Peinelt, C., and Stoklosa, P. (2021). TRPM4 in cancer-A new potential drug target. Biomolecules 11, 229. doi:10.3390/biom11020229
Borthwick, L. A., Neal, A., Hobson, L., Gerke, V., Robson, L., and Muimo, R. (2008). The annexin 2-S100A10 complex and its association with TRPV6 is regulated by cAMP/PKA/CnA in airway and gut epithelia. Cell Calcium 44, 147–157. doi:10.1016/j.ceca.2007.11.001
Bouras, H., Roig, S. R., Kurstjens, S., Tack, C. J. J., Kebieche, M., de Baaij, J. H. F., et al. (2020). Metformin regulates TRPM6, a potential explanation for magnesium imbalance in type 2 diabetes patients. Can. J. Physiol. Pharmacol. 98, 400–411. doi:10.1139/cjpp-2019-0570
Brackley, A. D., Gomez, R., Guerrero, K. A., Akopian, A. N., Glucksman, M. J., Du, J., et al. (2017). A-kinase anchoring protein 79/150 scaffolds transient receptor potential A 1 phosphorylation and sensitization by metabotropic glutamate receptor activation. Sci. Rep. 7, 1842. doi:10.1038/s41598-017-01999-4
Brandao, K., Deason-Towne, F., Zhao, X., Perraud, A. L., and Schmitz, C. (2014). TRPM6 kinase activity regulates TRPM7 trafficking and inhibits cellular growth under hypomagnesic conditions. Cell Mol. Life Sci. 71, 4853–4867. doi:10.1007/s00018-014-1647-7
Brauchi, S., Krapivinsky, G., Krapivinsky, L., and Clapham, D. E. (2008). TRPM7 facilitates cholinergic vesicle fusion with the plasma membrane. Proc. Natl. Acad. Sci. U. S. A. 105, 8304–8308. doi:10.1073/pnas.0800881105
Brauchi, S., Orio, P., and Latorre, R. (2004). Clues to understanding cold sensation: thermodynamics and electrophysiological analysis of the cold receptor TRPM8. Proc. Natl. Acad. Sci. U. S. A. 101, 15494–15499. doi:10.1073/pnas.0406773101
Brazer, S. C., Singh, B. B., Liu, X., Swaim, W., and Ambudkar, I. S. (2003). Caveolin-1 contributes to assembly of store-operated Ca2+ influx channels by regulating plasma membrane localization of TRPC1. J. Biol. Chem. 278, 27208–27215. doi:10.1074/jbc.M301118200
Brenner, J. S., and Dolmetsch, R. E. (2007). TrpC3 regulates hypertrophy-associated gene expression without affecting myocyte beating or cell size. PLoS One 2, e802. doi:10.1371/journal.pone.0000802
Brixel, L. R., Monteilh-Zoller, M. K., Ingenbrandt, C. S., Fleig, A., Penner, R., Enklaar, T., et al. (2010). TRPM5 regulates glucose-stimulated insulin secretion. Pflugers Arch. 460, 69–76. doi:10.1007/s00424-010-0835-z
Brownlow, S. L., Harper, A. G., Harper, M. T., and Sage, S. O. (2004). A role for hTRPC1 and lipid raft domains in store-mediated calcium entry in human platelets. Cell Calcium 35, 107–113. doi:10.1016/j.ceca.2003.08.002
Brozyna, A. A., Guo, H. Z., Yang, S. E., Cornelius, L., Linette, G., Murphy, M., et al. (2017). TRPM1 (melastatin) expression is an independent predictor of overall survival in clinical AJCC stage I and II melanoma patients. J. Cutan. Pathol. 44, 328–337. doi:10.1111/cup.12872
Burglen, L., Van Hoeymissen, E., Qebibo, L., Barth, M., Belnap, N., Boschann, F., et al. (2023). Gain-of-function variants in the ion channel gene TRPM3 underlie a spectrum of neurodevelopmental disorders. Elife 12, e81032. doi:10.7554/eLife.81032
Cabezas-Bratesco, D., Brauchi, S., González-Teuber, V., Steinberg, X., Valencia, I., and Colenso, C. (2015). The different roles of the channel-kinases TRPM6 and TRPM7. Curr. Med. Chem. 22, 2943–2953. doi:10.2174/0929867322666150716115644
Cabezas-Bratesco, D., McGee, F. A., Colenso, C. K., Zavala, K., Granata, D., Carnevale, V., et al. (2022). Sequence and structural conservation reveal fingerprint residues in TRP channels. Elife 11, e73645. doi:10.7554/eLife.73645
Cai, N., Lou, L., Al-Saadi, N., Tetteh, S., and Runnels, L. W. (2018). The kinase activity of the channel-kinase protein TRPM7 regulates stability and localization of the TRPM7 channel in polarized epithelial cells. J. Biol. Chem. 293, 11491–11504. doi:10.1074/jbc.RA118.001925
Cai, R., and Chen, X. Z. (2023). Roles of intramolecular interactions in the regulation of TRP channels. Rev. Physiol. Biochem. Pharmacol. 186, 29–56. doi:10.1007/112_2022_74
Camacho, J. E., Tian, Q., Hammer, K., Schröder, L., Camacho Londoño, J., Reil, J. C., et al. (2015). A background Ca2+ entry pathway mediated by TRPC1/TRPC4 is critical for development of pathological cardiac remodelling. Eur. Heart J. 36, 2257–2266. doi:10.1093/eurheartj/ehv250
Camprubi-Robles, M., Planells-Cases, R., and Ferrer-Montiel, A. (2009). Differential contribution of SNARE-dependent exocytosis to inflammatory potentiation of TRPV1 in nociceptors. FASEB J. 23, 3722–3733. doi:10.1096/fj.09-134346
Cao, E. (2020). Structural mechanisms of transient receptor potential ion channels. J. Gen. Physiol. 152, e201811998. doi:10.1085/jgp.201811998
Cao, Y., Posokhova, E., and Martemyanov, K. A. (2011). TRPM1 forms complexes with nyctalopin in vivo and accumulates in postsynaptic compartment of ON-bipolar neurons in mGluR6-dependent manner. J. Neurosci. 31, 11521–11526. doi:10.1523/JNEUROSCI.1682-11.2011
Cayouette, S., and Boulay, G. (2007). Intracellular trafficking of TRP channels. Cell Calcium 42, 225–232. doi:10.1016/j.ceca.2007.01.014
Cayouette, S., Lussier, M. P., Mathieu, E. L., Bousquet, S. M., and Boulay, G. (2004). Exocytotic insertion of TRPC6 channel into the plasma membrane upon Gq protein-coupled receptor activation. J. Biol. Chem. 279, 7241–7246. doi:10.1074/jbc.M312042200
Cerda, O., Cáceres, M., Park, K.-S., Leiva-Salcedo, E., Romero, A., Varela, D., et al. (2015). Casein kinase-mediated phosphorylation of serine 839 is necessary for basolateral localization of the Ca2+-activated non-selective cation channel TRPM4. Pflugers Arch. 467, 1723–1732. doi:10.1007/s00424-014-1610-3
Chen, L. Y., Zhu, L. Y., Lu, X. Y., Ming, X. Y., and Yang, B. S. (2022). TRPM2 regulates autophagy to participate in hepatitis B virus replication. J. Viral Hepat. 29, 627–636. doi:10.1111/jvh.13710
Chen, Y., Zhang, X., Yang, T., Bi, R., Huang, Z., Ding, H., et al. (2019). Emerging structural biology of TRPM subfamily channels. Cell Calcium 79, 75–79. doi:10.1016/j.ceca.2019.02.011
Cheng, H., Beck, A., Launay, P., Gross, S. A., Stokes, A. J., Kinet, J.-P., et al. (2007). TRPM4 controls insulin secretion in pancreatic beta-cells. Cell Calcium 41, 51–61. doi:10.1016/j.ceca.2006.04.032
Cho, C. H., Kim, E., Lee, Y. S., Yarishkin, O., Yoo, J. C., Park, Y. J., et al. (2014). Depletion of 14-3-3γ reduces the surface expression of Transient Receptor Potential Melastatin 4b (TRPM4b) channels and attenuates TRPM4b-mediated glutamate-induced neuronal cell death. Mol. Brain 7, 52. doi:10.1186/s13041-014-0052-3
Chubanov, V., Mittermeier, L., and Gudermann, T. (2018). Role of kinase-coupled TRP channels in mineral homeostasis. Pharmacol. Ther. 184, 159–176. doi:10.1016/j.pharmthera.2017.11.003
Chubanov, V., Schlingmann, K. P., Waring, J., Heinzinger, J., Kaske, S., Waldegger, S., et al. (2007). Hypomagnesemia with secondary hypocalcemia due to a missense mutation in the putative pore-forming region of TRPM6. J. Biol. Chem. 282, 7656–7667. doi:10.1074/jbc.M611117200
Chubanov, V., Waldegger, S., Mederos y Schnitzler, M., Vitzthum, H., Sassen, M. C., Seyberth, H. W., et al. (2004). Disruption of TRPM6/TRPM7 complex formation by a mutation in the TRPM6 gene causes hypomagnesemia with secondary hypocalcemia. Proc. Natl. Acad. Sci. U. S. A. 101, 2894–2899. doi:10.1073/pnas.0305252101
Clapham, D. E. (2003). TRP channels as cellular sensors. Nature 426, 517–524. doi:10.1038/nature02196
Clapham, D. E., Montell, C., Schultz, G., Julius, D., and International Union of, P. (2003). International Union of Pharmacology. XLIII. Compendium of voltage-gated ion channels: transient receptor potential channels. Pharmacol. Rev. 55, 591–596. doi:10.1124/pr.55.4.6
Clark, K., Middelbeek, J., and van Leeuwen, F. N. (2008). Interplay between TRP channels and the cytoskeleton in health and disease. Eur. J. Cell Biol. 87, 631–640. doi:10.1016/j.ejcb.2008.01.009
Cohen, M. R., and Moiseenkova-Bell, V. Y. (2014). Structure of thermally activated TRP channels. Curr. Top. Membr. 74, 181–211. doi:10.1016/B978-0-12-800181-3.00007-5
Cornejo, V. H., Gonzalez, C., Campos, M., Vargas-Saturno, L., Juricic, M. L. A., Miserey-Lenkei, S., et al. (2020). Non-conventional axonal organelles control TRPM8 Ion Channel trafficking and peripheral cold sensing. Cell Rep. 30, 4505–4517. doi:10.1016/j.celrep.2020.03.017
Cosens, D. J., and Manning, A. (1969). Abnormal electroretinogram from a Drosophila mutant. Nature 224, 285–287. doi:10.1038/224285a0
Csanády, L., and Törocsik, B. (2009). Four Ca2+ ions activate TRPM2 channels by binding in deep crevices near the pore but intracellularly of the gate. J. Gen. Physiol. 133, 189–203. doi:10.1085/jgp.200810109
Cuajungco, M. P., Grimm, C., Oshima, K., D'Hoedt, D., Nilius, B., Mensenkamp, A. R., et al. (2006). PACSINs bind to the TRPV4 cation channel. PACSIN 3 modulates the subcellular localization of TRPV4. J. Biol. Chem. 281, 18753–18762. doi:10.1074/jbc.M602452200
Dai, Y., Wang, S., Tominaga, M., Yamamoto, S., Fukuoka, T., Higashi, T., et al. (2007). Sensitization of TRPA1 by PAR2 contributes to the sensation of inflammatory pain. J. Clin. Invest. 117, 1979–1987. doi:10.1172/JCI30951
De Caro, C., Cristiano, C., Avagliano, C., Bertamino, A., Ostacolo, C., Campiglia, P., et al. (2019). Characterization of new TRPM8 modulators in pain perception. Int. J. Mol. Sci. 20, 5544. doi:10.3390/ijms20225544
Deeds, J., Cronin, F., and Duncan, L. M. (2000). Patterns of melastatin mRNA expression in melanocytic tumors. Hum. Pathol. 31, 1346–1356. doi:10.1053/hupa.2000.19441
de Jong, P. R., Takahashi, N., Peiris, M., Bertin, S., Lee, J., Gareau, M. G., et al. (2015). TRPM8 on mucosal sensory nerves regulates colitogenic responses by innate immune cells via CGRP. Mucosal Immunol. 8, 491–504. doi:10.1038/mi.2014.82
Delprat, B., Crouzier, L., Su, T. P., and Maurice, T. (2020). At the crossing of ER stress and MAMs: a key role of sigma-1 receptor? Adv. Exp. Med. Biol. 1131, 699–718. doi:10.1007/978-3-030-12457-1_28
Desai, B. N., Krapivinsky, G., Navarro, B., Krapivinsky, L., Carter, B. C., Febvay, S., et al. (2012). Cleavage of TRPM7 releases the kinase domain from the ion channel and regulates its participation in Fas-induced apoptosis. Dev. Cell 22, 1149–1162. doi:10.1016/j.devcel.2012.04.006
Dhaka, A., Earley, T. J., Watson, J., and Patapoutian, A. (2008). Visualizing cold spots: TRPM8-expressing sensory neurons and their projections. J. Neurosci. official J. Soc. Neurosci. 28, 566–575. doi:10.1523/JNEUROSCI.3976-07.2008
Dhennin-Duthille, I., Gautier, M., Faouzi, M., Guilbert, A., Brevet, M., Vaudry, D., et al. (2011). High expression of transient receptor potential channels in human breast cancer epithelial cells and tissues: correlation with pathological parameters. Cell Physiol. Biochem. 28, 813–822. doi:10.1159/000335795
Diaz-Franulic, I., Verdugo, C., Gonzalez, F., Gonzalez-Nilo, F., and Latorre, R. (2021). Thermodynamic and structural basis of temperature-dependent gating in TRP channels. Biochem. Soc. Trans. 49, 2211–2219. doi:10.1042/BST20210301
Dietrich, A., Fahlbusch, M., and Gudermann, T. (2014). Classical transient receptor potential 1 (TRPC1): channel or channel regulator? Cells 3, 939–962. doi:10.3390/cells3040939
Dietrich, A., Mederos y Schnitzler, M., Emmel, J., Kalwa, H., Hofmann, T., and Gudermann, T. (2003). N-linked protein glycosylation is a major determinant for basal TRPC3 and TRPC6 channel activity. J. Biol. Chem. 278, 47842–47852. doi:10.1074/jbc.M302983200
Donate-Macian, P., Bano-Polo, M., Vazquez-Ibar, J. L., Mingarro, I., and Peralvarez-Marin, A. (2015). Molecular and topological membrane folding determinants of transient receptor potential vanilloid 2 channel. Biochem. Biophys. Res. Commun. 462, 221–226. doi:10.1016/j.bbrc.2015.04.120
Doñate-Macián, P., Enrich-Bengoa, J., Dégano, I. R., Quintana, D. G., and Perálvarez-Marín, A. (2019). Trafficking of stretch-regulated TRPV2 and TRPV4 channels inferred through interactomics. Biomolecules 9, 791. doi:10.3390/biom9120791
Dong, X. P., Wang, X., and Xu, H. (2010). TRP channels of intracellular membranes. J. Neurochem. 113, 313–328. doi:10.1111/j.1471-4159.2010.06626.x
Du, S.-L., Jia, Z.-Q., Zhong, J.-C., and Wang, L.-F. (2021). TRPC5 in cardiovascular diseases. Rev. Cardiovasc Med. 22, 127–135. doi:10.31083/j.rcm.2021.01.212
Duan, J., Li, J., Zeng, B., Chen, G. L., Peng, X., Zhang, Y., et al. (2018). Structure of the mouse TRPC4 ion channel. Nat. Commun. 9, 3102. doi:10.1038/s41467-018-05247-9
Duncan, L. M., Deeds, J., Hunter, J., Shao, J., Holmgren, L. M., Woolf, E. A., et al. (1998). Down-regulation of the novel gene melastatin correlates with potential for melanoma metastasis. Cancer Res. 58, 1515–1520.
Dutta, D., Martin, L. E., Freichel, M., Torregrossa, A. M., and Medler, K. F. (2018). TRPM4 and TRPM5 are both required for normal signaling in taste receptor cells. Proc. Natl. Acad. Sci. U. S. A. 115, 772–781. doi:10.1073/pnas.1718802115
Elzamzamy, O. M., Johnson, B. E., Chen, W. C., Hu, G., Penner, R., and Hazlehurst, L. A. (2021). Transient receptor potential C 1/4/5 is a determinant of MTI-101 induced calcium influx and cell death in multiple myeloma. Cells 10, 1490. doi:10.3390/cells10061490
Elzamzamy, O. M., Penner, R., and Hazlehurst, L. A. (2020). The role of TRPC1 in modulating cancer progression. Cells 9, 388. doi:10.3390/cells9020388
Enders, M., Heider, T., Ludwig, A., and Kuerten, S. (2020). Strategies for neuroprotection in multiple sclerosis and the role of calcium. Int. J. Mol. Sci. 21, 1663. doi:10.3390/ijms21051663
Englisch, C. N., Paulsen, F., and Tschernig, T. (2022). TRPC channels in the physiology and pathophysiology of the renal tubular system: what do we know? Int. J. Mol. Sci. 24, 181. doi:10.3390/ijms24010181
Erler, I., Al-Ansary, D. M., Wissenbach, U., Wagner, T. F., Flockerzi, V., and Niemeyer, B. A. (2006). Trafficking and assembly of the cold-sensitive TRPM8 channel. J. Biol. Chem. 281, 38396–38404. doi:10.1074/jbc.M607756200
Estadella, I., Pedros-Gamez, O., Colomer-Molera, M., Bosch, M., Sorkin, A., and Felipe, A. (2020). Endocytosis: a turnover mechanism controlling Ion Channel function. Cells 9, 1833. doi:10.3390/cells9081833
Fallah, H. P., Ahuja, E., Lin, H., Qi, J., He, Q., Gao, S., et al. (2022). A review on the role of TRP Channels and their potential as drug Targets_An insight into the TRP channel drug discovery methodologies. Front. Pharmacol. 13, 914499. doi:10.3389/fphar.2022.914499
Fan, C., Choi, W., Sun, W., Du, J., and Lu, W. (2018). Structure of the human lipid-gated cation channel TRPC3. Elife 7, e36852. doi:10.7554/eLife.36852
Ferrandiz-Huertas, C., Mathivanan, S., Wolf, C. J., Devesa, I., and Ferrer-Montiel, A. (2014a). Trafficking of ThermoTRP channels. Membr. (Basel) 4, 525–564. doi:10.3390/membranes4030525
Ferrandiz-Huertas, C., Mathivanan, S., Wolf, C. J., Devesa, I., and Ferrer-Montiel, A. (2014b). Trafficking of ThermoTRP channels. Membr. (Basel) 4, 525–564. doi:10.3390/membranes4030525
Feske, S., Gwack, Y., Prakriya, M., Srikanth, S., Puppel, S. H., Tanasa, B., et al. (2006). A mutation in Orai1 causes immune deficiency by abrogating CRAC channel function. Nature 441, 179–185. doi:10.1038/nature04702
Fischer, M. J., Balasuriya, D., Jeggle, P., Goetze, T. A., McNaughton, P. A., Reeh, P. W., et al. (2014). Direct evidence for functional TRPV1/TRPA1 heteromers. Pflugers Arch. 466, 2229–2241. doi:10.1007/s00424-014-1497-z
Fleig, A., and Penner, R. (2004). The TRPM ion channel subfamily: molecular, biophysical and functional features. Trends Pharmacol. Sci. 25, 633–639. doi:10.1016/j.tips.2004.10.004
Fonfria, E., Murdock, P. R., Cusdin, F. S., Benham, C. D., Kelsell, R. E., and McNulty, S. (2006). Tissue distribution profiles of the human TRPM cation channel family. J. Recept Signal Transduct. Res. 26, 159–178. doi:10.1080/10799890600637506
Fowler, M. A., Sidiropoulou, K., Ozkan, E. D., Phillips, C. W., and Cooper, D. C. (2007). Corticolimbic expression of TRPC4 and TRPC5 channels in the rodent brain. PLoS One 2, e573. doi:10.1371/journal.pone.0000573
Frühwald, J., Londoño, J. C., Dembla, S., Mannebach, S., Lis, A., Drews, A., et al. (2012). Alternative splicing of a protein domain indispensable for function of transient receptor potential melastatin 3 (TRPM3) ion channels. J. Biol. Chem. 287, 36663–36672. doi:10.1074/jbc.M112.396663
Fuessel, S., Sickert, D., Meye, A., Klenk, U., Schmidt, U., Schmitz, M., et al. (2003). Multiple tumor marker analyses (PSA, hK2, PSCA, trp-p8) in primary prostate cancers using quantitative RT-PCR. Int. J. Oncol. 23, 221–228. doi:10.3892/ijo.23.1.221
Fujita, M., Sato, T., Yajima, T., Masaki, E., and Ichikawa, H. (2017). TRPC1, TRPC3, and TRPC4 in rat orofacial structures. Cells Tissues Organs 204, 293–303. doi:10.1159/000477665
Furukawa, C., Fujii, N., Manabe, A., Matsunaga, T., Endo, S., Hasegawa, H., et al. (2017). Up-regulation of transient receptor potential melastatin 6 channel expression by tumor necrosis factor-α in the presence of epidermal growth factor receptor tyrosine kinase inhibitor. J. Cell Physiol. 232, 2841–2850. doi:10.1002/jcp.25709
Gaudet, R. (2008). TRP channels entering the structural era. J. Physiol. 586, 3565–3575. doi:10.1113/jphysiol.2008.155812
Gebhard, A. W., Jain, P., Nair, R. R., Emmons, M. F., Argilagos, R. F., Koomen, J. M., et al. (2013). MTI-101 (cyclized HYD1) binds a CD44 containing complex and induces necrotic cell death in multiple myeloma. Mol. Cancer Ther. 12, 2446–2458. doi:10.1158/1535-7163.MCT-13-0310
Gerke, V., and Moss, S. E. (2002). Annexins: from structure to function. Physiol. Rev. 82, 331–371. doi:10.1152/physrev.00030.2001
Ghosh, D., Pinto, S., Danglot, L., Vandewauw, I., Segal, A., Van Ranst, N., et al. (2016). VAMP7 regulates constitutive membrane incorporation of the cold-activated channel TRPM8. Nat. Commun. 7, 10489. doi:10.1038/ncomms10489
Ghosh, D., Segal, A., and Voets, T. (2014). Distinct modes of perimembrane TRP channel turnover revealed by TIR-FRAP. Sci. Rep. 4, 7111. doi:10.1038/srep07111
Gkika, D., Lemonnier, L., Shapovalov, G., Gordienko, D., Poux, C., Bernardini, M., et al. (2015). TRP channel-associated factors are a novel protein family that regulates TRPM8 trafficking and activity. J. Cell Biol. 208, 89–107. doi:10.1083/jcb.201402076
Goel, M., Zuo, C. D., Sinkins, W. G., and Schilling, W. P. (2007). TRPC3 channels colocalize with Na+/Ca2+ exchanger and Na+ pump in axial component of transverse-axial tubular system of rat ventricle. Am. J. Physiol. Heart Circ. Physiol. 292, H874–H883. doi:10.1152/ajpheart.00785.2006
Gonzalez-Cobos, J. C., and Trebak, M. (2010). TRPC channels in smooth muscle cells. Front. Biosci. Landmark Ed. 15, 1023–1039. doi:10.2741/3660
Greka, A., Navarro, B., Oancea, E., Duggan, A., and Clapham, D. E. (2003). TRPC5 is a regulator of hippocampal neurite length and growth cone morphology. Nat. Neurosci. 6, 837–845. doi:10.1038/nn1092
Groenestege, W. M., Hoenderop, J. G., van den Heuvel, L., Knoers, N., and Bindels, R. J. (2006). The epithelial Mg2+ channel transient receptor potential melastatin 6 is regulated by dietary Mg2+ content and estrogens. J. Am. Soc. Nephrol. 17, 1035–1043. doi:10.1681/ASN.2005070700
Guilbert, A., Gautier, M., Dhennin-Duthille, I., Rybarczyk, P., Sahni, J., Sevestre, H., et al. (2013). Transient receptor potential melastatin 7 is involved in oestrogen receptor-negative metastatic breast cancer cells migration through its kinase domain. Eur. J. Cancer 49, 3694–3707. doi:10.1016/j.ejca.2013.07.008
Guo, H., Carlson, J. A., and Slominski, A. (2012). Role of TRPM in melanocytes and melanoma. Exp. Dermatol 21, 650–654. doi:10.1111/j.1600-0625.2012.01565.x
Hagmann, H., Mangold, N., Rinschen, M. M., Koenig, T., Kunzelmann, K., Schermer, B., et al. (2018). Proline-dependent and basophilic kinases phosphorylate human TRPC6 at serine 14 to control channel activity through increased membrane expression. Faseb J. 32, 208–219. doi:10.1096/fj.201700309R
Hall, B. E., Prochazkova, M., Sapio, M. R., Minetos, P., Kurochkina, N., Binukumar, B. K., et al. (2018). Phosphorylation of the transient receptor potential ankyrin 1 by cyclin-dependent kinase 5 affects chemo-nociception. Sci. Rep. 8, 1177. doi:10.1038/s41598-018-19532-6
Hanner, M., Moebius, F. F., Flandorfer, A., Knaus, H. G., Striessnig, J., Kempner, E., et al. (1996). Purification, molecular cloning, and expression of the mammalian sigma1-binding site. Proc. Natl. Acad. Sci. U. S. A. 93, 8072–8077. doi:10.1073/pnas.93.15.8072
Hantute-Ghesquier, A., Haustrate, A., Prevarskaya, N., and Lehen'kyi, V. (2018). TRPM family channels in cancer. Pharmaceuticals-Base 11, 58. doi:10.3390/ph11020058
Hara, Y., Wakamori, M., Ishii, M., Maeno, E., Nishida, M., Yoshida, T., et al. (2002). LTRPC2 Ca2+-permeable channel activated by changes in redox status confers susceptibility to cell death. Mol. Cell 9, 163–173. doi:10.1016/s1097-2765(01)00438-5
Hardie, R. C., and Minke, B. (1992). The trp gene is essential for a light-activated Ca2+ channel in Drosophila photoreceptors. Neuron 8, 643–651. doi:10.1016/0896-6273(92)90086-s
Hasan, N., Pangeni, G., Cobb, C. A., Ray, T. A., Nettesheim, E. R., Ertel, K. J., et al. (2019). Presynaptic expression of LRIT3 transsynaptically organizes the postsynaptic glutamate signaling complex containing TRPM1. Cell Rep. 27, 3107–3116. doi:10.1016/j.celrep.2019.05.056
He, Z., Jia, C., Feng, S., Zhou, K., Tai, Y., Bai, X., et al. (2012). TRPC5 channel is the mediator of neurotrophin-3 in regulating dendritic growth via CaMKIIα in rat hippocampal neurons. J. Neurosci. 32, 9383–9395. doi:10.1523/JNEUROSCI.6363-11.2012
Held, K., and Tóth, B. I. (2021). TRPM3 in brain (Patho)Physiology. Front. Cell Dev. Biol. 9, 635659. doi:10.3389/fcell.2021.635659
Held, K., Voets, T., and Vriens, J. (2015). TRPM3 in temperature sensing and beyond. Temp. (Austin) 2, 201–213. doi:10.4161/23328940.2014.988524
Hofmann, T., Obukhov, A. G., Schaefer, M., Harteneck, C., Gudermann, T., and Schultz, G. (1999). Direct activation of human TRPC6 and TRPC3 channels by diacylglycerol. Nature 397, 259–263. doi:10.1038/16711
Hong, C., Jeong, B., Park, H. J., Chung, J. Y., Lee, J. E., Kim, J., et al. (2020). TRP channels as emerging therapeutic targets for neurodegenerative diseases. Front. Physiol. 11, 238. doi:10.3389/fphys.2020.00238
Hong, C., Kwak, M., Myeong, J., Ha, K., Wie, J., Jeon, J. H., et al. (2015). Extracellular disulfide bridges stabilize TRPC5 dimerization, trafficking, and activity. Pflugers Arch. 467, 703–712. doi:10.1007/s00424-014-1540-0
Hoth, M., and Penner, R. (1992). Depletion of intracellular calcium stores activates a calcium current in mast cells. Nature 355, 353–356. doi:10.1038/355353a0
Hou, J., Renigunta, V., Nie, M., Sunq, A., Himmerkus, N., Quintanova, C., et al. (2019). Phosphorylated claudin-16 interacts with Trpv5 and regulates transcellular calcium transport in the kidney. Proc. Natl. Acad. Sci. U. S. A. 116, 19176–19186. doi:10.1073/pnas.1902042116
Hsu, Y. J., Hoenderop, J. G., and Bindels, R. J. (2007). TRP channels in kidney disease. Biochim. Biophys. Acta 1772, 928–936. doi:10.1016/j.bbadis.2007.02.001
Hu, Q., Ahmad, A. A., Seidel, T., Hunter, C., Streiff, M., Nikolova, L., et al. (2020). Location and function of transient receptor potential canonical channel 1 in ventricular myocytes. J. Mol. Cell Cardiol. 139, 113–123. doi:10.1016/j.yjmcc.2020.01.008
Huang, Y. H., Winkler, P. A., Sun, W. N., Lü, W., and Du, J. (2018). Architecture of the TRPM2 channel and its activation mechanism by ADP-ribose and calcium. Nature 562, 145–149. doi:10.1038/s41586-018-0558-4
Hynkova, A., Marsakova, L., Vaskova, J., and Vlachova, V. (2016). N-terminal tetrapeptide T/SPLH motifs contribute to multimodal activation of human TRPA1 channel. Sci. Rep. 6, 28700. doi:10.1038/srep28700
Iftinca, M., and Altier, C. (2020). The cool things to know about TRPM8. Channels (Austin). 14, 413–420. doi:10.1080/19336950.2020.1841419
Iordanov, I., Mihalyi, C., Toth, B., and Csanady, L. (2016). The proposed channel-enzyme transient receptor potential melastatin 2 does not possess ADP ribose hydrolase activity. Elife 5, e17600. doi:10.7554/eLife.17600
Iosifidis, C., Liu, J., Gale, T., Ellingford, J. M., Campbell, C., Ingram, S., et al. (2022). Clinical and genetic findings in TRPM1-related congenital stationary night blindness. Acta Ophthalmol. 100, e1332–e1339. doi:10.1111/aos.15186
Isshiki, M., and Anderson, R. G. (2003). Function of caveolae in Ca2+ entry and Ca2+-dependent signal transduction. Traffic 4, 717–723. doi:10.1034/j.1600-0854.2003.00130.x
Jahn, R., Lang, T., and Sudhof, T. C. (2003). Membrane fusion. Cell 112, 519–533. doi:10.1016/s0092-8674(03)00112-0
Jiang, Q., Fu, X., Tian, L., Chen, Y., Yang, K., Chen, X., et al. (2014). NOX4 mediates BMP4-induced upregulation of TRPC1 and 6 protein expressions in distal pulmonary arterial smooth muscle cells. PLoS One 9, e107135. doi:10.1371/journal.pone.0107135
Jimenez, I., Prado, Y., Marchant, F., Otero, C., Eltit, F., Cabello-Verrugio, C., et al. (2020). TRPM channels in human diseases. Cells 9, 2604. doi:10.3390/cells9122604
Kalia, J., and Swartz, K. J. (2013). Exploring structure-function relationships between TRP and Kv channels. Sci. Rep. 3, 1523. doi:10.1038/srep01523
Kanda, S., Harita, Y., Shibagaki, Y., Sekine, T., Igarashi, T., Inoue, T., et al. (2011). Tyrosine phosphorylation-dependent activation of TRPC6 regulated by PLC-γ1 and nephrin: effect of mutations associated with focal segmental glomerulosclerosis. Mol. Biol. Cell 22, 1824–1835. doi:10.1091/mbc.E10-12-0929
Kanzaki, M., Zhang, Y. Q., Mashima, H., Li, L., Shibata, H., and Kojima, I. (1999). Translocation of a calcium-permeable cation channel induced by insulin-like growth factor-I. Nat. Cell Biol. 1, 165–170. doi:10.1038/11086
Karashima, Y., Prenen, J., Meseguer, V., Owsianik, G., Voets, T., and Nilius, B. (2008). Modulation of the transient receptor potential channel TRPA1 by phosphatidylinositol 4,5-biphosphate manipulators. Pflugers Arch. 457, 77–89. doi:10.1007/s00424-008-0493-6
Kaske, S., Krasteva, G., Konig, P., Kummer, W., Hofmann, T., Gudermann, T., et al. (2007). TRPM5, a taste-signaling transient receptor potential ion-channel, is a ubiquitous signaling component in chemosensory cells. BMC Neurosci. 8, 49. doi:10.1186/1471-2202-8-49
Ketterer, C., Mussig, K., Heni, M., Dudziak, K., Randrianarisoa, E., Wagner, R., et al. (2011). Genetic variation within the TRPM5 locus associates with prediabetic phenotypes in subjects at increased risk for type 2 diabetes. Metabolism 60, 1325–1333. doi:10.1016/j.metabol.2011.02.002
Khan, S., Patra, P. H., Somerfield, H., Benya-Aphikul, H., Upadhya, M., and Zhang, X. (2023). IQGAP1 promotes chronic pain by regulating the trafficking and sensitization of TRPA1 channels. Brain 146, 2595–2611. doi:10.1093/brain/awac462
Khanna, R., Myers, M. P., Laine, M., and Papazian, D. M. (2001). Glycosylation increases potassium channel stability and surface expression in mammalian cells. J. Biol. Chem. 276, 34028–34034. doi:10.1074/jbc.M105248200
Kheradpezhouh, E., Zhou, F. H., Barritt, G. J., and Rychkov, G. Y. (2018). Oxidative stress promotes redistribution of TRPM2 channels to the plasma membrane in hepatocytes. Biochem. Biophys. Res. Commun. 503, 1891–1896. doi:10.1016/j.bbrc.2018.07.132
Kim, B. J., So, I., and Kim, K. W. (2006). The relationship of TRP channels to the pacemaker activity of interstitial cells of Cajal in the gastrointestinal tract. J. Smooth Muscle Res. 42, 1–7. doi:10.1540/jsmr.42.1
Kim, D., Cavanaugh, E. J., and Simkin, D. (2008). Inhibition of transient receptor potential A1 channel by phosphatidylinositol-4,5-bisphosphate. Am. J. Physiol. Cell Physiol. 295, C92–C99. doi:10.1152/ajpcell.00023.2008
Kim, H. Y., Chung, G., Jo, H. J., Kim, Y. S., Bae, Y. C., Jung, S. J., et al. (2011). Characterization of dental nociceptive neurons. J. Dent. Res. 90, 771–776. doi:10.1177/0022034511399906
Kim, J., Ko, J., Hong, C., and So, I. (2019). Structure-function relationship and physiological roles of transient receptor potential canonical (TRPC) 4 and 5 channels. Cells 9, 73. doi:10.3390/cells9010073
Kim, Y. S., Son, J. Y., Kim, T. H., Paik, S. K., Dai, Y., Noguchi, K., et al. (2010). Expression of transient receptor potential ankyrin 1 (TRPA1) in the rat trigeminal sensory afferents and spinal dorsal horn. J. Comp. Neurol. 518, 687–698. doi:10.1002/cne.22238
Kiselyov, K., Xu, X., Mozhayeva, G., Kuo, T., Pessah, I., Mignery, G., et al. (1998). Functional interaction between InsP3 receptors and store-operated Htrp3 channels. Nature 396, 478–482. doi:10.1038/24890
Kobayashi, K., Fukuoka, T., Obata, K., Yamanaka, H., Dai, Y., Tokunaga, A., et al. (2005). Distinct expression of TRPM8, TRPA1, and TRPV1 mRNAs in rat primary afferent neurons with adelta/c-fibers and colocalization with trk receptors. J. Comp. Neurol. 493, 596–606. doi:10.1002/cne.20794
Koike, C., Obara, T., Uriu, Y., Numata, T., Sanuki, R., Miyata, K., et al. (2010). TRPM1 is a component of the retinal ON bipolar cell transduction channel in the mGluR6 cascade. Proc. Natl. Acad. Sci. U. S. A. 107, 332–337. doi:10.1073/pnas.0912730107
Koivisto, A. P., Belvisi, M. G., Gaudet, R., and Szallasi, A. (2022). Advances in TRP channel drug discovery: from target validation to clinical studies. Nat. Rev. Drug Discov. 21, 41–59. doi:10.1038/s41573-021-00268-4
Krapivinsky, G., Krapivinsky, L., Manasian, Y., and Clapham, D. E. (2014). The TRPM7 chanzyme is cleaved to release a chromatin-modifying kinase. Cell. 157, 1061–1072. doi:10.1016/j.cell.2014.03.046
Krapivinsky, G., Mochida, S., Krapivinsky, L., Cibulsky, S. M., and Clapham, D. E. (2006). The TRPM7 ion channel functions in cholinergic synaptic vesicles and affects transmitter release. Neuron 52, 485–496. doi:10.1016/j.neuron.2006.09.033
Kruse, M., Schulze-Bahr, E., Corfield, V., Beckmann, A., Stallmeyer, B., Kurtbay, G., et al. (2009). Impaired endocytosis of the ion channel TRPM4 is associated with human progressive familial heart block type I. J. Clin. Invest. 119, 2737–2744. doi:10.1172/JCI38292
Lainez, S., Schlingmann, K. P., van der Wijst, J., Dworniczak, B., van Zeeland, F., Konrad, M., et al. (2014). New TRPM6 missense mutations linked to hypomagnesemia with secondary hypocalcemia. Eur. J. Hum. Genet. 22, 497–504. doi:10.1038/ejhg.2013.178
Laínez, S. V. P., Ontoria-Oviedo, I., Estévez-Herrera, J., Camprubí-Robles, M., Ferrer-Montiel, A., Planells-Cases, R., et al. (2010). GABA A receptor associated protein (GABARAP) modulates TRPV1 expression and channel function and desensitization. FASEB J. 24, 1958–1970. doi:10.1096/fj.09-151472
Lameris, A. L., Nevalainen, P. I., Reijnen, D., Simons, E., Eygensteyn, J., Monnens, L., et al. (2015). Segmental transport of Ca2+ and Mg2+ along the gastrointestinal tract. Am. J. Physiol. Gastrointest. Liver Physiol. 308, G206–G216. doi:10.1152/ajpgi.00093.2014
Laude, A. J., and Prior, I. A. (2004). Plasma membrane microdomains: organization, function and trafficking. Mol. Membr. Biol. 21, 193–205. doi:10.1080/09687680410001700517
Launay, P., Fleig, A., Perraud, A. L., Scharenberg, A. M., Penner, R., and Kinet, J. P. (2002). TRPM4 is a Ca2+-activated nonselective cation channel mediating cell membrane depolarization. Cell 109, 397–407. doi:10.1016/s0092-8674(02)00719-5
Lavanderos, B., Silva, I., Cruz, P., Orellana-Serradell, O., Saldias, M. P., and Cerda, O. (2020). TRP channels regulation of rho GTPases in brain context and diseases. Front. Cell Dev. Biol. 8, 582975. doi:10.3389/fcell.2020.582975
Lee, B. C., Hong, S. E., Lim, H. H., Kim, D. H., and Park, C. S. (2011). Alteration of the transcriptional profile of human embryonic kidney cells by transient overexpression of mouse TRPM7 channels. Cell Physiol. Biochem. 27, 313–326. doi:10.1159/000327958
Lepage, P. K., Lussier, M. P., Barajas-Martinez, H., Bousquet, S. M., Blanchard, A. P., Francoeur, N., et al. (2006). Identification of two domains involved in the assembly of transient receptor potential canonical channels. J. Biol. Chem. 281, 30356–30364. doi:10.1074/jbc.M603930200
Li, C., Ma, W., Yin, S., Liang, X., Shu, X., Pei, D., et al. (2016). Sorting nexin 11 regulates lysosomal degradation of plasma membrane TRPV3. Traffic 17, 500–514. doi:10.1111/tra.12379
Li, H. S., Xu, X. Z., and Montell, C. (1999). Activation of a TRPC3-dependent cation current through the neurotrophin BDNF. Neuron 24, 261–273. doi:10.1016/s0896-6273(00)80838-7
Lin, W., Ezekwe, E. A., Zhao, Z., Liman, E. R., and Restrepo, D. (2008). TRPM5-expressing microvillous cells in the main olfactory epithelium. BMC Neurosci. 9, 114. doi:10.1186/1471-2202-9-114
Liu, D., and Liman, E. R. (2003). Intracellular Ca2+ and the phospholipid PIP2 regulate the taste transduction ion channel TRPM5. Proc. Natl. Acad. Sci. U. S. A. 100, 15160–15165. doi:10.1073/pnas.2334159100
Liu, H., Chatel, S., Simard, C., Syam, N., Salle, L., Probst, V., et al. (2013). Molecular genetics and functional anomalies in a series of 248 Brugada cases with 11 mutations in the TRPM4 channel. PLoS One 8, e54131. doi:10.1371/journal.pone.0054131
Liu, H., El Zein, L., Kruse, M., Guinamard, R., Beckmann, A., Bozio, A., et al. (2010). Gain-of-function mutations in TRPM4 cause autosomal dominant isolated cardiac conduction disease. Circ. Cardiovasc Genet. 3, 374–385. doi:10.1161/CIRCGENETICS.109.930867
Liu, X., Wang, W., Singh, B. B., Lockwich, T., Jadlowiec, J., O'Connell, B., et al. (2000). Trp1, a candidate protein for the store-operated Ca2+ influx mechanism in salivary gland cells. J. Biol. Chem. 275, 3403–3411. doi:10.1074/jbc.275.5.3403
Liu, Y., Mikrani, R., He, Y., Faran Ashraf Baig, M. M., Abbas, M., Naveed, M., et al. (2020). TRPM8 channels: a review of distribution and clinical role. Eur. J. Pharmacol. 882, 173312. doi:10.1016/j.ejphar.2020.173312
Lockwich, T., Singh, B. B., Liu, X., and Ambudkar, I. S. (2001). Stabilization of cortical actin induces internalization of transient receptor potential 3 (Trp3)-associated caveolar Ca2+ signaling complex and loss of Ca2+ influx without disruption of Trp3-inositol trisphosphate receptor association. J. Biol. Chem. 276, 42401–42408. doi:10.1074/jbc.M106956200
Lockwich, T. P., Liu, X., Singh, B. B., Jadlowiec, J., Weiland, S., and Ambudkar, I. S. (2000). Assembly of Trp1 in a signaling complex associated with caveolin-scaffolding lipid raft domains. J. Biol. Chem. 275, 11934–11942. doi:10.1074/jbc.275.16.11934
Luongo, F., Pietropaolo, G., Gautier, M., Dhennin-Duthille, I., Ouadid-Ahidouch, H., Wolf, F. I., et al. (2018). TRPM6 is essential for magnesium uptake and epithelial cell function in the colon. Nutrients 10, 784. doi:10.3390/nu10060784
Lüscher, T. F. (2015). Predictors as well as surrogate and hard endpoints in cardiovascular disease. Eur. Heart J. 36, 2197–2199. doi:10.1093/eurheartj/ehv327
Lussier, M. P., Cayouette, S., Lepage, P. K., Bernier, C. L., Francoeur, N., St-Hilaire, M., et al. (2005). MxA, a member of the dynamin superfamily, interacts with the ankyrin-like repeat domain of TRPC. J. Biol. Chem. 280, 19393–19400. doi:10.1074/jbc.M500391200
Ma, H. T., Patterson, R. L., van Rossum, D. B., Birnbaumer, L., Mikoshiba, K., and Gill, D. L. (2000). Requirement of the inositol trisphosphate receptor for activation of store-operated Ca2+ channels. Science 287, 1647–1651. doi:10.1126/science.287.5458.1647
Ma, X., Cao, J., Luo, J., Nilius, B., Huang, Y., Ambudkar, I. S., et al. (2010). Depletion of intracellular Ca2+ stores stimulates the translocation of vanilloid transient receptor potential 4-C1 heteromeric channels to the plasma membrane. Arterioscler. Thromb. Vasc. Biol. 30, 2249–2255. doi:10.1161/ATVBAHA.110.212084
Malko, P., Mortadza, S. A. S., McWilliam, J., and Jiang, L. H. (2019). TRPM2 channel in microglia as a new player in neuroinflammation associated with a spectrum of central nervous system pathologies. Front. Pharmacol. 10, 239. doi:10.3389/fphar.2019.00239
Marcotti, A., Fernandez-Trillo, J., Gonzalez, A., Vizcaino-Escoto, M., Ros-Arlanzon, P., Romero, L., et al. (2023). TRPA1 modulation by Sigma-1 receptor prevents oxaliplatin-induced painful peripheral neuropathy. Brain 146, 475–491. doi:10.1093/brain/awac273
McKemy, D. D., Neuhausser, W. M., and Julius, D. (2002). Identification of a cold receptor reveals a general role for TRP channels in thermosensation. Nature 416, 52–58. doi:10.1038/nature719
McNulty, S., and Fonfria, E. (2005). The role of TRPM channels in cell death. Pflugers Arch. 451, 235–242. doi:10.1007/s00424-005-1440-4
Meents, J. E., Fischer, M. J., and McNaughton, P. A. (2017). Sensitization of TRPA1 by protein kinase A. PLoS One 12, e0170097. doi:10.1371/journal.pone.0170097
Mehta, D., Ahmmed, G. U., Paria, B. C., Holinstat, M., Voyno-Yasenetskaya, T., Tiruppathi, C., et al. (2003). RhoA interaction with inositol 1,4,5-trisphosphate receptor and transient receptor potential channel-1 regulates Ca2+ entry. Role in signaling increased endothelial permeability. J. Biol. Chem. 278, 33492–33500. doi:10.1074/jbc.M302401200
Mei, Z. Z., and Jiang, L. H. (2009). Requirement for the N-terminal coiled-coil domain for expression and function, but not subunit interaction of, the ADPR-activated TRPM2 channel. J. Membr. Biol. 230, 93–99. doi:10.1007/s00232-009-9190-4
Meng, J., Wang, J., Steinhoff, M., and Dolly, J. O. (2016). TNFα induces co-trafficking of TRPV1/TRPA1 in VAMP1-containing vesicles to the plasmalemma via Munc18-1/syntaxin1/SNAP-25 mediated fusion. Sci. Rep. 6, 21226. doi:10.1038/srep21226
Mery, L., Strauss, B., Dufour, J. F., Krause, K. H., and Hoth, M. (2002). The PDZ-interacting domain of TRPC4 controls its localization and surface expression in HEK293 cells. J. Cell Sci. 115, 3497–3508. doi:10.1242/jcs.115.17.3497
Mickle, A. D., Shepherd, A. J., and Mohapatra, D. P. (2015). Sensory TRP channels: the key transducers of nociception and pain. Prog. Mol. Biol. Transl. 131, 73–118. doi:10.1016/bs.pmbts.2015.01.002
Moiseenkova-Bell, V., and Wensel, T. G. (2011). Functional and structural studies of TRP channels heterologously expressed in budding yeast. Adv. Exp. Med. Biol. 704, 25–40. doi:10.1007/978-94-007-0265-3_2
Montecinos-Oliva, C., Schuller, A., Parodi, J., Melo, F., and Inestrosa, N. C. (2014). Effects of tetrahydrohyperforin in mouse hippocampal slices: neuroprotection, long-term potentiation and TRPC channels. Curr. Med. Chem. 21, 3494–3506. doi:10.2174/0929867321666140716091229
Montell, C. (2005). The TRP superfamily of cation channels. Sci. STKE 2005, re3. doi:10.1126/stke.2722005re3
Montell, C., and Rubin, G. M. (1989). Molecular characterization of the Drosophila trp locus: a putative integral membrane protein required for phototransduction. Neuron 2, 1313–1323. doi:10.1016/0896-6273(89)90069-x
Moran, M. M., McAlexander, M. A., Biro, T., and Szallasi, A. (2011). Transient receptor potential channels as therapeutic targets. Nat. Rev. Drug Discov. 10, 601–620. doi:10.1038/nrd3456
Morenilla-Palao, C., Pertusa, M., Meseguer, V., Cabedo, H., and Viana, F. (2009). Lipid raft segregation modulates TRPM8 channel activity. J. Biol. Chem. 284, 9215–9224. doi:10.1074/jbc.M807228200
Morenilla-Palao, C., Planells-Cases, R., Garcia-Sanz, N., and Ferrer-Montiel, A. (2004). Regulated exocytosis contributes to protein kinase C potentiation of vanilloid receptor activity. J. Biol. Chem. 279, 25665–25672. doi:10.1074/jbc.M311515200
Mori, Y., Takada, N., Okada, T., Wakamori, M., Imoto, K., Wanifuchi, H., et al. (1998). Differential distribution of TRP Ca2+ channel isoforms in mouse brain. Neuroreport 9, 507–515.
Müller, I., Alt, P., Rajan, S., Schaller, L., Geiger, F., and Dietrich, A. (2022). Transient receptor potential (TRP) channels in airway toxicity and disease: an update. Cells 11, 2907. doi:10.3390/cells11182907
Myeong, J., Ko, J., Kwak, M., Kim, J., Woo, J., Ha, K., et al. (2018). Dual action of the Gαq-PLCβ-PI(4,5)P2 pathway on TRPC1/4 and TRPC1/5 heterotetramers. Sci. Rep. 8, 12117. doi:10.1038/s41598-018-30625-0
Myeong, J., Kwak, M., Hong, C., Jeon, J. H., and So, I. (2014). Identification of a membrane-targeting domain of the transient receptor potential canonical (TRPC)4 channel unrelated to its formation of a tetrameric structure. J. Biol. Chem. 289, 34990–35002. doi:10.1074/jbc.M114.584649
Nadler, M. J., Hermosura, M. C., Inabe, K., Perraud, A. L., Zhu, Q., Stokes, A. J., et al. (2001). LTRPC7 is a Mg.ATP-regulated divalent cation channel required for cell viability. Nature 411, 590–595. doi:10.1038/35079092
Nakamura, M., Sanuki, R., Yasuma, T. R., Onishi, A., Nishiguchi, K. M., Koike, C., et al. (2010). TRPM1 mutations are associated with the complete form of congenital stationary night blindness. Mol. Vis. 16, 425–437.
Nazıroglu, M., and Demirdas, A. (2015). Psychiatric disorders and TRP channels: focus on psychotropic drugs. Curr. Neuropharmacol. 13, 248–257. doi:10.2174/1570159x13666150304001606
Neuillé, M., Morgans, C. W., Cao, Y., Orhan, E., Michiels, C., Sahel, J. A., et al. (2015). LRIT3 is essential to localize TRPM1 to the dendritic tips of depolarizing bipolar cells and may play a role in cone synapse formation. Eur. J. Neurosci. 42, 1966–1975. doi:10.1111/ejn.12959
Nilius, B. (2007). Transient receptor potential (TRP) cation channels: rewarding unique proteins. Bull. Mem. Acad. R. Med. Belg 162, 244–253.
Nilius, B., Prenen, J., and Owsianik, G. (2011). Irritating channels: the case of TRPA1. J. Physiol. 589, 1543–1549. doi:10.1113/jphysiol.2010.200717
Nugent, M., Yusef, Y. R., Meng, J., Wang, J., and Dolly, J. O. (2018). A SNAP-25 cleaving chimera of botulinum neurotoxin/A and/E prevents TNFα-induced elevation of the activities of native TRP channels on early postnatal rat dorsal root ganglion neurons. Neuropharmacology 138, 257–266. doi:10.1016/j.neuropharm.2018.06.016
Oancea, E., and Wicks, N. L. (2011). TRPM1: new trends for an old TRP. Adv. Exp. Med. Biol. 704, 135–145. doi:10.1007/978-94-007-0265-3_7
Oancea, E., Wolfe, J. T., and Clapham, D. E. (2006). Functional TRPM7 channels accumulate at the plasma membrane in response to fluid flow. Circ. Res. 98, 245–253. doi:10.1161/01.RES.0000200179.29375.cc
Oberwinkler, J., and Philipp, S. E. (2014). Trpm3. Handb. Exp. Pharmacol. 222, 427–459. doi:10.1007/978-3-642-54215-2_17
Oda, S., Numaga-Tomita, T., Kitajima, N., Toyama, T., Harada, E., Shimauchi, T., et al. (2017). TRPC6 counteracts TRPC3-Nox2 protein complex leading to attenuation of hyperglycemia-induced heart failure in mice. Sci. Rep. 7, 7511. doi:10.1038/s41598-017-07903-4
Ong, H. L., and Ambudkar, I. S. (2011). The dynamic complexity of the TRPC1 channelosome. Channels (Austin). 5, 424–431. doi:10.4161/chan.5.5.16471
Palmer, K. J., Konkel, J. E., and Stephens, D. J. (2005). PCTAIRE protein kinases interact directly with the COPII complex and modulate secretory cargo transport. J. Cell Sci. 118, 3839–3847. doi:10.1242/jcs.02496
Patterson, R. L., van Rossum, D. B., Ford, D. L., Hurt, K. J., Bae, S. S., Suh, P. G., et al. (2002). Phospholipase C-γ is required for agonist-induced Ca2+ entry. Cell 111, 529–541. doi:10.1016/s0092-8674(02)01045-0
Perez, C. A., Huang, L., Rong, M., Kozak, J. A., Preuss, A. K., Zhang, H., et al. (2002). A transient receptor potential channel expressed in taste receptor cells. Nat. Neurosci. 5, 1169–1176. doi:10.1038/nn952
Perraud, A.-L., Fleig, A., Dunn, C. A., Bagley, L. A., Launay, P., Schmitz, C., et al. (2001). ADP-ribose gating of the calcium-permeable LTRPC2 channel revealed by Nudix motif homology. Nature 411, 595–599. doi:10.1038/35079100
Philipp, S., Trost, C., Warnat, J., Rautmann, J., Himmerkus, N., Schroth, G., et al. (2000). TRP4 (CCE1) protein is part of native calcium release-activated Ca2+-like channels in adrenal cells. J. Biol. Chem. 275, 23965–23972. doi:10.1074/jbc.M003408200
Prawitt, D., Monteilh-Zoller, M. K., Brixel, L., Spangenberg, C., Zabel, B., Fleig, A., et al. (2003). TRPM5 is a transient Ca2+-activated cation channel responding to rapid changes in [Ca2+]i. Proc. Natl. Acad. Sci. U. S. A. 100, 15166–15171. doi:10.1073/pnas.2334624100
Proudfoot, C. J., Garry, E. M., Cottrell, D. F., Rosie, R., Anderson, H., Robertson, D. C., et al. (2006). Analgesia mediated by the TRPM8 cold receptor in chronic neuropathic pain. Curr. Biol. 16, 1591–1605. doi:10.1016/j.cub.2006.07.061
Pumroy, R. A., Fluck, E. C., Ahmed, T., and Moiseenkova-Bell, V. Y. (2020). Structural insights into the gating mechanisms of TRPV channels. Cell Calcium 87, 102168. doi:10.1016/j.ceca.2020.102168
Puram, S. V., Riccio, A., Koirala, S., Ikeuchi, Y., Kim, A. H., Corfas, G., et al. (2011). A TRPC5-regulated calcium signaling pathway controls dendrite patterning in the mammalian brain. Genes and Dev. 25, 2659–2673. doi:10.1101/gad.174060.111
Putney, J. W., Trebak, M., Vazquez, G., Wedel, B., and Bird, G. S. (2004). Signalling mechanisms for TRPC3 channels. Novartis Found. Symp. 258, 123–266.
Ramon, L., Sebastian, B., Gerardo, O., Cristián, Z., and Guillermo, V. (2007). ThermoTRP channels as modular proteins with allosteric gating. Cell Calcium 42, 427–438. doi:10.1016/j.ceca.2007.04.004
Ramsey, I. S., Delling, M., and Clapham, D. E. (2006). An introduction to TRP channels. Annu. Rev. Physiol. 68, 619–647. doi:10.1146/annurev.physiol.68.040204.100431
Reid, G., and Flonta, M. L. (2001). Physiology. Cold current in thermoreceptive neurons. Nature 413, 480. doi:10.1038/35097164
Reinach, P. S., Mergler, S., Okada, Y., and Saika, S. (2015). Ocular transient receptor potential channel function in health and disease. BMC Ophthalmol. 15 (Suppl. 1), 153. doi:10.1186/s12886-015-0135-7
Rivas, J., Díaz, N., Silva, I., Morales, D., Lavanderos, B., Alvarez, A., et al. (2020). KCTD5, a novel TRPM4-regulatory protein required for cell migration as a new predictor for breast cancer prognosis. Faseb J. 34, 7847–7865. doi:10.1096/fj.201901195RRR
Rivera, B., Moreno, C., Lavanderos, B., Hwang, J. Y., Fernandez-Trillo, J., Park, K. S., et al. (2021). Constitutive phosphorylation as a key regulator of TRPM8 channel function. J. Neurosci. official J. Soc. Neurosci. 41, 8475–8493. doi:10.1523/JNEUROSCI.0345-21.2021
Royle, S. J., and Murrell-Lagnado, R. D. (2003). Constitutive cycling: a general mechanism to regulate cell surface proteins. Bioessays 25, 39–46. doi:10.1002/bies.10200
Runnels, L. W., Yue, L., and Clapham, D. E. (2001). TRP-PLIK, a bifunctional protein with kinase and ion channel activities. Science 291, 1043–1047. doi:10.1126/science.1058519
Ryskamp, D. A., Witkovsky, P., Barabas, P., Huang, W., Koehler, C., Akimov, N. P., et al. (2011). The polymodal ion channel transient receptor potential vanilloid 4 modulates calcium flux, spiking rate, and apoptosis of mouse retinal ganglion cells. J. Neurosci. official J. Soc. Neurosci. 31, 7089–7101. doi:10.1523/JNEUROSCI.0359-11.2011
Saldías, M. P., Maureira, D., Orellana-Serradell, O., Silva, I., Lavanderos, B., Cruz, P., et al. (2021). TRP channels interactome as a novel therapeutic target in breast cancer. Front. Oncol. 11, 621614. doi:10.3389/fonc.2021.621614
Sasase, T., Fatchiyah, F., and Ohta, T. (2022). Transient receptor potential vanilloid (TRPV) channels: basal properties and physiological potential. Gen. Physiol. Biophys. 41, 165–190. doi:10.4149/gpb_2022016
Schattling, B., Steinbach, K., Thies, E., Kruse, M., Menigoz, A., Ufer, F., et al. (2012). TRPM4 cation channel mediates axonal and neuronal degeneration in experimental autoimmune encephalomyelitis and multiple sclerosis. Nat. Med. 18, 1805–1811. doi:10.1038/nm.3015
Schlingmann, K. P., Waldegger, S., Konrad, M., Chubanov, V., and Gudermann, T. (2007). TRPM6 and TRPM7-Gatekeepers of human magnesium metabolism. Biochim. Biophys. Acta 1772, 813–821. doi:10.1016/j.bbadis.2007.03.009
Schlingmann, K. P., Weber, S., Peters, M., Niemann Nejsum, L., Vitzthum, H., Klingel, K., et al. (2002). Hypomagnesemia with secondary hypocalcemia is caused by mutations in TRPM6, a new member of the TRPM gene family. Nat. Genet. 31, 166–170. doi:10.1038/ng889
Schmidt, M., Dubin, A. E., Petrus, M. J., Earley, T. J., and Patapoutian, A. (2009). Nociceptive signals induce trafficking of TRPA1 to the plasma membrane. Neuron 64, 498–509. doi:10.1016/j.neuron.2009.09.030
Schmitz, C., Dorovkov, M. V., Zhao, X., Davenport, B. J., Ryazanov, A. G., and Perraud, A.-L. (2005). The channel kinases TRPM6 and TRPM7 are functionally nonredundant. J. Biol. Chem. 280, 37763–37771. doi:10.1074/jbc.M509175200
Schmitz, C., Perraud, A. L., Johnson, C. O., Inabe, K., Smith, M. K., Penner, R., et al. (2003). Regulation of vertebrate cellular Mg2+ homeostasis by TRPM7. Cell 114, 191–200. doi:10.1016/s0092-8674(03)00556-7
Seebohm, G., and Schreiber, J. A. (2021). Beyond hot and spicy: TRPV channels and their pharmacological modulation. Cell Physiol. Biochem. 55, 108–130. doi:10.33594/000000358
Serafini, N., Dahdah, A., Barbet, G., Demion, M., Attout, T., Gautier, G., et al. (2012). The TRPM4 channel controls monocyte and macrophage, but not neutrophil, function for survival in sepsis. J. Immunol. 189, 3689–3699. doi:10.4049/jimmunol.1102969
Shaham, O., Gueta, K., Mor, E., Oren-Giladi, P., Grinberg, D., Xie, Q., et al. (2013). Pax6 regulates gene expression in the vertebrate lens through miR-204. Plos Genet. 9, e1003357. doi:10.1371/journal.pgen.1003357
Shari, L. W., Fan-Yan, W., and Angus, C. N. (2010). “Chapter 79-the EF2K/MHCK/TRPM7 family of atypical protein kinases,” in Handbook of cell signaling. Editors R. A. Bradshaw, and E. A. Dennis 2nd edn (Elsevier), 587–599.
Sharma, S., and Hopkins, C. R. (2019). Review of transient receptor potential canonical (TRPC5) channel modulators and diseases: miniperspective. J. Med. Chem. 62, 7589–7602. doi:10.1021/acs.jmedchem.8b01954
Shin, S. H., Lee, E. J., Hyun, S., Chun, J., Kim, Y., and Kang, S. S. (2012). Phosphorylation on the Ser 824 residue of TRPV4 prefers to bind with F-actin than with microtubules to expand the cell surface area. Cell Signal 24, 641–651. doi:10.1016/j.cellsig.2011.11.002
Singh, B. B., Lockwich, T. P., Bandyopadhyay, B. C., Liu, X., Bollimuntha, S., Brazer, S. C., et al. (2004). VAMP2-dependent exocytosis regulates plasma membrane insertion of TRPC3 channels and contributes to agonist-stimulated Ca2+ influx. Mol. Cell 15, 635–646. doi:10.1016/j.molcel.2004.07.010
Singh, B. B., Zheng, C., Liu, X., Lockwich, T., Liao, D., Zhu, M. X., et al. (2001). Trp1-dependent enhancement of salivary gland fluid secretion: role of store-operated calcium entry. FASEB J. 15, 1652–1654. doi:10.1096/fj.00-0749fje
Sita, G., Hrelia, P., Graziosi, A., Ravegnini, G., and Morroni, F. (2018). TRPM2 in the brain: role in health and disease. Cells 7, 82. doi:10.3390/cells7070082
Smedlund, K., Bah, M., and Vazquez, G. (2012). On the role of endothelial TRPC3 channels in endothelial dysfunction and cardiovascular disease. Cardiovasc Hematol. Agents Med. Chem. 10, 265–274. doi:10.2174/187152512802651051
Smyth, J. T., Lemonnier, L., Vazquez, G., Bird, G. S., and Putney, J. W. (2006). Dissociation of regulated trafficking of TRPC3 channels to the plasma membrane from their activation by phospholipase C. J. Biol. Chem. 281, 11712–11720. doi:10.1074/jbc.M510541200
Stallmeyer, B., Zumhagen, S., Denjoy, I., Duthoit, G., Hebert, J. L., Ferrer, X., et al. (2012). Mutational spectrum in the Ca(2+)--activated cation channel gene TRPM4 in patients with cardiac conductance disturbances. Hum. Mutat. 33, 109–117. doi:10.1002/humu.21599
Stojilkovic, S. S., Zemkova, H., and Van Goor, F. (2005). Biophysical basis of pituitary cell type-specific Ca2+ signaling-secretion coupling. Trends Endocrinol. Metab. 16, 152–159. doi:10.1016/j.tem.2005.03.003
Story, G. M., Peier, A. M., Reeve, A. J., Eid, S. R., Mosbacher, J., Hricik, T. R., et al. (2003). ANKTM1, a TRP-like channel expressed in nociceptive neurons, is activated by cold temperatures. Cell 112, 819–829. doi:10.1016/s0092-8674(03)00158-2
Su, W., Qiao, X., Wang, W., He, S., Liang, K., and Hong, X. (2023). TRPV3: structure, diseases and modulators. Molecules 28, 774. doi:10.3390/molecules28020774
Sudhof, T. C., and Rothman, J. E. (2009). Membrane fusion: grappling with SNARE and SM proteins. Science 323, 474–477. doi:10.1126/science.1161748
Sulak, M. A., Ghosh, M., Sinharoy, P., Andrei, S. R., and Damron, D. S. (2018). Modulation of TRPA1 channel activity by Cdk5 in sensory neurons. Channels (Austin) 12, 65–75. doi:10.1080/19336950.2018.1424282
Sundivakkam, P. C., Kwiatek, A. M., Sharma, T. T., Minshall, R. D., Malik, A. B., and Tiruppathi, C. (2009). Caveolin-1 scaffold domain interacts with TRPC1 and IP3R3 to regulate Ca2+ store release-induced Ca2+ entry in endothelial cells. Am. J. Physiol. Cell Physiol. 296, C403–C413. doi:10.1152/ajpcell.00470.2008
Suzuki, Y., Chitayat, D., Sawada, H., Deardorff, M. A., McLaughlin, H. M., Begtrup, A., et al. (2018). TRPV6 variants interfere with maternal-fetal calcium transport through the placenta and cause transient neonatal hyperparathyroidism. Am. J. Hum. Genet. 102, 1104–1114. doi:10.1016/j.ajhg.2018.04.006
Syam, N., Chatel, S., Ozhathil, L. C., Sottas, V., Rougier, J. S., Baruteau, A., et al. (2016). Variants of transient receptor potential melastatin member 4 in childhood atrioventricular block. J. Am. Heart Assoc. 5, e001625. doi:10.1161/JAHA.114.001625
Syam, N., Rougier, J. S., and Abriel, H. (2014). Glycosylation of TRPM4 and TRPM5 channels: molecular determinants and functional aspects. Front. Cell. Neurosci. 8, 52. doi:10.3389/fncel.2014.00052
Talbot, B. E., Vandorpe, D. H., Stotter, B. R., Alper, S. L., and Schlondorff, J. S. (2019). Transmembrane insertases and N-glycosylation critically determine synthesis, trafficking, and activity of the nonselective cation channel TRPC6. J. Biol. Chem. 294, 12655–12669. doi:10.1074/jbc.RA119.008299
Tang, Y., Tang, J., Chen, Z., Trost, C., Flockerzi, V., Li, M., et al. (2000). Association of mammalian trp4 and phospholipase C isozymes with a PDZ domain-containing protein, NHERF. J. Biol. Chem. 275, 37559–37564. doi:10.1074/jbc.M006635200
Tano, J. Y., Smedlund, K., and Vazquez, G. (2010). Endothelial TRPC3/6/7 proteins at the edge of cardiovascular disease. Cardiovasc Hematol. Agents Med. Chem. 8, 76–86. doi:10.2174/187152510790796138
Thebault, S., Alexander, R. T., Tiel Groenestege, W. M., Hoenderop, J. G., and Bindels, R. J. (2009). EGF increases TRPM6 activity and surface expression. J. Am. Soc. Nephrol. 20, 78–85. doi:10.1681/ASN.2008030327
Tiapko, O., and Groschner, K. (2018). TRPC3 as a target of novel therapeutic interventions. Cells 7, 83. doi:10.3390/cells7070083
Torihashi, S., Fujimoto, T., Trost, C., and Nakayama, S. (2002). Calcium oscillation linked to pacemaking of interstitial cells of Cajal: requirement of calcium influx and localization of TRP4 in caveolae. J. Biol. Chem. 277, 19191–19197. doi:10.1074/jbc.M201728200
Toro, C. A., Arias, L. A., and Brauchi, S. (2011). Sub-cellular distribution and translocation of TRP channels. Curr. Pharm. Biotechnol. 12, 12–23. doi:10.2174/138920111793937899
Toro, C. A., and Brauchi, S. (2015). Modulating the surface expression of cold receptors. Temp. (Austin). 2, 160–162. doi:10.1080/23328940.2015.1017087
Tóth, B., and Csanády, L. (2012). Pore collapse underlies irreversible inactivation of TRPM2 cation channel currents. Proc. Natl. Acad. Sci. U. S. A. 109, 13440–13445. doi:10.1073/pnas.1204702109
Trebak, M., Lemonnier, L., Smyth, J. T., Vazquez, G., and Putney, J. W. (2007). Phospholipase C-coupled receptors and activation of TRPC channels. Handb. Exp. Pharmacol. 179, 593–614. doi:10.1007/978-3-540-34891-7_35
Tsavaler, L., Shapero, M. H., Morkowski, S., and Laus, R. (2001). Trp-p8, a novel prostate-specific gene, is up-regulated in prostate cancer and other malignancies and shares high homology with transient receptor potential calcium channel proteins. Cancer Res. 61, 3760–3769.
Tsuruda, P. R., Julius, D., and Minor, D. L. (2006). Coiled coils direct assembly of a cold-activated TRP channel. Neuron 51, 201–212. doi:10.1016/j.neuron.2006.06.023
Uchiyama, M., Nakao, A., Kurita, Y., Fukushi, I., Takeda, K., Numata, T., et al. (2020). O2-Dependent protein internalization underlies astrocytic sensing of acute hypoxia by restricting multimodal TRPA1 channel responses. Curr. Biol. 30, 3378–3396. doi:10.1016/j.cub.2020.06.047
Ullrich, N. D., Voets, T., Prenen, J., Vennekens, R., Talavera, K., Droogmans, G., et al. (2005). Comparison of functional properties of the Ca2+-activated cation channels TRPM4 and TRPM5 from mice. Cell Calcium 37, 267–278. doi:10.1016/j.ceca.2004.11.001
van de Graaf, S. F., Hoenderop, J. G., Gkika, D., Lamers, D., Prenen, J., Rescher, U., et al. (2003). Functional expression of the epithelial Ca2+ channels (TRPV5 and TRPV6) requires association of the S100A10-annexin 2 complex. EMBO J. 22, 1478–1487. doi:10.1093/emboj/cdg162
van de Graaf, S. F. J., Rescher, U., Hoenderop, J. G. J., Verkaart, S., Bindels, R. J. M., and Gerke, V. (2008). TRPV5 is internalized via clathrin-dependent endocytosis to enter a Ca2+-controlled recycling pathway. J. Biol. Chem. 283, 4077–4086. doi:10.1074/jbc.M706959200
Van den Eynde, C., De Clercq, K., and Vriens, J. (2021). Transient receptor potential channels in the epithelial-to-mesenchymal transition. Int. J. Mol. Sci. 22, 8188. doi:10.3390/ijms22158188
van Rossum, D. B., Patterson, R. L., Ma, H. T., and Gill, D. L. (2000). Ca2+ entry mediated by store depletion, S-nitrosylation, and TRP3 channels. Comparison of coupling and function. J. Biol. Chem. 275, 28562–28568. doi:10.1074/jbc.M003147200
Vazquez, G., Wedel, B. J., Aziz, O., Trebak, M., and Putney Jr, J. W. (2004a). The mammalian TRPC cation channels. Biochim. Biophys. Acta 1742, 21–36. doi:10.1016/j.bbamcr.2004.08.015
Vazquez, G., Wedel, B. J., Kawasaki, B. T., Bird, G. S., and Putney, J. W. (2004b). Obligatory role of Src kinase in the signaling mechanism for TRPC3 cation channels. J. Biol. Chem. 279, 40521–40528. doi:10.1074/jbc.M405280200
Veliz, L. A., Toro, C. A., Vivar, J. P., Arias, L. A., Villegas, J., Castro, M. A., et al. (2010). Near-membrane dynamics and capture of TRPM8 channels within transient confinement domains. PLoS One 5, e13290. doi:10.1371/journal.pone.0013290
Venkatachalam, K., and Montell, C. (2007). TRP channels. Annu. Rev. Biochem. 76, 387–417. doi:10.1146/annurev.biochem.75.103004.142819
Venkatachalam, K., van Rossum, D. B., Patterson, R. L., Ma, H. T., and Gill, D. L. (2002). The cellular and molecular basis of store-operated calcium entry. Nat. Cell Biol. 4, E263–E272. doi:10.1038/ncb1102-e263
Venkatachalam, K., Zheng, F., and Gill, D. L. (2003). Regulation of canonical transient receptor potential (TRPC) channel function by diacylglycerol and protein kinase C. J. Biol. Chem. 278, 29031–29040. doi:10.1074/jbc.M302751200
Verma, P., Kumar, A., and Goswami, C. (2010). TRPV4-mediated channelopathies. Channels (Austin) 4, 319–328. doi:10.4161/chan.4.4.12905
Vig, M., Peinelt, C., Beck, A., Koomoa, D. L., Rabah, D., Koblan-Huberson, M., et al. (2006). CRACM1 is a plasma membrane protein essential for store-operated Ca2+ entry. Science 312, 1220–1223. doi:10.1126/science.1127883
Visser, D., Middelbeek, J., van Leeuwen, F. N., and Jalink, K. (2014). Function and regulation of the channel-kinase TRPM7 in health and disease. Eur. J. Cell Biol. 93, 455–465. doi:10.1016/j.ejcb.2014.07.001
Vriens, J., Owsianik, G., Hofmann, T., Philipp, S. E., Stab, J., Chen, X. D., et al. (2011). TRPM3 is a nociceptor channel involved in the detection of noxious heat. Neuron 70, 482–494. doi:10.1016/j.neuron.2011.02.051
Wagner, T. F. J., Drews, A., Loch, S., Mohr, F., Philipp, S. E., Lambert, S., et al. (2010). TRPM3 channels provide a regulated influx pathway for zinc in pancreatic beta cells. Pflugers Arch. 460, 755–765. doi:10.1007/s00424-010-0838-9
Walsh, L., Reilly, J. F., Cornwall, C., Gaich, G. A., Gipson, D. S., Heerspink, H. J., et al. (2021). Safety and efficacy of GFB-887, a TRPC5 channel inhibitor, in patients with focal segmental glomerulosclerosis, treatment-resistant minimal change disease, or diabetic nephropathy: TRACTION-2 trial design. Kidney Int. Rep. 6, 2575–2584. doi:10.1016/j.ekir.2021.07.006
Wang, C., Chen, J., Kuang, Y., Cheng, X., Deng, M., Jiang, Z., et al. (2022). A novel methylated cation channel TRPM4 inhibited colorectal cancer metastasis through Ca(2+)/Calpain-mediated proteolysis of FAK and suppression of PI3K/Akt/mTOR signaling pathway. Int. J. Biol. Sci. 18, 5575–5590. doi:10.7150/ijbs.70504
Wang, C., Naruse, K., and Takahashi, K. (2018). Role of the TRPM4 channel in cardiovascular physiology and pathophysiology. Cells 7, 62. doi:10.3390/cells7060062
Wang, J., Liao, Q. J., Zhang, Y., Zhou, H., Luo, C. H., Tang, J., et al. (2014). TRPM7 is required for ovarian cancer cell growth, migration and invasion. Biochem. Biophys. Res. Commun. 454, 547–553. doi:10.1016/j.bbrc.2014.10.118
Wang, L., Li, J., Zhang, J., He, Q., Weng, X., Huang, Y., et al. (2017a). Inhibition of TRPC3 downregulates airway hyperresponsiveness, remodeling of OVA-sensitized mouse. Biochem. Biophys. Res. Commun. 484, 209–217. doi:10.1016/j.bbrc.2016.12.138
Wang, L.-K., Chen, X., Zhang, C.-Q., Liang, C., Wei, Y.-J., Yue, J., et al. (2017b). Elevated expression of TRPC4 in cortical lesions of focal cortical dysplasia II and tuberous sclerosis complex. J. Mol. Neurosci. 62, 222–231. doi:10.1007/s12031-017-0923-z
Wang, S., Dai, Y., Fukuoka, T., Yamanaka, H., Kobayashi, K., Obata, K., et al. (2008). Phospholipase C and protein kinase A mediate bradykinin sensitization of TRPA1: a molecular mechanism of inflammatory pain. Brain 131, 1241–1251. doi:10.1093/brain/awn060
Wang, Y., Fu, X., Gaiser, S., Kottgen, M., Kramer-Zucker, A., Walz, G., et al. (2007). OS-9 regulates the transit and polyubiquitination of TRPV4 in the endoplasmic reticulum. J. Biol. Chem. 282, 36561–36570. doi:10.1074/jbc.M703903200
Watanabe, H., Murakami, M., Ohba, T., Takahashi, Y., and Ito, H. (2008). TRP channel and cardiovascular disease. Pharmacol. Ther. 118, 337–351. doi:10.1016/j.pharmthera.2008.03.008
Wedel, B. J., Vazquez, G., McKay, R. R., Bird, G., St, J., and Putney Jr, J. W. (2003). A calmodulin/inositol 1,4,5-trisphosphate (IP3) receptor-binding region targets TRPC3 to the plasma membrane in a calmodulin/IP3 receptor-independent process. J. Biol. Chem. 278, 25758–25765. doi:10.1074/jbc.M303890200
Wegierski, T., Hill, K., Schaefer, M., and Walz, G. (2006). The HECT ubiquitin ligase AIP4 regulates the cell surface expression of select TRP channels. EMBO J. 25, 5659–5669. doi:10.1038/sj.emboj.7601429
Wegierski, T., Lewandrowski, U., Muller, B., Sickmann, A., and Walz, G. (2009). Tyrosine phosphorylation modulates the activity of TRPV4 in response to defined stimuli. J. Biol. Chem. 284, 2923–2933. doi:10.1074/jbc.M805357200
Weinman, E. J., Steplock, D., Wang, Y., and Shenolikar, S. (1995). Characterization of a protein cofactor that mediates protein kinase A regulation of the renal brush border membrane Na(+)-H+ exchanger. J. Clin. Invest. 95, 2143–2149. doi:10.1172/JCI117903
Wes, P. D., Chevesich, J., Jeromin, A., Rosenberg, C., Stetten, G., and Montell, C. (1995). TRPC1, a human homolog of a Drosophila store-operated channel. Proc. Natl. Acad. Sci. U. S. A. 92, 9652–9656. doi:10.1073/pnas.92.21.9652
Woo, S. K., Kwon, M. S., Ivanov, A., Geng, Z., Gerzanich, V., and Simard, J. M. (2013). Complex N-glycosylation stabilizes surface expression of transient receptor potential melastatin 4b protein. J. Biol. Chem. 288, 36409–36417. doi:10.1074/jbc.M113.530584
Wu, C., Xie, N., Lian, Y., Xu, H., Chen, C., Zheng, Y., et al. (2016b). Central antinociceptive activity of peripherally applied botulinum toxin type A in lab rat model of trigeminal neuralgia. Springerplus 5, 431. doi:10.1186/s40064-016-2071-2
Wu, D., Huang, W., Richardson, P. M., Priestley, J. V., and Liu, M. (2008). TRPC4 in rat dorsal root ganglion neurons is increased after nerve injury and is necessary for neurite outgrowth. J. Biol. Chem. 283, 416–426. doi:10.1074/jbc.M703177200
Wu, M., Zhai, Y. J., Li, Y. X., Hu, Q. Q., Wang, Z. R., Wei, S. P., et al. (2016a). Hydrogen peroxide suppresses TRPM4 trafficking to the apical membrane in mouse cortical collecting duct principal cells. Am. J. Physiol. Ren. Physiol. 311, F1360–F1368. doi:10.1152/ajprenal.00439.2016
Wu, X., Zagranichnaya, T. K., Gurda, G. T., Eves, E. M., and Villereal, M. L. (2004). A TRPC1/TRPC3-mediated increase in store-operated calcium entry is required for differentiation of H19-7 hippocampal neuronal cells. J. Biol. Chem. 279, 43392–43402. doi:10.1074/jbc.M408959200
Xia, M., Liu, D., and Yao, C. (2015). TRPC3: a new target for therapeutic strategies in chronic pain-DAG-mediated activation of non-selective cation currents and chronic pain (mol pain 2014;10:43). J. Neurogastroenterol. Motil. 21, 445–447. doi:10.5056/jnm15078
Xie, J., An, S. W., Jin, X., Gui, Y., and Huang, C. L. (2020). Munc13 mediates klotho-inhibitable diacylglycerol-stimulated exocytotic insertion of pre-docked TRPC6 vesicles. PLoS One 15, e0229799. doi:10.1371/journal.pone.0229799
Xie, M. X., Cao, X. Y., Zeng, W. A., Lai, R. C., Guo, L., Wang, J. C., et al. (2021). ATF4 selectively regulates heat nociception and contributes to kinesin-mediated TRPM3 trafficking. Nat. Commun. 12, 1401. doi:10.1038/s41467-021-21731-1
Xu, H., Fu, Y., Tian, W., and Cohen, D. M. (2006). Glycosylation of the osmoresponsive transient receptor potential channel TRPV4 on Asn-651 influences membrane trafficking. Am. J. Physiol. Ren. Physiol. 290, 1103–1109. doi:10.1152/ajprenal.00245.2005
Xu, X. Z., Moebius, F., Gill, D. L., and Montell, C. (2001). Regulation of melastatin, a TRP-related protein, through interaction with a cytoplasmic isoform. Proc. Natl. Acad. Sci. U. S. A. 98, 10692–10697. doi:10.1073/pnas.191360198
Xu, Y., Dhingra, A., Fina, M. E., Koike, C., Furukawa, T., and Vardi, N. (2012). mGluR6 deletion renders the TRPM1 channel in retina inactive. J. Neurophysiol. 107, 948–957. doi:10.1152/jn.00933.2011
Yamaguchi, S., Tanimoto, A., Iwasa, S., and Otsuguro, K. (2019). TRPM4 and TRPM5 channels share crucial amino acid residues for Ca2+ sensitivity but not significance of PI(4,5)P2. Int. J. Mol. Sci. 20, 2012. doi:10.3390/ijms20082012
Yamamoto, S., Ishii, T., Mikami, R., Numata, T., and Shimizu, S. (2019). Short TRPM2 prevents the targeting of full-length TRPM2 to the surface transmembrane by hijacking to ER associated degradation. Biochem. Biophys. Res. Commun. 520, 520–525. doi:10.1016/j.bbrc.2019.10.065
Yamamoto, S., Shimizu, S., Kiyonaka, S., Takahashi, N., Wajima, T., Hara, Y., et al. (2008). TRPM2-mediated Ca2+ influx induces chemokine production in monocytes that aggravates inflammatory neutrophil infiltration. Nat. Med. 14, 738–747. doi:10.1038/nm1758
Yamashita, S., Mizumoto, H., Sawada, H., Suzuki, Y., and Hata, D. (2019). TRPV6 gene mutation in a dizygous twin with transient neonatal hyperparathyroidism. J. Endocr. Soc. 3, 602–606. doi:10.1210/js.2018-00374
Yang, H., Mergler, S., Sun, X., Wang, Z., Lu, L., Bonanno, J. A., et al. (2005). TRPC4 knockdown suppresses epidermal growth factor-induced store-operated channel activation and growth in human corneal epithelial cells. J. Biol. Chem. 280, 32230–32237. doi:10.1074/jbc.M504553200
Yang, L.-P., Jiang, F.-J., Wu, G.-S., Deng, K., Wen, M., Zhou, X., et al. (2015). Acute treatment with a novel TRPC4/C5 channel inhibitor produces antidepressant and anxiolytic-like effects in mice. PloS one 10, e0136255. doi:10.1371/journal.pone.0136255
Yang, T. J., Yu, Y., Yang, J. Y., Li, J. J., Zhu, J. Y., Vieira, J. A. C., et al. (2022). Involvement of transient receptor potential channels in ocular diseases: a narrative review. Ann. Transl. Med. 10, 839. doi:10.21037/atm-21-6145
Yu, M., Ledeboer, M. W., Daniels, M., Malojcic, G., Tibbitts, T. T., Coeffet-Le Gal, M., et al. (2019). Discovery of a potent and selective TRPC5 inhibitor, efficacious in a focal segmental glomerulosclerosis model. ACS Med. Chem. Lett. 10, 1579–1585. doi:10.1021/acsmedchemlett.9b00430
Yu, W., Hill, W. G., Apodaca, G., and Zeidel, M. L. (2011). Expression and distribution of transient receptor potential (TRP) channels in bladder epithelium. Am. J. Physiol. Ren. Physiol. 300, 49–59. doi:10.1152/ajprenal.00349.2010
Yu, Y., Chen, S., Xiao, C., Jia, Y., Guo, J., Jiang, J., et al. (2014). TRPM7 is involved in angiotensin II induced cardiac fibrosis development by mediating calcium and magnesium influx. Cell Calcium 55, 252–260. doi:10.1016/j.ceca.2014.02.019
Yuan, J. P., Kiselyov, K., Shin, D. M., Chen, J., Shcheynikov, N., Kang, S. H., et al. (2003). Homer binds TRPC family channels and is required for gating of TRPC1 by IP3 receptors. Cell. 114, 777–789. doi:10.1016/s0092-8674(03)00716-5
Zhang, M., Ma, Y., Ye, X., Zhang, N., Pan, L., and Wang, B. (2023). TRP (transient receptor potential) ion channel family: structures, biological functions and therapeutic interventions for diseases. Signal Transduct. Target Ther. 8, 261. doi:10.1038/s41392-023-01464-x
Zhang, W., Na, T., and Peng, J. B. (2008). WNK3 positively regulates epithelial calcium channels TRPV5 and TRPV6 via a kinase-dependent pathway. Am. J. Physiol. Ren. Physiol. 295, 1472–1484. doi:10.1152/ajprenal.90229.2008
Zhang, Y., Hoon, M. A., Chandrashekar, J., Mueller, K. L., Cook, B., Wu, D., et al. (2003). Coding of sweet, bitter, and umami tastes: different receptor cells sharing similar signaling pathways. Cell 112, 293–301. doi:10.1016/s0092-8674(03)00071-0
Zhang, Z., Tang, J., Tikunova, S., Johnson, J. D., Chen, Z., Qin, N., et al. (2001). Activation of Trp3 by inositol 1,4,5-trisphosphate receptors through displacement of inhibitory calmodulin from a common binding domain. Proc. Natl. Acad. Sci. U. S. A. 98, 3168–3173. doi:10.1073/pnas.051632698
Zhao, P. Y., Gan, G., Peng, S., Wang, S. B., Chen, B., Adelman, R. A., et al. (2015). TRP channels localize to subdomains of the apical plasma membrane in human fetal retinal pigment epithelium. Invest. Ophthalmol. Vis. Sci. 56, 1916–1923. doi:10.1167/iovs.14-15738
Zhou, Y., Castonguay, P., Sidhom, E.-H., Clark, A. R., Dvela-Levitt, M., Kim, S., et al. (2017). A small-molecule inhibitor of TRPC5 ion channels suppresses progressive kidney disease in animal models. Science 358, 1332–1336. doi:10.1126/science.aal4178
Zhou, Y. F., Bennett, T. M., and Shiels, A. (2021). Mutation of the TRPM3 cation channel underlies progressive cataract development and lens calcification associated with pro-fibrotic and immune cell responses. Faseb J. 35, e21288. doi:10.1096/fj.202002037R
Zielinska, W., Zabrzynski, J., Gagat, M., and Grzanka, A. (2021). The role of TRPM2 in endothelial function and dysfunction. Int. J. Mol. Sci. 22, 7635. doi:10.3390/ijms22147635
Keywords: TRP channels, trafficking, post-translational modifications, protein-protein interaction (PPI), ion channel mutations
Citation: Rivera B, Orellana-Serradell O, Servili E, Santos R, Brauchi S and Cerda O (2024) The odyssey of the TR(i)P journey to the cellular membrane. Front. Cell Dev. Biol. 12:1414935. doi: 10.3389/fcell.2024.1414935
Received: 09 April 2024; Accepted: 31 May 2024;
Published: 23 July 2024.
Edited by:
Elaine L. Bearer, University of New Mexico Health Sciences Center, United StatesReviewed by:
Suvrajit Saha, California Life Company (Calico), United StatesIlias Gkikas, Foundation for Research and Technology Hellas (FORTH), Greece
Copyright © 2024 Rivera, Orellana-Serradell, Servili, Santos, Brauchi and Cerda. This is an open-access article distributed under the terms of the Creative Commons Attribution License (CC BY). The use, distribution or reproduction in other forums is permitted, provided the original author(s) and the copyright owner(s) are credited and that the original publication in this journal is cited, in accordance with accepted academic practice. No use, distribution or reproduction is permitted which does not comply with these terms.
*Correspondence: Sebastián Brauchi, c2JyYXVjaGlAdWFjaC5jbA==; Oscar Cerda, b3NjYXJjZXJkYUB1Y2hpbGUuY2w=
†These authors have contributed equally to this work