- 1Department of Cell Biology, Naval Medical University, Shanghai, China
- 2Experimental Teaching Center, Naval Medical University, Shanghai, China
- 3Shanghai Key Laboratory of Cell Engineering, Naval Medical University, Shanghai, China
Hepatocyte transplantation (HTx) has been a novel cell-based therapy for severe liver diseases, as the donor livers for orthotopic liver transplantation are of great shortage. However, HTx has been confronted with two main hurdles: limited high-quality hepatocyte sources and low cell engraftment and repopulation rate. To cope with, researchers have investigated on various strategies, including small molecule drugs with unique advantages. Small molecules are promising chemical tools to modulate cell fate and function for generating high quality hepatocyte sources. In addition, endothelial barrier, immune responses, and low proliferative efficiency of donor hepatocytes mainly contributes to low cell engraftment and repopulation rate. Interfering these biological processes with small molecules is beneficial for improving cell engraftment and repopulation. In this review, we will discuss the applications and advances of small molecules in modulating cell differentiation and reprogramming for hepatocyte resources and in improving cell engraftment and repopulation as well as its underlying mechanisms.
Highlights
1. Small molecule modulation of signaling pathways (e.g., Wnt, TGF-beta, HGF) and epigenetic profiles can promote the differentiation of stem cells into hepatocyte-like cells or direct reprogramming of fibroblasts into hepatocytes.
2. Small molecule can maintain hepatocyte function and hepatic progenitor cells self-renewal and expansion.
3. Small molecules can improve hepatocyte engraftment by disrupting endothelial integrity, preventing microcirculatory obstruction, and inhibiting instant blood-mediated inflammatory reaction.
4. Small molecules can establish proliferation advantage of transplanted hepatocytes to enhance liver repopulation.
1 Introduction
Huge amounts of patients are being inflicted on liver diseases in the world. Orthotopic liver transplantation (OLT) is the standard treatment for end-stage liver diseases, acute liver failure (ALF), and inherited metabolic liver diseases (Lucey et al., 2023), but many patients die waiting due to the donor scarcity. Hepatocyte transplantation (HTx) is a promising alternative as it can directly restore liver function or bridge patients to OLT (Nulty et al., 2024; Peng et al., 2021). Advantages over OLT include less invasiveness, wider donor sources, repeat injections, and modifiability. Originally proposed in 1976 as a new treatment for Crigler-Najjar syndrome type 1 and validated in hyperbilirubinemic Gunn rat models, HTx has undergone substantial advances over time (Matas et al., 1976). Carrying this work into clinical application, the first human HTx procedure was performed in 1992 utilizing autologous hepatocytes for individuals with cirrhotic liver (Mito et al., 1992). Over the last 3 decades, more than 150 clinical trials of HTx have been reported and manifested efficacy (Gramignoli et al., 2015). Clinical indications for HTx include liver-based metabolic diseases, ALF, and chronic liver failure. In liver-based metabolic diseases, HTx initially improves biochemical indicators and clinical symptoms, but sustained responses are limited, with orthotopic liver transplantation (OLT) typically required after 9–12 months (Nguyen et al., 2020). For instance, Meyburg J et al. reported 4–13 months of metabolic stabilization in four children with urea cycle disorder following primary human hepatocyte (PHH) transplantation. (Meyburg et al., 2009). HTx has shown efficacy in ALF, albeit with variable outcomes. In a clinical trial of seven patients with acute-on-chronic liver failure, three fully recovered, one survived and later underwent OLT, and three died within 2.5–12 months post-transplantation (Wang et al., 2014). Notably, transplanted hepatocytes were detected by MRI in the spleen of two long-term survivors at 48 months post-transplantation (Wang et al., 2014). A recent trial using alginate-coated human hepatocyte microbeads in eight children with ALF demonstrated full recovery in four patients and successful bridging to OLT in three (Dhawan et al., 2020). For chronic liver failure, a phase I-II matched case-control trial of intrasplenic HTx using fetal hepatocytes showed improvements in Child-Pugh scores and encephalopathy compared to the control group, with stable clinical scores and absence of encephalopathy at 1-year follow-up (Pietrosi et al., 2015).
Despite its promising potential, limited high-quality donor hepatocytes and poor engraftment and repopulation hinder large-scale clinical use. PHHs have been widely used in clinical trials which easily lose functions during cell isolation, culture, and cryopreservation (Sun et al., 2023). Hepatocyte-like cells (HLCs) induced from stem cells by growth factors, small molecules, and/or transcription factor transduction are less functional than PHHs(Gao et al., 2020). Low cell engraftment is common in HTx due to instant blood-mediated inflammatory reaction (IBMIR) and chronic immune rejection (reviewed by Sun et al.). The cell engraftment was only 0.1%–0.3% in mice receiving 1 cell infusion of 3%–5% of the total recipient liver cells (Wang et al., 2002). Lack of proliferation and quantity of transplanted hepatocytes contributes to poor liver repopulation.
To improve hepatocyte engraftment, preconditioning strategies including irradiation and partial hepatectomy have been established but chemical intervention provide a less invasive approach. Small molecules are widely used tools in stem cell research and manipulating biology through protein interactions (Li et al., 2013; Wang et al., 2023). Compared to genetic techniques, small molecules are convenient to use, concentration-dependent, rapidly reversible, and spatially controlled. This review discusses mechanisms and applications of small molecules in generating high-quality hepatocytes via differentiation and reprogramming and in improving hepatocyte engraftment and repopulation (Figure 1).
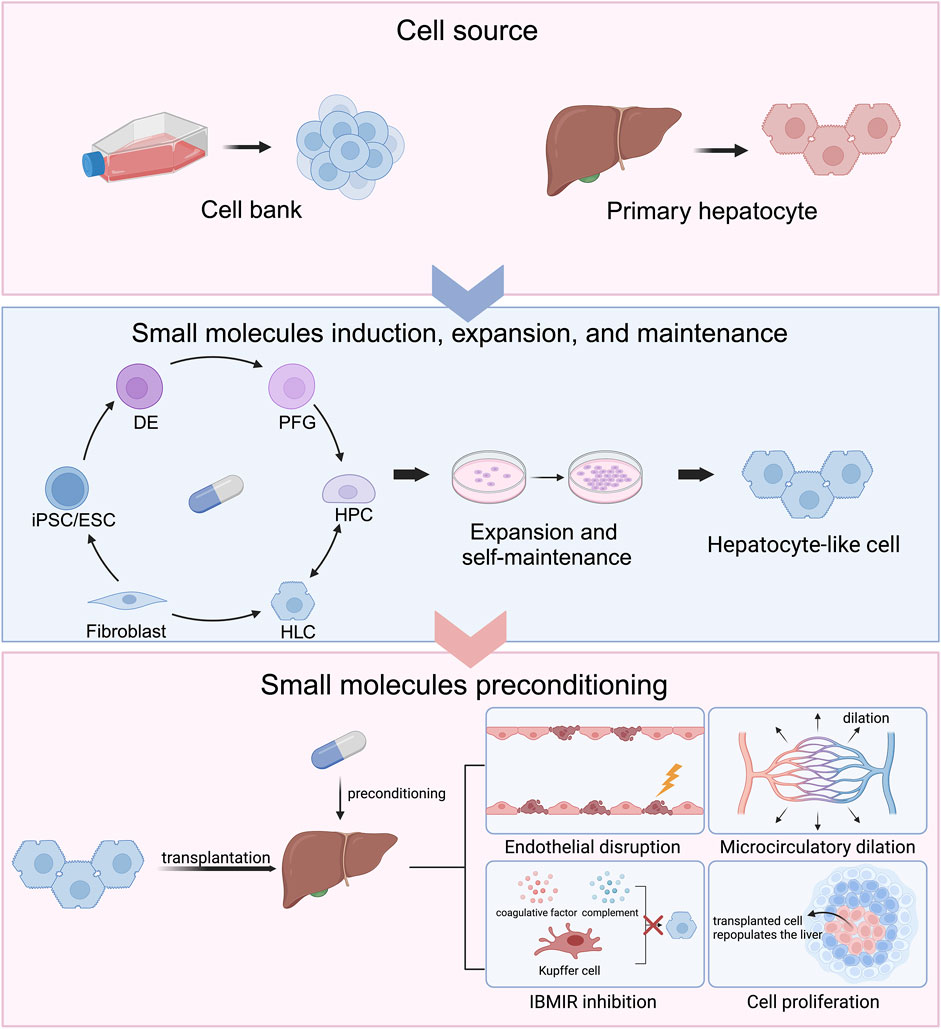
Figure 1. Chemical approaches to generate hepatocytes for cell transplantation and improve cell engraftment and repopulation.
Chemical approaches are applied in modulating cell identity in cell differentiation and reprogramming. Primary human hepatocytes (PHHs) are currently used in clinical hepatocyte transplantation (HTx) whereas their loss of function affects HTx efficiency. Small molecule cocktails are established to alleviate the loss of function and promote hepatocyte proliferation. Chemically induced hepatocyte-like cells (HLCs) are another hepatocyte source for HTx. induced pluripotent stem cells (iPSCs) or embryonic stem cells (ESCs) can be sequentially differentiated into definite endoderm (DE), posterior foregut (PFG), hepatic progenitor cells (HPCs), and HLCs under small molecule induction. Hepatocytes can convert to HPCs and thereby expand. HPCs can remain their ability of proliferation and self-maintenance and act as hepatocyte pool by small molecule modulations. Fibroblasts are able to be directly reprogrammed into HLCs under chemical modulation. The host liver can be preconditioned by small molecules to create amicable hepatic microenvironments, including endothelial disruption, microcirculatory dilation, instant blood-mediated inflammatory reaction (IBMIR) inhibition, and cell proliferation, which favor the engraftment and repopulation of the transplanted hepatocytes. Created in BioRender. Yind, K. (2024) BioRender.com/e30g211.
2 Signaling pathways and transcription factors in liver development
The manipulation of hepatocyte fate by small molecules relies on the understanding and recapitulation of liver development. In this section, we will elaborate on the role of signaling pathways and transcription factors in liver development. Under the activation of TGFβ signaling by Nodal/Activin A and canonical Wnt/β-catenin signaling, the primitive steak specifies into three germ layers, endoderm, mesoderm, and ectoderm (Camacho-Aguilar et al., 2024; Hayes et al., 2021). Combination of low concentrations of bone morphogenic protein (BMP), high concentrations of Nodal/Activin A, and Wnt/β-catenin signaling generates definitive endoderm (DE) (Pour et al., 2022). Wnt/β-catenin signaling promotes DE specification by induction of NODAL gene expression (Wild et al., 2020). As embryonic development proceeds, DE patterns into foregut, midgut, and hindgut. The liver and pancreas arise from posterior foregut (PFG) (Lotto et al., 2023). At mouse embryonic day (E) 8.0, a proportion of cells in ventral foregut proximal to mesodermal cells adopt a hepatoblast (HB) fate, being bipotent for hepatocytes and cholangiocytes (Lotto et al., 2023). HB specification is tuned by fibroblast growth factors (FGFs) and BMPs, secreted by cardiac mesoderm and septum transversum, respectively, while pancreatic fate is activated by TGFβ signaling (Lee et al., 2021). The hepatic signaling pathways inhibit pancreatic fate commitment and vice versa (Lotto et al., 2023). Additionally, retinoic acid and Wnt/β-catenin inhibition drive liver bud formation (Perugorria et al., 2019; Lotto et al., 2023). Proliferating HBs expand the liver bud based on cues from HGF, FGF, Wnt signaling, and Hippo signaling (Macchi and Sadler, 2020). Near E13.5, HBs commit to hepatocytes or cholangiocytes depending on periportal Notch and TGFβ signalings, which favor cholangiocytes differentiation whereas prevent hepatocyte differentiation (Yang et al., 2019). On contrary, Hippo signaling drive hepatocyte differentiation. Inhibition of Hippo signaling promotes cholangiocytes by increasing TGFβ signaling (Russell and Camargo, 2022). Together, temporally and spatially regulated signaling action patterns the hepatic lineage in a multi-step developmental process.
The concerted network of signaling pathways induces downstream transcription factors, including FOXA1-3, GATA4/6, TBX3, HEX, PROX1, HNF4α, HNF1α/β and C/EBPα/β, promoting hepatic differentiation (Tachmatzidi et al., 2021). The role of these transcription factors is evidenced by several studies which directly converted fibroblasts into hepatocytes by transducing specific combination of these transcription factors in fibroblasts (Garcia-Llorens et al., 2023; Rombaut et al., 2021). FoxA and Gata family are the ‘pioneer factors’ during liver bud formation. Prior to liver bud formation, FOXA1/2 and GATA4/6 bind to liver-specific genes and prime the compacted chromatin allowing other proteins to bind to their target genes (Tachmatzidi et al., 2021; Macchi and Sadler, 2020; Lee et al., 2005). Specifically, FOXA proteins bind to nucleosome and upregulate the levels of histone three lysine 4 (H3K4) me1 by recruiting lysine-specific demethylase 1 (LSD1) (Tachmatzidi et al., 2021). During HBs specification, the pioneer factors recruit EZH2, a histone methyltransferase (KMTs) that catalyzes histone three lysine 27 (H3K27) me3, to silent pancreatic-specific genes in HBs (Dumasia and Pethe, 2020). Different from FOXA1/2, FOXA3 expression delays to E10.5 and remains high level throughout liver development (Tachmatzidi et al., 2021), which exerts unique functions in liver-specific gene activation by interacting and travelling with RNA polymerase II from the distal to proximal regions of transcription start sites (Horisawa et al., 2020). HNF1β and HNF4α also play a crucial role in hepatic differentiation. HNF1β cooperates with GATA6 to upregulate HNF4α expression (Lau et al., 2018), which recruits RNA polymerase II to hepatic genes during liver bud formation (DeLaForest et al., 2018). By adulthood, HNF1β expression is substituted by HNF1α interacting with HNF4α (Tachmatzidi et al., 2021), which maintains hepatocyte identity and metabolic functions together with FOXA, TBX3, and CEBPα (Kotulkar et al., 2023).
Together, the liver development requires complicated networks of signaling pathways and transcription factors. The special and temporal regulation of Wnt/β-catenin, TGFβ, FGF, BMP, and HGF as well as the liver-specific transcription factors including FOXA and HNFs are crucial in liver development. Hepatic differentiation and reprogramming needs initiation and reactivation of these mediators, respectively.
3 Small molecule induction yields cell sources for HTx
A main hurdle of HTx is the limited availability of high-quality cells for transplantation. Attention has turned to using stem cell-derived hepatocytes since pluripotent stem cells (PSCs) and mesenchymal stem cells sources are abundant. Methods using combinations of signaling modulators have been developed to derive specific lineages by mimicking embryonic development. This review focuses on signaling pathways and epigenetic changes involved in hepatic differentiation and reprogramming and their chemical modulations (Figure 1).
3.1 Signaling modulation for hepatic differentiation
By mimicking the development signaling, stepwise chemical treatment induces differentiation of human embryonic stem cells (hESCs), induced PSCs (iPSCs), and human PSCs into hepatocytes. The target and mechanism of small molecules are summarized in Table 1. GSK3β inhibitor CHIR99021 (CHIR) activated Wnt/β-catenin to induce DE from hESCs combined with Nodal signaling activator activin A in RPMI + B27 medium (Raggi et al., 2022). Another GSK3β inhibitor BIO was also used to activate Wnt/β-catenin pathways (Tasnim et al., 2015; Onai, 2019). Activin A is able to be substituted with IDE1, an activator of Nodal signaling (Luo et al., 2021). The combination of IDE1, CHIR, and LY294002 (a PI3K inhibitor) induced DE specification from human umbilical cord-derived mesenchymal stem cells (Luo et al., 2021). However, this combination induced mesoderm formation in human PSCs probably due to the excessive level of Wnt signaling favoring mesoderm formation (Pan et al., 2022). To cope with, PD0332991, a CDK4/6 inhibitor was added to the combination of IDE1 and CHIR, resulting in more specific DE differentiation (Pan et al., 2022). To promote DE differentiation to PFG, Touboul T et al. treated hESCs with Wnt/β-catenin inhibitor IWR-1 together with activin A and noggin, resulting in induction of PFG gene expression (Touboul et al., 2016), although noggin was later found to impede with the promoting effect of IWR-1 on PFG induction. For further differentiation into HBs, a combined protocol of BMP4, IWR-1, and TGFβ inhibitor SB431542 eliciting hepatic markers, including fetoprotein (AFP), albumin (ALB), and prospero homeobox 1 (PROX1) was established (Touboul et al., 2016). Du, C et al. combined A83-01, a TGFβ signaling inhibitor, with two epigenetic modulators sodium butyrate (NaB) and dimethyl sulfoxide (DMSO) to induce hepatic differentiation from DE (Du et al., 2018). Finally, Touboul T et al. showed that CHIR treatment generated proliferative HBs with co-expression of HNF6 and PROX1, although activation of Wnt/β-catenin pathways theoretically promote biliary differentiation (Touboul et al., 2016).
Hepatocyte growth factor (HGF) and its receptor c-Met regulates hepatic maturation during liver development. In developing livers, the expression of HGF and c-Met upregulated between 4 and 21 days after birth (Hu et al., 1993). In fetal hepatocytes, treatment of HGF together with glucocorticoids promoted the expression of ALB (Kamiya et al., 2001), suggesting the effect of HGF on hepatic maturation. N-hexanoic-Tyr-Ile-(6) aminohexanoic amide (Dihexa), a small-molecule angiotensin IV analog, was found to efficiently activate HGF/c-Met pathway (Benoist et al., 2014). Treatment of Dihexa and glucocorticoids successfully induced functional HLCs from hepatic progenitor cells (HPCs)(Siller et al., 2015; Asumda et al., 2018). In addition, HGF can be replaced by other small molecules. Shan, J et al. identified two chemical compounds functional hits 1 (FH1) and functional proliferation hit 1 (FPH1) through high-throughput screen (Shan et al., 2013). Later, Du, C et al. established a protocol including FH1, FPH1, A83-01, and glucocorticoids, which yielded functional HLCs more efficiently compared to growth factor groups (Du et al., 2018). Luo S et al. combined FH1 with growth factors to induce hepatocyte differentiation from HPCs. FH1 induced hepatocytes with higher efficiency and similar maturity compared to HGF induced ones (Luo et al., 2021).
Recently, the hepatic differentiation of current good manufacturing practice (cGMP) compliant human iPSC (hiPSC) and hESC lines have been validated. The protocol successfully generated hepatocytes from cGMP cell lines using small molecules combined with growth factors in 21 days (Blackford et al., 2019). The ALB expression was detected but insignificant in the induced hepatocytes, suggesting that the hepatocytes was immature (Blackford et al., 2019). In comparison, the pure chemical protocol from Du et al. produced hepatocytes from hESCs within 13 days. The ALB positive hepatocytes account for 67.7% versus 37.1% in the growth factor group (Du et al., 2018). The hepatic differentiation using growth factor-free small molecule cocktails should also be validated in cGMP compliant hiPSC and hESC lines for future clinical application. Moreover, the combination and dosage of small molecules vary between research groups. Comparison studies are needed to evaluate the differentiation efficiency of protocols and to conclude a cGMP compliant protocol.
3.2 Small molecules for direct reprogramming to hepatic lineage
Recently, studies have shown that somatic cells can be directly reprogrammed into specific cell lineage, including hepatocytes. Direct reprogramming without entering pluripotent cell state shortens the time for cell differentiation and lower the risks of tumorigenesis, which is deemed as a promising method to yield hepatocytes for HTx.
Early in 2011, Sekiya et al. established three combinations comprising two transcription factors: HNF4α with FOXA1, FOXA2 or FOXA3, which successfully converted mouse embryonic fibroblasts (MEFs) into induced hepatocyte-like cells (iHeps) (Sekiya and Suzuki, 2011). However, the conversion efficiency of the protocol was insufficient (only 0.3%) (Sekiya and Suzuki, 2011). Hui’s group discovered a specific combination of three transcription factors consisting of GATA4, HNF1α and FOXA3, which converted p19Arf−/− mouse tail-tip fibroblasts to iHeps with a higher conversion efficiency (Huang et al., 2011; Ji et al., 2013). The researchers suggested that Hnf1a and Foxa3 transduction was already sufficient to induce iHeps and addition of Gata4 enhanced the conversion efficiency (Ji et al., 2013). Later, Hui et al. showed that transduction of Hnf1β and Foxa3 directly induced MEFs into hepatic stem cells which had the bipotential of differentiating into hepatocytes and cholangiocytes (Yu et al., 2013).
The clinical application of iHep generated by viral transduction in HTx have been limited due to the safe uncertainty, genetic instability and tumorigenesis risk. Therefore, studies about chemical induction of hepatocytes from fibroblasts have thrived in the field (Tables 1, 2).
Guo, R et al. showed that combination of a single transcription factor FOXA1, FOXA2, or FOXA3 with six compound cocktails, including CHIR, RepSox, valproic acid (VPA), Parnate, TTNPB, Dznep (termed CRVPTD), directly induced MEFs into iHeps (Guo et al., 2017). Researchers postulated that FOXAs upregulated liver-specific genes independent of any specific signaling pathway and thus was difficult to replace. Horisawa, K et al. demonstrated that liver-specific gene expression was promoted in MEFs by sequential and cooperative binding of FOXAs and HNF-4α to chromatin. FOXA3 exert unique regulations on the gene expressions among FOXA proteins, that is, by binding to and co-transversing the target genes with RNA polymerase II, which is indispensable for reprogramming MEFs to hepatocytes (Horisawa et al., 2020).
Later studies developed protocols independent of ectopic expression for direct reprogramming. Li, X et al. established a seven-compound cocktail (VPA [V], TD114-2 [T], E−616452 [6], tranylcypromine/Parnate [P], forskolin [F], AM580 [A], and EPZ004777 [E]) to regulate reprogramming-related signaling pathways (T6FA) and to modulate the epigenetic profile (VPE) (Li et al., 2017a). The small-molecule cocktail induced extra-embryonic endoderm (XEN)-like cells from fibroblasts bypassing the pluripotent state, which could differentiate towards hepatocytes (Li et al., 2017b). A later study elucidated that compounds CHIR, E−616452, and forskolin firstly worked in a cooperative manner to activate Sox7; CHIR/forskolin and E−616452 then activated Gata4 and Sall4 expression, respectively. The consecutive activation of the crucial transcription factors attributed to the conversion of MEF to XEN-like cells (Yang et al., 2020). Bai, Y et al. established a two-stage chemical cocktail to directly reprogram MEFs to iHeps (Bai et al., 2023), modified from the cocktails (C6FAE and C6F5UE) presented in Yang’s work (Yang et al., 2020). The researchers found that addition of vitamin C in the second stage was the optimal protocol to induce iHeps, with the iHep subpopulation of 15% in total cells (Bai et al., 2023). Principal component analysis and RNA-seq showed that the iHeps resembled PHHs (Bai et al., 2023). Finally, the iHeps was capable of engrafting the liver in Fah−/− mice and improving liver functions in vivo in a similar manner to PHHs (Bai et al., 2023). Noteworthy, Zhong Z et al. directly reprogramed MEFs into iHeps with one step chemical induction consisting of SB431542, CHIR99021, BIX01294 (G9a KMT inhibitor), LDN193189, and DAPT (Zhong et al., 2024). During 12-day induction, no pluripotency gene expression (Oct4, Sox2, and Nanog), immature HB markers, and HPC markers were detected, suggesting that the conversion of MEFs to iHeps bypassed the intermediate stem cell stage (Zhong et al., 2024). Mechanistically, the chemical cocktail suppressed SNAI1 expression, which induced mesenchymal to epithelial transition and HNF4α expression, thereby promoting iHep generation (Zhong et al., 2024).
Hui’s group reported that the induction of iHep generated by transcription factor cost 14 days and the induction efficiency was around 23% (Huang et al., 2011). In comparison, in studies using chemical approaches to produce iHep, the induction time was 20, 12, 12 days and the efficiency was over 20%, 15%, and 80%, respectively (Li et al., 2017a; Bai et al., 2023; Zhong et al., 2024). Collectively, the chemical approach inducing iHep from fibroblasts has a similar and even more robust induction efficiency to genetic approach, making it a promising method to produce hepatocytes from MEFs.
3.3 Epigenetic modulation for differentiation and reprogramming
Epigenetic modulations are important mechanisms of transcriptional regulation during normal development. During differentiation from PFG towards HBs, histone acetylation significantly increased at the liver regulatory elements mediated by histone acetyltransferase P300 (Xu et al., 2011). Moreover, H3K27 KMTs enriched at Pdx1 gene (a pancreatic gene) upstream which suppressed the pancreatic development while favored hepatic development (Xu et al., 2011). The methylation of CpG islands is regulated by DNA methyltransferases (DNMTs) and mediate gene expressions by mediating promoters (Wilkinson et al., 2023). Based on the mechanisms, histone deacetylase (HDAC) inhibitors and DNA/histone methylation mediators are used to promote hepatic differentiation. It was reported that combination of NaB with DMSO elicited hepatic differentiation from DE with comparable hepatic marker expression (AFP and HNF4α) to the growth factor-treated group (Tasnim et al., 2015). Demethylation of CpG by DNMT inhibitors allowed liver-specific gene expression, including AFP and ALB (Snykers et al., 2009). It was reported that combination of nanaomycin A, a selective DNMT3B inhibitor, with FGF4 and BMP4 promoted the differentiation from DE to HPCs (Nakamae et al., 2018).
Epigenetic modulation is also required in cell reprogramming. In differentiated cells, high levels of DNA methylation occurred in the CpG-rich promoters of pluripotency-related genes (Baral et al., 2022; Ghazimoradi and Farivar, 2020). Therefore, inhibition of DNMTs promotes demethylation of CpG islands and thereby removes the epigenetic barrier of cell reprogramming. It was demonstrated that the combination of RG108 (a DNMT inhibitor) and with BIX01294 promoted the reprogramming of MEFs to iPSC upon Oct4 and Klf4 transduction (Shi et al., 2008).
Histone acetylation/deacetylation is mediated by histone acetyltransferases and HDACs, respectively. Histone acetylation is related to open chromatin structure and transcriptional activation (Baral et al., 2022). HDAC inhibitors, including VPA and NaB, were shown to facilitate cell reprogramming in several studies (Aguirre-Vázquez et al., 2021; Duan et al., 2019; Mali et al., 2010). Histone lysine methylation is tuned by KMTs and histone lysine demethylases. The effect of histone lysine methylation on gene transcription varies, either activating or inhibitory. LSD1 specifically catalyzes the demethylation of H3K4/9me1/2 (Dai et al., 2021). Enhancer of zeste homolog 2 (EZH2) and DOT1L catalyzes methylation of H3K27 and histone three lysine 79 (H3K79), respectively (Wu et al., 2023; Lee et al., 2022). Small molecule inhibitor of LSD1 (Parnate), EZH2 (DZNep), and DOT1L (EPZ004777) promoted somatic reprogramming efficiency (Martinez-Gamero et al., 2021; Liuyang et al., 2023).
Based on the aforementioned mechanisms, researchers have applied small molecules to modulate epigenetic profiles in somatic cells and thus to promote somatic reprogramming into hepatocytes bypassing the iPSC stage (Table 1 and 2). Zhu, S et al. demonstrated that combination of NaB, Parnate, and RG108 with a Wnt signaling activator significantly reprogrammed MEFs into multipotent progenitor cells with transient expression of three transcription factors (OCT4, SOX2 and KLF4) (Zhu et al., 2014). Multipotent progenitor cells differentiated towards EPCs and hepatocytes sequentially in the hepatocyte induction medium (Zhu et al., 2014). Guo R et al. used VPA, Parnate, TTNPB, and Dznep combining with other small molecules to induce direct reprogramming from MEFs to iHeps with only a single factor FOXA3 (Guo et al., 2017). Li, X et al. developed a 7-compound protocol consisting of VPA, EPZ004777, Parnate and other small molecules which directly induced the XEN-like cells from MEFs (Li et al., 2017b). Zhong Z et al. combined BIX01294 with other signaling modulators to directly converted MEFs to iHep (Zhong et al., 2024).
3.4 Small molecule modulation of cell expansion and maturation
HPCs can be yielded either from livers or iPSCs and thus becomes one of accessible cell sources for HTx. However, the limited ability of ex vivo proliferation impedes with its application. During liver development, Wnt signaling promotes the proliferation, expansion, and differentiation of HPCs (Perugorria et al., 2019). Hedgehog signaling and BMP4 facilitates the proliferation of fetal HPCs in vitro (Hirose et al., 2009; Wang et al., 2015), while TGFβ signaling remarkably inhibits HPC colony formation in vitiro (Clark et al., 2005). Notch signaling activation promotes HPC differentiation towards cholangiocytes (Duan et al., 2018). These data suggested that fine modulation of these critical signalings might contribute to HPC expansion.
Our lab established a protocol consisting of EGF, CHIR, E−616452 and two bioactive lipids lysophosphatidic acid and sphingosine 1-phosphate, hereafter termed as ECELS. Mouse HBs maintained self-renewal in the Matrigel-coated media containing ECELS and bovine serum albumin (Lv et al., 2015). These expandable HBs could differentiated to mature hepatocytes by chemical induction (Lv et al., 2015). Pan, T et al. established a chemical cocktail of CHIR, A8301, and SAG (a Hedgehog signaling activator) combined with EGF, HGF and BMP4, which significantly promoted the expansion and stemness maintenance of HPCs (Pan et al., 2019). Later, researchers replaced EGF, HGF, and BMP4 with forskolin, Dihexa, and vitamin C, respectively. This chemical cocktail enabled the long-term self-renewal with efficient proliferation rate for at least 20 passages and the capability of differentiating into hepatocyte and cholangiocytes (Pan et al., 2021).
Mature hepatocytes converted to liver progenitor-like cells and re-differentiated into hepatocytes in response to chronic periportal liver injuries in vivo (Li et al., 2020). The liver progenitor-like cells generated duct-like cells when liver damage persisted (Li et al., 2020). Yan et al. established a transition and expansion medium which consisted of EGF, HGF, CHIR, lipids lysophosphatidic acid, sphingosine 1-phosphate, A83-01, and Y-27632. This protocol elicited significant transition of hepatocytes to duct-like cells and promoted the proliferation of duct-like cells (Wu et al., 2017). Miyoshi T et al. converted PHHs harvested from cirrhotic livers to liver progenitor cells by a protocol including Y-27632, A83-01, and CHIR (YAC). The induced liver progenitor cells differentiated into mature hepatocyte under induction of YAC, oncostatin M, and dexamethasone (Miyoshi et al., 2022).
The quick loss of functions in isolated hepatocytes is one of the main hurdles in hepatocyte-based cell therapy. Therefore, it is urgent to investigate on the underlying mechanism and discover corresponding strategies. Our lab demonstrated that mechanical tension represented by actin remodulation elicited hepatocyte dedifferentiation towards HPCs through Yap activation. Yap deletion in isolated hepatocytes resulted in maintenance of ALB and HNF4α expression (Sun et al., 2019). Based on the iterative chemical screening, we found that combination of an actin polymerization inhibitor Latrunculin B and actomyosin contraction inhibitor Blebbistatin led to increased levels of ALB and HNF4α. Addition of Dasatinib, XAV939, and LY294002 (Yap mediators) further facilitated hepatic genes expression (Sun et al., 2019). This chemical cocktail (termed LBDXL) was able to maintain the hepatic gene expression and metabolic functions of hepatocytes cultured in Matrigel-coated medium for up to 3 weeks (Sun et al., 2019).
The activation of epithelial to mesenchymal transition in hepatocytes results in loss of normal hepatic functions and regenerative capacity, where TGF-β signaling pathway play an important role (Xue et al., 2013). Xiang C et al. confirmed that the expression of components of TGF-β signaling pathway significantly upregulated in cultured PHHs. The researchers established a 5-compound (5C) protocol, consisting of SB431542, forskolin, DAPT (Notch inhibitor), IWP2 (Wnt inhibitor), and LDN193189 (BMP inhibitor) to suppress the expression of epithelial to mesenchymal transition marker genes (Xiang et al., 2019). 5C-cultured PHHs still supported hepatitis B virus infection after 4-week culture (Xiang et al., 2019). Additionally, Chen Y et al. developed a small-molecule cocktail consisting of SB431542, acetylcysteine (ROS inhibitor), CHIR, and Y-27632 (SACY)(Chen et al., 2021). Compared to 5C culture system, SACY exhibited higher level of ALB and α1 antitrypsin production and urea synthesis (Chen et al., 2021).
Loss of functions in hepatocytes was also observed in vivo, specifically in chronically injured liver. Lin P et al. showed that damaged hepatocytes harvested from CCl4-induced mice liver regained hepatocyte phenotypes and functions with treatment of five compounds (forskolin, CID755673, GSK429286A, ETC-1002, and phenylpropanoid glycoside) (Lin et al., 2023). Injection of revitalized hepatocytes promoted liver regeneration in CCl4-induced liver injury (Lin et al., 2023).
4 Small molecules improve hepatocyte engraftment and repopulation
Low cell engraftment and repopulation rate is another hurdle of HTx. To improve hepatocyte engraftment, preconditioning strategies including irradiation and partial hepatectomy have been established but chemical intervention provide a less invasive approach, which are reviewed in the following section. The potential mechanisms and clinical trials of small molecules improving poor cell engraftment and repopulation in hepatocyte transplantation were shown in Figure 2; Table 2.
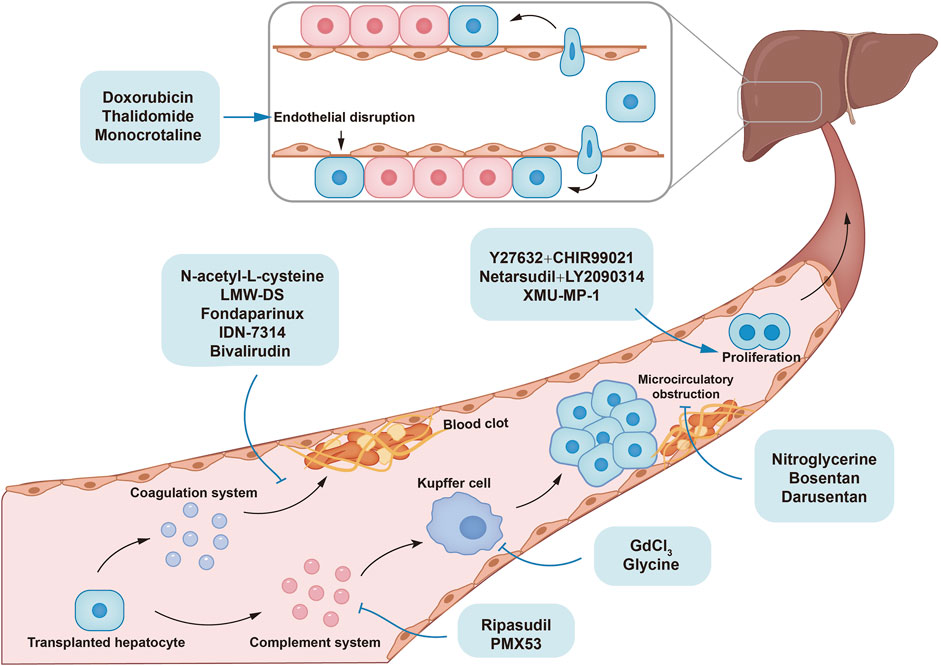
Figure 2. The mechanisms of small molecules improving poor cell engraftment and repopulation in hepatocyte transplantation.
The transplanted hepatocytes are cleared by instant blood-mediated inflammatory reaction (IBMIR) in the early period after HTx. Coagulation system, complement system, and innate immune cells are important components involved in IBMIR. Microcirculatory obstruction also participates in IBMIR by activating Kupffer cells. Advantageous proliferation of transplanted cells improves liver repopulation. N-acetyl-L-cysteine (NAC), low-molecular-weight dextran sulfate (LMW-DS), fondaparinux, and bivalirudin inhibit the coagulation system, while PMX53, ripasudil, gadolinium chloride (GdCl3), and glycine inhibit innate immune system, thereby alleviating hepatocyte clearance. Nitroglycerine, bosentan, and darusentan mitigate microcirculatory obstruction by dilating hepatic sinusoid. XMU-MP-1 enhanced cell proliferation by targeting Hippo pathway. Combination of Y27632 and CHIR99021, or netarsudil and LY2090314 improved liver repopulation in vivo by promoting the dediffereantiation and redifferentiation of hepatocytes.
4.1 Disruption of endothelial integrity in liver
Gupta et al. observed disrupted sinusoidal endothelium structure at 24 h post-HTx, suggesting endothelial disruption permits transplanted cell entrance into liver parenchyma (Gupta et al., 1999b; Gupta et al., 1999a). Therefore, facilitating sinusoidal endothelium disruption can enhance engraftment efficiency by promoting the entrance of hepatocytes into liver plates (Figure 2).
Small-molecule drugs including monocrotaline, doxorubicin, rifampicin, and phenytoin increased cell engraftment versus controls by causing hepatic endothelial damage (Joseph et al., 2006; Kim et al., 2005; Wu et al., 2008). Thalidomide has the anti-inflammatory properties via selective inhibitory activity of tumour necrosis factor-α (TNFα) and the anti-angiogenesis effect via downregulating VEGF levels. (Amare et al., 2021; Barbarossa et al., 2022). Viswanathan et al. found that Thalidomide was able to induce transient upregulation of serum hyaluronic acid levels versus control, suggesting the rapid endothelium damage (Viswanathan et al., 2016). Thalidomide pretreatment markedly upregulated transplanted cell numbers in liver versus non-treatment groups (Viswanathan et al., 2016), also improving engraftment via immune regulation discussed later. It is speculated that the effect of Thalidomide on hepatic endothelial damage is related to its anti-angiogenesis property. Thalidomide inhibited proliferation of human umbilical vein endothelial cells in vitro probably via downregulation of transcription factor SP1 (Moreira et al., 1999). Another study demonstrated that Thalidomide suppressed VEGF production by human peritoneal mesothelial cells and thereby inhibited endothelial tube formation via STAT3/SP4 signaling (Zhu et al., 2021). These data offer a mechanistic insight of how Thalidomide disrupt hepatic endothelial integrity, although further studies are needed.
In conclusion, using small molecules to induce endothelial disruption benefits cell engraftment. Mechanistically, endothelial disruption increases sinusoid permeability, allowing hepatocytes to pass the endothelial barrier and enter liver parenchyma.
4.2 Prevention of hepatic microcirculatory obstruction
Slehria et al. observed sinusoidal blood flow cessation in liver after HTx, persisting over 3 h and ameliorating by 24 h (Slehria et al., 2002). Researchers suggested that the overall sinusoidal perfusion declined from 94% to 84% on day 1% and to 76% on day 7 after HTx (Wilhelm et al., 2004), suggesting transplanted hepatocytes cause immediate and long-term disruption to sinusoidal blood flow and microcirculation.
Hepatic microcirculatory obstruction affects transplanted cell distribution. Cells entering sinusoids and integrating into liver plates avoid immune clearance, while those remaining in portal areas confront clearance (Gupta et al., 1999b; Koenig et al., 2005). Rajvanshi P et al. suggested hepatocyte location relates to portal vein radicles size. The intrasplenically-transplanted hepatocytes were largely located in periportal area (zone 1) in mice, while in downstream midlobular (zone 2) or perivenous (zone 3) areas in larger animals (Rajvanshi et al., 1996). Indeed, occlusion is more likely in smaller vessels versus larger ones, hindering sinusoidal distribution while increasing portal areas exposure, leading to immune clearance of transplanted hepatocytes.
Hepatic microcirculatory obstruction causes ischemia-related cell injury in the liver. After HTx, transient ischemia in portal areas leads to an immediate sinusoidal blood flow cessation (Wilhelm et al., 2004; Slehria et al., 2002). Wilhelm A et al. observed markedly increased Kupffer cells (KCs) on day 1 post-HTx (Wilhelm et al., 2004), which release ROS and proinflammatory cytokines including TNF-α, IL-1, IFN-γ (Peralta et al., 2013). ROS induces transplanted cell injury by inducing mitochondria dysfunction, protein synthesis disruption and cell membrane damage (Bardallo et al., 2022). The proinflammatory cytokines promote neutrophil and peripheral macrophage infiltration, which further enhance the immune reaction. Indeed, Gupta S et al. observed the infiltration of OX-43 antibody-positive granulocytes, phagocytes, and activated macrophages in portal areas (Gupta et al., 1999b). Overall, ischemia activates the immune response, leading to transplanted cell injury.
This evidence suggests that hepatic microcirculatory obstruction interferes with cell distribution and triggers immune responses. Thus, ameliorating the hepatic microcirculation with vascular dilators is a promising method to improve transplanted hepatocyte engraftment.
Nitroglycerine relaxes vascular smooth muscle cells and cause vascular dilation by increasing NO levels and activating guanylate cyclase. Slehria S et al. first reported that nitroglycerine treatment in rats significantly reduced periportal blood flow perturbation after HTx and increased transplanted hepatocytes in the liver and specifically in zone 2 at 2h after HTx but not long-term (Slehria et al., 2002), showing sinusoid dilatation contributed to hepatocyte distribution but not translocation. Other mechanisms may play a role in hepatocyte entry into liver plates. In another study, nitroglycerine significantly decreased KC activation and endothelial injury (Bahde et al., 2013), both of which are crucial in preventing ischemia-reperfusion injury. Bosentan (BOS), an unselective endothelin receptor blocker, increased desmin + hepatic stellate cells (HSCs) numbers and VEGF release of HSCs in rat (Bahde et al., 2013), potentially aiding cell entry via increasing hepatic sinusoid permeability. Moreover, conditioned medium of BOS-treated HSCs containing BOS and VEGF prevented TNF-α- or H2O2-induced cytotoxicity against hepatocytes (Bahde et al., 2013). BOS-incubated cells engrafted 2-fold higher than controls while systemic treatment of BOS in recipient rats did not (Bahde et al., 2013). A selective endothelin-1 receptor A blocker, Darusentan (DAR), was effective in dilating hepatic sinusoids, which allowed greater entry of hepatocytes and thus enhanced cell engraftment. Moreover, DAR reduced endothelial injury, KC activation and hepatic ischemia (Bahde et al., 2014). Contrary to BOS, DAR failed to prevent cytotoxicity and negatively affect hepatocyte proliferation in vitro (Bahde et al., 2014), suggesting that DAR favors systemic use. Noteworthy, the different effect of BOS and DAR on hepatocytes and host indicates the endothelin receptor A or B signaling pathway exert distinct biological functions in the liver, whereas the underlying mechanism warrants further investigations.
4.3 Inhibition of instant blood-mediated inflammatory reaction (IBMIR)
IBMIR was first described in islet transplantation which cause instant islet loss (van der Windt et al., 2007; Mou et al., 2022; Yan et al., 2022), involving interactions between platelet aggregation, coagulation system, complement system, and neutrophil/macrophage infiltration (Kale and Rogers, 2023). Hepatocyte-induced IBMIR occurs both in vitro and patients receiving HTx and is a dominant factor contributing to early cell loss of HTx (Lee et al., 2016).
Tissue factor (TF) is the initiating factor of coagulation extrinsic pathway, which expressed on cell membrane of mice and human hepatocytes (Kopec and Luyendyk, 2014). In the tubing loop system model, infusion of 5 × 105 hepatocytes into the whole-blood significantly increased D-dimer levels and decreased platelet counts (Stéphenne et al., 2007). The increase of D-dimer levels was also observed in a Crigler-Najjar patient after the first infusion of hepatocytes (Stéphenne et al., 2007). The changes were prevented by anti-TF monoclonal antibody, suggesting the procoagulant activity of hepatocytes depended on TF (Stéphenne et al., 2007).
Complement system can be directly activated by hepatocytes through antibody recognition, lack of membrane-bound regulators, and extracellular matrix proteins exposure (Nilsson et al., 2014), and indirectly by the crosstalk with coagulation system. For example, fXa and thrombin can convert C3 into C3a (Dzik, 2019). TF-induced thrombin cleaves C5 at a novel R947 site and forms new fragments, C5T and C5bT (Krisinger et al., 2012). Reciprocally, complement proteins interact with coagulation system. C5a can elicit significant increase in TF expression of endothelial cells (Dzik, 2019). Activation of coagulation and complement contributes to the infiltration of neutrophils, monocytes and macrophages, resulting in hepatocyte loss.
Collectively, TF-initiated coagulation activates the IBMIR with interactions between coagulation, complement system, and immune response promote IBMIR process. Interventions with each step with small molecules can attenuate IBMIR and enhance cell engraftment (Figure 2).
4.3.1 Inhibition of coagulation system
N-acetyl-L-cysteine (NAC), used in treating paracetamol overdose as an antioxidant, decreased coagulation factor activity and delayed prothrombin time (PT) in vitro (Knudsen et al., 2005; Jang et al., 2013; Pizon et al., 2011). Although the mechanism of anti-coagulation property of NAC is elusive, some studies offer a possible mechanistic insight. In a study, NAC markedly reversed the procoagulant activity of monocyte-derived microvesicles induced by high glucose levels via inhibiting p38/MAPK signaling (Li et al., 2017a). In HTx, Stéphenne X et al. reported that NAC impaired the TF-dependent procoagulant activity of hepatocytes in a dose-dependent manner, preventing platelet depletion and D-dimer increase (Stéphenne et al., 2007). Moreover, under hypoxia environment inevitable in HTx due to cell occlusion, NAC treatment increased the Bcl-2/Bax ratio and vital hepatocytes (Heil et al., 2018). Reactive oxygen species (ROS) are produced during hypoxia environment, NAC, as an ROS inhibitor, mitigated oxidative stress and downregulated apoptotic factors during hepatic ischemia-reperfusion injury (IRI) (Singh et al., 2024). With its dual functions promoting engraftment by interfering coagulation and enhancing viability, NAC shows promise as a small molecule for clinical HTx application.
Low-molecular-weight dextran sulfate (LMW-DS), a type of glycosaminoglycans, has been reported to inhibit coagulation and complement system. LMW-DS inhibited fXIa and C1s by potentiating C1 inhibitor (Wuillemin et al., 1997; Mauron et al., 1998; Wuillemin et al., 1996) and prevented the deposition of C3 and C4 (Wuillemin et al., 1997). LMW-DS impaired fXa activity and prolonged PT, activated partial thromboplastin time, and thrombin time in human plasma (Drozd et al., 2017). Based on these, LMW-DS has been used in inhibition of IBMIR in animal models and patients of islet transplantation (von Zur-Mühlen et al., 2019; Naziruddin et al., 2014; Kourtzelis et al., 2015), showing similar efficacy and safety with heparin (von Zur-Mühlen et al., 2019). Noteworthy, Gustafson EK et al. reported that LMW-DS remarkably prevented hepatocyte-induced IBMIR, with a maintenance of platelet count, decrease of thrombin-antithrombin complex (TAT), and inhibition of complement system (Gustafson et al., 2011; Gustafson et al., 2017). Mechanistically, LMW-DS inhibited the fibrin-induced activation of contact activation pathway and the lectin complement pathway (Gustafson et al., 2017). Compared to heparin, LMW-DS more effectively inhibited coagulation and complement cascades (Gustafson et al., 2017). Therefore, LMW-DS is a promising agent against hepatocyte-induced IBMIR.
Small molecule inhibitors of coagulative factors have also been investigated to prevent IBMIR in cell transplantation. Fondaparinux (antithrombin activator) and the direct thrombin inhibitor drugs including hirudin and bivalirudin significantly inhibited procoagulant activity of hepatocytes in vitro (Stephenne et al., 2012). The effect of thrombin inhibitor on thrombo-inflammation has also been studied in hepatic IRI. Dabigatran treatment upregulated the thrombomodulin levels and reduced damage-associated molecular high-mobility group box-1 release from injured cells triggering inflammation (Noguchi et al., 2021). Through this mechanism, sinusoidal endothelial cells are protected from hypoxia-reoxygenation damage, indirectly preventing hepatocyte injury via paracrine effects of sinusoidal endothelial cells (Noguchi et al., 2021). In addition, dabigatran reduced the neutrophil infiltration and pro-inflammatory cytokines (Noguchi et al., 2021). As hypoxia-reoxygenation damage occurs in HTx due to cell occlusion, thrombin inhibition may hinder coagulation activation and inflammatory reaction triggered by hypoxia-reoxygenation damage in HTx.
Hepatocyte apoptosis is an important feature of acute and chronic liver diseases. Kopec AK et al. found that Fas-induced apoptosis increased the TF activity of mouse hepatocytes by upregulating caspase 3. Pretreating hepatocytes with IDN-7314, a pan-caspase inhibitor, impaired the TF activity and inhibit the coagulation activation in vitro and in vivo (Kopec et al., 2018). Although it is unclear whether the endogenous TF in liver microenvironment of recipients promotes IBMIR, a new sight into the relationship between coagulation and apoptosis is given for further studies.
4.3.2 Inhibition of the innate immune system
The innate immune system, including complement system, cytokines, and innate immune cells, functions downstream of coagulation system and determines transplanted hepatocytes viability. Around 70% of hepatocytes were lost early after transplantation due to neutrophil and macrophage/monocyte infiltration elicited by complement activation and cytokine release (Gupta et al., 1999b). Therefore, small molecules targeting these aspects are effective in improving hepatocyte engraftment.
Complement system initializes innate immune system activation and shares a crosstalk with coagulation system. Direct inhibition of complement system hinders the activation of innate immune system and thus enhances cell engraftment. Kusakabe J et al. reported that PMX53, a C5a receptor 1 (C5aR-1) antagonist, mitigated hepatic IRI through inhibiting pro-inflammatory cytokine release and neutrophil/macrophage infiltration and platelet aggregation (Kusakabe et al., 2020), suggesting the crosstalk between coagulation and complement system. The C5aR inhibitor may also exert effect in hepatocyte induced IBMIR due to similar process with IRI. Noteworthy, our lab demonstrated that a clinically-used ROCK inhibitor, ripasudil, significantly enhanced hepatocyte engraftment and liver repopulation by blocking membrane attack complex (MAC) formation in vitro/vivo and thereby inhibiting KCs functions (Ma et al., 2023). In isolated hepatocytes, the endocytosis of cell membrane protein, including CD59a, was observed (Ma et al., 2023). Mechanistically, ripasudil safeguarded membrane localization of CD59a, a MAC inhibitor highly expressed on hepatocytes, hindering the MAC formation (Ma et al., 2023). In addition, ripasudil improved liver repopulation (3.96% versus 0.52%) and increased alanine aminotransferase and aspartate aminotransferase levels at 4 weeks post-transplantation, suggesting that ripasudil exerted long-term effect on engrafted hepatocytes and liver function. Therefore, ROCK inhibitor ripasudil is a promising chemical to inhibit complement activation and thus promote hepatocyte engraftment.
Innate immune cells are the most important scavengers responsible for hepatocyte clearance in the short period after HTx. Thus, investigators have developed various strategies to inhibit innate immune cells for improving hepatocyte engraftment. Gadolinium chloride (GdCl3), a lanthanide compound, depletes KCs irreversibly and has been widely used in research. Joseph B et al. indicated that depleting KCs with GdCl3 increased the number of transplanted hepatocytes in short and long-term after HTx, resulting in significantly improved liver repopulation (Joseph et al., 2002). However, GdCl3 permanently destroys KCs by blocking K-type calcium channels (Pałasz and Czekaj, 2000), thus unsuitable for clinical use. Transcription factor GATA-1 competed with c-Jun for the β3/β4 region of PU.1 protein, thereby preferring erythropoiesis and suppressing myelopoiesis (Raghav and Gangenahalli, 2021). Activation of GATA-1 by small molecules may decrease Kupffer cell number in the liver and improve hepatocyte engraftment. Glycine, a nonessential amino acid, has also been used to inhibit KC function in liver without decreasing KC numbers (Rentsch et al., 2005). After human adipose-derived stem cells transplantation, the number of KCs and TNFα release markedly increased (Al-Saeedi et al., 2019). Glycine treatment did not decrease KC numbers but significantly downregulated TNFα levels, resulting in improved cell engraftment in liver (Al-Saeedi et al., 2019).
In addition to direct KC inhibitors, several small molecules mentioned above impaired KC fuctions and enhanced cell engraftment. Darusentan treatment significantly declined KC numbers likely by downregulating macrophage chemokines Ccl2 and Cx3cL (Bahde et al., 2014). Thalidomide decreased KC-associated inflammatory cytokines/chemokines expression and infiltrated KCs (Viswanathan et al., 2016), probably via inhibiting NF-kB signaling and TNFα (Mercurio et al., 2017). In hepatic IRI model, neutrophil infiltration and TNF-α release caused by IRI was impaired by dabigatran (Noguchi et al., 2021). Thrombin, also known as factor II, upregulates proinflammatory cytokine release and promotes leukocyte infiltration (Esmon, 2014). Therefore, inhibition of thrombin by dabigatran may impair the IRI-induced inflammatory response. The dual or multiple anti-IBMIR functions of these small molecules indicate close crosstalk between coagulation, ischemia, and inflammation. Simultaneous targeting of these processes by single molecule is the future direction of improving cell engraftment.
4.4 Promote the proliferation of transplanted cells
In Fah−/− mice, donor hepatocytes have a proliferative advantage for liver repopulation since host cell proliferation is impaired. (Overturf et al., 1996). Our lab demonstrated that host hepatocytes in Fah−/− mice liver experienced senescence characterized by cell cycle arrest, which favored the proliferation of donor hepatocytes (Chen et al., 2019). Senescence disrupts cell connections and degrades extracellular matrix, providing engraftment space (Chen et al., 2019). Among patients with Crigler–Najjar disease where the proliferation of host hepatocytes is impaired, transplanted hepatocytes successfully alleviated the liver functions (Jorns et al., 2012). Moreover, in α1-antitrypsin deficiency mice models with inhibited host hepatocytes proliferation, wild-type donor hepatocytes replaced 20%–98% of host hepatocytes (Ding et al., 2011). However, in most inherited metabolic disorders host hepatocytes viability is not significantly compromised (Barahman et al., 2019a). The proliferation advantages can be achieved by: (1) inhibit host cells proliferation and (2) promote transplanted cells proliferation. For the former one, several precondition strategies including hepatic irradiation, portal embolization, and partial hepatectomy are used in clinical trials (Nguyen et al., 2020). However, these methods are invasive to patients’ body. Using small molecules to intervene with host and donor hepatocytes viability is an ideal, noninvasive, and controllable method.
The Hippo pathway, critical for liver regeneration, comprised of MST1/2 phosphorylating and activating the LATS1/2, which then phosphorylates and inactivates YAP and TAZ. Deletion of MST1/2 or LATS1/2 genes increased nuclear level of YAP/TAZ and expression of downstream genes (Driskill and Pan, 2021). Fan F et al. identified a MST1/2 inhibitor, XMU-MP-1, effectively suppressed MST1/2 kinase and thereby activated downstream YAP in various cells. In Fah−/−Rag2−/−IL2rg−/−(FRG) mice treated with XMU-MP-1, transplanted hepatocyte proliferation was remarkably promoted, improving liver repopulation and ALB levels (Fan et al., 2016).
HGF/c-Met signaling pathway plays an important role in liver regeneration. HGF binds to its specific receptor c-Met, activating downstream pathways and leading to cell proliferation (Zhao et al., 2022). It was reported that the c-Met receptor agonist antibody 5D5 induced significant proliferation in hiPSC-derived hepatocyte-like cells (hiPSC-HLCs) in vitro (Yuan et al., 2019). In FRG-SCID mice, 5D5 remarkably promoted liver repopulation rate of hiPSC-HLCs(Yuan et al., 2019). Small molecule agonists targeting c-Met receptor warrant further development.
Jiang M et al. observed that the HPC and cell cycle genes significantly increased from day 4 after HTx in Fah−/− mice, peaked at day 30 and then decreased to normal. The labeled hepatocytes expanded at day 30 and almost repopulated the liver at D120 (Jiang et al., 2023). These results suggested that the transplanted hepatocytes dedifferentiate to an HPC stage to proliferate and then re-differentiate to hepatocyte after repopulation. Combination of ROCK inhibitor Y27632 and CHIR99021 (termed YC) markedly increased the expression of HPC and cell cycle genes in vivo and thereby promoted hepatocytes proliferation in treated mice (Jiang et al., 2023). Clinically used small molecule drugs of ROCK signaling Netarsudil (N) and Wnt signaling LY2090314 (L) were also tested. Similar to YC, NL treatment also promoted the dediffereantiation and redifferentiation of hepatocytes and favored the liver repopulation in vivo (Jiang et al., 2023).
5 Conclusion and future perspectives
Hepatocyte transplantation (HTx) provides an alternative way of liver transplantation for patients with irreversible liver diseases. Translation of HTx from lab to bedside is of great emergency due to the scarcity of donor livers. However, the wide application of HTx is hindered by two main problems: shortage of high-quality donor hepatocytes and low cell engraftment and repopulation rate.
5.1 Cell source for HTx
Chemical induction of hepatic fate from pluripotent stem cells (PSCs) can provide an unlimited hepatocyte source. The insights into the signaling pathway and transcription factor network of liver development provide cues for yielding hepatocyte-like cells (HLCs) from PSCs by chemical modulation. During embryonic liver development, bone morphogenic protein (BMP), Wnt/β-catenin and Notching signaling promote definitive endoderm (DE) formation; fibroblast growth factors (FGFs) and BMPs promote hepatoblasts (HBs) specification from DE; Notch and TGFβ signaling inhibition and Hippo signaling activation drive HBs into hepatocyte fate. Downstream of signaling pathways, transcription factors including FOXAs, GATA4/6, HNF4α, and HNF1 interact with each other and activate liver-specific gene expression, accompanied by epigenetic changes. For instance, pioneer factors recruit the histone acetyltransferase p300 to deposit H3K9ac/14ac at liver-specific gene regulatory elements, directing hepatic programs (Xu et al., 2011). To recapitulate liver development, the differentiation of PCSs comprises three main steps: ‘PSCs to DE’, ‘DE to hepatic progenitor cells (HPCs)’, and ‘HPCs to HLCs’. Stepwise chemical approaches derive HLCs by regulating these developmental paradigms and epigenetic landscapes. Additionally, reopening liver genes with small molecules enables direct fibroblast reprogramming (Rombaut et al., 2021; Park et al., 2019; Bai et al., 2023).
A comparative study showed that the chemically induced hepatocytes resemble growth-factor derived counterparts (Gao et al., 2020). The expression of liver drug-metabolizing enzymes, transporters, and nuclear receptors in primary hepatocytes are significantly higher than that in HLCs (Gao et al., 2020).
Despite the progress made in chemical induction of hepatocyte, it is noteworthy to mention that consensus on optimal small molecule combinations, doses and hepatocyte quality is lacking, hindering protocol standardization and clinical translation. Future work establishing current good manufacturing practice (cGMP)-grade protocols and improving functional stability is needed for transplantation applications. Comparing differential gene expression between primary and induced hepatocytes may identify targets to enhance cell competence for transplantation.
5.2 Strategies to improve low cell engraftment
In general, there are two strategies to improve low cell engraftment: prime the host hepatic microenvironment for HTx (hepatic irradiation and partial hepatectomy) and prime the donor hepatocytes (alginate encapsulation) (Nguyen et al., 2020). Hepatic irradiation (HIR) has manifested its efficiency in animal preclinical studies and clinical trials (Soltys et al., 2017; Barahman et al., 2019b). However, the efficiency depends on irradiation parameters and host radiosensitivity. It is noteworthy that HIR has hepatoxicity and may cause side effects in patients. Therefore, combination of HIR and systemic small molecules including anti-IBMIR and chemotherapeutic drugs may reduce dosage of both radiation and small molecules, lowering the risks of adverse drug reactions. Recent studies have shown that hepatocyte microbeads encapsuled by alginate are protected from immune rejection and improve cell engraftment (Jitraruch et al., 2014). A recent clinical trial has validated its efficiency and safety in pediatric patients with ALF (Dhawan et al., 2020). However, other problems involved in HTx such as microcirculatory obstruction cannot be tackled with alginate encapsulation. It is interesting to envisage that load alginate encapsulation with small molecules can improve cell engraftment.
Challenges to chemical approaches still remains to be solved before wide applications, including drug specificity, target accuracy, pharmacokinetic properties, and safety. For instance, CHIR99021, a GSK3β inhibitor that activates Wnt/β-catenin signaling, is widely used in regulating cell differentiation, reprogramming, and proliferation. However, Wnt/β-catenin signaling activation promotes the development of liver cancer and the resistance to immunotherapy (Zhou et al., 2022; He and Tang, 2020). Hippo pathway play critical role in liver physiology and tumorigenesis. Depletion of MST1/2 drives the formation of hepatocellular carcinoma and/or cholangiocarcinoma (Driskill and Pan, 2021). Whether in vivo administration of Wnt/β-catenin (CHIR990221) and Hippo signaling activators (XMU-MP-1) will lead to tumorigenesis or affect tumor development in chronic liver failure recipients with hepatocellular carcinoma needs to be studied. TGFβ signaling, ROCK signaling, and others are ubiquitous and regulates numerous critical physiologic processes in healthy cells. How to minimize the systemic effect while maximize the hepatic effect of in vivo administration of small molecules is an important problem before the large-scale clinical use. Developing liver-specific or hepatocyte-specific drug delivery system is one of the feasible strategies, including nanoparticle-based and small molecule-based drug delivery systems (Unagolla et al., 2024; Sharma et al., 2021). Off-target effects are another challenge of clinical application of small molecules. In vitro screening is insufficient to mimic the in vivo complicated microenvironment. Therefore, small molecules may cause off-target effects in vivo despite its in vitro efficacy. Target prediction models based on deep learning are prosperous strategies to mitigate off-target effects.
Despite the obstacles, some small molecules mentioned in this review have already applied clinically, whose application in HTx can be quickly translated due to its confirmative safety and efficiency in human (Table 3). For instance, ripasudil has already been licensed for treating ocular hypertension and open-angle glaucoma, which has potential for rapid translation as a therapeutic to promote hepatocyte engraftment (Table 3). It is noteworthy that systemic adverse drug reactions need further investigation if ripasudil is used systemically, as it rarely enters systemic circulation through the blood-ocular barrier when administered as eye drops in current clinical trials (Kusuhara and Nakamura, 2020). The indication and clinical trials of other small molecules in this review was accessible in Table 1 and 2. In the future, studies comparing various chemical protocols of hepatocyte differentiation and reprogramming are urgently needed in order to standardize a paradigm protocol for potential clinical trials. Additionally, studies on simultaneous interventions of each process during hepatocyte engraftment are warranted, because the reason of low cell engraftment is multifactorial. We believe that the in-depth probes into stem cell biology and progress in chemical screenings and deliver systems will promote the application of HTx by ingenious chemical formula in the future.
Author contributions
HS: Writing–original draft. YD: Writing–original draft. PS: Writing–original draft. ZL: Writing–review and editing. CnW: Writing–review and editing. HM: Writing–review and editing. JL: Writing–review and editing. BY: Methodology, Writing–review and editing. WL: Conceptualization, Writing–review and editing. CoW: Funding acquisition, Supervision, Writing–review and editing.
Funding
The author(s) declare that financial support was received for the research, authorship, and/or publication of this article. This study was supported by the China National Natural Science Foundation (Grant Nos 32370890, 32100642, 32170851, and 92168118), Postdoctoral Science Foundation of China (Grant No. 2022M713842).
Conflict of interest
The authors declare that the research was conducted in the absence of any commercial or financial relationships that could be construed as a potential conflict of interest.
Publisher’s note
All claims expressed in this article are solely those of the authors and do not necessarily represent those of their affiliated organizations, or those of the publisher, the editors and the reviewers. Any product that may be evaluated in this article, or claim that may be made by its manufacturer, is not guaranteed or endorsed by the publisher.
References
Aguirre-Vázquez, A., Salazar-Olivo, L. A., Flores-Ponce, X., Arriaga-Guerrero, A. L., Garza-Rodríguez, D., Camacho-Moll, M. E., et al. (2021). 5-Aza-2'-Deoxycytidine and valproic acid in combination with CHIR99021 and A83-01 induce pluripotency genes expression in human adult somatic cells. Molecules 26, 1909. doi:10.3390/molecules26071909
AL-Saeedi, M., Liang, R., Schultze, D. P., Nickkholgh, A., Herr, I., Zorn, M., et al. (2019). Glycine protects partial liver grafts from Kupffer cell-dependent ischemia-reperfusion injury without negative effect on regeneration. Amino Acids 51, 903–911. doi:10.1007/s00726-019-02722-5
Amare, G. G., Meharie, B. G., and Belayneh, Y. M. (2021). A drug repositioning success: the repositioned therapeutic applications and mechanisms of action of thalidomide. J. Oncol. Pharm. Pract. 27, 673–678. doi:10.1177/1078155220975825
Asumda, F. Z., Hatzistergos, K. E., Dykxhoorn, D. M., Jakubski, S., Edwards, J., Thomas, E., et al. (2018). Differentiation of hepatocyte-like cells from human pluripotent stem cells using small molecules. Differentiation 101, 16–24. doi:10.1016/j.diff.2018.03.002
Bahde, R., Kapoor, S., Bandi, S., Bhargava, K. K., Palestro, C. J., and Gupta, S. (2013). Directly acting drugs prostacyclin or nitroglycerine and endothelin receptor blocker bosentan improve cell engraftment in rodent liver. Hepatology 57, 320–330. doi:10.1002/hep.26005
Bahde, R., Kapoor, S., Viswanathan, P., Spiegel, H. U., and Gupta, S. (2014). Endothelin-1 receptor A blocker darusentan decreases hepatic changes and improves liver repopulation after cell transplantation in rats. Hepatology 59, 1107–1117. doi:10.1002/hep.26766
Bai, Y., Yang, Z., Xu, X., Ding, W., Qi, J., Liu, F., et al. (2023). Direct chemical induction of hepatocyte-like cells with capacity for liver repopulation. Hepatology 77, 1550–1565. doi:10.1002/hep.32686
Barahman, M., Asp, P., Roy-Chowdhury, N., Kinkhabwala, M., Roy-Chowdhury, J., Kabarriti, R., et al. (2019a). Hepatocyte transplantation: quo vadis? Int. J. Radiat. Oncol. Biol. Phys. 103, 922–934. doi:10.1016/j.ijrobp.2018.11.016
Barahman, M., Zhang, W., Harris, H. Y., Aiyer, A., Kabarriti, R., Kinkhabwala, M., et al. (2019b). Radiation-primed hepatocyte transplantation in murine monogeneic dyslipidemia normalizes cholesterol and prevents atherosclerosis. J. Hepatol. 70, 1170–1179. doi:10.1016/j.jhep.2019.01.010
Baral, I., Varghese, P. C., and Dutta, D. (2022). Epigenetics as “conductor” in “orchestra” of pluripotent states. Cell Tissue Res. 390, 141–172. doi:10.1007/s00441-022-03667-0
Barbarossa, A., Iacopetta, D., Sinicropi, M. S., Franchini, C., and Carocci, A. (2022). Recent advances in the development of thalidomide-related compounds as anticancer drugs. Curr. Med. Chem. 29, 19–40. doi:10.2174/0929867328666210623143526
Bardallo, R. G, Panisello-Roselló, A., Sanchez-Nuno, S., Alva, N., Roselló-Catafau, J., and Carbonell, T. (2022). Nrf2 and oxidative stress in liver ischemia/reperfusion injury. Febs J. 289 (18), 5463–5479. doi:10.1111/febs.16336
Benoist, C. C., Kawas, L. H., Zhu, M., Tyson, K. A., Stillmaker, L., Appleyard, S. M., et al. (2014). The procognitive and synaptogenic effects of angiotensin IV-derived peptides are dependent on activation of the hepatocyte growth factor/c-met system. J. Pharmacol. Exp. Ther. 351, 390–402. doi:10.1124/jpet.114.218735
Blackford, S. J. I., Ng, S. S., Segal, J. M., King, A. J. F., Austin, A. L., Kent, D., et al. (2019). Validation of current good manufacturing practice compliant human pluripotent stem cell-derived hepatocytes for cell-based therapy. Stem Cells Transl. Med. 8, 124–137. doi:10.1002/sctm.18-0084
Camacho-Aguilar, E., Yoon, S. T., Ortiz-Salazar, M. A., DU, S., Guerra, M. C., and Warmflash, A. (2024). Combinatorial interpretation of BMP and WNT controls the decision between primitive streak and extraembryonic fates. Cell Syst. 15, 445–461.e4. doi:10.1016/j.cels.2024.04.001
Chen, J., Yang, T., Song, S., Liu, Q., Sun, Y., Zhao, L., et al. (2019). Senescence suppressed proliferation of host hepatocytes is precondition for liver repopulation. Biochem. Biophys. Res. Commun. 516, 591–598. doi:10.1016/j.bbrc.2019.06.103
Chen, Y., Tang, D., Wu, H., Wu, Y., Yuan, T., Zhang, H., et al. (2021). Assessment of long-term functional maintenance of primary human hepatocytes to predict drug-induced hepatoxicity in vitro. Arch. Toxicol. 95, 2431–2442. doi:10.1007/s00204-021-03050-y
Clark, J. B., Rice, L., Sadiq, T., Brittain, E., Song, L., Wang, J., et al. (2005). Hepatic progenitor cell resistance to TGF-beta1's proliferative and apoptotic effects. Biochem. Biophys. Res. Commun. 329, 337–344. doi:10.1016/j.bbrc.2005.01.129
Dai, X. J., Liu, Y., Xue, L. P., Xiong, X. P., Zhou, Y., Zheng, Y. C., et al. (2021). Reversible lysine specific demethylase 1 (LSD1) inhibitors: a promising wrench to impair LSD1. J. Med. Chem. 64, 2466–2488. doi:10.1021/acs.jmedchem.0c02176
Delaforest, A., DI Furio, F., Jing, R., Ludwig-Kubinski, A., Twaroski, K., Urick, A., et al. (2018). HNF4A regulates the formation of hepatic progenitor cells from human iPSC-derived endoderm by facilitating efficient recruitment of RNA pol II. Genes (Basel) 10, 21. doi:10.3390/genes10010021
Dhawan, A., Chaijitraruch, N., Fitzpatrick, E., Bansal, S., Filippi, C., Lehec, S. C., et al. (2020). Alginate microencapsulated human hepatocytes for the treatment of acute liver failure in children. J. Hepatol. 72, 877–884. doi:10.1016/j.jhep.2019.12.002
Ding, J., Yannam, G. R., Roy-Chowdhury, N., Hidvegi, T., Basma, H., Rennard, S. I., et al. (2011). Spontaneous hepatic repopulation in transgenic mice expressing mutant human α1-antitrypsin by wild-type donor hepatocytes. J. Clin. Invest 121, 1930–1934. doi:10.1172/JCI45260
Driskill, J. H., and Pan, D. (2021). The Hippo pathway in liver homeostasis and pathophysiology. Annu. Rev. Pathol. 16, 299–322. doi:10.1146/annurev-pathol-030420-105050
Drozd, N. N., Logvinova, Y. S., Torlopov, M. A., and Udoratina, E. V. (2017). Effect of sulfation and molecular weight on anticoagulant activity of dextran. Bull. Exp. Biol. Med. 162, 462–465. doi:10.1007/s10517-017-3640-2
Duan, J. L., Ruan, B., Yan, X. C., Liang, L., Song, P., Yang, Z. Y., et al. (2018). Endothelial Notch activation reshapes the angiocrine of sinusoidal endothelia to aggravate liver fibrosis and blunt regeneration in mice. Hepatology 68, 677–690. doi:10.1002/hep.29834
Duan, Q., Li, S., Wen, X., Sunnassee, G., Chen, J., Tan, S., et al. (2019). Valproic acid enhances reprogramming efficiency and neuronal differentiation on small molecules staged-induction neural stem cells: suggested role of mTOR signaling. Front. Neurosci. 13, 867. doi:10.3389/fnins.2019.00867
DU, C., Feng, Y., Qiu, D., Xu, Y., Pang, M., Cai, N., et al. (2018). Highly efficient and expedited hepatic differentiation from human pluripotent stem cells by pure small-molecule cocktails. Stem Cell Res. Ther. 9, 58. doi:10.1186/s13287-018-0794-4
Dumasia, N. P., and Pethe, P. S. (2020). Pancreas development and the Polycomb group protein complexes. Mech. Dev. 164, 103647. doi:10.1016/j.mod.2020.103647
Dzik, S. (2019). Complement and coagulation: cross talk through time. Transfus. Med. Rev. 33, 199–206. doi:10.1016/j.tmrv.2019.08.004
Esmon, C. T. (2014). Targeting factor Xa and thrombin: impact on coagulation and beyond. Thromb. Haemost. 111, 625–633. doi:10.1160/TH13-09-0730
Fan, F., He, Z., Kong, L. L., Chen, Q., Yuan, Q., Zhang, S., et al. (2016). Pharmacological targeting of kinases MST1 and MST2 augments tissue repair and regeneration. Sci. Transl. Med. 8, 352ra108. doi:10.1126/scitranslmed.aaf2304
Gao, X., Li, R., Cahan, P., Zhao, Y., Yourick, J. J., and Sprando, R. L. (2020). Hepatocyte-like cells derived from human induced pluripotent stem cells using small molecules: implications of a transcriptomic study. Stem Cell Res. Ther. 11, 393. doi:10.1186/s13287-020-01914-1
Garcia-Llorens, G., Martínez-Sena, T., Pareja, E., Tolosa, L., Castell, J. V., and Bort, R. (2023). A robust reprogramming strategy for generating hepatocyte-like cells useable in pharmaco-toxicological studies. Stem Cell Res. Ther. 14, 94. doi:10.1186/s13287-023-03311-w
Ghazimoradi, M. H., and Farivar, S. (2020). The role of DNA demethylation in induction of stem cells. Prog. Biophys. Mol. Biol. 153, 17–22. doi:10.1016/j.pbiomolbio.2019.12.005
Gramignoli, R., Vosough, M., Kannisto, K., Srinivasan, R. C., and Strom, S. C. (2015). Clinical hepatocyte transplantation: practical limits and possible solutions. Eur. Surg. Res. 54, 162–177. doi:10.1159/000369552
Guo, R., Tang, W., Yuan, Q., Hui, L., Wang, X., and Xie, X. (2017). Chemical cocktails enable hepatic reprogramming of mouse fibroblasts with a single transcription factor. Stem Cell Rep. 9, 499–512. doi:10.1016/j.stemcr.2017.06.013
Gupta, S., Bhargava, K. K., and Novikoff, P. M. (1999a). Mechanisms of cell engraftment during liver repopulation with hepatocyte transplantation. Semin. Liver Dis. 19, 15–26. doi:10.1055/s-2007-1007094
Gupta, S., Rajvanshi, P., Sokhi, R., Slehria, S., Yam, A., Kerr, A., et al. (1999b). Entry and integration of transplanted hepatocytes in rat liver plates occur by disruption of hepatic sinusoidal endothelium. Hepatology 29, 509–519. doi:10.1002/hep.510290213
Gustafson, E. K., Elgue, G., Hughes, R. D., Mitry, R. R., Sanchez, J., Haglund, U., et al. (2011). The instant blood-mediated inflammatory reaction characterized in hepatocyte transplantation. Transplantation 91, 632–638. doi:10.1097/TP.0b013e31820ae459
Gustafson, E., Asif, S., Kozarcanin, H., Elgue, G., Meurling, S., Ekdahl, K. N., et al. (2017). Control of IBMIR induced by fresh and cryopreserved hepatocytes by low molecular weight dextran sulfate versus heparin. Cell Transpl. 26, 71–81. doi:10.3727/096368916X692609
Hayes, K., Kim, Y. K., and Pera, M. F. (2021). A case for revisiting Nodal signaling in human pluripotent stem cells. Stem Cells 39, 1137–1144. doi:10.1002/stem.3383
Heil, J., Schultze, D., Schemmer, P., and Bruns, H. (2018). N-acetylcysteine protects hepatocytes from hypoxia-related cell injury. Clin. Exp. Hepatol. 4, 260–266. doi:10.5114/ceh.2018.80128
He, S., and Tang, S. (2020). WNT/β-catenin signaling in the development of liver cancers. Biomed. Pharmacother. 132, 110851. doi:10.1016/j.biopha.2020.110851
Hirose, Y., Itoh, T., and Miyajima, A. (2009). Hedgehog signal activation coordinates proliferation and differentiation of fetal liver progenitor cells. Exp. Cell Res. 315, 2648–2657. doi:10.1016/j.yexcr.2009.06.018
Horisawa, K., Udono, M., Ueno, K., Ohkawa, Y., Nagasaki, M., Sekiya, S., et al. (2020). The dynamics of transcriptional activation by hepatic reprogramming factors. Mol. Cell 79, 660–676. doi:10.1016/j.molcel.2020.07.012
Huang, P., He, Z., Ji, S., Sun, H., Xiang, D., Liu, C., et al. (2011). Induction of functional hepatocyte-like cells from mouse fibroblasts by defined factors. Nature 475, 386–389. doi:10.1038/nature10116
Hu, Z., Evarts, R. P., Fujio, K., Marsden, E. R., and Thorgeirsson, S. S. (1993). Expression of hepatocyte growth factor and c-met genes during hepatic differentiation and liver development in the rat. Am. J. Pathol. 142, 1823–1830.
Jang, D. H., Weaver, M. D., and Pizon, A. F. (2013). In vitro study of N-acetylcysteine on coagulation factors in plasma samples from healthy subjects. J. Med. Toxicol. 9, 49–53. doi:10.1007/s13181-012-0242-2
Jiang, M., Guo, R., Ai, Y., Wang, G., Tang, P., Jia, X., et al. (2023). Small molecule drugs promote repopulation of transplanted hepatocytes by stimulating cell dedifferentiation. JHEP Rep. 5, 100670. doi:10.1016/j.jhepr.2023.100670
Ji, S., Zhang, L., and Hui, L. (2013). Cell fate conversion: direct induction of hepatocyte-like cells from fibroblasts. J. Cell Biochem. 114, 256–265. doi:10.1002/jcb.24380
Jitraruch, S., Dhawan, A., Hughes, R. D., Filippi, C., Soong, D., Philippeos, C., et al. (2014). Alginate microencapsulated hepatocytes optimised for transplantation in acute liver failure. PLoS One 9, e113609. doi:10.1371/journal.pone.0113609
Jorns, C., Ellis, E. C., Nowak, G., Fischler, B., Nemeth, A., Strom, S. C., et al. (2012). Hepatocyte transplantation for inherited metabolic diseases of the liver. J. Intern Med. 272, 201–223. doi:10.1111/j.1365-2796.2012.02574.x
Joseph, B., Kumaran, V., Berishvili, E., Bhargava, K. K., Palestro, C. J., and Gupta, S. (2006). Monocrotaline promotes transplanted cell engraftment and advances liver repopulation in rats via liver conditioning. Hepatology 44, 1411–1420. doi:10.1002/hep.21416
Joseph, B., Malhi, H., Bhargava, K. K., Palestro, C. J., Mccuskey, R. S., and Gupta, S. (2002). Kupffer cells participate in early clearance of syngeneic hepatocytes transplanted in the rat liver. Gastroenterology 123, 1677–1685. doi:10.1053/gast.2002.36592
Kale, A., and Rogers, N. M. (2023). No time to die-how islets meet their demise in transplantation. Cells 12, 796. doi:10.3390/cells12050796
Kamiya, A., Kinoshita, T., and Miyajima, A. (2001). Oncostatin M and hepatocyte growth factor induce hepatic maturation via distinct signaling pathways. FEBS Lett. 492, 90–94. doi:10.1016/s0014-5793(01)02140-8
Kim, K. S., Joseph, B., Inada, M., and Gupta, S. (2005). Regulation of hepatocyte engraftment and proliferation after cytotoxic drug-induced perturbation of the rat liver. Transplantation 80, 653–659. doi:10.1097/01.tp.0000173382.11916.bf
Knudsen, T. T., Thorsen, S., Jensen, S. A., Dalhoff, K., Schmidt, L. E., Becker, U., et al. (2005). Effect of intravenous N-acetylcysteine infusion on haemostatic parameters in healthy subjects. Gut 54, 515–521. doi:10.1136/gut.2004.043505
Koenig, S., Stoesser, C., Krause, P., Becker, H., and Markus, P. M. (2005). Liver repopulation after hepatocellular transplantation: integration and interaction of transplanted hepatocytes in the host. Cell Transpl. 14, 31–40. doi:10.3727/000000005783983322
Kopec, A. K., and Luyendyk, J. P. (2014). Coagulation in liver toxicity and disease: role of hepatocyte tissue factor. Thromb. Res. 133 (Suppl. 1), S57–S59. doi:10.1016/j.thromres.2014.03.023
Kopec, A. K., Spada, A. P., Contreras, P. C., Mackman, N., and Luyendyk, J. P. (2018). Caspase inhibition reduces hepatic tissue factor-driven coagulation in vitro and in vivo. Toxicol. Sci. 162, 396–405. doi:10.1093/toxsci/kfx268
Kotulkar, M., Robarts, D. R., and Apte, U. (2023). HNF4α in hepatocyte health and disease. Semin. Liver Dis. 43, 234–244. doi:10.1055/a-2097-0660
Kourtzelis, I., Magnusson, P. U., Kotlabova, K., Lambris, J. D., and Chavakis, T. (2015). Regulation of instant blood mediated inflammatory reaction (IBMIR) in pancreatic islet xeno-transplantation: points for therapeutic interventions. Adv. Exp. Med. Biol. 865, 171–188. doi:10.1007/978-3-319-18603-0_11
Krisinger, M. J., Goebeler, V., Lu, Z., Meixner, S. C., Myles, T., Pryzdial, E. L., et al. (2012). Thrombin generates previously unidentified C5 products that support the terminal complement activation pathway. Blood 120, 1717–1725. doi:10.1182/blood-2012-02-412080
Kusakabe, J., Hata, K., Tamaki, I., Tajima, T., Miyauchi, H., Wang, Y., et al. (2020). Complement 5 inhibition ameliorates hepatic ischemia/reperfusion injury in mice, dominantly via the C5a-mediated cascade. Transplantation 104, 2065–2077. doi:10.1097/TP.0000000000003302
Kusuhara, S., and Nakamura, M. (2020). Ripasudil hydrochloride hydrate in the treatment of glaucoma: safety, efficacy, and patient selection. Clin. Ophthalmol. 14, 1229–1236. doi:10.2147/OPTH.S216907
Lau, H. H., Ng, N. H. J., Loo, L. S. W., Jasmen, J. B., and Teo, A. K. K. (2018). The molecular functions of hepatocyte nuclear factors - in and beyond the liver. J. Hepatol. 68, 1033–1048. doi:10.1016/j.jhep.2017.11.026
Lee, C. A., Dhawan, A., Smith, R. A., Mitry, R. R., and Fitzpatrick, E. (2016). Instant blood-mediated inflammatory reaction in hepatocyte transplantation: current status and future perspectives. Cell Transpl. 25, 1227–1236. doi:10.3727/096368916X691286
Lee, C. S., Friedman, J. R., Fulmer, J. T., and Kaestner, K. H. (2005). The initiation of liver development is dependent on Foxa transcription factors. Nature 435, 944–947. doi:10.1038/nature03649
Lee, J. H., Lee, J. H., and Rane, S. G. (2021). TGF-Β signaling in pancreatic islet β cell development and function. Endocrinology 162, bqaa233. doi:10.1210/endocr/bqaa233
Lee, S. H., Li, Y., Kim, H., Eum, S., Park, K., and Lee, C. H. (2022). The role of EZH1 and EZH2 in development and cancer. BMB Rep. 55, 595–601. doi:10.5483/BMBRep.2022.55.12.174
Li, M., Zhang, X., Yu, J., Wu, X., and Sun, L. (2017a). Monocyte-derived procoagulant microvesicles induced by high glucose can Be attenuated by the antioxidant N-Acetyl-L-Cysteine, partly through the P38/MAPK pathway. Metab. Syndr. Relat. Disord. 15, 521–526. doi:10.1089/met.2017.0089
Lin, P., Bai, Y., Nian, X., Chi, J., Chen, T., Zhang, J., et al. (2023). Chemically induced revitalization of damaged hepatocytes for regenerative liver repair. iScience 26, 108532. doi:10.1016/j.isci.2023.108532
Liuyang, S., Wang, G., Wang, Y., He, H., Lyu, Y., Cheng, L., et al. (2023). Highly efficient and rapid generation of human pluripotent stem cells by chemical reprogramming. Cell Stem Cell 30, 450–459 e9. doi:10.1016/j.stem.2023.02.008
Li, W., Li, K., Wei, W., and Ding, S. (2013). Chemical approaches to stem cell biology and therapeutics. Cell Stem Cell 13, 270–283. doi:10.1016/j.stem.2013.08.002
Li, W., Li, L., and Hui, L. (2020). Cell plasticity in liver regeneration. Trends Cell Biol. 30, 329–338. doi:10.1016/j.tcb.2020.01.007
Li, X., Liu, D., Ma, Y., DU, X., Jing, J., Wang, L., et al. (2017b). Direct reprogramming of fibroblasts via a chemically induced XEN-like state. Cell Stem Cell 21, 264–273. doi:10.1016/j.stem.2017.05.019
Lotto, J., Stephan, T. L., and Hoodless, P. A. (2023). Fetal liver development and implications for liver disease pathogenesis. Nat. Rev. Gastroenterol. Hepatol. 20, 561–581. doi:10.1038/s41575-023-00775-2
Lucey, M. R., Furuya, K. N., and Foley, D. P. (2023). Liver transplantation. N. Engl. J. Med. 389, 1888–1900. doi:10.1056/NEJMra2200923
Luo, S., Ai, Y., Xiao, S., Wang, B., and Wang, Y. (2021). Functional hit 1 (FH1)-based rapid and efficient generation of functional hepatocytes from human mesenchymal stem cells: a novel strategy for hepatic differentiation. Ann. Transl. Med. 9, 1087. doi:10.21037/atm-21-2829
Lv, L., Han, Q., Chu, Y., Zhang, M., Sun, L., Wei, W., et al. (2015). Self-renewal of hepatoblasts under chemically defined conditions by iterative growth factor and chemical screening. Hepatology 61, 337–347. doi:10.1002/hep.27421
Macchi, F., and Sadler, K. C. (2020). Unraveling the epigenetic basis of liver development, regeneration and disease. Trends Genet. 36, 587–597. doi:10.1016/j.tig.2020.05.002
Ma, H., Wang, C., Liang, S., Yu, X., Yuan, Y., Lv, Z., et al. (2023). ROCK inhibition enhanced hepatocyte liver engraftment by retaining membrane CD59 and attenuating complement activation. Mol. Ther. 31, 1846–1856. doi:10.1016/j.ymthe.2023.02.018
Mali, P., Chou, B.-K., Yen, J., Ye, Z., Zou, J., Dowey, S., et al. (2010). Butyrate greatly enhances derivation of human induced pluripotent stem cells by promoting epigenetic remodeling and the expression of pluripotency-associated genes. Stem Cells 28, 713–720. doi:10.1002/stem.402
Martinez-Gamero, C., Malla, S., and Aguilo, F. (2021). LSD1: expanding functions in stem cells and differentiation. Cells 10, 3252. doi:10.3390/cells10113252
Matas, A. J., Sutherland, D. E., Steffes, M. W., Mauer, S. M., Sowe, A., Simmons, R. L., et al. (1976). Hepatocellular transplantation for metabolic deficiencies: decrease of plasms bilirubin in Gunn rats. Science 192, 892–894. doi:10.1126/science.818706
Mauron, T., Lämmle, B., and Wuillemin, W. A. (1998). Influence of low molecular weight heparin and low molecular weight dextran sulfate on the inhibition of coagulation factor XIa by serpins. Thromb. Haemost. 80, 82–86. doi:10.1055/s-0037-1615143
Mercurio, A., Adriani, G., Catalano, A., Carocci, A., Rao, L., Lentini, G., et al. (2017). A mini-review on thalidomide: chemistry, mechanisms of action, therapeutic potential and anti-angiogenic properties in multiple myeloma. Curr. Med. Chem. 24, 2736–2744. doi:10.2174/0929867324666170601074646
Meyburg, J., Das, A. M., Hoerster, F., Lindner, M., Kriegbaum, H., Engelmann, G., et al. (2009). One liver for four children: first clinical series of liver cell transplantation for severe neonatal urea cycle defects. Transplantation 87, 636–641. doi:10.1097/TP.0b013e318199936a
Mito, M., Kusano, M., and Kawaura, Y. (1992). Hepatocyte transplantation in man. Transpl. Proc. 24, 3052–3053.
Miyoshi, T., Hidaka, M., Miyamoto, D., Sakai, Y., Murakami, S., Huang, Y., et al. (2022). Successful induction of human chemically induced liver progenitors with small molecules from damaged liver. J. Gastroenterol. 57, 441–452. doi:10.1007/s00535-022-01869-5
Moreira, A. L., Friedlander, D. R., Shif, B., Kaplan, G., and Zagzag, D. (1999). Thalidomide and a thalidomide analogue inhibit endothelial cell proliferation in vitro. J. Neurooncol 43, 109–114. doi:10.1023/a:1006202700039
Mou, L., Shi, G., Cooper, D. K. C., Lu, Y., Chen, J., Zhu, S., et al. (2022). Current topics of relevance to the xenotransplantation of free pig islets. Front. Immunol. 13, 854883. doi:10.3389/fimmu.2022.854883
Nakamae, S., Toba, Y., Takayama, K., Sakurai, F., and Mizuguchi, H. (2018). Nanaomycin A treatment promotes hepatoblast differentiation from human iPS cells. Stem Cells Dev. 27, 405–414. doi:10.1089/scd.2017.0251
Naziruddin, B., Iwahashi, S., Kanak, M. A., Takita, M., Itoh, T., and Levy, M. F. (2014). Evidence for instant blood-mediated inflammatory reaction in clinical autologous islet transplantation. Am. J. Transpl. 14, 428–437. doi:10.1111/ajt.12558
Nguyen, M. P., Jain, V., Iansante, V., Mitry, R. R., Filippi, C., and Dhawan, A. (2020). Clinical application of hepatocyte transplantation: current status, applicability, limitations, and future outlook. Expert Rev. Gastroenterology and Hepatology 14, 185–196. doi:10.1080/17474124.2020.1733975
Nilsson, B., Teramura, Y., and Ekdahl, K. N. (2014). The role and regulation of complement activation as part of the thromboinflammation elicited in cell therapies. Mol. Immunol. 61, 185–190. doi:10.1016/j.molimm.2014.06.009
Noguchi, D., Kuriyama, N., Hibi, T., Maeda, K., Shinkai, T., Gyoten, K., et al. (2021). The impact of dabigatran treatment on sinusoidal protection against hepatic ischemia/reperfusion injury in mice. Liver Transpl. 27, 363–384. doi:10.1002/lt.25929
Nulty, J., Anand, H., and Dhawan, A. (2024). Human hepatocyte transplantation: three decades of clinical experience and future perspective. Stem Cells Transl. Med. 13, 204–218. doi:10.1093/stcltm/szad084
Onai, T. (2019). Canonical Wnt/β-catenin and Notch signaling regulate animal/vegetal axial patterning in the cephalochordate amphioxus. Evol. Dev. 21, 31–43. doi:10.1111/ede.12273
Overturf, K., AL-Dhalimy, M., Tanguay, R., Brantly, M., Ou, C. N., Finegold, M., et al. (1996). Hepatocytes corrected by gene therapy are selected in vivo in a murine model of hereditary tyrosinaemia type I. Nat. Genet. 12, 266–273. doi:10.1038/ng0396-266
Pałasz, A., and Czekaj, P. (2000). Toxicological and cytophysiological aspects of lanthanides action. Acta Biochim. Pol. 47, 1107–1114. doi:10.18388/abp.2000_3963
Pan, T., Chen, Y., Zhuang, Y., Yang, F., Xu, Y., Tao, J., et al. (2019). Synergistic modulation of signaling pathways to expand and maintain the bipotency of human hepatoblasts. Stem Cell Res. Ther. 10, 364. doi:10.1186/s13287-019-1463-y
Pan, T., Tao, J., Chen, Y., Zhang, J., Getachew, A., Zhuang, Y., et al. (2021). Robust expansion and functional maturation of human hepatoblasts by chemical strategy. Stem Cell Res. Ther. 12, 151. doi:10.1186/s13287-021-02233-9
Pan, T., Wang, N., Zhang, J., Yang, F., Chen, Y., Zhuang, Y., et al. (2022). Efficiently generate functional hepatic cells from human pluripotent stem cells by complete small-molecule strategy. Stem Cell Res. Ther. 13, 159. doi:10.1186/s13287-022-02831-1
Park, S., Hwang, S., Kim, J., Hwang, S., Kang, S., Yang, S., et al. (2019). The therapeutic potential of induced hepatocyte-like cells generated by direct reprogramming on hepatic fibrosis. Stem Cell Res. Ther. 10, 21. doi:10.1186/s13287-018-1127-3
Peng, W. C., Kraaier, L. J., and Kluiver, T. A. (2021). Hepatocyte organoids and cell transplantation: what the future holds. Exp. Mol. Med. 53, 1512–1528. doi:10.1038/s12276-021-00579-x
Peralta, C., Jimenez-Castro, M. B., and Gracia-Sancho, J. (2013). Hepatic ischemia and reperfusion injury: effects on the liver sinusoidal milieu. J. Hepatol. 59, 1094–1106. doi:10.1016/j.jhep.2013.06.017
Perugorria, M. J., Olaizola, P., Labiano, I., Esparza-Baquer, A., Marzioni, M., Marin, J. J. G., et al. (2019). Wnt-β-catenin signalling in liver development, health and disease. Nat. Rev. Gastroenterol. Hepatol. 16, 121–136. doi:10.1038/s41575-018-0075-9
Pietrosi, G., Vizzini, G., Gerlach, J., Chinnici, C., Luca, A., Amico, G., et al. (2015). Phases I-II matched case-control study of human fetal liver cell transplantation for treatment of chronic liver disease. Cell Transpl. 24, 1627–1638. doi:10.3727/096368914X682422
Pizon, A. F., Jang, D. H., and Wang, H. E. (2011). The in vitro effect of N-acetylcysteine on prothrombin time in plasma samples from healthy subjects. Acad. Emerg. Med. 18, 351–354. doi:10.1111/j.1553-2712.2011.01041.x
Pour, M., Kumar, A. S., Farag, N., Bolondi, A., Kretzmer, H., Walther, M., et al. (2022). Emergence and patterning dynamics of mouse-definitive endoderm. iScience 25, 103556. doi:10.1016/j.isci.2021.103556
Raggi, C., M'Callum, M. A., Pham, Q. T., Gaub, P., Selleri, S., Baratang, N. V., et al. (2022). Leveraging interacting signaling pathways to robustly improve the quality and yield of human pluripotent stem cell-derived hepatoblasts and hepatocytes. Stem Cell Rep. 17, 584–598. doi:10.1016/j.stemcr.2022.01.003
Raghav, P. K., and Gangenahalli, G. (2021). PU.1 mimic synthetic peptides selectively bind with GATA-1 and allow c-jun PU.1 binding to enhance myelopoiesis. Int. J. Nanomedicine 16, 3833–3859. doi:10.2147/IJN.S303235
Rajvanshi, P., Kerr, A., Bhargava, K. K., Burk, R. D., and Gupta, S. (1996). Studies of liver repopulation using the dipeptidyl peptidase IV-deficient rat and other rodent recipients: cell size and structure relationships regulate capacity for increased transplanted hepatocyte mass in the liver lobule. Hepatology 23, 482–496. doi:10.1002/hep.510230313
Rentsch, M., Puellmann, K., Sirek, S., Iesalnieks, I., Kienle, K., Mueller, T., et al. (2005). Benefit of Kupffer cell modulation with glycine versus Kupffer cell depletion after liver transplantation in the rat: effects on postischemic reperfusion injury, apoptotic cell death graft regeneration and survival. Transpl. Int. 18, 1079–1089. doi:10.1111/j.1432-2277.2005.00185.x
Rombaut, M., Boeckmans, J., Rodrigues, R. M., VAN Grunsven, L. A., Vanhaecke, T., and De Kock, J. (2021). Direct reprogramming of somatic cells into induced hepatocytes: cracking the Enigma code. J. Hepatol. 75, 690–705. doi:10.1016/j.jhep.2021.04.048
Russell, J. O., and Camargo, F. D. (2022). Hippo signalling in the liver: role in development, regeneration and disease. Nat. Rev. Gastroenterol. Hepatol. 19, 297–312. doi:10.1038/s41575-021-00571-w
Sekiya, S., and Suzuki, A. (2011). Direct conversion of mouse fibroblasts to hepatocyte-like cells by defined factors. Nature 475, 390–393. doi:10.1038/nature10263
Shan, J., Schwartz, R. E., Ross, N. T., Logan, D. J., Thomas, D., Duncan, S. A., et al. (2013). Identification of small molecules for human hepatocyte expansion and iPS differentiation. Nat. Chem. Biol. 9, 514–520. doi:10.1038/nchembio.1270
Sharma, R., Porterfield, J. E., An, H. T., Jimenez, A. S., Lee, S., Kannan, S., et al. (2021). Rationally designed galactose dendrimer for hepatocyte-specific targeting and intracellular drug delivery for the treatment of liver disorders. Biomacromolecules 22, 3574–3589. doi:10.1021/acs.biomac.1c00649
Shi, Y., Desponts, C., Do, J. T., Hahm, H. S., Scholer, H. R., and Ding, S. (2008). Induction of pluripotent stem cells from mouse embryonic fibroblasts by Oct4 and Klf4 with small-molecule compounds. Cell Stem Cell 3, 568–574. doi:10.1016/j.stem.2008.10.004
Siller, R., Greenhough, S., Naumovska, E., and Sullivan, G. J. (2015). Small-molecule-driven hepatocyte differentiation of human pluripotent stem cells. Stem Cell Rep. 4, 939–952. doi:10.1016/j.stemcr.2015.04.001
Singh, K., Gupta, J. K., Kumar, S., Mukherjee, S., and Patel, A. (2024). Hepatic ischemia-reperfusion injury: protective approaches and treatment. Curr. Mol. Pharmacol. 17, e030823219400. doi:10.2174/1874467217666230803114856
Slehria, S., Rajvanshi, P., Ito, Y., Sokhi, R. P., Bhargava, K. K., Palestro, C. J., et al. (2002). Hepatic sinusoidal vasodilators improve transplanted cell engraftment and ameliorate microcirculatory perturbations in the liver. Hepatology 35, 1320–1328. doi:10.1053/jhep.2002.33201
Snykers, S., Henkens, T., De Rop, E., Vinken, M., Fraczek, J., De Kock, J., et al. (2009). Role of epigenetics in liver-specific gene transcription, hepatocyte differentiation and stem cell reprogrammation. J. Hepatol. 51, 187–211. doi:10.1016/j.jhep.2009.03.009
Soltys, K. A., Setoyama, K., Tafaleng, E. N., Soto Gutiérrez, A., Fong, J., Fukumitsu, K., et al. (2017). Host conditioning and rejection monitoring in hepatocyte transplantation in humans. J. Hepatol. 66, 987–1000. doi:10.1016/j.jhep.2016.12.017
Stephenne, X., Nicastro, E., Eeckhoudt, S., Hermans, C., Nyabi, O., Lombard, C., et al. (2012). Bivalirudin in combination with heparin to control mesenchymal cell procoagulant activity. PLoS One 7, e42819. doi:10.1371/journal.pone.0042819
Stéphenne, X., Vosters, O., Najimi, M., Beuneu, C., Dung, K. N., Wijns, W., et al. (2007). Tissue factor-dependent procoagulant activity of isolated human hepatocytes: relevance to liver cell transplantation. Liver Transpl. 13, 599–606. doi:10.1002/lt.21128
Sun, P., Zhang, G., Su, X., Jin, C., Yu, B., Yu, X., et al. (2019). Maintenance of primary hepatocyte functions in vitro by inhibiting mechanical tension-induced YAP activation. Cell Rep. 29, 3212–3222. doi:10.1016/j.celrep.2019.10.128
Sun, Z., Yuan, X., Wu, J., Wang, C., Zhang, K., Zhang, L., et al. (2023). Hepatocyte transplantation: the progress and the challenges. Hepatol. Commun. 7, e0266. doi:10.1097/HC9.0000000000000266
Tachmatzidi, E. C., Galanopoulou, O., and Talianidis, I. (2021). Transcription control of liver development. Cells 10, 2026. doi:10.3390/cells10082026
Tasnim, F., Phan, D., Toh, Y. C., and Yu, H. (2015). Cost-effective differentiation of hepatocyte-like cells from human pluripotent stem cells using small molecules. Biomaterials 70, 115–125. doi:10.1016/j.biomaterials.2015.08.002
Touboul, T., Chen, S., To, C. C., Mora-Castilla, S., Sabatini, K., Tukey, R. H., et al. (2016). Stage-specific regulation of the WNT/β-catenin pathway enhances differentiation of hESCs into hepatocytes. J. Hepatol. 64, 1315–1326. doi:10.1016/j.jhep.2016.02.028
Unagolla, J. M., Das, S., Flanagan, R., Oehler, M., and Menon, J. U. (2024). Targeting chronic liver diseases: molecular markers, drug delivery strategies and future perspectives. Int. J. Pharm. 660, 124381. doi:10.1016/j.ijpharm.2024.124381
VAN Der Windt, D. J., Bottino, R., Casu, A., Campanile, N., and Cooper, D. K. (2007). Rapid loss of intraportally transplanted islets: an overview of pathophysiology and preventive strategies. Xenotransplantation 14, 288–297. doi:10.1111/j.1399-3089.2007.00419.x
Viswanathan, P., Gupta, P., Kapoor, S., and Gupta, S. (2016). Thalidomide promotes transplanted cell engraftment in the rat liver by modulating inflammation and endothelial integrity. J. Hepatol. 65, 1171–1178. doi:10.1016/j.jhep.2016.07.008
Von Zur-Mühlen, B., Lundgren, T., Bayman, L., Berne, C., Bridges, N., Eggerman, T., et al. (2019). Open randomized multicenter study to evaluate safety and efficacy of low molecular weight sulfated dextran in islet transplantation. Transplantation 103, 630–637. doi:10.1097/TP.0000000000002425
Wang, L. J., Chen, Y. M., George, D., Smets, F., Sokal, E. M., Bremer, E. G., et al. (2002). Engraftment assessment in human and mouse liver tissue after sex-mismatched liver cell transplantation by real-time quantitative PCR for Y chromosome sequences. Liver Transpl. 8, 822–828. doi:10.1053/jlts.2002.34891
Wang, Z. M., Yuan, X. H., and Shen, H. (2015). BMP-4 induced proliferation and oriented differentiation of rat hepatic oval cells into hepatocytes. Asian Pac J. Trop. Med. 8, 412–416. doi:10.1016/S1995-7645(14)60353-9
Wang, F., Zhou, L., Ma, X., Ma, W., Wang, C., Lu, Y., et al. (2014). Monitoring of intrasplenic hepatocyte transplantation for acute-on-chronic liver failure: a prospective five-year follow-up study. Transpl. Proc. 46, 192–198. doi:10.1016/j.transproceed.2013.10.042
Wang, J., Sun, S., and Deng, H. (2023). Chemical reprogramming for cell fate manipulation: methods, applications, and perspectives. Cell Stem Cell 30, 1130–1147. doi:10.1016/j.stem.2023.08.001
Wild, S. L., Elghajiji, A., Grimaldos Rodriguez, C., Weston, S. D., Burke, Z. D., and Tosh, D. (2020). The canonical Wnt pathway as a key regulator in liver development, differentiation and homeostatic renewal. Genes (Basel) 11, 1163. doi:10.3390/genes11101163
Wilhelm, A., Leister, I., Sabandal, P., Krause, P., Becker, H., and Markus, P. M. (2004). Acute impairment of hepatic microcirculation and recruitment of nonparenchymal cells by intrasplenic hepatocyte transplantation. J. Pediatr. Surg. 39, 1214–1219. doi:10.1016/j.jpedsurg.2004.04.020
Wilkinson, A. L., Zorzan, I., and Rugg-Gunn, P. J. (2023). Epigenetic regulation of early human embryo development. Cell Stem Cell 30, 1569–1584. doi:10.1016/j.stem.2023.09.010
Wu, Y. M., Joseph, B., Berishvili, E., Kumaran, V., and Gupta, S. (2008). Hepatocyte transplantation and drug-induced perturbations in liver cell compartments. Hepatology 47, 279–287. doi:10.1002/hep.21937
Wu, D., Zhang, J., Jun, Y., Liu, L., Huang, C., Wang, W., et al. (2023) “The emerging role of DOT1L in cell proliferation and differentiation: friend or foe,”, 18658. University of Murcia, Murcia, Spain: Histol Histopathol.
Wu, H., Zhou, X., Fu, G. B., He, Z. Y., Wu, H. P., You, P., et al. (2017). Reversible transition between hepatocytes and liver progenitors for in vitro hepatocyte expansion. Cell Res. 27, 709–712. doi:10.1038/cr.2017.47
Wuillemin, W. A., Eldering, E., Citarella, F., De Ruig, C. P., Ten Cate, H., and Hack, C. E. (1996). Modulation of contact system proteases by glycosaminoglycans. Selective enhancement of the inhibition of factor XIa. J. Biol. Chem. 271, 12913–12918. doi:10.1074/jbc.271.22.12913
Wuillemin, W. A., Te Velthuis, H., Lubbers, Y. T., De Ruig, C. P., Eldering, E., and Hack, C. E. (1997). Potentiation of C1 inhibitor by glycosaminoglycans: dextran sulfate species are effective inhibitors of in vitro complement activation in plasma. J. Immunol. 159, 1953–1960. doi:10.4049/jimmunol.159.4.1953
Xiang, C., DU, Y., Meng, G., Soon Yi, L., Sun, S., Song, N., et al. (2019). Long-term functional maintenance of primary human hepatocytes in vitro. Science 364, 399–402. doi:10.1126/science.aau7307
Xu, C. R., Cole, P. A., Meyers, D. J., Kormish, J., Dent, S., and Zaret, K. S. (2011). Chromatin “prepattern” and histone modifiers in a fate choice for liver and pancreas. Science 332, 963–966. doi:10.1126/science.1202845
Xue, Z. F., Wu, X. M., and Liu, M. (2013). Hepatic regeneration and the epithelial to mesenchymal transition. World J. Gastroenterol. 19, 1380–1386. doi:10.3748/wjg.v19.i9.1380
Yan, L. L., Ye, L. P., Chen, Y. H., He, S. Q., Zhang, C. Y., Mao, X. L., et al. (2022). The influence of microenvironment on survival of intraportal transplanted islets. Front. Immunol. 13, 849580. doi:10.3389/fimmu.2022.849580
Yang, L., Li, L. C., Wang, X., Wang, W. H., Wang, Y. C., Xu, C. R., et al. (2019). The contributions of mesoderm-derived cells in liver development. Semin. Cell Dev. Biol. 92, 63–76. doi:10.1016/j.semcdb.2018.09.003
Yang, Z., Xu, X., Gu, C., Li, J., Wu, Q., Ye, C., et al. (2020). Chemicals orchestrate reprogramming with hierarchical activation of master transcription factors primed by endogenous Sox17 activation. Commun. Biol. 3, 629. doi:10.1038/s42003-020-01346-w
Yuan, L., Zhang, Y., Liu, X., Chen, Y., Zhang, L., Cao, J., et al. (2019). Agonist c-met monoclonal antibody augments the proliferation of hiPSC-derived hepatocyte-like cells and improves cell transplantation therapy for liver failure in mice. Theranostics 9, 2115–2128. doi:10.7150/thno.30009
Yu, B., He, Z. Y., You, P., Han, Q. W., Xiang, D., Chen, F., et al. (2013). Reprogramming fibroblasts into bipotential hepatic stem cells by defined factors. Cell Stem Cell 13, 328–340. doi:10.1016/j.stem.2013.06.017
Zhao, Y., Ye, W., Wang, Y. D., and Chen, W. D. (2022). HGF/c-Met: a key promoter in liver regeneration. Front. Pharmacol. 13, 808855. doi:10.3389/fphar.2022.808855
Zhong, Z., DU, J., Zhu, X., Guan, L., Hu, Y., Zhang, P., et al. (2024). Highly efficient conversion of mouse fibroblasts into functional hepatic cells under chemical induction. J. Mol. Cell Biol. 15, mjad071. doi:10.1093/jmcb/mjad071
Zhou, Y., Xu, J., Luo, H., Meng, X., Chen, M., and Zhu, D. (2022). Wnt signaling pathway in cancer immunotherapy. Cancer Lett. 525, 84–96. doi:10.1016/j.canlet.2021.10.034
Zhu, N., Wang, L., Guo, H., Jia, J., Gu, L., Wang, X., et al. (2021). Thalidomide suppresses angiogenesis through the signal transducer and activator of transcription 3/SP4 signaling pathway in the peritoneal membrane. Front. Physiol. 12, 712147. doi:10.3389/fphys.2021.712147
Zhu, S., Rezvani, M., Harbell, J., Mattis, A. N., Wolfe, A. R., Benet, L. Z., et al. (2014). Mouse liver repopulation with hepatocytes generated from human fibroblasts. Nature 508, 93–97. doi:10.1038/nature13020
Glossary
HTx hepatocyte transplantation
OLT orthotopic liver transplantation
ALF acute liver failure
PHHs primary human hepatocyte
HLC hepatocyte-like cells
IBMIR instant blood-mediated inflammatory reaction
BMP bone morphogenic protein
DE definitive endoderm
PFG posterior foregut
HB hepatoblast
FGFs fibroblast growth factors
LSD1 lysine-specific demethylase 1
PSCs pluripotent stem cells
hESCs human embryonic stem cells
CHIR CHIR99021
AFP fetoprotein
ALB albumin
PROX1 prospero homeobox 1
NaB sodium butyrate
DMSO dimethyl sulfoxide
HGF hepatocyte growth factor
HPCs hepatic progenitor cells
FH1 functional hits 1
FPH1 functional proliferation hit 1
cGMP current good manufacturing practice
hiPSC human iPSC
MEFs mouse embryonic fibroblasts
iHeps induced hepatocyte-like cells
VPA valproic acid
XEN extra-embryonic endoderm
KMTs histone methyltransferases
DNMTs DNA methyltransferases
HDAC histone deacetylase
KCs Kupffer cells
HSCs hepatic stellate cells
TF tissue factor
MAC membrane attack complex
GdCl3 Gadolinium chloride
HIR hepatic irradiation
Keywords: hepatocyte transplantation, small molecule, cell-based therapy, induced pluripotent stem cell, instant blood-mediated inflammatory reaction
Citation: Shi H, Ding Y, Sun P, Lv Z, Wang C, Ma H, Lu J, Yu B, Li W and Wang C (2024) Chemical approaches targeting the hurdles of hepatocyte transplantation: mechanisms, applications, and advances. Front. Cell Dev. Biol. 12:1480226. doi: 10.3389/fcell.2024.1480226
Received: 13 August 2024; Accepted: 21 October 2024;
Published: 31 October 2024.
Edited by:
Ting Li, Southern Medical University, ChinaReviewed by:
Pawan Kumar Raghav, University of California, San Francisco, United StatesXiaolei Li, University of Pennsylvania, United States
Copyright © 2024 Shi, Ding, Sun, Lv, Wang, Ma, Lu, Yu, Li and Wang. This is an open-access article distributed under the terms of the Creative Commons Attribution License (CC BY). The use, distribution or reproduction in other forums is permitted, provided the original author(s) and the copyright owner(s) are credited and that the original publication in this journal is cited, in accordance with accepted academic practice. No use, distribution or reproduction is permitted which does not comply with these terms.
*Correspondence: Chao Wang, d2FuZ2NoYW9zbW11QDEyNi5jb20=; Wenlin Li, bGl3ZW5saW5Ac21tdS5lZHUuY24=; Bing Yu, c21tdWNlbGx5dUAxNjMuY29t
†These authors have contributed equally to this work and share first authorship