- 1Department of Hepatopancreatobiliary Surgery, The First Affiliated Hospital of Soochow University, Suzhou, China
- 2Department of Anesthesiology, Xinyi People’s Hospital, Xinyi, Jiangsu, China
- 3Department of General Surgery, Wujin Affiliated Hospital of Jiangsu University and The Wujin Clinical College of Xuzhou Medical University, Changzhou, Jiangsu, China
Ferritinophagy, the selective autophagic degradation of ferritin to release iron, is emerging as a critical regulator of iron homeostasis and a key player in the pathogenesis of various liver diseases. This review comprehensively examines the mechanisms, regulation, and multifaceted roles of ferritinophagy in liver health and disease. Ferritinophagy is intricately regulated by several factors, including Nuclear Receptor Coactivator 4 (NCOA4), Iron regulatory proteins and signaling pathways such as mTOR and AMPK. These regulatory mechanisms ensure proper iron utilization and prevent iron overload, which can induce oxidative stress and ferroptosis. In liver diseases, ferritinophagy exhibits dual roles. In liver fibrosis, promoting ferritinophagy in hepatic stellate cells (HSCs) can induce cell senescence and reduce fibrosis progression. However, in non-alcoholic fatty liver disease (NAFLD), chronic ferritinophagy may exacerbate liver injury through iron overload and oxidative stress. In hepatocellular carcinoma (HCC), ferritinophagy can be harnessed as a novel therapeutic strategy by inducing ferroptosis in cancer cells. Additionally, ferritinophagy is implicated in drug-induced liver injury and sepsis-associated liver damage, highlighting its broad impact on liver pathology. This review also explores the crosstalk between ferritinophagy and other selective autophagy pathways, such as mitophagy and lipophagy, which collectively influence cellular homeostasis and disease progression. Understanding these interactions is essential for developing comprehensive therapeutic strategies targeting multiple autophagy pathways. In summary, ferritinophagy is a complex and dynamic process with significant implications for liver diseases. This review provides an in-depth analysis of ferritinophagy’s regulatory mechanisms and its potential as a therapeutic target, emphasizing the need for further research to elucidate its role in liver health and disease.
Introduction
The phenomenon of autophagy was first discovered by scholars Ashford and Porter in 1962. However, at that time, they interpreted their observations as the process of lysosome formation and believed that lysosomes were not independent organelles but rather a part of mitochondria (Ashford and Porter, 1962). It was not until 1967 that Duve and his colleagues formally named this phenomenon “autophagy” and identified lysosomes as the sites where autophagy occurs (Deter et al., 1967). Over the next 30 years, numerous researchers conducted studies based on this foundation, discovering and naming several autophagy-related proteins. To minimize confusion and complications arising from different naming conventions, a unified gene and protein nomenclature was established (Klionsky et al., 2003).
Autophagy is an intracellular degradation and recycling pathway. It involves encapsulating target substances into autophagosomes, which are then sent to lysosomes for degradation. This process, which helps to mitigate damage caused by metabolic stress and clear out unnecessary or harmful substances within the cell, plays a crucial role in maintaining cellular homeostasis (Patel et al., 2013). Ferroptosis, another emerging research hotspot, was initially regarded as a novel form of cell death dependent on intracellular iron. Unlike traditional apoptosis, autophagy, and necrosis, ferroptosis is primarily caused by the excessive accumulation of iron ions and lipid peroxidation within cells (Dixon et al., 2012). However, in recent years, researchers have discovered a close link between autophagy and ferroptosis. The most representative example is ferritinophagy, which involves the degradation of ferritin to release free Fe2+ ions. These Fe2+ ions generate excessive ROS through the Fenton reaction, thereby inducing ferroptosis (Qian et al., 2024).
Ferritin, the primary iron storage protein in the human body, is crucial for maintaining iron homeostasis. It regulates intracellular iron balance by storing and releasing iron as needed (Knovich et al., 2009). Structurally, ferritin is a 24-subunit macromolecular iron-storage complex composed of ferritin heavy chain (FTH1) and ferritin light chain (FTL) subunits. FTH1 can oxidize ferrous iron (Fe2⁺) to ferric iron (Fe3⁺), allowing it to be safely stored within the ferritin complex. While FTL promotes iron nucleation, enhancing the stability of the ferritin structure (Pantopoulos et al., 2012). As early as 2005, Theodros and his colleagues discovered that lysosomes play a crucial role in degrading ferritin and releasing iron ions (Kidane et al., 2006). But It was not until 2014 that a paper published in Nature demonstrated that NCOA4 is a key receptor for ferritinophagy. NCOA4 mediates the localization of ferritin to autophagosomes, which then fuse with lysosomes to complete the degradation process. (Mancias et al., 2014). Under normal circumstances, ferritinophagy adjusts according to intracellular iron levels. When there is an excess of iron, NCOA4 can be ubiquitinated and degraded, promoting the storage of surplus iron and preventing its toxic effects. In conditions of iron deficiency, NCOA4 selectively binds to ferritin, facilitating its degradation through autophagy to release iron, thereby maintaining normal cellular iron levels and physiological activities (Mancias et al., 2015; Wu H. et al., 2023).
Recent research has found that ferritinophagy is not only crucial for maintaining iron balance under normal physiological conditions but also closely related to the development and progression of various diseases. Under conditions of toxicity or oxidative stress, overexpression of ferritinophagy can lead to a range of liver diseases. For example, exposure to metals and chemicals such as cadmium, copper, and arsenic (As) can induce ferritinophagy and result in liver damage (He et al., 2022; Yu L. et al., 2023; Zhong et al., 2024). In patients with NAFLD, short-term ferritinophagy can reduce lipotoxicity. However, chronic ferritinophagy often indicates worsening of the condition, progressing from reversible fatty liver to irreversible liver cirrhosis (Li et al., 2022). From another perspective, harnessing ferritinophagy to target specific detrimental factors can also benefit certain diseases. Such as, in liver fibrosis, employing specific drugs to enhance ferritinophagy in HSCs can effectively mitigate the progression of liver fibrosis (Kong et al., 2019). In this context, this review aims to summarize the fundamental mechanisms and regulatory pathways of ferritinophagy, along with its potential roles in various diseases. The goal is to offer new insights and advance research in this field.
Regulation of ferritinophagy
Ferritinophagy is a specialized form of autophagy. It shares many similarities with the classical autophagy pathway, primarily involving three steps: the formation of autophagosomes, the fusion of autophagosomes with lysosomes, and the degradation and recycling of the contents (Figure 1). Ferritinophagy plays a crucial role in regulating cellular iron balance, ensuring that iron is properly utilized in metabolism. If ferritinophagy is impaired, it can lead to iron overload, where excess iron participates in the Fenton reaction. This generates reactive hydroxyl radicals (•OH), which are highly oxidative and can damage cell membranes, potentially triggering lipid peroxidation and ferroptosis, a form of cell death that can disrupt liver function (Qian et al., 2024; Szarka et al., 2021).
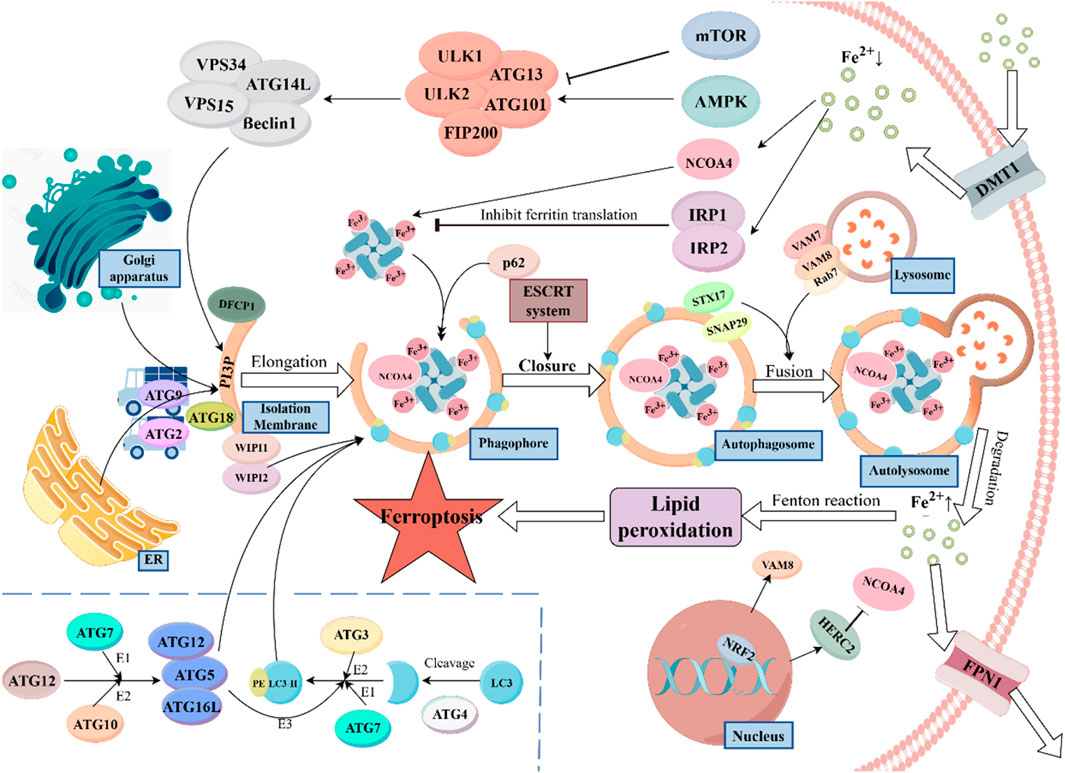
Figure 1. Ferritinophagy, a specific form of autophagy, is vital for maintaining cellular iron homeostasis. The process begins with the recognition of ferritin by Nuclear Receptor Coactivator 4 (NCOA4), which directs ferritin to the isolation membrane (IM). Upon autophagosome maturation, ferritin is degraded in the lysosome, releasing iron ions, which are exported by Ferroportin 1 (FPN1). Divalent Metal Transporter 1 (DMT1) mediates iron uptake through endosomal formation. The autophagic process is regulated by mechanistic target of rapamycin (mTOR) and AMP-activated protein kinase (AMPK) signaling in response to nutrient availability. The Autophagy-Related Gene 16-Like (ATG16L) complex facilitates the lipidation of Light Chain 3 (LC3), essential for autophagosome formation. Transport proteins such as ATG9, ATG2, and ATG18 are involved in lipid transfer, supporting the expansion of the autophagosome. Additionally, the protein WD Repeat Domain Phosphoinositide Interacting Proteins 1/2 (WIPI1/2) plays a crucial role in recruiting downstream ATG proteins, while Double FYVE Domain Containing Protein 1 (DFCP1) promotes isolation membrane formation. P62 acts as an autophagy adaptor protein, binding to NCOA4 and ferritin to target them to the autophagosome. The ESCRT system assists in the closure of the autophagosome membrane during later stages. STX17 (Syntaxin 17) and SNAP29 (synaptosome associated protein 29) promote the fusion of autophagosomes with lysosomes, aided by Vesicle-Associated Membrane Protein (VAMP) seven and VAMP8 on the lysosome membrane. Ras-Related Protein 7 (Rab7) facilitates the maturation of autophagosomes and their fusion with lysosomes. Nuclear Factor Erythroid 2–Related Factor 2 (NRF2), a key regulator of oxidative stress, controls ferroptosis via HERC2 and VAMP8, influencing NCOA4 degradation and the fusion process. Disruption in ferritinophagy can lead to iron overload, generating reactive hydroxyl radicals, which can induce ferroptosis and disrupt cellular function.
Ferritinophagy is regulated by several factors that help maintain iron balance in the body. IRPs, such as IRP1 and IRP2, play a central role in controlling iron uptake, storage, and usage. When iron is abundant, IRP1 binds to Fe-S clusters, losing its ability to regulate ferritin production. However, in low iron conditions, IRP1 binds to iron-responsive elements (IREs) in ferritin mRNA, reducing ferritin synthesis to limit iron storage (Tong and Rouault, 2007). In contrast, IRP2 does not have Fe-S clusters. When iron is sufficient, IRP2 is degraded via the FBXL5-mediated ubiquitination pathway. Iron deficiency affects the stability of FBXL5, thereby enhancing the binding of IRP2 to IREs (Zhang et al., 2014; Sanchez et al., 2011). Additionally, the expression of NCOA4 is adjusted according to intracellular iron levels. When iron is sufficient, HERC2 recognizes NCOA4 and degrades it via ubiquitination to prevent iron toxicity. When iron is deficient, the expression of NCOA4 increases to promote ferritinophagy, thereby releasing more iron ions (Mancias et al., 2015). Besides, there are two classic pathways that respond to external nutrients and energy: the mTOR signaling pathway and the AMPK pathway. The mTOR pathway remains active when nutrients are sufficient, inhibiting autophagy, while the AMPK pathway acts in the opposite manner (Bao et al., 2022).
Upon receiving activation signals, the ULK complex (including proteins like ULK1/2 and ATG13) recruits and activates the PI3K complex (composed of VPS34, ATG14L, VPS15, and Beclin1) to generate phosphatidylinositol-3-phosphate (PtdIns3P). PtdIns3P accumulates at the autophagy initiation site, the isolation membrane (IM), and recruits other effector proteins, such as DFCP1 and WIPI1/2 (23, 24). Among them, DFCP1 can promote the formation and expansion of the isolation membrane (Nähse et al., 2024), WIPI1/2 act as effector proteins of PtdIns3P and are crucial for recruiting downstream ATG proteins (Gaugel et al., 2012). WIPI2’s primary function is to recruit the ATG16L complex (ATG5-ATG12-ATG16L). The ATG16L complex acts as an E3 enzyme and facilitates the lipidation of microtubule-associated proteins 1A/1B light chain 3 (LC3), converting it to LC3-II. And LC3 is one of the most important markers in the autophagy process, it integrates into the IM like bricks, providing essential structural support for the formation of the autophagosome. Additionally, the ATG16L complex can help in the expansion and closure of the IM membrane by affecting membrane curvature (Wilson et al., 2014; Noda and Yoshimori, 2009; Nakatogawa, 2013). How is the ATG16L complex formed? The process is as follows: First, ATG12 is activated through a process that requires ATP and interacts with other proteins like ATG7 and ATG10. This activation is essential for the formation of the autophagosome. Subsequently, under the catalysis of ATG10, ATG12 is covalently linked to ATG5, forming the ATG12-ATG5 covalent complex. Finally, the ATG12-ATG5 complex binds with ATG16L1 to form the final complex (Mizushima, 2020). It is also noteworthy that before being acted upon by E3 ligase, LC3 must first be cleaved at its C-terminus by ATG4, exposing a glycine residue that can conjugate with phosphatidylethanolamine (PE). Subsequently, LC3 is activated by ATG7 (E1) and ATG3 (E2), and finally conjugates with PE (29, 31).
In addition to the proteins involved in forming the autophagic membrane, certain transport proteins are essential for providing the necessary lipids and other materials. ATG9 is a membrane protein that helps transfer lipids and proteins from organelles like the endoplasmic reticulum and Golgi apparatus to the site of autophagosome formation, ensuring the membrane can expand. ATG2 also aids in this process by facilitating lipid transfer between the endoplasmic reticulum and the autophagic membrane. Together, ATG2 and ATG18 help recruit lipids to the membrane, which is crucial for the expansion of the autophagosome (Obara et al., 2008; Osawa and Noda, 2019; He and Klionsky, 2007).
Ferritinophagy, as a specific form of autophagy, relies on the precise recognition of ferritin. NCOA4 is the key regulatory factor in this process. It binds to ferritin and directs it to the IM. Once the autophagosome matures and fuses with the lysosome, ferritin is degraded, releasing iron ions. These ions are then transported out of the cell by ferroportin1 (FPN1), participating in systemic iron metabolism. It is also important to note that intracellular iron uptake requires another transporter, Divalent Metal Transporter 1 (DMT1), and iron transport mediated by DMT1 is typically facilitated through the formation of endosomes (Yanatori and Kishi, 2019; Ajoolabady et al., 2021; Anderson and Frazer, 2017). In this process, P62/SQSTM1 acts as an autophagy adaptor protein, regulating NCOA4 and inducing the localization of the NCOA4-ferritin complex to the autophagosome (Liu et al., 2023). In the later stages of autophagy, the ESCRT system assists in the closure of the autophagosome membrane, enabling the autophagosome to mature into a complete vesicle (Lefebvre et al., 2018). STX17 and SNAP29, which are localized on the autophagosome, can also promote the fusion of autophagosomes and lysosomes by forming a complex with VAMP7/8, which is located on the lysosome (Tian et al., 2021). Meanwhile, Rab7 can also promote the maturation of autophagosomes and their fusion with lysosomes by interacting with effector proteins (Ao et al., 2014). The transcription factor nuclear factor erythroid 2–related factor 2 (NRF2) is primarily responsible for regulating the cellular antioxidant stress response. Under normal conditions, NRF2 binds to Keap1, which promotes NRF2 ubiquitination, thus maintaining its low levels within the cell. When cells are exposed to oxidative stress or toxic substances, the structure of Keap1 changes, allowing NRF2 to be released and translocate into the nucleus. Once in the nucleus, NRF2 binds to the antioxidant responsive element (ARE), initiating the transcription of antioxidant and detoxifying genes (Fan et al., 2017). Anandhan et al. discovered that NRF2 can regulate ferroptosis through HERC2 and VAMP8. HERC2 is an E3 ubiquitin ligase for NCOA4, facilitating its degradation, while VAMP8 plays a role in the fusion of autophagosomes and lysosomes (Anandhan et al., 2023).
Ferritinophagy in biochemical factor-induced liver injury
With the rapid progress of urbanization and industrialization, heavy metal pollution has become an increasingly severe issue. These metals pose a significant threat to public health, as many can induce liver damage through ferritinophagy. For instance, cadmium (Cd), a high-risk heavy metal, is prominently used in industries such as metallurgy, mining, battery manufacturing, pigments, and plastics (Wang M. et al., 2021). Research has shown that Cd induces liver damage in a dose-dependent manner. The underlying mechanism is closely linked to the ER stress-ferritinophagy axis. Intervening with an ER stress inhibitor, such as GSK, can significantly inhibit ferritinophagy in cells exposed to Cd, thereby alleviating ferroptosis (He et al., 2022). In addition, As is also a common chemical pollutant. It poses serious health risks to humans through food chain transmission and bioaccumulation (Clemens and Ma, 2016). Although the toxicity of As is not as high as that of heavy metals like Cd, lead, or mercury, its widespread presence in the environment poses significant risks. As can enter the human body through drinking water, food, air, and occupational exposure, making it more frequently encountered than other heavy metals. Consequently, the Agency for Toxic Substances and Disease Registry (ATSDR) has placed arsenic at the top of its Substance Priority List (Shakya et al., 2023; Chen and Costa, 2021; Garbinski et al., 2019). Recent reports have increasingly highlighted the hepatotoxic effects of arsenic. Wu and colleagues have found that extended exposure to low levels of As compounds can result in liver fibrosis (Wu M. et al., 2023). Wang et al. found that long-term consumption of drinking water containing inorganic arsenic (iAs) increases the risk of liver cancer (Wang et al., 2014). Additionally, As has been shown to induce the accumulation of intracellular ROS and trigger ferroptosis (Gao et al., 2024). However, it remains unclear whether chronic As exposure induces ferroptosis by enhancing hepatic ferritinophagy. To investigate this, Yu et al. conducted a study and discovered that chronic arsenic exposure activates the AMPK/mTOR/ULK1 axis, triggering ferritinophagy-mediated ferroptosis and leading to liver damage (Yu L. et al., 2023). Another heavy metal commonly encountered in daily life is cuprum (Cu). The widespread misuse of cuprum-based fungicides and fertilizers in agriculture, along with the indiscriminate discharge of industrial wastewater, has led to increasing soil contamination with cuprum (Li et al., 2020). The hepatotoxic mechanisms of cuprum are complex and multifaceted. On one hand, cuprum induces oxidative stress through a mitochondrial-mediated caspase-dependent pathway (Yang et al., 2019), On the other hand, it can cause mitochondrial autophagy through the PINK1/Parkin pathway (Yang et al., 2021), Additionally, it promotes ferroptosis by inducing NCOA4-mediated ferritinophagy, inhibiting the antioxidant stress signaling pathway NRF2/Keap1, and suppressing Ferroptosis Suppressor Protein 1 (FSP1) (Zhong et al., 2024).
Silica nanoparticles (SiNPs) are inorganic materials with unique physicochemical properties. Due to these properties, they are widely used in various fields, including biomedicine, chemical engineering, pharmaceuticals, and cosmetics, playing a crucial role in modern technology (Huang et al., 2022; Wang et al., 2018). According to the ‘Guidelines on Protecting Workers from Potential Risks of Engineered Nanomaterials’ published by the World Health Organization, SiNPs are the second most produced engineered nanomaterials globally, with an annual production reaching up to 1.5 million tons (WHO Guidelines Approved by the Guidelines Review Committee, 2017). With the widespread use of SiNPs, the potential for human exposure has increased, drawing significant attention from researchers and regulatory agencies. Previous studies have demonstrated that SiNPs can induce autophagy in various cell lines (Ma et al., 2022; Zhu Y. et al., 2023). A 2018 study found that silica nanoparticles can mediate autophagy in hepatocytes through the EIF2AK3 and ATF6 UPR pathways (Wang et al., 2018). Building on this, Liang et al. found through both in vivo and in vitro experiments that prolonged exposure to SiNPs exacerbates liver fibrosis, with the underlying mechanism involving NCOA4-mediated ferritinophagy. Furthermore, upon cessation of SiNPs exposure, the activation of ferritinophagy decreased, thereby alleviating the severity of liver fibrosis (Liang et al., 2023). Deoxynivalenol (DON), also known as vomitoxin, is a mycotoxin produced by certain Fusarium species, particularly Fusarium graminearum and Fusarium culmorum. These fungi commonly infect essential cereal crops such as wheat, barley, rye, oats, and maize, posing a significant challenge to public food safety (Liao et al., 2018). The liver, being the most important detoxification organ in the body, is inevitably damaged by the metabolism of most mycotoxins. In 2020, Peng et al. demonstrated that prolonged exposure to DON impairs liver function, with molecular-level observations showing significant increases in both apoptosis and autophagy in hepatocytes (Peng et al., 2020). Jiang et al. further explored the connection between ferritinophagy and DON-induced liver injury. They found that DON promotes autophagy by activating the expression of mammalian target of rapamycin complex 1 (mTORC1). Additionally, DON enhances the phosphorylation of NCOA4 through ataxia-telangiectasia mutated kinase (ATM), increasing its affinity for ferritin and thereby promoting ferritinophagy and ferroptosis (Jiang et al., 2024a). Recent research has proposed a potential therapeutic approach for DON-induced liver injury: glycyrrhetinic acid (GA) can bind to programmed cell death protein 4 (PDCD4) to inhibit its ubiquitination and degradation. The stabilization of PDCD4 downregulates NCOA4 expression through the JNK-Jun-NCOA4 axis, thereby inhibiting ferritinophagy and effectively mitigating liver injury induced by DON(64).
Ferritinophagy in drug-induced acute liver injury
Drug-Induced Liver Injury (DILI) is a common liver disease in clinical practice. In severe cases, it can lead to liver failure and even be life-threatening. Notably, in Western societies, DILI is the leading cause of acute liver failure (Katarey and Verma, 2016). Specifically, one of the major causes of DILI is acetaminophen (APAP) overdose. As one of the most widely used analgesic and anti-inflammatory drugs globally, APAP has been beneficial to patients around the world. Excessive consumption, on the other hand, can lead to severe liver damage (Ramachandran and Jaeschke, 2019). The exact mechanism by which APAP impacts liver function remains unclear. Existing research indicates that APAP produces a toxic metabolite, N-acetyl-p-benzoquinone imine (NAPQI). Normally, NAPQI is neutralized and rendered non-toxic by binding with glutathione (GSH). When NAPQI accumulates excessively in the liver, it leads to GSH depletion, contributing to liver damage (Nguyen and Stamper, 2017). GSH, as a crucial antioxidant in cells, plays a key role in preventing lipid peroxidation. When GSH is depleted, the levels of ROS in the cell increase, leading to lipid peroxidation and ultimately resulting in ferroptosis (Niu et al., 2021). Researchers have explored this issue by synthesizing a novel antioxidant enzyme nanomaterial—flower-shaped MnO₂ nanoparticles (MnO₂Nfs). These nanoparticles effectively improve acute liver injury induced by APAP. They not only possess various antioxidant enzyme activities to reduce ROS generation but also inhibit ferritinophagy by suppressing FTH/L degradation and LC3-II expression (Wu et al., 2024). Similarly, Shan and colleagues utilized manganese to create poly (acrylic) acid-coated Mn₃O₄ nanoparticles (PAA@Mn₃O₄-NPs, PMO). This material demonstrates antioxidant properties and good biocompatibility, allowing for liver accumulation and presence in lysosomes. By eliminating ROS, it inhibits ferritinophagy and counters acute liver injury induced by APAP (70).
Another noteworthy drug is methotrexate (MTX). As a folate antagonist, MTX inhibits dihydrofolate reductase (DHFR) to block the synthesis of DNA, RNA, and proteins. Low doses (5–25 mg/week) of MTX are first-line treatments for rheumatoid arthritis and other inflammatory joint diseases, while high doses, typically defined as 500 mg/m2, are commonly used to treat various malignant tumors (Pivovarov and Zipursky, 2019; Howard et al., 2016). However, MTX’s hepatotoxicity has long been a concern for clinicians. Although recent studies suggest that the risk of MTX-induced liver fibrosis might be overestimated (Andrade and Björnsson, 2023), there is broad consensus that long-term use of MTX can lead to liver damage (Ezhilarasan, 2021). Wang and colleagues investigated the mechanism of MTX-induced hepatotoxicity and found that MTX triggers ferritinophagy mediated by NCOA4 within liver cells through high-mobility group box 1 (HMGB1), ultimately leading to liver damage. Additionally, they discovered that GA, an HMGB1 inhibitor, can effectively alleviate MTX-induced liver injury (Wang C. et al., 2024).
Tuberculosis (TB) is a common infectious disease caused by Mycobacterium TB and is a leading cause of death from infectious diseases among adults worldwide. The standard treatment regimen typically involves a 6-month multi-drug therapy, which commonly includes isoniazid (INH), rifampin (RIF), pyrazinamide (PZA), and ethambutol (EMB) (Furin et al., 2019). During anti-TB treatment, one of the most frequent adverse reactions is anti-TB drug-induced liver injury. Studies have shown that RIF, INH, and PZA can induce hepatotoxicity through mitochondrial autophagy (Elmorsy et al., 2017). Zhou and colleagues analyzed RIF-induced liver toxicity from the perspective of ferritinophagy. They found that RIF exacerbates liver damage by inhibiting the expression of Human 71 kDa heat shock cognate protein (HSPA8), which increases ferritinophagy (Zhou et al., 2022).
Ferritinophagy in liver fibrosis
Liver fibrosis is a pathological process in which normal liver tissue is gradually replaced by fibrous tissue (scar tissue) due to chronic injury. The development of liver fibrosis involves several key steps: 1. Chronic liver damage from various causes, such as hepatitis viruses, alcoholic hepatitis, and NAFLD. 2. Inflammatory cells release cytokines and chemical signals that promote the activation of HSCs. 3. Activated HSCs transform into myofibroblast-like cells and begin to synthesize and secrete large amounts of extracellular matrix (ECM), including collagen. 4. As fibrous tissue accumulates in the liver, the liver’s structure is progressively destroyed, leading to a decline in liver function (Aydın and Akçalı, 2018; Roehlen et al., 2020).
Previously, liver fibrosis was generally regarded as a passive and irreversible process. However, current understanding suggests that early-stage liver fibrosis can be slowed, arrested, or even reversed with prompt and suitable intervention. Once fibrosis advances to late-stage cirrhosis, reversal becomes highly challenging, and therapeutic options are significantly constrained. In such advanced cases, liver transplantation may be the only effective recourse (Aydın and Akçalı, 2018; Bataller and Brenner, 2005). The activation of HSCs is a hallmark event in liver fibrosis. Regulating HSC activation could enable precise antifibrotic therapies, offering the potential to effectively treat liver fibrosis (Higashi et al., 2017).
Kong et al. discovered that artesunate, a stable derivative of artemisinin, can promote ferritinophagy in activated HSCs in vitro, thereby inhibiting the progression of liver fibrosis. PCR results indicated that artesunate can enhance the expression of several ferritinophagy-related genes, including Atg3, Atg5, Atg6/beclin1, Atg12, and LC3 (17). Another compound, Oroxylin A (OA), extracted from the traditional Chinese herb Scutellaria baicalensis, was shown by Sun and colleagues to mediate ferritinophagy through the cGAS-STING pathway, inducing HSC senescence and ultimately inhibiting liver fibrosis. In their experiments, they found that increasing doses of OA led to a corresponding increase in NCOA4 expression, while the expression of FTH1 decreased. FTH is a crucial protein responsible for storing and regulating iron ions within cells. A decrease in FTH1 implies an increase in intracellular free iron ions, which typically leads to increased ferritinophagy. Additionally, autophagy markers and initiators such as LC3 and Beclin1 showed dose-dependent expression increases. This indicates that OA can induce ferritinophagy in HSCs. Furthermore, when HSCs were treated with NCOA4 siRNA, the expression of senescence markers (p16, p21, and HMGA1) was significantly downregulated compared to the control group, leading to the conclusion that OA regulates HSC senescence through ferritinophagy. Subsequently, using cGAS siRNA to inhibit the cGAS-STING pathway in HSCs resulted in decreased expression of NCOA4, LC3, and Beclin1, along with reduced intracellular ROS levels and iron ion content. This demonstrated that the effect of OA on ferritinophagy in HSCs is regulated by the cGAS-STING pathway (Sun et al., 2023). Schisandra chinensis, another traditional Chinese herb, has recently been found to possess various biological activities, including antioxidant, anti-inflammatory, hepatoprotective, and anti-tumor properties (Zhang W. et al., 2020). Recent studies have shown that Schisandrin B, an active compound extracted from Schisandra chinensis, inhibits the activation of HSCs and induces macrophage polarization, thereby alleviating liver fibrosis (Chen et al., 2017; Chen et al., 2021). Ma et al. studied Schisandrin B from the perspective of HSCs senescence. They found that Schisandrin B can regulate ferritinophagy mediated by NCOA4 in HSCs, inducing iron overload and subsequent oxidative stress. This promotes the senescence of activated HSCs, ultimately improving the degree of liver fibrosis (Ma et al., 2023). Taurine, one of the most abundant amino acids in mammals, has a protective effect against liver fibrosis caused by cellular oxidative stress (Miyazaki et al., 2005). Although Li et al. did not conduct NCOA4 knockout and overexpression experiments to confirm the relationship between taurine and ferritinophagy, their bioinformatics analysis revealed a strong molecular docking interaction between taurine and NCOA4, suggesting that taurine may potentially target NCOA4-mediated ferritinophagy, thereby promoting ferroptosis in HSCs and reducing ECM deposition (Li et al., 2024). Future researchers could use this as a starting point to further investigate and elucidate the underlying mechanisms.
In addition to the natural compounds mentioned above, research in molecular biology has also explored this area. BECN1 was discovered as early as 2018 to promote lipid peroxidation and iron death by inhibiting the important regulatory system of iron death, system xc−(90). Zhang et al. found that the RNA-binding protein ELAVL1/HuR can bind to the 3′untranslated region of BECN1 mRNA, increasing its stability and thereby activating ferritinophagy to induce ferroptosis in HSCs. Additionally, sorafenib (SF) has been shown to upregulate ELAVL1 expression, which alleviates the severity of liver fibrosis. This suggests a potential therapeutic pathway where SF modulates ferroptosis-related pathways to mitigate liver damage (Zhang et al., 2018). Mesenchymal Stem Cells (MSCs) are recognized for their pluripotency, immune regulation, and self-renewal capabilities, which suggest their potential clinical application value. Previous studies have demonstrated that MSCs and their derived exosomes (MSC-ex) have shown considerable abilities in inhibiting liver fibrosis, acute liver injury, and acute liver failure (Shokravi et al., 2022; Shao et al., 2020; Li et al., 2013). After a deeper understanding of MSCs’ mechanisms, Tan and colleagues found that MSC-ex promotes the expression of BECN1 to increase intracellular LC3 and Fe2+ levels, thereby inducing ferritinophagy and promoting HSCs iron death to reduce liver fibrosis (Tan et al., 2022). On the other hand, another RNA-binding protein, ZFP36/TTP, exhibits a contrasting role. It binds to ATG16L1 mRNA, promoting its degradation and thus leading to inactivation of ferritinophagy in HSCs and resulting in resistance to ferroptosis (Zhang Z. et al., 2020).
Ferritinophagy in liver cancer
HCC is the most common type of primary liver cancer. According to the Global Cancer Statistics 2020, HCC ranks sixth in global cancer incidence and third in cancer-related mortality (Sung et al., 2021). While SF is a first-line treatment for advanced HCC, it offers limited improvement in patient survival, and many HCC patients develop adaptive resistance to SF(98, 99). Therefore, finding suitable adjunctive drugs to enhance the efficacy of SF may be highly beneficial for HCC patients.
Caryophyllene Oxide, a naturally occurring sesquiterpene compound, possesses various biological activities. Historically used in cosmetics and food additives for its unique aroma, recent studies have highlighted its significant antitumor and analgesic properties (Fidyt et al., 2016; Kim et al., 2014). Research in 2019 demonstrated that β-Caryophyllene Oxide could indirectly increase the sensitivity of HCC to SF, enhancing its cytotoxic effects (Di Giacomo et al., 2019). Further investigation by Xiu and colleagues explored the association between Caryophyllene Oxide and ferritinophagy in HCC cells. They found that Caryophyllene Oxide could trigger ferritinophagy and mediate ferroptosis in HCC cells by increasing the expression levels of NCOA4 and LC3Ⅱ, while decreasing FTH1 expression. This led to a significant reduction in liver tumor volume, suggesting that Caryophyllene Oxide could be a potential therapeutic agent for HCC(103). Additionally, Artesunate, recognized for its ability to induce ferritinophagy, is considered an ideal adjunctive therapy. Li and colleagues investigated the combined effects of Artesunate and SF on HCC cells, finding that the combination treatment enhanced mitochondrial damage, lysosomal activation, and ferritinophagy, ultimately leading to ferroptosis. These findings highlight the potential value of the synergistic effects between artemisinin derivatives and SF in HCC treatment, suggesting that this combination could offer an innovative and clinically promising strategy for managing HCC(104).
Esculetin, a natural dihydroxycoumarin commonly derived from the bark of Fraxinus chinensis Roxb, has garnered significant research interest due to its diverse pharmacological activities, including antioxidant, anti-inflammatory, antitumor, and antibacterial effects (Garg et al., 2022). As early as 2015, studies demonstrated that esculetin could induce ROS accumulation and apoptosis in gastric cancer cells through a caspase-dependent pathway, achieving its antitumor effects (Pan et al., 2015). However, research on esculetin’s role in inducing ferritinophagy in HCC cells is limited. Xiu et al. investigated this aspect and found that, similar to Caryophyllene Oxide, esculetin can regulate ferritinophagy through the NCOA4/LC3-II/FTH1 signaling pathway. This regulation helps suppress HCC cell proliferation and differentiation (Xiu et al., 2023). Eupatorium chinense L., a traditional Chinese medicinal herb, is known for its antitumor, antibacterial, and anti-inflammatory properties. Zhu and colleagues extracted a sesquiterpene from Eupatorium chinense L. and discovered that this compound can upregulate NCOA4 expression to mediate ferritinophagy and disrupt mitochondrial function, thereby enhancing HCC cell apoptosis (Zhu ZH. et al., 2023).
Research from the molecular biology perspective has provided valuable insights into the treatments of liver cancer. Polypyrimidine Tract Binding Protein 1 (PTBP1) is a crucial RNA-binding protein with significant regulatory roles across various systems, including the nervous, immune, and cardiovascular systems, as well as in tumors (Yu Q. et al., 2023). Yang and colleagues discovered that PTBP1 can promote ferritinophagy in HCC cells by binding to NCOA4 mRNA, which enhances the sensitivity of HCC cells to ferroptosis following SF treatment (Yang et al., 2023). Additionally, Wang and colleagues found that in HCC cell models, activators of ferroptosis (such as SF, erastin, and sulfasalazine) induce ferritinophagy, leading to the activation of AMPK phosphorylation. BCAT2 is a key enzyme in glutamate production, and the function of system Xc–is directly regulated by glutamate levels. AMPK/SREBP1 signaling pathway inhibits the nuclear translocation of the transcription factor SREBP1 and suppresses the transcription of BCAT2. Inhibition of BCAT2 results in decreased intracellular glutamate, causing reduced cystine uptake and thereby inducing ferroptosis in tumor cells (Wang K. et al., 2021).
Ferritinophagy in Non-Alcoholic fatty liver disease
Non-Alcoholic Fatty Liver Disease (NAFLD) is characterized by the accumulation of fat in the liver without the involvement of excessive alcohol consumption. As one of the most prevalent chronic liver diseases worldwide, NAFLD’s high prevalence is closely linked to modern dietary and lifestyle habits. Metabolic syndrome (MS) is a key risk factor for NAFLD, encompassing conditions such as obesity, hypertension, hyperglycemia, and hyperlipidemia (Friedman et al., 2018; Huang, 2009). Research and epidemiological studies have demonstrated that dysregulation of iron homeostasis, leading to iron overload, plays a significant role in the development of NAFLD (114). Kobi et al. discovered that iron overload can promote the nuclear translocation of transcription factor EB (TFEB) in NAFLD, which enhances lysosomal expression and increases the occurrence of ferritinophagy, and ultimately aggravating the severity of disease (Honma et al., 2023). Ferritinophagy plays a critical role in releasing iron from ferritin stores, aiding in the oxidative desaturation of fatty acids and the synthesis of triglycerides and cardiolipins, which helps mitigate lipotoxicity. This process plays a critical role in cellular metabolism, ensuring that iron is available for key biochemical pathways. While beneficial in the short term by preventing the accumulation of toxic lipid intermediates, chronic induction of ferritinophagy can have detrimental effects. Over time, the sustained mobilization of iron may lead to excessive iron accumulation in the liver, promoting oxidative stress and inflammation. This contributes to the development of hepatic steatosis, a condition characterized by the abnormal retention of fat in liver cells, and can progress to more severe liver diseases such as non-alcoholic steatohepatitis (NASH), fibrosis, and ultimately cirrhosis. Therefore, while ferritinophagy is a vital adaptive response to metabolic stress, its persistent activation underscores the delicate balance required to maintain liver health and prevent long-term hepatic damage (Li et al., 2022).
Insulin resistance, characterized by the reduced sensitivity of the body to insulin and the diminished ability to promote glucose uptake and utilization (Samuel and Shulman, 2016), is a common feature of metabolic disorders. The prevailing view is that insulin resistance and the resulting hyperinsulinemia are key factors in the pathogenesis of NAFLD (117). Prolonged high-fat diets have been found to inhibit ferritinophagy, disrupting iron homeostasis, leading to endoplasmic reticulum stress, and ultimately mediating the development of insulin resistance. This may explain why individuals who excessively consume high-fat foods are more prone to NAFLD (118). Chronic intermittent hypobaric hypoxia (CIHH) refers to a treatment method that induces adaptation and enhances tolerance to hypoxic environments through intermittent exposure to low oxygen conditions. Although CIHH has shown potential in some studies and experiments, its widespread clinical use requires further research and validation (Zhang et al., 2020c). Cui and colleagues discovered a link between CIHH and ferritinophagy. They found that CIHH treatment in MS rats upregulated the expression of NCOA4, promoting ferritinophagy and the expression of FPN, thereby reducing oxidative stress and iron overload. This ultimately inhibited hepatocyte ferroptosis and alleviated NAFLD (114). Insulin Growth Factor Binding Protein 7 (IGFBP7) is a protein that regulates the activity of insulin-like growth factors (IGF), which are crucial for cell growth, differentiation, and metabolism. IGFBP7 modulates the biological functions of IGF by binding to it (Jin et al., 2020). Previous studies have found that IGFBP7 exacerbates hepatic steatosis in the development of NAFLD, although the exact mechanisms remain unclear (Yan et al., 2019). Wang and colleagues conducted further research and discovered that IGFBP7 promotes the progression of NAFLD by inducing ferroptosis through NCOA4-mediated ferritinophagy. Depletion of IGFBP7 effectively improved liver inflammation, fibrosis, and steatosis (Wang et al., 2023).
Ferritinophagy in sepsis-associated liver injury
Sepsis is characterized by a systemic infection and a strong inflammatory response, potentially leading to multiple organ dysfunction or failure, and even death. The liver, as a critical metabolic and detoxification organ, is a primary target of sepsis-associated damage. Liver dysfunction frequently occurs in the early stages of sepsis and is often considered a marker of poor prognosis. Understanding the underlying mechanisms of sepsis-induced liver injury is essential for developing effective therapeutic strategies (Yan et al., 2014).
YAP1 (Yes-associated protein 1) and TAZ (WW domain-containing transcription regulator 1) are important downstream effectors of the Hippo signaling pathway, which regulates gene expression through transcription factors. The Hippo/YAP1 pathway plays significant roles in tissue growth, organ size regulation, and cancer development (Panciera et al., 2017). Additionally, there is evidence that YAP1 has positive effects in liver-related diseases, such as promoting liver regeneration and repair and inhibiting liver fibrosis (Liu et al., 2019; Wang et al., 2020). Wang et al. discovered that in a sepsis-induced liver injury model, YAP1 can inhibit NCOA4-mediated ferritinophagy, thereby suppressing the expression of SFXN1 (a mitochondrial membrane iron transport protein). This inhibition prevents ferroptosis in hepatocytes and effectively alleviates liver damage (Wang et al., 2022). Similarly, Qi et al. observed comparable effects in a NAFLD model. They found that curcumol can reduce NCOA4 expression through YAP, regulating ferritinophagy and ultimately preventing hepatocyte senescence (Qi et al., 2021). There are few studies on ferritinophagy and sepsis-associated liver injury, but based on literature review, I believe the following targets may be related to ferritinophagy: GPR116, an adhesion G protein-coupled receptor (GPCR), can inhibit key ferroptosis targets such as system Xc, GSH, and GPX4, inducing sepsis-related liver injury (Yan et al., 2014). GLI Family Zinc Finger 2 (GLI2) is a key regulator in the Hedgehog (Hh) signaling pathway, which plays an important role in tissue development during embryogenesis and is thought to promote liver regeneration (Omenetti et al., 2011), Sun et al. found that GLI2 exacerbates sepsis-related liver injury by regulating the expression of synovial apoptosis inhibitor 1 (SYVN1), which inhibits PPARα-mediated autophagy (Sun et al., 2024). Hypoxia-Inducible Factor 1-Alpha (HIF-1α) is a transcription factor induced under hypoxic conditions. Several studies have shown that HIF-1α can inhibit ferritinophagy under hypoxia, protecting cells from ferroptosis (Ni et al., 2021; Wang Y. et al., 2024). In sepsis-associated liver injury, Zhu et al. discovered that fibroblast growth factor 21 (FGF21) can promote HIF-1α expression, effectively suppressing macrophage activation and reducing inflammation after liver injury. However, whether ferritinophagy is involved in this process requires further investigation (Zhu et al., 2024). While analyzing recurrently dysregulated genes in sepsis, Deng et al. discovered that the long non-coding RNA Mir22 hg contributes to ferritinophagy-mediated ferroptosis by recruiting the m6A reader YTHDC1 and stabilizing Angptl4 mRNA. This finding suggests that Mir22 hg could serve as a potential therapeutic target for treating sepsis through the modulation of ferroptosis (Deng et al., 2024).
At the end of this article, we provide a summary of the role of ferritinophagy in various liver diseases, as well as its regulatory factors. To facilitate comprehension, we have compiled (Table 1), which lists factors that can exacerbate liver diseases through ferritinophagy related pathways, and (Table 2), which lists factors that may ameliorate liver diseases through these pathways.
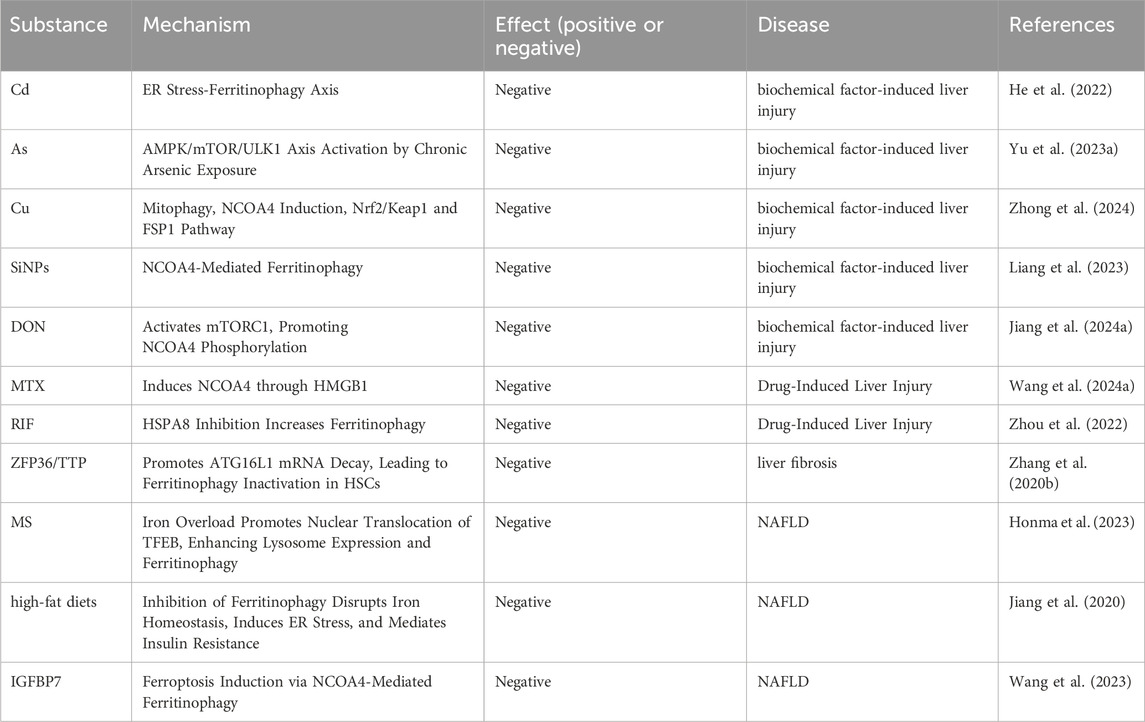
Table 1. Regulatory core substances participate in the aggravation of liver diseases by regulating ferritinophagy.
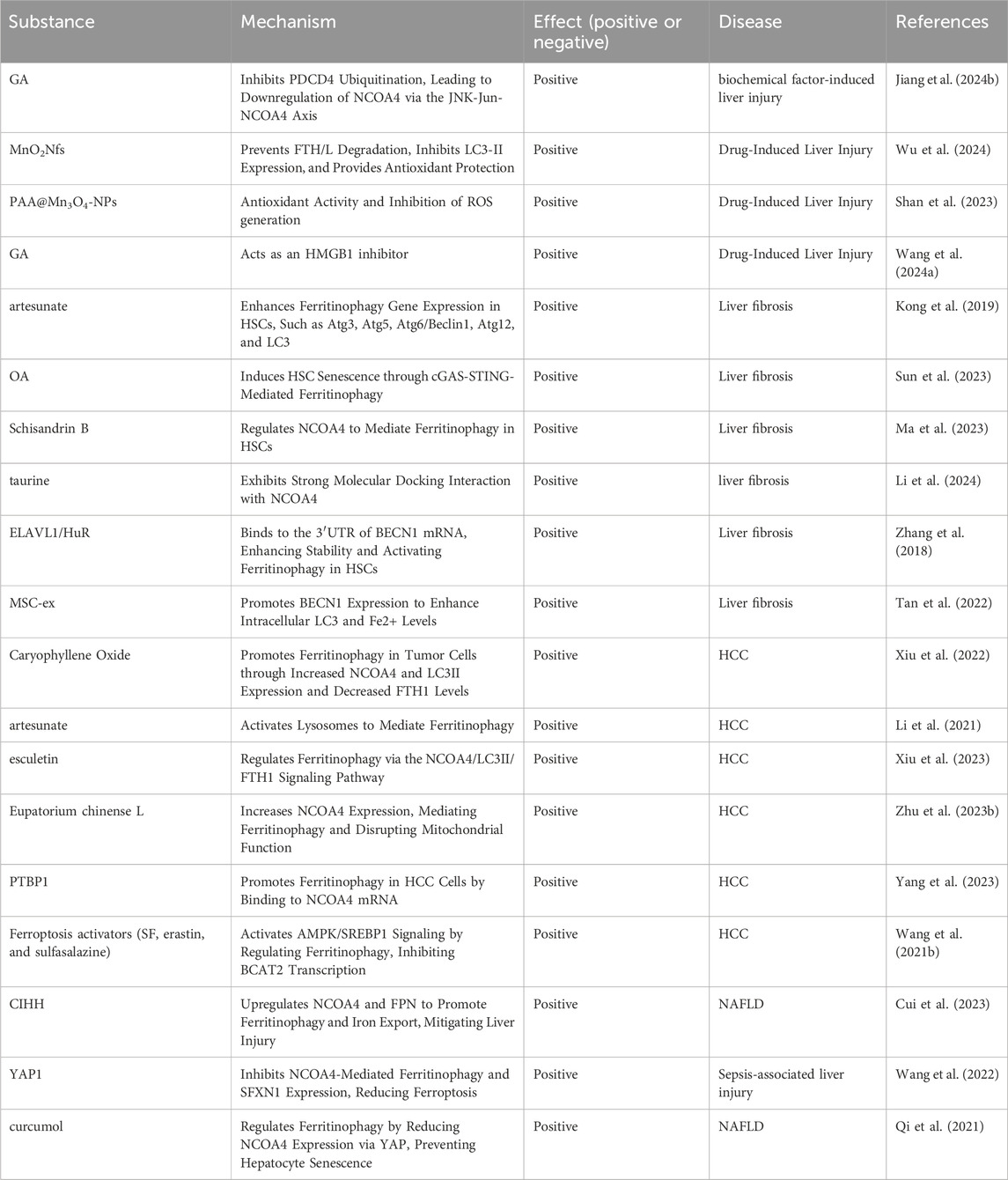
Table 2. Regulatory core substances participate in the improvement of liver diseases by regulating ferritinophagy.
Interactions of ferritinophagy with other forms of selective autophagy
Ferritinophagy is not an isolated process, it interacts with other forms of selective autophagy, such as mitophagy (mitochondrial autophagy) and lipophagy (lipid droplet autophagy). These interactions play a critical role in maintaining cellular homeostasis and can significantly impact disease progression. Under conditions of oxidative stress, ferritinophagy is upregulated to release iron for essential metabolic processes. However, excessive iron release, through the Fenton reaction, leads to the generation of ROS, which in turn causes a reduction in mitochondrial membrane potential and mitochondrial dysfunction. This dysfunction activates the PINK1/Parkin signaling pathway to inhibit mitophagy (Zhang et al., 2020d). Furthermore, iron overload activates autophagy-related signaling pathways by generating ROS and increasing intracellular calcium levels. Concurrently, lipophagy, mediated by specific receptors such as Rab10 and DNM2, promotes ferroptosis, thereby exacerbating the pathological progression of non-alcoholic steatohepatitis (Honma et al., 2023). Understanding the complex crosstalk between ferritinophagy and other autophagy pathways is essential for the development of comprehensive therapeutic strategies. Future research should focus on elucidating these interactions and exploring the potential for combined therapeutic approaches targeting multiple autophagy pathways to better manage NASH and related diseases.
Discussion on the clinical application prospects of traditional Chinese medicines
Several Traditional Chinese Medicines (TCMs) have been reported to modulate ferritinophagy, suggesting their potential therapeutic applications in liver diseases. For example, artesunate (a derivative of artemisinin) and Oroxylin A (extracted from Scutellaria baicalensis) have been shown to induce ferritinophagy in HSCs, thereby mitigating liver fibrosis. Another compound, Schisandrin B from Schisandra chinensis, has demonstrated potential in regulating ferritinophagy and alleviating liver fibrosis. While these compounds show promise in preclinical studies, none have been specifically approved for clinical use targeting ferritinophagy.
Future directions for drug discovery:
1. Target Identification and Validation: Future research should focus on identifying specific TCM compounds that modulate ferritinophagy and elucidate their mechanisms of action.
2. Clinical Trials: Conducting well-designed clinical trials to assess the safety and efficacy of TCMs in modulating ferritinophagy is essential for their approval and integration into clinical practice.
3. Combination Therapies: Exploring the combination of TCMs with conventional therapies (e.g., for cancer or fibrosis) may enhance therapeutic outcomes by targeting multiple pathways, including ferritinophagy.
4. Biomarker Development: Developing biomarkers to monitor ferritinophagy activity in clinical settings could facilitate the evaluation of TCM efficacy and guide personalized treatment strategies.
Conclusion
Ferritinophagy has garnered significant attention for its pivotal role in liver health and disease, influencing not only iron metabolism and cellular homeostasis but also various pathological liver conditions. Abnormal activation or inhibition of ferritinophagy is strongly linked to liver damage, fibrosis, and the development of cancer. Therefore, a comprehensive understanding of the mechanisms underlying ferritinophagy and its involvement in liver diseases is critical for the development of novel therapeutic strategies. Future research must focus on precisely regulating ferritinophagy to offer new insights into the prevention and treatment of liver diseases.
Despite promising findings in animal models, translating these results to clinical applications for human patients presents several challenges. First, significant physiological and pathological differences exist between animal models and humans. Liver metabolic pathways, the regulation of ferritinophagy, and drug responses can vary across species, meaning that dosages, mechanisms of action, and therapeutic effects effective in animals may not directly apply to humans. Moreover, human diseases are far more complex and heterogeneous than those observed in animal models. For example, the pathogenesis of NAFLD involves a range of factors, including genetic background, lifestyle, and metabolic syndrome components, which are difficult to fully replicate in animal models. Even when modulation of ferritinophagy shows positive effects in animals, the same therapeutic strategy may yield varying results in human patients due to individual variations.
Furthermore, drug safety and side effects pose critical challenges in the translational process. Drugs that appear safe in animal studies may lead to unpredictable side effects in human clinical trials, as they may interfere with other essential cellular processes while regulating ferritinophagy. This underscores the need for comprehensive toxicological evaluations in preclinical studies and close monitoring of patient responses during clinical trials.
Clinical trial design and execution also present significant hurdles. Recruiting the appropriate patient population, defining valid treatment endpoints, and ensuring ethical and scientific integrity require careful planning. For instance, in liver cancer trials, factors such as tumor stage, treatment history, and comorbidities must be considered to ensure the reliability of results.
In summary, while compelling evidence from biological models supports the potential role of ferritinophagy in liver disease treatment, substantial obstacles remain in translating these findings to human clinical practice. Ongoing research must continue to explore the mechanisms and regulation of ferritinophagy and its implications for human liver diseases, with a focus on optimizing clinical trial designs and ensuring the safety and efficacy of treatments for human patients.
Author contributions
KW: Data curation, Software, Writing–original draft. WZo: Data curation, Software, Writing–review and editing. ZH: Software, Writing–review and editing. WZn: Data curation, Writing–review and editing. LQ: Writing–original draft. JQ: Conceptualization, Investigation, Writing–review and editing. DW: Methodology, Writing–review and editing. LZ: Writing–review and editing. XX: Data curation, Writing–review and editing. DS: Writing–original draft.
Funding
The author(s) declare that financial support was received for the research, authorship, and/or publication of this article. This research was supported by Jiangsu Science and Technology Talent Promotion Project (TJ2022052), Soochow University Young Physician Scientist Program and Soochow University Affiliated First Hospital Fund, and Young Talent Development Plan of Changzhou Health Commission (CZQM2021028).
Acknowledgments
We would like to show sincere appreciation to the reviewers for their many useful comments on the early version of the manuscript.
Conflict of interest
The authors declare that the research was conducted in the absence of any commercial or financial relationships that could be construed as a potential conflict of interest.
Generative AI statement
The author(s) declare that no Generative AI was used in the creation of this manuscript.
Publisher’s note
All claims expressed in this article are solely those of the authors and do not necessarily represent those of their affiliated organizations, or those of the publisher, the editors and the reviewers. Any product that may be evaluated in this article, or claim that may be made by its manufacturer, is not guaranteed or endorsed by the publisher.
References
Ajoolabady, A., Aslkhodapasandhokmabad, H., Libby, P., Tuomilehto, J., Lip, G. Y. H., Penninger, J. M., et al. (2021). Ferritinophagy and ferroptosis in the management of metabolic diseases. Trends Endocrinol. Metab. 32 (7), 444–462. doi:10.1016/j.tem.2021.04.010
Anandhan, A., Dodson, M., Shakya, A., Chen, J., Liu, P., Wei, Y., et al. (2023). NRF2 controls iron homeostasis and ferroptosis through HERC2 and VAMP8. Sci. Adv. 9 (5), eade9585. doi:10.1126/sciadv.ade9585
Anderson, G. J., and Frazer, D. M. (2017). Current understanding of iron homeostasis. Am. J. Clin. Nutr. 106 (Suppl. 6), 1559s–66s. doi:10.3945/ajcn.117.155804
Andrade, R. J., and Björnsson, E. S. (2023). Liver fibrosis with methotrexate — an overestimated risk? Nat. Rev. Gastroenterology and Hepatology 20 (6), 347–348. doi:10.1038/s41575-023-00782-3
Ao, X., Zou, L., and Wu, Y. (2014). Regulation of autophagy by the Rab GTPase network. Cell Death Differ. 21 (3), 348–358. doi:10.1038/cdd.2013.187
Ashford, T. P., and Porter, K. R. (1962). Cytoplasmic components in hepatic cell lysosomes. J. Cell Biol. 12 (1), 198–202. doi:10.1083/jcb.12.1.198
Aydın, M. M., and Akçalı, K. C. (2018). Liver fibrosis. Turk J. Gastroenterol. 29 (1), 14–21. doi:10.5152/tjg.2018.17330
Bao, L., Zhao, C., Feng, L., Zhao, Y., Duan, S., Qiu, M., et al. (2022). Ferritinophagy is involved in Bisphenol A-induced ferroptosis of renal tubular epithelial cells through the activation of the AMPK-mTOR-ULK1 pathway. Food Chem. Toxicol. 163, 112909. doi:10.1016/j.fct.2022.112909
Bataller, R., and Brenner, D. A. (2005). Liver fibrosis. J. Clin. investigation 115 (2), 209–218. doi:10.1172/jci24282
Chen, Q., Bao, L., Lv, L., Xie, F., Zhou, X., Zhang, H., et al. (2021). Schisandrin B regulates macrophage polarization and alleviates liver fibrosis via activation of PPARγ. Ann. Transl. Med. 9 (19), 1500. doi:10.21037/atm-21-4602
Chen, Q., Zhang, H., Cao, Y., Li, Y., Sun, S., Zhang, J., et al. (2017). Schisandrin B attenuates CCl4-induced liver fibrosis in rats by regulation of Nrf2-ARE and TGF-β/Smad signaling pathways. Drug Des. Devel Ther. 11, 2179–2191. doi:10.2147/dddt.S137507
Chen, Q. Y., and Costa, M. (2021). Arsenic: a global environmental challenge. Annu. Rev. Pharmacol. Toxicol. 61, 47–63. doi:10.1146/annurev-pharmtox-030220-013418
Clemens, S., and Ma, J. F. (2016). Toxic heavy metal and metalloid accumulation in crop plants and foods. Annu. Rev. Plant Biol. 67, 489–512. doi:10.1146/annurev-arplant-043015-112301
Cui, F., Mi, H., Wang, R., Du, Y., Li, F., Chang, S., et al. (2023). The effect of chronic intermittent hypobaric hypoxia improving liver damage in metabolic syndrome rats through ferritinophagy. Pflugers Arch. 475 (11), 1251–1263. doi:10.1007/s00424-023-02860-6
Deng, W., Zhong, L., Ye, S., Luo, J., Ren, G., Huang, J., et al. (2024). Mir22hg facilitates ferritinophagy-mediated ferroptosis in sepsis by recruiting the m6A reader YTHDC1 and enhancing Angptl4 mRNA stability. J. Bioenerg. Biomembr. 56 (4), 405–418. doi:10.1007/s10863-024-10022-1
Deter, R. L., Baudhuin, P., and De Duve, C. (1967). Participation of lysosomes in cellular autophagy induced in rat liver by glucagon. J. Cell Biol. 35 (2), C11–C16. doi:10.1083/jcb.35.2.c11
Di Giacomo, S., Briz, O., Monte, M. J., Sanchez-Vicente, L., Abete, L., Lozano, E., et al. (2019). Chemosensitization of hepatocellular carcinoma cells to sorafenib by β-caryophyllene oxide-induced inhibition of ABC export pumps. Arch. Toxicol. 93 (3), 623–634. doi:10.1007/s00204-019-02395-9
Dixon, S. J., Lemberg, K. M., Lamprecht, M. R., Skouta, R., Zaitsev, E. M., Gleason, C. E., et al. (2012). Ferroptosis: an iron-dependent form of nonapoptotic cell death. Cell 149 (5), 1060–1072. doi:10.1016/j.cell.2012.03.042
Elmorsy, E., Attalla, S. M., Fikry, E., Kocon, A., Turner, R., Christie, D., et al. (2017). Adverse effects of anti-tuberculosis drugs on HepG2 cell bioenergetics. Hum. Exp. Toxicol. 36 (6), 616–625. doi:10.1177/0960327116660751
Ezhilarasan, D. (2021). Hepatotoxic potentials of methotrexate: understanding the possible toxicological molecular mechanisms. Toxicology 458, 152840. doi:10.1016/j.tox.2021.152840
Fan, Z., Wirth, A. K., Chen, D., Wruck, C. J., Rauh, M., Buchfelder, M., et al. (2017). Nrf2-Keap1 pathway promotes cell proliferation and diminishes ferroptosis. Oncogenesis 6 (8), e371. doi:10.1038/oncsis.2017.65
Fidyt, K., Fiedorowicz, A., Strządała, L., and Szumny, A. (2016). β-caryophyllene and β-caryophyllene oxide-natural compounds of anticancer and analgesic properties. Cancer Med. 5 (10), 3007–3017. doi:10.1002/cam4.816
Friedman, S. L., Neuschwander-Tetri, B. A., Rinella, M., and Sanyal, A. J. (2018). Mechanisms of NAFLD development and therapeutic strategies. Nat. Med. 24 (7), 908–922. doi:10.1038/s41591-018-0104-9
Furin, J., Cox, H., and Pai, M. (2019). Tuberc. Lancet. 393 (10181), 1642–1656. doi:10.1016/s0140-6736(19)30308-3
Gao, X., Su, Q., Pan, H., You, Y., Ruan, Z., Wu, Y., et al. (2024). Arsenic-induced ferroptosis in chicken hepatocytes via the mitochondrial ROS pathway. Biol. Trace Elem. Res. 202 (9), 4180–4190. doi:10.1007/s12011-023-03968-7
Garbinski, L. D., Rosen, B. P., and Chen, J. (2019). Pathways of arsenic uptake and efflux. Environ. Int. 126, 585–597. doi:10.1016/j.envint.2019.02.058
Garg, S. S., Gupta, J., Sahu, D., and Liu, C. J. (2022). Pharmacological and therapeutic applications of esculetin. Int. J. Mol. Sci. 23 (20), 12643. doi:10.3390/ijms232012643
Gaugel, A., Bakula, D., Hoffmann, A., and Proikas-Cezanne, T. (2012). Defining regulatory and phosphoinositide-binding sites in the human WIPI-1 β-propeller responsible for autophagosomal membrane localization downstream of mTORC1 inhibition. J. Mol. Signal 7 (1), 16. doi:10.1186/1750-2187-7-16
He, C., and Klionsky, D. J. (2007). Atg9 trafficking in autophagy-related pathways. Autophagy 3 (3), 271–274. doi:10.4161/auto.3912
He, Z., Shen, P., Feng, L., Hao, H., He, Y., Fan, G., et al. (2022). Cadmium induces liver dysfunction and ferroptosis through the endoplasmic stress-ferritinophagy axis. Ecotoxicol. Environ. Saf. 245, 114123. doi:10.1016/j.ecoenv.2022.114123
Higashi, T., Friedman, S. L., and Hoshida, Y. (2017). Hepatic stellate cells as key target in liver fibrosis. Adv. Drug Deliv. Rev. 121, 27–42. doi:10.1016/j.addr.2017.05.007
Honma, K., Kirihara, S., Nakayama, H., Fukuoka, T., Ohara, T., Kitamori, K., et al. (2023). Selective autophagy associated with iron overload aggravates non-alcoholic steatohepatitis via ferroptosis. Exp. Biol. Med. (Maywood, NJ) 248 (13), 1112–1123. doi:10.1177/15353702231191197
Howard, S. C., McCormick, J., Pui, C. H., Buddington, R. K., and Harvey, R. D. (2016). Preventing and managing toxicities of high-dose methotrexate. Oncologist 21 (12), 1471–1482. doi:10.1634/theoncologist.2015-0164
Huang, P. L. (2009). A comprehensive definition for metabolic syndrome. Dis. Model Mech. 2 (5-6), 231–237. doi:10.1242/dmm.001180
Huang, Y., Li, P., Zhao, R., Zhao, L., Liu, J., Peng, S., et al. (2022). Silica nanoparticles: biomedical applications and toxicity. Biomed. Pharmacother. 151, 113053. doi:10.1016/j.biopha.2022.113053
Jiang, C., Zhang, S., Li, D., Chen, L., Zhao, Y., Mei, G., et al. (2020). Impaired ferritinophagy flux induced by high fat diet mediates hepatic insulin resistance via endoplasmic reticulum stress. Food Chem. Toxicol. 140, 111329. doi:10.1016/j.fct.2020.111329
Jiang, J., Ruan, Y., Liu, X., Ma, J., and Chen, H. (2024a). Ferritinophagy is critical for deoxynivalenol-induced liver injury in mice. J. Agric. Food Chem. 72 (12), 6660–6671. doi:10.1021/acs.jafc.4c00556
Jiang, J., Zhou, X., Chen, H., Wang, X., Ruan, Y., Liu, X., et al. (2024b). 18β-Glycyrrhetinic acid protects against deoxynivalenol-induced liver injury via modulating ferritinophagy and mitochondrial quality control. J. Hazard Mater 471, 134319. doi:10.1016/j.jhazmat.2024.134319
Jin, L., Shen, F., Weinfeld, M., and Sergi, C. (2020). Insulin growth factor binding protein 7 (IGFBP7)-Related cancer and IGFBP3 and IGFBP7 crosstalk. Front. Oncol. 10, 727. doi:10.3389/fonc.2020.00727
Katarey, D., and Verma, S. (2016). Drug-induced liver injury. Clin. Med. (Lond). 16 (Suppl. 6), s104–s109. doi:10.7861/clinmedicine.16-6-s104
Kidane, T. Z., Sauble, E., and Linder, M. C. (2006). Release of iron from ferritin requires lysosomal activity. Am. J. Physiol. Cell Physiol. 291 (3), C445–C455. doi:10.1152/ajpcell.00505.2005
Kim, C., Cho, S. K., Kim, K. D., Nam, D., Chung, W. S., Jang, H. J., et al. (2014). β-Caryophyllene oxide potentiates TNFα-induced apoptosis and inhibits invasion through down-modulation of NF-κB-regulated gene products. Apoptosis 19 (4), 708–718. doi:10.1007/s10495-013-0957-9
Klionsky, D. J., Cregg, J. M., Dunn, W. A., Emr, S. D., Sakai, Y., Sandoval, I. V., et al. (2003). A unified nomenclature for yeast autophagy-related genes. Dev. Cell 5 (4), 539–545. doi:10.1016/s1534-5807(03)00296-x
Knovich, M. A., Storey, J. A., Coffman, L. G., Torti, S. V., and Torti, F. M. (2009). Ferritin for the clinician. Blood Rev. 23 (3), 95–104. doi:10.1016/j.blre.2008.08.001
Kong, Z., Liu, R., and Cheng, Y. (2019). Artesunate alleviates liver fibrosis by regulating ferroptosis signaling pathway. Biomed. Pharmacother. 109, 2043–2053. doi:10.1016/j.biopha.2018.11.030
Lefebvre, C., Legouis, R., and Culetto, E. (2018). ESCRT and autophagies: endosomal functions and beyond. Seminars cell and Dev. Biol. 74, 21–28. doi:10.1016/j.semcdb.2017.08.014
Li, N., Liao, Y., Huang, H., and Fu, S. (2022). Co-regulation of hepatic steatosis by ferritinophagy and unsaturated fatty acid supply. Hepatol. Commun. 6 (10), 2640–2653. doi:10.1002/hep4.2040
Li, S., Ren, Q. J., Xie, C. H., Cui, Y., Xu, L. T., Wang, Y. D., et al. (2024). Taurine attenuates activation of hepatic stellate cells by inhibiting autophagy and inducing ferroptosis. World J. gastroenterology 30 (15), 2143–2154. doi:10.3748/wjg.v30.i15.2143
Li, T., Yan, Y., Wang, B., Qian, H., Zhang, X., Shen, L., et al. (2013). Exosomes derived from human umbilical cord mesenchymal stem cells alleviate liver fibrosis. Stem Cells Dev. 22 (6), 845–854. doi:10.1089/scd.2012.0395
Li, X., Zhang, J., Gong, Y., Liu, Q., Yang, S., Ma, J., et al. (2020). Status of copper accumulation in agricultural soils across China (1985-2016). Chemosphere 244, 125516. doi:10.1016/j.chemosphere.2019.125516
Li, Z. J., Dai, H. Q., Huang, X. W., Feng, J., Deng, J. H., Wang, Z. X., et al. (2021). Artesunate synergizes with sorafenib to induce ferroptosis in hepatocellular carcinoma. Acta Pharmacol. Sin. 42 (2), 301–310. doi:10.1038/s41401-020-0478-3
Liang, Q., Ma, Y., Wang, F., Sun, M., Lin, L., Li, T., et al. (2023). Ferritinophagy was involved in long-term SiNPs exposure induced ferroptosis and liver fibrosis. Nanotoxicology 17 (2), 157–175. doi:10.1080/17435390.2023.2197055
Liao, Y., Peng, Z., Chen, L., Nüssler, A. K., Liu, L., and Yang, W. (2018). Deoxynivalenol, gut microbiota and immunotoxicity: a potential approach? Food Chem. Toxicol. 112, 342–354. doi:10.1016/j.fct.2018.01.013
Liu, L., Zheng, B., Luo, M., Du, J., Yang, F., Huang, C., et al. (2023). Suppression of USP8 sensitizes cells to ferroptosis via SQSTM1/p62-mediated ferritinophagy. Protein Cell 14 (3), 230–234. doi:10.1093/procel/pwac004
Liu, Y., Lu, T., Zhang, C., Xu, J., Xue, Z., Busuttil, R. W., et al. (2019). Activation of YAP attenuates hepatic damage and fibrosis in liver ischemia-reperfusion injury. J. hepatology 71 (4), 719–730. doi:10.1016/j.jhep.2019.05.029
Ma, M., Wei, N., Yang, J., Ding, T., Song, A., Chen, L., et al. (2023). Schisandrin B promotes senescence of activated hepatic stellate cell via NCOA4-mediated ferritinophagy. Pharm. Biol. 61 (1), 621–629. doi:10.1080/13880209.2023.2189908
Ma, Y., Liang, Q., Wang, F., Yan, K., Sun, M., Lin, L., et al. (2022). Silica nanoparticles induce pulmonary autophagy dysfunction and epithelial-to-mesenchymal transition via p62/NF-κB signaling pathway. Ecotoxicol. Environ. Saf. 232, 113303. doi:10.1016/j.ecoenv.2022.113303
Mancias, J. D., Pontano Vaites, L., Nissim, S., Biancur, D. E., Kim, A. J., Wang, X., et al. (2015). Ferritinophagy via NCOA4 is required for erythropoiesis and is regulated by iron dependent HERC2-mediated proteolysis. eLife 4, e10308. doi:10.7554/eLife.10308
Mancias, J. D., Wang, X., Gygi, S. P., Harper, J. W., and Kimmelman, A. C. (2014). Quantitative proteomics identifies NCOA4 as the cargo receptor mediating ferritinophagy. Nature 509 (7498), 105–109. doi:10.1038/nature13148
Miyazaki, T., Karube, M., Matsuzaki, Y., Ikegami, T., Doy, M., Tanaka, N., et al. (2005). Taurine inhibits oxidative damage and prevents fibrosis in carbon tetrachloride-induced hepatic fibrosis. J. hepatology 43 (1), 117–125. doi:10.1016/j.jhep.2005.01.033
Mizushima, N. (2020). The ATG conjugation systems in autophagy. Curr. Opin. Cell Biol. 63, 1–10. doi:10.1016/j.ceb.2019.12.001
Nähse, V., Stenmark, H., and Schink, K. O. (2024). Omegasomes control formation, expansion, and closure of autophagosomes. Bioessays 46 (6), e2400038. doi:10.1002/bies.202400038
Nakatogawa, H. (2013). Two ubiquitin-like conjugation systems that mediate membrane formation during autophagy. Essays Biochem. 55, 39–50. doi:10.1042/bse0550039
Nguyen, N. U., and Stamper, B. D. (2017). Polyphenols reported to shift APAP-induced changes in MAPK signaling and toxicity outcomes. Chem. Biol. Interact. 277, 129–136. doi:10.1016/j.cbi.2017.09.007
Ni, S., Yuan, Y., Qian, Z., Zhong, Z., Lv, T., Kuang, Y., et al. (2021). Hypoxia inhibits RANKL-induced ferritinophagy and protects osteoclasts from ferroptosis. Free Radic. Biol. Med. 169, 271–282. doi:10.1016/j.freeradbiomed.2021.04.027
Niu, B., Liao, K., Zhou, Y., Wen, T., Quan, G., Pan, X., et al. (2021). Application of glutathione depletion in cancer therapy: enhanced ROS-based therapy, ferroptosis, and chemotherapy. Biomaterials 277, 121110. doi:10.1016/j.biomaterials.2021.121110
Noda, T., and Yoshimori, T. (2009). Molecular basis of canonical and bactericidal autophagy. Int. Immunol. 21 (11), 1199–1204. doi:10.1093/intimm/dxp088
Obara, K., Sekito, T., Niimi, K., and Ohsumi, Y. (2008). The Atg18-Atg2 complex is recruited to autophagic membranes via phosphatidylinositol 3-phosphate and exerts an essential function. J. Biol. Chem. 283 (35), 23972–23980. doi:10.1074/jbc.M803180200
Omenetti, A., Choi, S., Michelotti, G., and Diehl, A. M. (2011). Hedgehog signaling in the liver. J. hepatology 54 (2), 366–373. doi:10.1016/j.jhep.2010.10.003
Osawa, T., and Noda, N. N. (2019). Atg2: a novel phospholipid transfer protein that mediates de novo autophagosome biogenesis. Protein Sci. 28 (6), 1005–1012. doi:10.1002/pro.3623
Pan, H., Wang, B. H., Lv, W., Jiang, Y., and He, L. (2015). Esculetin induces apoptosis in human gastric cancer cells through a cyclophilin D-mediated mitochondrial permeability transition pore associated with ROS. Chem. Biol. Interact. 242, 51–60. doi:10.1016/j.cbi.2015.09.015
Panciera, T., Azzolin, L., Cordenonsi, M., and Piccolo, S. (2017). Mechanobiology of YAP and TAZ in physiology and disease. Nat. Rev. Mol. Cell Biol. 18 (12), 758–770. doi:10.1038/nrm.2017.87
Pantopoulos, K., Porwal, S. K., Tartakoff, A., and Devireddy, L. (2012). Mechanisms of mammalian iron homeostasis. Biochemistry 51 (29), 5705–5724. doi:10.1021/bi300752r
Patel, A. S., Morse, D., and Choi, A. M. (2013). Regulation and functional significance of autophagy in respiratory cell biology and disease. Am. J. Respir. Cell Mol. Biol. 48 (1), 1–9. doi:10.1165/rcmb.2012-0282TR
Peng, Z., Liao, Y., Wang, X., Chen, L., Wang, L., Qin, C., et al. (2020). Heme oxygenase-1 regulates autophagy through carbon-oxygen to alleviate deoxynivalenol-induced hepatic damage. Arch. Toxicol. 94 (2), 573–588. doi:10.1007/s00204-019-02649-6
Pivovarov, K., and Zipursky, J. S. (2019). Low-dose methotrexate toxicity. Cmaj 191 (15), E423. doi:10.1503/cmaj.181054
Qi, X., Song, A., Ma, M., Wang, P., Zhang, X., Lu, C., et al. (2021). Curcumol inhibits ferritinophagy to restrain hepatocyte senescence through YAP/NCOA4 in non-alcoholic fatty liver disease. Cell Prolif. 54 (9), e13107. doi:10.1111/cpr.13107
Qian, Z. B., Li, J. F., Xiong, W. Y., and Mao, X. R. (2024). Ferritinophagy: a new idea for liver diseases regulated by ferroptosis. Hepatobiliary Pancreat. Dis. Int. 23 (2), 160–170. doi:10.1016/j.hbpd.2023.10.005
Ramachandran, A., and Jaeschke, H. (2019). Acetaminophen hepatotoxicity. Seminars liver Dis. 39 (2), 221–234. doi:10.1055/s-0039-1679919
Roehlen, N., Crouchet, E., and Baumert, T. F. (2020). Liver fibrosis: mechanistic concepts and therapeutic perspectives. Cells 9 (4), 875. doi:10.3390/cells9040875
Samuel, V. T., and Shulman, G. I. (2016). The pathogenesis of insulin resistance: integrating signaling pathways and substrate flux. J. Clin. investigation 126 (1), 12–22. doi:10.1172/jci77812
Sanchez, M., Galy, B., Schwanhaeusser, B., Blake, J., Bähr-Ivacevic, T., Benes, V., et al. (2011). Iron regulatory protein-1 and -2: transcriptome-wide definition of binding mRNAs and shaping of the cellular proteome by iron regulatory proteins. Blood 118 (22), e168–e179. doi:10.1182/blood-2011-04-343541
Shakya, A., Dodson, M., Artiola, J. F., Ramirez-Andreotta, M., Root, R. A., Ding, X., et al. (2023). Arsenic in drinking water and diabetes. Water (Basel) 15 (9), 1751. doi:10.3390/w15091751
Shan, X., Li, J., Liu, J., Feng, B., Zhang, T., Liu, Q., et al. (2023). Targeting ferroptosis by poly(acrylic) acid coated Mn(3)O(4) nanoparticles alleviates acute liver injury. Nat. Commun. 14 (1), 7598. doi:10.1038/s41467-023-43308-w
Shao, M., Xu, Q., Wu, Z., Chen, Y., Shu, Y., Cao, X., et al. (2020). Exosomes derived from human umbilical cord mesenchymal stem cells ameliorate IL-6-induced acute liver injury through miR-455-3p. Stem Cell Res. Ther. 11 (1), 37. doi:10.1186/s13287-020-1550-0
Shokravi, S., Borisov, V., Zaman, B. A., Niazvand, F., Hazrati, R., Khah, M. M., et al. (2022). Mesenchymal stromal cells (MSCs) and their exosome in acute liver failure (ALF): a comprehensive review. Stem Cell Res. Ther. 13 (1), 192. doi:10.1186/s13287-022-02825-z
Sun, C., Gao, M., Hu, H., Qi, J., Tang, Y., Cao, X., et al. (2024). IGF2BP3 modified GLI2 transcriptionally regulates SYVN1 and facilitates sepsis liver injury through autophagy. iScience 27 (6), 109870. doi:10.1016/j.isci.2024.109870
Sun, Y., Weng, J., Chen, X., Ma, S., Zhang, Y., Zhang, F., et al. (2023). Oroxylin A activates ferritinophagy to induce hepatic stellate cell senescence against hepatic fibrosis by regulating cGAS-STING pathway. Biomed. Pharmacother. 162, 114653. doi:10.1016/j.biopha.2023.114653
Sung, H., Ferlay, J., Siegel, R. L., Laversanne, M., Soerjomataram, I., Jemal, A., et al. (2021). Global cancer Statistics 2020: GLOBOCAN estimates of incidence and mortality worldwide for 36 cancers in 185 countries. CA a cancer J. Clin. 71 (3), 209–249. doi:10.3322/caac.21660
Szarka, A., Kapuy, O., Lőrincz, T., and Bánhegyi, G. (2021). Vitamin C and cell death. Antioxid. Redox Signal 34 (11), 831–844. doi:10.1089/ars.2019.7897
Tan, Y., Huang, Y., Mei, R., Mao, F., Yang, D., Liu, J., et al. (2022). HucMSC-derived exosomes delivered BECN1 induces ferroptosis of hepatic stellate cells via regulating the xCT/GPX4 axis. Cell death and Dis. 13 (4), 319. doi:10.1038/s41419-022-04764-2
Tian, X., Teng, J., and Chen, J. (2021). New insights regarding SNARE proteins in autophagosome-lysosome fusion. Autophagy 17 (10), 2680–2688. doi:10.1080/15548627.2020.1823124
Tong, W. H., and Rouault, T. A. (2007). Metabolic regulation of citrate and iron by aconitases: role of iron-sulfur cluster biogenesis. Biometals 20 (3-4), 549–564. doi:10.1007/s10534-006-9047-6
Wang, C., Leng, M., Ding, C., Zhu, X., Zhang, Y., Sun, C., et al. (2024a). Ferritinophagy-mediated ferroptosis facilitates methotrexate-induced hepatotoxicity by high-mobility group box 1 (HMGB1). Liver Int. official J. Int. Assoc. Study Liver 44 (3), 691–705. doi:10.1111/liv.15811
Wang, J., Li, Y., Duan, J., Yang, M., Yu, Y., Feng, L., et al. (2018). Silica nanoparticles induce autophagosome accumulation via activation of the EIF2AK3 and ATF6 UPR pathways in hepatocytes. Autophagy 14 (7), 1185–1200. doi:10.1080/15548627.2018.1458174
Wang, J., Zhu, Q., Li, R., Zhang, J., Ye, X., and Li, X. (2022). YAP1 protects against septic liver injury via ferroptosis resistance. Cell Biosci. 12 (1), 163. doi:10.1186/s13578-022-00902-7
Wang, K., Zhang, Z., Tsai, H. I., Liu, Y., Gao, J., Wang, M., et al. (2021b). Branched-chain amino acid aminotransferase 2 regulates ferroptotic cell death in cancer cells. Cell Death Differ. 28 (4), 1222–1236. doi:10.1038/s41418-020-00644-4
Wang, M., Chen, Z., Song, W., Hong, D., Huang, L., and Li, Y. (2021a). A review on cadmium exposure in the population and intervention strategies against cadmium toxicity. Bull. Environ. Contam. Toxicol. 106 (1), 65–74. doi:10.1007/s00128-020-03088-1
Wang, W., Cheng, S., and Zhang, D. (2014). Association of inorganic arsenic exposure with liver cancer mortality: a meta-analysis. Environ. Res. 135, 120–125. doi:10.1016/j.envres.2014.08.034
Wang, X., Wang, G., Qu, J., Yuan, Z., Pan, R., and Li, K. (2020). Calcipotriol inhibits NLRP3 signal through YAP1 activation to alleviate cholestatic liver injury and fibrosis. Front. Pharmacol. 11, 200. doi:10.3389/fphar.2020.00200
Wang, Y., Bo, J., Zhao, Z., Han, Y., Zhang, Q., and Liu, L. (2023). Depletion of Igfbp7 alleviates zebrafish NAFLD progression through inhibiting hepatic ferroptosis. Life Sci. 332, 122086. doi:10.1016/j.lfs.2023.122086
Wang, Y., Ding, H., Zheng, Y., Wei, X., Yang, X., Wei, H., et al. (2024b). Alleviated NCOA4-mediated ferritinophagy protected RA FLSs from ferroptosis in lipopolysaccharide-induced inflammation under hypoxia. Inflamm. Res. official J. Eur. Histamine Res. Soc. [et al] 73 (3), 363–379. doi:10.1007/s00011-023-01842-9
WHO Guidelines Approved by the Guidelines Review Committee (2017). WHO Guidelines on protecting Workers from potential risks of manufactured nanomaterials. Geneva: World Health Organization.
Wilson, M. I., Dooley, H. C., and Tooze, S. A. (2014). WIPI2b and Atg16L1: setting the stage for autophagosome formation. Biochem. Soc Trans 42 (5), 1327–1334. doi:10.1042/bst20140177
Wu, A., Li, M., Chen, Y., Zhang, W., Li, H., Chen, J., et al. (2024). Multienzyme active manganese oxide alleviates acute liver injury by mimicking redox regulatory system and inhibiting ferroptosis. Adv Healthc Mater 13 (11), e2302556. doi:10.1002/adhm.202302556
Wu, H., Liu, Q., Shan, X., Gao, W., and Chen, Q. (2023a). ATM orchestrates ferritinophagy and ferroptosis by phosphorylating NCOA4. Autophagy 19 (7), 2062–2077. doi:10.1080/15548627.2023.2170960
Wu, M., Sun, J., Wang, L., Wang, P., Xiao, T., Wang, S., et al. (2023b). The lncRNA HOTAIR via miR-17-5p is involved in arsenite-induced hepatic fibrosis through regulation of Th17 cell differentiation. J Hazard Mater 443 (Pt B), 130276. doi:10.1016/j.jhazmat.2022.130276
Xiu, Z., Li, Y., Fang, J., Han, J., Li, S., Li, Y., et al. (2023). Inhibitory effects of esculetin on liver cancer through triggering NCOA4 pathway-mediation ferritinophagy in vivo and in vitro. J Hepatocell Carcinoma 10, 611–629. doi:10.2147/jhc.S395617
Xiu, Z., Zhu, Y., Han, J., Li, Y., Yang, X., Yang, G., et al. (2022). Caryophyllene oxide induces ferritinophagy by regulating the NCOA4/FTH1/LC3 pathway in hepatocellular carcinoma. Front Pharmacol 13, 930958. doi:10.3389/fphar.2022.930958
Yan, H., Li, T., Wang, Y., Xu, J., and Lu, X. (2019). Insulin-like growth factor binding protein 7 accelerates hepatic steatosis and insulin resistance in non-alcoholic fatty liver disease. Clin Exp Pharmacol Physiol 46 (12), 1101–1110. doi:10.1111/1440-1681.13159
Yan, J., Li, S., and Li, S. (2014). The role of the liver in sepsis. Int Rev Immunol 33 (6), 498–510. doi:10.3109/08830185.2014.889129
Yanatori, I., and Kishi, F. (2019). DMT1 and iron transport. Free Radic Biol Med 133, 55–63. doi:10.1016/j.freeradbiomed.2018.07.020
Yang, F., Liao, J., Yu, W., Qiao, N., Guo, J., Han, Q., et al. (2021). Exposure to copper induces mitochondria-mediated apoptosis by inhibiting mitophagy and the PINK1/parkin pathway in chicken (Gallus gallus) livers. J Hazard Mater 408, 124888. doi:10.1016/j.jhazmat.2020.124888
Yang, F., Pei, R., Zhang, Z., Liao, J., Yu, W., Qiao, N., et al. (2019). Copper induces oxidative stress and apoptosis through mitochondria-mediated pathway in chicken hepatocytes. Toxicol In Vitro 54, 310–316. doi:10.1016/j.tiv.2018.10.017
Yang, H., Sun, W., Bi, T., Wang, Q., Wang, W., Xu, Y., et al. (2023). The PTBP1-NCOA4 axis promotes ferroptosis in liver cancer cells. Oncology reports 49 (2), 45. doi:10.3892/or.2023.8482
Yu, L., Lv, Z., Li, S., Jiang, H., Han, B., Zheng, X., et al. (2023a). Chronic arsenic exposure induces ferroptosis via enhancing ferritinophagy in chicken livers. Sci Total Environ 890, 164172. doi:10.1016/j.scitotenv.2023.164172
Yu, Q., Wu, T., Xu, W., Wei, J., Zhao, A., Wang, M., et al. (2023b). PTBP1 as a potential regulator of disease. Mol Cell Biochem. 479, 2875–2894. doi:10.1007/s11010-023-04905-x
Zhang, D. L., Ghosh, M. C., and Rouault, T. A. (2014). The physiological functions of iron regulatory proteins in iron homeostasis - an update. Front Pharmacol 5, 124. doi:10.3389/fphar.2014.00124
Zhang, L., Hou, L., Liu, Z., Huang, S., Meng, Z., and Liang, L. (2020d). A mitophagic response to iron overload-induced oxidative damage associated with the PINK1/Parkin pathway in pancreatic beta cells. J Trace Elem Med Biol 60, 126493. doi:10.1016/j.jtemb.2020.126493
Zhang, L., Jin, L., Guo, J., Bao, K., Hu, J., Zhang, Y., et al. (2020c). Chronic intermittent hypobaric hypoxia enhances bone fracture healing. Front Endocrinol (Lausanne) 11, 582670. doi:10.3389/fendo.2020.582670
Zhang, W., Zhu, Y. D., Zhang, Q. Y., Ma, L. J., Yang, L., Guo, W. Z., et al. (2020a). Research progress in application and mechanism of Schisandrae Chinensis Fructus for prevention and treatment of liver diseases. Zhongguo Zhong Yao Za Zhi 45 (16), 3759–3769. doi:10.19540/j.cnki.cjcmm.20200513.601
Zhang, Z., Guo, M., Li, Y., Shen, M., Kong, D., Shao, J., et al. (2020b). RNA-binding protein ZFP36/TTP protects against ferroptosis by regulating autophagy signaling pathway in hepatic stellate cells. Autophagy 16 (8), 1482–1505. doi:10.1080/15548627.2019.1687985
Zhang, Z., Yao, Z., Wang, L., Ding, H., Shao, J., Chen, A., et al. (2018). Activation of ferritinophagy is required for the RNA-binding protein ELAVL1/HuR to regulate ferroptosis in hepatic stellate cells. Autophagy 14 (12), 2083–2103. doi:10.1080/15548627.2018.1503146
Zhong, G., Li, Y., Ma, F., Huo, Y., Liao, J., Han, Q., et al. (2024). Copper exposure induced chicken hepatotoxicity: involvement of ferroptosis mediated by lipid peroxidation, ferritinophagy, and inhibition of FSP1-CoQ10 and nrf2/slc7a11/GPX4 Axis. Biol Trace Elem Res 202 (4), 1711–1721. doi:10.1007/s12011-023-03773-2
Zhou, J., Tan, Y., Hu, L., Fu, J., Li, D., Chen, J., et al. (2022). Inhibition of HSPA8 by rifampicin contributes to ferroptosis via enhancing autophagy. Liver international official journal of the International Association for the Study of the Liver 42 (12), 2889–2899. doi:10.1111/liv.15459
Zhu, J., Jin, Z., Wang, J., Wu, Z., Xu, T., Tong, G., et al. (2024). FGF21 ameliorates septic liver injury by restraining proinflammatory macrophages activation through the autophagy/HIF-1α axis. J Adv Res. doi:10.1016/j.jare.2024.04.004
Zhu, Y., Zhang, Y., Fan, Z., Fang, Y., Zheng, Y., Li, Y., et al. (2023a). Silica nanoparticles trigger chaperone HSPB8-assisted selective autophagy via TFEB activation in hepatocytes. Small 19 (5), e2204310. doi:10.1002/smll.202204310
Zhu, Z. H., Xu, X. T., Shen, C. J., Yuan, J. T., Lou, S. Y., Ma, X. L., et al. (2023b). A novel sesquiterpene lactone fraction from Eupatorium chinense L. suppresses hepatocellular carcinoma growth by triggering ferritinophagy and mitochondrial damage. Phytomedicine 112, 154671. doi:10.1016/j.phymed.2023.154671
Keywords: ferritinophagy, autophagy, ferroptosis, NAFLD, HCC, liver fibrosis
Citation: Wu K, Zhao W, Hou Z, Zhang W, Qin L, Qiu J, Wang D, Zhuang L, Xue X and Sun D (2025) Ferritinophagy: multifaceted roles and potential therapeutic strategies in liver diseases. Front. Cell Dev. Biol. 13:1551003. doi: 10.3389/fcell.2025.1551003
Received: 24 December 2024; Accepted: 06 February 2025;
Published: 25 February 2025.
Edited by:
Kurt Sartorius, University of the Witwatersrand, South AfricaReviewed by:
Yuh-Jin Liang, Taipei Veterans General Hospital, TaiwanTerisha Ghazi, University of KwaZulu-Natal, South Africa
Mengjie Xiao, Guangdong Academy of Sciences (China National Analytical Center, Guangzhou), China
Copyright © 2025 Wu, Zhao, Hou, Zhang, Qin, Qiu, Wang, Zhuang, Xue and Sun. This is an open-access article distributed under the terms of the Creative Commons Attribution License (CC BY). The use, distribution or reproduction in other forums is permitted, provided the original author(s) and the copyright owner(s) are credited and that the original publication in this journal is cited, in accordance with accepted academic practice. No use, distribution or reproduction is permitted which does not comply with these terms.
*Correspondence: Lin Zhuang, emh1YW5nbGluQHdqcm15eS5jbg==; Xiaofeng Xue, eGZ4dWVAc3VkYS5lZHUuY24=; Ding Sun, c3VuZGluZ0BzdWRhLmVkdS5jbg==
†These authors have contributed equally to this work