- 1Department of Biological Sciences, Faculty of Science, Concordia University of Edmonton, Edmonton, AB, Canada
- 2Department of Microbiology, Faculty of Medicine, Northern Border University, Arar, Saudi Arabia
- 3Department of Pathology, Faculty of Medicine, Northern Border University, Arar, Saudi Arabia
- 4Department of Pharmacology, Faculty of Medicine, Northern Border University, Arar, Saudi Arabia
- 5Department of Family and Community Medicine, Faculty of Medicine, Northern Border University, Arar, Saudi Arabia
- 6Department of Anatomy, Faculty of Medicine, Northern Border University, Arar, Saudi Arabia
Inflammation is a critical part of innate immune response that is essential for exclusion of harmful stimuli and restoration of tissue homeostasis. Nonetheless, failure to resolve inflammation results in chronic inflammatory conditions, including autoimmune diseases. Conventionally, resolution of inflammation was deemed a passive process; however, evidence indicates that it entails active, highly regulated molecular and cellular events involving efferocytosis-driven macrophage reprogramming, post-transcriptional regulatory mechanisms and the production of specialized pro-resolving mediators (SPMs). These processes collectively restore tissue homeostasis and prevent chronic inflammation. Emerging therapeutic approaches targeting these pathways demonstrate promising results in preclinical studies and clinical trials, enhancing resolution and improving overall disease outcome. This resulted in a paradigm shift from conventional anti-inflammatory strategies to resolution-focused treatment. Yet, challenges remain due to the complexity of resolution mechanisms and tissue-specific differences. This review summarizes current advances in inflammation resolution, emphasizing emerging concepts of resolution pharmacology. By employing endogenous mechanisms facilitating resolution, novel therapeutic applications can effectively manage several chronic inflammatory disorders.
1 Introduction
Inflammation is a physiological immune response triggered upon injury and/or infection to eliminate harmful stimuli, promote tissue repair and establish immune memory for future encounters (Kumar et al., 2004). The acute inflammatory response involves a complex and coordinated series of molecular and cellular events, including release of soluble mediators such as chemokines, cytokines, free radicals and eicosanoids, regulating its initiation and resolution (Rankin, 2004). Traditionally, the termination of inflammatory responses was mainly credited to passive dissipation of pro-inflammatory inducers; however, the expanding literature implies inflammation resolution to be highly regulated. Canon of Medicine, compiled in 1025 by the physician-philosopher Avicenna, was first to highlight the concept of active resolution of inflammation, (Sina, 2005). In the late 1970s, Kumar et al reemphasized resolution as a distinctive process mediated by cellular and soluble effectors with well-characterized events (Kumar et al., 2021). Following that, key insights into cellular dynamics of inflammation resolution emerged following studies revealing that macrophages phagocytosed apoptotic polymorphonuclear cells (PMNs), as a key event in establishing resolution (Savill et al., 1989). Macrophages were subsequently recognized for their role in coordinating inflammation resolution processes. This was accentuated with complete resolution as the ideal inflammatory outcome, and chronic inflammation attributed to resolution failure (Cotran et al., 1999).
Discoveries of arachidonic acid metabolism and cytokine signaling enhanced our understanding of acute inflammation as a complex and biochemically driven process. The 1982 Nobel Prize was awarded to Bergström, Samuelsson and Vane for emphasizing the significance of prostaglandin biosynthesis and aspirin’s mechanism of action (Vane, 1971; Samuelsson, 1983). The work on arachidonic acid derivatives highlighted the role of lipid autacoids in inflammation induction and resolution (Samuelsson, 1983). The discovery of lipoxins (LXs) by Serhan et al. revealed their anti-inflammatory and pro-resolving activities, such as suppressing neutrophil recruitment and enhancing clearance of tissue debris by macrophages (Serhan et al., 1984; Serhan, 2005), emphasizing a dual effect of LXs in limiting neutrophil activity while promoting monocyte trafficking for tissue repair. As a result, LXs are currently perceived as active mediators of tissue restoration rather than passive anti-inflammatory agents (Maddox and Serhan, 1996). Additional molecules were reported over the years to be actively involved in inflammation resolution and were termed specialized pro-resolving mediators (SPMs). These mediators cemented the concept of resolution as a dynamic biologically orchestrated process crucial for restoring tissue homeostasis (Panigrahy et al., 2021).
Over the past two decades, significant progress has been made in understanding the resolution of cardinal signs of inflammation: heat (calor), pain (dolor), redness (rubor), swelling (tumor) and loss of function (functio laesa) (Heidland et al., 2006; Tracy, 2006). Interplay between effective resolution, innate immunity and adaptive immunity was reported, where unresolved acute inflammation led to maladaptive immune responses (Newson et al., 2014). This supported the idea that chronic inflammatory diseases may not only be driven by persistent pro-inflammatory processes but also by impaired resolution, with therapies aimed at activating resolution potentially guiding inflammation down a pro-resolution pathway. Unlike traditional anti-inflammatory approaches, pro-resolution strategies offer broader potential; however, challenges remain, such as defining the diverse tissue- and stimulus-specific nature of resolution pathways (Fullerton and Gilroy, 2016). Additionally, while central mediators of resolution have been identified, it is uncertain whether a single pro-resolution therapy can address multiple diseases.
The literature is progressively distinguishing the terms “anti-inflammation” and “pro-resolution,” emphasizing the concept that resolution is an active process, facilitating restoration of tissue homeostasis and the transition from innate to adaptive immunity (Serhan et al., 2007; Buckley et al., 2014). Efforts to target pro-resolution pathways in chronic inflammatory diseases are ongoing, with new therapeutic models being explored. This evolving understanding encourages a shift in how chronic inflammation is treated, suggesting that pro-resolving agents may be as effective, or even synergistic with, or superior to, conventional anti-inflammatory drugs (Fullerton and Gilroy, 2016). Although many effective anti-inflammatory treatments are available, including NSAIDs, anti-cytokine therapies and steroids, addressing underlying disease mechanisms remains a significant challenge. Diseases driven by persistent inflammation could be treated by activating pro-resolution pathways that are either pathologically suppressed or by enhancing functional pro-resolution mechanisms. To target resolution specifically, the aim should be to alter the trajectory of established inflammation-driven disease in a clinically relevant manner by leveraging endogenous “off switches,” such as signaling cascades or cellular interactions, that lead to inflammation resolution (Fullerton and Gilroy, 2016). This review offers an update on the field of inflammation resolution, focusing on key molecular and cellular participants and mechanisms that influence their behavior, fate and clearance while emphasizing ongoing efforts to develop therapies targeting pro-resolution pathways.
A typical acute inflammatory response and its resolution goes through four main phases that each comprises several critical events (summarized in Figure 1): 1) initiation of acute inflammation, 2) suppression of inflammation and onset of resolution, 3) active resolution and 4) post-resolution.
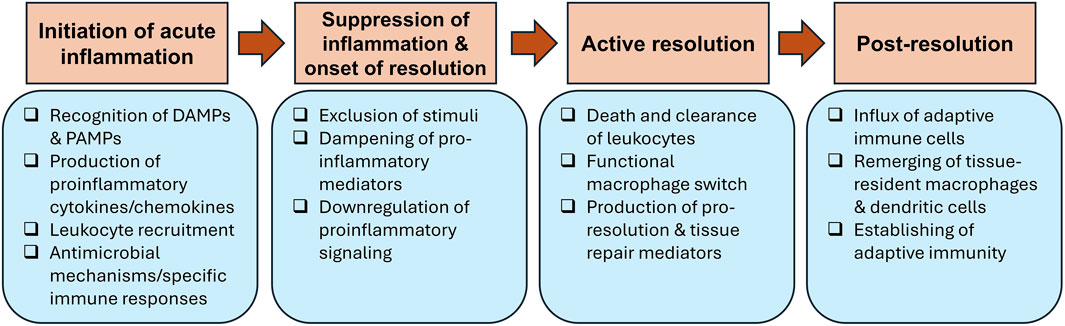
Figure 1. Steps of acute inflammation and its resolution. Acute inflammation is triggered after recognition of damage-associated molecular patterns (DAMPs) and pathogen-associated molecular patterns (PAMPs) by receptors expressed on resident immune cells. This results in the secretion of pro-inflammatory mediators that aid in recruitment of granulocytes to the inflammation site. Several antimicrobial mechanisms are deployed to remove the injurious stimuli, which kicks off the suppression of inflammation and onset of resolution by downregulating pro-inflammatory mediators and signaling. Active resolution is activated by efferocytosis, resulting in macrophage phenotypic switch from classically activated (M1) to alternatively activated (M2) macrophages and production of pro-resolving molecules. The post-resolution phase occurs after the completion of resolution, when adaptive immune cells infiltrate tissues to establish adaptive immune responses.
2 Initiation of acute inflammation
Following injury and/or infection, damage-associated molecular patterns (DAMPs) (Roh and Sohn, 2018), released by necrotic cells, and pathogen-associated molecular patterns (PAMPs) (Mogensen, 2009), including conserved motifs of invading pathogens, are detected by innate receptors such as toll-like receptors (TLRs) on tissue-resident cells. Recognizing DAMPs or PAMPs triggers the onset of an acute inflammatory response (Medzhitov and Janeway, 2000), leading to release of various pro-inflammatory mediators facilitating cellular recruitment and modulating immune responses to clear infections (Abdallah et al., 2017). Previously, we discussed mediators, cellular effectors and pathways involved during onset and peak phase of acute inflammation, and importantly demonstrated that mechanisms involved in induction of inflammation are evolutionarily conserved among cold- and warm-blooded animals (Soliman and Barreda, 2023). These mechanisms involve exudation of proteins, upregulation of cell adhesion molecules on endothelial cells and influx of granulocytes (PMNs in nonspecific inflammation or eosinophils in allergic responses) (Fullerton and Gilroy, 2016). Recruited immune cells act through intracellular mechanisms, such as superoxide radicals, myeloperoxidase, proteases and lactoferrins, or extracellularly via neutrophil extracellular traps (NETs) to neutralize pathogens (Schmid-Schönbein, 2006). Drugs such as NSAIDs and cytokine-neutralizing antibodies, e.g., tumor necrosis factor (TNF)-specific antibodies, are frequently utilized to suppress inflammation to treat chronic inflammatory diseases (Yeung et al., 2018).
3 Suppression of inflammation and onset of resolution
Inflammation resolution is triggered by effector cells that induce inhibition of pro-inflammatory profiles and transition toward tissue repair and homeostasis (Buckley et al., 2014). Indeed, the process depends on the extent to which inflammatory cells have neutralized provocative stimuli (Buckley et al., 2014). The transition from pro-inflammatory to anti-inflammatory profile and subsequent restoration of tissue homeostasis, traditionally referred to as resolution, is now understood to be an active process rather than a passive dissipation of inflammation (Buckley et al., 2013). Initially, dampening of acute inflammation starts only once the injurious agents responsible for triggering the inflammatory response are eliminated (exclusion of the stimuli). Afterward, the production of pro-inflammatory mediators ceases, and any remaining mediators are degraded and cleared (dampening of pro-inflammatory mediators). This results in the downregulation of pro-inflammatory signaling and halting further leukocyte recruitment and edema formation.
3.1 Exclusion of stimuli
For resolution to proceed effectively, agents that triggered the inflammatory response must be cleared. We previously highlighted antimicrobial responses by which the immune system can remove bacterial infections (Soliman et al., 2021; Soliman and Barreda, 2022; Soliman and Barreda, 2023). These include NADPH oxidase-dependent killing by PMNs, antimicrobial peptides, phagocytosis and NETs. Additionally, leukocytes, such as neutrophils, engulf debris, clearing the injury site of dead tissue and creating pathways for angiogenesis necessary for restoring tissue repair (Peiseler and Kubes, 2019). Dysregulated inflammatory responses in the case of immunodeficiency disorders (e.g., chronic granulomatous disease) have been attributed to defects in mechanisms of bacterial clearance (Pollock et al., 1995), causing resolution failure. Likewise, autoimmune diseases such as rheumatoid arthritis are driven by persistent endogenous antigens (Firestein, 2003).
3.2 Dampening of pro-inflammatory mediators
After exclusion of stimuli, levels of pro-inflammatory mediators (cytokines, chemokines, eicosanoids and cell adhesion molecules) must return to their pre-inflammatory baseline. The depletion of chemokines through mechanisms such as proteolytic cleavage and sequestration is essential for creating a resolving environment and halting neutrophil influx (Ortega-Gómez et al., 2013). Prostaglandins contributing to vasodilation and edema formation were found to undergo catabolism during resolution (Hansen et al., 1999). Leukotrienes are degraded by β-oxidation pathway, breaking down carboxyl ends of molecules into short-chain metabolites (Hansen et al., 1999; Freire and Van Dyke, 2013).
Proteolysis of chemokines has been shown to be a key mechanism for their depletion. Matrix metalloproteinases (MMPs), known for their role in breaking down extracellular matrix proteins during many physiological and pathological conditions, contribute to modulating activity of several bioactive molecules involved during inflammation (Tam et al., 2004; Dean and Overall, 2007). Levels of molecules including chemokines (McQuibban et al., 2000; Moelants et al., 2013), defensins (Wilson et al., 1999), mannose-binding lectin (Butler et al., 2002) and TNF-α (Gearing et al., 1994) are controlled by MMPs during inflammation resolution. At the cellular level, macrophages regulate acute inflammatory responses by selectively cleaving chemokines via MMP-12 (Dean et al., 2008). For example, macrophage-derived MMP-12 targets ELR motif in CXC chemokines, a domain essential for receptor binding, depicting these chemokines ineffective (Dean et al., 2008). These findings emphasize these endogenous mechanisms clearing pro-inflammatory mediators and suggest that their dysregulation can lead to chronic inflammation. Indeed, leveraging pro-inflammatory catabolic pathways therapeutically could help shift ongoing inflammation toward resolution.
Chemokines drive cell migration via conventional G protein-coupled chemokine receptors. A subset of chemokines is recognized by a unique class of atypical chemokine receptors (ACKRs), previously referred to as decoys or chemokine-binding proteins, including chemokine-binding protein D6 and CCR5 (Ariel et al., 2006; Nibbs and Graham, 2013; Vacchini et al., 2016). Unlike conventional receptors, ACKRs do not induce leukocyte migration because they cannot activate classical G protein-dependent signaling pathways (Vacchini et al., 2016). These receptors bind ligands without triggering classical signaling pathways, acting as scavengers for pro-inflammatory signals. Instead, ACKRs sequester chemokines from the environment, which is critical for shaping chemokine gradients (Nibbs and Graham, 2013). As a result, ACKRs are increasingly recognized as vital regulatory elements in chemokine networks across various physiological and pathological conditions. For example, CCR5 expressed on apoptotic neutrophils can scavenge chemokines CCL3 and CCL5 in mice (Ariel et al., 2006). In Ccr5−/− mice, CCL3 and CCL5 were elevated in peritoneal exudates during the resolution of acute peritonitis (Ariel et al., 2006).
3.3 Downregulation of pro-inflammatory signaling
After removal of stimuli and lowering levels of pro-inflammatory mediators at the inflammation site, various pro-inflammatory receptor families and their signaling pathways are deactivated to prevent collateral damage from a sustained pro-inflammatory state. A failure of this process would result in a cytokine storm that can occur even in the absence of positive bacterial blood cultures (i.e., sepsis), indicating excessive and prolonged activation of the innate immune system despite eliminating the initial trigger (Buckley et al., 2013). The “stop signals” of inflammation consist of negative feedback regulators. These signals counteract pro-inflammatory signaling pathways and suppress further production of pro-inflammatory mediators.
Inflammation is regulated by both transcriptional and post-transcriptional mechanisms, which control the expression of proteins involved in its initiation and resolution (Sugimoto et al., 2016). While transcription is the initial step in gene expression, post-transcriptional regulation is essential for rapidly suppressing inflammation by facilitating mRNA degradation. This is particularly important because mRNA can be long-lived, and simply halting its synthesis does not immediately stop inflammation. mRNAs of pro-inflammatory cytokines are frequently controlled through mRNA decay or blocking their translation (Anderson, 2008). These mechanisms protect the host from the pathological overexpression of inflammatory proteins. Many mRNAs contain adenine- or uridine-rich elements (AREs) within their 3′-untranslated regions (UTRs), which attract destabilizing factors and translational silencers (Anderson, 2008). Among the ARE-binding proteins that promote mRNA destabilization are tristetraprolin (TTP) (Carballo et al., 2000) and steroid receptor co-activator 3 (SRC3) (Li et al., 2012). Additionally, microRNAs (miRNAs) can post-transcriptionally regulate mRNA stability and translation, limiting the expression of inflammatory mediators. Although the precise role of post-transcriptional regulation in inflammation resolution is not entirely understood, it presents exciting opportunities for pharmacological interventions to address the overproduction of inflammatory proteins (Mazumder et al., 2010). Downregulators of pro-inflammatory signaling, summarized in Table 1, can be categorized into 1) anti-inflammatory mediators that can negatively regulate signaling of pro-inflammatory pathways and their activated transcription programs; 2) post-transcriptional regulation promoting mRNA degradation or inhibiting its translation; and 3) miRNAs.
4 Active resolution of inflammation
Cell death and removal of inflammatory leukocytes are the main drivers of resolution, offering a wealth of potential targets for developing pro-resolution therapeutics. Active resolution of inflammation involves: 1) death and clearance of inflammatory leukocytes; 2) functional macrophage switch; and 3) production of pro-resolution and tissue repair mediators. During resolution phase, regardless of whether the initial response was driven by PMNs, eosinophils, or lymphocytes responding to recall antigens, immune cells are cleared from inflamed tissues (Buckley et al., 2014). While some inflammatory leukocytes may return to systemic circulation, many PMNs, eosinophils and lymphocytes undergo local apoptosis or necrosis, followed by engulfing apoptotic cells by monocyte-derived macrophages, a process known as efferocytosis (Buckley et al., 2013). This process is governed by complex signaling pathways involving cell-to-cell receptor interactions and humoral mediators, notably bioactive lipids. After completing neutrophil apoptosis and efferocytosis, a shift towards a pro-resolution profile is triggered by effector cells including macrophages via releasing pro-resolving mediators (Serhan and Savill, 2005).
4.1 Death and clearance of inflammatory leukocytes
Three primary means by which inflammatory cells can be cleared from inflammatory sites: 1) retro-transendothelial migration back into systemic circulation, 2) lymphatic drainage, facilitating their role in adaptive immunity, and 3) cell death within inflamed tissues (Fullerton and Gilroy, 2016).
Many mechanisms, including autophagy, pyroptosis, necrosis, apoptosis and necroptosis mediate localized cell death (Fullerton and Gilroy, 2016). Granulocytes (e.g., neutrophils) are known to have a short lifespan, though pro-inflammatory cytokines (e.g., IL-6, IL-8 and GM-CSF) enhance survival of these cells. Likewise, eosinophil endurance is promoted by IL-3, IL-5 and GM-CSF (Filep, 2022). Pathways such as NF-κB enhance pro-survival molecules of granulocytes while reducing pro-apoptotic ones. Downregulating these mediators via dampening pro-inflammatory pathways is the initial step in activating pro-apoptotic pathways in inflammatory cells. The regulation of cell death pathways plays a crucial role in inflammation and its resolution, with several key mechanisms and signaling pathways influencing leukocyte survival. Inducing apoptotic pathways in inflammatory cells like PMNs, eosinophils and T helper (Th)1 lymphocytes can aid in resolving chronic inflammation. However, this strategy requires precise targeting to avoid disrupting pro-resolving and homeostatic cells (Alessandri et al., 2011; Leitch et al., 2012). Granulocyte apoptosis during resolution has been extensively studied, with key molecules and pathways regulating leukocyte survival or death, including CDK, NF-κB, PI3K–AKT, cAMP and MAPK. The following summarizes some of these important pathways and potential therapeutic targets.
4.1.1 NF-κB pathway
NF-κB is a critical transcription factor involved in regulating expression of several pro-inflammatory mediators. Activation of NF-κB pathway influences cellular survival by regulating intracellular pro-survival proteins, including BCL-2 family members like BCL-XL and XIAP. Pharmacological inhibition of NF-κB and IKKα can enhance granulocyte apoptosis, promoting inflammatory resolution (Savill, 1997; Sousa et al., 2009; Lawrence and Fong, 2010; Remijsen et al., 2011; Vanden Berghe et al., 2014).
4.1.2 cAMP pathway
Cyclic AMP (cAMP) plays a dual role in apoptosis, with its effects varying based on the context and cell type. cAMP was shown to inhibit granulocyte apoptosis and impair efferocytosis in vitro (Rossi et al., 1998; Lawrence and Fong, 2010). However, in vivo studies demonstrate that therapeutic increase of cAMP, via rolipram or forskolin, in LPS-induced pleurisy promotes PMN apoptosis and inhibits neutrophil accumulation (Sousa et al., 2010). Additionally, rolipram induces a cAMP-mediated switch of M1-like macrophages to M2 pro-resolving macrophages, highlighting cAMP’s therapeutic potential in regulating inflammation (Bystrom et al., 2008).
4.1.3 PI3K–AKT pathway
The pro-apoptotic protein BAD, a downstream target of PI3K–AKT pathway, is phosphorylated by AKT to suppress apoptosis and promote cell survival (Song et al., 2005). In experimental autoimmune encephalomyelitis (EAE), a multiple sclerosis model, PI3Kγ-deficient mice showed reduced disease severity, lower CCL2 and CCL5 levels in brain tissue, and increased leukocyte apoptosis compared to wild-type controls (Rodrigues et al., 2010). Notably, neither PI3Kγ deficiency nor its pharmacological inhibition (e.g., with AS-605240) affected the acute phase of disease but instead reduced inflammation by promoting leukocyte apoptosis.
4.1.4 MAPK pathway
Mitogen-activated protein kinase (MAPK) pathways have various roles in inflammation resolution ranging from enhancing neutrophil apoptosis to inducing polarization of anti-inflammatory macrophages. MAPK subtypes (e.g., ERK1/2, JNK, p38 MAPK) are involved in regulating cellular apoptosis (Junttila et al., 2008). ERK1/2 is typically linked to cell survival, but its inhibition can promote resolution of pleurisy by triggering PMNs apoptosis (Sawatzky et al., 2006; Chapman and Miner, 2011). Controversial roles of p38 MAPK were reported, with evidence for both pro-apoptotic (Langereis et al., 2010) and anti-apoptotic (Allaeys et al., 2014) effects. MAPK phosphatase 1 (MKP-1), an enzyme that regulates activity of MAPK, limits activation of p38 MAPK, aiding macrophage polarization and release of anti-inflammatory cytokines (Perdiguero et al., 2012).
4.1.5 CDK inhibition
Cyclin-dependent kinase (CDK), which generally promotes cell survival by driving cell cycle progression, is increasingly recognized as emerging therapeutic tool prompting inflammation resolution (Rossi et al., 2006). By targeting CDK, therapies facilitate efferocytosis and enhance clearing of inflammatory cells. For example, inhibitors such as roscovitine induce apoptosis in granulocytes, enhancing resolution in GM-CSF- and LPS- stimulated human PMNs (Leitch et al., 2010; Perdiguero et al., 2012).
Therapeutic inducing of apoptosis is a promising strategy, however, effective efferocytosis is further required to prevent accumulation of apoptotic cells (Leitch et al., 2010). On the other hand, efferocytosis is an immunosuppressive process, with the potential to increase susceptibility to infections (Medeiros et al., 2009). Understanding interactions between apoptotic cells, phagocytosis and soluble mediators regulating these processes is essential for interpreting mechanisms underlying chronic inflammatory diseases and developing therapies. Efferocytosis is mediated by complex interplay of signals and receptors that assist in recognizing and engulfing apoptotic cells. Apoptotic PMNs release “find-me” signals such as ATP, which activate purinergic receptors on macrophages (Elliott et al., 2009). ICAM3 (CD50) binding to CD14 on macrophages and thrombospondin (TSP1) engaging CD36, further enhance apoptotic cell recognition (Savill et al., 1992; Moffatt et al., 1999). Lysophosphatidylcholine (LPC) and sphingosine-1-phosphate (S1P) interact with G-protein-coupled receptors G2A and S1P1-5, respectively, to guide phagocytes toward apoptotic PMNs (Lauber et al., 2003; Gude et al., 2008). In addition to “find-me” signal, “eat-me” signal (e.g., phosphatidylserine; PS) is crucial for specific recognition of apoptotic cells. Externalization of PS during apoptosis allows direct or opsonin-mediated binding to phagocytic receptors including αvβ3 (Poon et al., 2014; Elliott and Ravichandran, 2016).
Bridging molecules are essential for connecting macrophages and apoptotic cells. For example, αvβ3 or αvβ5 integrins on macrophages and PS on apoptotic cells are bound by the milk fat globule-EGF factor 8 protein (MFG-E8) (Hanayama et al., 2002). Likewise, developmental endothelial locus-1 (DEL-1) facilitates efferocytosis and inflammation resolution by connecting PS to αvβ3 integrins (Hajishengallis and Chavakis, 2019). Annexin A1 (ANXA1), galectin-3 and protein S are additional bridging molecules that promote PS-dependent clearance of apoptotic cells by interacting with TAM receptors (e.g., Tyro-3, Axl, Mer) (Anderson et al., 2003; Arur et al., 2003; Lemke and Burstyn-Cohen, 2010; Caberoy et al., 2012). By identifying PS, macrophage phagocytic receptors such TIM-1, TIM-4 and stabilin-2 aid in efferocytosis (Kobayashi et al., 2007; Park et al., 2008). Additional receptors, including CD14, scavenger receptor CD36 and integrin CD11b/CD18, are involved in regulating apoptotic cell clearance (Devitt et al., 1998; Mevorach et al., 1998). Defective clearance of apoptotic cells is associated with autoimmune disorders (Shao and Cohen, 2011) and chronic inflammatory diseases (Brown et al., 2003), making efferocytosis a critical process for resolving inflammation and maintaining immune tolerance (Brown et al., 2003).
4.2 Functional macrophage switch
By triggering immunological reactions through releasing pro-inflammatory mediators, classically activated macrophages (M1) act as early respondents in inflammatory sites (Soliman and Barreda, 2022). However, these cells shift to alternatively activated anti-inflammatory (M2) phenotype during the resolution phase, which is necessary to eliminate apoptotic cells and facilitate resolution. Efferocytosis enables this transition, resulting in a decrease in pro-inflammatory cytokines and increased levels of anti-inflammatory mediators such as IL-10 and TGF-β (Soliman and Barreda, 2022). Several mediators and signaling pathways regulating the functional switch of macrophages from M1 (classical) to M2 (alternative) phenotype are summarized in Figure 2.
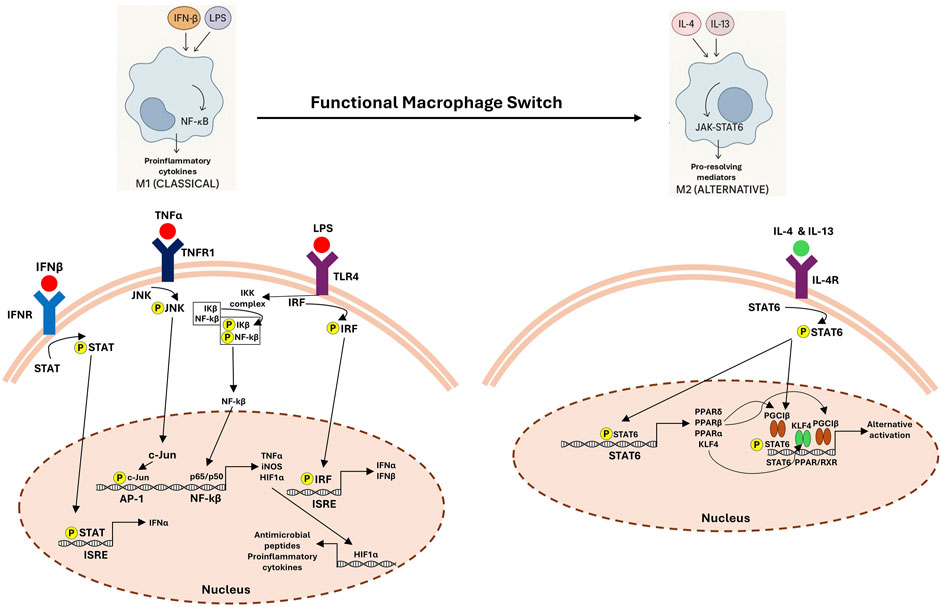
Figure 2. Functional macrophage switch: signaling pathways in M1 and M2 polarization. The left panel shows classical (M1) macrophage activation driven by lipopolysaccharide (LPS), tumor necrosis factor-alpha (TNF-α) and interferon-β (IFN-β), which activate Toll-like receptor 4 (TLR4), tumor necrosis factor receptor (TNFR) and interferon receptor (IFNR), respectively. This leads to downstream activation of STAT, JNK, IRF, and NF-κB signaling cascades, promoting the transcription of proinflammatory cytokines (e.g., TNFα), inducible nitric oxide synthase (iNOS), and antimicrobial peptides. The right panel depicts alternative (M2) activation mediated by interleukin-4 (IL-4) and interleukin-13 (IL-13) via IL-4 receptor (IL-4R), resulting in STAT6 phosphorylation and induction of anti-inflammatory and tissue repair-associated genes through PPAR and KLF4-dependent pathways. This switch supports the resolution of inflammation and tissue remodeling.
Prostaglandin E2 (PGE2) plays a complex role in inflammation, initially acting as a pro-inflammatory mediator by promoting vasodilation, edema and neutrophil recruitment during the onset phase (Ricciotti and FitzGerald, 2011). This is mediated through COX-2-derived PGE2, which is a key target of Nonsteroidal anti-inflammatory drugs (NSAIDs). However, during resolution, PGE2 contributes to macrophage reprogramming by suppressing TNF-α and IL-1β production while enhancing IL-10 release, thereby facilitating the transition to a pro-resolving environment (Ricciotti and FitzGerald, 2011). PGE2 further reduces synthesis and secretion of TNF-α and IL-1β from macrophages (Kunkel et al., 1986; Katakami et al., 1988). This oppressive effect allows PGE2 to act as self-regulator of pro-inflammatory cytokines (Kunkel et al., 1986). Recently, PGE2 has been shown to enhance IL-10 release in LPS-stimulated macrophages (MacKenzie et al., 2013). PGE2 induces production of IL-10 in alternatively active macrophages through EP receptor signaling, suggesting a continuous role in macrophage reprogramming (Németh et al., 2009). Others reported that PGE2 displays a bipolar effect on IL-6 transcription, promoting its expression initially before limiting it (MacKenzie et al., 2013). High levels of PGE2 reduced mucosal inflammation and colitis symptoms in DSS-induced colitis murine model (Zhang Y. et al., 2015). In lungs, PGE2 was released by fibroblasts and alveolar macrophages, limiting inflammatory reactions and facilitating tissue repair (Vancheri et al., 2004). Conversely, low levels of PGE2 have been linked to lung fibrosis in human and animal models, highlighting its role in effective inflammation resolution (Wilborn et al., 1995; Hodges et al., 2004).
Macrophages undergo metabolic adaptations as they process the metabolites of apoptotic cells, influencing their pro-resolving functions (Schilperoort et al., 2023b). Apoptotic cell-derived arginine facilitates multiple cycles of efferocytosis, termed continual efferocytosis, in murine macrophages generated in vitro or isolated during resolution (Yurdagul et al., 2020). In contrast, human monocyte-derived macrophages primarily utilize apoptotic cell-derived ornithine to sustain continual efferocytosis (Yurdagul et al., 2020). These findings highlight cellular metabolic dependencies, underscoring the necessity of mechanistic investigations of immunometabolism in human macrophages. Macrophages engaging in efferocytosis activate distinct metabolic pathways that enhance production of pro-resolving mediators (Zhang et al., 2019). For example, suppression of Cpt1, which encodes a key enzyme in mitochondrial long-chain fatty acid oxidation, in murine macrophages co-cultured with apoptotic cells, led to decreased IL-10 levels. Interestingly, when glycolysis was inhibited, IL-10 production remained unaffected (Zhang et al., 2019). These findings indicate that fatty acid oxidation is a crucial metabolic pathway for efferocytosis-driven IL-10 production in macrophages. Other reports indicated that glycolysis is non-replaceable for definite efferocytosis persistence in murine as well as human macrophages (Schilperoort et al., 2023a).
Additionally, GLS1-modified by glutaminase 1 (GLS1)-mediated glutaminolysis was required for established efferocytosis. Macrophages that were removed from liver and spleens of Gls1-deficient mice were identified to be defective in efferocytosis when they were subsequently challenged with labeled apoptotic cells (Merlin et al., 2021). In atherosclerosis murine model, efferocytosis was impaired in Gls1-deficient mice, facilitating the deterioration of disease (Merlin et al., 2021). Interestingly, macrophages no matter if differentiated in vitro or isolated from tissues (e.g., spleen and thymus), maintained clearing of apoptotic cells under hypoxic conditions by developing the required metabolic profile (Wang et al., 2023). Table 2 summarizes various drugs and compounds that promote M2 macrophage polarization by targeting specific signaling pathways. These interventions have been studied in different experimental models, demonstrating their potential to modulate inflammation and enhance tissue repair. Future studies should investigate how efferocytosis of cells undergoing pro-inflammatory cell death (i.e., pyroptosis, necroptosis, ferroptosis) might impact macrophage-mediated resolution of inflammation.
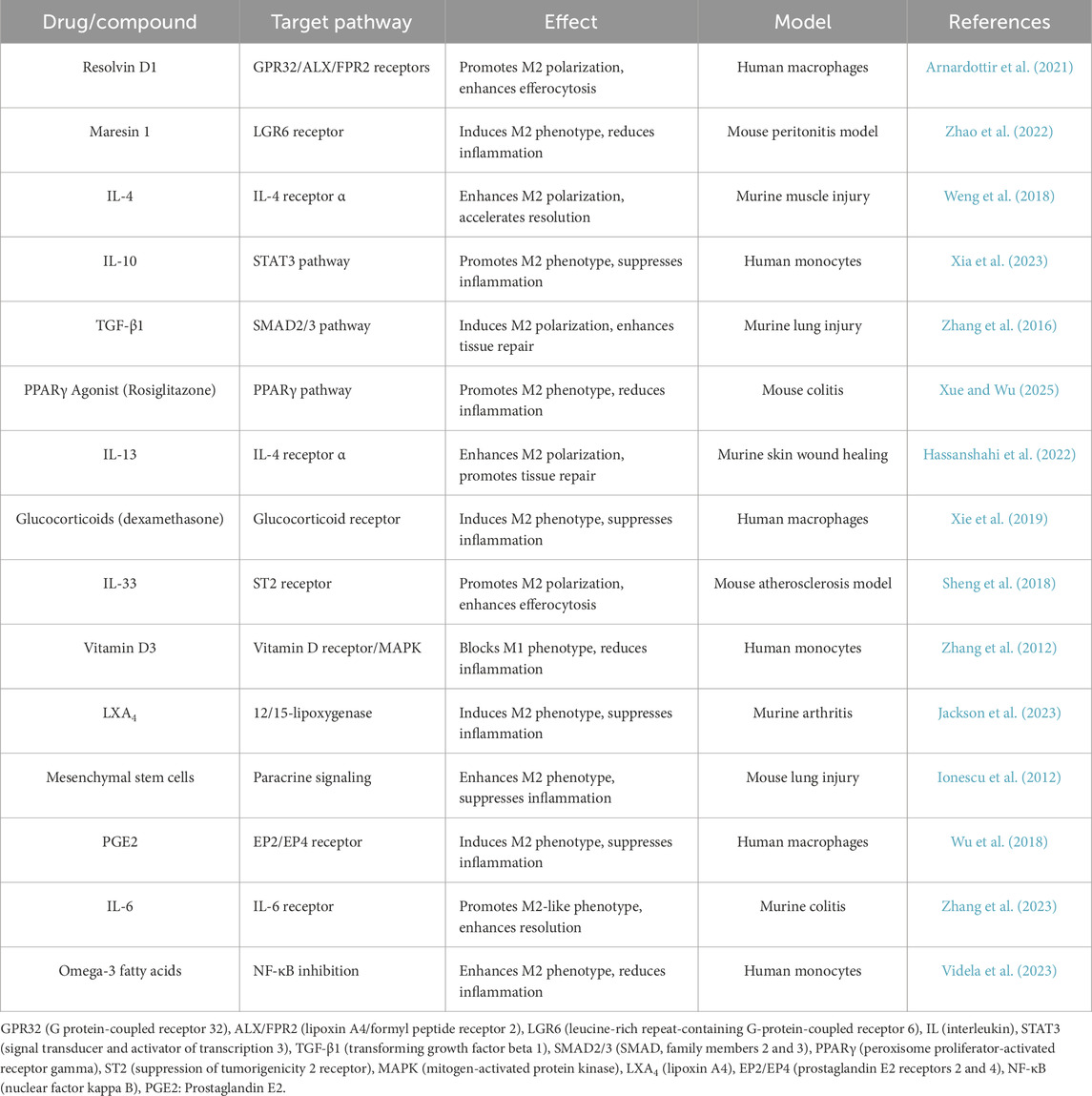
Table 2. Recent advances in targeting macrophage phenotype transitions during inflammation resolution.
4.3 Production of pro-resolution and tissue repair mediators
Macrophages engulfing apoptotic cells shift toward a pro-resolution phenotype (Junttila et al., 2008), that is characterized by increased expression of inhibitory molecules (e.g., PDL1 and ICOS ligand), secretion of anti-inflammatory cytokines, and reduced release of pro-inflammatory cytokines (e.g., TNF, IL-1β, IL-18) (Poon et al., 2014). They also produce pro-resolving lipid mediators, including SPMs (Schwab et al., 2007; Serhan et al., 2012), which contribute to many steps of resolving inflammation and subsequent restoration of tissue homeostasis (Figure 3). Table 3 details to a large extent pro-resolution mediators and their mechanisms of action in overcoming inflammation. SPMs, including lipoxins, resolvins, maresins and protectins, play a crucial role in actively resolving inflammation (Figure 4). These mediators not only counteract excessive immune activation but also promote tissue repair and immune balance (Serhan and Levy, 2018). The exact mechanisms by which SPMs exert their anti-inflammatory and pro-resolving effects is not fully understood. SPMs promote inflammation resolution as well as switch the balance from effector to regulatory T cell (Treg). Other SPMs such as protectins, maresins, and D-series resolvins have been shown to function as biased positive allosteric modulators (PAM) of the prostaglandin E2 (PGE2) receptor (EP4), promoting anti-inflammatory signaling of EP4 (Alnouri et al., 2024).
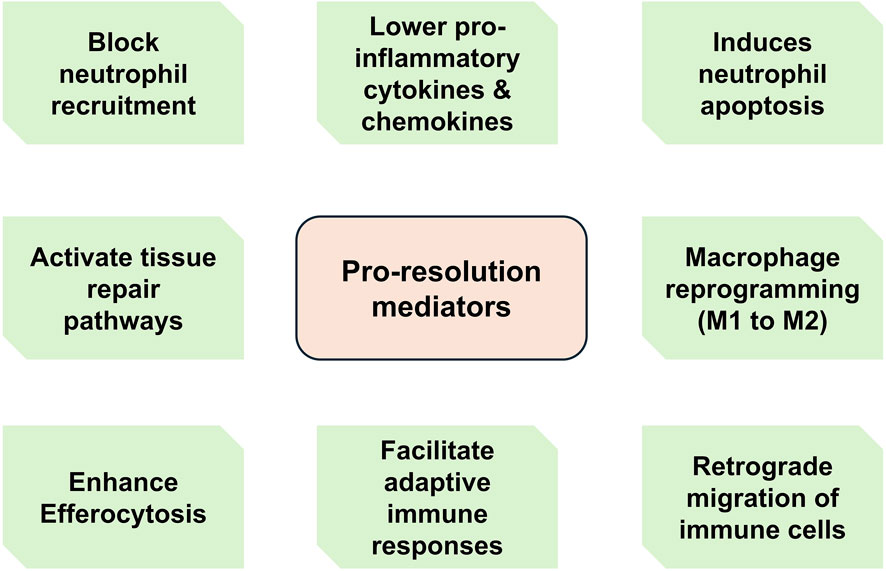
Figure 3. Main functions of pro-resolution mediators. During active resolution, a variety of effector cells including macrophages secrete pro-resolving molecules such as specialized pro-resolving mediators (SPMs). These molecules mediate various functions ranging from suppressing granulocyte recruitment to regulating tissue repair pathways and facilitating adaptive immune responses.
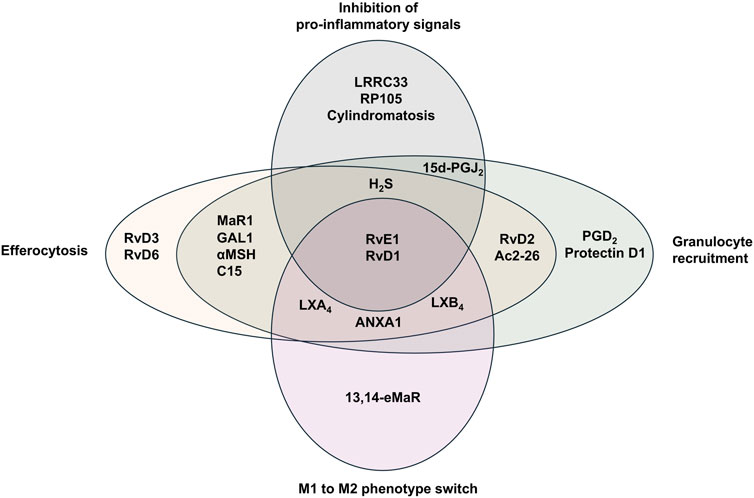
Figure 4. Specialized pro-resolving mediators and their reported functions during resolution of inflammation. Specialized pro-resolving mediators (SPMs) mediate several effector functions during resolution of inflammation. LX (lipoxin), Rv (resolvin), ANXA1 (annexin A1), PGD (prostaglandin D), 15d-PGJ2 (15-deoxy-Δ12,14-prostaglandin J2), MaR1 (maresin 1), αMSH (alpha-melanocyte-stimulating hormone), C15 (chemerin-derived peptide), H2S (hydrogen sulfide).
Resolvin E4 (RvE4), a newly identified member of resolvin family, has been reported to enhance efferocytosis (Serhan and Levy, 2018). Meanwhile, RvE1 reduces differentiation of Th17 and thus IL-17 production (Oner et al., 2021). RvD1 suppresses inflammation via post-transcriptional regulation, linking miRNA modulation to macrophage reprogramming (Amin Mohedin et al., 2024). Explicitly, RvD1 prevented age-related inflammation by reducing macrophage senescence and fibrosis in spleens of aged mice, indicating its anti-aging and anti-fibrotic potential (Groenen et al., 2024). Precise delivery of RvD1 using nanotechnology enhanced its anti-inflammatory effects within vascular lesions (He et al., 2024). RvD2 activation of GPR18 on macrophages reduces plaque burden and inflammation in atherosclerosis murine models, showing targeted SPM signaling benefits (Lipscomb et al., 2024). Likewise RvT4 promotes macrophage cholesterol efflux, reducing atherosclerosis and highlighting a novel role for SPMs in lipid homeostasis in cardiovascular diseases (Walker et al., 2024). Mechanistically, resolvin enhances mitochondrial metabolism and reduces inflammatory gene expression, supporting long-term macrophage function under inflammatory stress (Padovani et al., 2024). Additionally, resolvins demonstrated diagnostic and prognostic potentials in experimental models and clinical trials (Perez-Hernandez et al., 2021). A clinical study demonstrated that low RvD1 and LXA4 levels correlated with inflammation severity in gallstone-induced pancreatitis patients (Mısırlıoglu et al., 2025).
SPMs exhibit various pro-resolution effects in organ-specific inflammation and injury. RvD4 mitigates LPS-induced lung injury via reducing neutrophil infiltration and cytokine levels (Inomata et al., 2024). RvE1 has been also shown to protect against one-lung ventilation-induced injury via diminishing cytokine storms and epithelial damage (Ji et al., 2024). In pressure-overload-induced heart failure model, RvD2/GPR18 axis ameliorated pro-inflammatory macrophage polarization (Zheng et al., 2024). RvD1 with exercise therapy was reported to mediate neuroprotective effects in intracranial hemorrhage mice models via BDNF/TrkB/PI3K/AKT signaling by enhancing neural recovery post-stroke (Xiaoyu et al., 2024). RvD2 induces dentin regeneration and pulp stem cell activation post-pulpotomy, showcasing dental regenerative potential of SPMs through stimulation of reparative processes in dental tissues (Yoneda et al., 2024).
SPMs have been found to change tumor-associated macrophages (TAMs) into an anti-tumor phenotype in cancer (Lavy et al., 2021). SPMs also include Protectin Conjugates in Tissue Regeneration 1 (PCTR1) and Protectin D1 (PD1). PCTR1 and PD1 were detected during the resolution phase of respiratory synthetical virus (RSV) infection where they demonstrated modulatory effects on secondary immune responses in lungs (Walker et al., 2021). Similarly, RvD1 inhibited IL-6/STAT3-driven epithelial mesenchymal transmission in colorectal cancer, suppressing cancer progression by interfering with inflammatory cytokine signaling (Du et al., 2024).
Maresin 1 (MaR1), a lipid mediator derived from docosahexaenoic acid, plays a crucial role in the resolution of inflammation by promoting macrophage-mediated efferocytosis and reducing pro-inflammatory cytokine production (Francos-Quijorna et al., 2017). Dysregulated maresin 1 production was associated with chronic rhinosinusitis. Maresin 1 is also involved in the regulation of adhesion molecule expression in phagocytes, resulting in promoting inflammation resolution (Beegun et al., 2021). Prostanoids, which include prostaglandins and thromboxanes, fuel the immune system to start normally but then induce inflammation resolution. Specifically, PGE2 and PGD2 affect immune cell activity by regulating neutrophil influx, enhancing macrophage-mediated clearance of apoptotic cells, and shifting from a pro-inflammatory to a reparative environment. These bioactive lipids are prominent factors leading the shift from inflammation to resolution, and as a result, they are promising therapeutic candidates for inflammatory diseases (Schmid and Brüne, 2021). Likewise, RvE1 enhances macrophage efferocytosis and restores hematopoiesis in aplastic anemia, suggesting a therapeutic role of RvE1 in bone marrow failure syndromes (Grazda et al., 2024). RvE1 and MaR1 enhance osteogenesis of bone marrow stem cells under inflammatory stress by resolving inflammation and promoting regenerative capacity through enhancing osteoblast differentiation (Alzahrani et al., 2024).
Recent studies indicated phytocannabinoids, like cannabidiol (CBD) and tetrahydrocannabinol (THC), as key modulators of inflammation resolution. CBD and THC activate the endocannabinoids system (ECS) through CB1 and CB2 receptors, triggering the endocannabinoid pathway. This system influences immune cell function, suppresses the production of pro-inflammatory mediators and promotes M2 macrophage efferocytosis (Nagarkatti et al., 2009; Burstein, 2015). Besides, phytocannabinoid treatment increases apoptosis and promotes tissue repair by suppressing oxidative stress and downregulating fibrotic activity. Immunomodulatory properties of these substances have made them favorable for utilizing them in chronic inflammatory diseases and infections, even in extreme cases such as COVID-19 (Paland et al., 2021).
5 Post-resolution
The post-resolution phase bridges innate and adaptive immunity, where molecular pathways regulating resolution events (e.g., macrophage reprogramming) directly influence long-term immune memory and tissue repair. For instance, resolvins and maresins have been found to not only assist in clearing apoptotic neutrophils but also modulating dendritic cell (DCs) trafficking to lymph nodes and priming T-cell responses (Chiurchiù et al., 2016). Subsequently, macrophages transitioning to an M2 phenotype secrete TGF-β and IL-10, which dampen inflammation while promoting Treg expansion, a critical step in preventing autoimmune reactivation (Chen et al., 2023). This crosstalk underscores how resolution pathways are not merely endpoints but active participants in immune homeostasis, with failures leading to chronic inflammation or fibrosis.
The traditional view that resolution marks the end of inflammatory responses is increasingly challenged by recent reports confirming a post-resolution phase (Newson et al., 2014). It was reported that after resolution of innate immune responses to fungal mimics (zymosan) or bacterial infection (S. pneumoniae), a second wave of leukocytes infiltrated tissues, consisting of monocyte-derived macrophages, myeloid-derived suppressor cells, DCs and macrophages (Ersland et al., 2010; Newson et al., 2014). Furthermore, tissue-resident embryonically derived macrophages, previously lost during acute inflammation, re-emerged (Blériot et al., 2020). These populations were further associated with increased memory T and B lymphocytes in tissues and absence of PMNs (Nakano et al., 2009; Ersland et al., 2010). Collectively, the data suggest that immune cells originating from resolving tissues or peripheral blood enhance adaptive immune responses during resolution by persisting in tissues for months post-inflammation (Yona et al., 2013; Panigrahy et al., 2021). Thus, these post-resolution processes shape a connection between innate and adaptive immunity, where induction of acute inflammation and its resolution are crucial for regulating humoral and cell-mediated immune responses.
Post-resolution is crucial for establishing long-term immune memory and ensuring effective tissue remodeling. SPMs, such as resolvins, protectins and maresins are not merely passive byproducts but actively orchestrate the cessation of inflammation, promote clearance of inflammatory cells and facilitate tissue repair and regeneration (Spite et al., 2014). The phase is thus crucial for restoring tissue homeostasis and preventing chronic inflammation, which can lead to fibrosis and impaired function. SPMs modulate adaptive immunity by influencing DCs function and T cell responses (Serhan and Levy, 2018). This modulation ensures that the immune system can remember and respond more effectively to future insults, thereby linking the resolution of inflammation directly to quality and durability of adaptive immune memory.
6 Epigenetic regulatory mechanisms during inflammation resolution
Emerging evidence highlights epigenetic mechanisms as pivotal regulators of inflammation resolution, primarily through the modulation of gene expression in innate immune cells (Zhang and Cao, 2021). Histone modifications, such as methylation and acetylation, dynamically regulate inflammatory gene transcription in macrophages and DCs. These modifications influence the accessibility of transcriptional machinery to gene of pro- and anti-inflammatory cytokines, thereby controlling the intensity and duration of immune responses (Zhang and Cao, 2021). For instance, histone demethylase Tet2 plays a crucial role in repressing IL-6 transcription during resolution phase, preventing prolonged inflammation and promoting tissue homeostasis (Zhang Q. et al., 2015).
Macrophage polarization is another critical aspect influenced by epigenetic regulation. Epigenetic modifications govern the transition between pro-inflammatory M1 and anti-inflammatory M2 macrophage phenotypes (Kapellos and Iqbal, 2016). DNA methylation (e.g., DNMT3A-mediated silencing of pro-inflammatory genes) and histone modifications (e.g., HDAC3-dependent deacetylation) dynamically modulate macrophage polarization (Jin et al., 2021). Jin et al. explored the potential of targeting epigenetic modifiers to reprogram macrophages in non-resolving inflammatory conditions, such as in atherosclerosis (Jin et al., 2021). By modulating epigenetic enzymes, it is possible to shift macrophage polarization towards a reparative phenotype, offering therapeutic avenues for chronic inflammatory diseases.
7 Translational research and therapeutic implications
Understanding the therapeutic potential of these pathways requires a detailed examination of how they can be targeted to accelerate inflammation resolution in clinical settings. Table 4 summarizes key molecular pathways involved in inflammation resolution, highlighting their downstream targets, effects on resolution, and the progress made in testing these pathways as therapeutic targets. Targeting resolution pathways in chronic inflammatory conditions may yield valuable insights for resolution pharmacology. As preclinical research continues to elucidate the functions of SPMs in inflammation, their potential as therapeutic agents is drawing increasing interest. For example, RvD2 in combination with omega-3 polyunsaturated fatty acid mitigated inflammatory bowel disease (IBD) symptoms in murine models through modulation of intestinal inflammation and epithelial repair (Chaim et al., 2024). Recently imidazole-derived RvD1 analogues with antioxidant activity demonstrated promising potentials for the treatment of oxidative stress-related diseases, including osteoarthritis (Kariminezhad et al., 2024). Another study showed that RvD1 promotes chondrocyte proliferation in osteoarthritis via NLRP3/caspase-1 suppression, highlighting mechanistic insights into how RvD1 can reduce inflammasome activation and restore cartilage cell health (Wang et al., 2024). RvD1 suppresses inflammation in synoviocytes via p38, NF-κB, and AKT pathways, supporting the use of RvD1 in treating inflammatory joint diseases like rheumatoid arthritis (Yanoshita et al., 2025). Ongoing clinical trials are investigating the efficacy of SPMs-based synthetic analogs, designed to resist metabolic degradation and extend their pharmacological effects. These analogs are being evaluated for their therapeutic potential in inflammatory diseases, including dry eye, asthma, periodontitis, inflammatory bowel disease and cardiovascular disorders. Table 5 summarizes key translational research efforts exploring SPMs interventions in clinical settings, highlighting their study outcomes and therapeutic potential.
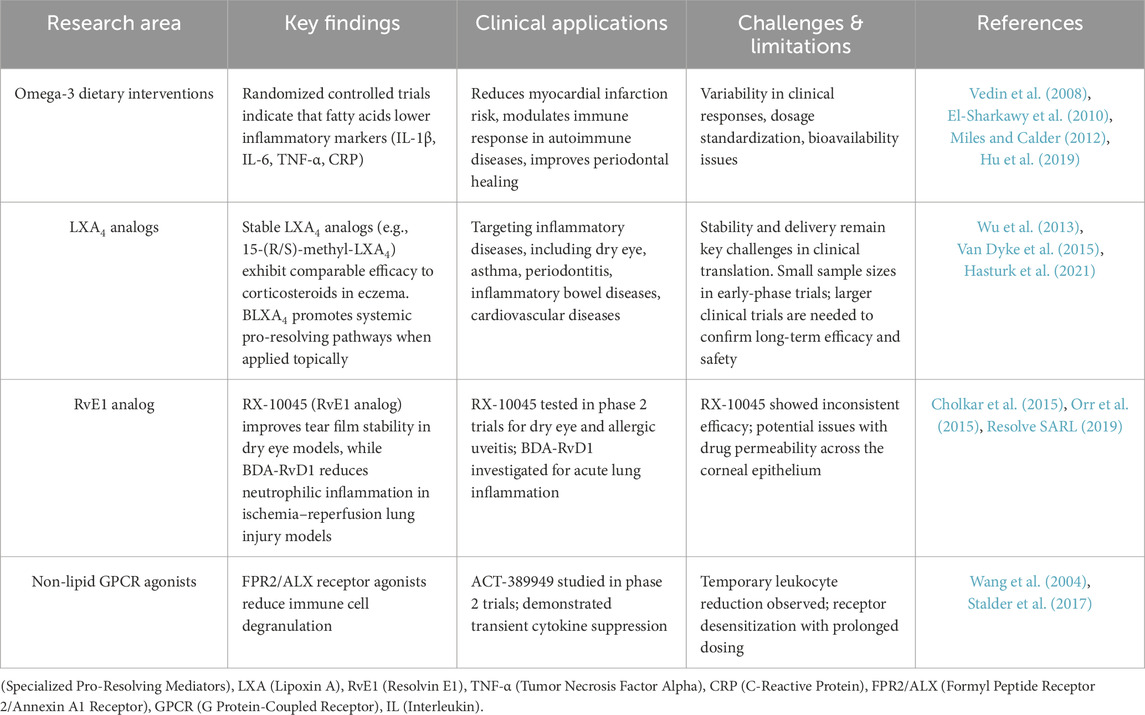
Table 5. Translational research on specialized pro-resolving mediators (SPMs) and related therapeutic interventions.
8 Conclusion
The resolution of inflammation is an active, highly regulated process that restores tissue homeostasis and prevents the transition to chronic inflammation. Over the past decades, significant progress has been made in elucidating the molecular and cellular mechanisms underlying resolution, including the roles of SPMs, macrophage reprogramming and efferocytosis. These insights have shifted the paradigm from traditional anti-inflammatory treatments to pro-resolution strategies, which offer a more targeted and physiological approach to managing chronic inflammatory diseases.
Despite these advances, challenges remain in translating fundamental discoveries into clinical therapies. The complexity of resolution pathways, their tissue-specific dynamics, and the interplay between innate and adaptive immunity necessitate further investigation. Emerging therapeutic approaches, including lipid mediator analogs, nanomedicine, and immunometabolic interventions, hold promises for enhancing resolution in disease contexts. Future research should focus on refining biomarker identification, optimizing drug delivery systems, and integrating computational models to advance resolution-targeted treatments. By leveraging these strategies, resolution pharmacology has the potential to revolutionize how inflammatory diseases are treated, shifting the focus from merely suppressing inflammation to actively restoring immune balance and tissue integrity.
Author contributions
AS: Conceptualization, Writing – review and editing, Writing – original draft. MS: Writing – review and editing. SS: Writing – review and editing. HB: Writing – review and editing. NG: Writing – review and editing. BA: Writing – review and editing. AA: Writing – review and editing. AH: Writing – review and editing. MJ: Writing – review and editing. EE: Writing – review and editing.
Funding
The author(s) declare that no financial support was received for the research and/or publication of this article.
Conflict of interest
The authors declare that the research was conducted in the absence of any commercial or financial relationships that could be construed as a potential conflict of interest.
Generative AI statement
The author(s) declare that no Generative AI was used in the creation of this manuscript.
Publisher’s note
All claims expressed in this article are solely those of the authors and do not necessarily represent those of their affiliated organizations, or those of the publisher, the editors and the reviewers. Any product that may be evaluated in this article, or claim that may be made by its manufacturer, is not guaranteed or endorsed by the publisher.
References
Abdallah, F., Mijouin, L., and Pichon, C. (2017). Skin immune landscape: inside and outside the organism. Mediat. Inflamm. 2017, 5095293. doi:10.1155/2017/5095293
Akhtar, N., Singh, A. K., and Ahmed, S. (2016). MicroRNA-17 suppresses TNF-α signaling by interfering with TRAF2 and cIAP2 association in rheumatoid arthritis synovial fibroblasts. J. Immunol. 197, 2219–2228. doi:10.4049/jimmunol.1600360
Alessandri, A. L., Duffin, R., Leitch, A. E., Lucas, C. D., Sheldrake, T. A., Dorward, D. A., et al. (2011). Induction of eosinophil apoptosis by the cyclin-dependent kinase inhibitor AT7519 promotes the resolution of eosinophil-dominant allergic inflammation. PLoS One 6, e25683. doi:10.1371/journal.pone.0025683
Allaeys, I., Gymninova, I., Canet-Jourdan, C., and Poubelle, P. E. (2014). IL-32γ delays spontaneous apoptosis of human neutrophils through MCL-1, regulated primarily by the p38 MAPK pathway. PLoS One 9, e109256. doi:10.1371/journal.pone.0109256
Alnouri, M. W., Roquid, K. A., Bonnavion, R., Cho, H., Heering, J., Kwon, J., et al. (2024). SPMs exert anti-inflammatory and pro-resolving effects through positive allosteric modulation of the prostaglandin EP4 receptor. Proc. Natl. Acad. Sci. U. S. A. 121, e2407130121. doi:10.1073/pnas.2407130121
Alzahrani, S., Shinwari, Z., Alaiya, A., and Al-Kahtani, A.-K. (2024). Impact of resolvin-E1 and maresin-1 on bone marrow stem cell osteogenesis under inflammatory stress. Cells 13, 932. doi:10.3390/cells13110932
Amici, S. A., Dong, J., and Guerau-de-Arellano, M. (2017). Molecular mechanisms modulating the phenotype of macrophages and microglia. Front. Immunol. 8, 1520. doi:10.3389/fimmu.2017.01520
Amin Mohedin, J., Rezaiemanesh, A., Asadi, S., Haddadi, M., Abdul Ahmed, B., Gorgin Karaji, A., et al. (2024). Resolvin D1 (Rvd1) attenuates in vitro LPS-stimulated inflammation through downregulation of miR-155, miR -146, miR -148 and krupple like factor 5. Rep. Biochem. Mol. Biol. 12, 566–574. doi:10.61186/rbmb.12.4.566
Anderson, H. A., Maylock, C. A., Williams, J. A., Paweletz, C. P., Shu, H., and Shacter, E. (2003). Serum-derived protein S binds to phosphatidylserine and stimulates the phagocytosis of apoptotic cells. Nat. Immunol. 4, 87–91. doi:10.1038/ni871
Anderson, P. (2008). Post-transcriptional control of cytokine production. Nat. Immunol. 9, 353–359. doi:10.1038/ni1584
Ansari, J., Senchenkova, E. Y., Vital, S. A., Al-Yafeai, Z., Kaur, G., Sparkenbaugh, E. M., et al. (2021). Targeting the AnxA1/Fpr2/ALX pathway regulates neutrophil function, promoting thromboinflammation resolution in sickle cell disease. Blood 137, 1538–1549. doi:10.1182/blood.2020009166
Ariel, A., Fredman, G., Sun, Y.-P., Kantarci, A., Van Dyke, T. E., Luster, A. D., et al. (2006). Apoptotic neutrophils and T cells sequester chemokines during immune response resolution through modulation of CCR5 expression. Nat. Immunol. 7, 1209–1216. doi:10.1038/ni1392
Ariel, A., Li, P.-L., Wang, W., Tang, W.-X., Fredman, G., Hong, S., et al. (2005). The docosatriene protectin D1 is produced by TH2 skewing and promotes human T cell apoptosis via lipid raft clustering. J. Biol. Chem. 280, 43079–43086. doi:10.1074/jbc.M509796200
Arnardottir, H., Thul, S., Pawelzik, S.-C., Karadimou, G., Artiach, G., Gallina, A. L., et al. (2021). The resolvin D1 receptor GPR32 transduces inflammation resolution and atheroprotection. J. Clin. Invest. 131, e142883. doi:10.1172/JCI142883
Arur, S., Uche, U. E., Rezaul, K., Fong, M., Scranton, V., Cowan, A. E., et al. (2003). Annexin I is an endogenous ligand that mediates apoptotic cell engulfment. Dev. Cell. 4, 587–598. doi:10.1016/s1534-5807(03)00090-x
Aubeux, D., Tessier, S., Pérez, F., Geoffroy, V., and Gaudin, A. (2023). In vitro phenotypic effects of Lipoxin A4 on M1 and M2 polarized macrophages derived from THP-1. Mol. Biol. Rep. 50, 339–348. doi:10.1007/s11033-022-08041-5
Auvynet, C., Moreno, S., Melchy, E., Coronado-Martínez, I., Montiel, J. L., Aguilar-Delfin, I., et al. (2013). Galectin-1 promotes human neutrophil migration. Glycobiology 23, 32–42. doi:10.1093/glycob/cws128
Bäck, M., Powell, W. S., Dahlén, S.-E., Drazen, J. M., Evans, J. F., Serhan, C. N., et al. (2014). Update on leukotriene, lipoxin and oxoeicosanoid receptors: IUPHAR Review 7. Br. J. Pharmacol. 171, 3551–3574. doi:10.1111/bph.12665
Barnig, C., Cernadas, M., Dutile, S., Liu, X., Perrella, M. A., Kazani, S., et al. (2013). Lipoxin A4 regulates natural killer cell and type 2 innate lymphoid cell activation in asthma. Sci. Transl. Med. 5, 174ra26. doi:10.1126/scitranslmed.3004812
Bazzoni, F., Rossato, M., Fabbri, M., Gaudiosi, D., Mirolo, M., Mori, L., et al. (2009). Induction and regulatory function of miR-9 in human monocytes and neutrophils exposed to proinflammatory signals. Proc. Natl. Acad. Sci. U. S. A. 106, 5282–5287. doi:10.1073/pnas.0810909106
Beegun, I., Koenis, D. S., Alusi, G., and Dalli, J. (2021). Dysregulated maresin concentrations in plasma and nasal secretions from patients with chronic rhinosinusitis. Front. Immunol. 12, 733019. doi:10.3389/fimmu.2021.733019
Benedetti, F., Curreli, S., Krishnan, S., Davinelli, S., Cocchi, F., Scapagnini, G., et al. (2017). Anti-inflammatory effects of H2S during acute bacterial infection: a review. J. Transl. Med. 15, 100. doi:10.1186/s12967-017-1206-8
Blériot, C., Chakarov, S., and Ginhoux, F. (2020). Determinants of resident tissue macrophage identity and function. Immunity 52, 957–970. doi:10.1016/j.immuni.2020.05.014
Brock, M., Trenkmann, M., Gay, R. E., Gay, S., Speich, R., and Huber, L. C. (2011). MicroRNA-18a enhances the interleukin-6-mediated production of the acute-phase proteins fibrinogen and haptoglobin in human hepatocytes. J. Biol. Chem. 286, 40142–40150. doi:10.1074/jbc.M111.251793
Brown, J. R., Goldblatt, D., Buddle, J., Morton, L., and Thrasher, A. J. (2003). Diminished production of anti-inflammatory mediators during neutrophil apoptosis and macrophage phagocytosis in chronic granulomatous disease (CGD). J. Leukoc. Biol. 73, 591–599. doi:10.1189/jlb.1202599
Brzoska, T., Böhm, M., Lügering, A., Loser, K., and Luger, T. A. (2010). Terminal signal: anti-inflammatory effects of α-melanocyte-stimulating hormone related peptides beyond the pharmacophore. Adv. Exp. Med. Biol. 681, 107–116. doi:10.1007/978-1-4419-6354-3_8
Buckley, C. D., Gilroy, D. W., and Serhan, C. N. (2014). Proresolving lipid mediators and mechanisms in the resolution of acute inflammation. Immunity 40, 315–327. doi:10.1016/j.immuni.2014.02.009
Buckley, C. D., Gilroy, D. W., Serhan, C. N., Stockinger, B., and Tak, P. P. (2013). The resolution of inflammation. Nat. Rev. Immunol. 13, 59–66. doi:10.1038/nri3362
Burstein, S. (2015). Cannabidiol (CBD) and its analogs: a review of their effects on inflammation. Bioorg Med. Chem. 23, 1377–1385. doi:10.1016/j.bmc.2015.01.059
Butler, G. S., Sim, D., Tam, E., Devine, D., and Overall, C. M. (2002). Mannose-binding lectin (MBL) mutants are susceptible to matrix metalloproteinase proteolysis: potential role in human MBL deficiency. J. Biol. Chem. 277, 17511–17519. doi:10.1074/jbc.M201461200
Bystrom, J., Evans, I., Newson, J., Stables, M., Toor, I., van Rooijen, N., et al. (2008). Resolution-phase macrophages possess a unique inflammatory phenotype that is controlled by cAMP. Blood 112, 4117–4127. doi:10.1182/blood-2007-12-129767
Caberoy, N. B., Alvarado, G., Bigcas, J.-L., and Li, W. (2012). Galectin-3 is a new MerTK-specific eat-me signal. J. Cell. Physiol. 227, 401–407. doi:10.1002/jcp.22955
Carballo, E., Lai, W. S., and Blackshear, P. J. (1998). Feedback inhibition of macrophage tumor necrosis factor-alpha production by tristetraprolin. Science 281, 1001–1005. doi:10.1126/science.281.5379.1001
Carballo, E., Lai, W. S., and Blackshear, P. J. (2000). Evidence that tristetraprolin is a physiological regulator of granulocyte-macrophage colony-stimulating factor messenger RNA deadenylation and stability. Blood 95, 1891–1899. doi:10.1182/blood.v95.6.1891
Cash, J. L., Bena, S., Headland, S. E., McArthur, S., Brancaleone, V., and Perretti, M. (2013). Chemerin15 inhibits neutrophil-mediated vascular inflammation and myocardial ischemia-reperfusion injury through ChemR23. EMBO Rep. 14, 999–1007. doi:10.1038/embor.2013.138
Ceppi, M., Pereira, P. M., Dunand-Sauthier, I., Barras, E., Reith, W., Santos, M. A., et al. (2009). MicroRNA-155 modulates the interleukin-1 signaling pathway in activated human monocyte-derived dendritic cells. Proc. Natl. Acad. Sci. U. S. A. 106, 2735–2740. doi:10.1073/pnas.0811073106
Chaim, F. H. M., Pascoal, L. B., de Castro, M. M., Palma, B. B., Rodrigues, B. L., Fagundes, J. J., et al. (2024). The resolvin D2 and omega-3 polyunsaturated fatty acid as a new possible therapeutic approach for inflammatory bowel diseases. Sci. Rep. 14, 28698. doi:10.1038/s41598-024-80051-8
Chapman, M. S., and Miner, J. N. (2011). Novel mitogen-activated protein kinase kinase inhibitors. Expert Opin. Investig. Drugs 20, 209–220. doi:10.1517/13543784.2011.548803
Chello, M., Mastroroberto, P., Marchese, A. R., Maltese, G., Santangelo, E., and Amantea, B. (1998). Nitric oxide inhibits neutrophil adhesion during experimental extracorporeal circulation. Anesthesiology 89, 443–448. doi:10.1097/00000542-199808000-00021
Chen, S., Saeed, A. F. U. H., Liu, Q., Jiang, Q., Xu, H., Xiao, G. G., et al. (2023). Macrophages in immunoregulation and therapeutics. Sig Transduct. Target Ther. 8, 207–235. doi:10.1038/s41392-023-01452-1
Chiang, N., Serhan, C. N., Dahlén, S.-E., Drazen, J. M., Hay, D. W. P., Rovati, G. E., et al. (2006). The lipoxin receptor ALX: potent ligand-specific and stereoselective actions in vivo. Pharmacol. Rev. 58, 463–487. doi:10.1124/pr.58.3.4
Chiurchiù, V., Leuti, A., Dalli, J., Jacobsson, A., Battistini, L., Maccarrone, M., et al. (2016). Pro-resolving lipid mediators Resolvin D1, Resolvin D2 and Maresin 1 are critical in modulating T cell responses. Sci. Transl. Med. 8, 353ra111. doi:10.1126/scitranslmed.aaf7483
Cholkar, K., Trinh, H. M., Vadlapudi, A. D., Wang, Z., Pal, D., and Mitra, A. K. (2015). Interaction studies of Resolvin E1 Analog (RX-10045) with efflux transporters. J. Ocular Pharmacol. Ther. 31, 248–255. doi:10.1089/jop.2014.0144
Cirino, G., Cicala, C., Sorrentino, L., Ciliberto, G., Arpaia, G., Perretti, M., et al. (1993). Anti-inflammatory actions of an N-terminal peptide from human lipocortin 1. Br. J. Pharmacol. 108, 573–574. doi:10.1111/j.1476-5381.1993.tb12843.x
Coll, R. C., Robertson, A. A. B., Chae, J. J., Higgins, S. C., Muñoz-Planillo, R., Inserra, M. C., et al. (2015). A small-molecule inhibitor of the NLRP3 inflammasome for the treatment of inflammatory diseases. Nat. Med. 21, 248–255. doi:10.1038/nm.3806
Cotran, R. S., Kumar, V., and Collins, T. (1999). Robbins pathologic basis of disease. Philadelphia, PA: W.B. Saunders Company, xv–1425.
Dean, R. A., Cox, J. H., Bellac, C. L., Doucet, A., Starr, A. E., and Overall, C. M. (2008). Macrophage-specific metalloelastase (MMP-12) truncates and inactivates ELR+ CXC chemokines and generates CCL2, -7, -8, and -13 antagonists: potential role of the macrophage in terminating polymorphonuclear leukocyte influx. Blood 112, 3455–3464. doi:10.1182/blood-2007-12-129080
Dean, R. A., and Overall, C. M. (2007). Proteomics discovery of metalloproteinase substrates in the cellular context by iTRAQ labeling reveals a diverse MMP-2 substrate degradome. Mol. Cell. Proteomics 6, 611–623. doi:10.1074/mcp.M600341-MCP200
Degraaf, A. J., Zasłona, Z., Bourdonnay, E., and Peters-Golden, M. (2014). Prostaglandin E2 reduces toll-like receptor 4 expression in alveolar macrophages by inhibition of translation. Am. J. Respir. Cell. Mol. Biol. 51, 242–250. doi:10.1165/rcmb.2013-0495OC
Devitt, A., Moffatt, O. D., Raykundalia, C., Capra, J. D., Simmons, D. L., and Gregory, C. D. (1998). Human CD14 mediates recognition and phagocytosis of apoptotic cells. Nature 392, 505–509. doi:10.1038/33169
Divanovic, S., Trompette, A., Atabani, S. F., Madan, R., Golenbock, D. T., Visintin, A., et al. (2005). Negative regulation of Toll-like receptor 4 signaling by the Toll-like receptor homolog RP105. Nat. Immunol. 6, 571–578. doi:10.1038/ni1198
Du, H., You, L., Wu, A., Wang, F., Yu, J., and Chen, C. (2024). Resolvin D1 inhibits IL-6-induced epithelial-mesenchymal transition of colorectal cancer cells by targeting IL-6/STAT3 signaling. Cell. Biochem. Biophys. 82, 1453–1461. doi:10.1007/s12013-024-01299-5
El Kebir, D., Gjorstrup, P., and Filep, J. G. (2012). Resolvin E1 promotes phagocytosis-induced neutrophil apoptosis and accelerates resolution of pulmonary inflammation. Proc. Natl. Acad. Sci. U. S. A. 109, 14983–14988. doi:10.1073/pnas.1206641109
Elliott, M. R., Chekeni, F. B., Trampont, P. C., Lazarowski, E. R., Kadl, A., Walk, S. F., et al. (2009). Nucleotides released by apoptotic cells act as a find-me signal to promote phagocytic clearance. Nature 461, 282–286. doi:10.1038/nature08296
Elliott, M. R., and Ravichandran, K. S. (2016). The dynamics of apoptotic cell clearance. Dev. Cell. 38, 147–160. doi:10.1016/j.devcel.2016.06.029
El-Sharkawy, H., Aboelsaad, N., Eliwa, M., Darweesh, M., Alshahat, M., Kantarci, A., et al. (2010). Adjunctive treatment of chronic periodontitis with daily dietary supplementation with omega-3 fatty acids and low-dose aspirin. J. Periodontology 81, 1635–1643. doi:10.1902/jop.2010.090628
Erriah, M., Pabreja, K., Fricker, M., Baines, K. J., Donnelly, L. E., Bylund, J., et al. (2019). Galectin-3 enhances monocyte-derived macrophage efferocytosis of apoptotic granulocytes in asthma. Respir. Res. 20, 1. doi:10.1186/s12931-018-0967-9
Ersland, K., Wüthrich, M., and Klein, B. S. (2010). Dynamic interplay among monocyte-derived, dermal, and resident lymph node dendritic cells during the generation of vaccine immunity to fungi. Cell. Host Microbe 7, 474–487. doi:10.1016/j.chom.2010.05.010
Filep, J. G. (2022). Targeting neutrophils for promoting the resolution of inflammation. Front. Immunol. 13, 866747. doi:10.3389/fimmu.2022.866747
Firestein, G. S. (2003). Evolving concepts of rheumatoid arthritis. Nature 423, 356–361. doi:10.1038/nature01661
Francos-Quijorna, I., Santos-Nogueira, E., Gronert, K., Sullivan, A. B., Kopp, M. A., Brommer, B., et al. (2017). Maresin 1 promotes inflammatory resolution, neuroprotection, and functional neurological recovery after spinal cord injury. J. Neurosci. 37, 11731–11743. doi:10.1523/JNEUROSCI.1395-17.2017
Freire, M. O., and Van Dyke, T. E. (2013). Natural resolution of inflammation. Periodontol. 2000 63, 149–164. doi:10.1111/prd.12034
Fullerton, J. N., and Gilroy, D. W. (2016). Resolution of inflammation: a new therapeutic frontier. Nat. Rev. Drug Discov. 15, 551–567. doi:10.1038/nrd.2016.39
Gao, J., Su, Y., and Wang, Z. (2023a). Lung inflammation resolution by RvD1 and RvD2 in a receptor-dependent manner. Pharmaceutics 15, 1527. doi:10.3390/pharmaceutics15051527
Gao, X.-W., Hu, H.-L., Xie, M.-H., Tang, C.-X., Ou, J., and Lu, Z.-H. (2023b). CX3CL1/CX3CR1 axis alleviates inflammation and apoptosis in human nucleus pulpous cells via M2 macrophage polarization. Exp. Ther. Med. 26, 359. doi:10.3892/etm.2023.12058
Gearing, A. J., Beckett, P., Christodoulou, M., Churchill, M., Clements, J., Davidson, A. H., et al. (1994). Processing of tumour necrosis factor-alpha precursor by metalloproteinases. Nature 370, 555–557. doi:10.1038/370555a0
Graham, G. J., and Locati, M. (2013). Regulation of the immune and inflammatory responses by the “atypical” chemokine receptor D6. J. Pathology 229, 168–175. doi:10.1002/path.4123
Grazda, R., Seyfried, A. N., Maddipati, K. R., Fredman, G., and MacNamara, K. C. (2024). Resolvin E1 improves efferocytosis and rescues severe aplastic anemia in mice. Cell. Death Dis. 15, 324–411. doi:10.1038/s41419-024-06705-7
Groenen, A. G., Lipscomb, M., Bossardi Ramos, R., Sadhu, S., Bazioti, V., Fredman, G., et al. (2024). Resolvin D1 suppresses macrophage senescence and splenic fibrosis in aged mice. Prostagl. Leukot. Essent. Fat. Acids 202, 102634. doi:10.1016/j.plefa.2024.102634
Gude, D. R., Alvarez, S. E., Paugh, S. W., Mitra, P., Yu, J., Griffiths, R., et al. (2008). Apoptosis induces expression of sphingosine kinase 1 to release sphingosine-1-phosphate as a “come-and-get-me” signal. FASEB J. 22, 2629–2638. doi:10.1096/fj.08-107169
Hajishengallis, G., and Chavakis, T. (2019). DEL-1-regulated immune plasticity and inflammatory disorders. Trends Mol. Med. 25, 444–459. doi:10.1016/j.molmed.2019.02.010
Hanayama, R., Tanaka, M., Miwa, K., Shinohara, A., Iwamatsu, A., and Nagata, S. (2002). Identification of a factor that links apoptotic cells to phagocytes. Nature 417, 182–187. doi:10.1038/417182a
Hansen, W. R., Keelan, J. A., Skinner, S. J. M., and Mitchell, M. D. (1999). Key enzymes of prostaglandin biosynthesis and metabolism. Coordinate regulation of expression by cytokines in gestational tissues: a review. Prostagl. & Other Lipid Mediat. 57, 243–257. doi:10.1016/S0090-6980(99)00008-8
Hassanshahi, A., Moradzad, M., Ghalamkari, S., Fadaei, M., Cowin, A. J., and Hassanshahi, M. (2022). Macrophage-mediated inflammation in skin wound healing. Cells 11, 2953. doi:10.3390/cells11192953
Hasturk, H., Schulte, F., Martins, M., Sherzai, H., Floros, C., Cugini, M., et al. (2021). Safety and preliminary efficacy of a novel host-modulatory therapy for reducing gingival inflammation. Front. Immunol. 12, 704163. doi:10.3389/fimmu.2021.704163
He, Y., Feng, D., Li, M., Gao, Y., Ramirez, T., Cao, H., et al. (2017). Hepatic mitochondrial DNA/Toll-like receptor 9/MicroRNA-223 forms a negative feedback loop to limit neutrophil overactivation and acetaminophen hepatotoxicity in mice. Hepatology 66, 220–234. doi:10.1002/hep.29153
He, Z., Chen, W., Hu, K., Luo, Y., Zeng, W., He, X., et al. (2024). Resolvin D1 delivery to lesional macrophages using antioxidative black phosphorus nanosheets for atherosclerosis treatment. Nat. Nanotechnol. 19, 1386–1398. doi:10.1038/s41565-024-01687-1
Heidland, A., Klassen, A., Rutkowski, P., and Bahner, U. (2006). The contribution of Rudolf Virchow to the concept of inflammation: what is still of importance? J. Nephrol. 19 (10), S102–S109.
Hesampour, F., Bernstein, C. N., and Ghia, J.-E. (2024). Brain-gut Axis: invasive and noninvasive vagus nerve stimulation, limitations, and potential therapeutic approaches. Inflamm. Bowel Dis. 30, 482–495. doi:10.1093/ibd/izad211
Hodges, R. J., Jenkins, R. G., Wheeler-Jones, C. P. D., Copeman, D. M., Bottoms, S. E., Bellingan, G. J., et al. (2004). Severity of lung injury in cyclooxygenase-2-deficient mice is dependent on reduced prostaglandin E(2) production. Am. J. Pathol. 165, 1663–1676. doi:10.1016/S0002-9440(10)63423-2
Hu, Y., Hu, F. B., and Manson, J. E. (2019). Marine omega-3 supplementation and cardiovascular disease: an updated meta-analysis of 13 randomized controlled trials involving 127 477 participants. J. Am. Heart Assoc. 8, e013543. doi:10.1161/JAHA.119.013543
Inomata, R., Tsubouchi, H., Takao, T., Kurokawa, M., Yanagi, S., Sakai, K., et al. (2024). Resolvin D4 mitigates lipopolysaccharide-induced lung injury in mice. Prostagl. Leukot. Essent. Fat. Acids 203, 102652. doi:10.1016/j.plefa.2024.102652
Ionescu, L., Byrne, R. N., van Haaften, T., Vadivel, A., Alphonse, R. S., Rey-Parra, G. J., et al. (2012). Stem cell conditioned medium improves acute lung injury in mice: in vivo evidence for stem cell paracrine action. Am. J. Physiol. Lung Cell. Mol. Physiol. 303, L967–L977. doi:10.1152/ajplung.00144.2011
Iqbal, A. J., Sampaio, A. L. F., Maione, F., Greco, K. V., Niki, T., Hirashima, M., et al. (2011). Endogenous galectin-1 and acute inflammation: emerging notion of a galectin-9 pro-resolving effect. Am. J. Pathology 178, 1201–1209. doi:10.1016/j.ajpath.2010.11.073
Ishida, T., Yoshida, M., Arita, M., Nishitani, Y., Nishiumi, S., Masuda, A., et al. (2010). Resolvin E1, an endogenous lipid mediator derived from eicosapentaenoic acid, prevents dextran sulfate sodium induced colitis. Inflamm. Bowel Dis. 16, 87–95. doi:10.1002/ibd.21029
Jackson, C. D., Hilliard, K. A., and Brown, C. R. (2023). 12/15-lipoxygenase activity promotes efficient inflammation resolution in a murine model of Lyme arthritis. Front. Immunol. 14, 1144172. doi:10.3389/fimmu.2023.1144172
Ji, L., Liu, G., Yu, G., Xia, C., Liu, S., and Lan, Y. (2024). Resolvin E1 and inhibition of BLT2 signaling attenuate the inflammatory response and improve one-lung ventilation-induced lung injury. Immunol. Invest. 53, 1293–1307. doi:10.1080/08820139.2024.2399587
Jin, F., Li, J., Guo, J., Doeppner, T. R., Hermann, D. M., Yao, G., et al. (2021). Targeting epigenetic modifiers to reprogramme macrophages in non-resolving inflammation-driven atherosclerosis. Eur. Heart J. Open 1, oeab022. doi:10.1093/ehjopen/oeab022
Jung, M., Ma, Y., Iyer, R. P., DeLeon-Pennell, K. Y., Yabluchanskiy, A., Garrett, M. R., et al. (2017). IL-10 improves cardiac remodeling after myocardial infarction by stimulating M2 macrophage polarization and fibroblast activation. Basic Res. Cardiol. 112, 33. doi:10.1007/s00395-017-0622-5
Junttila, M. R., Li, S.-P., and Westermarck, J. (2008). Phosphatase-mediated crosstalk between MAPK signaling pathways in the regulation of cell survival. FASEB J. 22, 954–965. doi:10.1096/fj.06-7859rev
Kapellos, T. S., and Iqbal, A. J. (2016). Epigenetic control of macrophage polarisation and soluble mediator gene expression during inflammation. Mediat. Inflamm. 2016, 6591703. doi:10.1155/2016/6591703
Kariminezhad, Z., Rahimi, M., Fernandes, J., Maltais, R., Sancéau, J.-Y., Poirier, D., et al. (2024). Development of new resolvin D1 analogues for osteoarthritis therapy: acellular and computational approaches to study their antioxidant activities. Antioxidants 13, 386. doi:10.3390/antiox13040386
Katakami, Y., Nakao, Y., Koizumi, T., Katakami, N., Ogawa, R., and Fujita, T. (1988). Regulation of tumour necrosis factor production by mouse peritoneal macrophages: the role of cellular cyclic AMP. Immunology 64, 719–724.
Kobayashi, N., Karisola, P., Peña-Cruz, V., Dorfman, D. M., Jinushi, M., Umetsu, S. E., et al. (2007). TIM-1 and TIM-4 glycoproteins bind phosphatidylserine and mediate uptake of apoptotic cells. Immunity 27, 927–940. doi:10.1016/j.immuni.2007.11.011
Kobayashi, Y. (2010). The regulatory role of nitric oxide in proinflammatory cytokine expression during the induction and resolution of inflammation. J. Leukoc. Biol. 88, 1157–1162. doi:10.1189/jlb.0310149
Kovalenko, A., Chable-Bessia, C., Cantarella, G., Israël, A., Wallach, D., and Courtois, G. (2003). The tumour suppressor CYLD negatively regulates NF-kappaB signalling by deubiquitination. Nature 424, 801–805. doi:10.1038/nature01802
Kumar, R., Clermont, G., Vodovotz, Y., and Chow, C. C. (2004). The dynamics of acute inflammation. J. Theor. Biol. 230, 145–155. doi:10.1016/j.jtbi.2004.04.044
Kumar, V., Abbas, A. K., Aster, J. C., Cotran, R. S., and Robbins, S. L. (2021). Robbins & Cotran pathologic basis of disease. 10th edition. Philadelphia, PA: Elsevier. Available online at: https://virtual.anu.edu.au/login/?url=https://www.clinicalkey.com/dura/browse/bookChapter/3-s2.0-C20160040871.
Kunkel, S. L., Chensue, S. W., and Phan, S. H. (1986). Prostaglandins as endogenous mediators of interleukin 1 production. J. Immunol. 136, 186–192. doi:10.4049/jimmunol.136.1.186
Langereis, J. D., Raaijmakers, H. A. J. A., Ulfman, L. H., and Koenderman, L. (2010). Abrogation of NF-κB signaling in human neutrophils induces neutrophil survival through sustained p38-MAPK activation. J. Leukoc. Biol. 88, 655–664. doi:10.1189/jlb.0809544
Lauber, K., Bohn, E., Kröber, S. M., Xiao, Y., Blumenthal, S. G., Lindemann, R. K., et al. (2003). Apoptotic cells induce migration of phagocytes via caspase-3-mediated release of a lipid attraction signal. Cell. 113, 717–730. doi:10.1016/s0092-8674(03)00422-7
Lavy, M., Gauttier, V., Poirier, N., Barillé-Nion, S., and Blanquart, C. (2021). Specialized pro-resolving mediators mitigate cancer-related inflammation: role of tumor-associated macrophages and therapeutic opportunities. Front. Immunol. 12, 702785. doi:10.3389/fimmu.2021.702785
Law, H. L., Wright, R. D., Iqbal, A. J., Norling, L. V., and Cooper, D. (2020). A pro-resolving role for galectin-1 in acute inflammation. Front. Pharmacol. 11, 274. doi:10.3389/fphar.2020.00274
Lawrence, T., and Fong, C. (2010). The resolution of inflammation: anti-inflammatory roles for NF-κB. Int. J. Biochem. & Cell. Biol. 42, 519–523. doi:10.1016/j.biocel.2009.12.016
Lee, H.-N., Kundu, J. K., Cha, Y.-N., and Surh, Y.-J. (2013). Resolvin D1 stimulates efferocytosis through p50/p50-mediated suppression of tumor necrosis factor-α expression. J. Cell. Sci. 126, 4037–4047. doi:10.1242/jcs.131003
Leitch, A. E., Lucas, C. D., Marwick, J. A., Duffin, R., Haslett, C., and Rossi, A. G. (2012). Cyclin-dependent kinases 7 and 9 specifically regulate neutrophil transcription and their inhibition drives apoptosis to promote resolution of inflammation. Cell. Death Differ. 19, 1950–1961. doi:10.1038/cdd.2012.80
Leitch, A. E., Riley, N. A., Sheldrake, T. A., Festa, M., Fox, S., Duffin, R., et al. (2010). The cyclin-dependent kinase inhibitor R-roscovitine down-regulates Mcl-1 to override pro-inflammatory signalling and drive neutrophil apoptosis. Eur. J. Immunol. 40, 1127–1138. doi:10.1002/eji.200939664
Lemke, G., and Burstyn-Cohen, T. (2010). TAM receptors and the clearance of apoptotic cells. Ann. N. Y. Acad. Sci. 1209, 23–29. doi:10.1111/j.1749-6632.2010.05744.x
Liang, J., Wang, J., Azfer, A., Song, W., Tromp, G., Kolattukudy, P. E., et al. (2008). A novel CCCH-zinc finger protein family regulates proinflammatory activation of macrophages. J. Biol. Chem. 283, 6337–6346. doi:10.1074/jbc.M707861200
Li, J., Liu, Y.-H., Ou, S., Dai, X.-M., Wang, J.-P., and Su, Y.-P. (2012). Steroid receptor coactivator-3 differentially regulates the inflammatory response in peritoneal macrophages. Mol. Med. Rep. 5, 1099–1105. doi:10.3892/mmr.2012.750
Linker, K., Pautz, A., Fechir, M., Hubrich, T., Greeve, J., and Kleinert, H. (2005). Involvement of KSRP in the post-transcriptional regulation of human iNOS expression-complex interplay of KSRP with TTP and HuR. Nucleic Acids Res. 33, 4813–4827. doi:10.1093/nar/gki797
Lipscomb, M., Walis, S., Marinello, M., Mena, H. A., MacNamara, K. C., Spite, M., et al. (2024). Resolvin D2 limits atherosclerosis progression via myeloid cell-GPR18. FASEB J. 38, e23555. doi:10.1096/fj.202302336RR
Liu, J., Zhang, Z., Chai, L., Che, Y., Min, S., and Yang, R. (2013). Identification and characterization of a unique leucine-rich repeat protein (LRRC33) that inhibits Toll-like receptor-mediated NF-κB activation. Biochem. Biophy. Res. Commun. 434, 28–34. doi:10.1016/j.bbrc.2013.03.071
MacKenzie, K. F., Clark, K., Naqvi, S., McGuire, V. A., Nöehren, G., Kristariyanto, Y., et al. (2013). PGE2 induces macrophage IL-10 production and a regulatory-like phenotype via a protein kinase A–SIK–CRTC3 pathway. J. Immunol. 190, 565–577. doi:10.4049/jimmunol.1202462
Maddox, J. F., and Serhan, C. N. (1996). Lipoxin A4 and B4 are potent stimuli for human monocyte migration and adhesion: selective inactivation by dehydrogenation and reduction. J. Exp. Med. 183, 137–146. doi:10.1084/jem.183.1.137
Maderna, P., Cottell, D. C., Toivonen, T., Dufton, N., Dalli, J., Perretti, M., et al. (2010). FPR2/ALX receptor expression and internalization are critical for lipoxin A4 and annexin-derived peptide-stimulated phagocytosis. FASEB J. 24, 4240–4249. doi:10.1096/fj.10-159913
Maderna, P., Yona, S., Perretti, M., and Godson, C. (2005). Modulation of phagocytosis of apoptotic neutrophils by supernatant from dexamethasone-treated macrophages and annexin-derived peptide Ac(2-26). J. Immunol. 174, 3727–3733. doi:10.4049/jimmunol.174.6.3727
Mangan, M. S. J., Olhava, E. J., Roush, W. R., Seidel, H. M., Glick, G. D., and Latz, E. (2018). Targeting the NLRP3 inflammasome in inflammatory diseases. Nat. Rev. Drug Discov. 17, 588–606. doi:10.1038/nrd.2018.97
Mazumder, B., Li, X., and Barik, S. (2010). Translation control: a multifaceted regulator of inflammatory response. J. Immunol. 184, 3311–3319. doi:10.4049/jimmunol.0903778
McArthur, S., Juban, G., Gobbetti, T., Desgeorges, T., Theret, M., Gondin, J., et al. (2020). Annexin A1 drives macrophage skewing to accelerate muscle regeneration through AMPK activation. J. Clin. Invest. 130, 1156–1167. doi:10.1172/JCI124635
McQuibban, G. A., Gong, J. H., Tam, E. M., McCulloch, C. A., Clark-Lewis, I., and Overall, C. M. (2000). Inflammation dampened by gelatinase A cleavage of monocyte chemoattractant protein-3. Science 289, 1202–1206. doi:10.1126/science.289.5482.1202
Medeiros, A. I., Serezani, C. H., Lee, S. P., and Peters-Golden, M. (2009). Efferocytosis impairs pulmonary macrophage and lung antibacterial function via PGE2/EP2 signaling. J. Exp. Med. 206, 61–68. doi:10.1084/jem.20082058
Medzhitov, R., and Janeway, C. (2000). Innate immunity. N. Engl. J. Med. 343, 338–344. doi:10.1056/NEJM200008033430506
Merlin, J., Ivanov, S., Dumont, A., Sergushichev, A., Gall, J., Stunault, M., et al. (2021). Non-canonical glutamine transamination sustains efferocytosis by coupling redox buffering to oxidative phosphorylation. Nat. Metab. 3, 1313–1326. doi:10.1038/s42255-021-00471-y
Mevorach, D., Mascarenhas, J. O., Gershov, D., and Elkon, K. B. (1998). Complement-dependent clearance of apoptotic cells by human macrophages. J. Exp. Med. 188, 2313–2320. doi:10.1084/jem.188.12.2313
Miao, L., Shen, X., Whiteman, M., Xin, H., Shen, Y., Xin, X., et al. (2016). Hydrogen sulfide mitigates myocardial infarction via promotion of mitochondrial biogenesis-dependent M2 polarization of macrophages. Antioxid. Redox Signal 25, 268–281. doi:10.1089/ars.2015.6577
Miles, E. A., and Calder, P. C. (2012). Influence of marine n-3 polyunsaturated fatty acids on immune function and a systematic review of their effects on clinical outcomes in rheumatoid arthritis. Br. J. Nutr. 107, S171–S184. doi:10.1017/S0007114512001560
Mısırlıoglu, N. F., Ergun, S., Kucuk, S. H., Himmetoglu, S., Ozen, G. D., Sayili, U., et al. (2025). The importance of resolvin D1, LXA4, and LTB4 in patients with acute pancreatitis due to gallstones. Med. (Kaunas) 61, 239. doi:10.3390/medicina61020239
Mitchell, S., Thomas, G., Harvey, K., Cottell, D., Reville, K., Berlasconi, G., et al. (2002). Lipoxins, aspirin-triggered epi-lipoxins, lipoxin stable analogues, and the resolution of inflammation: stimulation of macrophage phagocytosis of apoptotic neutrophils in vivo. J. Am. Soc. Nephrol. 13, 2497–2507. doi:10.1097/01.asn.0000032417.73640.72
Moelants, E. A. V., Mortier, A., Van Damme, J., and Proost, P. (2013). In vivo regulation of chemokine activity by post-translational modification. Immunol. Cell. Biol. 91, 402–407. doi:10.1038/icb.2013.16
Moffatt, O. D., Devitt, A., Bell, E. D., Simmons, D. L., and Gregory, C. D. (1999). Macrophage recognition of ICAM-3 on apoptotic leukocytes. J. Immunol. 162, 6800–6810. doi:10.4049/jimmunol.162.11.6800
Mogensen, T. H. (2009). Pathogen recognition and inflammatory signaling in innate immune defenses. Clin. Microbiol. Rev. 22, 240–273. doi:10.1128/CMR.00046-08
Mukhopadhyay, R., Jia, J., Arif, A., Ray, P. S., and Fox, P. L. (2009). The GAIT system: a gatekeeper of inflammatory gene expression. Trends Biochem. Sci. 34, 324–331. doi:10.1016/j.tibs.2009.03.004
Nagarkatti, P., Pandey, R., Rieder, S. A., Hegde, V. L., and Nagarkatti, M. (2009). Cannabinoids as novel anti-inflammatory drugs. Future Med. Chem. 1, 1333–1349. doi:10.4155/fmc.09.93
Nakano, H., Lin, K. L., Yanagita, M., Charbonneau, C., Cook, D. N., Kakiuchi, T., et al. (2009). Blood-derived inflammatory dendritic cells in lymph nodes stimulate acute T helper type 1 immune responses. Nat. Immunol. 10, 394–402. doi:10.1038/ni.1707
Napimoga, M. H., Vieira, S. M., Dal-Secco, D., Freitas, A., Souto, F. O., Mestriner, F. L., et al. (2008). Peroxisome proliferator-activated receptor-γ ligand, 15-deoxy-Δ12,14-prostaglandin J2, reduces neutrophil migration via a nitric oxide pathway. J. Immunol. 180, 609–617. doi:10.4049/jimmunol.180.1.609
Németh, K., Leelahavanichkul, A., Yuen, P. S. T., Mayer, B., Parmelee, A., Doi, K., et al. (2009). Bone marrow stromal cells attenuate sepsis via prostaglandin E2—dependent reprogramming of host macrophages to increase their interleukin-10 production. Nat. Med. 15, 42–49. doi:10.1038/nm.1905
Newson, J., Stables, M., Karra, E., Arce-Vargas, F., Quezada, S., Motwani, M., et al. (2014). Resolution of acute inflammation bridges the gap between innate and adaptive immunity. Blood 124, 1748–1764. doi:10.1182/blood-2014-03-562710
Nguyen-Chi, M., Luz-Crawford, P., Balas, L., Sipka, T., Contreras-López, R., Barthelaix, A., et al. (2020). Pro-resolving mediator protectin D1 promotes epimorphic regeneration by controlling immune cell function in vertebrates. Br. J. Pharmacol. 177, 4055–4073. doi:10.1111/bph.15156
Nibbs, R. J. B., and Graham, G. J. (2013). Immune regulation by atypical chemokine receptors. Nat. Rev. Immunol. 13, 815–829. doi:10.1038/nri3544
Obora, K., Onodera, Y., Takehara, T., Frampton, J., Hasei, J., Ozaki, T., et al. (2017). Inflammation-induced miRNA-155 inhibits self-renewal of neural stem cells via suppression of CCAAT/enhancer binding protein β (C/EBPβ) expression. Sci. Rep. 7, 43604. doi:10.1038/srep43604
Ogilvie, R. L., Abelson, M., Hau, H. H., Vlasova, I., Blackshear, P. J., and Bohjanen, P. R. (2005). Tristetraprolin down-regulates IL-2 gene expression through AU-rich element-mediated mRNA decay. J. Immunol. 174, 953–961. doi:10.4049/jimmunol.174.2.953
Ogilvie, R. L., SternJohn, J. R., Rattenbacher, B., Vlasova, I. A., Williams, D. A., Hau, H. H., et al. (2009). Tristetraprolin mediates interferon-γ mRNA decay. J. Biol. Chem. 284, 11216–11223. doi:10.1074/jbc.M901229200
Oner, F., Alvarez, C., Yaghmoor, W., Stephens, D., Hasturk, H., Firatli, E., et al. (2021). Resolvin E1 regulates Th17 function and T cell activation. Front. Immunol. 12, 637983. doi:10.3389/fimmu.2021.637983
Orr, S. K., Colas, R. A., Dalli, J., Chiang, N., and Serhan, C. N. (2015). Proresolving actions of a new resolvin D1 analog mimetic qualifies as an immunoresolvent. Am. J. Physiology-Lung Cell. Mol. Physiology 308, L904–L911. doi:10.1152/ajplung.00370.2014
Ortega-Gómez, A., Perretti, M., and Soehnlein, O. (2013). Resolution of inflammation: an integrated view. EMBO Mol. Med. 5, 661–674. doi:10.1002/emmm.201202382
Ou, G., Liu, Q., Yu, C., Chen, X., Zhang, W., Chen, Y., et al. (2021). The protective effects of maresin 1 in the OVA-induced asthma mouse model. Mediat. Inflamm. 2021, 4131420. doi:10.1155/2021/4131420
Padovani, C. M., Wilson, R. M., Rodriguez, A., Spur, B. W., and Yin, K. (2024). Resolvin D2 attenuates LPS-induced macrophage exhaustion. FASEB J. 38, e23569. doi:10.1096/fj.202302521R
Paland, N., Pechkovsky, A., Aswad, M., Hamza, H., Popov, T., Shahar, E., et al. (2021). The immunopathology of COVID-19 and the cannabis paradigm. Front. Immunol. 12, 631233. doi:10.3389/fimmu.2021.631233
Panigrahy, D., Gilligan, M. M., Serhan, C. N., and Kashfi, K. (2021). Resolution of inflammation: an organizing principle in biology and medicine. Pharmacol. & Ther. 227, 107879. doi:10.1016/j.pharmthera.2021.107879
Park, S.-Y., Jung, M.-Y., Kim, H.-J., Lee, S.-J., Kim, S.-Y., Lee, B.-H., et al. (2008). Rapid cell corpse clearance by stabilin-2, a membrane phosphatidylserine receptor. Cell. Death Differ. 15, 192–201. doi:10.1038/sj.cdd.4402242
Peiseler, M., and Kubes, P. (2019). More friend than foe: the emerging role of neutrophils in tissue repair. J. Clin. Invest. 129, 2629–2639. doi:10.1172/JCI124616
Perdiguero, E., Kharraz, Y., Serrano, A. L., and Muñoz-Cánoves, P. (2012). MKP-1 coordinates ordered macrophage-phenotype transitions essential for stem cell-dependent tissue repair. Cell. Cycle 11, 877–886. doi:10.4161/cc.11.5.19374
Perez-Hernandez, J., Chiurchiù, V., Perruche, S., and You, S. (2021). Regulation of T-cell immune responses by pro-resolving lipid mediators. Front. Immunol. 12, 768133. doi:10.3389/fimmu.2021.768133
Perretti, M., and Dalli, J. (2023). Resolution pharmacology: focus on pro-resolving annexin A1 and lipid mediators for therapeutic innovation in inflammation. Annu. Rev. Pharmacol. Toxicol. 63, 449–469. doi:10.1146/annurev-pharmtox-051821-042743
Phillips, K., Kedersha, N., Shen, L., Blackshear, P. J., and Anderson, P. (2004). Arthritis suppressor genes TIA-1 and TTP dampen the expression of tumor necrosis factor alpha, cyclooxygenase 2, and inflammatory arthritis. Proc. Natl. Acad. Sci. U. S. A. 101, 2011–2016. doi:10.1073/pnas.0400148101
Piecyk, M., Wax, S., Beck, A. R., Kedersha, N., Gupta, M., Maritim, B., et al. (2000). TIA-1 is a translational silencer that selectively regulates the expression of TNF-alpha. EMBO J. 19, 4154–4163. doi:10.1093/emboj/19.15.4154
Pollock, J. D., Williams, D. A., Gifford, M. A. C., Li, L. L., Du, X., Fisherman, J., et al. (1995). Mouse model of X–linked chronic granulomatous disease, an inherited defect in phagocyte superoxide production. Nat. Genet. 9, 202–209. doi:10.1038/ng0295-202
Poon, I. K. H., Lucas, C. D., Rossi, A. G., and Ravichandran, K. S. (2014). Apoptotic cell clearance: basic biology and therapeutic potential. Nat. Rev. Immunol. 14, 166–180. doi:10.1038/nri3607
Rankin, J. A. (2004). Biological mediators of acute inflammation. AACN Adv. Crit. Care 15, 3–17. doi:10.1097/00044067-200401000-00002
Rao, S. P., Ge, X. N., and Sriramarao, P. (2017). Regulation of eosinophil recruitment and activation by galectins in allergic asthma. Front. Med. (Lausanne) 4, 68. doi:10.3389/fmed.2017.00068
Remijsen, Q., Kuijpers, T. W., Wirawan, E., Lippens, S., Vandenabeele, P., and Vanden Berghe, T. (2011). Dying for a cause: NETosis, mechanisms behind an antimicrobial cell death modality. Cell. Death Differ. 18, 581–588. doi:10.1038/cdd.2011.1
Resolve Sarl, A. T. (2019). A multicenter, double-masked, parallel-group, vehicle-controlled study to assess the efficacy and safety of RX-10045 nanomicellar ophthalmic solution for treatment of ocular inflammation and pain in subjects undergoing cataract surgery. clinicaltrials.gov. Available online at: https://clinicaltrials.gov/study/NCT02329743 (Accessed February 3, 2025).
Ricciotti, E., and FitzGerald, G. A. (2011). Prostaglandins and inflammation. Arterioscler. Thromb. Vasc. Biol. 31, 986–1000. doi:10.1161/ATVBAHA.110.207449
Rodrigues, D. H., Vilela, M. de C., Barcelos, L. da S., Pinho, V., Teixeira, M. M., and Teixeira, A. L. (2010). Absence of PI3Kγ leads to increased leukocyte apoptosis and diminished severity of experimental autoimmune encephalomyelitis. J. Neuroimmunol. 222, 90–94. doi:10.1016/j.jneuroim.2010.02.016
Roh, J. S., and Sohn, D. H. (2018). Damage-associated molecular patterns in inflammatory diseases. Immune Netw. 18, e27. doi:10.4110/in.2018.18.e27
Rossi, A. G., McCutcheon, J. C., Roy, N., Chilvers, E. R., Haslett, C., and Dransfield, I. (1998). Regulation of macrophage phagocytosis of apoptotic cells by cAMP. J. Immunol. 160, 3562–3568. doi:10.4049/jimmunol.160.7.3562
Rossi, A. G., Sawatzky, D. A., Walker, A., Ward, C., Sheldrake, T. A., Riley, N. A., et al. (2006). Cyclin-dependent kinase inhibitors enhance the resolution of inflammation by promoting inflammatory cell apoptosis. Nat. Med. 12, 1056–1064. doi:10.1038/nm1468
Samuelsson, B. (1983). From studies of biochemical mechanism to novel biological mediators: prostaglandin endoperoxides, thromboxanes, and leukotrienes. Nobel Lecture, 8 December 1982. Biosci. Rep. 3, 791–813. doi:10.1007/BF01133779
Sauer, I., Schaljo, B., Vogl, C., Gattermeier, I., Kolbe, T., Müller, M., et al. (2006). Interferons limit inflammatory responses by induction of tristetraprolin. Blood 107, 4790–4797. doi:10.1182/blood-2005-07-3058
Savill, J. (1997). Apoptosis in resolution of inflammation. J. Leukoc. Biol. 61, 375–380. doi:10.1002/jlb.61.4.375
Savill, J., Hogg, N., Ren, Y., and Haslett, C. (1992). Thrombospondin cooperates with CD36 and the vitronectin receptor in macrophage recognition of neutrophils undergoing apoptosis. J. Clin. Invest. 90, 1513–1522. doi:10.1172/JCI116019
Savill, J. S., Henson, P. M., and Haslett, C. (1989). Phagocytosis of aged human neutrophils by macrophages is mediated by a novel “charge-sensitive” recognition mechanism. J. Clin. Invest. 84, 1518–1527. doi:10.1172/JCI114328
Sawada, Y., Honda, T., Hanakawa, S., Nakamizo, S., Murata, T., Ueharaguchi-Tanada, Y., et al. (2015). Resolvin E1 inhibits dendritic cell migration in the skin and attenuates contact hypersensitivity responses. J. Exp. Med. 212, 1921–1930. doi:10.1084/jem.20150381
Sawatzky, D. A., Willoughby, D. A., Colville-Nash, P. R., and Rossi, A. G. (2006). The involvement of the apoptosis-modulating proteins ERK 1/2, Bcl-xL and Bax in the resolution of acute inflammation in vivo. Am. J. Pathol. 168, 33–41. doi:10.2353/ajpath.2006.050058
Schilperoort, M., Ngai, D., Katerelos, M., Power, D. A., and Tabas, I. (2023a). PFKFB2-mediated glycolysis promotes lactate-driven continual efferocytosis by macrophages. Nat. Metab. 5, 431–444. doi:10.1038/s42255-023-00736-8
Schilperoort, M., Ngai, D., Sukka, S. R., Avrampou, K., Shi, H., and Tabas, I. (2023b). The role of efferocytosis-fueled macrophage metabolism in the resolution of inflammation. Immunol. Rev. 319, 65–80. doi:10.1111/imr.13214
Schmid, T., and Brüne, B. (2021). Prostanoids and resolution of inflammation – beyond the lipid-mediator class switch. Front. Immunol. 12, 714042. doi:10.3389/fimmu.2021.714042
Schmid-Schönbein, G. W. (2006). Analysis of inflammation. Annu. Rev. Biomed. Eng. 8, 93–151. doi:10.1146/annurev.bioeng.8.061505.095708
Schwab, J. M., Chiang, N., Arita, M., and Serhan, C. N. (2007). Resolvin E1 and protectin D1 activate inflammation-resolution programmes. Nature 447, 869–874. doi:10.1038/nature05877
Serhan, C. N. (2005). Lipoxins and aspirin-triggered 15-epi-lipoxins are the first lipid mediators of endogenous anti-inflammation and resolution. Prostagl. Leukot. Essent. Fat. Acids 73, 141–162. doi:10.1016/j.plefa.2005.05.002
Serhan, C. N., Brain, S. D., Buckley, C. D., Gilroy, D. W., Haslett, C., O’Neill, L. A. J., et al. (2007). Resolution of inflammation: state of the art, definitions and terms. FASEB J. 21, 325–332. doi:10.1096/fj.06-7227rev
Serhan, C. N., Dalli, J., Karamnov, S., Choi, A., Park, C.-K., Xu, Z.-Z., et al. (2012). Macrophage proresolving mediator maresin 1 stimulates tissue regeneration and controls pain. FASEB J. 26, 1755–1765. doi:10.1096/fj.11-201442
Serhan, C. N., Hamberg, M., and Samuelsson, B. (1984). Lipoxins: novel series of biologically active compounds formed from arachidonic acid in human leukocytes. Proc. Natl. Acad. Sci. 81, 5335–5339. doi:10.1073/pnas.81.17.5335
Serhan, C. N., and Levy, B. D. (2018). Resolvins in inflammation: emergence of the pro-resolving superfamily of mediators. J. Clin. Invest. 128, 2657–2669. doi:10.1172/JCI97943
Serhan, C. N., and Savill, J. (2005). Resolution of inflammation: the beginning programs the end. Nat. Immunol. 6, 1191–1197. doi:10.1038/ni1276
Shaked, I., Meerson, A., Wolf, Y., Avni, R., Greenberg, D., Gilboa-Geffen, A., et al. (2009). MicroRNA-132 potentiates cholinergic anti-inflammatory signaling by targeting acetylcholinesterase. Immunity 31, 965–973. doi:10.1016/j.immuni.2009.09.019
Shao, W.-H., and Cohen, P. L. (2011). Disturbances of apoptotic cell clearance in systemic lupus erythematosus. Arthritis Res. Ther. 13, 202. doi:10.1186/ar3206
Sheedy, F. J., Palsson-McDermott, E., Hennessy, E. J., Martin, C., O’Leary, J. J., Ruan, Q., et al. (2010). Negative regulation of TLR4 via targeting of the proinflammatory tumor suppressor PDCD4 by the microRNA miR-21. Nat. Immunol. 11, 141–147. doi:10.1038/ni.1828
Sheng, Y.-R., Hu, W.-T., Wei, C.-Y., Tang, L.-L., Liu, Y.-K., Liu, Y.-Y., et al. (2018). IL-33/ST2 axis affects the polarization and efferocytosis of decidual macrophages in early pregnancy. Am. J. Reprod. Immunol. 79, e12836. doi:10.1111/aji.12836
Soliman, A. M., and Barreda, D. R. (2022). Acute inflammation in tissue healing. Int. J. Mol. Sci. 24, 641. doi:10.3390/ijms24010641
Soliman, A. M., and Barreda, D. R. (2023). The acute inflammatory response of teleost fish. Dev. & Comp. Immunol. 146, 104731. doi:10.1016/j.dci.2023.104731
Soliman, A. M., Yoon, T., Wang, J., Stafford, J. L., and Barreda, D. R. (2021). Isolation of skin leukocytes uncovers phagocyte inflammatory responses during induction and resolution of cutaneous inflammation in fish. Front. Immunol. 12, 725063. doi:10.3389/fimmu.2021.725063
Song, G., Ouyang, G., and Bao, S. (2005). The activation of Akt/PKB signaling pathway and cell survival. J. Cell. Mol. Med. 9, 59–71. doi:10.1111/j.1582-4934.2005.tb00337.x
Sousa, L. P., Carmo, A. F., Rezende, B. M., Lopes, F., Silva, D. M., Alessandri, A. L., et al. (2009). Cyclic AMP enhances resolution of allergic pleurisy by promoting inflammatory cell apoptosis via inhibition of PI3K/Akt and NF-kappaB. Biochem. Pharmacol. 78, 396–405. doi:10.1016/j.bcp.2009.04.030
Sousa, L. P., Lopes, F., Silva, D. M., Tavares, L. P., Vieira, A. T., Rezende, B. M., et al. (2010). PDE4 inhibition drives resolution of neutrophilic inflammation by inducing apoptosis in a PKA-PI3K/Akt-dependent and NF-kappaB-independent manner. J. Leukoc. Biol. 87, 895–904. doi:10.1189/jlb.0809540
Spite, M., Clària, J., and Serhan, C. N. (2014). Resolvins, specialized proresolving lipid mediators, and their potential roles in metabolic diseases. Cell. Metab. 19, 21–36. doi:10.1016/j.cmet.2013.10.006
Stalder, A. K., Lott, D., Strasser, D. S., Cruz, H. G., Krause, A., Groenen, P. M. A., et al. (2017). Biomarker-guided clinical development of the first-in-class anti-inflammatory FPR2/ALX agonist ACT-389949. Br. J. Clin. Pharmacol. 83, 476–486. doi:10.1111/bcp.13149
Sugimoto, M. A., Sousa, L. P., Pinho, V., Perretti, M., and Teixeira, M. M. (2016). Resolution of inflammation: what controls its onset? Front. Immunol. 7, 160. doi:10.3389/fimmu.2016.00160
Sun, F., Luo, J., Yue, T., Wang, F., Yang, C., Zhang, S., et al. (2021). The role of hydrogen sulphide signalling in macrophage activation. Immunology 162, 3–10. doi:10.1111/imm.13253
Szukiewicz, D. (2024). CX3CL1 (Fractalkine)-CX3CR1 Axis in inflammation-induced angiogenesis and tumorigenesis. Int. J. Mol. Sci. 25, 4679. doi:10.3390/ijms25094679
Tam, E. M., Morrison, C. J., Wu, Y. I., Stack, M. S., and Overall, C. M. (2004). Membrane protease proteomics: isotope-coded affinity tag MS identification of undescribed MT1–matrix metalloproteinase substrates. Proc. Natl. Acad. Sci. U. S. A. 101, 6917–6922. doi:10.1073/pnas.0305862101
Tang, B., Xiao, B., Liu, Z., Li, N., Zhu, E.-D., Li, B.-S., et al. (2010). Identification of MyD88 as a novel target of miR-155, involved in negative regulation of Helicobacter pylori-induced inflammation. FEBS Lett. 584, 1481–1486. doi:10.1016/j.febslet.2010.02.063
Tili, E., Michaille, J.-J., Cimino, A., Costinean, S., Dumitru, C. D., Adair, B., et al. (2007). Modulation of miR-155 and miR-125b levels following lipopolysaccharide/TNF-alpha stimulation and their possible roles in regulating the response to endotoxin shock. J. Immunol. 179, 5082–5089. doi:10.4049/jimmunol.179.8.5082
Tracy, R. P. (2006). The five cardinal signs of inflammation: calor, dolor, rubor, tumor … and penuria (apologies to aulus cornelius celsus, de medicina, c. A.D. 25). Journals Gerontology Ser. A 61, 1051–1052. doi:10.1093/gerona/61.10.1051
Truman, L. A., Ford, C. A., Pasikowska, M., Pound, J. D., Wilkinson, S. J., Dumitriu, I. E., et al. (2008). CX3CL1/fractalkine is released from apoptotic lymphocytes to stimulate macrophage chemotaxis. Blood 112, 5026–5036. doi:10.1182/blood-2008-06-162404
Vacchini, A., Locati, M., and Borroni, E. M. (2016). Overview and potential unifying themes of the atypical chemokine receptor family. J. Leukoc. Biol. 99, 883–892. doi:10.1189/jlb.2MR1015-477R
Vago, J. P., Nogueira, C. R. C., Tavares, L. P., Soriani, F. M., Lopes, F., Russo, R. C., et al. (2012). Annexin A1 modulates natural and glucocorticoid-induced resolution of inflammation by enhancing neutrophil apoptosis. J. Leukoc. Biol. 92, 249–258. doi:10.1189/jlb.0112008
Vancheri, C., Mastruzzo, C., Sortino, M. A., and Crimi, N. (2004). The lung as a privileged site for the beneficial actions of PGE2. Trends Immunol. 25, 40–46. doi:10.1016/j.it.2003.11.001
Vanden Berghe, T., Linkermann, A., Jouan-Lanhouet, S., Walczak, H., and Vandenabeele, P. (2014). Regulated necrosis: the expanding network of non-apoptotic cell death pathways. Nat. Rev. Mol. Cell. Biol. 15, 135–147. doi:10.1038/nrm3737
Van Dyke, T. E., Hasturk, H., Kantarci, A., Freire, M. O., Nguyen, D., Dalli, J., et al. (2015). Proresolving nanomedicines activate bone regeneration in periodontitis. J. Dent. Res. 94, 148–156. doi:10.1177/0022034514557331
Vane, J. R. (1971). Inhibition of prostaglandin synthesis as a mechanism of action for aspirin-like drugs. Nat. New Biol. 231, 232–235. doi:10.1038/newbio231232a0
Vedin, I., Cederholm, T., Freund Levi, Y., Basun, H., Garlind, A., Faxén Irving, G., et al. (2008). Effects of docosahexaenoic acid-rich n-3 fatty acid supplementation on cytokine release from blood mononuclear leukocytes: the OmegAD study. Am. J. Clin. Nutr. 87, 1616–1622. doi:10.1093/ajcn/87.6.1616
Videla, L. A., Valenzuela, R., Del Campo, A., and Zúñiga-Hernández, J. (2023). Omega-3 lipid mediators: modulation of the M1/M2 macrophage phenotype and its protective role in chronic liver diseases. Int. J. Mol. Sci. 24, 15528. doi:10.3390/ijms242115528
Vyas, K., Chaudhuri, S., Leaman, D. W., Komar, A. A., Musiyenko, A., Barik, S., et al. (2009). Genome-wide polysome profiling reveals an inflammation-responsive posttranscriptional operon in gamma interferon-activated monocytes. Mol. Cell. Biol. 29, 458–470. doi:10.1128/MCB.00824-08
Walker, K. H., Krishnamoorthy, N., Brüggemann, T. R., Shay, A. E., Serhan, C. N., and Levy, B. D. (2021). Protectins PCTR1 and PD1 reduce viral load and lung inflammation during respiratory syncytial virus infection in mice. Front. Immunol. 12, 704427. doi:10.3389/fimmu.2021.704427
Walker, M. E., De Matteis, R., Perretti, M., and Dalli, J. (2024). Resolvin T4 enhances macrophage cholesterol efflux to reduce vascular disease. Nat. Commun. 15, 975. doi:10.1038/s41467-024-44868-1
Wallace, J. L., and Wang, R. (2015). Hydrogen sulfide-based therapeutics: exploiting a unique but ubiquitous gasotransmitter. Nat. Rev. Drug Discov. 14, 329–345. doi:10.1038/nrd4433
Wang, C., Rosas, F., Ramirez, M., Nioko, V., Verdu, A., Dosa, P., et al. (2004). Inhibition of pulmonary inflammatory diseases, asthma and pulmonary fibrosis, by lipoxin A4 and a novel series of selective small molecule FPRL1 agonists. J. Allergy Clin. Immunol. 113, S220. doi:10.1016/j.jaci.2004.01.245
Wang, L., Li, W., Xu, Y., Wei, Q., Zhao, H., and Jiang, X. (2011). Annexin 1-derived peptide Ac2-26 inhibits eosinophil recruitment in vivo via decreasing prostaglandin D2. Int. Arch. Allergy Immunol. 154, 137–148. doi:10.1159/000320228
Wang, X., Yang, M., Yu, G., Qi, J., Jia, Q., Liu, S., et al. (2024). Promoting the proliferation of osteoarthritis chondrocytes by resolvin D1 regulating the NLRP3/caspase-1 signaling pathway. Cell. Signal 113, 110960. doi:10.1016/j.cellsig.2023.110960
Wang, Y.-T., Trzeciak, A. J., Rojas, W. S., Saavedra, P., Chen, Y.-T., Chirayil, R., et al. (2023). Metabolic adaptation supports enhanced macrophage efferocytosis in limited-oxygen environments. Cell. Metab. 35, 316–331.e6. doi:10.1016/j.cmet.2022.12.005
Ward, C., Dransfield, I., Murray, J., Farrow, S. N., Haslett, C., and Rossi, A. G. (2002). Prostaglandin D2 and its metabolites induce caspase-dependent granulocyte apoptosis that is mediated via inhibition of IκBα degradation using a peroxisome proliferator-activated receptor-γ-independent mechanism. J. Immunol. 168, 6232–6243. doi:10.4049/jimmunol.168.12.6232
Weng, S.-Y., Wang, X., Vijayan, S., Tang, Y., Kim, Y. O., Padberg, K., et al. (2018). IL-4 receptor alpha signaling through macrophages differentially regulates liver fibrosis progression and reversal. EBioMedicine 29, 92–103. doi:10.1016/j.ebiom.2018.01.028
Wilborn, J., Crofford, L. J., Burdick, M. D., Kunkel, S. L., Strieter, R. M., and Peters-Golden, M. (1995). Cultured lung fibroblasts isolated from patients with idiopathic pulmonary fibrosis have a diminished capacity to synthesize prostaglandin E2 and to express cyclooxygenase-2. J. Clin. Investigation 95, 1861–1868. doi:10.1172/JCI117866
Wilson, C. L., Ouellette, A. J., Satchell, D. P., Ayabe, T., López-Boado, Y. S., Stratman, J. L., et al. (1999). Regulation of intestinal alpha-defensin activation by the metalloproteinase matrilysin in innate host defense. Science 286, 113–117. doi:10.1126/science.286.5437.113
Wu, J. M. F., Cheng, Y., Tang, T. W. H., Shih, C., Chen, J., and Hsieh, P. C. H. (2018). Prostaglandin E2 receptor 2 modulates macrophage activity for cardiac repair. J. Am. Heart Assoc. 7, e009216. doi:10.1161/JAHA.118.009216
Wu, S.-H., Chen, X.-Q., Liu, B., Wu, H.-J., and Dong, L. (2013). Efficacy and safety of 15(R/S)-methyl-lipoxin A4 in topical treatment of infantile eczema. Br. J. Dermatology 168, 172–178. doi:10.1111/j.1365-2133.2012.11177.x
Wu, X.-Y., Fan, W.-D., Fang, R., and Wu, G.-F. (2014). Regulation of microRNA-155 in endothelial inflammation by targeting nuclear factor (NF)-κB P65. J. Cell. Biochem. 115, 1928–1936. doi:10.1002/jcb.24864
Xia, T., Zhang, M., Lei, W., Yang, R., Fu, S., Fan, Z., et al. (2023). Advances in the role of STAT3 in macrophage polarization. Front. Immunol. 14, 1160719. doi:10.3389/fimmu.2023.1160719
Xiang, S., Ye, Y., Yang, Q., Xu, H., Shen, C., Ma, M., et al. (2021). RvD1 accelerates the resolution of inflammation by promoting apoptosis of the recruited macrophages via the ALX/FasL-FasR/caspase-3 signaling pathway. Cell. Death Discov. 7, 339–410. doi:10.1038/s41420-021-00708-5
Xiaoyu, L., Dandan, L., Tianzhao, O., Ziyou, Z., Zhenlin, L., Zhuang, L., et al. (2024). Resolvin D1 combined with exercise rehabilitation alleviates neurological injury in mice with intracranial hemorrhage via the BDNF/TrkB/PI3K/AKT pathway. Sci. Rep. 14, 31447. doi:10.1038/s41598-024-83019-w
Xie, W., Wang, H., Liu, Q., Li, Y., Wang, J., Yao, S., et al. (2016). ResolvinD1 reduces apoptosis and inflammation in primary human alveolar epithelial type 2 cells. Lab. Invest. 96, 526–536. doi:10.1038/labinvest.2016.31
Xie, Y., Tolmeijer, S., Oskam, J. M., Tonkens, T., Meijer, A. H., and Schaaf, M. J. M. (2019). Glucocorticoids inhibit macrophage differentiation towards a pro-inflammatory phenotype upon wounding without affecting their migration. Dis. Model. Mech. 12, dmm037887. doi:10.1242/dmm.037887
Xue, L., and Wu, Y.-Y. (2025). Activation of PPARγ regulates M1/M2 macrophage polarization and attenuates dextran sulfate sodium salt-induced inflammatory bowel disease via the STAT-1/STAT-6 pathway. Kaohsiung J. Med. Sci. n/a 41, e12927. doi:10.1002/kjm2.12927
Yanoshita, M., Hirose, N., Nishiyama, S., Tsuboi, E., Kubo, N., Kita, D., et al. (2025). Resolvin D1 suppresses inflammation in human fibroblast-like synoviocytes via the p-38, NF-κB, and AKT signaling pathways. Vitro Cell. Dev. Biol. Anim. 61, 331–339. doi:10.1007/s11626-024-01008-9
Yaseen, H., Butenko, S., Polishuk-Zotkin, I., Schif-Zuck, S., Pérez-Sáez, J. M., Rabinovich, G. A., et al. (2020). Galectin-1 facilitates macrophage reprogramming and resolution of inflammation through IFN-β. Front. Pharmacol. 11, 901. doi:10.3389/fphar.2020.00901
Yeung, Y. T., Aziz, F., Guerrero-Castilla, A., and Arguelles, S. (2018). Signaling pathways in inflammation and anti-inflammatory therapies. Curr. Pharm. Des. 24, 1449–1484. doi:10.2174/1381612824666180327165604
Yona, S., Kim, K.-W., Wolf, Y., Mildner, A., Varol, D., Breker, M., et al. (2013). Fate mapping reveals origins and dynamics of monocytes and tissue macrophages under homeostasis. Immunity 38, 79–91. doi:10.1016/j.immuni.2012.12.001
Yoneda, M., Ideguchi, H., Nakamura, S., Arias, Z., Ono, M., Omori, K., et al. (2024). Resolvin D2-induced reparative dentin and pulp stem cells after pulpotomy in a rat model. Heliyon 10, e34206. doi:10.1016/j.heliyon.2024.e34206
Yurdagul, A., Subramanian, M., Wang, X., Crown, S. B., Ilkayeva, O. R., Darville, L., et al. (2020). Macrophage metabolism of apoptotic cell-derived arginine promotes continual efferocytosis and resolution of injury. Cell. Metab. 31, 518–533.e10. doi:10.1016/j.cmet.2020.01.001
Zhang, F., Wang, H., Wang, X., Jiang, G., Liu, H., Zhang, G., et al. (2016). TGF-β induces M2-like macrophage polarization via SNAIL-mediated suppression of a pro-inflammatory phenotype. Oncotarget 7, 52294–52306. doi:10.18632/oncotarget.10561
Zhang, J., Wang, M., Ye, J., Liu, J., Xu, Y., Wang, Z., et al. (2020). The anti-inflammatory mediator resolvin E1 protects mice against lipopolysaccharide-induced heart injury. Front. Pharmacol. 11, 203. doi:10.3389/fphar.2020.00203
Zhang, M., Li, X., Zhang, Q., Yang, J., and Liu, G. (2023). Roles of macrophages on ulcerative colitis and colitis-associated colorectal cancer. Front. Immunol. 14, 1103617. doi:10.3389/fimmu.2023.1103617
Zhang, Q., and Cao, X. (2021). Epigenetic remodeling in innate immunity and inflammation. Annu. Rev. Immunol. 39, 279–311. doi:10.1146/annurev-immunol-093019-123619
Zhang, Q., Yuan, L., Liu, D., Wang, J., Wang, S., Zhang, Q., et al. (2014). Hydrogen sulfide attenuates hypoxia-induced neurotoxicity through inhibiting microglial activation. Pharmacol. Res. 84, 32–44. doi:10.1016/j.phrs.2014.04.009
Zhang, Q., Zhao, K., Shen, Q., Han, Y., Gu, Y., Li, X., et al. (2015a). Tet2 is required to resolve inflammation by recruiting Hdac2 to specifically repress IL-6. Nature 525, 389–393. doi:10.1038/nature15252
Zhang, S., Weinberg, S., DeBerge, M., Gainullina, A., Schipma, M., Kinchen, J. M., et al. (2019). Efferocytosis fuels requirements of fatty acid oxidation and the electron transport chain to polarize macrophages for tissue repair. Cell. Metab. 29, 443–456.e5. doi:10.1016/j.cmet.2018.12.004
Zhang, Y., Desai, A., Yang, S. Y., Bae, K. B., Antczak, M. I., Fink, S. P., et al. (2015b). Inhibition of the prostaglandin-degrading enzyme 15-PGDH potentiates tissue regeneration. Science 348, aaa2340. doi:10.1126/science.aaa2340
Zhang, Y., Leung, D. Y. M., Richers, B. N., Liu, Y., Remigio, L. K., Riches, D. W., et al. (2012). Vitamin D inhibits monocyte/macrophage proinflammatory cytokine production by targeting MAPK phosphatase-1. J. Immunol. 188, 2127–2135. doi:10.4049/jimmunol.1102412
Zhao, M., Li, C., Zhang, J., Yin, Z., Zheng, Z., Wan, J., et al. (2022). Maresin-1 and its receptors RORα/LGR6 as potential therapeutic target for respiratory diseases. Pharmacol. Res. 182, 106337. doi:10.1016/j.phrs.2022.106337
Zhao, M., Zheng, Z., Yin, Z., Zhang, J., Qin, J., Wan, J., et al. (2023). Resolvin D2 and its receptor GPR18 in cardiovascular and metabolic diseases: a promising biomarker and therapeutic target. Pharmacol. Res. 195, 106832. doi:10.1016/j.phrs.2023.106832
Zheng, Z., Zhao, M., Xu, Y., Zhang, J., Peng, S., Liu, J., et al. (2024). Resolvin D2/GPR 18 axis ameliorates pressure overload-induced heart failure by inhibiting pro-inflammatory macrophage polarization. J. Lipid Res. 65, 100679. doi:10.1016/j.jlr.2024.100679
Keywords: acute inflammation, resolution, specialized pro-resolving molecules, proinflammatory pathways, resolution pharmacology
Citation: Soliman AM, Soliman M, Shah SSH, Baig HA, Gouda NS, Alenezi BT, Alenezy A, Hegazy AMS, Jan M and Eltom EH (2025) Molecular dynamics of inflammation resolution: therapeutic implications. Front. Cell Dev. Biol. 13:1600149. doi: 10.3389/fcell.2025.1600149
Received: 25 March 2025; Accepted: 23 April 2025;
Published: 08 May 2025.
Edited by:
Kihong Lim, University of Rochester, United StatesReviewed by:
Hong Nian, Tianjin Medical University Eye Hospital, ChinaPietro Asproni, Institut de Recherche en Sémiochimie et Ethologie Appliquée (IRSEA), France
Copyright © 2025 Soliman, Soliman, Shah, Baig, Gouda, Alenezi, Alenezy, Hegazy, Jan and Eltom. This is an open-access article distributed under the terms of the Creative Commons Attribution License (CC BY). The use, distribution or reproduction in other forums is permitted, provided the original author(s) and the copyright owner(s) are credited and that the original publication in this journal is cited, in accordance with accepted academic practice. No use, distribution or reproduction is permitted which does not comply with these terms.
*Correspondence: Amro M. Soliman, YW1yby5zb2xpbWFuQGNvbmNvcmRpYS5hYi5jYQ==