- School of Tropical Agriculture and Forestry, Hainan University, Haikou, China
Salmonella is a foodborne pathogen that enters the host’s body through contaminated food and water, leading to gastroenteritis and systemic diseases. It is a significant veterinary and human pathogen capable of infecting both humans and animals, with substantial impacts on public health, human well-being, and the economic development of the livestock and poultry farming industry. Small non-coding RNAs (sRNAs), typically 50–500 nucleotides (nt) in length, have been identified in various bacteria, including Escherichia coli, Brucella, Pseudomonas aeruginosa, and Salmonella. These sRNAs play crucial roles in regulating diverse physiological processes within bacteria. This review emphasizes recent advances in understanding how sRNAs regulate the virulence of Salmonella spp, such as the discovery of novel sRNAs like SaaS and new regulatory mechanisms of known sRNAs like RyhB-1/RyhB-2 and SdsR/Spot 42. It also outlines critical future directions, including exploring the multifaceted functions of sRNAs in lifestyle or infection phase transitions, fully elucidating their roles in regulating the host immune response, studying the combined actions of multiple sRNAs on host pathogenesis and expanding research to more Salmonella serotypes and diverse animal models. Through these efforts, this review aims to enhance our understanding of Salmonella sRNAs and their infection mechanisms.
1 Introduction
Salmonella is a facultative anaerobic bacterium belonging to the Enterobacteriaceae family and is classified as a Gram-negative bacterium. It possesses a wide array of antigens and a highly complex structure, which can be broadly categorized into three types: somatic (O) antigens, flagellar (H) antigens, and surface (Vi) antigens. To date, Over 2,600 serotypes of Salmonella have been identified globally (Kabir et al., 2025; Vakili et al., 2025). Most Salmonella strains possess flagella, except Salmonella Pullorum and Salmonella Gallinarum. Salmonella is a common pathogen responsible for zoonotic infections and is a leading cause of foodborne illness worldwide (Traore et al., 2024). In the intestinal tract, Salmonella is excreted through feces and can contaminate water and food via insects or other biological vectors. When contaminated water or food is ingested by humans or animals, the pathogens are once again excreted through feces, perpetuating a continuous cycle of transmission. According to survey statistics, Salmonella-related food poisoning cases rank among the highest globally, and Salmonella is widely distributed (Chlebicz and Śliżewska, 2018). Salmonella is commonly found in meat, eggs, poultry and milk. Contamination can occur due to substandard environmental conditions in food preparation areas and improper cooking practices, leading to illness in consumers. The presence of Salmonella poses a significant threat to food safety and the sustainable development of animal husbandry.
sRNAs are a class of non-coding RNAs ranging from 50 nt to 500 nt in length and are widely found in bacterial RNAs (Tsai et al., 2015; Meng et al., 2022). Previous studies have found that sRNAs are predominantly found in Gram-negative bacteria such as Brucella, Escherichia coli, Pseudomonas aeruginosa, and Salmonella and are involved in the regulation of several bacterial physiological processes including bacteriophage metabolism, iron stabilization, carbon and amino acid utilization, metal ion utilization, expression of outer membrane proteins, periplasmic membrane formation, stress response, community sensing, and virulence gene regulation (Fröhlich et al., 2016; Kim, 2016; Meng et al., 2022). For the past few years, the role played by sRNAs in bacterial-host interactions has been of great interest, and it has been demonstrated that sRNAs play an indispensable role in the process of regulating the mechanisms of bacterial pathogenesis. Therefore, the present study highlights the functions played by sRNAs in Salmonella-host interactions in recent years to provide ideas for improving host resistance to Salmonella infection.
2 Mechanisms of action of Salmonella infected hosts
Salmonella enters the host with food and water, and since the host stomach contains gastric acid, the bacteria are stimulated by the low PH environment and need to react to counteract this stimulus (Foster and Hall, 1990). This regulation enables the bacteria to stabilize their internal pH, allowing them to survive in the acidic environment. After passing through the stomach and entering the small intestine, Salmonella adheres to the intestinal epithelium using adhesins and invades the epithelium via bacterial-mediated endocytosis or through M cells (Shaji et al., 2023). Upon entering host cells, Salmonella utilizes its Type III secretion system (T3SS) to release proteins that interact with the host cells, aiding bacterial survival and replication. These interactions induce host cytoskeletal rearrangements, disrupt the normal epithelial brush border structure, and form membrane ruffles that encapsulate the bacteria in a large vesicle known as Salmonella-containing vacuoles (SCVs) (Chatterjee et al., 2023). Upon invading intestinal epithelial cells, an inflammatory response is triggered in the host, leading to symptoms such as diarrhea and fever. After invading the intestinal epithelium, Salmonella is phagocytosed by immune cells such as macrophages, dendritic cells, and neutrophils. From there, the bacteria can translocate to other organs, including the liver, spleen, and bone marrow, via the small intestinal lymphoid tissues, mesenteric lymph nodes, or through hematogenous spread (Shaji et al., 2023; Zhang et al., 2024). During this process, antimicrobial effectors, such as antimicrobial peptides, are produced within macrophages. However, Salmonella is able to survive and replicate within these cells by utilizing a large number of virulence factors that resist and evade the antimicrobial effects of macrophages, ultimately achieving systemic infection (Shaji et al., 2023). The infection pathway of Salmonella is shown in Figure 1.
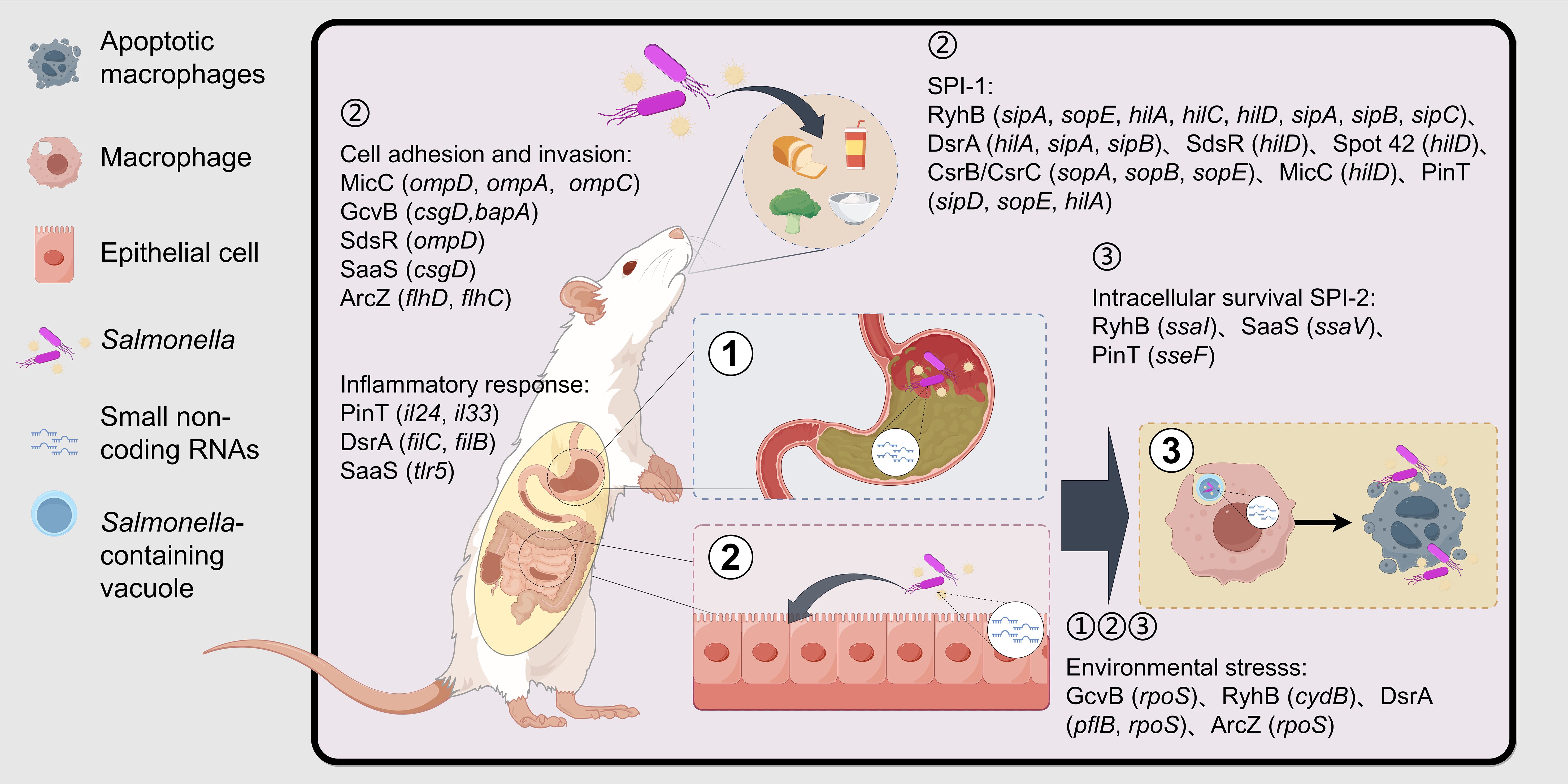
Figure 1. Associated processes involved in sRNAs after Salmonella invasion of the host. Salmonella is ingested by the host along with water and food and reaches the host’s stomach. Subsequently, it adheres to and invades intestinal epithelial cells with the assistance of biofilms, adhesins, and SPI-1 effector proteins. Finally, it survives within the SCV of macrophages with the help of SPI-2 effector factors. During the infection process, Salmonella needs to withstand a series of environmental stresses, including gastric acid, nutritional limitations in the intestine, and the antibacterial system of macrophages, and evade the host’s immune response to survive ultimately. sRNAs are involved in the regulation of environmental stress, epithelial cell adhesion and invasion, macrophage intracellular survival, and immunomodulation. The genes in the brackets are the key target genes of sRNA. (Created by Figdraw.).
Salmonella Pathogenicity Islands (SPIs) play a crucial role in the pathogenesis of Salmonella. Among these, Salmonella Pathogenicity Island 1 (SPI-1) was one of the first SPIs to be recognized and is primarily responsible for bacterial invasiveness and inflammation induction (Worley, 2023). SPI-1 encodes a T3SS that directs effector proteins into host cells, leading to alterations in host cell membrane structure and facilitating bacterial invasion (Chatterjee et al., 2023). Key effector proteins encoded by SPI-1, such as sipA, sipB, and sopC, promote bacterial entry into host cells via the T3SS (Azimi et al., 2020). Salmonella Pathogenicity Island 2 (SPI-2) is primarily responsible for bacterial replication and survival within phagocytes. Like SPI-1, SPI-2 also encodes a T3SS, but this system functions primarily after Salmonella has entered the host cell, helping the bacteria to survive and multiply within the host cell and avoid clearance by the host immune system (Schultz et al., 2021). Effector proteins encoded by SPI-2, such as sseF and sseG, help maintain the stability of SCVs and regulate nutrient uptake (Schultz et al., 2021). In addition to SPI-1 and SPI-2, Salmonella possesses multiple other virulence islands that encode proteins for a variety of functions, including metal ion transport, metabolic pathways adapted to the host environment, cell adhesion, and evasion of the host immune system. For example:SPI-3 is associated with the acquisition of magnesium ions; SPI-4 mediates adhesion to gastrointestinal epithelium; SPI-5 triggers an inflammatory response; SPI-6 possesses antimicrobial activity and promotes intracellular survival in macrophages, as well as systemic infections; SPI-7 attenuates the innate immune response and helps bacteria resist the killing effects of phagocytes. Together, these virulence islands enable Salmonella to adapt to the host environment, evade the host immune system, acquire necessary nutrients, and promote bacterial proliferation and survival within the host (Worley, 2023).
3 Mechanisms of sRNAs function in Salmonella
sRNAs are non-coding RNA molecules that are transcribed but typically do not encode proteins. They can form stable secondary structures after transcription. Based on the functions and modes of action, sRNAs can be categorized into three main groups: sRNAs with housekeeping functions, sRNAs that bind to target proteins and sRNAs that bind to target mRNAs through base pairing. Most of the currently known sRNAs belong to the third category. sRNAs can also be divided into cis- and trans-encoded sRNAs based on their base pairing patterns (Meng et al., 2022). Cis-encoded sRNAs are transcribed from the antisense strand of their regulatory target genes and bind to their corresponding mRNA targets in a partially complementary manner, leading to transcription termination or translation initiation (Aiba, 2007). Trans-encoded sRNAs, on the other hand, are typically produced by independent transcription from intergenic regions of the chromosome or by processing of the 5’ or 3’ untranslated regions (UTRs) of mRNAs. They interact with multiple mRNA targets through incomplete base complementation, inhibiting or facilitating translation initiation and accelerating or mitigating the degradation of target mRNAs (King et al., 2022). The pairing of many trans-encoded sRNAs with target mRNAs often requires the involvement of the RNA chaperone protein (Hfq) to enhance their interaction, and homologous Hfq has been found in a wide range of bacteria (Jørgensen et al., 2020). Hfq proteins are stable, cyclic hexameric structures composed of six identical subunits that can stabilize and protect RNAs as well as promote inter-pairing between RNAs (Watkins and Arya, 2023), As shown in Figure 2.
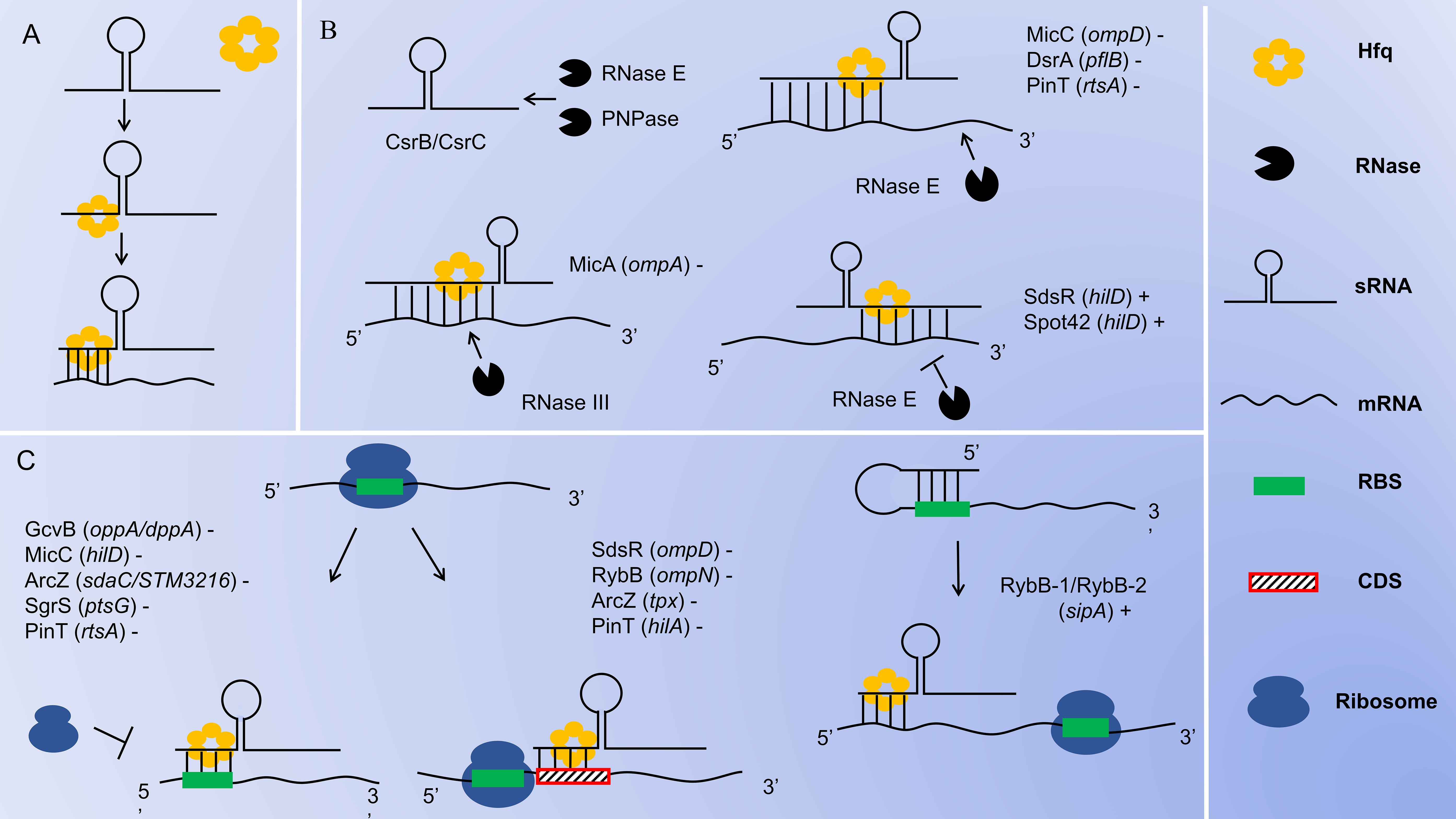
Figure 2. Mechanisms of sRNA Function in Salmonella. (A) Hfq stabilizes sRNA-mRNA complexes. (B) sRNA-mediated degradation or protection. RNase E and PNPase are capable of inducing the degradation of sRNAs CsrB/CsrC; The sRNAs bind to the 5’-UTR of the target mRNAs, recruiting RNase E to cleave and degrade the target mRNAs from the 3’ end; The sRNAs bind to the 5’-UTR of the target mRNAs, recruiting RNase III to cleave and degrade the target mRNAs at the double-stranded region; The sRNAs bind to the 3’-UTR of the target mRNAs, interfering with the cleavage of the target mRNAs by RNase E and promoting the translation of the mRNAs. (C) sRNA-mediated translational inhibition or activation. sRNAs block ribosome binding by directly attaching to the RBS in the 5’-UTR of target mRNAs, inhibiting translation initiation; sRNAs prevent ribosomal translation by binding to the CDS region of the target mRNAs; sRNAs bind to the 5’-UTR of the target mRNAs, unwind its secondary structure and expose the RBS, which promotes the binding of ribosomes to the RBS and facilitates the initiation of translation. The genes in the brackets are the target genes of sRNAs. The “+” or “-” indicates the up-regulation or down-regulation of the target genes by the sRNAs.
In Salmonella, most identified sRNAs block translation by directly binding to the ribosome binding site (RBS) in the 5’ UTR of target mRNAs, thereby down-regulating the expression of related genes. For example, GcvB blocks the translation of oppA and dppA mRNAs (Sharma et al., 2007); MicC blocks the translation of hilD mRNA (Cakar et al., 2022); ArcZ blocks the translation of sdaC and STM3216 mRNAs (Papenfort et al., 2009); SgrS blocks the translation of ptsG mRNA (Bobrovskyy and Vanderpool, 2014); and PinT blocks the translation of rtsA mRNA (Kim et al., 2019b). This process is illustrated in Figure 2. Alternatively, some sRNAs inhibit translation initiation by binding to the coding sequence (CDS) region of target mRNAs. For instance, RybB inhibits the translation initiation of ompN mRNA (Bouvier et al., 2008); SdsR inhibits the translation initiation of ompD mRNA (Fröhlich et al., 2012); ArcZ inhibits the translation initiation of tpx mRNA (Papenfort et al., 2009); and PinT inhibits the translation initiation of hilA mRNA (Kim et al., 2019b). This process is illustrated in Figure 2. In contrast, some sRNAs activate translation by binding to the 5’ UTR of target mRNAs. Typically, these target mRNAs contain intrinsic secondary structures within their 5’ UTR that inhibit ribosome binding. Therefore, when sRNAs bind to the inhibitory sequences within the 5’ UTR, the RBS becomes accessible for ribosome binding, promoting translation initiation, and thus up-regulating the expression of related genes (Lalaouna et al., 2013). For example, RyhB-1 and/or RyhB-2 bind to the 5’ UTR region of sipA, opening up the secondary structure to reveal the hidden RBS and enhancing sipA expression (Chen et al., 2021). This process is illustrated in Figure 2. Through this interaction with the target mRNAs, sRNAs achieve direct regulation of the target gene.
sRNAs can also mediate the decay of target mRNAs. Ribonucleases (RNases) are key regulators of RNA decay, and sRNAs pair with target mRNAs to trigger RNase-mediated degradation (Lalaouna et al., 2013). Most decay processes are carried out by RNase E. RNase E is a single-strand-specific endoribonuclease that can enter from the 3’ end of a double-stranded RNA structure, extend linearly along single-stranded regions, and cleave the target mRNAs at appropriate sites, thereby down-regulating the expression of related genes (Waters et al., 2017). For example, MicC, with the assistance of Hfq, recognizes ompD mRNA and induces RNase E-mediated cleavage downstream of ompD mRNA (Pfeiffer et al., 2009; Bandyra et al., 2024); The base pairing of DsrA with pflB mRNA recruiting RNase E to degrade pflB mRNA (Dong et al., 2022); The base pairing of PinT with rtsA mRNA recruiting RNase E to degrade rtsA mRNA (Kim et al., 2019b). This process is illustrated in Figure 2. Conversely, SdsR and Spot 42 target the 3’ UTR of hilD mRNA. These sRNAs stabilize hilD mRNA levels by interfering with RNase E-dependent mRNA degradation mechanisms, thus up-regulating the expression of hilD (Abdulla et al., 2023). This process is illustrated in Figure 2. Moreover, RNase E is essential for the processing of sRNAs derived from the 3’ UTR of mRNAs. Hfq assists in guiding RNase E to cleave stable 3’ fragments from various precursor RNAs, leading to the formation of functional mature sRNAs. One notable example of this process is the maturation of ArcZ, which is cleaved by RNase E (Chao et al., 2017). RNase III is an endoribonuclease with specificity for double-stranded RNA (Quendera et al., 2020). In Salmonella, MicA interacts with its target ompA mRNA to form a double-stranded RNA structure. RNase III then targets this double-stranded region and mediates its degradation, thereby down-regulating the expression of ompA (Viegas et al., 2011). As shown in Figure 2. sRNAs achieve indirect regulation of target genes by recruiting RNase E or RNase III to induce the decay of target mRNAs, or by interfering with RNase E to prevent the decay of target mRNAs. PNPase is an exoribonuclease that progressively degrades RNA. In Salmonella, both CsrB and CsrC are highly structured molecules. The decay process of CsrB and CsrC involves multiple endonucleolytic cleavages by RNase E, which are then followed by exonucleolytic degradation by PNPase. PNPase has been identified as a key factor in the decay of CsrB and CsrC sRNAs in Salmonella (Viegas et al., 2007). As shown in Figure 2.
4 Functions of Salmonella virulence-associated sRNAs
To further understand the functions of virulence-associated sRNAs in Salmonella, we have listed several sRNAs that have been extensively studied in recent years. These sRNAs can indirectly regulate bacterial virulence by participating in various physiological processes, such as the regulation of outer membrane proteins, iron metabolism, glucose metabolism, carbon source utilization, redox balance, and antibiotic resistance. Additionally, these sRNAs play crucial roles in different stages of Salmonella infection, including gastrointestinal stress, invasion of intestinal epithelial cells, survival within macrophages, and the regulation of SPI-1 and SPI-2 (as shown in Figure 1), to promote bacterial colonization and infection. These sRNAs interact with multiple targets, forming a complex regulatory network that is involved in Salmonella survival and pathogenesis. We have integrated the basic information of the sRNAs mentioned below into Table 1, including their names, sizes, encoding modes, target mRNAs, and functions.
4.1 GcvB
GcvB is a highly conserved sRNA found in various bacterial families, including Enterobacteriaceae, Actinobacteriaceae, Pasteurellaceae, Photobacteriaceae, and Vibrionaceae (Ju et al., 2021; Liu et al., 2022). Approximately 200 nt in length, GcvB is a trans-encoded sRNA that requires the chaperone protein Hfq for its auxiliary actions (Miyakoshi et al., 2022). GcvB expression is closely linked to glycine metabolism, and it can activate the transcription of GcvB when glycine levels are high (Urbanowski et al., 2000; Ernst and Downs, 2016). The target genes of GcvB encode products that are primarily amino acid transport proteins, particularly the ABC transporter proteins Dpp and Opp (Miyakoshi et al., 2015). ABC transporters are essential membrane transport systems that play a critical role in bacterial survival, virulence, and pathogenicity (Davidson et al., 2008). In addition to its role in amino acid transport, GcvB is involved in various physiological processes, including bacterial biofilm formation, acid tolerance responses, and oxidative stress responses. The rpoS gene encodes the σ subunit of RNA polymerase, which regulates the transcription of genes involved in general stress responses in Escherichia coli (Metaane et al., 2022). Studies have shown that GcvB positively regulates the rpoS gene post-transcriptionally, although the exact mechanism of this regulation remains to be elucidated (Bak et al., 2014). csgD is a transcriptional regulator of the csgBAC operon, which encodes curli fibrillar proteins. These proteins are major components of the extracellular matrix in Escherichia coli and Salmonella and are crucial for bacterial adhesion and biofilm formation (Long et al., 2024). It has been demonstrated that GcvB down-regulates the expression of the csgD mRNA, thereby controlling the production of curli fibrils (Andreassen et al., 2018). Research by Ma et al. (2024) has shown that the deletion of GcvB in Salmonella Typhimurium significantly enhances the bacterium’s biofilm formation ability and up-regulates the mRNA expression of the biofilm-related protein BapA. Additionally, the virulence of the GcvB deletion strain in mice was reduced compared to wild-type and complementary strains, leading to an increased survival rate of the mice. This indicates that GcvB promotes the virulence of Salmonella Typhimurium. The oxidative stress resistance of the GcvB deletion strain was also significantly enhanced. These findings suggest that GcvB plays a regulatory role in Salmonella Typhimurium biofilm formation, oxidative stress resistance, and virulence.
4.2 RyhB
RyhB was initially discovered in Escherichia coli and is approximately 90 nt in length (Massé and Gottesman, 2002). Subsequently, two homologous and functionally similar sRNAs were identified in Salmonella: RyhB-1 (also known as RyhB or RfrA) and RyhB-2 (also known as IsrE or RfrB), with lengths of 96 nt and 98 nt, respectively (Kim and Kwon, 2013). In addition to regulating iron metabolism, Salmonella RyhB is involved in resistance to oxidative stress, acid resistance, bacterial motility, and host cell invasion and intracellular survival, among other life processes.
In Escherichia coli, iron acquisition and storage are controlled by the global iron uptake regulator Fur protein and the sRNA RyhB. The Fur protein can recognize the RyhB sequence in iron-rich environments and negatively regulate the expression of the RyhB (Porcheron and Dozois, 2015). When bacteria are in an iron-rich environment, Fur binds to Fe2+ and forms the Fur-Fe2+ complex, which binds to the Fur box of RyhB, inhibiting its transcription. This leads to the derepression of iron storage-related proteins and the decreased expression of iron uptake genes activated by RyhB, thus increasing intracellular free iron storage and reducing extracellular iron uptake functions. Conversely, when cells are iron-deficient, Fur dissociates from Fe2+, lifting the inhibition on RyhB. Under low iron conditions, RyhB is abundantly expressed and reduces the stability and translational efficiency of mRNAs encoding nonessential iron-sulfur proteins and iron storage proteins (Kim, 2016). This regulation of iron metabolism by RyhB ensures that iron is taken up and used in essential pathways, playing a crucial role in maintaining intracellular iron homeostasis.
Moreover, RyhB can regulate gene expression by incomplete base pairing with target mRNAs, promoting Salmonella invasiveness (Malabirade et al., 2018). Specifically, Salmonella enterica RyhB-1 and RyhB-2 enhance the invasion of Caco-2 cells by up-regulating the expression of T3SS effector proteins like SipA and SopE under simulated intestinal conditions, thereby promoting the bacterial virulence (Chen et al., 2021). When Salmonella invades macrophages, it faces harsh conditions, including acidification, oxidative stress, and nutrient deficiencies. Peñaloza et al. (2021) found that in a model of Salmonella Typhimurium infection of mouse macrophage RAW264.7, deletion of RyhB-1 and/or RyhB-2 resulted in increased intracellular survival and accelerated intracellular replication within the macrophage. These mutant strains exhibited higher intracellular ATP levels and lower NAD/NADH ratios compared to the wild-type. RyhB-1 and RyhB-2 also inhibit Salmonella virulence within RAW264.7 by down-regulating transcriptional regulators such as hilA, hilC, and hilD, as well as SPI-1-related genes encoding effector proteins like sipA, sipB, and sipC. Meng et al. (2023) found that deletion of RyhB-1 and/or RyhB-2 similarly increased the survival of Salmonella enteritidis in HD11 cells through a model of Salmonella enteritidis infection in chicken macrophage HD11 cells. Additionally, RyhB-1 negatively regulates the SPI-2 gene, and both RyhB-1 and RyhB-2 directly interact with the ssaI CDS region through an incomplete complementary base-pairing mechanism, down-regulating ssaI expression to inhibit its mRNA translation, thereby inhibiting the virulence of Salmonella enteritidis within HD11 cells. Phagocytes have two important antimicrobial systems: the Phagocyte Oxidase (PHOX) pathway and the inducible Nitric Oxide Synthase (iNOS) pathway. These pathways produce Reactive Oxygen Species (ROS) and Reactive Nitrogen Species (RNS), respectively. ROS plays a crucial role in the early response to bacterial infection, while RNS restricts bacterial survival within host cells (Meng et al., 2022). Salmonella Typhimurium RyhB-1 and RyhB-2 are highly induced by nitrosoglutathione (S-nitrosoglutathione, GSNO), a nitric oxide donor compound used to induce nitrosative stress in macrophages, which is an extremely potent antibacterial agent (Calderón et al., 2014a). Significant up-regulation of RyhB-1 and RyhB-2 expression in Salmonella Typhimurium after H2O2 treatment leads to increased intracellular ROS levels, helping the bacteria resist oxidative stress (Calderón et al., 2014b).
4.3 DsrA
DsrA is a trans-encoded sRNA that is 87 nt in length (Lease et al., 1998). It tends to accumulate in large quantities at low temperatures (Repoila and Gottesman, 2001). DsrA regulates the expression of the hns and rpoS genes. rpoS encodes a sigma factor that responds to general stress and is highly conserved in Escherichia coli, Salmonella, and other related enteric bacteria. When bacteria encounter harsh conditions, they need to integrate multiple stress signals and initiate appropriate cellular responses to survive. rpoS is a major regulator of the general stress response (Jones et al., 2006). DsrA activates the translation of rpoS by base pairing with the 5’ UTR of rpoS mRNA. On the other hand, hns encodes the H-NS protein, a major repressor and silencer of numerous genes. The expression of DsrA triggers an increase in the turnover of hns mRNA, leading to a decrease in the amount of H-NS protein in the cells (Lalaouna et al., 2015).
The known functions of DsrA are primarily focused on its role in the acid tolerance response (ATR). Salmonella are exposed to various stress conditions during pathogenesis, with acid stress being one of the main host defense mechanisms. This acid stress environment is typically encountered in the host’s stomach and in phagocytic and non-phagocytic cells containing SCVs (Allam et al., 2012). Ryan et al. (2016) found that DsrA was induced threefold under conditions of pH 3.1 compared to normal conditions. Homozygous mutants lacking DsrA (ΔDsrA) exhibited lower viability under ATR, along with a 1.8-fold up-regulation of the hns gene in ΔDsrA compared to the wild strain. Additionally, the expression of invasion-associated SPI-1 effectors such as hilA, sipA, and sipB was down-regulated in ΔDsrA, resulting in reduced motility, weak adhesion, and impaired invasion efficacy, indicating that DsrA can promote the virulence of the bacteria. Furthermore, ΔDsrA was unable to induce intestinal inflammation in C57BL/6 mice 72 hours post-infection, which may be related to a significant reduction in the expression of flagellin structural genes fliC and fliB in ΔDsrA, considered key pro-inflammatory determinants.
Dong et al. (2022) found that genes involved in glycolysis, pyruvate metabolism, the tricarboxylic acid (TCA) cycle, and NADH-dependent respiration showed significantly different expression patterns between the wild strain of Salmonella Typhimurium and the DsrA deletion mutant (ΔDsrA), both before and after H2O2 treatment, as revealed by RNA-Seq. This suggests that DsrA plays a crucial role in regulating central carbon metabolism (CCM) and NAD(H) homeostasis in Salmonella Typhimurium. Target gene prediction and experimental validation revealed that DsrA down-regulates the expression of pflB, which encodes pyruvate-formate lyase. Additionally, the NAD/NADH ratio in the pflB-DsrA co-overexpression strain (WT-ppflB-pDsrA) was significantly lower than that in the pflB overexpression strain (WT-ppflB), suggesting that the inhibition of pflB by DsrA significantly affects the redox homeostasis of Salmonella Typhimurium. Changes in redox status are often closely related to alterations in bacterial metabolic pathways, activation of stress responses or expression of virulence factors.
4.4 SdsR(RyeB)
SdsR is a member of the stationary phase sigma factor (σS) regulator and is one of the most conserved sRNAs in Enterobacteriaceae (Ryan et al., 2017). It is present in Escherichia coli, Salmonella, and other enterobacteria. SdsR binds to target mRNAs through partial base complementary pairing (Melamed et al., 2016), with the binding region often consisting of six to eight adjacent bases (Bouvier et al., 2008). Hfq protects SdsR from degradation by cytosolic ribonucleases and promotes trans base pairing with multiple targets, such as mutS and tolC in Escherichia coli and stpA, crp, tolC, and ompD in Salmonella (Gupta et al., 2019).
Previous studies have found that SdsR can affect the translation of membrane proteins (Fröhlich et al., 2016), antibiotic susceptibility (Gutierrez et al., 2013), survival time during the stationary phase (Monteiro et al., 2012), and biofilm formation (Doetsch et al., 2011). SdsR also down-regulates the expression of tolC, a gene encoding an outer membrane protein component of drug-resistant efflux pumps, thereby reducing bacterial resistance to new antibiotics and crystal violet (Parker and Gottesman, 2016). In recent years, a study by Abdulla et al. (2023) found that the 3’ UTR of hilD renders hilD mRNA susceptible to RNase E-dependent degradation. However, SdsR alters the accessibility of hilD mRNA to RNase E cleavage by targeting the binding site within the first 90 nt of the 3’ UTR, thereby up-regulating the expression of hilD and SPI-1 genes. The transcriptional regulator hilD is a major regulatory element for SPI-1 virulence gene expression, and the regulatory effect of SdsR on hilD is essential for Salmonella invasion. SdsR accumulates in large quantities during the stationary phase, osmotic shock, and heat shock, and its expression is negatively correlated with that of the sRNA SraC (RyeA). The sRNA homologs of SdsR and SraC are located in the intergenic region (IGR) between the pphA and yebY genes, with opposite polarity (Gupta et al., 2019). Unlike SdsR, SraC does not depend on the regulation of Hfq and σ factors and is less conserved (Gupta et al., 2020). Consequently, SraC has been rarely studied in Salmonella. SraC is 270 nt long, and its internal fragment is fully complementary to the 104 nt long SdsR.
4.5 Spot 42
Spot 42 is a 109 nt sRNA specifically found in γ-proteobacteria, including Escherichia coli and Salmonella (Bækkedal and Haugen, 2015). The cyclic adenosine monophosphate receptor protein (CRP) is a transcription factor that becomes active upon binding to the intracellular second messenger cyclic adenosine monophosphate (cAMP). The interaction of CRP-cAMP with DNA results in the activation or inhibition of its target genes (El Mouali et al., 2021). Spot 42 is highly expressed when bacteria are grown under glucose conditions, while its transcription is inhibited by cAMP-CRP when the bacteria are grown on non-preferred carbon sources (Bækkedal and Haugen, 2015). Spot 42 can be divided into two functional domains. The 5’ part comprises three distinct single-stranded regions involved in base pairing with mRNA targets, while the 3’ region contains the Hfq binding module, consisting of two stem-loop structures (Stenum and Holmqvist, 2022). Spot 42 RNA has been shown to down-regulate several targets, including galK, srlA, fucI, and nanC, which encode proteins involved in secondary metabolism and the uptake and utilization of non-preferred carbon sources (Beisel and Storz, 2011). Aoyama et al. (2022) found that Spot 42 encodes a 15-amino acid protein, SpfP, which plays a role in metabolic inhibition. This indicates that Spot 42 is a dual-function sRNA, affecting both carbon source utilization through its base-pairing activity and via the small protein it encodes. Additionally, Abdulla et al. (2023) demonstrated that two sRNAs, SdsR and Spot 42, regulate SPI-1 by targeting different regions of the hilD mRNA 3’ UTR. Salmonella mutants lacking both SdsR and Spot 42 showed reduced virulence in mouse infection models, indicating that SdsR and Spot 42 promote bacterial virulence. Unlike the binding site of SdsR on hilD, Spot 42 targets a region of hilD closer to the terminator.
4.6 SaaS
SaaS is a newly identified regulatory factor screened from meat-source Salmonella enteritidis in recent years. Hu et al. (2020) investigated the transcription and sRNA expression of Salmonella enteritidis exposed to meat thawing loss broth (MTLB) during biofilm formation. Through RNA-Seq, they identified 19 candidate sRNAs involved in biofilm regulation. Preliminary findings suggest that SaaS is associated with the biofilm formation of Salmonella enteritidis, which is closely linked to bacterial colonization. This implies a potential connection between SaaS and the virulence of Salmonella enteritidis. Cai et al. (2023a) studied the role of SaaS in the virulence phenotype of Salmonella enteritidis by constructing SaaS mutant and complementary strains. They found that SaaS is activated in a simulated intestinal environment (SIE) and can directly target the mRNA of the virulence protein SsaV, up-regulating the expression of ssaV. Experiments using human colorectal adenocarcinoma cells (Caco-2) and mouse macrophages (RAW264.7) showed that SaaS promotes the invasion and destruction of epithelial cells by Salmonella enteritidis and inhibits the overgrowth and destruction of macrophages. Additionally, BALB/c mouse models demonstrated that the removal of SaaS significantly reduced mortality, mitigated pathophysiological deterioration, and decreased the spread of bacteria to the systemic circulation and systemic inflammation. SaaS also promoted bacterial colonization in the cecum and colon of the BALB/c mouse model. Furthermore, Cai et al. (2023b) found that SaaS enhances damage to the mucosal barrier by affecting the expression of antibacterial products, reducing the number of goblet cells, inhibiting the expression of mucin genes, and ultimately decreasing the thickness of the mucosal layer. High-throughput 16S rRNA gene sequencing revealed that SaaS alters gut balance by depleting beneficial gut microbiota and increasing harmful ones. ELISA and Western blot analyses confirmed that SaaS regulates intestinal inflammation by continuously activating the P38-JNK-ERK MAPK signaling pathway, achieving immune escape in the initial infection stage and enhancing pathogenesis in later stages. These studies all indicate that SaaS promotes the virulence of Salmonella enteritidis.
4.7 ArcZ
ArcZ is a conserved trans-acting sRNA in Enterobacteria that relies on Hfq and is cleaved by RNase E into a processed form of 55 to 60 nt. This processed form is highly conserved for controlling the expression of target mRNAs (Chao et al., 2017). High oxygen levels can induce the expression of ArcZ, which peaks during the stationary growth phase. This regulation is mediated by the oxygen-responsive two-component system ArcA/ArcB, which restricts the transcription of ArcZ under low oxygen conditions (Brown et al., 2022). ArcZ can control multiple targets and influences stress response, motility, and virulence by regulating major regulators such as flhDC and rpoS. Recent RL-SEQ data suggest that ArcZ may interact directly with more than 10% of mRNAs in the Escherichia coli and Salmonella genomes, making ArcZ one of the largest target regulators among sRNAs (Melamed et al., 2016; Liu et al., 2023). In Escherichia coli, the general stress response mediated by RpoS has been extensively studied, and the first clear target of ArcZ in Escherichia coli is rpoS mRNA (Mandin and Gottesman, 2010; Battesti et al., 2011). ArcZ can directly act on rpoS mRNA, up-regulating the expression of the rpoS gene. Another sRNA, CyaR, can interact with rpoS mRNA and down-regulate rpoS mRNA expression (Kim and Lee, 2020). ArcZ can directly interact with CyaR and inhibit its function by degrading CyaR through RNase E (Kim and Lee, 2020), while CyaR does not affect the activity of ArcZ (Iosub et al., 2020). ArcZ can control the biofilm formation of Salmonella Typhimurium by regulating the transcriptional factor CsgD (Mika and Hengge, 2014). CsgD regulates multiple genes responsible for the assembly, transport, and synthesis of curli, which are crucial for biofilm formation. Recently, Kim et al. (2019a) found that in Salmonella Typhimurium, two sRNAs, FnrS and ArcZ, directly affect the activation of the SPI1 T3SS by regulating hilD translation. ArcZ and the two-component system FnrS inhibit the translation of hilD mRNA through direct interaction with the mRNA of the virulence gene hilD, thereby down-regulating the expression of hilD and hilA. Under aerobic conditions, ArcZ is expressed and inhibits hilD translation. Under hypoxic conditions, the two-component Fnr system activates the transcription of FnrS, which in turn inhibits hilD translation. This regulatory network allows the T3SS genes to be expressed most efficiently when exposed to fluctuating oxygen levels.
4.8 CsrB/CsrC
The sequence-specific RNA-binding protein CsrA is a global regulator that controls the expression of SPI-1 and SPI-2 by directly inhibiting hilD translation initiation and down-regulating the expression of hilD. Additionally, CsrA regulates the expression of genes encoding metabolic regulators and biofilm formation in Salmonella. It plays a crucial role in modulating the pathogenicity, metabolism, biofilm formation, and motility of bacteria in response to changes in nutritional conditions. The activity of CsrA is regulated by two sRNAs, CsrB and CsrC, which sequester and antagonize the CsrA protein (Potts et al., 2019). The BarA/SirA two-component system induces the expression of CsrB and CsrC. Together, the BarA/SirA and Csr global regulatory systems control a wide range of cellular processes. Nava-Galeana et al. (2023) found through proteomic analysis that in Salmonella Typhimurium, the BarA/SirA and CsrB/CsrC systems up-regulate the expression of SPI-1-related genes such as sopA, sopB and sopE in a cascade manner, and down-regulate the genes expressed in the intestinal lumen of Salmonella, such as pdu and cbi-cob. Additionally, a study by El Mouali et al. (2021) found that in Salmonella enteritidis, the central metabolic regulator CRP-cAMP can regulate CsrB and CsrC to varying degrees in a growth-stage-dependent manner.
4.9 MicC
MicC is capable of binding to the mRNA of the outer membrane protein OmpD and indirectly down-regulate its expression in Salmonella Typhimurium by accelerating the decay of ompD mRNA through RNase E-dependent mechanisms (Pfeiffer et al., 2009). Wang et al. (2020) exposed five strains of Salmonella to acid stress and found that acid exposure induced high levels of MicC expression. Meng et al. (2021) constructed a mutant and a replacement strain of Salmonella enteritidis MicC using a λRed recombination system. qRT-PCR results showed that MicC down-regulated the expression of ompA and ompC. Additionally, challenge experiments using 6-week-old BALB/c mice and 1-day-old chickens demonstrated that MicC may inhibit the virulence of Salmonella enteritidis to mice and chickens by down-regulating the expression of outer membrane protein genes. Cakar et al. (2022) found that MicC prevents the translation of hilD mRNA by base pairing near the ribosome binding site, and down-regulates the expression of hilD. MicC is activated by the transcription factor SlyA, and SlyA enters the SPI-1 regulatory network exclusively through MicC. The transcription of MicC is negatively regulated by the OmpR/EnvZ two-component system, but this regulation is dependent on SlyA. Deletion mutants of MicC were more competitive in the small intestines of BALB/c mice compared to wild-type strains, indicating that MicC inhibits bacterial virulence during infection.
4.10 PinT
PinT is an 80 nt long sRNA derived from a horizontally acquired Salmonella-specific site that also encodes the co-activating protein RtsA of the invasive gene (Westermann et al., 2016). Studies by Westermann et al. (2016) identified PhoP-activated PinT, which increases 100-fold during infection after entry into host cells. PinT can affect the expression of invasion-related effectors and virulence genes required for intracellular survival. Specifically, the expression of SPI-1 invasion-related genes (such as sipD and sopE) decreases, while the expression of SPI-2 genes that promote intracellular survival (such as sseF) increases. Additionally, NF-κB-related immune genes are strongly activated in the invading host cells. The expression of the SPI-1 and SPI-2 T3SS is no longer required after Salmonella invades intestinal epithelial cells or during intracellular replication in macrophages. Kim et al. (2019b) found that PhoPQ-regulated sRNA PinT promotes the effective down-regulation of the SPI-1 T3SS by preventing the translation of hilA and rtsA. PinT base-pairs with the mRNAs of hilA and rtsA, inhibits the initiation of hilA translation, and directly down-regulates the expression of hilA. Meanwhile, it also induces the degradation of rtsA transcripts and indirectly down-regulates the expression of rtsA. PinT also indirectly down-regulates the expression of fliZ, a post-translational regulator of HilD, and directly inhibits the translation of SsrB, a major regulator of SPI-2 T3SS, thus down-regulating the expression of ssrB. In vivo mouse competition tests have shown that PinT controls a series of virulence genes at the post-transcriptional level, and PinT is able to inhibit the virulence of bacteria to mice. Additionally, PinT is capable of inhibiting the mRNA of the effector kinase SteC in Salmonella Typhimurium, thereby down-regulating the expression of steC and ultimately delaying actin rearrangement in infected host cells (Correia Santos et al., 2021).
4.11 SgrS
SgrS is a 227 nt Hfq-bound sRNA that is widely present in γ-proteobacteria. Its 5’ terminal also encodes a small 43-amino acid protein called SgrT, which inhibits the activity of major glucose transporters. SgrS has two distinct functions: it base-pairs with ptsG mRNA, which encodes the glucose transporter EIICBGlc, to inhibit its translation, and it also translates the small protein SgrT from its own open reading frame (ORF). These functions operate through independent regulatory mechanisms, both of which can reduce the accumulation of phosphorylated sugars, thereby relieving stress and promoting growth (Bobrovskyy and Vanderpool, 2014). SgrS can pair with the mRNA of the virulence gene sopD and inhibit its translation, thus down-regulating the expression of sopD (Papenfort et al., 2012). c-di-GMP is a central signaling molecule that determines the transition between motile and sessile lifestyles in many bacteria. At high concentrations, c-di-GMP stimulates the formation of biofilms, while at low concentrations, it leads to the dispersion and planktonic state of biofilms. In recent years, Baek and Yoon (2023) have studied the potential of c-di-GMP to alter the carbon metabolic pathway of Salmonella Typhimurium. They found that the loss of SgrS alleviates the down-regulation of the carbohydrate phosphotransferase system (PTS) genes by c-di-GMP. These results indicate that SgrS mediates the negative effects of c-di-GMP on PTS regulation.
5 Conclusion and prospect
Base pairing between sRNA and target mRNA can lead to mRNA translation inhibition or activation, as well as promote mRNA stabilization or degradation. This interaction forms a complex regulatory network in Salmonella, playing a crucial role in bacterial adaptation, virulence, and pathogenesis. Salmonella is an enteric pathogen and intracellular parasite. When the bacteria enter the host through contaminated water and food, they encounter various pressures and stresses in the host’s gastrointestinal tract and macrophages. sRNAs such as GcvB, RyhB, DsrA, and ArcZ are involved in regulating the stress response, enabling the bacteria to adapt to environmental changes. Upon entering the intestine, the bacteria must adhere to and invade intestinal epithelial cells. Bacterial biofilm formation promotes adhesion to these cells, and sRNAs like GcvB, SdsR, SaaS, ArcZ, and CsrB/CsrC participate in regulating biofilm formation, thus enhancing adhesion to epithelial cells. Additionally, SPI-1 is a key virulence island involved in the adhesion and invasion of intestinal epithelial cells. sRNAs such as RyhB, DsrA, SdsR, Spot 42, SaaS, CsrB/CsrC, MicC, SgrS and PinT regulate the translation of SPI-1-related virulence gene mRNAs, thereby modulating their virulence. After Salmonella enters macrophages, it needs to survive and replicate within the SCV. SPI-2 plays a crucial role in the intracellular survival stage of the bacteria, and sRNAs such as RyhB, SaaS and PinT regulate the translation of SPI-2-related gene mRNAs, promoting bacterial survival within the cells (as shown in Figure 1). Moreover, sRNAs are involved in the regulation of outer membrane proteins, iron metabolism, glucose metabolism, carbon source utilization, redox balance, antibiotic resistance, and numerous other physiological processes.
In the past five years, numerous new advancements have been achieved in the research of sRNAs. In previous studies, although a large number of sRNAs were identified, only a few of them have been proven to be capable of regulating target genes and had their signal regulatory pathways elucidated. Recently, researchers have identified a novel sRNA named SaaS from meat-derived Salmonella enteritidis. SaaS can enhance bacterial virulence and continuously activate the P38-JNK-ERK MAPK signaling pathway to regulate intestinal inflammation, which represents a significant new discovery regarding the regulation of the interaction between bacteria and the host by sRNAs (Cai et al., 2023b). In addition, RyhB was initially discovered in Escherichia coli, and subsequently, its homologous counterparts RyhB-1 and RyhB-2 were identified in Salmonella (Kim and Kwon, 2013). The more well-known function of RyhB-1 and RyhB-2 is related to the uptake and utilization of iron (Porcheron and Dozois, 2015; Kim, 2016). In recent years, multiple researchers have found that RyhB-1 and RyhB-2 can promote the invasion of intestinal epithelial cells in the early stage of infection (within the intestine), while in the late stage of infection (inside macrophages), they can inhibit the virulence of bacteria in the macrophages of mice and chickens (Chen et al., 2021; Peñaloza et al., 2021; Meng et al., 2023). This stage-dependent regulation reflects the precise regulatory ability of sRNAs in complex infectious microenvironments. In previous studies on the mechanism of sRNA-mediated decay of target mRNAs, sRNAs typically targeted the 5’-UTR of mRNAs and recruited RNases to cleave the target mRNAs. However, recent research has revealed that SdsR and Spot42 can target the 3’-UTR of hilD, interfering with the degradation function of RNase E and thus up-regulating the expression of hilD (Abdulla et al., 2023). This new discovery has revealed a novel regulatory pathway of bacterial virulence and expanded our understanding of the regulatory mechanism of sRNAs on target mRNAs. However, despite the new breakthroughs achieved in the research progress of sRNAs in recent years, there are still some issues that need to be improved and resolved:
1. In Salmonella, most studies have focused on the single functions of sRNAs. For example, as previously mentioned, SgrS acts as an intermediary in the c-di-GMP-mediated down-regulation of PTS genes and may indirectly influence bacterial biofilm formation by regulating PTS systems (Baek and Yoon, 2023). In addition, some sRNAs listed in Table 1 primarily regulate biofilm formation indirectly by modulating outer membrane proteins or rpoS mRNAs. Although these sRNAs are known to play important roles in biofilm formation, whether they also play crucial roles in lifestyle or infection phase transitions remains unclear.
2. Additionally, the function of sRNAs in the process of bacterial infection, especially in regulating the host immune response, remains to be fully elucidated. Many sRNAs have been found to be capable of influencing bacterial virulence. Apart from regulating the expression of virulence factors, it remains unclear whether they also participate in the relevant signaling pathways of immune regulation. Moreover, the specific molecular recognition and signal transduction mechanisms between sRNAs and immune cells are still not well-defined.
3. There are various sRNAs in bacteria, and there may be synergistic or antagonistic effects among them, jointly regulating the physiological functions of bacteria and their interactions with the host. However, most current studies only focus on the functions of individual sRNAs, and there is relatively little research on the impacts of the combined actions of multiple sRNAs on host pathogenesis. For instance, different sRNAs may regulate different virulence genes and cooperate with each other to influence the infection process of bacteria. Nevertheless, the mechanisms of such combined actions have not been fully revealed.
4. Currently, there are certain limitations in the research field of Salmonella sRNAs. From the perspective of serotypes, research mainly focuses on Salmonella Typhimurium and Salmonella enteritidis. In contrast, for numerous other serotypes of Salmonella, the research on sRNAs is rather scarce, making it difficult for us to comprehensively understand the functions and regulatory mechanisms of sRNAs within the entire genus of Salmonella. In terms of the selection of animal models, the types of hosts are relatively limited, mainly being mice and chickens. This limitation has led to a lack of understanding of the mechanisms of action of Salmonella sRNAs within other important human or animal hosts, as well as their impacts on the pathological processes of the hosts. This not only restricts our grasp of the universal laws of Salmonella infections but also poses challenges to the transformation and application of relevant research findings in different host backgrounds.
Therefore, the role of sRNAs warrants further attention. With the advancement of bioinformatics and the continuous progress and improvement of second-generation sequencing technology, more sRNAs and their specific functions will be discovered in the future, leading to a deeper understanding of bacterial survival and pathogen-host interactions. This review summarizes the regulatory roles of several sRNAs in Salmonella-host cell interactions that have been well studied in recent years, providing valuable insights and new research directions in the field. It is expected to have a significant impact on the research, diagnosis, control, and treatment of the disease. For example, in terms of disease diagnosis, sRNAs can serve as stage-specific markers of infection (such as the high expression of SaaS in the intestinal environment). Early diagnosis can be achieved by detecting the levels of sRNAs in clinical samples. In terms of disease control, targeting biofilm-related sRNAs (such as GcvB) can inhibit bacterial adhesion and colonization, reducing the risk of foodborne contamination. In terms of disease treatment, taking sRNAs that regulate virulence (such as SdsR) as targets, developing specific inhibitors to promote their degradation or suppress their functions can weaken the pathogenicity of Salmonella, making it easier for the body to eliminate the bacteria.
Author contributions
TH: Conceptualization, Data curation, Investigation, Methodology, Software, Writing – original draft. YD: Conceptualization, Data curation, Visualization, Writing – review & editing. YS: Conceptualization, Validation, Writing – review & editing. TL: Funding acquisition, Project administration, Resources, Supervision, Writing – review & editing.
Funding
The author(s) declare that financial support was received for the research and/or publication of this article. This work was supported by the Hainan Provincial Natural Science Foundation of China (Grant No. 823RC468); the Hainan Provincial Graduate Student Innovative Research Project (Grant No. Qhys2023-229) and the National Natural Science Foundation of China (Grant No. 32460914).
Acknowledgments
Thanks to all who worked on this study.
Conflict of interest
The authors declare that the research was conducted in the absence of any commercial or financial relationships that could be construed as a potential conflict of interest.
Generative AI statement
The author(s) declare that no Generative AI was used in the creation of this manuscript.
Publisher’s note
All claims expressed in this article are solely those of the authors and do not necessarily represent those of their affiliated organizations, or those of the publisher, the editors and the reviewers. Any product that may be evaluated in this article, or claim that may be made by its manufacturer, is not guaranteed or endorsed by the publisher.
References
Abdulla, S. Z., Kim, K., Azam, M. S., Golubeva, Y. A., Cakar, F., Slauch, J. M., et al. (2023). Small RNAs Activate Salmonella Pathogenicity Island 1 by Modulating mRNA Stability through the hilD mRNA 3′ Untranslated Region. J. Bacteriol. 205, e00333–e00322. doi: 10.1128/jb.00333-22
Aiba, H. (2007). Mechanism of RNA silencing by Hfq-binding small RNAs. Curr. Opin. Microbiol. 10, 134–139. doi: 10.1016/j.mib.2007.03.010
Allam, U. S., Krishna, M. G., Sen, M., Thomas, R., Lahiri, A., Gnanadhas, D. P., et al. (2012). Acidic pH induced STM1485 gene is essential for intracellular replication of Salmonella. Virulence 3, 122–135. doi: 10.4161/viru.19029
Andreassen, P. R., Pettersen, J. S., Szczerba, M., Valentin-Hansen, P., Møller-Jensen, J., Jørgensen, M. G. (2018). sRNA-dependent control of curli biosynthesis in Escherichia coli: McaS directs endonucleolytic cleavage of csgD mRNA. Nucleic Acids Res. 46, 6746–6760. doi: 10.1093/nar/gky479
Aoyama, J. J., Raina, M., Zhong, A., Storz, G. (2022). Dual-function Spot 42 RNA encodes a 15–amino acid protein that regulates the CRP transcription factor. Proc. Natl. Acad. Sci. U.S.A. 119, e2119866119. doi: 10.1073/pnas.2119866119
Azimi, T., Zamirnasta, M., Sani, M. A., Soltan Dallal, M. M., Nasser, A. (2020). Molecular mechanisms of Salmonella effector proteins: A comprehensive review. IDR 13, 11–26. doi: 10.2147/IDR.S230604
Baek, J., Yoon, H. (2023). Cyclic di-GMP Modulates a Metabolic Flux for Carbon Utilization in Salmonella enterica Serovar Typhimurium. Microbiol. Spectr. 11, e03685–e03622. doi: 10.1128/spectrum.03685-22
Bækkedal, C., Haugen, P. (2015). The Spot 42 RNA: A regulatory small RNA with roles in the central metabolism. RNA Biol. 12, 1071–1077. doi: 10.1080/15476286.2015.1086867
Bak, G., Han, K., Kim, D., Lee, Y. (2014). Roles of rpoS- activating small RNA s in pathways leading to acid resistance of Escherichia coli. MicrobiologyOpen 3, 15–28. doi: 10.1002/mbo3.143
Bandyra, K. J., Fröhlich, K. S., Vogel, J., Rodnina, M., Goyal, A., Luisi, B. F. (2024). Cooperation of regulatory RNA and the RNA degradosome in transcript surveillance. Nucleic Acids Res. 52, 9161–9173. doi: 10.1093/nar/gkae455
Battesti, A., Majdalani, N., Gottesman, S. (2011). The RpoS-mediated general stress response in Escherichia coli. Annu. Rev. Microbiol. 65, 189–213. doi: 10.1146/annurev-micro-090110-102946
Beisel, C. L., Storz, G. (2011). The base-pairing RNA spot 42 participates in a multioutput feedforward loop to help enact catabolite repression in Escherichia coli. Mol. Cell 41, 286–297. doi: 10.1016/j.molcel.2010.12.027
Bobrovskyy, M., Vanderpool, C. K. (2014). The small RNA SgrS: roles in metabolism and pathogenesis of enteric bacteria. Front. Cell. Infect. Microbiol. 4. doi: 10.3389/fcimb.2014.00061
Bouvier, M., Sharma, C. M., Mika, F., Nierhaus, K. H., Vogel, J. (2008). Small RNA binding to 5′ mRNA coding region inhibits translational initiation. Mol. Cell 32, 827–837. doi: 10.1016/j.molcel.2008.10.027
Brown, A. N., Anderson, M. T., Bachman, M. A., Mobley, H. L. T. (2022). The ArcAB two-component system: function in metabolism, redox control, and infection. Microbiol. Mol. Biol. Rev. 86, e00110–e00121. doi: 10.1128/mmbr.00110-21
Cai, L., Xie, Y., Hu, H., Xu, X., Wang, H., Zhou, G. (2023a). A small RNA, SaaS, promotes Salmonella pathogenicity by regulating invasion, intracellular growth, and virulence factors. Microbiol. Spectr. 11, e02938–e02922. doi: 10.1128/spectrum.02938-22
Cai, L., Xie, Y., Shao, L., Hu, H., Xu, X., Wang, H., et al. (2023b). SaaS sRNA promotes Salmonella intestinal invasion via modulating MAPK inflammatory pathway. Gut Microbes 15, 2211184. doi: 10.1080/19490976.2023.2211184
Cakar, F., Golubeva, Y. A., Vanderpool, C. K., Slauch, J. M. (2022). The Small RNA MicC Downregulates hilD Translation To Control the Salmonella Pathogenicity Island 1 Type III Secretion System in Salmonella enterica Serovar Typhimurium. J. Bacteriol. 204, e00378–e00321. doi: 10.1128/JB.00378-21
Calderón, P. F., Morales, E. H., Acuña, L. G., Fuentes, D. N., Gil, F., Porwollik, S., et al. (2014b). The small RNA RyhB homologs from Salmonella typhimurium participate in the response to S-nitrosoglutathione-induced stress. Biochem. Biophys. Res. Commun. 450, 641–645. doi: 10.1016/j.bbrc.2014.06.031
Calderón, I. L., Morales, E. H., Collao, B., Calderón, P. F., Chahuán, C. A., Acuña, L. G., et al. (2014a). Role of Salmonella Typhimurium small RNAs RyhB-1 and RyhB-2 in the oxidative stress response. Res. Microbiol. 165, 30–40. doi: 10.1016/j.resmic.2013.10.008
Chao, Y., Li, L., Girodat, D., Förstner, K. U., Said, N., Corcoran, C., et al. (2017). In vivo cleavage map illuminates the central role of RNase E in coding and non-coding RNA pathways. Mol. Cell 65, 39–51. doi: 10.1016/j.molcel.2016.11.002
Chatterjee, R., Chowdhury, A. R., Mukherjee, D., Chakravortty, D. (2023). From Eberthella typhi to Salmonella typhi: the fascinating journey of the virulence and pathogenicity of Salmonella typhi. ACS Omega 8, 25674–25697. doi: 10.1021/acsomega.3c02386
Chen, B., Meng, X., Ni, J., He, M., Chen, Y., Xia, P., et al. (2021). Positive regulation of Type III secretion effectors and virulence by RyhB paralogs in Salmonella enterica serovar Enteritidis. Vet Res. 52, 44. doi: 10.1186/s13567-021-00915-z
Chlebicz, A., Śliżewska, K. (2018). Campylobacteriosis, salmonellosis, yersiniosis, and listeriosis as zoonotic foodborne diseases: A review. IJERPH 15, 863. doi: 10.3390/ijerph15050863
Correia Santos, S., Bischler, T., Westermann, A. J., Vogel, J. (2021). MAPS integrates regulation of actin-targeting effector SteC into the virulence control network of Salmonella small RNA PinT. Cell Rep. 34, 108722. doi: 10.1016/j.celrep.2021.108722
Davidson, A. L., Dassa, E., Orelle, C., Chen, J. (2008). Structure, function, and evolution of bacterial ATP-binding cassette systems. Microbiol. Mol. Biol. Rev. 72, 317–364. doi: 10.1128/MMBR.00031-07
Doetsch, M., Schroeder, R., Fürtig, B. (2011). Transient RNA–protein interactions in RNA folding. FEBS J. 278, 1634–1642. doi: 10.1111/j.1742-4658.2011.08094.x
Dong, R., Liang, Y., He, S., Cui, Y., Shi, C., He, Y., et al. (2022). DsrA modulates central carbon metabolism and redox balance by directly repressing pflB expression in Salmonella typhimurium. Microbiol. Spectr. 10, e01522–e01521. doi: 10.1128/spectrum.01522-21
El Mouali, Y., Esteva-Martínez, G., García-Pedemonte, D., Balsalobre, C. (2021). Corrigendum: differential regulation of CsrC and CsrB by CRP-cAMP in Salmonella enterica. Front. Microbiol. 12. doi: 10.3389/fmicb.2021.723431
Ernst, D. C., Downs, D. M. (2016). 2-Aminoacrylate Stress Induces a Context-Dependent Glycine Requirement in ridA Strains of Salmonella enterica. J. Bacteriol. 198, 536–543. doi: 10.1128/JB.00804-15
Foster, J. W., Hall, H. K. (1990). Adaptive acidification tolerance response of Salmonella Typhimurium. J. Bacteriol. 172, 771–778. doi: 10.1128/jb.172.2.771-778.1990
Fröhlich, K. S., Haneke, K., Papenfort, K., Vogel, J. (2016). The target spectrum of SdsR small RNA in Salmonella. Nucleic Acids Res. 44, 10406–10422. doi: 10.1093/nar/gkw632
Fröhlich, K. S., Papenfort, K., Berger, A. A., Vogel, J. (2012). A conserved RpoS-dependent small RNA controls the synthesis of major porin OmpD. Nucleic Acids Res. 40, 3623–3640. doi: 10.1093/nar/gkr1156
Gupta, A. K., Siddiqui, N., Dutta, T. (2020). A novel mechanism of RyeA/SraC induction under acid stress. Biochem. Biophys. Res. Commun. 525, 298–302. doi: 10.1016/j.bbrc.2020.02.085
Gupta, A. K., Siddiqui, N., Yadav, D., Arora, L., Dutta, T. (2019). Regulation of RyeA/SraC expression in Escherichia coli. Biochem. Biophys. Res. Commun. 516, 661–665. doi: 10.1016/j.bbrc.2019.06.110
Gutierrez, A., Laureti, L., Crussard, S., Abida, H., Rodríguez-Rojas, A., Blázquez, J., et al. (2013). β-lactam antibiotics promote bacterial mutagenesis via an RpoS-mediated reduction in replication fidelity. Nat. Commun. 4, 1610. doi: 10.1038/ncomms2607
Hu, H., Jia, K., Wang, H., Xu, X., Zhou, G., He, S. (2020). Novel sRNA and regulatory genes repressing the adhesion of Salmonella enteritidis exposed to meat-related environment. Food Control 110, 107030. doi: 10.1016/j.foodcont.2019.107030
Iosub, I. A., Van Nues, R. W., McKellar, S. W., Nieken, K. J., Marchioretto, M., Sy, B., et al. (2020). Hfq CLASH uncovers sRNA-target interaction networks linked to nutrient availability adaptation. eLife 9, e54655. doi: 10.7554/eLife.54655
Jones, A. M., Goodwill, A., Elliott, T. (2006). Limited Role for the DsrA and RprA Regulatory RNAs in rpoS Regulation in Salmonella enterica. J. Bacteriol. 188, 5077–5088. doi: 10.1128/JB.00206-06
Jørgensen, M. G., Pettersen, J. S., Kallipolitis, B. H. (2020). sRNA-mediated control in bacteria: An increasing diversity of regulatory mechanisms. Biochim. Biophys. Acta (BBA) Gene Regul. Mech. 1863, 194504. doi: 10.1016/j.bbagrm.2020.194504
Ju, X., Fang, X., Xiao, Y., Li, B., Shi, R., Wei, C., et al. (2021). Small RNA GcvB regulates oxidative stress response of Escherichia coli. Antioxidants 10, 1774. doi: 10.3390/antiox10111774
Kabir, A., Kelley, W. G., Glover, C., Erol, E., Helmy, Y. A. (2025). Phenotypic and genotypic characterization of antimicrobial resistance and virulence profiles of Salmonella enterica serotypes isolated from necropsied horses in Kentucky. Microbiol. Spectr. 13, e0250124. doi: 10.1128/spectrum.02501-24
Kim, J. N. (2016). Roles of two RyhB paralogs in the physiology of Salmonella enterica. Microbiol. Res. 186–187, 146–152. doi: 10.1016/j.micres.2016.04.004
Kim, K., Golubeva, Y. A., Vanderpool, C. K., Slauch, J. M. (2019a). Oxygen-dependent regulation of SPI1 type three secretion system by small RNAs in Salmonella enterica serovar Typhimurium. Mol. Microbiol. 111, 570–587. doi: 10.1111/mmi.14174
Kim, J. N., Kwon, Y. M. (2013). Genetic and phenotypic characterization of the RyhB regulon in Salmonella Typhimurium. Microbiol. Res. 168, 41–49. doi: 10.1016/j.micres.2012.06.007
Kim, W., Lee, Y. (2020). Mechanism for coordinate regulation of rpoS by sRNA-sRNA interaction in Escherichia coli. RNA Biol. 17, 176–187. doi: 10.1080/15476286.2019.1672514
Kim, K., Palmer, A. D., Vanderpool, C. K., Slauch, J. M. (2019b). The small RNA PinT contributes to PhoP-mediated regulation of the Salmonella pathogenicity island 1 type III secretion system in Salmonella enterica serovar typhimurium. J. Bacteriol. 201, e00312–19. doi: 10.1128/JB.00312-19
King, K. A., Caudill, M. T., Caswell, C. C. (2022). A comprehensive review of small regulatory RNAs in Brucella spp. Front. Vet. Sci. 9. doi: 10.3389/fvets.2022.1026220
Lalaouna, D., Morissette, A., Carrier, M., Massé, E. (2015). DsrA regulatory RNA represses both hns and rbs D m RNA s through distinct mechanisms in E scherichia coli. Mol. Microbiol. 98, 357–369. doi: 10.1111/mmi.13129
Lalaouna, D., Simoneau-Roy, M., Lafontaine, D., Massé, E. (2013). Regulatory RNAs and target mRNA decay in prokaryotes. Biochim. Biophys. Acta (BBA) Gene Regul. Mech. 1829, 742–747. doi: 10.1016/j.bbagrm.2013.02.013
Lease, R. A., Cusick, M. E., Belfort, M. (1998). Riboregulation in Escherichia coli: DsrA RNA acts by RNA: RNA interactions at multiple loci. Proc. Natl. Acad. Sci. U.S.A. 95, 12456–12461. doi: 10.1073/pnas.95.21.12456
Liu, F., Chen, Z., Zhang, S., Wu, K., Bei, C., Wang, C., et al. (2023). In vivo RNA interactome profiling reveals 3’UTR-processed small RNA targeting a central regulatory hub. Nat. Commun. 14, 8106. doi: 10.1038/s41467-023-43632-1
Liu, B., Fang, J., Chen, H., Sun, Y., Yang, S., Gao, Q., et al. (2022). GcvB regulon revealed by transcriptomic and proteomic analysis in vibrio alginolyticus. IJMS 23, 9399. doi: 10.3390/ijms23169399
Long, J., Yang, C., Liu, J., Ma, C., Jiao, M., Hu, H., et al. (2024). Tannic acid inhibits Escherichia coli biofilm formation and underlying molecular mechanisms: Biofilm regulator CsgD. Biomed. Pharmacother. 175, 116716. doi: 10.1016/j.biopha.2024.116716
Ma, Z., Li, N., Jiao, J., Zuo, Y., Ling, Y., Cai, X., et al. (2024). The regulatory roles of sRNA GcvB in virulence in Salmonella typhimurium. J. Nuclear Agric. Sci. 38, 1048–1056. doi: 10.11869/j.issn.1000-8551.2024.06.1048
Malabirade, A., Habier, J., Heintz-Buschart, A., May, P., Godet, J., Halder, R., et al. (2018). The RNA complement of outer membrane vesicles from Salmonella enterica serovar typhimurium under distinct culture conditions. Front. Microbiol. 9. doi: 10.3389/fmicb.2018.02015
Mandin, P., Gottesman, S. (2010). Integrating anaerobic/aerobic sensing and the general stress response through the ArcZ small RNA. EMBO J. 29, 3094–3107. doi: 10.1038/emboj.2010.179
Massé, E., Gottesman, S. (2002). A small RNA regulates the expression of genes involved in iron metabolism in Escherichia coli. Proc. Natl. Acad. Sci. U.S.A. 99, 4620–4625. doi: 10.1073/pnas.032066599
Melamed, S., Peer, A., Faigenbaum-Romm, R., Gatt, Y. E., Reiss, N., Bar, A., et al. (2016). Global mapping of small RNA-target interactions in bacteria. Mol. Cell 63, 884–897. doi: 10.1016/j.molcel.2016.07.026
Meng, X., Cui, W., Meng, X., Wang, J., Wang, J., Zhu, G. (2021). A non-coding small RNA MicC contributes to virulence in outer membrane proteins in Salmonella Enteritidis. JoVE, e61808. doi: 10.3791/61808
Meng, X., He, M., Chen, B., Xia, P., Wang, J., Zhu, C., et al. (2023). RyhB paralogs downregulate the expressions of multiple survival-associated genes and attenuate the survival of Salmonella enteritidis in the chicken macrophage HD11. Microorganisms 11, 214. doi: 10.3390/microorganisms11010214
Meng, X., He, M., Xia, P., Wang, J., Wang, H., Zhu, G. (2022). Functions of small non-coding RNAs in Salmonella–host interactions. Biology 11, 1283. doi: 10.3390/biology11091283
Metaane, S., Monteil, V., Ayrault, S., Bordier, L., Levi-Meyreuis, C., Norel, F. (2022). The stress sigma factor σS/RpoS counteracts Fur repression of genes involved in iron and manganese metabolism and modulates the ionome of Salmonella enterica serovar Typhimurium. PloS One 17, e0265511. doi: 10.1371/journal.pone.0265511
Mika, F., Hengge, R. (2014). Small RNAs in the control of RpoS, CsgD, and biofilm architecture of Escherichia coli. RNA Biol. 11, 494–507. doi: 10.4161/rna.28867
Miyakoshi, M., Chao, Y., Vogel, J. (2015). Cross talk between ABC transporter m RNA s via a target m RNA -derived sponge of the G cv B small RNA. EMBO J. 34, 1478–1492. doi: 10.15252/embj.201490546
Miyakoshi, M., Okayama, H., Lejars, M., Kanda, T., Tanaka, Y., Itaya, K., et al. (2022). Mining RNA-seq data reveals the massive regulon of GcvB small RNA and its physiological significance in maintaining amino acid homeostasis in Escherichia coli. Mol. Microbiol. 117, 160–178. doi: 10.1111/mmi.14814
Monteiro, C., Papenfort, K., Hentrich, K., Ahmad, I., Le Guyon, S., Reimann, R., et al. (2012). Hfq and Hfq-dependent small RNAs are major contributors to multicellular development in Salmonella enterica serovar Typhimurium. RNA Biol. 9, 489–502. doi: 10.4161/rna.19682
Nava-Galeana, J., Núñez, C., Bustamante, V. H. (2023). Proteomic analysis reveals the global effect of the BarA/SirA-Csr regulatory cascade in Salmonella Typhimurium grown in conditions that favor the expression of invasion genes. J. Proteomics 286, 104960. doi: 10.1016/j.jprot.2023.104960
Papenfort, K., Podkaminski, D., Hinton, J. C. D., Vogel, J. (2012). The ancestral SgrS RNA discriminates horizontally acquired Salmonella mRNAs through a single G-U wobble pair. Proc. Natl. Acad. Sci. U.S.A. 109, E757–E764. doi: 10.1073/pnas.1119414109
Papenfort, K., Said, N., Welsink, T., Lucchini, S., Hinton, J. C. D., Vogel, J. (2009). Specific and pleiotropic patterns of mRNA regulation by ArcZ, a conserved, Hfq-dependent small RNA. Mol. Microbiol. 74, 139–158. doi: 10.1111/j.1365-2958.2009.06857.x
Parker, A., Gottesman, S. (2016). Small RNA regulation of TolC, the outer membrane component of bacterial multidrug transporters. J. Bacteriol. 198, 1101–1113. doi: 10.1128/JB.00971-15
Peñaloza, D., Acuña, L. G., Barros, M. J., Núñez, P., Montt, F., Gil, F., et al. (2021). The small RNA RyhB homologs from Salmonella typhimurium restrain the intracellular growth and modulate the SPI-1 gene expression within RAW264.7 macrophages. Microorganisms 9, 635. doi: 10.3390/microorganisms9030635
Pfeiffer, V., Papenfort, K., Lucchini, S., Hinton, J. C. D., Vogel, J. (2009). Coding sequence targeting by MicC RNA reveals bacterial mRNA silencing downstream of translational initiation. Nat. Struct. Mol. Biol. 16, 840–846. doi: 10.1038/nsmb.1631
Porcheron, G., Dozois, C. M. (2015). Interplay between iron homeostasis and virulence: Fur and RyhB as major regulators of bacterial pathogenicity. Vet. Microbiol. 179, 2–14. doi: 10.1016/j.vetmic.2015.03.024
Potts, A. H., Guo, Y., Ahmer, B. M. M., Romeo, T. (2019). Role of CsrA in stress responses and metabolism important for Salmonella virulence revealed by integrated transcriptomics. PloS One 14, e0211430. doi: 10.1371/journal.pone.0211430
Quendera, A. P., Seixas, A. F., Dos Santos, R. F., Santos, I., Silva, J. P. N., Arraiano, C. M., et al. (2020). RNA-binding proteins driving the regulatory activity of small non-coding RNAs in bacteria. Front. Mol. Biosci. 7. doi: 10.3389/fmolb.2020.00078
Repoila, F., Gottesman, S. (2001). Signal transduction cascade for regulation of RpoS: temperature regulation of DsrA. J. Bacteriol. 183, 4012–4023. doi: 10.1128/JB.183.13.4012-4023.2001
Ryan, D., Mukherjee, M., Suar, M. (2017). The expanding targetome of small RNAs in Salmonella Typhimurium. Biochimie 137, 69–77. doi: 10.1016/j.biochi.2017.03.005
Ryan, D., Ojha, U. K., Jaiswal, S. (2016). The small RNA DsrA influences the acid tolerance response and virulence of Salmonella enterica serovar typhimurium. Front. Microbiol. 7. doi: 10.3389/fmicb.2016.00599
Schultz, B. M., Melo-Gonzalez, F., Salazar, G. A., Porto, B. N., Riedel, C. A., Kalergis, A. M., et al. (2021). New insights on the early interaction between typhoid and non-typhoid Salmonella serovars and the host cells. Front. Microbiol. 12. doi: 10.3389/fmicb.2021.647044
Shaji, S., Selvaraj, R. K., Shanmugasundaram, R. (2023). Salmonella infection in poultry: A review on the pathogen and control strategies. Microorganisms 11, 2814. doi: 10.3390/microorganisms11112814
Sharma, C. M., Darfeuille, F., Plantinga, T. H., Vogel, J. (2007). A small RNA regulates multiple ABC transporter mRNAs by targeting C/A-rich elements inside and upstream of ribosome-binding sites. Genes Dev. 21, 2804–2817. doi: 10.1101/gad.447207
Stenum, T. S., Holmqvist, E. (2022). CsrA enters Hfq’s territory: Regulation of a base-pairing small RNA. Mol. Microbiol. 117, 4–9. doi: 10.1111/mmi.14785
Traore, K. A., Aboubacar-Paraiso, A. R., Bouda, S. C., Ouoba, J. B., Kagambèga, A., Roques, P., et al. (2024). Characteristics of nontyphoid Salmonella isolated from human, environmental, animal, and food samples in Burkina Faso: A systematic review and meta-analysis. Antibiotics 13, 556. doi: 10.3390/antibiotics13060556
Tsai, C.-H., Liao, R., Chou, B., Palumbo, M., Contreras, L. M. (2015). Genome-wide analyses in bacteria show small-RNA enrichment for long and conserved intergenic regions. J. Bacteriol. 197, 40–50. doi: 10.1128/JB.02359-14
Urbanowski, M. L., Stauffer, L. T., Stauffer, G. V. (2000). The gcvB gene encodes a small untranslated RNA involved in expression of the dipeptide and oligopeptide transport systems in Escherichia coli. Mol. Microbiol. 37, 856–868. doi: 10.1046/j.1365-2958.2000.02051.x
Vakili, S., Haeili, M., Feizi, A., Moghaddasi, K., Omrani, M., Ghodousi, A., et al. (2025). Whole-genome sequencing-based characterization of Salmonella enterica Serovar Enteritidis and Kentucky isolated from laying hens in northwest of Iran 2022–2023. Gut Pathog. 17, 2. doi: 10.1186/s13099-025-00679-3
Viegas, S. C., Pfeiffer, V., Sittka, A., Silva, I. J., Vogel, J., Arraiano, C. M. (2007). Characterization of the role of ribonucleases in Salmonella small RNA decay. Nucleic Acids Res. 35, 7651–7664. doi: 10.1093/nar/gkm916
Viegas, S. C., Silva, I. J., Saramago, M., Domingues, S., Arraiano, C. M. (2011). Regulation of the small regulatory RNA MicA by ribonuclease III: a target-dependent pathway. Nucleic Acids Res. 39, 2918–2930. doi: 10.1093/nar/gkq1239
Wang, H., Huang, M., Zeng, X., Peng, B., Xu, X., Zhou, G. (2020). Resistance profiles of Salmonella isolates exposed to stresses and the expression of small non-coding RNAs. Front. Microbiol. 11. doi: 10.3389/fmicb.2020.00130
Waters, S. A., McAteer, S. P., Kudla, G., Pang, I., Deshpande, N. P., Amos, T. G., et al. (2017). Small RNA interactome of pathogenic E. coli revealed through crosslinking of RNase E. EMBO J. 36, 374–387. doi: 10.15252/embj.201694639
Watkins, D., Arya, D. (2023). Models of Hfq interactions with small non-coding RNA in Gram-negative and Gram-positive bacteria. Front. Cell. Infect. Microbiol. 13. doi: 10.3389/fcimb.2023.1282258
Westermann, A. J., Förstner, K. U., Amman, F., Barquist, L., Chao, Y., Schulte, L. N., et al. (2016). Dual RNA-seq unveils noncoding RNA functions in host–pathogen interactions. Nature 529, 496–501. doi: 10.1038/nature16547
Worley, M. J. (2023). Salmonella bloodstream infections. TropicalMed 8, 487. doi: 10.3390/tropicalmed8110487
Keywords: Salmonella, sRNA, infection, virulence, regulation
Citation: He T, Ding Y, Sun Y and Li T (2025) Advances in sRNA-mediated regulation of Salmonella infection in the host. Front. Cell. Infect. Microbiol. 15:1503337. doi: 10.3389/fcimb.2025.1503337
Received: 28 September 2024; Accepted: 23 April 2025;
Published: 15 May 2025.
Edited by:
Asis Das, University of Connecticut, United StatesReviewed by:
Juan F. González, Nationwide Children’s Hospital, United StatesRhishita Chourashi, University of Maryland, United States
Copyright © 2025 He, Ding, Sun and Li. This is an open-access article distributed under the terms of the Creative Commons Attribution License (CC BY). The use, distribution or reproduction in other forums is permitted, provided the original author(s) and the copyright owner(s) are credited and that the original publication in this journal is cited, in accordance with accepted academic practice. No use, distribution or reproduction is permitted which does not comply with these terms.
*Correspondence: Tiansen Li, dHNsaV9ka3lAaGFpbmFudS5lZHUuY24=