- 1Division of Pulmonary, Allergy, and Critical Care Medicine, Department of Medicine, The University of Alabama at Birmingham, Birmingham, AL, United States
- 2Gregory Fleming James Cystic Fibrosis Research Center, The University of Alabama at Birmingham, Birmingham, AL, United States
- 3Department of Microbiology, The University of Alabama at Birmingham, Birmingham, AL, United States
The leading cause of death for people with cystic fibrosis (pwCF) continues to be due to respiratory-related illnesses. Both wound repair and immune cell responses are dysregulated in the CF airways, creating a cycle of unresolved injury and perpetuating inflammation. PwCF are predisposed to colonization and infections with opportunistic bacteria like Pseudomonas aeruginosa (Pa), the most common adult pathogen in CF. Pa possesses key virulence factors that can exacerbate chronic inflammation and lung injury. With the approval of highly effective modulator therapies like elexacaftor/tezacaftor/ivacaftor (ETI), pwCF eligible for ETI have seen drastic improvements in lung function and clinical outcomes, including an increased life expectancy. While modulator therapies are improving bronchial epithelial cellular processes in wound repair and some areas of immunity, many of these processes do not reach a non-CF baseline state or have not been thoroughly studied. The effect of modulator therapy on Pa may lead to a reduction in infection, but in more longitudinal studies, there is not always eradication of Pa, and colonization and infection frequency can return to pre-modulator levels over time. Finally, in this review we explore the current state of additional treatments for CF lung disease, independent of CFTR genotype, including anti-inflammatories, phage-therapies, and Pa vaccines.
1 Introduction
Cystic Fibrosis (CF) is an autosomal recessive disorder that leads to ion imbalance in the epithelium of organs such as the pancreas, gastrointestinal tract, and the lungs (Shteinberg et al., 2021). Mutations in the cystic fibrosis transmembrane conductance regulator (CFTR) gene lead to dysfunctional protein, impaired protein trafficking, or in some cases, complete absence of the CFTR protein. Without treatment, CFTR dysfunction causes multisystem complications with significant disease burden and a decreased life (Elborn, 2016). The leading cause of death for people with CF (pwCF) in 2023 was due to respiratory-related illnesses (Cystic Fibrosis Foundation, 2024). The absence or dysfunction of CFTR at the epithelial membrane results in poor mucociliary clearance in the lung along with several other physiological changes that provide a suitable environment for opportunistic infection with pathogens such as Pseudomonas aeruginosa (Pa) (Elborn, 2016). Pa is a gram-negative bacterium that poses a significant threat to pwCF due to its ability to establish chronic lung infections, and such infections strongly correlate with decreased lung function and increased mortality (Lyczak et al., 2002; Bhagirath et al., 2016). Pa is the most common lung pathogen of adult pwCF and can produce many virulence factors that cause and exacerbate lung damage, leading to colonization and recurrent infections (Bhagirath et al., 2016; Cystic Fibrosis Foundation, 2024).
Wound repair is a dynamic process with various cellular regulators, many of which are dysfunctional in CF (Hilliard et al., 2007). This dysfunction leads to a delayed repair process, exacerbating already increased inflammation and reducing the CF lung’s ability to combat threats like bacterial infection with Pa (Conese and Di Gioia, 2021). At least 50% of pwCF aged 35 or older are expected to develop advanced lung disease, which is characterized by severely impaired lung function and airway remodeling as a result of aberrant wound repair (Conese and Di Gioia, 2021; Cystic Fibrosis Foundation, 2024). Therefore, research to advance the understanding of wound repair mechanisms in the chronically infected CF lung is of high importance to ultimately develop therapeutic approaches to restore these processes.
In addition to the cellular regulators of wound repair and the abnormal properties (e.g. thick mucus) caused by defective CFTR, specific immune cell populations are also negatively affected in CF. Neutrophils and macrophages, in particular, exhibit compromised phagocytic capabilities and inflammatory signaling, leading to chronic infection and inflammation (Hayes et al., 2011; Harwood et al., 2021). Lymphoid cells and eosinophils also play a role in CF pathogenesis, but their contributions remain less studied (Liu et al., 1999; Harwood et al., 2021).
Over the past decade, the development of CFTR modulator therapies which include potentiators, correctors, stabilizers, and amplifiers has significantly improved the prognosis of pwCF (Lopes-Pacheco, 2019). There has been robust improvement in lung pathophysiology in pwCF, particularly by the highly effective modulator combination therapy of elexacaftor/tezacaftor/ivacaftor (ETI) (Lupas et al., 2024). One of the remaining questions that continues to be a source of debate in this field is the long-term effect of CFTR modulators on cellular processes in the CF lung, specifically on wound repair mechanisms, immune cell function, and susceptibility to Pa infection.
This review will focus on the elucidation of mechanisms behind CF lung repair in response to 1- injury following infection; 2- virulence strategies employed by Pa to damage and impair these processes; and 3- the complex immune system mechanisms to combat Pa. Moreover, this review will explore how recent advances in CFTR modulator therapies have affected all these processes and discuss other therapeutic strategies on the horizon for pwCF. We aim to provide a broad overview of wound repair, Pa infection, and related immune cell processes in the CF lung prior to modulator therapy in order to consider the implications of ETI on these process and further future directions in understanding the CF lung environment.
2 Wound repair mechanisms
2.1 Injury repair in the bronchial epithelium
The mature lung has traditionally been viewed as a quiescent organ with minimal regenerative capacity. However, the lung possesses a unique and remarkable capacity to maintain homeostasis despite injury through extensive wound repair mechanisms that are similar to the mechanisms of general wound repair. Pulmonary epithelial wound repair has been reviewed at great length elsewhere (Crosby and Waters, 2010; Croasdell Lucchini et al., 2021); however, we will provide a brief overview here.
In response to an epithelial insult in the lung, an initial acute inflammatory phase results in the activation of tissue resident macrophages followed by the recruitment of additional inflammatory cells to the site of injury (Wynn and Vannella, 2016). Injured epithelial cells as well as the immune cell environment release a variety of soluble mediators and chemokines such as epidermal growth factor, which recruit progenitor cell populations to the site of injury and initiate the wound repair process (Van Winkle et al., 1997; Kim et al., 1998; Croasdell Lucchini et al., 2021). This signaling milieu, moreover, results in the expression of tissue remodeling and extracellular matrix (ECM) proteins such as collagens, fibronectin, and matrix metalloproteinases (MMPs), among others, which aid in the migration and proliferation of epithelial cells about the site of injury (Rickard et al., 1993; Legrand et al., 1999; Coraux et al., 2008; Ojo et al., 2015). Transforming growth factor beta (TGF-β) signaling further plays a vital role in the re-epithelialization process as its initiation results in the epithelial-mesenchymal transition (EMT), which aids in the formation of a provision ECM network across which cell migration and remodeling can occur (Kim et al., 2006; Câmara and Jarai, 2010). Importantly, the EMT is often dysregulated in pathogenesis and has been described in a variety of lung disease states including idiopathic pulmonary fibrosis, pulmonary hypertension, and cancer.
A growing body of evidence suggests that following lung injury, stem cell progenitor populations and/or self-renewing cell types are recruited to reestablish a continuous and functional epithelial surface (Kotton and Morrisey, 2014). One of the greatest challenges to understanding the injury response in the airway epithelium is great redundancy in the regenerative capacity of the many subpopulations within the bronchial epithelium. The basal cell has classically been identified as a pulmonary stem population, particularly in the proximal airways, with the capacity to give rise to an assortment of cell populations including ciliated and goblet cells (Ruysseveldt et al., 2021). The field of progenitor cell biology in the lung is a frontier of novel research as new regenerative cell types are still being discovered (Basil et al., 2022).
2.2 Wound repair in the CF lung
Chronic inflammation and aberrant injury repair are constants in the CF lung. Only recently, some of the mechanisms in which this occurs have been elucidated including protease/anti-protease imbalances of neutrophil elastase (NE), MMPs, and other proteases which have been noted in CF pathogenesis, all of which contribute to tissue damage and remodeling (Hilliard et al., 2007) (Fischer et al., 2013). Additionally, molecular markers of injury repair are increased for an extended period, including MMP-9, TGF-β1, fibronectin, vimentin, and integrin β1, and a decrease in E-cadherin (Conese and Di Gioia, 2021). While many of these proteins are associated with EMT, which as discussed previously, is an essential step in healthy injury repair, the CF lung appears to have a prolonged and aberrant EMT state, contributing to further chronic inflammation and injury.
The perpetuating cycle of chronic inflammation found in the CF lung results in the imbalance of pro- and anti-inflammatory mediators, such as increases in interleukin-8 (IL-8) and IL-6, and decreases in IL-10 (Courtney et al., 2004). This pattern of increased inflammation has been shown to play a role in not only damaging the epithelium, but also in dysregulating repair and remodeling (Shute et al., 2003; Courtney et al., 2004; Virella-Lowell et al., 2004; Conese and Di Gioia, 2021).
Cellular senescence is defined by irreversible cell cycle growth arrest, which occurs in response to cellular stressors such as inflammation (Campisi and d’Adda di Fagagna, 2007; Herranz and Gil, 2018). Senescent cells are resistant to apoptosis and acquire a senescence associated secretory phenotype (SASP), which stimulates secretion of pro-inflammatory mediators that perpetuate tissue damage and inflammation (Hernandez-Segura et al., 2018). Transient cellular senescence is a crucial part of normal wound repair, but disease states occur with persistent senescence, which can overwhelm the immune system to effectively clear these cells (Wilkinson and Hardman, 2020). Several studies including one from Easter et al. found that cellular senescence markers are upregulated in the CF bronchial epithelium when compared to non-CF controls at baseline, suggesting another mechanism that could be dysregulating wound repair in the CF bronchial epithelium and one of many reasons for the compounding lung injury that pwCF deal with overtime (Nakamura et al., 1992; Fischer et al., 2013; Easter et al., 2024).
In summary, many studies have shown evidence for dysregulated wound repair in the CF lung, which can be further complicated by chronic airway infection with Pa modulating wound repair in the CF lung even further (Figure 1).
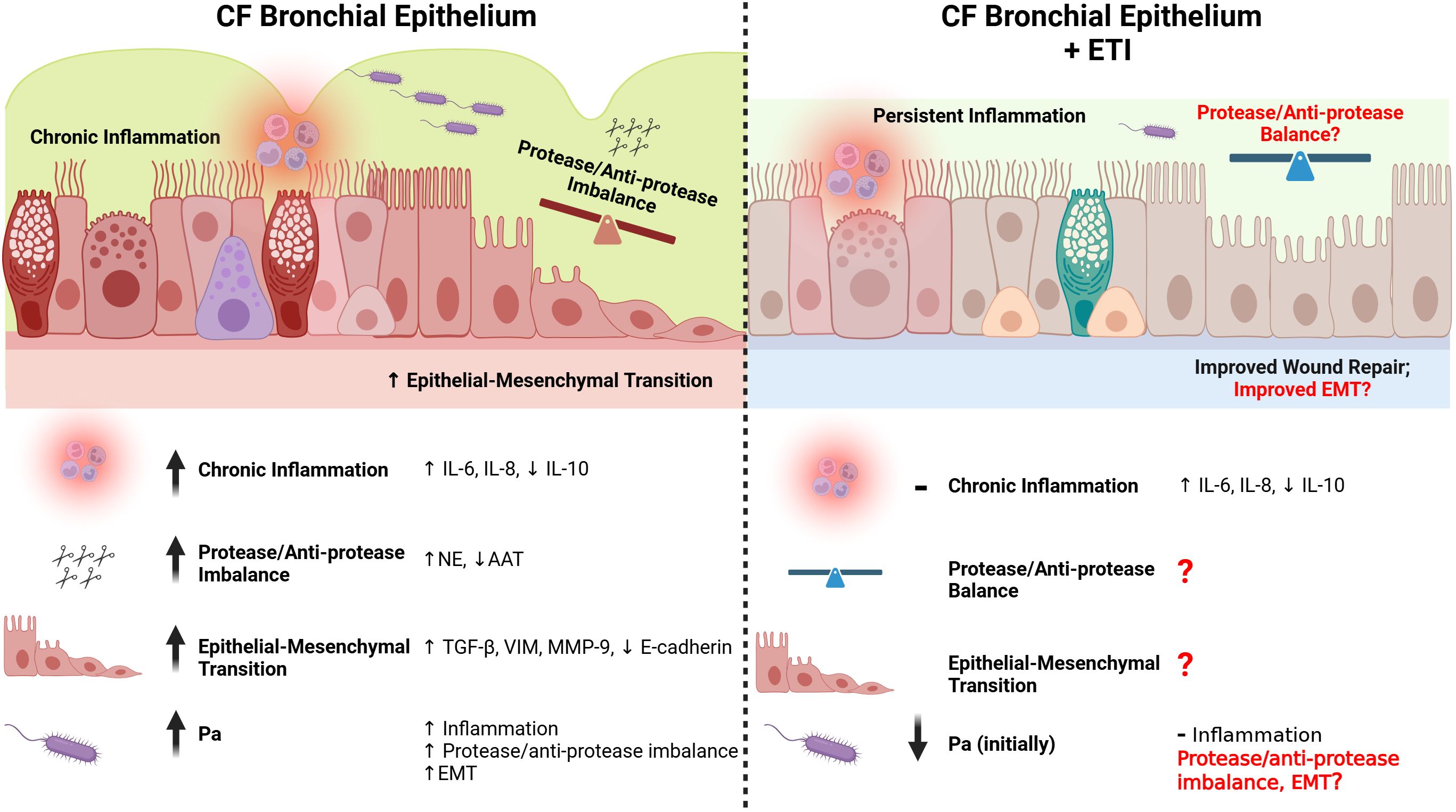
Figure 1. Repair in the CF bronchial epithelium before and after ETI therapy. The CF bronchial epithelium is subject to chronic inflammation, partially as a result of Pa infection and a subsequent dysfunctional immune response (see Figure 2). Pro-inflammatory cytokines (e.g. IL-6, IL-8) are upregulated in the CF bronchial epithelium, while anti-inflammatory cytokines are downregulated (e.g. IL-10). Even after the initiation of ETI, many markers of chronic inflammation are only slightly improved or are unchanged. The protease/anti-protease ratios are imbalanced in the CF bronchial epithelium, with increased NE and decreased AAT. This imbalance causes weaker cell-cell junctions, and a delayed healing process. The protease/anti-protease axis has not been well explored after initiation of ETI, especially in the context of Pa infection. The epithelial-mesenchymal transition (EMT) is prolonged in the CF bronchial epithelium, with greater increases in TGF-β, vimentin, MMP-9, and a decrease in E-cadherin when responding to injury. While ETI causes an improvement in wound repair rates in the CF bronchial epithelium, there have not been studies on the effect of ETI on EMT with or without Pa infection. Created in https://BioRender.com.
3 The effects of Pseudomonas aeruginosa on wound repair
Pa is a gram-negative opportunistic pathogen and a major causative organism in a variety of chronic pneumonia, eye infections, burns, diabetic ulcers, etc. This versatility is due not only to its broad arsenal of virulence factors, but its dynamic production of these factors over time. It’s long been established that Pa poorly interacts with intact, healthy, and well polarized epithelium (Tsang et al., 1994; Schwarzer et al., 2016). However, in pwCF, the airway epithelium demonstrates increased tight junction leakage, cell death, senescence, remodeling, and division. These factors increase the capacity for the establishment of Pa infection. The prevalence of Pa in pwCF <2 years-old is under 30%, but at the age of 25 years and older, greater than 50% of pwCF are infected with Pa (Rosenfeld et al., 2003; Foundation, 2021).
Pa infections can be separated into either acute or chronic infections. These infections exist on a spectrum, but acute infections are more directly cytotoxic and invasive whereas Pa strains in chronic infections tend to be more resistant to treatment, mucoid, and sessile. In acute infections, Pa breaches the epithelium and accessing the basolateral side of the cell layer where most cytotoxic factors are delivered. Once colonized, Pa will become less cytotoxic and more resistant over time. This transition is marked by increased biofilm production and decreased motility factors (Iwanska et al., 2023), leading to the formation of non-moving, resistant infections (Mahenthiralingam et al., 1994; Ramsey and Wozniak, 2005). This resistant Pa state can be disrupted, and shift towards more acute Pa phenotypes that can lead to pulmonary exacerbations (Aaron et al., 2004).
Throughout this process, Pa employs a multitude of virulence factors that will either induce the damage or impair the repair processes. For the purposes of this review, we will only discuss a few examples of Pa virulence factors found to both induce damage and affect wound repair in the lung.
3.1 Factors contributing to wound induction
Cell-junction destruction is crucial in Pa exacerbating pulmonary symptoms in pwCF, as this destruction allows for cytotoxic products to be released in deeper cell layers. Pa rhamnolipids can displace important linker proteins and disrupt the tight junctions at the lung basolateral membrane (Zulianello et al., 2006). There is also a positive correlation between rhamnolipid production and acute infection isolates (Laabei et al., 2014). LecA and LecB have been demonstrated to increase Pa-induced alveolar capillary permeability (Chemani et al., 2009). LecB has been shown to be crucial to Pa’s ability to loosen cell-substrate adhesion (Thuenauer et al., 2020). Other enzymes such as LasB elastase have been shown to degrade key proteins in the extracellular matrix and basement membrane (Guzzo et al., 1991; Coin et al., 1997).
Once the barrier to the basolateral side has been subverted Pa can release substances that lead to direct cytotoxicity, exacerbating cell death and tissue injury. Examples include the Type III secretion system (T3SS) effectors ExoS and ExoT that have been shown to induce apoptosis and cause actin cytoskeleton collapse (Ganesan et al., 1999; Shaver and Hauser, 2004).
Lipopolysaccharide (LPS) can trigger inflammatory responses and pyroptotic cell death, which is highly pro-inflammatory in nature (Schoeniger et al., 2016; Huang et al., 2019). Pyocyanin is a secondary metabolite capable of generating reactive oxygen species (ROS) (O’Malley et al., 2003), and has been detected in large quantities in the sputum of pwCF infected with Pa, enough to inhibit ciliary beating and cause toxicity in the in vitro respiratory epithelium (Kanthakumar et al., 1993).
3.2 Factors of wound repair alteration
Several of these Pa virulence factors have also been shown to alter cellular repair rates. LecB, has been shown to impair repair processes through decreasing migration and proliferation of human lung epithelial cells and diminishing cell-cell contacts (Cott et al., 2016). ExoT has also been shown to impair repair in A549 alveolar cell monolayers, secondary to actin cytoskeleton disorganization (Geiser et al., 2001). Pyocyanin inhibits wound repair by inducing premature growth arrest of human diploid fibroblasts, causing the development of characteristics associated with cellular senescence (Muller, 2006).
LasB elastase promotes collagen degradation by inducing the conversion of the inactive precursors of several MMP to active enzymes, thus inhibiting proper collagen placement (de Bentzmann et al., 2000). LasB, as well as some previously described factors, are controlled under quorum sensing (QS) systems. One group demonstrated that inhibiting QS dampened the combined action of LasB and LasA proteases, highlighting an alternative strategy to allow for efficient repair of the airway epithelium despite infection (Ruffin et al., 2016; Ruffin and Brochiero, 2019).
In total, Pa has a multitude of virulence factors that can cause lung injury directly and indirectly by dysregulating host injury repair mechanisms. How Pa virulence factors directly alter repair mechanisms is still an area that needs more investigation, especially in the context of CF affecting not only the airway epithelium but also impacting the immune system of the lung.
4 Immune cells and their effect on lung repair in the CF lung
CFTR dysfunction in selected immune cell populations has been shown to have a negative effect on tissue repair and exacerbates the inflammatory phenotype already prevalent in CF. This section will go into further detail on the altered phenotypes of these cell populations, their role in tissue injury/repair, and their interaction with Pa.
4.1 Neutrophils
PwCF have higher baseline levels of neutrophil recruitment factors including IL-6, IL-8, and TNF-α, leading to an increase in neutrophil recruitment with disputes on whether this occurs prior to infection or after the first infection (Hayes et al., 2011; Giacalone et al., 2020; Aslanhan et al., 2021). When compared to non-CF neutrophils, CF neutrophils shed less L-selectin and are less responsive to IL-8 due to the downregulation of CXCR2, increased mucus in the bronchial epithelium, and the presence of Pa-produced alginate (Hayes et al., 2011; Aslanhan et al., 2021; Laucirica et al., 2022; Hu et al., 2024). These factors lead to a reduction in neutrophil recruitment and their bactericidal effects (Zhang et al., 2021).
During the recruitment process, CF neutrophils exhibit the GRIM (Granule releasing, immunoregulatory, and metabolically active) phenotype (Margaroli et al., 2021; Laucirica et al., 2022), which is characterized by a reduced ability to phagocytose bacteria (Giacalone et al., 2020). This phenotype occurs due to the CFTR defect leading to impaired chloride transport and lack of hypochlorous acid production, a vital component of the antibacterial activity of neutrophils (Cabrini et al., 2020).
GRIM neutrophils have decreased CD16 expression post migration and have increased levels of CD63, which is activated by CF mucus and further increased in the presence of Pa infection (Laucirica et al., 2022; Hu et al., 2024). It is also known that GRIM neutrophils exocytose high levels of cathepsin G, MPO, Arg1, and NE, which are parts of the secondary defense that neutrophils display during degranulation (Giacalone et al., 2020). However, when transcription is inhibited, GRIM neutrophils had decreased degranulation with increased levels of bacterial killing compared to baseline (Margaroli et al., 2021).
There is debate on whether ROS, secreted by neutrophils in CF can exacerbate inflammation and injury in the lung. Some reports state that oxidative burst and ROS exacerbate CF-associated inflammatory changes and infection in the CF lung (Cabrini et al., 2020; Aslanhan et al., 2021), while others claim no difference in ROS and C-reactive protein between pwCF and healthy controls (Kelk et al., 2022).
The protease to anti-protease ratio is severely skewed in CF as NE dominates the airway and cleaves anti-proteases such as secretory leukocyte inhibitor (SLPI) and alpha-1 antitrypsin (AAT) (Hayes et al., 2011; Houston et al., 2024). The CFTR protein is an identified substrate for NE along with IL-8 receptor CXCR1, elastin, collagen, Fcγ receptors, and MMP9 (Houston et al., 2024).
Finally, CF neutrophils also have dysregulated cell death pathways with increased levels of the anti-apoptotic marker MCL-1 along with increases in p21 and neutrophil survival regulator proliferating cell nuclear antigen (PCNA) (Chiara et al., 2012). This study implicates these proteins as potential targets for inducing apoptosis in CF neutrophils. Additionally, CF neutrophils do not undergo spontaneous apoptosis due to decreases in Fas and FasL along with TNF-α death receptors (Hayes et al., 2011).
NE serum levels are elevated in CF patients and together with increased AAT levels, they attenuate inflammation promoting wound closure in a CF in vitro model (Garratt et al., 2016).
CF neutrophils are less responsive to recruitment signals, have reduced bactericidal effects when recruited, exocytose high levels of degranulation molecules (NE) that lead to injury, have decreased wound repair signal levels (AAT), and have delayed apoptosis compared to healthy neutrophil functions.
4.2 Macrophages
CF macrophages have been found to be dysfunctional in several ways, including a decreased ability to phagocytose due to defective autophagy (Harwood et al., 2021; Badr et al., 2022; Aridgides et al., 2023). Circulating monocytes from pwCF show significantly higher baseline levels of activation compared to non-CF donors, but their ability to mount a type 1 IFN signaling response against Pa LPS is diminished (Gillan et al., 2023) and their response to attenuate inflammation is delayed (Slimmen et al., 2023; Wellems et al., 2023).
In addition, CF macrophages have decreased iron metabolism protein heme oxygenase-1 leading to more iron availability, which is utilized by Pa as a nutrient promoting Pa growth and biofilm formation (Hazlett et al., 2020; Harwood et al., 2021; Kircheva et al., 2024; Zhang et al., 2024). The combination of increased pro-inflammatory cytokines and an impaired phagocytotic activity and an arrest in M1 leads to over recruitment of CF neutrophils creating a positive feedback loop contributing to continuous lung damage in the CF airway.
Oz et al. demonstrated that CF macrophages positive for the CCL2 and CCL7 ligand receptor (CCR2) can drive pathogenic TGFβ signaling and sustain a pro-inflammatory environment by constitutively recruiting neutrophils. The same study found that CF mice had higher levels of M1 macrophages and neutrophils than the WT after Pa LPS stimulation and had more severe lung damage, collagen deposition, airway wall thickening and higher levels of TGFβ (Oz et al., 2022).
4.3 Lymphoid cells
Lymphoid cells express the CFTR protein and in CF, they are ineffective in clearing Pa at least partially due to being Th17 and Th2 cells rather than Th1, which leads to impaired antigen presentation, increased levels of immunoglobulin (Ig) E, and excessive neutrophil infiltration (Harwood et al., 2021). This Th17 and Th2 phenotype is likely in part due to increased levels of IL-17 and IL-10, which hinder antigen presentation, and of IL-6, essential for neutrophil recruitment, in pwCF (Hagner et al., 2021; Harwood et al., 2021). In an in vivo model mimicking CF lung disease, knockout of IL-17A led to reduced structural lung damage and decreased levels of lymphocytes, implicating IL-17A and lymphocytes as important mediators of CF lung inflammation (Hagner et al., 2021).
From a B-cell perspective, pwCF have increased lymphoid follicles and Pa infection leads to upregulation of the B-cell chemoattractants CXCL13, CCL19, and CCL21 (Neill et al., 2014; Polverino et al., 2019). However, there is conflicting evidence for elevation of B-cell activating factor (BAFF) in pwCF at baseline and how this affects Pa bacterial burden (Neill et al., 2014; Garic et al., 2019; Polverino et al., 2019).
Up to now, lymphocytes have not been shown to be involved directly in wound repair mechanisms in the CF lung.
4.4 Eosinophils
Eosinophils are well characterized in allergic asthma and have been described in several studies in CF. Most CF patients do not show any change in the absolute eosinophil count (AEC) in their lungs, however, the levels of eosinophil cationic protein (EPA), an eosinophil activation marker, is upregulated in the sputum and serum of pwCF (Koller et al., 1994; Liu et al., 1999). This could be due to NE mediated degranulation of eosinophils indirectly increasing EPA levels (Liu et al., 1999).
There is no evidence of correlation between Pa infection and eosinophil count (Albon et al., 2023), however, there is a population of pwCF that have both CF and asthma which have elevated levels of AECs, which was correlated with more symptoms and higher exacerbation numbers (Ye et al., 2022).
Overall, it does not appear that eosinophils in CF play a key role in Pa infection but contribute to the already dysfunctional immune response with increased levels of EPA.
Eosinophils have been found to play a role in lung injury repair. Eosinophils produce TGF-β, which is a well-known inducer of EMT (Phipps et al., 2002; Siddiqui et al., 2023). Eosinophils also express fibroblast growth factor 2, vascular endothelial growth factor, and IL-4, which have been shown in wound repair of the skin (Phipps et al., 2002). Therefore, eosinophils are potentially one of the cell types that initiate and activate remodeling and repair processes in wound healing, but more research is needed in CF eosinophils and their influence on lung epithelial repair.
In summary, neutrophils and macrophages are affected in CF, and their dysfunction causes a delay in eradicating Pa and wound repair after Pa -induced injury. Lymphoid cells are also dysregulated in CF, contributing to the often-ineffective immune response to Pa infection. In contrast, eosinophils have not been widely implicated in CF immune responses to Pa but have been found to be important in lung injury repair processes in general (Figure 2).
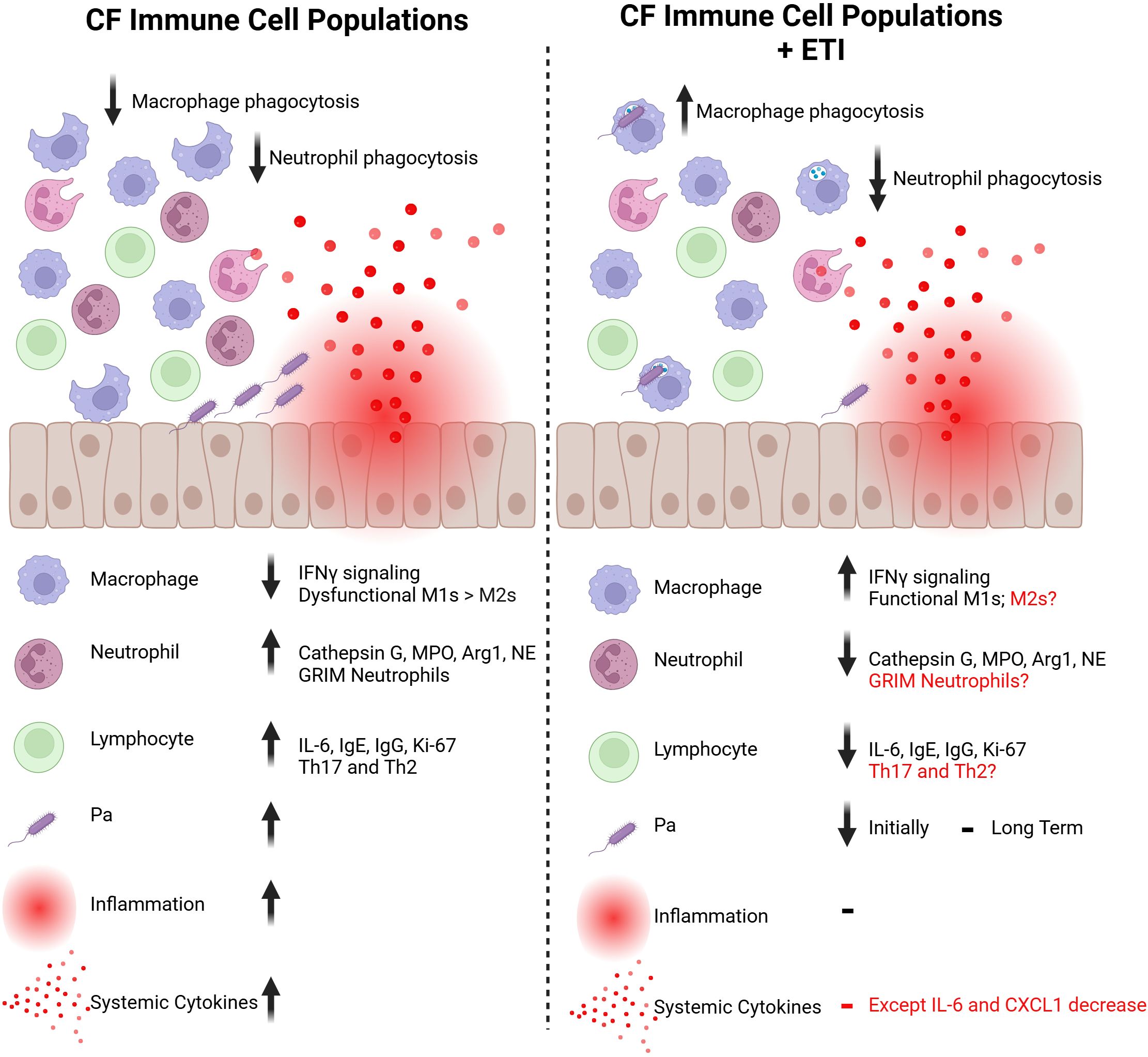
Figure 2. Immune cell populations in the CF bronchial epithelium before and after ETI therapy. The immune cell populations that reside in and interact with the CF bronchial epithelium have attenuated responses to injury. Macrophages and neutrophils have reduced phagocytic capabilities against bacteria like Pa and exacerbate inflammatory signals. With ETI therapy, CF macrophages show improved phagocytosis through an increase in IFNγ signaling. However, CF neutrophils have further decreased phagocytic capabilities. CF neutrophils tend to be over-recruited to sites of injury, which is improved with ETI initiation. Lymphocytes are also upregulated at baseline in the CF bronchial epithelium but the impact of ETI treatment on this population is largely unknown. The dysregulated immune response allows for higher levels of colonization with bacteria like Pa. With ETI therapy, levels of Pa colonization are reduced in short-term studies. However, levels of Pa have been shown to rebound with long-term ETI treatment. Due to this dysfunctional immune response, the CF bronchial epithelium has high levels of inflammation, resulting in higher levels of systemic cytokines. This local and systemic inflammation is not returned to baseline with ETI therapy, but improvements are seen in cytokines such as IL-6. Created in https://BioRender.com.
5 Highly effective modulator therapy in CF
The highly effective modulator therapy consisting of ETI was first approved for use in the United States in 2019 to treat pwCF with at least one F508del mutation, which is the most common genetic mutation, as well as at least 177 other mutations to date (Cystic Fibrosis Foundation, 2020; Dailymed, 2020). Several studies have shown that lung function and sweat chloride significantly improve with ETI therapy (Zemanick et al., 2021). This section of the review will focus on the effect of ETI on the CF lung including the airway epithelium, wound repair, and the immune system.
5.1 Effects of ETI on CF bronchial epithelium
ETI can efficiently restore CFTR function in CF bronchial epithelial cells from donors with eligible mutations; ETI attenuates lipid dysregulation, such as an increased fatty acid ratio, and oxidative stress in CF bronchial epithelial cell cultures (Veltman et al., 2021). However, ETI treatment led to a two-fold increase in de-novo synthesis of sphingolipids in primary epithelial cell cultures in both CF and non-CF subjects, implicating that this phenomenon is a potential off-target effect of ETI therapy (Liessi et al., 2023).
5.2 Effects of ETI on wound repair
A 2018 study suggested that the combination of lumacaftor (VX-809) + ivacaftor (VX-770) improved wound repair rates and transepithelial resistance in primary epithelial cell cultures from CF donors with CFTR trafficking mutations (Adam et al., 2018). This effect was also observed in the presence of Pa exoproducts. In another study, Laselva et al. demonstrated that ETI-treated bronchial epithelial cells that overexpress the F508del mutation exhibit accelerated wound repair relative to vehicle treated controls (Laselva and Conese, 2022), whereas Easter et al. did not find any attenuation in the pro-inflammatory response nor reduction in cellular senescence in the bronchial epithelium (Easter et al., 2024). However, these studies did not explore the effect of ETI on any wound repair specific markers in CF airways (Figure 1).
5.3 Effects of ETI on Pa infection
Introduction of ETI was presumed to lead to eradication of Pa in the CF lung, given the rapid decrease in Pa bacterial burden found with initiation of Ivacaftor and only modest increase in Pa one year post-Ivacaftor initiation (Hisert et al., 2017). Early studies assessing the effect of Pa burden on pwCF post ETI initiation have demonstrated a modest decrease in positive Pa cultures; however, Pa is not eradicated in pwCF on ETI therapy for more than one year (Gushue et al., 2023; Burgel et al., 2024; Dittrich et al., 2024; Szabo et al., 2024). Recent reports have shown that reduction or eradication of Pa is more likely in pwCF, who are younger and colonized for a shorter period of time, when compared to older pwCF, who are colonized for longer and with a multi-drug resistant Pa (Dittrich et al., 2024; Szabo et al., 2024).
Taken this together, Pa colonization and recurrent infections are still evident in the post-ETI era. Analysis of the Pa genome pre- and post- ETI initiation demonstrates strains do not change post-ETI initiation, but Pa has the ability to adapt in this new environment showing mutations in iron metabolism, alginate production, and biofilm formation (Armbruster et al., 2024; Ledger et al., 2024).
5.4 Effects of ETI on immune cells
Although lung function and mucociliary clearance improves in the majority with ETI therapy, the effect of ETI on the innate immune system and systemic inflammation is controversial (Figure 2). Although there seems to be a trend of ETI-mediated decrease in pro-inflammatory markers, those levels do not reach a non-CF baseline level (Casey et al., 2023; Schmidt et al., 2023; Wang et al., 2023; Westholter et al., 2023; Jarosz-Griffiths et al., 2024). Most studies have found decreases in pro-inflammatory markers including IL-6, MMP9, and CXCL1, but no differences in IL-8 levels, which is the key cytokine mediating neutrophil recruitment in the CF lung.
The effect of ETI on neutrophils is an ongoing area of research with one study observing no differences in neutrophil activation marker baseline levels between pwCF before ETI and 6 months post initiation (Schmidt et al., 2023). Furthermore, ROS production and chemotactic activity did not change post ETI, and the phagocytotic capacity decreased down post ETI initiation, in contrary to the expected improvement.
Neutrophil cytokine secretion of IL-1β, TNF, and IL-6 decreased with ETI therapy, along with caspase-1 levels (Jarosz-Griffiths et al., 2024) and the number of sputum neutrophils remained reduced from 3 months to 1 year post ETI initiation in addition to decreases in NE, AAT, and systemic decrease in IL-8 (Casey et al., 2023).
These data would indicate that although neutrophils secrete less proinflammatory mediators, their ability to be activated and phagocytose is not fully restored by ETI treatment. These data also highlight the importance of further studies, especially longitudinal studies to determine the long-term effects of ETI on the neutrophil and other immune cell populations at different stages of CF lung disease severity.
Some studies have shown that in the presence of ETI therapy and either Pa or Burkolderia cepacia complex infection, CF macrophage phagocytosis and killing improved but cytokine secretion was not affected (Aridgides et al., 2023; Zhang et al., 2023). These papers did not address whether the total number of macrophages was impacted by ETI but did show that ETI enhanced the M1 phenotype with little benefit for M2 macrophages, which are involved in injury repair and resolution of inflammation. Additionally, it has been shown that the stunted IFN signaling in macrophages is rescued with ETI therapy (Gillan et al., 2023).
The current literature is controversial in regard to the benefits of modulator therapy on lymphoid cells. One report did not mention differences in circulating T cells with mono- or dual modulator therapy, whereas another study observed increased levels post ETI therapy (Eschenhagen et al., 2023; Westholter et al., 2023). Westholter et al. noted decreased IL-6 serum levels 6 months post ETI initiation and Eschenhagen et al. reported decreases in IgE, IgG, and Ki-67 levels post ETI initiation (Eschenhagen et al., 2023). In the presence of Pa infection, some reports show decreased levels of regulatory T cells (Tregs) (Westholter et al., 2023).
There is very little known regarding the effect of ETI therapy on the eosinophil population, and eosinophils are not key mediator cells in Pa infections. However, one recent study showed that ETI led to a decrease in IgE levels but did not affect eosinophil count in a study population of 108 pwCF (Mehta et al., 2023).
6 Future directions and discussion
6.1 Treatments to benefit the CF lung
Although modulator therapy has greatly increased the life expectancy and quality of life in most pwCF, there are still patients not eligible for HEMT and others who experience a limited impact on various cell populations in the CF lung. Therefore, continued research is needed to better characterize short- and long-term effects of ETI that takes the severity of disease, as well as severity of lung injury, into consideration.
6.1.1 CFTR modulators
Current studies in the field of modulators include small molecule screening to target nonsense-mediated decay of the CFTR transcript due to stop codon mutations (Sharma et al., 2021; Kim et al., 2022; Chen et al., 2023). There are also ongoing clinical trials for mRNA correction therapy (Rowe et al., 2023).
Additionally, to add to the current modulators, compounds able to stabilize or amplify the CFTR protein are another area of research that is being done in this field to increase the half-life of the CFTR protein (Donaldson et al., 2017; Bengtson et al., 2022).
6.1.2 Anti-inflammatory treatments
To combat the chronic inflammation found in pwCF, there are a few avenues being researched as potential therapeutic targets for regulating the inflammatory axis. TNF signaling, more specifically the LTa-TNFN2 axis, has been implicated as a therapeutic target given the protective role that Etanercept, a molecule that increases oxidative stress resistance, exerts on epithelial cells in vitro when challenged by H2O2 (Checa et al., 2024).
IL-22 signaling is also being considered as a therapeutic target given its role as an integral molecule to prevent Pa-mediated lung damage. PwCF have lower levels of IL-22 which is associated with increased levels of serine protease-3 (Guillon et al., 2019). Therefore, either supplementation with IL-22 and/or reinstating the protease to anti-protease ratio will be pivotal for resolving chronic inflammation in CF.
Other studies explore ways to improve inflammation in CF by acting on specific immune populations. One such study found that the compound Tanshinone IIA promotes decreased inflammation and tissue repair by inducing neutrophil reverse migration and induced apoptosis in an in vivo CFTR KO zebrafish model, which could also be a potential therapeutic target for the delayed apoptosis of the CF neutrophil (Bernut et al., 2020). Another potential therapeutic target could be formyl peptide receptor 1 (FPR-1) which regulates neutrophil function and is expressed on their cell surface. Mice deficient in this protein were protected from lung injury via a bleomycin model (Leslie et al., 2020).
The use of IL-6-elafin (a serine protease inhibitor) genetically modified macrophages have been found to better fight Pa infections and were found to increase epithelial cells proliferation and tissue repair in a murine model (Kheir et al., 2022). Finally, Cappiello et al. found esculentin (Esc) (1-21) and Esc(1-21)-1-c, both antimicrobial peptides, detoxify LPS shown through decreases in IL-6 and cyclooxygenase-2 and further lead to cell migration in epithelial cells (Cappiello et al., 2021). Suggesting that these molecules could also be therapeutic targets for chronic infections such as Pa and assist the immune cell population.
Another avenue to potentially promote resolution of chronic inflammation is to elucidate the role of extracellular vesicles (EVs) in the context of Pa infection. EVs have been found to be particles that contain miRNA, ECM proteins, cytokines, and much more (Useckaite et al., 2020; Sarkar et al., 2024). They have been implicated in several disease states including CF. Recently, a study found that EVs secreted by bronchial epithelial cells were able to reduce Pa burden and inflammation in CF mouse lungs (Sarkar et al., 2024). Given this, further research regarding the role that EVs play in these mechanisms will be crucial to finding therapeutic targets for both chronic inflammation and chronic infection with Pa.
One study explored treatment with the senolytic cocktail Dasatinib + Quercetin (D+Q) that not only decreased cellular senescence markers in a Cftr-/- knockout rat trachea model but also decreased pro-inflammatory cytokine secretion and significantly improved mucociliary clearance (Easter et al., 2024). This same study by Easter et al. also explored the mechanisms governing this increase in senescent cells and found that fibroblast growth factor receptor inhibition attenuated senescence markers and improved mucociliary clearance.
Continued research in this field will be vital to uncovering ways to control the chronic inflammation that exacerbates respiratory complications found in CF.
6.1.3 Treatment strategies to promote tissue repair
There are not many studies on the promotion of tissue repair in the CF lung, but one such study found that in addition to being preventative for inflammation, IL-22 was also found to lead to higher wound-closure rates in vitro (Guillon et al., 2019). Leading to the possibility of IL-22 treatment being a functional treatment for multiple aspects in tissue repair and inflammation.
Other interesting discoveries include the use of electro-sprayed mesenchymal stromal cell extracellular matrix nanoparticles to accelerate cellular wound healing and also reduce Pa growth (Wandling et al., 2023). Another study found that antipseudomonal peptides esculentin-1a(1-21)NH2 and Esc(1-21)-1c were found to counteract the inhibitory effect of Pa -LPS on wound healing which in turn enhanced IL-8 production and MMP9 activity (Cappiello et al., 2019).
6.2 Advancements in treatments to target Pa infection in the CF lung
Pa is notoriously difficult to eradicate due to adaptive systems that can evade antibiotics. These include biofilm-mediated resistance, hypermutation of antibiotic targets, and formation of multidrug-resistant cells (Mulcahy et al., 2010; Breidenstein et al., 2011). The current treatment regimen for Pa in the CF lung is to attempt early eradication with inhaled antibiotics, tobramycin or tobramycin/ciprofloxacin (Mogayzel et al., 2014). This approach aims to attenuate Pa-mediated airway remodeling, but has been not very successful for most pwCF. Most develop Pa colonization and chronic suppressive therapy with inhaled antibiotics, such as tobramycin, colistin, or aztreonam lysine, becomes the mainstay of treatment (Smith and Rowbotham, 2022).
While the development of novel antimicrobials is desperately under explored, some promising and innovative examples are currently in the pipeline. This section is not exhaustive of all new potential Pa treatments; however, it highlights some of the areas that are generating the most interest currently. Treatments targeting Pa colonization and/or infection will indirectly improve the processes of wound repair, as Pa possesses many virulence factors that directly affect damage and repair (as reviewed in Section 3). Lowering the levels of Pa in the lung would also indirectly affect immune function, as Pa is highly pro-inflammatory.
6.2.1 Conventional antibiotics
Avibactam, vaborbactam, and relebactam are among the new β-lactam–β-lactamase inhibitors which have shown promising results when combined with other conventional pseudomonal antibiotics (Yahav et al., 2020). Cefiderocol is a novel siderophore cephalosporin with activity against a wide spectrum of Gram-negative micro-organisms, including multidrug-resistant Pa infections (Hackel et al., 2018; Meschiari et al., 2021). Plazomicin is a parenteral aminoglycoside recently approved by the FDA for the management of complicated urinary tract infections and pyelonephritis caused by susceptible organisms, including Pa (Losito et al., 2022). While it does not seem to be superior to other aminoglycosides such as amikacin, gentamicin, and tobramycin against Pa, it does give health-care providers a new tool.
6.2.2 Anti-biofilm and anti-quorum sensing
Biofilms and bacterial communications are a source of antibiotic resistance; therefore, some groups are developing strategies to directly target these systems. One example is a low molecular weight alginate oligomer, known as OligoG. In vitro studies have demonstrated it was able to inhibit Pa mucoid biofilm formation and disrupt established mucoid biofilms. It was also able to potentiate the effects of other antimicrobials by increasing diffusion within biofilms (Powell et al., 2018). OligoG has now entered phase 2b clinical trials to assess safety, efficacy and tolerability in adult pwCF (2019). Another example of biofilm disruption is PLG0206, an engineered cationic antimicrobial peptide. Initial studies have demonstrated broad-spectrum activity and the capacity to significantly inhibit in vitro Pa biofilm growth on airway epithelial cells (Lashua et al., 2016), which ultimately can attenuate chronic inflammation and aberrant wound repair.
6.2.3 Phage therapy
Phage therapy has been an increasingly popular alternative for antibiotic treatment, especially for patients who have been unresponsive to conventional antibiotics. Due to limited production and distribution, most cases of successful bacteriophage usage are described in individual case reports, however, few examples are promising. A personalized aerosolized bacteriophage treatment was successfully used to treat a patient with chronic lung infection caused by multidrug-resistant (MDR) Pa (Kohler et al., 2023) resulting in clinical improvement and progressive clearance of lung consolidations. Another case described by Law et al. used bacteriophage therapy in a 26-year-old cystic fibrosis (CF) patient awaiting lung transplantation for pneumonia treatment. The patient did not have recurrence of Pa pneumonia and CF exacerbations within 100 days following the end of therapy and underwent successful bilateral lung transplantation 9 months later (Law et al., 2019).
There are several clinical trials currently underway to study the safety and efficacy of using single and multi-phage treatments to target Pa in pwCF (2020; Tamma et al., 2022; Ling et al., 2023). While these treatments and trials are exciting, there still are many unanswered questions regarding phage therapy, specifically the effect of phages on wound repair mechanisms in the chronically infected lung.
6.2.4 Vaccines
Finally, a true breakthrough discovery for Pa infection prevention would be an effective vaccine, the hunt for which has been underway for nearly 50 years. An important challenge for obtaining a good vaccine is the identification of ideal antigens. Antigen variability, plasticity of virulence factor expression during acute to chronic infection and bacterial localization are a challenge (Hoggarth et al., 2019; Sainz-Mejias et al., 2020; Lopez-Siles et al., 2021). However, if successfully developed, a vaccine against Pa for at-risk patients could reduce the prevalence of infections, the overall burden of disease, and the use of antibiotic treatment. Four vaccines have entered phase III trials with some showing promising results, but no anti- Pa vaccine has yet been approved. As discussed for other anti- Pa treatments, it remains to be studied whether vaccines would have direct effects on wound repair mechanisms.
7 Conclusion
In conclusion, the wound repair mechanisms and immune cell function are both altered in the CF lung, which is further exacerbated by many virulence factors of Pa. There are several cellular mechanisms we discussed that could use more exploration, such as specific cell signaling pathways altered in dysfunctional wound repair, or the possible roles that eosinophils can play in wound repair.
The FDA approval of HEMT has changed the clinical landscape of care for over 90% of pwCF. We discussed the current evidence that ETI is improving metrics in inflammation and bacterial burden, but many of these metrics are not returned to baseline states when compared to non-CF. Notably, Pa is not always eradicated from the CF lung after ETI initiation, and the same Pa strains have been observed in pwCF pre- and post-ETI initiation. In addition, Pa has impressive versatility and the ability to adapt quickly in new environments, raising concerns on the continued effects of Pa on repair mechanisms in the ETI-treated CF bronchial epithelium.
ETI treatment has also not been well explored in the context of cell signaling mechanisms, leaving many questions on how this treatment will change the CF lung’s ability to “repair itself”. Additionally, we explored other potential avenues of treatment for pwCF, ranging from anti-inflammatories to phage therapy. These studies demonstrate the need for further research into the cellular mechanisms attributing to CF disease states, highlight the currently evolving landscape of treatment with ETI, and shed light on treatments that could be available in the future for pwCF, regardless of their CF mutations.
Author contributions
ELM: Writing – original draft, Writing – review & editing, Project administration. MH: Visualization, Writing – original draft, Writing – review & editing. FP: Writing – original draft, Writing – review & editing. LJ: Visualization, Writing – original draft, Writing – review & editing. EM: Writing – review & editing. JB: Writing – review & editing. SK: Supervision, Writing – review & editing.
Funding
The author(s) declare that no financial support was received for the research and/or publication of this article.
Conflict of interest
The authors declare that the research was conducted in the absence of any commercial or financial relationships that could be construed as a potential conflict of interest.
Generative AI statement
The author(s) declare that no Generative AI was used in the creation of this manuscript.
Publisher’s note
All claims expressed in this article are solely those of the authors and do not necessarily represent those of their affiliated organizations, or those of the publisher, the editors and the reviewers. Any product that may be evaluated in this article, or claim that may be made by its manufacturer, is not guaranteed or endorsed by the publisher.
References
Aaron, S. D., Ramotar, K., Ferris, W., Vandemheen, K., Saginur, R., Tullis, E., et al. (2004). Adult cystic fibrosis exacerbations and new strains of Pseudomonas aeruginosa. Am. J. Respir. Crit. Care Med. 169, 811–815. doi: 10.1164/rccm.200309-1306OC
Adam, D., Bilodeau, C., Sognigbe, L., Maille, E., Ruffin, M., Brochiero, E. (2018). CFTR rescue with VX-809 and VX-770 favors the repair of primary airway epithelial cell cultures from patients with class II mutations in the presence of Pseudomonas aeruginosa exoproducts. J. Cyst. Fibros. 17, 705–714. doi: 10.1016/j.jcf.2018.03.010
Albon, D., Zhang, L., Patrie, J., Jones, M., Li, Z. G., Noonan, E., et al. (2023). Association between Cystic Fibrosis exacerbations, lung function, T2 inflammation and microbiological colonization. Allergy Asthma Clin. Immunol. 19, 15. doi: 10.1186/s13223-023-00760-z
Aridgides, D. S., Mellinger, D. L., Gwilt, L. L., Hampton, T. H., Mould, D. L., Hogan, D. A., et al. (2023). Comparative effects of CFTR modulators on phagocytic, metabolic and inflammatory profiles of CF and nonCF macrophages. Sci. Rep. 13, 11995. doi: 10.1038/s41598-023-38300-9
Armbruster, C. R., Hilliam, Y. K., Zemke, A. C., Atteih, S., Marshall, C. W., Moore, J., et al. (2024). Persistence and evolution of Pseudomonas aeruginosa following initiation of highly effective modulator therapy in cystic fibrosis. mBio 15, e0051924. doi: 10.1128/mbio.00519-24
Aslanhan, U., Cakir, E., Pur Ozyigit, L., Kucuksezer, U. C., Gelmez, Y. M., Yuksel, M., et al. (2021). Pseudomonas aeruginosa colonization in cystic fibrosis: Impact on neutrophil functions and cytokine secretion capacity. Pediatr. Pulmonol. 56, 1504–1513. doi: 10.1002/ppul.25294
(2019). A Phase 2b Randomised, Double-blind, Parallel-group Study of Alginate Oligosaccharide (OligoG) Dry Powder Inhalation in Addition to Standard of Care Compared to Placebo in Addition to Standard of Care in Patients with Cystic Fibrosis (CF).
(2020). CYstic Fibrosis bacterioPHage Study at Yale (CYPHY): A Single-site, Randomized, Double-blind, Placebo-controlled Study of Bacteriophage Therapy YPT-01 for Pseudomonas Aeruginosa Infections in Adults With Cystic Fibrosis.
Badr, A., Eltobgy, M., Krause, K., Hamilton, K., Estfanous, S., Daily, K. P., et al. (2022). CFTR modulators restore acidification of autophago-lysosomes and bacterial clearance in cystic fibrosis macrophages. Front. Cell Infect. Microbiol. 12. doi: 10.3389/fcimb.2022.819554
Basil, M. C., Cardenas-Diaz, F. L., Kathiriya, J. J., Morley, M. P., Carl, J., Brumwell, A. N., et al. (2022). Human distal airways contain a multipotent secretory cell that can regenerate alveoli. Nature 604, 120–126. doi: 10.1038/s41586-022-04552-0
Bengtson, C., Silswal, N., Baumlin, N., Yoshida, M., Dennis, J., Yerrathota, S., et al. (2022). The CFTR amplifier nesolicaftor rescues TGF-beta1 inhibition of modulator-corrected F508del CFTR function. Int. J. Mol. Sci. 23, 10956. doi: 10.3390/ijms231810956
Bernut, A., Loynes, C. A., Floto, R. A., Renshaw, S. A. (2020). Deletion of cftr leads to an excessive neutrophilic response and defective tissue repair in a zebrafish model of sterile inflammation. Front. Immunol. 11. doi: 10.3389/fimmu.2020.01733
Bhagirath, A. Y., Li, Y., Somayajula, D., Dadashi, M., Badr, S., Duan, K. (2016). Cystic fibrosis lung environment and Pseudomonas aeruginosa infection. BMC Pulm. Med. 16, 174. doi: 10.1186/s12890-016-0339-5
Breidenstein, E. B., de la Fuente-Nunez, C., Hancock, R. E. (2011). Pseudomonas aeruginosa: all roads lead to resistance. Trends Microbiol. 19, 419–426. doi: 10.1016/j.tim.2011.04.005
Burgel, P. R., Ballmann, M., Drevinek, P., Heijerman, H., Jung, A., Mainz, J. G., et al. (2024). Considerations for the use of inhaled antibiotics for Pseudomonas aeruginosa in people with cystic fibrosis receiving CFTR modulator therapy. BMJ Open Respir. Res. 11, e002049. doi: 10.1136/bmjresp-2023-002049
Cabrini, G., Rimessi, A., Borgatti, M., Lampronti, I., Finotti, A., Pinton, P., et al. (2020). Role of cystic fibrosis bronchial epithelium in neutrophil chemotaxis. Front. Immunol. 11. doi: 10.3389/fimmu.2020.01438
Câmara, J., Jarai, G. (2010). Epithelial-mesenchymal transition in primary human bronchial epithelial cells is Smad-dependent and enhanced by fibronectin and TNF-alpha. Fibrogen. Tissue Repair 3, 2. doi: 10.1186/1755-1536-3-2
Campisi, J., d’Adda di Fagagna, F. (2007). Cellular senescence: when bad things happen to good cells. Nat. Rev. Mol. Cell Biol. 8, 729–740. doi: 10.1038/nrm2233
Cappiello, F., Carnicelli, V., Casciaro, B., Mangoni, M. L. (2021). Antipseudomonal and immunomodulatory properties of esc peptides: promising features for treatment of chronic infectious diseases and inflammation. Int. J. Mol. Sci. 22, 557. doi: 10.3390/ijms22020557
Cappiello, F., Ranieri, D., Carnicelli, V., Casciaro, B., Chen, H. T., Ferrera, L., et al. (2019). Bronchial epithelium repair by Esculentin-1a-derived antimicrobial peptides: involvement of metalloproteinase-9 and interleukin-8, and evaluation of peptides’ immunogenicity. Sci. Rep. 9, 18988. doi: 10.1038/s41598-019-55426-x
Casey, M., Gabillard-Lefort, C., McElvaney, O. F., McElvaney, O. J., Carroll, T., Heeney, R. C., et al. (2023). Effect of elexacaftor/tezacaftor/ivacaftor on airway and systemic inflammation in cystic fibrosis. Thorax 78, 835–839. doi: 10.1136/thorax-2022-219943
Checa, J., Fiol, P., Guevara, M., Aran, J. M. (2024). TNFRSF1B signaling blockade protects airway epithelial cells from oxidative stress. Antioxid. (Basel) 13, 368. doi: 10.3390/antiox13030368
Chemani, C., Imberty, A., de Bentzmann, S., Pierre, M., Wimmerova, M., Guery, B. P., et al. (2009). Role of LecA and LecB lectins in Pseudomonas aeruginosa-induced lung injury and effect of carbohydrate ligands. Infect. Immun. 77, 2065–2075. doi: 10.1128/IAI.01204-08
Chen, J., Thrasher, K., Fu, L., Wang, W., Aghamohammadzadeh, S., Wen, H., et al. (2023). The synthetic aminoglycoside ELX-02 induces readthrough of G550X-CFTR producing superfunctional protein that can be further enhanced by CFTR modulators. Am. J. Physiol. Lung Cell Mol. Physiol. 324, L756–L770. doi: 10.1152/ajplung.00038.2023
Chiara, A. D., Pederzoli-Ribeil, M., Burgel, P. R., Danel, C., Witko-Sarsat, V. (2012). Targeting cytosolic proliferating cell nuclear antigen in neutrophil-dominated inflammation. Front. Immunol. 3. doi: 10.3389/fimmu.2012.00311
Coin, D., Louis, D., Bernillon, J., Guinand, M., Wallach, J. (1997). LasA, alkaline protease and elastase in clinical strains of Pseudomonas aeruginosa: quantification by immunochemical methods. FEMS Immunol. Med. Microbiol. 18, 175–184. doi: 10.1111/j.1574-695X.1997.tb01043.x
Conese, M., Di Gioia, S. (2021). Pathophysiology of lung disease and wound repair in cystic fibrosis. Pathophysiology 28, 155–188. doi: 10.3390/pathophysiology28010011
Coraux, C., Roux, J., Jolly, T., Birembaut, P. (2008). Epithelial cell-extracellular matrix interactions and stem cells in airway epithelial regeneration. Proc. Am. Thorac. Soc. 5, 689–694. doi: 10.1513/pats.200801-010AW
Cott, C., Thuenauer, R., Landi, A., Kuhn, K., Juillot, S., Imberty, A., et al. (2016). Pseudomonas aeruginosa lectin LecB inhibits tissue repair processes by triggering beta-catenin degradation. Biochim. Biophys. Acta 1863, 1106–1118. doi: 10.1016/j.bbamcr.2016.02.004
Courtney, J. M., Ennis, M., Elborn, J. S. (2004). Cytokines and inflammatory mediators in cystic fibrosis. J. Cyst. Fibros. 3, 223–231. doi: 10.1016/j.jcf.2004.06.006
Croasdell Lucchini, A., Gachanja, N. N., Rossi, A. G., Dorward, D. A., Lucas, C. D. (2021). Epithelial cells and inflammation in pulmonary wound repair. Cells 10, 339. doi: 10.3390/cells10020339
Crosby, L. M., Waters, C. M. (2010). Epithelial repair mechanisms in the lung. Am. J. Physiol. Lung Cell Mol. Physiol. 298, L715–L731. doi: 10.1152/ajplung.00361.2009
Cystic Fibrosis Foundation (2020). FDA Approves Expansion of Modulators for People With Certain Rare Mutations. CF Foundation. Available online at: https://web.archive.org/web/20211003191030/https:/www.cff.org/News/News-Archive/2020/FDA-Approves-Expansion-of-Modulators-for-People-With-Certain-Rare-Mutations/ (Accessed 11/20/2024).
Dailymed (2020). TRIKAFTA- elexacaftor, tezacaftor, and ivacaftor kit (U.S. National Library of Medicine: NIH). Available online at: https://web.archive.org/web/20200721105004/https:/dailymed.nlm.nih.gov/dailymed/drugInfo.cfm?setid=f354423a-85c2-41c3-a9db-0f3aee135d8d (Accessed 2024).
de Bentzmann, S., Polette, M., Zahm, J. M., Hinnrasky, J., Kileztky, C., Bajolet, O., et al. (2000). Pseudomonas aeruginosa virulence factors delay airway epithelial wound repair by altering the actin cytoskeleton and inducing overactivation of epithelial matrix metalloproteinase-2. Lab. Invest. 80, 209–219. doi: 10.1038/labinvest.3780024
Dittrich, A. M., Sieber, S., Naehrlich, L., Burkhart, M., Hafkemeyer, S., Tummler, B. (2024). Use of elexacaftor/tezacaftor/ivacaftor leads to changes in detection frequencies of Staphylococcus aureus and Pseudomonas aeruginosa dependent on age and lung function in people with cystic fibrosis. Int. J. Infect. Dis. 139, 124–131. doi: 10.1016/j.ijid.2023.11.013
Donaldson, S. H., Solomon, G. M., Zeitlin, P. L., Flume, P. A., Casey, A., McCoy, K., et al. (2017). Pharmacokinetics and safety of cavosonstat (N91115) in healthy and cystic fibrosis adults homozygous for F508DEL-CFTR. J. Cyst. Fibros. 16, 371–379. doi: 10.1016/j.jcf.2017.01.009
Easter, M., Hirsch, M. J., Harris, E., Howze, P., Matthews, E. L., Jones, L. I., et al. (2024). FGF receptors mediate cellular senescence in the cystic fibrosis airway epithelium. JCI Insight 9, e174888. doi: 10.1172/jci.insight.174888
Eschenhagen, P. N., Bacher, P., Grehn, C., Mainz, J. G., Scheffold, A., Schwarz, C. (2023). Proliferative activity of antigen-specific CD154+ T cells against bacterial and fungal respiratory pathogens in cystic fibrosis decreases after initiation of highly effective CFTR modulator therapy. Front. Pharmacol. 14. doi: 10.3389/fphar.2023.1180826
Fischer, B. M., Wong, J. K., Degan, S., Kummarapurugu, A. B., Zheng, S., Haridass, P., et al. (2013). Increased expression of senescence markers in cystic fibrosis airways. Am. J. Physiol. Lung Cell Mol. Physiol. 304, L394–L400. doi: 10.1152/ajplung.00091.2012
Ganesan, A. K., Vincent, T. S., Olson, J. C., Barbieri, J. T. (1999). Pseudomonas aeruginosa exoenzyme S disrupts Ras-mediated signal transduction by inhibiting guanine nucleotide exchange factor-catalyzed nucleotide exchange. J. Biol. Chem. 274, 21823–21829. doi: 10.1074/jbc.274.31.21823
Garic, D., Tao, S., Ahmed, E., Youssef, M., Kanagaratham, C., Shah, J., et al. (2019). Depletion of BAFF cytokine exacerbates infection in Pseudomonas aeruginosa infected mice. J. Cyst. Fibros. 18, 349–356. doi: 10.1016/j.jcf.2018.11.015
Garratt, L. W., Sutanto, E. N., Ling, K. M., Looi, K., Iosifidis, T., Martinovich, K. M., et al. (2016). Alpha-1 antitrypsin mitigates the inhibition of airway epithelial cell repair by neutrophil elastase. Am. J. Respir. Cell Mol. Biol. 54, 341–349. doi: 10.1165/rcmb.2015-0074OC
Geiser, T. K., Kazmierczak, B. I., Garrity-Ryan, L. K., Matthay, M. A., Engel, J. N. (2001). Pseudomonas aeruginosa ExoT inhibits in vitro lung epithelial wound repair. Cell Microbiol. 3, 223–236. doi: 10.1046/j.1462-5822.2001.00107.x
Giacalone, V. D., Margaroli, C., Mall, M. A., Tirouvanziam, R. (2020). Neutrophil adaptations upon recruitment to the lung: new concepts and implications for homeostasis and disease. Int. J. Mol. Sci. 21, 851. doi: 10.3390/ijms21030851
Gillan, J. L., Chokshi, M., Hardisty, G. R., Clohisey Hendry, S., Prasca-Chamorro, D., Robinson, N. J., et al. (2023). CAGE sequencing reveals CFTR-dependent dysregulation of type I IFN signaling in activated cystic fibrosis macrophages. Sci. Adv. 9, eadg5128. doi: 10.1126/sciadv.adg5128
Guillon, A., Brea, D., Luczka, E., Herve, V., Hasanat, S., Thorey, C., et al. (2019). Inactivation of the interleukin-22 pathway in the airways of cystic fibrosis patients. Cytokine 113, 470–474. doi: 10.1016/j.cyto.2018.10.015
Gushue, C., Eisner, M., Bai, S., Johnson, T., Holtzlander, M., McCoy, K., et al. (2023). Impact of Elexacaftor-Tezacaftor-Ivacaftor on lung disease in cystic fibrosis. Pediatr. Pulmonol. 58, 2308–2316. doi: 10.1002/ppul.26485
Guzzo, J., Pages, J. M., Duong, F., Lazdunski, A., Murgier, M. (1991). Pseudomonas aeruginosa alkaline protease: evidence for secretion genes and study of secretion mechanism. J. Bacteriol. 173, 5290–5297. doi: 10.1128/jb.173.17.5290-5297.1991
Hackel, M. A., Tsuji, M., Yamano, Y., Echols, R., Karlowsky, J. A., Sahm, D. F. (2018). In vitro activity of the siderophore cephalosporin, cefiderocol, against carbapenem-nonsusceptible and multidrug-resistant isolates of gram-negative bacilli collected worldwide in 2014 to 2016. Antimicrob. Agents Chemother. 62, e01968–17. doi: 10.1128/AAC.01968-17
Hagner, M., Albrecht, M., Guerra, M., Braubach, P., Halle, O., Zhou-Suckow, Z., et al. (2021). IL-17A from innate and adaptive lymphocytes contributes to inflammation and damage in cystic fibrosis lung disease. Eur. Respir. J. 57, 1900716. doi: 10.1183/13993003.00716-2019
Harwood, K. H., McQuade, R. M., Jarnicki, A., Schneider-Futschik, E. K. (2021). Anti-inflammatory influences of cystic fibrosis transmembrane conductance regulator drugs on lung inflammation in cystic fibrosis. Int. J. Mol. Sci. 22, 7606. doi: 10.3390/ijms22147606
Hayes, E., Pohl, K., McElvaney, N. G., Reeves, E. P. (2011). The cystic fibrosis neutrophil: a specialized yet potentially defective cell. Arch. Immunol. Ther. Exp. (Warsz) 59, 97–112. doi: 10.1007/s00005-011-0113-6
Hazlett, H. F., Hampton, T. H., Aridgides, D. S., Armstrong, D. A., Dessaint, J. A., Mellinger, D. L., et al. (2020). Altered iron metabolism in cystic fibrosis macrophages: the impact of CFTR modulators and implications for Pseudomonas aeruginosa survival. Sci. Rep. 10, 10935. doi: 10.1038/s41598-020-67729-5
Hernandez-Segura, A., Nehme, J., Demaria, M. (2018). Hallmarks of cellular senescence. Trends Cell Biol. 28, 436–453. doi: 10.1016/j.tcb.2018.02.001
Herranz, N., Gil, J. (2018). Mechanisms and functions of cellular senescence. J. Clin. Invest. 128, 1238–1246. doi: 10.1172/JCI95148
Hilliard, T. N., Regamey, N., Shute, J. K., Nicholson, A. G., Alton, E. W., Bush, A., et al. (2007). Airway remodelling in children with cystic fibrosis. Thorax 62, 1074–1080. doi: 10.1136/thx.2006.074641
Hisert, K. B., Heltshe, S. L., Pope, C., Jorth, P., Wu, X., Edwards, R. M., et al. (2017). Restoring cystic fibrosis transmembrane conductance regulator function reduces airway bacteria and inflammation in people with cystic fibrosis and chronic lung infections. Am. J. Respir. Crit. Care Med. 195, 1617–1628. doi: 10.1164/rccm.201609-1954OC
Hoggarth, A., Weaver, A., Pu, Q., Huang, T., Schettler, J., Chen, F., et al. (2019). Mechanistic research holds promise for bacterial vaccines and phage therapies for Pseudomonas aeruginosa. Drug Des. Devel. Ther. 13, 909–924. doi: 10.2147/DDDT.S189847
Houston, C. J., Alkhatib, A., Einarsson, G. G., Tunney, M. M., Taggart, C. C., Downey, D. G. (2024). Diminished airway host innate response in people with cystic fibrosis who experience frequent pulmonary exacerbations. Eur. Respir. J. 63, 2301228. doi: 10.1183/13993003.01228-2023
Hu, Y., Bojanowski, C. M., Britto, C. J., Wellems, D., Song, K., Scull, C., et al. (2024). Aberrant immune programming in neutrophils in cystic fibrosis. J Leukoc Biol. 115 (3), 420–434. doi: 10.1101/2023.01.22.23284619
Huang, X., Feng, Y., Xiong, G., Whyte, S., Duan, J., Yang, Y., et al. (2019). Caspase-11, a specific sensor for intracellular lipopolysaccharide recognition, mediates the non-canonical inflammatory pathway of pyroptosis. Cell Biosci. 9, 31. doi: 10.1186/s13578-019-0292-0
Iwanska, A., Trafny, E. A., Czopowicz, M., Augustynowicz-Kopec, E. (2023). Phenotypic and genotypic characteristics of Pseudomonas aeruginosa isolated from cystic fibrosis patients with chronic infections. Sci. Rep. 13, 11741. doi: 10.1038/s41598-023-39005-9
Jarosz-Griffiths, H. H., Gillgrass, L., Caley, L. R., Spoletini, G., Clifton, I. J., Etherington, C., et al. (2024). Anti-inflammatory effects of elexacaftor/tezacaftor/ivacaftor in adults with cystic fibrosis heterozygous for F508del. PloS One 19, e0304555. doi: 10.1371/journal.pone.0304555
Kanthakumar, K., Taylor, G., Tsang, K. W., Cundell, D. R., Rutman, A., Smith, S., et al. (1993). Mechanisms of action of Pseudomonas aeruginosa pyocyanin on human ciliary beat in vitro. Infect. Immun. 61, 2848–2853. doi: 10.1128/iai.61.7.2848-2853.1993
Kelk, D., Logan, J., Andersen, I., Gutierrez Cardenas, D., Bell, S. C., Wainwright, C. E., et al. (2022). Neutrophil respiratory burst activity is not exaggerated in cystic fibrosis. J. Cyst. Fibros. 21, 707–712. doi: 10.1016/j.jcf.2021.12.015
Kheir, S., Villeret, B., Garcia-Verdugo, I., Sallenave, J. M. (2022). IL-6-elafin genetically modified macrophages as a lung immunotherapeutic strategy against Pseudomonas aeruginosa infections. Mol. Ther. 30, 355–369. doi: 10.1016/j.ymthe.2021.08.007
Kim, K. K., Kugler, M. C., Wolters, P. J., Robillard, L., Galvez, M. G., Brumwell, A. N., et al. (2006). Alveolar epithelial cell mesenchymal transition develops in vivo during pulmonary fibrosis and is regulated by the extracellular matrix. Proc. Natl. Acad. Sci. U.S.A. 103, 13180–13185. doi: 10.1073/pnas.0605669103
Kim, J. S., McKinnis, V. S., Nawrocki, A., White, S. R. (1998). Stimulation of migration and wound repair of Guinea-pig airway epithelial cells in response to epidermal growth factor. Am. J. Respir. Cell Mol. Biol. 18, 66–74. doi: 10.1165/ajrcmb.18.1.2740
Kim, Y. J., Nomakuchi, T., Papaleonidopoulou, F., Yang, L., Zhang, Q., Krainer, A. R. (2022). Gene-specific nonsense-mediated mRNA decay targeting for cystic fibrosis therapy. Nat. Commun. 13, 2978. doi: 10.1038/s41467-022-30668-y
Kircheva, N., Dobrev, S., Petkova, V., Yocheva, L., Angelova, S., Dudev, T. (2024). In silico analysis of the ga(3+)/fe(3+) competition for binding the iron-scavenging siderophores of P. aeruginosa-implementation of three gallium-based complexes in the “Trojan horse” Antibacterial strategy. Biomolecules 14, 487. doi: 10.3390/biom14040487
Kohler, T., Luscher, A., Falconnet, L., Resch, G., McBride, R., Mai, Q. A., et al. (2023). Personalized aerosolised bacteriophage treatment of a chronic lung infection due to multidrug-resistant Pseudomonas aeruginosa. Nat. Commun. 14, 3629. doi: 10.1038/s41467-023-39370-z
Koller, D. Y., Gotz, M., Eichler, I., Urbanek, R. (1994). Eosinophilic activation in cystic fibrosis. Thorax 49, 496–499. doi: 10.1136/thx.49.5.496
Kotton, D. N., Morrisey, E. E. (2014). Lung regeneration: mechanisms, applications and emerging stem cell populations. Nat. Med. 20, 822–832. doi: 10.1038/nm.3642
Laabei, M., Jamieson, W. D., Lewis, S. E., Diggle, S. P., Jenkins, A. T. (2014). A new assay for rhamnolipid detection-important virulence factors of Pseudomonas aeruginosa. Appl. Microbiol. Biotechnol. 98, 7199–7209. doi: 10.1007/s00253-014-5904-3
Laselva, O., Conese, M. (2022). Elexacaftor/tezacaftor/ivacaftor accelerates wound repair in cystic fibrosis airway epithelium. J. Pers. Med. 12, 1577. doi: 10.3390/jpm12101577
Lashua, L. P., Melvin, J. A., Deslouches, B., Pilewski, J. M., Montelaro, R. C., Bomberger, J. M. (2016). Engineered cationic antimicrobial peptide (eCAP) prevents Pseudomonas aeruginosa biofilm growth on airway epithelial cells. J. Antimicrob. Chemother. 71, 2200–2207. doi: 10.1093/jac/dkw143
Laucirica, D. R., Schofield, C. J., McLean, S. A., Margaroli, C., Agudelo-Romero, P., Stick, S. M., et al. (2022). Pseudomonas aeruginosa modulates neutrophil granule exocytosis in an in vitro model of airway infection. Immunol. Cell Biol. 100, 352–370. doi: 10.1111/imcb.12547
Law, N., Logan, C., Yung, G., Furr, C. L., Lehman, S. M., Morales, S., et al. (2019). Successful adjunctive use of bacteriophage therapy for treatment of multidrug-resistant Pseudomonas aeruginosa infection in a cystic fibrosis patient. Infection 47, 665–668. doi: 10.1007/s15010-019-01319-0
Ledger, E. L., Smith, D. J., Teh, J. J., Wood, M. E., Whibley, P. E., Morrison, M., et al. (2024). Impact of CFTR modulation on Pseudomonas aeruginosa infection in people with cystic fibrosis. J. Infect. Dis. 230, e536–e547. doi: 10.1093/infdis/jiae051
Legrand, C., Gilles, C., Zahm, J. M., Polette, M., Buisson, A. C., Kaplan, H., et al. (1999). Airway epithelial cell migration dynamics. MMP-9 role in cell-extracellular matrix remodeling. J. Cell Biol. 146, 517–529. doi: 10.1083/jcb.146.2.517
Leslie, J., Millar, B. J., Del Carpio Pons, A., Burgoyne, R. A., Frost, J. D., Barksby, B. S., et al. (2020). FPR-1 is an important regulator of neutrophil recruitment and a tissue-specific driver of pulmonary fibrosis. JCI Insight 5, e125937. doi: 10.1172/jci.insight.125937
Liessi, N., Tomati, V., Capurro, V., Loberto, N., Garcia-Aloy, M., Franceschi, P., et al. (2023). The combination elexacaftor/tezacaftor/ivacaftor (ETI) modulates the de novo synthethic pathway of ceramides in a genotype-independent manner. J. Cyst. Fibros. 22, 680–682. doi: 10.1016/j.jcf.2023.04.012
Ling, K. M., Stick, S. M., Kicic, A. (2023). Pulmonary bacteriophage and cystic fibrosis airway mucus: friends or foes? Front. Med. (Lausanne) 10. doi: 10.3389/fmed.2023.1088494
Liu, H., Lazarus, S. C., Caughey, G. H., Fahy, J. V. (1999). Neutrophil elastase and elastase-rich cystic fibrosis sputum degranulate human eosinophils in vitro. Am. J. Physiol. 276, L28–L34. doi: 10.1152/ajplung.1999.276.1.L28
Lopes-Pacheco, M. (2019). CFTR modulators: the changing face of cystic fibrosis in the era of precision medicine. Front. Pharmacol. 10. doi: 10.3389/fphar.2019.01662
Lopez-Siles, M., Corral-Lugo, A., McConnell, M. J. (2021). Vaccines for multidrug resistant Gram negative bacteria: lessons from the past for guiding future success. FEMS Microbiol. Rev. 45, 054. doi: 10.1093/femsre/fuaa054
Losito, A. R., Raffaelli, F., Del Giacomo, P., Tumbarello, M. (2022). New drugs for the treatment of Pseudomonas aeruginosa infections with limited treatment options: A narrative review. Antibiotics (Basel) 11, 579. doi: 10.3390/antibiotics11050579
Lupas, D., Chou, F. Y., Hakani, M. A. A., Kuthiala, I., Srikrishnaraj, A., Li, X., et al. (2024). The clinical effectiveness of elexacaftor/tezacaftor/ivacaftor (ETI) for people with CF without a F508del variant: A systematic review and meta-analysis. J. Cystic Fibros. 23, 950–958. doi: 10.1016/j.jcf.2024.07.012
Lyczak, J. B., Cannon, C. L., Pier, G. B. (2002). Lung infections associated with cystic fibrosis. Clin. Microbiol. Rev. 15, 194–222. doi: 10.1128/CMR.15.2.194-222.2002
Mahenthiralingam, E., Campbell, M. E., Speert, D. P. (1994). Nonmotility and phagocytic resistance of Pseudomonas aeruginosa isolates from chronically colonized patients with cystic fibrosis. Infect. Immun. 62, 596–605. doi: 10.1128/iai.62.2.596-605.1994
Margaroli, C., Moncada-Giraldo, D., Gulick, D. A., Dobosh, B., Giacalone, V. D., Forrest, O. A., et al. (2021). Transcriptional firing represses bactericidal activity in cystic fibrosis airway neutrophils. Cell Rep. Med. 2, 100239. doi: 10.1016/j.xcrm.2021.100239
Mehta, A. M., Lee, I., Li, G., Jones, M. K., Hanson, L., Lonabaugh, K., et al. (2023). The impact of CFTR modulator triple therapy on type 2 inflammatory response in patients with cystic fibrosis. Allergy Asthma Clin. Immunol. 19, 66. doi: 10.1186/s13223-023-00822-2
Meschiari, M., Volpi, S., Faltoni, M., Dolci, G., Orlando, G., Franceschini, E., et al. (2021). Real-life experience with compassionate use of cefiderocol for difficult-to-treat resistant Pseudomonas aeruginosa (DTR-P) infections. JAC Antimicrob. Resist. 3, dlab188. doi: 10.1093/jacamr/dlab188
Mogayzel, P. J., Jr., Naureckas, E. T., Robinson, K. A., Brady, C., Guill, M., Lahiri, T., et al. (2014). Cystic Fibrosis Foundation pulmonary guideline. pharmacologic approaches to prevention and eradication of initial Pseudomonas aeruginosa infection. Ann. Am. Thorac. Soc. 11, 1640–1650. doi: 10.1513/AnnalsATS.201404-166OC
Mulcahy, L. R., Burns, J. L., Lory, S., Lewis, K. (2010). Emergence of Pseudomonas aeruginosa strains producing high levels of persister cells in patients with cystic fibrosis. J. Bacteriol. 192, 6191–6199. doi: 10.1128/JB.01651-09
Muller, M. (2006). Premature cellular senescence induced by pyocyanin, a redox-active Pseudomonas aeruginosa toxin. Free Radic. Biol. Med. 41, 1670–1677. doi: 10.1016/j.freeradbiomed.2006.09.004
Nakamura, H., Yoshimura, K., McElvaney, N. G., Crystal, R. G. (1992). Neutrophil elastase in respiratory epithelial lining fluid of individuals with cystic fibrosis induces interleukin-8 gene expression in a human bronchial epithelial cell line. J. Clin. Invest. 89, 1478–1484. doi: 10.1172/JCI115738
Neill, D. R., Saint, G. L., Bricio-Moreno, L., Fothergill, J. L., Southern, K. W., Winstanley, C., et al. (2014). The B lymphocyte differentiation factor (BAFF) is expressed in the airways of children with CF and in lungs of mice infected with Pseudomonas aeruginosa. PloS One 9, e95892. doi: 10.1371/journal.pone.0095892
O’Malley, Y. Q., Reszka, K. J., Rasmussen, G. T., Abdalla, M. Y., Denning, G. M., Britigan, B. E. (2003). The Pseudomonas secretory product pyocyanin inhibits catalase activity in human lung epithelial cells. Am. J. Physiol. Lung Cell Mol. Physiol. 285, L1077–L1086. doi: 10.1152/ajplung.00198.2003
Ojo, O. O., Ryu, M. H., Jha, A., Unruh, H., Halayko, A. J. (2015). High-mobility group box 1 promotes extracellular matrix synthesis and wound repair in human bronchial epithelial cells. Am. J. Physiol. Lung Cell Mol. Physiol. 309, L1354–L1366. doi: 10.1152/ajplung.00054.2015
Oz, H. H., Cheng, E. C., Di Pietro, C., Tebaldi, T., Biancon, G., Zeiss, C., et al. (2022). Recruited monocytes/macrophages drive pulmonary neutrophilic inflammation and irreversible lung tissue remodeling in cystic fibrosis. Cell Rep. 41, 111797. doi: 10.1016/j.celrep.2022.111797
Phipps, S., Ying, S., Wangoo, A., Ong, Y. E., Levi-Schaffer, F., Kay, A. B. (2002). The relationship between allergen-induced tissue eosinophilia and markers of repair and remodeling in human atopic skin. J. Immunol. 169, 4604–4612. doi: 10.4049/jimmunol.169.8.4604
Polverino, F., Lu, B., Quintero, J. R., Vargas, S. O., Patel, A. S., Owen, C. A., et al. (2019). CFTR regulates B cell activation and lymphoid follicle development. Respir. Res. 20, 133. doi: 10.1186/s12931-019-1103-1
Powell, L. C., Pritchard, M. F., Ferguson, E. L., Powell, K. A., Patel, S. U., Rye, P. D., et al. (2018). Targeted disruption of the extracellular polymeric network of Pseudomonas aeruginosa biofilms by alginate oligosaccharides. NPJ Biofilms Microbiomes 4, 13. doi: 10.1038/s41522-018-0056-3
Ramsey, D. M., Wozniak, D. J. (2005). Understanding the control of Pseudomonas aeruginosa alginate synthesis and the prospects for management of chronic infections in cystic fibrosis. Mol. Microbiol. 56, 309–322. doi: 10.1111/j.1365-2958.2005.04552.x
Rickard, K. A., Taylor, J., Rennard, S. I., Spurzem, J. R. (1993). Migration of bovine bronchial epithelial cells to extracellular matrix components. Am. J. Respir. Cell Mol. Biol. 8, 63–68. doi: 10.1165/ajrcmb/8.1.63
Rosenfeld, M., Ramsey, B. W., Gibson, R. L. (2003). Pseudomonas acquisition in young patients with cystic fibrosis: pathophysiology, diagnosis, and management. Curr. Opin. Pulm. Med. 9, 492–497. doi: 10.1097/00063198-200311000-00008
Rowe, S. M., Zuckerman, J. B., Dorgan, D., Lascano, J., McCoy, K., Jain, M., et al. (2023). Inhaled mRNA therapy for treatment of cystic fibrosis: Interim results of a randomized, double-blind, placebo-controlled phase 1/2 clinical study. J. Cyst. Fibros. 22, 656–664. doi: 10.1016/j.jcf.2023.04.008
Ruffin, M., Bilodeau, C., Maille, E., LaFayette, S. L., McKay, G. A., Trinh, N. T., et al. (2016). Quorum-sensing inhibition abrogates the deleterious impact of Pseudomonas aeruginosa on airway epithelial repair. FASEB J. 30, 3011–3025. doi: 10.1096/fj.201500166R
Ruffin, M., Brochiero, E. (2019). Repair process impairment by Pseudomonas aeruginosa in epithelial tissues: major features and potential therapeutic avenues. Front. Cell Infect. Microbiol. 9. doi: 10.3389/fcimb.2019.00182
Ruysseveldt, E., Martens, K., Steelant, B. (2021). Airway basal cells, protectors of epithelial walls in health and respiratory diseases. Front. Allergy 2. doi: 10.3389/falgy.2021.787128
Sainz-Mejias, M., Jurado-Martin, I., McClean, S. (2020). Understanding Pseudomonas aeruginosa-host interactions: the ongoing quest for an efficacious vaccine. Cells 9, 2617. doi: 10.3390/cells9122617
Sarkar, S., Barnaby, R., Nymon, A. B., Taatjes, D. J., Kelley, T. J., Stanton, B. A. (2024). Extracellular vesicles secreted by primary human bronchial epithelial cells reduce Pseudomonas aeruginosa burden and inflammation in cystic fibrosis mouse lung. Am. J. Physiol. Lung Cell Mol. Physiol. 326, L164–L174. doi: 10.1152/ajplung.00253.2023
Schmidt, H., Hopfer, L. M., Wohlgemuth, L., Knapp, C. L., Mohamed, A. O. K., Stukan, L., et al. (2023). Multimodal analysis of granulocytes, monocytes, and platelets in patients with cystic fibrosis before and after Elexacaftor-Tezacaftor-Ivacaftor treatment. Front. Immunol. 14. doi: 10.3389/fimmu.2023.1180282
Schoeniger, A., Fuhrmann, H., Schumann, J. (2016). LPS- or Pseudomonas aeruginosa-mediated activation of the macrophage TLR4 signaling cascade depends on membrane lipid composition. PeerJ 4, e1663. doi: 10.7717/peerj.1663
Schwarzer, C., Fischer, H., Machen, T. E. (2016). Chemotaxis and binding of Pseudomonas aeruginosa to scratch-wounded human cystic fibrosis airway epithelial cells. PloS One 11, e0150109. doi: 10.1371/journal.pone.0150109
Sharma, J., Du, M., Wong, E., Mutyam, V., Li, Y., Chen, J., et al. (2021). A small molecule that induces translational readthrough of CFTR nonsense mutations by eRF1 depletion. Nat. Commun. 12, 4358. doi: 10.1038/s41467-021-24575-x
Shaver, C. M., Hauser, A. R. (2004). Relative contributions of Pseudomonas aeruginosa ExoU, ExoS, and ExoT to virulence in the lung. Infect. Immun. 72, 6969–6977. doi: 10.1128/IAI.72.12.6969-6977.2004
Shteinberg, M., Haq, I. J., Polineni, D., Davies, J. C. (2021). Cystic fibrosis. Lancet 397, 2195–2211. doi: 10.1016/S0140-6736(20)32542-3
Shute, J., Marshall, L., Bodey, K., Bush, A. (2003). Growth factors in cystic fibrosis - when more is not enough. Paediatr. Respir. Rev. 4, 120–127. doi: 10.1016/s1526-0542(03)00028-9
Siddiqui, S., Bachert, C., Bjermer, L., Buchheit, K. M., Castro, M., Qin, Y., et al. (2023). Eosinophils and tissue remodeling: Relevance to airway disease. J. Allergy Clin. Immunol. 152, 841–857. doi: 10.1016/j.jaci.2023.06.005
Slimmen, L. J. M., Giacalone, V. D., Schofield, C., Horati, H., Manai, B., Estevao, S. C., et al. (2023). Airway macrophages display decreased expression of receptors mediating and regulating scavenging in early cystic fibrosis lung disease. Front. Immunol. 14. doi: 10.3389/fimmu.2023.1202009
Smith, S., Rowbotham, N. J. (2022). Inhaled anti-pseudomonal antibiotics for long-term therapy in cystic fibrosis. Cochrane Database Syst. Rev. 11, CD001021. doi: 10.1002/14651858.CD001021.pub4
Szabo, M. M., Foushee, S. E., McPheeters, C. M., O’Hagan, A. R., Ramirez, A. M., O’Reilly, E. A. (2024). Impact of elexacaftor/tezacaftor/ivacaftor on respiratory colonization in an adult cystic fibrosis clinic. Am. J. Med. Sci. 367, 337–342. doi: 10.1016/j.amjms.2024.02.001
Tamma, P. D., Souli, M., Billard, M., Campbell, J., Conrad, D., Ellison, D. W., et al. (2022). Safety and microbiological activity of phage therapy in persons with cystic fibrosis colonized with Pseudomonas aeruginosa: study protocol for a phase 1b/2, multicenter, randomized, double-blind, placebo-controlled trial. Trials 23, 1057. doi: 10.1186/s13063-022-07047-5
Thuenauer, R., Landi, A., Trefzer, A., Altmann, S., Wehrum, S., Eierhoff, T., et al. (2020). The Pseudomonas aeruginosa lectin lecB causes integrin internalization and inhibits epithelial wound healing. mBio 11, e03260–19. doi: 10.1128/mBio.03260-19
Tsang, K. W., Rutman, A., Tanaka, E., Lund, V., Dewar, A., Cole, P. J., et al. (1994). Interaction of Pseudomonas aeruginosa with human respiratory mucosa in vitro. Eur. Respir. J. 7, 1746–1753. doi: 10.1183/09031936.94.07101746
Useckaite, Z., Ward, M. P., Trappe, A., Reilly, R., Lennon, J., Davage, H., et al. (2020). Increased extracellular vesicles mediate inflammatory signalling in cystic fibrosis. Thorax 75, 449–458. doi: 10.1136/thoraxjnl-2019-214027
Van Winkle, L. S., Isaac, J. M., Plopper, C. G. (1997). Distribution of epidermal growth factor receptor and ligands during bronchiolar epithelial repair from naphthalene-induced Clara cell injury in the mouse. Am. J. Pathol. 151, 443–459.
Veltman, M., De Sanctis, J. B., Stolarczyk, M., Klymiuk, N., Bähr, A., Brouwer, R. W., et al. (2021). CFTR Correctors and Antioxidants Partially Normalize Lipid Imbalance but not Abnormal Basal Inflammatory Cytokine Profile in CF Bronchial Epithelial Cells. Front. Physiol. 12. doi: 10.3389/fphys.2021.619442
Virella-Lowell, I., Herlihy, J. D., Liu, B., Lopez, C., Cruz, P., Muller, C., et al. (2004). Effects of CFTR, interleukin-10, and Pseudomonas aeruginosa on gene expression profiles in a CF bronchial epithelial cell Line. Mol. Ther. 10, 562–573. doi: 10.1016/j.ymthe.2004.06.215
Wandling, E. N., Rhoads, K., Ohman, D. E., Heise, R. L. (2023). Electrosprayed mesenchymal stromal cell extracellular matrix nanoparticles accelerate cellular wound healing and reduce gram-negative bacterial growth. Pharmaceutics 15, 1277. doi: 10.3390/pharmaceutics15041277
Wang, S., Niroula, S., Hoffman, A., Khorrami, M., Khorrami, M., Yuan, F., et al. (2023). Inflammatory activity of epithelial stem cell variants from cystic fibrosis lungs is not resolved by CFTR modulators. Am. J. Respir. Crit. Care Med. 208, 930–943. doi: 10.1164/rccm.202305-0818OC
Wellems, D., Hu, Y., Jennings, S., Wang, G. (2023). Loss of CFTR function in macrophages alters the cell transcriptional program and delays lung resolution of inflammation. Front. Immunol. 14. doi: 10.3389/fimmu.2023.1242381
Westholter, D., Raspe, J., Uebner, H., Pipping, J., Schmitz, M., Strassburg, S., et al. (2023). Regulatory T cell enhancement in adults with cystic fibrosis receiving Elexacaftor/Tezacaftor/Ivacaftor therapy. Front. Immunol. 14. doi: 10.3389/fimmu.2023.1107437
Wilkinson, H. N., Hardman, M. J. (2020). Senescence in wound repair: emerging strategies to target chronic healing wounds. Front. Cell Dev. Biol. 8. doi: 10.3389/fcell.2020.00773
Wynn, T. A., Vannella, K. M. (2016). Macrophages in tissue repair, regeneration, and fibrosis. Immunity 44, 450–462. doi: 10.1016/j.immuni.2016.02.015
Yahav, D., Giske, C. G., Gramatniece, A., Abodakpi, H., Tam, V. H., Leibovici, L. (2020). New beta-lactam-beta-lactamase inhibitor combinations. Clin. Microbiol. Rev. 34, e00115–20. doi: 10.1128/CMR.00115-20
Ye, S. C., Desai, S., Karlsen, E., Kwong, E., Wilcox, P. G., Quon, B. S. (2022). Association between elevated peripheral blood eosinophil count and respiratory outcomes in adults with cystic fibrosis. J. Cyst. Fibros. 21, 1048–1052. doi: 10.1016/j.jcf.2022.03.009
Zemanick, E. T., Taylor-Cousar, J. L., Davies, J., Gibson, R. L., Mall, M. A., McKone, E. F., et al. (2021). A Phase 3 Open-Label Study of Elexacaftor/Tezacaftor/Ivacaftor in Children 6 through 11 Years of Age with Cystic Fibrosis and at Least One F508del Allele. Am. J. Respir. Crit. Care Med. 203, 1522–1532. doi: 10.1164/rccm.202102-0509OC
Zhang, Q., Kwon, W. Y., Vlkova, B., Rica, I., Kaczmarek, E., Park, J., et al. (2021). Direct airway instillation of neutrophils overcomes chemotactic deficits induced by injury. Shock 56, 119–124. doi: 10.1097/SHK.0000000000001691
Zhang, S., Shrestha, C. L., Robledo-Avila, F., Jaganathan, D., Wisniewski, B. L., Brown, N., et al. (2023). Cystic fibrosis macrophage function and clinical outcomes after elexacaftor/tezacaftor/ivacaftor. Eur. Respir. J. 61, 2102861. doi: 10.1183/13993003.02861-2021
Zhang, H., Yang, J., Cheng, J., Zeng, J., Ma, X., Lin, J. (2024). PQS and pyochelin in Pseudomonas aeruginosa share inner membrane transporters to mediate iron uptake. Microbiol. Spectr. 12, e0325623. doi: 10.1128/spectrum.03256-23
Keywords: wound repair, Pseudomonas aeruginosa, cystic fibrosis, bronchial epithelium, immune system, modulator therapy, elexacaftor/tezacaftor/ivacaftor(ETI)
Citation: Matthews EL, Hirsch MJ, Prokopczuk F, Jones LI, Martínez E, Barnes JW and Krick S (2025) Wound repair and immune function in the Pseudomonas infected CF lung: before and after highly effective modulator therapy. Front. Cell. Infect. Microbiol. 15:1566495. doi: 10.3389/fcimb.2025.1566495
Received: 24 January 2025; Accepted: 04 April 2025;
Published: 28 April 2025.
Edited by:
Washington LC Dos-Santos, Oswaldo Cruz Foundation (Fiocruz), BrazilReviewed by:
Massimo Conese, University of Foggia, ItalyTimothy James Wells, The University of Queensland, Australia
Copyright © 2025 Matthews, Hirsch, Prokopczuk, Jones, Martínez, Barnes and Krick. This is an open-access article distributed under the terms of the Creative Commons Attribution License (CC BY). The use, distribution or reproduction in other forums is permitted, provided the original author(s) and the copyright owner(s) are credited and that the original publication in this journal is cited, in accordance with accepted academic practice. No use, distribution or reproduction is permitted which does not comply with these terms.
*Correspondence: Stefanie Krick, c2tyaWNrQHVhYm1jLmVkdQ==
†These authors have contributed equally to this work and share first authorship