- 1Cell Cycle Laboratory, Butantan Institute, São Paulo, Brazil
- 2Department of Infection Biology, London School of Hygiene and Tropical Medicine, London, United Kingdom
Trypanosoma cruzi is a protozoan parasite that is the etiological agent of Chagas disease, which is endemic to Latin America with reported cases in non-endemic regions such as Europe, Asia, and Oceania due to migration. During its lifecycle, T. cruzi alternates between replicative and non-replicative infective lifeforms. Metacyclogenesis is the most studied transition of the T. cruzi life cycle, where replicative epimastigotes differentiate into infective metacyclic trypomastigotes inside the gut of the triatomine vector. This early-branching organism expresses a divergent pre-replication complex (pre-RC) where the only conserved component is the MCM2–7 protein family. Given the role of pre-RC components in cell cycle regulation, we investigated whether MCM expression and location could be involved in proliferation control in epimastigotes and during metacyclogenesis. Using CRISPR/Cas9, we tagged MCM subunits and tracked their expression and subcellular localization. Our findings reveal that MCM subunits are consistently expressed and localized to the nucleus throughout the epimastigote cell cycle, including in G1/G0-arrested cells. However, MCM subunits are degraded during metacyclogenesis as cells enter the G0 state, marking the transition to replication arrest. Therefore, epimastigotes arrested in G1/G0 can either maintain MCM complex expression and resume the cell cycle when conditions become favorable, or they can undergo metacyclogenesis, exiting the cell cycle and entering a G0 state, where MCM subunits are degraded as part of the replication repression mechanism.
Introduction
Cellular commitment to the cell cycle is strongly influenced by environmental conditions, such as nutrient availability. Under favorable conditions, cells pass through the G1 checkpoint, and progress irreversibly through the cell cycle, ultimately generating two daughter cells (Blagosklonny and Pardee, 2002; Johnson and Skotheim, 2013). Accurate DNA replication is essential during this process. DNA replication is initiated at specific chromosomal sites named origins of replication. During the cell cycle, two main events must occur at these origins: licensing and firing. The licensing process occurs in late mitosis and early G1 phases and is accomplished by assembling the pre-Replicative Complex (pre-RC). The Origin Recognition Complex (ORC1-6) recognizes and binds to the origins of replication and allows the subsequent loading of Cdt1 and Cdc6, which carries the Mini-Chromosome Maintenance complex (MCM2-7), completing pre-RC assembly (Stoeber et al., 2001). The MCM2–7 complex has a ring-shaped structure with helicase activity that is only activated after CDC45 and GINS recruitment, at the beginning of the S phase, when origins of replication are fired (Im et al., 2009). To guarantee that each origin of replication is fired only once per cell cycle, the pre-RC components are subject to degradation, detachment from chromatin, and export from the nucleus, thus avoiding DNA re-replication (Arias and Walter, 2007). In both budding yeast and fission yeast, all ORC subunits remain bound to chromatin throughout the cell cycle, however, Orc2 and Orc6 are phosphorylated and lose their capacity to load the MCM2–7 complex (Fujita et al., 1998; Diffley et al., 1995; Liang and Stillman, 1997; Lygerou and Nurse, 1999). In metazoans, pre-RC subunits are modified in a cell cycle-specific manner that controls chromatin affinity, cellular localization and/or stability (DePamphilis, 2005). For instance, Orc1 can be ubiquitinated and degraded during the S phase in HeLa cells (Méndez et al., 2002), or detached from chromatin and accumulated in the cytoplasm of CHO cells (Saha et al., 2006). Cdc6 is phosphorylated and degraded during the S phase in yeast (Drury et al., 2000; Jallepalli et al., 1997) and during mitosis in metazoans, but it can also be exported to the cytoplasm (Petersen et al., 2000; Kim and Kipreos, 2008). Cdt1 is exported from the nucleus in budding yeast during the S phase (Tanaka and Diffley, 2002) and is degraded in fission yeast and metazoans, also in S phase (Blow and Dutta, 2005; Machida et al., 2005). Additionally, in metazoans, Cdt1 can be inhibited by the binding protein geminin (Kim and Kipreos, 2007). In budding yeast, MCM2–7 is exported from the nucleus (Dalton and Whitbread, 1995), and in fission yeast and metazoans, MCM2–7 remains nuclear but is detached from chromatin at the end of DNA replication. Therefore, regulation of the pre-RC complex is conserved among eukaryotes, with distinct mechanisms observed across different systems.
When cells face unfavorable conditions, such as nutritional deprivation, the restriction point is not crossed and the cells exit the cell cycle (G0 phase). At this point, cells can either remain quiescent until the cell cycle resumes under favorable environmental conditions or they can terminally differentiate, entering a permanent non-proliferative state where the cell cycle does not resume (Sagot and Laporte, 2019) (Matson and Cook, 2017; Fiore et al., 2018). In both situations, the pre-RC are downregulated (Blow and Hodgson, 2002; Stoeber et al., 2001), but in terminally differentiated cells some pre-RC can be completely degraded (Carroll et al., 2018).
Trypanosoma cruzi is a protozoan parasite that causes Chagas’ disease, a potentially life-threatening illness endemic to Latin America with an estimated 6 to 7 million people infected (World Health Organization (WHO), 2024). During its life cycle, T. cruzi transitions from replicative to non-replicative lifeforms inside the mammalian host (amastigote and bloodstream trypomastigotes, respectively), and the triatomine insect vector (epimastigote and metacyclic trypomastigotes, respectively) (Martín-Escolano et al., 2022). In the vector, the process is called metacyclogenesis, and nutritional stress plays a crucial role in triggering the transition to infectious trypomastigotes (Melo et al., 2020). T. cruzi, Trypanosoma brucei and Leishmania spp. are the three main human-infectious trypanosomatids, and their pre-replication complexes differ from other eukaryotes. The ORC is divergent (Godoy et al., 2009; Tiengwe et al., 2012; Marques et al., 2016), no Cdt1 and Cdc6 equivalents have been found, but all components of the MCM2–7 complex are conserved (Dang and Li, 2011; Tiengwe et al., 2012; da Silva et al., 2017). Since MCM2–7 appeared to be the only pre-RC conserved component, we questioned whether MCM expression and location could be involved in proliferation control in T. cruzi. For this, we analyzed replicating and stationary epimastigotes, and those undergoing metacyclogenesis. We have previously found that subunit 7 of the MCM2–7 complex is only expressed in replicative lifeforms and is abolished in non-replicative trypomastigotes (metacyclic and bloodstream) (Calderano et al., 2014). Here, we investigated other MCM subunits and found that MCMs are expressed and localized inside the nucleus of replicating and stationary epimastigotes, and that degradation only occurs during metacyclogenesis, when cells are arrested in G0. While epimastigotes retain an ability to re-enter the cell cycle, MCM complex remains expressed, and cytoplasmic export is not involved as a regulatory mechanism. Degradation occurs only after differentiation into non-proliferative metacyclic trypomastigotes, and MCM complex expression is restored after differentiation to the replicative lifeform amastigotes within infected host cells.
Methodology
Cell culture
Epimastigotes: T. cruzi strains CL Brener and Dm28c, were maintained as epimastigotes in LIT medium (Camargo, 1964) supplemented with 10% fetal bovine serum (FBS), 60 µg/mL of penicillin, 100 µg/mL of streptomycin, and incubated at 28°C.
Hydroxyurea synchronization: Epimastigote cultures were synchronized with Hydroxyurea (HU) as described previously (Galanti et al., 1994). Briefly, epimastigote cultures were diluted in fresh medium to a final concentration of 3x106/mL. After 24h, HU was added at a final concentration of 20 mM and incubated for 24h at 28°C. Cells were washed three times with PBS and suspended in a fresh medium. Aliquots were collected at 0h, 4h, 6h, and 8h after HU removal, which were fixed for FACs analysis and total protein extraction.
Metacyclogenesis: The in vitro metacyclogenesis process was based on Contreras et al., 1985, with some changes. Epimastigote cultures in the stationary growth phase (~108 parasites/mL) were stressed in TAU medium (190 mM NaCl, 8 mM phosphate buffer pH 6.0, 17 mM KCl, 2 mM CaCl2, 2 mM MgCl2) at 5x108 parasites/mL for 2h at 28°C. Afterwards, they were diluted to 5x106 parasites/mL in TAU3aaG (TAU supplemented with 10 mM L-proline, 50 mM L-sodium glutamate, 2 mM L-sodium aspartate and 10 mM D-glucose) and incubated for 5 days at 28°C, in 5% CO2. To determine the percentage of differentiation, cells were fixed on a slide and stained with DAPI. Nucleus, kinetoplast, and cellular morphology were analyzed to determine epimastigotes, intermediates and fully differentiated trypomastigotes (Gonçalves et al., 2018).
In vitro infective cycle of T. cruzi: A total of 2.5 x 105 LLC-MK2 cells were seeded in a 175 cm2 flask with 40 mL of DMEM supplemented with 10% FBS and incubated at 37°C in 5% CO2. After 24h, 106 TCTs (Tissue Cultured Trypomastigotes) were added to the LLC-MK2 culture and incubated for 24h under the same conditions. The remaining TCTs were removed by washing with PBS and fresh medium added. After 5–6 days, TCTs were collected from the medium, following their egress from host cells.
CRISPR/Cas9
Gene editing was performed as described (Costa et al., 2018) targeted at the following genes in the CL Brener (MCM2: TcCLB.506933.40, MCM3: TcCLB.511109.100, MCM4: TcCLB.511127.140, MCM5: TcCLB.508647.140, MCM6: TcCLB.507527.30) and Dm28c strains (MCM6: C4B63_6g251, MCM7: C4B63_80g19). Briefly epimastigotes expressing the Cas9 enzyme and T7 RNA polymerase were transfected using program X-014 from Nucleofactor2b (Lonza) (Burkard et al., 2007) and the electroporation buffer (90 mM sodium phosphate, 5 mM potassium chloride, 0.15 mM calcium chloride, 50 mM Hepes, pH 7.2).
CRISPR/Cas9 transfections were carried out with two PCR products (~5 µg of each) representing single guide RNA (sgRNA) and donor DNA as described previously (Costa et al., 2018). Primers used to amplify donor DNA and sgRNA are listed in Tables 1 and 2. Donor DNA to insert 3 copies of mNeonGreen, 6 copies of Myc and blasticidin resistance gene were amplified from pPOTv6-blast-3Myc::3mNG::3Myc plasmid (Paterou et al., 2023). Donor DNA to insert 3 copies of Myc and the puromycin resistance gene were amplified from pMOTag (Lander et al., 2017).
Epimastigotes cloning
Epimastigotes were counted in a Neubauer chamber and diluted to a final concentration of 1 parasite/mL of mixed LIT medium (50% fresh medium and 50% conditioned medium), supplemented with 10% FBS and resistance drugs (5 µg/mL puromycin, 10 µg/mL blasticidin, and 100 µg/mL G418). Then, 200 µL aliquots of this diluted culture were applied to each well of a 96-well plate and incubated at 28°C. The protein expression of each clone was confirmed by western blotting. No differences in protein expression levels were observed among clones.
Conditioned medium
Epimastigotes were diluted to 3x106 parasites/mL in fresh LIT medium supplemented with 10% FBS. After 48h incubation at 28°C, the cell culture was centrifuged, and the medium was collected and filtered to eliminate any remaining parasites.
Immunofluorescence
Extracellular life cycle stages: For immunofluorescence assays, 3x106 cells were applied to poly-D-lysine-coated glass slides, incubated at room temperature for 5 minutes and washed with PBS. Then cells were fixed with 2% paraformaldehyde for 10 minutes, washed twice with PBS and permeabilized with PBS/0.3% Triton X-100 for 5 minutes. After washing twice with PBS, the cells were blocked for 1h in PBS containing 3% bovine serum albumin, 1% bovine gelatin and 50% FBS. The cells were then washed twice with PBS and incubated at room temperature for 4h in PBS/3% BSA with mouse anti-Myc (1:1000) (Myc-Tag 9B11 Mouse mAB, Cell Signalling). The cells were then washed 5x with PBS and incubated for 1h at room temperature with PBS/BSA containing anti-mouse 488 plus (1:1000) (Thermo Fisher Scientific). After several washes with PBS, the slide was mounted using Vecta-Shield with DAPI (VectorLabs). Images were acquired using a 100× 1.35NA lens and cell F software in an Olympus BX51 microscope (Tokyo, Japan). Brightness and contrast were adjusted using Photoshop. Raw images of control and edited cell lines are presented in Supplementary Figure 2.
EdU staining: Cells were incubated for 30 minutes with 5-ethynyl-2′-deoxyuridine (EdU) at a final concentration of 100 µM, washed 3x with PBS, and processed as above for microscopy, as previously described. EdU detection followed the manufacturer’s instructions (Click-iT™ EdU Cell Proliferation Kit for Imaging, Alexa Fluor™ 647 dye-Thermo Fisher Scientific). Images were acquired through a z-series of 0.2 μm using a 100× 1.35 NA lens and cell R software in an Olympus IX81 microscope. Deconvolution was performed using AutoQuant X software.
Intracellular amastigotes: LLC-MK2 cells (5 × 10³) were seeded on 13 mm round coverslips in a 12-well plate using DMEM supplemented with 10% FBS. The cells were incubated for 24h at 37°C with 5% CO2. Cells were infected with Dm28c strain trypomastigotes at an MOI of 1:10. After 24h, the medium was removed, and the cells were washed with PBS to eliminate remaining parasites. Fresh DMEM with 10% FBS was then added to the wells. Following a 48h incubation period, the cells underwent immunofluorescence staining. The steps for fixation, permeabilization, blocking, and antibody incubation were performed as previously described.
Western blotting
RIPA Lysis and Extraction Buffer (Thermo Fisher Scientific) supplemented with a protease inhibitor cocktail (Pierce™ Protease Inhibitor Tablets, EDTA free-Thermo Fisher Scientific) and a phosphatase inhibitor cocktail (Halt™ Phosphatase Inhibitor Cocktail-Thermo Ficher Scientific) were used to extract total proteins, using 20 µL of RIPA buffer for each 107 cells. Total protein extract concentration was determined using a Pierce™ BCA Protein assay kit (Thermo Fisher Scientific).
Western blotting band quantification using Photoshop
Western blotting images were acquired using a UVITEC system (Cambridge), employing automatic exposure times to prevent band saturation. Subsequently, images were analyzed using Photoshop software to quantify band intensity. Signal (anti-Myc) and control (anti-Flag) images were juxtaposed in the same JPEG file, enabling uniform band quantification under consistent parameters. To ensure accurate measurement, the rectangle selection tool was utilized to delineate the band area. A consistent selection size was applied across all bands under analysis, encompassing both the anti-Myc signal and control bands. Additionally, a nearby region of each band was selected as the background signal. The intensity of each selected area (anti-Myc, anti-Flag, and background) was quantified to facilitate the calculation of the relative expression levels of MCM proteins. The background intensity was subtracted from the intensities of the anti-Myc and anti-Flag signals to correct for non-specific signal contributions. The corrected anti-Myc intensity was normalized to the corrected anti-Flag intensity by dividing the anti-Myc intensity by the anti-Flag intensity. This step accounts for any variability in the total protein load or other experimental inconsistencies. The relative expression levels were determined by dividing the normalized anti-Myc/anti-Flag ratio for each sample by the normalized anti-Myc/anti-Flag ratio of a reference sample (e.g., a control or baseline sample). This provided a relative measure of MCM expression across different samples. Data visualization and statistical analyses were performed using GraphPad Prism software. Graphs were created to illustrate the results, and appropriate statistical tests were applied to assess the significance of the differences observed.
Flow cytometry
Propidium iodide: 107 cells were pelleted, washed twice with PBS and fixed by resuspending in 1 mL of cold 70% ethanol/30% PBS and kept at –20°C for at least 4h. Fixed cells were pelleted, washed twice with PBS and resuspended in 500 µL PBS containing RNAse A (Invitrogen) and propidium iodide (100 µM final concentration) (Thermo Fisher Scientific). The cells were incubated at 37°C for 30 minutes and analyzed by flow cytometry using an Attune NxT (Life Technologies).
EdU incorporation: after 30 minutes incubation with 100 µM EdU (final concentration) (Thermo Fisher Scientific), 107 cells were pelleted, washed 3x with 1 mL of PBS. The cell pellet was then fixed and EdU detection performed as previously described and analyzed using an Attune NxT flow cytometer.
Data acquired by the Attune NxT were analyzed by FlowJo software. The cell cycle stages were determined by the cell cycle modeling tool for TCTs histograms and by gate selection for synchronization.
Results
MCM6-Myc and MCM7-Myc expression is constitutive throughout epimastigote cell cycle
We utilized the CRISPR/Cas9 system to tag MCM proteins in T. cruzi CL Brener and DM28c epimastigotes. Specifically, we inserted three copies of mNeonGreen and six copies of a c-Myc epitope at the 3’ end of the MCM 2, 3, 4 and 6 genes in the CL Brener strain (Figure 1A), and three copies of the c-Myc tag at the 3’end of the MCM6 and 7 genes (Figure 1B) in Dm28c. The expressed tagged proteins were detectable in all cell lines (Figures 1A, B).
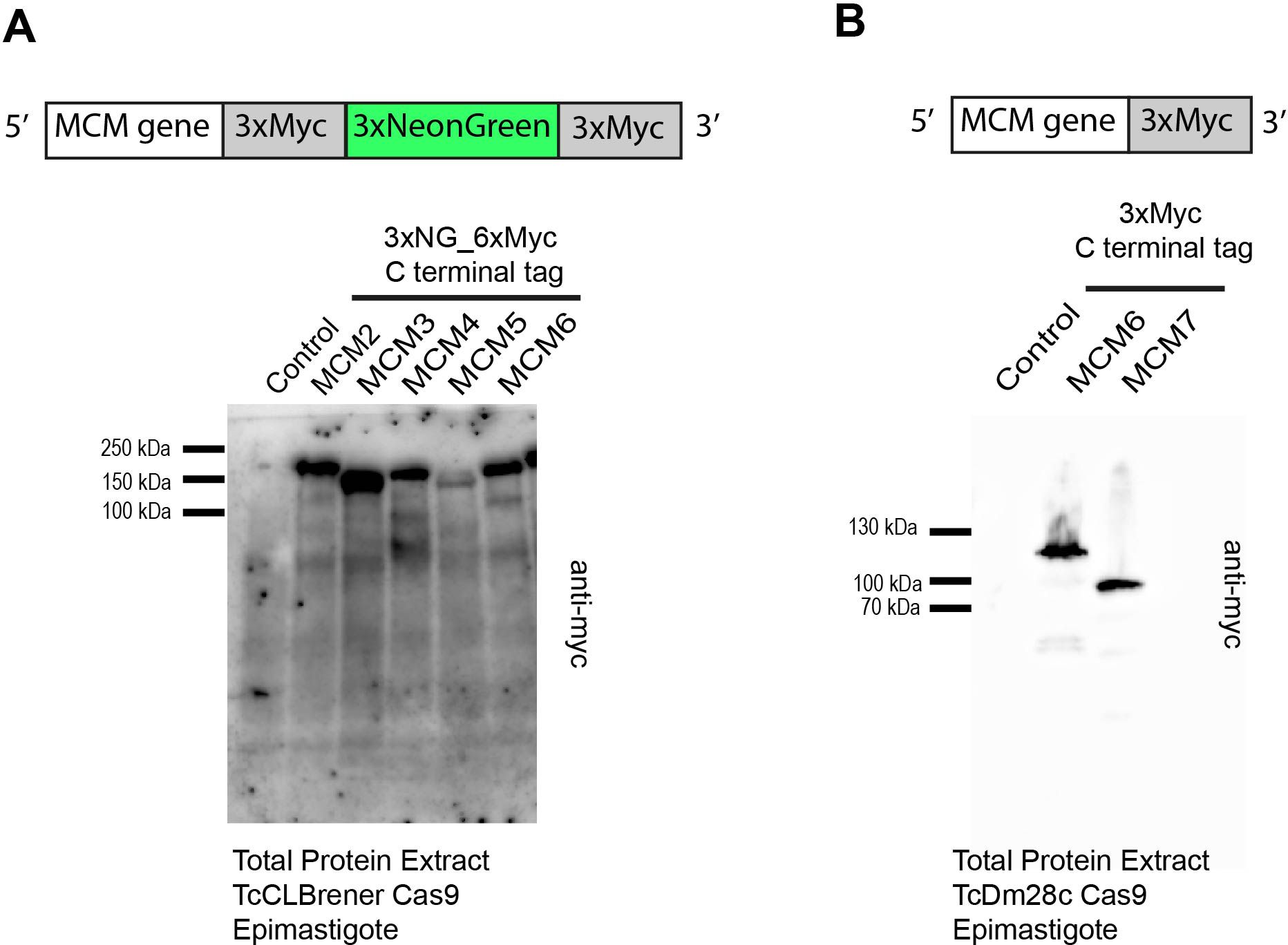
Figure 1. T. cruzi epimastigote cell lines modified by CRISPR/Cas9. (A) Western blot analysis of total protein from T. cruzi epimastigotes (CL Brener strain). As indicated in the illustration, three copies of mNeonGreen and six copies of the c-Myc epitope were inserted into the 3’ end of the MCM 2, 3, 4, 5, 6 genes. An anti-Myc antibody was used to identify the tagged proteins in total extracts. Protein extract of epimastigotes (TcCLBrener-Cas9) was used as a control. Expected band masses: ~196 kDa for MCM2-Myc_NG; ~178 kDa for MCM3-Myc_NG; ~184 kDa for MCM4-Myc_NG; ~172 kDa for MCM5-Myc_NG; ~169 kDa for MCM6-Myc_NG. (B) Western blot analysis of total protein from T. cruzi epimastigote (Dm28c strain) cell lines. As indicated by the illustration, three copies of the Myc epitope were inserted into the 3’ end of the MCM6 and MCM7 genes. An anti-Myc antibody was used to identify the tagged proteins in total extracts. Protein extract of epimastigotes (Dm28c-Cas9) was used as a control. Expected band masses: ~103 kDa for MCM6-Myc and ~86 kDa for MCM7-Myc.
To determine the expression levels of MCM6 and MCM7 throughout the cell cycle, we synchronized T. cruzi Dm28c strain epimastigotes with hydroxyurea (Figure 2A). We obtained cell populations enriched at each cell cycle phase with the MCM6-Myc (Figure 2B) and MCM7-Myc modified cell lines (Figure 2E): 0h enriched for G1 and S phases, 4h enriched for S phase, 6h enriched for S and G2 phases, and 8h enriched for G2 phase. Whole protein extracts from these synchronized cells were used to assess the expression levels of MCM6-Myc (Figure 2C) and MCM7-Myc (Figure 2F) by western blotting, with Flag-tagged Cas9 serving as the loading control. Quantification of band intensity from western blots of three independent replicates revealed that MCM6-Myc (Figure 2D) and MCM7-Myc (Figure 2G) are constitutively expressed throughout the epimastigote cell cycle with no significant variation among the phases.
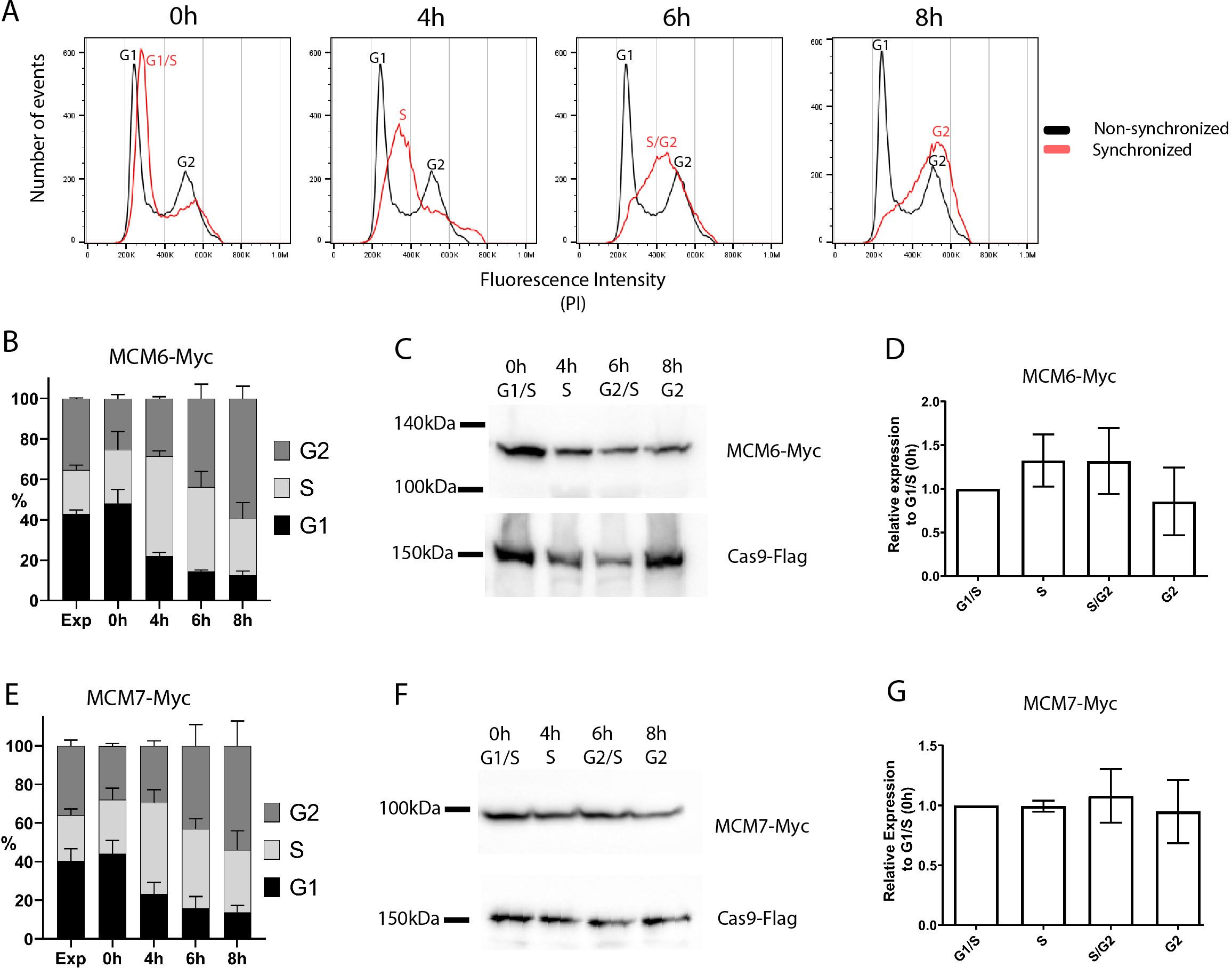
Figure 2. Cell cycle synchronization by hydroxyurea. T. cruzi epimastigotes from the Dm28c strain (MCM6-Myc and MCM7-Myc) were synchronized by hydroxyurea (HU) (see Methods for further details). (A) DNA content was measured by propidium iodide (PI) fluorescence intensity, using Flow Cytometry, and the histograms of number of events x PI intensity were analyzed in FlowJo software. The black line represents the cell cycle distribution of a non-synchronous population, and the two peaks for G1 and G2 populations are indicated (black letters). The red line represents the synchronous population at different time points after HU release (0h, 4h, 6h, 8h), and the cell cycle-enriched populations are marked on the peak histogram in red letters. (B, E) The histograms of number of events x PI intensity were used to determine the percentage of cells in each cell cycle stage (G1, S, G2) by FlowJo software, The graphs show this distribution at each time point of the synchronized and non-synchronized populations (B for MCM6-Myc cell line, E for MCM7-Myc cell line). (C, F) Western blot analysis of the synchronized cell population. Anti-Myc was used to detect (C) MCM6-Myc and (F) MCM7-Myc and anti-Flag were used to detect the tagged Cas9 enzyme (input control). (D, G) Bands from the blot were quantified using Photoshop software, and the relative expression compared to the 0h point is represented in the graphs: (D) MCM6-Myc and (G) MCM7-Myc.
MCM subunits are nuclear localized throughout the epimastigote cell cycle
We next investigated whether the cellular location of MCM subunits presents a different profile through the cell cycle, by performing immunofluorescence with clonal populations of the MCM-Myc tagged CL Brener (Supplementary Figure 1) and Dm28c (Figure 3A) strains. Using morphological features such as the number of nuclei, kinetoplasts and flagella (Elias et al., 2007), we were able to determine the epimastigote cell cycle phase and location of MCM subunits. MCM 2, 3, 4, 6 and 7 in CL Brener strain (Supplementary Figure 1) and MCM6 (Figure 3A) and 7 (Figure 3B) in Dm28c strain were nuclear in all cell cycle phases, and no specific cytoplasmatic signal was identified. We also used the incorporation of the thymidine analogue EdU, to identify cells in S phase. Analysis of Z-stack images allowed us to reveal colocalization of MCM6-Myc, MCM7-Myc and EdU - regions visualized as yellow on merged images of anti-Myc (green) and EdU (red) staining (Figures 3C, D). As the MCM complex are part of the replisome (Polasek-Sedlackova et al., 2022), colocalization would be expected if MCM6 and MCM7 have a role in the DNA replication process.
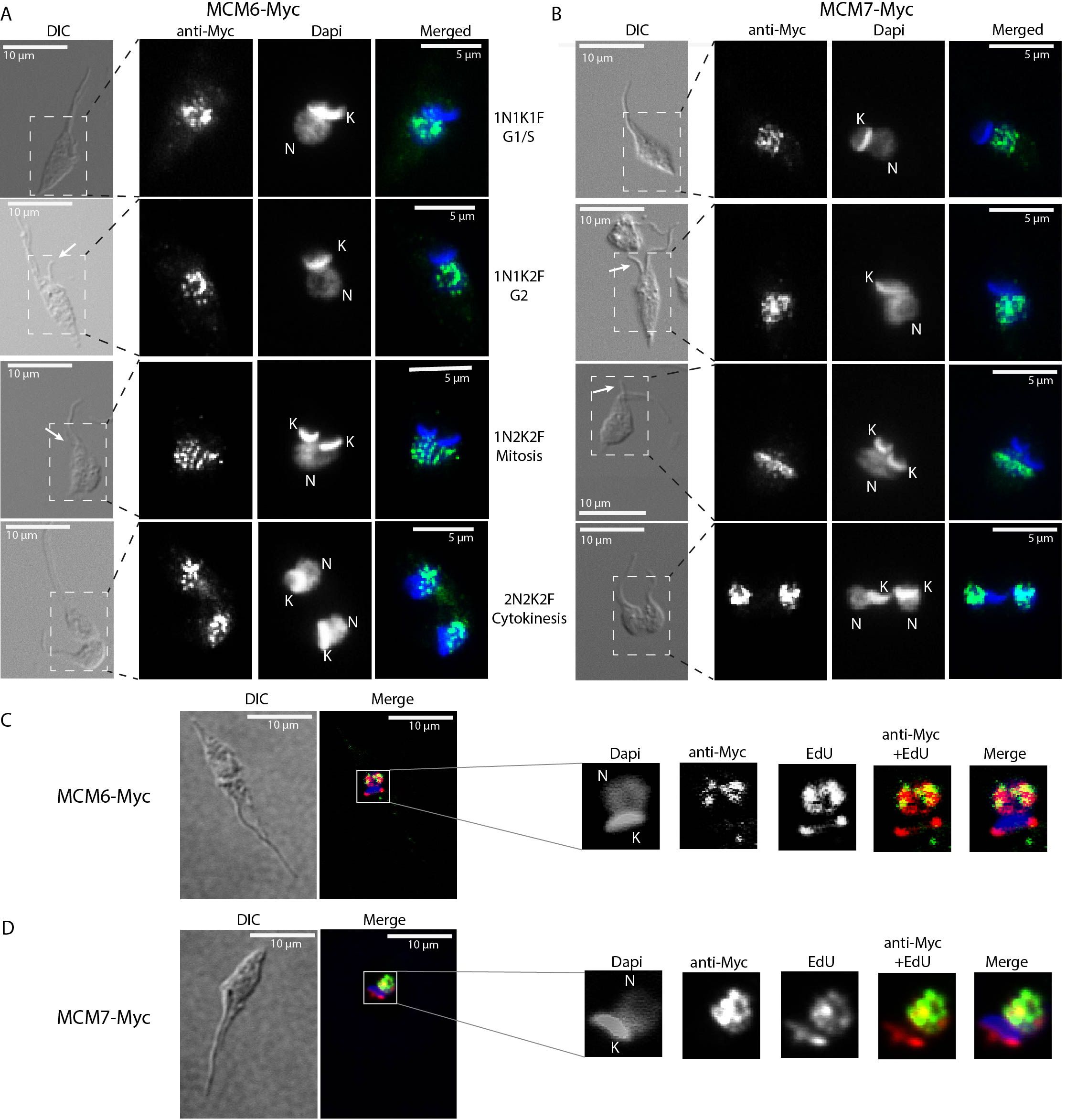
Figure 3. Immunofluorescence of MCM-tagged T. cruzi epimastigotes (Dm28c strain) using anti-Myc antibody. Epimastigotes from (A, C) the MCM6-Myc cell line (Dm28c strain) and (B, D) the MCM7-Myc cell line (Dm28c strain) were subjected to immunofluorescence using anti-Myc antibody (see Methods for further details). (A, B) Different cell cycle phases are represented and were identified by the N (Nucleus), K (Kinetoplast) and F (Flagellum) numbers. The white arrows indicate emerging new flagella. The dashed rectangle in the DIC images highlight the regions shown in the corresponding fluorescent images (ani-Myc, DAPi and merged). (C, D) Epimastigotes in S phase of the cell cycle were identified by EdU incorporation (red). The yellow areas are sites of colocalization of (C) MCM6-Myc and EdU, and (D) MCM7-Myc and EdU. (A, B) Images were acquired on a Microscope Olympus BX51. (C, D) Images were acquired on a Microscope Olympus IX81 and are a layer from a Z-stack acquisition, deconvoluted by software Auto Quant. White bar scale (10 µm), Green (anti-Myc signal, yellow when on a red background), Blue (DAPI signal), Red (EdU signal), DIC (Differential Interference Contrast).
MCM6-Myc and MCM7-Myc cells are arrested in G1 phase during metacyclogenesis
During the T. cruzi life cycle, replicative epimastigotes differentiate into metacyclic trypomastigotes, which are infective but non-replicative. This differentiation process, known as metacyclogenesis, occurs within the vector gut (Ferreira et al., 2023) and can also be induced in vitro (Contreras et al., 1985) (Figure 4A). We induced in vitro metacyclogenesis and collected samples at four-time points to analyze the cell cycle and the expression of MCM6-Myc and MCM7-Myc. Epimastigotes were cultured until they reached stationary growth phase (point 2 in Figure 4A), and then subjected to stress in TAU medium for 2h (point 3 in Figure 4A). Subsequently, they were incubated for 5 days in TAU3aaG medium (point 4 in Figure 4A) to allow metacyclogenesis. As a control, epimastigotes from the stationary phase were diluted in fresh medium and collected 24h later, representing replicative epimastigotes recovered from a non-replicative state. At the end of metacyclogenesis, we quantified the percentage of cells that had completely differentiated into metacyclic trypomastigotes, as well as intermediate forms and non-differentiated epimastigotes (Figure 4B). Cellular morphology, including the shape and positioning of the nucleus and kinetoplast, was used to identify epimastigotes, intermediates, and metacyclic trypomastigotes (Gonçalves et al., 2018). For the MCM6-Myc cell line, 37.5% of the cells had completely differentiated into metacyclic trypomastigotes, while for the MCM7-Myc cell line, 32.7% of the cells were metacyclic trypomastigotes (Figure 4B).
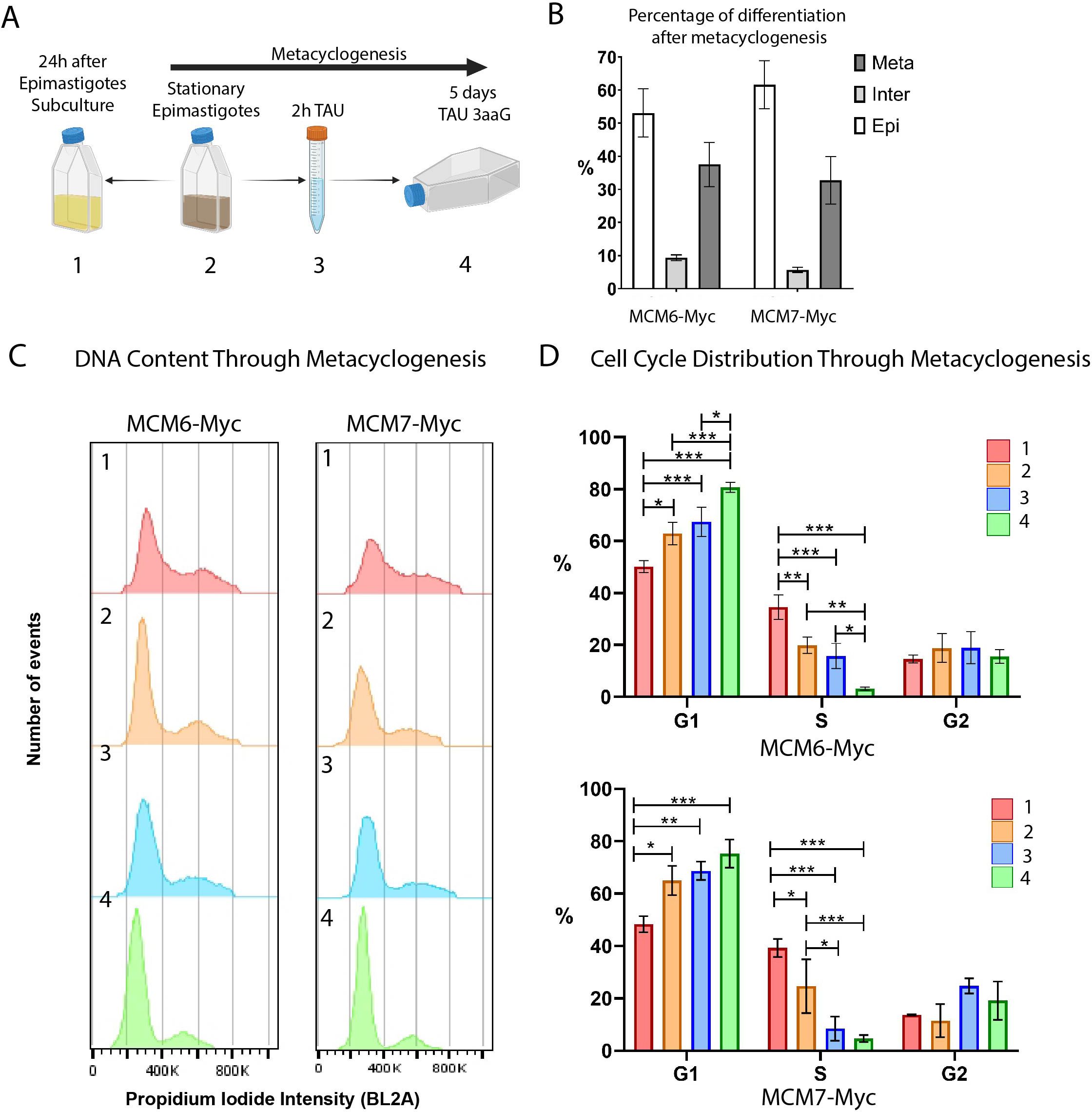
Figure 4. Metacyclogenesis of MCM6-Myc and MCM7-Myc cell lines (Dm28c strain). (A) Schematic representation of the metacyclogenesis process in vitro. (1) Epimastigotes in the stationary phase of growth were diluted in fresh medium and collected 24h later, serving as the control for replicating epimastigotes. (2) Epimastigotes in the stationary phase were (3) stressed for 2 h in TAU medium and (4) differentiated over 5 days in TAU 3aaG medium (see Methods for further details). (B) After metacyclogenesis, cells were fixed on slides and stained with DAPI. Epimastigotes, intermediate forms, and metacyclic trypomastigotes were counted. The bar chart shows the percentage of each cell type, averaged from three replicates. (C) The DNA content of each sample collected during metacyclogenesis was analyzed by flow cytometry. The histograms represent the number of events versus propidium iodide intensity (BL2A) for each metacyclogenesis time point. (D) The histograms from (C) were analyzed using FlowJo software, and cell cycle phases were determined by Dean-Jett-Fox cell cycle modeling. The bar charts show the percentage of parasites in each cell cycle phase throughout metacyclogenesis, averaged from three replicates. A two-way ANOVA was applied, and significance is indicated by * (p ≤ 0.01), ** (p ≤ 0.001), and *** (p ≤ 0.0001).
Using flow cytometry, we analyzed the DNA content by propidium iodide (PI) intensity for each time point during metacyclogenesis (Figure 4C) and quantified the cell cycle distribution (Figure 4D).
In both cell lines, MCM6-Myc and MCM7-Myc, we observed that the percentage of cells in G1 phase increased as epimastigotes transitioned from the replicative phase (point 1) to the stationary growth phase (point 2) (Santos et al., 2018), reaching a maximum at the end of metacyclogenesis (Figure 4D). In the MCM6-Myc cell line, the percentage of cells in G1 phase in replicative epimastigotes (point 1) was 50.2%, compared to 62.9% in epimastigotes in the stationary growth phase (point 2). At the end of metacyclogenesis, (point 4) the percentage of cells in G1 was 80.7% (Figure 4D, upper graph). There was a similar trend with MCM7-Myc cell line, with 48.3% in G1 phase in replicative epimastigotes (point 1), 65% in epimastigotes in the stationary growth phase (point 2), and 75.3% at the end of metacyclogenesis (point 4 in Figure 4D, lower graph).
Simultaneously, in both cell lines, the percentage of parasites in S phase gradually decreased during the transition from replicative epimastigotes to non-replicative metacyclics. In the MCM6-Myc cell line, the percentage of epimastigotes in S phase (point 1) decreased from 34.6%, to 19.9% in the stationary growth phase (point 2). At the end of metacyclogenesis (point 4), the percentage of parasites in S phase was 3.1% (Figure 4D, upper graph). In the MCM7-Myc cell line, the percentage of epimastigotes in S phase (point 1) was 39.2%, compared to 24.6% in the stationary growth phase (point 2). At the end of metacyclogenesis (point 4), the percentage of cells in the S phase was 4.6% (Figure 4D, lower graph). Finally, in the tagged lines, there was no significant difference in the percentage of parasites in G2 at the 4 points collected during metacyclogenesis.
Expression levels of MCM6-Myc and MCM7-Myc decrease through metacyclogenesis
Given that cells exiting the cell cycle exhibit decreased levels of MCM subunits (Madine et al., 2000) and that we have previously observed that metacyclic trypomastigotes do not express MCM7 (Calderano et al., 2014), we investigated the expression profiles of MCM6-Myc and MCM7-Myc at four distinct points during metacyclogenesis (Figure 5A). Our observations revealed a decrease in expression levels in both parasite lines, from replicative epimastigotes (point 1) to parasites post-metacyclogenesis (point 4, Figure 5A).
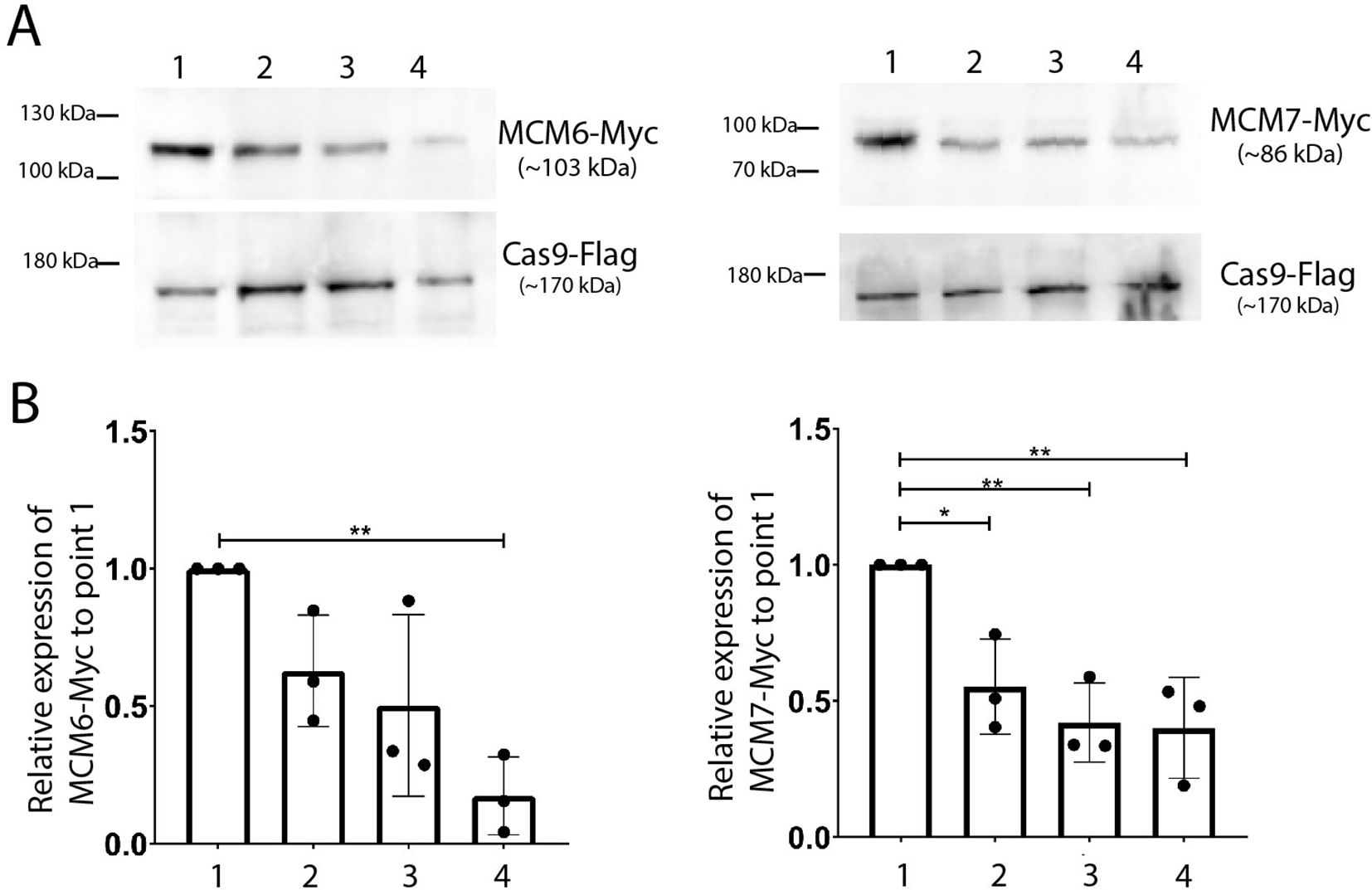
Figure 5. Western blotting analysis of MCM6-Myc and MCM7-Myc during metacyclogenesis of T. cruzi (Dm28c strain). (A) Whole protein extracts from each metacyclogenesis point were subjected to western blot analysis. An anti-Myc antibody was used to identify MCM6-Myc and MCM7-Myc, while an anti-Flag antibody was used to detect Cas9 expression, serving as an input control. (B) The bands detected in (A) were quantified using Photoshop software. The bar chart shows the expression levels relative to replicative epimastigotes (point 1), averaged from 3 replicates. One-way ANOVA test was applied, and significance is indicated by * (p<0.05) and ** (p<0.01).
To quantify these changes, we measured band intensity from three independent Western blot replicates and analyzed the MCM expression levels at each point (points 1 to 4, Figure 5A) relative to the levels in replicative epimastigotes (point 1, Figure 5A). In the MCM6-Myc cell line, the expression levels in stationary epimastigotes (point 2), and in epimastigotes after 2h in TAU (point 3), followed a reducing trend compared to those in replicative epimastigotes (Figure 5B). The reduction in expression levels was more pronounced and statistically significant in parasites post-metacyclogenesis (point 4). In the MCM7-Myc cell line, a similar pattern was observed. The decreased expression levels from replicative epimastigotes (point 1, right graph, Figure 5B) to stationary epimastigotes (point 2), 2h TAU-stressed epimastigotes (point 3), and parasites post-metacyclogenesis (point 4) were statistically significant across all points (Figure 5B).
MCM6-Myc and MCM7-Myc are nuclear-localized in amastigotes and stationary-epimastigotes, but are not expressed in metacyclic or tissue-cultured trypomastigotes
MCM subunits are nuclear-localized throughout the entire cell cycle in replicative epimastigotes (Figure 3). Given that expression of MCM6-Myc and MCM7-Myc diminishes in stationary epimastigotes, we investigated the impact on their cellular location. To confirm that epimastigotes in the “stationary growth phase” are non-replicating, we assessed their proliferation capacity using EdU incorporation, followed by analysis via flow cytometry (Figures 6A, B). The results showed that replicating epimastigotes incorporated EdU, whereas stationary epimastigotes did not (Figures 6A, B). When we analyzed the cellular location of MCM6-Myc and MCM7-Myc, we found that they had the same nuclear pattern as in stationary-epimastigotes (Figures 6C, D). Additionally, after metacyclogenesis process, in epimastigotes that did not differentiate, MCM6-Myc and MCM7-Myc were also nuclear-localized (Supplementary Figure 3). However, no specific signal was detectable in fully differentiated metacyclic trypomastigotes (Figure 7A).
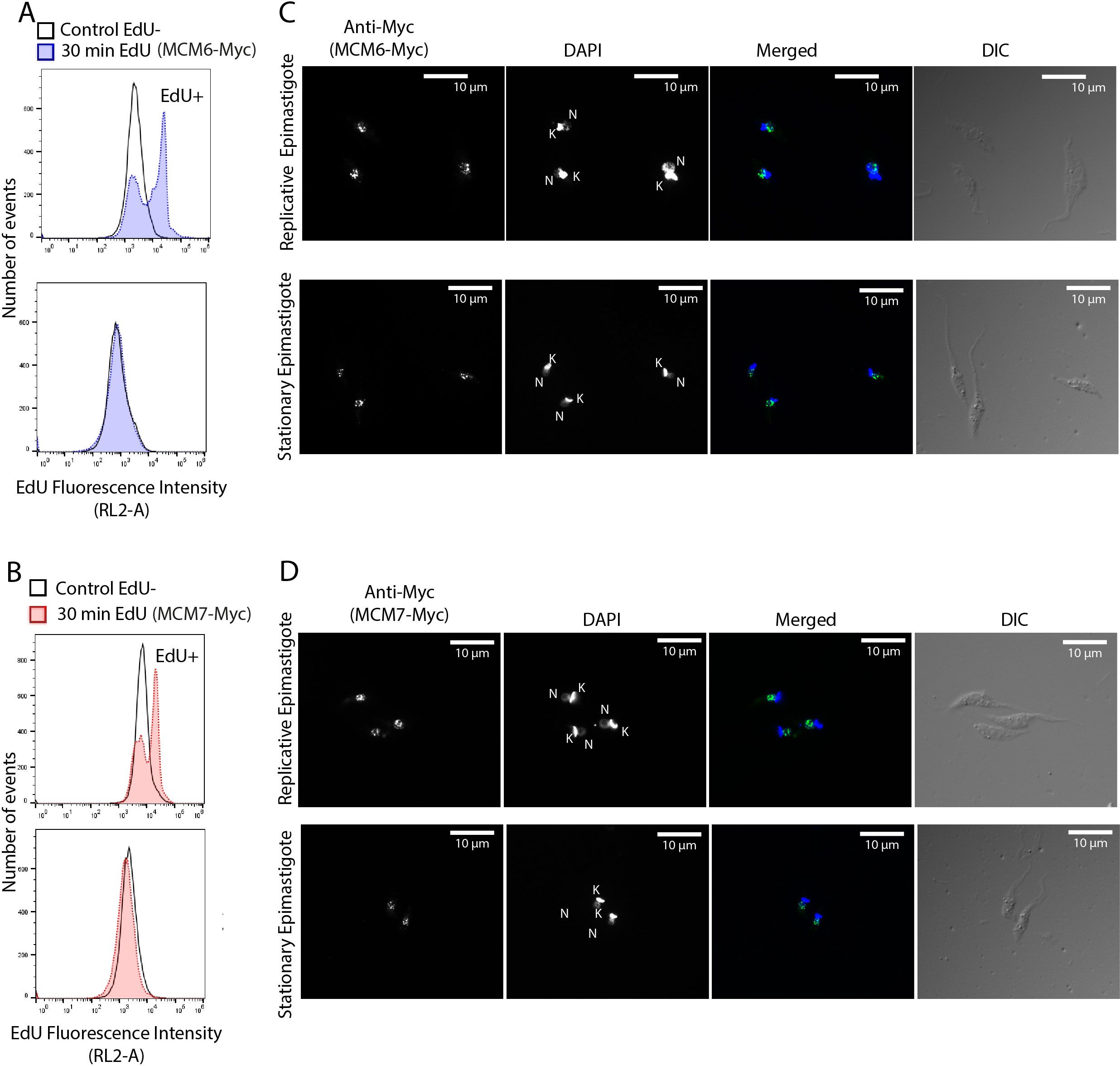
Figure 6. Cellular localization of MCM6-Myc and MCM7-Myc in replicative and non-replicative T. cruzi epimastigotes (Dm28c strain). T. cruzi epimastigotes in log phase (replicative) and stationary phase (non-replicative) were analyzed to determine MCM localization (A, B) Cells were incubated with EdU for 30 minutes (see Methods for further details) and analyzed by flow cytometry. The histograms show the number of cells versus EdU fluorescence intensity. Blank histograms represent the negative control, where parasites (MCM6-Myc cell line and MCM7-Myc cell line) were not incubated with EdU. In (A), the blue histograms represent the MCM6-Myc line incubated with EdU. In (B), the red histograms represent the MCM7-Myc line incubated with EdU. (C, D) Immunofluorescence of epimastigotes using an anti-Myc antibody to detect (C) MCM6-Myc and (D) MCM7-Myc. White bar scale represents 10 µm. N, nucleus; K, kinetoplast; green, anti-Myc signal; blue, DAPI (DNA); DIC, Differential Interference Contrast.
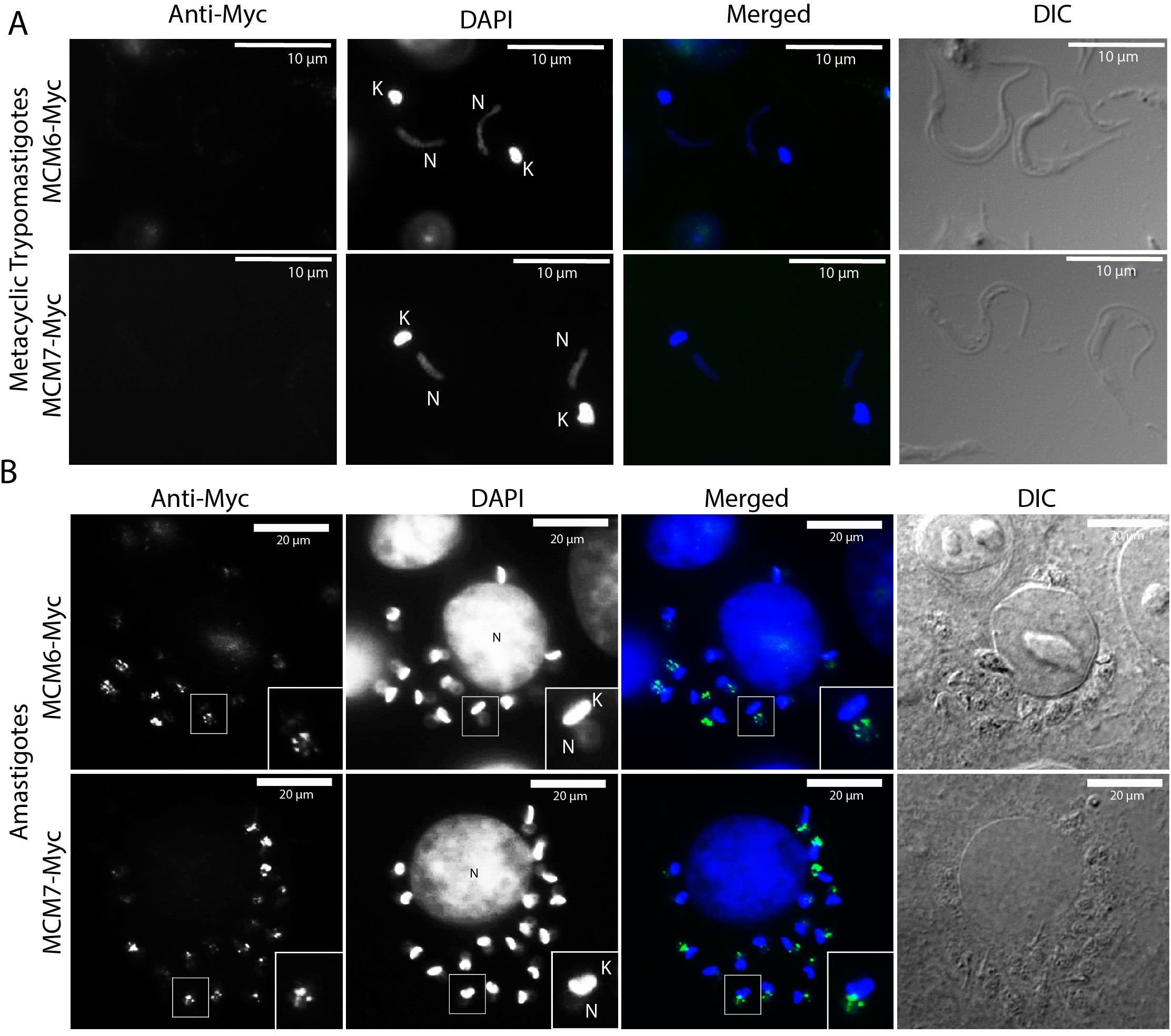
Figure 7. Immunofluorescence of T. cruzi metacyclic trypomastigotes and amastigotes (Dm28c strain). (A) Metacyclic trypomastigotes and (B) intracellular amastigotes derived from the MCM6-Myc (Dm28c strain) and MCM7-Myc cell lines (Dm28c strain) were subjected to immunofluorescence using anti-Myc antibody. White bar scale represents 10 µm in (A), and 20 µm in (B). N, nucleus; K, kinetoplast; green, anti-Myc; blue, DAPI (DNA); DIC, Differential Interference Contrast. In B, the image corresponds to one slice of a Z-stack image, and the framed amastigote in the image is magnified twofold in the bottom right corner of anti-Myc, DAPI, and merged images.
Metacyclic trypomastigotes were then used to infect mammalian cells to produce amastigotes and TCTs (Tissue-Culture Trypomastigotes). Using immunofluorescence to assess MCM6-Myc and MCM7-Myc locations within intracellular amastigotes, we observed that these proteins were also confined to the nucleus in this replicative life cycle stage (Figure 7B). Unlike epimastigotes, the cell cycle stage of amastigotes cannot be inferred from morphology. However, no cytoplasmic signal was detected for either MCM protein, with localization being exclusively nuclear.
Tissue-culture trypomastigotes are arrested in G1 phase of the cell cycle
Given our previous observation that cells are arrested in the G1 phase following metacyclogenesis, we characterized the cell cycle profile of TCTs using the Dm28c MCM6-Myc and MCM7-Myc strains, with cell cycle stages defined using the FlowJo software modeling tool. The cell cycle profiles of replicative epimastigotes and TCTs of MCM6-Myc and MCM7-Myc cell lines were compared (Figures 8A, B, respectively). ~50% of epimastigotes were in G1 phase (Figures 8C, D), 50.2% and 48.3% in the MCM6-Myc and MCM7-Myc cell lines, respectively. By comparison, TCTs exhibited a higher proportion, with around ~74% of cells in G1 phase (Figures 8C, D). Therefore, G1 arrest was also observed in TCTs. We performed immunofluorescence on both epimastigotes and TCTs to further assess MCM protein expression. As shown (Figure 8E), specific nuclear signals were observed exclusively in epimastigotes, while TCTs did not exhibit expression of MCM6-Myc or MCM7-Myc.
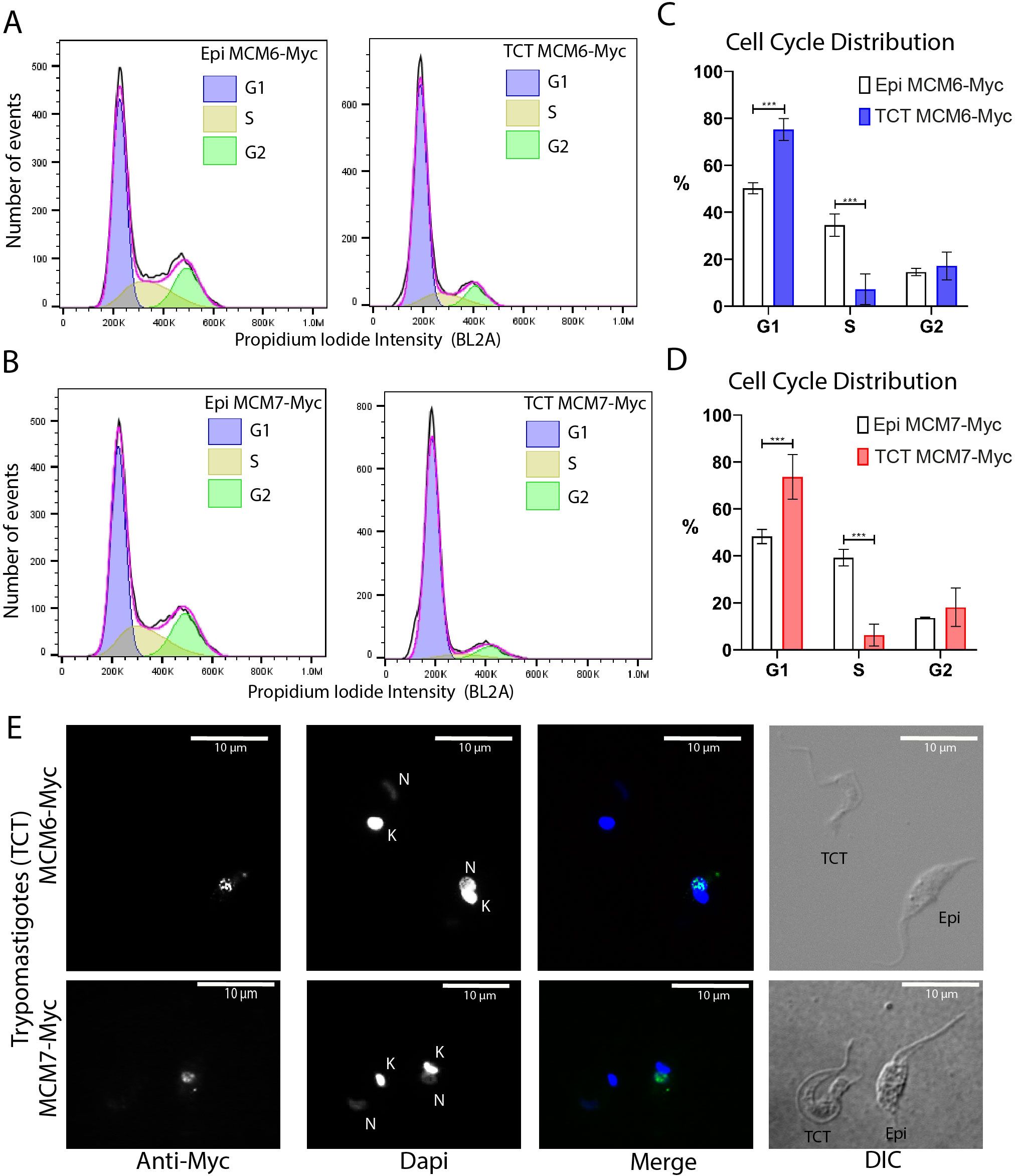
Figure 8. Cell cycle distribution and immunofluorescence analysis of tissue culture trypomastigotes (Dm28c strain). (A, B) Histograms of event counts versus propidium iodide intensity from flow cytometry analysis of epimastigotes and TCTs. Cell cycle distribution was determined using the cell cycle modeling tool in FlowJo software. (A) MCM6-Myc cell line. (B) MCM7-Myc cell line. (C, D) Bar charts illustrating the cell cycle distribution of epimastigotes and TCTs. (C) MCM6-Myc cell line. (D) MCM7-Myc cell line. (E) Immunofluorescence analysis of TCTs and epimastigotes on the same slide, using an anti-Myc antibody in the MCM6-Myc and MCM7-Myc cell lines. The white bar scale represents 10 µm. N, nucleus; K, kinetoplast; green, anti-Myc; blue, DAPI (DNA); DIC, Differential Interference Contrast.
Discussion
MCM subunits are nuclear in amastigotes and epimastigotes, and their regulation (throughout the cell cycle) does not involve nuclear export or downregulation
The MCM2–7 complex is the only pre-RC component that is integrated into the replisome during DNA replication (Polasek-Sedlackova et al., 2022). In T. cruzi, MCM expression is exclusive to the replicative forms (Calderano et al., 2014) (Figures 3, 7; Supplementary Figure 1), localizes inside the nucleus (Figures 3A, B; Supplementary Figure 1) and colocalizes with the site of EdU incorporation (Figures 3C, D), supporting the view that the MCM complex has a conserved role in DNA replication and is part of the replisome in this organism.
The pre-RC complex is involved in DNA replication control, so their components can be subject to three main regulation mechanisms: degradation, nuclear export, and chromatin detachment. In T. cruzi, we found that MCM subunits are expressed and nuclear-localized throughout the epimastigote cell cycle (Figures 2, 3; Supplementary Figure 1), in line with most eukaryotes (Forsburg, 2004), including T. brucei (Dang and Li, 2011). This nuclear pattern of MCM complex expression in epimastigotes was consistent across all analyzed conditions, including in those that were replicating (Figure 3; Supplementary Figure 1), stationary (Figure 6), or committed to the differentiation process (Supplementary Figure 3). Therefore, mechanisms other than nuclear export and degradation may be involved in regulating MCM complex activity in epimastigotes.
MCM subunits are downregulated during metacyclogenesis and are absent from trypomastigotes (metacyclic and TCTs)
When cells exit the cell cycle, MCM subunits are downregulated, and quiescent cells maintain low levels of expression (Blow and Hodgson, 2002; Stoeber et al., 2001). However, MCM subunits expression is abolished in terminally differentiated cells (Carroll et al., 2018).
In T. cruzi, downregulation of the MCM complex was observed during metacyclogenesis (Calderano et al., 2014) (Figures 4D, 5). Protein degradation rates increase in stationary epimastigotes (Henriquez et al., 1993), and higher levels of turn-over occur during metacyclogenesis, where autophagy and proteasome-mediated protein degradation play a crucial role (Losinno et al., 2020; Cardoso et al., 2011, 2008). However, maintaining MCM subunits expression in stationary epimastigotes guarantees their readiness to re-enter the cell cycle and initiate DNA replication promptly when favorable conditions are restored (Lee and Osley, 2021). MCM subunits degradation represents a potential additional regulatory mechanism that acts to inhibit DNA replication in differentiated metacyclic trypomastigotes (Calderano et al., 2014). Different proteomic approaches have identified proteins differentially expressed in stationary epimastigotes (Avila et al., 2018) and during metacyclogenesis (Amorim et al., 2017; Lucena et al., 2019), however, MCM subunits were not detected in these studies. Detection of MCM subunits by proteomics seems to be challenging; studies investigating the cell cycle (Chávez et al., 2021), nuclear (dos Santos Júnior Ade et al., 2015) and chromatin (Leandro de Jesus et al., 2017) proteome profiles have been unable to detect these proteins, with the exception of MCM2 (TcCLB.506933.40). This protein was detected in the proteome of the two cell cycle phases analyzed, G1 and S (Chávez et al., 2021), consistent with the constitutive expression of MCM6 and 7 reported here (Figures 2C, F). With this exception, the limited ability to date of proteome techniques to detect MCM subunits prevents more in-depth comparisons between our data and previous studies.
G1/G0 arrest: possible G1 checkpoint in trypanosomatids
The cell cycle arrest observed during metacyclogenesis and in TCTs was defined by DNA content analysis (Figures 4C, D, Figures 8A–D), however, this does not allow differentiation between G1 and G0 arrested cells due to their identical DNA content. In other eukaryotes and metazoans, it is known that G1 and G0 populations exhibit different transcriptome (Coller et al., 2006) and proteome (Ly et al., 2015) profiles. Several markers for G0 cells have been identified (Breeden and Tsukiyama, 2022), including unlicensed origins of replication (Carroll et al., 2018; Stoeber et al., 1998), reduced rRNA transcription (Hannan et al., 2000), decreased translation (Pereira et al., 2015; Liu and Sabatini, 2020), diminished transcription rates (Choder, 1991; Young et al., 2017; McKnight et al., 2015; Mews et al., 2014), and condensed chromatin (Evertts et al., 2013; Rawlings et al., 2011; Schmiady and Sperling, 1981; Swygert et al., 2019). Additionally, S. cerevisiae in the stationary phase is heterogeneous and consists of quiescent (G0) and non-quiescent cells (Allen et al., 2006; Aragon et al., 2008). In this context, we categorize stationary epimastigotes as being in a G1/G0 arrested state, and the non-replicative trypomastigotes lifeforms (both metacyclic and TCTs) to be G0 arrested, as they exhibit several hallmarks of G0 cells, including reduced transcriptional (Elias et al., 2001) and translational (Smircich et al., 2015) activities, increased chromatin condensation (Lima et al., 2022), absence of a nucleolus (Lima et al., 2022), and unlicensed DNA replication origins (Calderano et al., 2014).
This G1/G0 arrest suggests that the exit from the cell cycle occurs at the G1 phase. Notably, in T. brucei, the transition from the replicative slender form to the non-replicative stumpy form occurs in early G1 (Briggs et al., 2021), and in amastigotes of T. cruzi, G1 arrest is also observed after stress induction (Dumoulin and Burleigh, 2018). Additionally, the primary factors required to pass the G1 restriction point (Johnston et al., 1977; Johnson and Skotheim, 2013) are also observed in T. cruzi; these are nutritional stress (Gonzales-Perdomo et al., 1988; Figueiredo et al., 2000; Hamedi et al., 2015; Barison et al., 2017) and cellular size (Campbell and De Graffenried, 2023). The G1 checkpoint is conserved among most eukaryotes and is typically regulated by transcriptional control (Johnson and Skotheim, 2013). However, in trypanosomatids, transcription is polycistronic (Imboden et al., 1987), meaning they cannot regulate transcription via RNA polymerase II as in other eukaryotes (Clayton, 2019). As a result, the mechanisms governing the G1-G0 transition in these parasites remain unclear. Nonetheless, it is intriguing that this G1 control checkpoint appears to be present in this early-branching eukaryote, which may also employ alternative regulatory mechanisms beyond transcriptional control.
Conclusion
Epimastigotes arrested in G1/G0 can either maintain MCM subunits expression and resume the cell cycle when conditions become favorable or undergo differentiation, where they enter G0 as MCM subunits are degraded as part of the replication repression mechanism. However, the regulatory mechanisms governing this process remain unclear and warrant further investigation.
Data availability statement
The raw data supporting the conclusions of this article will be made available by the authors, without undue reservation.
Author contributions
BS: Formal Analysis, Investigation, Methodology, Validation, Visualization, Writing – review & editing. ÉM: Data curation, Formal Analysis, Methodology, Writing – review & editing. AC: Data curation, Methodology, Writing – review & editing. FC: Methodology, Writing – review & editing. MT: Methodology, Writing – review & editing. JK: Funding acquisition, Resources, Supervision, Writing – review & editing. ME: Conceptualization, Funding acquisition, Resources, Writing – review & editing. SC: Conceptualization, Data curation, Formal Analysis, Funding acquisition, Investigation, Methodology, Project administration, Supervision, Visualization, Writing – original draft, Writing – review & editing.
Funding
The author(s) declare that financial support was received for the research and/or publication of this article. This study was financed, in part, by the São Paulo Research Foundation (FAPESP), Brazil. Process Numbers #2021/01013-5; # 2020/00694-6; #2018/15553-9; #2022/05264-5 (BS master fellowship); #2022/08866-6 (AC master fellowship); # 2022/02243-7 (ÉM master fellowship). ME is fellowship from National Council for Scientific and Technological Development (CNPq) (31125/2021).
Acknowledgments
We thank Dr. Noelia Lander and Dr. Roberto Docampo for pMOTag23M plasmid and FAPESP for grant and fellowship funding.
Conflict of interest
The authors declare that the research was conducted in the absence of any commercial or financial relationships that could be construed as a potential conflict of interest.
Generative AI statement
The author(s) declare that Generative AI was used in the creation of this manuscript. Artificial intelligence was used to improve the English writing, as the original draft was written by a non-native English speaker. AI was not used to generate content, but only to improve and correct the writing.
Publisher’s note
All claims expressed in this article are solely those of the authors and do not necessarily represent those of their affiliated organizations, or those of the publisher, the editors and the reviewers. Any product that may be evaluated in this article, or claim that may be made by its manufacturer, is not guaranteed or endorsed by the publisher.
Supplementary material
The Supplementary Material for this article can be found online at: https://www.frontiersin.org/articles/10.3389/fcimb.2025.1584812/full#supplementary-material
Supplementary Figure 1 | Fluorescence imaging of T. cruzi CL Brener strain epimastigotes modified using CRISPR/Cas9. T. cruzi epimastigotes were genetically modified by CRISPR/Cas9 to incorporate three copies of the mNeonGreen gene at the 3’ end of the MCM2, MCM3, MCM4, and MCM6 genes. Fluorescence microscopy was employed to capture images of mNeonGreen fluorescence (green), DAPI-stained DNA (blue), and Differential Interference Contrast (DIC). Cells at different stages of the cell cycle are shown. The black scale bar represents 10 µm. N indicates the nucleus, K indicates the kinetoplast, and F indicates the flagellum. (A) MCM2-3mNG cell line; (B) MCM3-3mNG cell line; (C) MCM4-3mNG cell line; (D) MCM6-3mNG cell line.
Supplementary Figure 2 | Immunofluorescence imaging of T. cruzi Dm28c strain epimastigotes and amastigotes genetically modified using CRISPR/Cas9. The epimastigotes were modified by inserting three copies of the Myc sequence at the 3’ end of the MCM6 and MCM7 genes. Immunofluorescence microscopy was performed to visualize anti-Myc staining (green), DAPI-stained DNA (blue), and Differential Interference Contrast (DIC) images. Here are shown raw images of intracellular amastigotes and epimastigotes from: Dm28c-Cas9 (control), Dm28c-MCM6-Myc, and Dm28c-MCM7-Myc cell lines. The black scale bar represents 10 µm, and the white scale bar represents 20 µm. Dashed rectangles highlight cells that are 2x magnified. For amastigotes, magnified images are on the bottom right (control and MCM6-Myc) and bottom left (MCM7-Myc). For epimastigotes, the magnified cells are located to the left of the immunofluorescence image. N denotes the nucleus and K the kinetoplast.
Supplementary Figure 3 | Immunofluorescence imaging of T. cruzi Dm28c cells after metacyclogenesis. Epimastigotes from the MCM6-Myc and MCM7-Myc cell lines (Dm28c strain) were subjected to metacyclogenesis. Immunofluorescence staining was performed using an anti-Myc antibody. The images show both differentiated metacyclic trypomastigotes and non-differentiated epimastigotes. (A) MCM6-Myc cell line and (B) MCM7-Myc cell line. Anti-Myc staining is shown in green, DAPI-stained DNA in blue, and Differential Interference Contrast (DIC) images are included. The white scale bar represents 10 µm. N denotes the nucleus, K the kinetoplast.
References
Allen, C., Büttner, S., Aragon, A. D., Thomas, J. A., Meirelles, O., Jaetao, J. E., et al. (2006). Isolation of quiescent and nonquiescent cells from yeast stationary-phase cultures. J. Cell Biol. 174, 89–100. doi: 10.1083/jcb.200604072
Amorim, J. C., Batista, M., Da Cunha, E. S., Lucena, A. C. R., Lima, C. V. P., Sousa, K., et al. (2017). Quantitative proteome and phosphoproteome analyses highlight the adherent population during Trypanosoma cruzi metacyclogenesis. Sci. Rep. 7, 9899. doi: 10.1038/s41598-017-10292-3
Aragon, A. D., Rodriguez, A. L., Meirelles, O., Roy, S., Davidson, G. S., Tapia, P. H., et al. (2008). Characterization of differentiated quiescent and nonquiescent cells in yeast stationary-phase cultures. Mol. Biol. Cell 19, 1271–1280. doi: 10.1091/mbc.e07-07-0666
Arias, E. E. and Walter, J. C. (2007). Strength in numbers: preventing rereplication via multiple mechanisms in eukaryotic cells. Genes Dev. 21, 497–518. doi: 10.1101/gad.1508907
Avila, C. C., Mule, S. N., Rosa-Fernandes, L., Viner, R., Barison, M. J., Costa-Martins, A. G., et al. (2018). Proteome-wide analysis of trypanosoma cruzi exponential and stationary growth phases reveals a subcellular compartment-specific regulation. Genes (Basel) 9. doi: 10.3390/genes9080413
Barison, M. J., Rapado, L. N., Merino, E. F., Furusho Pral, E. M., Mantilla, B. S., Marchese, L., et al. (2017). Metabolomic profiling reveals a finely tuned, starvation-induced metabolic switch in Trypanosoma cruzi epimastigotes. J. Biol. Chem. 292, 8964–8977. doi: 10.1074/jbc.M117.778522
Blagosklonny, M. V. and Pardee, A. B. (2002). The restriction point of the cell cycle. Cell Cycle 1, 103–110. doi: 10.4161/cc.1.2.108
Blow, J. J. and Dutta, A. (2005). Preventing re-replication of chromosomal DNA. Nat. Rev. Mol. Cell Biol. 6, 476–486. doi: 10.1038/nrm1663
Blow, J. J. and Hodgson, B. (2002). Replication licensing–defining the proliferative state? Trends Cell Biol. 12, 72–78. doi: 10.1016/S0962-8924(01)02203-6
Breeden, L. L. and Tsukiyama, T. (2022). Quiescence in saccharomyces cerevisiae. Annu. Rev. Genet. 56, 253–278. doi: 10.1146/annurev-genet-080320-023632
Briggs, E. M., Rojas, F., Mcculloch, R., Matthews, K. R., and Otto, T. D. (2021). Single-cell transcriptomic analysis of bloodstream Trypanosoma brucei reconstructs cell cycle progression and developmental quorum sensing. Nat. Commun. 12, 5268. doi: 10.1038/s41467-021-25607-2
Burkard, G., Fragoso, C. M., and Roditi, I. (2007). Highly efficient stable transformation of bloodstream forms of Trypanosoma brucei. Mol. Biochem. Parasitol. 153, 220–223. doi: 10.1016/j.molbiopara.2007.02.008
Calderano, S., Godoy, P., Soares, D., Sant’anna, O. A., Schenkman, S., and Elias, M. C. (2014). ORC1/CDC6 and MCM7 distinct associate with chromatin through Trypanosoma cruzi life cycle. Mol. Biochem. Parasitol. 193, 110–113. doi: 10.1016/j.molbiopara.2014.03.004
Camargo, E. P. (1964). Growth and differentiation in trypanosoma cruzi. I. Origin of metacyclic trypanosomes in liquid media. Rev. Inst. Med. Trop. Sao Paulo 6, 93–100.
Campbell, P. C. and De Graffenried, C. L. (2023). Morphogenesis in Trypanosoma cruzi epimastigotes proceeds via a highly asymmetric cell division. PloS Negl. Trop. Dis. 17, e0011731. doi: 10.1371/journal.pntd.0011731
Cardoso, J., Lima, C. E. P., Leal, T., Gradia, D. F., Fragoso, S. P., Goldenberg, S., et al. (2011). Analysis of proteasomal proteolysis during the in vitro metacyclogenesis of Trypanosoma cruzi. PLoS One 6, e21027. doi: 10.1371/journal.pone.0021027
Cardoso, J., Soares, M. J., Menna-Barreto, R. F., Le Bloas, R., Sotomaior, V., Goldenberg, S., et al. (2008). Inhibition of proteasome activity blocks Trypanosoma cruzi growth and metacyclogenesis. Parasitol. Res. 103, 941–951. doi: 10.1007/s00436-008-1081-6
Carroll, T. D., Newton, I. P., Chen, Y., Blow, J. J., and Nathke, I. (2018). Lgr5(+) intestinal stem cells reside in an unlicensed G1 phase. J. Cell Biol. 217, 1667–1685. doi: 10.1083/jcb.201708023
Chávez, S., Urbaniak, M. D., Benz, C., Smircich, P., Garat, B., Sotelo-Silveira, J. R., et al. (2021). Extensive Translational Regulation through the Proliferative Transition of Trypanosoma cruzi Revealed by Multi-Omics. mSphere 6, e0036621. doi: 10.1128/mSphere.00366-21
Choder, M. (1991). A general topoisomerase I-dependent transcriptional repression in the stationary phase in yeast. Genes Dev. 5, 2315–2326. doi: 10.1101/gad.5.12a.2315
Clayton, C. (2019). Regulation of gene expression in trypanosomatids: living with polycistronic transcription. Open Biol. 9, 190072. doi: 10.1098/rsob.190072
Coller, H. A., Sang, L., and Roberts, J. M. (2006). A new description of cellular quiescence. PLoS Biol. 4, e83. doi: 10.1371/journal.pbio.0040083
Contreras, V. T., Salles, J. M., Thomas, N., Morel, C. M., and Goldenberg, S. (1985). In vitro differentiation of Trypanosoma cruzi under chemically defined conditions. Mol. Biochem. Parasitol. 16, 315–327. doi: 10.1016/0166-6851(85)90073-8
Costa, F. C., Francisco, A. F., Jayawardhana, S., Calderano, S. G., Lewis, M. D., Olmo, F., et al. (2018). Expanding the toolbox for Trypanosoma cruzi: A parasite line incorporating a bioluminescence-fluorescence dual reporter and streamlined CRISPR/Cas9 functionality for rapid in vivo localisation and phenotyping. PLoS Negl. Trop. Dis. 12, e0006388. doi: 10.1371/journal.pntd.0006388
Dalton, S. and Whitbread, L. (1995). Cell cycle-regulated nuclear import and export of Cdc47, a protein essential for initiation of DNA replication in budding yeast. Proc. Natl. Acad. Sci. U. S. A. 92, 2514–2518. doi: 10.1073/pnas.92.7.2514
Dang, H. Q. and Li, Z. (2011). The Cdc45·Mcm2-7·GINS protein complex in trypanosomes regulates DNA replication and interacts with two Orc1-like proteins in the origin recognition complex. J. Biol. Chem. 286, 32424–32435. doi: 10.1074/jbc.M111.240143
da Silva, M. S., Pavani, R. S., Damasceno, J. D., Marques, C. A., Mcculloch, R., Tosi, L. R. O., et al. (2017). Nuclear DNA replication in trypanosomatids: there are no easy methods for solving difficult problems. Trends Parasitol. 33, 858–874. doi: 10.1016/j.pt.2017.08.002
DePamphilis, M. L. (2005). Cell cycle dependent regulation of the origin recognition complex. Cell Cycle 4, 70–79. doi: 10.4161/cc.4.1.1333
Diffley, J. F., Cocker, J. H., Dowell, S. J., Harwood, J., and Rowley, A. (1995). Stepwise assembly of initiation complexes at budding yeast replication origins during the cell cycle. J. Cell Sci. Suppl. 19, 67–72. doi: 10.1242/jcs.1995.Supplement_19.9
dos Santos Júnior Ade, C., Kalume, D. E., Camargo, R., Gómez-Mendoza, D. P., Correa, J. R., Charneau, S., et al. (2015). Unveiling the trypanosoma cruzi nuclear proteome. PLoS One 10, e0138667. doi: 10.1371/journal.pone.0138667
Drury, L. S., Perkins, G., and Diffley, J. F. (2000). The cyclin-dependent kinase Cdc28p regulates distinct modes of Cdc6p proteolysis during the budding yeast cell cycle. Curr. Biol. 10, 231–240. doi: 10.1016/S0960-9822(00)00355-9
Dumoulin, P. C. and Burleigh, B. A. (2018). Stress-induced proliferation and cell cycle plasticity of intracellular trypanosoma cruzi amastigotes. MBio 9. doi: 10.1128/mBio.00673-18
Elias, M. C., Da Cunha, J. P., De Faria, F. P., Mortara, R. A., Freymüller, E., and Schenkman, S. (2007). Morphological events during the Trypanosoma cruzi cell cycle. Protist 158, 147–157. doi: 10.1016/j.protis.2006.10.002
Elias, M. C., Marques-Porto, R., Freymüller, E., and Schenkman, S. (2001). Transcription rate modulation through the Trypanosoma cruzi life cycle occurs in parallel with changes in nuclear organisation. Mol Biochem Parasitol 112, 79–90. doi: 10.1016/s0166-6851(00)00349-2
Evertts, A. G., Manning, A. L., Wang, X., Dyson, N. J., Garcia, B. A., and Coller, H. A. (2013). H4k20 methylation regulates quiescence and chromatin compaction. Mol. Biol. Cell. 24, 3025–3037. doi: 10.1091/mbc.e12-07-0529
Ferreira, A. Z. L., De Araújo, C. N., Cardoso, I. C. C., De Souza Mangabeira, K. S., Rocha, A. P., Charneau, S., et al. (2023). Metacyclogenesis as the starting point of chagas disease. Int. J. Mol. Sci. 25. doi: 10.3390/ijms25010117
Figueiredo, R. C., Rosa, D. S., and Soares, M. J. (2000). Differentiation of Trypanosoma cruzi epimastigotes: metacyclogenesis and adhesion to substrate are triggered by nutritional stress. J. Parasitol. 86, 1213–1218. doi: 10.1645/0022-3395(2000)086[1213:DOTCEM]2.0.CO;2
Fiore, A. P. Z. P., Ribeiro, P. D. F., and Bruni-Cardoso, A. (2018). Sleeping beauty and the microenvironment enchantment: microenvironmental regulation of the proliferation-quiescence decision in normal tissues and in cancer development. Front. Cell Dev. Biol. 6, 59–59. doi: 10.3389/fcell.2018.00059
Forsburg, S. L. (2004). Eukaryotic MCM proteins: beyond replication initiation. Microbiol. Mol. Biol. Rev. 68, 109–131. doi: 10.1128/MMBR.68.1.109-131.2004
Fujita, M., Hori, Y., Shirahige, K., Tsurimoto, T., Yoshikawa, H., and Obuse, C. (1998). Cell cycle dependent topological changes of chromosomal replication origins in Saccharomyces cerevisiae. Genes Cells 3, 737–749. doi: 10.1046/j.1365-2443.1998.00226.x
Galanti, N., Dvorak, J. A., Grenet, J., and Mcdaniel, J. P. (1994). Hydroxyurea-induced synchrony of DNA replication in the Kinetoplastida. Exp. Cell Res. 214, 225–230. doi: 10.1006/excr.1994.1252
Godoy, P. D., Nogueira-Junior, L. A., Paes, L. S., Cornejo, A., Martins, R. M., Silber, A. M., et al. (2009). Trypanosome prereplication machinery contains a single functional orc1/cdc6 protein, which is typical of archaea. Eukaryot. Cell 8, 1592–1603. doi: 10.1128/EC.00161-09
Gonçalves, C. S., Ávila, A. R., De Souza, W., Motta, M. C. M., and Cavalcanti, D. P. (2018). Revisiting the Trypanosoma cruzi metacyclogenesis: morphological and ultrastructural analyses during cell differentiation. Parasit. Vectors 11, 83. doi: 10.1186/s13071-018-2664-4
Gonzales-Perdomo, M., Romero, P., and Goldenberg, S. (1988). Cyclic AMP and adenylate cyclase activators stimulate Trypanosoma cruzi differentiation. Exp. Parasitol. 66, 205–212. doi: 10.1016/0014-4894(88)90092-6
Hamedi, A., Botelho, L., Britto, C., Fragoso, S. P., Umaki, A. C., Goldenberg, S., et al. (2015). In vitro metacyclogenesis of Trypanosoma cruzi induced by starvation correlates with a transient adenylyl cyclase stimulation as well as with a constitutive upregulation of adenylyl cyclase expression. Mol. Biochem. Parasitol. 200, 9–18. doi: 10.1016/j.molbiopara.2015.04.002
Hannan, K. M., Kennedy, B. K., Cavanaugh, A. H., Hannan, R. D., Hirschler-Laszkiewicz, I., Jefferson, L. S., et al. (2000). RNA polymerase I transcription in confluent cells: Rb downregulates rDNA transcription during confluence-induced cell cycle arrest. Oncogene 19, 3487–3497. doi: 10.1038/sj.onc.1203690
Henriquez, D. A., Perez, N., Pance, A., and Bradley, C. (1993). Mechanisms of protein degradation in Trypanosoma cruzi. Biol. Res. 26, 151–157.
Im, J. S., Ki, S. H., Farina, A., Jung, D. S., Hurwitz, J., and Lee, J. K. (2009). Assembly of the Cdc45-Mcm2-7-GINS complex in human cells requires the Ctf4/And-1, RecQL4, and Mcm10 proteins. Proc. Natl. Acad. Sci. U. S. A. 106, 15628–15632. doi: 10.1073/pnas.0908039106
Imboden, M. A., Laird, P. W., Affolter, M., and Seebeck, T. (1987). Transcription of the intergenic regions of the tubulin gene cluster of Trypanosoma brucei: evidence for a polycistronic transcription unit in a eukaryote. Nucleic Acids Res. 15, 7357–7368. doi: 10.1093/nar/15.18.7357
Jallepalli, P. V., Brown, G. W., Muzi-Falconi, M., Tien, D., and Kelly, T. J. (1997). Regulation of the replication initiator protein p65cdc18 by CDK phosphorylation. Genes Dev. 11, 2767–2779. doi: 10.1101/gad.11.21.2767
Johnson, A. and Skotheim, J. M. (2013). Start and the restriction point. Curr. Opin. Cell Biol. 25, 717–723. doi: 10.1016/j.ceb.2013.07.010
Johnston, G. C., Pringle, J. R., and Hartwell, L. H. (1977). Coordination of growth with cell division in the yeast Saccharomyces cerevisiae. Exp. Cell Res. 105, 79–98. doi: 10.1016/0014-4827(77)90154-9
Kim, Y. and Kipreos, E. T. (2007). Cdt1 degradation to prevent DNA re-replication: conserved and non-conserved pathways. Cell Div. 2, 18. doi: 10.1186/1747-1028-2-18
Kim, J. and Kipreos, E. T. (2008). Control of the Cdc6 replication licensing factor in metazoa: the role of nuclear export and the CUL4 ubiquitin ligase. Cell Cycle 7, 146–150. doi: 10.4161/cc.7.2.5282
Lander, N., Chiurillo, M. A., Vercesi, A. E., and Docampo, R. (2017). Endogenous C-terminal tagging by CRISPR/cas9 in trypanosoma cruzi. Bio Protoc. 7. doi: 10.21769/BioProtoc.2299
Leandro de Jesus, T. C., Calderano, S. G., Vitorino, F. N., Llanos, R. P., Lopes, M. C., De Araújo, C. B., et al. (2017). Quantitative Proteomic Analysis of Replicative and Nonreplicative Forms Reveals Important Insights into Chromatin Biology of Trypanosoma cruzi. Mol. Cell Proteomics 16, 23–38. doi: 10.1074/mcp.M116.061200
Lee, P. H. and Osley, M. A. (2021). Chromatin structure restricts origin utilization when quiescent cells re-enter the cell cycle. Nucleic Acids Res. 49, 864–878. doi: 10.1093/nar/gkaa1148
Liang, C. and Stillman, B. (1997). Persistent initiation of DNA replication and chromatin-bound MCM proteins during the cell cycle in cdc6 mutants. Genes Dev. 11, 3375–3386. doi: 10.1101/gad.11.24.3375
Lima, A. R. J., Silva, H. G. S., Poubel, S., Rosón, J. N., De Lima, L. P. O., Costa-Silva, H. M., et al. (2022). Open chromatin analysis in Trypanosoma cruzi life forms highlights critical differences in genomic compartments and developmental regulation at tDNA loci. Epigenetics Chromatin 15, 22. doi: 10.1186/s13072-022-00450-x
Liu, G. Y. and Sabatini, D. M. (2020). mTOR at the nexus of nutrition, growth, ageing and disease. Nat Rev Mol Cell Biol 21, 183–203. doi: 10.1038/s41580-019-0199-y
Losinno, A. D., Martínez, S. J., Labriola, C. A., Carrillo, C., and Romano, P. S. (2020). Induction of autophagy increases the proteolytic activity of reservosomes during. Autophagy 17, 439–456. doi: 10.1080/15548627.2020.1720428
Lucena, A. C. R., Amorim, J. C., De Paula Lima, C. V., Batista, M., Krieger, M. A., De Godoy, L. M. F., et al. (2019). Quantitative phosphoproteome and proteome analyses emphasize the influence of phosphorylation events during the nutritional stress of Trypanosoma cruzi: the initial moments of in vitro metacyclogenesis. Cell Stress Chaperones. 24, 927–936. doi: 10.1007/s12192-019-01018-7
Ly, T., Endo, A., and Lamond, A. I. (2015). Proteomic analysis of the response to cell cycle arrests in human myeloid leukemia cells. eLife 4, e04534. doi: 10.7554/eLife.04534
Lygerou, Z. and Nurse, P. (1999). The fission yeast origin recognition complex is constitutively associated with chromatin and is differentially modified through the cell cycle. J. Cell Sci. 112, 3703–3712. doi: 10.1242/jcs.112.21.3703
Machida, Y. J., Hamlin, J. L., and Dutta, A. (2005). Right place, right time, and only once: replication initiation in metazoans. Cell 123, 13–24. doi: 10.1016/j.cell.2005.09.019
Madine, M. A., Swietlik, M., Pelizon, C., Romanowski, P., Mills, A. D., and Laskey, R. A. (2000). The roles of the MCM, ORC, and Cdc6 proteins in determining the replication competence of chromatin in quiescent cells. J. Struct. Biol. 129, 198–210. doi: 10.1006/jsbi.2000.4218
Marques, C. A., Tiengwe, C., Lemgruber, L., Damasceno, J. D., Scott, A., Paape, D., et al. (2016). Diverged composition and regulation of the Trypanosoma brucei origin recognition complex that mediates DNA replication initiation. Nucleic Acids Res. 44, 4763–4784. doi: 10.1093/nar/gkw147
Martín-Escolano, J., Marín, C., Rosales, M. J., Tsaousis, A. D., Medina-Carmona, E., and Martín-Escolano, R. (2022). An updated view of the trypanosoma cruzi life cycle: intervention points for an effective treatment. ACS Infect. Dis. 8, 1107–1115. doi: 10.1021/acsinfecdis.2c00123
Matson, J. P. and Cook, J. G. (2017). Cell cycle proliferation decisions: the impact of single cell analyses. FEBS J. 284, 362–375. doi: 10.1111/febs.2017.284.issue-3
McKnight, J. N., Boerma, J. W., Breeden, L. L., and Tsukiyama, T. (2015). Global promoter targeting of a conserved lysine deacetylase for transcriptional shutoff during quiescence entry. Mol. Cell 59, 732–743. doi: 10.1016/j.molcel.2015.07.014
Melo, R. F. P., Guarneri, A. A., and Silber, A. M. (2020). The influence of environmental cues on the development of. Front. Cell Infect. Microbiol. 10, 27. doi: 10.3389/fcimb.2020.00027
Méndez, J., Zou-Yang, X. H., Kim, S. Y., Hidaka, M., Tansey, W. P., and Stillman, B. (2002). Human origin recognition complex large subunit is degraded by ubiquitin-mediated proteolysis after initiation of DNA replication. Mol. Cell 9, 481–491. doi: 10.1016/S1097-2765(02)00467-7
Mews, P., Zee, B. M., Liu, S., Donahue, G., Garcia, B. A., and Berger, S. L. (2014). Histone methylation has dynamics distinct from those of histone acetylation in cell cycle reentry from quiescence. Mol. Cell Biol. 34, 3968–3980. doi: 10.1128/MCB.00763-14
Paterou, A., Sáez Conde, J., Týč, J., Sunter, J. D., Vaughan, S., Gull, K., et al. (2025). A comprehensive toolkit for protein localization and functional analysis in trypanosomatids. Open Biol 15, 240361. doi: 10.1098/rsob.240361
Pereira, S. F., Gonzalez, R. L., Jr., and Dworkin, J. (2015). Protein synthesis during cellular quiescence is inhibited by phosphorylation of a translational elongation factor. Proc Natl Acad Sci U S A 112, E3274–E3281. doi: 10.1073/pnas.1505297112
Petersen, B. O., Wagener, C., Marinoni, F., Kramer, E. R., Melixetian, M., Lazzerini Denchi, E., et al. (2000). Cell cycle- and cell growth-regulated proteolysis of mammalian CDC6 is dependent on APC-CDH1. Genes Dev. 14, 2330–2343. doi: 10.1101/gad.832500
Polasek-Sedlackova, H., Miller, T. C. R., Krejci, J., Rask, M.-B., and Lukas, J. (2022). Solving the MCM paradox by visualizing the scaffold of CMG helicase at active replisomes. Nat. Commun. 13, 6090. doi: 10.1038/s41467-022-33887-5
Rawlings, J. S., Gatzka, M., Thomas, P. G., and Ihle, J. N. (2011). Chromatin condensation via the condensin II complex is required for peripheral T-cell quiescence. EMBO J. 30, 263–276. doi: 10.1038/emboj.2010.314
Sagot, I. and Laporte, D. (2019). The cell biology of quiescent yeast - a diversity of individual scenarios. J. Cell Sci. 132. doi: 10.1242/jcs.213025
Saha, T., Ghosh, S., Vassilev, A., and Depamphilis, M. L. (2006). Ubiquitylation, phosphorylation and Orc2 modulate the subcellular location of Orc1 and prevent it from inducing apoptosis. J. Cell Sci. 119, 1371–1382. doi: 10.1242/jcs.02851
Santos, C., Ludwig, A., Kessler, R. L., Rampazzo, R. C. P., Inoue, A. H., Krieger, M. A., et al. (2018). Trypanosoma cruzi transcriptome during axenic epimastigote growth curve. Mem. Inst. Oswaldo Cruz 113, e170404. doi: 10.1590/0074-02760170404
Schmiady, H. and Sperling, K. (1981). Length of human prematurely condensed chromosomes during G0 and G1 phase. Exp. Cell Res. 134, 461–465. doi: 10.1016/0014-4827(81)90446-8
Smircich, P., Eastman, G., Bispo, S., Duhagon, M. A., Guerra-Slompo, E. P., Garat, B., et al. (2015). Ribosome profiling reveals translation control as a key mechanism generating differential gene expression in Trypanosoma cruzi. BMC Genomics 16, 443. doi: 10.1186/s12864-015-1563-8
Stoeber, K., Mills, A. D., Kubota, Y., Krude, T., Romanowski, P., Marheineke, K., et al. (1998). Cdc6 protein causes premature entry into S phase in a mammalian cell-free system. EMBO J. 17, 7219–7229. doi: 10.1093/emboj/17.24.7219
Stoeber, K., Tlsty, T. D., Happerfield, L., Thomas, G. A., Romanov, S., Bobrow, L., et al. (2001). DNA replication licensing and human cell proliferation. J. Cell Sci. 114, 2027–2041. doi: 10.1242/jcs.114.11.2027
Swygert, S. G., Kim, S., Wu, X., Fu, T., Hsieh, T. H., Rando, O. J., et al. (2019). Condensin-dependent chromatin compaction represses transcription globally during quiescence. Mol. Cell 73, 533–546.e4. doi: 10.1016/j.molcel.2018.11.020
Tanaka, S. and Diffley, J. F. (2002). Interdependent nuclear accumulation of budding yeast Cdt1 and Mcm2–7 during G1 phase. Nat. Cell Biol. 4, 198–207. doi: 10.1038/ncb757
Tiengwe, C., Marcello, L., Farr, H., Gadelha, C., Burchmore, R., Barry, J. D., et al. (2012). Identification of ORC1/CDC6-interacting factors in Trypanosoma brucei reveals critical features of origin recognition complex architecture. PLoS One 7, e32674. doi: 10.1371/journal.pone.0032674
World Health Organization (WHO). (2024). Chagas disease, also known as American trypanosomiasis, is a potentially life-threatening illness caused by the protozoan parasite Trypanosoma cruzi (T. cruzi). 4 April. Available online at: https://www.who.int/news-room/facts-in-pictures/detail/chagas-disease (Accessed December 10, 2024).
Keywords: Mini-Chromosome Maintenance, MCM, Trypanosoma cruzi, metacyclogenesis, replication control, G0, G1 arrest, cell cycle arrest
Citation: Santarossa BA, Mariani É, Corrêa AdP, Costa FC, Taylor MC, Kelly JM, Elias MC and Calderano SG (2025) Stage-specific MCM protein expression in Trypanosoma cruzi: insights into metacyclogenesis and G1 arrested epimastigotes. Front. Cell. Infect. Microbiol. 15:1584812. doi: 10.3389/fcimb.2025.1584812
Received: 27 February 2025; Accepted: 16 April 2025;
Published: 26 May 2025.
Edited by:
Rubem Figueiredo Sadok Menna-Barreto, Oswaldo Cruz Foundation (Fiocruz), BrazilReviewed by:
Izabela Marques Dourado Bastos, University of Brasilia, BrazilCarlos Robello, Universidad de la República, Uruguay
Ranjeet Kumar, Banaras Hindu University, India
Copyright © 2025 Santarossa, Mariani, Corrêa, Costa, Taylor, Kelly, Elias and Calderano. This is an open-access article distributed under the terms of the Creative Commons Attribution License (CC BY). The use, distribution or reproduction in other forums is permitted, provided the original author(s) and the copyright owner(s) are credited and that the original publication in this journal is cited, in accordance with accepted academic practice. No use, distribution or reproduction is permitted which does not comply with these terms.
*Correspondence: Simone Guedes Calderano, c2ltb25lLmNhbGRlcmFub0BidXRhbnRhbi5nb3YuYnI=