- Division of Diabetes, Endocrinology and Metabolism, Imperial College London, London, United Kingdom
Over the last century, our knowledge of the processes which control appetite and weight regulation has developed significantly. The understanding of where gut hormones fit into the control of energy homeostasis in addition to the rapid advancement of pharmacotherapeutics has paved the way for the development of novel gut hormone analogs to target weight loss. Currently, bariatric surgery remains the most efficacious treatment for obesity. The emergence of gut hormone analogs may provide a useful non-surgical addition to the armamentarium in treating obesity. Simply targeting single gut hormone pathways may be insufficiently efficacious, and combination/multiple-agonist approaches may be necessary to obtain the results required for clear clinical impact.
Obesity is the accumulation of excessive fat that may impair health. It is the driver for several serious non-communicable diseases, including type 2 diabetes, cardiovascular disease, hypertension, dyslipidaemia, cancer, obstructive sleep apnoea and musculoskeletal problems. Aetiologically, obesity derives from the interaction of multiple genetic factors that bias metabolism toward the accumulation of fat, combined with environmental factors including the supply of easily available energy-dense food and reduced opportunities for physical activity. The prevalence of obesity is increasing year on year. Global estimates for obesity prevalence have tripled from 4.3% (1975) to 13.2% (2016) (1).
Current Options for Obesity
Lifestyle modifications remain the first line treatment for obesity, with evidence from large studies demonstrating only a modest benefit in outcomes. For example, meta-analysis of studies with a minimum of 1 year follow-up demonstrate 5–9% short term weight loss and 3–6% long term weight loss (2). It is possible to lose much more weight with incentivized high-intensity lifestyle changes, however the weight loss is often not sustainable due to counter-regulatory changes after such weight loss. This was highlighted by the long-term follow up of participants who participated in a weight loss competition, losing an average of 58.3 ± 24.9 kg at the end of the competition (3). Participants subsequently exhibited significant metabolic adaptations to counteract weight loss efforts, in particular a sustained reduction in energy expenditure up to 6 years following initial weight loss. This led to weight regain despite continued lifestyle changes. Strikingly, this reduction in energy expenditure persisted despite weight regain. This is a clear (if somewhat extreme) example of how the body defends itself against weight loss. Current lifestyle recommendations are not a sufficiently effective and/or durable strategy for weight loss and patients require other treatment options.
The choices for the pharmacological treatment of obesity have until recently been limited to orlistat, a pancreatic lipase inhibitor preventing fat absorption within the gut. Average weight loss is around 3% over 1 year, but patient perseverance with treatment is limited due to undesirable gastrointestinal side effects (4). Treatments such as sibutramine and rimonabant have been withdrawn due to psychiatric and cardiovascular side effects respectively (5, 6). Lorcaserin (Belviq®) and phentermine/topiramate (Qsymia®) are both licensed in the US but are not currently licensed in Europe. Bupropion/naltrexone has been approved in the US under the trade name Contrave® and was more recently approved in the European Union in 2015 under the trade name Mysimba®. Overall, these pharmacological treatments are characterized by relatively weak efficacies (5–10% weight loss) and potential side effects, including the possible exacerbation of anxiety or depression, which are common co-morbidities in patients with obesity.
Bariatric treatment of obesity is established as the most effective method of obtaining sustained weight loss. A recent long-term (12-year follow up) study of patients with severe obesity undergoing Roux-en-Y gastric bypass, showed an average weight loss from baseline of 45 kg at 2 years, 36.3 kg at 6 years, and 35 kg at 12 years (7). The data from the Swedish Obesity Study shows that bariatric surgery improves mortality, and reduces the rate of cardiovascular events, cancer and diabetes (8). Long-term follow-up data also show that for obese patients with diabetes, bariatric surgery is more effective than medical therapy alone in improving glycaemic control, lipid profile and quality of life, with up to 3 in 10 patients obtaining a long-term remission, defined as a HbA1c of ≤6.5% without anti-diabetic medication (9). Furthermore, mortality rates associated with bariatric surgery are low, similar to a laparoscopic cholecystectomy operation. Despite the highly effective outcomes with bariatric surgery, there are associated post-surgical complications such as post-prandial hypoglycaemia, nutritional deficiencies, and psychosocial issues which may cause long-term morbidity and should not be overlooked (10). In addition, surgery does require considerable resources in terms of specialist surgeons, facilities and follow-up; these limit the deliverability of bariatric surgery to all patients with obesity. Lastly, surgery is a “one size” solution where patients obtain varying levels of weight loss ranging from the inadequate to the excessive from the same procedure, i.e., “one size does not fit all.”
Over the last 20 years, a gap has emerged between relatively ineffective pharmacological therapy and effective (but non-titratable and non-reversible) surgical treatments (Figure 1). Within this gap, there is a need for pharmacotherapies capable of inducing significant weight loss, sustaining weight loss and which are proven in reducing comorbidities such as the risk of developing overt diabetes and cardiovascular disease. In the following review, we examine the state-of-the art in gut hormone research, and the current considerable efforts to turn these into practical and safe therapies for obesity.
Appetite and Energy Homeostasis
The long-term control of body weight is tightly regulated through various homeostatic physiological processes to allow the body to conserve energy. Traditionally, food consumption is thought of in terms of short and long-term signals. In the acute setting, food consumption is regulated by sensory stimuli, gut mechanoreceptors, nutrient concentrations in the plasma, and changes in gut hormones secreted by specialized enteroendocrine cells. Longer term adiposity signals, for example, leptin and insulin, also influence feeding behavior. These homeostatic mechanisms rely on gut-neuronal circuits or the “gut-brain axis” allowing signaling between the periphery and central nervous system to coordinate systemic changes in our physiology (Figure 2). Both peripheral circulating peptides and vagal afferents are important in the signaling pathway controlling appetite. The main central regions where “anorexic” or “orexigenic” signals converge include brainstem regions including the dorsal vagal complex (DVC) in the medulla and the hypothalamic nuclei, most importantly the arcuate nucleus. Of the neuronal cell populations within the arcuate nucleus, two leptin-sensitive neuronal cell subtypes are characterized by the expression of specific weight regulating neuropeptides. The first contains Neuropeptide Y (NPY) and Agouti-related peptide (AgRP). The second contains alpha-melanocyte stimulating hormone (alpha-MSH) and cocaine-and amphetamine regulated transcript (CART). Activation of the orexigenic NPY/AgRP neurons leads to food intake, partly by NPY activation of neuropeptide Y1R receptors and partly by AgRP inverse agonism of the MC4R receptor. On the other hand, activation of the anorexigenic alpha-MSH/CART neurons inhibits food intake via activation of MC4R by alpha-MSH. Both pathways operate exclusively and receive signals via the nucleus of the solitary tract (NTS) and the dorsal vagal complex (DVC) of the brainstem, relaying important afferent vagal signals from peripheral gut hormone receptors, for example in the vagal innervation of the hepatic portal vein. The circumventricular organs, such as the median eminence, subfornical organs and area postrema, act as points that allow access for peripheral peptides through the blood-brain barrier or may themselves have receptors for the gut hormones, transducing these peripheral signals into the CNS (e.g., area postrema to the NTS). In addition to homeostatic mechanisms, food intake is controlled by regions of the brain regulating hedonistic pathways, circadian rhythms and cultural/learned experiences and therefore the hypothalamic nuclei also receive important signals from the cortex and mesolimbic system.
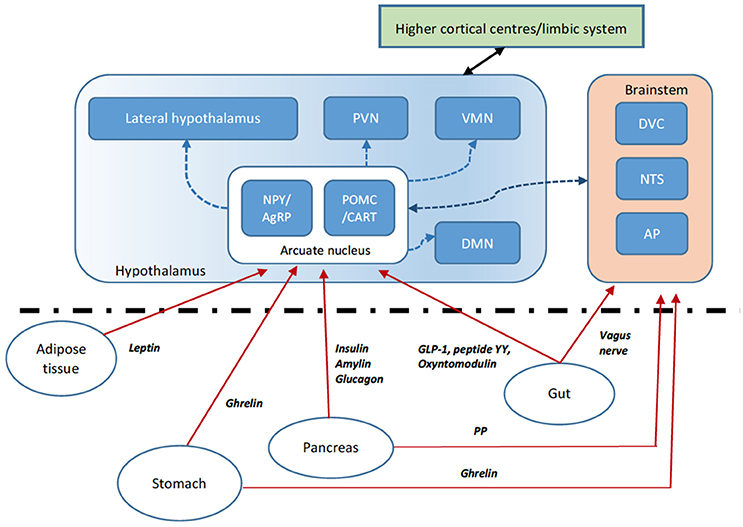
Figure 2. Gut brain axis schematic. The hypothalamus integrates anorexigenic and orexigenic signals. Peripheral signals such as gut peptides, leptin from adipose tissue and pancreatic signals are able to cross the blood-brain barrier at the median eminence (a circumventricular organ) or activate their cognate receptors at other circumventricular organs such as the area postrema (AP). These signals relay to the hypothalamic arcuate nucleus (ARC) via direct activation or indirectly via brainstem regions including the dorsal vagal complex (DVC) and nucleus tractus solitarus (NTS). Within the ARC, two key populations of neurons include those that express the orexigenic neuropeptide Y (NPY) and agouti-related peptide (AgRP—an inverse agonist of the melanocortin receptor MC4R) and those expressing the anorexigenic pro-opiomelanocortin (POMC), processed to the MC4R agonist alpha-MSH, and cocaine- and amphetamine regulated transcript (CART). The ARC signals to second-order neurons in various hypothalamic nuclei including the paraventricular nucleus (PVN), dorsomedial nucleus (DMN), ventromedial nucleus (VMN) and lateral hypothalamic area (LHA). Second-order neurons express anorexigenic and orexigenic neuropeptides further modulating appetite and energy homeostasis. Vagal afferents also signal directly to brainstem regions. Higher central nervous system centers including the mesolimbic pathways also signal to the hypothalamic nuclei.
Gut Hormones
The impact of gut hormones on the regulation of appetite was discovered early on when N.F. Maclagan in 1937 investigated the effect of a crude preparation of intestinal mucosa, called enterogastrone which was administered to rabbits at a dose of 2–3 mg/kg (11). He found a transient reduction in food intake in the rabbits and reported this extract was not active by mouth. This observation is now understood to be due to the rapid degradation of gut peptides in the stomach, a process which to this day limits the widespread use of gut hormone therapy in obesity pharmacotherapy. Since Maclagan's early discovery, several gut hormones have been isolated and described (Table 1).
CCK—the First “Satiety Signal”
The first isolated gut hormone to be investigated was cholecystokinin (CCK), a peptide released by the neuroendocrine I-cells in the duodenum and jejunum in response to fat and protein rich meals (12). The “enterogastrone” preparation used by Maclagan in his experiments contained CCK (13). Gerard Smith and colleagues showed that intraperitoneal injection of CCK into fasted rats inhibited food intake by 50% (14). To further understand the physiological role of CCK, the feeding behavior of rats with chronic gastric fistulas were studied. In a series of these experiments, CCK was proposed as the first “satiety signal” (15). CCK, in addition to inhibition of food intake, delays gastric emptying, stimulates pancreatic enzyme secretion and gall bladder contraction. The finding that CCK is a satiety signal led to research into the peptide's potential as an obesity therapy. However, CCK receptor agonists such as loxiglumide did not apparently show any anorectic activity in human volunteers and therefore their development has been abandoned (16).
GLP-1 and its Analogues as Current Clinical Treatments for Diabetes and Obesity
The most well-known therapeutic gut hormone is GLP-1 which has been developed into analogs for the treatment of type 2 diabetes mellitus over the past 20 years. GLP-1 is released from the neuroendocrine L-cells of the gut. It is the product of post-translational processing of preproglucagon, by prohormone convertase 1 (PC-1) and the peptidyl-glycine alpha-amidating monooxygenase (PHM) which adds the C-terminal amide (Figure 3). A variety of GLP-1 variants are secreted in vivo including the inactive forms GLP-11−37 and GLP-11−36NH2, in addition to the active forms, GLP-17−37 and GLP-17−36NH2 (18). GLP-1's role in mediating the “incretin” effect, whereby insulin secretion is enhanced when enteral glucose is given in comparison to parenteral glucose was observed early on. Wolfgang Schmidt and colleagues first postulated that GLP-1 stimulated insulin secretion and demonstrated that GLP1−36NH2 was able to stimulate glucose-dependent insulin release from isolated rat pancreatic islets, albeit at high concentrations (19). Not long after this, GLP-17−36NH2 was shown to be a much more potent incretin in humans (20). The interest in this peptide as an obesity therapy followed in vivo experiments whereby GLP-17−36NH2 was administered intracerebroventricularly (ICV) in rats, leading to a dose-dependent reduction in food intake (21). Following on from this, continuous subcutaneous infusion of GLP-17−36NH2 in both lean and obese humans was shown to cause an increase in satiety and reduction of food intake (22, 23). In contrast to CCK, GLP-17−36NH2 retained its anorectic effects during continuous infusion and when administered at a dose of 4.8 pmol/kg for 6 weeks led to an average weight loss of 2% (24).
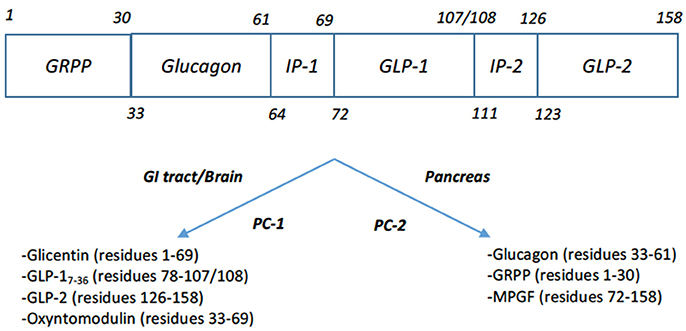
Figure 3. Post-translational products of Preproglucagon (17). Tissue specific processing by proprotein convertase 1 (PC-1) in the intestine and brain of proglucagon leads to formation of glicentin, GLP-1, GLP-2, oxyntomodulin. Note that GLP-1's C-terminal residue 108 is processed to the C-terminal amide. Processing of proglucagon by proprotein convertase 2 (PC-2) in the pancreas leads to the formation of glucagon, glicentin-related polypeptide (GRPP), and major proglucagon fragment (MPGF).
One of the major breakthroughs in this area came when Buckley and Lundquist described the degradation of GLP-1 by cleavage of the N-terminal 2 residues from the peptide (25). It was later found that dipeptidyl peptidase-IV (DPP4) was responsible for this inactivation (26). Around the same time, the GLP-1R agonist exendin-4/exenatide was isolated from the saliva of the Gila monster lizard (Heloderma suspectum) by Eng et al. (27). This was developed into the first marketed GLP-1 analog (Byetta®) due to its resistance to DPP4 degradation, extending its half-life and making it practicable as a treatment. Since these discoveries, several GLP-1 analogs have been developed with different structural modifications to implement DPP4 resistance and prolong their half-lives. These GLP-1 analogs are mostly licensed for the treatment of type 2 diabetes, including liraglutide (Victoza®), exenatide (Byetta®), and lixisenatide (Lyxumia®). Longer acting preparations are available and include exenatide LAR (Bydureon®), albiglutide (Tanzeum®), semaglutide (Ozempic®), and dulaglutide (Trulicity®).
The advantage of the GLP-1 analogs over other types of diabetes treatment is the associated weight loss. For example, exenatide and its longer acting preparation exenatide LAR (encapsulated in poly(lactic-co-glycolic) microspheres), lead to mean weight reductions of 3.7 and 3.6 kg, respectively, over 30 weeks in a comparative study (28). When given via an annually renewed osmotic mini-pump, exenatide can reduce weight by 2.4–4.2 kg in obese type 2 diabetic patients (29). Liraglutide has a longer half-life (13 h) than exenatide, owing to the linkage of a palmitate fatty acid group by a glutamic acid spacer at position 26, therefore increasing binding to circulating albumin. Licensed doses used in clinical practice range from 0.6 to 1.8 mg daily. Liraglutide 1.8 mg has been shown to have a similar effect on weight loss to exenatide, with weight loss of 3.24 kg over 26 weeks (30). The weekly analog semaglutide, at doses of 0.5 or 1 mg weekly, has also been shown to cause mean weight reductions of 3.5-6.1 kg in trials in obese type 2 diabetics (31).
GLP-1 analogs have also been investigated as a treatment for obesity in non-diabetic patients. Astrup et al. initially showed that liraglutide at doses up to 3 mg daily reduced weight by 7.2 kg on average in a double-blind randomized placebo controlled 20-week proof of concept trial, however there was a dose-related nausea as the main side-effect (32). This proof of concept led to a 56-week randomized placebo-controlled trial in 3731 patients, where it was shown that 3 mg liraglutide led to a reduction in body weight by an average of 8.4 kg, versus the placebo group who lost an average of 2.6 kg (33). As a result, liraglutide 3 mg is now licensed as a treatment in non-diabetic obese patients (Saxenda®). Semaglutide has also been tested in non-diabetic obese subjects in a small double-blinded randomized cross-over study (at a dose of 1.0 mg weekly) and shown to reduce weight on average by 5 kg over 12 weeks in comparison with a 1 kg weight gain in the placebo arm (34). In a Phase II dose-ranging study in non-diabetic obese subjects, semaglutide was given at doses of up to 0.4 mg daily for 52 weeks and compared to liraglutide 3 mg daily: it was shown to reduce weight on average by up to 13.8% at the highest dose of 0.4 mg, compared to 7.8% with liraglutide (35).
Despite the modest weight loss effects described with the GLP-1 analogs, there are a significant proportion of patients who do not respond adequately to GLP-1 analogs in terms of weight loss: the SCALE study showed, for example, that 37% of patients assigned to liraglutide 3 mg either lost <5% baseline weight or even gained weight, in other words they were non-responders (33). Even with semaglutide 0.4 mg daily, the non-response rate was 17% (35). Another issue with GLP-1 analog therapy for obesity are the common gastrointestinal side effects (nausea, vomiting, diarrhea, constipation) which occur in up to 40% of patients, thus limiting the maximal doses that can be given.
The PP-Fold Anorectic Gut Hormones: Peptide YY, Pancreatic Polypeptide
Peptide tyrosine tyrosine (PYY) is a peptide co-secreted with GLP-1 from the neuroendocrine L-cells of the intestine and is a member of the pancreatic polypeptide (PP) family of proteins. The PP family of proteins contains pancreatic polypeptide (PP), PYY, and the neurotransmitter neuropeptide Y (NPY), which have a common structural hair-pin fold. Full length PYY1−36 is processed by DPP4 to PYY3−36 which then selectively binds to Y2 neuropeptide Y receptors. PYY is secreted post-prandially and acts as a satiety signal (36). Administration of PYY by infusion in human volunteers leads to reduced food intake and this effect is retained in patients with obesity (37, 38). PYY has a short half-life, limiting its therapeutic practicability. Specific alterations to the PYY3−36 sequence can be made to obtain an analog with a prolonged duration of action. Long-acting analogs of PYY which enable weekly injections are being tested in Phase I (ClinicalTrials.gov Identifier NCT01515319). Another analog, with an alpha-helix stabilizing sequence and histidine residues, demonstrated efficacy in reducing food intake for up to 24 h in animal studies (39). Reversible PEGylation of PYY(3–36) maintains its functional activity and significantly prolongs its half-life in vivo (40). An oral preparation of PYY3−36 has been tested and does reduce food intake during an ad libitum test meal, but did not affect total 24 h food intake after a single dose, suggesting that the effect of a single dose is short-lived (41).
Pancreatic polypeptide (PP) is a 36-amino acid peptide hormone released by the PP cells of the pancreas after a nutrient stimulus. It is secreted in proportion to the calories ingested and remains elevated for up to 6 h (42). Intraperitoneal injections of PP reduce food intake over a 4 h period in both lean and obese mice (43). A short 90-min infusion of PP significantly reduced food intake in healthy human volunteers, and this effect seems to last for 24 h, reducing cumulative food intake by a mean of 25% (44). A stable analog of pancreatic polypeptide, PP 1420, has undergone Phase I trials to confirm the tolerability of single ascending subcutaneous doses of the peptide, PP 1420, in healthy subjects (45). The 7TM Pharma PP analog did not lower body weight in Phase II trials and development of the drug appears to have been terminated.
Amylin and its Synergistic Effects
Amylin is a peptide hormone co-secreted with insulin by the pancreatic beta cells in response to nutrient stimulus. It is a 37-amino acid peptide, derived from an 89 amino-acid precursor protein, referred to as preProIAPP. PreProIAPP is cleaved at the N-terminus resulting in ProIAPP and which is subsequently processed by PC-2. It has various roles including inhibiting glucagon secretion, delaying gastric emptying and acting as a satiety signal. Initial in vivo studies showed that intrahypothalamic injection of rat amylin dose dependently reduced feeding in rats for up to 8 h (46). There is also some evidence that amylin is capable of influencing food reward pathways (47). Pramlintide, an analog of Amylin, has been modified to prevent the formation of fibrils preserving stability and solubility of the peptide and has been approved by the FDA for diabetes treatment but not specifically for obesity. In a double-blind placebo-controlled study over 4 months, patients lost approximately 3 kg on average (48). Interestingly, amylin appears to have a synergistic effect with leptin: pre-treatment with amylin reduces the leptin resistance observed in obesity and leads to a mean weight loss of 12.7% when given in combination with recombinant leptin in a proof of concept trial (49, 50). Newer amylin analogs such as davalintide, PEGylated amylin and dual amylin/calcitonin agonists are in pre-clinical development for diabetes/obesity treatment, as yet there are no published clinical trials (47).
Inhibiting Ghrelin to Reduce Appetite
Ghrelin, a 28-amino acid peptide derived from preproghrelin, is secreted by the X/A like cells of the stomach and to a lesser extent the small intestine (51, 52). Through the action of ghrelin-O-transferase (GOAT), the peptide undergoes posttranslational acylation at the serine-3 residue linking it to octanoic acid (53). This modification allows the peptide to cross the blood-brain barrier and bind to its cognate receptor, the growth hormone secretagogue (GHS) receptor. Ghrelin levels rise to a maximum when fasting and fall after feeding (54). Ghrelin, unlike the other gut hormones, increases food intake when given to human volunteers (55), i.e., it is a “hunger hormone” that drives appetite. Consequently, there has been considerable interest in targeting the GHS receptor or inhibiting GOAT as an anti-obesity therapy, for example, utilizing antagonists, inverse agonists, ghrelin vaccines and GOAT enzyme inhibitors (56, 57). There has been varied success. For example, an in vivo study using a small molecule ghrelin receptor antagonist in diet-induced obese mice led to reduced food intake and weight loss of up to 15% (58). The ligand-independent constitutive activity of GHS receptor can be inhibited by inverse agonists to the receptor and one such drug has been trialed in Phase I. Interestingly this trial reports that the most common side effect is that of somnolence as well as a positive chronotropic effect on heart rate; these may limit the tolerability and long-term safety of the treatment, however this may open up the possibility that the drug is useful for insomnia (59).
Oxyntomodulin and the Dual GLP-1/Glucagon Agonist Concept
Due to the limitations of using single gut hormones (for example GLP-1 alone), researchers have investigated the idea of using the synergism between certain gut hormones to obtain benefits in metabolism and weight regulation. The most well-developed example of this is the dual agonism of GLP-1 and glucagon. Glucagon is secreted by alpha-cells of the pancreatic islets after PC-2 processing of proglucagon (60). In contrast to GLP-1, glucagon's classical effects are to increase hepatic glucose output by stimulating gluconeogenesis and glycogenolysis (61), although knockout of the glucagon receptor in mice only modestly reduces glucose levels by 1–2 mmol/l (62). Glucagon inhibits food intake when given to humans (63).
The interest in glucagon as a partner for GLP-1 comes from three other observations. Glucagon increases energy expenditure, unlike GLP-1, and this effect is retained when the two are co-infused (64, 65). Although animal studies suggest that this thermogenesis is via the activation of brown adipose tissue (BAT), studies in human subjects show that glucagon does not increase BAT activation as assessed by infrared thermometry nor 18F-FDG uptake (66). This BAT-independent effect of glucagon on energy expenditure may be due to substrate cycling (67), however direct evidence for this effect in humans is currently lacking. The second observation is that the acute hyperglycaemia from glucagon can be counter-balanced by GLP-1 co-administration (64). The third observation is that the co-infusion of GLP-1 and glucagon leads to a synergistic suppression of food intake (65). Therefore, dual agonism of glucagon and GLP-1 receptors promises to deliver weight loss efficacy beyond that seen with GLP-1 alone, via synergistic anorectic effects, increased energy expenditure and without any hyperglycaemia arising from the glucagon component.
The prototypical dual GLP-1/glucagon agonist is oxyntomodulin (OXM), which is a peptide hormone secreted by the neuroendocrine L-cells of the intestine. Like glucagon and GLP-1, it is a product of preproglucagon post translational processing by PC1 in the gut. It was first discovered following early experiments showing that intestinal extracts contained an activity similar to pancreatic glucagon. An isolated fraction with glucagon properties was termed “enteroglucagon,” later found to include two peptides containing the entire glucagon sequence, glicentin and OXM (68). In addition to containing the glucagon sequence, OXM has an additional C-terminal 8-amino acid octapeptide (labeled IP-1 in Figure 3) (69). In vitro experiments show that OXM is a full agonist at both the GLP-1 and glucagon receptors, albeit with reduced binding affinities of 10- and 100-fold respectively compared to the cognate peptides (70, 71). Consistent with these receptor binding actions, research has shown that OXM potentiates glucose stimulated insulin secretion via the GLP-1 receptor and is an incretin in its own right (72, 73). Dakin and colleagues showed that ICV injection of OXM into rats inhibits feeding. Importantly, comparison with a “pair-fed group” given the mean food intake of the OXM-treated group showed that OXM treatment led to enhanced weight loss relative to the pair-fed group, implying that the peptide leads to an increase in energy expenditure (74). GLP-1 receptor activity mediates the anorectic action of OXM, but the additional weight loss effect requires glucagon receptor activity (71, 75–77). Thus, OXM represents a dual agonist at the GLP-1 and glucagon receptors, with each receptor respectively mediating anorectic and energy expenditure effects.
In a double-blind, placebo-controlled, cross over study, Cohen and colleagues showed that an intravenous infusion of OXM at 3 pmol/kg/min reduced mean food intake by 19% in healthy human volunteers (78). Wynne and colleagues carried out a double-blind, placebo-controlled, parallel group study, showing preprandial subcutaneous injection of 400 nmol OXM in healthy overweight or obese volunteers for 4 weeks resulted in a mean reduction of body weight by 2.4% (79). This was associated with a 19% reduction in energy intake. OXM, when given as subcutaneous injections for 4 days, does not alter resting energy expenditure, but increased activity-related energy expenditure and total energy expenditure (80).
Due to the intrinsic dual receptor activity of OXM, analogs can therefore be used as unimolecular GLP-1/glucagon dual agonists (81, 82). Structural modifications to OXM to stabilize it have been developed including PEGylation (83), fatty-acid acylation (84, 85), amino acid substitution and other modifications (84). Furthermore, structure-activity studies have identified analogs with superior potency compared to the native peptide (84). Day and colleagues described a PEGylated co-agonist, which when administered once a week, normalized adiposity and glucose tolerance in diet-induced obese mice (86). Another PEGylated long acting dual GLP-1/glucagon receptor agonist was tested in vivo in diet-induced obese mice and was shown to improve glycaemia, reduce food intake and induce substantial weight loss (87). Given the positive findings from these studies, several clinical trials of GLP-1/glucagon co-agonists are being carried out by a variety of pharmaceutical companies (82). One of the earliest candidates in this class, MEDI0382, has recently reported Phase I/II results with promising efficacy in improving glycaemia and reducing weight in obese diabetic patients when given for up to 41 days (88).
Other Dual/Triple Agonist Combinations (PYY/GLP-1, PYY/OXM, GLP-1/GIP, GLP-1/GIP/Glucagon)
Other combinations of analogs with putative synergistic effects on reduction in food intake have been explored. PYY(3-36) has been shown to possess synergistic anorectic effects when combined with GLP-1 or OXM in infusion studies (89, 90). Beglinger's group also showed that an oral combination of PYY/GLP-1 can reduce food intake during an ad libitum test meal to a greater extent than either of the two hormones alone (41). However, not all gut hormone combinations give additive/synergistic effects on food intake, for example PYY(3-36) and PP (91).
Another interesting “dual agonist” concept that is being explored is the glucose-dependent insulinotropic polypeptide GIP/GLP-1 co-agonist. GIP is an insulinotropic peptide released by the K-cells of the small intestine in response to nutrient ingestion (18). Therefore, GIP/GLP-1 co-agonists were explored as a means of enhancing the incretin effect. Finan and co-workers engineered peptides with balanced activity at the GLP-1 and GIP receptors, and these were modified with fatty-acid acylation or PEGylation to improve their pharmacokinetics. These GIP/GLP-1 dual agonists produced enhanced weight loss and improvements in glucose levels compared to GLP-1 agonists alone in animal studies. In a Phase I study in diabetic volunteers, they showed that one of the dual agonists can reduce HbA1c with minimal gastrointestinal side effects (92). This dual agonist (NNC0090-2746) went on to Phase II studies in obese diabetic patients over 12 weeks where it was shown to reduce HbA1c by 0.96% compared to placebo and to reduce weight by up to 1.8% relative to placebo, albeit its efficacy was not demonstrably superior to liraglutide (93). Extending this work on dual agonism, Finan and colleagues have also devised triple agonists of the GLP-1, GIP and glucagon receptors. In pre-clinical models, these new agents demonstrate better weight loss than the GLP-1/GIP dual agonist mentioned above (94). Clinical study results are awaited with the GLP-1/GIP/glucagon triple agonists.
Triple Agonism with GLP-1/OXM/PYY—the Pathway to the “Medical Bypass”?
In our continuing efforts to devise a better therapy for obesity, we have taken a tack which is based on the known effects of bariatric surgery on gut hormone physiology. Patients who have undergone RYGB have much higher post-prandial levels of PYY, GLP-1, and OXM (95–98), secretion being triggered by direct and early exposure of jejeunal L-cells to nutrients through the bypass. It is postulated that the release of these hormones leads to a synergistic effect on food intake (via all three hormones) and increased insulin secretion (via GLP-1 and OXM). The latter process could account for the rapid improvements in glycaemia which are observed early on after surgery, although other factors such as the very low-calorie liquid diet imposed after surgery are also likely to contribute. To test the hypothesis that the elevation of these three hormones were responsible for the benefits of RYGB directly, our group devised a study in which a triple infusion of GLP-1, OXM, and PYY (the “GOP infusion”) was given to recreate the post-prandial levels observed in RYGB patients. This led to a significant mean reduction in food intake by 32% when given for 10 h or so to healthy volunteers even though the individual doses of each hormone were calibrated to be sub-anorectic (98). No significant issues with nausea were observed. Continuing studies are underway to examine the effects of GOP infusion when this is given for up to 28 days to obese diabetic patients. Regardless, the GOP infusion study suggests that triple hormone/agonist therapy is feasible and capable of synergistically reducing food intake to achieve better weight loss than single or even dual-agonism, perhaps recapitulating the effect of the RYGB without surgery: the “medical bypass.”
Conclusions
GLP-1 therapy for obesity represents the first generation of gut hormone-based therapies for obesity. In the search for better efficacy than presently available, it seems that approaches targeting multiple satiety and metabolic pathways for the optimal effects on food intake and metabolism will be required, and there is much pharmaceutical development in this sector at present. It is hoped that these efforts will pay off in the form of an efficacious, well-tolerated, safe and even beneficial tool for fighting the global pandemic of obesity.
Author Contributions
Review written by DH and edited by TT and SB.
Funding
The Section of Endocrinology and Investigative Medicine is funded by grants from the MRC, BBSRC, NIHR, an Integrative Mammalian Biology (IMB) Capacity Building Award, an FP7- HEALTH- 2009- 241592 EuroCHIP grant and is supported by the NIHR Imperial Biomedical Research Centre Funding Scheme. DH is supported by an NIHR Academic Clinical Fellowship. TT and SB are supported by the MRC and NIHR Imperial BRC. The views expressed are those of the authors and not necessarily those of the MRC, the NHS, the NIHR or the Department of Health.
Conflict of Interest Statement
The authors declare that the research was conducted in the absence of any commercial or financial relationships that could be construed as a potential conflict of interest.
References
1. WHO Global Health Observatory. Global Health Observatory Repository: Overweight/Obesity. (2018) Available online at: http://apps.who.int/gho/data/node.main.A896, 2018
2. Franz MJ, VanWormer JJ, Crain AL, Boucher JL, Histon T, Caplan W, et al. Weight-loss outcomes: a systematic review and meta-analysis of weight-loss clinical trials with a minimum 1-year follow-up. J Am Diet Assoc. (2007) 107:1755–67. doi: 10.1016/j.jada.2007.07.017
3. Fothergill E, Guo J, Howard L, Kerns JC, Knuth ND, Brychta R, et al. Persistent metabolic adaptation 6 years after “The Biggest Loser” competition. Obesity (2016) 24:1612–9. doi: 10.1002/oby.21538
4. Rucker D, Padwal R, Li SK, Curioni C, Lau DC. Long term pharmacotherapy for obesity and overweight: updated meta-analysis. BMJ (2007) 335:1194–9. doi: 10.1136/bmj.39385.413113.25
5. James WP, Caterson ID, Coutinho W, Finer N, Van Gaal LF, Maggioni AP, et al. Effect of sibutramine on cardiovascular outcomes in overweight and obese subjects. N Engl J Med. (2010) 363:905–17. doi: 10.1056/NEJMoa1003114
6. Christensen R, Kristensen PK, Bartels EM, Bliddal H, Astrup A. Efficacy and safety of the weight-loss drug rimonabant: a meta-analysis of randomised trials. Lancet (2007) 370:1706–13. doi: 10.1016/S0140-6736(07)61721-8
7. Adams TD, Davidson LE, Litwin SE, Kim J, Kolotkin RL, Nanjee MN, et al. Weight and metabolic outcomes 12 years after gastric bypass. N Engl J Med. (2017) 377:1143–55. doi: 10.1056/NEJMoa1700459
8. Sjostrom L. Review of the key results from the Swedish Obese Subjects (SOS) trial - a prospective controlled intervention study of bariatric surgery. J Intern Med. (2013) 273:219–34. doi: 10.1111/joim.12012
9. Schauer PR, Bhatt DL, Kirwan JP, Wolski K, Aminian A, Brethauer SA, et al. Bariatric surgery versus intensive medical therapy for diabetes - 5-year outcomes. N Engl J Med. (2017) 376:641–51. doi: 10.1056/NEJMoa1600869
10. Tharakan G, Behary P, Wewer Albrechtsen NJ, Chahal H, Kenkre J, Miras AD, et al. Roles of increased glycaemic variability, GLP-1 and glucagon in hypoglycaemia after Roux-en-Y gastric bypass. Eur J Endocrinol. (2017) 177:455–64. doi: 10.1530/EJE-17-0446
11. Maclagan NF. The role of appetite in the control of body weight. J Physiol. (1937) 90:385–94. doi: 10.1113/jphysiol.1937.sp003524
12. Ivy AC, Oldberg E. Observations on the Cause of Gall-Bladder Contraction and Evacuation. Proc Soc Exp Biol Med. (1928) 25:251–2. doi: 10.3181/00379727-25-3800
13. Kosaka T, Lim RKS. Demonstration of the Humoral Agent in Fat Inhibition of Gastric Secretion. Proc Soc Exp Biol Med. (1930) 27:890–1. doi: 10.3181/00379727-27-5024
14. Gibbs J, Young RC, Smith GP. Cholecystokinin decreases food intake in rats. J Comp Physiol Psychol. (1973) 84:488–95. doi: 10.1037/h0034870
15. Gibbs J, Young RC, Smith GP. Cholecystokinin elicits saiety in rats with open gastric fistulas. Nature (1973) 245:323–5. doi: 10.1038/245323a0
16. Lieverse RJ, Masclee AA, Jansen JB, Rovati LC, Lamers CB. Satiety effects of the type A CCK receptor antagonist loxiglumide in lean and obese women. Biol Psychiatry (1995) 37:331–5. doi: 10.1016/0006-3223(94)00136-Q
17. Drucker DJ. Glucagon-like peptides: regulators of cell proliferation, differentiation, and apoptosis. Mol Endocrinol. (2003) 17:161–71. doi: 10.1210/me.2002-0306
18. Baggio LL, Drucker DJ. Biology of incretins: GLP-1 and GIP. Gastroenterology (2007) 132:2131–57. doi: 10.1053/j.gastro.2007.03.054
19. Schmidt W, Siegel EG, Creutzfeldt W. Glucagon-like peptide-1 but not glucagon-like peptide-2 stimulates insulin release from isolated rat pancreatic islets. Diabetologia (1985) 28:704–7. doi: 10.1007/BF00291980
20. Kreymann B, Williams G, Ghatei MA, Bloom SR. Glucagon-like peptide-1 7-36: a physiological incretin in man. Lancet (1987) 2:1300–4. doi: 10.1016/S0140-6736(87)91194-9
21. Turton MD, O'Shea D, Gunn I, Beak SA, Edwards CM, Meeran K, et al. A role for glucagon-like peptide-1 in the central regulation of feeding. Nature (1996) 379:69–72. doi: 10.1038/379069a0
22. Flint ARA, Astrup A, Holst JJ. Glucagon-like peptide 1 promotes satiety and suppresses energy intake in humans. J Clin Invest. (1997) 101:515–20. doi: 10.1172/JCI990
23. Naslund E, Bogefors J, Skogar S, Gryback P, Jacobsson H, Holst JJ, et al. GLP-1 slows solid gastric emptying and inhibits insulin, glucagon, and PYY release in humans. Am J Physiol. (1999) 277:R910–6. doi: 10.1152/ajpregu.1999.277.3.R910
24. Zander M, Madsbad S, Madsen JL, Holst JJ. Effect of 6-week course of glucagon-like peptide 1 on glycaemic control, insulin sensitivity, and beta-cell function in type 2 diabetes: a parallel-group study. Lancet (2002) 359:824–30. doi: 10.1016/S0140-6736(02)07952-7
25. Buckley Dl, Lundquist P. Analysis of the degradation of insulinotropin [GLP-1(7–37)] in human plasma and production of degradation resistant analogs. Regul Peptides (1992) 40:117. doi: 10.1016/0167-0115(92)90152-K
26. Mentlein R, Gallwitz B, Schmidt WE. Dipeptidyl-peptidase IV hydrolyses gastric inhibitory polypeptide, glucagon-like peptide-1(7-36)amide, peptide histidine methionine and is responsible for their degradation in human serum. Eur J Biochem. (1993) 214:829–35. doi: 10.1111/j.1432-1033.1993.tb17986.x
27. Eng J, Kleinman WA, Singh L, Singh G, Raufman JP. Isolation and characterization of exendin-4, an exendin-3 analogue, from Heloderma suspectum venom. Further evidence for an exendin receptor on dispersed acini from guinea pig pancreas. J Biol Chem. (1992) 267:7402–5.
28. Drucker DJ, Buse JB, Taylor K, Kendall DM, Trautmann M, Zhuang D, et al. Exenatide once weekly versus twice daily for the treatment of type 2 diabetes: a randomised, open-label, non-inferiority study. Lancet (2008) 372:1240–50. doi: 10.1016/S0140-6736(08)61206-4
29. Henry RR, Rosenstock J, Logan D, Alessi T, Luskey K, Baron MA. Continuous subcutaneous delivery of exenatide via ITCA 650 leads to sustained glycemic control and weight loss for 48 weeks in metformin-treated subjects with type 2 diabetes. J Diabetes Complications (2014) 28:393–8. doi: 10.1016/j.jdiacomp.2013.12.009
30. Buse JB, Rosenstock J, Sesti G, Schmidt WE, Montanya E, Brett JH, et al. Liraglutide once a day versus exenatide twice a day for type 2 diabetes: a 26-week randomised, parallel-group, multinational, open-label trial (LEAD-6). Lancet (2009) 374:39–47. doi: 10.1016/S0140-6736(09)60659-0
31. Madsbad S, Holst JJ. Glycaemic control and weight loss with semaglutide in type 2 diabetes. Lancet Diabetes Endocrinol. (2017) 5:315–7. doi: 10.1016/S2213-8587(17)30094-3
32. Astrup A, Rossner S, Van Gaal L, Rissanen A, Niskanen L, Al Hakim M, et al. Effects of liraglutide in the treatment of obesity: a randomised, double-blind, placebo-controlled study. Lancet (2009) 374:1606–16. doi: 10.1016/S0140-6736(09)61375-1
33. Pi-Sunyer X, Astrup A, Fujioka K, Greenway F, Halpern A, Krempf M, et al. SCALE Obesity and Prediabetes NN8022-1839 Study Group. A Randomized, Controlled Trial of 3.0 mg of Liraglutide in Weight Management. N Engl J Med. (2015) 373:11–22. doi: 10.1056/NEJMoa1411892
34. Blundell J, Finlayson G, Axelsen M, Flint A, Gibbons C, Kvist T, et al. Effects of once-weekly semaglutide on appetite, energy intake, control of eating, food preference and body weight in subjects with obesity. Diabetes Obes Metab. (2017) 19:1242–51. doi: 10.1111/dom.12932
35. O'Neil PM, Birkenfeld AL, McGowan B, Mosenzon O, Pedersen SD, Wharton S, et al. A Randomised, Phase II, Placebo- and Active-Controlled Dose-Ranging Study of Semaglutide for Treatment of Obesity in Subjects Without Diabetes. Chicago, IL: ENDO (2018).
36. Adrian TE, Ferri GL, Bacarese-Hamilton AJ, Fuessl HS, Polak JM, Bloom SR. Human distribution and release of a putative new gut hormone, peptide YY. Gastroenterology (1985) 89:1070–7. doi: 10.1016/0016-5085(85)90211-2
37. Batterham RL, Cowley MA, Small CJ, Herzog H, Cohen MA, Dakin CL, et al. Gut hormone PYY(3-36) physiologically inhibits food intake. Nature (2002) 418:650–4. doi: 10.1038/nature00887
38. Batterham RL, Cohen MA, Ellis SM, Le Roux CW, Withers DJ, Frost GS, et al. Inhibition of food intake in obese subjects by peptide YY3-36. N Engl J Med. (2003) 349:941–8. doi: 10.1056/NEJMoa030204
39. Cegla J, Cuenco J, Minnion J, Ghourab S, Hostomska K, Tan T, et al. Pharmacokinetics and pharmacodynamics of subcutaneously administered PYY3-36 and its analogues in vivo. Lancet (2015) 385 (Suppl. 1):S28. doi: 10.1016/S0140-6736(15)60343-9
40. Shechter Y, Tsubery H, Mironchik M, Rubinstein M, Fridkin M. Reversible PEGylation of peptide YY3-36 prolongs its inhibition of food intake in mice. FEBS Lett. (2005) 579:2439–44. doi: 10.1016/j.febslet.2005.03.044
41. Steinert RE, Poller B, Castelli MC, Drewe J, Beglinger C. Oral administration of glucagon-like peptide 1 or peptide YY 3-36 affects food intake in healthy male subjects. Am J Clin Nutr. (2010) 92:810–7. doi: 10.3945/ajcn.2010.29663
42. Adrian TE, Bloom SR, Bryant MG, Polak JM, Heitz PH, Barnes AJ. Distribution and release of human pancreatic polypeptide. Gut (1976) 17:940–4. doi: 10.1136/gut.17.12.940
43. Asakawa A, Uemoto M, Ueno N, Katagi M, Fujimiya M, Fujino K, et al. Peptide YY3-36 and pancreatic polypeptide suppress food intake. J Gastroenterol Hepatol. (2006) 21:1501–2. doi: 10.1111/j.1440-1746.2006.04338.x
44. Batterham RL, Le Roux CW, Cohen MA, Park AJ, Ellis SM, Patterson M, et al. Pancreatic polypeptide reduces appetite and food intake in humans. J Clin Endocrinol Metab. (2003) 88:3989–92. doi: 10.1210/jc.2003-030630
45. Tan TM, Field BC, Minnion JS, Cuenco-Shillito J, Chambers ES, Zac-Varghese S, et al. Pharmacokinetics, adverse effects and tolerability of a novel analogue of human pancreatic polypeptide, PP 1420. Br J Clin Pharmacol. (2012) 73:232–9. doi: 10.1111/j.1365-2125.2011.04082.x
46. Chance WT, Balasubramaniam A, Zhang FS, Wimalawansa SJ, Fischer JE. Anorexia following the intrahypothalamic administration of amylin. Brain Res. (1991) 539:352–4. doi: 10.1016/0006-8993(91)91644-G
47. Boyle CN, Lutz TA, Le Foll C. Amylin–Its role in the homeostatic and hedonic control of eating and recent developments of amylin analogs to treat obesity. Mol Metab. (2018) 8:203–10. doi: 10.1016/j.molmet.2017.11.009
48. Smith SR, Aronne LJ, Burns CM, Kesty NC, Halseth AE, Weyer C. Sustained weight loss following 12-month pramlintide treatment as an adjunct to lifestyle intervention in obesity. Diabetes Care (2008) 31:1816–23. doi: 10.2337/dc08-0029
49. Roth JD, Roland BL, Cole RL, Trevaskis JL, Weyer C, Koda JE, et al. Leptin responsiveness restored by amylin agonism in diet-induced obesity: evidence from nonclinical and clinical studies. Proc Natl Acad Sci USA. (2008) 105:7257–62. doi: 10.1073/pnas.0706473105
50. Ravussin E, Smith SR, Mitchell JA, Shringarpure R, Shan K, Maier H, et al. Enhanced weight loss with pramlintide/metreleptin: an integrated neurohormonal approach to obesity pharmacotherapy. Obesity (Silver Spring) (2009) 17:1736–43. doi: 10.1038/oby.2009.184
51. Kojima M, Hosoda H, Date Y, Nakazato M, Matsuo H, Kangawa K. Ghrelin is a growth-hormone-releasing acylated peptide from stomach. Nature (1999) 402:656–60. doi: 10.1038/45230
52. Date Y, Kojima M, Hosoda H, Sawaguchi A, Mondal MS, Suganuma T, et al. Ghrelin, a novel growth hormone-releasing acylated peptide, is synthesized in a distinct endocrine cell type in the gastrointestinal tracts of rats and humans. Endocrinology (2000) 141:4255–61. doi: 10.1210/endo.141.11.7757
53. Yang J, Brown MS, Liang G, Grishin NV, Goldstein JL. Identification of the acyltransferase that octanoylates ghrelin, an appetite-stimulating peptide hormone. Cell (2008) 132:387–96. doi: 10.1016/j.cell.2008.01.017
54. Tschop M, Smiley DL, Heiman ML. Ghrelin induces adiposity in rodents. Nature (2000) 407:908–13. doi: 10.1038/35038090
55. Wren AM, Seal LJ, Cohen MA, Brynes AE, Frost GS, Murphy KG, et al. Ghrelin enhances appetite and increases food intake in humans. J Clin Endocrinol Metab. (2001) 86:5992. doi: 10.1210/jcem.86.12.8111
56. Asakawa A, Inui A, Kaga T, Katsuura G, Fujimiya M, Fujino MA, et al. Antagonism of ghrelin receptor reduces food intake and body weight gain in mice. Gut (2003) 52:947–52. doi: 10.1136/gut.52.7.947
57. Khatib MN, Gaidhane S, Gaidhane AM, Simkhada P, Zahiruddin QS. Ghrelin O Acyl Transferase (GOAT) as a Novel Metabolic Regulatory Enzyme. J Clin Diagn Res. (2015) 9:LE01–5. doi: 10.7860/JCDR/2015/9787.5514
58. Esler WP, Rudolph J, Claus TH, Tang W, Barucci N, Brown SE, et al. Small-molecule ghrelin receptor antagonists improve glucose tolerance, suppress appetite, and promote weight loss. Endocrinology (2007) 148:5175–85. doi: 10.1210/en.2007-0239
59. Denney WS, Sonnenberg GE, Carvajal-Gonzalez S, Tuthill T, Jackson VM. Pharmacokinetics and pharmacodynamics of PF-05190457: the first oral ghrelin receptor inverse agonist to be profiled in healthy subjects. Br J Clin Pharmacol. (2017) 83:326–38. doi: 10.1111/bcp.13127
60. Knop FK. EJE PRIZE 2018: a gut feeling about glucagon. Eur J Endocrinol. (2018) 178:R267–80. doi: 10.1530/EJE-18-0197
61. Sandoval DA, D'Alessio DA. Physiology of proglucagon peptides: role of glucagon and GLP-1 in health and disease. Physiol Rev. (2015) 95:513–48. doi: 10.1152/physrev.00013.2014
62. Gelling RW, Du XQ, Dichmann DS, Romer J, Huang H, Cui L, et al. Lower blood glucose, hyperglucagonemia, and pancreatic alpha cell hyperplasia in glucagon receptor knockout mice. Proc Natl Acad Sci USA. (2003) 100:1438–43. doi: 10.1073/pnas.0237106100
63. Geary N, Kissileff HR, Pi-Sunyer FX, Hinton V. Individual, but not simultaneous, glucagon and cholecystokinin infusions inhibit feeding in men. Am J Physiol. (1992) 262:R975–80. doi: 10.1152/ajpregu.1992.262.6.R975
64. Tan TM, Field BC, McCullough KA, Troke RC, Chambers ES, Salem V, et al. Coadministration of glucagon-like peptide-1 during glucagon infusion in humans results in increased energy expenditure and amelioration of hyperglycemia. Diabetes (2013) 62:1131–8. doi: 10.2337/db12-0797
65. Cegla JTR, Jones B, Tharakan G, Kenkre J, McCullough KA, Lim CT, et al. Co-infusion of low-dose GLP-1 and glucagon in man results in a reduction in food intake. Diabetes (2014) 63:3711–20. doi: 10.2337/db14-0242
66. Salem V, Izzi-Engbeaya C, Coello C, Thomas DB, Chambers ES, Comninos AN, et al. Glucagon increases energy expenditure independently of brown adipose tissue activation in humans. Diabetes Obes Metab. (2016) 18:72–81. doi: 10.1111/dom.12585
67. Nair KS. Hyperglucagonemia increases resting metabolic rate in man during insulin deficiency. J Clin Endocrinol Metab. (1987) 64:896–901. doi: 10.1210/jcem-64-5-896
68. Holst JJ. Enteroglucagon. Annu Rev Physiol. (1997) 59:257–71. doi: 10.1146/annurev.physiol.59.1.257
69. Bataille D, Tatemoto K, Coudray AM, Rosselin G, Mutt V. [Bioactive “enteroglucagon” (oxyntomodulin): evidence for a C-terminal extension of the glucagon molecule]. C R Seances Acad Sci III (1981) 293:323–8.
70. Schepp W, Dehne K, Riedel T, Schmidtler J, Schaffer K, Classen M. Oxyntomodulin: a cAMP-dependent stimulus of rat parietal cell function via the receptor for glucagon-like peptide-1 (7-36)NH2. Digestion (1996) 57:398–405. doi: 10.1159/000201367
71. Pocai A, Carrington PE, Adams JR, Wright M, Eiermann G, Zhu L, et al. Glucagon-like peptide 1/glucagon receptor dual agonism reverses obesity in mice. Diabetes (2009) 58:2258–66. doi: 10.2337/db09-0278
72. Schjoldager BT, Baldissera FG, Mortensen PE, Holst JJ, Christiansen J. Oxyntomodulin: a potential hormone from the distal gut. Pharmacokinetics and effects on gastric acid and insulin secretion in man. Eur J Clin Invest. (1988) 18:499–503. doi: 10.1111/j.1365-2362.1988.tb01046.x
73. Shankar SS, Shankar RR, Mixson LA, Miller DL, Pramanik B, O'Dowd AK, et al. Native Oxyntomodulin has significant glucoregulatory effects independent of weight loss in obese humans with and without type 2 diabetes. Diabetes (2018) 67:1105–12. doi: 10.2337/db17-1331
74. Dakin CL, Small CJ, Batterham RL, Neary NM, Cohen MA, Patterson M, et al. Peripheral oxyntomodulin reduces food intake and body weight gain in rats. Endocrinology (2004) 145:2687–95. doi: 10.1210/en.2003-1338
75. Sowden GL, Drucker DJ, Weinshenker D, Swoap SJ. Oxyntomodulin increases intrinsic heart rate in mice independent of the glucagon-like peptide-1 receptor. Am J Physiol Regul Integr Comp Physiol. (2007) 292:R962–970. doi: 10.1152/ajpregu.00405.2006
76. Baggio LL, Huang Q, Brown TJ, Drucker DJ. Oxyntomodulin and glucagon-like peptide-1 differentially regulate murine food intake and energy expenditure. Gastroenterology (2004) 127:546–58. doi: 10.1053/j.gastro.2004.04.063
77. Kosinski JR, Hubert J, Carrington PE, Chicchi GG, Mu J, Miller C, et al. The glucagon receptor is involved in mediating the body weight-lowering effects of oxyntomodulin. Obesity (2012) 20:1566–71. doi: 10.1038/oby.2012.67
78. Cohen MA, Ellis SM, Le Roux CW, Batterham RL, Park A, Patterson M, et al. Oxyntomodulin suppresses appetite and reduces food intake in humans. J Clin Endocrinol Metab. (2003) 88:4696–701. doi: 10.1210/jc.2003-030421
79. Wynne K, Park AJ, Small CJ, Patterson M, Ellis SM, Murphy KG, et al. Subcutaneous oxyntomodulin reduces body weight in overweight and obese subjects: a double-blind, randomized, controlled trial. Diabetes (2005) 54:2390–5. doi: 10.2337/diabetes.54.8.2390
80. Wynne K, Park AJ, Small CJ, Meeran K, Ghatei MA, Frost GS, et al. Oxyntomodulin increases energy expenditure in addition to decreasing energy intake in overweight and obese humans: a randomised controlled trial. Int J Obes. (2006) 30:1729–36. doi: 10.1038/sj.ijo.0803344
81. Pocai A. Action and therapeutic potential of oxyntomodulin. Mol Metab. (2014) 3:241–51. doi: 10.1016/j.molmet.2013.12.001
82. Sanchez-Garrido MA, Brandt SJ, Clemmensen C, Muller TD, DiMarchi RD, Tschop MH. GLP-1/glucagon receptor co-agonism for treatment of obesity. Diabetologia (2017) 60:1851–61. doi: 10.1007/s00125-017-4354-8
83. Bianchi E, Carrington PE, Ingallinella P, Finotto M, Santoprete A, Petrov A, et al. A PEGylated analog of the gut hormone oxyntomodulin with long-lasting antihyperglycemic, insulinotropic and anorexigenic activity. Bioorg Med Chem. (2013) 21:7064–73. doi: 10.1016/j.bmc.2013.09.016
84. Druce MR, Minnion JS, Field BC, Patel SR, Shillito JC, Tilby M, et al. Investigation of structure-activity relationships of Oxyntomodulin (Oxm) using Oxm analogs. Endocrinology (2009) 150:1712–22. doi: 10.1210/en.2008-0828
85. Zhou J, Cai X, Huang X, Dai Y, Sun L, Zhang B, et al. A novel glucagon-like peptide-1/glucagon receptor dual agonist exhibits weight-lowering and diabetes-protective effects. Eur J Med Chem. (2017) 138:1158–69. doi: 10.1016/j.ejmech.2017.07.046
86. Day JW, Ottaway N, Patterson JT, Gelfanov V, Smiley D, Gidda J, et al. A new glucagon and GLP-1 co-agonist eliminates obesity in rodents. Nat Chem Biol. (2009) 5:749–57. doi: 10.1038/nchembio.209
87. Hershkovitz O, Bar-IIan A, Hart G, Fima E. 2013 The long-acting dual GLP-1/Glucagon Agonist, MOD-6030 improves glycaemic control and induces a prolonged weight loss in diet induced obesity mice following a once weekly administration. In: The Endocrine Society's 95th Annual Meeting and Expo. San Francisco, CA (2013).
88. Ambery P, Parker VE, Stumvoll M, Posch MG, Heise T, Plum-Moerschel L, et al. MEDI0382, a GLP-1 and glucagon receptor dual agonist, in obese or overweight patients with type 2 diabetes: a randomised, controlled, double-blind, ascending dose and phase 2a study. Lancet (2018) 391:2607–18. doi: 10.1016/S0140-6736(18)30726-8
89. Neary NM, Small CJ, Druce MR, Park AJ, Ellis SM, Semjonous NM, et al. Peptide YY3-36 and glucagon-like peptide-17-36 inhibit food intake additively. Endocrinology (2005) 146:5120–7. doi: 10.1210/en.2005-0237
90. Field BC, Wren AM, Peters V, Baynes KC, Martin NM, Patterson M, et al. PYY3-36 and oxyntomodulin can be additive in their effect on food intake in overweight and obese humans. Diabetes (2010) 59:1635–9. doi: 10.2337/db09-1859
91. Neary NM, McGowan BM, Monteiro MP, Jesudason DR, Ghatei MA, Bloom SR. No evidence of an additive inhibitory feeding effect following PP and PYY 3-36 administration. Int J Obes. (2008) 32:1438–40. doi: 10.1038/ijo.2008.95
92. Finan B, Ma T, Ottaway N, Muller TD, Habegger KM, Heppner KM, et al. Unimolecular dual incretins maximize metabolic benefits in rodents, monkeys, and humans. Sci Transl Med. (2013) 5:209ra151. doi: 10.1126/scitranslmed.3007218
93. Frias JP, Bastyr EJ III, Vignati L, Tschop MH, Schmitt C, Owen K, et al. The Sustained Effects of a Dual GIP/GLP-1 Receptor Agonist, NNC0090-2746, in Patients with Type 2 Diabetes. Cell Metab. (2017) 26:343–352 e342. doi: 10.1016/j.cmet.2017.07.011
94. Finan B, Yang B, Ottaway N, Smiley DL, Ma T, Clemmensen C, et al. A rationally designed monomeric peptide triagonist corrects obesity and diabetes in rodents. Nat Med. (2015) 21:27–36. doi: 10.1038/nm.3761
95. Korner J, Inabnet W, Febres G, Conwell IM, McMahon DJ, Salas R, et al. Prospective study of gut hormone and metabolic changes after adjustable gastric banding and Roux-en-Y gastric bypass. Int J Obes. (2009) 33:786–95. doi: 10.1038/ijo.2009.79
96. Laferrere B, Swerdlow N, Bawa B, Arias S, Bose M, Olivan B, et al. Rise of oxyntomodulin in response to oral glucose after gastric bypass surgery in patients with type 2 diabetes. J Clin Endocrinol Metab. (2010) 95:4072–6. doi: 10.1210/jc.2009-2767
97. Falken Y, Hellstrom PM, Holst JJ, Naslund E. Changes in glucose homeostasis after Roux-en-Y gastric bypass surgery for obesity at day three, two months, and one year after surgery: role of gut peptides. J Clin Endocrinol Metab. (2011) 96:2227–35. doi: 10.1210/jc.2010-2876
Keywords: gut hormones, obesity, GLP-1, Oxyntomodulin, glucagon, gastric bypass surgery, diabetes mellitus
Citation: Hope DCD, Tan TMM and Bloom SR (2018) No Guts, No Loss: Toward the Ideal Treatment for Obesity in the Twenty-First Century. Front. Endocrinol. 9:442. doi: 10.3389/fendo.2018.00442
Received: 19 December 2017; Accepted: 17 July 2018;
Published: 15 August 2018.
Edited by:
Jeff M. P. Holly, University of Bristol, United KingdomReviewed by:
Claire Joanne Stocker, University of Buckingham, United KingdomJens Juul Holst, University of Copenhagen, Denmark
Copyright © 2018 Hope, Tan and Bloom. This is an open-access article distributed under the terms of the Creative Commons Attribution License (CC BY). The use, distribution or reproduction in other forums is permitted, provided the original author(s) and the copyright owner(s) are credited and that the original publication in this journal is cited, in accordance with accepted academic practice. No use, distribution or reproduction is permitted which does not comply with these terms.
*Correspondence: Tricia M. M. Tan, dC50YW5AaW1wZXJpYWwuYWMudWs=