- 1Section of Endocrinology, Diabetes and Metabolism, University of Chicago, Chicago, IL, United States
- 2Synthonics Inc., Blacksburg, VA, United States
- 3Department of Chemistry, Virginia Tech, Blacksburg, VA, United States
The standard of care to treat hypothyroidism is daily administration of levo-thyroxine (LT4). This is based on the understanding that deiodinases can restore production of T3 and compensate for the small amounts of T3 that are normally produced by the thyroid gland. However, pre-clinical and clinical evidence indicating that deiodinases fall short of restoring T3 production is accumulating, opening the possibility that liothyronine (LT3) might have a role in the treatment of some hypothyroid patients. LT3 tablets taken orally result in a substantial peak of circulating T3 that is dissipated during the next several hours, which is markedly distinct from the relative stability of T3 levels in normal individuals. Thus, the effort to developing new delivery strategies for LT3, including slow release tablets, liquid formulations, use of T3-related/hybrid molecules such as T3 sulfate, poly-zinc-T3 and glucagon-T3, nanoparticles containing T3, subcutaneous implant of T3-containing matrices, and stem cells for de novo development of the thyroid gland. This article reviews these strategies, their applicability in animal models and translatability to humans.
Introduction
Hypothyroidism is a common disorder that in most cases results from insufficient activity of the thyroid gland (1). The thyroid secretion contains both thyroxine (T4) and triiodothyronine (T3), with the latter being the active form of thyroid hormone (TH). It is estimated that healthy adult subjects produce approximately 100 ug T4 daily; the daily production rate of T3 is approximately 30 ug, of which only about 5 ug are secreted from the thyroid, with the remaining 25 ug produced via T4 deiodination outside of the thyroid parenchyma (2). During hypothyroidism, the reduction in circulating TH levels is detected by the hypothalamus-pituitary-thyroid (HPT), hence elevating serum TSH and defining the diagnosis of hypothyroidism (3). From the time it was originally described in the late 18th century until the 1970s, hypothyroidism was treated predominantly with desiccated thyroid extract, which contains both T4 and T3 (4). The discovery that humans convert T4 to T3 and the development of a TSH immunoassay that could be used to titrate the thyroid replacement dose, levothyroxine (LT4), became the standard of care and the need for supplementation with LT3 obviated; more recently combined therapy with LT4 and LT3 has been the subject of much discussion and controversy (4).
The thyroid function is activated by the pituitary hormone TSH, which is under direct hypothalamic stimulation via TRH and inhibition via a feed-back negative mediated by circulating T4 and T3, each playing independent roles (5). Plasma T3 is detected directly by the hypothalamic paraventricular nucleus, where TRH is produced, and in the pituitary thyrotrophes, where TSH is secreted. In contrast, in order to slow down expression of TRH and TSH, plasma T4 requires local conversion to T3 via the type 2 deiodinase (D2), which is present in the hypothalamus and in the anterior pituitary gland (6). The independent role of T4 is observed as serum TSH increases with the drop in serum T4 during iodine deficiency or mild hypothyroidism, while circulating T3 remains within normal range (7, 8). In turn, the acute administration of large doses of PTU to thyroidectomized individuals kept on L-T4-replacement therapy revealed how serum T3 per se has an important role in TSH secretion (9). The approximately 20% drop in serum T3 that follows as a result of D1 inhibition is sufficient to double serum TSH levels, even as serum T4 levels remain stable (9).
TH signaling is initiated via binding of T3 to nuclear receptors (TR) (10). Based on the affinity of TRs for T3, it is considered that normal circulating levels of T3 account for the bulk of TH signaling in target tissues (11). In some organs, however, the activity of local deiodinases can modulate TH signaling provided by plasma T3. Tissues that express D2 such as brain, pituitary gland and brown adipose tissue, have enhanced TH signaling because D2 produces T3 locally, which adds to the incoming T3 from circulation. In contrast, TH signaling is dampened in tissues expressing the type 3 deiodinase (D3), such as placenta, pancreatic beta cells, skin, which inactivates both T4 and T3 (11). Given that tissues and plasma T3 are in equilibrium, deiodinases produce most circulating T3 and play a role in maintaining circulating levels of T3 relatively stable. Notwithstanding, the thyroid gland also sustains plasma T3 levels as seen in animal models of deiodinase deficiency (12, 13). In fact, TSH acts on the thyroid by preferentially accelerating T3 secretion (over T4) (14–16). For example, the minimal circadian rhythmicity observed in plasma T3 levels is thought to result from an elevation in circulating TSH in the early morning hours [see (11) for review].
The standard of care for hypothyroidism is treatment with levothyroxine (LT4) that is adjusted based on serum TSH levels. The goal is to give patients sufficient amounts of LT4 to bring serum TSH within the normal reference range (3). However, since the early 70s it became apparent that therapy with LT4 results in significantly higher T4 and lower T3 serum levels, what was attributed to the absence of thyroidal T3 secretion (17). Nevertheless, a later study from the same group reported that LT4-treated patients have normal T3 and significantly higher T4 serum levels (18). In addition, a non-cross sectional study that looked at serum T3 levels in 50 euthyroid individuals before and after thyroid surgery found that therapy with LT4 can restore both circulating TSH and T3 levels (19). Hence the expectation that in LT4-treated patients the deiodinase system will metabolize LT4 and produce T3 in amounts equivalent to what is produced by the healthy thyroid gland. However, there is evidence indicating that this indeed might not be the case (20, 21). In a series of approximately 2,000 LT4-treated hypothyroid patients, serum T3 is relatively lower and serum T4 is relatively higher when compared to control individuals; in about 15% of the patients, serum T3 is below normal range (22). Similar findings were obtained through the analyzes of publically available NHANES data, showing that approximately 500 individuals maintained on LT4 have lower serum T3 levels when compared with control individuals matched for age, sex, ethnic background and serum TSH (23).
Preclinical studies of LT4-treated thyroidectomized rats indicate that minimally reduced plasma T3 levels are sufficient to cause widespread dampening in TH signaling, including in the liver, skeletal muscle and brain (24). There is also evidence that TH signaling might not be fully normalized in LT4-treated hypothyroid patients. For example, in association studies LT4-treated patients that have a normal serum TSH weigh about 10 pounds more despite ingesting less calories; they report less physical activity and are more likely to be on statins and anti-depressive therapy (23). A meta-analysis of LT4-treated patients revealed that both total cholesterol and LDL cholesterol remains elevated despite serum TSH that was within the reference range (25). In fact, these findings go along with the observation that non-objective symptoms of hypothyroidism remain in about 15–20% of the LT4-treated hypothyroid patients (difficulty with weight management, low energy, depressed mood, and memory impairment) despite achieving normal TSH and free T4 levels (26, 27). However, progress in this area is slow given the multiple biases which impact on subject self-reported quality of life that are not thyroid related.
The use of liothyronine (LT3) in combination with LT4 to treat hypothyroidism is not frequent but it is popular among patients that, despite normal circulating TH and TSH, experience residual symptoms (28). However, available oral LT3 preparations result in rapid T3 absorption that is followed by fast metabolization (29). This typically results in a peak of serum T3 at about 3h after dosing, which is followed by a relatively fast decline in serum T3 levels (29–31) (Figure 1). In patients taking 10 ug of LT3, the serum T3 peak is approximately 40% above the baseline levels (32); multiple daily doses might partially mitigate this problem but its practicality is a challenge. The peak of serum T3 observed after oral administration of LT3 is not physiological and has given pause to physicians that wish to prescribe LT3. Thus, the American Thyroid Association recommends “against the routine use of combination treatment with LT4 and LT3 as a form of thyroid replacement therapy in patients with primary hypothyroidism” (3). Subsequently, in an attempt to identify adverse outcomes for patients on LT3 tablets, cardiovascular, skeleton, and mental outcomes were assessed through an observational study in the Scottish town of Tayside (33). Compared to the about 34,000 patients taking only LT4, those using LT4 plus LT3 (n = 327) or LT3 alone (n = 73) had no increased mortality or morbidity risk due to cardiovascular disease, atrial fibrillation, or fractures after adjusting for age; the number of prescriptions for bisphosphonates or statins were also similar. A novel finding was an increased risk of new prescriptions for antipsychotic medication, proportional to the number of LT3 prescriptions (33). However, prospective safety studies to evaluate potential long term undesirable cardiovascular and skeleton associated with such pharmacokinetics (PK) have not been performed (34).
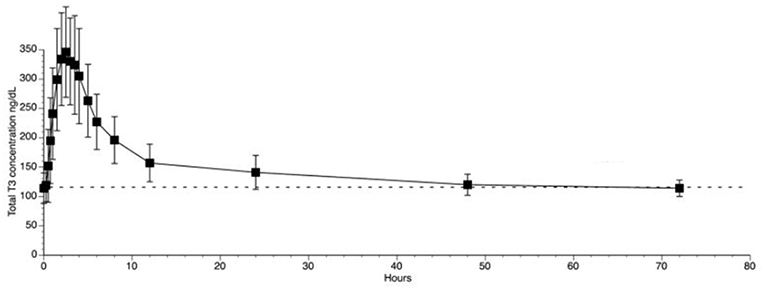
Figure 1. T3 serum concentrations for 3 days following oral administration of a single 50 mcg dose of liothyronine to volunteers. Adapted from Jonklaas et al. (30).
Delivery Systems That Aim at Physiological Replacement of T3
The utilization of animal models to develop systems that restore physiological levels of T3 has two main purposes. First, to study thyroid economy and TH action in other species. This is justified because hypothyroidism not only affects about 5% of the human population but is also frequently observed in other animal species such as dogs and cats (35, 36). Second, to develop delivery systems that eventually could be transitioned/adapted to human use, which has been done mostly in small laboratory rodents such as rats and mice. When studying or using animal models, however, one should be aware that thyroid economy in various species might not emulate what happens in humans (37). For example, rodents secrete relatively more T3 from the thyroid gland (about 40% of the daily production rate) and exhibit an approximately 12–24 times faster turnover rate for both serum T4 and T3 (2). However, when focusing on the absorption kinetics, rodents exhibit a similar PK profile as humans, i.e., a serum peak at 3 h after dosing that is followed by a fast decline in its circulating levels (38).
Enteral/Gastrointestinal Preparations
Oral
Administration of LT3 through the mouth can be utilized to restore TH signaling in laboratory animals that have been made hypothyroid. Adding LT3 to the drinking water or to the diet avoids the administration of a single dose of LT3 and has been shown to restore thyroid status in thyroidectomized rodents (37). However, consistency of dosing and the inevitable circadian rhythmicity associated with food and water intake remain a limiting factor. For example, LT3 supplied in the drinking water (dilution from a stock solution prepared in 40 mM NaOH) was given to different mouse strains aged 6–8 weeks, with variable results. Some strains exhibited complete (>90%) suppression of serum T4 and TSH whereas in others, a much weaker effect was observed. In addition, female and male animals responded differently, and heavier mice had higher post mortem levels of serum T3, which might have been due to increased water intake and/or changes in volume of distribution (39, 40). Notably, liquid preparations for oral administration of LT3 are available for humans (IBSA, Switzerland). Some patients might find it practical to use LT3 solutions, but few studies have been performed to date (41). Only one systematic study exists with this formulation but unfortunately PK profiles were not obtained (41).
New platforms continue to be developed to improve dosing flexibility and PK properties for oral administration of LT3. An original concept is the utilization of thermal inkjet (TIJ) 2D printing to enable the deposit of LT3 and/or LT4 onto orodispersible films (ODFs) (42). In a recent study, a two-cartridge TIJ printer was used to print separate solutions of LT3 and LT4. Dose adjustments allowed for LT3 (15–50 μg) and LT4 dosages (60–180 μg) to be successfully printed onto ODF. When placed in water, ODFs disintegrated in less than 45s (42). PK studies have not been performed but the prospect of fine customization of LT4 and LT3 dosages is exciting. Furthermore, it is conceivable that the utilization of different ODFs could effectively modify the PK properties of orally administered LT3.
An alternative approach to improve LT3 PK properties when given orally is to delay its absorption. This has been used for LT3 or combinations of LT4+LT3. For example, “slow release” LT3 tablets containing a hydrophilic swellable matrix system made with hydroxypropylmethylcellulose, sodium carboxymethylcellulose, calcium phosphate and magnesium stearate have been prepared (US Patent #5,324,522). Other combinations of salt and matrices have also been tested, including mannitol, magnesium stearate, calcium phosphate, and microporous polypropylene (US Patent #5,324,522). When tested in vitro the rate of LT3 release from such capsules can be modulated according to their content and grade of Methocel, and/or SimpleCap/Lactose (43). In vivo tests were also performed. In one of the two clinical studies performed to date, slow release LT3 tablets were given to 17 hypothyroid individuals. The results indicated that slowing down the release of LT3 in the intestine decreases the peak T3 in the serum (Cmax) by about 9% and prolonged the time to Cmax from ~3.2 to 5 h (44). However, in the other study in which LT3 tablets were prepared with microcrystalline cellulose and magnesium stearate (BCT303), sustained serum T3 levels were not observed (30). Another approach still under testing is to deliver LT3 via chewable ion exchange resin that form a drug resin complex (Spectrix, Southlake TX). This technology utilizes ion exchange resin to form a multi-particulate drug-resin complex that could potentially provide an enhanced drug release profile and PK profile in humans, but clinical studies have yet to be performed.
To meet the challenge of creating sustained and/or slow release delivery systems for LT3, investigators utilized T3 derivatives. For example, it is well-known that phenolic hydroxyl within the T3 molecule can be sulfated (T3-S), a reaction that inactivates T3 but dramatically enhances its solubility in water and loss to the environment. However, sulfatases in the liver can reactivate T3-S via de-sulfation and prevent its loss to the environment (45). Studies in hypothyroid rats revealed that substantial amounts of parentally administered T3-S were converted back to T3, triggering systemic thyromimetic effects (46). Similar studies were conducted in 28 thyroidectomized individuals given T3-S orally. Tmax for T3-S was between 3 and 4 h. At the same time, T3 levels (generated through T3-S de-sulfation) increased rapidly in the circulation, with an early peak between 2 and 4 h that was followed by a variable plateau that depended on the dose of T3-S administered and lasted up to 48 h. These observations suggest that sufficient amounts of orally administered T3-S are converted to T3 in humans and could be tested as a tool to restore T3 levels in hypothyroid individuals (47). However, studies in euthyroid individuals have not been conducted. This is important because T3-S is metabolized through the D1 pathway through inner ring deiodination, which leads to irreversible inactivation of the T3 molecule (45). In fact, D1 is expressed in such high levels in the liver and kidney that it has been proposed that D1 plays a scavenger role by minimizing the loss of iodine through urine and bile (48). This is critical given that D1 is a T3-responsive gene, hence its levels are low in tissues of hypothyroid patients. Studies could be conducted in hypothyroid individuals after thyroid status was normalized with LT4 to ensure that at the normal D1 levels there is sufficient amounts of T3-S to be de-sulfated to T3. Nevertheless, this remains an ingenious strategy that should be pursued further.
Another T3 derivative was prepared using metal coordination, which resulted in LT3 polymers with Zinc. Poly-Zinc-Liothyronine (PZL) is a copolymer of zinc and T3 that forms a supramolecular complex (Figure 2). These supramolecular complexes of the form [M(T3)]n have superior mucoadhesive properties, and when coupled with the hydrolysis behavior of [M(T3)]n complexes, translate into a slow release formulation of LT3 (38). The properties of a controlled release, orally delivered drug product via metal coordination of a drug ligand, relies on known principles of mucoadhesion and coordination chemistry (49). For illustrative purposes only, consider PZL as a prodrug. Then the process of modified drug release and absorption can be seen to involve three distinct steps: (a) Mucoadhesion of PZL to an area of the gastrointestinal tract, (b) Controlled ligand exchange (e.g., hydrolysis) of T3 from PZL, followed by c) Drug absorption of the LT3. Many metal complexes, including PZL, exhibit mucoadhesion due to the interaction of the metal, acting as a Lewis acid, with anionic components of the mucosa (Figure 3) (50). Mucoadhesion prolongs the residence time (i.e., delays transit time) of a drug in the gastrointestinal tract (51). PZL interacts with the mucosa by a variety of additional mechanisms, including coordinate covalent bonding, hydrogen bonding, halogen bonding, metal- halogen bonding, electrostatic interactions and particle size (52–55). Thus, PZL adheres to the intestinal lining creating a “drug depot” from which LT3 gradually releases into the intestinal lumen and is ultimately absorbed into the bloodstream (Figure 4). Intestinal contents, such as bile acids and pancreatic secretion, accelerate breaking of the bonds between the metal and T3. Once freed from the metal, the released T3 reverts to its original form and quickly travels through the epithelial cell layer and into the blood stream. These processes increase the length of time PZL remains in the intestine and slows down the rate at which LT3 becomes available for absorption. Of note, Zn is an essential mineral involved in multiple physiological functions and the amount contained in a 30 ug dose of PZL is <1/1,000 of the daily recommended allowance.
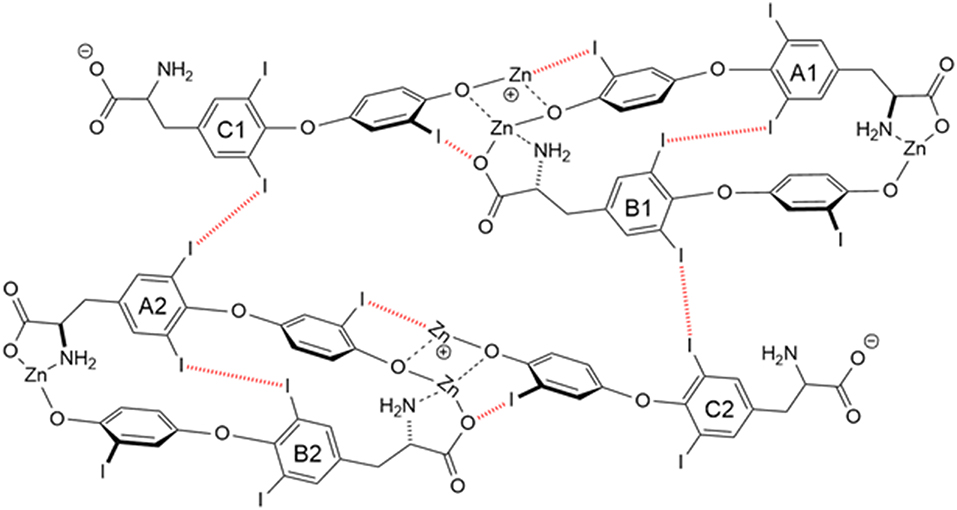
Figure 2. Strong bonding interactions (coordinate covalent bonds) between Zn and ligand donor atoms of T32- are shown in black; weak bonding interactions (halogen bonds) between iodine and X-bond acceptor atoms of T32- are shown in red dashed line. Both bonding modes contribute to polymer formation and stabilization. Two and 3-D structures, including metal organic frameworks, are possible.
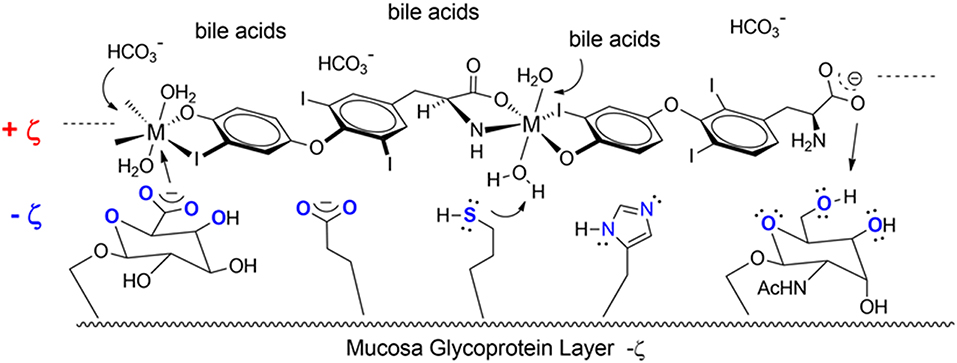
Figure 3. Endogenous ligands in the upper GI tract can affect hydmlysis rate. These include HCI (stomach), bile acids and carbonate buffers (upper intestines).
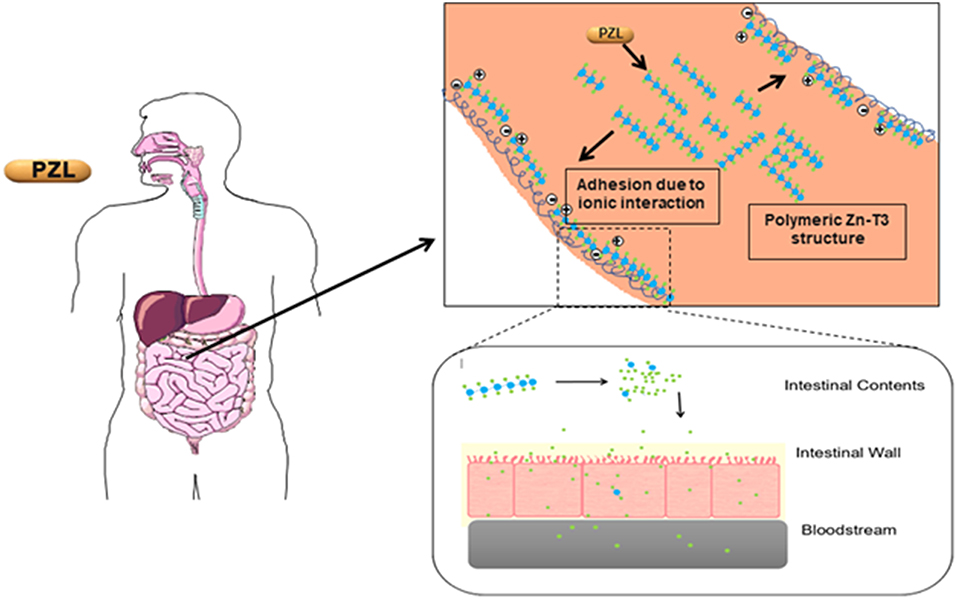
Figure 4. Increasing the period PZL remains at the site of absorption and slowing the rate at which T3 becomes available for absorption, more closely matches T3 production in a healthy subject. By creating a unique “drug depot” from which T3 slowly releases, PZL addresses many of the limitations that have plagued previous attempts to mimic the natural production of T3 in healthy subjects.
Capsules of PZL were tested in hypothyroid rats via oral gavage, with a significant modification in PK properties (38). After PZL administration serum T3 exhibited about 30% lower peak (lower Cmax) that was delayed (longer Tmax) by about 6h as compared to rats given equimolar amounts of LT3. These figures could be further improved by packaging PZL in slow release capsules made with hydrophilic swellable matrix as described above for LT3 (44); the T3 clearance rate did not show differences between PZL- and LT3-treated rats. TSH levels, which were elevated, declined rapidly after LT3 administration but in PZL-treated rats the decline was delayed by about 4 h (Figure 5). Daily administration of PZL for 8 days showed that PZL and LT3 had similar long-term biological effects such as reduction of serum cholesterol, restauration of growth rate and induction of T3-dependent genes in the heart, liver and brain (38).
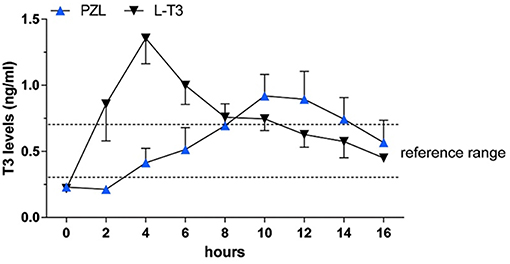
Figure 5. Serum triiodothyronine (T3) levels in hypothyroid rats given 24 μg/kg body weight LT3 or equimolar amounts of PZL through gavage; adapted fivm Da Conceicao et al. (38).
Rectal
The walls of the rectum are lined with a mucosa that is highly vascularized, allowing for rapid absorption of medications. Formal PK studies after the administration of the LT4 suppository have been performed in thyroidectomized rats (56). Tmax is at about 15 h, similar to when LT4 is given orally, but the area under the curve is about 3 times smaller indicating incomplete absorption. Similar results were obtained in hypothyroid patients (56). Unfortunately, no such studies have been performed with LT3 but should be explored.
Parenteral Preparations
Subcutaneous
One of the most common forms of TH replacement in small rodents is the subcutaneous injection of aqueous solutions of LT3. While this is a very reliable way of delivering exact amounts of LT3, its absorption is relatively fast, followed by a peak of LT3 in the circulation. Peaks can be minimized by splitting the dose into multiple injections every 24 h, but with a half-life of ~2 h, the peaks of T3 in the circulation will inevitably remain (37). A more stable T3 profile could in theory be achieved by injecting an oil suspension of LT3, which should slow down its absorption rate, but formal PK studies remain to be done.
The gold standard in parenteral preparations for animals is the use of osmotic pumps or pellets that are implanted subcutaneously, releasing fixed amounts of T3 daily for a pre-defined number of days. The pump is a small cylindrical reservoir that uses osmotic driving agent to release a pharmaceutical of interest at a rate of 0.1 to 10 ul/h up to 6 weeks. Both the pumps and the pellets are suitable to be surgically implanted in rodents as well as in larger animals (37). Osmotic minipumps (Alzet, Cupertino CA) operate due to a difference in osmotic pressure between the pump and the subcutaneous area where the pump is implanted. The higher osmolality of the pump flux water through a semipermeable membrane into the pump. As the water builds up into the pump, it compresses the reservoir, displacing the pharmaceutical solution into the subcutaneous at a known rate (Figure 6). The pellet system (Innovative Research of America, Sarasota FL) consists of a pellet with a biodegradable matrix that continuously releases the pharmaceutical product in the animal. Pellets vary in diameter (1/8″ to 1/2″) depending on the pharmaceutical load and release rate. Pellets might contain from 0.001 mg up to 200 mg, which is released over a predefined period of time, e.g., 21 days, 60 days or 90 days. Thyroidectomized rodents implanted with either osmotic pumps or pellet devices do exhibit rather stable levels of T3 in the circulation (37).
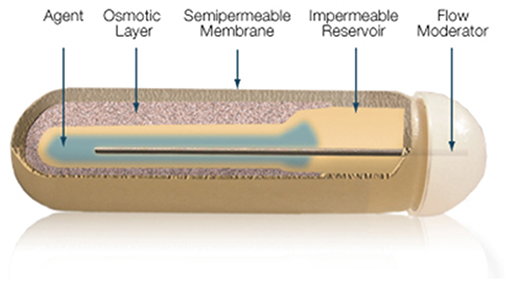
Figure 6. Mechanism of ALZET osmotic pumps which relies on the osmotic pressure difference between pump's compartments. Reproduced from www.Alzet.com.
Similar technology is being developed by pharmaceutical companies which could provide stable delivery of LT3 to patients and be applicable in the treatment of hypothyroidism. Titan Pharmaceuticals (South San Francisco CA) utilizes a proprietary technology (ProNeura®) to develop a platform to deliver LT3 subcutaneously. It consists of a solid rod made with a mixture of LT3 and ethylene-vinyl acetate that is placed subcutaneously, normally in the inner part of the upper arm, and can be removed at the end of the treatment period. Preliminary studies have been performed in thyroidectomized rats and in intact beagle dogs (57). LT3 implants continuously released T3 in both species, in a dose dependent manner for over 6 months. Following implantation, there was a transient serum peak of T3 followed by a sustained period of relatively stable circulating levels of T3 (57).
MedinCell (Montpellier, France) takes advantage of the fact that copolymers combined with pharmaceuticals are solubilized in a biocompatible solvent, which forms a fully bioresorbable depot once injected subcutaneously. BEPO® consists of copolymers containing hydrophilic blocks (polyethylene glycol—PEG) linked with hydrophobic blocks (Poly(D,L-lactic acid)—PLA), which precipitate and create a depot when placed an aqueous environment. The pharmaceutical is trapped within this matrix and later released by diffusion. The kinetics of pharmaceutical release can be fine-tuned by adjusting the hydrophilicity and relative ratio of the copolymers. Preliminary studies are not available but this technology could also potentially provide a steady release of LT3 molecules for days, weeks or months.
Intravenous and intraperitoneal
Both routes have been used to administer LT3 dissolved in aqueous solutions but in both cases a major peak of T3 in the circulation occurs (37).
Regenerative Approaches
Thyroid transplant was one of the first approaches to treat hypothyroidism, as many patients improved after receiving animal (sheep or goat) or human thyroid glands (from patients with Graves' disease or goiter) (4). Despite early successes this form of treatment was abandoned as symptoms of hypothyroidism kept recurring. More recently, a method was described that generate thyroid cells from stem cells by overexpressing the transcription factors NKX2.1 and PAX8 in mouse embryonic stem-cells (mESC) (58). This leads to fully differentiated follicular cells that express thyroid-specific markers such as TSH receptor, sodium/iodide symporter NIS and thyroglobulin. When exposed to human TSH, these cells organize with a three dimensional follicular architecture. These structures were next grafted under the kidney capsules of hypothyroid mice to examine their in vivo functionality. Serum FT4 became detectable after 4 weeks of transplant along with a reduction in serum TSH levels when compared to levels before grafting (58). Similar experiments were performed by other groups and, as a whole, it is clear that hypothyroid mice can be made euthyroid by transplanting functional thyroid follicular constellations (59). Whether this will ever be feasible to be utilized in humans remains to be seen. Patients complaining of persistent hypothyroidism symptoms could benefit from a new thyroid gland and physiological secretion of both T3 and T4.
Tissue Targeting of LT3
Substantial progress on LT3 delivery technology has been achieved recently with the development of tissue-specific targeting of T3 molecules. For example, engineered chemical conjugates of glucagon and T3 enabled delivery of T3 to the liver (60). Treatment with this conjugate corrected hyperlipidemia, steatohepatitis, atherosclerosis, glucose intolerance, and obesity in mouse models of obesity. Liver-directed T3 action spared the cardiovascular system from adverse T3 effects. These findings support the therapeutic utility of integrating T3 and a second hormone into a single molecular entity in order to obtain tissue-specific effects of T3 (60).
Nanotechnology, which involves manipulation of matter sized from 1 to 100 nm, is another tool that has been utilized to achieve tissue-specific delivery of LT3. Nanoparticles containing LT3 have been manufactured using poly(ethylene imine) (PEI) complexed with dodecanoic acid (PEI-C12) with a lamellar nanostructure and a repeat unit of 2.9 nm. In this context PEI-C12 functions as a guest matrix that dissolves LT3 without crystallization (61). This approach was used to test the hypothesis that efficacy of T3 delivery to the brain can be enhanced by encapsulation in nanoparticulate vehicles (62). T3 was encapsulated in poly-(lactide-co-glycolide)-polyethyleneglycol (PLGA-b-PEG) nanoparticles that were coated with glutathione, an efficient means of brain targeted drug delivery. Efficiency of T3 delivery was tested by measuring its ability to protect against ischemic damage in middle cerebral artery occlusion model of ischemic brain stroke. Indeed, administering T3 in nanoparticulate form resulted in significant benefit over injection of a LT3 solution (62).
Conclusion
LT3 is commercially available as sodium salt packaged in tablets that result in rapid duodenal availability and absorption (Table 1). The development of slow release formulations that could be used in humans has been in the works for years. Claims of slow release LT3 formulations based on changing the composition of the tablets have not been confirmed in clinical trials or have only minimally affected Cmax and Tmax. A number of new strategies and compounds are being explored but details aren't always available due to commercial interests and protection of intellectual property. So far, T3-S and PZL have shown promising results in animal models but formal phase-1 clinical trials have not been conducted. There is no doubt that the momentum around developing new delivery methods of LT3 for humans is building. This will pave the road to better understand and evaluate the use of LT3/LT4 combination therapy and possibly improve patients' quality of life.
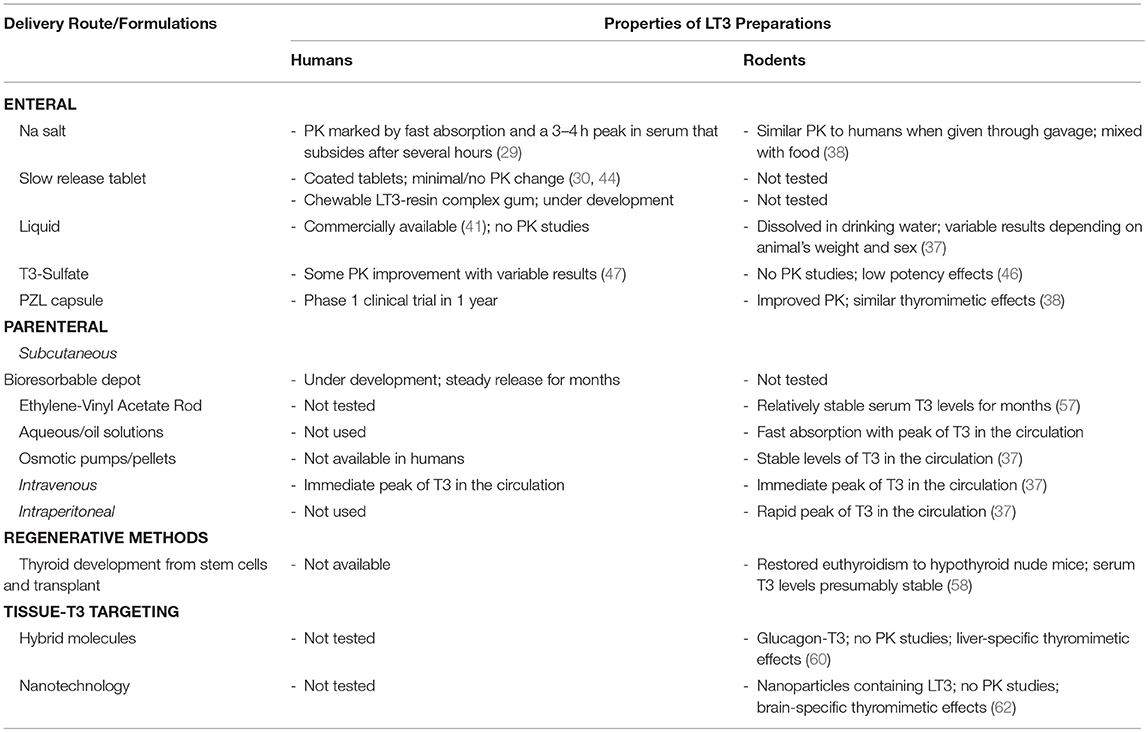
Table 1. Developmental stage of different LT3 products, their main properties and PK studies in rodents and humans.
Author Contributions
TI performed a bibliographic search and compiled a draft of the manuscript. JP and TP worked on the section on PZL and metal coordination. AB coordinated all work and edited the whole manuscript.
Conflict of Interest Statement
TI is the President and Chief Science Officer at Synthonics and Adjunct Faculty at Virginia Tech's Department of Chemistry; JP is Vice President of Research and Development at Synthonics; AB is a consultant for Synthonics Inc, Allergan Inc, and BLA Technology LLC; he served as consultant for Sentier LLC during 2018.
The remaining author declares that the research was conducted in the absence of any commercial or financial relationships that could be construed as a potential conflict of interest.
Acknowledgments
This work supported by NIDDK 2 R44 DK116396-02A1.
Abbreviations
AUC, area under the curve; Cmax, maximum concentration; D2, type 2 deiodinase; D3, type 3 deiodinase; FT4, free thyroxine; FT3, free triiodothyronine; HR, (place in column) heart rate; HPT, hypothalamus-pituitary-thyroid; LT3, liothyronine; LT4, levothyroxine; mESC, mouse embryonic stem-cells; PZL, Poly-Zinc-Liothyronine; PK, Pharmacokinetics; T3S, T3 sulfate; T3, triiodothyronine; T4, thyroxine; Tmax, time to maximum concentration; TSH, thyroid stimulating hormone.
References
1. Chaker L, Bianco AC, Jonklaas J, Peeters RP. Hypothyroidism. Lancet. (2017) 390:1550–62. doi: 10.1016/S0140-6736(17)30703-1
2. Bianco AC, Salvatore D, Gereben B, Berry MJ, Larsen PR. Biochemistry, cellular and molecular biology, and physiological roles of the iodothyronine selenodeiodinases. Endocrine Rev. (2002) 23:38–89. doi: 10.1210/edrv.23.1.0455
3. Jonklaas J, Bianco AC, Bauer AJ, Burman KD, Cappola AR, Celi FS, et al. Guidelines for the treatment of hypothyroidism: prepared by the american thyroid association task force on thyroid hormone replacement. Thyroid Offic J Am Thyroid Assoc. (2014) 24:1670–751. doi: 10.1089/thy.2014.0028
4. McAninch EA, Bianco AC. The history and future of treatment of Hypothyroidism. Ann Inter Med. (2016) 164:50–6. doi: 10.7326/M15-1799
5. Fekete C, Lechan RM. Central regulation of hypothalamic-pituitary-thyroid axis under physiological and pathophysiological conditions. Endocrine Rev. (2014) 35:159–94. doi: 10.1210/er.2013-1087
6. Fonseca TL, Correa-Medina M, Campos MP, Wittmann G, Werneck-de-Castro JP, Arrojo e Drigo R, et al. Coordination of hypothalamic and pituitary T3 production regulates TSH expression. J Clin Invest. (2013) 123:1492–500. doi: 10.1172/JCI61231
7. Riesco G, Taurog A, Larsen PR, Krülich L. Acute and chronic responses to iodine deficiency in rats. Endocrinology. (1977) 100:303–13. doi: 10.1210/endo-100-2-303
8. Santisteban P, Obregon MJ, Rodriguez-Pena A, Lamas L, Del Rey FE, De Escobar GM. Are iodine-deficient rats euthyroid? Endocrinology. (1982) 110:1780–9. doi: 10.1210/endo-110-5-1780
9. Geffner DL, Azukizawa M, Hershman JM. Propylthiouracil blocks extrathyroidal conversion of thyroxine to triiodothyronine and augments thyrotropin secretion in man. J Clin Invest. (1975) 55:224–9. doi: 10.1172/JCI107925
10. Brent GA. Mechanisms of thyroid hormone action. J Clin Invest. (2012) 122:3035–43. doi: 10.1172/JCI60047
11. Bianco AC, Dumitrescu A, Gereben B, Ribeiro MO, Fonseca TL, Fernandes GW, et al. Paradigms of dynamic control of thyroid hormone signaling. Endocrine Rev. (2019) 40:1000–47. doi: 10.1210/er.2018-00275
12. Christoffolete MA, Arrojo e Drigo R, Gazoni F, Tente SM, Goncalves V, Amorim BS, et al. Mice with impaired extrathyroidal thyroxine to 3,5,3′-triiodothyronine conversion maintain normal serum 3,5,3′-triiodothyronine concentrations. Endocrinology. (2007) 148:954–60. doi: 10.1210/en.2006-1042
13. Galton VA, Schneider M, Clark AS, Germain DL. Life without T4 to T3 conversion: studies in mice devoid of the 5′-deiodinases. Endocrinology. (2009) 150:2957–63. doi: 10.1210/en.2008-1572
14. Citterio CE, Morishita Y, Dakka N, Veluswamy B, Arvan P. Relationship between the dimerization of thyroglobulin and its ability to form triiodothyronine. J Biol Chem. (2018) 293:4860–9. doi: 10.1074/jbc.RA118.001786
15. Citterio CE, Veluswamy B, Morgan SJ, Galton VA, Banga JP, Atkins S, et al. De novo triiodothyronine formation from thyrocytes activated by thyroid-stimulating hormone. J Biol Chem. (2017) 292:15434–44. doi: 10.1074/jbc.M117.784447
16. Di Jeso B, Arvan P. Thyroglobulin from molecular and cellular biology to clinical endocrinology. Endocrine Rev. (2016) 37:2–36. doi: 10.1210/er.2015-1090
17. Stock JM, Surks MI, Oppenheimer JH. Replacement dosage of L-thyroxine in hypothyroidism. A re-evaluation. N Engl J Med. (1974) 290:529–33. doi: 10.1056/NEJM197403072901001
18. Fish LH, Schwartz HL, Cavanaugh J, Steffes MW, Bantle JP, Oppenheimer JH. Replacement dose, metabolism, and bioavailability of levothyroxine in the treatment of hypothyroidism. N Engl J Med. (1987) 316:764–70. doi: 10.1056/NEJM198703263161302
19. Jonklaas J, Davidson B, Bhagat S, Soldin SJ. Triiodothyronine levels in athyreotic individuals during levothyroxine therapy. JAMA. (2008) 299:769–77. doi: 10.1001/jama.299.7.769
20. McAninch EA, Bianco AC. New insights into the variable effectiveness of levothyroxine monotherapy for hypothyroidism. Lancet Diabetes Endocrinol. (2015) 3:756–8. doi: 10.1016/S2213-8587(15)00325-3
21. Gereben B, McAninch EA, Ribeiro MO, Bianco AC. Scope and limitations of iodothyronine deiodinases in hypothyroidism. Nat Rev Endocrinol. (2015) 11:642–52. doi: 10.1038/nrendo.2015.155
22. Gullo D, Latina A, Frasca F, Le Moli R, Pellegriti G, Vigneri R. Levothyroxine monotherapy cannot guarantee euthyroidism in all athyreotic patients. PLoS ONE. (2011) 6:e22552. doi: 10.1371/journal.pone.0022552
23. Peterson SJ, McAninch EA, Bianco AC. Is a Normal TSH Synonymous with “Euthyroidism” in Levothyroxine Monotherapy? J Clin Endocrinol Metabol. (2016) 2016:jc20162660. doi: 10.1210/jc.2016-2660
24. Werneck de Castro JP, Fonseca TL, Ueta CB, McAninch EA, Abdalla S, Wittmann G, et al. Differences in hypothalamic type 2 deiodinase ubiquitination explain localized sensitivity to thyroxine. J Clin Invest. (2015) 125:769–81. doi: 10.1172/JCI77588
25. McAninch EA, Rajan KB, Miller CH, Bianco AC. Systemic thyroid hormone status during levothyroxine therapy in hypothyroidism: a systematic review and meta-analysis. J Clin Endocrinol Metabol. (2018) 103:4533–42. doi: 10.1210/jc.2018-01361
26. Saravanan P, Visser TJ, Dayan CM. Psychological well-being correlates with free thyroxine but not free 3,5,3'-triiodothyronine levels in patients on thyroid hormone replacement. J Clin Endocrinol Metabol. (2006) 91:3389–93. doi: 10.1210/jc.2006-0414
27. Saravanan P, Chau WF, Roberts N, Vedhara K, Greenwood R, Dayan CM. Psychological well-being in patients on 'adequate' doses of l-thyroxine: results of a large, controlled community-based questionnaire study. Clin Endocrinol. (2002) 57:577–85. doi: 10.1046/j.1365-2265.2002.01654.x
28. Peterson SJ, Cappola AR, Castro MR, Dayan CM, Farwell AP, Hennessey JV, et al. An online survey of hypothyroid patients captured predominantly dissatis-fied individuals. Thyroid Offic J Am Thyroid Assoc. (2018) 28:707–21. doi: 10.1089/thy.2017.0681
29. Surks MI, Schadlow AR, Oppenheimer JH. A new radioimmunoassay for plasma L-triiodothyronine: measurements in thyroid disease and in patients maintained on hormonal replacement. J Clin Invest. (1972) 51:3104–13. doi: 10.1172/JCI107137
30. Jonklaas J, Burman KD, Wang H, Latham KR. Single-dose T3 administration: kinetics and effects on biochemical and physiological parameters. Ther Drug Monit. (2015) 37:110–8. doi: 10.1097/FTD.0000000000000113
31. Jonklaas J, Burman KD. Daily administration of short-acting liothyronine is associated with significant triiodothyronine excursions and fails to alter thyroid-responsive parameters. Thyroid Offic J Am Thyroid Assoc. (2016) 26:770–8. doi: 10.1089/thy.2015.0629
32. Saravanan P, Siddique H, Simmons DJ, Greenwood R, Dayan CM. Twenty-four hour hormone profiles of TSH, Free T3 and free T4 in hypothyroid patients on combined T3/T4 therapy. Exp Clin Endocrinol Diabetes. (2007) 115:261–7. doi: 10.1055/s-2007-973071
33. Leese GP, Soto-Pedre E, Donnelly LA. Liothyronine use in a 17 year observational population-based study - the tears study. Clin Endocrinol. (2016) 85:918–25. doi: 10.1111/cen.13052
34. Jonklaas J. Risks and safety of combination therapy for hypothyroidism. Expert Rev Clin Pharmacol. (2016) 9:1057–67. doi: 10.1080/17512433.2016.1182019
35. Mooney CT. Canine hypothyroidism: a review of aetiology and diagnosis. N Z Vet J. (2011) 59:105–14. doi: 10.1080/00480169.2011.563729
36. Scott-Moncrieff JC. Clinical signs and concurrent diseases of hypothyroidism in dogs and cats. Vet Clin North Am Small Anim Pract. (2007) 37:709–22, vi. doi: 10.1016/j.cvsm.2007.03.003
37. Bianco AC, Anderson G, Forrest D, Galton VA, Gereben B, Kim BW, et al. American thyroid association guide to investigating thyroid hormone economy and action in rodent and cell models. Thyroid. (2014) 24:88–168. doi: 10.1089/thy.2013.0109
38. Da Conceicao RR, Fernandes GW, Fonseca TL, Bocco B, Bianco AC. Metal coordinated poly-zinc-liothyronine provides stable circulating triiodothyronine levels in hypothyroid rats. Thyroid Offic J Am Thyroid Assoc. (2018) 28:1425–33. doi: 10.1089/thy.2018.0205
39. East-Palmer J, Szkudlinski MW, Lee J, Thotakura NR, Weintraub BD. A novel, nonradioactive in vivo bioassay of thyrotropin (TSH). Thyroid Offic J Am Thyroid Assoc. (1995) 5:55–9. doi: 10.1089/thy.1995.5.55
40. Hamidi S, Aliesky H, Chen CR, Rapoport B, McLachlan SM. Variable suppression of serum thyroxine in female mice of different inbred strains by triiodothyronine administered in drinking water. Thyroid Offic J Am Thyroid Assoc. (2010) 20:1157–62. doi: 10.1089/thy.2010.0117
41. Trimboli P, Centanni M, Virili C. Liquid liothyronine to obtain target TSH in differentiated thyroid cancer patients. Endocr J. (2016) 63:563–7. doi: 10.1507/endocrj.EJ16-0040
42. Alomari M, Vuddanda PR, Trenfield SJ, Dodoo CC, Velaga S, Basit AW, et al. Printing T3 and T4 oral drug combinations as a novel strategy for hypothyroidism. Int J Pharm. (2018) 549:363–9. doi: 10.1016/j.ijpharm.2018.07.062
43. Bakhteyar H, Cassone C, Kohan HG, Sani SN. Kinetic analysis of drug release from compounded slow-release capsules of liothyronine sodium (T3). Int J Pharm Compd. (2017) 21:418–25.
44. Hennemann G, Docter R, Visser TJ, Postema PT, Krenning EP. Thyroxine plus low-dose, slow-release triiodothyronine replacement in hypothyroidism: proof of principle. Thyroid Offic J Am Thyroid Assoc. (2004) 14:271–5. doi: 10.1089/105072504323030924
45. Peeters RP, Visser TJ. Metabolism of thyroid hormone. In: Feingold KR, Anawalt B, Boyce A, Chrousos G, Dungan K, Grossman A, Hershman JM, Kaltsas G, Koch C, Kopp P, Korbonits M, McLachlan R, Morley JE, New M, Perreault L, Purnell J, Rebar R, Singer F, Trence DL, Vinik A, Wilson DP, editors. Endotext. South Dartmouth, MA: MDText.com, Inc. (2000).
46. Santini F, Hurd RE, Lee B, Chopra IJ. Thyromimetic effects of 3,5,3′-triiodothyronine sulfate in hypothyroid rats. Endocrinology. (1993) 133:105–10. doi: 10.1210/endo.133.1.8319558
47. Santini F, Giannetti M, Ricco I, Querci G, Saponati G, Bokor D, et al. Steady state serum T3 concentrations for 48 hours following the oral administration of a single dose of 3,5,3′-Triiodothyronine Sulfate (T3S). Endocrine Prac. (2014) 20:680–9. doi: 10.4158/EP13331.OR
48. Schneider MJ, Fiering SN, Thai B, Wu SY, St Germain E, Parlow AF, et al. Targeted disruption of the type 1 selenodeiodinase gene (dio1) results in marked changes in thyroid hormone economy in mice. Endocrinology. (2006) 147:580–9. doi: 10.1210/en.2005-0739
50. Smart JD. The basics and underlying mechanisms of mucoadhesion. Adv Drug Deliv Rev. (2005) 57:1556–68. doi: 10.1016/j.addr.2005.07.001
51. Shaikh R, Raj Singh TR, Garland MJ, Woolfson AD, Donnelly RF. Mucoadhesive drug delivery systems. J Pharm Bioallied Sci. (2011) 3:89–100. doi: 10.4103/0975-7406.76478
52. Katrusiak A, Katrusiak A. Thyroxine revisited. J Pharm Sci. (2004) 93:3066–75. doi: 10.1002/jps.20155
53. Mondal S, Mugesh G. Conformational flexibility and halogen bonding in thyroid hormones and their metabolites. Cryst Growth Des. (2016) 16:5896–906. doi: 10.1021/acs.cgd.6b00945
54. Parra RD. Concerted halogen bonding and orthogonal metal-halogen interactions in dimers of lithium formamidinate and halogenated formamidines: An ab initio study. Molecules. (2014) 19:1069–84. doi: 10.3390/molecules19011069
55. Tur KM, Ch'ng HS. Evaluation of possible mechanism(s) of bioadhesion. Int J Pharm. (1998) 160:61–74. doi: 10.1016/S0378-5173(97)00297-4
56. Kashiwagura Y, Uchida S, Tanaka S, Watanabe H, Masuzawa M, Sasaki T, et al. Clinical efficacy and pharmacokinetics of levothyroxine suppository in patients with hypothyroidism. Biol Pharm Bull. (2014) 37:666–70. doi: 10.1248/bpb.b13-00998
57. Sreedharan S, Patel R. Long-term sustained delivery of liothyronine (L-T3) with subdermal proneuratm implants. In: 87th Annual Meeting of the American Thyroid Association Vancouver, BC (2017).
58. Antonica F, Kasprzyk DF, Opitz R, Iacovino M, Liao XH, Dumitrescu AM, et al. Generation of functional thyroid from embryonic stem cells. Nature. (2012) 491:66–71. doi: 10.1038/nature11525
59. Kurmann AA, Serra M, Hawkins F, Rankin SA, Mori M, Astapova I, et al. Regeneration of thyroid function by transplantation of differentiated pluripotent stem cells. Cell Stem Cell. (2015) 17:527–42. doi: 10.1016/j.stem.2015.09.004
60. Finan B, Clemmensen C, Zhu Z, Stemmer K, Gauthier K, Muller L, et al. Chemical hybridization of glucagon and thyroid hormone optimizes therapeutic impact for metabolic disease. Cell. (2016) 167:843–57 e14. doi: 10.1016/j.cell.2016.09.014
61. Thunemann AF, General S. Nanoparticles of a polyelectrolyte-fatty acid complex: carriers for Q10 and triiodothyronine. J Control Release. (2001) 75:237–47. doi: 10.1016/S0168-3659(01)00352-2
Keywords: hypothyroidism, liothyronine, combination therapy, animals, thyroid, levothyroxine
Citation: Idrees T, Price JD, Piccariello T and Bianco AC (2019) Sustained Release T3 Therapy: Animal Models and Translational Applications. Front. Endocrinol. 10:544. doi: 10.3389/fendo.2019.00544
Received: 31 May 2019; Accepted: 22 July 2019;
Published: 13 August 2019.
Edited by:
Jacqueline Jonklaas, Georgetown University, United StatesReviewed by:
Rudolf Hoermann, Klinikum Lüdenscheid, GermanyJames Vincent Hennessey, Harvard Medical School, United States
Richard Ross, University of Sheffield, United Kingdom
Copyright © 2019 Idrees, Price, Piccariello and Bianco. This is an open-access article distributed under the terms of the Creative Commons Attribution License (CC BY). The use, distribution or reproduction in other forums is permitted, provided the original author(s) and the copyright owner(s) are credited and that the original publication in this journal is cited, in accordance with accepted academic practice. No use, distribution or reproduction is permitted which does not comply with these terms.
*Correspondence: Antonio C. Bianco, abianco@deiodinase.org