- 1Barts and the London School of Medicine and Dentistry, London, United Kingdom
- 2Imperial College School of Medicine, Imperial College London, United Kingdom
- 3Nuffield Department of Population Health, University of Oxford, Oxford, United Kingdom
- 4Division of Cardiovascular Medicine, Radcliffe Department of Medicine, University of Oxford, Oxford, United Kingdom
- 5National Institute for Health Research Oxford Biomedical Research Centre, Oxford University Hospitals NHS Foundation Trust, Oxford, United Kingdom
- 6MRC Lifecourse Epidemiology Centre, University of Southampton, Southampton, United Kingdom
- 7NIHR Southampton Biomedical Research Centre, University of Southampton and University Hospital Southampton NHS Foundation Trust, Southampton, United Kingdom
- 8William Harvey Research Institute, NIHR Barts Biomedical Research Centre, Queen Mary University of London, London, United Kingdom
- 9Barts Heart Centre, St Bartholomew’s Hospital, Barts Health NHS Trust, West Smithfield, United Kingdom
Objective: Obesity and cardiovascular disease are major global public health problems. Maternal obesity has been linked to multiple adverse health consequences for both mother and baby. Obesity during pregnancy may adversely alter the intrauterine environment, which has been hypothesised to predispose the offspring to poorer cardiovascular health throughout life. In this paper, we systematically review current literature examining the links between maternal obesity and offspring cardiovascular health.
Methods: This study is registered with PROSPERO (CRD42021278567) and was conducted in accordance with the PRISMA guidelines. A comprehensive systematic literature search was conducted, including two electronic databases (Ovid Medline, Embase), cross-referencing, author searching, and grey literature searches. We selected studies exploring the relationship between maternal obesity and offspring cardiovascular health, using pre-defined eligibility criteria. Studies were critically appraised using the ROBINS-I tool.
Results: From 1,214 results, 27 articles met the eligibility criteria. Multiple cardiovascular outcomes were considered, including congenital heart disease, cardiometabolic parameters, and cardiovascular diseases in neonates, children, and adults. In these studies, maternal obesity was consistently associated with congenital heart disease, several adverse cardiometabolic parameters throughout life including higher body mass index and insulin levels, and greater risk of cardiovascular disease in adulthood. Hypothesized underlying mechanisms are complex and multifactorial comprising genetic, environmental, and socioeconomic components, which can be difficult to quantify. Heterogeneity in study designs, highly selected study samples, and high risk of bias in some studies limit conclusions regarding causality.
Conclusions: We identified consistent evidence of links between maternal obesity and poorer offspring cardiovascular health throughout the lifecourse, extending from the neonatal period into adulthood. Although underlying mechanisms are unclear, our findings support consideration of targeted maternal obesity prevention for promotion of offspring cardiovascular health. This all-encompassing systematic review provides critical appraisal of the latest evidence, defines gaps and biases of existing literature, and may inform potential new public health strategies for cardiovascular disease prevention.
Systematic Review Registration: [https://www.crd.york.ac.uk/prospero], identifier PROSPERO (CRD42021278567).
Introduction
The World Health Organisation (WHO) has characterised the rising prevalence of obesity as an epidemic. As a risk factor for a multitude of diseases, obesity poses a major global public health challenge (1).
The prevalence of obesity in women of reproductive age is increasing alongside that of the general population (2). Current estimates suggest that 30% of reproductive age women in the UK are obese (3). Maternal obesity is a risk factor for numerous pregnancy complications, including miscarriage, pre-eclampsia, and gestational diabetes mellitus (GDM). Obesity is also a major risk factor for the leading causes of maternal death: cardiovascular disease (CVD) and pulmonary embolism (4, 5). Indeed, between 2015-2017, almost 60% of the women who died within the first 6 weeks post-partum were overweight or obese (5). Maternal obesity also increases the risk of still-birth and neonatal mortality and is associated with significantly greater risk of foetal macrosomia, intra-uterine growth restriction, and congenital structural abnormalities (2).
Increasing evidence suggests that the adverse health consequences of maternal obesity for the offspring may extend beyond pregnancy throughout the entire life course (6, 7). Several studies have linked maternal obesity with greater likelihood of congenital heart disease (CHD) (8, 9), adverse cardiometabolic profile in childhood (10–13), and higher risk of CVD in adulthood (14). Whilst some studies have suggested that the period in-utero is a critical determinant of long-term offspring health (6, 7), others have not corroborated these findings (14–16). Another key consideration is the influence of shared home lifestyle on the mother-child cardiovascular risk profile. Indeed, a shared obesogenic diet and similar attitudes to physical exercise can importantly influence cardiovascular health of both mother and child, with such behavioural traits persisting into adulthood (17, 18). Furthermore, the genetic inheritance of obesogenic traits is likely to play an additionally important role in driving poorer cardiovascular health in the offspring of mothers with obesity. These factors are complex and intimately intertwined. Greater understanding of the relationship between maternal obesity and offspring cardiovascular health may provide insight into potential disease mechanisms and may inform targeted population-level CVD prevention strategies.
With the increasing rates of maternal obesity and the rising global burden of CVD, examining the relationships between maternal obesity and offspring life-long cardiovascular health is a public health priority. Research in this area faces unique challenges with multiple potential sources of bias. Previous reviews of literature in this area have limited to specific cardiovascular outcomes and life-stages, and as such do not capture the entire spectrum of cardiovascular consequences that may be related to maternal obesity. The aim of this paper is to provide an all-encompassing systematic review and critical appraisal of the literature exploring the impact of maternal obesity on offspring cardiovascular health across the entire life course.
Methods
This systematic review was performed according to the Preferred Reporting Items for Systemic Reviews and Meta-analyses (PRISMA) protocol. Prior to conducting the review, the study was registered on the International Prospective Register of Systematic Reviews (PROSPERO; https://www.crd.york.ac.uk/prospero/; Registration Number: CRD42021278567) (19). Methods are aligned with the PRISMA statement (Transparent Reporting of Systematic Reviews and Meta-Analyses) (20) and the PRISMA checklist is provided in Supplementary Table 1.
Eligibility Criteria
We set a broad remit for the review with pre-defined study inclusion criteria (Table 1). We included studies with any measure or estimate of maternal obesity set as the exposure of interest, and the outcome as any measures of offspring cardiovascular health at any age. We included studies of both adult and paediatric populations in the English language.
Search Strategy
The searches were carried out by two independent investigators (LK, MA) using the Ovid Medline (1946-October 2020) and EMBASE electronic databases. In Ovid Medline, we used the advanced search option to select relevant MeSH (Medical Subject Headings) terms. To capture broad results, we used the explode function, included all subheadings, and included a keyword search for each term using the multi-purpose (mp) function. Search terms were combined using Boolean operators. The results were limited to English language articles and human studies (Table 2). The titles and abstracts of the search results were visually screened for eligibility according to pre-defined criteria by LK and MA (Table 1). A similar approach was taken to searching the Embase database to identify further unique hits. Additional studies were sought through cross-referencing, author searches, and grey-literature searches. The full text of selected papers was then examined to confirm eligibility. Papers that did not meet these criteria were excluded. In cases of ambiguity, eligibility for inclusion was determined after full text review and discussion with project supervisors (ZRE, SEP).
Risk of Bias Assessment
The studies were assessed for their risk of bias using the ROBINS-I (Risk of Bias in Non-randomised Studies – of Interventions) tool (21). This tool assesses the risk of bias in seven domains by asking a series of questions, before coming to an overall judgement about risk of bias (low, moderate, serious, critical). The seven domains comprise potential bias from confounding, participant selection, intervention (exposure) classification, deviations from intended intervention (exposure), missing data, outcome measurement, and selective reporting of results. Further explanations of these domains and criteria for assessment of overall risk of bias are available in a dedicated publication (21).
Results
The search generated 1,213 results, including 9 duplicates. One study was identified from unpublished literature (doctoral thesis) searching. 1,159 papers were excluded following the title and abstract screen. This left 46 articles, which were read in full. Of these, 27 studies met the eligibility criteria and are included in the review (Figure 1).
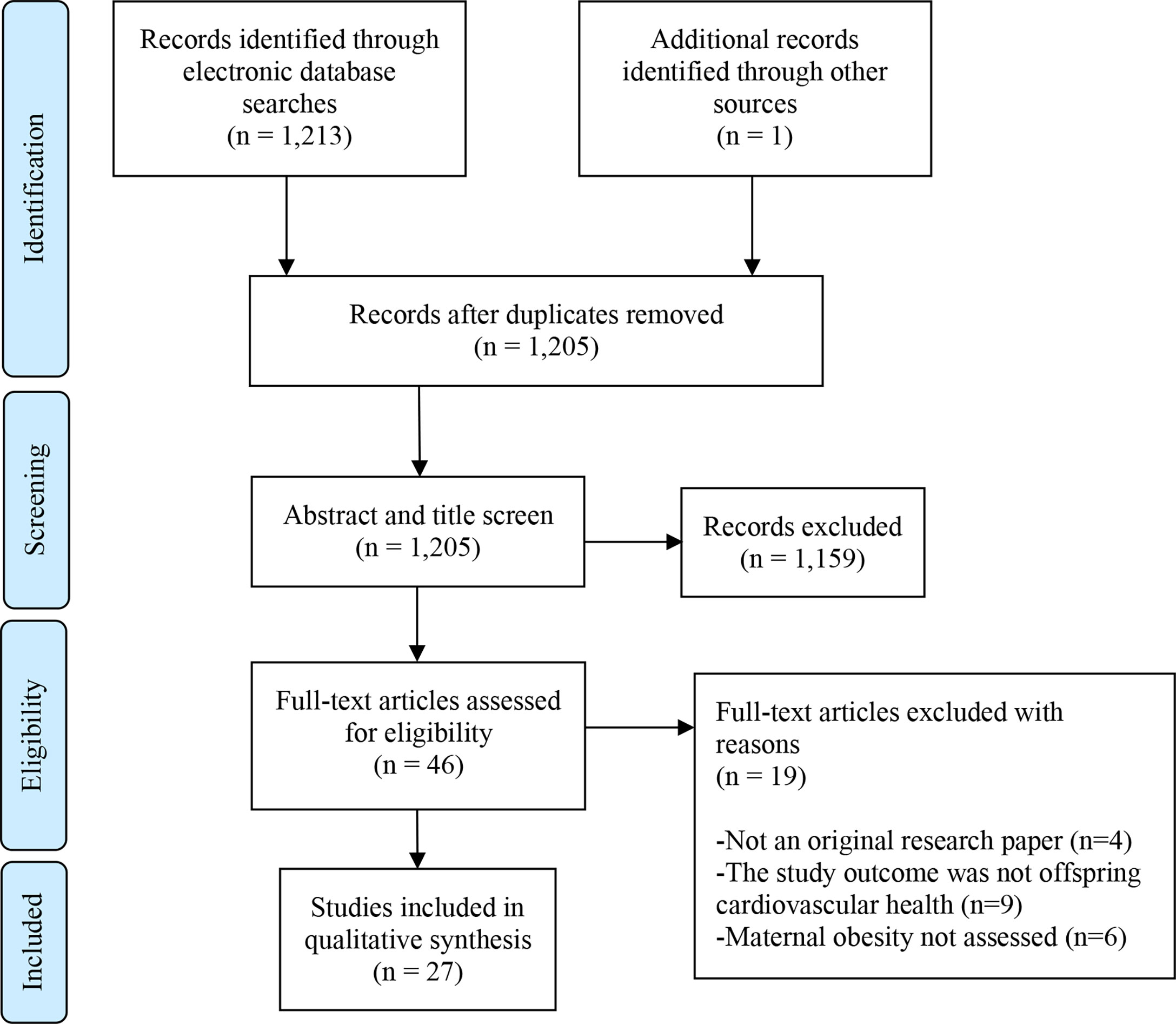
Figure 1 PRISMA Flow Diagram. Flow chart as per: Moher D, Liberati A, Tetzlaff J, Altman DG, The PRISMA Group (2009). Preferred Reporting Items for Systematic Reviews and Meta-Analyses: The PRISMA Statement. PLoS Med 6(7): e1000097. doi: 10.1371/journal.pmed1000097.
Study Characteristics
The 27 included studies were published between 2011 and 2020 in 11 different countries. All were observational studies. All used Maternal pre-pregnancy body mass index (MppBMI) or maternal body mass index (BMI) during pregnancy as the main exposure of interest. Six studies used gestational weight gain (GWG) as an additional exposure. One study also used skinfold thickness and fat percentage by bioimpedance. The studies measured cardiovascular health outcomes in offspring at various ages: 10 papers studied neonates, 11 studied children (0-18years), and eight studied adults (17-86 years). A diverse range of outcomes were considered, including CHD, cardiometabolic parameters (such as, BMI, blood pressure (BP) measurement, serum lipids, and serum insulin levels), and incident/prevalent CVD.
We organised the identified studies according to the age range studied and the outcome measured: neonatal CHD (n=7), neonatal cardiometabolic parameters (n=1), childhood cardiometabolic parameters (n=11), adult cardiometabolic parameters (n=5) and adult CVD (n=3). The characteristics, outcomes, and exposures of studies are summarised in Figure 2, and in Tables 3–7.
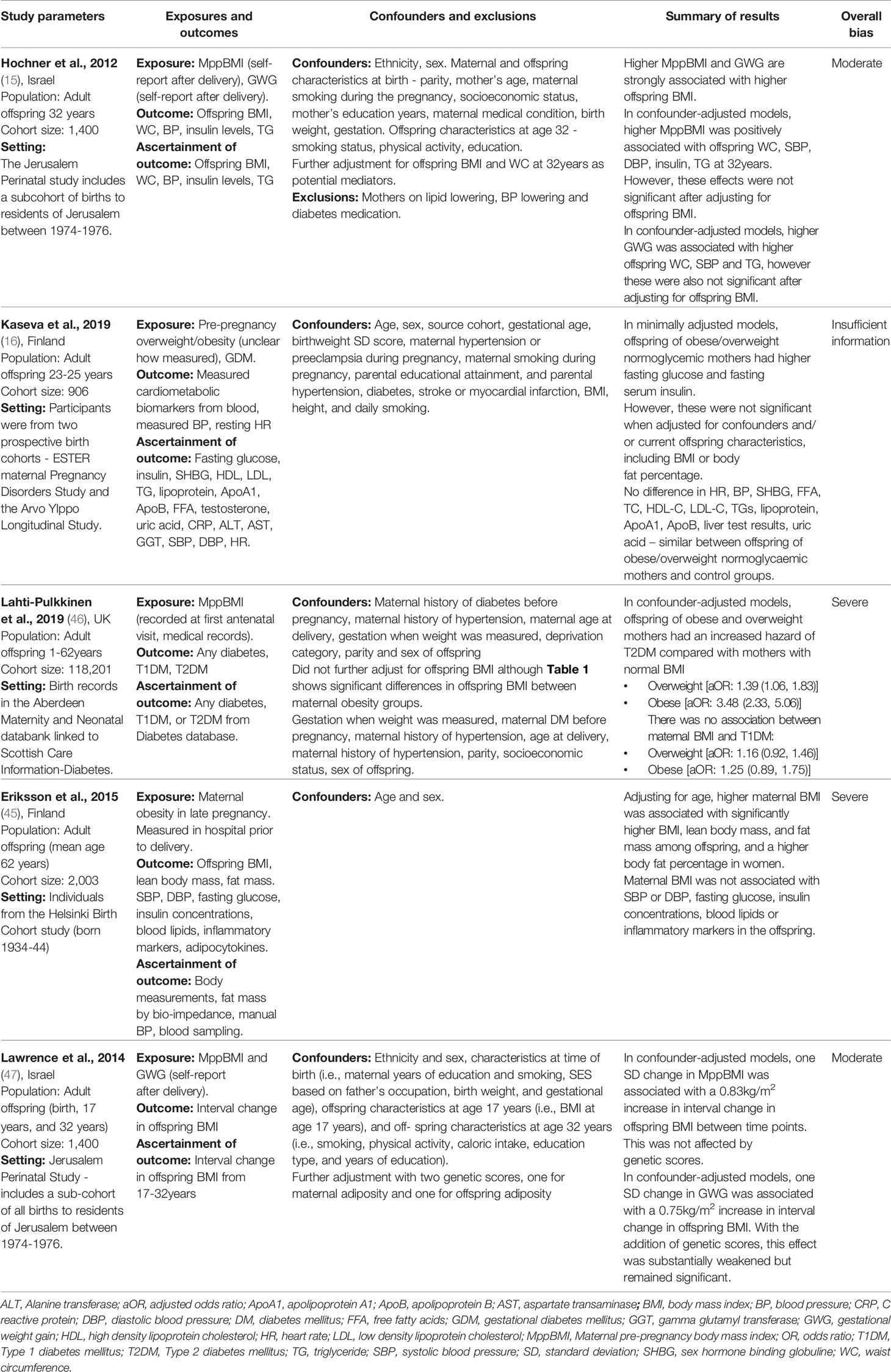
Table 6 Characteristics of the studies: Maternal obesity and cardiometabolic parameters in adulthood.
Risk of Bias Assessment
The studies were evaluated for their risk of bias using the ROBINS-I tool. 16 studies were appraised to be of moderate risk of bias, while 5 were of severe risk and 5 of critical risk. One study did not have enough information available to come to a bias assessment. No studies were at a low risk of bias. The results of the bias assessment are displayed in Supplementary Table 2 and summarised in Tables 3–7.
Neonatal Congenital Heart Disease
Several studies examined the link between maternal obesity and neonatal CHD. Brite et al. (24) conducted a retrospective electronic records review of maternal and offspring records of 121,815 deliveries across 19 hospitals included in the Consortium on Safe Labour cohort study. Odds of any offspring CHD ascertained from hospital discharge records are reported for different pre-pregnancy BMI categories (overweight, obese, and morbidly obese) compared to normal weight mothers as defined by WHO categories. Additionally, odds of disease-specific CHDs were considered in mothers with obesity (BMI ≥30kg/m2) compared to mothers with BMI<30kg/m2. Models were adjusted for study site, age, race, insurance status, and maternal smoking. A further analysis on a subset of 5,131 pairs was also adjusted for maternal glucose tolerance. The sample included 1,388 (1%) cases of CHD. The authors report a dose-dependent increase in odds of any CHD across increasing BMI categories. Compared to normal weight mothers, mothers with morbid obesity (BMI ≥40kg/m2) were at highest risk of having a child with CHD [OR 1.36 (1.03-1.78)], followed by mothers with obesity [OR 1.25 (1.08-1.45)], and mothers who were overweight [OR 1.18 (1.03-1.34)]. Compared to mothers without obesity, those with BMI ≥30kg/m2 had significantly greater odds of having an infant with conotruncal defects [OR 1.33 (1.03–1.72)], atrial septal defects [OR 1.22 (1.04–1.43)], or ventricular septal defects [OR 1.38 (1.06–1.79)]. In the subset of 5,131 pairs adjusted for maternal glucose tolerance, these results remained consistent.
Madsen et al. (26) report a large population-based case-control study evaluating the relationship between maternal obesity and offspring CHD using linked birth-hospital discharge records between 1992-2007. The authors considered any CHD recorded within three years of birth. The sample comprised 14,142 infants with CHD and 141,420 comparators without CHD selected at random and matched on year of delivery. In models adjusted for gestational diabetes, infants with CHD were more likely to have a mother with obesity [OR 1.22, (1.15–1.30)]. Consistent with the observations by Brite et al. (24), Madsen et al. (26) also report a dose-response relationship between increasing BMI and higher risk of offspring CHD. They also report association of higher BMI with greater risk of specific CHDs, namely left [OR 1.27 (1.02–1.59)] and right [OR 1.43 (1.20–1.69)] ventricular outflow tract defects, and hypoplastic left heart syndrome [OR 1.86, 95% (1.13–3.05)]. In contrast to Brite et al. (24), they report no statistically significant association with conotruncal defects [OR 1.04 (0.82–1.33)]. These studies have several strengths, both making use of large, standardised health records to ascertain maternal BMI and offspring health outcomes. However, notably, Brite et al. (24) report missing BMI data for 77,000 women, and noted a higher prevalence of CHD in women with missing BMI data. This suggests not-at-random missingness in a manner relevant to the outcome of interest and may thus be a source of systematic bias. Similarly, Madsen et al. (26) used self-reported BMI for women who had missing physical measurements of BMI, potentially introducing recall/reporting bias in ascertainment of the exposure. Furthermore, neither study considered key modifying variables, such as exposure to teratogens, folic acid intake, and maternal co-morbidities other than gestational diabetes, which may represent important sources of confounding.
A small case control study by Alvarado-Terrones et al. (22), investigating maternal factors associated with incidence of total anomalous pulmonary venous connection (TAPVC) presented findings consistent with these observations. This study included 55 mother-child cases with isolated TAPVC and 152 healthy mother-child cases. Both groups had no maternal history of addiction, pre-eclampsia, type 1 diabetes, type 2 diabetes, or GDM. They report no difference in rates of family history, addiction, folic acid supplementation, or alcoholism in cases vs controls. The authors report that compared to normal weight mothers, those with pre-pregnancy BMI in the overweight and obese categories had significantly higher risk of infant TAPVC [OR 1.9 (0.9-3.8); OR 3.7 (1.5-9.5), respectively].
Complementary to these findings, Tang et al. (23) report association of elevated MppBMI with increased risk of obstructive heart defects (OHDs). This study identified several infant and maternal single nucleotide polymorphisms (SNPs) that interacted with maternal obesity to increase the risk of OHDs, some of which were protective in normal weight women (23) but not in those with obesity. These findings suggest important genetic evidence supporting the association between maternal BMI and infant CHD.
Several studies have failed to reproduce the observed associations between maternal BMI and infant CHD risk. Ghaderian et al. (25) present a retrospective case-control study of 164 CHD consecutive cases of infants referred to their paediatric unit and 158 infants without major malformations and their mothers. Infants with syndromic CHD, genetic syndrome, or chromosomal defects were excluded, as were mothers with gestational or pre-pregnancy diabetes. Maternal BMI was extracted from medical records and categorised into WHO obesity groups. In contrast with previously mentioned studied (22, 24, 26), Ghaderian et al. (25) report no statistically significant association between maternal obesity and infant CHD. It is possible that by more careful exclusion of genetic causes of CHD and mothers with history of diabetes, Ghaderian et al. (25) were able to elucidate the true nature of the maternal obesity-CHD relationship isolated from these confounding factors. However, the authors may also have been underpowered to detect an association with obesity due to the small number of mothers in the obesity categories (n=23 cases, 14%) as indicated by the wide confidence intervals reported by these authors (Table 3). There is also ambiguity around the timing of BMI measurement, without explicit reporting that the measures were taken before or during pregnancy.
Dolk et al. (27) also present a case control study of babies diagnosed with CHD before 6 months of age, excluding those with genetic syndromes and children born to mothers with pre-gestational diabetes. In a different approach to other studies, they also included stillborn babies with prenatal diagnosis of CHD, mitigating the effect of survivor bias. This is an important consideration, as CHD may result in intra-uterine death and maternal obesity is also associated with an increased risk of stillbirth. Therefore, in excluding stillborn babies, cases of CHD may be missed, underestimating the effect of MppBMI on CHD. In the study by Dolk et al. (27) cases were ascertained by paediatric cardiologists based on clinical records and International Paediatric Cardiology Code criteria. Controls were babies without CHD recruited from routine maternity outpatient clinics. BMI data was extracted from maternity health records. Mothers with pre-gestational diabetes were excluded. Similar to Ghaderian et al. (25), Dolk et al. (27) report no significant association between maternal BMI category and infant CHD in crude or multivariable models (adjusted for: maternal age, previous pregnancy, maternal education, socioeconomic deprivation, diet).
In a further study, Kaplinski et al. (28) investigated the association between genetically determined maternal obesity and offspring conotruncal defects in 466 patients identified via Children’s Hospital of Philadelphia (1992-2010) and the Paediatric Cardiac Genomic Consortium (2010-2012). This study reported no significant association (in contrast to Brite et al. (24) and Madsen et al. (26)), suggesting that the relationship may not be causal (28). However, only the genetic risk of obesity was assessed, not the actual presence of maternal pre-pregnancy obesity phenotype. Since obesity develops through a complex interaction of genetic and lifestyle factors, genetic non-compliance may be an issue in this study. Nevertheless, these studies highlight the need to consider the role of the genetic risk of obesity as well as BMI itself.
Neonatal Cardiometabolic Parameters
Lemas et al. (29) recruited 753 mothers and infants from the Healthy Heart Study and 1,012 controls (Table 4). The exposure of interest was MppBMI. The outcomes considered were neonatal cardio-metabolic markers including cord blood glucose, insulin, glucose-insulin ratio, total and high-density lipoprotein cholesterol (HDL-c), TG, free fatty acids, and leptin (29). The study found that MppBMI was positively associated with cord-blood insulin and glucose, and negatively associated with HDL-c (29). Adjustment for neonatal adiposity and foetal growth rendered the association with insulin levels non-significant, suggesting the effects may be partially mediated by neonatal adiposity. This study adjusted for multiple potential confounders relating to health and environmental status of mother and child. A potential limitation is measurement of MppBMI, which was obtained from medical records in 90% and self-report in 10%; this approach may introduce erroneous and non-standardised reports as well as recall and information bias.
Childhood Cardiometabolic Parameters
A total of 12 studies investigated the association between maternal BMI and cardiometabolic parameters during childhood (Table 5). The age range was 2-18 years. The papers studied a range of different outcomes split into six domains: childhood anthropometrics, BP, insulin levels, lipids, cardiovascular phenotypic measures.
Childhood Anthropometrics
Nine studies report positive associations between MppBMI and offspring childhood anthropometrics (30, 31, 33, 35–38, 40, 41). Of these, three studies had critical and two had severe risk of bias, predominantly due to confounding and selection bias (Table 5 and Supplementary Table 2).
Filler et al. (34) conducted a study in children from emergency departments and clinical hospital appointments, and found that in confounder-adjusted models, higher MppBMI was associated with greater BMI, SBP, HDL-c, insulin, and all body fat measures in the offspring at 6 years. However, recruitment from hospital clinics may have introduced selection bias (34). As the children included in the study were in hospital for a medical problem, they are unlikely to be representative of the general population. Furthermore, disease processes for which the children are hospitalised can have independent effects on BMI and cardiometabolic markers which may importantly influence associations in this sample. Therefore, associations observed by Filler et al. (34) may not be widely generalisable. Techur-Pedro’s doctoral thesis (35), includes a retrospective longitudinal cohort study of live births registered on the Palau Ministry of Health Maternal and Child Health Birth Registry. This study similarly reports that among children aged 4-6 years, the risk of overweight or obesity was 2.57-times higher among those born to mothers with pre-pregnancy obesity compared to children born to mothers with normal MppBMI. However, this study had a 72% attrition rate at offspring follow-up, severely limiting the internal validity of the study due to attrition bias (35).
Despite shortcomings in several of the studies in this category, there is broad consistency of findings with other, more methodologically robust studies. Perng et al. (37) report a prospective cohort of pregnant women and their children in the USA. They studied 1,090 mother-child pairs and measured offspring overall and central adiposity at 6-10 years, along with blood metabolic parameters. The study found that every 5kg/m2 increase in MppBMI was associated with 0.92kg higher total fat, 0.27 BMI z-score and 0.39kg trunk fat in the offspring (37). An important strength of this study was its adjustment for offspring lifestyle factors during childhood, including diet and television watching habits, as well as paternal BMI. Having a parent with obesity means that, beyond genetic factors, the child is more likely to grow up in an obesogenic environment, with unhealthy diet and exercise habits. Offspring lifestyle must be considered in studies in this area to ensure any association is not due to the lifestyle impact of having a parent with obesity. No other studies adjusted for these variables, making confounding bias due to these factors a major issue in all other studies.
Across these studies, a range of different methods were used to assess offspring anthropometrics. BMI is a useful measure of obesity because it is easy to calculate and correlates well with subcutaneous fat mass. However, this correlation is not true of all body types and some studies suggest that measures of visceral adiposity are more predictive of adverse cardiovascular complications of obesity. It was therefore valuable that several studies measured additional markers of offspring obesity and visceral adiposity (Table 5). In summary, published studies provide consistent evidence that MppBMI is positively associated with offspring BMI during childhood, with higher BMI categories conferring an increased risk.
Childhood Blood Pressure
Six studies measured the impact of MppBMI on offspring BP. Five studies found that MppBMI was significantly associated with higher BP in the offspring (30, 33, 34, 37, 38) (Table 5). Perng et al. (37) found a positive association between MppBMI and higher offspring systolic BP. A similar association was found by Gaillard et al. (33) who used a prospective cohort study to assess the BMI of 5,908 mothers throughout pregnancy and followed up their offspring at 6 years. Higher weight in early pregnancy was associated with higher childhood systolic BP, although this was attenuated by adjustment for the child’s BMI, suggesting partial mediation by this variable (33). However, this study is limited by the fact that MppBMI was ascertained from self-report (33).
Veena et al. (31) recruited 504 pregnant women without gestational diabetes at their booking appointment and followed-up their offspring at 9.5 years, measuring offspring BMI, BP and insulin levels, as well as paternal BMI at five years. They report no association between maternal obesity during pregnancy and offspring BP after accounting for childhood BMI (Table 5). However, in this study maternal BMI was measured at 30 weeks’ gestation, which could reflect GWG, as opposed to MppBMI. Indeed, Gaillard et al. (33) found only early, not mid or late, pregnancy BMI to be positively associated with offspring BP. Therefore, the measurement of maternal BMI at such a late stage in pregnancy may have missed any correlation with offspring BP and could have contributed to the contradictory result to other studies in this section. Although gestational weight gain does correlate with maternal BMI, it is a proxy estimate and introduces measurement error and noise into the measurement, potentially diluting the effects of MppBMI. Finally, Wang et al. (39) investigated the interaction of maternal serum homocysteine levels and MppBMI with offspring SBP. Elevated blood homocysteine levels are known to be associated with increase CVD risk, and this study found a significant combined effect of maternal homocysteine concentration and pre-pregnancy obesity on offspring SBP, giving an example of how cardiovascular risk factors may compound offspring risk (39).
Currently available evidence on the association between MppBMI and childhood BP is high in quality and consistent, indicating a likely underlying causal effect. Gaillard et al. (33) further demonstrate attenuation (but not complete disappearance) of this relationship with adjustment for childhood BMI, suggesting a partial mediating effect of this variable. Thus overall, the current evidence suggests that maternal obesity is linked to higher offspring BP, but that this is almost completely mediated through greater obesity in childhood.
Childhood Insulin Levels
Five studies assessed the impact of MppBMI on offspring insulin levels. All studies used fasting insulin levels as the outcome measure, and consistently found a positive correlation between MppBMI and offspring insulin levels (31, 33, 37, 38, 40). Generally, the studies were of good quality, with only the study by Gaillard et al. (33) at a serious risk of bias due to the use of self-report MppBMI.
However, it is insulin resistance, rather than absolute insulin levels, that underlies the pathophysiology of type 2 diabetes (T2DM). To assess this, Perng et al. (37) and Tan et al. (38) used the Homeostasis Model Assessment of Insulin Resistance (HOMA-IR), an accurate tool for assessing insulin resistance (42). Both studies found a positive association between MppBMI and HOMA-IR. However, in non-diabetic subjects, the HOMA-IR is less useful and fasting insulin levels are an acceptable surrogate for insulin resistance (42). Therefore, the additional use of HOMA-IR by these studies may be of limited benefit.
Tan et al. (38) also used the Whole Body Insulin Sensitivity Index (WBISI), which is of greater benefit than HOMA-IR as the use of an Oral Glucose Tolerance Test integrates both insulin sensitivity and secretion (43). When comparing the offspring of normoglycaemic mothers with overweight/obesity to the offspring of normoglycaemic normal weight mothers, Tan et al. (38) found that MppBMI was positively associated with WBISI. However, confounders such as sociodemographic factors were not considered, and self-reported MppBMI was used to measure the exposure for some participants.
Adiposity has been strongly linked to insulin resistance. Therefore, it was important that studies adjusted for current offspring anthropometrics: in the four studies that adjusted for this, the association was partially attenuated (31, 33, 38, 40). Brandt et al. (40) also showed individuals with elevated insulin levels at age eight years had a significantly higher BMI trajectory from one year onwards. In summary, MppBMI has been consistently associated with both higher levels of insulin and insulin resistance in child offspring, supporting a potential underlying causal relationship, and this association is only partially explained (and therefore mediated) by the offspring BMI.
Childhood Lipid Profile
Four studies investigated the role of MppBMI on offspring lipid levels, although results were mixed. Gaillard et al. (33) and Tan et al. (38) found a significant association between higher MppBMI and adverse offspring lipid levels: lower HDL-c and higher low density lipoprotein-cholesterol (LDL-c) levels, though this analysis was not adjusted for by child body fat percentage or BMI and sociodemographic confounders, and consequently, the study by Gaillard et al. is at serious risk of bias from unadjusted confounders. Additionally, these findings were not supported by the study by Veena et al. (31), which found no association, although maternal BMI in this study was measured late in pregnancy at 30 weeks’ gestation. Finally, Perng et al. (37) investigated only the impact of MppBMI on triglyceride levels, reporting no significant relationship. Considering current evidence, no definitive conclusions can be made regarding the association between MppBMI and offspring lipid levels as the results are contradictory and the quality of the studies is mixed.
Childhood Cardiac Phenotypes
Two studies used echocardiography to measure offspring cardiovascular structure and function in childhood (32, 36). In a study of 4,852 parents and children, Toemen et al. (32) found that MppBMI (self-reported) and early pregnancy BMI (measured) were associated with higher left ventricular mass and left ventricular mass index in the offspring at six years. However, these associations were attenuated to non-significance when adjusted for offspring BMI. Also, no significant association was found with relative wall thickness or fractional shortening. Similarly, Litwin et al. (36) found no association with offspring LV diastolic and systolic function, this study was at serious risk of bias as GDM was the only confounder considered.
There is a paucity of evidence in this area, which limits the conclusions that can be drawn. The results available suggest that higher MppBMI is associated with adverse cardiovascular phenotypes in childhood, but that this may be wholly related to childhood BMI. Further studies are required before definitive conclusions can be drawn in this field.
Childhood Vascular Parameters
Two studies assessed the association between MppBMI on the offspring vasculature (30, 32). Toemen et al. (32) found MppBMI was positively associated with greater aortic root diameter of offspring measured on echocardiography. Cox et al. (30) used fundal image analysis to calculate the retinal tortuosity index, an indirect measure of hypertension, coronary heart disease and stroke risk (44). Higher MppBMI was significantly associated with an increased retinal tortuosity index, independent of BP, in the offspring at age 4-6 years (30). However, only 56% of participants were followed-up, placing the study at serious risk of bias (30). The low number of studies and heterogenous designs mean conclusions cannot be drawn about the impact of MppBMI on offspring vasculature. However, these results suggest a potential association that warrants further investigation.
Adult Anthropometrics
Two studies measured offspring anthropometrics in adulthood (15, 45). Both studies found a positive association between MppBMI and offspring BMI (Table 6). Hochner et al. (15) prospectively followed-up 1,400 offspring at 32 years, using a self-report questionnaire to measure MppBMI and GWG. For every one standard deviation (SD) increase in MppBMI, offspring BMI increased by 1.8 kg/m2 and waist circumference (WC) increased by 3.5 cm, and similarly, greater GWG was associated with higher offspring BMI in adulthood (15). Eriksson et al. (45) followed up 2,003 offspring at 62 years and found MppBMI was positively associated with lean body mass, fat mass, and BMI of the offspring. However, this study failed to adjust for confounders, except birthweight. Maternal BMI was also measured before delivery, so measurements may reflect gestational weight gain, as opposed to MppBMI. This study is likely impacted by survival bias as those who survived until 62 years may have had a more favourable body composition than those who died. Lawrence et al. (47) measured the change in offspring BMI from 17-32 years and found a significant association between higher MppBMI and a greater interval increase in offspring BMI, even after adjustment for genetic risk scores. Overall, the results are suggestive of a positive association between MppBMI and offspring obesity in adulthood. However, the extent to which this is caused by genetic factors, environmental factors or pregnancy-related factors is difficult to isolate.
Adult Blood Pressure
Three studies measured the impact of MppBMI on offspring BP in adulthood (15, 16, 45). Only Hochner et al. (15) found a significant positive association between MppBMI and offspring systolic and diastolic BP. However, the association was attenuated after adjustment for the current offspring BMI, indicating that offspring BMI is a full mediator of this association. Kaseva et al. (16) found no significant association between MppBMI and offspring BP at 24 years. Eriksson et al. (45) found no significant association at 62 years. In summary, MppBMI may be associated with offspring BP during adulthood, though this relationship, similar to relationships with childhood BP, may be mediated by offspring BMI.
Adult Insulin Levels
Three studies addressed the role of MppBMI on offspring insulin levels during adulthood (15, 16, 45). Hochner et al. (15) and Kaseva et al. (16) found a significant association between MppBMI and offspring insulin levels at 32 and 24 years respectively. Kaseva et al. (16) also found a significant association with fasting glucose levels, although there is insufficient information available in the study to assess bias. The associations were attenuated to non-significance in both studies when adjusted for current offspring BMI. Eriksson et al. (45) found no association between maternal obesity and neither insulin or fasting glucose levels in offspring at 62 years but was at a serious risk of bias. While the results suggest there may be an association between MppBMI and offspring adult insulin and glucose levels, it is likely to be mediated by the offspring’s current BMI.
Two studies assessed the role of MppBMI in the risk of offspring type 2 diabetes. Lahti-Pulkkinen et al. (46) and Eriksson et al. (48) found a significant association between MppBMI and offspring diabetes risk. Lahti-Pulkkinen et al. (46) linked the birth records of 118,201 children born in Aberdeen between 1950-2011 to the Scottish register for diabetes (up to 2012). Offspring of mothers who were overweight or obese had an increased hazard of T2DM compared to normal BMI mothers (hazard ratio 3.48 and 1.39 respectively) (46).
Eriksson et al. (48) used the Helsinki Birth Cohort study to follow disease outcomes in 13,345 individuals born in Helsinki between 1934 to 1944. Maternal BMI was associated with an increased risk of diabetes (48). However, maternal BMI was measured at the end of pregnancy and did not adjust for the gestation at which the BMI was measured, failing to distinguish between GWG and MppBMI. Furthermore, Eriksson et al. identified diabetes cases through prescriptions of medication, missing diet-controlled and undiagnosed cases. This method also relies on the completeness and accuracy of medication records. Some diabetes medications can be used for other diseases, such as metformin, so there is also a risk of misclassification.
The results suggest an association between MppBMI and offspring type 2 diabetes. However, the studies have several key limitations and neither adjusted for current offspring BMI, limiting definitive conclusions.
Adult Lipid Profile
Three studies investigated the impact on adult offspring lipid levels (15, 16, 45). Only Hochner et al. (15) found a positive association between MppBMI and offspring triglyceride levels at 32 years, but this was wholly mediated by offspring current BMI, suggesting no direct association between MppBMI and triglyceride levels. Kaseva et al. (16) and Eriksson et al. (45) similarly found no association between MppBMI and offspring lipid measures (Total Cholesterol, LDL-c, HDL-c and triglycerides), although both have key issues. Overall, it remains unclear whether there is an association between MppBMI and offspring lipid levels as existing evidence is limited to a few studies with high risk of bias.
Adult Cardiovascular Disease
Three studies investigated the effect of maternal BMI during pregnancy on offspring CVD. All found that an elevated MppBMI was associated with increased risk of offspring hospital admission and death due to CVD (14, 48, 49) (Table 7).
Reynolds et al. (14) linked the Aberdeen Maternity and Neonatal Databank to national death and morbidity records for 37,709 individuals aged 34-61years. The study found that the children of mothers with obesity were more likely to be admitted to hospital for a cardiovascular event than children of mothers with a normal BMI (14).
Razaz et al. (49) used the Swedish Birth Register to follow up offspring between 1-25 years. The children of mothers who were overweight or obese were more likely to develop CVD compared to those of normal BMI mothers (49). Interestingly, Razaz et al. (49) conducted a sibling analysis, where CVD cases were matched to their siblings, in order to remove the impact of childhood environment, which the siblings likely shared. The study found that increase in maternal BMI between pregnancies was associated with an increase in offspring CVD risk (49). This finding is suggestive of a causal relationship between MppBMI and offspring CVD. However, it is important to note that despite the sibling study design, there may still be a degree of persistent environmental confounding.
Importantly, none of the studies exploring the role of MppBMI in CVD measured or adjusted for the current offspring BMI, lifestyle factors or sociodemographic factors, so residual confounding is very likely in these studies. Overall, the results suggest there is a positive association between MppBMI and risk of hospital admission and death from CVD, but this may be partly or wholly explained by confounding, and further investigations are needed to address this question.
Main Limitations in Appraised Studies
A main limitation of existing studies is inadequate consideration of confounding. Residual confounding reduces specificity and severely limits causal conclusions. Our study question cannot ethically or practically be answered in human randomised controlled trial. Therefore, controlling for confounders needs to be a key focus of future observational studies. While controlling for all variables may not be feasible, we have identified key areas where confounding should be addressed (Supplementary Table 3). Furthermore, experimental studies using animal models may have a role in isolating causal relationships in this context.
Accurate and non-biased measurement of maternal obesity is clearly highly important, given that it is the exposure of interest. However, very few studies performed dedicated prospective measurement of maternal BMI as part of a research protocol. In most studies, MppBMI was ascertained from self-report questionnaires or medical records. Using self-report methods, women generally underestimate MppBMI in higher BMI categories (50). This approach may also introduce recall bias as women may forget their pre-pregnancy BMI over time. Importantly such reporting biases may differently influence self-reports of BMI in mothers of children with cardiovascular illnesses compared to those with healthy children thereby introducing systematic bias. Other studies obtained BMI from medical records. Although this is a more objective method for ascertainment of BMI, there is also potential to introduce biases given that recording of BMI is not performed using pre-defined protocols or standardised equipment.
Another important consideration is timing of maternal obesity measurement. This is clearly important given that women gain weight during pregnancy and that exposure to maternal obesity may have different consequences for the offspring depending on gestational age of exposure. Furthermore, variation in time of measurement of maternal obesity limits between study comparisons. Measuring MppBMI at a later stage in pregnancy may reflect GWG, as opposed to MppBMI. Studies should therefore aim to measure the BMI as soon as possible at a standard gestation. However, this is difficult to achieve as women may not always have a reason to attend a medical facility to have their BMI checked. Women gain minimal weight during the first trimester, indicating BMI at the 10 week booking appointment is a reliable substitute for MppBMI (51). In our review, many studies measured maternal BMI at an unknown, late, or inconsistent time.
In studies investigating the offspring during childhood or adulthood, offspring were followed up for 2-18 years and 24-72 years respectively. The risk of obesity, hypertension, insulin resistance, and hypercholesterolaemia increase with age; Lawrence et al. (47) found that the children of mothers with obesity had a higher increase in BMI between 17-32years. This makes it difficult to draw conclusions about the impact of MppBMI on cardiometabolic parameters because the effects are potentially only manifest at certain ages. To understand how the risk changes with time, measurements should be repeated at different ages. However, drop-out, time, and cost limit the feasibility of this recommendation.
A further consideration is the potential time-varying nature of exposure to maternal obesity on offspring cardiovascular risk. It is possible, for example, that the importance of maternal obesity as a driver of adverse cardiovascular health may be more important at younger rather than older ages. As an individual transitions to adulthood other environmental exposures may contribute more to the overall cardiovascular risk than maternal obesity. However, it is also reasonable to suggest, based on available evidence, that early life exposure to maternal obesity is both a direct and indirect driver of adverse cardiometabolic profile and lifestyle behaviours throughout life. Indeed, the importance of early life exposures as determinants of health through to older age is the key tenet of lifecourse epidemiology theory (52). Further research, using different approaches, is needed to determine with greater certainty the mechanisms through which maternal obesity impacts offspring cardiovascular health and to isolate its independent effect over environmental exposures in later life. Animal studies and genetic studies are likely to provide greatest insight into such mechanism as the separation of such tightly intertwined factors (maternal obesity, environment/lifestyle) using observational approaches is extremely challenging and may not be possible.
Discussion
In this study, we systematically reviewed studies investigating the association between maternal obesity and cardiovascular health in the offspring. A large number of studies were examined with variable designs and quality, measuring the impact on CHD, cardiometabolic parameters, and CVD across a range of age groups.
Congenital Heart Disease
Our results were suggestive of a positive association between MppBMI and CHD. Previous studies have proposed several underlying mechanisms, although no definitive cause has been found. Firstly, impaired glycaemic control increases CHD risk and individuals with obesity are more likely to experience type 2 and gestational diabetes. However, most studies in this review excluded mothers with diabetes, reducing the likelihood of this as a potential explanation of the relationship.
Folic acid supplementation decreases the risk of neural tube defects and CHD (53–55). Most studies in this review controlled for folic acid supplementation, which, in theory, would indicate folic acid deficiency does not explain the elevated risk of CHD. However, women with obesity are more likely to be deficient in folic acid despite supplementation and may require higher doses (54, 56). Therefore, future studies should measure serum folate levels, as well as supplementation. Furthermore, other teratogenic exposures were not adequately considered in existing studies.
Finally, previous studies have suggested women with obesity may be less likely to comply with antenatal screening. Similarly, increased abdominal fat may make defects less visible via ultrasound scan. Without a prenatal diagnosis, women with obesity may be less likely to terminate an affected pregnancy, increasing CHD rates compared to normal weight women.
Cardiometabolic Parameters and Cardiovascular Disease
We conclude that maternal obesity during pregnancy is associated with an adverse offspring cardiometabolic profile and higher CVD risk. The next question is whether these associations are simply due to the genetic and environmental impact of having a parent with obesity, or if exposure to maternal obesity in-utero independently programs the foetus to have worse cardiovascular health. The first refers to the widely accepted view that obesity and CVD occur as a consequence of unhealthy lifestyle habits and genetics. A mother with obesity is more likely to eat an unhealthy diet and exercise infrequently (57). Her children are more likely to adopt these habits, increasing their risk of obesity, hypercholesterolaemia, hypertension, and insulin resistance. These risk factors would increase the offspring’s risk of CVD later in life. Furthermore, a mother with obesity may have several genetic predispositions to obesity that could further increase the offspring’s obesity risk, should these be inherited. It is possible that any associations between MppBMI and offspring cardiovascular health are simply due to the lifestyle effect of having a mother with obesity and the inheritance of obesogenic genes.
However, we found evidence that adverse cardiometabolic changes may be present in neonates of mothers with obesity. The neonatal period is not yet strongly influenced by environmental and lifestyle factors, suggesting the conditions in-utero may influence offspring cardiovascular health at very early ages. Furthermore, if the elevated risk was only due to lifestyle and genetic factors, paternal obesity would be expected to confer an equal risk to offspring health. Three studies in this review adjusted for paternal BMI, of these two found a significant association with an adverse offspring cardiometabolic profile even after adjustment for paternal BMI. Furthermore, Perng et al. (37) found a significant association between higher MppBMI and an adverse offspring BMI and cardiometabolic profile after adjustment for offspring television watching habits and diet during childhood. These studies suggest that offspring exposure to maternal obesity during pregnancy confers an additional risk, over that expected from lifestyle and genetic factors.
The second mechanism involves the Developmental Origins of Health and Disease (DOHaD). This was described by Barker following the observation that death rates distributions from coronary heart disease mirrored infant mortality and low birth-weight rates 70 years earlier (7). The theory postulates that exposure to less favourable conditions in-utero triggers physiological adaptations that help the foetus to survive (6, 7). Since these adaptations occur during critical periods of organ development, the changes are likely irreversible and impact foetal long-term development (6, 7). While these compensatory adaptations may be helpful for in-utero survival, the infant may be left with increased risk of developing certain non-communicable diseases later in life (6, 7). For example, the changes that help a foetus to survive conditions of poor nutrient availability in-utero could be maladaptive in a post-natal environment where there is sufficient food availability, increasing the offspring’s later risk of coronary heart disease. Whilst direct causal evidence in this context is lacking, several lines of evidence provide support for the hypothesis (58, 59).
The Foetal Overnutrition Hypothesis suggests that mothers with obesity are more likely to have a diet high in calories and fat, which exposes the foetus to excess nutrients in-utero (12). Several observational studies have shown an association between excessive maternal fat and sugar intake and the development of childhood obesity in the offspring (12). This hypothesis is further supported by research into diabetes during pregnancy. Here, the foetus is exposed to excessive sugar, lipids and amino acids, which contribute to foetal macrosomia and an increased risk of childhood obesity (60). Furthermore, high calorie diets during pregnancy have been associated with changes in hypothalamic gene expression in animal models, which could mediate appetite and satiety in the offspring and therefore offspring obesity levels and cardiovascular risk (61).
Epigenetic changes are the second proposed underlying mechanism for the DOHaD (62). Epigenetic changes are heritable alterations in gene expression, but not the underlying genetic sequence, through DNA methylation, histone modification and RNA processes (63). The environment in-utero is a key period for the development of epigenetic markers; and nutrition influences this process (63). For example, offspring born during the Dutch Hunger Winter were exposed to famine conditions in utero. Exposed offspring were more likely to develop coronary heart disease in adulthood and at a younger age (64). Such individuals were also found to have less DNA methylation of the IGF-2 gene 60 years later compared to unexposed siblings (63). Similar epigenetic changes have been reported in animal studies for maternal obesity. In primates, a high calorie maternal diet during pregnancy was shown to cause covalent modification of histone proteins at specific sites, which altered gene expression in the offspring (65). A mouse-model found that a high-fat maternal diet during pregnancy decreased global and gene-specific DNA methylation in offspring, which altered gene expression of dopamine and opioids (66). These offspring showed an increased preference for sucrose and fat (66). Therefore, animal studies have shown exposure to pre-natal maternal obesity causes epigenetic changes that adversely influence offspring diet. However, the impact on disease risk is unclear and human studies are limited. A systematic review by Van Dijk et al. (67) found that obesity was associated with different levels of methylation at several specific sites, and some evidence that this may be reversible with weight loss programs. Liu et al. (68) measured the impact of MppBMI on CpG methylation in neonatal cord blood DNA. While one significant site was identified, the impact on gene expression and offspring long term health were not investigated, so the clinical relevance of this observation is unknown.
Public Health Implications
The rising prevalence of obesity worldwide is a major public health concern. Obesity is a key risk factor for numerous conditions, and its rising prevalence is causing an increase in the incidence of CVD, type 2 diabetes, and several cancers. We found several associations between an elevated MppBMI and adverse offspring cardiovascular health, including an increased risk of CHD, obesity, worse cardiometabolic parameters, and greater CVD burden. The children of mothers with obesity are more likely to be obese themselves and have worse cardiovascular health. These observations highlight the importance of early life factors on later health outcomes (52). These findings are concerning for many reasons. 30% of reproductive age women are obese, which is likely to impact the health of the next generation, further perpetuating the increasing trend in the prevalence of obesity and CVD. Secondly, our results suggest the associations with offspring cardiovascular health may not be adequately explained by sharing lifestyle and genetic factors with an obese parent. Exposure to maternal obesity in-utero could program offspring to have worse cardiovascular health. These offspring would be high risk for developing CVD, given the likely presence of genetic, environmental, and developmental risk factors for obesity. These children could then grow up to also be mother with obesity, creating a vicious cycle, where the obesity crisis worsens with each generation.
To protect the offspring, we should address maternal BMI during and before pregnancy (59). If developmental programming is occurring, obesity prevention strategies could hypothetically reverse such programming. Meanwhile, if developmental programming is not occurring, MppBMI could be used as a screening tool to identify offspring at a high risk of cardiovascular complications. Pre- and post-natal interventions could be targeted towards such groups, reinforcing good lifestyle habits in the mother that she can pass on to her child. However, it is not enough to only address diet and lifestyle through educational interventions (69–71). Obesity is strongly influenced by health inequalities such as socioeconomic or educational status. Access to fresh healthy food is more difficult in some, predominantly poorer, areas and healthy food is often more expensive than unhealthy options, limiting its accessibility. Therefore, poverty must be addressed, and health education must have a particular focus on individuals in areas with greater socio-economic deprivation.
Strengths and Limitations
The broad framing of the remit of this review, in terms of definition of the exposure, offspring cardiovascular health indicators, and age range covered permitted a wide-reaching assessment of the associations of maternal obesity with offspring cardiovascular health. We used a systematic and standardised approach validated by two independent investigators. However, despite a thorough and broad review of the topic, we were unable to draw definitive conclusions in several areas due to limitations of the source literature. We cannot exclude the possibility of publication bias. Finally, the heterogenous design of the studies precluded meta-analysis of the results.
Conclusions
The world is facing an obesity epidemic. Currently, 30% of reproductive age women are obese in the UK. We conducted a systematic review of 27 papers to understand the impact of maternal obesity on offspring cardiovascular health. We conclude that higher MppBMI is associated with adverse offspring cardiovascular health throughout life. Developmental programming in-utero may contribute to the association, as the lifestyle impact of having an obese parent does not fully explain reported findings. However, the observational design and mixed quality of the studies limit conclusions about causality. Future studies must minimise confounding and measure MppBMI using standardised methods. In the meantime, public health strategies targeting maternal obesity are warranted to improve health of mother and child across multiple cardiovascular health areas throughout the life course.
Data Availability Statement
The original contributions presented in the study are included in the article/Supplementary Material. Further inquiries can be directed to the corresponding author.
Author Contributions
LK, SEP, and ZR-E conceptualised the idea and planned the study. LK led on running literature searches, critical appraisal of the literature, and writing of the manuscript. MA acted as a second independent searcher and assisted with critical appraisal and manuscript preparation. CM assisted with manuscript preparation. ZR-E and SEP provided key edits and the final version of the manuscript. ZR-E provided overall supervision of the work. All coauthors provided critical feedback on the final version of the manuscript and approved the submitted version.
Funding
ZR-E recognizes the National Institute for Health Research (NIHR) Integrated Academic Training programme which supports her Academic Clinical Lectureship post and was also supported by British Heart Foundation Clinical Research Training Fellowship No. FS/17/81/33318. SEP acknowledges support from the ‘SmartHeart’ EPSRC programme grant (www.nihr.ac.uk; EP/P001009/1) and also from the CAP-AI programme, London’s first AI enabling programme focused on stimulating growth in the capital’s AI Sector. CAP-AI is led by Capital Enterprise in partnership with Barts Health NHS Trust and Digital Catapult and is funded by the European Regional Development Fund and Barts Charity. SEP has also received funding from the European Union’s Horizon 2020 research and innovation programme under grant agreement No. 825903 (euCanSHare project). CM and SN were supported by the Oxford NIHR Biomedical Research Centre (IS-BRC-1215-20008). SN was additionally supported by the Oxford British Heart Foundation Centre of Research Excellence. NCH acknowledges support from the UK Medical Research Council (MRC #405050259 and #MC_UU_12011/1), NIHR Southampton Biomedical Research Centre, University of Southampton, and University Hospital Southampton. AJL is funded by a British Heart Foundation Intermediate Research Fellowship (FS/18/3/33292). The funders provided support in the form of salaries for authors as detailed above but did not have any additional role in the study design, data collection and analysis, decision to publish, or preparation of the manuscript.
Conflict of Interest
The authors declare that the research was conducted in the absence of any commercial or financial relationships that could be construed as a potential conflict of interest.
Publisher’s Note
All claims expressed in this article are solely those of the authors and do not necessarily represent those of their affiliated organizations, or those of the publisher, the editors and the reviewers. Any product that may be evaluated in this article, or claim that may be made by its manufacturer, is not guaranteed or endorsed by the publisher.
Acknowledgments
Figure 2 created in BioRender.com.
Supplementary Material
The Supplementary Material for this article can be found online at: https://www.frontiersin.org/articles/10.3389/fendo.2022.868441/full#supplementary-material
References
1. Apovian CM. The Obesity Epidemic — Understanding the Disease and the Treatment. N Engl J Med (2016) 374:177–9. doi: 10.1056/NEJMe1514957
2. Chandrasekaran S, Neal-Perry G. Long-Term Consequences of Obesity on Female Fertility and the Health of the Offspring. Curr Opin Obstet Gynecol (2017) 29:180–7. doi: 10.1097/GCO.0000000000000364
3. Huda SS, Brodie LE, Sattar N. Obesity in Pregnancy: Prevalence and Metabolic Consequences. Semin Fetal Neonatal Med (2010) 15:70–6. doi: 10.1016/j.siny.2009.09.006
5. Knight M, Bunch K, Tuffnell D, Shakespeare J, Kotnis R, Kenyon S, Kurinczuk JJ. Saving Lives, Improving Mothers’ Care - Lessons learned to inform maternity care from the UK and Ireland Confidential Enquiries into Maternal Deaths and Morbidity 2015-17. Oxford: National Perinatal Epidemiology Unit, University of Oxford 2019.
6. Mandy M, Nyirenda M. Developmental Origins of Health and Disease: The Relevance to Developing Nations. Int Health (2018) 10:66–70. doi: 10.1093/inthealth/ihy006
7. Barker DJP. The Origins of the Developmental Origins Theory. J Intern Med (2007) 261:412–7. doi: 10.1111/j.1365-2796.2007.01809.x
8. Liu X, Ding G, Yang W, Feng X, Li Y, Liu H, et al. Maternal Body Mass Index and Risk of Congenital Heart Defects in Infants: A Dose-Response Meta-Analysis. BioMed Res Int (2019) 2019:1315796. doi: 10.1155/2019/1315796
9. Cai G, Sun X, Zhang L, Hong Q. Association Between Maternal Body Mass Index and Congenital Heart Defects in Offspring: A Systematic Review. Am J Obstet Gynecol (2014) 211:91–117. doi: 10.1016/j.ajog.2014.03.028
10. Heslehurst N, Vieira R, Akhter Z, Bailey H, Slack E, Ngongalah L, et al. The Association Between Maternal Body Mass Index and Child Obesity: A Systematic Review and Meta-Analysis. PloS Med (2019) 16:e1002817. doi: 10.1371/journal.pmed.1002817
11. Yu Z, Han S, Zhu J, Sun X, Ji C, Guo X. Pre-Pregnancy Body Mass Index in Relation to Infant Birth Weight and Offspring Overweight/Obesity: A Systematic Review and Meta-Analysis. PloS One (2013) 8:e61627. doi: 10.1371/journal.pone.0061627
12. Gaillard R. Maternal Obesity During Pregnancy and Cardiovascular Development and Disease in the Offspring. Eur J Epidemiol (2015) 30:1141–52. doi: 10.1007/s10654-015-0085-7
13. Ludwig-Walz H, Schmidt M, Günther ALB, Kroke A. Maternal Prepregnancy BMI or Weight and Offspring’s Blood Pressure: Systematic Review. Matern Child Nutr (2018) 14:e12561. doi: 10.1111/mcn.12561
14. Reynolds RM, Allan KM, Raja EA, Bhattacharya S, McNeill G, Hannaford PC, et al. Maternal Obesity During Pregnancy and Premature Mortality From Cardiovascular Event in Adult Offspring: Follow-Up of 1 323 275 Person Years. BMJ (2013) 347:f4539. doi: 10.1136/bmj.f4539
15. Hochner H, Friedlander Y, Calderon-Margalit R, Meiner V, Sagy Y, Avgil-Tsadok M, et al. Associations of Maternal Prepregnancy Body Mass Index and Gestational Weight Gain With Adult Offspring Cardiometabolic Risk Factors. Circulation (2012) 125:1381–9. doi: 10.1161/CIRCULATIONAHA.111.070060
16. Kaseva N, Vääräsmäki M, Sundvall J, Matinolli H-M, Sipola M, Tikanmäki M, et al. Gestational Diabetes But Not Prepregnancy Overweight Predicts for Cardiometabolic Markers in Offspring Twenty Years Later. J Clin Endocrinol Metab (2019) 104:2785–95. doi: 10.1210/jc.2018-02743
17. Mei K, Huang H, Xia F, Hong A, Chen X, Zhang C, et al. State-Of-the-Art of Measures of the Obesogenic Environment for Children. Obes Rev (2021) 22:e13093. doi: 10.1111/OBR.13093
18. Dhana K, Haines J, Liu G, Zhang C, Wang X, Field AE, et al. Association Between Maternal Adherence to Healthy Lifestyle Practices and Risk of Obesity in Offspring: Results From Two Prospective Cohort Studies of Mother-Child Pairs in the United States. BMJ (2018) 362:k2486. doi: 10.1136/BMJ.K2486
19. PROSPERO: Inernational Prospective Register of Systematic Reviews . Available at: https://www.crd.york.ac.uk/prospero/.
20. Moher D, Liberati A, Tetzlaff J, Altman DG. Preferred Reporting Items for Systematic Reviews and Meta-Analyses: The PRISMA Statement. PloS Med (2009) 6:e1000097. doi: 10.1371/journal.pmed.1000097
21. Sterne JA, Hernán MA, Reeves BC, Savović J, Berkman ND, Viswanathan M, et al. ROBINS-I: A Tool for Assessing Risk of Bias in Non-Randomised Studies of Interventions. BMJ (2016) 355:i4919. doi: 10.1136/bmj.i4919
22. Alvarado-Terrones EG, Perea-Cabrera M, Klünder-Klünder M, Segura-Stanford B, Erdmenger-Orellana JR, Lopez-Yañez Blanco A, et al. Maternal Obesity as a Risk Factor for the Development of Total Anomalous Pulmonary Venous Connection in Their Offspring. Arch Med Res (2018) 49:109–13. doi: 10.1016/j.arcmed.2018.06.001
23. Tang X, Cleves MA, Nick TG, Li M, MacLeod SL, Erickson SW, et al. Obstructive Heart Defects Associated With Candidate Genes, Maternal Obesity, and Folic Acid Supplementation. Am J Med Genet Part A (2015) 167:1231–42. doi: 10.1002/ajmg.a.36867
24. Brite J, Laughon SK, Troendle J, Mills J. Maternal Overweight and Obesity and Risk of Congenital Heart Defects in Offspring. Int J Obes (2014) 38:878–82. doi: 10.1038/ijo.2013.244
25. Ghaderian M, Emami-Moghadam A-R, Khalilian M-R, Riahi K, Ghaedi F. Prepregnancy Maternal Weight and Body Mass Index of Children With and Without Congenital Heart Disease. Iran J Pediatr (2014) 24:313–8.
26. Madsen NL, Schwartz SM, Lewin MB, Mueller BA. Prepregnancy Body Mass Index and Congenital Heart Defects Among Offspring: A Population-Based Study. Congenit Heart Dis (2013) 8:131–41. doi: 10.1111/j.1747-0803.2012.00714.x
27. Dolk H, McCullough N, Callaghan S, Casey F, Craig B, Given J, et al. Risk Factors for Congenital Heart Disease: The Baby Hearts Study, A Population-Based Case-Control Study. PloS One (2020) 15:e0227908. doi: 10.1371/journal.pone.0227908
28. Kaplinski M, Taylor D, Mitchell LE, Hammond DA, Goldmuntz E, Agopian AJ. The Association of Elevated Maternal Genetic Risk Scores for Hypertension, Type 2 Diabetes and Obesity and Having a Child With a Congenital Heart Defect. PloS One (2019) 14:e0216477. doi: 10.1371/journal.pone.0216477
29. Lemas DJ, Brinton JT, Shapiro ALB, Glueck DH, Friedman JE, Dabelea D. Associations of Maternal Weight Status Prior and During Pregnancy With Neonatal Cardiometabolic Markers at Birth: The Healthy Start Study. Int J Obes (2015) 39:1437–42. doi: 10.1038/ijo.2015.109
30. Cox B, Luyten LJ, Dockx Y, Provost E, Madhloum N, De Boever P, et al. Association Between Maternal Prepregnancy Body Mass Index and Anthropometric Parameters, Blood Pressure, and Retinal Microvasculature in Children Age 4 to 6 Years. JAMA Netw Open (2020) 3:e204662. doi: 10.1001/jamanetworkopen.2020.4662
31. Veena SR, Krishnaveni GV, Karat SC, Osmond C, Fall CH. Testing the Fetal Overnutrition Hypothesis; The Relationship of Maternal and Paternal Adiposity to Adiposity, Insulin Resistance and Cardiovascular Risk Factors in Indian Children. Public Health Nutr (2013) 16:1656–66. doi: 10.1017/S1368980012003795
32. Toemen L, Gishti O, van Osch-Gevers L, Steegers EAP, Helbing WA, Felix JF, et al. Maternal Obesity, Gestational Weight Gain and Childhood Cardiac Outcomes: Role of Childhood Body Mass Index. Int J Obes (2016) 40:1070–8. doi: 10.1038/ijo.2016.86
33. Gaillard R, Steegers EAP, Franco OH, Hofman A, Jaddoe VWV. Maternal Weight Gain in Different Periods of Pregnancy and Childhood Cardio-Metabolic Outcomes. The Generation R Study. Int J Obes (2015) 39:677–85. doi: 10.1038/ijo.2014.175
34. Filler G, Yasin A, Kesarwani P, Garg AX, Lindsay R, Sharma AP. Big Mother or Small Baby: Which Predicts Hypertension? J Clin Hypertens (2011) 13:35–41. doi: 10.1111/j.1751-7176.2010.00366.x
35. Techur-Pedro A. Maternal Prepregnancy Nutritional Status as a Key Link of Intergenerational Risk of Obesity and Chronic Disease in Childhood: University of Hawai'i at Manoa (2015). Available at: https://www.proquest.com/docview/1714412442.
36. Litwin L, Sundholm JKM, Rönö K, Koivusalo SB, Eriksson JG, Sarkola T. No Effect of Gestational Diabetes or Pre-Gestational Obesity on 6-Year Offspring Left Ventricular Function—RADIEL Study Follow-Up. Acta Diabetol (2020) 57:1463–72. doi: 10.1007/s00592-020-01571-z
37. Perng W, Gillman MW, Mantzoros CS, Oken E. A Prospective Study of Maternal Prenatal Weight and Offspring Cardiometabolic Health in Midchildhood. Ann Epidemiol (2014) 24:793–800.e1. doi: 10.1016/j.annepidem.2014.08.002
38. Tan HC, Roberts J, Catov J, Krishnamurthy R, Shypailo R, Bacha F. Mother’s Pre-Pregnancy BMI is an Important Determinant of Adverse Cardiometabolic Risk in Childhood. Pediatr Diabetes (2015) 16:419–26. doi: 10.1111/pedi.12273
39. Wang H, Xu BP, Xu RB, Walker SO, Wang G. Joint Effect of Maternal Plasma Homocysteine and Prepregnancy Obesity on Child Blood Pressure: A Prospective Birth Cohort Study. Int J Obes (2017) 41:1447–53. doi: 10.1038/ijo.2017.109
40. Brandt S, Moß A, Lennerz B, Koenig W, Weyermann M, Rothenbacher D, et al. Plasma Insulin Levels in Childhood are Related to Maternal Factors - Results of the Ulm Birth Cohort Study. Pediatr Diabetes (2014) 15:453–63. doi: 10.1111/pedi.12109
41. Sundholm JKM, Litwin L, Rönö K, Koivusalo SB, Eriksson JG, Sarkola T. Maternal Obesity and Gestational Diabetes: Impact on Arterial Wall Layer Thickness and Stiffness in Early Childhood - RADIEL Study Six-Year Follow-Up. Atherosclerosis (2019) 284:237–44. doi: 10.1016/j.atherosclerosis.2019.01.037
42. Antuna-Puente B, Disse E, Rabasa-Lhoret R, Laville M, Capeau J, Bastard J-P. How Can We Measure Insulin Sensitivity/Resistance? Diabetes Metab (2011) 37:179–88. doi: 10.1016/j.diabet.2011.01.002
43. Yeckel CW, Weiss R, Dziura J, Taksali SE, Dufour S, Burgert TS, et al. Validation of Insulin Sensitivity Indices From Oral Glucose Tolerance Test Parameters in Obese Children and Adolescents. J Clin Endocrinol Metab (2004) 89:1096–101. doi: 10.1210/jc.2003-031503
44. Witt N, Wong TY, Hughes AD, Chaturvedi N, Klein BE, Evans R, et al. Abnormalities of Retinal Microvascular Structure and Risk of Mortality From Ischemic Heart Disease and Stroke. Hypertension (2006) 47:975–81. doi: 10.1161/01.HYP.0000216717.72048.6c
45. Eriksson JG, Sandboge S, Salonen M, Kajantie E, Osmond C. Maternal Weight in Pregnancy and Offspring Body Composition in Late Adulthood: Findings From the Helsinki Birth Cohort Study (HBCS). Ann Med (2015) 47:94–9. doi: 10.3109/07853890.2015.1004360
46. Lahti-Pulkkinen M, Bhattacharya S, Wild SH, Lindsay RS, Räikkönen K, Norman JE, et al. Consequences of Being Overweight or Obese During Pregnancy on Diabetes in the Offspring: A Record Linkage Study in Aberdeen, Scotland. Diabetologia (2019) 62:1412–9. doi: 10.1007/s00125-019-4891-4
47. Lawrence GM, Shulman S, Friedlander Y, Sitlani CM, Burger A, Savitsky B, et al. Associations of Maternal Pre-Pregnancy and Gestational Body Size With Offspring Longitudinal Change in BMI. Obesity (2014) 22:1165–71. doi: 10.1002/oby.20643
48. Eriksson JG, Sandboge S, Salonen MK, Kajantie E, Osmond C. Long-Term Consequences of Maternal Overweight in Pregnancy on Offspring Later Health: Findings From the Helsinki Birth Cohort Study. Ann Med (2014) 46:434–8. doi: 10.3109/07853890.2014.919728
49. Razaz N, Villamor E, Muraca GM, Bonamy A-KE, Cnattingius S. Maternal Obesity and Risk of Cardiovascular Diseases in Offspring: A Population-Based Cohort and Sibling-Controlled Study. Lancet Diabetes Endocrinol (2020) 8:572–81. doi: 10.1016/S2213-8587(20)30151-0
50. Stommel M, Schoenborn CA. Accuracy and Usefulness of BMI Measures Based on Self-Reported Weight and Height: Findings From the NHANES & NHIS 2001-2006. BMC Public Health (2009) 9:421. doi: 10.1186/1471-2458-9-421
51. Fattah C, Farah N, O’Toole F, Barry S, Stuart B, Turner MJ. Body Mass Index (BMI) in Women Booking for Antenatal Care: Comparison Between Selfreported and Digital Measurements. Eur J Obstet Gynecol Reprod Biol (2009) 144:32–4. doi: 10.1016/j.ejogrb.2009.01.015
52. Kuh D, Ben-Shlomo Y, Lynch J, Hallqvist J, Power C. Life Course Epidemiology. J Epidemiol Community Heal (2003) 57:778–83. doi: 10.1136/JECH.57.10.778
53. Feng Y, Wang S, Chen R, Tong X, Wu Z, Mo X. Maternal Folic Acid Supplementation and the Risk of Congenital Heart Defects in Offspring: A Meta-Analysis of Epidemiological Observational Studies. Sci Rep (2015) 5:8506. doi: 10.1038/srep08506
54. Zheng Z, Yang T, Chen L, Wang L, Zhang S, Wang T, et al. Increased Maternal Body Mass Index is Associated With Congenital Heart Defects: An Updated Meta-Analysis of Observational Studies. Int J Cardiol (2018) 273:112–20. doi: 10.1016/j.ijcard.2018.09.116
55. Rasmussen SA, Chu SY, Kim SY, Schmid CH, Lau J. Maternal Obesity and Risk of Neural Tube Defects: A Metaanalysis. Am J Obstet Gynecol (2008) 198:611–9. doi: 10.1016/j.ajog.2008.04.021
56. Mojtabai R. Body Mass Index and Serum Folate in Childbearing Age Women. Eur J Epidemiol (2004) 19:1029–36. doi: 10.1007/s10654-004-2253-z
57. Mozaffarian D, Hao T, Rimm EB, Willett WC, Hu FB. Changes in Diet and Lifestyle and Long-Term Weight Gain in Women and Men. N Engl J Med (2011) 364:2392–404. doi: 10.1056/NEJMOA1014296
58. Barker DJP. Obesity and Early Life. Obes Rev (2007) 8:45–9. doi: 10.1111/J.1467-789X.2007.00317.X
59. Hanson M, Barker M, Dodd JM, Kumanyika S, Norris S, Steegers E, et al. Interventions to Prevent Maternal Obesity Before Conception, During Pregnancy, and Post Partum. Lancet Diabetes Endocrinol (2017) 5:65–76. doi: 10.1016/S2213-8587(16)30108-5
60. Fall CHD. Evidence for the Intra-Uterine Programming of Adiposity in Later Life. Ann Hum Biol (2011) 38:410–28. doi: 10.3109/03014460.2011.592513
61. Poston L. Maternal Obesity, Gestational Weight Gain and Diet as Determinants of Offspring Long Term Health. Best Pract Res Clin Endocrinol Metab (2012) 26:627–39. doi: 10.1016/j.beem.2012.03.010
62. Gluckman PD, Hanson MA, Cooper C, Thornburg KL. Effect of In Utero and Early-Life Conditions on Adult Health and Disease. N Engl J Med (2008) 359:61–73. doi: 10.1056/NEJMra0708473
63. Heijmans BT, Tobi EW, Stein AD, Putter H, Blauw GJ, Susser ES, et al. Persistent Epigenetic Differences Associated With Prenatal Exposure to Famine in Humans. Proc Natl Acad Sci U.S.A. (2008) 105:17046–9. doi: 10.1073/pnas.0806560105
64. Painter RC, De Rooij SR, Bossuyt PM, Simmers TA, Osmond C, Barker DJ, et al. Early Onset of Coronary Artery Disease After Prenatal Exposure to the Dutch Famine. Am J Clin Nutr (2006) 84:322–7. doi: 10.1093/ajcn/84.1.322
65. Aagaard-Tillery KM, Grove K, Bishop J, Ke X, Fu Q, McKnight R, et al. Developmental Origins of Disease and Determinants of Chromatin Structure: Maternal Diet Modifies the Primate Fetal Epigenome. J Mol Endocrinol (2008) 41:91–102. doi: 10.1677/JME-08-0025
66. Vucetic Z, Kimmel J, Totoki K, Hollenbeck E, Reyes TM. Maternal High-Fat Diet Alters Methylation and Gene Expression of Dopamine and Opioid-Related Genes. Endocrinology (2010) 151:4756–64. doi: 10.1210/en.2010-0505
67. Van Dijk SJ, Molloy PL, Varinli H, Morrison JL, Muhlhausler BS, Buckley M, et al. Epigenetics and Human Obesity. Int J Obes (2015) 39:85–97. doi: 10.1038/ijo.2014.34
68. Liu X, Chen Q, Tsai H-J, Wang G, Hong X, Zhou Y, et al. Maternal Preconception Body Mass Index and Offspring Cord Blood DNA Methylation: Exploration of Early Life Origins of Disease. Environ Mol Mutagen (2014) 55:223–30. doi: 10.1002/em.21827
69. Adams J, Mytton O, White M, Monsivais P. Why Are Some Population Interventions for Diet and Obesity More Equitable and Effective Than Others? The Role of Individual Agency. PloS Med (2016) 13:e1001990. doi: 10.1371/JOURNAL.PMED.1001990
70. McGill R, Anwar E, Orton L, Bromley H, Lloyd-Williams F, O’Flaherty M, et al. Are Interventions to Promote Healthy Eating Equally Effective for All? Systematic Review of Socioeconomic Inequalities in Impact Health Behavior, Health Promotion and Society. BMC Public Health (2015) 15:1–15. doi: 10.1186/S12889-015-1781-7/TABLES/4
Keywords: obesity, maternal obesity, women’s health, cardiovascular disease, congenital heart disease, cardiometabolic disease, lifecourse epidemiology
Citation: Kankowski L, Ardissino M, McCracken C, Lewandowski AJ, Leeson P, Neubauer S, Harvey NC, Petersen SE and Raisi-Estabragh Z (2022) The Impact of Maternal Obesity on Offspring Cardiovascular Health: A Systematic Literature Review. Front. Endocrinol. 13:868441. doi: 10.3389/fendo.2022.868441
Received: 03 February 2022; Accepted: 14 April 2022;
Published: 20 May 2022.
Edited by:
Claire Joanne Stocker, Aston University, United KingdomReviewed by:
Zheng Liu, Peking University, ChinaCharis Bridger Staatz, University College London, United Kingdom
Alexandre A. da Silva, University of Mississippi Medical Center, United States
Copyright © 2022 Kankowski, Ardissino, McCracken, Lewandowski, Leeson, Neubauer, Harvey, Petersen and Raisi-Estabragh. This is an open-access article distributed under the terms of the Creative Commons Attribution License (CC BY). The use, distribution or reproduction in other forums is permitted, provided the original author(s) and the copyright owner(s) are credited and that the original publication in this journal is cited, in accordance with accepted academic practice. No use, distribution or reproduction is permitted which does not comply with these terms.
*Correspondence: Zahra Raisi-Estabragh, emFocmFyYWlzaUBkb2N0b3JzLm9yZy51aw==
†These authors have contributed equally to this work and share last authorship