- 1Department of Urology, Institute of Urology, West China Hospital, Sichuan University, Chengdu, China
- 2Department of Urology, Affiliated Hospital of Guizhou Medical University, Guiyang, China
- 3Department of Urology, Affiliated Hospital of North Sichuan Medical College, Nanchong, China
The circadian rhythm generated by circadian clock genes functions as an internal timing system. Since the circadian rhythm controls abundant physiological processes, the circadian rhythm evolved in organisms is salient for adaptation to environmental change. A disturbed circadian rhythm is a trigger for numerous pathological events. Recently, accumulated data have indicated that kidney stone disease (KSD) is related to circadian rhythm disturbance. However, the mechanism between them has not been fully elucidated. In this narrative review, we summarized existing evidence to illustrate the possible association between circadian rhythm disturbance and KSD based on the epidemiological studies and risk factors that are linked to circadian rhythm disturbance and discuss some chronotherapies for KSD. In summary, KSD is associated with systemic disorders. Metabolic syndrome, inflammatory bowel disease, and microbiome dysbiosis are the major risk factors supported by sufficient data to cause KSD in patients with circadian rhythm disturbance, while others including hypertension, vitamin D deficiency, parathyroid gland dysfunction, and renal tubular damage/dysfunction need further investigation. Then, some chronotherapies for KSD were confirmed to be effective, but the molecular mechanism is still unclear.
1 Introduction
With the Earth’s planetary rotation, there is a 24-h oscillating light-dark cycle (1). To adapt to this environmental cycle, all animals and plants have evolved universal internal circadian rhythms (2). Such rhythms are observed in cellular, physiological, and biological behavioral processes within a 24-hour cycle. For instance, heart rate and body temperature increase in the morning and decrease in the evening (1, 3). It is also found in the diet, sleep-wake cycle, endocrine, absorption, and reproduction (4–7). Hence, circadian rhythm is of great significance for maintaining homeostasis and normal physiological activities.
Before artificial light was created, humans adjusted their lives to a natural day-night alteration cycle (8). With the great development of technology and society, life patterns have changed greatly, and the phenomenon of circadian rhythm disorder is now common due to social jet lag (SJL), shift work, and sleep disruption, which all contribute to abnormal daily rest/wake cycles and chronically disrupt endogenous circadian rhythms. The asynchrony between endogenous circadian rhythm and the sleep-wake cycle is defined as circadian rhythm disruption (9, 10). Growing epidemiological and genetic evidence shows that circadian disruption leads to various diseases, such as insomnia, hypertension, type 2 diabetes (T2D), chronic kidney disease (CKD), and even cancer (10–12). All of these factors finally cause immense loss of public health.
Kidney stone disease (KSD) is a common health concern with increasing incidence during the past few decades and occurs in a wide range of ages, including children, adolescents, and adults (13). The prevalence is approximately 10% globally, with a high recurrence rate of 50% within 5-10 years and 75% within 20 years (14–16). It causes such a large burden on public health since it is not only a transient acute symptom but is also linked to cardiovascular disease (CVD), CKD, end-stage renal disease (ESRD), and renal cancer (17, 18). An updated meta-analysis signifies that KSD is associated with an approximately 20%-40% higher risk for coronary artery disease, transient ischemia/stroke, and arterial disease (19). Then, the risks of ESRD, renal cell carcinoma, and transient cell carcinoma are all increased in patients with prior KSD history (18, 20). Although great progress has been achieved in traditional surgical management to provide better prognosis, the incidence and recurrence rates are still very high with little breakthrough in prevention methods (especially drug interventions) (21). Therefore, it is pivotal to explore the potential pathophysiological mechanisms of KSD to provide insights for prevention and therapy.
There is plenty of evidence to suggest that a circadian rhythm disturbance can promote KSD (22, 23). Herein, this review summarizes the relationship between circadian rhythm disturbance and KSD and discusses the potential mechanisms by which circadian rhythm affects KSD.
2 Biological characteristics of the circadian clock
The central circadian rhythm clock in the suprachiasmatic nucleus (SCN) is in the anterior part of the hypothalamus. It synchronizes with Earth’s time and feeds back to the downstream brain and peripheral regions by sympathetic nervous system transduction and hormone secretion after light signals from the light-dark cycle are received by the retina and transmitted to the SCN as electrical signals (10, 24). Some synchronization factors also called “time givers” or zeitgebers vary with temperature, diet, pharmacological manipulation, and social interactions (25). Additionally, peripheral organs, including the heart, liver, and kidney, participate in the “peripheral clock” and regulate cyclic physiological functions by manipulating the transcription of circadian genes, protein synthesis, energy metabolism, and so on. Both the central and peripheral clocks essentially share the same molecular structure, but the relationship between them remains unclear (10, 26).
At the molecular level, approximately 10% of genes are clock-controlled genes (CCGs) with circadian oscillations, also called circadian clock genes. The maintenance of circadian rhythm depends on a transcription-translation negative feedback loop formed by a series of interacting clock genes (25, 27, 28) (see Figure 1). The cycle of potential molecular mechanisms generating circadian rhythm is approximately 24 hours without synchronizing input; the central-peripheral network can adapt to a limited range of day lengths (29, 30). Some genes and regulators that are vital for initiating and maintaining circadian rhythm have been well investigated, such as the circadian locomotor output cycles kaput (CLOCK), a gene encoding the protein related to the length and persistence of a circadian circle (2); brain and muscle aryl hydrocarbon receptor nuclear translocator-like protein-1 coding gene (BMAL1), encoding the basic-helix–loop-helix transcription factor BMAL1 protein (2); the period family (PER1/2/3), key regulators in the cell cycle (31); cryptochrome 1&2 (CRY1/2), the main part of the negative feedback loop of the circadian clock and REV-ERBα/β, members of the orphan nuclear receptor family, which play a key role in regulating the expression of CLOCK and BMAL1 (32, 33). These circadian clock genes regulate the day-night cycles by both positive and negative feedback cycles in the SCN and peripheral tissues and organs (10).
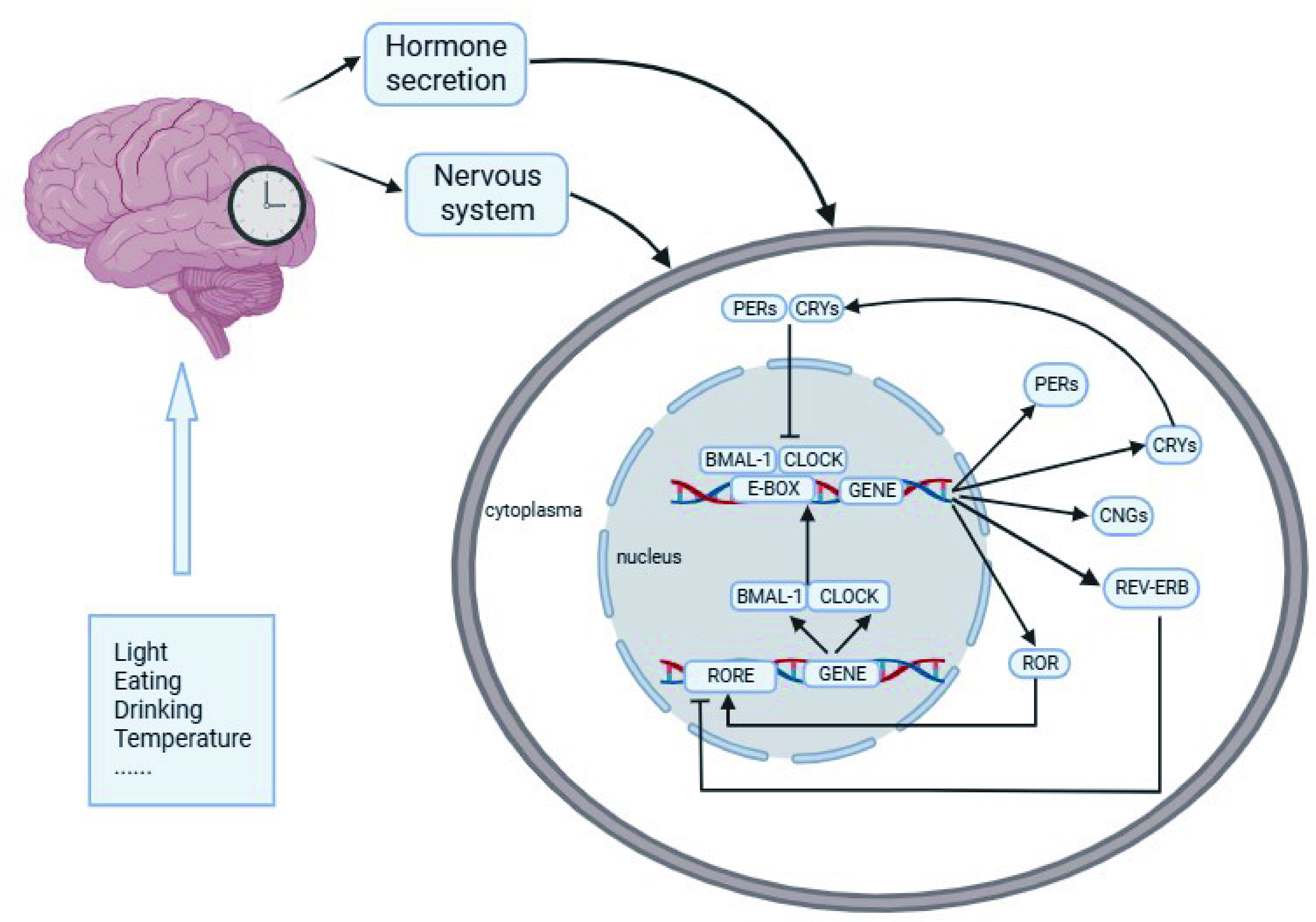
Figure 1 Molecular mechanism of the circadian rhythm. After cues from Zeitgebers of light, temperature, and feeding are perceived and transmitted to the SCN as electrical signals, the central circadian clock system will synchronize with geophysical time and feedback to the downstream brain regions and peripheral organs through the nervous system and hormone release. CLOCK and BMAL1 act as the center transcription factors of a heterodimer complex and activate the transcription of PER, CRY, REV-ERβ and RORα by cis-acting E-box and ROR elements. A multimeric complex formed by the PER/CRY proteins subsequently enters the nucleus to inhibit CLOCK/BMAL1 activity. Then, REV-ERBα and ROR proteins compete for response RORα/CLOCK/BMAL1 transcription. REV-ERBα reduces CLOCK/BMAL1 transcription, while RORα induces it. The main circadian genes are reactivated by the last with a new cycle beginning, and this feedback loop occurs at approximately 24 h.
3 Mechanism of KSD
KSD is a common urological disease with a complicated pathological process. The major types include calcium stones (calcium oxalate [CaOx] & calcium phosphate [CaP]), uric acid (UA) stones (UAS), struvite stones (infection stones), and cystine stones. The biochemical process of stone formation is successive and complex and involves various physicochemical changes. Generally, four steps participate in pathogenesis: urinary supersaturation, crystal nucleation, growth, and aggregation. Crystal formation from supersaturated urine retained in the kidney is the driving force (16, 34). Then, the crystals gather together to grow to a size as further aggregation, which can interact with intrarenal structures (also called crystal-cell interaction) to cause renal tubular epithelial cell (RTEC) injury (34, 35). Crystal-cell interaction leads to the movement of crystals from the basolateral side of cells to the basement membrane and results in the retention of crystals in the kidney or collecting duct to eventually form the clinical stone (34, 36). Furthermore, a plaque of calcium deposited in the interstitial tissue of the renal papilla observed by electron microscopy, called Randall’s plaque (RP), appears to be the origin of urinary stones since it contributes greatly to crystal retention (37, 38). It should be noted that some factors are reported to be critical modulators for stone formation, including promoters and inhibitors. Promoters are receptors or receptor-like features that play vital roles in crystal−cell interactions for crystal retention in the kidney, while inhibitors can decrease crystallization and inhibit crystal aggregation and/or adhesion to RTECs. For example, serum calcium and vitamin D (vit D) act as promoters in KSD, while some metallic cations, such as magnesium, can inhibit crystal growth and aggregation (16, 39, 40). It is now widely accepted that stone formation usually depends on the imbalance between promoters and inhibitors.
4 Epidemiological evidence of circadian rhythm disruption in KSD
Light is the main zeitgeber that regulates the 24-h circadian cycle. Sleep and wakefulness can coregulate the circadian rhythm and maintain sleep homeostasis with light exposure. However, the day-night circadian rhythm has been altered greatly with the invention of incandescent lighting (2, 41). Circadian sleep disorder is defined medically as an inability to sleep at the desired time rather than an inability to sleep, for instance, staying up late working (night shift work) and rapidly travelling to new time zones (social jet lag [SJL]). Both shift work and SJL are popular and involve a large population and diverse professions (42, 43). Altered sleep-wake cycles disturb the internal circadian clock. Growing evidence shows that disordered circadian sleep and sleep insufficiency are closely related to multiple disorders, including KSD. Yin et al. (44) investigated the relationship between sleep status and KSD risk in a cross-sectional study and found that short sleep duration (< 7 h) was associated with a higher KSD prevalence than normal sleep duration (7-9 h) (odds ratio [OR] = 1.21, 95% confidence interval [CI]: 1.08 to 1.35). Another large population-based study obtained similar results, and it estimated the sleep score to assess sleep quality according to a previous study and indicated that a reduced sleep score led to increased KSD risk (hazard ratio [HR] = 1.07, 95% CI: 1.05 to 1.10) (45, 46). A prospective study in China measured sleep quality using the Pittsburgh Sleep Quality Index (PSQI) and KSD prevalence, and the PSQI was positively correlated with KSD prevalence (OR=1.18, 95% CI: 1.08 to 1.28) (47). Another sleep disturbance, obstructive sleep apnea (OSA), is common, with an incidence of 4.0% to 32.8% for middle age and 22.4% for older than 60 years (42), and has been confirmed to be a risk factor for KSD (HR=1.35, 95% CI: 1.23 to 1.48) (48). A retrospective study including 127 patients performed a univariable comparison of 24-h urine components and found that OSA is related to changes in urinary analytes that promote KSD (49).
Although several studies have elucidated that disturbed circadian rhythm can induce KSD, the endogenous mechanism is poorly understood. KSD is a multifactorial systemic disease with diverse triggers. Based on epidemiological studies, metabolic syndrome, hypertension, inflammatory bowel disease, microbiome dysbiosis, parathyroid hormone disorder, and vitamin D deficiency can induce KSD, and they are related to a disrupted circadian clock (50–52) (Figure 2).
5 Association between circadian rhythm disturbance and KSD
Sleep and circadian rhythm disturbance is associated with alterations in circadian gene expression, and mammals with specific circadian gene deficiencies or mutations exhibit abnormal sleep/awake rhythms (1, 53–55). Here, we comprehensively summarize some potential links between circadian rhythm disturbance and KSD. The factors related to KSD that are affected by sleep and circadian rhythm disturbance and the mechanisms are described as follows.
5.1 Glycolipid metabolism disorder (metabolic syndrome)
Metabolic syndrome (MetS) is a cluster of conditions that usually occur together to increase the risk of T2D and CVD, including hypertension, chronic hyperglycemia (T2D and impaired glucose tolerance [IGT]), and dyslipidemia (obesity, decreased high-density lipoprotein [HDL] level, increased low-density lipoprotein [LDL] or total cholesterol [TC] level), and its prevalence is approximately 25% of adults with an increasing trend at advanced ages (56, 57). MetS is confirmed to be an independent risk factor for KSD (OR=1.49, 95% CI: 1.26 to 1.76) (58, 59). The pathological mechanism of MetS in KSD mainly consists of insulin resistance (IR), hyperglycemia, and vascular dysfunction (59, 60).
MetS is significantly associated with circadian rhythm disturbance. A new concept, circadian syndrome (CirS), was suggested to indicate MetS related to disrupted circadian rhythm (61, 62). Xiao et al. first reported the relationship between CirS and KSD prevalence in a cross-sectional study and obtained similar results (OR=1.42, 95% CI: 1.06 to 1.91) (23). A longitudinal analysis from Canada investigated 393 participants for a mean 6-year follow-up and found that short sleepers were at significantly higher risk of MetS (relative risk [RR]=1.74; 95% CI: 1.05-2.72) (63). The large population-based study by Bayon et al. revealed that shift work was correlated with higher MetS risk (OR=4.45, 95% CI: 1.36 to 14.56) (64). The cross-sectional data from the New Hoorn Study cohort indicated that SJL was correlated with MetS with a prevalence ratio of 1.62 (65). Additionally, OSA can cause MetS and increased waist circumference (WC) and triglyceride (TG) levels in MetS can induce OSA (66, 67). Here, we reviewed the effects of MetS on KSD from two aspects.
5.1.1 Type 2 Diabetes (T2D)
The KSD prevalence was higher in T2D patients than in healthy controls (21% vs. 8%, p < 0.05), and the recurrence rate was twice as high (2.1 vs. 1.3, p < 0.05) (68, 69). A cohort study showed that the population with T2D had a higher KSD risk (OR=2.44, 95% CI: 1.84 to 3.25) (70). IR can hamper the ability of ammonia genesis to respond to acid load in the kidney, which results in hyperacid urine and elevated urinary calcium excretion by compensatory hyperinsulinemia (60, 68). Oxidative stress (OS) is another link between T2D and KSD; increased reactive oxygen species (ROS) generated by disordered glycometabolism, oxidative damage of β-cells in the pancreas, and endothelial dysfunction cause OS and inflammation in RTECs, resulting in increased levels of superoxide dismutase and malondialdehyde and decreased levels of antioxidants (71). These factors eventually lead to KSD.
Glycometabolism is a complicated physiological process and presents a significant diurnal oscillation. Variations in both the secretion and sensitivity of insulin display obvious circadian rhythms, and glucose tolerance (GT) is higher in the morning than in the evening in healthy people, but this oscillation disappears in T2D patients and IGT appears when the circadian oscillation is damaged (72–74). Many studies have revealed that the risk of IR and T2D is higher in populations with sleep disorders, SJL, and shift work, and it may be reduced by extended sleep duration and circadian readjustment strategies (11, 75). Cui et al. investigated the association between sleep duration and the risk of T2D in a case-control study and found a U-shaped association (≤ 6 h, OR = 1.74, 95% CI: 1.01 to 3.01; 8-9 h, OR = 1.46, 95% CI: 1.04 to 2.06) compared to sleep duration of 6-8 h, in which abnormal sleep duration increased the T2D risk (76). A prospective study including night workers and day workers with 4 years of follow-up showed that the risk of obesity and T2D was 5 times higher in night shift workers than in daytime workers (77). Then, a meta-analysis assessed the association between shift work and T2D and revealed that shift work increased the risk of T2D (RR=1.10, 95% CI: 1.05 to 1.14), and the trend was similar in the population with SJL (78, 79).
The possible relationship between disrupted circadian rhythm and T2D has been clarified by numerous studies. Rodents with SCN dysfunction display global or partial circadian gene disruption and easily developed IGT, hyperglycemia, hyperinsulinemia, decreased insulin secretion and sensitivity, and β-cell defects in the pancreas (80–82). Clock mutant mice show hyperglycemia, decreased levels of expression, and phase shifts of RNA oscillation of genes that participated in glucose sensing, insulin signaling, islet growth, and development (83, 84). It also disrupts hepatic glycogen oscillation and changes the circadian mRNA and protein expression of glycogen synthase 2 (limiting enzyme in glycogenesis) in mice (85). Whole-body Bmal1 knockout (KO) obliterates the systemic insulin sensitivity rhythm to develop increased fasting blood glucose, glucose intolerance, and hypoinsulinemia (83, 86). Severe IGT, increased glycemia levels, and decreased insulin secretion are found in pancreas- or β-cell-specific Bmal1 KO mice (87). The whole-body Cry1/2 KO severely damages glucose homeostasis with IGT (88). Another study by Zhang et al. demonstrated that the hepatic overexpression of Cry1 results in decreased gluconeogenesis and lower glycemia in diabetic mice (89). Qian et al. investigated Per1:LUC transgenic rats exposed to light during the night for 10 weeks and showed disruption of islet circadian clock through impairment in the amplitude, phase, islet synchrony of clock transcriptional oscillations, and diminished glucose-stimulated insulin secretion (90). Furthermore, Per2 inhibition decreases glycemia levels and gluconeogenesis and stimulates insulin secretion (91). Then, global Rer-erbα/β dual KO mice exhibit hyperglycemia by disturbing the insulin signaling pathway (92). Meanwhile, Rev-erbα mutant mice fed a chow diet have slight hyperglycemia without IR, which can be explained by REV-ERBα affecting glycemia by regulating glucose-6-phosphatase and phosphoenolpyruvate carboxylase (93).
In summary, primary findings indicated that a disturbed circadian rhythm leads to T2D mainly by regulating enzymes and insulin in glycometabolism. However, direct evidence about how circadian disorders affect T2D and KSD remains unclear.
5.2 Lipid abnormalities and obesity
Lipid metabolism disorders are common in KSD patients. A retrospective study enrolled 2242 patients with KSD and found that high TC levels were significantly associated with higher UAS risk (p=0.006), and the 24-h urine analysis presented a significant positive correlation between low HDL levels and lower urine pH and higher urinary oxalate and uric acid levels (94, 95). A demonstrably increased KSD risk in the obese population was observed in a cohort study, and in particular, an increased level of visceral adiposity was a risk factor for hypercalciuria and UAS (OR=3.64, 95% CI: 1.22 to 10.85) (96, 97). Obesity promotes systemic inflammation and OS, leading to tissue immune cell infiltration and contributing to stone formation. It facilitates the expression of adipokines and some inflammatory molecules, such as tumor necrosis factor-α and interleukin-6, which were detected in the renal tissue and urine of KSD patients (98). Furthermore, calcifications by lipid deposition within the hyperosmotic turbulent vasa recta erode into the nearby collecting system and interstitium to promote RP formation, which is further confirmed by the presence of cholesterol identified in stones and renal papillary (95).
Lipid metabolism is precisely regulated by the circadian clock. Plasma lipids present a day-night variation within a narrow range independent of food intake, with the peak level of HDL in the early rest phase and a decrease in the active phase (99, 100). Circadian rhythm disturbance causes lipid abnormalities and obesity. A study investigated the association between sleep duration and obesity in children and adolescents and revealed that short sleep duration increases the risk of obesity (OR=1.69, 95% CI: 1.25 to 2.29) and elevated WC (OR=1.49, 95% CI: 1.13 to 1.97), which is similar to the study by Brocato et al. (101, 102). Another longitudinal investigation including 815 workers showed that workers with greater SJL are more likely to be obese (OR=1.20, 95% CI: 1.00 to 1.50) (103). In addition, circadian rhythm disturbance alters the plasma lipid profile by increasing the levels of cholesterol, TGs, and LDL and decreasing the level of HDL (104, 105).
Clock gene expression alteration has been comprehensively verified in both human and animal models. Vieira et al. analyzed the 24-h pattern of clock gene expression in an obese population and showed that the expression of CRY2 and REV-ERBα was upregulated in obese participants. A positive correlation was observed between REV-ERBα expression and body mass index and WC in the obese population. The expression of CLOCK was positively correlated with LDL and RORα with HDL levels. Obese people with MetS presented a positive correlation between PER2 expression and LDL, while REV-ERBα was correlated with WC. CRY2 and REV-ERBα are considered clock genes upregulated in obesity (106). Hepatic PER2, PER3, and CRY2 showed lower expression in the obese groups than in the normal control group (107). In animal experiments, the diurnal feeding rhythm is significantly impaired in homozygous Clock mutant mice, and they are more likely to develop hyperphagia, obesity, hyperleptinemia, and hepatic steatosis (80, 83). Microsomal TG transfer protein levels in the enterocytes of ClockΔ19/Δ19Apoe−/− mice are higher, and enterocytes secret more chylomicrons. ClockΔ19/Δ19 protein enhances intestinal cholesterol absorption, as well as the secretion of chylomicrons and cholesterol (108). The Bmal1 KO mice display ectopic body and liver fat formation, hyperlipidemia, increased circulating leptin levels, and the absence of glucose fluctuation, also presenting earlier signs of obesity under a high-fat diet (HFD) (86, 109). In liver-specific and global Bmal1−/− mice, an elevation of circulating free fatty acids and higher TG formation is detected and can be reversed by Bmal1 overexpression (110). Adipose-specific Bmal1−/− mice show increased weight gain and fat formation with increased calorie intake during the daytime (84). Per1/2/3 triple-deficient mice are more likely to be obese, suggesting a potential role in body weight regulation (111). Moreover, PER1 is identified to bind with major hepatic enzymes in bile acid synthesis, and Per1 expression can be enhanced to increase fat absorption and accumulation in mice (112). Per2 KO mice gain an altered lipid profile and downregulated triacylglycerol levels, while Per2 deficiency in fibroblasts can promote adipocyte differentiation via the direct interaction with PPARα/γ and their target genes (113, 114). Per3 KO promotes adipogenesis in vivo by a clock output pathway in which PER3 and BMAL1 directly affect transcription factor Klf15 expression in adipocyte precursor cells (115). In addition, Cry1/2 double null mice have abnormal serum and hepatic TG levels (88). Furthermore, Rev-erbα deficiency increases plasma lipid levels and decreases hepatic cholesterol and TG levels (116).
Although circadian genes in lipid abnormalities have been extensively studied, the pathological process in KSD deserves further research.
5.3 Hypertension
The kidney is the central organ that regulates blood pressure (BP). Poorly controlled BP results in kidney diseases and influences BP regulation in positive feedback (117). According to historical clinical investigations, hypertension (HTN) may correlate with KSD. Cappuccio et al. recruited 688 male workers in a cross-sectional study and found the relative risk of hypertensive participants having a history of KSD was twice that of the normal group (OR=2.11, 95% CI: 1.17 to 3.81), which is similar to the larger cohort study based on U.S. population by Hill et al. (RR=1.79, 95% CI: 1.19 to 2.71) (118). Data from a prospective cohort proved this finding (119, 120). A study confirmed that HTN can be an independent predictive determinant for recurrent KSD, especially in non-obese SFs (121). Inconsistently, the studies published by Madore et al. showed that the KSD incidence was comparable in both the hypertensive population and normal population (OR= 0.99, 95% CI: 0.82 to 1.21), and similar results were obtained when limited to middle-aged women (122, 123). The inconsistency of diagnostic criteria for HTN can lead to opposite results, and it is essential to remeasure the association in new criteria. Considering the widespread popularity of HTN and the link between HTN and metabolism, it cannot be ignored. Currently, the etiology of HTN in KSD consists of an alteration of urine components, IR, inflammation, and OS (124). Increased urine calcium excretion is caused by central volume expansion (the ‘central blood volume’ theory), and higher excretion levels of oxalate and uric acid were detected in hypertensive patients (119, 124, 125). Furthermore, ROS overgeneration by the activated renin-angiotensin-aldosterone system (RAAS) promotes RTEC injury and crystal formation (124, 126).
BP in healthy individuals exhibits precise daily variation, which is characterized by an increase after awakening followed by a decrease at night during sleep (1, 127). Disturbed circadian rhythm eliminates the diurnal rhythm of BP, resulting in elevated BP that transfers to HTN (127–129). Grandner et al. analyzed more than 700,000 adults from two large cohorts to illustrate that the HTN risk was higher in sleep deficiency compared to 7 h (≤ 4 h: OR=1.86, ≤ 5 h: OR=1.56, ≤ 6 h: OR=1.27, p < 0.0005 for all) (130). A dose-response meta-analysis found a higher HTN risk for shorter sleep duration, and previous meta-analyses reported similar results to strengthen this association (47, 131). For OSA patients, the HTN risk was higher than that in healthy controls (OR=2.84, 95% CI: 1.70 to 3.98) and was positively correlated with the OSA grade (132). Additionally, night shift workers have a higher HTN risk than normal controls, which increases with an increasing frequency of night shift work (133). Although there is no significant association between SJL and HTN in historical studies, it is worth noting that a recent study presented a morning BP surge caused by acute SJL (134, 135).
The underlying mechanism of disturbed circadian rhythm in HTN is complicated. First, in OSA patients, sympathetic nervous system overactivity, disruption by OS, and inflammation in vascular structure and functions contribute to the abnormal diurnal pattern of BP (132, 136). Intermittent hypoxia (IH) and negative pressure against obstruction activate adrenal, renal, and peripheral chemoreceptors to increase the circulating levels of hormones such as renin and catecholamine and decrease nitric oxide (NO) synthesis, leading to upregulated sympathetic system activity (136, 137). The interactions between the sympathetic system and the kidney secondarily activate RAAS to increase BP (138). Additionally, IH disrupts endothelial NO expression by promoting ROS generation. Endothelial dysfunction is mainly caused by inhibited NO bioactivity and bioavailability impairs vascular vasodilation and enhances vasoconstriction (25, 136).
Circadian gene abnormalities also trigger HTN. Clock mutation represses the expression level of Atp1b1, which encodes the β1 subunit of the Na+/K+-ATPase to elevate BP (139). In hypertensive rodents, myeloid-specific deficiency of Bmal1 exacerbates vascular remodeling and accelerates HTN formation by influencing the profibrotic macrophage phenotype (140). A human study showed a higher level of Per1 mRNA in the renal medulla in the hypertensive group than in the normal control group, suggesting a role for Per1 in the regulation of BP by renin (141). Doi et al. examined BP regulation in global Cry1/2 double null mice and revealed that Cry1/2 KO mice developed salt-sensitive HTN compared to wild-type (WT) mice (142). Surprisingly, KO or mutation of circadian genes also causes the absence of diurnal rhythm in BP and significantly decreased BP (127, 128, 143). To explain this, glycolipid metabolism disorders should be considered due to the close link between HTN and MetS (143, 144). Moreover, the relationship between circadian genes and the proteins expression in the local kidney that play roles in water and electrolyte balance is inspiring. The sodium chloride (NaCl) co-transporter (NCC) is involved in sodium reabsorption and BP maintenance and Richards et al. proved that Per1 inhibition reduces NCC expression and results in lower BP in mice (145). Zuber et al. also investigated the intrinsic circadian rhythm system and found that Clock mutant mice exhibit significant alterations in the renal expression of several key regulators of water or sodium balance (vasopressin V2 receptor, aquaporin-2, aquaporin-4, epithelial sodium channel), which functionally leads to dysregulation of sodium excretion rhythms and a significant decrease in BP (146). In addition to circadian genes, serum- and glucocorticoid-induced kinase 1 (SGK1) in renal tubules, a clock-controlled and glucocorticoid receptor- and mineralocorticoid receptor-induced gene was recently shown to participate in BP circadian regulation. Staub et al. generated a tubular-specific Sgk1 KO model and found that Sgk1 deletion elevates pulse pressure by increasing the circulating aldosterone level and disrupts the BP rhythm (147).
Together, these studies indicated that circadian rhythm disturbance can disrupt BP homeostasis bidirectionally. However, since epidemiological studies focused on KSD and HTN are full of controversies, this hypothesis needs further verification.
5.4 Inflammatory bowel disease
Inflammatory bowel disease (IBD) is an autoimmune disease characterized by chronic intestinal granulomatous inflammation and includes two main types: Crohn’s disease (CD) and ulcerative colitis (UC) (148, 149). KSD is one of the most frequent extraintestinal manifestations of IBD, with a higher prevalence in IBD patients than in normal controls. In the cohort from Mississippi, 6% and 6.7% of CD and UC patients developed KSD, respectively, which is similar to the data in Switzerland (150–152). An observational study revealed that UC was a risk factor for KSD (OR = 4.2, 95% CI: 1.1-15), and the KSD prevalence in UC and CD was comparable (153). The pathological process of IBD in KSD was exhaustively reviewed by Corica et al. (152). Briefly, UA supersaturation by low urine volume and pH, hypercalciuria by bile salt malabsorption, increased colonic epithelium permeability to oxalate, and decolonization of Oxalobacter formigenes (O. formigenes) are radical.
IBD is strongly affected by circadian rhythm disturbance. The IBD incidence was significantly higher in patients with shorter sleep durations than in normal controls (HR = 1.51, 95% CI: 1.10 to 2.09) in a 10-year follow-up analysis (154). A retrospective study enrolled 115 IBD subjects and 76 healthy controls to measure chronotype, SJL and sleep debt (SD), which showed that later chronotype was negatively correlated with severe IBD (r = -0.209, p < 0.05) and that SJL was higher in the IBD group than in the controls (1.32 h ± 1.03 vs. 1.05 h ± 0.97, p < 0.05), while SD was also elevated in the IBD group compared to the controls (21.90 min ± 25.37 vs. 11. 49 min ± 13.58, p < 0.05) (155).
In IBD patients and animals, abnormal expression and status of clock genes are considered initial manifestations. Weintraub et al. analyzed clock genes in peripheral blood and intestinal mucosa and found that the expression levels of clock genes (CLOCK, BMAL1, CRY1, CRY2, PER1, and PER2) were significantly lower in both inflamed intestinal mucosa and leukocytes than in healthy controls, which was also reported in different tissues (peripheral blood monocytes, colon) (156–158). Rodents with artificially induced colitis displayed decreased Per2, Cry1, Rev-erbα, and Npas2 levels and increased Rorα in colon tissue (159). Kyoko et al. measured the tight junction proteins occludin and claudin-1 in Clock mutation ((Clock△19/△19) mice to show that mice lacking Clock have persistently low levels of these two proteins and were more susceptible to intestinal injury (160). Similarly, Bmal1 KO mice show worse UC and the absence of time-dependent variation in disease activity compared to Bmal1+/+ controls, and epithelial proliferation in the colon presents a daily rhythm in Bmal1+/+ controls but is absent in the Bma1 KO group, resulting in poor regeneration (161). Mice lacking Rorα or Bmal1-driven Lnc-UC (a long noncoding RNA that is associated with colitis, particularly by reducing Rev-erbα expression) are more likely to have colitis than the control group. Lnc-UC deactivates the activity of NLR family pyrin domain (NLRP) 3, which is essential in the induction of proinflammatory cytokines (159, 162, 163). Bmal1 KO also leads to a lower level of regulatory B cells in the intraepithelial region, which expresses highly programmed death ligand 1 to alleviate colitis severity (164). Then, colitis is more severe in Per1/2 KO mice than in WT mice, with decreased Paneth cells, goblet cells, lysozyme transcripts, and lysozyme proteins (165). Oh et al. presented that intestinal epithelial-specific Rorα KO leads to severe inflammation by reducing the level of Ki67, a cell proliferation marker, and p-DRP1, a molecule active in ATP production (162). All the evidence indicates that IBD is related to abnormal expression of circadian genes.
All these studies demonstrated that circadian rhythm disturbance can promote KSD via IBD. However, further research is necessary to better clarify the internal molecular pathways in the process of KSD.
5.5 Microbiome dysbiosis
Microbiomes coexisting with humans are important in maintaining health and causing diseases. The gut microbiome (GMB) is a large set of microorganisms that colonize our digestive tract, and the diverse mixture of bacteria within the genitourinary tract (often at low levels) is defined as the urinary microbiome (UMB) (166–168). Dysbiosis of GMB and UMB contributes greatly to KSD (168). The colonization rate of O. formigenes in CaOx stones has attracted great attention. Several studies have proven that the colonization rate of O. formigenes is lower in SFs than in controls (169–171). Stern et al. studied the distinct GMB in SFs and non-SFs and revealed that Bacteroides was 3.4 times more abundant in SFs than in non-SFs (34.9% vs. 10.2%, p = 0.001), and Prevotella was 2.8 times more abundant in non-SFs than in SFs (34.7% vs. 12.3%, p = 0.005). In urinary analysis, Eubacterium was negatively correlated with oxalate levels, and Escherichia tended to have an inverse correlation with citrate levels (172). Compared to GMB, UMB in KSD is underexplored due to dramatic variation (168, 173). A case-control investigation profiling the UMB in male patients with calcium-based stones found that the UMB diversity was markedly lower than that in healthy controls, and the components were also different in the two groups (p < 0.001). The taxa at the genus level that significantly differentiated the two groups were Prevotella in the normal group and Acinetobacter in the KSD group (174). A meta-analysis including 8 studies indicated that the abundance of Bacteroides, Lactobacillus, and Prevotella showed the most significant difference in GMB between KSD patients and healthy controls (175). In UMB, Escherichia coli (E. coli), Lactobacillus, Staphylococcus, Streptococcus, and Klebsiella are considered vital in KSD based on evidence in vivo and in vitro (166, 168, 176–178). The pathogenic mechanism of GMB is poorly understood, oxalate accumulation by oxalate degradation dysfunction and related metabolic disorders is widely recognized (166, 179). Meanwhile, urease enzymes and inflammation are also important (16, 180).
Microbiomes have been found to follow a strict circadian rhythm (167, 181). Up to 60% of the total microbial composition oscillates rhythmically, which translates to diurnal fluctuations in 20% of commensal species of the GMB in mice and 10% in humans. The GMB abundance of ad libitum-fed mice under a strict light-dark cycle was measured at the changing point, and significant diurnal fluctuations were identified in the abundance of more than 15% of the GMB (182). Circadian misalignment disrupts GMB homeostasis and causes diseases. Smith et al. explored the correlation between GBM diversity and sleep physiology to show that diversity was positively correlated with sleep efficiency and duration. Sleep duration reduction can significantly decrease GBM diversity (183, 184). Additionally, exposure to SJL exacerbated GBM and metabolite homeostasis in the jejunum and colon of mice and was also detected in humans (181, 185).
Studies support that clock gene expression alterations contribute to GMB dysbiosis. Compared to WT mice, Clock mutant (ClockΔ19/Δ19) mice have a decreased Firmicutes/Bacteroidetes ratio, especially when paired with alcohol consumption or HFD (186). Liang et al. constructed Bmal1 KO mice and found that Bmal1 KO abolishes the oscillation and composition of GMB with a decreased abundance of Prevotella and an increased abundance of Bacteroides (187). In Per1/2-deficient mice, Bacteroides and Lactobacillus lost oscillations in relative abundance (182). Furthermore, in the interaction between GBM and MetS, dysbiosis significantly promotes the development of MetS. A high-sugar diet and HFD can aggravate the impact of circadian disorganization on GBM and further disturb glycolipid metabolism (188–190). In UMB, studies are limited. The diurnal oscillation in Streptococcus pneumoniae (S. pneumoniae) is driven by external clues, such as temperature (191). Per1 mutant flies are more sensitive to S. pneumoniae, and the elevated infection sensitivity can be a consequence of the circadian regulation disturbance of phagocytosis in these fly mutants (192, 193). In E. coli, circadian rhythms are driven by special genes, including radA and KaiC (194). Furthermore, a red and blue photoreceptor was contained in E. coli to adapt to the day-night cycle, which may provide evidence for dysbiosis in abnormal light exposure by circadian disturbance (195).
These studies provide a preliminary indication of the correlation between MB and KSD. However, data from mammals are still necessary, and since there is an interaction between GMB and UMB, further research is needed.
5.6 Other factors
5.6.1 Parathyroid gland hormone disorder
Disordered calcium metabolism contributes greatly to KSD. Goodman et al. concluded that the risk of hypercalcemia was 9 times higher in KSD patients than in normal controls (196). Parathyroid hormone (PTH) is essential to enhance calcium reabsorption and inhibit phosphorus reabsorption in the kidney (197). Elevated PTH with hypercalcemia in primary hyperparathyroidism (PTHP) is a well-recognized reason for KSD (198). An observational study revealed that parathyroidectomy was effective in the therapy of KSD recurrence by idiopathic hypercalciuria and that stone activity significantly decreased after surgery (0.05-0.15 vs 0.50-0.75, p < 0.001) (199, 200).
The PTH level exhibits a bimodal pattern over 24 hours, with a maximum peak in the afternoon and a small peak at night (201). Circadian impairment leads to abnormal PTH levels. Higher serum PTH level was observed in patients with moderate OSA and severe OSA than in healthy controls (63.11 ± 36.11 and 53.16 ± 25.29 vs 43.71 ± 24.45, p < 0.05) (202). Then, Sleep disturbance is common in PTHP patients, but the causation is unclear (203, 204).
Clock genes in the parathyroid gland (PTG) were explored, and their disturbance caused parathyroid gland dysfunction (205). Normal circadian clock operation was confirmed in animal models with a periodicity of 24 hours and was significant for Bmal1, Npas2, Per1,2,3, Cry1,2, and Rev-Erbα. In hyperplastic PTG tissue, circadian genes were deregulated, with significant upregulation of Per1,2 and Rev-Erbα and downregulation of Npas2 (206). Egstrand et al. investigated the alteration of circadian genes during a 24-h cycle in murine PTG and found rhythmic expression of parathyroid signature genes, and this rhythm is essential for PTG function regulation. Mice with PTG-specific Bmal1 knockdown (PTHcre;Bmal1flox/flox) were created, and a global decrease in circadian genes was observed, including Clock, Npas2, Cry1,2, and Per1,2,3. Compared to WT, PTHcre;Bmal1flox/flox shows a higher parathyroid cell proliferation response and led to PTHP (205). The identification of transcriptional patterns in human PTG tissues presents that the transcript expression levels of PER1 and CRY1/2 are significantly lower in PHPT tissue than in healthy tissue (207).
Taken together, these studies indicate that circadian gene disturbance leads to PTG disorder and is a risk factor for KSD. However, more investigations are needed.
5.6.2 Vitamin D Deficiency
Vitamin D (vit D) participates in maintaining calcium homeostasis, and vit D deficiency (VDD) is defined as a serum 25-hydroxyvitamin-D [25(OH)D] concentration less than 30 ng/ml (208). VDD affects a large population globally and is more prevalent in KSD patients (209). The investigation by Elkoushy et al. showed that more than 80% of KSD patients have VDD, which is consistent with a multicenter study (210, 211). A case-control study in Spain including 366 participants found that calcium SFs had lower levels of Vit D (25.7 vs. 28.4 ng/ml, p = 0.02) and a higher percentage of VDD than non-SFs (28.0% vs. 15.7%, p = 0.009) (212). Vit D is a fundamental regulator of systemic inflammation, OS, and mitochondrial respiratory function. Based on current studies, cell injury by OS and inflammation by overproduced ROS are the cardinal pathogenic factors for KSD in VDD (213, 214).
Vit D status is significantly influenced by a circadian rhythm in which serum Vit D presents a significant diurnal rhythm with a nadir in the morning and is followed by a rapid increase to a plateau during the day (215). VDD is widespread in populations with abnormal light exposure or insufficient sleep (216). Piovezan et al. indicated that short sleep duration showed an independent association with VDD (OR = 1.61, 95% CI: 1.25 to 2.26) (217). Then, shift workers are more likely to develop VDD. An investigation in Italy measured serum 25(OH)D in workers and observed that the level was lower in night shift workers than in daily workers (13.4 ± 5.3 ng/mL vs. 21.9 ± 10.7 ng/mL, p < 0.001) (218, 219).
Endogenous vit D is synthesized in the skin from the cholesterol-like precursor (7-dehydrocholesterol) present in epidermal cells by exposure to ultraviolet B (UV-B) from sunlight (220). Therefore, a lack of UV exposure reduces vit D levels and exacerbates OS in RTECs, which leads to KSD (129, 218). Moreover, research on the interaction between VDD and circadian genes is limited. Kwai et al. created an intestinal Bmal1 KO model (Bmal1Int-/- mice) and found that the vit D receptor (VDR) target genes in the intestine are disrupted. The expression of VDR and Vdr peaks at ZT8 (zeitgeber time [ZT]: light on, ZT0–ZT12; lights off, ZT12–ZT24) in the control group but disappears in Bmal1Int-/- mice. The experiment in Caco-2 cell lines also reveals that the BMAL1 KO reduced VDR and VDR expression (221). However, other circadian genes are still unclear in VDD, which is worth more analysis.
5.6.3 Renal Tubular Damage or Dysfunctions
The combination of urinary supersaturation and renal tubular damage is vital in stone formation. Renal tubular damage is related to sleep disorders, especially OSA (222, 223). The high oxygen demand of renal tubules makes them vulnerable to hypoxia by chronic IH in OSA and easily advanced to renal tubular injury (223). However, such a theory needs to be backed up by more research, and its relationship with KSD should be further elucidated based on direct evidence.
Distal renal tubular acidosis (dRTA) syndrome is a condition caused by the acidification defect in the collection tubule and the inability of the distal nephron to maximally increase the urinary secretion of protons ([H+]) in the presence of metabolic acidosis, characterized by a persistent hyperchloremia, normal plasma anion gap and metabolic acidosis with a relatively normal glomerular filtration rate. Patients with dRTA have elevated urinary calcium, recurrent CaOx or CaP stone formation, and nephrocalcinosis (224, 225). In addition to inherited dRTA, secondary dRTA is caused by numerous triggers, including autoimmune diseases, nephrotoxins, and miscellaneous aetiologies. Currently, there are insufficient epidemiological and basic studies to confirm the relationship between dRTA and circadian rhythm disturbance.
6 Future perspective
Growing evidence indicates that some therapeutic strategies enhancing circadian clock function or circadian gene expression may be beneficial for the prevention of KSD. First, feeding time is one of the most important external Zeitgebers in peripheral tissues, and unhealthy feeding time promotes diseases. A systematic review suggested that fasting results in altered urine metabolites and density, although this did not transfer to clinical outcomes. Safe fasting practices are vital for high-risk patients to prevent KSD (226). For OSA patients, continuous positive air treatment significantly reduces tubular damage, which may decrease the KSD risk (223). Moreover, melatonin (Mel), a hormone released from the pineal gland against SJL and sleep disorders with anti-inflammatory and antioxidative functions, can prevent crystalluria and kidney damage caused by crystal formation and aggregation (227, 228). Song et al. found that Mel has protective effects on oxalate-induced endoplasmic reticulum stress and apoptosis via the activation of the adenosine 5’-monophosphate-activated protein kinase pathway in HK-2 cells (229). In addition, BMAL1 is a therapeutic target in vitro. BMAL1 overexpression stimulated the OS-related NRF2/HO-1 pathway to reduce CaOx stone formation. This suggests that maintaining normal rhythms and properly intervening in some related circadian genes and downstream antioxidant pathways may benefit the prevention of CaOx stones (230). According to current limited studies, it can be speculated that artificial interventions in sleep status and circadian rhythm have the potential to prevent KSD. However, more clinical and basic research is needed.
7 Conclusions
Since KSD is a major challenge for global health, its potential mechanism should be investigated. Increasing convincing evidence has elucidated that a disordered circadian clock is a putative factor for KSD. This review summarizes the biological characteristics of the circadian rhythm, the mechanism of KSD, and the putative mechanism of the circadian rhythm disturbance in KSD. Existing clinical and basic studies have indicated that circadian rhythm-based interventions have potential clinical value in the management of KSD, but the specific and accurate mechanism of KSD caused by circadian rhythm disturbance is still unclear. KSD is not an isolated kidney disease, but a systemic disorder affected by various factors. Understanding the relationship between circadian rhythm and systemic multi-organ and multi-system health status is essential. In addition, both behavioral and pharmacological interventions related to rhythm modification deserve more research. A comprehensive and in-depth exploration of the mechanism of KSD caused by sleep and circadian rhythm disturbance and the efficacy of chronothrapies for KSD are necessary and can provide a new strategy for the clinical management of KSD.
Author contributions
SH: Conceptualization, Writing – original draft. JHW: Writing – original draft. TL: Writing – original draft. SY: Data curation, Writing – review & editing. JC: Writing – review & editing. YT: Supervision, Writing – review & editing. YX: Data curation, Writing – review & editing. JW: Conceptualization, Writing – review & editing. YB: Conceptualization, Writing – review & editing.
Funding
The author(s) declare financial support was received for the research, authorship, and/or publication of this article. This study was funded by the National Natural Science Foundation of China (Grant no. 82203298), the PostDoctor Research Project, West China Hospital, Sichuan University (2020HXBH027), and the Sichuan Science and Technology Program (2022YFS0306).
Acknowledgments
The authors sincerely thank the authors who shared the original dataset in this study.
Conflict of interest
The authors declare that the research was conducted in the absence of any commercial or financial relationships that could be construed as a potential conflict of interest.
Publisher’s note
All claims expressed in this article are solely those of the authors and do not necessarily represent those of their affiliated organizations, or those of the publisher, the editors and the reviewers. Any product that may be evaluated in this article, or claim that may be made by its manufacturer, is not guaranteed or endorsed by the publisher.
References
1. Fagiani F, Di Marino D, Romagnoli A, Travelli C, Voltan D, Di Cesare Mannelli L, et al. Molecular regulations of circadian rhythm and implications for physiology and diseases. Signal Transduct Target Ther (2022) 7:41. doi: 10.1038/s41392-022-00899-y
2. Chan M-C, Spieth PM, Quinn K, Parotto M, Zhang H, Slutsky AS. Circadian rhythms: from basic mechanisms to the intensive care unit. Crit Care Med (2012) 40:246–53. doi: 10.1097/CCM.0b013e31822f0abe
3. Fatima N, Rana S. Metabolic implications of circadian disruption. Pflugers Arch (2020) 472:513–26. doi: 10.1007/s00424-020-02381-6
4. Estanislau TB, Kruger K, Rosa-Neto JC, Padilha CS, Gonçalves DC, Minuzzi LG, et al. Feeding pattern, circadian rhythm, and immune function: what do we know about? Curr Pharm Des (2022) 28(30):2478–87. doi: 10.2174/1381612828666220729091451
5. Rhodes V, Maguire M, Shetty M, McAloon C, Smeaton AF. Periodicity intensity of the 24 h circadian rhythm in newborn calves show indicators of herd welfare. Sensors (Basel) (2022) 22:5843. doi: 10.3390/s22155843
6. Yamaguchi ST, Kobayashi R, Tomita J, Kume K. The regulation of circadian rhythm by insulin signaling in Drosophila. Neurosci Res (2022) S0168-0102(22):00194–8. doi: 10.1016/j.neures.2022.07.005
7. Konishi T, Takahashi Y, Shiina Y, Oike H, Oishi K. Time-of-day effects of consumption of fish oil-enriched sausages on serum lipid parameters and fatty acid composition in normolipidemic adults: A randomized, double-blind, placebo-controlled, and parallel-group pilot study. Nutrition (2021) 90:111247. doi: 10.1016/j.nut.2021.111247
8. Walker WH, Bumgarner JR, Walton JC, Liu JA, Meléndez-Fernández OH, Nelson RJ, et al. Light pollution and cancer. Int J Mol Sci (2020) 21:9360. doi: 10.3390/ijms21249360
9. Reutrakul S, Van Cauter E. Interactions between sleep, circadian function, and glucose metabolism: implications for risk and severity of diabetes. Ann N Y Acad Sci (2014) 1311:151–73. doi: 10.1111/nyas.12355
10. McAlpine CS, Swirski FK. Circadian influence on metabolism and inflammation in atherosclerosis. Circ Res (2016) 119:131–41. doi: 10.1161/CIRCRESAHA.116.308034
11. Masri S, Sassone-Corsi P. The emerging link between cancer, metabolism, and circadian rhythms. Nat Med (2018) 24:1795–803. doi: 10.1038/s41591-018-0271-8
12. Li H, Ou J, Li Y, Xu N, Li Q, Wu P, et al. ISX-9 potentiates CaMKIIδ-mediated BMAL1 activation to enhance circadian amplitude. Commun Biol (2022) 5:750. doi: 10.1038/s42003-022-03725-x
13. Peerapen P, Thongboonkerd V. Kidney stone prevention. Adv Nutr (2023) 14:555–69. doi: 10.1016/j.advnut.2023.03.002
14. D’Ambrosio V, Moochhala S, Unwin RJ, Ferraro PM. Why is diagnosis, investigation, and improved management of kidney stone disease important? Non-pharmacological and pharmacological treatments for nephrolithiasis. Expert Rev Clin Pharmacol (2022) 15:407–14. doi: 10.1080/17512433.2022.2082943
15. Barghouthy Y, Corrales M, Doizi S, Somani BK, Traxer O. Tea and coffee consumption and the risk of urinary stones—a systematic review of the epidemiological data. World J Urol (2021) 39:2895–901. doi: 10.1007/s00345-020-03561-w
16. Wang Z, Zhang Y, Zhang J, Deng Q, Liang H. Recent advances on the mechanisms of kidney stone formation (Review). Int J Mol Med (2021) 48:149. doi: 10.3892/ijmm.2021.4982
17. van de Pol JAA, van den Brandt PA, Schouten LJ. Kidney stones and the risk of renal cell carcinoma and upper tract urothelial carcinoma: the Netherlands Cohort Study. Br J Cancer (2019) 120:368–74. doi: 10.1038/s41416-018-0356-7
18. Dhondup T, Kittanamongkolchai W, Vaughan LE, Mehta RA, Chhina JK, Enders FT, et al. Risk of ESRD and mortality in kidney and bladder stone formers. Am J Kidney Dis (2018) 72:790–7. doi: 10.1053/j.ajkd.2018.06.012
19. Luke M, Ankith M, Shabbir HM, Rukshana S, Manuel FP. Epidemiological and biological associations between cardiovascular disease and kidney stone formation: A systematic review and meta-analysis. Nutrition Metab Cardiovasc Dis (2023) S0939475323003691. doi: 10.1016/j.numecd.2023.09.011
20. Cheungpasitporn W, Thongprayoon C, O’Corragain OA, Edmonds PJ, Ungprasert P, Kittanamongkolchai W, et al. The risk of kidney cancer in patients with kidney stones: a systematic review and meta-analysis. QJM (2015) 108:205–12. doi: 10.1093/qjmed/hcu195
21. Rodríguez D, Sacco DE. Minimally invasive surgical treatment for kidney stone disease. Adv Chronic Kidney Dis (2015) 22:266–72. doi: 10.1053/j.ackd.2015.03.005
22. Costello HM, Johnston JG, Juffre A, Crislip GR, Gumz ML. Circadian clocks of the kidney: function, mechanism, and regulation. Physiol Rev (2022) 102:1669–701. doi: 10.1152/physrev.00045.2021
23. Xiao Y, Yin S, Bai Y, Yang Z, Wang J, Cui J, et al. Association between circadian syndrome and the prevalence of kidney stones in overweight adults: a cross-sectional analysis of NHANES 2007-2018. BMC Public Health (2023) 23:960. doi: 10.1186/s12889-023-15934-y
24. Lucas RJ, Freedman MS, Lupi D, Munoz M, David-Gray ZK, Foster RG. Identifying the photoreceptive inputs to the mammalian circadian system using transgenic and retinally degenerate mice. Behav Brain Res (2001) 125:97–102. doi: 10.1016/s0166-4328(01)00274-1
25. Li T, Bai Y, Jiang Y, Jiang K, Tian Y, Wang Z, et al. Potential effect of the circadian clock on erectile dysfunction. Aging Dis (2022) 13:8. doi: 10.14336/AD.2021.0728
26. Fan X, Chen Z, Li W, Qin H, Huang S, Lu Z, et al. Exploring the effects of large-area dorsal skin irradiation on locomotor activity and plasm melatonin level in C3H/He mice. Chronobiol Int (2021) 38:1776–85. doi: 10.1080/07420528.2021.1962904
27. Hergenhan S, Holtkamp S, Scheiermann C. Molecular interactions between components of the circadian clock and the immune system. J Mol Biol (2020) 432:3700–13. doi: 10.1016/j.jmb.2019.12.044
28. Xu H, Huang L, Zhao J, Chen S, Liu J, Li G. The circadian clock and inflammation: A new insight. Clin Chim Acta (2021) 512:12–7. doi: 10.1016/j.cca.2020.11.011
29. Zhang EE, Kay SA. Clocks not winding down: unravelling circadian networks. Nat Rev Mol Cell Biol (2010) 11:764–76. doi: 10.1038/nrm2995
30. Meijer JH, Michel S, Vanderleest HT, Rohling JHT. Daily and seasonal adaptation of the circadian clock requires plasticity of the SCN neuronal network. Eur J Neurosci (2010) 32:2143–51. doi: 10.1111/j.1460-9568.2010.07522.x
31. Deng F, Yang K, Zheng G. Period family of clock genes as novel predictors of survival in human cancer: A systematic review and meta-analysis. Dis Markers (2020) 2020:6486238. doi: 10.1155/2020/6486238
32. Munroe SH, Lazar MA. Inhibition of c-erbA mRNA splicing by a naturally occurring antisense RNA. J Biol Chem (1991) 266:22083–6. doi: 10.1016/S0021-9258(18)54535-X
33. Lazar MA, Hodin RA, Cardona G, Chin WW. Gene expression from the c-erbA alpha/Rev-ErbA alpha genomic locus. Potential regulation of alternative splicing by opposite strand transcription. J Biol Chem (1990) 265:12859–63. doi: 10.1016/S0021-9258(19)38238-9
34. Gupta S, Sk S. Kidney stones: Mechanism of formation, pathogenesis and possible treatments. (2018).
35. Paliouras C, Tsampikaki E, Alivanis P, Aperis G. Pathophysiology of nephrolithiasis. Nephrol Res Rev (2012) 4:58–65. doi: 10.4081/nr.2012.e14
36. Alelign T, Petros B. Kidney stone disease: an update on current concepts. Adv Urol (2018) 2018:1–12. doi: 10.1155/2018/3068365
37. Randall A. THE ORIGIN AND GROWTH OF RENAL CALCULI. Ann Surg (1937) 105:1009–27. doi: 10.1097/00000658-193706000-00014
38. Khan SR, Canales BK, Dominguez-Gutierrez PR. Randall’s plaque and calcium oxalate stone formation: role for immunity and inflammation. Nat Rev Nephrol (2021) 17:417–33. doi: 10.1038/s41581-020-00392-1
39. Ketha H, Singh RJ, Grebe SK, Bergstralh EJ, Rule AD, Lieske JC, et al. Altered calcium and vitamin D homeostasis in first-time calcium kidney stone-formers. PloS One (2015) 10:e0137350. doi: 10.1371/journal.pone.0137350
40. Chung J, Granja I, Taylor MG, Mpourmpakis G, Asplin JR, Rimer JD. Molecular modifiers reveal a mechanism of pathological crystal growth inhibition. Nature (2016) 536:446–50. doi: 10.1038/nature19062
41. Brainard J, Gobel M, Scott B, Koeppen M, Eckle T. Health implications of disrupted circadian rhythms and the potential for daylight as therapy. Anesthesiology (2015) 122:1170–5. doi: 10.1097/ALN.0000000000000596
42. Cho JW, Duffy JF. Sleep, sleep disorders, and sexual dysfunction. World J Mens Health (2019) 37:261–75. doi: 10.5534/wjmh.180045
43. Pallesen S, Bjorvatn B, Waage S, Harris A, Sagoe D. Prevalence of shift work disorder: A systematic review and meta-analysis. Front Psychol (2021) 12:638252. doi: 10.3389/fpsyg.2021.638252
44. Yin S, Wang J, Bai Y, Yang Z, Cui J, Wang J. Association between sleep duration and kidney stones in 34 190 American adults: A cross-sectional analysis of NHANES 2007-2018. Sleep Health (2022) 8:671–7. doi: 10.1016/j.sleh.2022.08.003
45. Wang H, Zhang YQ, Yu CQ, Guo Y, Pei P, Chen JS, et al. [Associations between sleep status and risk for kidney stones in Chinese adults: a prospective cohort study]. Zhonghua Liu Xing Bing Xue Za Zhi (2022) 43:1002–9. doi: 10.3760/cma.j.cn112338-20210930-00760
46. Fan M, Sun D, Zhou T, Heianza Y, Lv J, Li L, et al. Sleep patterns, genetic susceptibility, and incident cardiovascular disease: a prospective study of 385 292 UK biobank participants. Eur Heart J (2020) 41:1182–9. doi: 10.1093/eurheartj/ehz849
47. Wang S, Zhou X, Qiu S, Cai B, Li Y, Zhang C, et al. Association between sleep quality and urolithiasis among general population in Western China: a cross-sectional study. BMC Public Health (2022) 22:1787. doi: 10.1186/s12889-022-14187-5
48. Tsai S-H, Stoller ML, Sherer BA, Chao Z-H, Tung T-H. Risk of nephrolithiasis in patients with sleep apnea: A population-based cohort study. J Clin Sleep Med (2018) 14:767–73. doi: 10.5664/jcsm.7102
49. Tallman JE, Stone BV, Sui W, Miller NL, Hsi RS. Association between obstructive sleep apnea and 24-h urine chemistry risk factors for urinary stone disease. Urolithiasis (2023) 51:46. doi: 10.1007/s00240-023-01421-x
50. Khalili P, Jamali Z, Sadeghi T, Esmaeili-Nadimi A, Mohamadi M, Moghadam-Ahmadi A, et al. Risk factors of kidney stone disease: a cross-sectional study in the southeast of Iran. BMC Urol (2021) 21:141. doi: 10.1186/s12894-021-00905-5
51. Jiang YG, He LH, Luo GT, Zhang XD. Prevalence of kidney stones and associated risk factors in the Shunyi District of Beijing, China. Hong Kong Med J (2017) 23:462–9. doi: 10.12809/hkmj164904
52. Liu W, Wang M, Liu J, Yan Q, Liu M. Causal effects of modifiable risk factors on kidney stones: a bidirectional mendelian randomization study. BMC Med Genomics (2023) 16:82. doi: 10.1186/s12920-023-01520-z
53. Foster RG. Sleep, circadian rhythms and health. Interface Focus (2020) 10:20190098. doi: 10.1098/rsfs.2019.0098
54. Goldbeter A, Leloup J-C. From circadian clock mechanism to sleep disorders and jet lag: Insights from a computational approach. Biochem Pharmacol (2021) 191:114482. doi: 10.1016/j.bcp.2021.114482
55. Wulff K, Porcheret K, Cussans E, Foster RG. Sleep and circadian rhythm disturbances: multiple genes and multiple phenotypes. Curr Opin Genet Dev (2009) 19:237–46. doi: 10.1016/j.gde.2009.03.007
56. Saklayen MG. The global epidemic of the metabolic syndrome. Curr Hypertens Rep (2018) 20:12. doi: 10.1007/s11906-018-0812-z
57. Nolan PB, Carrick-Ranson G, Stinear JW, Reading SA, Dalleck LC. Prevalence of metabolic syndrome and metabolic syndrome components in young adults: A pooled analysis. Prev Med Rep (2017) 7:211–5. doi: 10.1016/j.pmedr.2017.07.004
58. Qiu F, Xu Y, Ji X, Pu J, Zhou J, Huang Y. Incidence and correlation of metabolic syndrome and kidney stones in a healthy screening population. Transl Androl Urol (2021) 10:3646–55. doi: 10.21037/tau-21-689
59. Chang C-W, Ke H-L, Lee J-I, Lee Y-C, Jhan J-H, Wang H-S, et al. Metabolic syndrome increases the risk of kidney stone disease: A cross-sectional and longitudinal cohort study. JPM (2021) 11:1154. doi: 10.3390/jpm11111154
60. Spatola L, Ferraro PM, Gambaro G, Badalamenti S, Dauriz M. Metabolic syndrome and uric acid nephrolithiasis: insulin resistance in focus. Metabolism (2018) 83:225–33. doi: 10.1016/j.metabol.2018.02.008
61. Zimmet P, Alberti KGMM, Stern N, Bilu C, El-Osta A, Einat H, et al. The Circadian Syndrome: is the Metabolic Syndrome and much more! J Intern Med (2019) 286:181–91. doi: 10.1111/joim.12924
62. Shi Z, Tuomilehto J, Kronfeld-Schor N, Alberti GK, Stern N, El-Osta A, et al. The circadian syndrome predicts cardiovascular disease better than metabolic syndrome in Chinese adults. J Intern Med (2021) 289:851–60. doi: 10.1111/joim.13204
63. Chaput J-P, McNeil J, Després J-P, Bouchard C, Tremblay A. Short sleep duration as a risk factor for the development of the metabolic syndrome in adults. Prev Med (2013) 57:872–7. doi: 10.1016/j.ypmed.2013.09.022
64. Bayon V, Berger M, Solelhac G, Haba-Rubio J, Marques-Vidal P, Strippoli M-P, et al. Impact of night and shift work on metabolic syndrome and its components: a cross-sectional study in an active middle-to-older-aged population-based sample. BMJ Open (2022) 12:e053591. doi: 10.1136/bmjopen-2021-053591
65. Koopman ADM, Rauh SP, van ‘t Riet E, Groeneveld L, van der Heijden AA, Elders PJ, et al. The association between social jetlag, the metabolic syndrome, and type 2 diabetes mellitus in the general population: the new hoorn study. J Biol Rhythms (2017) 32:359–68. doi: 10.1177/0748730417713572
66. Kim DH, Kim B, Han K, Kim SW. The relationship between metabolic syndrome and obstructive sleep apnea syndrome: a nationwide population-based study. Sci Rep (2021) 11:8751. doi: 10.1038/s41598-021-88233-4
67. Drager LF, Lopes HF, Maki-Nunes C, Trombetta IC, Toschi-Dias E, Alves MJNN, et al. The impact of obstructive sleep apnea on metabolic and inflammatory markers in consecutive patients with metabolic syndrome. PloS One (2010) 5:e12065. doi: 10.1371/journal.pone.0012065
68. Libório AB, Figueiredo PRL, Montenegro Junior RM, Montenegro RM, Martins MRA, Silva Junior GB, et al. Urinary calcium excretion and insulin resistance in patients with acromegaly. Int Urol Nephrol (2012) 44:1473–7. doi: 10.1007/s11255-011-0116-6
69. Meydan N, Barutca S, Caliskan S, Camsari T. Urinary stone disease in diabetes mellitus. Scandinavian J Urol Nephrol (2003) 37:64–70. doi: 10.1080/00365590310008730
70. Weinberg AE, Patel CJ, Chertow GM, Leppert JT. Diabetic severity and risk of kidney stone disease. Eur Urol (2014) 65:242–7. doi: 10.1016/j.eururo.2013.03.026
71. Khand TU, Channa NA, Khand F. Oxidative stress is the common causal link between renal stones and diabetes mellitus in adults. J Pak Med Assoc (2022) 72:1302–5. doi: 10.47391/JPMA.1188
72. Tan E, Scott EM. Circadian rhythms, insulin action, and glucose homeostasis. Curr Opin Clin Nutr Metab Care (2014) 17:343–8. doi: 10.1097/MCO.0000000000000061
73. Henry CJ, Kaur B, Quek RYC. Chrononutrition in the management of diabetes. Nutr Diabetes (2020) 10:6. doi: 10.1038/s41387-020-0109-6
74. Polonsky KS, Given BD, Hirsch LJ, Tillil H, Shapiro ET, Beebe C, et al. Abnormal patterns of insulin secretion in non-insulin-dependent diabetes mellitus. N Engl J Med (1988) 318:1231–9. doi: 10.1056/NEJM198805123181903
75. Song L, Shen J, Wang J, Zhang Y, Zhou Z, Sang L, et al. Shift patterns, physical exercise, and Type 2 diabetes mellitus (T2DM): a prospective cohort study in China. Transl Behav Med (2023) 13:183–91. doi: 10.1093/tbm/ibac089
76. Cui S, Li Y, Chen Y, Ren P, Fan M, Yang X, et al. Association of sleep duration with risk of type 2 diabetes mellitus in a rural Chinese population: a nested case-control study. Sleep Breath (2022) 26:2025–33. doi: 10.1007/s11325-021-02535-5
77. Pietroiusti A, Neri A, Somma G, Coppeta L, Iavicoli I, Bergamaschi A, et al. Incidence of metabolic syndrome among night-shift healthcare workers. Occup Environ Med (2010) 67:54–7. doi: 10.1136/oem.2009.046797
78. Gao Y, Gan T, Jiang L, Yu L, Tang D, Wang Y, et al. Association between shift work and risk of type 2 diabetes mellitus: a systematic review and dose-response meta-analysis of observational studies. Chronobiol Int (2020) 37:29–46. doi: 10.1080/07420528.2019.1683570
79. Chan K, Wong FS, Pearson JA. Circadian rhythms and pancreas physiology: A review. Front Endocrinol (Lausanne) (2022) 13:920261. doi: 10.3389/fendo.2022.920261
80. Turek FW, Joshu C, Kohsaka A, Lin E, Ivanova G, McDearmon E, et al. Obesity and metabolic syndrome in circadian Clock mutant mice. Science (2005) 308:1043–5. doi: 10.1126/science.1108750
81. Shi S, Ansari TS, McGuinness OP, Wasserman DH, Johnson CH. Circadian disruption leads to insulin resistance and obesity. Curr Biol (2013) 23:372–81. doi: 10.1016/j.cub.2013.01.048
82. Lee J, Moulik M, Fang Z, Saha P, Zou F, Xu Y, et al. Bmal1 and β-cell clock are required for adaptation to circadian disruption, and their loss of function leads to oxidative stress-induced β-cell failure in mice. Mol Cell Biol (2013) 33:2327–38. doi: 10.1128/MCB.01421-12
83. Marcheva B, Ramsey KM, Buhr ED, Kobayashi Y, Su H, Ko CH, et al. Disruption of the clock components CLOCK and BMAL1 leads to hypoinsulinaemia and diabetes. Nature (2010) 466:627–31. doi: 10.1038/nature09253
84. Shi D, Chen J, Wang J, Yao J, Huang Y, Zhang G, et al. Circadian clock genes in the metabolism of non-alcoholic fatty liver disease. Front Physiol (2019) 10:423. doi: 10.3389/fphys.2019.00423
85. Baek M, Virgilio S, Lamb TM, Ibarra O, Andrade JM, Gonçalves RD, et al. Circadian clock regulation of the glycogen synthase (gsn) gene by WCC is critical for rhythmic glycogen metabolism in Neurospora crassa. Proc Natl Acad Sci U.S.A. (2019) 116:10435–40. doi: 10.1073/pnas.1815360116
86. Jouffe C, Weger BD, Martin E, Atger F, Weger M, Gobet C, et al. Disruption of the circadian clock component BMAL1 elicits an endocrine adaption impacting on insulin sensitivity and liver disease. Proc Natl Acad Sci U.S.A. (2022) 119:e2200083119. doi: 10.1073/pnas.2200083119
87. Zhang Z, Yu B, Wang X, Luo C, Zhou T, Zheng X, et al. Circadian rhythm and atherosclerosis (Review). Exp Ther Med (2020) 20:96. doi: 10.3892/etm.2020.9224
88. Lamia KA, Papp SJ, Yu RT, Barish GD, Uhlenhaut NH, Jonker JW, et al. Cryptochromes mediate rhythmic repression of the glucocorticoid receptor. Nature (2011) 480:552–6. doi: 10.1038/nature10700
89. Zhang EE, Liu Y, Dentin R, Pongsawakul PY, Liu AC, Hirota T, et al. Cryptochrome mediates circadian regulation of cAMP signaling and hepatic gluconeogenesis. Nat Med (2010) 16:1152–6. doi: 10.1038/nm.2214
90. Qian J, Block GD, Colwell CS, Matveyenko AV. Consequences of exposure to light at night on the pancreatic islet circadian clock and function in rats. Diabetes (2013) 62:3469–78. doi: 10.2337/db12-1543
91. Zani F, Breasson L, Becattini B, Vukolic A, Montani J-P, Albrecht U, et al. PER2 promotes glucose storage to liver glycogen during feeding and acute fasting by inducing Gys2 PTG and G L expression. Mol Metab (2013) 2:292–305. doi: 10.1016/j.molmet.2013.06.006
92. Cho H, Zhao X, Hatori M, Yu RT, Barish GD, Lam MT, et al. Regulation of circadian behaviour and metabolism by REV-ERB-α and REV-ERB-β. Nature (2012) 485:123–7. doi: 10.1038/nature11048
93. Delezie J, Dumont S, Dardente H, Oudart H, Gréchez-Cassiau A, Klosen P, et al. The nuclear receptor REV-ERBα is required for the daily balance of carbohydrate and lipid metabolism. FASEB J (2012) 26:3321–35. doi: 10.1096/fj.12-208751
94. Torricelli FCM, De SK, Gebreselassie S, Li I, Sarkissian C, Monga M. Dyslipidemia and kidney stone risk. J Urol (2014) 191:667–72. doi: 10.1016/j.juro.2013.09.022
95. Ding Q, Ouyang J, Fan B, Cao C, Fan Z, Ding L, et al. Association between dyslipidemia and nephrolithiasis risk in a chinese population. Urol Int (2019) 103:156–65. doi: 10.1159/000496208
96. Antonelli JA, Maalouf NM, Pearle MS, Lotan Y. Use of the National Health and Nutrition Examination Survey to calculate the impact of obesity and diabetes on cost and prevalence of urolithiasis in 2030. Eur Urol (2014) 66:724–9. doi: 10.1016/j.eururo.2014.06.036
97. Zhou T, Watts K, Agalliu I, DiVito J, Hoenig DM. Effects of visceral fat area and other metabolic parameters on stone composition in patients undergoing percutaneous nephrolithotomy. J Urol (2013) 190:1416–20. doi: 10.1016/j.juro.2013.05.016
98. Wu H, Ballantyne CM. Metabolic inflammation and insulin resistance in obesity. Circ Res (2020) 126:1549–64. doi: 10.1161/CIRCRESAHA.119.315896
99. Chua EC-P, Shui G, Lee IT-G, Lau P, Tan L-C, Yeo S-C, et al. Extensive diversity in circadian regulation of plasma lipids and evidence for different circadian metabolic phenotypes in humans. Proc Natl Acad Sci U.S.A. (2013) 110:14468–73. doi: 10.1073/pnas.1222647110
100. Gooley JJ. Circadian regulation of lipid metabolism. Proc Nutr Soc (2016) 75:440–50. doi: 10.1017/S0029665116000288
101. Seo SH, Shim YS. Association of sleep duration with obesity and cardiometabolic risk factors in children and adolescents: A population-based study. Sci Rep (2019) 9:9463. doi: 10.1038/s41598-019-45951-0
102. Brocato J, Wu F, Chen Y, Shamy M, Alghamdi MA, Khoder MI, et al. Association between sleeping hours and cardiometabolic risk factors for metabolic syndrome in a Saudi Arabian population. BMJ Open (2015) 5:e008590. doi: 10.1136/bmjopen-2015-008590
103. Parsons MJ, Moffitt TE, Gregory AM, Goldman-Mellor S, Nolan PM, Poulton R, et al. Social jetlag, obesity and metabolic disorder: investigation in a cohort study. Int: J Obes (Lond) (2015) 39:842–8. doi: 10.1038/ijo.2014.201
104. Hirayama S, Soda S, Ito Y, Matsui H, Ueno T, Fukushima Y, et al. Circadian change of serum concentration of small dense LDL-cholesterol in type 2 diabetic patients. Clin Chim Acta (2010) 411:253–7. doi: 10.1016/j.cca.2009.11.017
105. Xing C, Huang X, Zhang Y, Zhang C, Wang W, Wu L, et al. Sleep disturbance induces increased cholesterol level by NR1D1 mediated CYP7A1 inhibition. Front Genet (2020) 11:610496. doi: 10.3389/fgene.2020.610496
106. Vieira E G, Ruano E, AL CF, Aranda G, Momblan D, Carmona F, et al. Altered clock gene expression in obese visceral adipose tissue is associated with metabolic syndrome. PloS One (2014) 9:e111678. doi: 10.1371/journal.pone.0111678
107. Valladares M, Obregón AM, Chaput J-P. Association between genetic variants of the clock gene and obesity and sleep duration. J Physiol Biochem (2015) 71:855–60. doi: 10.1007/s13105-015-0447-3
108. Pan X, Jiang X-C, Hussain MM. Impaired cholesterol metabolism and enhanced atherosclerosis in clock mutant mice. Circulation (2013) 128:1758–69. doi: 10.1161/CIRCULATIONAHA.113.002885
109. Pérez-Mendoza M, Rivera-Zavala JB, Díaz-Muñoz M. Daytime restricted feeding modifies the daily variations of liver gluconeogenesis: adaptations in biochemical and endocrine regulators. Chronobiol Int (2014) 31:815–28. doi: 10.3109/07420528.2014.908898
110. Pan X, Bradfield CA, Hussain MM. Global and hepatocyte-specific ablation of Bmal1 induces hyperlipidaemia and enhances atherosclerosis. Nat Commun (2016) 7:13011. doi: 10.1038/ncomms13011
111. Dallmann R, Weaver DR. Altered body mass regulation in male mPeriod mutant mice on high-fat diet. Chronobiol Int (2010) 27:1317–28. doi: 10.3109/07420528.2010.489166
112. Ge W, Sun Q, Yang Y, Ding Z, Liu J, Zhang J. Circadian PER1 controls daily fat absorption with the regulation of PER1-PKA on phosphorylation of bile acid synthetase. J Lipid Res (2023) 64:100390. doi: 10.1016/j.jlr.2023.100390
113. Grimaldi B, Bellet MM, Katada S, Astarita G, Hirayama J, Amin RH, et al. PER2 controls lipid metabolism by direct regulation of PPARγ. Cell Metab (2010) 12:509–20. doi: 10.1016/j.cmet.2010.10.005
114. Yang S, Liu A, Weidenhammer A, Cooksey RC, McClain D, Kim MK, et al. The role of mPer2 clock gene in glucocorticoid and feeding rhythms. Endocrinology (2009) 150:2153–60. doi: 10.1210/en.2008-0705
115. Aggarwal A, Costa MJ, Rivero-Gutiérrez B, Ji L, Morgan SL, Feldman BJ. The circadian clock regulates adipogenesis by a per3 crosstalk pathway to klf15. Cell Rep (2017) 21:2367–75. doi: 10.1016/j.celrep.2017.11.004
116. Le Martelot G, Claudel T, Gatfield D, Schaad O, Kornmann B, Lo Sasso G, et al. REV-ERBalpha participates in circadian SREBP signaling and bile acid homeostasis. PloS Biol (2009) 7:e1000181. doi: 10.1371/journal.pbio.1000181
117. Wadei HM, Textor SC. The role of the kidney in regulating arterial blood pressure. Nat Rev Nephrol (2012) 8:602–9. doi: 10.1038/nrneph.2012.191
118. Hill AJ, Basourakos SP, Lewicki P, Wu X, Arenas-Gallo C, Chuang D, et al. Incidence of kidney stones in the United States: the continuous national health and nutrition examination survey. J Urol (2022) 207:851–6. doi: 10.1097/JU.0000000000002331
119. Borghi L, Meschi T, Guerra A, Briganti A, Schianchi T, Allegri F, et al. Essential arterial hypertension and stone disease. Kidney Int (1999) 55:2397–406. doi: 10.1046/j.1523-1755.1999.00483.x
120. Cappuccio FP, Siani A, Barba G, Mellone MC, Russo L, Farinaro E, et al. A: prospective study of hypertension and the incidence of kidney stones in men. J: Hypertens (1999) 17:1017–22. doi: 10.1097/00004872-199917070-00019
121. Kim Y-J, Park MS, Kim W-T, Yun S-J, Kim W-J, Lee S-C. Hypertension influences recurrent stone formation in nonobese stone formers. Urology (2011) 77:1059–63. doi: 10.1016/j.urology.2010.07.492
122. Madore F, Stampfer MJ, Rimm EB, Curhan GC. Nephrolithiasis and risk of hypertension. Am J Hypertens (1998) 11:46–53. doi: 10.1016/s0895-7061(97)00371-3
123. Madore F, Stampfer MJ, Willett WC, Speizer FE, Curhan GC. Nephrolithiasis and risk of hypertension in women. Am J Kidney Dis (1998) 32:802–7. doi: 10.1016/s0272-6386(98)70136-2
124. Cupisti A, D’Alessandro C, Samoni S, Meola M, Egidi MF. Nephrolithiasis and hypertension: possible links and clinical implications. J Nephrol (2014) 27:477–82. doi: 10.1007/s40620-014-0068-x
125. Taylor EN, Mount DB, Forman JP, Curhan GC. Association of prevalent hypertension with 24-hour urinary excretion of calcium, citrate, and other factors. Am J Kidney Dis (2006) 47:780–9. doi: 10.1053/j.ajkd.2006.01.024
126. Davalos M, Konno S, Eshghi M, Choudhury M. Oxidative renal cell injury induced by calcium oxalate crystal and renoprotection with antioxidants: a possible role of oxidative stress in nephrolithiasis. J Endourol (2010) 24:339–45. doi: 10.1089/end.2009.0205
127. Gumz ML, Shimbo D, Abdalla M, Balijepalli RC, Benedict C, Chen Y, et al. Toward precision medicine: circadian rhythm of blood pressure and chronotherapy for hypertension - 2021 NHLBI workshop report. Hypertension (2023) 80:503–22. doi: 10.1161/HYPERTENSIONAHA.122.19372
128. Douma LG, Gumz ML. Circadian clock-mediated regulation of blood pressure. Free Radical Biol Med (2018) 119:108–14. doi: 10.1016/j.freeradbiomed.2017.11.024
129. Smolensky MH, Sackett-Lundeen LL, Portaluppi F. Nocturnal light pollution and underexposure to daytime sunlight: Complementary mechanisms of circadian disruption and related diseases. Chronobiol Int (2015) 32:1029–48. doi: 10.3109/07420528.2015.1072002
130. Grandner M, Mullington JM, Hashmi SD, Redeker NS, Watson NF, Morgenthaler TI. Sleep duration and hypertension: analysis of > 700,000 adults by age and sex. J Clin Sleep Med (2018) 14:1031–9. doi: 10.5664/jcsm.7176
131. Li H, Ren Y, Wu Y, Zhao X. Correlation between sleep duration and hypertension: a dose-response meta-analysis. J Hum Hypertens (2019) 33:218–28. doi: 10.1038/s41371-018-0135-1
132. Hou H, Zhao Y, Yu W, Dong H, Xue X, Ding J, et al. Association of obstructive sleep apnea with hypertension: A systematic review and meta-analysis. J Glob Health (2018) 8:10405. doi: 10.7189/jogh.08.010405
133. Xiao Z, Xu C, Liu Q, Yan Q, Liang J, Weng Z, et al. Night shift work, genetic risk, and hypertension. Mayo Clin Proc (2022) 97:2016–27. doi: 10.1016/j.mayocp.2022.04.007
134. Makarem N, Zuraikat FM, Aggarwal B, Jelic S, St-Onge M-P. Variability in sleep patterns: an emerging risk factor for hypertension. Curr Hypertens Rep (2020) 22:19. doi: 10.1007/s11906-020-1025-9
135. Nakamura N, Akiyama H, Nishimura M, Zhu K, Suzuki K, Higuchi M, et al. Acute social jetlag augments morning blood pressure surge: a randomized crossover trial. Hypertens Res (2023) 46(9):2179–91. doi: 10.1038/s41440-023-01360-5
136. Dopp JM, Reichmuth KJ, Morgan BJ. Obstructive sleep apnea and hypertension: Mechanisms, evaluation, and management. Curr Sci Inc (2007) 9:529–34. doi: 10.1007/s11906-007-0095-2
137. Salman LA, Shulman R, Cohen JB. Obstructive sleep apnea, hypertension, and cardiovascular risk: epidemiology, pathophysiology, and management. Curr Cardiol Rep (2020) 22:6. doi: 10.1007/s11886-020-1257-y
138. Foster GE, Hanly PJ, Ahmed SB, Beaudin AE, Pialoux V, Poulin MJ. Intermittent hypoxia increases arterial blood pressure in humans through a Renin-Angiotensin system-dependent mechanism. Hypertension (2010) 56:369–77. doi: 10.1161/HYPERTENSIONAHA.110.152108
139. Nakashima A, Kawamoto T, Noshiro M, Ueno T, Doi S, Honda K, et al. Dec1 and CLOCK regulate na+/K+-ATPase β1 subunit expression and blood pressure. Hypertension (2018) 72:746–54. doi: 10.1161/HYPERTENSIONAHA.118.11075
140. Huo M, Cao X, Zhang H, Lau CW, Hong H, Chen FM, et al. Loss of myeloid Bmal1 exacerbates hypertensive vascular remodelling through interaction with STAT6 in mice. Cardiovasc Res (2022) 118:2859–74. doi: 10.1093/cvr/cvab336
141. Marques FZ, Campain AE, Tomaszewski M, Zukowska-Szczechowska E, Yang YHJ, Charchar FJ, et al. Gene expression profiling reveals renin mRNA overexpression in human hypertensive kidneys and a role for microRNAs. Hypertension (2011) 58:1093–8. doi: 10.1161/HYPERTENSIONAHA.111.180729
142. Doi M, Takahashi Y, Komatsu R, Yamazaki F, Yamada H, Haraguchi S, et al. Salt-sensitive hypertension in circadian clock–deficient Cry-null mice involves dysregulated adrenal Hsd3b6. Nat Med (2010) 16:67–74. doi: 10.1038/nm.2061
143. Richards J, Diaz AN, Gumz ML. Clock genes in hypertension: novel insights from rodent models. Blood Pressure Monit (2014) 19:249–54. doi: 10.1097/MBP.0000000000000060
144. Mancusi C, Izzo R, di Gioia G, Losi MA, Barbato E, Morisco C. Insulin resistance the hinge between hypertension and type 2 diabetes. High Blood Press Cardiovasc Prev (2020) 27:515–26. doi: 10.1007/s40292-020-00408-8
145. Richards J, Ko B, All S, Cheng K-Y, Hoover RS, Gumz ML. A role for the circadian clock protein per1 in the regulation of the naCl co-transporter (NCC) and the with-no-lysine kinase (WNK) cascade in mouse distal convoluted tubule cells. J Biol Chem (2014) 289:11791–806. doi: 10.1074/jbc.M113.531095
146. Zuber AM, Centeno G, Pradervand S, Nikolaeva S, Maquelin L, Cardinaux L, et al. Molecular clock is involved in predictive circadian adjustment of renal function. Proc Natl Acad Sci U.S.A. (2009) 106:16523–8. doi: 10.1073/pnas.0904890106
147. Staub O, Debonneville A, Stifanelli M, Juffre A, Maillard MP, Gumz ML, et al. Renal tubular SGK1 is required to achieve blood pressure surge and circadian rhythm. Am J Physiol Renal Physiol (2023) 325:F629–37. doi: 10.1152/ajprenal.00211.2023
148. Chang JT. Pathophysiology of inflammatory bowel diseases. N Engl J Med (2020) 383:2652–64. doi: 10.1056/NEJMra2002697
149. Dincer MT, Dincer ZT, Bakkaloglu OK, Yalin SF, Trabulus S, Celik AF, et al. Renal manifestations in inflammatory bowel disease: A cohort study during the biologic era. Med Sci Monit (2022) 28:e936497-1–e936497-10. doi: 10.12659/MSM.936497
150. Fagagnini S, Heinrich H, Rossel J-B, Biedermann L, Frei P, Zeitz J, et al. Risk factors for gallstones and kidney stones in a cohort of patients with inflammatory bowel diseases. PloS One (2017) 12:e0185193. doi: 10.1371/journal.pone.0185193
151. Herbert J, Teeter E, Burstiner LS, Doka R, Royer A, Owings AH, et al. Urinary manifestations in African American and Caucasian inflammatory bowel disease patients: a retrospective cohort study. BMC Urol (2022) 22:1. doi: 10.1186/s12894-021-00951-z
152. Corica D, Romano C. Renal involvement in inflammatory bowel diseases. ECCOJC (2016) 10:226–35. doi: 10.1093/ecco-jcc/jjv138
153. Cury D, Moss A, Schor N. Nephrolithiasis in patients with inflammatory bowel disease in the community. IJNRD (2013) 6:139–42. doi: 10.2147/IJNRD.S45466
154. Ananthakrishnan AN, Khalili H, Konijeti GG, Higuchi LM, de Silva P, Fuchs CS, et al. Sleep duration affects risk for ulcerative colitis: a prospective cohort study. Clin Gastroenterol Hepatol (2014) 12:1879–86. doi: 10.1016/j.cgh.2014.04.021
155. Chakradeo PS, Keshavarzian A, Singh S, Dera AE, Esteban JPG, Lee AA, et al. Chronotype, social jet lag, sleep debt and food timing in inflammatory bowel disease. Sleep Med (2018) 52:188–95. doi: 10.1016/j.sleep.2018.08.002
156. Weintraub Y, Cohen S, Chapnik N, Ben-Tov A, Yerushalmy-Feler A, Dotan I, et al. Clock gene disruption is an initial manifestation of inflammatory bowel diseases. Clin Gastroenterol Hepatol (2020) 18:115–122.e1. doi: 10.1016/j.cgh.2019.04.013
157. Liu X, Yu R, Zhu L, Hou X, Zou K. Bidirectional regulation of circadian disturbance and inflammation in inflammatory bowel disease. Inflammatory Bowel Dis (2017) 23:1741–51. doi: 10.1097/MIB.0000000000001265
158. Weintraub Y, Cohen S, Anafy A, Chapnik N, Tsameret S, Ben-Tov A, et al. Inverse relationship between clock gene expression and inflammatory markers in ulcerative colitis patients undergoing remission. Dig Dis Sci (2023) 68:2454–62. doi: 10.1007/s10620-023-07847-y
159. Wang S, Lin Y, Yuan X, Li F, Guo L, Wu B. REV-ERBα integrates colon clock with experimental colitis through regulation of NF-κB/NLRP3 axis. Nat Commun (2018) 9:4246. doi: 10.1038/s41467-018-06568-5
160. Kyoko O, Kono H, Ishimaru K, Miyake K, Kubota T, Ogawa H, et al. Expressions of tight junction proteins Occludin and Claudin-1 are under the circadian control in the mouse large intestine: implications in intestinal permeability and susceptibility to colitis. PloS One (2014) 9:e98016. doi: 10.1371/journal.pone.0098016
161. Taleb Z, Carmona-Alcocer V, Stokes K, Haireek M, Wang H, Collins SM, et al. BMAL1 regulates the daily timing of colitis. Front Cell Infect Microbiol (2022) 12:773413. doi: 10.3389/fcimb.2022.773413
162. Oh SK, Kim D, Kim K, Boo K, Yu YS, Kim IS, et al. RORα is crucial for attenuated inflammatory response to maintain intestinal homeostasis. Proc Natl Acad Sci U.S.A. (2019) 116:21140–9. doi: 10.1073/pnas.1907595116
163. Wang S, Lin Y, Li F, Qin Z, Zhou Z, Gao L, et al. An NF-κB-driven lncRNA orchestrates colitis and circadian clock. Sci Adv (2020) 6:eabb5202. doi: 10.1126/sciadv.abb5202
164. Liu J-L, Wang C-Y, Cheng T-Y, Rixiati Y, Ji C, Deng M, et al. Circadian clock disruption suppresses PDL1+ Intraepithelial B cells in experimental colitis and colitis-associated colorectal cancer. Cell Mol Gastroenterol Hepatol (2021) 12:251–76. doi: 10.1016/j.jcmgh.2021.02.008
165. Pagel R, Bär F, Schröder T, Sünderhauf A, Künstner A, Ibrahim SM, et al. Circadian rhythm disruption impairs tissue homeostasis and exacerbates chronic inflammation in the intestine. FASEB J (2017) 31:4707–19. doi: 10.1096/fj.201700141RR
166. Miller AW, Penniston KL, Fitzpatrick K, Agudelo J, Tasian G, Lange D. Mechanisms of the intestinal and urinary microbiome in kidney stone disease. Nat: Rev Urol (2022) 19:695–707. doi: 10.1038/s41585-022-00647-5
167. Parizadeh M, Arrieta M-C. The global human gut microbiome: genes, lifestyles, and diet. Trends Mol Med (2023) S1471-4914(23):00152–1. doi: 10.1016/j.molmed.2023.07.002
168. Patel SR, Ingram C, Scovell JM, Link RE, Mayer WA. The microbiome and: urolithiasis: current advancements and future challenges. Curr Urol Rep (2022) 23:47–56. doi: 10.1007/s11934-022-01088-8
169. Kumar R, Mukherjee M, Bhandari M, Kumar A, Sidhu H, Mittal RD. Role of Oxalobacter formigenes in calcium oxalate stone disease: a study from North India. Eur Urol (2002) 41:318–22. doi: 10.1016/s0302-2838(02)00040-4
170. Kaufman DW, Kelly JP, Curhan GC, Anderson TE, Dretler SP, Preminger GM, et al. Oxalobacter formigenes may reduce the risk of calcium oxalate kidney stones. J: Am Soc Nephrol (2008) 19:1197–203. doi: 10.1681/ASN.2007101058
171. Troxel SA, Sidhu H, Kaul P, Low RK. Intestinal Oxalobacter formigenes colonization in calcium oxalate stone formers and its relation to urinary oxalate. J: Endourol (2003) 17:173–6. doi: 10.1089/089277903321618743
172. Stern JM, Moazami S, Qiu Y, Kurland I, Chen Z, Agalliu I, et al. Evidence for a distinct gut microbiome in kidney stone formers compared to non-stone formers. Urolithiasis (2016) 44:399–407. doi: 10.1007/s00240-016-0882-9
173. Lewis DA, Brown R, Williams J, White P, Jacobson SK, Marchesi JR, et al. The human urinary microbiome; bacterial DNA in voided urine of asymptomatic adults. Front Cell Infect Microbiol (2013) 3:41. doi: 10.3389/fcimb.2013.00041
174. Xie J, Huang J, Huang X, Peng J, Yu Z, Yuan Y, et al. Profiling the urinary microbiome in men with calcium-based kidney stones. BMC Microbiol (2020) 20:41. doi: 10.1186/s12866-020-01734-6
175. Yuan T, Xia Y, Li B, Yu W, Rao T, Ye Z, et al. Gut microbiota in patients with kidney stones: a systematic review and meta-analysis. BMC Microbiol (2023) 23:143. doi: 10.1186/s12866-023-02891-0
176. Amimanan P, Tavichakorntrakool R, Fong-Ngern K, Sribenjalux P, Lulitanond A, Prasongwatana V, et al. Elongation factor Tu on Escherichia coli isolated from urine of kidney stone patients promotes calcium oxalate crystal growth and aggregation. Sci: Rep (2017) 7:2953. doi: 10.1038/s41598-017-03213-x
177. Chutipongtanate S, Sutthimethakorn S, Chiangjong W, Thongboonkerd V. Bacteria can promote calcium oxalate crystal growth and aggregation. J Biol Inorg Chem (2013) 18:299–308. doi: 10.1007/s00775-012-0974-0
178. Gao H, Lin J, Xiong F, Yu Z, Pan S, Huang Y. Urinary microbial and metabolomic profiles in kidney stone disease. Front Cell Infect Microbiol (2022) 12:953392. doi: 10.3389/fcimb.2022.953392
179. Ticinesi A, Nouvenne A, Meschi T. Gut microbiome and kidney stone disease: not just an Oxalobacter story. Kidney Int (2019) 96:25–7. doi: 10.1016/j.kint.2019.03.020
180. Jones-Freeman B, Chonwerawong M, Marcelino VR, Deshpande AV, Forster SC, Starkey MR. The microbiome and host mucosal interactions in urinary tract diseases. Mucosal Immunol (2021) 14:779–92. doi: 10.1038/s41385-020-00372-5
181. Thaiss CA, Levy M, Korem T, Dohnalová L, Shapiro H, Jaitin DA, et al. Microbiota diurnal rhythmicity programs host transcriptome oscillations. Cell (2016) 167:1495–1510.e12. doi: 10.1016/j.cell.2016.11.003
182. Thaiss CA, Zeevi D, Levy M, Zilberman-Schapira G, Suez J, Tengeler AC, et al. Transkingdom control of microbiota diurnal oscillations promotes metabolic homeostasis. Cell (2014) 159:514–29. doi: 10.1016/j.cell.2014.09.048
183. Smith RP, Easson C, Lyle SM, Kapoor R, Donnelly CP, Davidson EJ, et al. Gut microbiome diversity is associated with sleep physiology in humans. PloS One (2019) 14:e0222394. doi: 10.1371/journal.pone.0222394
184. Agrawal R, Ajami NJ, Malhotra S, Chen L, White DL, Sharafkhaneh A, et al. Habitual sleep duration and the colonic mucosa-associated gut microbiota in humans-A pilot study. Clocks Sleep (2021) 3:387–97. doi: 10.3390/clockssleep3030025
185. Li Q, Wang B, Qiu H-Y, Yan X-J, Cheng L, Wang Q-Q, et al. Chronic jet lag exacerbates jejunal and colonic microenvironment in mice. Front Cell Infect Microbiol (2021) 11:648175. doi: 10.3389/fcimb.2021.648175
186. Voigt RM, Summa KC, Forsyth CB, Green SJ, Engen P, Naqib A, et al. The circadian clock mutation promotes intestinal dysbiosis. Alcohol Clin Exp Res (2016) 40:335–47. doi: 10.1111/acer.12943
187. Liang X, Bushman FD, FitzGerald GA. Rhythmicity of the intestinal microbiota is regulated by gender and the host circadian clock. Proc Natl Acad Sci U.S.A. (2015) 112:10479–84. doi: 10.1073/pnas.1501305112
188. Leone V, Gibbons SM, Martinez K, Hutchison AL, Huang EY, Cham CM, et al. Effects of diurnal variation of gut microbes and high-fat feeding on host circadian clock: function and metabolism. Cell Host Microbe (2015) 17:681–9. doi: 10.1016/j.chom.2015.03.006
189. Voigt RM, Forsyth CB, Green SJ, Mutlu E, Engen P, Vitaterna MH, et al. Circadian disorganization alters intestinal microbiota. PloS One (2014) 9:e97500. doi: 10.1371/journal.pone.0097500
190. Zarrinpar A, Chaix A, Yooseph S, Panda S. Diet and feeding pattern affect the diurnal dynamics of the gut microbiome. Cell Metab (2014) 20:1006–17. doi: 10.1016/j.cmet.2014.11.008
191. Paulose JK, Cassone CV, Graniczkowska KB, Cassone VM. Entrainment of the circadian clock of the enteric bacterium klebsiella aerogenes by temperature cycles. iScience (2019) 19:1202–13. doi: 10.1016/j.isci.2019.09.007
192. Stone EF, Fulton BO, Ayres JS, Pham LN, Ziauddin J, Shirasu-Hiza MM. The: circadian clock protein timeless regulates phagocytosis of bacteria in Drosophila. PloS Pathog (2012) 8:e1002445. doi: 10.1371/journal.ppat.1002445
193. Shirasu-Hiza MM, Dionne MS, Pham LN, Ayres JS, Schneider DS. Interactions between circadian rhythm and immunity in Drosophila melanogaster. Curr Biol (2007) 17:R353–355. doi: 10.1016/j.cub.2007.03.049
194. Diallo AB, Mezouar S, Boumaza A, Fiammingo O, Coiffard B, Pontarotti P, et al. RadA, a key gene of the circadian rhythm of escherichia coli. IJMS (2022) 23:6136. doi: 10.3390/ijms23116136
195. Gomelsky M, Klug G. BLUF: a novel FAD-binding domain involved in sensory transduction in microorganisms. Trends Biochem Sci (2002) 27:497–500. doi: 10.1016/s0968-0004(02)02181-3
196. Goodman HO, Holmes RP, Assimos DG. Genetic factors in calcium oxalate stone disease. J Urol (1995) 153:301–7. doi: 10.1097/00005392-199502000-00003
197. Ducy P. A central regulation of PTH secretion and function. Neuron (2023) 111:1847–9. doi: 10.1016/j.neuron.2023.05.018
198. Ganesan C, Weia B, Thomas I-C, Song S, Velaer K, Seib CD, et al. Analysis of: primary hyperparathyroidism screening among US veterans with kidney stones. JAMA Surg (2020) 155:861–8. doi: 10.1001/jamasurg.2020.2423
199. Charles P-Y, Letavernier E, Périé S, Gauthé M, Daudon M, Haymann J-P. Effect of parathyroidectomy on renal stone recurrence. Urolithiasis (2021) 49:327–34. doi: 10.1007/s00240-020-01239-x
200. Perez AA, Schneider DF, Long KL, Pitt SC, Sippel RS. Timely evaluation and management of primary hyperparathyroidism in patients with kidney stones. J Surg Res (2018) 232:564–9. doi: 10.1016/j.jss.2018.07.028
201. Schou A, Jørgensen NR, Maro VP, Kilonzo K, Ramaiya K, Sironga J, et al. The circadian rhythm of calcium and bone homeostasis in Maasai. Am J Hum Biol (2022) 34:e23756. doi: 10.1002/ajhb.23756
202. Erden ES, Genc S, Motor S, Ustun I, Ulutas KT, Bilgic HK, et al. Investigation of serum bisphenol A, vitamin D, and parathyroid hormone levels in patients with obstructive sleep apnea syndrome. Endocrine (2014) 45:311–8. doi: 10.1007/s12020-013-0022-z
203. Murray SE, Pathak PR, Schaefer SC, Chen H, Sippel RS. Improvement of sleep disturbance and insomnia following parathyroidectomy for primary hyperparathyroidism. World J Surg (2014) 38:542–8. doi: 10.1007/s00268-013-2285-1
204. Chou F-F, Lee C-H, Chen J-B, Huang S-C, Lee C-T. Sleep disturbances before and after parathyroidectomy for secondary hyperparathyroidism. Surgery (2005) 137:426–30. doi: 10.1016/j.surg.2004.12.010
205. Egstrand S, Mace ML, Morevati M, Nordholm A, Engelholm LH, Thomsen JS, et al. Hypomorphic expression of parathyroid Bmal1 disrupts the internal parathyroid circadian clock and increases parathyroid cell proliferation in response to uremia. Kidney Int (2022) 101:1232–50. doi: 10.1016/j.kint.2022.02.018
206. Egstrand S, Nordholm A, Morevati M, Mace ML, Hassan A, Naveh-Many T, et al. A molecular circadian clock operates in the parathyroid gland and is disturbed in chronic kidney disease associated bone and mineral disorder. Kidney Int (2020) 98:1461–75. doi: 10.1016/j.kint.2020.06.034
207. Sadowski SM, Pusztaszeri M, Brulhart-Meynet M-C, Petrenko V, De Vito C, Sobel J, et al. Identification of differential transcriptional patterns in primary and secondary hyperparathyroidism. J Clin Endocrinol Metab (2018) 103:2189–98. doi: 10.1210/jc.2017-02506
208. Bouillon R, Carmeliet G. Vitamin D insufficiency: Definition, diagnosis and management. Best Pract Res Clin Endocrinol Metab (2018) 32:669–84. doi: 10.1016/j.beem.2018.09.014
209. Johri N, Jaeger P, Ferraro PM, Shavit L, Nair D, Robertson WG, et al. Vitamin D deficiency is prevalent among idiopathic stone formers, but does correction pose any risk? Urolithiasis (2017) 45:535–43. doi: 10.1007/s00240-016-0954-x
210. Elkoushy MA, Sabbagh R, Unikowsky B, Andonian S. Prevalence and metabolic abnormalities of vitamin D–inadequate patients presenting with urolithiasis to a tertiary stone clinic. Urology (2012) 79:781–5. doi: 10.1016/j.urology.2011.09.004
211. Eisner BH, Thavaseelan S, Sheth S, Haleblian G, Pareek G. Relationship between serum vitamin D and 24-hour urine calcium in patients with nephrolithiasis. Urology (2012) 80:1007–10. doi: 10.1016/j.urology.2012.04.041
212. Girón-Prieto MS, Del Carmen Cano-García M, Arrabal-Polo MÁ, Poyatos-Andujar A, Quesada-Charneco M, De Haro-Muñoz T, et al. Analysis of vitamin D deficiency in calcium stone-forming patients. Int Urol Nephrol (2016) 48:1243–6. doi: 10.1007/s11255-016-1290-3
213. Tavasoli S, Taheri M. Vitamin D and calcium kidney stones: a review and a proposal. Int Urol Nephrol (2019) 51:101–11. doi: 10.1007/s11255-018-1965-z
214. Wimalawansa SJ. Vitamin D deficiency: effects on oxidative stress, epigenetics, gene regulation, and aging. Biol (Basel) (2019) 8:30. doi: 10.3390/biology8020030
215. Masood T, Kushwaha RS, Singh R, Sailwal S, Pandey H, Varma A, et al. Circadian rhythm of serum 25 (OH) vitamin D, calcium and phosphorus levels in the treatment and management of type-2 diabetic patients. Drug Discovery Ther (2015) 9:70–4. doi: 10.5582/ddt.2015.01002
216. Alefishat E, Abu Farha R. Determinants of vitamin d status among Jordanian employees: Focus on the night shift effect. Int J Occup Med Environ Health (2016) 29:859–70. doi: 10.13075/ijomeh.1896.00657
217. Piovezan RD, Hirotsu C, Feres MC, Cintra FD, Andersen ML, Tufik S, et al. Obstructive sleep apnea and objective short sleep duration are independently associated with the risk of serum vitamin D deficiency. PloS One (2017) 12:e0180901. doi: 10.1371/journal.pone.0180901
218. Lehnert M, Beine A, Burek K, Putzke S, Schlösser S, Pallapies D, et al. Vitamin D supply in shift working nurses. Chronobiol Int (2018) 35:724–9. doi: 10.1080/07420528.2018.1424719
219. Romano A, Vigna L, Belluigi V, Conti DM, Barberi CE, Tomaino L, et al. Shift work and serum 25-OH vitamin D status among factory workers in Northern Italy: Cross-sectional study. Chronobiol Int (2015) 32:842–7. doi: 10.3109/07420528.2015.1048867
220. Martelli M, Salvio G, Santarelli L, Bracci M. Shift work and serum vitamin D levels: A systematic review and meta-analysis. Int J Environ Res Public Health (2022) 19:8919. doi: 10.3390/ijerph19158919
221. Kawai M, Kinoshita S, Yamazaki M, Yamamoto K, Rosen CJ, Shimba S, et al. Intestinal clock system regulates skeletal homeostasis. JCI Insight (2019) 4:e121798. doi: 10.1172/jci.insight.121798. 121798.
222. Pochetti P, Azzolina D, Ragnoli B, Tillio PA, Cantaluppi V, Malerba M. Interrelationship among obstructive sleep apnea, renal function and survival: A cohort study. Int J Environ Res Public Health (2020) 17:4922. doi: 10.3390/ijerph17144922
223. Moriya R, Hokari S, Ohshima Y, Suzuki R, Nagai A, Fujito N, et al. Continuous positive airway pressure treatment reduces renal tubular damage in patients with obstructive sleep apnea: A retrospective single-center cohort study. Sleep Med (2023) 106:106–15. doi: 10.1016/j.sleep.2023.03.028
224. Domrongkitchaiporn S, Stitchantrakul W. Renal tubular acidosis and stone formation. In: Talati JJ, Tiselius H-G, Albala DM, Ye Z, editors. Urolithiasis. London: Springer London (2012). p. 195–8. doi: 10.1007/978-1-4471-4387-1_23
225. Giglio S, Montini G, Trepiccione F, Gambaro G, Emma F. Distal renal tubular acidosis: a systematic approach from diagnosis to treatment. J Nephrol (2021) 34:2073–83. doi: 10.1007/s40620-021-01032-y
226. Kirubarajan A, Lam ACL, Khan S, Yau M, Golda N, Buckley R. The association between renal stones and fasting: A systematic review. Canadian Urological Association Journal (2021) 15(3):E169–74. doi: 10.5489/cuaj.6664
227. Sener TE, Sener G, Cevik O, Eker P, Cetinel S, Traxer O, et al. The effects of melatonin on ethylene glycol-induced nephrolithiasis: role on osteopontin mRNA gene expression. Urology (2017) 99:287.e9–287.e15. doi: 10.1016/j.urology.2016.09.032
228. Vasey C, McBride J, Penta K. Circadian rhythm dysregulation and restoration: the role of melatonin. Nutrients (2021) 13:3480. doi: 10.3390/nu13103480
229. Song Q, He Z, Li B, Liu J, Liu L, Liao W, et al. Melatonin inhibits oxalate-induced endoplasmic reticulum stress and apoptosis in HK-2 cells by activating the AMPK pathway. Cell Cycle (2020) 19:2600–10. doi: 10.1080/15384101.2020.1810401
Keywords: circadian rhythm disturbance, circadian clock, sleep disorder, circadian clock gene, kidney stone disease
Citation: He S-K, Wang J-H, Li T, Yin S, Cui J-W, Xiao Y-F, Tang Y, Wang J and Bai Y-J (2023) Sleep and circadian rhythm disturbance in kidney stone disease: a narrative review. Front. Endocrinol. 14:1293685. doi: 10.3389/fendo.2023.1293685
Received: 13 September 2023; Accepted: 08 November 2023;
Published: 27 November 2023.
Edited by:
Mohammed S. Razzaque, Lake Erie College of Osteopathic Medicine, United StatesReviewed by:
Yu Pan, Shanghai Jiao Tong University, ChinaRicardo Adrian Nugraha, Airlangga University, Indonesia
Jens Djurhuus, Aarhus University, Denmark
Copyright © 2023 He, Wang, Li, Yin, Cui, Xiao, Tang, Wang and Bai. This is an open-access article distributed under the terms of the Creative Commons Attribution License (CC BY). The use, distribution or reproduction in other forums is permitted, provided the original author(s) and the copyright owner(s) are credited and that the original publication in this journal is cited, in accordance with accepted academic practice. No use, distribution or reproduction is permitted which does not comply with these terms.
*Correspondence: Yun-Jin Bai, YmFpeXVuamluQHdjaHNjdS5jbg==
†These authors have contributed equally to this work